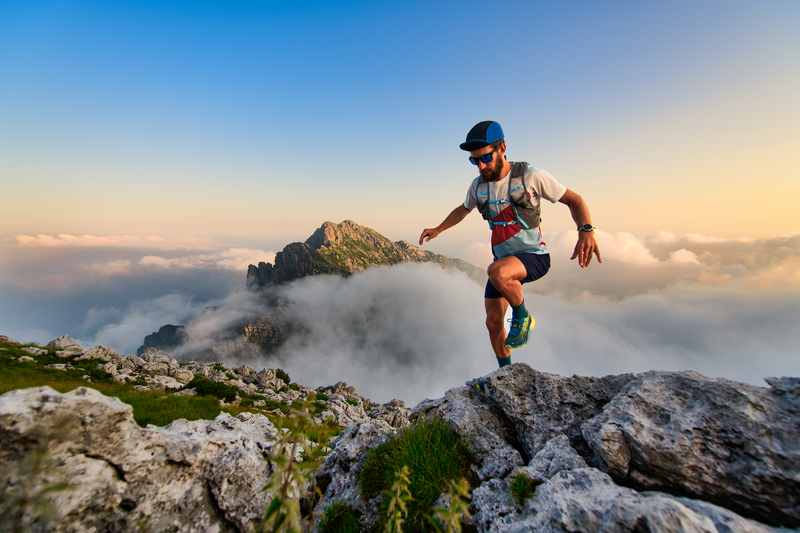
95% of researchers rate our articles as excellent or good
Learn more about the work of our research integrity team to safeguard the quality of each article we publish.
Find out more
HYPOTHESIS AND THEORY article
Front. Plant Sci. , 01 September 2021
Sec. Plant Biophysics and Modeling
Volume 12 - 2021 | https://doi.org/10.3389/fpls.2021.662425
Ribulose-1,5-bisphosphate (RuBP) carboxylase/oxygenase (RuBisCO) is the carbon-fixing enzyme present in most photosynthetic organisms, converting CO2 into organic matter. Globally, photosynthetic efficiency in terrestrial plants has become increasingly challenged in recent decades due to a rapid increase in atmospheric CO2 and associated changes toward warmer and dryer environments. Well adapted for these new climatic conditions, the C4 photosynthetic pathway utilizes carbon concentrating mechanisms to increase CO2 concentrations surrounding RuBisCO, suppressing photorespiration from the oxygenase catalyzed reaction with O2. The energy efficiency of C3 photosynthesis, from which the C4 pathway evolved, is thought to rely critically on an uninterrupted supply of chloroplast CO2. Part of the homeostatic mechanism that maintains this constancy of supply involves the CO2 produced as a byproduct of photorespiration in a negative feedback loop. Analyzing the database of RuBisCO kinetic parameters, we suggest that in genera (Flaveria and Panicum) for which both C3 and C4 examples are available, the C4 pathway evolved only from C3 ancestors possessing much lower than the average carboxylase specificity relative to that of the oxygenase reaction (SC/O=SC/SO), and hence, the higher CO2 levels required for development of the photorespiratory CO2 pump (C2 photosynthesis) essential in the initial stages of C4 evolution, while in the later stage (final optimization phase in the Flaveria model) increased CO2 turnover may have occurred, which would have been supported by the higher CO2 levels. Otherwise, C4 RuBisCO kinetic traits remain little changed from the ancestral C3 species. At the opposite end of the spectrum, C3 plants (from Limonium) with higher than average SC/O, which may be associated with the ability of increased CO2, relative to O2, affinity to offset reduced photorespiration and chloroplast CO2 levels, can tolerate high stress environments. It is suggested that, instead of inherently constrained by its kinetic mechanism, RuBisCO possesses the extensive kinetic plasticity necessary for adaptation to changes in photorespiration that occur in the homeostatic regulation of CO2 supply under a broad range of abiotic environmental conditions.
What makes Ribulose-1,5-bisphosphate (RuBP) carboxylase/oxygenase (RuBisCO) kinetic parameters the way they are? This question has now persisted for several decades, without a definitive explanation. Although RuBisCO is the principal carbon-fixing enzyme in the biosphere, its product turnover rate (circa 3s−1 per active site for plants) may be considered, if not particularly slow, rather unexceptional (Bar-Even et al., 2011). The primary substrate (CO2) must also compete for binding to the RuBP in the RuBisCO active site with the more abundant O2 in the atmosphere leading to photorespiration, consuming additional energy and compromising the process of photosynthesis. The combined effects of increasing population and anthropogenic climate change have motivated efforts to enhance carbon fixation in plants for increasing both agricultural crop yield and carbon sequestration generally (Niinemets et al., 2017; Andralojc et al., 2018; Erb and Zarzycki, 2018; Fernie et al., 2020; Lawson and Flexus, 2020). Although the possibility of enhancing photosynthesis by improving RuBisCO kinetic traits has been given due consideration (Whitney et al., 2011; Sharwood et al., 2016; Gomez-Fernandez et al., 2018; Wilson et al., 2018; Zhou and Whitney, 2019; Davidi et al., 2020; Lin et al., 2020; Bouvier et al., 2021), a conclusive picture of RuBisCO’s molecular mechanism (Cleland et al., 1998; Tcherkez, 2013, 2015; Cummins et al., 2018b, 2019b; Kannappan et al., 2019; Bathellier et al., 2020; Cummins and Gready, 2020) and a general consensus understanding of the observed tradeoffs between RuBisCO’s kinetic parameters remains elusive, despite having been analyzed in varying ways with the objective of gaining insights into the possible connection between evolutionary and biochemical (or catalytic) constraints (Bouvier et al., 2021). The earliest of these studies, (Tcherkez et al., 2006; Savir et al., 2010) based on general mechanistic assumptions and limited data samples, concluded that variations in the elementary rate constants must be tightly constrained by the limitations inherent in RuBisCOs kinetic mechanism, resulting in an enzyme which provides only limited scope for further optimization (Tcherkez et al., 2006; Tcherkez, 2013, 2015), while later studies of more extensive data sets have challenged this view, revealing greater flexibility (Cummins et al., 2018a, 2019a; Flamholz et al., 2019). Other studies have examined the coevolution of RuBisCO kinetics and carbon concentrating mechanism (CCMs) (Goudet et al., 2020; Iñiguez et al., 2020).
Various forms of CCMs have occurred independently and at different times in a wide range of photosynthetic organisms from diverse environments, directing the evolution of RuBisCO kinetics (Iñiguez et al., 2020). In higher plants, the vast majority follow the C3 photosynthetic pathway initiated by CO2 fixing to the bound form of activated RuBP substrate (Cleland et al., 1998) which proceeds through hydrolysis to break down into two molecules of the 3-carbon compound 3-phosphoglyceric acid (3PGA). The C4 pathway, although present in only about 8,000 species (Sage, 2016), including some important agricultural crops (maize and sorghum), accounts for about 25% of terrestrial photosynthesis. In C4 photosynthesis, CO2 is initially converted by carbonic anhydrase (CA) to bicarbonate which is fixed by phosphoenolpyruvate carboxylase into oxaloacetate. Oxaloacetate is converted into malate (4-carbon compound) or aspartate for diffusion into the bundle sheath cells where they are decarboxylated in high concentrations and the released CO2 is then, as in C3 photosynthesis, fixed into 3PGA by RuBisCO. By increasing CO2 supplies and thereby suppressing photorespiration, plant CCMs began evolving from C3 species, probably in the early Oligocene (circa 30ma) in response to decreasing CO2 levels, as an efficient way of increasing photosynthesis in the more challenging environmental conditions (Sage, 2004). The evolutionary success of C4 photosynthesis and the observation of increased carbon assimilation in some C3 crops under elevated CO2 (Xu et al., 2016; Thompson et al., 2017) have stimulated research into the possibility of incorporating CCMs into C3 plant species via genetic modification (McGrath and Long, 2014; Jurić et al., 2019; Kubis and Bar-Even, 2019; Atkinson et al., 2020).
Selective abiotic forces have determined the evolution of C4 photosynthesis over a number of distinct phases (Sage et al., 2018). C2 photosynthesis, a crucial step in the early stages of this evolution (Sage et al., 2012; Bräutigam and Gowik, 2016), is characterized by the formation of a photorespiratory CO2 pump that utilizes the two-carbon compound, glycine, to transport and concentrate the photorespiratory CO2 into the bundle sheath cells where it can be re-fixed by RuBisCO. The processes involved in elevating levels of photorespiration relative to photosynthesis in C3 plants are, therefore, a fundamentally important selection factor initiating the evolution to C4 photosynthesis (Sage et al., 2018). This ratio can be modelled using the rate, or velocity, v, of the oxygenase relative to the carboxylase catalyzed reaction, given by (Laing et al., 1974; Jordan and Ogren, 1984)
where O and C are, respectively, the O2 and CO2 concentrations at carboxylation sites, and SC/O is the specificity of the carboxylase relative to the oxygenase reaction which can be expressed in various algebraic forms as
In an obvious notation, the RuBisCO kinetic parameters V and K denote the maximum catalytic (turnover) rates (Vmax or kcat) and Michaelis constant (KM), respectively. A recent data compilation contains RuBisCO kinetic parameters (at 25° C) from over 300 species (Flamholz et al., 2019), with more than 50% derived from higher plants. Of these plant species, the ubiquitous C3 plants constitute by far the largest sample, followed by around 40 examples of C4 plants, while samples of C3-C4 intermediate (extant plants exhibiting C2 photosynthesis on the pathway to C4) and some C4-like plants from Flaveria, a genus adopted as a model for the evolutionary pathway of C4 photosynthesis (McKown et al., 2005; Kapralov et al., 2011; Sage et al., 2013; Schulze et al., 2013; Mallmann et al., 2014), and other miscellaneous plants, including examples of CCM-containing plants that follow the crassulacean acid metabolism pathway, are fewer in number.
The reduction of atmospheric CO2 levels and associated increase in O2 would have undoubtedly enforced adaptation of plants to increased photorespiration over geological time. Increasing temperature also increases vO/C (Jordan and Ogren, 1984) by decreasing both SC/O and the ratio of substrate concentrations, C/O, and is an important environmental factor driving the evolution of the C4 pathway (Sage et al., 2018). However, while photorespiration is not particularly advantageous to C3 plants due to net loss of carbon, it is also important to recognize that a significant amount of photorespiratory CO2 can feed into chloroplasts (Sage and Sage, 2009; Tholen and Zhu, 2011; Busch et al., 2013), increasing the potential for the recapture of carbon for photosynthesis and thus facilitating evolution along the C4 pathway. This creates a negative feedback loop that mitigates the photorespiratory response and thus limiting the increases in vO/C. Moreover, the combined action of CA (releasing CO2 from bicarbonate) and photorespiration have been postulated to form the basis of a homeostatic mechanism that ensures a stable supply of CO2 to RuBisCO, essential for the energy efficient maintenance of photosynthesis (Riazunnisa et al., 2006; Igamberdiev and Roussel, 2012; Igamberdiev, 2015).
While the importance of abiotic environmental conditions leading to carbon restriction (reduced atmospheric CO2, higher temperatures, and lack of water) in driving evolution of the photorespiratory CO2 pump is well understood (Sage et al., 2018), the role of RuBisCO kinetic variability, which underpins SC/O, warrants further critical investigation (Sage, 2013; Sage et al., 2018). In the present study, we have attempted to delineate possible coevolutionary relationships between RuBisCO, photorespiration, and CCMs by analyzing RuBisCO kinetic parameter data for higher plant species derived from the most recent compilation (Flamholz et al., 2019). In two genera, Flaveria and Panicum, the results suggest that in addition to abiotic conditions that increase photorespiration by lowering SC/O, much lower than average SC/O in the C3 populations could also be a critically important precondition in C4 evolution. In contrast, C3 plants (e.g., Limonium) that have adapted to extreme abiotic environments are typically characterized by higher than average SC/O (Galmés et al., 2005), which compensates for the lower levels of photorespiratory CO2 (Equation 1) through higher CO2 affinity.
The data sets for our analysis were accessed from the compilation by Flamholz et al. (2019). Where there are multiple entries per species in this database, these were averaged prior to statistical analysis. The complete list of species and associated kinetic parameters used in the analysis is provided in the Supplementary Material (Supplementary Tables S1 and S2). It is worth noting here that correlations between these kinetic parameters have been recently analyzed using Phylogenetic Generalized Least Squares (PGLS) as opposed to standard least-squares regression (Bouvier et al., 2021). Standard regression analysis assumes independence of the residuals, which may not necessarily be true when looking for correlations between traits in evolutionary biology as those taxa with a more common ancestor (more related) would exhibit similar traits and hence dependent residuals. PGLS methods are often used to account for such dependencies (for a general overview of PGLS methodology, see Mundry, 2014). The PGLS study by Bouvier et al., (2021) suggests that correlations between RuBisCO kinetic parameters are over estimated by standard regression; i.e., a significant phylogenetic signal is present. Nevertheless, the covariance between KC and VC appears not affected, which is perhaps not surprising given the interdependence between K and V (Briggs and Haldane, 1925). In view of this underlying covariance present in the enzyme kinetics, which is quite distinct from phylogenetic and catalytic constraints, standard linear least-squares regression analysis was carried out on the total C3 (excluding Limonium), C3 Limonium collected from high stress (high temperature and water restricted) habitat (Galmés et al., 2014), Flaveria sample and total C4 samples. The vast majority of grass species are split fairly evenly between the closely related Panicoideae, Arundinoideae, Chloridoideae, Micrairoideae, Aristidoideae, and Danthonioideae (PACMAD) and Bamboos, Oryzoideae, and Pooideae (BOP) sister clades. Among grasses, C4 photosynthesis has evolved from C3 only in PACMAD species (for an overview, see Christin et al., 2013). As both clades are relatively well represented in the kinetic data, correlations are also examined separately for Oryza, Aegilops, Puccinellia (all BOP), and Panicum (PACMAD) species.
The estimation of effect sizes (Cumming, 2012) of primary interest in our analysis is both the differences between mean kinetic parameters and, in particular, the differences between coefficients obtained from linear least-squares regression. As by definition KM is expressed as an explicit function of Vmax (Briggs and Haldane, 1925), they are not independent variables. The Michaelis constant for the carboxylase and oxygenase reactions can be written in the general linear in Vmax form (Cummins et al., 2018a),
The coefficient of the KM intercept and coefficient of Vmax obtained by linear regression of the data can be interpreted as the sample mean values of b and m, respectively, which are functions of the rate constants for the elementary steps in the kinetic mechanism (Cummins et al., 2018a). Prior linear regression analysis of the explicit dependence of KM on Vmax indicates that KM is to some extent dependent on the value of b, which is a function of the dissociation rates (among other rate constants) of the CO2 and O2 gas substrates (Cummins et al., 2018a, 2019a).
The margin of error in the difference between two means is estimated using confidence intervals (CIs), calculated from the standard errors of the means (SE). For two independent samples of size n1 and n2 with means M1 and M2 (e.g., regression coefficients for C3 and C4 samples), the combined SE for the difference in the means (∆M) can be obtained by quadrature, giving the CI as follows:
Alternatively, quadrature may be used with fractional errors to express differences as a percentage, as
In Equations (4) and (5), Student’s inverse cumulative distribution function (t−1) is given by
The CIs can be readily interpreted in terms of p values. If │∆M│> CI, the difference may be considered “statistically significant” (null hypothesis may be rejected) at α=p. We consider that only very small p values (at best, p <0.01) should provide a reliable foundation for rejecting a null hypothesis (Cumming, 2012). The statistical analysis was generated using the Real Statistics in Excel software package (Zaiontz, 2020).
Mean values of the RuBisCO kinetic parameters are given in Table 1 for the total samples of C3 (excluding Limonium), C3 Limonium and C4 plants. Since KC differences increase in proportion to the VC (probably by around 40% of mean C3 values), carboxylase specificity (SC=VC/KC) does not make a significant contribution to differences in mean SC/O. The difference in SC/O between C3 and C4 is therefore determined largely by SO, primarily through the increase maximum turnover rate for the oxygenase reaction, VO. The transition from C3 to C4 plants is accompanied by an estimated 10–25% decrease in mean SC/O, due overwhelmingly to changes in the kinetics of the oxygenase reaction alone. Overall, the results in Table 1 are similar to those in the PGLS study (Bouvier et al., 2021); in C4 species, SC/O is lower than in C3, and both VC and KC are higher than in C3 species. The sample of C3 Limonium species differs from the main C3 sample in both higher SC and SO means, determined largely by decreases in KC and KO. The resulting mean SC/O in Limonium is 15% higher than the mean of the C3 sample.
Table 1. Sample sizes (n) and mean values of RuBisCO kinetic parameters: maximum turnover rates, Vmax (s−1), Michaelis constants, KM (μM), and specificities, S=Vmax/KM (s−1.mM−1) and relative specificities SC/O with standard errors in the means (SE) for C3, C3Limonium, and C4 plants.
Regression coefficients and their standard errors are given in Table 2 for the C3 (excluding Limonium), C3 Limonium, and C4 samples. Scatter plots of the data and lines of best fit with R2 are shown in Figure 1 for correlations between parameters from the same reaction (carboxylase or oxygenase), and in Figure 2 for correlations between carboxylase and oxygenase parameters. Results obtained for carboxylase (Figure 1A) indicate differences between carboxylation parameters in C3, C3 Limonium, and C4 plant RuBisCOs. On average, b is negligible (when compared to the product, mVmax) in the C3 plant sample. For the carboxylase reaction, the gradients (m) and intercept (b) of the lines of best fit decrease and increase, respectively, from C3 to C3 Limonium, to C4. Also of note are the lower R2 values (higher variance) for the C3 and C4 samples as compared to C3 Limonium sample.
Table 2. Results of linear regression analysis for C3 (excluding Limonium), C3Limonium, and C4 plants.
Figure 1. Correlations between parameters Vmax, KM, and S from (A,B) carboxylase and (C,D) oxygenase reactions. Scatter plots and lines (dash) of best fit with R2 for C3 (), C3 Limonium (highlighted in red), and C4 (
) plant RuBisCO parameters. Solid lines in (B; SC vs. VC) and (D; SO vs. VO) are predictions of the actual curves (Equation 7) based on coefficients (Table 2) obtained from regression of data in (A,C), respectively.
Figure 2. Correlations (A-H) between carboxylase and oxygenase kinetic parameters. Scatter plots and lines of best fit with R2 for C3 (), C3 genus Limonium (highlighted in red), and C4 (
) plant RuBisCO parameters.
The actual trend lines for S vs. Vmax (Figures 1B,D) can be calculated by expressing the specificity (Vmax/KM) in the general hyperbolic in Vmax form
and substituting m and b with the corresponding KM vs. Vmax regression coefficients given in Table 2. S increases from zero with increasing Vmax, reaching the asymptotic limit value m−1 when mVmax>>b. It is also clearly apparent that the linear equation obtained from regression of the Limonium data (with higher R2) is tangential to the predicted curves in the vicinity of the data points. That the coefficient corresponding to m−1 should approximate the mean value of S is easily verified by comparing the results in Tables 1 and 2, justifying the underlying assumptions of the regression analysis. The rate at which this limit is reached depends on m.b−1 (verifiable from the corresponding coefficients in Table 2). For carboxylase, we find that the rate of increase in S with respect to increasing Vmax is relatively fast in the sample of C3 plants, while significantly slower for C3 Limonium, and in C4 plants, the limiting value is reached very slowly. Unlike carboxylase, the regression coefficients obtained for the oxygenase reaction (Figure 1C) indicate little difference between the C3, C3 Limonium, and C4 plant groupings. In contrast to the C3 sample which shows practically no correlation between SC and VC (Figure 1B), all groups exhibit positive correlations for SO vs. VO (Figure 1D).
Varying degrees of correlation are evident between carboxylase and oxygenase kinetic parameters (Figure 2). Positive correlations are observed in both VO vs. VC (Figure 2A) and KO vs. KC (Figure 2B), although the differences between C3, C3 Limonium and C4 are not significant. Nevertheless, differences between the three groups do become apparent in many of the other correlations. In particular, very strong (R2>0.9) SC vs. SO correlations (Figure 2D) clearly distinguish the three groups. The different forms in Equation (2) suggest similarly strong linear correlations should also be obtained for VC/VO vs. KC/KO (Figure 2F). Inverting the linear equations obtained from the VC/VO vs. KC/KO regression provide hyperbolic-like functions which accurately predict the reciprocal VC/VO vs. KO/KC plots (Figure 2H) for each of the three group samples. Other correlations, SC/O vs. VC, SC/O vs. VC/O, and SC/O vs. KO/C, relevant for the discussion and interpretation of Equation (2) are also shown in Figure 2.
As shown in Figure 1, the correlation coefficients obtained from the linear regression of KM vs. Vmax in Table 2 can be used to predict the correlations in S vs. Vmax. The relatively high level of correlation in the Limonium data suggests that an accurate estimation of the curve for SC vs. SO should be obtainable using parametric equations derived from SC vs. VC and SO vs. VC. Equations for SC vs. VC and SO vs. VO are already defined (Figures 1B,D), and an equation (Figure 3C) for VO in terms of VC derived from nonlinear regression of VO vs. VC (Figure 2A) can be substituted for VO in the equation for SO vs. VO, yielding the desired equation for SO vs. VC (Figure 3B). In each case, the linear equations obtained from regression of data in Figure 3 are quite clearly tangential to the predicted curves in the vicinity of the data points. Moreover, the predicted curve for SC vs. SO (Figure 3A) is in fact very nearly linear.
Figure 3. The predicted trend (solid line) in (A) SC vs. SO for genus C3 Limonium, determined using the set of parametric equations describing the trends in SC vs. VC (Figure 1B) and (B) SO vs. VC. In (B), the solid trend line for SO vs. VC is determined by substituting (C) the hyperbolic equation obtained from the nonlinear least squares fit of the VO vs. VC, data for VO in the equation for the predicted SO vs. VO curve (Figure 1D).
For each genus, the near-linear trend predicted in Figure 3A suggests performing the linear regression for SC vs. SO with the intercept fixed at zero (Figure 4A). Further, the gradient (regression coefficient) of the regression line in this way quite accurately predicts the sample SC/O means for each of the genera (Figure 4B), which again validates our basic assertion that the coefficients of the linear regression should correspond to sample means (Cummins et al., 2018a, 2019a). Examples of genera with data for more than a few species are rather limited. In addition to Limonium, for which there is a reasonably sized sample (Table 1), C3 plant data are available for numbers of BOP species from Oryza, Aegilops, and Puccinellia. Both C3 and C4 plant data are available for Panicum, while Flaveria is the only genus for which there are parameters for C3, C4, and transitional (C3-C4 and C4-like) species (Supplementary Tables S1 and S2). The results in Figure 4A reveal a high level (R2>0.90) of SC vs. SO correlation between species within the various genera, irrespective of photosynthetic (C3 or C4) pathway. The distribution of SC/O in these genera is illustrated more clearly in Figure 4B. For the vast majority of Panicum and Flaveria species (both C3 and C4), the SC/O is more than one SD below the median of the total C3 sample.
Figure 4. (A) Plots of SC vs. SO for genera with lines of best fit passing through SC=0. (B) Diagram illustrating the variance of SC/O. The sample means are well approximated by the gradients (regression coefficient) of the trend lines in (A). The error bars correspond to 95% (α=0.05) confidence intervals. The lower shaded area, less than one SD below the median, represents around 10% of the total C3 species sample, with a mean SC/O of 83.4 (VC=2.85s−1, KC=12.1μM, SC=245s−1.mM−1, VO=1.43s−1, KO=489μM, SO=2.85s−1.mM−1).
There are some other correlations of note that distinguish the various genera (Figure 5). Significant SC/O vs. VC correlations are obtained for both Limonium and Flaveria (5A), but only for Limonium in SO vs. VC (5B). In Figure 5C, increased SC/O in Limonium, Oryza, Aegilops, and Puccinellia (exclusively C3) species correlates with increased CO2 affinity (KO/C), while for Panicum and Flaveria (predominantly C4 species), increased KO/C has little tendency to increase SC/O, which appears to be true generally for species that have evolved C4 photosynthesis (Figure 2G).
Figure 5. Scatter plots of (A) SC/O vs. VC, (B) SO vs. VC, (C) SC/O vs. KO/KC, and (D) VO vs. VC for various genera. Description of symbols used is the same as in Figure 4.
The evolutionary pathway to C4 photosynthesis necessitates extensive structural, biochemical, and genetic modifications in the ancestral C3 plants (Gowik et al., 2011). Considering the current understanding of C4 evolution has been achieved through a broad multidisciplinary approach (Sage et al., 2018), it is noteworthy that the numbers of published RuBisCO kinetic studies over the last decade have shown a steep decline (Hanson, 2016). C4 photosynthesis is a prime example of convergent evolution (Blount et al., 2018), having arisen on at least 66 occasions over the past 30ma (Sage et al., 2011), producing many thousands of species spread globally over many diverse plant families (Sage, 2016). Despite being the most extensively studied enzyme, at least in terms of kinetics (Jeske et al., 2019), compilations of the C4 RuBisCO kinetic parameters (Flamholz et al., 2019) barely scratch the surface of the total global C4, and for that matter, C3 populations.
Despite the large gaps in the available data, what exactly can be understood in relation to the coevolution of RuBisCO kinetics and C4 photosynthesis? Table 1 shows clear differences between sample means of most C3 and C4 kinetic parameters. However, it seems that the fundamental question is whether, or to what extent, these differences arise from adaptation over time along the evolutionary C4 pathway, or they are mostly traits inherited, with minimal change, from the ancestral C3 species? Comparing sample means can only provide the answer if the evolving C4 plants were randomly selected from the broader C3 population. The evidence suggests this may not be the case, as both Flaveria and Panicun C3 species exhibit SC/O values much lower than the C3 average (Figure 4B), which could well be an advantage in the early-stage evolution of C4 plants, as the first stages of C4 evolution involve establishment of the photorespiratory CO2 pump (C2 photosynthesis). Given this initial requirement for photorespiratory CO2, it would not be unexpected to find positive selection of C3 species with low SC/O.
While C4 evolution may have followed a number of different pathways (Schüssler et al., 2017), in the Flaveria model, C2 photosynthesis is associated with intermediate C3-C4 species, transitioning to C4-like in the final “optimization” stages (Sage et al., 2018); phylogenetic analysis of C3, transitional and C4 species in Flaveria (Kubien et al., 2008; Kapralov et al., 2011) reveals correlations with variation in kinetic parameters. While not so apparent in the combined C3 and C4 samples (Figure 2C), the expected negative SC/O vs. VC trend (Tcherkez et al., 2006) found in Flaveria (Kubien et al., 2008) is reproduced in Figure 5A. One interpretation of this result is that increased CO2 and decreased O2 levels favor selection of RuBisCO with lower SC and higher VC, with little change in oxygenase kinetics. This appears to be supported in Figure 5B which reveals that the trend is determined exclusively by decreasing SC (increasing KC), as SO vs. VC in Flavaria shows no correlation, so that by inference the observed reduction of SC/O with increasing VC in Flaveria must arise from SC alone. However, as we explain below, these trends in the adaptation of RuBisCO would most likely have arisen much later in the evolution of C4, well after the establishment of a functional CCM.
There is no difference between the C3 and C4 mean SC to suggest there is adaptation toward decreased carboxylation (KC) in favor of speed (VC); increased VC alone causes the increase in KC, maintaining the stability in SC (Table 1). Rather, the difference between C3 and C4 mean SC/O stems from SO alone, predominately through higher oxygenase turnover (VO). If sampling is restricted to the C3 species with SC/O less than one SD below the median (Figure 4B), the resulting mean values of VO (1.43s−1) and KO (489μM) are comparable to the corresponding values obtained for the C4 sample. The C3 species with lower SC/O and C4 species exhibit very similar oxygenase traits. These similarities suggest that the C4 plants sampled (Panicum and Flaveria) may have evolved from C3 with SC/O well below the mean of the total C3 population. Notwithstanding the other preconditions (Gowik et al., 2011), if this restriction extends more generally to the Poaceae and Asteraceae families, it alone would have significantly limited the numbers of C4 species that could have evolved. The increased mean VC observed in the C4 sample is consistent with adaptation in response to increased supply of CO2 to the enzyme following development of the CCM, decreasing the selection pressure to optimize the oxygenase reaction (VO, KO) and carboxylation (KC) traits.
Although the mitigation of photorespiration is seen as a pathway for improving crop yields, it is well recognized that under some conditions it is likely essential for healthy plant growth (Betti et al., 2016). Photorespiration can protect photosynthesis from light damage and help maintain cellular redox balance as well as plant immune responses (Voss et al., 2013). While the current evidence is largely circumstantial (Ratcliffe, 2018), another study suggests that photorespiration may not waste as much energy a first thought and enhances nitrate assimilation (Bloom and Lancaster, 2018). The scavenging of photorespiratory CO2 in plant cells helps maintain chloroplast C levels in C3 photosynthesis (Sage and Sage, 2009; Tholen and Zhu, 2011; Busch et al., 2013). The flow of chloroplast CO2 should be sufficient to occupy all available RuBisCO sites (Igamberdiev, 2015). When ambient CO2 decreases to lower than normal levels (as under extreme climatic conditions), CA may be unable to produce enough CO2 from the reservoir of bicarbonate to fuel RuBisCO, resulting in the underutilization of the energy produced by the light reactions, but this can be mitigated by the supply of photorespiratory CO2 (Igamberdiev, 2015). Moreover, the efficient operation of C3 photosynthesis may require that fluctuations in O and C be contained within certain limits (Roussel and Igamberdiev, 2011), which we expect would then tend to limit vO/C (Equation 1). Based on these considerations, we might posit that vO/C should also be maintained within certain limits. The availability of photorespiratory CO2 to chloroplasts supports a homeostatic mechanism that helps renormalizes vO/C and CO2 levels by negative feedback of photorespiratory CO2 into chloroplasts in response to decreasing C levels.
Apart from substrate concentrations (C and O), the other factor that determines vO/C is SC/O, which must then also be somehow constrained to keep vO/C within certain limits. Loosely correlated changes in SC and SO, which may result in an increase in one and a decrease in the other, have the potential to produce much larger variations in SC/O than are observed (Figure 2D). The strong (R2>0.90) positive correlations between SC and SO produce tightly constrained SC/O variability (Figure 2D), particularly within C3 genera (Figure 4A). An increase in SC is accompanied by a proportionate increase in SO, facilitating the containment of vO/C within the limits required for efficient photosynthesis. Such correlations between kinetic traits have usually been attributed to constraints inherent in RuBisCO’s catalytic mechanism (Tcherkez et al., 2006; Tcherkez, 2013, 2015; Flamholz et al., 2019), which we consider in detail below. However, another recent study suggests phylogenetic, rather than catalytic (or mechanistic), constraints have largely determine RuBisCO adaptation (Bouvier et al., 2021).
A fundamental understanding of correlations between RuBisCO parameters requires consideration of its kinetic mechanism (Figure 6), together with a knowledge of the functional dependence of Vmax, m and b in Equation (3) on the rate constants (ki) for the elementary steps in both carboxylase and oxygenase reactions. As indicated in Figure 6, Vmax, m and b share a number of ki associated with product turnover, while only m and b depend on the ki that determine substrate affinity. Correlations between kinetic traits arising out of RuBisCOs mechanism must derive from correlations between the ki. However, the ki are unknown quantities, difficult if not impossible to determine empirically with any certainty, and without simplifying assumptions (Tcherkez et al., 2006), complexity of the functional relationships hinders efforts to uncover such correlations (for details of the actual kinetic equations, see Cummins et al., 2018a). Nevertheless, several important observations can be made based on the results of the present analysis.
Figure 6. The kinetic mechanism of RuBisCO. RuBisCO processes the three substrates, ribulose-1,5-bisphosphate (RuBP), and CO2 or O2, the complete reactions taking place over several stages. RuBP (R) binds first, forming a complex (ER) with the activated form of the enzyme (E), followed by enolization of RuBP (ER*) which facilitates binding with the CO2 (C) or O2 (O) molecule to R* forming the ERC or ERO enzyme-substrate complexes. The 6-carbon compound formed by the addition of carbon dioxide to RuBP breaks apart forming a product complex (EP) which dissociates into two molecules of the 3-carbon compound (P), 3-phosphoglyceric acid (3PGA). Oxygenation proceeds through analogous steps except that the dissociation products (X) are one 3PGA molecule and one of 2-phospho-glycolate (2PG) to be recycled into 3PGA by photorespiration, producing CO2 which can be made available for photosynthesis. The rate constants can be associated with turnover of product or affinity of CO2 or O2 substrate molecules for RuBP. The functional dependence of Vmax, m and b in KM (Equation 3) on the elementary rate constants is indicated for each of the reactions. A derivation of the actual kinetic equations is given in the Supplementary Material.
The absence of correlation between SC and VC in the C3 sample (Figure 1B) demonstrates that the ki on which VC depends are not correlated to that associated with CO2 affinity for enolized RuBP (k5). When mVC>>b, SC converges asymptotically to the mean value for the sample. Compared to C3 Limonium and C4 plants, SC converges very quickly to this mean value for the C3 sample (Table 1), and so the dependence of KC on b, which depends on the decarboxylation rate (k6), may be neglected. This would suggest a lesser significance of k6 in C3 species, resolving to some extent the apparent differences of opinion on the issue of decarboxylation (Tcherkez et al., 2018; Cummins et al., 2019a). Nevertheless, the results suggest significant (mVmax ≈ b) decarboxylation in both C3 Limonium and C4 samples (Figure 1A) and deoxygenation (k12, i.e., breakdown of the Michaelis complex by dissociation of O2) in all three (Figure 1C).
Correlations between carboxylase and oxygenase reactions are not precluded. The carboxylase and oxygenase reactions are preceded by enolization of the bound RuBP required for activation of CO2 or O2 binding, which has been shown to co-limit Vmax (Tcherkez et al., 2013). As a consequence of this co-limitation, the forward rate constant (k3) for the enolization of RuBP is a common factor of VC and VO, which effectively couples the two reaction rates. However, the actual correlation observed between VC and VO is overall weak (Figure 2A) and variable between genera (Figure 5D), with only Limonium and Aegilops (both C3) exhibiting a moderate level of correlation. Consequently, these correlations are more likely due to adaptation, rather than by a tradeoff enforce by RuBisCO’s mechanism. In Limonium, the linear correlation between VC and VO maintains a more or less constant ratio VC/O (in the range 2–3; Figure 2E), limiting variation in SC/O, and hence photorespiration, to mainly the ratio of KMs, i.e., KO/C (Figure 2G).
In contrast, the positive correlations between SC and SO seem to establish a manifest constraint between carboxylase and oxygenase (Figures 2D, 4A) kinetic parameters. The correlation becomes stronger (as measured by R2) the more closely related the species; R2 values tend to be somewhat higher within genera (Figure 4A), than within the general C3, C4, or total plant sample (Figure 2D). The positive correlation seems to be preserved in mutants when the changes in kinetic parameters are relatively small (Genkov et al., 2010), while breaks for mutations that cause large perturbations to the kinetics, sometimes resulting in decreased SC and increased SO, i.e., increasing photorespiration (Whitney et al., 1999). This tends to suggest some level of correlation between carboxylase and oxygenase ki, most likely with those associated with substrate affinity (Figure 6). Both substrates present similar electrostatic potentials to the RuBisCO active site (Kannappan and Gready, 2008), so that the binding of CO2 to enolized RuBP induces a redistribution of charge similar to that induced by O2 binding (Cummins et al., 2018b; Kannappan et al., 2019; Bathellier et al., 2020; Cummins and Gready, 2020) which will then interact similarly with the external (to the active site) electrostatic field, which is thought to be the primary driver in enzyme catalysis (Warshel et al., 2006; Fried and Boxer, 2017). Moreover, evolutionary changes in this electrostatic field have been linked to RuBisCO substrate specificity (Poudel et al., 2020) This electrostatic field would change slightly with sequence variation outside the highly conserved active site to produce the small free energy changes required to maintain the correlation between SC and SO when mutations occur. However, other types of biophysical constraints may limit the fitness of some mutations (Studer et al., 2014; Duraõ et al., 2015), and while superior traits are still being discovered (Davidi et al., 2020), the practical limits of RuBisCO’s kinetic variability remain unclear.
The effect of abiotic environmental stress on RuBisCO in C3 plants may be succinctly rationalized in terms of the ratios of Vmax, VC/O=VC/VO, and KMs, KO/C=KO/KC (VC/O vs. KO/C in Figure 2H). Both the carboxylase and oxygenase reactions produce 3PGA (Figure 6), although RuBisCO processes the primary substrate (CO2) more efficiently than O2 due to superior kinetic traits (Vmax, KM) and the fact that one of the oxygenase products (2PG) has to be reprocessed into 3PGA by photorespiration, albeit costing a certain amount of additional energy and carbon. On the other hand, the additional CO2 produced as a byproduct in photorespiration may be reutilized in photosynthesis if captured by chloroplasts. Thus VC/O seems to strike a practical balance between photosynthesis and photorespiration as a measure of 3PGA production efficiency; the higher VC/O the more energy efficiently 3PGA is produced. According to the familiar form of the classical Michaelis-Menten (MM) equation (Michaelis and Menten, 1913; Briggs and Haldane, 1925; Michaelis et al., 2011),
when the reaction rate (v) reaches half of Vmax, KM is equivalent to the concentration of substrate, [S]. Consequently, for a given Vmax, a substrate with a lower value of KM saturates the enzyme with a smaller concentration of substrate. Actually (in vivo), the rate of carbon assimilation in plants typically deviates from the classical MM curve (Equation 8) reaching only about 50% of VC (Laisk, 1985; Laisk and Oja, 1998; Ruuska et al., 1998) due to some other limiting factors (Igamberdiev, 2015). To reach saturation, lower values of KC require less CO2, and higher values of KO more O2. In terms of the RuBisCO kinetic mechanism (Figure 6), lower KC can be best achieved by increasing CO2 affinity (k5/k6), and higher KO by decreasing O2 affinity (k11/k12) for the enolizied form of RuBP.
The curvature of the predicted trend lines in Figure 7 is largely determined by the explicit linear dependence of KM on Vmax (Equation 3); increasing VC/O produces the monotonic decrease in KO/C. There is no obvious explanation for the divergence of KO/C, and hence SC/O, curves between C3 Limonium and the mainstream C3 species at lower VC/O as arising from mechanistic constrains imposed by the enzyme. Alternatively, it is posited that the observed trends arise from adaptation of RuBisCO in response to changes in chloroplast carbon (C) levels according to the prevailing environmental conditions. Under more temperate conditions (applicable to most of the C3 sample), in species with less than the mean VC/O of about three (Table 1), C can be supplemented by increased photorespiration (decreasing SC/O). Increasing SC/O reduces photorespiration and hence the maintenance of C, requiring increased KO/C (higher CO2 relative to O2 affinity) to maintain sustainable levels of photosynthesis under high stress at the lower end of VC/O. In fact, a unique study provides some empirical evidence that those (Limonium) species with higher SC/O are associated with reduced C (Galmés et al., 2014).
Figure 7. The effect of abiotic environmental stress on RuBisCO kinetics in C3 plants. The trend lines (Figure 2H) in relative specificity, SC/O, are obtained by the product of VC/O=VC/VO, a measure of 3PGA product turnover efficiency, and KO/C=KO/KC, a measure of CO2 relative to O2 affinity for enolizied RuBP. The efficiency of C3 photosynthesis also relies on the constant supply of CO2 to RuBisCO, which photorespiration can help maintain. Abiotic stress factors increase the photorespiration relative to photosynthesis (Equation 1). Most C3 species, with more or less average SC/O, are situated in usually low stress (or temperate) habitats; however, some level of photorespiratory CO2 may be necessary to maintain photosynthesis during short periods of increased stress (Igamberdiev, 2015). Some C3 plants can leverage off the additional photorespiratory CO2 produced by their much lower than average SC/O in high stress environments (C2 photosynthesis). Some of these C2 plants may go on to evolve fully developed carbon concentrating mechanisms (C4 photosynthesis). Alternatively, the much higher than average SC/O in other C3 plants, while mitigating photorespiration, may compensate for the carbon restriction (reduced levels of chloroplast CO2) associated with high stress environments by gains in CO2 relative to O2 affinity.
As VC/O increases from its mean toward the maximum value, the requirement for additional carbon appears to diminish; photorespiratory CO2 declines with a drift toward increased SC/O. Thus, there is a positive correlation between VC/O and photorespiratory C, which is also clearly apparent in C4 evolution where the establishment of increased C levels (by the CCM) is followed by optimization of VC. Increased throughput of product can only be maintained by increased supply of CO2 substrate, necessitating the adaptation of RuBisCO kinetic traits. Most of the C3 sample is tightly clustered about the mean VC or VC/O (Figures 2C,E). If VC/O falls much below the mean, C requires supplementation depending on environmental conditions, either by increased photorespiration (low stress) or by increased CO2 relative to O2 affinity (high stress), necessitating RuBisCO accommodate an expansive range of SC/O. As discussed, C4 plants have likely evolved only from C3 with below average SC/O, and this appears to be supported by the parallel trends in C3 and C4 illustrated in Figure 7. C3 species with the minimal values of SC/O (lower values for KO/C) produce additional photorespiratory CO2 under high stress conditions, as required for the evolution of C2 and C4 photosynthesis. The CCM maintains consistently higher C levels, regardless of stress factors, reducing the pressure on RuBisCO to adapt, so that its kinetics have remained, except perhaps for a tendency toward higher VC, relatively unchanged by evolution.
Analysis of the RuBisCO kinetic data presented here suggests that the evolution of kinetic parameters in higher plants, rather than being highly constrained or subjected to tradeoffs imposed by the enzyme’s kinetic mechanism, has adapted to variations in photorespiration as part the homeostatic maintenance of a constant CO2 supply to the enzyme under disparate environmental conditions. The positive correlations observed between SC and SO, particularly between phylogenetically related species, reflect similarities in the physical binding properties of the two substrates CO2 and O2 to RuBP, which serve to contain SC/O within the limits required for maintaining balance between photosynthesis and photorespiration in the regulation of carbon flux when mutations occur. Significantly, the limitation on product turnover (VC) is the extent to which RuBisCO kinetics can adapt to the availability of carbon. Apparent tradeoffs between turnover and specificity are not symptomatic of an inefficient enzyme, but reflects the necessary adaptation of a flexible one to the changing levels of accessible CO2 as a consequence of changes in abiotic environmental conditions.
Over the past 30ma, the evolution of C4 photosynthesis has dramatically reduced photorespiration in a relatively small number (a few percent) of plant species by maintaining high levels of chloroplast CO2, although somewhat paradoxically high levels of photorespiration were instrumental at the beginning the evolutionary process. The C4 RuBisCOs in the Panicum and Flaveria samples do not exhibit the “average” C3 kinetics, but inherit traits largely unchanged from a small proportion of the ancestral C3 population (i.e., those with much lower than the mean SC/O and, therefore, increased photorespiration). Nevertheless, differences in leaf anatomy and biochemistry indicate that a C4 plant is not simply a C3 with an attached CCM, and prodigious efforts to artificially introduce CCMs into commercial C3 crops are ongoing but have yet to bear fruit. Recent modeling suggests that achieving C2 photosynthesis in rice may be a more realistic goal (Bellasio and Farquhar, 2019).
While this research continues, however, are there any prospects for reengineering C3 RuBisCOs with the objective of improving its kinetic traits? Despite decades of research, while some progress has been made through directed evolution, a demonstrably better RuBisCO for agriculture also remains elusive (Zhou and Whitney, 2019). What is meant by a “better” RuBisCO needs to be carefully defined, and the traits to be improved should at least, if not necessarily improve yield under the prevailing settings, increase the fitness of a species to survive the expected increases in the frequency and severity of hot and dry weather events over the coming decades. In this regard, C4 plants demonstrate the importance of a secure supply of carbon under such climatic conditions, and the evolution of increases in product turnover (VC), although perhaps modest compared to VC found in other taxa (Davidi et al., 2020), may well only have been maintained by the increased availability of carbon provided by the CCM. C3 plants with higher SC/O suffer from the concomitant reduction in the contributions to chloroplast CO2 levels that would otherwise had been made by photorespiration. In these cases, increasing the affinity of CO2 relative to O2 for RuBP makes more efficient uptake of the meager supply of carbon under high stress environmental conditions. In the absence of a functional CCM, the reengineering of RuBisCOs with increased SC/O, if possible, may help to futureproof C3 crops in a rapidly changing climate.
Publicly available data sets were analyzed in this study. This data can be found at: https://pubs.acs.org/doi/abs/10.1021/acs.biochem.9b00237/supplfile/bi9b00237si005.xlsx.
The author confirms being the sole contributor of this work and has approved it for publication.
This research did not receive any specific grant from funding agencies in the public, commercial, or not-for-profit sectors.
The author declares that the research was conducted in the absence of any commercial or financial relationships that could be construed as a potential conflict of interest.
All claims expressed in this article are solely those of the authors and do not necessarily represent those of their affiliated organizations, or those of the publisher, the editors and the reviewers. Any product that may be evaluated in this article, or claim that may be made by its manufacturer, is not guaranteed or endorsed by the publisher.
The Supplementary Material for this article can be found online at: https://www.frontiersin.org/articles/10.3389/fpls.2021.662425/full#supplementary-material, www.real-statistics.com, www.c4rice.com
Andralojc, P. J., Carmo-Silva, E., Degen, G. E., and Parry, M. A. J. (2018). Increasing metabolic potential: C-fixation. Essays Biochem. 62, 109–118. doi: 10.1042/EBC20170014
Atkinson, N., Mao, Y., Chan, K. X., and McCormick, A. J. (2020). Condensation of Rubisco into a proto-pyrenoid in higher plant chloroplasts. Nat. Commun. 11:6303. doi: 10.1038/s41467-020-20132-0
Bar-Even, A., Noor, E., Savir, Y., Liebermeister, W., Davidi, D., Tawfik, D. S., et al. (2011). The moderately efficient enzyme: evolutionary and physicochemical trends shaping enzyme parameters. Biochemistry 50, 4402–4410. doi: 10.1021/bi2002289
Bathellier, C., Li-Juan Yu, L.-J., Farquhar, G. D., Michelle, L., Coote, M. L., Lorimer, G. H., et al. (2020). Ribulose 1,5-bisphosphate carboxylase/oxygenase activates O2 by electron transfer. Proc. Natl. Acad. Sci. doi: 10.1073/pnas.2008824117
Bellasio, C., and Farquhar, G. (2019). A leaf-level biochemical model simulating the introduction of C2 and C4 photosynthesis in C3rice: gains, losses and metabolitefluxes. New Phytol. 223, 150–166. doi: 10.1111/nph.15787
Betti, M., Bauwe, H., Busch, F. A., Fernie, A. R., Keech, O., Levey, M., et al. (2016). Manipulating photorespiration to increase plant productivity: recent advances and perspectives for crop improvement. J. Exp. Bot. 67, 2977–2988. doi: 10.1093/jxb/erw076
Bloom, A. J., and Lancaster, K. M. (2018). Manganese binding to Rubisco could drive a photorespiratory pathway that increases the energy efficiency of photosynthesis. Nat. Plants 4, 414–422. doi: 10.1038/s41477-018-0191-0
Blount, Z. D., Lenski, R. E., and Losos, J. B. (2018). Contingency and determinism in evolution: replaying life’s tape. Science 362:eaam5979. doi: 10.1126/science.aam5979
Bouvier, J. W., Emms, D. M., Rhodes, T., Nielsen, J. R., Bolton, J. S., Eddershaw, A., et al. (2021). Rubisco adaptation is more limited by phylogenetic constraint than by catalytic trade-off. Mol. Biol. Evol. 25, 2880–2896. doi: 10.1093/molbev/msab079
Bräutigam, A., and Gowik, U. (2016). Photorespiration connects C3 and C4 photosynthesis. J. Exp. Bot. 67, 2953–2962. doi: 10.1093/jxb/erw056
Briggs, G. E., and Haldane, J. B. S. (1925). A note on the kinetics of enzyme action. Biochem. J. 19, 338–339. doi: 10.1042/bj0190338
Busch, F. A., Sage, T. L., Cousins, A. B., and Sage, R. F. (2013). C3 plants enhance rates of photosynthesis by reassimilating photorespired and respired CO2. Plant Cell Environ. 36, 200–212. doi: 10.1111/j.1365-3040.2012.02567.x
Christin, P. A., Osborne, C. P., Chatelet, D. S., Columbus, J. T., Besnard, G., Hodkinson, T. R., et al. (2013). Anatomical enablers and the evolution of C4 photosynthesis in grasses. Proc. Natl. Acad. Sci. U. S. A. 110, 1381–1386. doi: 10.1073/pnas.1216777110
Cleland, W. W., Andrews, T. J., Gutteridge, S., Hartmann, F. C., and Lorimer, G. H. (1998). Mechanism of Rubisco: the carbamate as general base. Chem. Rev. 98, 549–562. doi: 10.1021/cr970010r
Cumming, G. (2012). Understanding the New Statistics: Effect Sizes, Confidence Interval and Meta Analysis. Taylor and Francis, New York, 53–117.
Cummins, P. L., Kannappan, B., and Gready, J. E. (2018a). Directions for optimization of photosynthetic carbon fixation: Rubisco's efficiency may not be so constrained after all. Front. Plant Sci. 9:183. doi: 10.3389/fpls.2018.00183
Cummins, P. L., Kannappan, B., and Gready, J. E. (2018b). Revised mechanism of carboxylation of ribulose-1,5-biphosphate by Rubisco from large scale quantum chemical calculations. J. Comput. Chem. 39, 1656–1665. doi: 10.1002/jcc.25343
Cummins, P. L., Kannappan, B., and Gready, J. E. (2019a). Response: commentary: directions for optimization of photosynthetic carbon fixation: Rubisco’s efficiency may not be so constrained after all. Front. Plant Sci. 10:1426. doi: 10.3389/fpls.2019.01426
Cummins, P. L., Kannappan, B., and Gready, J. E. (2019b). Ab initio molecular dynamics simulation and energetics of the ribulose-1,5-biphosphate carboxylation reaction catalyzed by Rubisco: toward elucidating the stereospecific protonation mechanism. J. Phys. Chem. B 123, 2679–2686. doi: 10.1021/acs.jpcb.8b12088
Cummins, P. L., and Gready, J. E. (2020). Kohn-Sham density functional calculations reveal proton wires in the enolization and carboxylase reactions catalyzed by Rubisco. J. Phys. Chem. B 124, 3015–3026. doi: 10.1021/acs.jpcb.0c01169
Davidi, D., Shamshoum, M., Guo, Z., Bar-On, Y. M., Prywes, N., Oz, A., et al. (2020). Highly active Rubiscos discovered by systematic interrogation of natural sequence diversity. EMBO J. 39:e104081. doi: 10.15252/embj.2019104081
Duraõ, P., Aigner, H., Nagy, P., Mueller-Cajar, O., Hartl, F. U., and Hayer-Hartl, M. (2015). Opposing effects of folding and assembly chaperones on evolvability of 694 Rubisco. Nat. Chem. Biol. 11, 148–155. doi: 10.1038/nchembio.1715
Erb, T. J., and Zarzycki, J. (2018). A short history of RubisCO: the rise and fall (?) of nature's predominant CO2 fixing enzyme. Curr. Opin. Biotechnol. 49, 100–107. doi: 10.1016/j.copbio.2017.07.017
Fernie, A. R., Bachem, C. W. B., Helariutta, Y., Neuhaus, E., Prat, S., Ruan, Y.-L., et al. (2020). Synchronization of developmental, molecular and metabolic aspects of source–sink interactions. Nat. Plants 6, 55–66. doi: 10.1038/s41477-020-0590-x
Flamholz, A. I., Prywes, N., Moran, U., Davidi, D., Bar-On, Y. M., Oltrogge, L. M., et al. (2019). Revisiting tradeoffs in Rubisco kinetic parameters. Biochemistry 58, 3365–3376. doi: 10.1021/acs.biochem.9b00237
Fried, S. D., and Boxer, S. G. (2017). Electric fields and enzyme catalysis. Annu. Rev. Biochem. 86, 387–415. doi: 10.1146/annurev-biochem-061516-044432
Gomez-Fernandez, B. J., Garcia-Ruiz, E., Martin-Diaz, J., Gomez de Santos, P., Santos-Moriano, P., Plou, F. J., et al. (2018). Directed -in vitro- evolution of precambrian and extant Rubiscos. Sci. Rep. 8:5532. doi: 10.1038/s41598-018-23869-3
Galmés, J., Flexas, J., Keys, A. J., Cifre, J., Mitchell, R. A. C., Madgwick, P. J., et al. (2005). Rubisco specificity factor tends to be larger in plant species from drier habitats and in species with persistent leaves. Plant Cell Environ. 28, 571–579. doi: 10.1111/j.1365-3040.2005.01300.x
Galmés, J., Andralojc, P. J., Kapralov, M. V., Flexas, J., Keys, A. J., Molins, A., et al. (2014). Environmentally driven evolution of Rubisco and improved photosynthesis and growth within the C3 genus Limonium (Plumbaginaceae). New Phytol. 203, 989–999. doi: 10.1111/nph.12858
Genkov, T., Meyer, M., Griffiths, H., and Spreitzer, R. J. (2010). Functional hybrid rubisco enzymes with plant small subunits and algal large subunits: engineered rbcS cDNA for expression in chlamydomonas. J. Biol. Chem. 285, 19833–19841. doi: 10.1074/jbc.M110.124230
Goudet, M., Orr, D., Melkonian, M., Muller, K., Meyer, M., Carmo-Silva, E., et al. (2020). Rubisco and carbon concentration mechanism (CCM) co-evolution across Chlorophytes and Streptophytes. New Phytol. 227, 810–823. doi: 10.1111/nph.16577
Gowik, U., Bräutigam, A., Weber, K. L., Weber, A. P. M., and Westhoff, P. (2011). Evolution of C4 photosynthesis in the genus Flaveria: how many and which genes does it take to make C4? Plant Cell 23, 2087–2105. doi: 10.1105/tpc.111.086264
Hanson, D. T. (2016). Breaking the rules of Rubisco catalysis. J. Exp. Bot. 67, 3180–3182. doi: 10.1093/jxb/erw197
Igamberdiev, A. U. (2015). Control of Rubisco function via homeostatic equilibration of CO2 supply. Front. Plant Sci. 6:106. doi: 10.3389/fpls.2015.00106
Igamberdiev, A. U., and Roussel, M. R. (2012). Feedforward non-Michaelis–Menten mechanism for CO2 uptake by Rubisco: contribution of carbonic anhydrases and photorespiration to optimization of photosynthetic carbon assimilation. Biosystems 107, 158–166. doi: 10.1016/j.biosystems.2011.11.008
Iñiguez, C., Capó-Bauçà, S., Niinemets, Ü., Stoll, H., Aguiló-Nicolau, P., and Galmés, J. (2020). Evolutionary trends in Rubisco kinetics and their co-evolution with CO2 concentrating mechanisms. Plant J. 101, 897–918. doi: 10.1111/tpj.14643
Jordan, D. B., and Ogren, W. L. (1984). The CO2/O2 specificity of ribulose 1,5-bisphosphate carboxylase oxygenase: dependence on ribulosebisphosphate concentration, pH and temperature. Planta 161, 308–313. doi: 10.1007/BF00398720
Jeske, L., Placzek, S., Schomburg, I., Chang, A., and Schomburg, D. (2019). BRENDA in 2019: a European ELIXIR core data resource. Nucleic Acids Res. 47, D542–D549. doi: 10.1093/nar/gky1048
Jurić, I., Hibberd, J. M., Blatt, M., and Burroughs, N. J. (2019). Computational modelling predicts substantial carbon assimilation gains for C3 plants with a single-celled C4 biochemical pump. PLoS Comput. Biol. 15:e1007373. doi: 10.1371/journal.pcbi.1007373
Kannappan, B., Cummins, P. L., and Gready, J. E. (2019). Mechanism of oxygenase pathway reactions catalyzed by Rubisco from large scale Kohn-Sham density functional calculations. J. Phys. Chem. B 123, 2833–2843. doi: 10.1021/acs.jpcb.9b00518
Kannappan, B., and Gready, J. E. (2008). Redefinition of rubisco carboxylase reaction reveals origin of water for hydration and new roles for active-site residues. J. Am. Chem. Soc. 130, 15063–15080. doi: 10.1021/ja803464a
Kapralov, M. V., Kubien, D. S., Andersson, I., and Filatov, D. A. (2011). Changes in Rubisco kinetics during the evolution of C4 photosynthesis in Flaveria (Asteraceae) are associated with positive selection on genes encoding the enzyme. Mol. Biol. Evol. 28, 1491–1503. doi: 10.1093/molbev/msq335
Kubien, D. S., Whitney, S. M., Moore, P. V., and Jesson, L. K. (2008). The biochemistry of Rubisco in Flaveria. J. Exp. Bot. 59, 1767–1777. doi: 10.1093/jxb/erm283
Kubis, A., and Bar-Even, A. (2019). Synthetic biology approaches for improving photosynthesis. J. Exp. Bot. 70, 1425–1433. doi: 10.1093/jxb/erz029
Laisk, A. (1985). “Kinetics of photosynthetic CO2 uptake in C3 plants,” in Kinetics of Photosynthetic Carbon Metabolism. eds. J. Viil, G. Grishina, and A. Laisk (Tallinn: Valgus Press), 21–34.
Laisk, A., and Oja, V. (1998). Dynamics of Leaf Photosynthesis: Rapid Response Measurements and Their Interpretations. Melbourne: CSIRO Publishing.
Laing, W. A., Ogren, W. L., and Hageman, R. H. (1974). Regulation of soybean net photosynthetic CO2 fixation by the interaction of CO2, O2 and ribulose 1,5-bisphosphate carboxylase. Plant Physiol. 54, 678–685. doi: 10.1104/pp.54.5.678
Lawson, T., and Flexus, J. (2020). Fuelling life: recent advances in photosynthesis research: editorial. Plant J. 101, 753–755. doi: 10.1111/tpj.14698
Lin, M. T., Stone, W. D., Chaudhari, V., and Hanson, M. R. (2020). Small subunits can determine enzyme kinetics of tobacco Rubisco expressed in Escherichia coli. Nat. Plants 6, 1289–1299. doi: 10.1038/s41477-020-00761-5
Mallmann, J., Heckmann, D., Bräutigam, A., Lercher, M. J., Weber, A. P., Westhoff, P., et al. (2014). The role of photorespiration during the evolution of C4 photosynthesis in the genus Flaveria. ELife 3:e02478. doi: 10.7554/eLife.02478
McGrath, J. M., and Long, S. P. (2014). Can the cyanobacterial carbon-concentrating mechanism increase photosynthesis in crop species? Plant Physiol. 164, 2247–2261. doi: 10.1104/pp.113.232611
McKown, A. D., Moncalvo, J. M., and Dengler, N. G. (2005). Phylogeny of Flaveria (Asteraceae) and inference of C4 photosynthesis evolution. Am. J. Bot. 92, 1911–1928. doi: 10.3732/ajb.92.11.1911
Michaelis, L., Menten, M. L., Johnson, K. A., and Goody, R. S. (2011). The original Michaelis constant: translation of the 1913 Michaelis-Menten paper. Biochemistry 50, 8264–8269. doi: 10.1021/bi201284u
Mundry, R. (2014). “Statistical Issues and Assumptions of Phylogenetic Generalized Least Squares,” in Modern Phylogenetic Comparative Methods and Their Application in Evolutionary Biology. ed. L. Z. Garamszegi (Springer-Verlag Berlin Heidelberg), 131–153.
Niinemets, U., Berry, J. A., von Caemmerer, S., Ort, D. R., Parry, M. A. J., and Poorter, H. (2017). Photosynthesis: ancient, essential, complex, diverse … and in need of improvement in a changing world. New Phytol. 213, 43–47. doi: 10.1111/nph.14307
Poudel, S., Pike, D. H., Raanan, H., Mancini, J. A., Nanda, V., Rickaby, R. E. M., et al. (2020). Biophysical analysis of the structural evolution of substrate specificity in Rubisco. Proc. Natl. Acad. Sci. 117, 30451–30457. doi: 10.1073/pnas.2018939117
Ratcliffe, G. (2018). Faculty opinions recommendation of Bloom A.J. and Lancaster K.M., Nat. Plants. Fac. Opin. 4, 414–422. doi: 10.3410/f.733575302.793548683
Riazunnisa, K., Padmavathi, L., Bauwe, H., and Raghavendra, A. S. (2006). Markedly low requirement of added CO2 for photosynthesis by mesophyll protoplasts of pea (Pisum sativum): possible roles of photorespiratory CO2 and carbonic anhydrase. Physiol. Plant. 128, 763–772. doi: 10.1111/j.1399-3054.2006.00803.x
Roussel, M. R., and Igamberdiev, A. U. (2011). Dynamics and mechanisms of oscillatory photosynthesis. Biosystems 103, 230–238. doi: 10.1016/j.biosystems.2010.07.020
Ruuska, S. A., Andrews, T. J., Badger, M. R., Hudson, G. S., Laisk, A., Price, G. D., et al. (1998). The interplay between limiting processes in C3 photosynthesis studied by rapid response gas exchange using transgenic tobacco impaired in photosynthesis. Aust. J. Plant Physiol. 25, 859–870. doi: 10.1071/PP98079
Sage, R. F. (2004). The evolution of C4 photosynthesis. New Phytol. 161, 341–370. doi: 10.1111/j.1469-8137.2004.00974.x
Sage, R. F. (2013). Photorespiratory compensation: a driver for biological diversity. Plant Biol. 15, 624–638. doi: 10.1111/plb.12024
Sage, R. F. (2016). A portrait of the C4 photosynthetic family on the 50th anniversary of its discovery: species number, evolutionary lineages, and Hall of Fame. J. Exp. Bot. 67, 4039–4056. doi: 10.1093/jxb/erw156
Sage, T. L., and Sage, R. F. (2009). The functional anatomy of rice leaves: implications for refixation of photorespiratory CO2 and efforts to engineer C4 photosynthesis into rice. Plant Cell Physiol. 50, 756–772. doi: 10.1093/pcp/pcp033
Sage, T. L., Busch, F. A., Johnson, D. C., Friesen, P. C., Stinson, C. R., Stata, M., et al. (2013). Initial events during the evolution of C4 photosynthesis in C3 species of Flaveria. Plant Physiol. 163, 1266–1276. doi: 10.1104/pp.113.221119
Sage, R. F., Christin, P.-A., and Edwards, E. J. (2011). The C4 plant lineages of planet Earth. J. Exp. Bot. 62, 3155–3169. doi: 10.1093/jxb/err048
Sage, R. F., Monson, R. K., Ehleringer, J. R., Adachi, S., and Pearcy, R. W. (2018). Some like it hot: the physiological ecology of C4 plant evolution. Oecologia 187, 941–966. doi: 10.1007/s00442-018-4191-6
Sage, R. F., Sage, T. L., and Kocacinar, F. (2012). Photorespiration and the evolution of C4 photosynthesis. Annu. Rev. Plant Biol. 63, 19–47. doi: 10.1146/annurev-arplant-042811-105511
Savir, Y., Noor, E., Milo, R., and Tlusty, T. (2010). Cross-species analysis traces adaptation of Rubisco toward optimality in a low-dimensional landscape. Proc. Natl. Acad. Sci. U. S. A. 107, 3475–3480. doi: 10.1073/pnas.0911663107
Schulze, S., Mallmann, J., Burscheidt, J., Koczor, M., Streubel, M., Bauwe, H., et al. (2013). Evolution of C4 photosynthesis in the genus Flaveria: establishment of a photorespiratory CO2 pump. Plant Cell 25, 2522–2535. doi: 10.1105/tpc.113.114520
Schüssler, C., Freitag, H., Koteyeva, N., Schmidt, D., Edwards, G., Vosnesenskya, E., et al. (2017). Molecular phylogeny and forms of photosynthesis in tribe Salsoleae (Chenopodiaceae). J. Exp. Bot. 68, 207–223. doi: 10.1093/jxb/erw432
Sharwood, R. E., Ghannoum, O., Kapralov, M. V., Gunn, L. H., and Whitney, S. M. (2016). Temperature responses of Rubisco from Paniceae grasses provide opportunities for improving C3 photosynthesis. Nat. Plants 2:16186. doi: 10.1038/nplants.2016.186
Studer, R. A., Christin, P., Williams, M. A., and Orengo, C. A. (2014). Stability activity tradeoffs constrain the adaptive evolution of RubisCO. Proc. Natl. Acad. Sci. U. S. A. 111, 2223–2228. doi: 10.1073/pnas.1310811111
Tcherkez, G. G., Farquhar, G. D., and Andrews, T. J. (2006). Despite slow catalysis and confused substrate specificity, all ribulose bisphosphate carboxylases may be nearly perfectly optimized. Proc. Natl. Acad. Sci. U. S. A. 103, 7246–7251. doi: 10.1073/pnas.0600605103
Tcherkez, G. G. B., Bathellier, C., Stuart-Williams, H., Whitney, S., Gout, E., Bligny, R., et al. (2013). D2O solvent isotope effects suggest uniform energy barriers in ribulose1,5-bisphosphate carboxylase/oxygenase catalysis. Biochemistry 52, 869–877. doi: 10.1021/bi300933u
Tcherkez, G. (2013). Modelling the reaction mechanism of ribulose-1,5-bisphosphate carboxylase/oxygenase and consequences for kinetic parameters. Plant Cell Environ. 36, 1586–1596. doi: 10.1111/pce.12066
Tcherkez, G. (2015). The mechanism of rubisco-catalyzed oxygenation. Plant Cell Environ. 39, 983–1596. doi: 10.1111/pce.12629
Tcherkez, G. G., Bathellier, C., Farquhar, G. D., and Lorimer, G. H. (2018). Commentary: directions for optimization of photosynthetic carbon fixation: Rubisco’s efficiency may not be so constrained after all. Front. Plant Sci. 9:183. doi: 10.3389/fpls.2018.00929
Tholen, D., and Zhu, X.-G. (2011). The mechanistic basis of internal conductance: a theoretical analysis of mesophyll cell photosynthesis and CO2 diffusion. Plant Physiol. 156, 90–105. doi: 10.1104/pp.111.172346
Thompson, M., Gamage, D., Hirotsu, N., Martin, A., and Seneweera, S. (2017). Effects of elevated carbon dioxide on photosynthesis and carbon partitioning: a perspective on root sugar sensing and hormonal crosstalk. Front. Physiol. 8:578. doi: 10.3389/fphys.2017.00578
Voss, I., Sunil, B., Scheibe, R., and Raghavendra, A. S. (2013). Emerging concept for the role of photorespiration as an important part of abiotic stress response. Plant Biol. 15, 713–722. doi: 10.1111/j.1438-8677.2012.00710.x
Warshel, A., Sharma, P. K., Kato, M., Xiang, Y., Liu, H., and Olsson, M. H. M. (2006). Electrostatic basis for enzyme catalysis. Chem. Rev. 106, 3210–3235. doi: 10.1021/cr0503106
Wilson, R. H., Martin-Avila, E., Conlan, C., and Whitney, S. M. (2018). An improved Escherichia coli screen for Rubisco identifies a protein–protein interface that can enhance CO2-fixation kinetics. J. Biol. Chem. 293, 18–27. doi: 10.1074/jbc.M117.810861
Whitney, S. M., von Caemmerer, S., Hudson, G. S., and Andrews, T. J. (1999). Directed mutation of the Rubisco large subunit of tobacco influences photorespiration and growth. Plant Physiol. 121, 579–588. doi: 10.1104/pp.121.2.579
Whitney, S. M., Houtz, R. L., and Alonso, H. (2011). Advancing our understanding and capacity to engineer nature's CO2-sequestering enzyme, Rubisco. Plant Physiol. 155, 27–35. doi: 10.1104/pp.110.164814
Xu, Z., Jiang, Y., Jia, B., and Zhou, G. (2016). Elevated-CO2 response of stomata and its dependence on environmental factors. Front. Plant Sci. 7:657. doi: 10.3389/fpls.2016.00657
Keywords: ribulose-1,5-bisphosphate carboxylase/oxygenase, photorespiration, carbon concentrating mechanism, photosynthesis, evolution, homeostasis, climate change
Citation: Cummins PL (2021) The Coevolution of RuBisCO, Photorespiration, and Carbon Concentrating Mechanisms in Higher Plants. Front. Plant Sci. 12:662425. doi: 10.3389/fpls.2021.662425
Received: 01 February 2021; Accepted: 26 July 2021;
Published: 01 September 2021.
Edited by:
Ingo Dreyer, University of Talca, ChileReviewed by:
John Albert Raven, University of Dundee, United KingdomCopyright © 2021 Cummins. This is an open-access article distributed under the terms of the Creative Commons Attribution License (CC BY). The use, distribution or reproduction in other forums is permitted, provided the original author(s) and the copyright owner(s) are credited and that the original publication in this journal is cited, in accordance with accepted academic practice. No use, distribution or reproduction is permitted which does not comply with these terms.
*Correspondence: Peter L. Cummins, cGV0ZXIuY3VtbWluc0BhbnUuZWR1LmF1
Disclaimer: All claims expressed in this article are solely those of the authors and do not necessarily represent those of their affiliated organizations, or those of the publisher, the editors and the reviewers. Any product that may be evaluated in this article or claim that may be made by its manufacturer is not guaranteed or endorsed by the publisher.
Research integrity at Frontiers
Learn more about the work of our research integrity team to safeguard the quality of each article we publish.