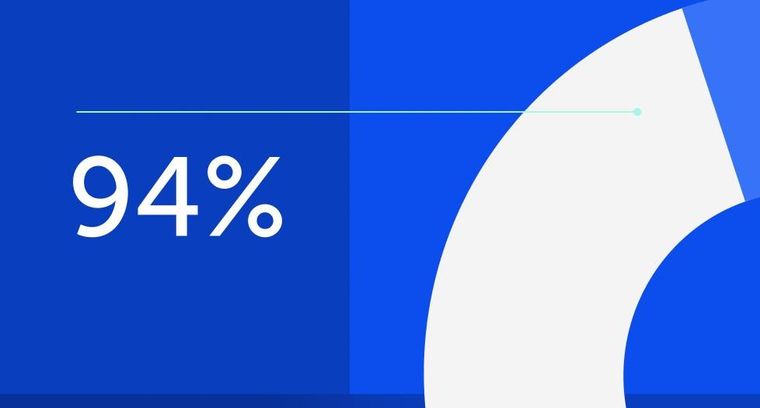
94% of researchers rate our articles as excellent or good
Learn more about the work of our research integrity team to safeguard the quality of each article we publish.
Find out more
ORIGINAL RESEARCH article
Front. Plant Sci., 09 March 2021
Sec. Plant Proteomics and Protein Structural Biology
Volume 12 - 2021 | https://doi.org/10.3389/fpls.2021.635962
This article is part of the Research TopicPlant Glycobiology - A Sweet World of Glycans, Glycoproteins, Glycolipids, and Carbohydrate-Binding ProteinsView all 33 articles
Complex N-glycan modification of secretory glycoproteins in plants is still not well understood. Essential in animals, where a lack of complex N-glycans is embryo-lethal, their presence in plants seemed less relevant for a long time mostly because Arabidopsis thaliana cgl1 mutants lacking N-acetyl-glucosaminyltransferase I (GNTI, the enzyme initiating complex N-glycan maturation in the Golgi apparatus) are viable and showed only minor impairments regarding stress tolerance or development. A different picture emerged when a rice (Oryza sativa) gntI T-DNA mutant was found to be unable to reach the reproductive stage. Here, we report on tomato (Solanum lycopersicum) lines that showed severe impairments upon two RNA interference (RNAi) approaches. Originally created to shed light on the role of core α1,3-fucose and β1,2-xylose residues in food allergy, plants with strongly reduced GNTI activity developed necrotic fruit-attached stalks and early fruit drop combined with patchy incomplete ripening. Correspondingly, semiquantitative RT-PCR of the abscission zone (az) revealed an increase of abscission markers. Also, GNTI-RNA interference (RNAi) plants were more susceptible to sporadic infection. To obtain vital tomatoes with comparable low allergenic potential, Golgi α-mannosidase II (MANII) was chosen as the second target. The resulting phenotypes were oppositional: MANII-reduced plants carried normal-looking fruits that remained attached for extended time without signs of necrosis. Fruits contained no or only few, but enlarged, seeds. Furthermore, leaves developed rolled-up rims simultaneously during the reproductive stage. Trials to cross MANII-reduced plants failed, while GNTI-reduced plants could be (back-)crossed, retaining their characteristic phenotype. This phenotype could not be overcome by ethephon or indole-3-acetic acid (IAA) application, but the latter was able to mimic patchy fruit ripening in wild-type. Phytohormones measured in leaves and 1-aminocyclopropane-1-carboxylic acid (ACC) contents in fruits showed no significant differences. Together, the findings hint at altered liberation/perception of protein-bound N-glycans, known to trigger auxin-like effects. Concomitantly, semiquantitative RT-PCR analysis revealed differences in auxin-responsive genes, indicating the importance of complex N-glycan modification for hormone signaling/crosstalk. Another possible role of altered glycoprotein life span seems subordinate, as concluded from transient expression of Arabidopsis KORRIGAN KOR1-GFP fusion proteins in RNAi plants of Nicotiana benthamiana. In summary, our analyses stress the importance of complex N-glycan maturation for normal plant responses, especially in fruit-bearing crops like tomato.
Plants are considered a safe and cost-effective way to produce therapeutic glycoproteins (Gomord et al., 2005; Schoberer and Strasser, 2018). N-glycosylation determines the physicochemical properties of glycoproteins, rendering this posttranslational modification indispensable for the functionality of most secretory proteins (Bosques et al., 2004; Gomord and Faye, 2004) and finally plant vitality (Lerouge et al., 1998; Strasser, 2016; Nagashima et al., 2018). The initial steps of N-glycan synthesis and protein attachment occur at/within the endoplasmic reticulum (ER). These basic steps are highly conserved in all eukaryotes due to folding assistance by lectin chaperones in the ER lumen (reviewed in Howell, 2013). Differences among taxa and species occur during N-glycan maturation, i.e., the conversion of ER-type high mannose to complex-type N-glycans in the Golgi apparatus (Varki et al., 2009). In animal and plant cells, in contrast to unicellular yeasts or microalgae (Mócsai et al., 2020), the initial N-glycan modifications in the Golgi stacks are similar (Varki, 2017). By the sequential action of several glycosyltransferases and glycosylhydrolases, N-glycans with less mannose but additional sugar moieties are formed (Kornfeld and Kornfeld, 1985). First, in the cis-Golgi, α-mannosidase I (Golgi MANI) removes terminal mannoses from the high mannose ER structure, resulting in Man5GlcNAc2∼Asn N-glycans (Kajiura et al., 2010). Then, N-acetyl-glucosaminyltransferase I (GNTI) adds an N-acetylglucosamine (GlcNAc) residue to the trimmed α1,3 arm (Harpaz and Schachter, 1980; Oppenheimer and Hill, 1981). Although it was reported that also abrogation of ER mannosidase I activity can lead to complex-type fucosylated and xylosylated N-glycans in the Golgi apparatus (Liebminger et al., 2009), no further N-glycan modification occurs when GNTI is missing. This was confirmed for the genetic model plant Arabidopsis by isolation and characterization of the first complex glycan-less1 (cgl1) mutant lines (von Schaewen et al., 1993; Frank et al., 2008).
Downstream of GNTI, Golgi MANII removes two mannoses from the α1,6 arm (van den Elsen et al., 2001; Strasser et al., 2006; Shah et al., 2008), before N-acetyl-glucosaminyltransferase II (GNTII) can add one GlcNAc in place. Regardless of prior action of Golgi MANII (Kornfeld and Kornfeld, 1985; Lerouge et al., 1998; Bencúr et al., 2005; Strasser et al., 2006; Kaulfürst-Soboll et al., 2011b), xylose and core fucose residues may be added by β1,2-xylosyltransferase (XYLT) (Strasser et al., 2000) and α1,3-fucosyltransferase, the latter being encoded by two genes in Arabidopsis, FUCTa/FUT11 and FUCTb/FUT12 (Wilson et al., 2001; Bondili et al., 2006). Further decoration of the terminal GlcNAc residues leads to the formation of Lewis-a epitopes (Léonard et al., 2002) via sequential action of β1,3-galactosyltransferase (GALT1) and α1,4-fucosyltransferase FUCTc/FUT13 (in Arabidopsis; Strasser et al., 2007). This bulky modification seems to occur on glycoproteins destined for the plasma membrane/apoplast, but not on those targeted to the tonoplast/vacuole (Takahashi et al., 1986; Fitchette-Lainé et al., 1997). Abundance of Lewis-a epitopes varies among different plant species and tissues (Wilson et al., 2001; Strasser et al., 2007).
Complex N-glycans of plant origin differ from those in mammals by their core α1,3-fucose linkage (also present in insects and certain helminths) and β1,2-xylose attached to the branching mannose (also found in helminths and some snails). In the medical literature, especially in the field of allergy, these epitopes are known as cross-reactive carbohydrate determinants (CCDs). Based on their ability to be bound by specific immunoglobulin E (IgE) antibodies, regardless of the origin, they appear to react similarly to pan-allergens, although in this case without clinical symptoms (Aalberse et al., 1981; reviewed by Altmann, 2007). However, crops are an essential part of the human diet, and oral intake/digestion of plant glycoproteins is usually well tolerated and causes no problems. Nevertheless, CCD-specific IgE antibodies are especially abundant in humans with pollen and/or hymenoptera (bee/wasp) allergy, where they are able to activate basophilic granulocytes (Mertens et al., 2010; Kaulfürst-Soboll et al., 2011a; and the references cited therein). Immunogenicity becomes more problematic with respect to the parenteral administration of glycoproteins containing non-human carbohydrate structures (Gomord et al., 2005; Walsh and Jefferis, 2006). This became evident when patients responded with severe acute symptoms to the injection of cetuximab (Erbitux®), a chimeric mouse–human monoclonal antibody carrying the non-primate glycan epitope Gal-α1,3-Gal of mouse, common with meat from cows and pigs, but also present in cats, dogs, and rats (Chung et al., 2008).
In order to overcome the risk of hypersensitive immune reactions connected with plant-made pharmaceuticals (PMPs), much effort went into altering the modification of N-glycans in different plant hosts (Saint-Jore-Dupas et al., 2004). First, retention within the ER was tested as a solution to protect glycoproteins from receiving core fucose or xylose residues in the Golgi. Fusion of the amino-acid motif H/KDEL to the C-terminus was found sufficient for ER retention of candidate proteins (Denecke et al., 1992) by carrying mostly high mannose N-glycans with eight to nine Man residues (Pagny et al., 2000). But since retention of heterologous proteins in the ER depends on the efficiency of the retrieval machinery (bringing proteins back from the Golgi apparatus), some glycoproteins were still found to be modified with complex-type N-glycans (e.g., cell wall invertase; Pagny et al., 2000). Nevertheless, plant-made antiviral antibodies were shown to be as functional as their mammalian counterparts- yet, with shorter half-life due to a higher clearance rate by mannose receptors in mammals (Engering et al., 1997; Ko et al., 2003).
Another approach to reduce the immunogenic potential of PMPs is the knockout or silencing of plant glycosyltransferases or glycosylhydrolases. Besides eliminating core fucose and xylose by a mutant combination or the engineering of Nicotiana benthamiana plants (Strasser et al., 2004, 2008), Arabidopsis complex glycan-less1 (cgl1 = gntI) produces mostly glycoproteins with mannose-terminating N-glycans of Man5GlcNAc2∼Asn structure (von Schaewen et al., 1993). Feasibility to produce an active human enzyme for therapeutic treatment of a congenital genetic disorder was proven by heterologous expression of glucocerebosidase in Arabidopsis cgl1 seeds (Downing et al., 2006). However, Arabidopsis is a small weed and usually not well suited for obtaining high yields, therefore gene-silencing approaches were pursued to suppress GNTI activity in crops. First approaches demonstrated moderate success: GNTI silencing by antisense approaches in potato and tobacco resulted in a clear, age-dependent reduction of the complex N-glycan pattern (Wenderoth and von Schaewen, 2000), but sometimes without visible changes (Strasser et al., 2004). Better suppression was later obtained by double strand-based RNA interference (dsRNAi) in potato and tomato (Kaulfürst-Soboll et al., 2011a).
Although knockout or silencing of GNTI is an efficient tool to reduce the allergenic potential of plant-specific N-glycan epitopes, it turned out to be disadvantageous for the plant itself. In Arabidopsis, lack of/reduced complex N-glycan formation resulted in increased salt sensitivity, showing as compromised root growth (Frank et al., 2008; Kang et al., 2008). Besides, an extended flowering period, reported by Boyes et al. (2001), and susceptibility to sporadic pathogen attack, already observed for Arabidopsis cgl1 (von Schaewen et al., 1993), was later also found for tomato GNTI-RNAi plants (Kaulfürst-Soboll et al., 2011a). More drastic effects were observed in rice gntI T-DNA plants that suffered from severe developmental problems and failed to reach the reproductive stage (Fanata et al., 2013).
So far only observed in tomato, stalk-associated fruit parts of GNTI-RNAi plants turned brown during ripening, leading to premature fruit drop (Kaulfürst-Soboll et al., 2011a). Interestingly, in Arabidopsis hybrid glycosylation1 (hgl1 = manII) mutants, both core fucose and xylose residues are masked from full immunogenic recognition (Kaulfürst-Soboll et al., 2011b); therefore, we chose the next enzyme in line, Golgi mannosidase II (MANII), as alternative for reducing the immunogenic potential of tomato fruits. To examine whether silencing of Golgi MANII may be better tolerated or provokes a similar ripening phenotype as observed upon GNTI silencing, we generated stable Micro-Tom MANII-RNAi lines using two different dsRNAi constructs. Comparative analyses with wild-type and GNTI-RNAi lines grown in parallel are presented here.
The Solanum lycopersicum variety Moneymaker dwarf cultivar Micro-Tom (Scott and Harbaugh, 1989) is defective in genes of brassinosteroid metabolism (which indirectly influences responsiveness to gibberellin) and carries a mutation in the self-pruning (sp) gene that leads to a determinate phenotype and another still uncharacterized mutation. In addition, supplemental resistance genes are present, e.g., for Fusarium wilt race 1, gray leafspot, and Cladosporium fulvum (Scott and Harbaugh, 1989; Martí et al., 2006). Still, Micro-Tom is considered a suitable model for the analyses of hormonal regulations in fruit development (Meissner et al., 1997; Eyal and Levy, 2002; Martí et al., 2006; Serrani et al., 2007). Transformation of Micro-Tom cotyledons by Agrobacteria cocultivation in tissue culture and further propagation were done as described previously (Kaulfürst-Soboll et al., 2011a). Tomato plants in soil (for perennials) were regularly watered with 0.4 ppm CALCINIT (93.5% NO3–, 6.5% NH4+, 27% Ca2+, water-soluble) and 0.2 ppm HaKaPhos basis3 (COMPO, Germany). N. benthamiana plants were watered with complete fertilizer HaKaPhos plus (52% NO3–, 48% NH4+ in N14+P5+K24, COMPO, Germany). In the greenhouse, plants received additional illumination (long day regime: 16-h light/8-h darkness at 20–21 to 24–25°C). When about 3 weeks old, N. benthamiana plants were transferred to the lab for agroinfiltration.
Tomato MANII-RNAi transformants were created analogous to the GNTI-RNAi transformants described previously (Kaulfürst-Soboll et al., 2011a). A circa 400-bp fragment of the gene coding for Golgi MANII was obtained via RT-PCR from total leaf RNA of Micro-Tom and inserted twice via compatible restriction sites into pUC-RNAi (SalI/BamHI and/or XhoI/BglII; Supplementary Figure S1), flanking the first intron of potato GA20 oxidase (Chen et al., 2003). One construct was assembled in sense-intron-antisense orientation, and another in antisense-intron-sense orientation, using the primers given in Supplementary Table S1. The two assemblies were inserted via PstI into SdaI opened plant-expression vector pBinAR (HygR) behind the constitutive CaMV 35S promoter (Höfgen and Willmitzer, 1990), introduced into Agrobacteria and used to transform wild-type Micro-Tom.
The sense-intron-antisense MANII-RNAi construct was also used to transform N. benthamiana using a tissue-culture protocol for Nicotiana tabacum (Faske et al., 1997). For RNAi suppression of GNTI, another construct was used (Supplementary Figure S1). A similar region as chosen for tomato GNTI-RNAi (Kaulfürst-Soboll et al., 2011a) was amplified from N. tabacum Samsun NN clone A9 (Wenderoth and von Schaewen, 2000) using the same primers as for tomato GNTI-RNAi and inserted twice into vector pUC-RNAi in sense-intron-antisense orientation. The assembly was first inserted via PstI into the SdaI site between the CaMV 35S promoter and octopine synthase terminator. The entire expression cassette was released by EcoRI and SspI and inserted into EcoRI/SnabI-opened binary vector pDE1001 (KanR). All constructs were verified by restriction analyses/sequencing prior to Agrobacteria-mediated plant transformation.
Immunoblot analyses and peptide:N-glycosidase F (PNGase F, NEB) treatment were done with fruit extracts as described earlier (Kaulfürst-Soboll et al., 2011a, b) using α-PHA-L rabbit antiserum for routine cgly detection. α-vINV rabbit antiserum served as the marker of the ripening stage-dependent vacuolar invertase (vINV), concanavalin A (ConA) for detection of mostly mannose-terminating N-glycans, and selected potato-tomato allergic patient sera for the detection of cross-reactive carbohydrate determinants (CCDs), all characterized in Kaulfürst-Soboll et al. (2011a).
The basophil activation tests (BATs) were performed as described earlier (Kaulfürst-Soboll et al., 2011a) with heparinized whole blood from potato–tomato allergic patients using flow cytometry.
Root growth responses of tomato seedlings to salt were analyzed as described for Arabidopsis in Kang et al. (2008). Prior to germination, seeds were surface-sterilized in 50-ml tubes, 1 × 10 min with 4% sodium hypochloride (NaOCl) and washed 2 × 10 min with sterile ddH2O. Seedlings were kept on normal 3MS medium (complete Murashige & Skoog with vitamins, 3% sucrose, pH 6.1) before transfer to vertical agar (1.5%) plates of the same medium with salt (100 mM NaCl).
To determine how tightly tomato fruits are attached to their mother plants, the force needed to pick a fruit was measured. Three-month-old Micro-Tom plants grown in the greenhouse were used for the experiments. Fruits of similar developmental stage were pulled in longitudinal direction, and the force was recorded with a computer-assisted dynamometer.
Tomato seeds of ripe fruits were acid-washed in 1 N HCl for several hours and then extensively washed with tap water before letting them dry on filter paper. Dried seeds were stored for several months before using them for seed weight determinations.
Ethephon treatment of fruits was essentially done as described in Mizrahi et al. (1976). Fruits were dipped for 1 min into an aqueous solution [2% ethephon in 10 mM 2-(N-morpholino)ethanesulfonic acid (MES), pH 5] and left to dry. Auxin was applied with a syringe. Prior to infiltration, relief holes were pinched into each fruit with the help of the injection needle. Then, ca. 100–300 μl of indole-3-acetic acid (IAA) (100 μM IAA in 10 mM MES, pH 5) was slowly injected, with one repetition on the following day. Buffered water served as control.
Leaves were harvested from 4–5-week-old tomato plants for analysis of salicylic acid (SA), auxin (IAA), abscisic acid (ABA), and jasmonic acid (JA). After grinding under liquid N2 to fine powder, 200 mg was either stored at −80°C or directly used for extraction. Extraction of the free analytes was carried out with 1.5 ml ethyl acetate, containing 0.1% (v/v) formic acid and the internal standards 3-hydroxybenzoeic acid (3HOBA), dihydro-jasmonic acid, and indole-5-carboxylic acid (5IFA) (50 ng ml–1). Samples were incubated at 28°C for 60 min after a 10-min sonification step in an ultrasonic bath. After centrifugation at 18,500 g, 1.2 ml of the supernatant was transferred to a new tube. The ethyl acetate was removed to dryness in a gentle stream of N2. Derivatization was performed with 70 μl of a 1:1 mixture of methanol:trimethylsilyldiazomethane (TMSDM; 2 M in diethyl ether) for 20 min at 25°C. Determination of the analytes in 1 μl injected volume was performed by gas chromatography/mass spectrometry (GC/MS) (Shimadzu TQ8040) using splitless injection mode and an SH-Rxi-17SIL-MS column (30 m, 0.25 mm internal diameter, 0.25 μm film, RESTEK GmbH, Germany). The GC oven temperature was held at 70°C for 5 min, then ramped at 15°C min–1 to 270°C, then ramped at 75°C min–1 to 280°C, and afterward held for an additional 10 min at 280°C. Helium was used as the carrier gas with a flow rate of 1 ml min–1. The mass spectrometer was operated in electron impact ionization (EI) and multiple reaction monitoring (MRM) mode, also described in Wan et al. (2019).
For measurements of leaf-emitted ethylene, leaves of 4–6-week-old tomato plants were cut in small squares (3 × 3 mm, using sizers). Leaf pieces were floated on water in a Petri dish overnight (at room temperature) for recovery, before three (each) were transferred into a 6-ml glass reaction tube containing 500 μl water (dd): without treatment, after wounding (3× squeezing with a spatula), or addition of 1 μM elicitor (flg22; Felix et al., 1999; Crip21; Hegenauer et al., 2020). Vials were sealed with rubber plugs and placed on a horizontal shaker (80–100 r/min) at room temperature for 3 h. Of the gas phase, 1 ml was analyzed with a GC-flame ionization detector (FID) (Shimadzu, GC-2014, glass column 3 mm × 1.6 m with Al2O3).
Ethylene was chemically released from 1-aminocyclopropane-1-carboxylic acid (ACC) as described by Lizada and Yang (1979). Frozen tomatoes were thawed, cut in half, and photographed. One fruit half was crushed with a mortar and pestle in liquid nitrogen. Then, 50% methanol solution (10 ml g–1 fresh weight) was added to the powder. The homogenate was heated to 60°C for 15 min. The suspension was distributed to the test tubes (1 ml per tube). HgCl2 was added to a final concentration of 100 μM, sodium hydroxide (NaOH) was added to a final concentration of 600 mM, and hypochlorite (NaOCl) to 0.35% v/v. Tubes were immediately sealed with rubber plugs and incubated on ice for 20 min. Afterward, 1 ml gas phase was analyzed with a GC-FID (Shimadzu, GC-2014, glass column 3 mm × 1.6 m with Al2O3).
Several fruit-bearing pedicels of the same ripening stage [extending 3 mm to both sides of the abscission zone (az)] were harvested and snap frozen in liquid nitrogen. Total RNA was extracted according to Sokolovsky et al. (1990) and treated with RNase-free DNase I. First-strand cDNA was synthesized from 2 μg total RNA in a volume of 40 μl using oligo-dT (18-mer), RiboLock RNase inhibitor (Thermo Scientific), and RevertAidTM H Minus M-MuLV Reverse Transcriptase (Fermentas). For PCR with Taq-DNA Polymerase (Biozyme), 1-μl aliquots and gene-specific primer pairs were used at optimized annealing temperatures and the cycles indicated in Supplementary Table S1.
Cloning of the KOR1-GFP construct was done by restriction digest of pGFP2ΔNco-KOR1 (Rips et al., 2014) and insertion via XbaI/EcoRI sites 5’ of the GFP reporter in pGPTVII.Hyg (Walter et al., 2004). The final construct pGPTVII.Hyg:KOR1-GFP was used to transform Agrobacteria (strain GV2260). Leaves of 4-week-old N. benthamiana plants (wild-type, GNTI-RNAi, and MANII-RNAi) were co-infiltrated with silencing suppressor strain 19K and placed for 24 h in the dark before transfer to constant light. Leaf samples were checked for GFP signals [confocal laser scanning microscopy (CLSM), Leica SP5] and infiltrated with 10 μM tunicamycin as described in Frank et al. (2008).
For each sample, two leaf discs each were excised with a cork borer (∅ 5 mm) and frozen in liquid nitrogen. Protein extraction and immunoblot analyses were essentially done as described earlier (Frank et al., 2008). A first extraction step was conducted without sodium dodecyl sulfate (SDS) to yield a more concentrated extract in the second step with SDS. The first extraction was done in 50 mM HEPES-NaOH, pH 7.5, 250 mM NaCl, 2 mM sodium pyrosulfite, 1 mM ethylenediaminetetraacetic acid (EDTA), 1 mM Pefabloc SC (Applichem), proteinase inhibitor cocktail for use with plant extracts (Sigma, 1 μl per 30 mg fresh weight), and 2% β-mercaptoethanol. Then, 1% [w/v] insoluble polyvinylpolypyrollidone (PVPP, Sigma) and 2% [w/v] inulin (Merck) were added to the buffer and thoroughly mixed before distribution to the samples. After centrifugation for 10 min in a tabletop centrifuge (4°C), the obtained pellet was extracted with 80 μl of the buffer containing 0.6% [w/v] SDS (w/o PVPP or inulin). SDS-loading buffer was added to 30 μl of the cleared supernatants (4°C), boiled for 10 min, and loaded onto a 6% polyacrylamide gel for SDS-polyacrylamide gel electrophoresis (PAGE) followed by immunoblot analysis.
According to analyses of Arabidopsis hgl1 mutants (Kaulfürst-Soboll et al., 2011b), suppression of Golgi MANII activity should result in reduced complex N-glycan (cgly) recognition. As previously done for GNTI (Kaulfürst-Soboll et al., 2011a), MANII was silenced in the tomato Moneymaker dwarf variety Micro-Tom via a stable dsRNAi approach. In addition to the usual sense-intron-antisense orientation, also the reciprocal antisense-intron-sense orientation was tested (Supplementary Figure S1A). Compared to GNTI-RNAi, many more regenerants were obtained for both MANII-RNAi constructs. Both leaf and fruit extracts of the primary transformants were tested for reduced cgly patterns on immunoblots using a rabbit α-cgly antiserum (α-PHA-L; Laurière et al., 1989), mostly containing xylose-specific but also core fucose-specific antibodies. Independent of the sense and antisense orientation, strong suppression of cgly recognition was achieved by both MANII-RNAi constructs similarly to GNTI-RNAi (Supplementary Figures S1B,C). Selected transformants were propagated further (Figure 1), and leaf extracts were analyzed in parallel to a GNTI-RNAi (T3) plant, side by side with Arabidopsis cgly mutants (Supplementary Figure S2), using α-PHA-L and α-HRP antisera with slightly different binding affinities (Kaulfürst-Soboll et al., 2011b). Strongly suppressed plants among the tested primary transformants were about 50% for both MANII-RNAi constructs compared to only 7% previously obtained for the GNTI-RNAi lines. Of initially 91 independent GNTI-RNAi transformants, only lines #20 and #45 could be used for the analyses because among six substantially silenced T0 plants, one of the strongest candidates (#91) was lost upon transfer from tissue culture to soil. Of note, using a fruit-specific promoter (B33, driving the same GNTI-RNAi cassette), not a single silenced plant was obtained, although proven effective for vINV-RNAi suppression (Kaulfürst-Soboll et al., 2011a). For MANII-RNAi, lines #5 (producing only few seeds), #6, #11, #13, #14, #15, and #18 were propagated further (Supplementary Figures S3A,B), with lines #11, #14, and #18 used for most of the experiments. Their analyses showed that MANII-RNAi silencing in tomato is stable, resulting in low cgly recognition, comparable to that of the Arabidopsis hgl1 (manII) mutant.
Figure 1. GNTI- and MANII-RNAi plants show opposite growth phenotypes. Micro-Tom wild-type (wt) next to GNTI-RNAi (#20) and MANII-RNAi (#14) plants in the greenhouse. Around the age of 4 months, leaves of wt plants started to develop yellow rims (center), while premature senescence was visible for GNTI-RNAi plants, with yellow to necrotic leaf sectors (center left). At this stage, MANII-RNAi plants showed no signs of senescence, but often rolled-up leaf rims (center right). When cut back, GNTI-RNAi plants were able to grow new green sprouts (bottom left). Leaves and fruits of MANII-RNAi plants had a darker, matte appearance (center and bottom right). For details on plant growth and flower and fruit phenotypes, see Supplementary Figure S3.
When grown in the greenhouse next to wild-type and GNTI-RNAi, MANII-RNAi plants appeared more vigorous (Figure 1, top) with delayed leaf senescence. In case of sporadic pathogen attack (or abiotic stress without clear specification), MANII-RNAi plants performed like wild-type, while GNTI-RNAi plants were highly susceptible. Especially stems and flowers of MANII-RNAi plants were larger, and fruits developed slightly differently (Supplementary Figure S3C). Another specific phenomenon of MANII-RNAi plants was that leaves had a matte appearance, which was also true for fruits (Figure 1 and Supplementary Figure S3A, bottom), and sometimes displayed less complex leaf shapes. Furthermore, they rolled up their rims, indicating enhanced growth of the lower and/or inhibited growth of the upper side. This happened synchronously (from one day to the next) and was only observed during the fruit-bearing period, pointing to involvement of a volatile phytohormone (perhaps ethylene as antagonist of auxin) without affecting the other genotypes cultivated in parallel. When cut back, GNTI-RNAi plants showing early senescence/necrosis were able to regenerate new green sprouts (Figure 1, bottom left). Interestingly, root growth of MANII-RNAi seedlings was more tolerant to salt (100 mM NaCl), whereas GNTI-RNAi seemed not affected (Supplementary Figure S4).
The abundance of glycoproteins in tomato fruits increases upon ripening (Priem and Gross, 1992), without structural differences between N-glycans of green and red fruits (Zeleny et al., 1999). To demonstrate this for wild-type and evaluate the extent of cgly recognition in MANII-RNAi versus GNTI-RNAi plants, fruits of different ripening stages were harvested for immunoblot analyses. Because the patchiness of GNTI-RNAi fruits matched the 35S-promoter expression pattern (as visualized by β-glucuronidase GUS fusions in ripe fruits; Moon and Callahan, 2004), green and red parts were separated. Although fruit color is a good indicator of ripeness (indicated by letters in Figure 2), vINV was used as an additional marker because mRNA levels are induced by ethylene and dramatically increase with fruit ripening (Klann et al., 1993; Alba et al., 2005). Moreover, the protein was undetectable in vINV-RNAi fruits (Kaulfürst-Soboll et al., 2011a). Red fruits with comparable amounts of mature vINV signal in wild-type, GNTI-RNAi, and MANII-RNAi extracts were marked for further analyses (Figure 2, gray stars). As expected, the complex glycan pattern increased upon fruit ripening in wild-type (α-cgly) but was significantly reduced by GNTI-RNAi and MANII-RNAi silencing (although remaining bands were detected for both). To determine the extent of cgly reduction in ripe fruits more accurately, selected extracts were serially diluted prior to SDS-PAGE. Blots were developed with anti-complex glycan antiserum (α-cgly; Supplementary Figure S5) and also by affinoblotting with the lectin ConA (Faye and Chrispeels, 1985; Brewer and Bhattacharyya, 1986). Binding of cgly-specific antibodies was similarly reduced in GNTI-RNAi and MANII-RNAi fruit extracts, about 16–32 times compared to wild-type. Inversely, ConA labeling increased to the same extent in both GNTI-RNAi and MANII-RNAi extracts, as shown for Arabidopsis gntI mutant cgl1 (von Schaewen et al., 1993).
Figure 2. Detection of complex N-glycosylated proteins during tomato fruit ripening. Blots of fruit extracts from Micro-Tom wild-type (wt), MANII-RNAi (#14), GNTI-RNAi (#20), and vInv-RNAi (#9) plants of different ripeness (G, green; O, orange; R, red fruits, with lowercase letters indicating fruit parts; +Et, ethephon treatment) were developed with antisera specific for vacuolar invertase (α-vINV, top) or complex N-glycans (α-cgly, PHA-L, center). The latter mainly recognizes β1,2-xylose and to a lesser extent core α1,3-fucoses (independent antibodies). The Ponceau S-stained blots (protein) are shown as loading reference. Since vacuolar invertase is induced during tomato fruit ripening (migrating ∼50 kDa, avoid in vINV-RNAi), its presence was used as the marker to select fruit extracts of similar ripeness for further use (gray stars). Note that vINV (carrying four complex N-glycans in wild-type) is not the most abundant glycoprotein in red fruits (black arrowheads). Proteins decorated with complex N-glycans accumulate during ripening in wild-type fruits (left), which is markedly reduced in the MANII-RNAi and GNTI-RNAi lines. Apparent molecular masses are indicated in kDa (PageRuler Prestained Protein Ladder, Fermentas).
In Kaulfürst-Soboll et al. (2011a), GNTI reduction in fruits was verified by immunoblot analyses of protein extracts treated with PNGase F, an enzyme that releases asparagine-bound N-glycans when α1,3-fucose is missing (Tretter et al., 1991). Glycoproteins of GNTI-RNAi plants (or gntI mutants) are PNGase F-sensitive (Figure 3A), as indicated by the vINV shift (four complex N-glycans in wild-type) and inverse loss of ConA binding to most glycoproteins (Figure 3B). No shifts were detected for wild-type and only minor ones for MANII-RNAi extracts (compare α-cgly to ConA), confirming the presence of core fucoses on most protein-bound N-glycans of MANII-RNAi fruits, as previously shown for the Arabidopsis manII mutant hgl1 (Strasser et al., 2006; Kaulfürst-Soboll et al., 2011b).
Figure 3. Peptide:N-glycosidase F (PNGase F) treatment verifies the presence of core fucoses on MANII-RNAi glycans. (A) N-glycan structures (www.proglycan.com) that may be produced in wild-type (wt), manII, and gntI mutant plants (known as hgl1 and cgl1 in Arabidopsis, respectively) with indicated potential release by PNGase F. This endoglycosidase can cleave asparagine-bound N-glycans when coreα1,3-fucose is missing. In the cell wall or vacuole, terminal sugar residues can be liberated by hexosaminidases (GlcNAc) and α-mannosidases (dotted lines). (B) Immunoblots of untreated (-) and PNGase F-treated (+) fruit extracts from MANII-RNAi (#14), Micro-Tom wild-type (wt), and GNTI-RNAi (#20) plants were developed with α-vINV, α-cgly (α-PHA-L), or the lectin ConA (detecting mannose-terminating N-glycans). The Ponceau S-stained blot (protein) is shown as the loading reference. In GNTI-RNAi, PNGase-F treatment results in a shift of vacuolar invertase (vINV, arrowheads) and loss of ConA binding. This is not the case for MANII-RNAi or wt samples, confirming the presence of core fucoses on most glycoproteins. Note that the upper band detected by α-vINV (stars) shifts in all PNGase F-treated extracts (protein with high mannose precursor), indicating completeness of the enzyme treatment. Apparent molecular masses are indicated in kDa (PageRuler Prestained Protein Ladder, Fermentas).
From the analyses of Arabidopsis hgl1 mutants, MANII emerged as an alternative target for lowering the immunogenic potential of plant-derived glycoproteins (Kaulfürst-Soboll et al., 2011b). This was proven first by Western blot analysis using CCD-positive sera of two potato/tomato-allergic patients that contain CCD-specific (s)IgE antibodies (mediators of immediate-type allergic reactions). Patient sera, mainly recognizing core fucose (PT-02) or xylose epitopes (PT-06; Kaulfürst-Soboll et al., 2011a; Kaulfürst-Soboll et al., 2011b), showed markedly reduced binding to MANII-RNAi fruit extracts. Of note, binding was comparable to GNTI-RNAi (Figure 4A), whereas binding to vINV-RNAi extracts resembled wild-type, as reported earlier (Kaulfürst-Soboll et al., 2011a). To confirm similar peptide-epitope composition, the serum of a latex-allergic patient that strongly binds to highly abundant tomato polygalacturonase 2A (PG), confirmed with the recombinant protein expressed in Escherichia coli (not shown), was included.
Figure 4. Patient sera reveal reduced cross-reactive carbohydrate determinants (CCD) binding to MANII-RNAi fruit extracts. (A) Immunoblots were prepared with extracts of Micro-Tom wild-type (wt) and RNAi-silenced fruits (GNTI-RNAi #20; vINV #9; MANII-RNAi lines #11 and #14). CCD-positive sera of potato/tomato-allergic patients PT-02 and PT-06 (Kaulfürst-Soboll et al., 2011a) were used for the detection of CCD-specific IgE (sIgE) antibodies. A serum of a non-allergic individual, also containing sIgE for polygalacturonase 2A (46 kDa, a major glycoprotein of tomato fruits), but only minor CCD-sIgE, was included as control. The Ponceau S-stained blot (protein) is shown as the loading reference. The black arrowhead points to vacuolar invertase (vINV, 52 kDa), which was not detected in vINV-RNAi fruits. Note that the CCD-positive patient sera (left) show strong binding to wt and vINV-RNAi, but reduced binding to GNTI-RNAi and MANII-RNAi extracts. Apparent molecular masses are indicated in kDa (PageRuler Prestained Protein Ladder, Fermentas). (B) Whole blood of patient PT-02 was used for a basophil activation test (BAT) with the indicated fruit extracts, demonstrating about 10 times reduced stimulation by GNTI-RNAi and also MANII-RNAi. Horseradish peroxidase (HRP; vacuolar glycoprotein with nine complex N-glycans) in PBS served as a positive control for CCD-dependent basophil stimulation and PBS as negative control.
To further investigate whether MANII-RNAi suppression in tomato also results in lower effector-cell triggering, as previously shown for GNTI-RNAi (Kaulfürst-Soboll et al., 2011a), an ex vivo BAT was performed with whole blood of PT-02 and native tomato fruit extracts. Basophils of this potato/tomato-allergic patient were strongly activated by horseradish peroxidase (HRP; nine complex N-glycans), routinely used to demonstrate activation by plant-derived CCD epitopes (Figure 4B). Activation by wild-type or vINV-RNAi extracts was similar but required about 10 times higher concentration of the GNTI-RNAi or MANII-RNAi extracts. Thus, both immunological tests of MANII-RNAi fruit extracts confirmed effective core fucose shielding by untrimmed mannoses on the α1,6 arm.
MANII-RNAi plants developed almost like wild-type, whereas fruits of GNTI-RNAi plants ripened irregularly, with a patchy coloration (from green, yellow to red) and necrotic stalk-attached parts before ripening was complete (Figures 5A,B, arrows). This is likely one reason for the early fruit drop observed when GNTI-RNAi plants still looked fine and green, not yet showing signs of premature senescence. By contrast, MANII-RNAi fruits remained attached to the plant for an extended time. In addition, larger seeds were produced (Figure 5C), however, at the expense of the seed number per fruit. Seed mass was about 1.8-fold higher compared to wild-type and GNTI-RNAi plants (Figure 5D). In some lines, seed number was extremely reduced, and often fruits without seeds were found (thus work was continued with lines that still produced seeds). Interestingly, for Arabidopsis hgl1 (manII) and cgl1 (gntI) mutants, no obvious changes in seed development were observed, with 1,000-grain weights comparable to wild-type (not shown). This pointed to a tomato-specific phenomenon perhaps due to interference with hormone crosstalk during fruit ripening. Of note, the GNTI-RNAi phenotypes were not overcome by back-crossing, although plants could easily be crossed with the tall wild-type variety Moneymaker (to exclude an influence of the dwarf mutant background; Supplementary Figure S6) and another Micro-Tom line (vINV-RNAi, not shown). An extreme MANII-RNAi line was used for reciprocal crosses with Micro-Tom wild-type (Supplementary Figure S7). Pollination with wild-type did not result in vital seeds, and no hygromycin-resistant progeny was found when MANII-RNAi was used as the pollen donor. This revealed problems for both female and male gametophytes.
Figure 5. ManII-RNAi fruits resemble wild-type, except for seed number and mass. (A) Comparison of GNTI-RNAi (#20) and MANII-RNAi (#15) Micro-Tom plants in the greenhouse. Mature GNTI-RNAi fruits show yellow-green areas, whereas MANII-RNAi fruits develop normally. (B) Stalk-associated fruit parts of GNTI-RNAi (#20) remain incompletely ripe (green parts) and show signs of necrosis (arrows), whereas MANII-RNAi (#15) fruits look normal but contain less seeds. (C) Acid-washed dried and stored tomato seeds, shown for Micro-Tom wild-type (wt), and exemplarily for a GNTI-RNAi (GTI) and MANII-RNAi line (MII). Each box measures 5 mm × 5 mm. (D) MANII-RNAi seeds are heavier and GNTI-RNAi seeds are slightly lighter than Micro-Tom wild-type. The box plot was compiled with seeds from several seed batches (biological replicates) of wild-type (wt; N = 14), GNTI-RNAi lines (GTI #20, N = 8; #45, N = 4), and MANII-RNAi lines (MII #11, N = 13; #13, N = 2; #14, N = 23; #15, N = 11; #18, N = 9). For details (one-way ANOVA), see Supplementary Excel Sheet.
Since fruits of GNTI-RNAi plants came loose easily, the force needed to pick a fruit was determined for the Micro-Tom genotypes at comparable ripening stages (Supplementary Figure S8). When red fruits were pulled in longitudinal direction, fruits of the wild-type and MANII-RNAi were released at 5.3 N, but GNTI-RNAi fruits already at 4.2 N. Thus, premature abscission observed for GNTI-RNAi plants manifested before fruits dropped naturally. Several assumptions can be made about the underlying mechanisms in the tomato GNTI- and MANII-RNAi plants, but interference with auxin versus ethylene signaling during fruit ripening would fit the observed phenotypes best.
Ethylene, important for fruit ripening, is also known to be involved in senescence (Lim et al., 2007) and defense (Wang et al., 2002), which appears enhanced in GNTI-RNAi plants (Figure 1). To address whether ethylene may influence the patchy phenotype, GNTI-RNAi fruits were treated with ethephon (an industrial agent used for acceleration of fruit ripening), which releases ethylene during decomposition. Ethephon was applied to fruits when separation into orange and yellow parts became visible (Figure 6A). Two days later, the orange parts had turned red, while the yellow parts remained more or less the same. When a normal-looking green fruit (of almost the size of the red fruits) was subjected to the ethephon treatment, 11 days later, the fruit showed a more homogeneous yellow-red coloration compared to an untreated fruit on the same plant, but patchiness and stalk-attached necrosis remained (Figure 6B). Hence, we reasoned that rather auxin responses may be influenced in the RNAi lines.
Figure 6. The fruit phenotype of GNTI-RNAi is alleviated by ethephon and mimicked by indole-3-acetic acid (IAA). (A) Fruits of Micro-Tom GNTI-RNAi line #20 were photographed before (-) and after (+) treatment with 2% ethephon (2-chloro-ethyl-phosphonic acid), whose decomposition results in ethylene release. Ethephon was applied when irregular fruit coloration appeared (the border between yellow and orange areas was marked with a water-resistant pen). After 2 days, orange areas had turned red, while yellow-green areas remained patchy yellow-green. (B) After ethephon treatment of green fruits, GNTI-RNAi fruits developed a more homogeneous red color, but necrotic stalk regions remained after 11 days, compared to an untreated fruit from the same plant. (C–F) Detached Micro-Tom fruits (breaker stage) were infiltrated in parallel with either tap water (+ H2O) or the auxin indole-3-acetic acid (+ IAA, 100 μM). Dpi, days post infiltration. After 4 days, IAA infiltration resulted in patchy coloration of both wild-type (wt) and MANII-RNAi fruits (#20), similar to GNTI-RNAi (#14) fruits. After 11 days, IAA-infiltrated fruits looked rotten. When opening the water-infiltrated fruits, signs of internal rotting were visible for GNTI-RNAi, and to some extent also for MANII-RNAi, but not for wt fruits.
Many studies have shown that auxin (IAA) plays an important role in fruit ripening and aging. Exogenous IAA treatment of immature tomato fruits is known to delay ripening and results in parthenocarpy (Kumar et al., 2014). When IAA was injected into mature green fruits of Micro-Tom wild-type, fruits ripened but started to rot after 1 week, while water-infiltrated fruits of the same plant ripened without rotting (not shown). This was also observed for detached fruits. Compared to water, IAA infiltration into green wild-type fruits resulted in delayed ripening and early rotting (Figure 6C). GNTI-RNAi fruits kept their patchy phenotype upon IAA injection (Figure 6D), and water-infiltrated MANII-RNAi fruits showed a patchy coloration after 4 days, reminiscent of the GNTI-RNAi phenotype (Figure 6E). Internal signs of rotting were also visible for detached, water-injected fruits of GNTI-RNAi, and to a much lesser extent also for MANII-RNAi, but not for wild-type (Figure 6F).
To investigate whether phytohormone levels might be altered in the RNAi lines, the contents of IAA, ABA, JA, and SA were determined in Micro-Tom leaf tissues (Figure 7A). Compared to the wild-type, no significant changes were recorded, except for JA that was not detected in the RNAi plants. SA levels were by trend slightly elevated in the GNTI-RNAi lines compared to wild-type and MANII-RNAi.
Figure 7. Hormone measurements of Micro-Tom leaf and fruit extracts. (A) Phytohormone levels were determined in leaf extracts of the wild-type (wt) and indicated RNAi lines (GT, GNTI-RNAi; MII, MANII-RNAi). IAA, indole acetic acid; ABA, abscisic acid; JA, jasmonic acid; SA, salicylic acid. Bars represent the mean of three biological replicates for three plants of wt, four of each GNTI-RNAi line, and two of MANII-RNAi line #11. SD, standard deviation. (B) Ethylene emission from leaf pieces after the indicated treatments: water (mock), a bacterial elicitor (1 μM flg22), a parasitic plant elicitor from Cuscuta (1 μM Crip21), or repeated squeezing with a forceps (wounding). Bars represent the mean of three measurements with three plants per genotype, i.e., Micro-Tom wild-type (wt), GNTI-RNAi lines (GT #20, #45), and one MANII-RNAi line (MII #11). SEM, standard error of the mean. (C) Ethylene was chemically released from 1-aminocyclopropane-1-carboxylic acid (ACC) of frozen fruit halves. Micro-Tom wild-type (wt), GNTI-RNAi lines (GT #20, #45), and MANII-RNAi line (MII #11) at the ripening stages shown (G, green; O, orange; Y, yellow; R, red). Bars represent the mean of three replicates. SD, standard deviation of the mean.
To test whether stress treatment may reveal differences between the genotypes, ethylene release was measured upon wounding or incubation with elicitors, which are perceived by the heavily N-glycosylated ectodomains of receptor-like kinases (RLKs) at the plasma membrane, i.e., bacterial flg22 by FLS2 (Häweker et al., 2010) or Crip21 by CuRe (18 potential N-glycosylation sites) of the plant pathogen Cuscuta reflexa (Hegenauer et al., 2016, 2020). Ethylene production was found to be highly variable in leaf samples of all genotypes, without clear differences (Figure 7B). Moreover, when chemically released from ACC of harvested fruits, the amounts correlated with the degree of fruit ripening (Figure 7C). Together, these findings pointed to a subtle interference of altered N-glycan modification with hormone crosstalk/signaling during fruit ripening in the RNAi lines.
To shed more light on altered hormone responses, we chose pedicels as investigation object. With respect to the observed early fruit drop in GNTI-RNAi lines, tissue around the az of green (G), yellow (Y), orange (O), and red (R) fruits (Figure 8 and Supplementary Figure S3) was used for semiquantitative RT-PCR analyses. Harvested parts are shown for green and mature red fruit-bearing plants of wild-type, GNTI-RNAi, and MANII-RNAi lines without visible changes in the pedicel (Figure 8A) but obvious differences in the attached fruits. Those of the GNTI-RNAi line exhibited the patchy ripening phenotype linked with necrotic signs in the region where the stalk was attached and in partly brown regions around the seeds. The MANII-RNAi line developed completely red fruits (Supplementary Figure S3). Semiquantitative RT-PCR analyses were conducted for several target genes with focus on auxin-responsiveness. Marker genes for either ripening or abscission were also included. Compared to two housekeeping genes (ELF1a and TUB4), known auxin-response and az-marker genes were found to be deregulated in the RNAi lines. Tomato ARR15 (Arabidopsis thaliana response regulator type A), induced by auxin-negatively regulating cytokinin (Leibfried et al., 2005; To et al., 2007; Müller and Sheen, 2008) accumulates during fruit ripening in the az of wild-type, declined in the GNTI-RNAi lines and remained at elevated levels in MANII-RNAi lines. H+ ATPase (LHA4; Mito et al., 1996) was more prominently expressed in the early ripening stages of both RNAi lines, whereas GH3.2 (group II IAA-amido synthetase) that is auxin- and ethylene-responsive (Sravankumar et al., 2018) showed a peak in the az of yellow MANII-RNAi fruits and seemed to be more present in red fruits of GNTI-RNAi lines compared to those of wild-type. AUX/IAA repressor IAA9, shown to decrease in az upon flower removal (Meir et al., 2010), decreased in GNTI-RNAi (stages Y and O) but showed high expression in MANII-RNAi, similarly to wild-type. IAA9 and, to a higher extent, IAA17 are both induced by auxin and repressed by ethylene (Audran-Delalande et al., 2012). In wild-type, IAA17 was expressed during all ripening stages, but only detected in the az of green fruits for GNTI-RNAi, and in those of both green and red fruits of MANII-RNAi plants. Az markers TAPG2 and TAPG4 (two tomato abscission-related polygalacturonases; Meir et al., 2010; Reichardt et al., 2020) were clearly elevated in GNTI-RNAi plants (especially TAPG2 in the az of red fruits), with deregulation in MANII-RNAi (high in the az of yellow fruit pedicels). Auxin-response factors (ARFs) ARF2a (ethylene-responsive) and ARF2b (auxin-responsive) are important during Micro-Tom fruit ripening (Hao et al., 2015). Differential expression in the RNAi lines was detected for ARF2a with highest levels in red fruit pedicels of the wild-type, yellow ones in GNTI-RNAi, and a mixture of both in MANII-RNAi. Ripening-related marker ERT10 (Meir et al., 2010; Reichardt et al., 2020) was elevated in the az of GNTI-RNAi and deregulated in those of MANII-RNAi plants (inverse to wild-type in the az of green fruit pedicels).
Figure 8. Marker gene expression in wild-type, GNTI-RNAi, and MANII-RNAi fruit pedicels. (A) Close-up view of pedicels attached to green (top) or red (ripe) fruits of Micro-Tom wild-type (wt), GNTI-RNAi (#20), and MANII-RNAi (#18). Parts ca. 3 mm to both sides of the abscission zone (az) were used for RNA isolation. Longitudinally cut open pedicels of red fruits are shown below. Fruits were attached to the left. Bars = 2 mm. (B) Semiquantitative RT-PCR of abscission zones of GNTI-RNAi (#20) and MANII-RNAi (mix of #11 and #18) with green (G), yellow (Y), orange (O), or red (R) fruits was performed with two housekeeping genes as reference: ELF1a, translation elongation factor; TUB4, tubulin 4 (top). Marker genes were chosen based on known responses to auxin/ethylene and expression during certain developmental stages (abscission, fruit ripening). ARR15, Arabidopsis response regulator type A, auxin-responsive-negatively regulating cytokinin; ATPase (H+), auxin/sugar-responsive; GH3.2, IAA-amido synthetase, auxin- and ethylene-responsive; IAA9/17, AUX/IAA transcription factors: IAA9, auxin-responsive, ethylene-repressed; important for leaf morphology/fruit set; IAA17, auxin-responsive, ethylene-repressed; TAPG2/4, tomato abscission-related polygalacturonases; ARF2a/b, auxin response factors important for tomato fruit ripening (auxin versus ethylene); ERT10, tomato ripening-related marker.
To address the possibility that secreted glycoproteins with altered N-glycan structures may be less stable in the RNAi plants, N. benthamiana (suitable for agroinfiltration) was stably transformed with GNTI-RNAi or MANII-RNAi constructs (Supplementary Figure S1A). Reduced cgly patterns were confirmed by immunoblotting in the T1 and T2 generation (not shown). To prevent KOR1 cycling between the plasma membrane and trans-Golgi network (Nagashima et al., 2020), a binary KOR1-GFP construct with masked C-terminus was cloned (Supplementary Figure S9A) and detected at the cell surface of transfected protoplasts (Supplementary Figure S9B), similar to GFP-KOR1 (von Schaewen et al., 2015). Immunoblot analysis of agroinfiltrated N. benthamiana wild-type leaves (Supplementary Figure S9C) showed similar expression for KOR1-GFP next to GFP-KOR1 (Rips et al., 2014).
Nicotiana benthamiana wild-type plants in the greenhouse, cultivated together with GNTI- and MANII-RNAi lines (T3, Figure 9A), showed reduced cgly patterns (Figure 9B). KOR1-GFP was agroinfiltrated into leaves of the wild-type, GNTI-RNAi, and MANII-RNAi plants. When KOR1-GFP signals were detected at the plasma membrane of all genotypes (Figure 9C), leaves were infiltrated with water (mock) or tunicamycin (10 μM, to abrogate N-glycosylation and prevent further secretion) and harvested over the following hours. Immunoblot analyses with anti-GFP antiserum (α-GFP) indicated that KOR1 may be slightly less stable in the RNAi plants compared to wild-type (Figure 9D). This was deduced from persistence of the top bands versus the newly emerging bottom bands over time and most evident 1–2 h after tunicamycin infiltration.
Figure 9. Analysis of glycoprotein stability in N. benthamiana GNTI- and MANII-RNAi plants. (A) N. benthamiana wild-type (wt), GNTI-RNAi (#10), and MANII-RNAi (#4) T3 plants prior to agroinfiltration. (B) Immunoblot analysis of indicated leaf extracts (membrane fractions) developed with anti-complex glycan antiserum (α-cgly = α-PHA-L). The Ponceau S-stained blot (protein) is shown as the loading reference. Apparent molecular masses are indicated in kDa (PageRuler Prestained Protein Ladder, Fermentas). (C) Confocal laser scanning microscopy (CLSM) images of KOR1-GFP expression in N. benthamiana wild-type and the indicated RNAi lines. Merging of GFP (green) and chlorophyll autofluorescence (blue) with bright field. Bars = 10 μM. (D) Immunoblot analysis of leaf extracts (membrane fractions) upon agroinfiltration and development with anti-GFP antiserum (α-GFP). Tunicamycin (Tunica, 10 μM) treatment results in the accumulation of non-glycosylated KOR1-GFP (band shift). The fading top bands indicate decay due to protein turnover. 19K, co-expressed Agrobacterium silencing suppressor strain (negative control). The Ponceau S-stained blots are shown as the protein loading reference. Molecular masses are indicated in kDa (PageRuler Prestained Protein Ladder, Fermentas).
Here, we compared RNAi suppression of two consecutive glycosyltransferases in the Golgi apparatus of tomato that either prevent (GNTI-RNAi) or alter (MANII-RNAi) complex N-glycan formation on secretory glycoproteins. Compared to GNTI-RNAi (resulting in signs of premature senescence, early fruit drop, and patchy fruit ripening), MANII-RNAi silencing was far better tolerated in tomato, which is likely due to the hybrid nature of the accumulating N-glycans. Nevertheless, MANII-RNAi fruit extracts showed reduced recognition by cgly-specific antibodies (similar to GNTI-RNAi), which independently confirmed shielding of core fucoses (by branched mannoses on the 1,6-arm) and an altered position of the xylose residue, as described for the corresponding Arabidopsis mutants (Kaulfürst-Soboll et al., 2011b). Subtle phenotypic differences were also observed for the Micro-Tom MANII-RNAi plants: first of all, more vigorous growth, visible as thicker stems and broader leaves—sometimes with less complex shape that rolled up their rims during the ripening stage (Figure 1 and Supplementary Figure S3). The latter is reminiscent of tomato dr12 lines with defective ARF, whose mRNA accumulation is ethylene-dependent (highest in early red fruits) but also important for seed development and seedling growth. Mutant dr12 plants showed upwardly curled leaves (Jones et al., 2002). Furthermore, the pectin fine structure and tissue architecture were altered (Guillon et al., 2008), which may also be affected in the RNAi plants. Interestingly, a blotchy fruit phenotype was also observed for isopentenyltransferase IPT-transformed tomato lines with enhanced cytokinin levels (Martineau et al., 1994; reviewed in Srivastava and Handa, 2005), which may hint at a link to cytokinin, as reported for rice gntI mutants (Fanata et al., 2013). Of note, severe ripening defects with yellow and orange patches (never reaching the typical red color of wild-type fruits, similar to GNTI-RNAi) were also observed for Micro-Tom ARF2ab-RNAi lines that are compromised in normal climacteric fruit ripening (auxin versus ethylene; Hao et al., 2015). Clearly, flowers of MANII-RNAi plants were bigger and produced fleshier fruits (Supplementary Figure S3) with matte appearance that showed earlier water loss than detached wild-type fruits (Figure 1). Here, one could speculate that the latter reflects altered cuticular wax precursor synthesis/transport that involves the ER and Golgi (reviewed in Trivedi et al., 2019). Moreover, MANII-RNAi fruits contained fewer, but larger, seeds. As observed during back-crossing, both male and female fertility was compromised, resulting in fruits with less or no seeds in several independent lines. Seedlessness is an attribute of parthenocarpy that develops when male or female gametophytic plant fertility is impaired. This is often accompanied by an untimely increase of auxins and gibberellins in the ovaries (Nitsch, 1970; George et al., 1984; Mazzucato et al., 1998), e.g., in tomato pat mutants that show accelerated ripening and higher fruit quality, but produce no seeds due to aberrant anther and ovule development (Mazzucato et al., 1998). Of note, parthenocarpy can be induced by application of auxin and gibberellin (Pandolfini, 2009) and was achieved in Micro-Tom by ectopic expression of genes for auxin synthesis or auxin responsiveness (Martinelli et al., 2009). Moreover, when red fruits of the tomato variety Ailsa Craig were Agrobacterium-infiltrated with the GNTI-RNAi construct, fruits dropped within a few days, but those infiltrated with the MANII-RNAi construct remained on the plant until harvested after 1–2 weeks (not shown). Thus, the observed phenotypes seem not to be cultivar-specific nor the result of a specific integration site within the genome.
The largely opposing fruit phenotypes of GNTI- and MANII-RNAi tomato plants suggest that auxin versus ethylene signaling/readout, most important during the last fruit ripening stages in tomato (Srivastava and Handa, 2005), might be affected. Indeed, fruit treatments using either ethephon or IAA point in this direction, whereas the measurement of hormone contents in leaves of Micro-Tom wild-type versus the RNAi lines showed only minor differences—except for JA that was absent from the RNAi lines. The latter may be a side effect of the slightly elevated SA levels detected in the GNTI-RNAi lines.
Failure to provoke differential ethylene release from leaves by various stress treatments further corroborated a specific role for complex-type N-glycans during tomato fruit ripening. In this context, it is interesting that tomato ACC-synthase2 tilling mutants, either overproducing (acs2-1) or underproducing (acs2-2) ethylene, showed opposite phenotypes (Sharma et al., 2020). Similar to the GNTI-RNAi lines, acs2-1 overproducers displayed faster leaf senescence (besides accelerated fruit ripening), but acs2-2 underproducers slower leaf senescence (besides prolonged fruit ripening), partially reminiscent of the MANII-RNAi lines. Since fruit ACC contents correlated with the ripening state in all genotypes, the underlying mechanism likely acts upstream.
Subtle differences of hormone crosstalk in the Micro-Tom RNAi lines became evident when pedicel tissue around the az was used for semiquantitative RT-PCR analyses. Auxin-responsive genes were differentially regulated in GNTI-RNAi and MANII-RNAi versus wild-type. Especially the decline of tomato ARR15 (an auxin-response regulator that negatively regulates cytokinin responses) in GNTI-RNAi, and its elevated expression in MANII-RNAi, correlates with the observed early fruit drop in GNTI-RNAi, which suggests a less or higher suppression of cytokinin-responsive genes, respectively. The low abundance of IAA9 and IAA17 mRNA expression (auxin-induced, ethylene-repressed) in the GNTI-RNAi lines is indicative of elevated ethylene levels, likely induced by too much auxin/signaling (Abeles and Rubinstein, 1964). In MANII-RNAi, az of the orange ripening stage showed low amounts of IAA17 transcript compared to wild-type, but similar levels in the red stage, hinting at less ethylene in ripe fruits of MANII-RNAi compared to GNTI-RNAi. This is in accordance with the higher transcript levels of the auxin-conjugating enzyme GH3.2 in the red fruit stage of GNTI-RNAi, whereas in MANII-RNAi, only a temporary increase was observed for the yellow stage.
Compared to wild-type, abscission markers TAPG2 and TAPG4 were both elevated in GNTI-RNAi (especially TAPG2) but seemed deregulated in MANII-RNAi. Moreover, ARF2a and ARF2b act as repressors of auxin-responsive genes in tomato, but only ARF2a is induced by ethylene (Hao et al., 2015). Interestingly, the az of both GNTI- and MANII-RNAi pedicels showed elevated ARF2a levels during the early ripening stages, hinting at elevated ethylene/readout. However, ripening marker ERT10 was much higher expressed in GNTI-RNAi and inversely regulated in MANII-RNAi (highest in the az of green fruits, i.e., opposite to wild-type). Thus, alterations in auxin signaling (as indicated by the DII Venus sensor in the roots of Arabidopsis cgly mutant seedlings; Frank et al., 2021) would best explain the observed phenotypic deviations, which should be linked to the different N-glycan structures.
Early investigations by Handa et al. (1985) deduced a possible effect of N-glycans on hormone crosstalk from experiments, in which abrogation of N-glycosylation by tunicamycin treatment interfered with fruit ripening. Later, application of free Man5GlcNAc was shown to prevent the inhibitory effect of tunicamycin, suggesting an involvement of released N-glycans (Yunovitz and Gross, 1994b). Indeed, experiments with unbound N-glycans had concentration-dependent stimulatory or inhibitory effects on tomato fruit ripening (Yunovitz and Gross, 1994a, b; Yunovitz et al., 1996). Of note, M3XF [Man3(Xyl) GlcNAc (Fuc)GlcNAc], characteristic for complex N-glycans in wild-type (regardless of the presence or absence of terminal GlcNAc residues), and Man5GlcNAc (M5), representing the N-glycan structure of GNTI-RNAi plants, were equally able to promote fruit ripening and senescence at elevated concentrations. Especially, M5 was found to have an inhibitory effect (Napier and Venis, 1991; Yunovitz and Gross, 1994a). Based on these criteria, N-glycans of the studied RNAi lines differ only by the absence (GNTI-RNAi) or presence (MANII-RNAi) of core fucose and xylose residues.
Another aspect is the ability of plant cells to enzymatically release sugar moieties or entire N-glycans in post-Golgi acidic compartments. ENGase and peptide:N-glycanase (PNG1 in Arabidopsis; Diepold et al., 2007) are confined to the cytosol, where they aid in the clearance of misfolded glycoproteins upon retro-translocation from the ER. To date, it is not clear whether and how these activities might also contribute to tomato fruit ripening (Nakamura et al., 2008; Maeda and Kimura, 2014). However, two aPNGase isoforms (Uemura et al., 2018) that are similar to PNGase A from almond, which can release core fucosylated N-glycans (Hossain et al., 2010), are probably located in acidic compartments of tomato fruits (apoplast/vacuole). Thus, they may release both complex-type (with core fucose) and high mannose-type N-glycans from secreted glycoproteins (Figure 10). We speculate that those released in wild-type and MANII-RNAi plants would carry core fucoses, whereas those of GNTI-RNAi plants may look “dangerous,” due to their accessible GlcNAc2 chitobiose part, which resembles the pathogen-associated molecular pattern PAMP chitin.
Figure 10. Model of differential N-glycan interpretation in the RNAi lines. Protein-bound N-glycans of wild-type (wt), GNTI-RNAi, or MANII-RNAi plants (left) may be either directly bound or liberated depending on capability for enzymatic release. Decoding is suspected to occur by proteins with lectin domains (for sugar symbols, see Figure 3A). Arrows indicate cleavage by peptide:N-glycosidase (PNGase A isoforms in different acidic compartments that may release core fucosylated N-glycans) or in the cytosol by endo-β-N-acetyl-glucosaminidase (ENGase). In GNTI-RNAi plants, the chito-oligosaccharide N-glycan core may be decoded upon release from the glycoprotein backbone, potentially resulting in HR (hypersensitive response). Abbreviations: ConA, concanavalin A; HEXO, hexosaminidases (apoplast/vacuole); WGA, wheat-germ agglutinin.
Prior to cleavage by hexosaminidases (HEXO), present in the apoplast and vacuole, wild-type N-glycans carry two terminal GlcNAc residues (with or without Lewis-a epitopes). Those of MANII-RNAi plants may only display one (on the 1,3-arm); GNTI-RNAi plants, none. This, and the presence of terminal mannoses on the 1,6-arm, is probably decoded by lectins (or proteins with lectin domains) that bind to terminal GlcNAc (WGA-type), mannose (ConA-type), or chitobiose units (GlcNAc2 without core fucose). Hence, immediately upon secretion, glycoproteins of the wild-type look “young”; those of MANII-RNAi, “intermediate”; and those of GNTI-RNAi plants, “old” (as if HEXO already clipped the terminal GlcNAc residues). This might determine their clearance rate from the plasma membrane/apoplast by endocytosis and explain why KOR1-GFP turnover was slightly accelerated in the N. benthamiana RNAi plants. Besides, also presence (in wild-type), absence (in GNTI-RNAi), or shielding of core fucoses (in MANII-RNAi) may alter signaling from the plasma membrane. One example is the transforming growth factor (TGF)-beta receptor of mammalian cells for which lack of core fucose caused aberrant lung development in mice (Wang H. et al., 2005; Wang X. et al., 2005). Possibly this is also the case in plants; however, not much is known about the role of core fucose yet in this emerging field of research (reviewed in Bellande et al., 2017; Van Holle and Van Damme, 2018).
Apparently, phytohormone signaling can be influenced by released N-glycans (for review, see Maeda and Kimura, 2014; Lannoo and van Damme, 2015). Since free N-glycans were also found in the xylem sap of tomato stems (Faugeron et al., 1999; Tsujimori et al., 2019), even systemic signaling throughout the entire plant is conceivable. We believe that a different capacity to release N-glycans in the GNTI-RNAi versus the MANII-RNAi lines could be linked to their opposite phenotypes in tomato. Accessibility of the chitobiose part in the GNTI-RNAi lines may additionally modulate growth and developmental readouts, especially concerning apoplastic lectins or receptor-like kinases with a lectin domain (LecRLKs; Bellande et al., 2017; Sun et al., 2020). In case of the terminal N-glycan parts, lectins with GlcNAc- or mannose-specific domains (WGA- or ConA-type, respectively; Figure 10) may compete for binding in MANII-RNAi plants—due to the hybrid N-glycan structure with one branched mannose arm—shared with GNTI-RNAi, and thus differ from wild-type.
Compared to wild-type, the phenotypes observed in MANII-RNAi Micro-Tom plants, showing increased ectopic growth and delayed senescence, may reflect stimulatory auxin-like effects, while in GNTI-RNAi plants, auxin-like signaling seems to be supra-optimal (“over the top”). Too much auxin, and maybe also auxin-like effects, influences ethylene signaling, as deduced from our RT-PCR results. Together with additional defense signaling in the GNTI-RNAi lines, this may be responsible for the observed cell death/necrosis. The latter is known to be spontaneously triggered in plant autoimmune responses that may be elicited via chitin elicitor receptor kinase CERK1 after pathogen attack (reviewed in van Wersch et al., 2016; Chakraborty et al., 2018). But also independent of a chitin-signaling cascade, other possibilities of chitobiose perception exist in the plant apoplast (Vanholme et al., 2014).
Concerning performance in the field, independent of the hormonal aspects, higher susceptibility of GNTI-RNAi plants to bacterial infection (observed in the greenhouse; for a recent review on glycan-based plant-microbe interactions see Wanke et al., 2021) may relate to the fact that bacterial chitinases can liberate N-glycans for nutritional purposes (Frederiksen et al., 2013). Here, it remains to be investigated whether lack of core fucose in GNTI-RNAi plants may render them an “easier” food source.
Several attempts in the field of glyco-engineering have been made concerning the reduction of unwanted side effects of plant-derived glycoproteins. Although promising, not all seem to be compatible with normal plant growth and development. In this and earlier work (Kaulfürst-Soboll et al., 2011a), we show that not all findings obtained with Arabidopsis (a genetic model organism) can be transferred to crops. For example, salt-stress assays of the tomato RNAi lines did not reflect the results previously obtained with the corresponding Arabidopsis cgly mutants. To the contrary, MANII-RNAi seedlings showed longer root growth on salt perhaps due to their enlarged seeds (with more storage reserves), while GNTI-RNAi seedlings performed equally to wild-type. The phenotypes observed in tomato during fleshy fruit development stress the relevance of complex N-glycan structures for/during hormonal crosstalk, most likely interfering with auxin, also in Arabidopsis roots (Frank et al., 2021). In light of our results, the previously reported cytokinin hyposensitivity of the rice gntI T-DNA mutant (Fanata et al., 2013) may also be caused by auxin-like effects. This matches the more recent finding that core fucose is important for basipetal auxin transport and gravitropic responses in rice (Harmoko et al., 2016). For clearer results, clustered regularly interspaced short palindromic repeats/caspase 9 (CRISPR/Cas9) lines should be generated and studied. Whether tomato fruits of GNTI-CRISPR plants will ripen at all, and MANII-CRISPR plants will still be able to reproduce, remains to be shown.
The original contributions presented in the study are included in the article/Supplementary Material. Further inquiries can be directed to the corresponding author/s.
AvS and HK-S designed the study and wrote the manuscript. HK-S, MM-B, MA, and AvS performed the experimental work. HK-S, MM-B, RB, MA, and AvS analyzed the data. All authors approved the manuscript.
This work was financially supported by the Deutsche Forschungsgemeinschaft (DFG) by funds to MA (AL 1426/4-1) and AvS (SCHA 541/11-4).
The authors declare that the research was conducted in the absence of any commercial or financial relationships that could be construed as a potential conflict of interest.
The authors thank Joachim Kilian and Volker Hegenauer (Tübingen) for hormone and ACC measurements and Julia Frank (Münster) for the KOR1-GFP constructs. Andreas Schaller (University of Hohenheim, Germany) provided RT-PCR primers and gave advice on abscission zone analyses. Kerstin Fischer (Münster), Olessia Becker (Münster), and Birgit Löffelhardt (Tübingen) provided technical assistance. Dirk Schmidt (Münster) took care of the greenhouse plants, and Wiltrud Krüger (Münster) helped harvesting tomato seeds. Special thanks to Manuel Frank for statistical analyses (Aarhus University, DK) and Thomas Thiersch and Peter Tumbrink (Institute of Physics, WWU Münster) for help with the dynamometer equipment.
The Supplementary Material for this article can be found online at: https://www.frontiersin.org/articles/10.3389/fpls.2021.635962/full#supplementary-material
Aalberse, R. C., Koshte, V., and Clemens, J. G. (1981). Immunoglobulin E antibodies that crossreact with vegetable foods, pollen, and Hymenoptera venom. J. Allergy Clin. Immunol. 68, 356–364. doi: 10.1016/0091-6749(81)90133-0
Abeles, F. B., and Rubinstein, B. (1964). Regulation of ethylene evolution and leaf abscission by Auxin. Plant Physiol. 39, 963–969. doi: 10.1104/pp.39.6.963
Alba, R., Payton, P., Fei, Z., McQuinn, R., Debbie, P., Martin, G. B., et al. (2005). Transcriptome and selected metabolite analyses reveal multiple points of ethylene control during tomato fruit development. Plant Cell 17, 2954–2965. doi: 10.1105/tpc.105.036053
Altmann, F. (2007). The role of protein glycosylation in allergy. Int. Arch. Allergy Immunol. 142, 99–115. doi: 10.1159/000096114
Audran-Delalande, C., Bassa, C., Mila, I., Regad, F., Zouine, M., and Bouzayen, M. (2012). Genome-wide identification, functional analysis and expression profiling of the Aux/IAA gene family in Tomato. Plant Cell Physiol. 53, 659–672. doi: 10.1093/pcp/pcs022
Bellande, K., Bono, J.-J., Savelli, B., Jamet, E., and Canut, H. (2017). Plant lectins and lectin receptor-like kinases: how do they sense the outside? Int. J. Mol. Sci. 18:1164. doi: 10.3390/ijms18061164
Bencúr, P., Steinkellner, H., Svoboda, B., Mucha, J., Strasser, R., Kolarich, D., et al. (2005). Arabidopsis thaliana beta1,2-xylosyltransferase: an unusual glycosyltransferase with the potential to act at multiple stages of the plant N-glycosylation pathway. Biochem. J. 388, 515–525. doi: 10.1042/bj20042091
Bondili, J. S., Castilho, A., Mach, L., Glössl, J., Steinkellner, H., Altmann, F., et al. (2006). Molecular cloning and heterologous expression of beta1,2-xylosyltransferase and core alpha1,3-fucosyltransferase from maize. Phytochemistry 67, 2215–2224. doi: 10.1016/j.phytochem.2006.07.007
Bosques, C. J., Tschampel, S. M., Woods, R. J., and Imperiali, B. (2004). Effects of glycosylation on peptide conformation: a synergistic experimental and computational study. J. Am. Chem. Soc. 126, 8421–8425. doi: 10.1021/ja0496266
Boyes, D. C., Zayed, A. M., Ascenzi, R., McCaskill, A. J., Hoffman, N. E., Davis, K. R., et al. (2001). Growth stage-based phenotypic analysis of Arabidopsis: a model for high throughput functional genomics in plants. Plant Cell 13, 1499–1510. doi: 10.1105/tpc.13.7.1499
Brewer, C. F., and Bhattacharyya, L. (1986). Specificity of concanavalin A binding to asparagine-linked glycopeptides. A nuclear magnetic relaxation dispersion study. J. Biol. Chem. 261, 7306–7310. doi: 10.1016/s0021-9258(17)38391-6
Chakraborty, J., Ghosh, P., and Das, S. (2018). Autoimmunity in plants. Planta 248, 751–767. doi: 10.1007/s00425-018-2956-0
Chen, S., Hofius, D., Sonnewald, U., and Börnke, F. (2003). Temporal and spatial control of gene silencing in transgenic plants by inducible expression of double-stranded RNA. Plant J. 36, 731–740. doi: 10.1046/j.1365-313x.2003.01914.x
Chung, C. H., Mirakhur, B., Chan, E., Le, Q. T., Berlin, J., Morse, M., et al. (2008). Cetuximab-induced anaphylaxis and IgE specific for galactose-alpha-1,3-galactose. N. Engl. J. Med. 358, 1109–1117.
Denecke, J., De Rycke, R., and Botterman, J. (1992). Plant and mammalian sorting signals for protein retention in the endoplasmic reticulum contain a conserved epitope. EMBO J. 11, 2345–2355. doi: 10.1002/j.1460-2075.1992.tb05294.x
Diepold, A., Li, G., Lennarz, W. J., Nurnberger, T., and Brunner, F. (2007). The Arabidopsis AtPNG1 gene encodes a peptide: N-glycanase. Plant J. 52, 94–104. doi: 10.1111/j.1365-313x.2007.03215.x
Downing, W. L., Galpin, J. D., Clemens, S., Lauzon, S. M., Samuels, A. L., Pidkowich, M. S., et al. (2006). Synthesis of enzymatically active human alpha-L-iduronidase in Arabidopsis cgl (complex glycan-deficient) seeds. Plant Biotechnol. J. 4, 169–181. doi: 10.1111/j.1467-7652.2005.00166.x
Engering, A. J., Cella, M., Fluitsma, D. M., Hoefsmit, E. C., Lanzavecchia, A., and Pieters, J. (1997). Mannose receptor mediated antigen uptake and presentation in human dendritic cells. Adv. Exp. Med. Biol. 417, 183–187. doi: 10.1007/978-1-4757-9966-8_31
Eyal, E., and Levy, A. A. (2002). Tomato mutants as tools for functional genomics. Curr. Opin. Plant Biol. 5, 112–117. doi: 10.1016/s1369-5266(02)00237-6
Fanata, W. I. D., Lee, K. H., Son, B. H., Yoo, J. Y., Harmoko, R., Ko, K. S., et al. (2013). N-glycan maturation is crucial for cytokinin-mediated development and cellulose synthesis in Oryza sativa. Plant J. 73, 966–979. doi: 10.1111/tpj.12087
Faske, M., Backhausen, J. E., Sendker, M., Singer-Bayrle, M., Scheibe, R., and von Schaewen, A. (1997). Transgenic tobacco plants expressing pea chloroplast Nmdh cDNA in sense and antisense orientation: effects on NADP-MDH level, stability of transformants, and plant growth. Plant Physiol. 115, 705–715. doi: 10.1104/pp.115.2.705
Faugeron, C., Sakr, S., Lhernould, S., Michalski, J.-C., Delrot, S., and Morvan, H. (1999). Long-distance transport and metabolism of unconjugated N-glycans in tomato plants. J. Exp. Bot. 50, 1669–1675. doi: 10.1093/jxb/50.340.1669
Faye, L., and Chrispeels, M. J. (1985). Characterization of N-linked oligosaccharides by affinoblotting with Concanavalin A-peroxidase and treatment of the blots with glycosidases. Anal. Biochem. 149, 218–244. doi: 10.1016/0003-2697(85)90498-1
Felix, G., Duran, J. D., Volko, S., and Boller, T. (1999). Plants have a sensitive perception system for the most conserved domain of bacterial flagellin. Plant J. 18, 265–276. doi: 10.1046/j.1365-313x.1999.00265.x
Fitchette-Lainé, A. C., Gomord, V., Cabanes, M., Michalski, J. C., Saint Macary, M., Foucher, B., et al. (1997). N-glycans harboring the Lewis a epitope are expressed at the surface of plant cells. Plant J. 12, 1411–1417. doi: 10.1046/j.1365-313x.1997.12061411.x
Frank, J., Kaulfürst-Soboll, H., Rips, S., Koiwa, H., and von Schaewen, A. (2008). Comparative analyses of Arabidopsis complex glycan 1 mutants and genetic interaction with staurosporin & temperature-sensitive 3a. Plant Physiol. 148, 1354–1367. doi: 10.1104/pp.108.127027
Frank, M., Kaulfürst-Soboll, H., Fischer, K., and von Schaewen, A. (2021). Complex-type n-glycans influence the root hair landscape of arabidopsis seedlings by altering the auxin output. Front. Plant Sci. 12:635714. doi: 10.3389/fpls.2021.635714
Frederiksen, R. F., Paspaliari, D. K., Larsen, T., Storgaard, B. G., Larsen, M. H., Ingmer, H., et al. (2013). Bacterial chitinases and chitin-binding proteins as virulence factors. Microbiology 159, 833–847. doi: 10.1099/mic.0.051839-0
George, W. L., Scott, J., and Splittstoesser, W. E. (1984). Parthenocarpy in tomato. Hort Rev. 6, 65–84. doi: 10.1002/9781118060797.ch2
Gomord, V., Chamberlain, P., Jefferis, R., and Faye, L. (2005). Biopharmaceutical production in plants: problems, solutions and opportunities. Trends Biotechnol. 23, 559–565. doi: 10.1016/j.tibtech.2005.09.003
Gomord, V., and Faye, L. (2004). Posttranslational modification of therapeutic proteins in plants. Curr. Opin. Plant Biol. 7, 171–181. doi: 10.1016/j.pbi.2004.01.015
Guillon, F., Philippe, S., Bouchet, B., Devaux, M. F., Frasse, P., Jones, B., et al. (2008). Down-regulation of an Auxin Response Factor in the tomato induces modification of fine pectin structure and tissue architecture. J. Exp. Bot. 59, 273–288. doi: 10.1093/jxb/erm323
Handa, A. K., Singh, N. K., and Biggs, M. S. (1985). Effect of tunicamycin on in vitro ripening of tomato pericarp tissue. Physiol. Plant. 63, 417–424. doi: 10.1111/j.1399-3054.1985.tb02320.x
Hao, Y., Hu, G., Breitel, D., Liu, M., Mila, I., Frasse, P., et al. (2015). Auxin response factor slarf2 is an essential component of the regulatory mechanism controlling fruit ripening in Tomato. PLoS Genetics 11:e1005649. doi: 10.1371/journal.pgen.1005649
Harmoko, R., Yoo, J. Y., Ko, K. S., Ramasamy, N. K., Hwang, B. Y., Lee, E. J., et al. (2016). N-glycan containing a core (1,3-fucose residue is required for basipetal auxin transport and gravitropic response in rice (Oryza sativa). New Phytol. 212, 108–122. doi: 10.1111/nph.14031
Harpaz, N., and Schachter, H. (1980). Control of glycoprotein synthesis. Bovine colostrum UDP-Nacetylglucosamine:(-D-mannoside (2-N-acetylglucosaminyltransferase I. Separation from UDP-Nacetylglucosamine:(-D-mannoside (2-N-acetylglucosaminyltransferase II, partial purification, and substrate specificity. J. Biol. Chem. 255, 4885–4893. doi: 10.1016/s0021-9258(19)85579-5
Häweker, H., Rips, S., Koiwa, H., Salomon, S., Saijo, Y., Chinchilla, D., et al. (2010). Pattern recognition receptors require N-glycosylation to mediate plant immunity. J. Biol. Chem. 285, 4629–4636. doi: 10.1074/jbc.m109.063073
Hegenauer, V., Fürst, U., Kaiser, B., Smoker, M., Zipfel, C., Felix, G., et al. (2016). Detection of the plant parasite Cuscuta reflexa by a tomato cell surface receptor. Science 353, 478–481.
Hegenauer, V., Slaby, P., Körner, M., Bruckmüller, J. A., Burggraf, R., Albert, I., et al. (2020). The tomato receptor CuRe1 senses a cell wall protein to identify Cuscuta as a pathogen. Nat. Commun. 11:5299.
Höfgen, R., and Willmitzer, L. (1990). Biochemical and genetic analysis of different patatin isoforms expressed in various organs of potato (Solanum tuberosum). Plant Sci. 66, 221–230. doi: 10.1016/0168-9452(90)90207-5
Hossain, M. A., Nakano, R., Nakamura, K., and Kimura, Y. (2010). Molecular identification and characterization of an acidic peptide:N-glycanase from tomato (Lycopersicum esculentum) fruits. J. Biochem. 147, 157–165. doi: 10.1093/jb/mvp157
Howell, S. H. (2013). Endoplasmic reticulum stress responses in plants. Annu. Rev. Plant Biol. 64, 477–499. doi: 10.1146/annurev-arplant-050312-120053
Jones, B., Frasse, P., Olmos, E., Zegzouti, H., Li, Z. G., Latché, A., et al. (2002). Down-regulation of DR12, an auxin-response-factor homolog, in the tomato results in a pleiotropic phenotype including dark green and blotchy ripening fruit. Plant J. 32, 603–613. doi: 10.1046/j.1365-313x.2002.01450.x
Kajiura, H., Koiwa, H., Nakazawa, Y., Okazawa, A., Kobayashi, A., Seki, T., et al. (2010). Two Arabidopsis thaliana Golgi alpha-mannosidase I enzymes are responsible for plant N-glycan maturation. Glycobiology 20, 235–247. doi: 10.1093/glycob/cwp170
Kang, J. S., Frank, J., Kang, C. H., Kajiura, H., Vikram, M., Ueda, A., et al. (2008). Salt tolerance of Arabidopsis thaliana requires maturation of N-glycosylated proteins in the Golgi apparatus. Proc. Natl. Acad. Sci. U.S.A. 105, 5933–5938. doi: 10.1073/pnas.0800237105
Kaulfürst-Soboll, H., Mertens, M., Brehler, R., and von Schaewen, A. (2011a). Reduction of Cross-reactive Carbohydrate Determinants in plant foodstuff: elucidation of clinical relevance and implications for allergy diagnosis. PLoS One 6:e17800. doi: 10.1371/journal.pone.0017800
Kaulfürst-Soboll, H., Rips, S., Koiwa, H., Kajiura, H., Fujiyama, K., and von Schaewen, A. (2011b). Reduced immunogenicity of Arabidopsis hybrid glycosylation1 (hgl1) N-glycans due to altered accessibility of xylose and core fucose epitopes. J. Biol. Chem. 286, 22955–22964. doi: 10.1074/jbc.m110.196097
Klann, E. M., Chetelat, R. T., and Bennett, A. B. (1993). Expression of acid invertase gene controls sugar composition in Tomato (Lycopersicon) fruit. Plant Physiol. 103, 863–870. doi: 10.1104/pp.103.3.863
Ko, K., Tekoah, Y., Rudd, P. M., Harvey, D. J., Dwek, R. A., Spitsin, S., et al. (2003). Function and glycosylation of plant-derived antiviral monoclonal antibody. Proc. Natl. Acad. Sci. U.S.A. 100, 8013–8018. doi: 10.1073/pnas.0832472100
Kornfeld, R., and Kornfeld, S. (1985). Assembly of asparagine-linked oligosaccharides. Annu. Rev. Biochem. 54, 631–664. doi: 10.1146/annurev.bi.54.070185.003215
Kumar, R., Khurana, A., and Sharma, A. K. (2014). Role of plant hormones and their interplay in development and ripening of fleshy fruits. J. Exp. Bot. 65, 4561–4575. doi: 10.1093/jxb/eru277
Lannoo, N., and van Damme, E. J. M. (2015). N-glycans: the making of a varied toolbox. Plant Sci. 239, 67–83. doi: 10.1016/j.plantsci.2015.06.023
Laurière, M., Laurière, C., Chrispeels, M. J., Johnson, K. D., and Sturm, A. (1989). Characterization of a Xylose-specific antiserum that reacts with the complex asparagin-linked glycans of extracellular and vacuolar glycoproteins. Plant Physiol. 90, 1182–1188. doi: 10.1104/pp.90.3.1182
Leibfried, A., To, J. P. C., Busch, W., Stehling, S., Kehle, A., Demar, M., et al. (2005). WUSCHEL controls meristem function by direct regulation of cytokinin-inducible response regulators. Nature 438, 1172–1175. doi: 10.1038/nature04270
Léonard, R., Costa, G., Darrambide, E., Lhernould, S., Fleurat-Lessard, P., Carlué, M., et al. (2002). The presence of Lewis a epitopes in Arabidopsis thaliana glycoconjugates depends on an active alpha4-fucosyltransferase gene. Glycobiology 12, 299–306. doi: 10.1093/glycob/12.5.299
Lerouge, P., Cabanes-Macheteau, M., Rayon, C., Fischette-Lainé, A. C., Gomord, V., and Faye, L. (1998). N-glycoprotein biosynthesis in plants: recent developments and future trends. Plant Mol. Biol. 38, 31–48. doi: 10.1007/978-94-011-5298-3_2
Liebminger, E., Hüttner, S., Vavra, U., Fischl, R., Schoberer, J., Grass, J., et al. (2009). Class I α-mannosidases are required for N-glycan processing and root development in Arabidopsis thaliana. Plant Cell 21, 3850–3867. doi: 10.1105/tpc.109.072363
Lizada, C. C., and Yang, S. F. (1979). A simple and sensitive assay for 1-aminocyclopropane-1-carboxylic acid. Anal. Biochem. 100, 140–145. doi: 10.1016/0003-2697(79)90123-4
Maeda, M., and Kimura, Y. (2014). Structural features of free N-glycans occurring in plants and functional features of de-N-glycosylation enzymes, ENGase, and PNGase: the presence of unusual plant complex type N-glycans. Front. Plant Sci. 5:429. doi: 10.3389/fpls.2014.00429
Martí, E., Gisbert, C., Bishop, G. J., Dixon, M. S., and García-Martínez, J. L. (2006). Genetic and physiological characterization of tomato cv. Micro-Tom. J. Exp. Bot. 57, 2037–2047. doi: 10.1093/jxb/erj154
Martineau, B., Houck, C. M., Sheehy, R. E., and Hiatt, W. R. (1994). Fruit-specific expression of the A. tumefaciens isopentenyl transferase gene in tomato: effects on fruit ripening and defense-related gene expression in leaves. Plant J. 5, 11–19. doi: 10.1046/j.1365-313x.1994.5010011.x
Martinelli, F., Uratsu, S. L., Reagan, R. L., Chen, Y., Tricoli, D., Fiehn, O., et al. (2009). Gene regulation in parthenocarpic tomato fruit. J. Exp. Bot. 60, 3873–3890. doi: 10.1093/jxb/erp227
Mazzucato, A., Taddei, A. R., and Soressi, G. P. (1998). The parthenocarpic fruit (pat) mutant of tomato (Lycopersicon esculentum Mill.) sets seedless fruits and has aberrant anther and ovule development. Development 125, 107–114.
Meir, S., Philosoph-Hadas, S., Sundaresan, S., Selvaraj, K. S. V., Burd, S., Ophir, R., et al. (2010). Microarray analysis of the abscission-related transcriptome in the tomato flower abscission zone in response to auxin depletion. Plant Physiol. 154, 1929–1956. doi: 10.1104/pp.110.160697
Meissner, R., Jacobson, Y., Melamed, S., Levyatuv, S., Shalev, G., Ashri, A., et al. (1997). A new model system for tomato genetics. Plant J. 12, 1465–1472. doi: 10.1046/j.1365-313x.1997.12061465.x
Mertens, M., Amler, S., Moerschbacher, B. M., and Brehler, R. (2010). Cross-reactive carbohydrate determinants strongly affect the results of the basophil activation test in hymenoptera-venom allergy. Clin. Exp. Allergy 40, 1333–1345. doi: 10.1111/j.1365-2222.2010.03535.x
Mito, N., Wimmers, L. E., and Bennett, A. B. (1996). Sugar regulates mRNA abundance of H+-ATPase gene family members in Tomato. Plant Physiol. 112, 1229–1236. doi: 10.1104/pp.112.3.1229
Mizrahi, J., Dostal, H. C., and Cherry, J. H. (1976). Descriptive physiology and biochemistry of the abnormally ripening Tomato Fruit (Lycopersicon esculentum) cv. Snowball. Physiol Plant 38, 309–312. doi: 10.1111/j.1399-3054.1976.tb04010.x
Mócsai, R., Figl, R., Sützl, L., Fluch, S., and Altmann, F. (2020). A first view on the unsuspected intragenus diversity of N-glycans in Chlorella microalgae. Plant J. 103, 184–196. doi: 10.1111/tpj.14718
Moon, H., and Callahan, A. M. (2004). Developmental regulation of peach ACC oxidase promoter-GUS fusions in transgenic tomato fruits. J. Exp. Bot. 55, 1519–1528. doi: 10.1093/jxb/erh162
Müller, B., and Sheen, J. (2008). Cytokinin and auxin interaction in root stem-cell specification during early embryogenesis. Nature 453, 1094–1097. doi: 10.1038/nature06943
Nagashima, Y., Ma, Z., Liu, X., Qian, X., Zhang, X., von Schaewen, A., et al. (2020). Multiple quality control mechanisms in the ER and TGN determine subcellular dynamics and salt-stress tolerance function of KORRIGAN1. Plant Cell 32, 470–485. doi: 10.1105/tpc.19.00714
Nagashima, Y., von Schaewen, A., and Koiwa, H. (2018). Function of N-glycosylation in plants. Plant Sci. 274, 70–79. doi: 10.1016/j.plantsci.2018.05.007
Nakamura, K., Inoue, M., Yoshiie, T., Hosoi, K., and Kimura, Y. (2008). Changes in structural features of free N-glycan and endoglycosidase activity during tomato fruit ripening. Biosci. Biotechnol. Biochem. 72, 2936–2945. doi: 10.1271/bbb.80414
Napier, R. M., and Venis, M. A. (1991). From auxin-binding protein to plant hormone receptor? Trends Biochem. Sci. 2, 72–75. doi: 10.1016/0968-0004(91)90028-t
Nitsch, J. P. (1970). “Hormonal factors in growth and development,” in Food Science and Technology, Vol. 1, ed. A. C. Hulme (London: Academic Press), 427–472.
Oppenheimer, C. L., and Hill, R. L. (1981). Purification and characterization of a rabbit liver (1,3 mannoside (-2 Nacetylgiucosaminyl transferase. J. Biol. Chem. 256, 799–804. doi: 10.1016/s0021-9258(19)70047-7
Pagny, S., Cabanes-Macheteau, M., Gillikin, J. W., Leborgne-Castel, N., Lerouge, P., Boston, R. S., et al. (2000). Protein recycling from the Golgi apparatus to the endoplasmic reticulum in plants and its minor contribution to calreticulin retention. Plant Cell 12, 739–756. doi: 10.2307/3870998
Pandolfini, T. (2009). Seedless fruit production by hormonal regulation of fruit set. Nutrients 1, 168–177. doi: 10.3390/nu1020168
Priem, B., and Gross, K. C. (1992). Mannosyl- and xylosyl-containing glycans promote tomato (Lycopersicon esculentum Mill.) fruit ripening. Plant Physiol. 98, 399–401. doi: 10.1104/pp.98.1.399
Reichardt, S., Piepho, H. P., Stintzi, A., and Schaller, A. (2020). Peptide signaling for drought-induced tomato flower drop. Science 367, 1482–1485. doi: 10.1126/science.aaz5641
Rips, S., Bentley, N., Jeong, I. S., Welch, J. L., von Schaewen, A., and Koiwa, H. (2014). Multiple N-glycans cooperate in the subcellular targeting and functioning of Arabidopsis KORRIGAN1. Plant Cell 26, 3792–3808. doi: 10.1105/tpc.114.129718
Saint-Jore-Dupas, C., Gomord, V., and Paris, N. (2004). Protein localization in the plant Golgi apparatus and the trans-Golgi network. Cell Mol. Life. Sci. 61, 159–171. doi: 10.1007/s00018-003-3354-7
Schoberer, J., and Strasser, R. (2018). Plant glyco-biotechnology. Semin. Cell Dev. Biol. 80, 133–141. doi: 10.1016/j.semcdb.2017.07.005
Scott, J. W., and Harbaugh, B. K. (1989). Micro-tom. a miniature dwarf tomato. Fla. Agric. Exp. Stn. Circ. S-370, 1–6.
Serrani, J. C., Fos, M., Atarés, A., and García-Martínez, J. L. (2007). Effect of gibberellin and auxin on parthenocarpic fruit growth induction in the cv Micro-Tom of tomato. J. Plant Growth Regul. 26, 211–221. doi: 10.1007/s00344-007-9014-7
Shah, N., Kuntz, D. A., and Rose, D. R. (2008). Golgi alpha-mannosidase II cleaves two sugars sequentially in the same catalytic site. Proc. Natl. Acad. Sci. U.S.A. 105, 9570–9575. doi: 10.1073/pnas.0802206105
Sharma, K., Gupta, S., Sarma, S., Rai, M., Sreelakshmi, Y., and Sharma, R. (2020). Mutations in Tomato ACC synthase2 uncover its role in development beside fruit ripening. bioRxiv [Preprint] doi: 10.1101/2020.05.12.090431
Sokolovsky, V., Kaldenhoff, R., Ricci, M., and Russo, V. E. A. (1990). Fast and reliable mini-prep RNA extraction from Neurospora crassa. Fungal Genet. Newslett. 67, 41–43.
Sravankumar, T., Akash, Naik, N., and Kumar, R. (2018). A ripening-induced SlGH3-2 gene regulates fruit ripening via adjusting auxin-ethylene levels in tomato (Solanum lycopersicum L.). Plant Mol. Biol. 98, 455–469. doi: 10.1007/s11103-018-0790-1
Srivastava, A., and Handa, A. K. (2005). Hormonal regulation of tomato fruit development: a molecular perspective. J. Plant Growth Regul. 24, 67–82. doi: 10.1007/s00344-005-0015-0
Strasser, R. (2016). Plant protein glycosylation. Glycobiology 26, 926–939. doi: 10.1093/glycob/cww023
Strasser, R., Altmann, F., Mach, L., Glössl, J., and Steinkellner, H. (2004). Generation of Arabidopsis thaliana plants with complex N-glycans lacking beta 1,2-linked xylose and core alpha 1,3-linked fucose. FEBS Lett. 561, 132–136. doi: 10.1016/s0014-5793(04)00150-4
Strasser, R., Bondili, J. S., Schoberer, J., Svoboda, B., Liebminger, E., Glössl, J., et al. (2007). Enzymatic properties and subcellular localization of Arabidopsis beta-N-acetylhexosaminidases. Plant Physiol. 145, 5–16. doi: 10.1104/pp.107.101162
Strasser, R., Mucha, J., Macha, L., Altmann, F., Wilson, I. B. H., Glössl, J., et al. (2000). Molecular cloning and functional expression of β1,2-xylosyltransferase cDNA from Arabidopsis thaliana. FEBS Lett. 472, 105–108. doi: 10.1016/s0014-5793(00)01443-5
Strasser, R., Schoberer, J., Jin, C., Glössl, J., Mach, L., and Steinkellner, H. (2006). Molecular cloning and characterization of Arabidopsis thaliana Golgi alpha-mannosidase II, a key enzyme in the formation of complex N-glycans in plants. Plant J. 45, 789–803. doi: 10.1111/j.1365-313x.2005.02648.x
Strasser, R., Stadlmann, J., Schähs, M., Stiegler, G., Quendler, H., Mach, L., et al. (2008). Generation of glyco-engineered Nicotiana benthamiana for the production of monoclonal antibodies with a homogeneous human-like N-glycan structure. Plant Biotechnol. J. 6, 392–402. doi: 10.1111/j.1467-7652.2008.00330.x
Sun, Y., Qiao, Z., Muchero, W., and Chen, J. G. (2020). Lectin receptor-like kinases: the sensor and mediator at the plant cell surface. Front. Plant Sci. 11:596301. doi: 10.3389/fpls.2020.596301
Takahashi, N., Hotta, T., Ishihara, H., Mori, M., Teiima, S., Bligny, R., et al. (1986). Xylose-containing common structural unit in N-linked oligosaccharides of laccase from sycamore cells. Biochemistry 25, 388–395. doi: 10.1021/bi00350a018
To, J. P. C., Deruère, J., Maxwell, B. B., Morris, V. F., Hutchison, C. E., Ferreira, F. J., et al. (2007). Cytokinin regulates type-A Arabidopsis response regulator activity and protein stability via two-component phosphorelay. Plant Cell 19, 3901–3914. doi: 10.1105/tpc.107.052662
Tretter, V., Altmann, F., and März, L. (1991). Peptide-N4-(N-acetyl-beta-glucosaminyl)asparagine amidase F cannot release glycans with fucose attached alpha 1-3 to the asparagine-linked N acetylglucosamine residue. Eur. J. Biochem. 199, 647–652. doi: 10.1111/j.1432-1033.1991.tb16166.x
Trivedi, P., Nguyen, N., Hykkerud, A. L., Häggman, H., Martinussen, I., Jaakola, L., et al. (2019). Developmental and environmental regulation of cuticular wax biosynthesis in fleshy fruits. Front. Plant Sci. 10:431. doi: 10.3389/fpls.2019.00431
Tsujimori, Y., Ogura, M., Rahman, M. Z., Maeda, M., and Kimura, Y. (2019). Plant complex type free N-glycans occur in tomato xylem sap. Biosci. Biotech. Biochem. 83, 1310–1314. doi: 10.1080/09168451.2019.1608803
Uemura, R., Ogura, M., Matsumaru, C., Akiyama, T., Maeda, M., and Kimura, Y. (2018). Novel assay system for acidic Peptide:N-glycanase (aPNGase) activity in crude plant extract. Biosci. Biotechnol. Biochem. 82, 1172–1175. doi: 10.1080/09168451.2018.1459464
van den Elsen, J. M., Kuntz, D. A., and Rose, D. R. (2001). Structure of Golgi alpha-mannosidase II: a target for inhibition of growth and metastasis of cancer cells. EMBO J. 20, 3008–3017. doi: 10.1093/emboj/20.12.3008
Van Holle, S., and Van Damme, E. J. M. (2018). Signaling through plant lectins: modulation of plant immunity and beyond. Biochem. Soc. Trans. 46, 217–233. doi: 10.1042/bst20170371
van Wersch, R., Li, X., and Zhang, Y. (2016). Mighty dwarfs: Arabidopsis autoimmune mutants and their usages in genetic dissection of plant immunity. Front. Plant Sci. 7:1717. doi: 10.3389/fpls.2016.01717
Vanholme, B., Vanholme, R., Turumtay, H., Goeminne, G., Cesarino, I., Goubet, F., et al. (2014). Accumulation of N-acetylglucosamine oligomers in the plant cell wall affects plant architecture in a dose-dependent and conditional manner. Plant Physiol. 165, 290–308. doi: 10.1104/pp.113.233742
Varki, A., Cummings, R. D., Esko, J. D., Freeze, H. H., Stanley, P., Bertozzi, C. R., et al. (2009). Essentials of Glycobiology, 2nd Edn. New York, NY: Cold Spring Harbor Laboratory Press.
von Schaewen, A., Rips, S., Jeong, I. S., and Koiwa, H. (2015). Arabidopsis thaliana KORRIGAN1 protein: N-glycan modification, localization, and function in cellulose biosynthesis and osmotic stress responses. Plant Signal. Behav. 10:e1024397. doi: 10.1080/15592324.2015.1024397
von Schaewen, A., Sturm, A., O’Neill, J., and Chrispeels, M. (1993). Isolation of a mutant Arabidopsis plant that lacks N-acetyl glucosaminyl transferase I and is unable to synthesize Golgi-modified complex N-linked glycans. Plant Physiol. 102, 1109–1118. doi: 10.1104/pp.102.4.1109
Walsh, G., and Jefferis, R. (2006). Post-translational modifications in the context of therapeutic proteins. Nat. Biotechnol. 24, 1241–1252. doi: 10.1038/nbt1252
Walter, M., Chaban, C., Schütze, K., Batistiè, O., Weckermann, K., Näke, C., et al. (2004). Visualization of protein interactions in living plant cells using bimolecular fluorescence complementation. Plant J. 40, 428–438.
Wan, W.-L., Zhang, L., Pruitt, R., Zaidem, M., Brugman, R., Ma, X., et al. (2019). Comparing Arabidopsis receptor kinase and receptor protein-mediated immune signaling reveals BIK1-dependent differences. New Phytol. 221, 2080–2095. doi: 10.1111/nph.15497
Wang, H., Jones, B., Li, Z., Frasse, P., Delalande, C., Regad, F., et al. (2005). The Tomato Aux/IAA transcription factor IAA9 Is involved in fruit development and leaf morphogenesis. Plant Cell 17, 2676–2692. doi: 10.1105/tpc.105.033415
Wang, K. L., Li, H., and Ecker, J. R. (2002). Ethylene biosynthesis and signaling networks. Plant Cell 14, S131–S151.
Wang, X., Inoue, S., Gu, J., Miyoshi, E., Noda, K., Li, W., et al. (2005). Dysregulation of TGF-beta1 receptor activation leads to abnormal lung development and emphysema-like phenotype in core fucose-deficient mice. Proc. Natl. Acad. Sci. U.S.A. 102, 15791–15796. doi: 10.1073/pnas.0507375102
Wanke, A., Malisic, M., Wawra, S., and Zuccaro, A. (2021). Unraveling the sugar code: the role of microbial extracellular glycans in plant-microbe interactions. J. Exp. Bot. 72, 15–35. doi: 10.1093/jxb/eraa414
Wenderoth, I., and von Schaewen, A. (2000). Isolation and characterization of plant N-acetylglucosaminyltransferase I (GntI) cDNA sequences. Functional analyses in the Arabidopsis cgl mutant and in antisense plants. Plant Physiol. 123, 1097–1108. doi: 10.1104/pp.123.3.1097
Wilson, I. B., Rendiæ, D., Freilinger, A., Dumiæ, J., Altmann, F., Mucha, J., et al. (2001). Cloning and expression of cDNAs encoding alpha1,3-fucosyltransferase homologues from Arabidopsis thaliana. Biochim. Biophys. Acta 1527, 88–96. doi: 10.1016/s0304-4165(01)00151-9
Yunovitz, H., and Gross, K. C. (1994a). Delay of tomato fruit ripening by an oligosaccharide N-glycan. Interactions with IAA, galactose and lectins. Physiol. Plant 90, 152–156. doi: 10.1034/j.1399-3054.1994.900122.x
Yunovitz, H., and Gross, K. C. (1994b). Effect of tunicamycin on metabolism of unconjugated N-glycans in relation to regulation of tomato fruit ripening. Phytochemistry 37, 663–668. doi: 10.1016/s0031-9422(00)90334-0
Yunovitz, H., Livsey, J. N., and Gross, K. C. (1996). Unconjugated Man5GlcNAc occurs in vegetative tissues of tomato. Phytochemistry 42, 607–610. doi: 10.1016/0031-9422(95)00956-6
Keywords: auxin-like effects, complex N-glycans, GNTI, hormone signaling, free N-glycans, fruit abscission, fruit ripening, MANII
Citation: Kaulfürst-Soboll H, Mertens-Beer M, Brehler R, Albert M and von Schaewen A (2021) Complex N-Glycans Are Important for Normal Fruit Ripening and Seed Development in Tomato. Front. Plant Sci. 12:635962. doi: 10.3389/fpls.2021.635962
Received: 30 November 2020; Accepted: 25 January 2021;
Published: 09 March 2021.
Edited by:
Richard Strasser, University of Natural Resources and Life Sciences Vienna, AustriaReviewed by:
Yoshinobu Kimura, Okayama University, JapanCopyright © 2021 Kaulfürst-Soboll, Mertens-Beer, Brehler, Albert and von Schaewen. This is an open-access article distributed under the terms of the Creative Commons Attribution License (CC BY). The use, distribution or reproduction in other forums is permitted, provided the original author(s) and the copyright owner(s) are credited and that the original publication in this journal is cited, in accordance with accepted academic practice. No use, distribution or reproduction is permitted which does not comply with these terms.
*Correspondence: Antje von Schaewen, c2NoYWV3ZW5AdW5pLW11ZW5zdGVyLmRl; c2NoYWV3ZW5Ad3d1LmRl
†Present address: Melanie Mertens-Beer, Allergopharma GmbH & Co. KG, Reinbek, Germany
Disclaimer: All claims expressed in this article are solely those of the authors and do not necessarily represent those of their affiliated organizations, or those of the publisher, the editors and the reviewers. Any product that may be evaluated in this article or claim that may be made by its manufacturer is not guaranteed or endorsed by the publisher.
Research integrity at Frontiers
Learn more about the work of our research integrity team to safeguard the quality of each article we publish.