- 1Department of Plant Biology and Biotechnology, Faculty of Biotechnology and Horticulture, University of Agriculture in Krakow, Kraków, Poland
- 2Department of Plant Product Technology and Nutrition Hygiene, Faculty of Food Technology, University of Agriculture in Krakow, Kraków, Poland
- 3Laboratory of Mass Spectrometry, Faculty of Biotechnology and Horticulture, University of Agriculture in Krakow, Kraków, Poland
- 4Department of Human Nutrition and Dietetics, Faculty of Food Technology, University of Agriculture in Krakow, Kraków, Poland
- 5Department of Agrochemistry and Plant Nutrition, Slovak University of Agriculture in Nitra, Nitra, Slovakia
The process of uptake and translocation of non-organic iodine (I) ions, I– and IO3–, has been relatively well-described in literature. The situation is different for low-molecular-weight organic aromatic I compounds, as data on their uptake or metabolic pathway is only fragmentary. The aim of this study was to determine the process of uptake, transport, and metabolism of I applied to lettuce plants by fertigation as KIO3, KIO3 + salicylic acid (KIO3+SA), and iodosalicylates, 5-iodosalicylic acid (5-ISA) and 3,5-diiodosalicylic acid (3,5-diISA), depending on whether additional fertilization with vanadium (V) was used. Each I compound was applied at a dose of 10 μM, SA at a dose of 10 μM, and V at a dose of 0.1 μM. Three independent 2-year-long experiments were carried out with lettuce; two with pot systems using a peat substrate and mineral soil and one with hydroponic lettuce. The effectiveness of I uptake and translocation from the roots to leaves was as follows: 5-ISA > 3,5-diISA > KIO3. Iodosalicylates, 5-ISA and 3,5-diISA, were naturally synthesized in plants, similarly to other organic iodine metabolites, i.e., iodotyrosine, as well as plant-derived thyroid hormone analogs (PDTHA), triiodothyronine (T3) and thyroxine (T4). T3 and T4 were synthesized in roots with the participation of endogenous and exogenous 5-ISA and 3,5-diISA and then transported to leaves. The level of plant enrichment in I was safe for consumers. Several genes were shown to perform physiological functions, i.e., per64-like, samdmt, msams5, and cipk6.
Introduction
Iodine in Plants
Iodine (I) is a beneficial element for plants and studies have determine the effectiveness of iodide (I–) or iodate (IO3–) uptake by plants through roots or leaves (upon foliar application) and their potential translocation (Medrano-Macías et al., 2016; Antonyak et al., 2018). The effectiveness of I ion accumulation has been determined for a number of species of plants. The majority of the studies have been performed in the context of I biofortification of plants. They were conducted with the aim to prepare an I deficiency prevention program, other than through the consumption of kitchen salt based on plant enrichment with I (White and Broadley, 2009). Among the papers published within the last 15 years, there has been research on species such as kohlrabi (Golob et al., 2020), strawberry (Budke et al., 2020), lettuce (Blasco et al., 2008; Dávila-Rangel et al., 2020), basil (Incrocci et al., 2019; Kiferle et al., 2019), green bean, lettuce (Dobosy et al., 2020), cabbage, cowpea (Ojok et al., 2019), broccoli raab, curly kale, mizuna, and red mustard (Gonnella et al., 2019). There was also research devoted to the I uptake mechanism (Kato et al., 2013; Humphrey et al., 2019).
Another subject of research was the impact of I on oxyreduction, e.g., in lettuce (Blasco et al., 2008; Dávila-Rangel et al., 2020), the process of photosynthesis in kohlrabi (Golob et al., 2020) and basil (Kiferle et al., 2019), nitrate (V) content in four Brassica genotypes (Gonnella et al., 2019), and changes in the content of macro- and microelements in green bean and lettuce (Dobosy et al., 2020). Furthermore, there was also research devoted to the efficacy of I– and/or IO3– uptake by plants, depending on the additional application of other elements, such as selenium in kohlrabi (Golob et al., 2020), zinc, selenium, and iron in wheat (Zou et al., 2019), and zinc and selenium in wheat and rice (Cakmak et al., 2020). The impact of I on the induction of plant resistance to diseases was also analyzed (Ajiwe et al., 2019). There are also works focused on the process of methylation, i.e., volatilization to the atmosphere, of volatile I forms (Leblanc et al., 2006; Itoh et al., 2009).
There were also studies that tackled a unique subject of the plants’ ability to take up I applied in the form of organic compounds, e.g., iodoacetate anion (Weng et al., 2008) or organic I complexes. For instance, Dávila-Rangel et al. (2020) showed that the application of the chitosan-I complex enhanced I uptake by lettuce. The ability of tomato plants to take up organic I compounds was also determined, in which I was bound with the aromatic ring (Halka et al., 2019). The conclusion for lettuce was that the effectiveness of I biofortification with 5-ISA was higher than upon application with KIO3 (Smoleń et al., 2017). Smoleń et al. (2020) showed the enrichment of lettuce with I using KIO3, 5-ISA, and 3,5-diISA; however, they did not study the molecular mechanisms associated with the process of uptake and metabolism of these three I compounds.
Previous literature on physiology and/or biochemistry of plants has not considered the issue of activity and function of plant-derived thyroid hormone analogs (PDTHA), which are compounds that contain I; hence, the scarcity of scientific data on the subject. Fowden (1959) demonstrated that radioactive I binds to I organic compounds, e.g., T3 in bean, barley, aster, and Salicornia plants. Lima et al. (2012) presented general information on the potential presence of PDTHA compounds in plants. Based on their model research, Pessoa et al. (2010) concluded that in Arabidopsis thaliana exogenous T4 may be bound by the transthyretin-like protein. Plants were observed to produce transthyretins, i.e., proteins that may potentially act as T3 and/or T4 transporters (Eneqvist et al., 2003). However, in the available literature, it is unclear whether T3/T4 receptors might be present in plants, and descriptions of the potential genes that might encode such proteins is lacking. Obtaining information on the issue seems crucial for the determination of functionality and mechanism of PDTHA activity in plants.
Vanadium vs. Iodine
Vanadium is classified as a beneficial element for plants (Welch and Huffman, 1973; Mengel and Kirkby, 1996) and is also beneficial for animals and humans (Anke et al., 2002). In the human body, V regulates the activity of a number of enzymes (WHO, 2001; Anke et al., 2002; Gruzewska et al., 2014). It also improves thyroid function (Afkhami et al., 2009). There is no recommended dietary allowance (RDA) established for humans (Trumbo et al., 2001). The official information on the effect of V on humans was issued by WHO (World Health Organization) several decades ago and only contains rough suggestions concerning V doses for humans (10 μg V⋅24 h–1).
A positive impact of V on the growth and development of plants was observed at low doses < 0.04 mg V⋅dm–3 in the nutrient solution (Kaplan et al., 1990; Pilbeam and Drihem, 2007). Such doses have been observed to have a synergistic effect on the uptake of selected macroelements by plants or to increase the foliar content of photosynthetically active pigments (Kaplan et al., 1990; Pilbeam and Drihem, 2007). Higher photosynthetic activity following V application results in the accumulation of sugars in sweetcorn (Sentíes-Herrera et al., 2018), leading to larger biomass growth in the aboveground parts of the plants (Basiouny, 1984).
In hydroponic systems, the availability of V for roots is higher than that in the soil. Therefore, the V tolerance/harmfulness for plants (Gil et al., 1995; Chongkid et al., 2007; Vachirapatama et al., 2011) is much lower than that in the soil (Zhang et al., 2012; Akoumianaki-Ioannidou et al., 2016; Imtiaz et al., 2018). Next to the dose, the plants’ response to V also depends on I oxidation and is a generic property of plants (Kaplan et al., 1990; Gil et al., 1995; Vachirapatama et al., 2011). The low root absorbability of V is due to its poor mobility in the soil (Cappuyns and Swennen, 2014). The process of V sorption is related to the fact that VO2+ very easily reacts with humic acids in soil organic matter (SOM), making V not easily available to plants (Pilbeam and Drihem, 2007). Vanadium has not been commonly included in the process of preparing nutrient solutions for hydroponic systems (Jones, 2016). The conducted research made it possible to determine the plant’s response to the interaction between the simultaneous fertilization of plants with I and V.
In marine algae, V functions in I uptake into cells. This functionality of V is attributed to its presence at the active site of iodoperoxidase and other haloperoxidases (vHPO), i.e., V-dependent bromoperoxidase or chloroperoxidase (Leblanc et al., 2006). The structure, regulatory activity, and functionality of vHPO was described, owing to research on a number of marine alga species (Almeida et al., 2000; Colin et al., 2003; Kongkiattikajorn and Pongdam, 2006; Leblanc et al., 2006). Haloperoxidases (HPOs) are responsible for the oxidation of halogens that was conducted in the presence of H2O2 (Colin et al., 2005).
The process of cell I uptake by marine algae, catalyzed by vHPO, consisted of the oxidation of I– to HIO, which was further transformed to molecular I2. HIO and I2 are produced within the cell wall. Being more lipophilic than I–, they easily penetrate through the wall to the cytosol (Leblanc et al., 2006). Medrano-Macías et al. (2016) reported that there is likely a relationship between I and V in terrestrial plants. However, the function of vHPO in domesticated plants is not known. Smoleń et al. (2020) identified vHPO-like enzyme activity in lettuce and a relationship between its activity and I root uptake in the setting of trace I content in the rhizosphere. Thus far, no gene in the genome of lettuce has been assigned the function of vHPO.
The response of plants to I and V application on physiological and biochemical properties has not been diagnosed. The issue has been, to a limited extent, described in preliminary research by Smoleń et al. (2020). This situation is different for marine algae, as these plants actively take up I, accumulate it in their tissues, and carry out methylation (Leblanc et al., 2006; Keng et al., 2020). The methylation process (I volatilization) has also been described for selected terrestrial plant species (Attieh et al., 2000; Nagatoshi and Nakamura, 2007; Itoh et al., 2009). Among the studies conducted were biotechnological studies on deactivation of the process in A. thaliana (Landini et al., 2012). Iodovolatilization is carried out with the participation of vHPO. In contrast, the methylation of iodic hydrocarbons (CHxIx) is carried out with the participation of S-adenosyl-l-methionine (SAM)-dependent halide methyltransferase (HMT) or SAM-dependent halide/thiol methyltransferase (HTMT). These enzymes use iodide as a substrate (Medrano-Macías et al., 2016; Gonzali et al., 2017). No gene encoding HMT or HTMT has been identified in the genome of lettuce.
Salicylic Acid (SA) and SA-Derivatives vs. Iodine
A volatile ester of methyl salicylic acid (MeSA) can be volatilized in roots and leaves. MeSA is produced in the process of esterification of salicylic acid, during which CH3 is joined to the SA carboxylic group (Taiz and Zeiger, 2010; Zhang et al., 2013). In tomatoes, MeSA is synthesized by an enzyme named salicylic acid carboxyl methyltransferase (SAMT) (Tieman et al., 2010). This volatile ester participates in SAR (systemic acquired resistance) in plants (Gao et al., 2014). SA is classified as a plant phytohormone (Gust and Nürnberger, 2012) or as a phytohormone-like compound (Hayat et al., 2010). Literature lacks information on whether endogenous and exogenous iodosalicylates, such as 5-ISA and 3,5-diISA, in plants may undergo further catabolic reactions. No enzymes related to the catabolism of 5-ISA and 3,5-diISA have been identified to date in plants. Additionally, there is no data indicating whether SAMT can participate in the methylation of 5-ISA, 3,5-diISA, or the SA produced as a result of the potential degradation of these iodosalicylates. Moreover, genes encoding proteins with a SAMT-like function in lettuce have not been found.
The aim of this study was to determine the process of uptake and metabolism of I applied to plants as KIO3 and iodosalicylates. Additionally, the study aimed to determine the effect of V on these processes. Another objective was to document the selected molecular processes in the metabolism of non-organic and organic I compounds in roots and leaves of lettuce, considering aspects related to the synthesis of PDTHA.
A novelty in this study, when compared with previously published ones, was research on the plants’ ability to take up non-organic and organic I compounds (iodosalicylates) through the roots, as well as whether and to what extent these compounds can be metabolized and transported within the plants’ roots-leaves system. Additionally, selected genes were examined and assigned a potential putative role for encoding enzyme proteins demonstrating functions typical of vHPO, SAMT, and HMT/HTMT.
Materials and Methods
Plant Material and Treatments
Lactuca sativa L. var. capitata cv. “Melodion” was planted in two pot studies and one hydroponic study. This research was performed within the camp of the University of Agriculture in Kraków (50°05′04.1″N 19°57′02.1″E).
A nutrient film technique (NFT) was used for the hydroponic system in a greenhouse setting. The hydroponic experiment was named Experiment 1 (Table 1). The pot studies were in turn conducted in a foil tunnel with the plants being farmed in 2 types of substrate, a peat substrate as organic soil (Experiment 2) and loam soil as an example of a heavy mineral soil (Experiment 3). Each of the 3 experiments was repeated twice in the spring season during 2 consecutive years of the study in 2018 and 2019.
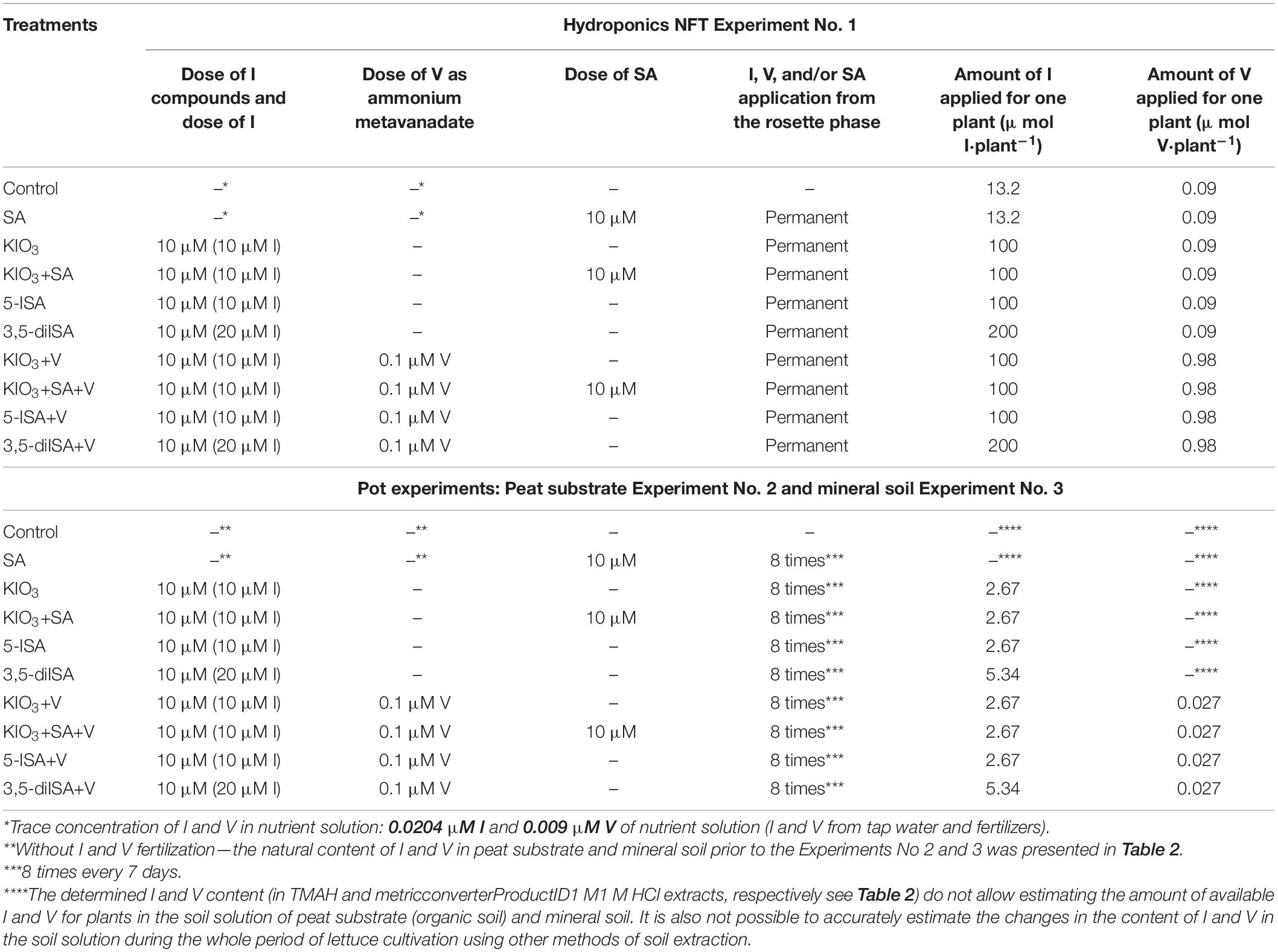
Table 1. Design and method of conducting experiments with lettuce cultivation in the hydroponics NFT Experiment No. 1 as well as in pot experiments: Experiment Nos. 2 and 3.
The subject of this study was plant fertilization with I (Table 1), that is, with potassium iodate (KIO3), 5-iodosalicylic acid (5-ISA), and 3,5-diiodosalicylic acid (3,5-diISA), as well as with ammonium metavanadate (V). An additional application of salicylic acid (SA) was also used to compare the effects of both iodosalicylic acids. The same configuration of the combinations tested was used in all 3 experiments: (1) Control; (2) SA; (3) KIO3; (4) KIO3+SA; (5) 5-ISA; (6) 3,5-diISA; (7) KIO3 + V; (8) KIO3 + SA + V; (9) 5-ISA + V; and (10) 3,5-diISA + V. In each experiment, application started at the rosette stage (5–6 true leaves). The following concentrations were used: 10 μM for all I compounds (molar mass equivalents), 10 μM for SA, and 0.1 μM for V. The experiments differed in the frequency of application. In hydroponic systems, the nutrient solution (containing the compounds tested) was applied continually (fixed concentration). A different application strategy was used in the pot systems. The I and V compounds and SA were applied to the soil once a week through manual fertigation (manual watering with solutions of the compounds studied, at a dose of 100 mL⋅pot–1 (one plant–1). In total, in Experiments 2 and 3, plants were fertilized with I, V, and SA 8 times. This research strategy was planned purposefully. Our aim was to avoid the risk of accumulation of I concentrations that would be toxic to plants in either the peat substrate or mineral soil. When designing the study, we had no information on whether or to what extent iodosalicylates applied to the soil would be taken up by the plants. Additionally, the aim was to measure the effectiveness of biofortification of lettuce using different I compounds with or without V, depending on the method of cultivation and substrate.
In a hydroponic system, it was possible to obtain the entire root system for chemical analyses without damaging it, among other things. This allowed model research on the uptake and transport of different forms of I in the roots-leaves system. In the pot system, it was impossible to isolate roots from the soil because the root system outgrew the volume of soil in the pots. Therefore, in Experiments 2 and 3, roots were not subjected to chemical analyses.
In each year of the study, seeds were sown in early March (13 March 2018 and 4 March 2019). They were sown in multi-pallets filled with substrate, that is, with peat substrate and sand 1:1 (V/V). The multi-pallets had 112 cells (14 rows × 8 cells), each sized 3.2 × 3.2 × 4 cm. Young plants in the phase of 4–5 true leaves were replanted to the NFT hydroponic system (in Experiment 1) or to pots (in Experiments 2 and 3; 10 April 2018 and 2 April 2019). The plants were potted together with the entire root ball in either peat substrate or heavy mineral soil. The volume of substrate in pots was 1.5 dm3. The chemical properties of the peat substrate and heavy mineral soil before cultivation are presented in Table 2; a detailed description of methods used for chemical analysis of soil is reported in the Supplementary Material. No fertilization was performed before or during lettuce cultivation in either of the two pot experiments. This was because the nutrient content, pH, and EC (electrical conductivity) were optimal for growing this species in the peat substrate or heavy mineral soil I (Sady, 2000; Table 2).
In the NFT hydroponic system, target replanting was preceded by thorough rinsing of the substrate between the seedlings’ roots with tap water. Seedlings were placed in the openings (spaced 25 cm apart) in Styrofoam slabs filling the NFT beds. No substrate was used in slabs. Once planted, seedlings in the NFT system were watered during the day. Each experiment consisted of four replicates in a randomized block design. In hydroponic Experiment 1, there were 15 plants per replicate and 60 plants per combination (600 plants per experiment). In pot Experiments 2 and 3, there were 7 plants per replicate and 28 plants per combination (a total of 560 plants in both pot experiments).
The type of nutrient solution, its preparation (content of macro- and microelements), regulation of pH and EC, and type of fertilizers in the NFT were the same as in our previous studies with lettuce in the same hydroponic system (Smoleń et al., 2019b). The I used in the base nutrient solutions (control) was iodide I– (25.52 μg I⋅dm–3) and iodate IO3– (0.29 μg I⋅dm–3). The content of I was natural (from water and dissolved fertilizers).
Once planted in spacers in the NFT systems, the plants were watered during the day between 5 am and 7 pm and at night between 1 and 2 am, for 1 min at 5-min intervals. In pot experiments, the plants were watered with tap water using drip irrigation. A single dose of water was about 100 mL⋅plant–1 (pot–1). The frequency of watering was adjusted to weather conditions and sizes of the plants; factors that determined the rate of substrate drying involved controlling watering by the irrigation computer with the option to sum the amount of solar radiation to start irrigation. The adopted watering strategy made it possible to eliminate water leaching from pots. On the days when the compound solutions were applied to the substrate in pot Experiments 2 and 3, the plants were not watered through the drip system.
Plants were harvested at the phase of head development, that is on 15 May 2018 and 7 May 2019 in hydroponic Experiment 1, and on 9 May 2018 and 16 May 2019 in pot Experiments 2 and 3. Then, the heads (lettuce leaves) were weighed. Hydroponic Experiment 1 was the only experiment where lettuce leaf harvesting was immediately followed by pipette collection of a secretion produced as a result of root pressure [white secretion (RootSec) on the surface of the root neck, visible after cutting the heads at collar level (lettuce leaves), as shown in Supplementary Figure 1]. The secretion was collected for the determination of the chemical forms of I, transported from roots to the aboveground parts of plants. A total of two samples were collected for each combination, each containing 5 mL of root secretion. Immediately after collection, the secretion was 1:1 mixed with buffer (20 mM Tris HCl buffer, pH 8.5). Then, the samples were frozen at −20°C and stored until analyzed using two mass spectrometry techniques. Iodides (I–) and iodates (IO3–) were analyzed using high-performance liquid chromatography with inductively coupled plasma mass spectrometry (HPLC-ICP-MS)/MS, while organic I compounds were analyzed using liquid chromatography-mass spectrometry (LC-MS)/MS. Methodologic details are presented in the following subsections.
In Experiment 1, the collection of root secretions was followed by the measurement of lettuce root biomass, while in pot Experiments 2 and 3 these measurements were abandoned, as the root biomass could not be separated from the soil without damaging the roots. Chemical analyses were performed on roots of all plants from the hydroponic system, and on four randomly selected heads from each biological replicate in all three experiments.
Activity of Vanadium-Dependent Haloperoxidases
Fresh leaf samples collected in all three experiments and root samples from Experiment 1 were used to measure the total activity of vHPO enzymes. The analysis was performed with a method adapted for lettuce by Smoleń et al. (2020) based on that for marine algae. The activity of vHPO was calculated based on the increase in absorbance within 20 min (wavelength: 620 nm) and converted into U⋅mg–1⋅min–1 protein. Protein content in enzyme extracts was measured using the Lowry method (Waterborg, 2002). Bovine serum albumin was used as a standard.
Freeze-Drying of Samples
Fresh root and leaf samples (lettuce) were washed in tap and distilled water. Samples of lettuce heads were vacuum-dried. Each head was halved (leaves were peeled in each growth phase) and mixed thoroughly as part of each replicate. Root and leaf samples were frozen at −20°C. The freeze-drying of frozen samples was performed with the Christ Alpha 1–4 unit (Martin Christ Gefriertrocknungsanlagen GmbH, Germany). Vacuum-dried samples of roots and leaves were ground in a lab mill (FRITSCH Pulverisette 14; FRITSCH GmbH, Weimar, Germany) and stored in sealed polyethylene bags until further chemical analyses (described in the next three sections).
Analysis of Total Iodine and Vanadium in Dry Samples of Roots and Leaves
The analysis of I content in samples of lettuce leaves and roots was performed by inductively coupled plasma mass spectrometry (ICP-MS/MS) with a triple quadruple spectrometer (iCAP TQ ICP-MS Thermo Fisher Scientific, Bremen, Germany), preceded by alkaline extraction of 0.2 g samples by tetramethylammonium hydroxide (TMAH; Smoleń et al., 2019a, c; based on Pn-En 15111, 2008).
Vanadium content in leaf and root samples was measured using inductively coupled plasma optical emission spectrometry (ICP-OES) (Prodigy Spectrometer, Leeman Labs, New Hampshire, MA, United States). The mineralization and measurement procedures were consistent with the method described by Smoleń et al. (2020).
The results of I and V content in the plant samples and biomass measurements were used to calculate I uptake (I-uptake) and V uptake (V-uptake) by plants.
Analysis of Iodides (I–) and Iodates (IO3–) in Roots and Leaves by HPLC-ICP-MS/MS
The content of iodides (I–) and iodates (IO3–) was only determined in root and leaf samples of hydroponic lettuce (Experiment 1); in pot experiments, there was no possibility to collect root samples for analysis. The content of these I ions was measured using a modified extraction procedure described by Smoleń et al. (2016). Briefly, a 0.05 g analytic portion of air-dried, ground plant samples was extracted (in 7 mL polypropylene tubes) using a solution containing 4 mL 25% TMAH (Sigma-Aldrich, St. Louis, MO, United States) and 10 mL 0.1 M NaOH (Chempur, Piekary Śla̧skie, Poland) dissolved to a final volume of 1 L with demineralized water. Once mixed, the samples were incubated for 1 h at 50°C in an ultrasonic bath, then cooled to approximately 20°C, mixed thoroughly, and centrifuged for 15 min at 4,500 rpm. The supernatants were filtered through a 0.22 μm syringe filter. The content of I ions in filtered samples was analyzed using HPLC-ICP-MS/MS. For I– and IO3– speciation forms, HPLC (Thermo Scientific Ultimate 3000; Thermo Fisher Scientific, Bremen, Germany) was coupled to ICP-MS/MS (iCAP TQ). This method employed a strong anion exchange column [Thermo Fisher Scientific; Dionex IonPac AS11 (4 × 250 mm)] and a precolumn [Thermo Fisher Scientific; Dionex IonPac AG11 (4 × 50 mm)]. The column temperature was set to 30°C. Demineralized water, 50 mM NaOH, and 0.5% TMAH were used as eluents. To separate both I ions, a mobile phase, containing 2.5 mM NaOH and 0.125% TMAH at an isocratic flow, was used. The flow-rate was 1.5 mL/min, with an injection volume of 10 μL and total analysis time of 7 min (Supplementary Figures 2, 3). The HPLC-column effluent was introduced directly into ICP-MS/MS (iCAP TQ ICP-MS). Iodine was determined at 127I.16O isotope, using S-TQ-O2 mode. Standards were prepared through dissolution of KI and KIO3 (Sigma-Aldrich, St. Louis, MO, United States) in demineralized water.
To ensure correct iodide and I measurements, a “standard addition method” was used; it was applied independently for each leaf and root sample from each of the 10 combinations (Supplementary Figures 4–6). The standard addition method has been applied because that the alternative and easier “standard series method,” which is described in numerous publications, would not provide correct analytical results. The difficulty to obtain reliable assaying results with the standard series method was due to the different matrix effects of root and leaf extracts obtained from each of the 10 combinations tested in Experiment 1. The effect could be eliminated through the use of the standard addition method, which allowed us to obtain correct analytic results.
Determination of Salicylic Acid, Benzoic Acid, Iodosalicylates, Iodobenzoates, and Plant-Derived Thyroid Hormone Analogs
Roots of plants from the hydroponic system (Experiment 1) and lettuce leaves in all three experiments were tested using LC-MS/MS to measure the content of SA, BeA (benzoic acid), 5-ISA, 3,5-diISA, 2-iodobenzoic acid (2-IBeA), 4-iodobenzoic acid (4-IBeA), 2,3,5-triiodobenzoic acid (2,3,5-triIBeA), iodotyrosine (I-Tyr), sodium salt triiodothyronine (T3-Na), triiodothyronine (T3), and thyroxine (T4) (Supplementary Figure 7). The root and leaf content of these compounds was analyzed in extracts prepared with 75% ethanol containing 50 ng⋅mL–1 of deuterated salicylic acid (SA-d4, Sigma-Aldrich). Sample extraction and filtration procedures were the same as described in our previous research (Smoleń et al., 2020).
The compounds were also measured in RootSec after lettuce harvesting. The RootSec were mixed with 20 mM Tris HCl buffer (pH 8.5). The collection and storage of RootSec is described in section “Plant Material and Treatments.”
Root secretions stored in the TRIS-HCl buffer were mixed in vortex prior to analysis and centrifuged at 5°C for 15 min at 4,500 rpm. Then, the supernatant was filtered with a 0.22 μm nylon syringe filters (FilterBio NY Syringe Filter, Phenomenex, Torrance, CA, United States) and analyzed using LC-MS/MS according to Smoleń et al. (2020).
Biofortification Target and the Safety of Iodine-Enriched Lettuce Consumers
The results of I measurements in lettuce leaves were used in each of the experiments to calculate the following coefficients: (1) recommended daily allowance of iodine (% RDA-I) and (2) hazard quotient for iodine (HQ-iodine). They were calculated for 100 g of fresh lettuce leaves, considering the daily I requirement of adults of 150 μg. The % RDA-I and HQ-iodine coefficients were calculated using mathematical formulas described in detail by Smoleń et al. (2019a).
Gene Expression Analysis
Plants cultivated in the hydroponic NFT system (Experiment 1) were used as material for gene expression analysis. Leaves and roots for RNA extraction were collected directly before harvest. The leaf and root samples were collected from 8 plants (2 plants from each of the 4 replications), separately for each of the 10 treatments. The third youngest leaf and root samples (portions of 5–10 cm, with tips) were collected for each plant. The samples were immediately frozen in liquid nitrogen and stored at −80°C until isolation of RNA. Total RNA extraction was carried out with a Direct-zolTM RNA MiniPrep Plus RNA isolation kit (Zymo Research, Irvine, CA, United States), according to the manufacturer’s instructions. RNA samples were treated with 1 U μl–1 RNase-free Dnase I (Ambion Inc., Austin, TX, United States) and 40 U μl–1 RiboLock RNase Inhibitor (Thermo Fisher Scientific, Wilmington, DE, United States) to avoid contamination by DNA and RNA degradation. The quality and integrity of RNA samples were verified by electrophoresis in 1% agarose gel in denaturing conditions. The concentration and quality of RNA were evaluated spectrophotometrically using NanoDrop 2000c (Thermo Fisher Scientific, Wilmington, DE, United States) at 230, 260, and 280 nm. cDNA synthesis was conducted in four biological replicates, each comprising two plants. One microgram of RNA from each sample was transcribed into cDNA using the iScript cDNA synthesis kit (BioRad laboratories, Hemel Hempstead, United Kingdom), according to the manufacturer’s instructions. The cDNA was frozen at −20°C until it was used as a template in real-time qPCR using the StepOnePlusTM Real-Time PCR System (Applied Biosystems, Foster City, CA, United States), according to the following steps: denaturation at 95°C for 10 min; 40 cycles at 95°C for 15 s, 60°C for 30 s, and 72°C for 30 s. The melting curves were obtained by melting the amplicons from 60 to 95°C for 15 s; the temperature was increased by 0.3°C per cycle.
For expression analysis, five genes possibly related to selected metabolic pathways for I and/or iodosalicylates in lettuce, i.e., per12-like, per-64-like, samdmt, cipk6, and msams5, were chosen (Supplementary Table 1). Moreover, differential expression patterns of those genes were shown by RNA-seq in leaves and roots of L. sativa ‘‘Melodion’’ of control plants and supplemented with SA, KIO3 and KIO3+V (data unpublished). Gene-specific primers for real-time qPCR were designed using Primer3Plus1 based on L. sativa var. capitata ‘‘Melodion’’ transcript sequences de novo assembled from RNAseq, deposited in the NCBI GeneBank (Acc. No MT649253, MT649254, MT663549-MT663551) and Lettuce Genome Resource2 (Supplementary Table 1).
The absence of primer-dimer and hairpin structures was determined using IDT-OligoAnalyzer 3.13. The utility of the designed primers was validated in a reverse-transcriptase polymerase chain reaction (RT-PCR) and confirmed by electrophoresis in 1% agarose gel (Supplementary Figure 8). Primer specificity was verified by observing single peaks in all melting curves. The total volume of the reaction mixture was 25 μL. The mixture included 12.5 μL Maxima SYBR Green/ROX qPCR Master Mix (2X) (Thermo Fisher Scientific), 0.7 μM of (5 μM) each primer (forward and reverse), 2 μL of a 5-fold diluted template cDNA, and a total volume of 25 μL made up with nuclease-free DEPC-treated water (diethylpyrocarbonate; Thermo Fisher Scientific, Wilmington, DE, United States). qPCR reactions were conducted in four biological and three technical replicates. No template controls were included. Amplification efficiencies for all primer pairs were evaluated using serial 10-fold dilutions of pooled cDNA. The efficiency of each primer pair was calculated from the slope of the standard curve using the formula E = 10– 1/slope and converted into percentage values according to the following formula: %E = (E − 1) × 100%.
Actin (act) (Smoleń et al., 2016) and protein phosphatase 2A regulatory subunit A3 (pp2aa3) (Sgamma et al., 2016) were used as endogenous reference genes. As Pp2aa3 expression was more stable than that of act (Supplementary Figure 9), relative quantification of gene expression was calculated using the 2–Δ Δ C(T) method (Livak and Schmittgen, 2001) with Ct value normalization to pp2aa3. In the case of per64-like, per12-like, cipk6, and msams5, the relative gene expression was compared with control samples from roots, whereas in samdmt, it was compared to control samples from leaves, since expression of samdmt in root control samples was not detectable (Supplementary Figure 8).
Statistical Analyses
All data were statistically verified using one-way analysis of variance (ANOVA) in the Statistica 12.0 PL (StatSoft Inc., Tulsa, OK 74104, United States)4 program at a significance level of p < 0.05. In the case of significant effects, homogenous groups were distinguished on the basis of a post-hoc Tukey HSD test. The results obtained were verified statistically by one-way ANOVA and post-hoc Tukey HSD test, separately for each of the three experiments and separately for leaves and roots of lettuce in Experiment 1.
Results
Plant Biomass
Hydroponic Experiment 1 was the only experiment where the application of 5-ISA and 3,5-diISA (without or with V) caused a reduction in the weight of leaves and whole plants (roots + leaves) compared with the control (Table 3). No negative impact of 3,5-diISA and 3,5-diISA+V on the weight of roots was observed. A comparable increase in root weight was observed for the application of 5-ISA and 5-ISA+V, compared with the control. In this experiment, combined fertilization with V and KIO3, KIO3+SA, 5-ISA, and 3,5-diISA had no effect on the weight of roots and heads (leaves) of lettuce, compared with the application of these compounds without V.
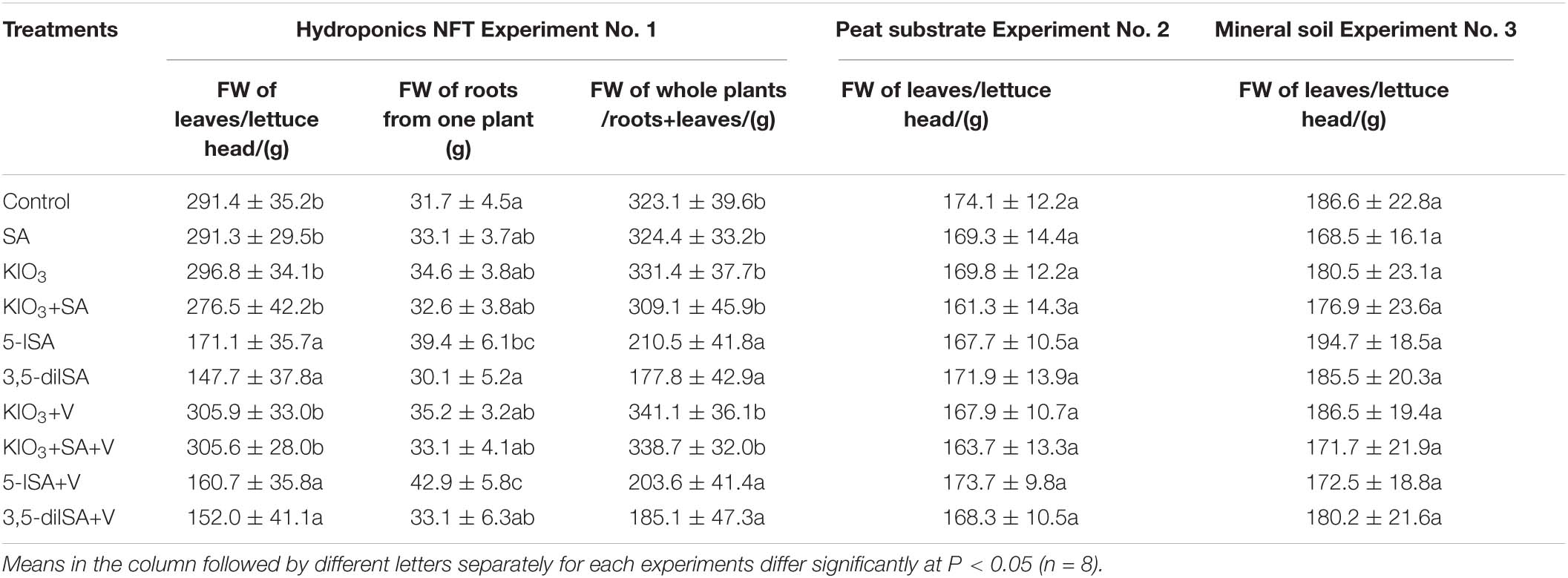
Table 3. Fresh weight of roots, leaves /lettuce head/ and whole plants /roots+leaves/ in hydroponic NFT Experiment No. 1 as well as in lettuce leaves /head/ in pot Experiment Nos. 2 and 3.
None of the combinations of SA, V, and I compounds had a significant impact on the weight of lettuce heads in either of the two pot experiments (Experiments 2 and 3).
Gene Expression in Roots and Leaves of Plants Cultivated in a Hydroponic System (Experiment 1)
I compounds, V, and SA applied to the nutrient solution had a statistically significant impact on the expression of the following genes in lettuce roots and leaves: per64-like, per12-like, samdmt, cipk6, and msams5 (Figures 1A–E). Basically, the expression of per64-like in leaves and samdmt in roots was relatively very low compared with roots and leaves, respectively. As for the remaining three genes (per12-like, cipk6, and msams5), their expression in roots was higher than in leaves.
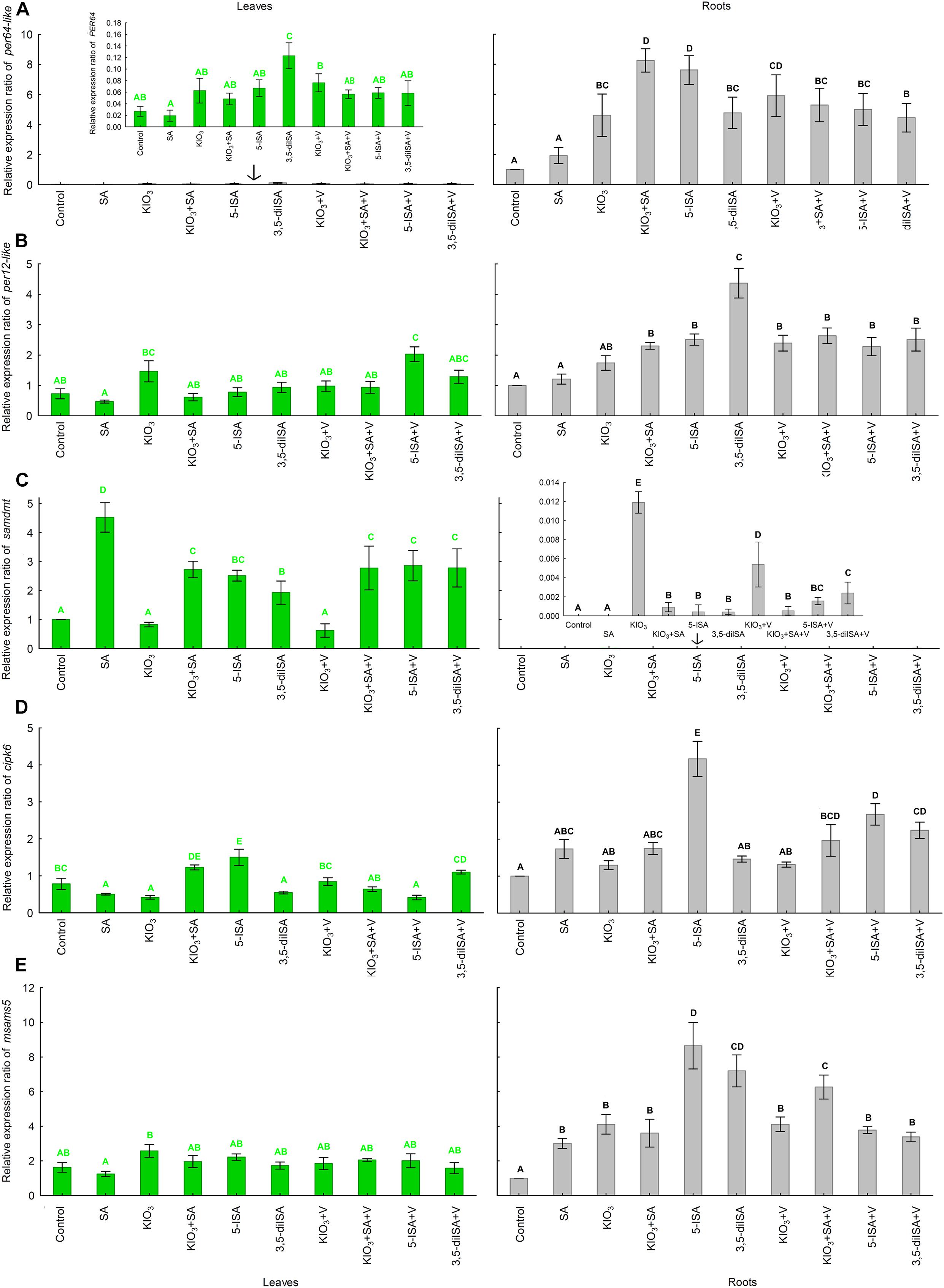
Figure 1. Relative expression of per64-like (A), per12-like (B), samdmt (C), cipk6 (D), and msams5 (E) genes in leaves and roots of lettuce plants cultivated in hydroponic NFT Experiment No. 1. Means followed by different letters for treatments differ significantly at P < 0.05. Bars indicate standard error (n = 4).
Compared with the control, all combinations with the application of I, I+SA, and V, caused increased expression of all five genes, i.e., per64-like, per12-like, samdmt, cipk6, and msams5, in roots (Figures 1A–E). The highest expression level of per64-like in roots was observed after application of KIO3+SA and 5-ISA. Additionally, exogenous 5-ISA in roots caused the highest expression of cipk6 and msams5; application of 3,5-diISA led to the most pronounced expression of per12-like, while plants fertilized with KIO3 had the highest expression of samdmt. Foliar activity of these five genes in lettuce was completely different that in roots compared with the control. The highest foliar expression of individual genes was as follows: per64-like following the application of exogenous 3,5-diISA, per12-like following the application of 5-ISA+V, samdmt following the application of SA, cipk6 following the application of exogenous 5-ISA, and msams5 following the application of KIO3.
Iodine Accumulation and Uptake by Lettuce
Inorganic (IO3–) and organic (5-ISA, 3,5-diISA) I accumulated in larger amounts in roots than in leaves (Figures 2A,B; hydroponic Experiment 1). Root accumulation of I upon the application of both iodosalicylates was higher than upon using KIO3 as a fertilizer. Vanadium added to the nutrient solution caused a significant reduction of I content in roots for the combination of KIO3+SA+V vs. KIO3+SA. Additionally, V caused a significant increase in I content in roots for the combination 5-ISA+V vs. 5-ISA, and for 3,5-diISA+V vs. 3,5-diISA (Figure 2B).
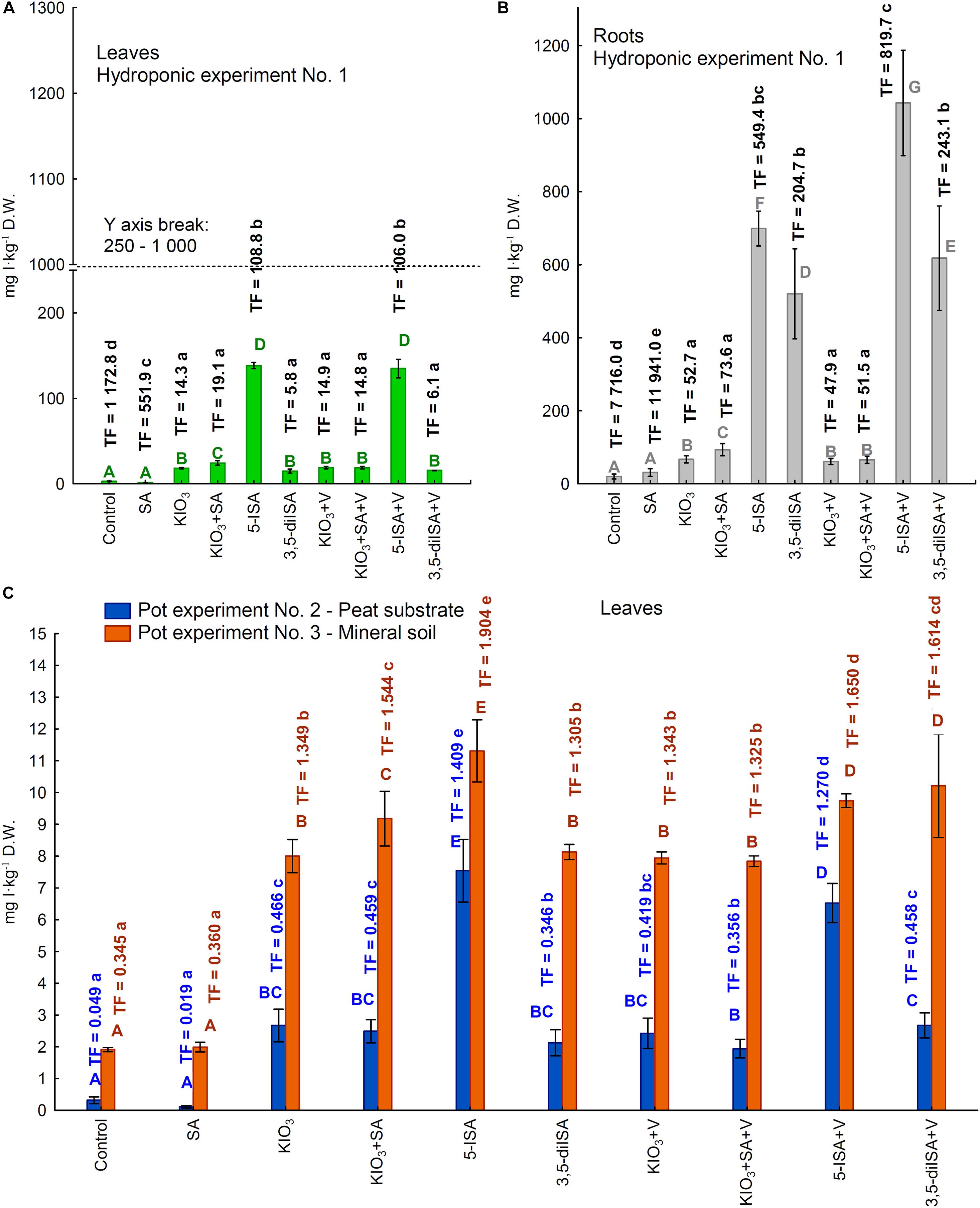
Figure 2. Concentrations of iodine in leaves /heads/ (A) and roots of lettuce (B) cultivated in hydroponic NFT Experiment No. 1 as well as in leaves of plants cultivated in pot Experiment No. 2 and 3 (C). Transfer factor /TF/ of iodine to roots and leaves /heads/ in the all experiments. Means followed by different letters for treatments, separately for each experiments differ significantly at P < 0.05. Color-coded capital letters for iodine content (green and gray for leaves and roots in Experiment 1 and blue and orange for leaves in Experiments 2 and 3); lowercase letters in the same colors for the TF factor in these experiments. Bars indicate standard error (n = 8).
Compared with the control and SA, application of each I compound (without V, with V, and with KIO3+SA) caused a significant increase in foliar I content (Figures 2A,C), RDA-I%, and HQ-iodine, as well as I uptake by a single lettuce head (leaves from one plant) in all three Experiments (Supplementary Table 2).
The highest foliar I content, RDA-I%, and HQ-iodine, as well as I uptake by a single lettuce head, was found in plants with applied 5-ISA in all three experiments (Figures 2A,C and Supplementary Table 2). Adding V to the compound (5-ISA+V vs. 5-ISA) reduced foliar I content in both pot experiments (Experiments 2 and 3) but not in the hydroponic system (Experiment 1). Consequently, RDA-I%, HQ-iodine, and I uptake by a single lettuce head was significantly lowered for 5-ISA+V vs. 5-ISA in both pot experiments (Supplementary Tables 2, 3).
Combined fertilization with KIO3+V had no impact on leaf I content in any of the three experiments, compared with KIO3 without V. As for the application of 3,5-diISA+V (compared with 3,5-diISA without V), only Experiment 3 with a peat substrate showed a significant increase in leaf I content, RDA-I%, HQ-iodine, and I uptake by a single lettuce head (Supplementary Tables 2, 3).
In all three experiments, the calculated TFs for I uptake were significantly modified by application of the I compounds tested, as well as SA and V (Figures 2A–C). The TF values reflected I uptake by plants (I content in roots or leaves) depending on its availability in the rhizosphere, i.e., in the nutrient solution in the hydroponic system (Experiment 1) or mineral soil or peat substrate (Experiments 2 and 3).
Vanadium Uptake and Accumulation by Lettuce
In hydroponic Experiment 1, V was accumulated in larger amounts in roots than in leaves (Figures 3A,B). Fertilization with V combined with KIO3, KIO3+SA, and 5-ISA caused an approximately 4-fold increase in V content in roots compared with the control (Figure 3B). Following fertilization with 3,5-diISA without applying ammonium metavanadate, the content of V in roots was lower than in the control (Figure 3B); V uptake by roots of a single plant and by whole plants (roots + head) was consequently lower (Supplementary Table 3). Application of 3,5-diISA+V resulted in a significant increase in V content in roots and V uptake by roots of a single plant and by whole plants (roots + head) compared with 3,5-diISA without V. However, the increase in root V content and in V uptake by roots and whole plants was less effective for fertilization with 3,5-diISA+V than for KIO3+V, KIO3+SA+V, and 5-ISA+V than for 3,5-diISA, KIO3, KIO3+SA, and 5-ISA, respectively. This was confirmed by TF values in roots for the respective combinations with and without V fertilization.
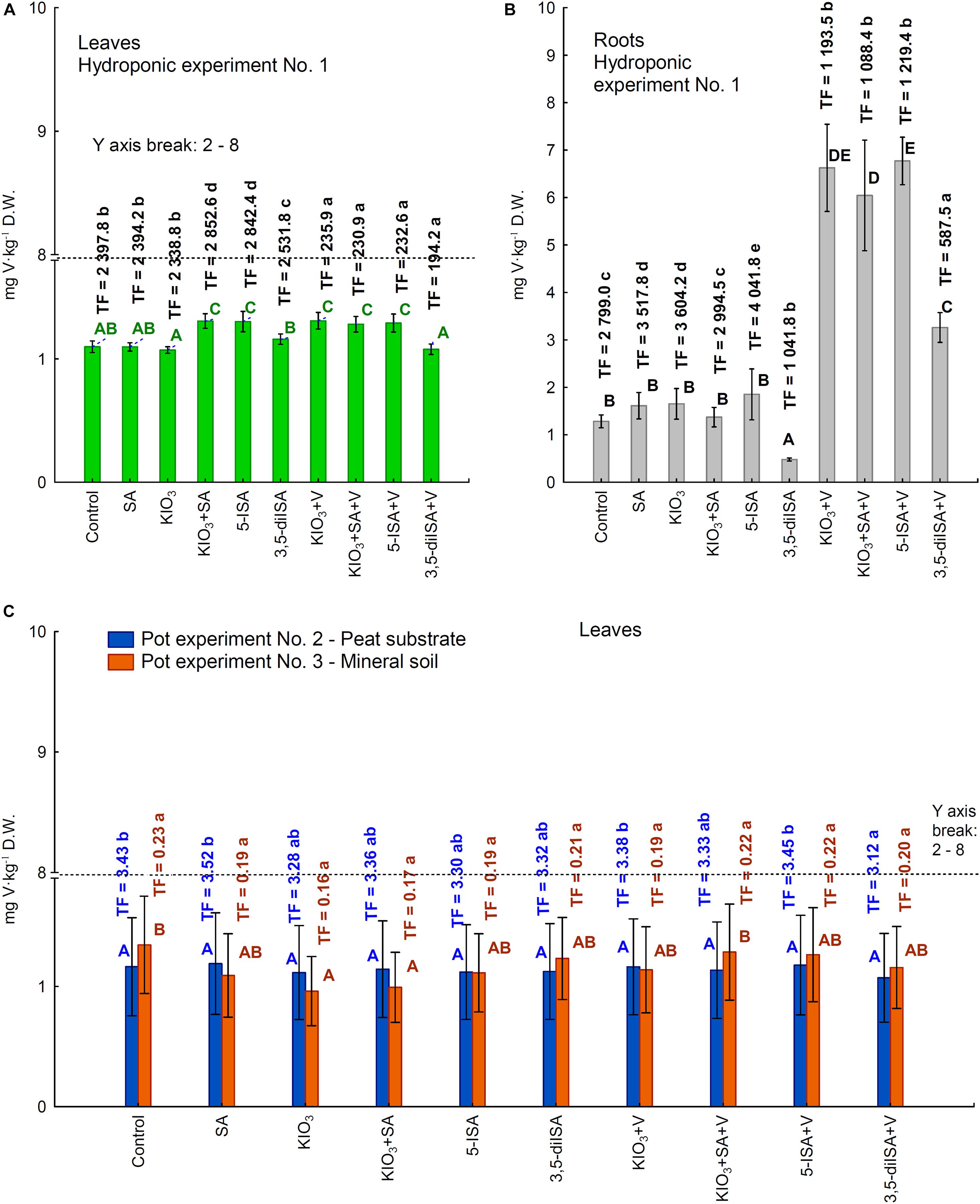
Figure 3. Concentrations of vanadium in leaves /heads/(A) and roots of lettuce (B) cultivated in hydroponic NFT Experiment No. 1 as well as in leaves of plants cultivated in pot Experiment No. 2 and 3 (C). Transfer factor /TF/ of vanadium to roots and leaves /heads/ in the all experiments. Means followed by different letters for treatments, separately for each experiments differ significantly at P < 0.05. Color-coded capital letters for vanadium content (green and gray for leaves and roots in Experiment 1 and blue and orange for leaves in Experiments 2 and 3); lowercase letters in the same colors for the TF factor in these experiments. Bars indicate standard error (n = 8).
Following the application of KIO3+V, KIO3+SA+V, and 5-ISA+V, the foliar content of V for hydroponic Experiment 1 was significantly higher than that for the control (Figure 3A); however, it was still at the same level as following the application of KIO3+SA and 5-ISA without ammonium metavanadate. Additionally, in Experiment 1, the combination of KIO3+V was the only one for which V uptake by a single head in Experiment 1 was significantly higher than that for KIO3 without ammonium metavanadate (Supplementary Table 3).
In the two pot experiments with lettuce, foliar V content (Figure 3C) and V uptake by a single head (leaves from one plant) (Supplementary Table 3) were significantly lower than in the control but only for the KIO3 and KIO3+SA combination in Experiment 3. For the remaining combinations, the content of V and V uptake by a single head was the same as in the control in both pot experiments (Figure 3C and Supplementary Table 3).
In Experiment 3, the TF for foliar V was about 20-fold lower than in Experiment 2 (Figure 3C), which was due to lower V content in the peat substrate than in mineral soil (Table 2).
vHPO Activity in Lettuce
The activity of vHPO in roots (Experiment 1) and leaves of lettuce in all three experiments was significantly modified by the application of the compounds studied to the nutrient solution or substrate in the pots (Figures 4A–C). In hydroponic Experiment 1, the vHPO activity measured in roots was 2.5- to 4.0-fold higher than that in leaves (Figures 4A,B). Foliar activity of vHPO in all three experiments was in the range between 0.08 and 1.43 U⋅ng–1 protein.
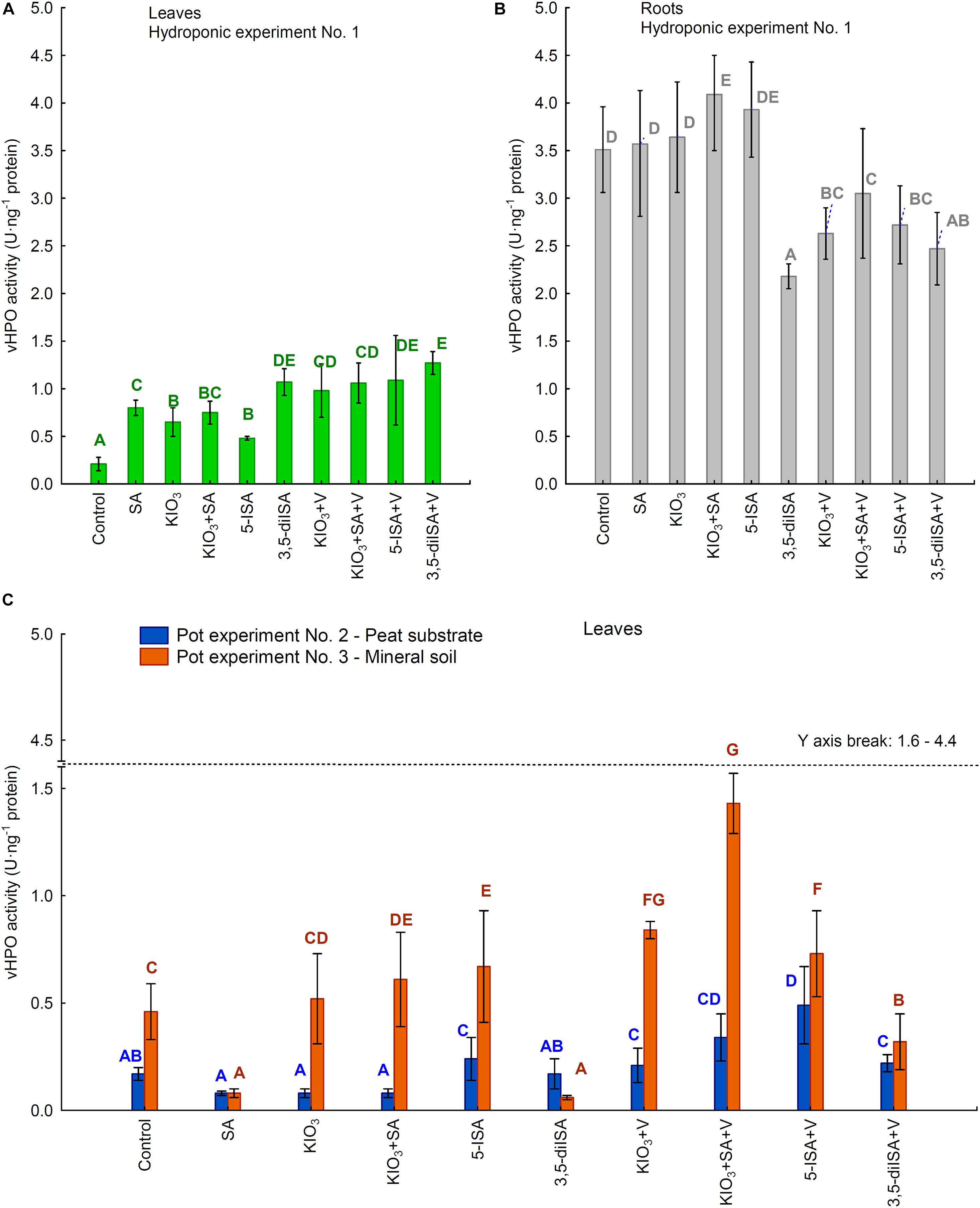
Figure 4. Activity of vanadium-dependent haloperoxidase (vHPO) in leaves /heads/(A) and roots (B) of lettuce cultivated in hydroponic NFT Experiment No. 1 as well as in leaves of plants cultivated in pot Experiment No. 2 and 3 (C). Means followed by different letters for treatments, separately for each experiments differ significantly at P < 0.05. Color-coded capital letters for vHPO activity—green and gray for leaves and roots in Experiment 1 and blue and orange for leaves in Experiments 2 and 3. Bars indicate standard error (n = 8).
The root activity of vHPO following the application of KIO3+SA and 5-ISA (Figure 4B) in the hydroponic system was significantly higher than that in the control. These two combinations also produced a significantly higher foliar activity of vHPO than that in the control (Figure 4A). For all four combinations with ammonium metavanadate fertilization and 3,5-diISA (without V), the root activity of vHPO was lower than in the control, while foliar activity of vHPO was significantly higher than that in the control (Figures 4A,B). Furthermore, 3,5-diISA (vs. 3,5-diISA+V) was the only combination where the activity of vHPO in both roots and leaves was the same. Additional fertilization with V together with KIO3, KIO3+SA, and 5-ISA caused a significant increase in vHPO in leaves and a decrease in the roots compared with the application of these compounds without V.
In each of the three experiments, the highest vHPO activity was measured in leaves of plants representing different combinations: 3,5-diISA+V in Experiment 1 (1.27 U⋅ng–1 protein), 5-ISA+V in Experiment 2 (0.49 U⋅ng–1 protein), and 5-ISA+V in Experiment 3 (1.43 U⋅ng–1 protein) (Figures 4A,C). The lowest foliar activity of vHPO was identified in the control in Experiment 1 (0.21 U⋅ng–1 protein) and for SA in Experiments 2 and 3 (0.08 U⋅ng–1 protein in each experiment).
BeA, SA, and Iodine Metabolites in Secretions Collected as a Result of Root Pressure
The contents of I–, IO3–, BeA, SA, 5-ISA, 2-IBeA, 4-IBeA, 2,3,5-triIBeA, I-Tyr, T3-Na, T3, and T4 measured in root secretions collected as a result of root pressure (RootSec) from plants in Experiment 1 differed significantly between the combinations studied (Table 4).
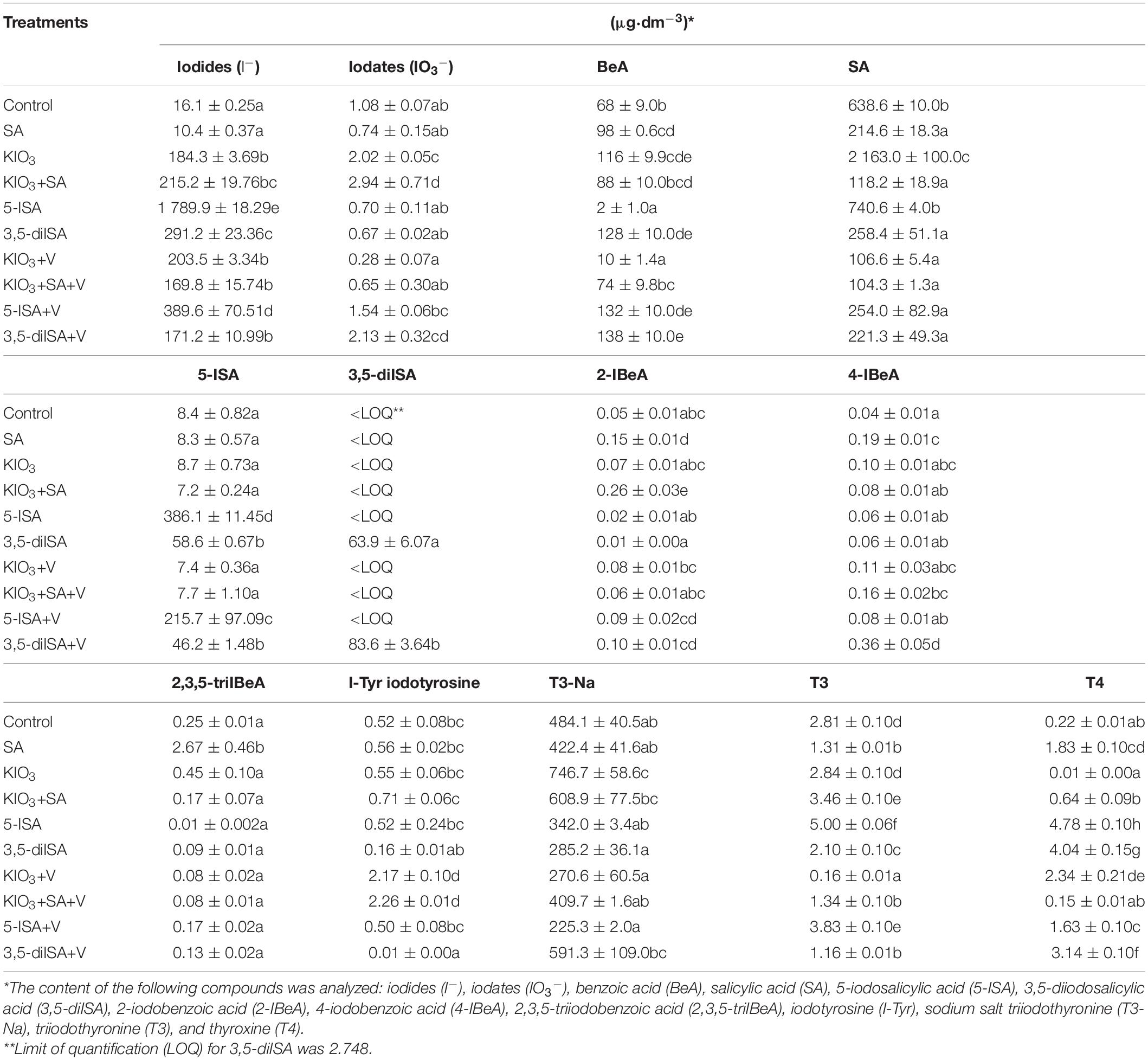
Table 4. Results of the determination of iodides, iodates, organic acids, and iodine metabolites in secretions collected as a result of root pressure (RootSec)—this is in white secretion on the surface of the root neck after cutting the heads (lettuce leaves).
Compared with the control and SA application in all remaining combinations, I content is RootSec was markedly higher. The highest content of I– was found after the application of 5-ISA (without V); it was 9.7-fold higher than when KIO3 was applied to the nutrient solution alone (Table 4). Compared with the control, a significant increase of IO3– was observed in RootSec following the application of KIO3, KIO3+SA, 5-ISA+V, and 3,5-diISA+V, with the highest RootSec content of IO3– being observed for fertilization with KIO3+SA.
3,5-diISA was detected in RootSec exclusively after the application of 3,5-diISA (with or without V). Additionally, the RootSec content of 5-ISA was on average 6.2-fold higher for these two combinations than for the control. However, the highest content of 5-ISA in RootSec (25- to 46-fold higher than in the control) was demonstrated following its application to the nutrient solution. Plants treated with exogenous 5-ISA were characterized by the highest content of T3 and T4 and the lowest content of BeA and 2,3,5-triIBeA in RootSec. The RootSec content of T4 was equally high for fertilization using 3,5-diISA.
For all combinations with fertilization using ammonium metavanadate, application of the compound caused a reduction in T3 content in RootSec; the comparison encompassed combinations with KIO3, KIO3+SA, 3,5-diISA, 5-ISA without and with V (Table 4). The application of 5-ISA+V vs. 5-ISA (without V) had a reduced content of SA, 5-ISA, T3, and T4 and increased 2-IBeA in RootSec. When it comes to fertilization using 3,5-diISA+V vs. 3,5-diISA (without V), it caused a significant increase in BeA, 2-IBeA, and 4-IBeA and a decrease in the content of 5-ISA, T3, and T4 in RootSec.
Application of exogenous SA to the nutrient solution caused a significant increase in the RootSec content of 2,3,5-triIBeA compared with all the remaining combinations (Table 4).
Determination of Iodides (I–) and Iodates (IO3–) in Roots and Leaves of Lettuce in a Hydroponic System (Experiment 1)
Roots and leaves had a higher content of iodides (I–) than iodates (IO3–), from 12.5 times in roots for SA treatment to 53,750 times in leaves for 5-ISA+V treatment (Table 5). There were trace amounts of IO3– in leaves and roots in all combinations subjected to analysis. Even in roots and leaves of plants fertilized with KIO3, the content of IO3– was lower than or similar to the control.
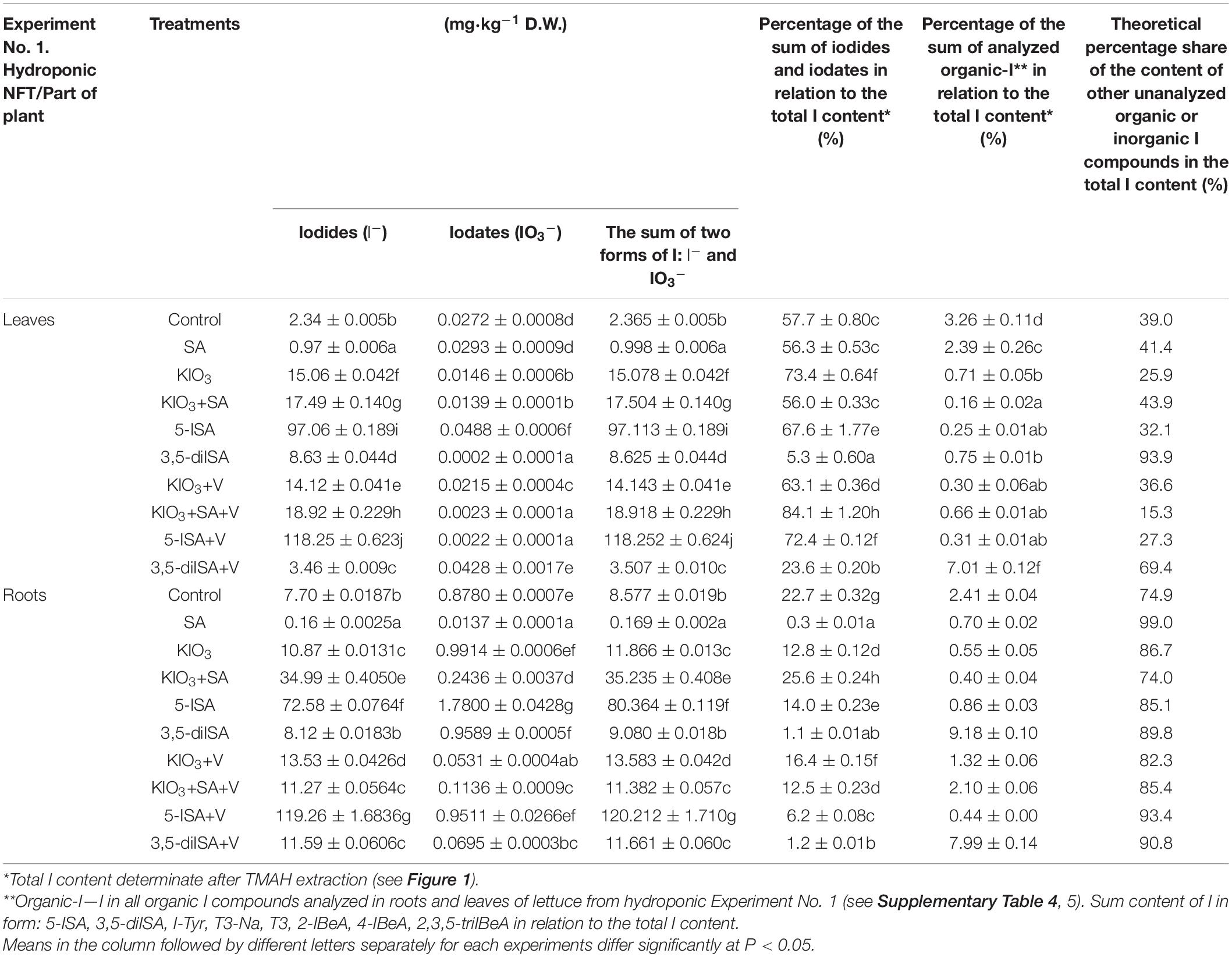
Table 5. Concentrations of iodides (I–) and iodates (IO3–) in roots and leaves of lettuce in hydroponics NFT Experiment No. 1 (speciation of iodine analyzed HPLC-ICP-MS/MS) as well percentage of the iodides and iodates in relation to the total iodine.
The lowest root and leaf content of I– (lower than in the control) was detected in plants treated with exogenous SA (Table 5). The highest content of I– in roots and leaves was found in plants treated with 5-ISA+V; it was significantly higher than in combinations where 5-ISA was used alone.
A comparison of combinations with I applied to the nutrient solution shows the following quantitative root content of I– (Table 5): 5-ISA+V > 5-ISA > KIO3+SA > KIO3+V > 3,5-diISA+V = KIO3+SA+V = KIO3 > 3,5-diISA. The quantitative content of I– in leaves was as follows: 5-ISA+V > 5-ISA > KIO3+SA+V > KIO3+SA > KIO3 > KIO3+V > 3,5-diISA > 3,5-diISA+V.
An additional application of V together with KIO3, KIO3+SA, 5-ISA, and 3,5-diISA in each of these four combinations had a different impact on the I– content in leaves and roots (Table 5) and resulted in an increase or decrease in I– content in leaves and roots, compared with application of exogenous I compounds without V.
The percentage of the sum of iodides (I–) and iodates (IO3–) in relation to total I content was within the range from 0.3 for SA to 25.6 for KIO3+SA in roots, and from 5.3 for 3,5-diISA to 84.1 for KIO3+SA+V in leaves (Table 5).
In the supporting information files were included the results of content of BeA, SA, iodine metabolites in roots and leaves (Supplementary Tables 4, 5) as well as content of I and V in soil after lettuce cultivation (Supplementary Table 6).
Discussion
The aim of research on I biofortification of plants is to establish biofortification regimens, define the threshold of I toxicity for plants, and optimize I biofortification of plants to make it safe and adequate to consumers’ needs (Lawson et al., 2016). Research on I biofortification must be connected with research targeted at expanding knowledge on biochemical, physiologic, and molecular aspects of the functions of trace elements in plants (White and Broadley, 2009).
Plant Biomass and Iodine Biofortification Efficiency Depending on the Chemical Form of Iodine, Vanadium Application, and Type of Cultivation
The dose of I in the hydroponic system was 37.5-fold higher than that in both pot experiments. In consequence, the I compounds (particularly as 5-ISA and secondarily as 3,5-diISA) were applied at a concentration that was too high, negatively impacting plants (reduced yield) in hydroponic Experiment 1. Smoleń et al. (2017) showed that in the hydroponic system, 5-ISA was toxic to lettuce when applied at a dose of 40.0 μM I; the symptoms were not reported after application of 5-ISA at a dose of 1.6 and 8.0 μM I. The authors did not conduct research on 3,5-diISA. Based on the results of a study by Smoleń et al. (2017) and the reduced lettuce biomass shown in this study (Experiment 1), we presume that the threshold of transition from harmful to toxic I activity in lettuce is somewhere between the dose of 8.0 μM and 10.0 μM of I applied as 5-ISA. Because only one dose of 3,5-diISA was tested, it was impossible to establish an exact threshold of harmfulness/toxicity of exogenous 3,5-diISA on lettuce, as was done for 5-ISA.
Welch and Huffman (1973) did not report a significant impact of 1 μM V (as NH4VO3) on the yield of lettuce or tomato compared with the control without V fertilization, for doses ranging from 0.05 to 0.40 μM V. In the three experiments described in the publication, simultaneous fertilization with KIO3, 5-ISA, and 3,5-diISA plus V at a dose of 0.1 μM V (vs. no V) had no negative impact on the biomass of lettuce.
The highest efficacy of I biofortification on lettuce following the application of 5-ISA (in each of the three experiments) compared with 3,5-diISA and KIO3 was justified in research by Smoleń et al. (2017). The authors demonstrated that 5-ISA at a dose of 8.0 μM I was enough to achieve a similar effect of I biofortification in lettuce leaves, as in the case of using KIO3 at a dose of 40.0 μM I. In Experiment 1, the dose of 5-ISA (or, to a lesser extent, 3,5-diISA and KIO3) was too high in the context of a need to balance I content in the daily diet of consumers. This is indicated by the RDA for I (%) in a 100 g portion of fresh lettuce leaves > 480% and HQ > 0.66; the compound would be harmful to consumers if HQ exceeded 1.0. The two iodosalicylates mentioned above, as well as 2-IBeA, 4-IBeA, 2,3,5-triIBeA, and I-Tyr, and T3 were naturally synthesized in lettuce, which further confirms the results of previous studies on lettuce (Smoleń et al., 2020). Halka et al. (2019) showed that these organic I compounds were present in tomato fruits and willow bark. The problem with defining a “target range” for I biofortification of lettuce with non-organic I compounds (KI and KIO3), as well as the determination of human demand of I and estimations of lettuce consumption by the general population were addressed in a study by Lawson et al. (2016). Vegetables enriched with KI and KIO3 have been found to be safe both for humans (Tonacchera et al., 2013) and laboratory rats (Pia̧tkowska et al., 2016). In our studies, exogenous 5-ISA and 3,5-diISA caused a significantly higher accumulation of these compounds in leaves and roots. This information may provoke questions on consumer safety where iodosalicylates are used for I enrichment of plants. There is no direct data on the effect of exogenous iodosalicylates used in vegetable growth on animals or humans. We presume that exogenous iodosalicylates may increase health-promoting effects of domesticated plants. This is because the fertilization of plants using inorganic KI or KIO3 compounds increases synthesis and accumulation of organic I metabolites in plants, as confirmed in research on lettuce (Smoleń et al., 2020) and tomato (Halka et al., 2019). Even though organic I metabolites, including T3, are present in marine algae in much higher amounts than in lettuce (Fenical, 1975), marine algae are still consumed by a number of people worldwide (González et al., 2019).
In hydroponic Experiment 1 with lettuce, I enrichment of plants was higher than in both pot experiments. This was probably because I taken up directly from the nutrient solution was more readily available to roots and because the dose of I per plant was higher than in Experiments 2 and 3. The effectiveness of I biofortification in plants is much higher in hydroponic and soilless systems than when soil fertilization is used (Blasco et al., 2008). This is due to high I sorption by soil, a phenomenon that is absent in hydroponic nutrient solutions. Iodine sorption in soil is attributable to the mineral fractions and SOM (Kashparov et al., 2005), especially the humified aromatic ring of organic matter but not fresh organic matter (Schlegel et al., 2006). After SOM, the following compounds also participate in I sorption by mineral soils: hydroxides Fe/Al (Yoshida et al., 1992), Cu(I)-Fe (III)-sulfides and Cu(I)-sulfides (Lefèvre et al., 2003), as well as Cu/Cr and Cu/Al (Pless et al., 2007). Additionally, I desorption by soil is very slow, which inhibits I uptake by roots (Dai et al., 2004). The SOM also contains SA and its derivatives (Hue et al., 1986). Molecular I or its non-organic anions in the soil may react with aromatic rings of compounds included in SOM (Yamada et al., 1996). Endogenous iodosalicylates and iodobenzoates were identified in the soil prior to lettuce cultivation. The peat substrate was richer in BeA, SA, 5-ISA, and 2-IBeA than mineral soil. In both pot experiments, lettuce heads grown in the peat substrate accumulated less I than those grown in mineral soil. The sorption of I anions (IO3–) by organic soils was higher than in mineral soils (Yamaguchi et al., 2005). This has also been confirmed by the results of analyses of the peat substrate and mineral soil after lettuce cultivation. Post-cultivation I content in the peat substrate was on average 1.5-fold higher than in mineral soil. The highest efficacy of I enrichment of lettuce following the application of 5-ISA in the peat substrate and mineral soil may have resulted from the low degradation or conversion of low-molecular-weight organic aromatic I compounds in soil, which then may be taken up by roots.
Relative Expression of Analyzed Genes vs. Iodine Uptake. Iodine Metabolism in Lettuce
The absence of impact or insignificant increase in V content in leaves following ammonium metavanadate fertilization reported in the three experiments was also confirmed in the literature. When V is applied through a soil fertilizer or nutrient solution, it accumulates in roots and its transport to aboveground parts of the plants is very limited. This was also reported for the cultivation of tomato, Chinese green mustard (Vachirapatama et al., 2011), soybean (Kaplan et al., 1990), rice (Chongkid et al., 2007), and lettuce (Gil et al., 1995). Increased V transfer to the aboveground parts of plants is possible if high concentrations of the element, which are potentially toxic to plants, are used in fertilization (Chongkid et al., 2007; Vachirapatama et al., 2011).
Peroxidases are linked to a number of physiological functions. These include the removal of H2O2, oxidation of toxic reductants, biosynthesis and degradation of lignin, and participation in many other biochemical processes (additional descriptions in Supplementary Data 1). The ion Ca2+ has been described as a cofactor for peroxidase (Pandey et al., 2017). Enzymes from the group of V-dependent haloperoxidases (vHPO) contain the bare metal oxide vanadate, as a prosthetic group (Wever and Hemrika, 2001) (see also Supplementary Data 1). In the presence of H2O2, they oxidize halides (I, Be, Cl) in the following reaction: H2O2 + X– + H+ → H2O + HOX, where X represents Cl–, Br–, or I– (Wever and Hemrika, 2001; Leblanc et al., 2006). The function of vHPO is well-described for marine algae (Leblanc et al., 2006; Verhaeghe et al., 2008). In marine algae, the vHPO enzyme plays a dual function. It can participate in the process of I uptake into cells and is involved in the process of I excretion from cells to the environment in the form of I2 (Leblanc et al., 2006).
An additional application of V with different I compounds and SA had no definitive impact on the expression of per64-like, per12-like, samdmt, cipk6, or msams5 in either roots or leaves. per64-like was the only gene whose expression decreased in the roots of plants treated with KIO3+SA+V, 5-ISA+V, and 3,5-diISA+V (in nutrient solution) when compared to the application of the same compounds without V. For these very same combinations (with and without V), the root activity of vHPO was reported to decrease. Therefore, the level of expression of the per64-like gene was correlated with the activity of vHPO in roots. The decreased activity of vHPO was accompanied by a lower expression of per64-like in plants treated with KIO3+SA+V, 5-ISA+V, and 3,5-diISA+V. These results are sufficient to assign vHPO-like activity to an enzyme encoded by per64-like, rather than the one encoded by per12-like. Perhaps peroxidase encoded by per64-like may have vHPO-like function (may be a V-dependent enzyme). The results of pairwise alignment of protein sequences of A. thaliana PER12 and PER64 with vIPO1 L. digitata and vBPO C. officinalis and A. nodosum showed common regions between them (see also Supplementary Data 1). Further in-silico research is needed to this end or research directed at isolating PER64-like enzyme to be able to examine its structure and functionality depending on the application of V.
Colin et al. (2005) proved that the in-vitro activity of vHPO isolated from Laminaria digitata grew intensively within the range 0–10 mM KI and dropped suddenly when KI > 20 mM was used. In the three experiments conducted as part of our study, exogenous KIO3, 5-ISA, and 3,5-diISA (applied without V) had a different effect on root and foliar activity of vHPO. Before its uptake by roots or immediately thereafter, IO3– must be reduced to I– (Kato et al., 2013). Surprisingly, there was no significant difference in vHPO activity in the roots of plants treated with KIO3 alone (Experiment 1) compared with the control, despite a simultaneous 2.6-fold increase in the expression of the per64-like gene. The results indirectly indicate that other molecular and biochemical mechanisms than vHPO must be involved in the process of root uptake and transport of I– produced following IO3– reduction. These mechanisms are probably responsible for chloride transport. The transport of I– within root cells and to the xylem is analogous to the translocation of Cl– ions and takes the form of symport (H+/anion) or antiport (Na:K/Cl) or is effected through I channels that are permeable to Cl–/I– (White and Broadley, 2001; Roberts, 2006; Colmenero-Flores et al., 2007).
The three experiments share the observation that none of the tested I compounds silenced foliar activity of vHPO. 3,5-diISA was the only compound that reduced the activity of vHPO in roots, an observation consistent with Smoleń et al. (2020). This may be because of the specific effect of 3,5-diISA on vHPO, which inhibited the activity of the enzyme (already at a dose of 10 μM; or 20 μM I) but did not suppress the expression of the per64-like gene, which has been linked with vHPO-like functions. Notably, lower expression of per64-like was reported in the roots of plants treated with 3,5-diISA but only compared with KIO3+SA and 5-ISA, with a simultaneous increase in gene expression in comparison with the control. 5-ISA increased the foliar activity of vHPO in all three experiments compared with the control, which translated into the highest I accumulation in lettuce leaves. The potential mechanism most likely to stimulate foliar activity of vHPO through 5-ISA and 3,5-diISA is not fully understood. It could be a result of catabolism in both iodosalicylates to I–, as only in this form could I be taken up intracellularly with the aid of vHPO in a mechanism resembling the one described for marine algae by Leblanc et al. (2006).
The activity of vHPO and expression of per64-like in leaves and roots were determined at the final stage of cultivation (shortly before lettuce harvesting), which means that the measurements were made after the plants have been exposed to exogenous V and I compounds for an extended time. While theoretically it may seem that V fertilization should increase the activity of vHPO in plants, due to the higher availability of the enzyme’s cofactor, lettuce fertilization with V caused decreased activity of vHPO and expression of the per64-like gene. Reduction in vHPO activity following test fertilization with I compounds + V is consistent with the previous findings of Smoleń et al. (2020). The authors showed that fertilization with V without the simultaneous use of I (plant cultivation with trace amounts of I in the nutrient solution) significantly enhanced the activity of vHPO. Given these results, the assignment of a vHPO-like function to the protein encoded by per64-like seems to be substantively justified.
Fertilization with V did not cause an increase in foliar V content in any of the experiments conducted as part of this research (except for KIO3+V vs. KIO3 in Experiment 1). However, a considerable increase in root V content following V fertilization was observed. The results were consistent with the literature. V fertilization of Chinese greens, at a dose of 0.39, 0.79, and 1.57 mM, caused a proportional increase in V content in the plants, with the following preserved concentration gradient: roots > stems > leaves system (Vachirapatama et al., 2011). A similar V concentration gradient was obtained by Akoumianaki-Ioannidou et al. (2016) for sweet basil fertilized with NH4VO3. The rate of transfer of V from roots to leaves can only be increased if it is used at very high doses, which may be harmful to plants. The negative effect of V on plants also depends on its chemical form. Findings for soybean showed that a harmful dose of VOSO4 was 1.2 mM V (Kaplan et al., 1990). A dose of 0.39 mM V was reported to be harmful for rice (Chongkid et al., 2007). The adverse effects of V may include root darkening, decreased number of secondary roots, decreased turgor pressure, loss of leaf firmness, and plastid degradation in plants (Gil et al., 1995). In Experiment 1, we did not observe any negative impact of V on the development of roots, which was demonstrated by the biomass obtained from the roots of one of the plants.
Expression of cipk6 Gene
The available literature describes the likely biochemical mechanisms of PDTHA activity in plants by comparing their function to thyroid hormones (T3 or T4) in humans. Eneqvist et al. (2003) stated that higher plants can produce a protein homologous to human transthyretin, which is responsible for T3 and T4 transport (TransThy-T3/T4trans). Additionally, the TransThy-T3/T4trans protein from higher plants, including A. thaliana, tomato, and potato was more closely related in the phylogenetic tree of the transthyretin protein family to the protein found in Homo sapiens than to TransThy-T3/T4trans from bacteria or fungi. In model research, Pessoa et al. (2010) showed that exogenous T4 can be bound by transthyretin-like protein in A. thaliana. Furthermore, Power et al. (2000) showed that transthyretin in humans belongs to the group of proteins that includes thyroxine-binding globulin and albumin. The chemical bond is responsible for transporting thyroid hormones in blood. Davis et al. (2000) showed that in humans, T4 can activate signal transduction proteins, such as mitogen-activated protein kinase (MAPK). Additionally, T4 can enhance the activity of several nuclear transactivator proteins, through serine phosphorylation by MAPK. A similar complex mechanism may exist in lettuce. Findings show that the protein encoded by cipk6 can likely perform the function of a T3 and/or T4 receptor (see the functional annotation of the cipk6 gene in Supplementary Data 2). This assumption is justified by results of chemical analyses of roots and RootSec. The synthesis of T3 and T4 (and possibly other isomers of these PDTHAs) probably occurs, with the participation of iodobenzoates and/or iodosalicylates (5-ISA and 3,5-diISA) as the substrates of PDTHA (Figures 5, 6). The results showed that the process occurs primarily in roots. T3 and T4 are then transported to the aboveground parts of plants (Figures 5, 6). The observation of root synthesis of T3 are consistent with the outcome of our previous study (Smoleń et al., 2020). In the present study, we measured T4 directly in leaves and roots. The key problem with T3 and T4 measurements in plant tissues is that there are no analytical protocols dedicated to the analysis of PDTHA content in plants. However, the content of T4 was measured directly in RootSec. Therefore, it is important that we postulate the need to search for and elaborate on the optimum methods or analytical procedures that would enable measurements of the total T4/T3 and other PDTHAs in the tissue of plants. We believe that the results of our analyses of non-organic and organic I metabolites (particularly T3 and T4) in roots, RootSec, and leaves, provide grounds to assign the function of a T3 and/or T4 receptor in lettuce to a protein encoded by cipk6. For cipk6, a significant correlation coefficient was reported between its expression vs.: 1) the content of T3 (r = 0.30∗), 5-ISA (r = 0.72∗), and total content of I (r = 0.84∗) directly in roots and 2) the content of T3 (r = 0.60∗), T4 (r = 0.58∗), 5-ISA (r = 0.89∗), and total content of I (r = 0.89∗) in RootSec. A statistically significant correlation between the expression of cipk6 and total I content (r = 0.26∗) was found in leaves; however, no correlation was reported between the expression of cipk6 and the content of T3 or 5-ISA. This led us to the conclusion that the root activity of cipk6 is closely related to the presence of PDTHA. The effect of PDTHA on cipk6 gene expression in leaves was smaller. This may be proof that the function of the dominant PDTHA receptor in aboveground parts of plants may be performed by a protein encoded by another gene/group of genes. However, the results of research presented herein are insufficient to thoroughly describe the physiological function of PDTHA in lettuce.
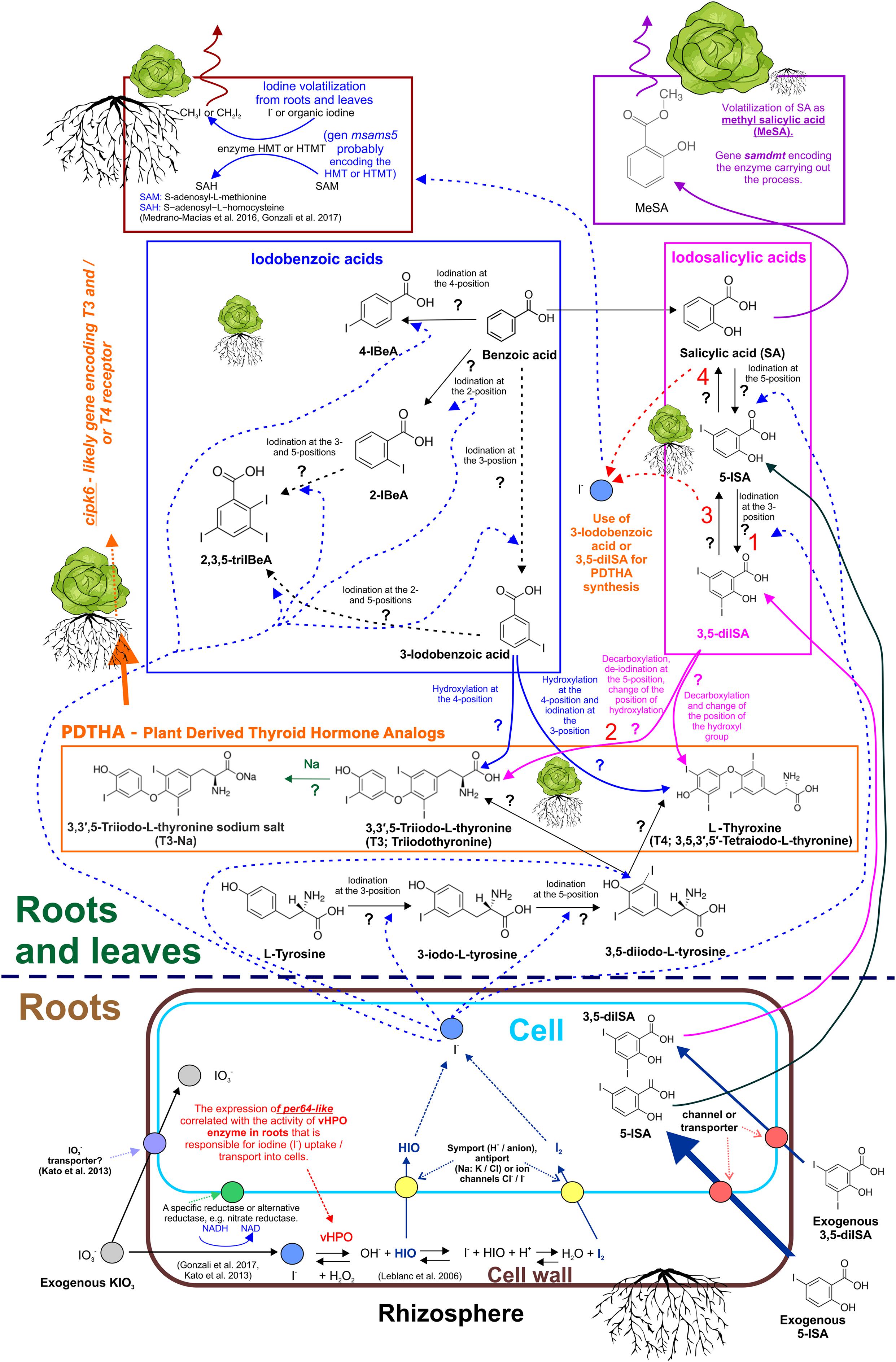
Figure 5. Mechanism of uptake of non-organic and organic iodine compounds. Theoretical metabolic pathway of iodosalicylates, iodobenzoates, and plant-derived thyroid hormone analogs (PDTHA) in lettuce—summary of the study and literature data. 1 and 2—Processes that mainly occur in roots. Foliar activity intensifies after application of 5-ISA and 3,5-diISA. 3—Deiodination of 3,5-diISA. Observed increased content of 5-ISA following exogenous application of 3,5-diISA. 4—Deiodination of 5-diSA. Observed increased content of SA following exogenous application of 5-diSA and 3,5-diISA. ?—Undefined enzymatic/metabolic processes that carry out these reactions.
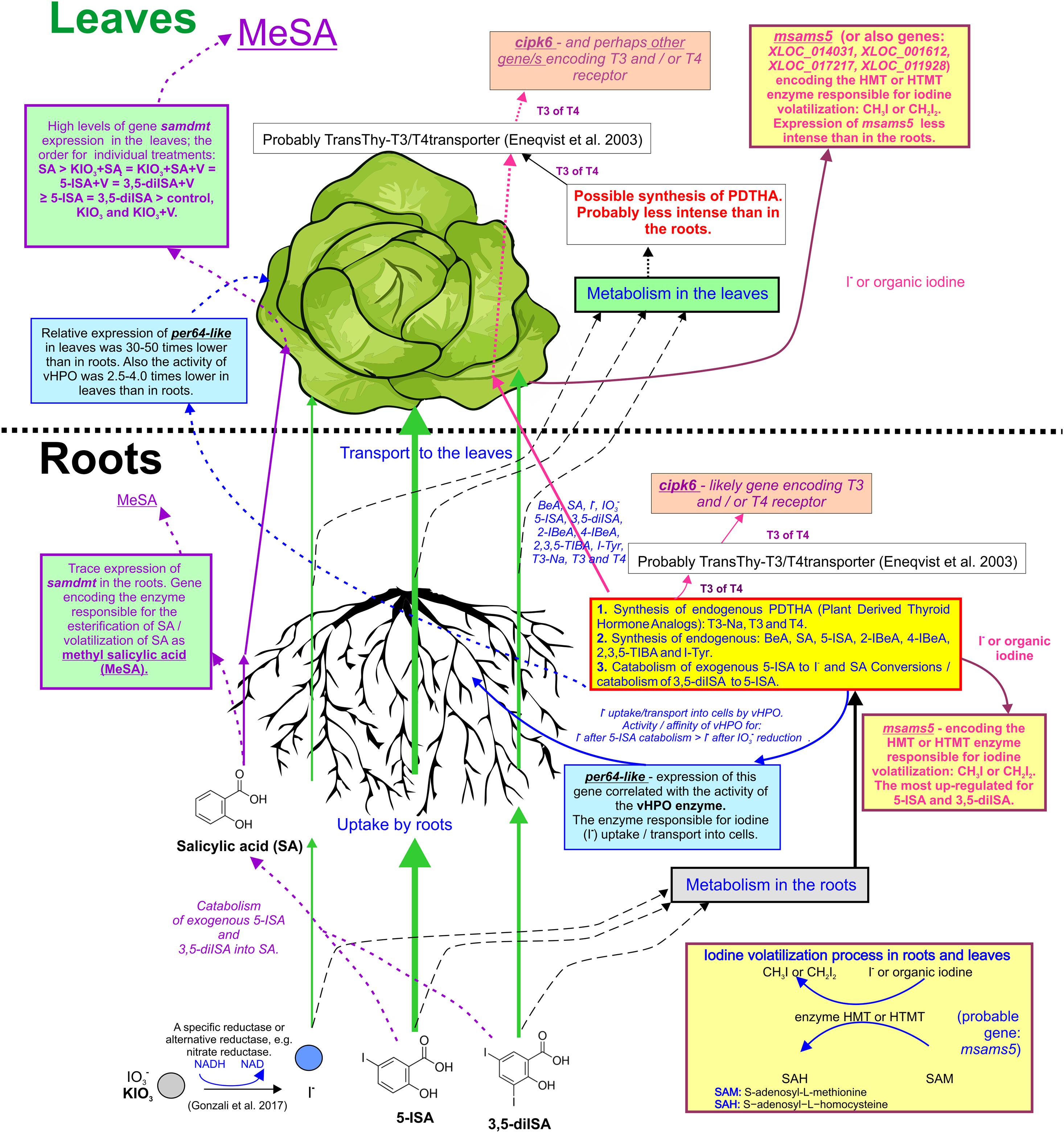
Figure 6. Graphic summary of study outcomes: uptake, transport, and metabolic pathways of iodine compounds and SA. Genes with described activity and function: Peroxidase 64-like (per64-like): the gene’s activity has been linked to the activity/functionality of V-dependent haloperoxidase (vHPO) in roots and leaves of lettuce. Peroxidase 12-like (per12-like): no correlation has been found between the gene’s expression and vHPO activity in roots. S-adenosyl-L-methionine-dependent methyltransferase (samdmt): gene that possibly encodes an enzyme that conducts the process of esterification/volatilization of methyl salicylic acid (MeSA). CBL-interacting serine/threonine-protein kinase 6 (cipk6): gene that likely encodes triiodothyronine (T3) and/or thyroxine (T4) receptors. S-adenosylmethionine synthase 5 (msams5): gene that possibly encodes an enzyme responsible for I volatilization in the process of methylation (synthesis of CH3I or CH2I2).
The highest expression of cipk6 in roots and leaves of plants treated with 5-ISA was unequivocally associated with I uptake and metabolism in these plants. The plants were found to demonstrate the highest I-uptake and total I content, and had increased 5-ISA, 2-IBeA, 3,5-diISA, I–, and IO3– levels in roots and leaves. In addition, RootSec from plants where 5-ISA had been applied were also found to have the highest content of 5-ISA and I metabolites, such as I–, T3, and T4. The results clearly showed that there is a close interdependence between a larger preference to take up 5-ISA (compared with IO3– and 3,5-diISA) and the metabolism of I compounds which, among others, induces the synthesis of PDTHA and increases the expression of cipk6, a gene of the T3 and/or T4 receptor.
Expression of the msams5 Gene
The functions of S-adenosyl-l-methionine (SAM)-dependent halide methyltransferase (HMT) or SAM-dependent halide/thiol methyltransferase (HTMT), enzymes responsible for I volatilization to CH3I or CH2I2, are subject to a complex control mechanism and are typical of a number of marine algae species (Keng et al., 2020) and terrestrial plants (Attieh et al., 2000; Nagatoshi and Nakamura, 2007; Itoh et al., 2009; Landini et al., 2012). These enzymes are not specific for I as a substrate but can also participate in the methylation of other group-17 elements (halogens) from the periodic table. A different, complicated affinity in the substrate-enzyme system is as follows: I– > Br– > Cl– (Manley, 2002; Murphy, 2003). In B. oleracea, HTMT was also involved in the process of methylation of [SH]- and [SCN]- groups to CH3SH (Attieh et al., 2000).
The available literature does not name the specific gene(s) responsible for I methylation in lettuce. The results justify the assignment of the potential HMT or HTMT function to an enzyme, S-adenosylmethionine synthase 5, encoded by the msams5 gene. Characteristics of the L. sativa MSAMS5 protein are shown in Supplementary Data 3. Significant overexpression (about 8.5-fold higher than the control) of the msams5 gene in the roots of plants treated with exogenous 5-ISA was detected. These results and, indirectly, the total root content of I and iodine metabolites show that a protein of the msams5-encoded enzyme may be immediately associated with I methylation by lettuce roots. Iodine volatilization through roots (with the participation of msams5-encoded enzyme) was higher following the application of iodosalicylates than when using KIO3. This indicates that the enzyme protein encoded by msams5 might have played a dominant role in I methylation in roots but not in leaves. Compared with the control, foliar expression of msams5 was slightly, yet significantly, increased only following the application of KIO3. Therefore, foliar expression of the msams5 gene did not reflect the reported accumulation of I or its organic or non-organic compounds. The process of methylation (volatilization) of gaseous I from leaves probably occurred with the participation of the enzyme(s) encoded by a gene(s) other than msams5. On the basis of the transcriptome analysis (Kȩska et al., 2019), we were able to identify other genes in lettuce leaves that may potentially be associated with synthesis of enzymes with HMT- or HTMT-like function, i.e., with the synthesis of CH3I or CH2I2. The list includes the following genes described in the genome of lettuce: S-adenosylmethionine synthase (XLOC_014031) (Lsat_1_v5_gn_6_117861.1), lysine-specific demethylase REF6 methyltransferase (XLOC_001612) (Lsat_1_v5_gn_1_28820.1), probable methyltransferase At1g27930 (XLOC_017217) (Lsat_1_v5_gn_8_148061.1), and histone-lysine N-methyltransferase (XLOC_011928) (Lsat_1_v5_gn_5_154240.1).
Notably, in vitro emission of CH3I, CH3Br, and CH3Cl by rice depended on the stadium of the plants’ development (Redeker et al., 2004). Secretion of CH3I by rice during the day was nearly twice that observed at night (Muramatsu and Yoshida, 1995; Muramatsu et al., 1995). Gonzali et al. (2017) suggested that because the structure of HMT or HTMT and other methyltransferases is homologic, they are probably involved in plant salinity tolerance or play a role in the protection of plants against diseases. The interpretation assumed in the literature is that the process of I methylation (synthesis of CH3I or CH2I2) serves to detoxify plants from excess I content in tissues (Landini et al., 2012; Medrano-Macías et al., 2016; Gonzali et al., 2017). The results justify the presumption that the process of volatilization of gaseous I compounds, i.e., CH3I or CH2I2 (associated with HMT- or HTMT-like enzymatic activity) may also perform a different physiological function that is not yet described in the literature.
The activity of S-adenosylmethionine synthases, including those encoded by msams5, requires divalent cations, such as Mg2+, Mn2+, or Ca2+, and monovalent cations, such as K+ or Na+ (Supplementary Data 3). The V cation (VO2+) may replace divalent cations at the active site of this type of enzyme (Chasteen, 1995). Morrell et al. (1986) showed that biotransformation (oxidation) of V from vanadate (VO3–) to vanadyl (VO2+) during its uptake by plants is possible. In the three experiments conducted as part of the research, V was applied as VO3–. The research results obtained imply that VO3– transformation to VO2+ was weaker in the presence of exogenous 5-ISA and 3,5-diISA. This is indicated by reduced activity of msams5, which encodes an enzyme dependent on VO2+ and not on VO3–, and decreased V uptake by roots and leaves, in particular for the 3,5-diISA+V combination (in Experiment 1).
Expression of samdmt Gene
The process of methylation (volatilization of methyl salicylic acid ester [MeSA]) occurs with the participation of an enzyme called salicylic acid carboxyl methyltransferase (SAMT) (Tieman et al., 2010). The synthesis of MeSA is one of the many processes in the production of SA derivatives in plants. MeSA participates in processes responsible for SAR. MeSA is volatilized from roots and overground parts of plants and can be transported by the phloem (Gao et al., 2014). The process of biosynthesis of MeSA is catalyzed by SA methyltransferases (SAMT/BSMT); the reconversion of MeSA back to SA by methyl esterase (MES, SABP2) is also possible (Park et al., 2009; Gao et al., 2014).
In our study the expression of the S-adenosyl-L-methionine-dependent methyltransferase gene (samdmt) (Supplementary Data 4) in lettuce leaves was clearly associated with application of exogenous SA, SA + KIO3 (KIO3+SA, KIO3+SA+V), and both iodosalicylates, 5-ISA and 3,5-diISA, with or without V. We reported a significant correlation between the foliar activity of samdmt and (1) the RootSec content of SA transported from roots to leaves (r = 0.80∗) and (2) the foliar content of SA and 5-ISA (r = 0.72∗ for SA and r = 0.77∗ for 5-ISA). Additionally, the results of measurements of all organic I metabolites and SA in roots and leaves showed that exogenous 5-ISA and 3,5-diISA underwent at least a 2-way transformation (Figures 5, 6). Conversely, 5-ISA and 3,5-diISA served as substrates in PDTHA synthesis, while they underwent a catabolic/decomposition reaction: (1) to I– ions that could be used as substrates in CH3I or CH2I2 synthesis with the participation of the msams5-encoded enzyme or (2) to the SA molecule. This is indicated by the elevated SA content in roots and leaves and, consequently, by an increased foliar expression of samdmt in plants treated with exogenous 5-ISA or 3,5-diISA. Therefore, the results justify the assignment of a SAMTase-like function (esterification of SA; volatilization of MeSA) to the samdmt gene. The process was probably far more intense in leaves than in roots. This was exemplified by the relatively high expression of samdmt in leaves and trace functionality of the gene in the roots. For this very reason, no significant correlation was found between the activity of samdmt and root content of SA, 5-ISA, and 3,5- diISA.
Conclusion
The direction of metabolic conversion of KIO3, 5-ISA, and 3,5-diISA in plants was documented. Both iodosalicylates were applied exogenously and underwent degradation inside the plants to I ions or served as precursors of synthesis of T3 and T4, classified as PDTHAs. The assignment of the role of encoding protein receptor T3 or T4, mainly in lettuce roots, to cipk6 was proposed.
There are reasons to believe that the per64-like, rather than the per12-like gene, may act as a V-dependent haloperoxidase (vHPO), an enzyme that participates in I uptake (expression of per64-like gene in roots > leaves). The expression of msams5 was sufficiently specific to link the gene to the functions of HMT/HTMT enzymes. This gene was overexpressed in roots in systems where exogenous iodosalicylates were applied. The expression of samdmt, in turn, makes it naturally shortlisted for the role of a gene encoding the enzyme responsible for esterification/volatilization of ethyl salicylic acid (activity: leaves > roots).
V added to the nutrient solution caused a significant reduction and growth of I content in roots, but not in leaves, for the combination of: KIO3+SA+V vs. KIO3+SA and 3,5-diISA+V vs. 3,5-diISA, respectively. V was mostly accumulated in roots, with its transfer to leaves being limited. The level of per64-like expression was correlated with root activity of vHPO.
Plant enrichment with I through 5-ISA and 3,5-diISA was more effective than that through KIO3. The results of pot experiments indicated that the I compounds tested, including 5-ISA and 3,5-diISA, in particular, may be used in I enrichment of plants through fertigation without the fear of harming the plants. The level of plant enrichment in I was safe for consumers. This is implied by the fact that the highest HQ-I in pot studies was 0.071. Consumers’ safety would be at risk if the HQ exceeded 1.0. In Experiment 1 (hydroponic system), the efficacy of I uptake from the nutrient solution was higher than in the mineral soil or peat substrate. However, in the context of balancing the reference daily allowance of I for humans, the achieved level of I accumulation (especially following application of 5-ISA) was too high [as shown by RDA-I (%) > 480%, HQ > 0.66]. This means that doses < 10 μM of I compounds can be recommended for hydroponic systems, especially where both iodosalicylates are used.
I-enriched lettuce strongly reduces the in vitro development of cancerous cells in colon cancer (Koronowicz et al., 2016). It seems appropriate to study the use of lettuce enriched with 5-ISA and 3,5-diISA in nutrigenomics.
Data Availability Statement
The datasets presented in this study can be found in the online repositories. The names of the repository/repositories and accession number(s) can be found in the article/Supplementary Material.
Author Contributions
SS, MC, IK, and JP: methodology. SS, MC, and KK: formal analysis. SS: funding acquisition. SS, IK, MH, MG, ŁS, JP, and AK: investigation of lettuce cultivation and chemical analyses of plant, nutrient solution, and soil samples. MC, KK, and DG: investigation of molecular analyses of lettuce. SS, MC, IK, and KK: resources. SS and MC: supervision. SS, MC, and KK: writing—original draft. SS, MC, IK, KK, MH, MG, DG, ŁS, and PK: writing—review and editing. All authors contributed to the article and approved the submitted version.
Funding
This research was financed by the National Science Centre, Poland (Grant No. UMO-2017/25/B/NZ9/00312), “Molecular and physiological mechanisms of vanadium influence on the uptake of iodates and iodosalicylates by lettuce and its anticancer effect tested on human cell lines.”
Conflict of Interest
The authors declare that the research was conducted in the absence of any commercial or financial relationships that could be construed as a potential conflict of interest.
Acknowledgments
The method of enriching plants with I, i.e., with 5-iodosalicylic acid and 3,5-diiodosalicylic acid, is patented by the Polish Patent Office. Patent number P.410806 (November 20, 2017).
Supplementary Material
The Supplementary Material for this article can be found online at: https://www.frontiersin.org/articles/10.3389/fpls.2021.653168/full#supplementary-material
Supplementary Data 1 | The functional annotation of per12-like i per64-like genes.
Supplementary Data 2 | The functional annotation of cipk6 gene.
Supplementary Data 3 | The functional annotation of msams5 gene.
Supplementary Data 4 | The functional annotation of samdmt gene.
Footnotes
- ^ https://primer3plus.com/cgi-bin/dev/primer3plus.cgi
- ^ https://lgr.genomecenter.ucdavis.edu
- ^ https://eu.idtdna.com/calc/analyzer
- ^ https://www.tibco.com/products/data-science
References
Afkhami, A. M., Karimi, M., Nourian, F., and Sohey, L. (2009). Comparison of the effects of sodium metavanadate and zinc sulfate supplementation on lipid and glucose in patients with type 2 diabetes. Iranian J. Diab. Obesit. 1, 22–29.
Ajiwe, S. T., Popoola, A. R., Afolabi, C. G., Oduwaye, O. A., Ganiyu, S. A., Fajinmi, O. B., et al. (2019). Effect of iodine biofortification on incidence and severity of Fusarium wilt and yield of tomato (Solanum lycopersicum L.). Nigerian J. Biotechnol. 36, 146–151. doi: 10.4314/njb.v36i1.19
Akoumianaki-Ioannidou, A., Barouchas, P. E., Ilia, E., Kyramariou, A., and Moustakas, N. K. (2016). Effect of vanadium on dry matter and nutrient concentration in sweet basil (’Ocimum basilicum’ L.). Aust. J. Crop. Sci. 10, 199–206.
Almeida, M. G., Humanes, M., Melo, R., Silva, J. A., Da Silva, J. F., and Wever, R. (2000). Purification and characterisation of vanadium haloperoxidases from the brown alga Pelvetia canaliculata. Phytochemistry 54, 5–11. doi: 10.1016/S0031-9422(99)00602-0
Anke, M., Illing-Günther, H., Gürtler, H., Holzinger, S., Jaritz, M., Anke, S., et al. (2002). Vanadium—an essential element for animals and humans? Trace Elem. Man Anim. 10, 221–225.
Antonyak, H. L., Panas, N. E., Pershyn, O. I, Polishchuk, A. I., and Hoyvanovych, N. K. (2018). Iodine in abiotic and biotic environments. Biol. Stud. 12, 117–134. doi: 10.30970/sbi.1202.567
Attieh, J., Sparace, S. A., and Saini, H. S. (2000). Purification and properties of multiple isoforms of a novel thiol methyltransferase involved in the production of volatile sulfur compounds from Brassica oleracea. Arch. Biochem. Biophys. 380, 257–[[4051]]266. doi: 10.1006/abbi.2000.1896
Basiouny, F. M. (1984). Distribution of vanadium and its influence on chlorophyll formation and iron metabolism in tomato plants. J. Plant Nutr. 7, 1059–1073. doi: 10.1080/01904168409363265
Blasco, B., Riosm, J. J., Cervillam, L. M., Sánchez-Rodrigezm, E., Ruizm, J. M., and Romero, L. (2008). Iodine biofortification and antioxidant capacity of lettuce: potential benefits for cultivation and human health. Ann. Appl. Biol. 152, 289–299. doi: 10.1111/j.1744-7348.2008.00217.x
Budke, C., thor Straten, S., Mühling, K. H., Broll, G., and Daum, D. (2020). Iodine biofortification of field-grown strawberries–APPROACHES and their limitations. Sci. Hort. 269:109317. doi: 10.1016/j.scienta.2020.109317
Cakmak, I., Marzorati, M., Van den Abbeele, P., Hora, K., Holwerda, H. T., Yazici, M. A., et al. (2020). Fate and bioaccessibility of iodine in food prepared from agronomically biofortified wheat and rice and impact of cofertilization with zinc and selenium. J. Agricult. Food Chem. 68, 1525–1535. doi: 10.1021/acs.jafc.9b05912
Cappuyns, V., and Swennen, R. (2014). Release of vanadium from oxidized sediments: insights from different extraction and leaching procedures. Environ. Sci. Pollut. Res. Int. 21, 2272–2282. doi: 10.1007/s11356-013-2149-0
Chongkid, B., Vachirapattama, N., and Jirakiattikul, Y. (2007). Effects of vanadium on rice growth and vanadium accumulation in rice tissues. Kasetsart J. Nat. Sci. 41, 28–33.
Colin, C., Leblanc, C., Michel, G., Wagner, E., Leize-Wagner, E., Van Dorsselaer, A., et al. (2005). Vanadium-dependent iodoperoxidases in Laminaria digitata, a novel biochemical function diverging from brown algal bromoperoxidases. JBIC J. Biol. Inor. Chem. 10, 156–166. doi: 10.1007/s00775-005-0626-8
Colin, C., Leblanc, C., Wagner, E., Delage, L., Leize-Wagner, E., Van Dorsselaer, A., et al. (2003). The brown algal kelp Laminaria digitata features distinct bromoperoxidase and iodoperoxidase activities. J. Biol. Chem. 278, 23545–23552. doi: 10.1074/jbc.M300247200
Colmenero-Flores, J. M., Martínez, G., Gamba, G., Vázquez, N., Iglesias, D. J., Brumós, J., et al. (2007). Identification and functional characterization of cation-chloride cotransporters in plants. Plant J. 50, 278–292. doi: 10.1111/j.1365-313X.2007.03048.x
Dai, J. L., Zhang, M., and Zhu, Y. G. (2004). Adsorption and desorption of iodine by various Chinese soils. I Iodate. Environ. Int. 30, 525–530. doi: 10.1016/j.envint.2003.10.007
Dávila-Rangel, I. E., Trejo Téllez, L. I., Ortega Ortiz, H., Juárez Maldonado, A., González Morales, S., Companioni González, B., et al. (2020). Comparison of iodide, iodate, and iodine-chitosan complexes for the biofortification of lettuce. Appl. Sci. 10:2378. doi: 10.3390/app10072378
Davis, P. J., Shih, A., Lin, H. Y., Martino, L. J., and Davis, F. B. (2000). Thyroxine promotes association of mitogen-activated protein kinase and nuclear thyroid hormone receptor (TR) and causes serine phosphorylation of TR. J. Biol. Chem. 275, 38032–38039. doi: 10.1074/jbc.M002560200
Dobosy, P., Kröpfl, K., Óvári, M., Sandil, S., Németh, K., Engloner, A., et al. (2020). Biofortification of green bean (Phaseolus vulgaris L.) and lettuce (Lactuca sativa L.) with iodine in a plant-calcareous sandy soil system irrigated with water containing KI. J. Food. Compos. Anal. 88:103434. doi: 10.1016/j.jfca.2020.103434
Eneqvist, T., Lundberg, E., Nilsson, L., Abagyan, R., and Sauer-Eriksson, A. E. (2003). The transthyretin-related protein family. Eur. J. Biochem. 270, 518–532. doi: 10.1046/j.1432-1033.2003.03408.x
Fenical, W. (1975). Halogenation in the rhodophyta 1, 2 a review. J. Phycol. 11, 245–259. doi: 10.1111/j.1529-8817.1975.tb02775.x
Fowden, L. (1959). Radioactive iodine incorporation into organic compounds of various angiosperms. Physiol. Plant. 12, 657–664. doi: 10.1111/j.1399-3054.1959.tb08900.x
Gao, Q. M., Kachroo, A., and Kachroo, P. (2014). Chemical inducers of systemic immunity in plants. J. Exp. Bot. 65, 1849–1855. doi: 10.1093/jxb/eru010
Gil, J., Álvarez, C. E., Martinez, M. C., and Pérez, N. (1995). Effect of vanadium on lettuce growth, cationic nutrition, and yield. J. Environ. Sci. Health Part A 30, 73–87. doi: 10.1080/10934529509376186
Golob, A., Novak, T., Maršić, N. K., Šircelj, H., Stibilj, V., Jerše, A., et al. (2020). Biofortification with selenium and iodine changes morphological properties of Brassica oleracea L. var. gongylodes) and increases their contents in tubers. Plant Physiol. Biochem. 150, 234–243. doi: 10.1016/j.plaphy.2020.02.044
Gonnella, M., Renna, M., D’Imperio, M., Santamaria, P., and Serio, F. (2019). Iodine biofortification of four brassica genotypes is effective already at low rates of potassium iodate. Nutrients 11:451. doi: 10.3390/nu11020451
González, A., Paz, S., Rubio, C., Gutiérrez, ÁJ., and Hardisson, A. (2019). Human exposure to iodine from the consumption of edible seaweeds. Biol. Trace Elem. Res. 197, 361–366. doi: 10.1007/s12011-019-01996-w
Gonzali, S., Kiferle, C., and Perata, P. (2017). Iodine biofortification of crops: agronomic biofortification, metabolic engineering and iodine bioavailability. Curr. Opin. Biotechnol. 44, 16–26. doi: 10.1016/j.copbio.2016.10.004
Gruzewska, K., Michno, A., Pawelczyk, T., and Bielarczyk, H. (2014). Essentiality and toxicity of vanadium supplements in health and pathology. J. Physiol. Pharmacol. 65, 603–611.
Gust, A. A., and Nürnberger, T. (2012). From plant immunology: a life or death switch. Nature 486, 198–199. doi: 10.1038/486198a
Halka, M., Smoleń, S., Czernicka, M., Klimek-Chodacka, M., Pitala, J., and Tutaj, K. (2019). Iodine biofortification through expression of HMT, SAMT and S3H genes in Solanum lycopersicum L. Plant Physiol. Biochem. 144, 35–48. doi: 10.1016/j.plaphy.2019.09.028
Hayat, Q., Hakat, S., Irfan, M., and Ahmad, A. (2010). Effect of exogenous salicylic acid under changing environment: a review. Environ. Exp. Bot. 68, 14–25. doi: 10.1016/j.envexpbot.2009.08.005
Hue, N. V., Craddock, G. R., and Adams, F. (1986). Effect of organic acids on aluminum toxicity in subsoils. Soil Sci. Soc. Am. J. 50, 28–34. doi: 10.2136/sssaj1986.03615995005000010006x
Humphrey, O. S., Young, S. D., Bailey, E. H., Crout, N. M. J., Ander, E. L., Hamilton, E. M., et al. (2019). Iodine uptake, storage and translocation mechanisms in spinach (Spinacia oleracea L.). Environ. Geochem. Health 41, 2145–2156. doi: 10.1007/s10653-019-00272-z
Imtiaz, M., Ashraf, M., Rizwan, M. S., Nawaz, M. A., Rizwan, M., Mehmood, S., et al. (2018). Vanadium toxicity in chickpea (Cicer arietinum L.) grown in red soil: effects on cell death, ROS and antioxidative systems. Ecotox. Environ. Safe 158, 139–144. doi: 10.1016/j.ecoenv.2018.04.022
Incrocci, L., Carmassi, G., Maggini, R., Poli, C., Saidov, D., Tamburini, C., et al. (2019). Iodine accumulation and tolerance in sweet basil (Ocimum basilicum L.) with green or purple leaves grown in floating system technique. Front Plant Sci. 10:1494. doi: 10.3389/fpls.2019.01494
Itoh, N., Toda, H., Matsuda, M., Negishi, T., Taniguchi, T., and Ohsawa, N. (2009). Involvement of S-adenosylmethionine-dependent halide/thiol methyltransferase (HTMT) in methyl halide emissions from agricultural plants: isolation and characterization of an HTMT-coding gene from Raphanus sativus (Daikon radish). BMC Plant Biol. 9:116. doi: 10.1186/1471-2229-9-116
Jones, J. B. Jr. (2016). Hydroponics: A Practical Guide for the Soilless Grower, 2nd Edn. Boca Raton, FL: CRC press.
Kaplan, D. I., Adriano, D. C., Carlson, C. L., and Sajwan, K. S. (1990). Vanadium: toxicity and accumulation by beans. Water Air Soil Pollut. 49, 81–91. doi: 10.1007/BF00279512
Kashparov, V., Colle, C., Zvarich, S., Yoschenko, V., Levchuk, S., and Lundin, S. (2005). Soil-to-plant halogens transfer studiem 1. Root uptake of radioiodine by plants. J. Environ. Radioact. 79, 187–204. doi: 10.1016/j.jenvrad.2004.06.005
Kato, S., Wachi, T., Yoshihira, K., Nakagawa, T., Ishikawa, A., Takagi, D., et al. (2013). Rice (Oryza sativa L.) roots have iodate reduction activity in response to iodine. Front. Plant Sci. 4:227. doi: 10.3389/fpls.2013.00227
Keng, F. S. L., Phang, S. M., Abd Rahman, N., Elvidge, E. C. L., Malin, G., and Sturges, W. T. (2020). The emission of volatile halocarbons by seaweeds and their response towards environmental changes. J. Appl. Phycol. 32, 1377–1394. doi: 10.1007/s10811-019-02026-x
Kȩska, K., Czernicka, M., Smoleń, S., and Kowalska, I. (2019). “Uptake of iodine in the form of KIO3 depending on the vanadium application in lettuce: transcriptomic approach,” in Proceedings of the PLANT BIOLOGY CS 2019 Conference 27-30.08.2019, České Budějovice.
Kiferle, C., Ascrizzi, R., Martinelli, M., Gonzali, S., Mariotti, L., Pistelli, L., et al. (2019). Effect of iodine treatments on Ocimum basilicum L.: biofortification, phenolics production and essential oil composition. PLoS One 15:e0229016. doi: 10.1371/journal.pone.0226559
Kongkiattikajorn, J., and Pongdam, S. (2006). Vanadium haloperoxidase from the red alga Gracilaria fisheri. Sci. Asia 32, 25–30. doi: 10.2306/scienceasia1513-1874.2006.32(s1).025
Koronowicz, A., Kopeć, A., Master, A., Smoleń, S., Pia̧tkowska, E., Bieżanowska-Kopeć, R., et al. (2016). Transcriptome profiling of Caco-2 cancer cell line following treatment with extracts from iodine-biofortified lettuce (Lactuca sativa L.). PLoS One 11:e0147336. doi: 10.1371/journal.pone.0147336
Landini, M., Gonzali, S., Kiferle, C., Tonacchera, M., Agretti, P., Dimida, A., et al. (2012). Metabolic engineering of the iodine content in Arabidopsis. Sci. Rep. 2:338. doi: 10.1038/srep00338
Lawson, P. G., Daum, D., Czauderna, R., and Vorsatz, C. (2016). Factors influencing the efficacy of iodine foliar sprays used for biofortifying butterhead lettuce (Lactuca sativa). J. Plant Nutri. Soil Sci. 179, 661–669. doi: 10.1002/jpln.201600213
Leblanc, C., Colin, C., Cosse, A., Delage, L., La Barre, S., Morin, P., et al. (2006). Iodine transfers in the coastal marine environment: the key role of brown algae and of their vanadium-dependent haloperoxidases. Biochemie 88, 1773–1785. doi: 10.1016/j.biochi.2006.09.001
Lefèvre, G., Bessière, J., Ehrhardt, J. J., and Walcarius, A. (2003). Immobilization of iodide on copper (I) sulfide minerals. J. Eviron. Radioactiv. 70, 73–83. doi: 10.1016/S0265-931X(03)00119-X
Lima, S. T. C., Merrigan, T. L., and Rodrigues, E. D. (2012). “Synthetic and plant derived thyroid hormone analogs,” in Thyroid and Parathyroid Diseases–New Insights into Some Old and Some New Issues, ed. L. S. Ward (London: IntechOpen), 221.
Livak, K. J., and Schmittgen, T. D. (2001). Analysis of relative gene expression data using real-time quantitative PCR and the 2–ΔΔct method. Methods 25, 402–408.
Manley, S. L. (2002). Phytogenesis of halomethanes: a product of selection or a metabolic accident? Biogeochemistry 60, 163–180. doi: 10.1023/A:1019859922489
Medrano-Macías, J., Leija-Martínez, P., González-Morales, S., Juárez-Maldonado, A., and Benavides-Mendoza, A. (2016). Use of iodine to biofortify and promote growth and stress tolerance in crops. Front. Plant Sci. 7:1146. doi: 10.3389/fpls.2016.01146
Mengel, K., and Kirkby, E. A. (1996). Principles of Plant Nutrition. Bern: International Potash Institute.
Morrell, B. G., Lepp, N. W., and Phipps, D. A. (1986). Vanadium uptake by higher plants: some recent developments. Environ. Geochem. Health 8, 14–18. doi: 10.1007/BF02280116
Muramatsu, Y., and Yoshida, S. (1995). Volatilization of methyl iodide from the soil-plant system. Atmosph. Environ. 29, 21–25. doi: 10.1016/1352-2310(94)00220-F
Muramatsu, Y., Yoshida, S., and Bannai, T. (1995). Tracer experiments on the behaviour of radioiodine in the soil-plant-atmosphere system. J. Radioanal. Nuclear Chem. 194, 303–310. doi: 10.1007/BF02038428
Murphy, C. (2003). New frontiers in biological halogenation. J. Appl. Microbiol. 94, 539–548. doi: 10.1046/j.1365-2672.2003.01900.x
Nagatoshi, Y., and Nakamura, T. (2007). Characterization of three halide methyltransferases in Arabidopsis thaliana. Plant Biotechnol. 24, 503–506. doi: 10.5511/plantbiotechnology.24.503
Ojok, J., Omara, P., Opolot, E., Odongo, W., Olum, S., Gijs, D. L., et al. (2019). Iodine agronomic biofortification of cabbage (Brassica oleracea var. capitata) and Cowpea (Vigna unguiculata L.) is effective under farmer field conditions. Agronomy 9:797. doi: 10.3390/agronomy9120797
Pandey, V. P., Awasthi, M., Singh, S., Tiwari, S., and Dwivedi, U. N. (2017). A comprehensive review on function and application of plant peroxidases. Biochem. Anal. Biochem. 6, 1–16. doi: 10.4172/2161-1009.1000308
Park, S. W., Liu, P. P., Forouhar, F., Vlot, A. C., Tong, L., Tietjen, K., et al. (2009). Use of a synthetic salicylic acid analog to investigate the roles of methyl salicylate and its esterases in plant disease resistance. J. Biol. Chem. 284, 7307–7317. doi: 10.1074/jbc.M807968200
Pessoa, J., Sárkány, Z., Ferreira-da-Silva, F., Martins, S., Almeida, M. R., Li, J., et al. (2010). Functional characterization of Arabidopsis thaliana transthyretin-like protein. BMC Plant Biol. 10:30. doi: 10.1186/1471-2229-10-30
Pia̧tkowska, E., Kopeć, A., Bieżanowska-Kopeć, R., Pysz, P., Kapusta-Duch, J., Koronowicz, A., et al. (2016). The impact of carrot enriched in iodine through soil fertilization on iodine concentration and selected biochemical parameters in Wistar rats. PLoS One 11:e0152680. doi: 10.1371/journal.pone.0152680
Pilbeam, D. J., and Drihem, K. (2007). “Vanadium,” in Handbook of Plant Nutrition, eds A. V. Barker and D. J. Pilbeam (Boca Raton, FL: CRC Press), 585–596.
Pless, J. D., Chwirka, J. B., and Krumhansl, J. L. (2007). Iodine sequestration using delafossites and layered hydroxides. Environ. Chem. Lett. 5, 85–89. doi: 10.1007/s10311-006-0084-8
Pn-En 15111 (2008). Foodstuffs – Determination of Trace Elements – Determination of Iodine by ICP-MS (Inductively Coupled Plasma Mass Spectrometry). Warsaw: Polish Committee of Standardization.
Power, D. M., Elias, N. P., Richardson, S. J., Mendes, J., Soares, C. M., and Santos, C. R. A. (2000). Evolution of the thyroid hormone-binding protein, transthyretin. Gen. Comp. Endocr. 119, 241–255. doi: 10.1006/gcen.2000.7520
Redeker, K. R., Manley, S. L., Walser, M., and Cicerone, R. J. (2004). Physiological and biochemical controls over methyl halide emissions from rice plants. Glob. Biogeochem. Cycle 18:GB1007. doi: 10.1029/2003GB002042
Roberts, S. K. (2006). Plasma membrane anion channels in higher plants and their putative functions in roots. New Phytol. 169, 647–666. doi: 10.1111/j.1469-8137.2006.01639.x
Schlegel, M. L., Reiller, P., Mercier-Bion, F., Barré, N., and Moulin, V. (2006). Molecular environment of iodine in naturally iodinated humic substances: insight from X-ray absorption spectroscopy. Geochim. Cosmochim. Acta 70, 5536–5551. doi: 10.1016/j.gca.2006.08.026
Sentíes-Herrera, H. E., Trejo-Téllez, L. I., Volke-Haller, V. H., Cadena-Íñiguez, J., Sánchez-García, P., and Gómez-Merino, F. C. (2018). Iodine, silicon, and vanadium differentially affect growth, flowering, and quality components of stalks in sugarcane. Sugar Tech. 20, 518–533. doi: 10.1007/s12355-017-0572-0
Sgamma, T., Pape, J., Massiah, A., and Jackson, S. (2016). Selection of reference genes for diurnal and developmental time-course real-time PCR expression analyses in lettuce. Plant Methods 12:21. doi: 10.1186/s13007-016-0121-y
Smoleń, S., Baranski, R., Ledwożyw-Smoleń, I., and Skoczylas, Ł, and Sady, W. (2019a). Combined biofortification of carrot with iodine and selenium. Food Chem. 300:125202. doi: 10.1016/j.foodchem.2019.125202
Smoleń, S., Kowalska, I., Czernicka, M., Halka, M., Kȩska, K., and Sady, W. (2016). Iodine and selenium biofortification with additional application of salicylic acid affects yield, selected molecular parameters and chemical composition of lettuce plants (Lactuca sativa L. var. capitata). Front. Plant Sci. 7:1553. doi: 10.3389/fpls.2016.01553
Smoleń, S., Kowalska, I., Halka, M., Ledwożyw-Smoleń, I., Grzanka, M., and Skoczylas, Ł, et al. (2020). Selected aspects of iodate and iodosalicylate metabolism in lettuce including the activity of vanadium dependent haloperoxidases as affected by exogenous vanadium. Agronomy 10, 1–21. doi: 10.3390/agronomy10010001
Smoleń, S., Kowalska, I., Kováčik, P., Halka, M., and Sady, W. (2019b). Biofortification of six varieties of lettuce (Lactuca sativa L.) with iodine and selenium in combination with the application of salicylic acid. Front. Plant Sci. 10:143. doi: 10.3389/fpls.2019.00143
Smoleń, S., Kowalska, I., Kováèik, P., Sady, W., Grzanka, M., and Kutman, U. B. (2019c). Changes in the chemical composition of six lettuce cultivars (Lactuca sativa L.) in response to biofortification with iodine and selenium combined with salicylic acid application. Agronomy 9:660. doi: 10.3390/agronomy9100660
Smoleń, S., Ledwożyw-Smoleń, I., Halka, M., Sady, W., and Kováčik, P. (2017). The absorption of iodine from 5-iodosalicylic acid by hydroponically grown lettuce. Sci. Hortic. 225, 716–725. doi: 10.1016/j.scienta.2017.08.009
Taiz, L., and Zeiger, E. (2010). “Plant physiology,” in The Benjamin Cummings Publishing Company, 3rd Edn (Redwood City, CA: The Benjamin Cummings Publishing Company).
Tieman, D., Zeigler, M., Schmelz, E., Taylor, M. G., Rushing, S., Jones, J. B., et al. (2010). Functional analysis of a tomato salicylic acid methyl transferase and its role in synthesis of the flavor volatile methyl salicylate. Plant J. 62, 113–123. doi: 10.1111/j.1365-313X.2010.04128.x
Tonacchera, M., Dimida, A., De Servi, M., Frigeri, M., Ferrarini, E., De Marco, G., et al. (2013). Iodine fortification of vegetables improves human iodine nutrition: in vivo evidence for a new model of iodine prophylaxis. J. Clin. Endoc. Methods 98, E694–E697. doi: 10.1210/jc.2012-3509
Trumbo, P., Yates, A. A., Schlicker, S., and Poos, M. (2001). Dietary reference intakes: vitamin A, vitamin K, arsenic, boron, chromium, copper, iodine, iron, manganese, molybdenum, nickel, silicon, vanadium, and zinc. J. Acad. Nutr. Diet 101, 294–301.
Vachirapatama, N., Jirakiattiku, Y., Dicinoski, G. W., Townsend, A. T., and Haddad, P. R. (2011). Effect of vanadium on plant growth and its accumulation in plant tissues. Songklanakarin J. Sci. Technol. 33, 255–261.
Verhaeghe, E., Buisson, D., Zekri, E., Leblanc, C., Potin, P., and Ambroise, Y. (2008). A colorimetric assay for steady-state analyses of iodo-and bromoperoxidase activities. Anal. Biochem. 379, 60–65. doi: 10.1016/j.ab.2008.04.041
Waterborg, J. H. (2002). “The lowry method for protein quantitation,” in The Protein Protocols Handbook, ed. J. M. Walker (Totowa, NJ: Humana Press).
Welch, R. M., and Huffman, E. W. (1973). Vanadium and plant nutrition: the growth of lettuce (Lactuca sativa L.) and tomato (Lycopersicon esculentum Mill.) plants in nutrient solutions low in vanadium. Plant Physiol. 52, 183–185. doi: 10.1104/pp.52.2.183
Weng, H. X., Yan, A. L., Hong, C. L., Xie, L. L., Qin, Y. C., and Cheng, C. Q. (2008). Uptake of different species of iodine by water spinach and its effect to growth. Biol. Trace Elem. Res. 124, 184–194. doi: 10.1007/s12011-008-8137-4
Wever, R., and Hemrika, W. (2001). “Vanadium haloperoxidases,” in Handbook of Metalloproteins, eds A. Messerschmidt, R. Huber, T. Poulos, and K. Wieghardt (Chichester: John Wiley & Sons, Ltd).
White, P. J., and Broadley, M. R. (2001). Chloride in soils and its uptake and movement within the plant: a review. Ann. Bot. 88, 967–988. doi: 10.1006/anbo.2001.1540
White, P. J., and Broadley, M. R. (2009). Biofortification of crops with seven mineral elements often lacking in human diets – iron, zinc, copper, calcium, magnesium, selenium and iodine. New Phytol. 182, 49–84.
WHO (2001). Vanadium Pentoxide and Other Inorganic Vanadium Compounds. Geneva: The World Health Organization.
Yamada, H., Sugahara, M., Kosaka, H., Katayama, A., Takahashi, K., and Yonebayashi, K. (1996). Determination of total and water soluble iodine in soil by high performance liquid chromatography. Soil Sci. Plant Nutr. 42, 367–374. doi: 10.1080/00380768.1996.10415107
Yamaguchi, N., Nakano, M., and Tanida, H. (2005). Transformation of Iodine Species in Soil Under Upland Field and Submerged Paddy Field Conditions. Hyogo: Spring-8.
Yoshida, S., Muramatsu, Y., and Uchida, S. (1992). Studies on the sorption of I–(iodide) and IO 3– (iodate) onto andosols. Water Air Soil Pollut. 63, 321–329. doi: 10.1007/BF00475499
Zhang, K., Halitschke, R., Yin, C., Liu, C. J., and Gan, S. S. (2013). Salicylic acid 3-hydroxylase regulates Arabidopsis leaf longevity by mediating salicylic acid catabolism. Proc. Natl. Acad. Sci. U.S.A. 110, 14807–14812. doi: 10.1073/pnas.1302702110
Zhang, L., Mao, X., and Xia, Z. (2012). Effects of sodium metavanadate and germination on the sprouting of chickpeas and its content of vanadium, formononetin and biochanin a in the sprouts. J. Diet Suppl. 9, 34–44. doi: 10.3109/19390211.2011.639858
Zou, C., Du, Y., Rashid, A., Ram, H., Savasli, E., Pieterse, P. J., et al. (2019). Simultaneous biofortification of wheat with zinc, iodine, selenium, and iron through foliar treatment of a micronutrient cocktail in six countries. J. Agr. Food Chem. 67, 8096–8106.
Glossary
2,3,5-triIBeA = 2,3,5-triiodobenzoic acid
2-IBeA = 2-iodobenzoic acid
3,5-diISA = 3,5-diiodosalicylic acid
4-IBeA = 4-iodobenzoic acid
5-ISA = 5-iodosalicylic acid
BeA = benzoic acid
HMT = halide methyltransferase
HPLC-ICP-MS)/MS = high-performance liquid chromatography with inductively coupled plasma mass spectrometry
HPOs = haloperoxidases
HTMT = SAM-dependent halide/thiol methyltransferase
ICP-OES = inductively coupled plasma optical emission spectrometry
I-Tyr = iodotyrosine
LC-MS/MS = liquid chromatography-mass spectrometry
MAPK = mitogen-activated protein kinase
MeSA = volatile ester of methyl salicylic acid
NFT = nutrient film technique
PDTHA = plant-derived thyroid hormone analogs
RDA = recommended dietary allowance
RootSec = secretion produced as a result of root pressure
SA = salicylic acid
SAM = S-adenosyl-l-methionine
SAMT = salicylic acid carboxyl methyltransferase
SAR = Systemic Acquired Resistance
SOM = soil organic matter
T3 = triiodothyronine
T3-Na = sodium salt triiodothyronine
T4 = thyroxine
TF = transfer factor
TMAH = tetramethylammonium hydroxide
TransThy-T3/T4trans = transthyretin, responsible for T3 and T4 transport
vHPO = vanadium-dependent haloperoxidases
per64-like = Peroxidase 64-like
per12-like = Peroxidase 12-like.
samdmt = S-adenosyl-L-methionine-dependent methyltransferase
cipk6 = CBL-interacting serine/threonine-protein kinase 6
msams5 = S-adenosylmethionine synthase 5
Keywords: 5-iodosalicylic acid, 3, 5-diiodosalicylic acid, vHPO, CBL-interacting serine/threonine-protein kinase 6, plant-derived thyroid hormone analogs
Citation: Smoleń S, Czernicka M, Kowalska I, Kȩska K, Halka M, Grzebelus D, Grzanka M, Skoczylas Ł, Pitala J, Koronowicz A and Kováčik P (2021) New Aspects of Uptake and Metabolism of Non-organic and Organic Iodine Compounds—The Role of Vanadium and Plant-Derived Thyroid Hormone Analogs in Lettuce. Front. Plant Sci. 12:653168. doi: 10.3389/fpls.2021.653168
Received: 13 January 2021; Accepted: 19 March 2021;
Published: 16 April 2021.
Edited by:
Francesco Di Gioia, Pennsylvania State University (PSU), United StatesReviewed by:
Luca Incrocci, University of Pisa, ItalyRosario Paolo Mauro, University of Catania, Italy
Copyright © 2021 Smoleń, Czernicka, Kowalska, Kȩska, Halka, Grzebelus, Grzanka, Skoczylas, Pitala, Koronowicz and Kováčik. This is an open-access article distributed under the terms of the Creative Commons Attribution License (CC BY). The use, distribution or reproduction in other forums is permitted, provided the original author(s) and the copyright owner(s) are credited and that the original publication in this journal is cited, in accordance with accepted academic practice. No use, distribution or reproduction is permitted which does not comply with these terms.
*Correspondence: Sylwester Smoleń, c3lsd2VzdGVyLnNtb2xlbkB1cmsuZWR1LnBs
†ORCID: Sylwester Smoleń, orcid.org/0000-0001-8093-3801; Małgorzata Czernicka, orcid.org/0000-0001-5768-5758; Iwona Kowalska, orcid.org/0000-0002-0012-6143; Kinga Kȩska, orcid.org/0000-0002-4329-1499; Maria Halka, orcid.org/0000-0002-3502-8930; Dariusz Grzebelus, orcid.org/0000-0001-6999-913X; Marlena Grzanka, orcid.org/0000-0002-3810-6906; Łukasz Skoczylas, orcid.org/0000-0002-8349-4477; Joanna Pitala, orcid.org/0000-0001-5906-4402; Aneta Koronowicz, orcid.org/0000-0002-1653-2867; Peter Kováčik, orcid.org/0000-0002-0185-3342