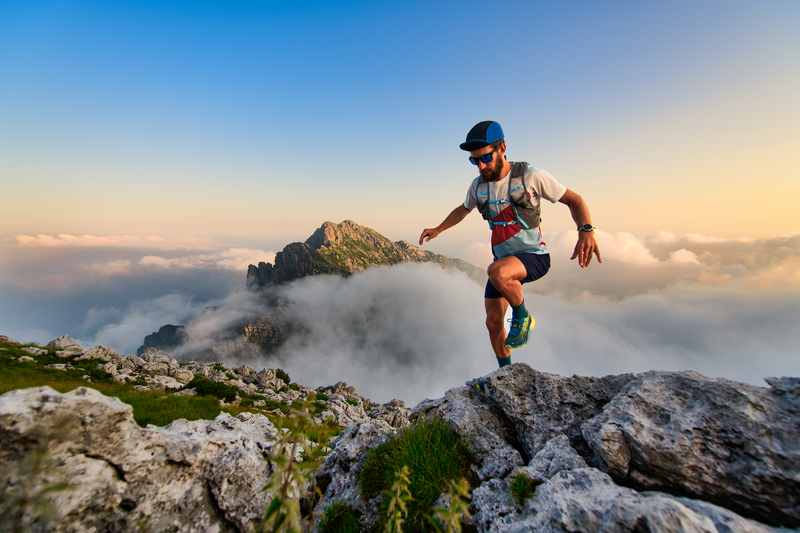
94% of researchers rate our articles as excellent or good
Learn more about the work of our research integrity team to safeguard the quality of each article we publish.
Find out more
ORIGINAL RESEARCH article
Front. Plant Sci. , 12 May 2021
Sec. Plant Biotechnology
Volume 12 - 2021 | https://doi.org/10.3389/fpls.2021.641420
This year, a respiratory virus caused an emergency pandemic alert in health services around the world, showing the need for biotechnological approaches to fight these diseases. The influenza virus is one of the main viral agents that generate pandemic outbreaks. Currently, the majority of co-circulating influenza A virus (IAV) strains are adamantine‐ and oseltamivir-resistant strains, and the challenge is to find new antivirals for more efficient treatments. The antiviral entry blocker (EB) peptide is a promising candidate for blocking the virus entry into cells. The aim of this research was to express the EB peptide in the microalgae Chlamydomonas reinhardtii and test its antiviral activity against IAV in vitro. The EB peptide nucleotide sequence was introduced into the nuclear genome of microalgae using Agrobacterium tumefaciens transformation. The EB peptide amount produced in transformed microalgae was 4.99 ± 0.067% of the total soluble protein. In hemagglutination inhibition assays using influenza A/H1N1 pdm and influenza A H1N1/Virginia/ATCC/2009 strains, we reported that the EB peptide extract from the microalgae showed 100-fold higher efficiency than the EB synthetic peptide. In addition, both the EB peptide extract and synthetic peptide inhibited viral replication in MDCK cells (IC50 = 20.7 nM and IC50 = 754.4 nM, respectively); however, the EB peptide extract showed a 32-fold higher antiviral effectiveness than the synthetic peptide against influenza A/H1N1 pdm. Extracts from untransformed and transformed microalgae and synthetic peptide did not show cytotoxic effect on MDCK cell monolayers. Thus, C. reinhardtii may be a fast, safe, and effective expression platform for production of peptides with significant antiviral activity and can be used as a prophylactic treatment to reduce viral propagation.
Influenza infections are the main cause of respiratory diseases in the world. The morbidity associated with influenza is high and many cases require hospitalization. The World Health Organization (2019) estimates between 290,000 and 650,000 influenza-associated respiratory deaths per year (Iuliano et al., 2018). Currently, the influenza virus is considered a global threat because it can be spread easily and has the ability to affect all age groups. Worldwide, the main co-circulating strains are influenza viruses A H1N1 and A H3N2. These viruses are dangerous and have caused various pandemic events in human history (Webby et al., 2013; Center for Disease Control and Prevention, 2018).
The viruses have evasion mechanisms for the immune system; therefore, new pandemic strains can arise; for this reason, the influenza vaccines need to be redesigned annually and new control strategies are required (Koel et al., 2015). Currently there are two classes of antiviral drugs in order to treat the flu: adamantine derivatives (such as adamantine and rimantadine) and neuraminidase inhibitors (oseltamivir and zanamavir). However, point mutations in neuraminidase and hemagglutinin have been detected in the circulating strain, which confers high resistance to these antiviral drugs (Jones et al., 2006; L’Huillier et al., 2015; World Health Organization, 2019). This event also demonstrates the urgent need for development of new strategies in order to control the influenza virus infection such as use of antiviral peptides.
The entry blocker (EB) peptide is a 20-amino-acid peptide derived from the fibroblast growth factor 4 signal sequence. This peptide exhibits a broad spectrum of antiviral effects against influenza viruses A, which was tested in vitro assays (Jones et al., 2006). This peptide has the ability to impede the virus entry into the cell due to the fact that the EB peptide docks to binding pockets of hemagglutinin and diminishes the affinity for sialic acid receptors. Moreover, it has been observed that EB induces virus aggregation and diminished interaction with permissive cells for the virus entry (Jones et al., 2011a,b).
Therefore, the use of the EB peptide is a promising alternative therapy against influenza virus; however, the organic synthesis is expensive; thus, to use new platforms to produce recombinant proteins is an alternative. Currently, the microalga Chlamydomonas reinhardtii has stood out as an economic expression system; moreover, the nuclear or chloroplast genetic transformation is relatively fast and stable, and the produced protein shows correct folding and a complex assembly. Additionally, this microalgae is recognized as GRAS (Generally Recognized as Safe) category platform (Mayfield et al., 2003, 2007). The aim of the current work was to express the EB peptide in C. reinhardtii microalgae and test its antiviral activity in vitro against influenza A virus H1N1.
The strain CC-137 mt (+) of C. reinhardtii was transformed with vectors harboring the EB sequences. Microalgae cultures were cultivated in Tris-Acetate-Phosphate medium (TAP) either solid or liquid (120 rpm of orbital agitation) at 23°C with 16/8 h light/dark cycle.
The nucleotide sequence of the EB antiviral peptide was codon optimized for nuclear microalgae expression. The peptide design contains the Pr1a signal peptide (Sharma et al., 2000), an enterokinase protease site, a KDEL retention sequence, and a histidine tag. The EB nucleotide sequence was synthesized by GenScript Inc. (Piscataway, NJ, United States). This sequence was cloned into pChlamy_1 vector using XbaI and KpnI restriction sites (Figure 1). This construct was confirmed by PCR assay, restriction analysis, and sequencing.
Figure 1. Components of the transformation vector for EB expression in Chlamydomonas reinhardtii. EB gene sequence optimized for expression in Chlamydomonas reinhardtii cloned between KpnI and XbaI restriction sites into pChlamy_1 vector. The EB peptide amino acid sequence has additional components: Signal peptide: Pr1a sequence; EK site: Enterokinase protease site; 6his: six-histidine tag; KDEL: reticulum endoplasmic retention tag. The pChlamy vector has the following elements: Hsp70A-RbcS2 promoter, Int-RbcS2 is RuBisCO Small Subunit 2 intron, B2 tubulin promoter, and Aph7 gene from hygromycin resistance.
Agrobacterium tumefaciens GV3101 strain was transformed with EB-pChlamy construct by electroporation (25 μF, 2.4 kV, and 200 Ω in BTX 630 ECM Electroporator). The transforming bacteria were grown in LB (Luria Bertani) medium containing ampicillin (100 mg/L). Then, the transformed bacteria were corroborated by PCR.
The C. reinhardtii nuclear transformation was achieved by co-cultivation of microalgae with A. tumefaciens GV3101 strain, following the protocol described by Kumar et al. (2004). The putative transformants were kept in successive selective rounds in solid TAP medium containing hygromycin (15 mg/L). After several rounds of selection, the resistant clones were grown in TAP liquid medium.
The DNA was extracted of biomass collected from 15 ml of liquid culture from wild type (WT) and TAP-hygromycin-resistant microalgae according to Newman et al. (1990). The identification of EB transgene into the microalgae genome was confirmed by PCR. For nuclear detection of EB gene, the specific primers F-5' TGCTGCTGTTCCTGGTGAT 3' and R-5'GTGGTGCTTGTCGTCGTC 3' were used. The PCR conditions were as follows: 5 min of denaturalization at 95°C, followed by 30 cycles (95°C for 30 s, 62°C for 30 s, and 72°C for 30 s), and a final cycle of 72°C for 5 min. The PCR products were visualized in 1% agarose gel electrophoresis.
The transformed line and WT microalgae were grown in 1 L of liquid TAP medium, which was inoculated with a pre-inoculum of 100 ml of culture (O.D.700 = 0.3). After 7 days, the biomass was collected by centrifugation at 10,000 rpm at 4°C and lyophilized. The chlorophyll was removed from the dry biomass by washing with acetone, acetone:methanol (50:50), and methanol. The biomass was suspended in an extraction buffer (Milli Q water or 100 mM Tris-HCl, pH 8) containing 0.1% protease inhibitors cocktail (Sigma Aldrich, St. Louis, MO, United States). The biomass was sonicated at 30% of amplitude (4 cycles, 30 s each), centrifuged at 12,000 rpm for 10 min, and supernatant was collected. The protein concentration was measured at 205 nm using a nanodrop 2000 (Thermo Scientific Waltham, MA, United States) according to Altmann et al. (2009). The extracts were analyzed by Tricine 18% SDS-PAGE and 6 M urea and stained with silver nitrate.
The total soluble protein (TSP) from transformed and WT microalgae were analyzed by Western blot. Twenty micrograms of TSP was resolved in Tricine 18% SDS-PAGE under reducing conditions and transferred to polyvinylidene fluoride in a Turbo Transfer System (Bio-Rad, Hercules, CA, United States). The membrane was blocked with Tris-buffered saline and 0.04% Tween-20 (TBS-T) containing 2% hydrolyzed casein. Subsequently, the membrane was incubated with a mouse anti-EB peptide polyclonal antibody at 1:250 dilution in TBS-T for 2 h. Finally, membrane was incubated with an Anti-Mouse antibody conjugated to Horseradish Peroxidase (HRP; A5420 Sigma-Aldrich, St. Louis, MO, United States) at 1:4000 in TBS-T for 2 h. Specific antibody detection was performed using the PIERCE ECL-Western Blotting Substrate kit following the manufacturer instructions, and the signal was captured in X-ray films (Cytiva Amersham™ Hyperfilm™ ECL, Thermo Fisher Scientific, Waltham, MA, United States).
The content of the EB peptide in transformed and WT microalgae of TSP was determined by indirect ELISA. Microtiter plates were coated with 5 μg of TSP. A synthetic EB peptide (RRKKAAVALLPAVLLALLAP) synthesized by GenScript Inc. (Piscataway, NJ. United States) was used to construct a standard curve (1.9–500 ng). The plate was washed with TBS-T and subsequently blocked with hydrolyzed casein at 2% in TBS-T and incubated with a mouse anti-EB peptide polyclonal antibody at 1:250 dilution. After, wells were incubated an Anti-Mouse Alkaline Phosphatase antibody produced in rabbits (A1902; Sigma Aldrich, St. Louis, MO, United States) at 1:1000 dilution. Colorimetric reaction with 3 mM solution of p-nitrophenyl phosphate was read at 405 nm in íMar™ Microplaque Absorbance Reader (Bio-Rad, Hercules, CA, United States). The data were analyzed for one-way analysis of variance (p < 0.001 was considered as a statistically significant difference) with Tukey multiple comparisons test. Statistical analysis and graphics were made in GraphPad Prism 6 Software.
Madin Darby Canine Kidney cells (MDCK) were routinely cultured at 37°C in a humid 5% CO2 atmosphere and grown in Minimum Essential Medium (MEM) supplemented with 10% fetal bovine serum (FBS). A viability standard curve was made using 0 to 1.6 × 105 cells in 96-microwell plates. Cells were incubated with 10 μl of MTT [5 mg/ml 3-(4,5-dimethylthiazol-2-yl) bromide-2,5-diphenyltetrazole in PBS] for 4 h at 37°C with 5% CO2. Then, the medium was removed, and the formazan crystals were dissolved with 50 μl of DMSO and read at 490 nm in íMar™ Microplaque Absorbance Reader (Bio-Rad, Hercules, CA, United States).
The cytotoxicity assay for the EB peptide was carried out in 1 × 104 MDCK cells. The cells were incubated for 72 h with medium alone, medium containing the synthetic EB peptide, or transformed WT TSP (0 to 50 μg). Medium containing 4 M urea was used as a positive control of damage to the cell monolayer. Cytotoxic effect was determined after 72 h by the MTT reduction method (van Meerloo et al., 2011). The data were analyzed for two-way analysis of variance (p < 0.001 was considered as a statistically significant difference) with Dunnett’s multiple comparisons. Statistical analysis and graphics were made in GraphPad Prism 6 Software.
Influenza pdm A/H1N1 (Zuñiga et al., 2011) and Influenza A H1N1 Virginia/ATCC/2009 strains were propagated in MDCK cell line using MEM medium containing 0.5 μg/ml Trypsin TPCK and 0.3% BSA, at 37°C for 72 h, when cytopathic effect was observed. Subsequently, the supernatant was collected and frozen in liquid nitrogen. Virus titer was determined by hemagglutination assays (Balish et al., 2013).
Virus infectivity was determined by plaque formation assays and tissue culture infectious dose 50% assay (TCID50). The plaque assays were done in MDCK cellular monolayer, adding 200 μl per well of 10-fold dilution series of viral stock and it was internalized 1 h at 37°C. Low melting agarose 0.6%, MEM medium, 0.5 μg/ml Trypsin TPCK, and 0.3% BSA were used as an immobilization medium. Plaque formation was monitored under a microscope daily until visible cytopathic effect. Also, the TCID50 of viral stock was performed as recommended (Balish et al., 2013).
Inhibition of hemagglutination induced by the influenza pdm A/H1N1 and influenza A H1N1 Virginia/ATTC/2009 strains was determined for the EB microalgae extract and the EB synthetic peptide. The EB synthetic peptide and EB expressed in microalgae (concentration from 0 to 4000 nM) were incubated with 64 hemagglutination units (HA) of virus for 1 h at 37°C. The WT extracts (0.02 to 200 μg of protein) were used for control. Subsequently, 50 μl of human red blood cell (RBC) suspension (0.05%) was added and incubated for 1 h at 37°C. Each concentration was tested in triplicate. The percentage of inhibition obtained from three independent assays was analyzed in GraphPad Prism 6 Software. The IC50 was determined by a dose-response curve (Kawaoka and Neumann, 2012; Spackman, 2014).
One hundred TCID50 of influenza pdm A/H1N1 virus was mixed with 0 to 4 μM of the synthetic peptide EB and EB expressed in microalgae, for 1 h at 37°C. Additionally, as a control, 0.02 to 200 μg of protein from WT extracts (MQ and Tris) was mixed with the virus for 1 h at 37°C, to demonstrate that microalgae proteins do not have an inhibitory effect on viral replication. Each concentration of EB was analyzed in quadruple. Mixtures were added to MDCK cell monolayers and viral adsorption was allowed for 1 h at 37°C. Then, the inoculum was removed, and cells were washed with PBS and incubated in MEM medium, 0.3% BSA, and 0.5 μg/ml TPCK trypsin. The cytopathic effect was observed at 48 h post-infection. Supernatants were collected and viral particles were determined by hemagglutination. The result from two independent assays was analyzed in GraphPad Prism 6 Software. The IC50 for each peptide was determined for a dose-response curve (Kawaoka and Neumann, 2012; Spackman, 2014).
The gene encoding the EB peptide was cloned into pChlamy_1 vector and was confirmed by PCR and sequencing analysis. The C. reinhardtii was transformed by this construct using A. tumefaciens. The 10 putative transformed microalgae were grown in TAP-hygromycin for multiple generations. A 116-bp specific amplicon of EB transgene was detected in the nuclear genome of three resistant microalgae (Figure 2). No change in phenotype characteristics was observed in comparison with the WT microalgae (data not shown). Furthermore, the transgenic line a showed stable characteristics after 4,000 generations and was selected for further studies.
Figure 2. PCR assay for the detection of EB peptide gene in nucleus transformed microalgae. Amplification of an expected 116-bp product corresponding to the EB gene of nuclear transgenic lines (a, b, f), (Agr): Positive control EB-pChlamy_1 purified from Agrobacterium tumefaciens; (+): Positive control EB-pChlamy_1 purified from E. coli; (−): negative control; (WT): wild type strain DNA; (M) DNA molecular weight marker (100 bp ladder).
It was necessary to remove the chlorophyll from the EB peptide in soluble extract because the chlorophyll inhibits the detection by Western blot. The EB peptide was successfully extracted with Tris and Milli Q water buffers from transformed microalgae. The EB peptide expected band is observed in TSP from transformed microalgae by Tricine SDS-PAGE, showing a 3.4-kDa size, which was the predicted size by bioinformatics programs (ProtParam tool, Expasy); in addition, the EB peptide was not observed in TSP from WT (Figure 3A).
Figure 3. Detection of an estimated 3.4-kDa band in transformed microalgae, corresponding to the EB peptide. (A) Protein electrophoresis from transformed and wild-type microalgae. The EB peptide (3.4 kDa estimated size) is observed in Tris and Milli Q water extracts from transformed microalgae lines EB a and EB b, respectively. (B) Western blot EB peptide detection with an anti-EB antibody in microalgae extracts. Tris and MQ water EB a line extracts from transformed microalgae are shown as well as Tris and MQ water WT from wild-type microalgae extracts. (MQ): Milli Q water.
The presence of the EB peptide in the extracts was validated by Western blot using a primary anti-EB peptide polyclonal antibody. Figure 3B shows the 3.4-kDa band from both Milli Q water and Tris-transformed microalgae extracts, while in the WT microalgae extracts, no signal was detected.
The EB quantification in transformed microalgae was determined by ELISA (Figure 4); Milli Q water extracts contains 4.99 ± 0.067% of EB in TSP (EB MQ) and TRIS extracts have 3.96 ± 0.046% of EB in TSP (EB TRIS). Regarding the yield of recombinant peptide production, C. reinhardtii produce 1.95 mg of EB per gram of dry weight; therefore, this represents 0.19% of dry biomass after chlorophyll extraction.
Figure 4. ELISA quantification of EB peptide produced in transformed microalgae. The EB concentration was estimated in transformed (EB) microalgae extracts with a mouse anti-EB peptide polyclonal antibody, using wild-type (WT) microalgae as controls. The amount of EB was expressed in percentages of total soluble protein (TSP; ANOVA one way, p < 0.001) with Tukey multiple comparisons test (**** = 0.0001). (MQ): Milli Q water extracts; (TRIS): Tris Buffer extracts.
The peptide cytotoxicity was assayed on MDCK cells by changes in cell viability, using MTT reduction assay. The cell viability did not decrease in the presence of the EB synthetic peptide and the EB or the WT microalgae extracts. These groups had no significant differences with respect to the control (medium alone) in all concentrations tested (0.3, 3, and 30 μg of protein; Figure 5). Consequently, we tested higher concentrations of protein (>30 μg) and neither showed a cytotoxic effect to MDCK cells (data not shown).
Figure 5. The cell viability is not affected for EB extracts. The EB and WT extracts in Tris and Milli Q water and the EB synthetic peptide (0.03, 3, and 30 μg/ml) did not decrease cellular viability in comparison with medium control (p < 0.001, ANOVA). Dunnett’s multiple comparisons test (*** = 0.001; * = 0.1). The % cell viability was determined by MTT reduction. Urea (4 M) was used as a negative control of cell viability reduction, and MEM medium was used as a positive control of cell viability reduction. (MQ): Milli Q water extracts; (TRIS): Tris Buffer extracts.
The hemagglutination inhibition test allows one to determine if the EB peptide is capable of binding to the viral hemagglutinin and blocks the interaction with N-acetylmuramic acid of glycoproteins in RBCs. The dose-response curve exhibited that the EB extracts and synthetic peptide can inhibit in a dose-dependent manner the erythrocyte hemagglutination of both influenza pdm and Virginia strains. For the influenza pdm strain, the half maximal inhibitory concentration for binding to receptor was lower for EB expressed in microalgae (2.202 ± 1.027 nM for EB MQ extract and 9.582 ± 1.027 nM for EB Tris extract) than for the EB synthetic peptide (256.3 ± 1.03 nM; Figure 6A; Table 1). This indicates that EB produced in algae is 100-fold more effective than the synthetic peptide. Another interesting fact is that MQ and Tris WT extracts are not able to inhibit the binding between hemagglutinin and its receptor.
Figure 6. EB blocks the binding of viral hemagglutinin to its receptor. EB expressed in microalgae and synthetic EB inhibited the viral hemagglutination. Dose-response curves of each peptide show the ability of EB to prevent viral hemagglutinin binding to sialic acid in red blood cell membrane. (A) EB inhibitory effects vs. influenza pdm A/H1N1 strain. (B) EB inhibitory effects vs. influenza A H1N1 Virginia/ATTC/2009 strain. The graph shows the mean hemagglutination inhibition in percentage for each EB ± SD. The results belong to three independent assays. (MQ): Milli Q water extracts; (TRIS): Tris Buffer extracts.
In the inhibition hemagglutination assay of influenza Virginia strain, the EB tris extract showed an IC50 of 2.338 ± 1.032 nM and EB MQ extract has 4.388 ± 1.01 nM, while the EB synthetic peptide showed an IC50 value of 613.8 ± 1.043 nM (Figure 6B; Table 1). Again, the EB peptide expressed in algae is more effective than the synthetically produced peptide against this influenza strain. We observed that WT microalgae extracts do not show the capacity for protection against the sialic acid-hemagglutinin binding.
The viral replication inhibition assay allows us to assess the ability of EB to inhibit the influenza pdm entry into MDCK cells. The first observation was 100 TCID50 of virus, and it caused a strong cytopathic effect that destroyed the entire cell monolayer at 48 h post-infection and produced new viral particles. The dose-response curve exhibits a reduction in viral infection when the EB amount increases (Figure 7). Both EB expressed in microalgae and the EB synthetic peptide inhibited viral replication, but comparing the IC50 between peptides, we determined that 36 times less amount of EB extracts (20.71 ± 1.03 nM) than the EB synthetic peptide (754.4 ± 1.02 nM) is necessary to inhibit the viral replication (Table 2). It can be concluded that EB prevents virus replication because we did not detect new viral particles (by hemagglutination assay) in the supernatant in wells without cytopathic effect. It is important to highlight that although the IC50 values are lower than those reported by Jones et al. (2006), here we used human RBCs instead of chicken RBCs, which can affect the hemagglutination activity of viruses, since there are specific expression patterns in 2,3‐ and 2,6-linked sialic acid moieties on RBC surfaces (Eisfeld et al., 2014). In addition, we used different multiplicity of infection (MOI) amounts (0.001 vs. 0.01).
Figure 7. Antiviral effect of EB synthetic and expressed in microalgae. EB expressed in microalgae and synthetic EB can inhibit viral replication of H1N1 A pdm in MDCK cells. Dose-response curves of each peptide show the ability of EB to prevent the replication of 100 TCID50 of Influenza pdm A/H1N1. In the graph, mean percentage ± SD of viral replication inhibition for each EB concentration is appreciated. The results belong to two independent assays. (MQ): Milli Q water extracts; (TRIS): Tris Buffer extracts.
Viruses have taken an unprecedented importance in Global Public Health, provoking human and economic losses and urging humanity to find solutions through the study of viruses, especially those that have a high mutation rate or a high transmission rate. The influenza virus is a pathogen that recurrently affects respiratory systems, and due to its special characteristics of genetic diversity mechanisms (such as rearrangement, recombination, and RNA polymerase errors), new resistant strains can arise (Webby et al., 2013). These situations thrust the importance of generating new antiviral drugs.
Currently, the most circulating influenza strains are resistant to adamantine and neuraminidase inhibitors. For this reason, other therapeutic targets are under investigation. One central point in the viral infection process is the binding of viral hemagglutinin to cell receptors. An excellent candidate to inhibit the binding is the EB peptide, by blocking the hemagglutinin-sialic acid interaction. In order to reduce production costs, we decided to express this peptide in C. reinhardtii. These microalgae have a great potential in biopharmaceuticals production, such as antibodies, growth factors, and enzymes because this platform production does not require a strict purification process, because it does not contain toxic elements for humans. This implies 80% cost reduction in the production process (Chen, 2008; Rosales-Mendoza et al., 2020).
C. reinhardtii has proven to be an effective platform for recombinant protein production reaching 0.02–2% TSP and a yield of 0.3–3 mg/L per culture medium in the case of expression in chloroplast (Rosales-Mendoza, 2013; Gimpel et al., 2015). Similarly, it is possible to express heterologous genes in the nuclear genome, but enhancing elements need to be used (Rasala et al., 2012). The EB peptide gene was codon optimized for expression in the nuclear genome of the microalgae. This avoids a depletion of the transcript because microalgae has an unusual use of codons with high GC content (>61%) and avoids heterochromatization (León and Galván, 2007). In the design of the EB peptide, the signal peptide Pr1a was added, which directs foreign proteins to the secretory pathway and, together with C-terminal KDEL sequence, prevents secretion of luminal ER proteins, therefore increasing the accumulation of recombinant proteins (Munro and Pelham, 1987; Sharma et al., 2000; Ziegler et al., 2000; Potvin and Zhang, 2010); this is an important approach to minimize foreign protein degradation by cytoplasmic proteases present in microalgae (Surzycki et al., 2009), since it keeps the recombinant protein bound to receptors into the inner membrane reticulum. Hempel et al. (2011) and Hempel and Maier (2012) demonstrated that there is an accumulation in the endoplasmic reticulum of Phaeodactylum tricornatum of an antibody against hepatitis B when an ER retention tag is added, and it prevented the export to the culture medium.
The maximum concentration of the EB peptide was obtained in aqueous extract reaching a yield of 4.99% of TSP. This high value is due to the endogenous proteins having less capacity to dissolve in water and remaining in the insoluble fraction. Therefore, the extraction microenvironment allows the EB peptide to have more access to the solvent; thus, the amount of EB is higher vs. endogenous proteins. The EB peptide yield represents 0.19% of dry biomass, which is higher than other biopharmaceutical products produced in microalgae. For example, Barahimipour et al. (2016) expressed the HIV antigen (P24) under the HSP70/RBCS2 fused promoter by nuclear transformation, and the yield was 0.25% of TSP (Barahimipour et al., 2016). The expression of erythropoietin has also been achieved with lower yield than in our work, rendering up to 0.03% of dry weight, in a work that includes the use of RBCS2 introns, which increase the transgene expression because the introns promote mRNA secondary structures causing higher efficiency of RNA polymerase II and longer messenger half-life (Eichler-Stahlberg et al., 2009). The differences in performance of recombinant protein production are not exclusive to microalgae, because protein accumulation is linked to stability of the messengers and the efficiency in the translation of mRNAs (Rasala et al., 2010).
Microalgae consumption in rats and humans has not shown toxicity; nevertheless, they have shown multiple benefits for gastrointestinal health (Tran et al., 2013; Endres and Murbach, 2018; Fields et al., 2020). Chávez et al. (2020) demonstrated that human pro-angiogenic growth factors (hVEGF-165, hPDGF-B, and hSDF-1) expressed in C. reinhardtii had the capacity of stimulating specific cellular processes without cytotoxic effect in mammalian cells. EB and WT extracts do not cause damage to MDCK cells; therefore, the EB peptide can be used as a prophylactic treatment to prevent viral spread in the respiratory tract.
Our results suggest that the EB peptide expressed in microalgae and also the synthetic peptide interact with viral hemagglutinin, avoiding binding with sialic acid site; therefore, the EB peptide prevents the first interaction between the virus and cell. Downard et al. (2016) reported that the EB peptide can stoichiometrically block the acid sialic binding sites. We observed that both peptides have wide protection ranges and a dose-dependent inhibitory effect, as reported by Jones et al. (2006). The effectiveness of the EB peptide expressed in microalgae is higher than the synthetic peptide against Pandemic and Virginia influenza strains. Different concentrations of the EB peptide are required for different viral strain inhibition. This effect was already reported by Jones et al. (2006), who reported that different glycosylation patterns of HA may have a slight effect in the interaction with EB. Therefore, additional experiments need to be performed for different influenza subtypes.
One goal of the antivirals is to prevent production of new infectious particles. The EB peptide’s efficiency to inhibit viral replication was demonstrated during the viral particle binding and internalization process (Downard et al., 2016). EB expressed in microalgae was able to inhibit viral replication with less amount than the synthetic peptide. This result is interesting because both peptides share the same functional consensus sequence RRKKLAVLLALLAP; they are hydrophobic amino acid clusters capable of interacting with the HA binding site (Bultmann et al., 2010; Jones et al., 2011a); however, the EB peptide expressed in microalgae has 10 amino acids more than the synthetic one (Enterokinase site and His-tag), which may provide more stability than the EB peptide alone. Another reason is that the synthetic EB peptide is highly hydrophobic and can render auto assembly (Bultmann et al., 2010), which compromises its bioavailability.
The soluble extract containing EB has an advantage regarding the synthetic peptide. This is the microenvironment of endogenous components of microalgae, which offers protection against degradation by adding trypsin in infection medium by virus processing; therefore, the peptide from microalgae water extract has an extended lifetime. The protective effect against mucosal protease degradation has been reported for biopharmaceuticals expressed in C. reinhardtii (Ochoa-Méndez et al., 2016; Carrizalez-López et al., 2018). Additionally, microalgae extracts have been shown to contain components enhancing biological activity, anti-inflammatory, and immunomodulatory properties (Rosales-Mendoza, 2013; Rosales-Mendoza et al., 2020). It will be necessary to prove the antiviral efficacy of EB peptide in an in vivo model.
C. reinhardtii has proven to be a good biopharmaceutical expression platform. We demonstrated that the EB peptide is expressed at high levels in transformed microalgae and can be extracted as part of total soluble fraction. We suggested that post-translational modifications conferred for microalgae to the EB peptide and the additional amino acids increased efficiency vs. the EB synthetic peptide. Our results show that microalgae as a platform can help us to improve the effectiveness of the EB peptide to inhibit flu virus replication. This promising result suggests that we can help to reduce the health burden caused by respiratory viral infections with antiviral peptides expressed in microalgae systems, which may reduce the spread of emerging viruses.
The original contributions presented in the study are included in the article/supplementary material; further inquiries can be directed to the corresponding author.
KR-B, AA-S, RS-G, LH, NS-J, CC-G, and RL-M designed the study. KR-B, LH, NS-J, and RL-M performed the experiments and collected the data. KR-B, AA-S, RS-G, LH, NS-J, RL-M, and CC-G analyzed, discussed, and interpreted the data. KR-B wrote the first manuscript and all co-authors contributed with substantive comments. LH and AA-S supervised the work and obtained the funding. All authors contributed to the article and approved the submitted version.
We thank CONACYT for the scholarship 331786 given to KR-B and for Grant No. 257528.
The authors declare that the research was conducted in the absence of any commercial or financial relationships that could be construed as a potential conflict of interest.
We want to thank our colleagues at the Plant Molecular Biology Laboratory at IPICYT, the Molecular Biotechnology of Plant Cells Laboratory at UASLP, and B108 Laboratory of the Immunology Department at UNAM. We appreciate technical support from MC Erica Segura Salinas and MC Onasis Vicente Fermín in BSL3 High Security Laboratory in UNAM, México. We also acknowledge the Servicio de Medicina Transfuncional at Instituto Nacional de Ciencias Médicas y Nutrición Salvador Zubirán, México, for the donation of red blood cells from healthy people and LANBAMA-IPICYT for the facilities provided.
Altmann, S. E., Jones, J. C., Schultz-Cherry, S., and Brandt, C. R. (2009). Inhibition of Vaccinia virus entry by a broad spectrum antiviral peptide. Virology 388, 248–259. doi: 10.1016/j.virol.2009.03.023
Balish, A. L., Kat, J. M., and Klimov, A. I. (2013). Influenza: Propagation, quantification, and storage. Curr. Protoc. Microbiol. 29, 15G.1.1–15G.1.24. doi: 10.1002/9780471729259.mc15g01s29
Barahimipour, R., Neupert, J., and Bock, R. (2016). Efficient expression of nuclear transgenes in the green alga Chlamydomonas: synthesis of an HIV antigen and development of a new selectable marker. Plant Mol. Biol. 90, 403–418. doi: 10.1007/s11103-015-0425-8
Bultmann, H., Girdaukas, G., Kwon, G. S., and Brandt, C. R. (2010). The virucidal EB peptide protects host cells from herpes simplex virus type 1 infection in the presence of serum albumin and aggregates proteins in a detergent-like manner. Antimicrob. Agents Chemother. 54, 4275–4289. doi: 10.1128/AAC.00495-10
Carrizalez-López, C., González-Ortega, O., Ochoa-Méndez, C. E., Galván-Moreno, F. U., Rosales-Mendoza, S., Monreal-Escalante, E., et al. (2018). Expression of multiple antihypertensive peptides as a fusion protein in the chloroplast of Chlamydomonas reinhardtii. J. Appl. Phycol. 30, 1701–1709. doi: 10.1007/s10811-017-1339-4
Center for Disease Control and Prevention (2018). 2018-2019 ACIP Background. IOP publishing. Available at: https://www.cdc.gov/flu/professionals/acip/2018-2019/background/background%20epidemiology.htm (Accessed February 26, 2019).
Chávez, M. N., Centeno-Cerdas, C., Bohne, A., Hopfner, U., Machens, H., Egaña, J. T., et al. (2020). Towards a biotechnological platform for the production of human pro-angiogenic growth factors in the green alga Chlamydomonas reinhardtii. Appl. Microbiol. Biotechnol. 104, 725–739. doi: 10.1007/s00253-019-10267-6
Chen, Q. (2008). Expression and purification of pharmaceutical proteins in plants. Biol. Eng. Trans. 1, 291–321. doi: 10.13031/2013.26854
Downard, K. M., Lu, R., and Mu, P. (2016). Molecular basis of influenza hemagglutinin inhibition with an entry-blocker peptide by computational docking and mass spectrometry. Antivir. Chem. Chemother. 24, 109–117. doi: 10.1177/2040206615622920
Eichler-Stahlberg, A., Weisheit, W., Ruecker, O., and Heitze, M. (2009). Strategies to facilitate transgene expression in Chlamydomonas reinhardtii. Planta 229, 873–883. doi: 10.1007/s00425-008-0879-x
Eisfeld, A. J., Neumann, G., and Kawaoka, Y. (2014). Influenza a virus isolation, culture and identification. Nat. Protoc. 9, 2663–2681. doi: 10.1038/nprot.2014.180
Endres, J. R., and Murbach, T. S. (2018). A toxicological evaluation of Chlamydomonas reinhardtii, a green algae. Int. J. Toxicol. 37, 53–62. doi: 10.1177/1091581817746109
Fields, F. J., Lejzerowicz, F., Schroeder, D., Ngoi, S. M., Tran, M., McDonald, D., et al. (2020). Effects of the microalgae Chlamydomonas on gastrointestinal health. J. Funct. Foods 65:103738. doi: 10.1016/j.jff.2019.103738
Gimpel, J. A., Hyun, J. S., Schoepp, N. G., and Mayfield, S. P. (2015). Production of recombinant proteins in microalgae at pilot greenhouse scale. Biotechnol. Bioeng. 112, 339–345. doi: 10.1002/bit.25357
Hempel, F., Lau, J., Klingl, A., and Maier, U. G. (2011). Algae as protein factories: expression of a human antibody and the respective antigen in the diatom Phaeodactylum tricornutum. PLoS One 6:e28424. doi: 10.1371/journal.pone.0028424
Hempel, F., and Maier, U. G. (2012). An engineered diatom acting like a plasma cell secreting human IgG antibodies with high efficiency. Microb. Cell Factories 11:126. doi: 10.1186/1475-2859-11-126
Iuliano, A. D., Roguski, K. M., Chang, H. H., Muscatello, D. J., Palekar, R., Tempia, S., et al. (2018). Estimates of global seasonal influenza-associated respiratory mortality: a modelling study. Lancet 391, 1285–1300. doi: 10.1016/S0140-6736(17)33293-2
Jones, J. C., Settles, E. W., Brandt, C. R., and Schultz-Cherry, S. (2011a). Identification of the minimal active sequence of an anti-influenza virus peptide. Antimicrob. Agents Chemother. 55, 1810–1813. doi: 10.1128/AAC.01428-10
Jones, J. C., Settles, E. W., Brandt, C. R., and Schultz-Cherry, S. (2011b). Virus aggregating peptide enhances the cell-mediated response to influenza virus vaccine. Vaccine 29, 7696–7703. doi: 10.1016/j.vaccine.2011.07.133
Jones, J. C., Turpin, E. A., Bultmann, H., Brandt, C. R., and Schultz-cherry, S. (2006). Inhibition of influenza virus infection by a novel antiviral peptide that targets viral attachment to cells. J. Virol. 80, 11960–11967. doi: 10.1128/JVI.01678-06
Kawaoka, Y., and Neumann, G. (2012). Influenza Virus. Methods and Protocols. New york: Humana Press.
Koel, B. F., Mögling, R., Chutinimitkul, S., Fraaij, P. L., Burke, D. F., van der Vliet, S., et al. (2015). Identification of amino acid substitutions supporting antigenic change of influenza a(H1N1)pdm09 viruses. J. Virol. 89, 3763–3775. doi: 10.1128/JVI.02962-14
Kumar, S. V., Misquitta, R. W., Reddy, V. S., Rao, B. J., and Rajam, M. V. (2004). Genetic transformation of the green alga ‐ Chlamydomonas reinhardtii by Agrobacterium tumefaciens. Plant Sci. 166, 731–738. doi: 10.1016/j.plantsci.2003.11.012
L’Huillier, A. G., Abed, Y., Petty, T. J., Cordey, S., Thomas, Y., Bouhy, X., et al. (2015). E119D neuraminidase mutation conferring pan-resistance to neuraminidase inhibitors in an a(H1N1)pdm09 isolate from a stem-cell transplant recipient. J. Infect. Dis. 212, 1726–1734. doi: 10.1093/infdis/jiv288
León, R., and Galván, A. F. E. (Eds.) (2007). Transgenic Microalgae as Green Cell Factories. Advances in Experimental Medicine and Biology. New York, NY: Springer.
Mayfield, S. P., Franklin, S. E., and Lerner, R. A. (2003). Expression and assembly of a fully active antibody in algae. Proc. Natl. Acad. Sci. 100, 438–442. doi: 10.1073/pnas.0237108100
Mayfield, S. P., Manuell, A. L., Chen, S., Wu, J., Tran, M., Siefker, D., et al. (2007). Chlamydomonas reinhardtii chloroplasts as protein factories. Curr. Opin. Biotechnol. 18, 126–133. doi: 10.1016/j.copbio.2007.02.001
Munro, S., and Pelham, H. R. B. (1987). A C-terminal signal prevents secretion of luminal ER proteins. Cell 48, 899–907. doi: 10.1016/0092-8674(87)90086-9
Newman, S. M., Boynton, J. E., Gillham, N. W., Randolph-Anderson, B. L., Johnson, A. M., and Harris, E. H. (1990). Transformation of chloroplast ribosomal RNA genes in Chlamydomonas: molecular and genetic characterization of integration events. Genetics 126, 875–888. doi: 10.1093/genetics/126.4.875
Ochoa-Méndez, C. E., Lara-Hernández, I., Martínez-Gonzáles, L., Aguirre-Bañuelos, P., Ibarra-Barajas, M., Castro-Moreno, P., et al. (2016). Bioactivity of an antihypertensive peptide expressed in Chlamydomonas reinhardtii. J. Biotechnol. 240, 76–84. doi: 10.1016/j.jbiotec.2016.11.001
Potvin, G., and Zhang, Z. (2010). Strategies for high-level recombinant protein expression in transgenic microalgae: a review. Biotechnol. Adv. 28, 910–918. doi: 10.1016/j.biotechadv.2010.08.006
Rasala, B. A., Lee, P. A., Shen, Z., Briggs, S. P., Mendez, M., and Mayfield, S. P. (2012). Robust expression and secretion of xylanase1 in Chlamydomonas reinhardtii by fusion to a selection gene and processing with the FMDV 2A peptide. PLoS One 7:e43349. doi: 10.1371/journal.pone.0043349
Rasala, B. A., Muto, M., Lee, P. A., Jager, M., Cardoso, R. M. F., Behnke, C. A., et al. (2010). Production of therapeutic proteins in algae, analysis of expression of seven human proteins in the chloroplast of Chlamydomonas reinhardtii. Plant Biotechnol. J. 8, 719–733. doi: 10.1111/j.1467-7652.2010.00503.x
Rosales-Mendoza, S. (2013). Future directions for the development of Chlamydomonas -based vaccines. Expert Rev. Vaccine. 12, 1011–1019. doi: 10.1586/14760584.2013.825455
Rosales-Mendoza, S., Solís-Andrade, K. I., Márquez-Escobar, V. A., González-Ortega, O., and Bañuelos-Hernandez, B. (2020). Current advances in the algae-made biopharmaceuticals field. Expert. Opin. Biol. Ther. 20, 751–766. doi: 10.1080/14712598.2020.1739643
Sharma, A., Sharma, R., Imamura, M., and Yamakawa, M. Y. H. M. (2000). Transgenic expression of cecropin B, an antibacterial peptide from Bombyx mori, confers enhanced resistance to bacterial leaf blight in rice. FEBS Lett. 484, 7–11. doi: 10.1016/S0014-5793(00)02106-2
Surzycki, R., Greenham, K., Kitayama, K., Dibal, F., Wagner, R., Rochaix, J., et al. (2009). Factors affecting expression of vaccines in microalgae. Biologicals 37, 133–138. doi: 10.1016/j.biologicals.2009.02.005
Tran, M., Henry, R. E., Siefker, D., Van, C., Newkirk, G., Kim, J., et al. (2013). Production of anti-cancer immunotoxins in algae: ribosome inactivating proteins as fusion partners. Biotechnol. Bioeng. 110, 2826–2835. doi: 10.1002/bit.24966
van Meerloo, J., Kaspers, G. J. L., and Cloos, J. (2011). “Cell Sensitivity Assays: the MTT Assay,” in Cancer Cell Culture. Methods in Molecular Biology (Methods and Protocols). ed. I. Cree (New York: Humana Press).
Webby, R. J., Aktories, K., Freiburg, L., and Cooper, M. D. (2013). Current Topics in Microbiology and Immunology Vol. 370: Swine Influenza. Available at: http://www.springer.com/series/82 (Accessed December 1, 2020)
World Health Organization (2019). Influenza. IOP publishing. Available at: https://www.who.int/influenza/en/ (Accessed February 26, 2019).
Ziegler, M. T., Thomas, S. R., and Danna, K. J. (2000). Accumulation of a thermostable endo-1,4-β-D-glucanase in the apoplast of Arabidopsis thaliana leaves. Mol. Breed. 6, 37–46. doi: 10.1023/A:1009667524690
Keywords: antiviral peptide, microalgae, nuclear expression, biopharma, hemagglutinin
Citation: Reyes-Barrera KL, Soria-Guerra RE, López-Martínez R, Huerta L, Salinas-Jazmín N, Cabello-Gutiérrez C and Alpuche-Solís ÁG (2021) The Entry Blocker Peptide Produced in Chlamydomonas reinhardtii Inhibits Influenza Viral Replication in vitro. Front. Plant Sci. 12:641420. doi: 10.3389/fpls.2021.641420
Received: 14 December 2020; Accepted: 23 March 2021;
Published: 12 May 2021.
Edited by:
Rima Menassa, Agriculture and Agri-Food Canada, CanadaReviewed by:
Kaushal Kumar Bhati, Catholic University of Louvain, BelgiumCopyright © 2021 Reyes-Barrera, Soria-Guerra, López-Martínez, Huerta, Salinas-Jazmín, Cabello-Gutiérrez and Alpuche-Solís. This is an open-access article distributed under the terms of the Creative Commons Attribution License (CC BY). The use, distribution or reproduction in other forums is permitted, provided the original author(s) and the copyright owner(s) are credited and that the original publication in this journal is cited, in accordance with accepted academic practice. No use, distribution or reproduction is permitted which does not comply with these terms.
*Correspondence: Ángel Gabriel Alpuche-Solís, YWxwdWNoZUBpcGljeXQuZWR1Lm14
Disclaimer: All claims expressed in this article are solely those of the authors and do not necessarily represent those of their affiliated organizations, or those of the publisher, the editors and the reviewers. Any product that may be evaluated in this article or claim that may be made by its manufacturer is not guaranteed or endorsed by the publisher.
Research integrity at Frontiers
Learn more about the work of our research integrity team to safeguard the quality of each article we publish.