- 1Department of Crop Science, College of Agricultural Sciences, São Paulo State University, Botucatu, Brazil
- 2Department of Soil Science and Agricultural Engineering, State University of Ponta Grossa, Ponta Grossa, Brazil
- 3Soils Department, Center of Rural Sciences, Federal University of Santa Maria, Santa Maria, Brazil
- 4Department of Biosystems Engineering, School of Sciences and Engineering, São Paulo State University, Tupã, Brazil
Long-term surface application of lime (L) and/or phosphogypsum (PG) in no-till (NT) systems can improve plant growth and physiological and biochemical processes. Although numerous studies have examined the effects of L on biomass and plant growth, comprehensive evaluations of the effects of this practice on net CO2 assimilation, antioxidant enzyme activities and sucrose synthesis are lacking. Accordingly, this study examined the effects of long-term surface applications of L and PG on soil fertility and the resulting impacts on root growth, plant nutrition, photosynthesis, carbon and antioxidant metabolism, and grain yield (GY) of maize established in a dry winter region. At the study site, the last soil amendment occurred in 2016, with the following four treatments: control (no soil amendments), L (13 Mg ha–1), PG (10 Mg ha–1), and L and PG combined (LPG). The long-term effects of surface liming included reduced soil acidity and increased the availability of P, Ca2+, and Mg2+ throughout the soil profile. Combining L with PG strengthened these effects and also increased SO42–-S. Amendment with LPG increased root development at greater depths and improved maize plant nutrition. These combined effects increased the concentrations of photosynthetic pigments and gas exchange even under low water availability. Furthermore, the activities of Rubisco, sucrose synthase and antioxidative enzymes were improved, thereby reducing oxidative stress. These improvements in the physiological performance of maize plants led to higher GY. Overall, the findings support combining soil amendments as an important strategy to increase soil fertility and ensure crop yield in regions where periods of drought occur during the cultivation cycle.
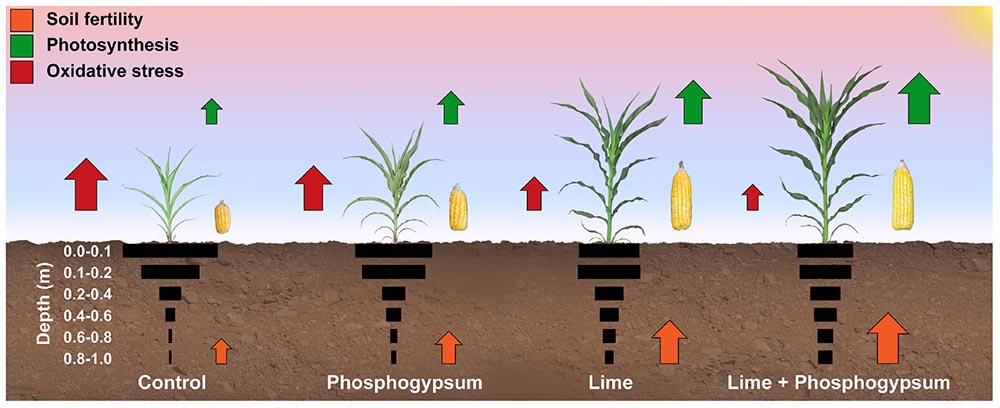
Graphical Abstract. Schematic summary of the main effects of lime and phosphogypsum on soil fertility, root growth and maize physiology.
Introduction
Soil degradation is a serious threat to food security worldwide (Bindraban et al., 2012; FAO, 2015; Gibbs and Salmon, 2015). Global drivers of soil degradation include erosion processes, contamination by heavy metals and other toxic substances, and soil acidification (FAO, 2015). Globally, ∼2.0 billion hectares (ha) of arable soil in the tropics is affected by high acidity (Bian et al., 2013), which is the most important factor limiting crop development and food production capacity in tropical crop systems (Costa and Crusciol, 2016).
Acidic and weathered soils are naturally less fertile due to the limited availability of calcium (Ca2+), magnesium (Mg2+), phosphorus (P), and high aluminum (Al3+) availability, especially in the deepest soil layers (Nora et al., 2017; Costa et al., 2018). Low nutrient availability coupled with high Al3+ levels leads to root growth inhibition and, consequently, decreased water and nutrient uptake, resulting in physiological dysfunction and lower yields (Eekhout et al., 2017; Reis et al., 2018). In addition, tropical regions are subject to periods of drought stress, mainly during the autumn/winter seasons (mid-March to September). The combination of low soil fertility and limited root development with prolonged periods of water limitation contributes strongly to lower yields, especially since the vast majority of the cultivated area is in upland conditions (Carmeis Filho et al., 2017). While advances in plant biotechnology have aided the development of plants that are more resistant to abiotic stresses (Gupta et al., 2020), soil management practices that enable crop development even under unfavorable conditions remain fundamental to food production (Gibbons et al., 2014; Holland et al., 2018).
Several management measures have been developed to mitigate the hazardous effects of soil acidification and improve and sustain soil productivity. In particular, liming has become a widespread practice to raise fertility levels and restore soil quality (Holland et al., 2018; Bossolani et al., 2020a, b, 2021a). Liming aims to increase soil pH, which in turn improves effective cation exchange capacity (ECEC) and base saturation (BS) and reduces Al3+ and manganese (Mn) concentrations (Crusciol et al., 2019; Bossolani et al., 2020a). However, due its low solubility, the effects of lime occur mostly in the top layers, mainly after surface applications under no-till (NT), and more slowly in deep (Caires et al., 2011). Amendment with phosphogypsum (PG) can also improve soil fertility but cannot correct soil acidity (Zoca and Penn, 2017). PG application increases Ca2+ and sulfate (SO42–) levels throughout the soil profile, which reduces Al3+ toxicity due to AlSO4+ ion pair formation and decreased Al3+ saturation (Caires et al., 2011; Zoca and Penn, 2017). These changes enhance root development in deeper soil layers (Costa et al., 2018), increasing crop tolerance to water limitation (Costa and Crusciol, 2016; Nora et al., 2017). Thus, the surface application of PG is an important complementary strategy to overcome the surface liming limitations (Bossolani et al., 2018, 2020b; Crusciol et al., 2019). Several types of abiotic stress can negatively affect the photosynthetic processes of plants and generate oxidative stress, with deleterious effects on key cellular components and functions (Gómez et al., 2019). Crops established in fertile soils tend to have a greater ability to maintain relatively high levels of growth, stomatal conductance, photosynthesis, and antioxidant metabolism under environmental stresses (e.g., drought) (Kleiner et al., 1992). In addition, less stressed plants exhibit delayed senescence, thereby further increasing photosynthetic capacity and enabling high yields (Gómez et al., 2019). In the present study, a detailed investigation was performed to determine (1) how amending soil with lime and PG under NT influences soil chemical properties and induces improvements in maize root development, nutrient uptake, physiology, and yield and (2) the main changes in the soil and crop nutrition that alter carbon and antioxidant metabolism in maize plants under field conditions.
Materials and Methods
Site Description, Experimental Design, and Treatments
The study site was a long-term field experiment (registered on the GLTEN Metadata Portal1) with lime and PG application that has been carried out in Botucatu, southeastern São Paulo State, Brazil (22° 83′ 3″ S, 48° 42′ 64″ W, 765 m above sea level), under NT since 2002. The soil was classified as a sandy clay loam kaolinitic and thermic Typic Haplorthox (USDA, 2014). Prior to the beginning of the experiment in 2002, soil granulometric and chemical properties (0.0–0.2 m depth) were determined according to the methods of Kiehl (1979) and van Raij et al. (2001), respectively, as follows: soil pH (0.01 M CaCl2 suspension): 4.2; soil organic carbon (SOC): 12.2 g kg–1; P (resin): 9.2 mg kg–1; exchangeable K+: 1.2 mmolc kg–1; exchangeable Ca2+: 14 mmolc kg–1; exchangeable Mg2+: 5 mmolc kg–1; total acidity at pH 7 (H + Al): 37 mmolc kg–1, cation exchange capacity (CEC): 57.2 mmolc kg–1; BS: 35%; aluminum saturation (AS): 65%; sand: 540 g kg–1; silt: 110 g kg–1; and clay: 350 g kg–1. In addition, the clay content at 0.2–0.4 m depth was 360 g kg–1. According to Köppen–Geiger’s climate classification (Alvares et al., 2013), the region is a mesothermic type (Cwa) with a humid subtropical climate, dry winters and hot summers. For the period 1956–2019, the average annual maximum and minimum temperatures were 26.1 and 15.3°C, respectively, and the average annual pluvial precipitation was 1,360 mm (Unicamp, 2020).
The experimental design was a randomized complete block with four treatments and four replicates. The treatments were composed by: (i) control (16 years without soil amendments with intensive crop cultivation and fertilizer inputs); (ii) exclusive application of PG; (iii) exclusive application of lime (L); and (iv) combined application of L and PG (LPG). Liming occurred by application of sedimentary dolomitic lime [CaMg(CO3)2]2, with 233 g kg–1 CaO and 175 g kg MgO. The PG contained 280 g kg–1 CaO, 150 g kg–1 S, <1 g kg–1 P, and <1 g kg–1 fluorine (F). The soil amendments were applied in 2002, 2004, 2010, and 2016 based on the results of annual sampling. The criterion for reapplying the treatments was BS ≤50% in the L treatment. The lime rate was calculated to increase the BS in the topsoil (0.0–0.2 m) to 70% according to the criterion proposed by van Raij et al. (1997) and was 2.7 Mg ha–1 in 2002 and 2.0 Mg ha–1 in 2004 and 2010. In October 2016, a new application was necessary, however, the lime rate calculation method used was revised considering the layer of 0.0–0.4 m depth, resulting in 13 Mg ha–1 of L, a rate used to rise the BS to 70%. When lime reapplication was required, PG was also reapplied. In 2002, 2004, and 2010, the rates of PG application were determined according to van Raij et al. (1997) by multiplying the clay content in the 0.2–0.4 m layer by a factor of 6, resulting in a rate of 2.1 Mg ha–1. In 2016, the most recent methodology for PG application in tropical soils proposed by Caires and Guimarães (2018), which is intended to increase Ca2+ saturation in the ECEC to 60% in the 0.2–0.4 m soil layer, was used, resulting in a higher PG rate of 10 Mg ha–1. After the last soil amendments reapplication, a micronutrient based fertilizer was applied over a total area at rates of 3 kg ha–1 B + 1 kg ha–1 Cu + 1 kg ha–1 Mn + 10 kg ha–1 Zn + 0.2 kg ha–1 Mo, in order to avoid unavailability of micronutrients due to the increase in pH by liming.
This study characterizes the long-term residual effects of these treatments in the first and second years after the last soil amendment reapplications in 2016. Several crops were grown during the agricultural years from 2002 to 2018. Previous crops grown and details of the L and PG applications are shown in Supplementary Table 1. Except for the first applications of lime and PG in 2002, when the experiment under NT began, all applications were applied to the soil surface.
Crop Management
Maize (simple hybrid P3707VYH; 60,000 plants ha–1; DuPont Pioneer®, Johnston, IA, United States) was sown in March 2017 and 2018. For both growing seasons, fertilization was performed at sowing with 28 kg ha–1 N, 98 kg ha–1 P2O5, and 56 kg ha–1 K2O. In addition, a topdressing fertilization with 90 kg N ha–1 (NH4)2SO4 (Cantarella et al., 1997) as ammonium sulfate was performed at the V4 phenological stage (Ritchie et al., 1993). The field plots consisted of 14 rows with a length of 9 m spaced 0.45 m apart (56.7 m2). The plots were spaced 8 m from each other to avoid cross-contamination from surface runoff containing fertilizers as a consequence of heavy rainfall or during treatment applications and sowing.
Meteorological Data
Throughout the experimental period, meteorological data (rainfall, solar radiation, wind speed, relative humidity, and maximum and minimum temperatures) were obtained through automatic meteorology station installed close to the experimental area. The evapotranspiration reference (ET0) was calculated using the Penman-Monteith method (Allen et al., 1998). The crop evapotranspiration (ETc) was calculated using the crop coefficient (Kc) for each stage of the crop’s phenological stage (Allen et al., 1998). Using the rainfall data, the climatological water balance was monitored and calculated using electronic spreadsheets (Rolim et al., 1998), following the procedure of Thornthwaite and Mather (1955) to determine the real evapotranspiration (ETr). The climatological water balance of the two growing seasons is shown in Figure 1.
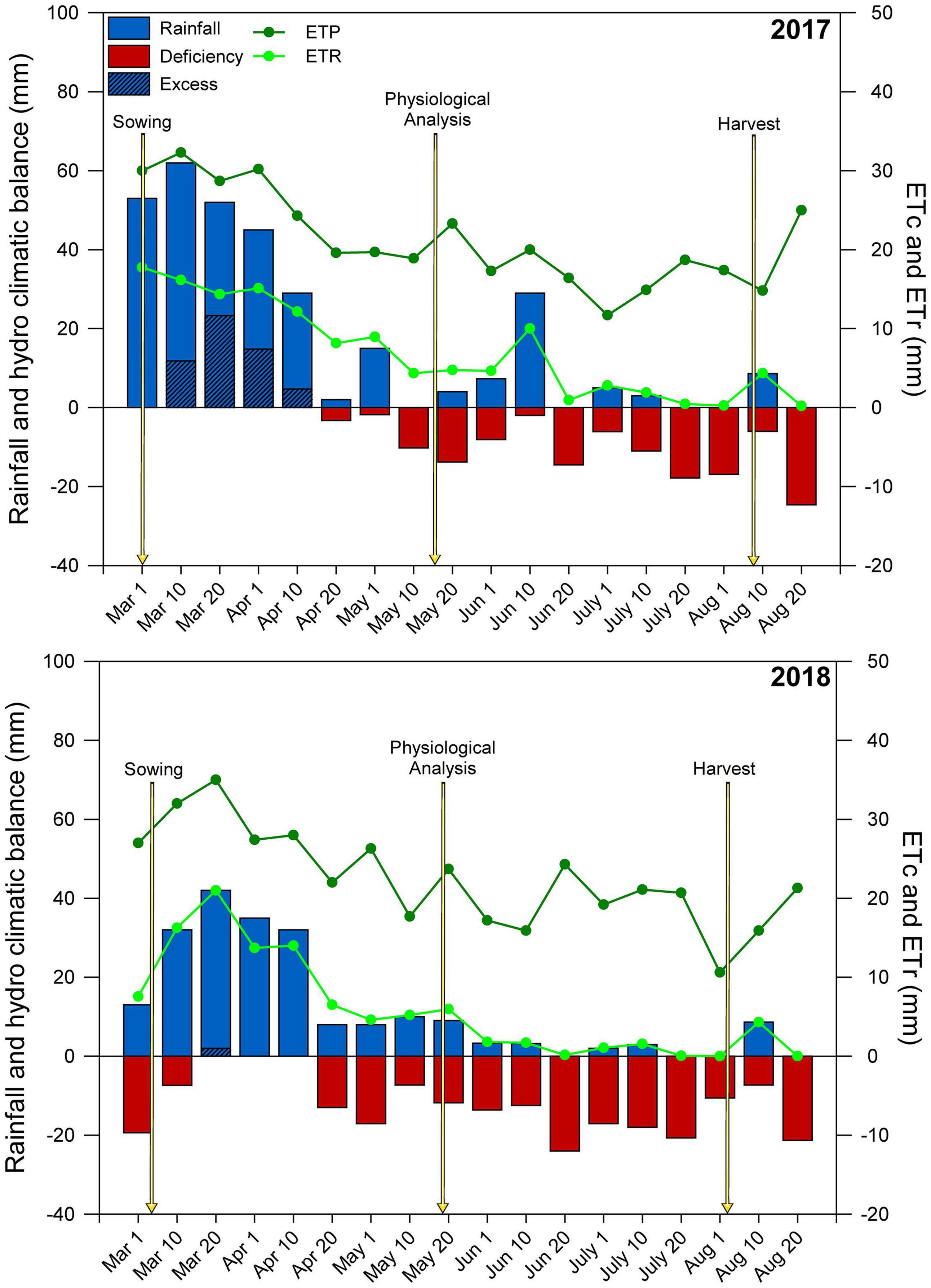
Figure 1. Climatological water balance at Botucatu-SP, Brazil, during the maize crop cycle. ETc, crop evapotranspiration; ETr, real evapotranspiration. The arrows indicate the managing and sampling time.
Soil Chemical Properties Analysis
In September 2018 (24 months after the last reapplication of soil amendments), eight individual soil subsamples were randomly taken at depths of 0.0–0.1, 0.1–0.2, 0.2–0.4, 0.4–0.6, 0.6–0.8, and 0.8–1.0 m in each plot, except for SOC, P, Fe, Mn, Cu, and Zn, that were sampled at a depth of 0.0–0.2 m. The samples were dried, sieved (2 mm mesh) and analyzed according to van Raij et al. (2001). The soil chemical analysis included soil pH (0.01 M CaCl2), SOC (Walkley and Black, 1934), exchangeable cations (K+, Ca2+, and Mg2+) and P extracted by ion-exchange resin and determined by atomic absorption spectrophotometry (AAS) and colorimetric method (van Raij et al., 2001), respectively. Total acidity (H + Al) was estimated using Smith-McLean-Pratt solution (Shoemaker et al., 1961), whereas exchangeable Al3+ was extracted using 1 M KCl and both determined by titration with 0.025 M NaOH solution. SO42–-S content was extracted by 0.01 M calcium phosphate solution (Bardsley and Lancaster, 1960), and determined by a turbidimetric method. Cationic micronutrients (Fe, Mn, Cu, and Zn) were extracted with a solution containing 0.005 M diethylenetriaminepentaacetic acid (DTPA) pH 7.3, 0.1 M triethanolamine (TEA) and 0.01 M CaCl2 and determined by AAS (van Raij et al., 2001).
Root Sampling and Dry Matter Determination
In both growing seasons, at maize full flowering (Ritchie et al., 1993), eight root subsamples (four subsamples from the plant rows and four subsamples from the middle of the inter-rows) were collected randomly from each plot and combined. A galvanized steel probe with a 82-mm-diameter cutting tip was used at depths of 0.0–0.1, 0.1–0.2, 0.2–0.4, 0.4–0.6, 0.6–0.8, and 0.8–1.0 m. Roots were carefully separated from soil and other residues by washing under a flow of swirling water over a 0.5-mm mesh sieve. The samples were dried in a forced-air oven at 60°C for 72 h to measure root dry matter, expressed in g m–3 and subsequently estimated to Mg ha–1 in the 0.0–1.0 m layer. The root dry matter distribution was calculated from the ratio of root dry matter in each layer to total root dry matter.
Leaves Sampling for Crop Nutrition and Physiologic Analysis
Nutritional, physiological, and biochemical analyses occurred on the same diagnostic leaves, when maize plants were at the full flowering (R2 phenological stage) (Ritchie et al., 1993). The diagnostic leaves selected were the fully expanded leaves in the top third of the maize canopy. Leaves sampling occurred immediately after the gas exchange analyses. Nutritional analysis occurred in dried and milled plant material, whereas the leaves for physiological analysis were placed in liquid nitrogen and stored at −80°C until further analysis.
Nutritional Determination
Leaf N was extracted by sulfuric digestion and determined by Kjeldahl method. Phosphorus, K, Ca, Mg, sulfur (S), Fe, Mn, Cu, and Zn extraction occurred by nitroperchloric digestion, and determined by AAS. Both methods are described by AOAC (2016).
Gas Exchange Parameters
Gas exchange assessments consisted of non-destructive analyses of fifteen diagnostic maize leaves using a using a Portable Infrared Gas Analyzer CIRAS-3 Portable Photosynthesis System (PP Systems Inc., Amesbury, MA, United States). The following parameters were determined on the diagnostic leaves of maize: net photosynthetic rate expressed as area (A; μmol CO2 m–2 s–1); stomatal conductance (gs; mol H2O m–2 s–1); internal CO2 concentration in the substomatal chamber (ic; mmol CO2 mol–1 air); leaf transpiration (E; mmol H2O m–2 s–1); and water use efficiency [WUE; μmol CO2 (mmol H2O)–1], calculated by the A/E ratio. Readings began after the air temperature in the chamber was adjusted to 28°C, with 380 ppm CO2 and 1,000 μmol m–2 s–1 of photosynthetically active radiation (PAR) supplied by LED lamps. The measurements were performed between 8:00 and 10:00 a.m. The minimum equilibration time before performing the reading was 3 min.
Photosynthetic Pigments
To determine photosynthetic pigments (chlorophyll a, b, total chlorophyll, and total carotenoids), five discs were cut between the edge and central rib of maize leaves using a paper punch (0.5 cm in diameter). The discs were stored for 24 h in capped glass vials wrapped in aluminum foil and containing 2 mL of N, N-dimethylformamide (DMF) according to the methodology proposed by Lichtenthaler (1987). The pigment concentrations were quantified in a spectrophotometer at wavelengths of 664, 647, and 480 nm for chlorophyll a, b and carotenoids, respectively. The absorbance was read after mixing 1 mL of the extract with 1 mL of distilled water. The calculations for the pigment concentrations were in accordance with the methods proposed by Wellburn (1994).
Ribulose-1,5-Bisphosphate Carboxylase/Oxygenase Activity (Rubisco, EC 4.1.1.39)
Total Rubisco activity (determined only in the second growing season) was measured according to the method described by Reid et al. (1997). Frozen plant material (0.3 g) was ground with a mortar and pestle under liquid nitrogen and suspended in extraction buffer containing 1.5 mL of 58 mM potassium phosphate and 1 mM ethylenediaminetetraacetic acid (EDTA). The homogenized material was centrifuged at 14,000 rpm for 25 min at 4°C, and the supernatant was stored at 4°C (adapted from Sage et al., 1988; Reid et al., 1997). The Rubisco incubation buffer contained 100 mM bicine-NaOH pH 8.0, 25 mM potassium bicarbonate (KHCO3), 20 mM magnesium chloride (MgCl2), 3.5 mM ATP, 5 mM phosphocreatine, 0.25 mM NADH, 80 nkat glyceraldehyde-3-phosphate dehydrogenase, 80 nkat 3-phosphoglyceric phosphokinase, and 80 nkat creatine phosphokinase. A 70-μL aliquot of the supernatant was incubated with 900 μl of the incubation buffer at 30°C for 5 min in the absence of ribulose-1,5-bisphosphate (RuBP) to enable carbamylation of Rubisco. NADP oxidation was initiated by adding 30 μL of 16.66 mM RuBP directly into the cuvette. Readings were obtained in a spectrophotometer at a wavelength of 340 nm. Rubisco activity was calculated from the difference in the absorbance readings at 0 and 1 min (without removing the cuvette from the spectrophotometer) and expressed in μmol min–1 mg protein–1.
Sucrose Synthase Activity (EC 2.4.1.13)
To extract sucrose synthase (Susy) (determined only in the second growing season), 0.5 g of frozen plant material was ground with a mortar and pestle under liquid nitrogen and suspended in extraction buffer containing 50 mM HEPES buffer pH 7.0, 2 mM MgCl2, 2 mM dithiothreitol (DTT) and 1 mM EDTA (Dejardin et al., 1997). The homogenized material was centrifuged at 14,000 rpm for 20 min at 4°C, and the supernatant was stored at 4°C. The incubation buffer consisted of 0.1 M MES buffer pH 6.0, 5 mM MgCl2, 0.3 M sucrose and 5 mM uridine 5′-trihydrogen diphosphate (UDP). A 0.5-mL aliquot of the supernatant was incubated with 3.5 mL of incubation buffer at 37°C for 30 min, and the reaction was terminated by adding 100 μL of potassium hydroxide (30% KOH; w/v) and heating at 100°C for 5 min. Readings were obtained in a spectrophotometer at a wavelength of 540 nm, and the results were expressed as μmol sucrose g–1 fresh weight (FW) h–1.
Sucrose Concentration
The sucrose concentration was determined from 1 g of frozen plant material extracted in 10 mL of MCW solution [60% methanol, 25% chloroform, and 15% water; (w/v)] after maceration by a mortar and pestle. The homogenized material was centrifuged at 8,000 rpm for 10 min at 4°C. An aliquot of 4 mL of the supernatant was removed and mixed with 1 mL of chloroform and 1.5 mL of distilled water in another tube. After separation of the phases, the sucrose content was determined in the aqueous phase as described by Bieleski and Turner (1966). In brief, a 50-μL aliquot of the aqueous phase was mixed with 500 μL of 30% KOH (w/v) and 2 mL of H2SO4. After homogenization by a vortex mixer, the tube was heated at 100°C for 10 min. After cooling, readings were obtained in a spectrophotometer at a wavelength of 490 nm. The sucrose concentration was determined by reference to a standard sucrose curve and expressed as mg g–1 FW.
Hydrogen Peroxide and Lipid Peroxidation
The hydrogen peroxide (H2O2) concentration was determined according to the methodology proposed by Alexieva et al. (2001). Frozen plant material (0.4 g) was ground with a mortar and pestle under liquid nitrogen, and 4 mL of 0.1% trichloroacetic acid (TCA) was added as extraction buffer. The homogenized material was centrifuged at 12,000 rpm for 15 min at 4°C. An aliquot of 200 μL of supernatant was mixed with 200 μL of 100 mM potassium phosphate buffer (pH 7.5) and 800 μL of 1 M potassium iodide and incubated at 1°C for 1 h. After warming to room temperature, readings were obtained in a spectrophotometer at a wavelength of 390 nm. The leaf concentration of H2O2 was determined by reference to a standard curve and expressed as μmol g–1 FW. Lipid peroxidation, or malondialdehyde (MDA), was measured according to the methodology proposed by Heath and Packer (1968). The extraction was carried out using 0.4 g of frozen plant material ground with a mortar and pestle under liquid nitrogen and suspended in 4 mL of 0.1% (w/v) TCA + 20% (w/v) polyvinylpolypyrrolidone (PVPP). The homogenized material was centrifuged at 10,000 rpm for 15 min at 4°C. A 250-μL aliquot of supernatant was mixed with 1 mL of 20% TCA + 0.5% thiobarbituric acid (w/v) solution to start the reaction. The reactions were heated at 95°C for 30 min and then placed on ice for 10 min. The reactions were centrifuged for 10 min at 10,000 rpm, and readings of the supernatant were obtained in a spectrophotometer at wavelengths of 535 and 600 nm. The results were expressed as nmol MDA g–1 FW.
Soluble Protein
Proteins were extracted from 1.5 g of frozen plant material ground with a mortar and pestle under liquid nitrogen and suspended in 20% PVPP and extraction buffer containing 100 mM potassium phosphate pH 7.5, 1 mM EDTA, and 1 mM DDT. The homogenized material was centrifuged at 10,000 rpm for 25 min at 4°C, and the supernatant was stored in Eppendorf tubes in a freezer at −80°C. The soluble protein concentration was determined using BSA (bovine serum albumin) as a standard according to the method proposed by Bradford (1976). Aliquots of 100 μL of the protein extract were mixed with 5 mL of Bradford reagent and analyzed in a spectrophotometer at a wavelength of 595 nm. The results and protein extract were used to determinate the activities of superoxide dismutase (SOD), catalase (CAT), ascorbate peroxidase (APX), and glutathione reductase (GR).
Superoxide Dismutase (EC:1.15.1.1)
Superoxide dismutase activity was determined according to Giannopolitis and Ries (1977). The reaction was conducted in a reaction chamber under illumination with a 15-W fluorescent lightbulb at 25°C after adding 2 mL of 50 mM potassium phosphate buffer pH 7.8, 250 μL of 13 mM methionine, 200 μL of 75 mM nitroblue tetrazolium (NBT), 200 μL of 0.1 mM EDTA and 250 μL of 2 μM riboflavin to 50 μL of protein extract. The tubes were vortexed and placed inside the chamber (total absence of ambient light) for 15 min top permit the formation of the blue formazan compound by photoreaction of NBT. A control was prepared for each sample using the same conditions, except that the tubes were covered with aluminum foil to prevent light exposure. After 15 min, the reactions were vortexed, and readings were obtained in a spectrophotometer at a wavelength of 560 nm. The results were expressed as U (unit) SOD mg–1 protein.
Catalase (1.11.1.6)
Catalase activity was determined by monitoring the degradation of H2O2 according to the methodology proposed by Azevedo et al. (1998). A 25-μL aliquot of protein extract was added to a mixture containing 1 mL of 100 mM potassium phosphate buffer pH 7.5 and 2 μL of 30% H2O2 (v/v) in a test tube and mixed quickly by vortexing. Enzyme activity was determined by the decomposition of H2O2 during a 2-min interval as measured using a spectrophotometer at a wavelength of 240 nm. The results were expressed as μmol min–1 mg–1 protein.
Ascorbate Peroxidase (EC:1.11.1.11)
Ascorbate peroxidase activity was determined according to Gratão et al. (2008). A 100-μL aliquot of protein extract was added to 600 μL of 80 mM potassium phosphate buffer pH 7.0, 100 μL of 5 mM ascorbate and 100 μL of 1 mM EDTA and homogenized by vortexing. The mixture was incubated for 5 min at 30°C in the dark. A 100-μL aliquot of 1 mM H2O2 was then added, and readings were obtained immediately in a spectrophotometer at a wavelength of 290 nm for 2 min. The results were expressed as μmol min–1 mg–1 protein.
Glutathione Reductase (EC 1.6.4.2)
Glutathione reductase activity was determined according to the methodology described by Gomes-Junior et al. (2006). One milliliter of 100 mM potassium phosphate buffer pH 7.5, 500 μL of 1 mM nitrobenzoic acid (DTNB), 100 μL of 1 mM oxidized glutathione (GSSG) and 100 μL of 0.1 mM NADPH were added to an Eppendorf tube and mixed thoroughly. A 50-μL aliquot of the supernatant was added and vortexed. The solution was then transferred to a cuvette, and the absorbance at 412 nm was immediately recorded for 2 min. The results were expressed as μmol min–1 mg–1 protein.
Shoot Dry Matter and Grain Yield of Maize
Maize shoot dry matter (SDM) and grain yield (GY) were evaluated at the harvest stage based on the relationship between the masses (shoot and grain) obtained in the interior (5.4 m2) of each plot and their respective water contents (0 and 130 g kg–1 of water for SDM and GY, respectively).
Statistical Analysis
Means were subjected to tests of homoscedasticity followed by the Anderson-Darling test of normality (Nelson, 1998). To evaluate homogeneity, Levene’s test in the Minitab statistical program was used. Subsequently, the means were subjected to analysis of individual variance (ANOVA) by the F-test (p ≤ 0.05) and, when significant, analyzed using the modified t-test [Fisher’s protected least significant difference (LSD) at p ≤ 0.05]. Redundancy analysis (RDA) was performed to determine the correlations among soil fertility × crop nutrition (average of two growing seasons), soil fertility × crop physiology (average of two growing seasons), and crop nutrition × crop physiology. The Monte Carlo permutation test was applied with 999 random permutations to verify the significance of soil chemical properties, crop nutrition, and for physiological responses. One-way PERMANOVA (Anderson, 2005) was used to group treatments by similarity. Heatmaps were constructed by calculating the Pearson’s correlation coefficients (p ≤ 0.05), and only significant correlations are shown.
Results
Weather Conditions
In the first and second growing seasons, maize received 315 and 210 mm of pluvial precipitation, respectively (Figure 1). From early-March (maize sowing) until early July (maize physiological maturity) of each year, only small amounts of rain occurred, resulting in a negative hydric balance.
Soil Fertility and Root Development
Twenty-four months after the last reapplication of soil amendments, significant variations (p < 0.01; Supplementary Table 2) in soil chemical properties among the treatments were observed in all layers of the soil profile (Figure 2). Surface amendment with L (regardless of PG addition) increased the soil pH in all soil layers (0.0–1.0 m); additionally, acidity neutralization was not improved by PG application compared with the control (Figure 2A). By contrast, significant increases in Ca2+ availability occurred in all soil layers when soil was amended with L and, in particular, LPG (Figure 2B). PG increased the levels of Ca2+ in topsoil in relation to the control, resulting in high BS values (Figure 2D), but BS remained lower in the PG treatment than in the L and LPG treatments. Mg2+ availability did not differ between L and LPG, but was higher in both of these treatments than in the control and PG treatments (Figure 2C). BS was slightly improved by LPG compared with L alone, mainly in deeper soil layers (0.1–0.8 m) (Figure 2D).
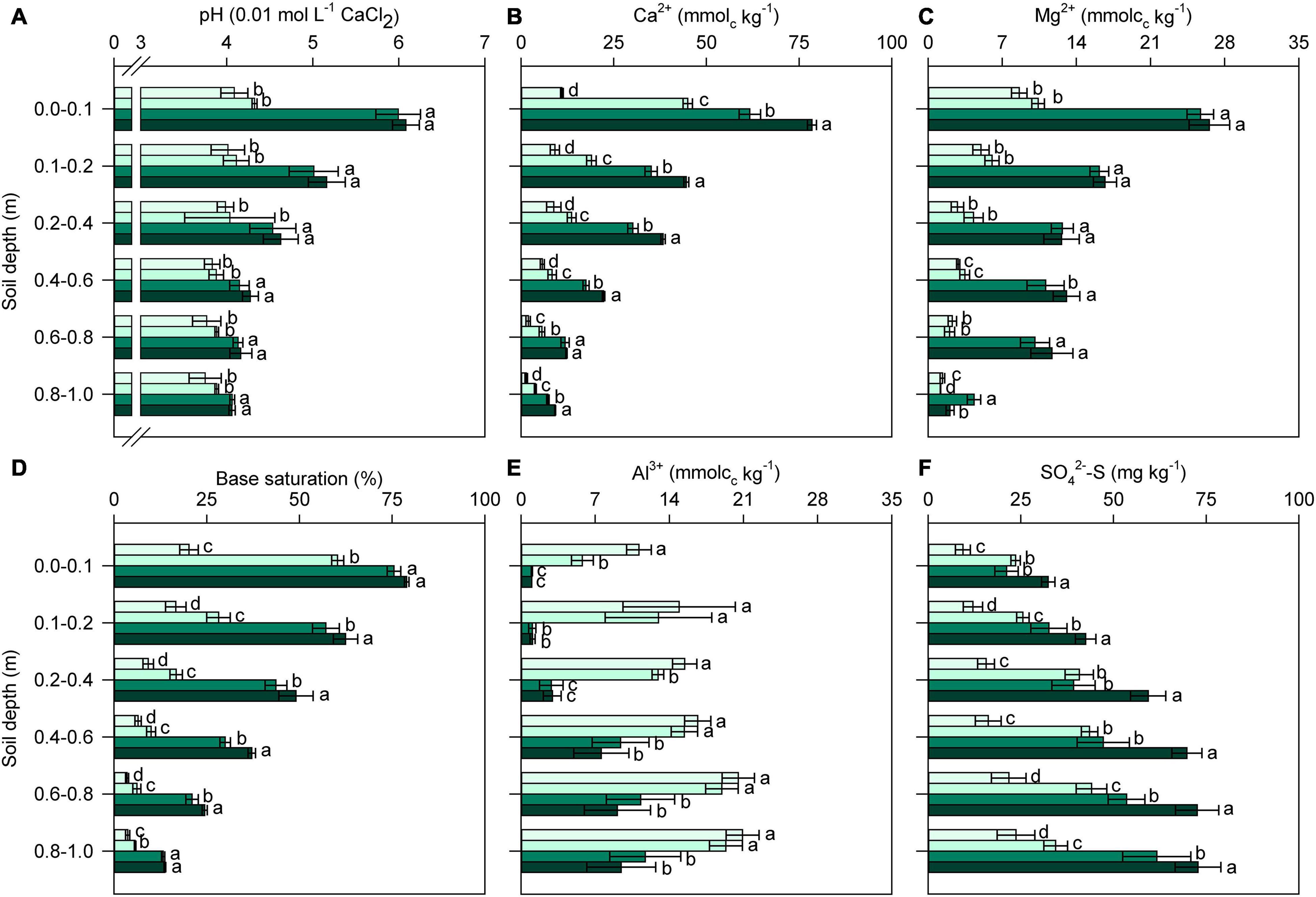
Figure 2. Changes in soil pH (A), exchangeable calcium (Ca2+) (B) and magnesium (Mg2+) (C), base saturation (BS) (D), exchangeable aluminum (Al3+) (E), and sulfate (SO42–-S) (F) in the soil profile as affected by surface-applied lime (L), phosphogypsum (PG), and lime + phosphogypsum (LPG) treatments. Different lower-case letters for each soil depth indicate significant differences between treatments by Student’s t-test at p ≤ 0.05. Error bars express the standard error of the mean (n = 4).
Aluminum availability was strongly reduced by the application of L and LPG, mainly in the uppermost layers (0.0–0.4 m depth); in soil depths below 0.4 m, there was no difference in aluminum availability between L and LPG (Figure 2E). In addition, the application of PG alone did not reduce levels of exchangeable Al3+ in layers below 0.4 m compared with the control treatment. The SO42–-S concentration was higher throughout the soil profile when LPG was applied; in the treatments with L or PG alone, the SO42–-S concentrations were similar and higher than that in the control treatment (Figure 2F). SOC, P, Fe, Mn, and Zn contents in the topsoil (0.0–0.2 m) were significantly altered (p < 0.01; Supplementary Table 3) by soil amendments (Figure 3). In general, L-amended soils (regardless of PG addition) provided the highest levels of SOC (Figure 3A) and P (Figure 3B) and the lowest concentration of Fe, Mn, Cu, and Zn (Figure 3).
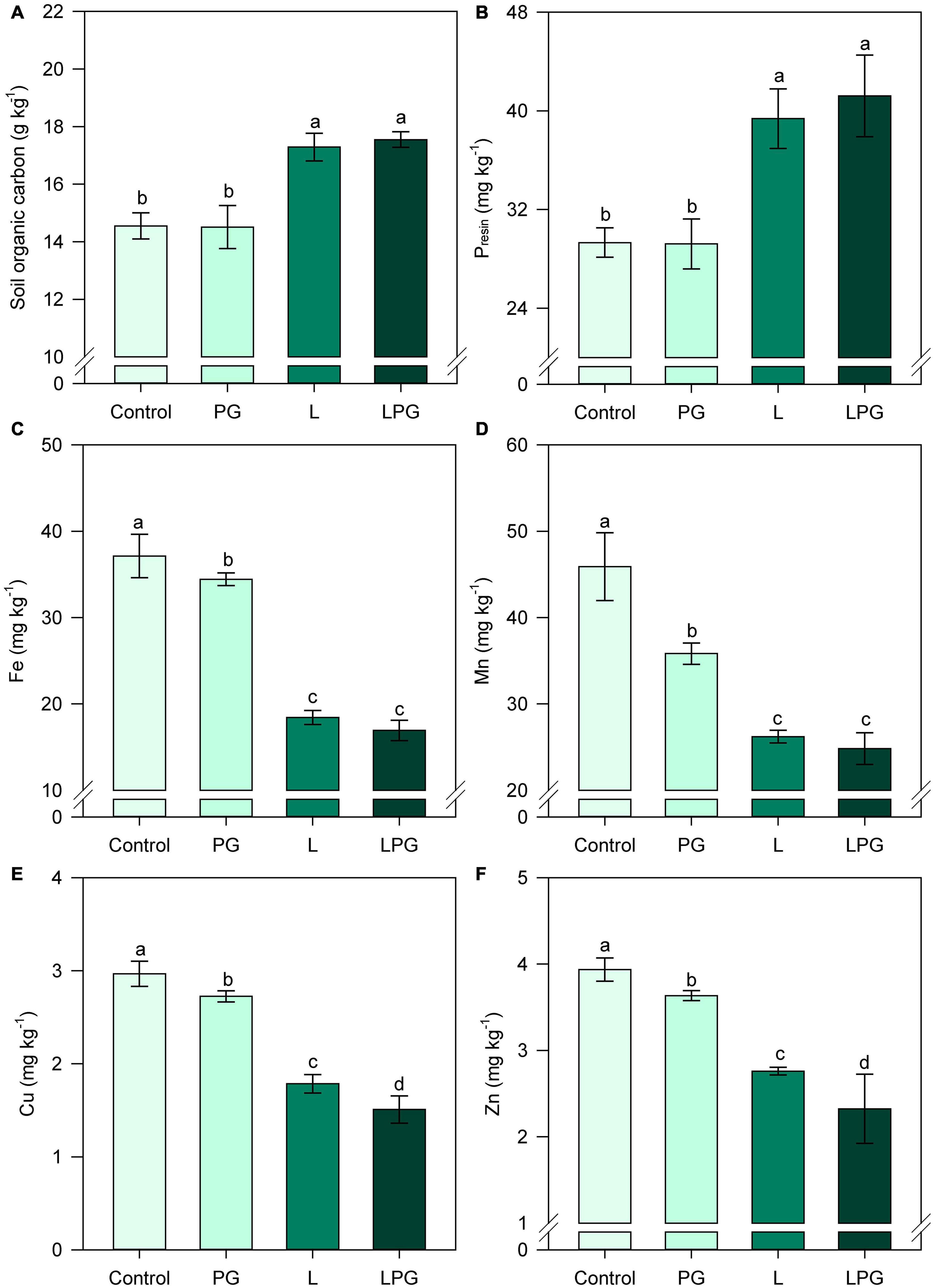
Figure 3. Changes in soil organic carbon (SOC) (A), phosphorus (P) (B), iron (Fe) (C), manganese (Mn) (D), copper (Cu) (E), and zinc (Zn) (F) at 0.0–0.2 m depth as affected by surface-applied lime (L), phosphogypsum (PG), and lime + phosphogypsum (LPG) treatments. Different lower-case letters for each soil depth indicate significant differences between treatments by Student’s t-test at p ≤ 0.05. Error bars express the standard error of the mean (n = 4).
Maize root development composed by dry matter production and distribution, changed (p < 0.01; Supplementary Table 4) by the long-term surface application of soil amendments (Figure 4). In both growing seasons, root dry matter increased in L-amended soil, but combining L to PG (LPG treatments), the effects were enhanced, especially at deeper layers (below 0.4 m depth) (Figures 4A,C). Between treatments became more evident as the soil depth increased. Regardless of growing season, the root system distribution in the soil profile revealed that the density of roots was highest at 0.0–0.2 m soil layer in the control and PG treatments, with a lower proportion of roots in layers deeper than 0.2 m. In L-amended and, in particular, LPG-amended soil, the root distribution was more uniform throughout the soil profile (Figures 4B,D). Treatment with PG alone improved root development and distribution compared with the control treatment, but the effects of PG alone were smaller than those of the L and LPG treatments. LPG-treatment provided the highest distribution of maize roots in the deepest soil depth.
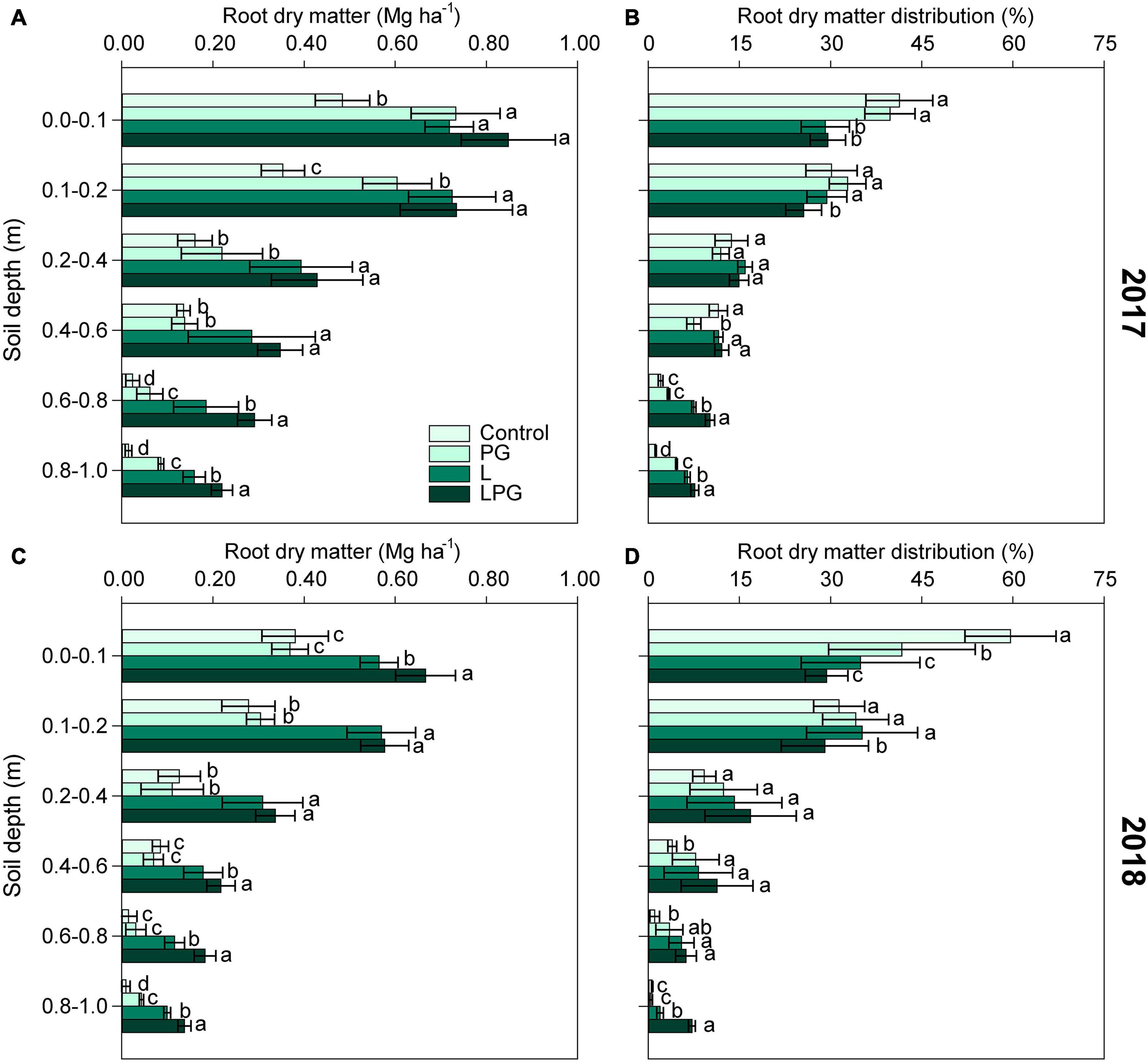
Figure 4. Root dry matter (A,C) and root dry matter distribution (B,D) in the soil profile as affected by surface-applied lime (L), phosphogypsum (PG), and lime + phosphogypsum (LPG) treatments. Different lower-case letters for each soil depth indicate significant differences between treatments for each growing season by Student’s t-test at p ≤ 0.05. Error bars express the standard error of the mean (n = 4).
Plant Nutrition
Leaf macronutrient concentrations increased (p < 0.05; Supplementary Table 5) in response to L and, in particular, LPG in both growing seasons (Table 1). With the exception of K which increased only in 2018, higher leaf concentration of N, P, Ca, Mg, and S occurred in both growing seasons in these treatments compared with the control and PG treatments. By contrast, the concentrations of Fe, Mn, and Zn reduced in treatments L-based (L and LPG), as occurred according to the soil chemical analysis (Figure 3). Among the micronutrients, leaf Cu concentration presented the smallest variation among micronutrients, showing changes only in 2018, where the control treatment provided higher concentrations than the other treatments.
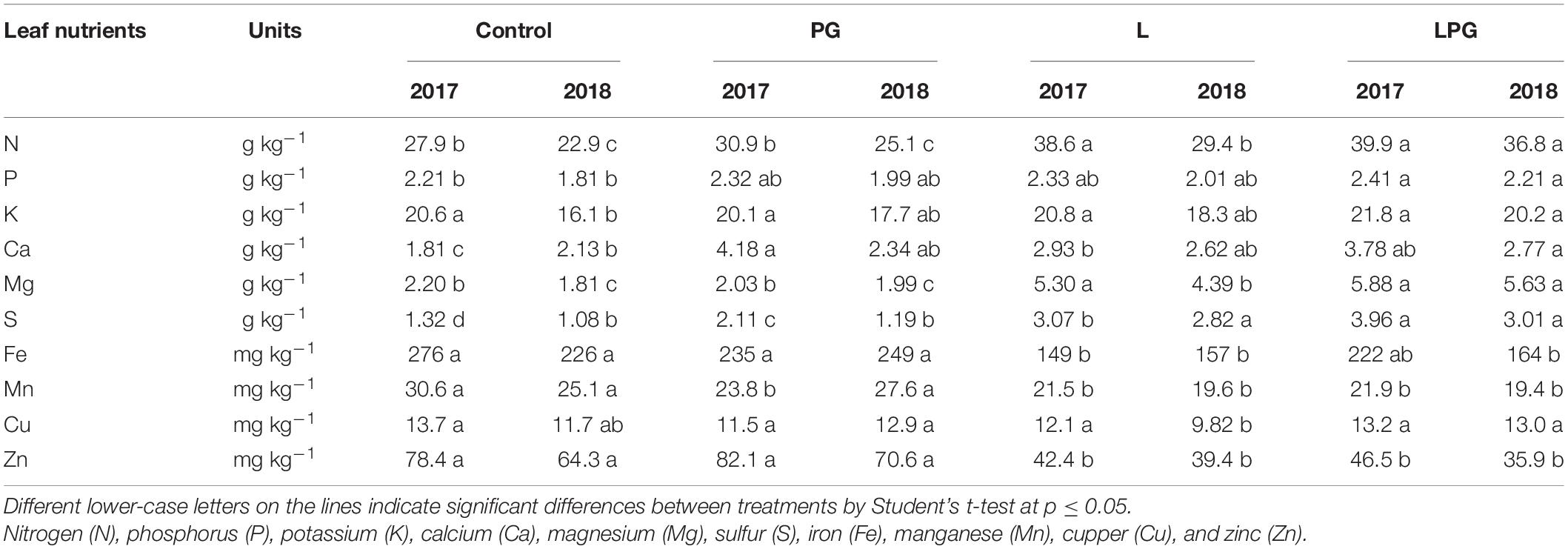
Table 1. Influence of surface-applied lime (L), phosphogypsum (PG), and lime + phosphogypsum (LPG) on nutrient (N, P, K, Ca, Mg, S, Fe, Mn, Cu, and Zn) concentrations in the leaves of maize cultivated in two growing seasons in a long-term no-till system.
Photosynthetic Pigments and Gas Exchange Measurements
Regardless of growing season, the application of L and particularly LPG increased (p < 0.01; Supplementary Table 6) the concentrations of chlorophylls (a, b, and total) and carotenoids compared with the control and PG treatments (Figure 5). On average, considering all photosynthetic pigments, LPG-amended soil provided maize plants with 137% more pigments than the control treatment, followed by 90% more in the treatment with L and 15% with PG. Leaf gas exchange of maize in both growing seasons improved by applying LPG, followed by L (Figure 6) compared with control and PG. In LPG-amended soil, maize plants presented the largest A rate compared with control treatments (LPG = 70%; L = 57%), as well as gs rates increased by 478% in LPG, by 380% in L, and by 76% in PG treatments (Figures 6A,B). Lowest rates of ic (Figure 6C) and E (Supplementary Figure 1) also occurred in these treatments (L and LPG), although differences did not occur between them. As a consequence of improvements in A and E rates, higher WUE occurred maize cultivated in L (250%) and LPG-amended soils (278%), both compared with control (Figure 6D).
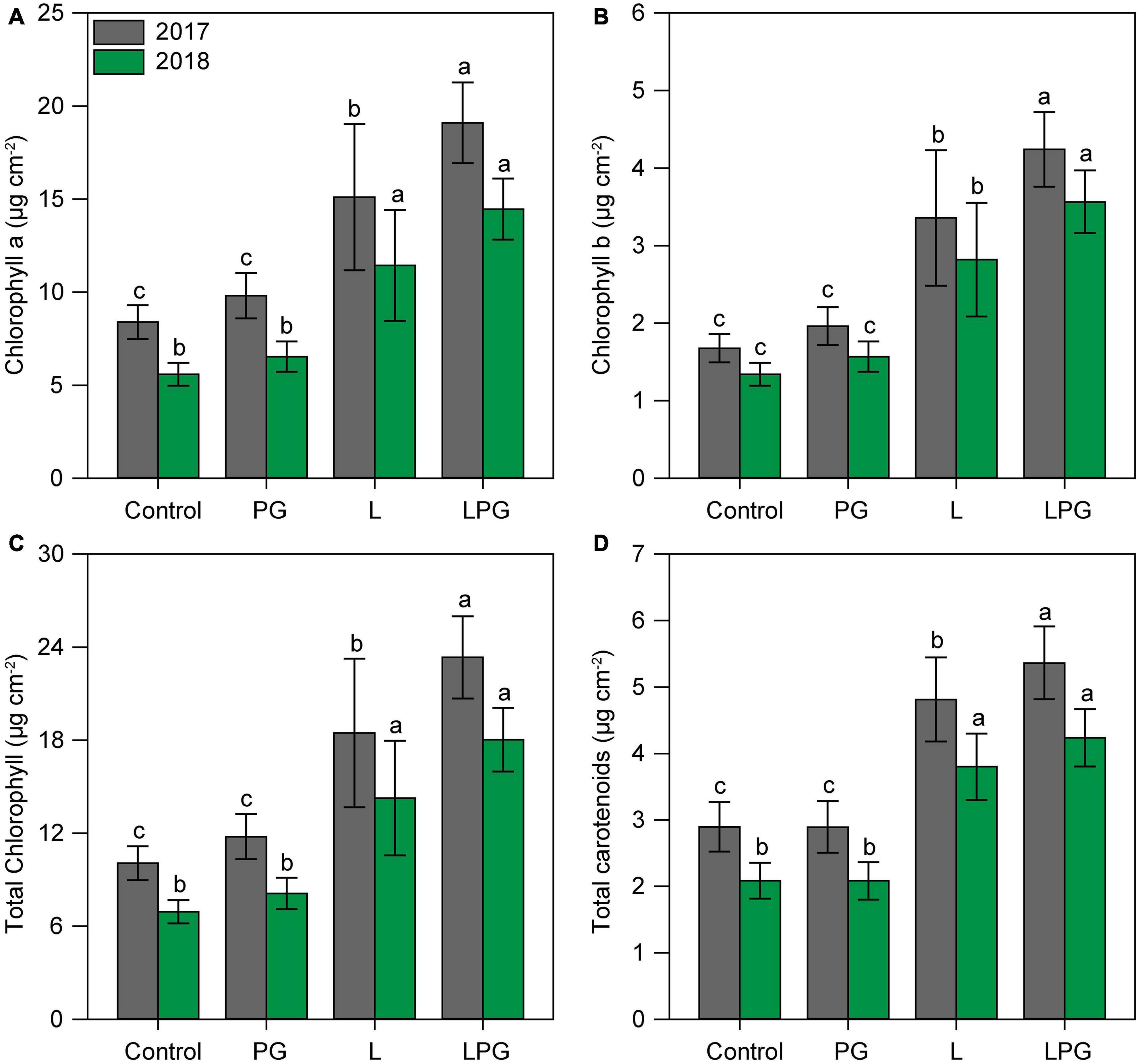
Figure 5. Chlorophyll a (A), chlorophyll b (B), total chlorophyll (C), and carotenoids (D) contents in maize leaves as affected by surface-applied lime (L), phosphogypsum (PG), and lime + phosphogypsum (LPG) treatments. Different lower-case letters indicate significant differences between treatments for each growing season by Student’s t-test at p ≤ 0.05. Error bars express the standard error of the mean (n = 4).
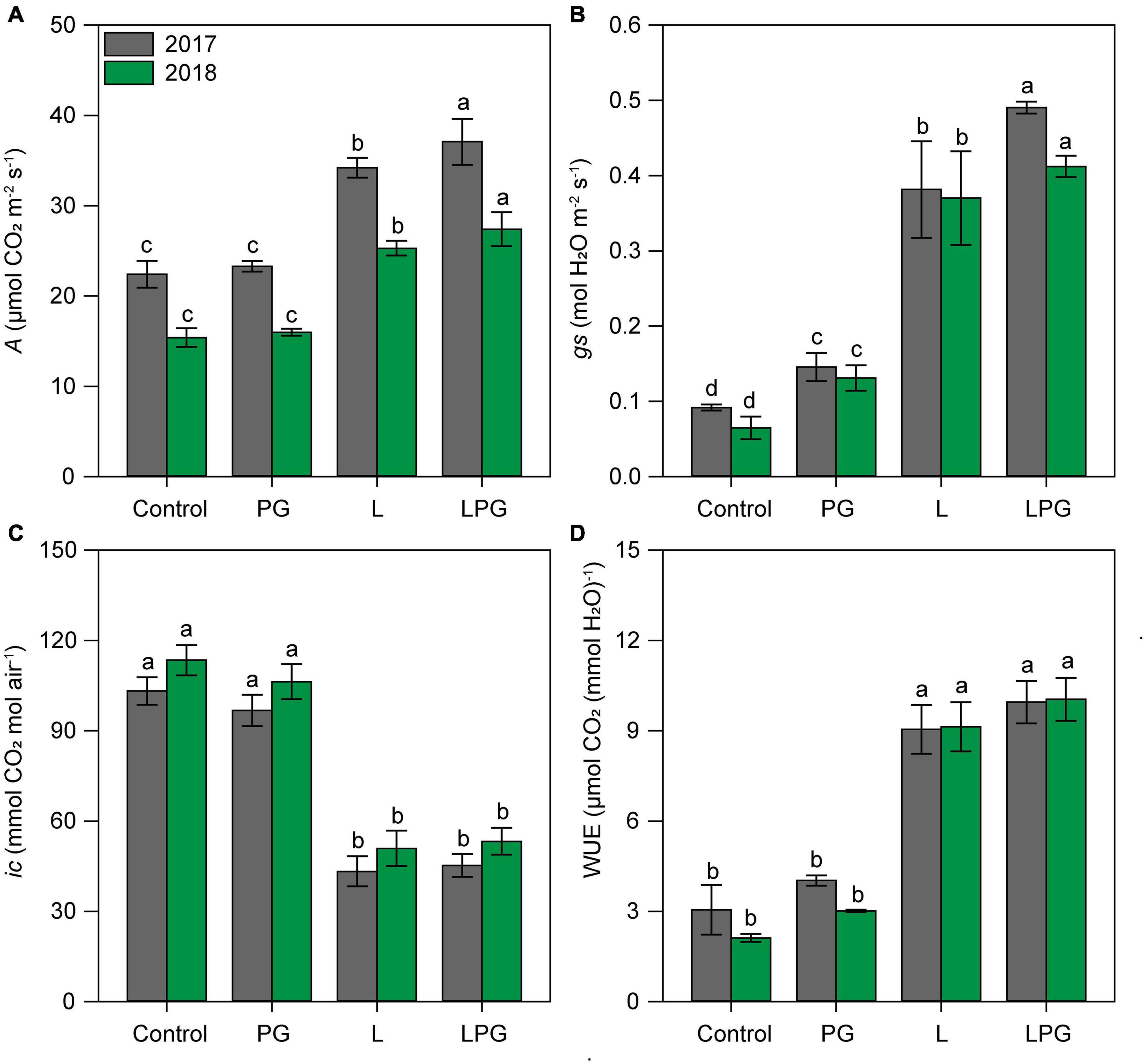
Figure 6. Net photosynthesis rate–A (A), stomatal conductance–gs (B), internal CO2 concentration–ic (C), and water use efficiency–WUE (D) in maize leaves as affected by surface-applied lime (L), phosphogypsum (PG), and lime + phosphogypsum (LPG) treatments. Different lower-case letters indicate significant differences between treatments for each growing season by Student’s t-test at p ≤ 0.05. Error bars express the standard error of the mean (n = 4).
Carbon Metabolism
The increased pigment concentrations in response to soil amendments were positively reflected in Rubisco activity (Figure 7A). Rubisco activity was highest (p < 0.01; Supplementary Table 6) in maize cultivated in LPG-amended soil (67% higher than control), followed by the L treatment (55% higher than control). Figure 7 The sucrose concentration was highest (p < 0.01; Supplementary Table 6) in the control treatment in both growing seasons (Figure 7B). In general, sucrose concentration in maize leaves decreased by 24% from control to the L and LPG treatments. The pattern of Susy activity was similar to that of Rubisco activity; Susy activity was highest (p < 0.01; Supplementary Table 6) in maize plants in the LPG-amended soil (120% higher than control), followed by the application of L alone (90% higher than control) (Figure 7C).
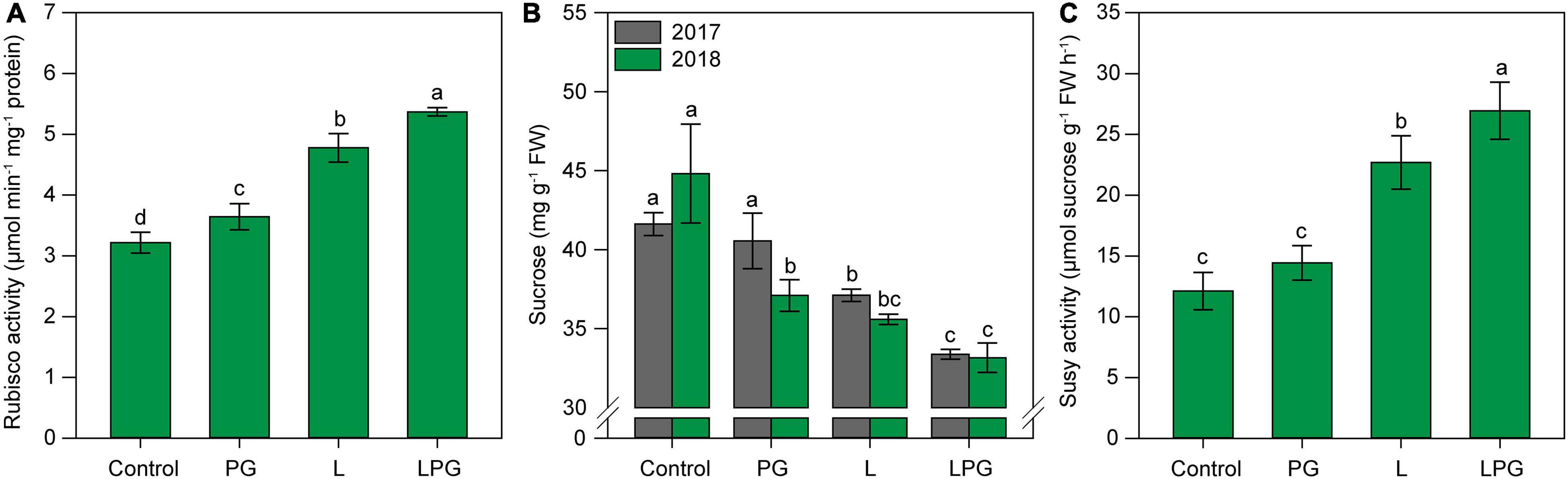
Figure 7. Rubisco activity (A), sucrose concentration (B), and Susy activity (C) in maize leaves as affected by surface-applied lime (L), phosphogypsum (PG), and lime + phosphogypsum (LPG) treatments. Different lower-case letters indicate significant differences between treatments for each growing season by Student’s t-test at p ≤ 0.05. Error bars express the standard error of the mean (n = 4).
Lipid Peroxidation and Antioxidant Metabolism
Hydrogen peroxide (H2O2) and malondialdehyde (MDA) concentrations in maize leaves were highest (p < 0.01; Supplementary Table 6) in the control treatment (unamended soil) (Figures 8A,B). Regardless of growing season, the concentrations of H2O2 and MDA were reduced (p < 0.01; Supplementary Table 6) by all of the soil amendments compared with the control; however, these reductions were greatest in the LPG treatment (H2O2 = 66%; MDA = 54%), followed by the treatments with L (H2O2 = 53%; MDA = 13.7%) and PG (H2O2 = 24.6%; MDA = 6.4%) alone.
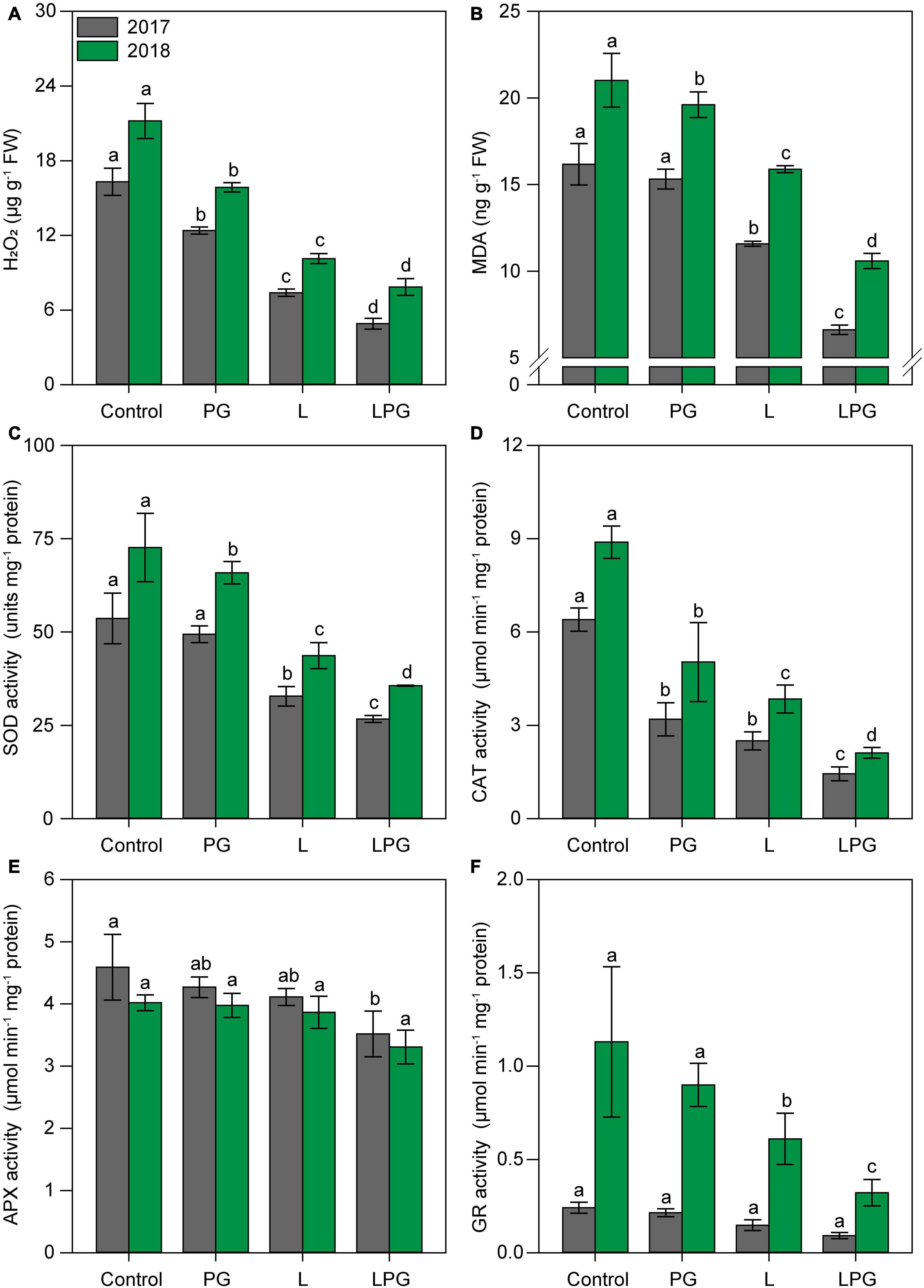
Figure 8. Oxidative stress [hydrogen peroxide (H2O2) (A) and malondialdehyde (MDA) (B) concentrations] and antioxidant enzyme activities [superoxide dismutase–SOD (C), catalase–CAT (D), ascorbate peroxidase–APX (E), and glutathione reductase–GR (F)] in maize leaves as affected by surface-applied lime (L), phosphogypsum (PG), and lime + phosphogypsum (LPG) treatments. Different lower-case letters indicate significant differences between treatments for each growing season by Student’s t-test at p ≤ 0.05. Error bars express the standard error of the mean (n = 4).
Antioxidant enzyme activities were highest (p < 0.01; Supplementary Table 6) in the leaves of maize in the control treatment or the treatment with PG alone (Figures 8C–F) in both growing seasons. On the other hand, enzymatic activity, i.e., SOD (Figure 8C), CAT (Figure 8D), APX (Figure 8E), and GR (Figure 8F) was lower (p < 0.01; Supplementary Table 6) in L-amended soil, especially in the LPG treatment. In general, considering both growing seasons, the antioxidant enzyme activities in maize cultivated in LPG-amended soil reduced by 51% for SOD, by 77% for CAT, by 21% for APX, and by 70% for GR, when compared with control treatment.
Maize Shoot Dry Matter Production and Grain Yield
Figure 9 considering the average between the two growing seasons, SDM and GY were highest (p < 0.01; Supplementary Table 6) in the LPG treatment (SDM = 92%; GY = 260%), compared with control, followed by the L (SDM = 70%; GY = 200%), and PG (SDM = 1.35%; GY = 31%) (Figures 9A,B). A clear trend of LPG > L > PG > control was also observed for the development of maize plants at 50 days after emergence and ears obtained at harvest in the second growing season (Figure 9C), although some similarities were found between the L and LPG treatments and between the control and PG treatments.
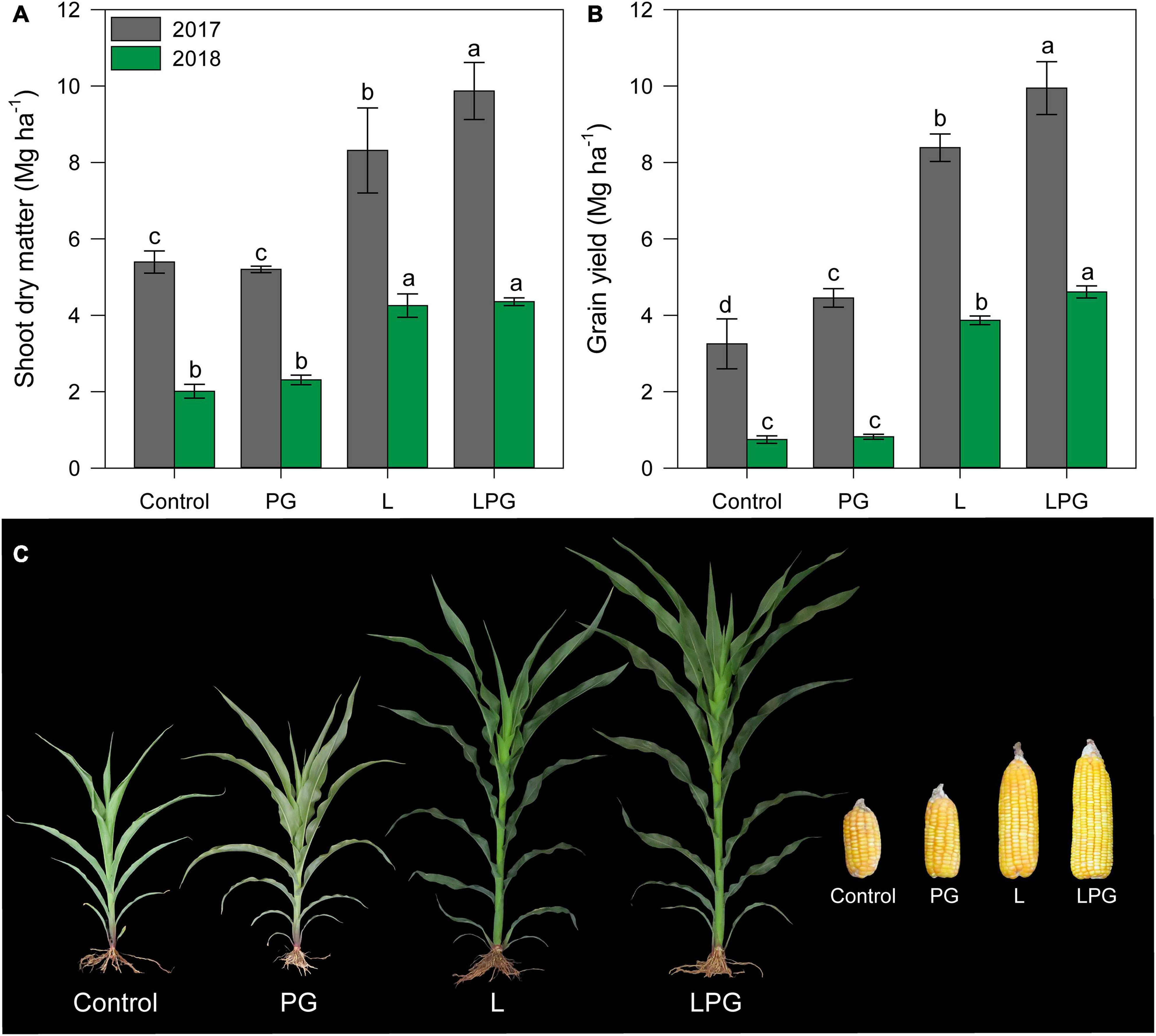
Figure 9. Shoot dry matter (A) and grain yield (B) of maize as affected by surface-applied lime (L), phosphogypsum (PG), and lime + phosphogypsum (LPG) treatments. Different lower-case letters indicate significant differences between treatments for each growing season by Student’s t-test at p ≤ 0.05. Error bars express the standard error of the mean (n = 4). Effect of soil amendments on maize development (2nd growing season = 2018) at 50 days after sowing and ear development at harvest (C).
Redundancy and Correlation Analyses of Soil and Maize Plant Measurements
To elucidate the effects of soil fertility (considering the average of soil attributes at the 0.0–1.0 m depth) on crop nutrition and crop physiology (average of the two growing seasons), three RDAs were performed (Figures 10A–C). The first RDA contemplates the relationship between soil fertility and crop nutrition, where axis 1 indicated that the soil factors explained 93.1% of the variation in nutrient concentration in maize leaves (Figure 10A). PERMANOVA analysis segregated the treatments into the following three groups: group 1 represented by the control, group 2 composed by PG, and group 3 composed by L and LPG treatments. In addition, according to Monte Carlo permutation, the main soil factors (p < 0.05) responsible for all variation in crop nutrition were the soil concentrations of Ca2+, Mg2+, Presin, and SO42–-S. Considering the role of soil fertility on crop physiology, PERMANOVA analysis segregated the treatments in four distinct groups, each represented by a treatment, with axis 1 indicating that soil fertility explained 93.8% of all variation in crop physiology (Figure 10B). The main soil factors (p < 0.05) responsible for these variations were the concentrations of Ca2+, Mg2+, Presin, and Mn. On the other hand, linking crop nutrition with maize physiology by RDA, axis 1 indicated that the crop nutrition was responsible for 91.5% of all variation in plant physiology (Figure 10C). In addition, PERMANOVA analysis segregated the treatments in two groups. Group 1 was composed by control and PG, whereas group 2 was composed by L and LPG treatments. Among the nutrients, Monte Carlo permutation indicated that the leaf concentrations of N, P, Mg, and Mn were the main responsible for changing (p < 0.05) the patterns of carbon and antioxidant metabolism in the maize. In general, Mn (soil or plant) has always been associated with an increase in oxidative stress, whereas the macronutrients (especially P and Mg) were associated with improved carbon metabolism.
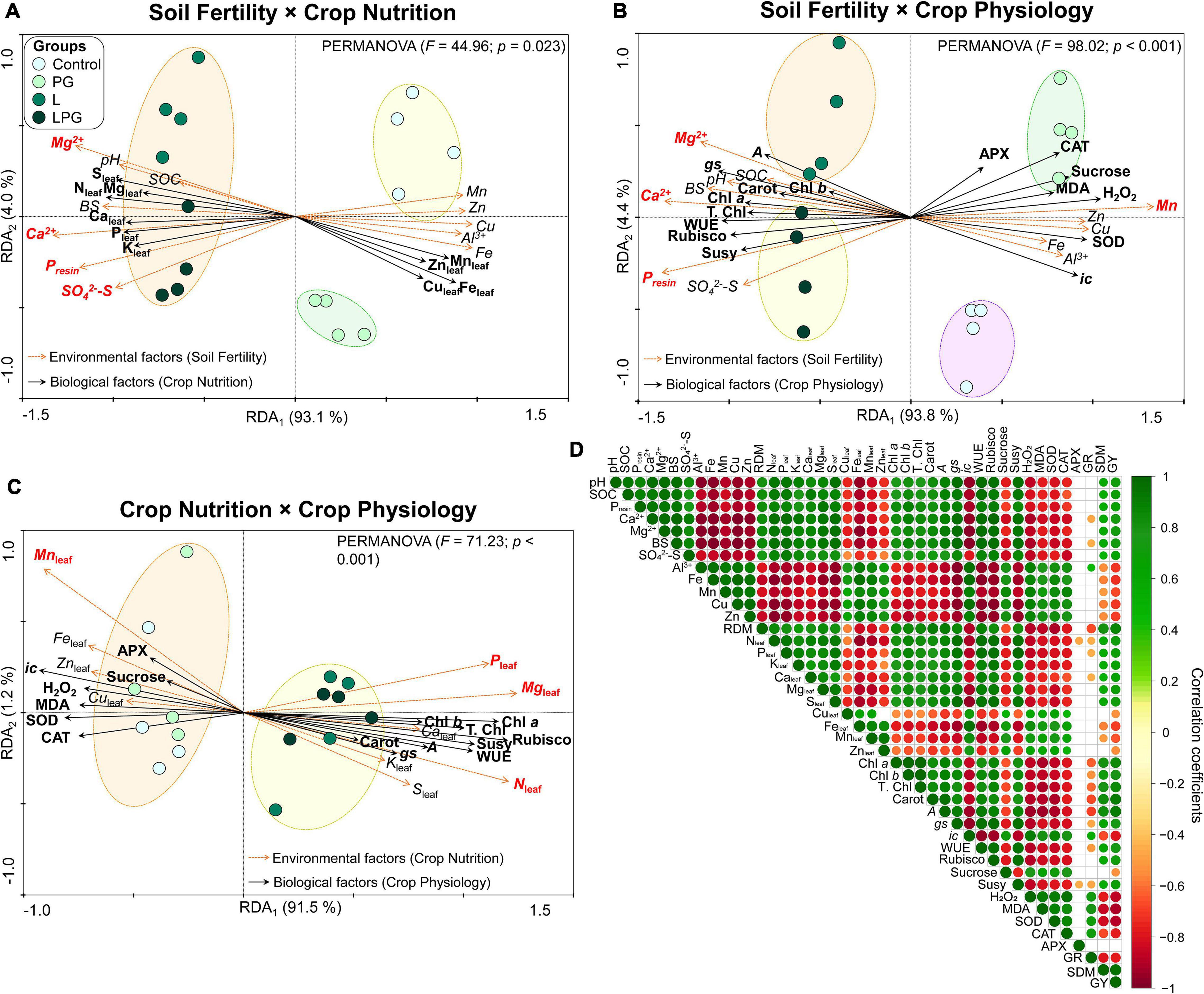
Figure 10. Redundancy analysis triplot (RDA) showing the relationship between the soil fertility × crop nutrition (A), soil fertility × crop physiology (B), and crop nutrition × crop physiology (C). The canonical axes are labeled with percentage of total variance explained (%). The arrows indicate correlations between factors. The significance of these correlations was evaluated by a Monte Carlo permutation test with 999 permutations and the significant soil properties are indicated by red color (p ≤ 0.05). The color dashed lines indicate significant clusters by permutation analysis (PERMANOVA, p ≤ 0.05). Heatmap showing the correlation coefficients (Pearson) among the soil fertility, root growth, crop nutrition, crop physiology, and agronomic parameters of maize plants (D). Only significant correlations at p ≤ 0.05 are shown. Soil organic matter (SOC), base saturation (BS), sulfate (SO42–-S), root dry matter (RDM), chlorophyll a (Chl a), Chlorophyll b (Chl b), total chlorophyll (T. Chl), carotenoids (Carot), Net photosynthesis rate (A), stomatal conductance (gs), internal CO2 concentration (ic), water use efficiency (WUE), hydrogen peroxide (H2O2), malondialdehyde (MDA), superoxide dismutase (SOD), catalase (CAT), ascorbate peroxidase (APX), glutathione reductase (GR), shoot dry matter (SDM), and grain yield (GY).
Overall, correlation analysis of soil fertility and maize nutritional, physiological and productive attributes demonstrated that improvements in soil chemical properties enhanced the development and distribution of roots in the soil profile, resulting in higher concentration of nutrients in maize leaves, as well as improved the carbon fixation, lower oxidative stress and greater SDM, and GY (Figure 10D). Increased pH and the corresponding effects on soil properties (i.e., increased SOC and exchangeable cations and low Al3+ toxicity up to 1.0 m depth) were positively correlated with root growth, leaf macronutrient concentration, pigment concentrations, gas exchange parameters, Rubisco and Susy activities, and shoot and grain production. Additionally, increased pH was negatively correlated with leaf and soil micronutrient concentrations, lipid peroxidation, and the activities of SOD, CAT, APX, and GR.
Discussion
Climatic Conditions
In both growing seasons, the amounts of rainfall received during maize cropping were much lower than the range (400–600 mm) considered sufficient for crop development (Fancelli, 2015), particularly in the second growing season (105 mm less than 2017), resulting in a severely negative hydric balance during maize development (Figure 1). In both growing seasons, the hydric conditions for second-crop maize were inappropriate and negatively influenced crop development and yield. According to Gupta et al. (2020), drought is responsible for more annual losses of crop GY than all plant pathogens combined. Climatic anomalies related to longer periods of rain scarcity are becoming increasingly common in tropical regions (Cunningham, 2020; Heinemann et al., 2021), limiting food production. Tropical regions with weathered and acidic soils present low nutrient availability and high Al3+ levels, leading to restricted root growth and lower uptake of water and nutrients, especially under lower water supply (Ritchey et al., 1982; Joris et al., 2013).
Changes in Soil Chemical Properties, Root Development, and Plant Nutrition
Remarkable effects of the soil amendments, primarily L, and LPG, were observed for most soil chemical properties, including higher soil pH, Ca2+, Mg2+, and SO42–-S levels and lower levels of exchangeable Al3+ throughout the soil profile (Figure 1). As a result, there were significant correlations among soil pH, macronutrient availability and BS (Figure 10B). Additionally, in the 0.0–0.2 m layer, SOC content and Presin concentration increased by the soil amendments application, although the increase in pH resulted in lower availability of cationic micronutrients (Figures 3, 8B). When PG was applied alone, soil attributes were slightly improved compared with the control treatment, but the effects were much smaller than those obtained by liming, regardless of the soil layer. However, when PG was applied in combination with L (LPG), the results were oftentimes greater than those obtained with L alone, especially in deeper soil layers. Unlike L, PG cannot correct soil acidity (Zoca and Penn, 2017), so its direct effects are linked to increased availability of Ca2+ and SO42–-S and reduced Al3+ content, mainly in deep layers (Costa et al., 2018; Crusciol et al., 2019; Bossolani et al., 2021b). Since most soil properties co-vary with pH (Lammel et al., 2018), PG is an important complementary amendment but not a substitute for L (Bossolani et al., 2020a).
Changes in soil nutrient concentrations can alter root development in the soil profile (Rellán-Álvarez et al., 2016). Positive correlations with soil fertility occurred for root dry matter (Figure 10B). During plant development, the architecture of the root system undergoes morphological changes depending on the availability of water and nutrients in order to improve the acquisition of environmental resources (Carmeis Filho et al., 2017; Bossolani et al., 2021b). In tropical acidic soils, low Ca2+ availability and high Mn and Al3+ availability in deeper layers are the main causes of restricted root development. Calcium plays a fundamental role in root growth as a component of the hormonal peptides responsible for cell elongation (Ritchey et al., 1995), and when it is absent in deeper layers, roots become superficial, increasing the susceptibility of plants to drought stress and limiting nutrient uptake (Crusciol et al., 2019; Bossolani et al., 2021b). The root distribution in the soil profile can be traced to mechanisms of cell division, elongation, and differentiation mediated by nutrient availability (Rellán-Álvarez et al., 2016). Longer and deeper roots can efficiently take up water from deep layers (Gupta et al., 2020).
Nutrient uptake by maize increased in L- and LPG-amended soils (Supplementary Table 5). In general, the leaf concentration of all macronutrients was positively influenced by L (regardless of PG addition). However, the leaf concentration of cationic micronutrients decreased in these treatments in response to their reduced availability in soil (Figure 1), as supported by correlation analysis (Figure 10D). In addition, our RDA analysis regarding the interactions between soil fertility and crop nutrition revealed the primary effects higher soil concentrations of Ca2+, Mg2+, Presin, and SO42–-S on improving the crop nutrition (Figure 10A). Thus, combining higher root development with increased soil nutrient concentrations, results on well-nourished plants that are able to withstand periods of low rainfall (Carmeis Filho et al., 2017; Bossolani et al., 2021b). In addition, according to Malavolta (1997), the macronutrient contents in maize were within the optimum range for full development only in soils amended with L or LPG, in both growing seasons.
Photosynthetic Parameters and Carbon Metabolism Response
Amendment with L or LPG (Figures 1, 3) enabled greater root development and improved root distribution throughout the soil profile (Figure 2), as well as plant nutrition (Supplementary Table 5). Under low water availability like that observed in this study, plants seek to adapt to the soil moisture gradient and, driven by nutrient availability, change their root architecture to enhance their ability to take up water and nutrients (Rellán-Álvarez et al., 2016). These changes are reflected in greater synthesis of chlorophylls and carotenoids (Figure 5). Chlorophyll is an important part of the Calvin cycle and is responsible for harvesting sunlight during plant photosynthesis (Croft et al., 2017; Busch, 2020). Carotenoids are responsible for adapting plastids to stress conditions, light and energy dissipation to avoid excessive production of reactive oxygen species (ROS) (Gómez et al., 2019).
Correlation analysis suggested that the increase in photosynthetic pigment concentrations was related to changes in leaf nutritional status, gas exchange parameters and photosynthetic potential (Figure 10D). Rates of A, gs, and WUE were also increased by applying L alone or LPG (Figures 6A,B,D). In addition, the increases in these parameters in association with the decreases in ic (Figure 6C) and E (Figure 5) imply greater productive capacity of plants under stress conditions (Reis et al., 2018). The regulation of stomatal conductance under conditions of low hydric availability is extremely important for increased WUE (Gupta et al., 2020). According to these authors, stomatal closure is the first defense against water loss. Adequate plant nutrition (e.g., K, Ca, and Mg) are essential for the mechanisms that modulate this response (Brodribb and McAdam, 2017; Gong et al., 2020). According to our RDAs linking soil fertility (Figure 10B) and crop nutrition (Figure 10C) with crop physiology, gs values were strongly associated with the soil concentrations of exchangeable bases (Ca2+, Mg2+, and BS) and the leaf concentrations of K and Ca, reinforcing the role of these nutrients in improving the use of water under hydric restrictions, as occurred in our study. Additionally, greater availability of nutrients in the soil, especially in deeper layers, increases root growth and the use of resources that enhance resilience to low water availability (Rellán-Álvarez et al., 2016).
The combination of these factors may imply greater carbon fixation by plants (Reis et al., 2018). The key enzyme involved in carbon fixation that drives the assimilation of CO2 is Rubisco (Busch, 2020). In this study, Rubisco activity was higher in the leaves of maize in soils amended with L and, in particular, LPG (Figure 7A). Susy activity was also increased in these treatments (Figure 7C), although the levels of sucrose decreased (Figure 7B). The response pathways of plants to environmental stress also include changes in the production and mobilization of metabolites (Bailey-Serres et al., 2019). Each Rubisco-mediated carboxylation reaction gives rise to triose phosphates that are exported and become substrates for the synthesis of most of the other organic compounds that make up the plant (Stein and Granot, 2019). Sucrose synthase (Susy) plays a key role in sugar metabolism and can reversibly cleave sucrose into fructose and glucose. These by-products can enter several metabolic pathways to provide energy and carbon skeletons for plant metabolism (Stein and Granot, 2019). The increases in Rubisco and Susy activity and low sucrose concentrations in leaves suggest enhancement of production and partitioning of sugars from source to sink tissues (Farhat et al., 2016).
Redundancy analysis enabled the identification of the most important soil and nutritional factors responsible for increasing carbon metabolism (Figures 10B,C), that were also supported by correlation analysis (Figure 10D). Both RDAs presented a similar pattern correlating the soil and nutritional factors on carbon metabolism, however, the segregation of treatments was assembled differently. Soil fertility was more sensitive in clustering the treatments, segregating each treatment in a group; on the other hand, crop nutrition separated the treatments into only two groups: control and PG, and L and LPG treatments. Possibly, the segregation of treatments occurred differently considering soil fertility and crop nutrition due to the strong role of soil chemistry in modulating the soil nutrients availability, and the root growth, which directly contributes to the acquisition of water for the full functioning of photosynthesis (Rellán-Álvarez et al., 2016; Busch, 2020; Gong et al., 2020). Additionally, although the concentration of nutrients is an important indicator of the nutritional status of plants, it is worth noting that the leaf concentration of nutrients does not reflect the amount of nutrients that accumulate in the plant tissues. The foliar concentration of nutrients can be subject to the concentration and dilution processes, depending on the biomass production by plants (Maia, 2012). For this reason, the soil chemistry may have been more sensitive to changes that occurred in carbon metabolism. In general, soil and leaf P, Ca2+, and Mg2+ were the main nutrients responsible for improving the dynamics of Rubisco and Susy activities in maize plants, whereas Mn was mainly responsible for reductions in these activities, as observed in the control and PG treatments. Phosphorus is an important supplier of energy for photosynthetic mechanisms and plant metabolism. Mg2+ is the most abundant divalent cation in the plant cytosol and participates in several aspects of photosynthesis, such as the composition of the chlorophyll molecule, activation of Rubisco in the carboxylation process and the partitioning of photoassimilates by plant tissues (Ceylan et al., 2016). Calcium also plays an important role in multiple photosynthetic pathways (Wang et al., 2019). This nutrient affects gas exchange related to photosynthesis by regulating stomatal movement (Song et al., 2014). Several photosynthetic proteins are regulated directly or indirectly by Ca (Wang et al., 2019). In addition, soil Ca plays an important role in signaling and root growth, increasing the capacity of plants to uptake water and other nutrients (Ritchey et al., 1995).
In acidic and low-fertility soils, Mn is generally abundant and limits plant growth (Roth and Pavan, 1991; Bossolani et al., 2020b). Mn can replace Mg in the Rubisco activation process and increases the affinity of the enzyme for oxygen by approximately 20-fold compared with Mg2+, thus increasing photorespiration by plants (Kitao et al., 1997). In addition, high concentrations of metallic nutrients such as Mn and Al3+ can reduce the WUE of plants (Reis et al., 2018), compromising the crops in periods of low water availability. Several authors have also associated these elements with reduced root development (Roth and Pavan, 1991; Ritchey et al., 1995; Caires et al., 2011; Costa and Crusciol, 2016).
Lipid Peroxidation and Antioxidant Metabolism Response
Oxidative stress in maize plants was strongly reduced by the application of L and LPG (Figures 8A,B), as evidenced by the reductions in the concentrations of H2O2 and MDA in these treatments. In addition to its role in cell defense signaling (Cuypers et al., 2010), H2O2 is one of the main forms of ROS responsible for lipid peroxidation (Loix et al., 2018).
The patterns of activity of antioxidant system enzymes (SOD, CAT, APX, and GR) (Figures 8C–F) were similar to those for lipid peroxidation, indicating that the low activity of these enzymes in maize established in L- and LPG-amended soils is the result of efficient catalysis of their substrates (ROS) (Silva et al., 2020). Lipid peroxidation can also be caused by other ROS [e.g., singlet oxygen (1O2–) and hydroxyl radicals (OH–)] (Farmer and Mueller, 2013). The first line of defense in antioxidant metabolism is the dismutation of 1O2– into H2O2 and H2O by the enzyme SOD, followed by the breakdown of H2O2 into H2O and O2 by CAT, APX, and GR (Noctor, 2002). In this process of ROS scavenging, enzymes are also consumed, resulting in reduced activity when measured in plant tissue (Noctor, 2002; Farmer and Mueller, 2013).
The increased oxidative stress in maize plants in the control and PG treatments is primarily attributable to low soil fertility and plant nutrition, as supported by RDA and correlation analysis (Figures 10A–D). Plants grown under low nutrient availability, mainly P, Ca2+, and Mg2+, and under high concentrations of toxic elements, such as Mn and Al3+, are more likely to reduce pigment levels (Figure 5) and photosynthetic activity (Figures 5, 6; Liu et al., 2008, 2015; Gong et al., 2020), in addition to accumulating sugars in source tissues (Figure 7B; Stein and Granot, 2019). All of these factors are positively correlated with increased oxidative stress (Figure 10B; Gong et al., 2020). The gradual loss of photosynthetic capacity results in electron accumulation in photosystems I and II, generating greater amounts of ROS and lipid peroxidation (Croft et al., 2017). The accumulation of sucrose in photosynthetically active leaves interferes with the production of ROS and increases oxidative stress in plants, especially for plants growing under soil Mg2+ deficiency (Cakmak and Kirkby, 2008). Calcium deficiency induces low stomatal conductance (Figures 6B, 8B) and, consequently, higher water loss via stomata, which can increase oxidative stress in plants (Gupta et al., 2020), particularly under low hydric availability, which is common during maize cropping. In summary, the mitigation of soil acidification, reduced toxicity of Mn and Al3+, and increased availability of nutrients such as P, Ca2+, and Mg2+ provided by liming (L), especially when combined with PG (LPG), can reduce oxidative stress in plants.
Maize Shoot Dry Matter Production and Grain Yield
This study revealed positive effects of the soil amendments on root development, plant carbon and antioxidant metabolism, SDM production and GY (Figure 9). In addition, during maize development, more turgid and vigorous plants were obtained, even under low pluvial precipitation (Figure 1). The long-term application of L and LPG improved soil fertility and root development, increasing the water and nutrients uptake by plants (Table 1). In addition, increased photosynthesis and better regulation of oxidative stress led to higher maize GYs under these soil amendments. Importantly, combining L and PG (LPG) provided better results than amendment with L alone. Understanding how soil amendments charges tropical soils and shape plant responses under drought is of paramount importance for the development of more productive agricultural systems under continuous climate change (Gibbons et al., 2014; Gupta et al., 2020). Therefore, amendment of soils with LPG should be considered a viable and promising alternative to improve the yield capacity of acidic tropical soils managed under NT (Costa and Crusciol, 2016; Crusciol et al., 2019; Bossolani et al., 2020a, b, 2021b).
Conclusion
After 2 years of the last surface reapplication of soil amendments (four reapplications of lime and/or phosphogypsum in 16 years of experiment), liming, especially combined with phosphogypsum, increased soil pH, P, Ca2+, Mg2+, and SO42–-S and reduced the concentrations of Al3+, Fe, Mn, Cu, and Zn beyond the depth where lime was applied. The improvement on soil fertility brought about by soil amendments, led to an increase on root growth of maize, which in turn, increased water and nutrient uptake by plants. As a result of this chain effect, maize plants grown under field drought conditions, improved their antioxidant system, photosynthetic pigment concentrations and, consequently, the carbon metabolism, including Rubisco and Susy activities, and sucrose production and partitioning. This study has not only confirmed that phosphogypsum potentiates the effects of lime on improving soil fertility, but has also highlighted that the increase in macronutrient concentrations (in soil and plants), especially P, Ca, and Mg, and reducing Mn concentrations, has a fundamental role in increasing antioxidant system and photosynthetic metabolism, benefits that eventually were reflected in the increase of the biomass production and GY.
Data Availability Statement
The raw data supporting the conclusions of this article will be made available by the authors, without undue reservation.
Author Contributions
JB and CC worked on the research designing and conduction, data analysis, and writing and formatting the manuscript. AG, LM, JP, VR, MF, JC, EC, TA, and AR revised this draft by rewriting, discussing, and commenting. All authors contributed significantly on the manuscript, confirm being contributor of this work, and approved it for publication.
Funding
This study was supported by São Paulo Research Foundation (FAPESP) (Grants 2018/11063-7 and 2019/12764-1) through financial support for scholarship to JB and for analysis. In addition, the National Council for Scientific and Technological Development (CNPq) (Universal Research Project: 421637/2018-8) provided financial support for analysis to CC.
Conflict of Interest
The authors declare that the research was conducted in the absence of any commercial or financial relationships that could be construed as a potential conflict of interest.
Acknowledgments
CC, EC, TA, JC, and AR would like to thank the CNPq for an award for excellence in research.
Supplementary Material
The Supplementary Material for this article can be found online at: https://www.frontiersin.org/articles/10.3389/fpls.2021.650296/full#supplementary-material
Footnotes
References
Alexieva, V., Sergiev, I., Mapelli, S., and Karanov, E. (2001). The effect of drought and ultraviolet radiation on growth and stress markers in pea and wheat. Plant. Cell Environ. 24, 1337–1344. doi: 10.1046/j.1365-3040.2001.00778.x
Allen, R. G., Pereira, L. S., Raes, D., and Smith, M. (1998). Crop Evapotranspiration-Guidelines for Computing Crop Water Requirements-FAO Irrigation and Drainage Paper 56. Rome: FAO.
Alvares, C. A., Stape, J. L., Sentelhas, P. C., de Moraes Gonçalves, J. L., and Sparovek, G. (2013). Köppen’s climate classification map for Brazil. Meteorol. Zeitschrift. 22, 711–728. doi: 10.1127/0941-2948/2013/0507
Anderson, M. J. (2005). Permutational multivariate analysis of variance. Dep. Stat. Univ. Auckland Auckl. 26, 32–46.
Azevedo, R. A., Alas, R. M., Smith, R. J., and Lea, P. J. (1998). Response of antioxidant enzymes to transfer from elevated carbon dioxide to air and ozone fumigation, in the leaves and roots of wild-type and a catalase-deficient mutant of barley. Physiol. Plant. 104, 280–292. doi: 10.1034/j.1399-3054.1998.1040217.x
Bailey-Serres, J., Parker, J. E., Ainsworth, E. A., Oldroyd, G. E. D., and Schroeder, J. I. (2019). Genetic strategies for improving crop yields. Nature 575, 109–118. doi: 10.1038/s41586-019-1679-0
Bardsley, C. E., and Lancaster, J. D. (1960). Determination of reserve sulfur and soluble sulfates in soils. Soil Sci. Soc. Am. J. 24, 265–268. doi: 10.2136/sssaj1960.03615995002400040015x
Bian, M., Zhou, M., Sun, D., and Li, C. (2013). Molecular approaches unravel the mechanism of acid soil tolerance in plants. Crop J. 1, 91–104. doi: 10.1016/j.cj.2013.08.002
Bieleski, R. L., and Turner, N. A. (1966). Separation and estimation of amino acids in crude plant extracts by thin-layer electrophoresis and chromatography. Anal. Biochem. 17, 278–293.
Bindraban, P. S., van der Velde, M., Ye, L., van den Berg, M., Materechera, S., Kiba, D. I., et al. (2012). Assessing the impact of soil degradation on food production. Curr. Opin. Environ. Sustain. 4, 478–488. doi: 10.1016/j.cosust.2012.09.015
Bossolani, J. W., Crusciol, C. A. C., Leite, M. F. A., Merloti, L. F., Moretti, L. G., Pascoaloto, I. M., et al. (2021a). Modulation of the soil microbiome by long-term Ca-based soil amendments boosts soil organic carbon and physicochemical quality in a tropical no-till crop rotation system. Soil Biol. Biochem. 156:108188. doi: 10.1016/j.soilbio.2021.108188
Bossolani, J. W., Crusciol, C. A. C., Portugal, J. R., Moretti, L. G., Garcia, A., Rodrigues, V. A., et al. (2021b). Long-term liming improves soil fertility and soybean root growth, reflecting improvements in leaf gas exchange and grain yield. Eur. J. Agron. 128:126308. doi: 10.1016/j.eja.2021.126308
Bossolani, J. W., Crusciol, C. A. C., Merloti, L. F., Moretti, L. G., Costa, N. R., Tsai, S. M., et al. (2020a). Long-term lime and gypsum amendment increase nitrogen fixation and decrease nitrification and denitrification gene abundances in the rhizosphere and soil in a tropical no-till intercropping system. Geoderma 375, 114476. doi: 10.1016/j.geoderma.2020.114476
Bossolani, J. W., dos Santos, F. L., Meneghette, H. H. A., Sanches, I. R., Moretti, L. G., Parra, L. F., et al. (2020b). Soybean in crop rotation with maize and palisade grass intercropping enhances the long-term effects of surface liming in no-till system. J. Soil Sci. Plant Nutr. 20, 1–12. doi: 10.1007/s42729-020-00347-2
Bossolani, J. W., Lazarini, E., Santos, F. L., Sanches, I. R., Meneghette, H. H. A., Parra, L. F., et al. (2018). Surface reapplication of lime and gypsum on maize cultivated sole and intercropped with Urochloa. Commun. Soil Sci. Plant Anal. 49, 1855–1868. doi: 10.1080/00103624.2018.1475565
Bradford, M. M. (1976). A rapid and sensitive method for the quantitation of microgram quantities of protein utilizing the principle of protein-dye binding. Anal. Biochem. 72, 248–254. doi: 10.1006/abio.1976.9999
Brodribb, T. J., and McAdam, S. A. M. (2017). Evolution of the stomatal regulation of plant water content. Plant Physiol. 174, 639–649. doi: 10.1104/pp.17.00078
Busch, F. A. (2020). Photorespiration in the context of Rubisco biochemistry, CO2 diffusion and metabolism. Plant J. 101, 919–939. doi: 10.1111/tpj.14674
Caires, E. F., and Guimarães, A. M. (2018). A novel phosphogypsum application recommendation method under continuous no-till management in Brazil. Agron. J. 110, 1987–1995. doi: 10.2134/agronj2017.11.0642
Caires, E. F., Joris, H. A. W., and Churka, S. (2011). Long-term effects of lime and gypsum additions on no-till corn and soybean yield and soil chemical properties in southern Brazil. Soil Use Manag. 27, 45–53. doi: 10.1111/j.1475-2743.2010.00310.x
Cakmak, I., and Kirkby, E. A. (2008). Role of magnesium in carbon partitioning and alleviating photooxidative damage. Physiol. Plant. 133, 692–704. doi: 10.1111/j.1399-3054.2007.01042.x
Cantarella, H., Raij, B., and van Camargo, C. E. O. (1997). “Adubação de cereais,” in Recomendações de Adubação e Calagem Para o Estado de São Paulo, 2nd Edn, eds B. V. Raij, H. Cantarella, J. A. Quaggio, and A. M. C. Furlani (Campinas: Instituto Agronomico/IAC), 43–50.
Carmeis Filho, A. C. A., Crusciol, C. A. C., and Castilhos, A. M. (2017). Liming demand and plant growth improvements for an Oxisol under long-term no-till cropping. J. Agric. Sci. 155, 1093–1112. doi: 10.1017/S0021859617000235
Ceylan, Y., Kutman, U. B., Mengutay, M., and Cakmak, I. (2016). Magnesium applications to growth medium and foliage affect the starch distribution, increase the grain size and improve the seed germination in wheat. Plant Soil 406, 145–156. doi: 10.1007/s11104-016-2871-8
Costa, C. H. M., Carmeis Filho, A. C. A., Crusciol, C. A. C., Soratto, R. P., and Guimarães, T. M. (2018). Intensive annual crop production and root development in a tropical acid soil under long-term no-till and soil-amendment management. Crop Pasture Sci. 69, 488–505. doi: 10.1071/CP17233
Costa, C. H. M., and Crusciol, C. A. C. (2016). Long-term effects of lime and phosphogypsum application on tropical no-till soybean-oat-sorghum rotation and soil chemical properties. Eur. J. Agron. 74, 119–132. doi: 10.1016/j.eja.2015.12.001
Croft, H., Chen, J. M., Luo, X., Bartlett, P., Chen, B., and Staebler, R. M. (2017). Leaf chlorophyll content as a proxy for leaf photosynthetic capacity. Glob. Chang. Biol. 23, 3513–3524. doi: 10.1111/gcb.13599
Crusciol, C. A. C., Marques, R. R., Carmeis Filho, A. C. A., Soratto, R. P., Costa, C. H. M., Ferrari Neto, J., et al. (2019). Lime and gypsum combination improves crop and forage yields and estimated meat production and revenue in a variable charge tropical soil. Nutr. Cycl. Agroecosyst. 115, 347–372. doi: 10.1007/s10705-019-10017-0
Cunningham, C. (2020). Characterization of dry spells in Southeastern Brazil during the monsoon season. Int. J. Climatol. 40, 4609–4621. doi: 10.1002/joc.6478
Cuypers, A., Plusquin, M., Remans, T., Jozefczak, M., Keunen, E., Gielen, H., et al. (2010). Cadmium stress: an oxidative challenge. Biometals 23, 927–940. doi: 10.1007/s10534-010-9329-x
Dejardin, A., Rochat, C., Wuilleme, S., and Boutin, J.-P. (1997). Contribution of sucrose synthase, ADP-glucose pyrophosphorylase and starch synthase to starch synthesis in developing pea seeds. Plant Cell Environ. 20, 1421–1430. doi: 10.1046/j.1365-3040.1997.d01-32.x
Eekhout, T., Larsen, P., and De Veylder, L. (2017). Modification of DNA checkpoints to confer aluminum tolerance. Trends Plant Sci. 22, 102–105. doi: 10.1016/j.tplants.2016.12.003
Fancelli, A. L. (2015). “Ecofisiologia, fenologia e implicações básicas de manejo,” in Milho do Plantio à Colheita, 1st Edn, eds A. Borém, J. C. C. Galvão, and M. A. Pimentel (Viçosa: UFV), 50–76.
Farhat, N., Elkhouni, A., Zorrig, W., Smaoui, A., Abdelly, C., and Rabhi, M. (2016). Effects of magnesium deficiency on photosynthesis and carbohydrate partitioning. Acta Physiol. Plant. 38:145. doi: 10.1007/s11738-016-2165-z
Farmer, E. E., and Mueller, M. J. (2013). ROS-mediated lipid peroxidation and RES-activated signaling. Annu. Rev. Plant Biol. 64, 429–450. doi: 10.1146/annurev-arplant-050312-120132
Giannopolitis, C. N., and Ries, S. K. (1977). Superoxide dismutases: I. occurrence in higher plants. Plant Physiol. 59, 309–314. doi: 10.1104/pp.59.2.309
Gibbons, J. M., Williamson, J. C., Williams, A. P., Withers, P. J. A., Hockley, N., Harris, I. M., et al. (2014). Sustainable nutrient management at field, farm and regional level: soil testing, nutrient budgets and the trade-off between lime application and greenhouse gas emissions. Agric. Ecosyst. Environ. 188, 48–56. doi: 10.1016/j.agee.2014.02.016
Gibbs, H. K., and Salmon, J. M. (2015). Mapping the world’s degraded lands. Appl. Geogr. 57, 12–21. doi: 10.1016/j.apgeog.2014.11.024
Gomes-Junior, R. A., Moldes, C. A., Delite, F. S., Pompeu, G. B., Gratao, P. L., Mazzafera, P., et al. (2006). Antioxidant metabolism of coffee cell suspension cultures in response to cadmium. Chemosphere 65, 1330–1337.
Gómez, R., Vicino, P., Carrillo, N., and Lodeyro, A. F. (2019). Manipulation of oxidative stress responses as a strategy to generate stress-tolerant crops. From damage to signaling to tolerance. Crit. Rev. Biotechnol. 39, 693–708. doi: 10.1080/07388551.2019.1597829
Gong, Z., Xiong, L., Shi, H., Yang, S., Herrera-Estrella, L. R., Xu, G., et al. (2020). Plant abiotic stress response and nutrient use efficiency. Sci. China Life Sci. 63, 635–674. doi: 10.1007/s11427-020-1683-x
Gratão, P. L., Monteiro, C. C., Antunes, A. M., Peres, L. E. P., and Azevedo, R. A. (2008). Acquired tolerance of tomato (Lycopersicon esculentum cv. Micro-Tom) plants to cadmium-induced stress. Ann. Appl. Biol. 153, 321–333. doi: 10.1016/j.chemosphere.2006.04.056
Gupta, A., Rico-Medina, A., and Caño-Delgado, A. I. (2020). The physiology of plant responses to drought. Science 368, 266–269. doi: 10.1126/science.aaz7614
Heath, R. L., and Packer, L. (1968). Photoperoxidation in isolated chloroplasts: I. Kinetics and stoichiometry of fatty acid peroxidation. Arch. Biochem. Biophys. 125, 189–198. doi: 10.1016/0003-9861(68)90654-1
Heinemann, A. B., Ramirez-Villegas, J., Stone, L. F., Silva, A. P. G. A., da Matta, D. H., and Diaz, M. E. P. (2021). The impact of El Niño Southern oscillation on cropping season rainfall variability across Central Brazil. Int. J. Climatol. 41, E283–E304. doi: 10.1002/joc.6684
Holland, J. E., Bennett, A. E., Newton, A. C., White, P. J., McKenzie, B. M., George, T. S., et al. (2018). Liming impacts on soils, crops and biodiversity in the UK: a review. Sci. Total Environ. 610-611, 316–332. doi: 10.1016/j.scitotenv.2017.08.020
Joris, H. A. W., Caires, E. F., Bini, A. R., Scharr, D. A., and Haliski, A. (2013). Effects of soil acidity and water stress on corn and soybean performance under a no-till system. Plant Soil 365, 409–424. doi: 10.1007/s11104-012-1413-2
Kitao, M., Lei, T. T., and Koike, T. (1997). Effects of manganese toxicity on photosynthesis of white birch (Betula platyphylla var. japonica) seedlings. Physiol. Plant. 101, 249–256. doi: 10.1034/j.1399-3054.1997.1010201.x
Kleiner, K., Abrams, M., and Schultz, J. (1992). The impact of water & nutrient deficiencies on the growth. Tree Physiol. 11, 271–287. doi: 10.1093/treephys/11.3.271
Lammel, D. R., Barth, G., Ovaskainen, O., Cruz, L. M., Zanatta, J. A., Ryo, M., et al. (2018). Direct and indirect effects of a pH gradient bring insights into the mechanisms driving prokaryotic community structures. Microbiome 6, 7–9. doi: 10.1186/s40168-018-0482-8
Lichtenthaler, H. K. (1987). Chlorophylls and carotenoids: pigments of photosynthetic biomembranes. Method. Enzymol., 148, 350–382. doi: 10.1016/0076-6879(87)48036-1
Liu, H., Chen, X., Chen, R., Song, S., and Sun, G. (2008). Effects of magnesium deficiency on growth and photosynthesis of flowering Chinese cabbage. Acta Hortic. 767, 169–176.
Liu, Y. F., Zhang, G. X., Qi, M. F., and Li, T. L. (2015). Effects of calcium on photosynthesis, antioxidant system, and chloroplast ultrastructure in tomato leaves under low night temperature stress. J. Plant Growth Regul. 34, 263–273. doi: 10.1007/s00344-014-9462-9
Loix, C., Huybrechts, M., Vangronsveld, J., Gielen, M., Keunen, E., and Cuypers, A. (2018). Reciprocal interactions between cadmium-induced cell wall responses and oxidative stress in plants. Front. Plant Sci. 9:391. doi: 10.3389/fpls.2018.00391
Maia, C. E. (2012). Sampling time of banana leaves for nutritional diagnosis. Rev. Bras. Ciência Solo 36, 859–864.
Malavolta, E. (1997). Avaliação do Estado Nutricional das Plantas: Princípios e Aplicações/Eurípedes Malavolta, Godofredo Cesar Vitti, Sebastião Alberto de Oliveira. Piracicaba: Potafos.
Noctor, G. (2002). Interactions between biosynthesis, compartmentation and transport in the control of glutathione homeostasis and signalling. J. Exp. Bot. 53, 1283–1304. doi: 10.1093/jexbot/53.372.1283
Nora, D. D., Amado, T. J. C., Nicoloso, R., da, S., Mazuco, A. C. B., and Piccin, M. (2017). Mitigation of the gradient of chemical properties in the rooting zone of dystrophic oxisols by gypsum and lime inputs under a no-till system. Rev. Bras. Cienc. Solo 41, 1–22. doi: 10.1590/18069657rbcs20150541
Reid, C. D., Tissue, D. T., Fiscus, E. L., and Strain, B. R. (1997). Comparison of spectrophotometric and radioisotopic methods for the assay of Rubisco in ozone-treated plants. Physiol. Plant. 101, 398–404. doi: 10.1111/j.1399-3054.1997.tb01014.x
Reis, A. R. D., Lisboa, L. A. M., Reis, H. P. G., Barcelos, J. P., de, Q., Santos, E. F., et al. (2018). Depicting the physiological and ultrastructural responses of soybean plants to Al stress conditions. Plant Physiol. Biochem. 130, 377–390. doi: 10.1016/j.plaphy.2018.07.028
Rellán-Álvarez, R., Lobet, G., and Dinneny, J. R. (2016). Environmental control of root system biology. Annu. Rev. Plant Biol. 67, 619–642. doi: 10.1146/annurev-arplant-043015-111848
Ritchey, K. D., Feldhake, C. M., Clark, R. B., and Sousa, D. M. G. (1995). “Improved water and nutrient uptake from subsurface layers of gypsum-amended soils,” in Agricultural Utilization of Urban and Industrial By-Products, ed. D. L. Karlen (Madison: ASA), 157–181.
Ritchey, K. D., Silva, J. E., and Costa, U. F. (1982). Calcium deficiency in clayey B horizons of savanna oxisols. Soil Sci. 133, 378–382.
Ritchie, S. W., Hanway, J. J., and Benson, G. O. (1993). How a Corn Plant Develops. Special Report no. 48. Ames, IA: Iowa State University of Science and Technology, Cooperative Extension Service.
Rolim, G. D. S., Sentelhas, P. C., and Barbieri, V. (1998). Planilhas no ambiente EXCEL TM para os cálculos de balanços hídricos: normal, sequencial, de cultura e de produtividade real e potencial. Rev. Bras. Agrometeorol. 6, 133–137.
Roth, C. H., and Pavan, M. A. (1991). Effects of lime and gypsum on clay dispersion and infiltration in samples of a Brazilian Oxisol. Geoderma 48, 351–361. doi: 10.1016/0016-7061(91)90053-V
Sage, R. F., Sharkey, T. D., and Seemann, J. R. (1988). The in-vivo response of the ribulose-1, 5-bisphosphate carboxylase activation state and the pool sizes of photosynthetic metabolites to elevated CO2 in Phaseolus vulgaris L.. Planta 174, 407–416. doi: 10.1007/BF00959528
Shoemaker, H. E., McLean, E. O., and Pratt, P. F. (1961). Buffer methods for determining lime requirement of soils with appreciable amounts of extractable aluminum. Soil Sci. Soc. Am. J. 25, 274–277. doi: 10.2136/sssaj1961.03615995002500040014x
Silva, V. M., Rimoldi Tavanti, R. F., Gratão, P. L., Alcock, T. D., and dos Reis, A. R. (2020). Selenate and selenite affect photosynthetic pigments and ROS scavenging through distinct mechanisms in cowpea (Vigna unguiculata (L.) walp) plants. Ecotoxicol. Environ. Saf. 201:110777. doi: 10.1016/j.ecoenv.2020.110777
Song, Y., Miao, Y., and Song, C. P. (2014). Behind the scenes: the roles of reactive oxygen species in guard cells. New Phytol. 201, 1121–1140.
Stein, O., and Granot, D. (2019). An overview of sucrose synthases in plants. Front. Plant Sci. 10:95. doi: 10.3389/fpls.2019.00095
Unicamp (2020). Center of Meteorological and Climatic Research Applied to Agriculture. Botucatu: UNICAMP.
van Raij, B., Cantarella, H., Quaggio, J. A., and Furlani, A. M. C. (1997). Recomendações de Adubação e Calagem para o Estado de São Paulo. São Paulo: Instituto Agronômico/Fundação IAC Campinas.
van Raij, B., Quaggio, J. A., Cantarella, H., and Abreu, C. A. (2001). “Os métodos de análise química do sistema IAC de análise de solo no contexto nacional,” in Análise química para Avaliação da Fertilidade de Solos Tropicais, eds B. V. Raij, J. C. Andrade, H. Cantarella, and J. A. Quaggio (Campinas: IAC), 5–39.
Walkley, A., and Black, I. A. (1934). An examination of the Degtjareff method for determining soil organic matter, and a proposed modification of the chromic acid titration method. Soil Sci. 37, 29–38.
Wang, Q., Yang, S., Wan, S., and Li, X. (2019). The significance of calcium in photosynthesis. Int. J. Mol. Sci. 20, 1–14. doi: 10.3390/ijms20061353
Wellburn, A. R. (1994). The spectral determination of chlorophyll a and b, as well as carotenoids using various solvents with spectrophotometers of different resolution. J. Plant Physiol 144, 307–313.
Keywords: soil fertility, soil amendments, root distribution, Rubisco, sucrose synthase, oxidative stress
Citation: Bossolani JW, Crusciol CAC, Garcia A, Moretti LG, Portugal JR, Rodrigues VA, Fonseca MC, Calonego JC, Caires EF, Amado TJC and Reis AR (2021) Long-Term Lime and Phosphogypsum Amended-Soils Alleviates the Field Drought Effects on Carbon and Antioxidative Metabolism of Maize by Improving Soil Fertility and Root Growth. Front. Plant Sci. 12:650296. doi: 10.3389/fpls.2021.650296
Received: 21 January 2021; Accepted: 21 June 2021;
Published: 12 July 2021.
Edited by:
Luciano Kayser Vargas, Department of Agricultural Research and Diagnosis, State Secretariat of Agriculture, Livestock and Irrigation, BrazilReviewed by:
André Auler, Federal University of Paraná, BrazilVolnei Pauletti, Federal University of Paraná, Brazil
Copyright © 2021 Bossolani, Crusciol, Garcia, Moretti, Portugal, Rodrigues, Fonseca, Calonego, Caires, Amado and Reis. This is an open-access article distributed under the terms of the Creative Commons Attribution License (CC BY). The use, distribution or reproduction in other forums is permitted, provided the original author(s) and the copyright owner(s) are credited and that the original publication in this journal is cited, in accordance with accepted academic practice. No use, distribution or reproduction is permitted which does not comply with these terms.
*Correspondence: Carlos Alexandre Costa Crusciol, Y2FybG9zLmNydXNjaW9sQHVuZXNwLmJy