- 1Institute of Food Science and Technology, Chinese Academy of Agricultural Sciences, Beijing, China
- 2Laboratory of Quality & Safety Risk Assessment on Agro-products Processing, Ministry of Agriculture and Rural Affairs, Beijing, China
- 3Institute of Medicinal Plant Sciences, Sanming Academy of Agricultural Sciences, Sanming, China
Dendrobium candidum is used as a traditional Chinese medicine and as a raw material in functional foods. D. candidum stems are green or red, and red stems are richer in anthocyanins. Light is an important environmental factor that induces anthocyanin accumulation in D. candidum. However, the underlying molecular mechanisms have not been fully unraveled. In this study, we exposed D. candidum seedlings to two different light intensities and found that strong light increased the anthocyanin content and the expression of genes involved in anthocyanin biosynthesis. Through transcriptome profiling and expression analysis, we identified a WD40-repeat transcription factor, DcTTG1, whose expression is induced by light. Yeast one-hybrid assays showed that DcTTG1 binds to the promoters of DcCHS2, DcCHI, DcF3H, and DcF3′H, and a transient GUS activity assay indicated that DcTTG1 can induce their expression. In addition, DcTTG1 complemented the anthocyanin deficiency phenotype of the Arabidopsis thaliana ttg1-13 mutant. Collectively, our results suggest that light promotes anthocyanin accumulation in D. candidum seedlings via the upregulation of DcTTG1, which induces anthocyanin synthesis-related gene expression.
Introduction
Dendrobium candidum, a perennial herb of the orchid family, has been used for thousands of years in China as a traditional Chinese medicine and in functional foods (Ng et al., 2012), including teas, juices, wines, stews, and soups (Wei et al., 2016). D. candidum stems are green or red, with the latter having a higher anthocyanin content. Anthocyanins have a high nutritional value and are beneficial for human health (de Pascual-Teresa and Sanchez-Ballesta, 2008). Further, they have a broad spectrum of medicinal effects, including protection against liver injury, reduction of blood pressure, improvement of eyesight, and strong anti-inflammatory activity and anticancer properties (Konczak and Zhang, 2004). In addition, anthocyanins function to attract insect pollinators and protect plants against environmental stresses. However, the gene transcription regulatory mechanism underlying color formation in D. candidum stems has not been fully unraveled.
Anthocyanin biosynthesis is catalyzed by a series of enzymes, including chalcone isomerase (CHI), flavanone 3-hydroxylase (F3H), flavonoid 3′-hydroxylase (F3′H), flavonoid 3′,5′-hydroxylase (F3′5′H), dihydroflavonol 4-reductase (DFR), and anthocyanin synthase (ANS). These enzymes are encoded by structural genes, the expression of which is regulated by transcription factors (TFs; Broun, 2005). Key anthocyanin biosynthetic enzymes have been extensively studied (Zhang et al., 2014). Anthocyanin biosynthesis starts from the flavonoid synthesis pathway with the formation of chalcone from 4-coumaroyl CoA and malonyl CoA by chalcone synthase (CHS). Anthocyanidins undergo glucose-flavonoid 3-O-glucosyl transferase (UFGT) modification to increase their stability (Holton and Cornish, 1995; Zhang et al., 2014). Anthocyanin aglycones include delphinidin, cyanidin, pelargonidin, peonidin, malvidin, and petunidin (Cabrita et al., 2000).
Numerous TFs regulating plant anthocyanin biosynthesis have been identified to date. They include three main types: MYB TFs, basic helix-loop-helix (bHLH) factors, and WD40-repeat proteins. MYB-bHLH-WD40 (MBW) complexes play an important role in anthocyanin biosynthesis in various plant species (Xu et al., 2015). Environmental biotic and abiotic factors play important roles in plant secondary metabolism, including anthocyanin, flavonoid, and polysaccharide metabolism. In Arabidopsis thaliana, environmental stress factors, such as light, cold, and drought stresses and pathogen invasion, enhance anthocyanin accumulation (Chalker-Scott, 1999). Light is one of the most important environmental factors regulating anthocyanin biosynthesis in plants. Light-regulated anthocyanin synthesis is mostly achieved through the regulation of specific TFs (Allan et al., 2008; Bai et al., 2019; Ni et al., 2019). In many fruit-bearing plants, such as pear, apple, Chinese bayberry, litchi, and eggplant, anthocyanin accumulation involves the light-mediated TF R2R3 MYB (Takos et al., 2006; Niu et al., 2010; Lai et al., 2014; Jiang et al., 2016; Bai et al., 2017). The basic leucine zipper TF HY5, which is regulated by light, directly binds to the gene promoters of R2R3 MYB regulators of anthocyanin biosynthesis to regulate anthocyanin biosynthesis (Shin et al., 2013; Jiang et al., 2016; An et al., 2017; Qiu et al., 2019). In Arabidopsis, the expression of anthocyanin biosynthesis-related genes, including production of anthocyanin pigment 1 (PAP1), PAP2, TT8, GL3, and EGL3, is induced by light (Cominelli et al., 2008). In Arabidopsis, the WD40-repeat protein TTG1 forms a complex with bHLH (GL3, EGL3, or TT8) and R2R3 MYB (PAP1, PAP2, MYB113, and MYB114) TFs to regulate anthocyanin synthesis (Zhang et al., 2003; Gonzalez et al., 2008). Low temperature is another important environmental factor that affects anthocyanin biosynthesis. MdbHLH3 binds to the MYC-binding site in the MdMYB1 promoter to induce anthocyanin accumulation in apple under low temperature (Xie et al., 2012).
The effects of environmental factors on the molecular mechanism of anthocyanin synthesis in D. candidum have not been fully elucidated. This study aimed to clarify the molecular mechanism of light-induced anthocyanin biosynthesis regulation in D. candidum. To this end, we grew plants under two different light intensities. The WD40-repeat TF DcTTG1 was found to respond to light signals and to regulate anthocyanin biosynthesis. These results provide new insights in the molecular basis of anthocyanin biosynthesis in D. candidum.
Materials and Methods
Plant Materials and Growth Conditions
Dendrobium candidum (cultivar JXH-1) tissues were cultured at the Institute of Medicinal Plants, Sanming Academy of Agricultural Sciences (Sanming, Fujian, China). Plant tissues were cultured in Murashige & Skoog (MS) medium supplemented with 6-BA (2.0 mg/L), sucrose (20 g/L), and agar (6 g/L), pH 5.8, under a 16-h light/8-h dark cycle, at 24°C. For light treatments, 3-cm-high seedlings were transferred to ½ MS medium and cultured under a 16-h light/8-h dark cycle at 24°C. The light intensity of fluorescent lamps (NH-GP-1200R, Nonghui, Shanghai, China) was set to 1,000 lx or 4,000 lx. Seedlings were collected after 30 days of light exposure. Tobacco (Nicotiana benthamiana) and wild-type (WT) and ttg1-13 mutant A. thaliana (ecotype Columbia) plants were grown in a mixed matrix of vermiculite/perlite/peat soil (1:1:1, v/v/v) or on MS medium in a greenhouse under a 16-h light/8-h dark cycle and a relative humidity of 40–60%, at 22°C.
Quantification of Anthocyanins
The total anthocyanin content of Arabidopsis leaves was extracted and determined using previously described methods (Zhao and Dixon, 2009; Zhao et al., 2011), using cyanidin 3-O-glucoside as a standard. Three biological replicates were analyzed.
Dendrobium candidum samples were ground into a fine powder in liquid nitrogen immediately after collection. One hundred milligram of the powder was extracted with 80% methanol aqueous solution containing 0.5% formic acid in a centrifuge tube under shaking for 2 min followed by ultrasonication at room temperature for 30 min. After the extraction, the mixture was centrifuged at 12000 rpm for 10 min. The supernatant liquid was transferred into a new tube and subjected to UPLC-MS/MS, using a 30A UPLC system with a phenylethyl chromatographic column, column temperature of 40°C, flow rate of 0.25 ml/min, and a total injection volume of 30 μl. Three biological replicates were analyzed for each sample.
Transcriptome Sequencing
RNA was isolated from plant tissue samples using an RNA isolation kit (Aidlab, Beijing, China). RNA quantity and integrity were assessed using a NanoDrop ND-1000 spectrophotometer (NanoDrop Technologies, Wilmington, DE, United States) and an Agilent 2100 Bioanalyzer (Agilent Technologies, Santa Clara, CA, United States), respectively. cDNA libraries were constructed with the NEB Next® Ultra™ RNA Library Prep Kit (New England Biolabs, Ipswich, MA, United States). The cDNA libraries were sequenced on the HiSeq4000 platform (Illumina, San Diego, CA, United States) at Biomarker Technology (Beijing, China). Three biological replicates were analyzed for each sample type. Gene expression levels were analyzed according to the “fragments per kilobase of transcript per million fragments mapped” method. The DESeq R package was used to identify differentially expressed genes (DEGs) in RDc4000 vs. RDc1000. Gene Ontology (GO) enrichment of the DEGs was analyzed using the GOseq R package (Young et al., 2010). Kyoto Encyclopedia of Genes and Genomes (KEGG) pathway enrichment was investigated using the KOBAS tool (Xie et al., 2012). The sequencing data have been submitted to NCBI Sequence Read Archive database under accession numbers SRR13577108, SRR13577107, SRR13577106, SRR13577105, SRR13577104, and SRR13577103.1
Phylogenetic Analysis and Subcellular Localization
Sequences of TTG1-like proteins were downloaded from the National Center for Biotechnology Information database. MEGA X was used for phylogenetic analysis of the TTG1-like proteins. Dendrobium candidum DNA was extracted using a DNA isolation kit (Aidlab). Using primers listed in Supplementary Table S1, a TTG1 sequence was cloned and a fusion vector DcTTG1::YFP was constructed. DcTTG1::YFP was transformed into Agrobacterium GV3101 for injection into tobacco (N. benthamiana) leaf epidermal cells. 4,6-Diamidino-2-phenylindole (DAPI) was used to stain the nuclei. Fluorescence signals were acquired using a confocal laser scanning microscope (model SP8; Leica Microsystems).
RNA Extraction, cDNA Synthesis, and Quantitative Reverse-Transcription PCR
RNA was extracted from D. candidum tissue samples using an RNA isolation kit (Aidlab). The RevertAid Premium First Strand cDNA Synthesis Kit (Fermentas/Thermo Fisher Scientific, Rochester, NY, United States) was used for cDNA synthesis. Quantitative reverse transcription PCRs (RT-qPCRs) were run on a 7500 Fast Quantitative PCR system (Applied Biosystems) using TB Green® Premix Ex Taq™ (Takara). DcACT was selected as a reference gene to normalize target gene expression according to the 2−ΔΔCT method. Three biological replicates were analyzed. The primers used for RT-qPCR are listed in Supplementary Table S2.
Y1H Assay
The coding region of DcTTG1 was ligated into the pGADT7 vector and promoter fragments of several target genes related to anthocyanin biosynthesis were ligated into the pHIS2 vector (Clontech, Palo Alto, CA, United States). Fusion products of the pGADT7 and pHIS2 vectors were transformed into yeast strain Y187 cells (Clontech, Palo Alto, CA, United States). The cells were cultured in synthetic defined (SD) medium –Leu/–Trp/–His with different concentrations of 3-amino-1,2,4-triazole (3-AT, mM) to prevent background histidine leakage of the pHIS2 vector at 28°C for 3 days. Empty pGADT7 vector was used as a control. The primers used for vector construction are listed in Supplementary Table S3.
Transcriptional Activity Assay
Transcriptional activity was analyzed according to previous reports (Liu et al., 2016; Jia et al., 2018). The coding sequence of DcTTG1 was fused into the 35S-LUC-GUS vector driven by the 35S promoter. Promoter fragments of DcCHs1, DcCHS2, DcCHI, DcF3H, DcF3'H, DcDFR1, DcDFR2, DcANS1, and DcUFGT were cloned into the 35S-LUC-GUS vector driven by the GUS reporter. The internal standard was LUC. The fusion vectors were transformed into Agrobacterium GV3101 cells. Single clones were cultured in liquid medium (10 mM MgCl2, 10 mM MES, and 100 μM acetosyringone) under shaking until an optical density at 600 nm of 0.5 was reached. Then, the Agrobacterium cells were injected into tobacco leaves. GUS and LUC activities were measured after 4 days. At least five biological replicates were included in each assay. The primers used for vector construction are listed in Supplementary Table S4.
Results
Red Pigmentation in RDc4000 Is Caused by Anthocyanin Accumulation
The phenotypes of D. candidum seedlings grown under 1,000 lx (RDc1000) and 4,000 lx (RDc4000) are shown in Figure 1A. RDc1000 stems had red pigmentation. The stems of RDc4000 were darker than those of RDc1000, and the leaves were dark red. In general, RDc4000 had more red pigment than RDc1000, indicating that higher-intensity light stimulates the accumulation of red pigment. Analysis of the anthocyanin contents to reveal the type and quantity of red pigmentation under the two light conditions revealed that D. candidum contained delphindin, delphindin-3-O-B-D-glucoside, delphindin-3,5-diglucoside, cyanin, and cyanidin under both conditions, but the contents of these five compounds were different. Delphindin-3-O-B-D-glucoside was the most abundant in RDc1000 and delphindin-3,5-diglucoside was the most abundant in RDc4000; however, the content of delphindin did not differ between the two light conditions. Notably, the contents of delphindin-3-O-B-D-glucoside, delphindin-3,5-diglucoside, cyanin, and cyanidin were significantly higher in RDc4000 than in RDc1000. These results indicated that a higher light intensity promotes the production of red pigment, mainly of the anthocyanin compounds delphindin-3-O-B-D-glucoside, delphindin-3,5-diglucoside, cyanin, and cyanidin, in D. candidum.
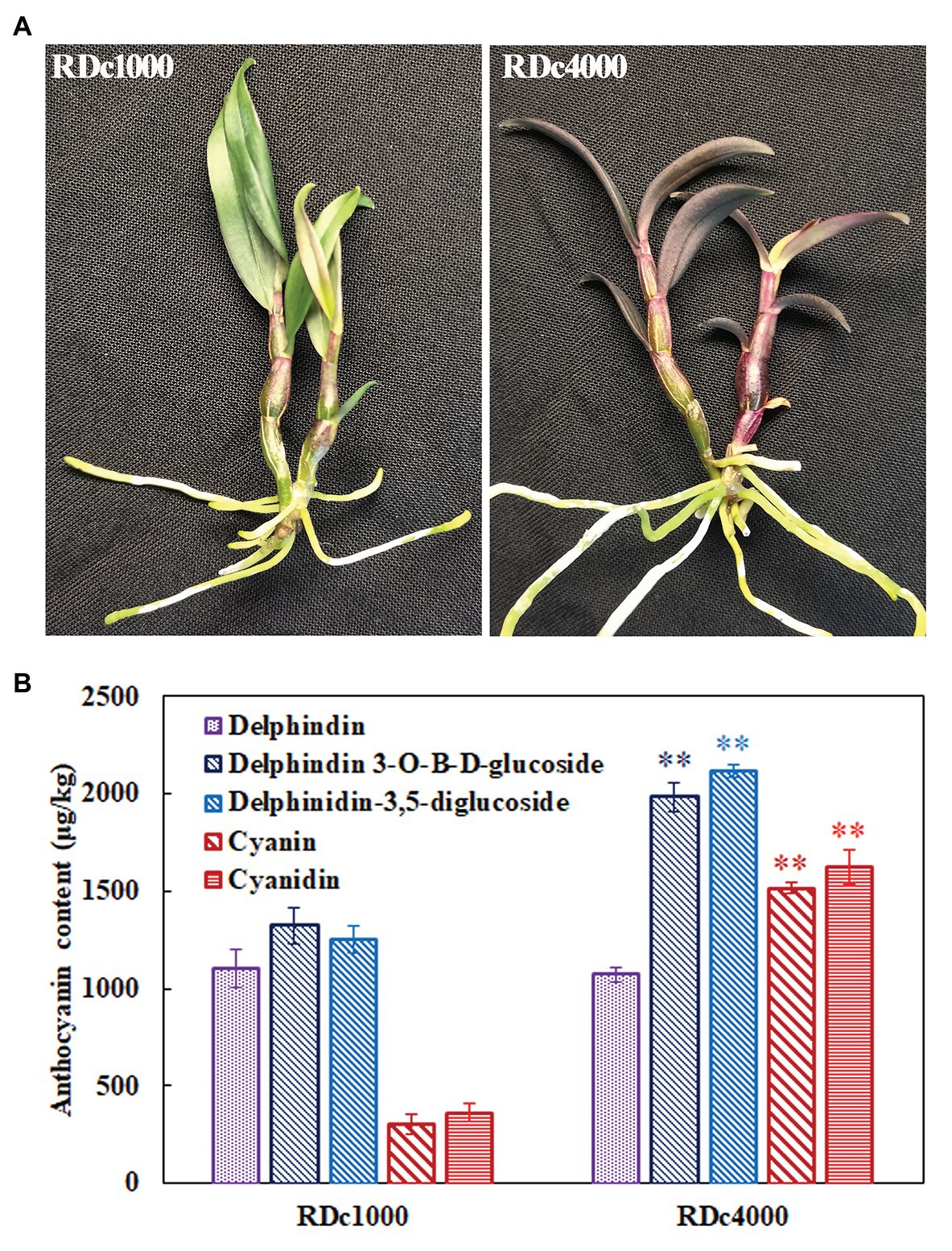
Figure 1. Photographs and anthocyanin contents of Dendrobium candidum under two different light treatments. (A) Photographs of RDc1000 (red D. candidum seedlings under 1,000 lx) and RDc4000 (red D. candidum seedlings under 4,000 lx). (B) Anthocyanin composition in RDc1000 and RDc4000, including delphindin, delphindin-3-O-B-D-glucoside, delphindin-3,5-diglucoside, cyanin, and cyanidin, as assessed by UPLC-MS/MS. All data represent the mean ± SE of three biological replicates. ∗∗p < 0.01, Student’s t-test.
Transcriptome Analysis of RDc1000 and RDc4000
Transcript-level differences in D. candidum under the different light intensities were analyzed by transcriptome sequencing of RDc1000 and RDc4000. The original sequencing data were transformed into raw reads via base calling. The total number of bases in the six libraries was 43.62 G, and the total number of bases in each sample was greater than 6.5 Gb. The Q20 and Q30 scores for each sample were above 97 and 94%, and the GC content was relatively consistent, around 46% (Supplementary Table S5). The mapping rate, i.e., the percentage of mapped reads in clean reads, the most directly reflects sequencing data utility. The mapping rate of the samples ranged from 90.5 to 91.08%. The percentage of uniquely and multi-mapped reads in clean reads ranged from 87.75 to 88.36% and from 2.38 to 2.74%, respectively (Supplementary Table S6). These findings indicated that the data met the quality requirements for analysis. In total, 130 unigenes could be assigned to 51 Gene Ontology (GO) terms in the cellular component, molecular function, and biological process categories. In the cellular component category, DEGs in RDc4000 vs. RDc1000 were mainly related to the plasma membrane, cell, cell parts, and organelles. In the molecular function category, most DEGs were related to catalytic activity and binding. In the biological process category, DEGs were mainly involved in metabolic processes, single-organism processes, and cellular processes (Figure 2A).
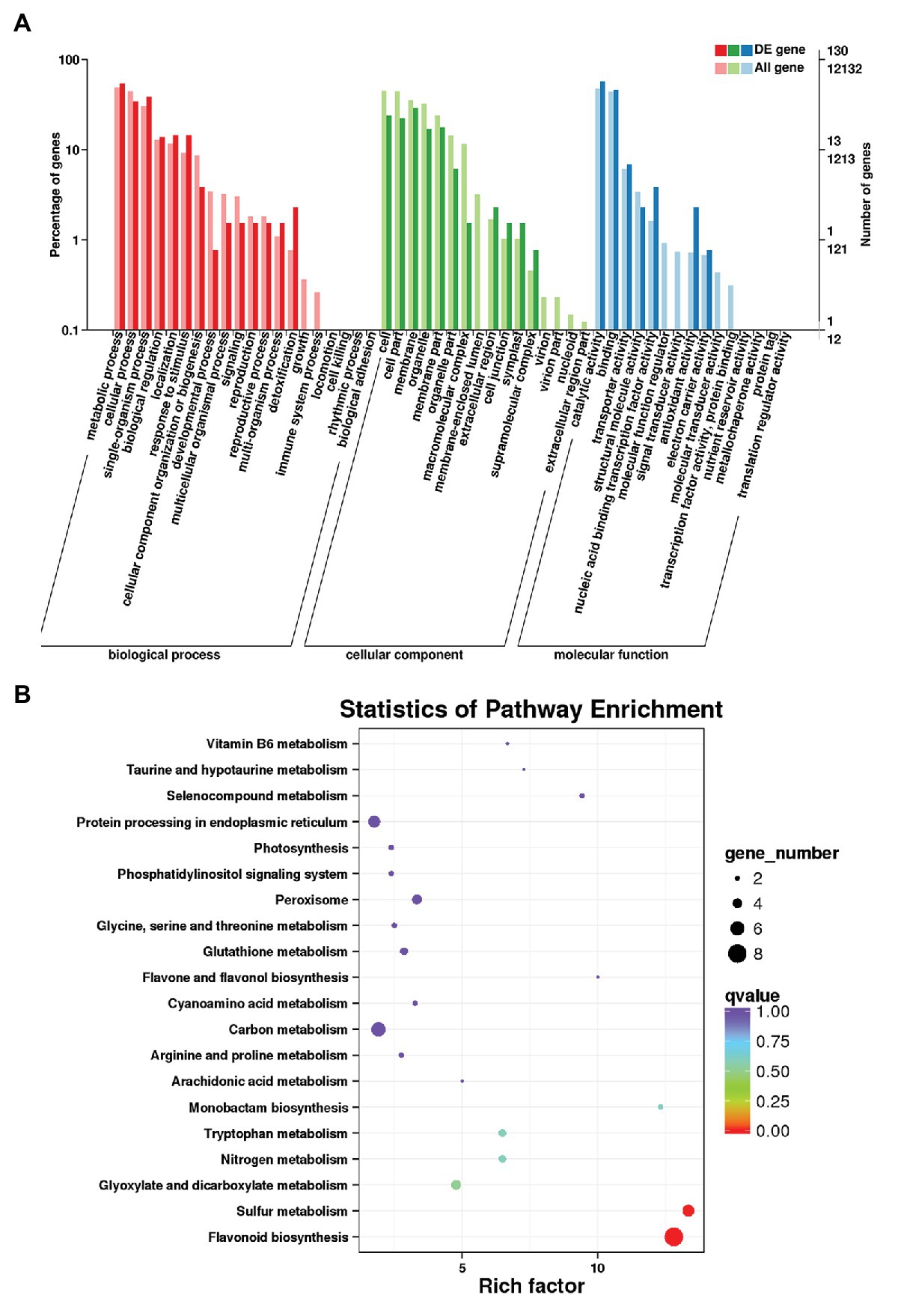
Figure 2. Transcriptome analysis of D. candidum stems. (A) Gene Ontology (GO) enrichment analysis results for differentially expressed genes (DEGs) between RDc1000 and RDc4000. (B) Kyoto Encyclopedia of Genes and Genomes (KEGG) enrichment analysis of the DEGs.
In total, 258 DEGs were found, 152 of which were upregulated and 106 of which were downregulated in RDc4000 vs. RDc1000. Among these, there were 22 TF genes, comprising eight upregulated and 14 downregulated genes, including MYB (two genes), bHLH (two genes), WRKY (two genes), AP2/ERF (two genes), bZIP (two genes), NAC (two genes), and the WD40-repeat TF gene, DcTTG1 (Supplementary Table S7). Among the 22 differentially expressed TF genes, DcTTG1 was upregulated the most strongly in RDc4000 vs. RDc1000 (Supplementary Table S7). Therefore, we speculated that DcTTG1 may be involved in the synthesis of anthocyanins. To identify the metabolic pathways involved in light-induced anthocyanin biosynthesis, 60 DEGs were subjected to KEGG pathway enrichment analysis, and the 20 most enriched pathways are shown in Figure 2B. In these pathways, we found eight DEGs involved in flavonoid biosynthesis, including genes encoding dihydroflavonol-4-reductase, naringenin, 2-oxoglutarate 3-dioxygenase, flavanone 3-dioxygenase, and leucoanthocyanidin dioxygenase, which are key genes in anthocyanin biosynthesis (Supplementary Table S8). These results indicated that these eight genes are the most important genes in the pathway of light-induced anthocyanin biosynthesis.
Expression Analysis and Characterization of DcTTG1
To better understand the role of this TF in anthocyanin production in D. candidum under the different light regimens, it was studied in depth. The DcTTG1 sequence was cloned from D. candidum cDNA. The relative expression of DcTTG1 in RDc1000 and RDc4000 was analyzed by RT-qPCR. The results are shown in Figure 3A. DcTTG1 expression in RDc4000 was 14 times higher than that in RDc1000, indicating that it is induced with increasing light intensity (Figure 3A). To study its regulation in anthocyanin biosynthesis, we conducted subcellular localization analysis using a Pro35S::DcTTG1-YFP vector construct that was transfected into Agrobacterium cells, which were injected into tobacco leaf epidermal cells. The fluorescence signal of Pro35S::DcTTG1-YFP was observed in the nucleus and overlapped with that of the nuclear dye, DAPI, indicating that DcTTG1 is located in the nucleus in D. candidum (Figure 3B). Next, we conducted phylogenetic analysis of DcTTG1 and TTG1-like proteins of other species (Figure 3C). DcTTG1 and PeTTG1 from Phalaenopsis equestris were in the same branch and were closely related. Together with the transcriptional expression differences after the different light treatments, this suggested that DcTTG1 plays an important role in light-regulated anthocyanin biosynthesis.
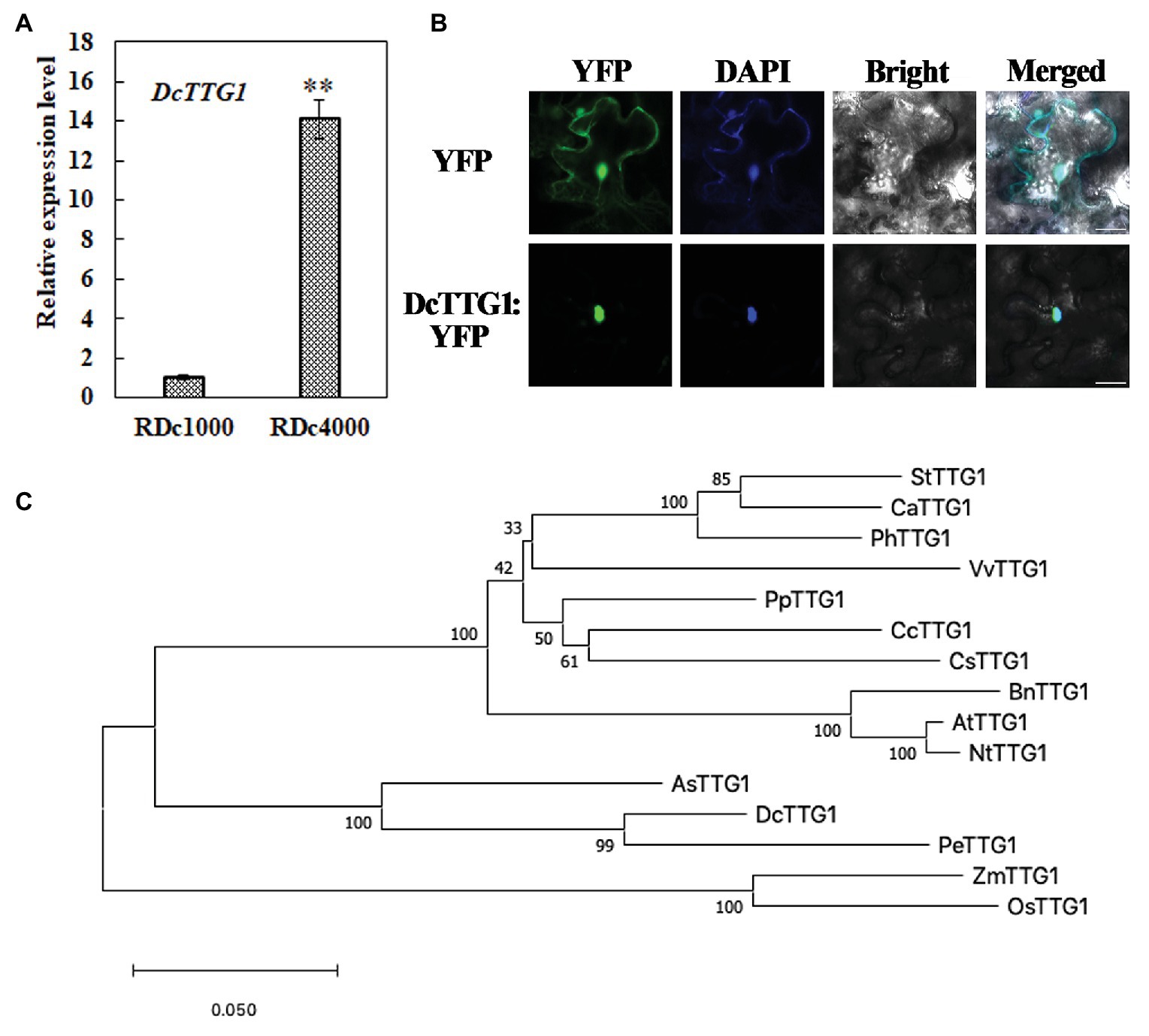
Figure 3. Phylogenetic, expression, and subcellular localization analyses of DcTTG1. (A) Relative expression of DcTTG1 in RDc1000 and RDc4000 as determined by quantitative reverse transcription PCR (RT-qPCR). DcACT was used as reference gene for normalization. Data represent the mean ± SE of three biological replicates. ∗∗p < 0.01, Student’s t-test. (B) Subcellular localization of DcTTG1. 4,6-Diamidino-2-phenylindole (DAPI) was used as a nuclear marker. The merged image shows the colocalization of the YFP fluorescence signal and the DAPI fluorescence signal. Bar = 20 μm. (C) Phylogenetic analysis of TTG1-like proteins. The following proteins were used to construct the phylogenetic tree: StTTG1 (NP_001305551.1), CaTTG1 (XP_016564215.1), PhTTG1 (AAC18914.1), VvTTG1 (CAN67365.1), PpTTG1 (ACQ65867.1), CcTTG1 (AMQ26245.1), CsTTG1 (NP_001306987.1), BnTTG1 (NP_001303154.1), AtTTG1 (CAC10524.1), NtTTG1 (ACJ06978.1), AsTTG1 (PKA51013.1), PeTTG1 (XP_020583423.1), ZmTTG1 (NP_001310302.1), and OsTTG1 (KAB8088430.1).
Expression Analysis of Key Anthocyanin Biosynthetic Genes
Anthocyanin accumulation affects plant pigmentation, resulting in different plant colors. To determine the effect of the different light intensity regimens on anthocyanin metabolism, the expression of 10 key anthocyanin biosynthetic genes, including DcCHS1, DcCHS2, DcCHI, DcF3H, DcF3'H, DcF3'5'H, DcDFR1, DcDFR2, DcANS1, and DcUFGT, was analyzed by RT-qPCR in RDc1000 and RDc4000 plants. The mRNA levels of DcCHS1, DcCHS2, DcF3H, DcF3'H, DcDFR1, DcDFR2, and DcANS1 were significantly higher in RDc4000 than in RDc1000. DcDFR1 was the most strongly induced, by approximately 27 times, followed by DcF3H. These results indicated that, the stronger the light intensity, the higher the expression of DFR, which greatly promotes anthocyanin accumulation in plants. These seven genes included early as well as late anthocyanin biosynthetic genes, indicating that high-intensity light has a significant effect on both the early and late stages of anthocyanin synthesis.
DcTTG1 May Regulate the Expression of DcCHS2, DcCHI1, DcF3H, and DcF3'H by Binding to Their Promoters
TTG1 is a WD40-repeat TF that can bind to the promoter regions of target genes to regulate their transcription. A Y1H assay was used to analyze the regulation of target genes by DcTTG1. The promoter sequences of nine anthocyanin biosynthetic genes, DcCHs1, DcCHS2, DcCHI, DcF3H, DcF3'H, DcDFR1, DcDFR2, DcANS1, and DcUFGT, were cloned separately to verify whether DcTTG1 has a regulatory effect on these genes. An appropriate concentration of 3-AT in the yeast growth medium (SD/–Leu/–Trp/–His) inhibited the self-activation of all target genes. The assay results suggested that DcTTG1 can bind to the promoters of DcCHS2, DcCHI, DcF3H, and DcF3'H (Figure 4).
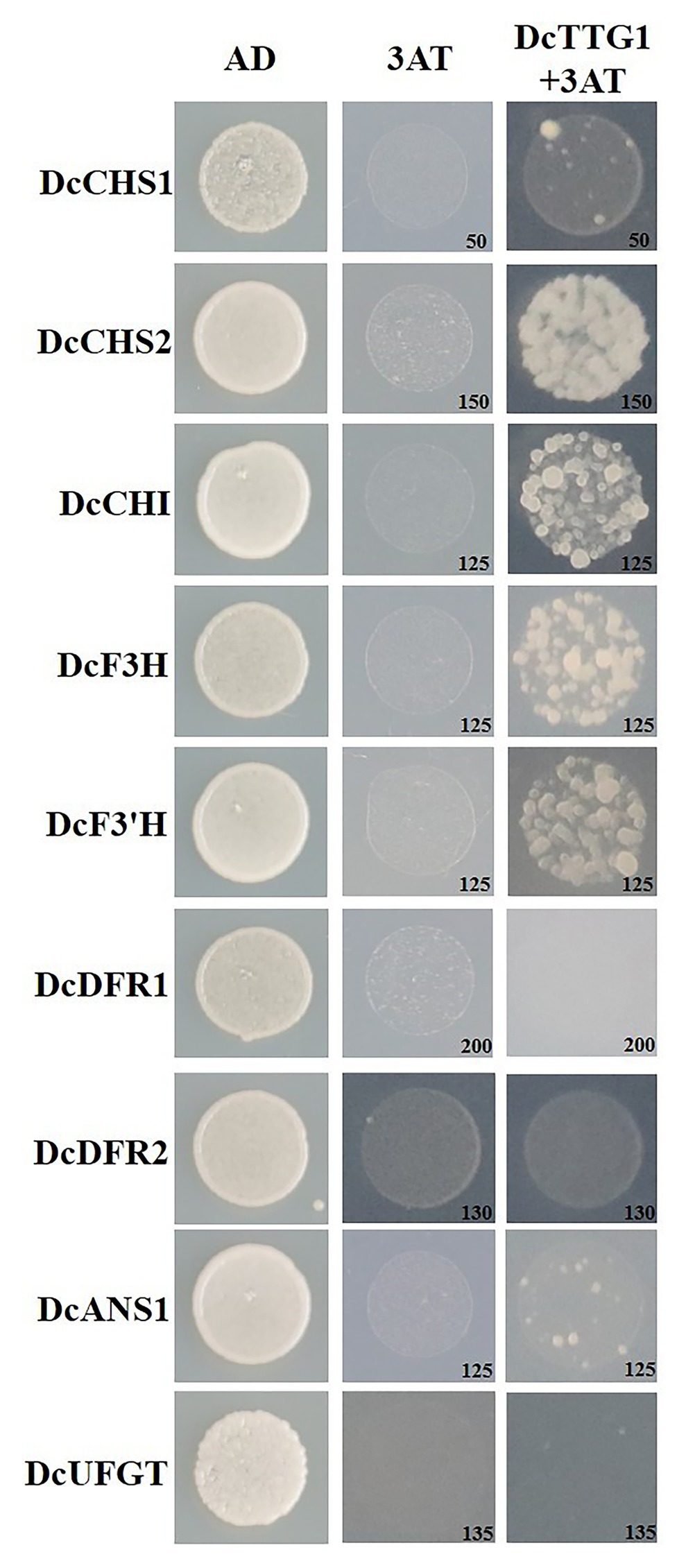
Figure 4. Y1H assays of DcTTG1 and anthocyanin biosynthesis-related gene promoters. The DcTTG1 sequence and promoter fragments of nine anthocyanin biosynthetic genes were cloned into pGADT7 and pHIS2 vectors, respectively. The plasmids were transformed into Y187 yeast cells (Clontech Laboratories, Mountain View, CA, United States) that were grown on SD (–Leu/–Trp/–His) medium at 28°C for 3 days. The optimal 3-AT concentration (in mM) to suppress background histidine leakiness after addition to the (–Leu/–Trp/–His) medium is indicated in the lower-right corner of each picture.
To verify the Y1H assay results, we used LUC/GUS analysis to detect the effect of DcTTG1 on the activation of key anthocyanin biosynthetic gene promoters. We cloned anthocyanin biosynthetic gene promoters, which we used to drive the GUS reporter gene, and DcTTG1 was driven by the CaMV35S promoter (Figure 5A). Transient expression of the reporter system in tobacco revealed that DcTTG1 activated reporter gene expression driven by the promoters of DcCHS2, DcCHI, DcF3H, and DcF3'H (Figure 5B), corroborating that DcTTG1 regulates the expression of these four genes. Thus, DcTTG1 modulates anthocyanin synthesis by regulating the expression of anthocyanin synthesis-related target genes under different light intensities.
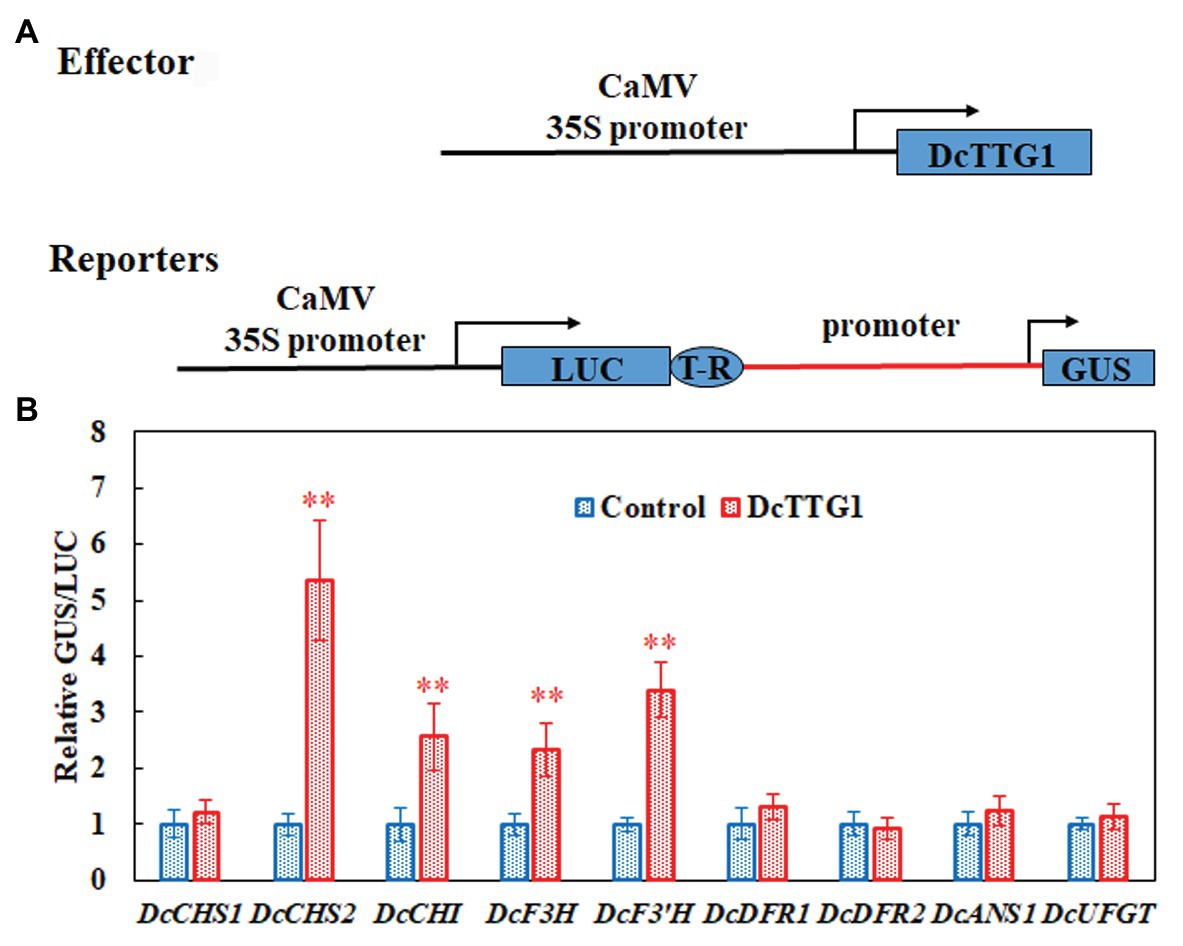
Figure 5. DcTTG1 can directly bind to the promoters of anthocyanin biosynthetic genes. (A) Effector-reporter systems were used to determine the ability of DcTT1 to activate the promoters of anthocyanin biosynthetic genes in tobacco leaves. The reporter systems contained the GUS reporter gene driven by the promoters of anthocyanin biosynthetic genes, as well as LUC driven by CaMV35S for standardization. The effector systems contained DcTTG1 driven by CaMV35S. Boxes, various DNA sequences. T-R, terminator. (B) Transcriptional activity of DcTTG1 on the promoters of anthocyanin biosynthetic genes as indicated by the reporter assay. Empty vector was used as a control, and the GUS/LUC ratio in the transformed leaves was set to 1. Data represent the mean ± SE of five biological replicates. ∗∗p < 0.01, Student’s t-test.
Phenotype Rescue of the Arabidopsis ttg1-13 Mutant
Establishing transgenic D. candidum lines is generally difficult and time-consuming. Therefore, we used the model plant Arabidopsis to verify the function of DcTTG1 in the regulation of anthocyanin biosynthesis. To further study the possible function of DcTTG1 as a regulator of anthocyanin biosynthesis, we overexpressed DcTTG1 driven by the CaMV35S promoter in the Arabidopsis ttg1-13 mutant, which is defect in seed coat anthocyanin pigmentation. We obtained 18 DcTTG1 overexpression lines and analyzed lines DcTTG1/ttg1-13#1 and DcTTG1/ttg1-13#2. The DcTTG1 expression levels in these lines were significantly higher than those in WT plants (Figure 6A), indicating successful overexpression. DcTTG1 overexpression restored the ttg1-13 mutant phenotype (Figure 6B; Supplementary Figure S1) as well as leaf anthocyanin pigmentation (Figure 6C), suggesting that DcTTG1 has a similar function as Arabidopsis TTG1.
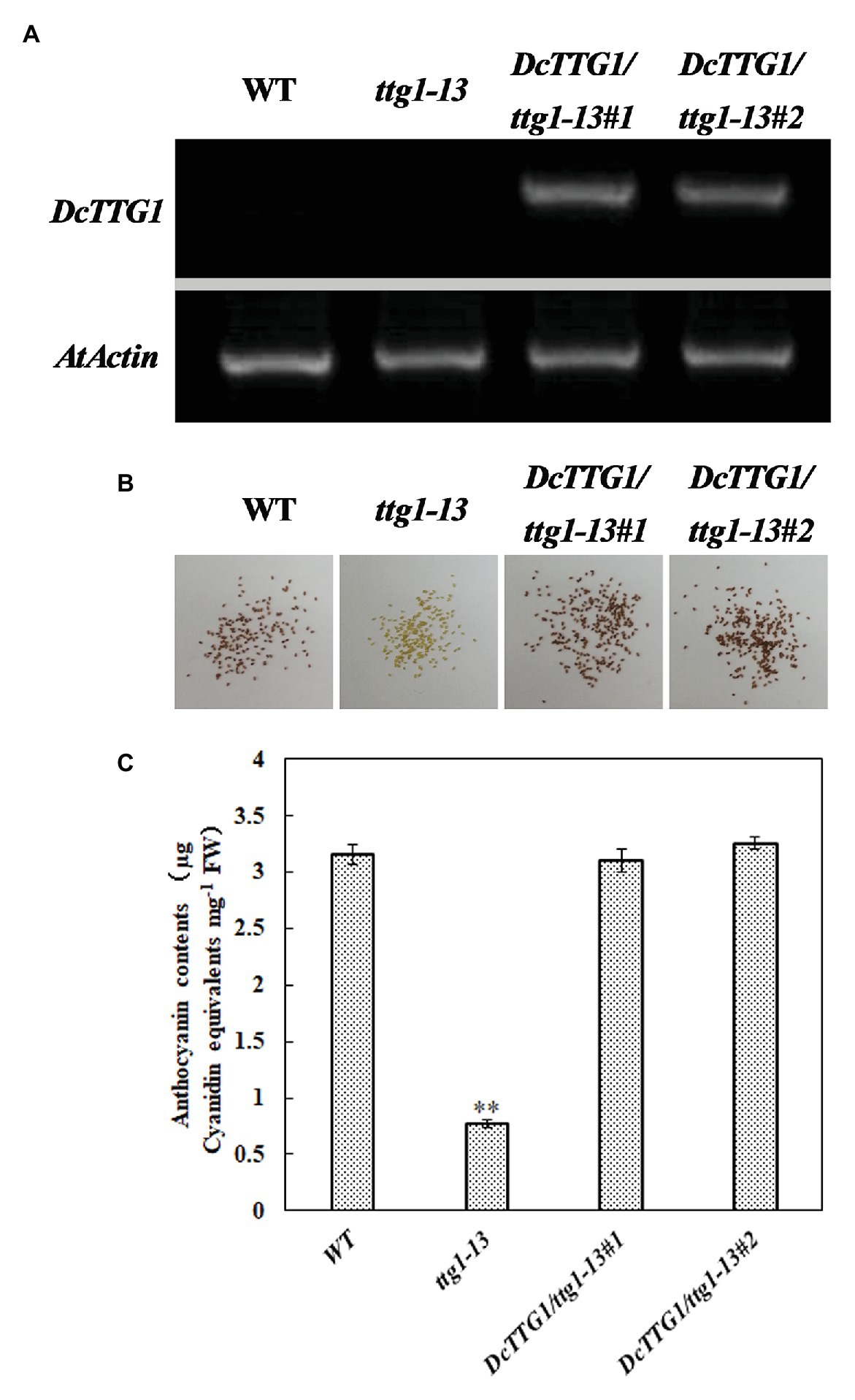
Figure 6. Genetic complementation of the Arabidopsis ttg1-13 mutant by DcTTG1. (A) DcTTG1 transcripts in Arabidopsis young seeds. (B) Restored seed pigments in ttg1-13 after overexpression of DcTTG1. (C) Quantification of anthocyanins in the leaves of WT, ttg1, DcTTG1/ttg1-13#1, and DcTTG1/ttg1-13#2. Data represent the mean ± SE of three biological replicates. ∗∗p < 0.01, Student’s t-test.
Discussion
This study revealed that the contents of delphindin-3-O-B-D-glucoside, delphindin-3,5-diglucoside, cyanin, and cyanidin were significantly increased in RDc4000 compared with RDc1000. Transcriptome sequencing and RT-qPCR showed that the WD40-repeat TF DcTTG1 was significantly more strongly expressed in RDc4000 than in RDc1000. Phylogenetic analysis and genetic complementation revealed the functional conservation of DcTTG1 and its orthologs in other plant species. Y1H and transcriptional activation assays indicated that DcTTG1 regulates DcCHS2, DcCHI, DcF3H, and DcF3'H expression by binding to their promoters. Thus, DcTTG1 may regulate anthocyanin biosynthesis in D. candidum under different light intensities.
Anthocyanin compositional and content analyses showed that the content of delphindin did not significantly differ between RDc1000 and RDc4000 (Figure 1B). In the anthocyanin biosynthetic pathway, the formation of delphindin requires F3'5'H catalysis (Matus et al., 2009). DcF3'5'H expression did not significantly differ between RDc1000 and RDc4000, which may explain the lack of a significant difference in delphindin content. However, the contents of delphindin-3-O-B-D-glucoside and delphindin-3,5-diglucoside were significantly different between RDc1000 and RDc4000, which may be due to significant increases in DcDFR1, DcDFR2, and DcANS1 expression in RDc4000 (Figure 7). The cyanin and cyanidin contents were significantly higher in RDc4000 than in RDc1000 (Figure 7). The formation of cyanin and cyanidin requires F3'H catalysis (Rinaldo et al., 2015), and DcF3'H expression was significantly higher in RDc4000 than in RDc1000 (Figure 7). Light provides energy for plant growth and development (Lau and Deng, 2010). It regulates plant secondary metabolism, including anthocyanin and flavonoid synthesis (Zhang et al., 2018; An et al., 2020). Different light intensities have different effects on anthocyanin accumulation, which is positively correlated with light intensity in many plant species (Jaakola, 2013; Zhang et al., 2018).
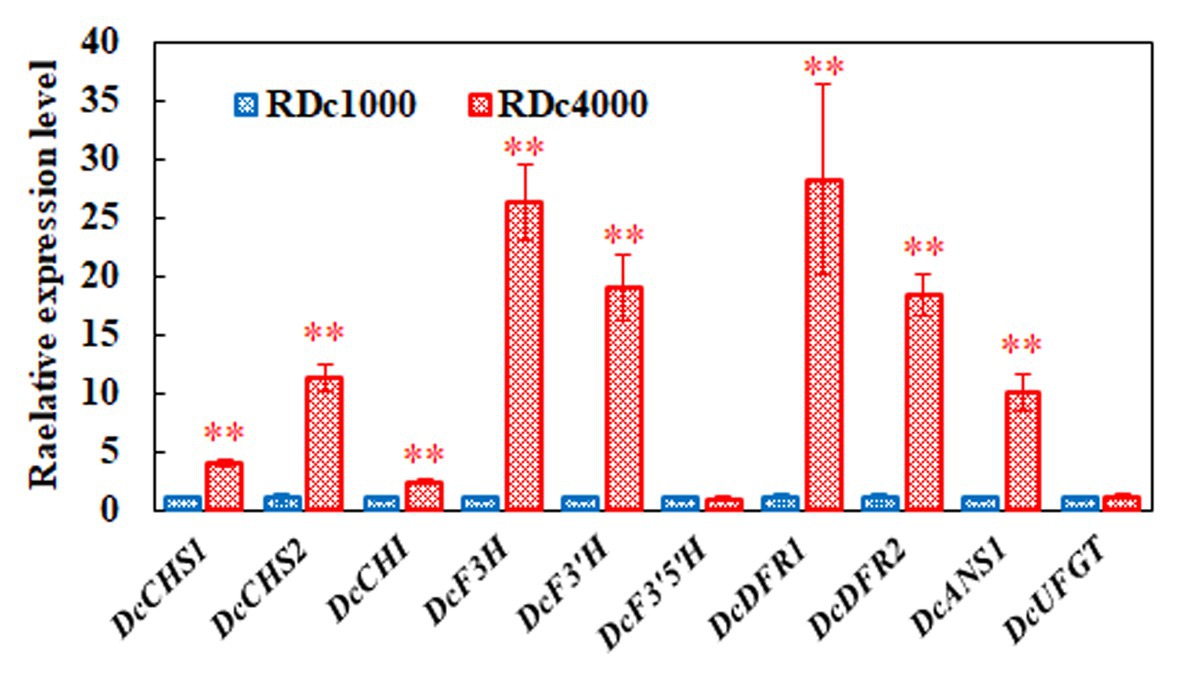
Figure 7. Expression of key anthocyanin biosynthetic genes. DcACTIN was used as reference gene for normalization. All gene expression levels in RDc1000 were set to 1. Error bars represent the mean ± SE of three biological replicates. ∗∗p < 0.01, Student’s t-test.
In Arabidopsis, AtTTG1 is expressed in the dark, but its expression increases immediately after illumination and then remains relatively stable over time (Cominelli et al., 2008). In our study, DcTTG1 expression increased significantly with increasing light intensity (Figure 3A). In Brassica, anthocyanins accumulate more strongly under LED irradiance with a photosynthetic photon flux of 220 μmol m–2 s−1 than under a flux of 330 or 440 μmol m–2 s−1 (Samuoliene et al., 2013). Within a certain range, anthocyanin accumulation gradually increases with increasing light intensity, but after a certain intensity, the amount of anthocyanin does not further increase due to photoprotection (Logan et al., 2015). Anthocyanin levels in lychee peels decreased rapidly after shading treatment, but anthocyanin biosynthesis resumed soon after the fruits were exposed to light again (Zhang et al., 2016). This shows that light can induce anthocyanin formation and is an important environmental factor affecting anthocyanin biosynthesis. Similar findings have been reported for Chinese bayberry (Niu et al., 2010), apple (Li et al., 2012), and grape berry (Azuma et al., 2012).
Transcriptome sequencing analysis was performed on RDc1000 and RDc4000 to study the relationship between light intensity and anthocyanin accumulation. Among the TFs identified by transcriptome sequencing, MYB, bHLH, and WD40 all showed significant differences in expression, indicating that MBW may be involved in anthocyanin biosynthesis induced by high light intensity in D. candidum. Transcriptome analysis of light-regulated anthocyanin biosynthesis in the pericarp of Litchi identified 76 TFs that regulate anthocyanin synthesis in response to light. Among these TFs, SQUAMOSA promoter binding protein-like constituted the largest population (21.1%), followed by MYB (19.7%), homologous domain leucine zipper protein (ATHB, 17.1%), and WD40 (13.2%; Zhang et al., 2016). Thus, light intensity is an important environmental factor in regulating anthocyanin biosynthesis.
The expression levels of DcCHI, DcF3'5'H, and DcUFGT did not differ significantly between the two light treatments, indicating that these genes are less responsive to different light intensities in D. candidum. Interestingly, our and previous findings indicate that the feedback regulation of anthocyanin synthetic genes in response to light varies among plant species. Leaves of Lactuca sativa L. var. capitata were green under 40 mmol m−2 s−1, but red under 100 mmol m−2 s−1, and the expression of LsCHI was upregulated (Zhang et al., 2018). However, in a study of light-induced regulation of anthocyanin biosynthesis in litchi fruit, the activation and expression of LcUFGT promoted the accumulation of anthocyanins and enhanced fruit redness (Zhang et al., 2016). Anthocyanin biosynthetic genes of different species differentially respond to light, which emphasizes the importance of studying the effects of different light treatments on anthocyanin synthesis in D. candidum.
DcTTG1 modulates anthocyanin synthesis by regulating the expression of anthocyanin synthesis-related target genes under different light intensities. In this study, no direct binding of TTG1 to late anthocyanin biosynthetic gene promoters was observed, which indicates that DcTTG1 has multiple modes of regulating key genes in anthocyanin biosynthesis. Gene regulatory networks in higher plants require the coordinated action of many gene interactions. Among them, the most widely studied is the regulation of anthocyanin biosynthesis by the MBW complex. TTG1 forms complexes with the R2R3 MYB TFs PAP1, PAP2, MYB113, MYB114, or TT2 and the bHLH TFs GL3, EGL3, or TT8, to form MBW (Xu et al., 2015). Research in Arabidopsis has shown that the TTG1-dependent MBW complex can directly bind to the promoters of TTG2, TT8, F3'H, DFR, ANS, UGT79B1, UGT75C1, 5MAT, and BLT (Wei et al., 2019). Therefore, it can be inferred that DcTTG1 alone cannot regulate the late anthocyanin biosynthetic genes, whereas different TTG1-dependent MBW complexes can simultaneously regulate the early and late anthocyanin biosynthetic genes. The different MBW complexes formed by TTG1 have diverse regulatory roles in anthocyanin biosynthesis. The TTG1-TT8/GL3-PAP1/PAP2/MYB113/MYB114 complex can activate the expression of late biosynthetic genes, including dihydroflavonol 4-reductase (DFR) and phthalate synthase (ANS), to affect anthocyanin biosynthesis (Shi and Xie, 2014). There is no relevant research on the characteristic roles of DcTTG1 and the DcTTG1-dependent MBW complex in the regulation of anthocyanin biosynthetic structural gene expression, especially under different light conditions. Our findings may contribute to improving plant production through the regulation of certain desired metabolic compounds.
In conclusion, we identified and isolated a WD40-repeat TF, DcTTG1, as a novel regulator of light-regulated anthocyanin accumulation in D. candidum. DcTTG1 regulates anthocyanin biosynthesis in various ways. The TF binds to the promoters of DcCHS2, DcCHI, DcF3H, and DcF3'H, which are anthocyanin biosynthesis-related genes. DcTTG1 restored the Arabidopsis ttg1-13 mutant phenotype, which is defect in seed coat anthocyanin pigmentation, suggesting that it has a similar function as Arabidopsis TTG1. We believe that the findings of this study will help improve the understanding of the mechanism of light-induced anthocyanin synthesis and accumulation.
Data Availability Statement
The transcriptome data of this study have been uploaded to NCBI Sequence Read Archive database (https://ncbiinsights.ncbi.nlm.nih.gov/tag/sra/), the accession number is PRJNA694810.
Author Contributions
NJ, FW, and BF designed the study. NJ, JW, YW, JL, JJ, JS, PY, and PW performed the experiments. NJ, JW, FW, and BF wrote the manuscript. All authors contributed to the article and approved the submitted version.
Funding
This work was supported by the special fund for Supervision of Quality and Safety of Agricultural Products (Risk Assessment) from the Chinese Ministry of Agriculture (grant GJFP2019019).
Conflict of Interest
The authors declare that the research was conducted in the absence of any commercial or financial relationships that could be construed as a potential conflict of interest.
Acknowledgments
We thank Qinhuangdao General Spectrometry Technology Co. Ltd. for providing testing technical services (anthocyanins).
Supplementary Material
The Supplementary Material for this article can be found online at: https://www.frontiersin.org/articles/10.3389/fpls.2021.633333/full#supplementary-material
Footnotes
References
Allan, A. C., Hellens, R. P., and Laing, W. A. (2008). MYB transcription factors that colour our fruit. Trends Plant Sci. 13, 99–102. doi: 10.1016/j.tplants.2007.11.012
An, J. P., Liu, Y. J., Zhang, X. W., Bi, S. Q., Wang, X. F., You, C. X., et al. (2020). Dynamic regulation of anthocyanin biosynthesis at different light intensities by the BT2-TCP46-MYB1 module in apple. J. Exp. Bot. 71, 3094–3109. doi: 10.1093/jxb/eraa056
An, J. P., Qu, F. J., Yao, J. F., Wang, X. N., You, C. X., Wang, X. F., et al. (2017). The bZIP transcription factor MdHY5 regulates anthocyanin accumulation and nitrate assimilation in apple. Hortic. Res. 4:17023. doi: 10.1038/hortres.2017.23
Azuma, A., Yakushiji, H., Koshita, Y., and Kobayashi, S. (2012). Flavonoid biosynthesis-related genes in grape skin are differentially regulated by temperature and light conditions. Planta 236, 1067–1080. doi: 10.1007/s00425-012-1650-x
Bai, S., Sun, Y., Qian, M., Yang, F., Ni, J., Tao, R., et al. (2017). Transcriptome analysis of bagging-treated red Chinese sand pear peels reveals light-responsive pathway functions in anthocyanin accumulation. Sci. Rep. 7:63. doi: 10.1038/s41598-017-00069-z
Bai, S., Tao, R., Tang, Y., Yin, L., Ma, Y., Ni, J., et al. (2019). BBX16, a B-box protein, positively regulates light-induced anthocyanin accumulation by activating MYB10 in red pear. Plant Biotechnol. J. 17, 1985–1997. doi: 10.1111/pbi.13114
Broun, P. (2005). Transcriptional control of flavonoid biosynthesis: a complex network of conserved regulators involved in multiple aspects of differentiation in Arabidopsis. Curr. Opin. Plant Biol. 8, 272–279. doi: 10.1016/j.pbi.2005.03.006
Cabrita, L., Fossen, T., and Andersen, Ø. M. (2000). Colour and stability of the six common anthocyanidin 3-glucosides in aqueous solutions. Food Chem. 68, 101–107. doi: 10.1016/S0308-8146(99)00170-3
Chalker-Scott, L. (1999). Environmental significance of anthocyanins in plant stress responses. Photochem. Photobiol. 70, 1–9. doi: 10.1111/j.1751-1097.1999.tb01944.x
Cominelli, E., Gusmaroli, G., Allegra, D., Galbiati, M., Wade, H. K., Jenkins, G. I., et al. (2008). Expression analysis of anthocyanin regulatory genes in response to different light qualities in Arabidopsis thaliana. J. Plant Physiol. 165, 886–894. doi: 10.1016/j.jplph.2007.06.010
de Pascual-Teresa, S., and Sanchez-Ballesta, M. T. (2008). Anthocyanins: from plant to health. Phytochem. Rev. 7, 281–299. doi: 10.1007/s11101-007-9074-0
Gonzalez, A., Zhao, M., Leavitt, J. M., and Lloyd, A. M. (2008). Regulation of the anthocyanin biosynthetic pathway by the TTG1/bHLH/Myb transcriptional complex in Arabidopsis seedlings. Plant J. 53, 814–827. doi: 10.1111/j.1365-313X.2007.03373.x
Holton, T. A., and Cornish, E. C. (1995). Genetics and biochemistry of anthocyanin biosynthesis. Plant Cell 7, 1071–1083. doi: 10.2307/3870058
Jaakola, L. (2013). New insights into the regulation of anthocyanin biosynthesis in fruits. Trends Plant Sci. 18, 477–483. doi: 10.1016/j.tplants.2013.06.003
Jia, N., Liu, J. Q., Sun, Y. F., Tan, P. H., Cao, H., Xie, Y. Y., et al. (2018). Citrus sinensis MYB transcription factors CsMYB330 and CsMYB308 regulate fruit juice sac lignification through fine-tuning expression of the Cs4CL1 gene. Plant Sci. 277, 334–343. doi: 10.1016/j.plantsci.2018.10.006
Jiang, M., Ren, L., Lian, H., Liu, Y., and Chen, H. (2016). Novel insight into the mechanism underlying light-controlled anthocyanin accumulation in eggplant (Solanum melongena L.). Plant Sci. 249, 46–58. doi: 10.1016/j.plantsci.2016.04.001
Konczak, I., and Zhang, W. (2004). Anthocyanins—more than nature’s colours. J. Biomed. Biotechnol. 2004:307613. doi: 10.1155/S1110724304407013
Lai, B., Li, X. J., Hu, B., Qin, Y. H., Huang, X. M., Wang, H. C., et al. (2014). LcMYB1 is a key determinant of differential anthocyanin accumulation among genotypes, tissues, developmental phases and ABA and light stimuli in Litchi chinensis. PLoS One 9:e86293. doi: 10.1371/journal.pone.0086293
Lau, O. S., and Deng, X. W. (2010). Plant hormone signaling lightens up: integrators of light and hormones. Curr. Opin. Plant Biol. 13, 571–577. doi: 10.1016/j.pbi.2010.07.001
Li, Y. Y., Mao, K., Zhao, C., Zhao, X. Y., Zhang, H. L., Shu, H. R., et al. (2012). MdCOP1 ubiquitin E3 ligases interact with MdMYB1 to regulate light-induced anthocyanin biosynthesis and red fruit coloration in apple. Plant Physiol. 160, 1011–1022. doi: 10.1104/pp.112.199703
Liu, J. Q., Chen, X. J., Liang, X. X., Zhou, X. G., Yang, F., Liu, J., et al. (2016). Alternative splicing of rice WRKY62 and WRKY76 transcription factor genes in pathogen defense. Plant Physiol. 171, 1427–1442. doi: 10.1104/pp.15.01921
Logan, B. A., Stafstrom, W. C., Walsh, M. J. L., Reblin, J. S., and Gould, K. S. (2015). Examining the photoprotection hypothesis for adaxial foliar anthocyanin accumulation by revisiting comparisons of green- and red-leafed varieties of coleus (Solenostemon scutellarioides). Photosynth. Res. 124, 267–274. doi: 10.1007/s11120-015-0130-0
Matus, J. T., Loyola, R., Vega, A., Pena-Neira, A., Bordeu, E., Arce-Johnson, P., et al. (2009). Post-veraison sunlight exposure induces MYB-mediated transcriptional regulation of anthocyanin and flavonol synthesis in berry skins of Vitis vinifera. J. Exp. Bot. 60, 853–867. doi: 10.1093/jxb/ern336
Ng, T. B., Liu, J., Wong, J. H., Ye, X., Wing Sze, S. C., Tong, Y., et al. (2012). Review of research on Dendrobium, a prized folk medicine. Appl. Microbiol. Biotechnol. 93, 1795–1803. doi: 10.1007/s00253-011-3829-7
Ni, J. B., Bai, S. L., Zhao, Y., Qian, M. J., Tao, R. Y., Yin, L., et al. (2019). Ethylene response factors Pp4ERF24 and Pp12ERF96 regulate blue light-induced anthocyanin biosynthesis in “Red Zaosu” pear fruits by interacting with MYB114. Plant Mol. Biol. 99, 67–78. doi: 10.1007/s11103-018-0802-1
Niu, S. S., Xu, C. J., Zhang, W. S., Zhang, B., Li, X., Lin-Wang, K., et al. (2010). Coordinated regulation of anthocyanin biosynthesis in Chinese bayberry (Myrica rubra) fruit by a R2R3 MYB transcription factor. Planta 231, 887–899. doi: 10.1007/s00425-009-1095-z
Qiu, Z., Wang, H., Li, D., Yu, B., Hui, Q., Yan, S., et al. (2019). Identification of candidate HY5-dependent and -independent regulators of anthocyanin biosynthesis in tomato. Plant Cell Physiol. 60, 643–656. doi: 10.1093/pcp/pcy236
Rinaldo, A. R., Cavallini, E., Jia, Y., Moss, S. M. A., McDavid, D. A. J., Hooper, L. C., et al. (2015). A grapevine anthocyanin acyltransferase, transcriptionally regulated by VvMYBA, can produce most acylated anthocyanins present in grape skins. Plant Physiol. 169, 1897–1916. doi: 10.1104/pp.15.01255
Samuoliene, G., Brazaityte, A., Jankauskiene, J., Virsile, A., Sirtautas, R., Novickovas, A., et al. (2013). LED irradiance level affects growth and nutritional quality of Brassica microgreens. Cent. Eur. J. Biol. 8, 1241–1249. doi: 10.2478/s11535-013-0246-1
Shi, M. Z., and Xie, D. Y. (2014). Biosynthesis and metabolic engineering of anthocyanins in Arabidopsis thaliana. Recent Pat. Biotechnol. 8, 47–60. doi: 10.2174/1872208307666131218123538
Shin, D. H., Choi, M., Kim, K., Bang, G., Cho, M., Choi, S. B., et al. (2013). HY5 regulates anthocyanin biosynthesis by inducing the transcriptional activation of the MYB75/PAP1 transcription factor in Arabidopsis. FEBS Lett. 587, 1543–1547. doi: 10.1016/j.febslet.2013.03.037
Takos, A. M., Jaffé, F. W., Jacob, S. R., Bogs, J., Robinson, S. P., and Walker, A. R. (2006). Light-induced expression of a MYB gene regulates anthocyanin biosynthesis in red apples. Plant Physiol. 142, 1216–1232. doi: 10.1104/pp.106.088104
Wei, Z., Cheng, Y., Zhou, C., Li, D., Gao, X., Zhang, S., et al. (2019). Genome-wide identification of direct targets of the TTG1–bHLH–MYB complex in regulating trichome formation and flavonoid accumulation in Arabidopsis thaliana. Int. J. Mol. Sci. 20:5014. doi: 10.3390/ijms20205014
Wei, W., Feng, L., Bao, W. -R., Ma, D. -L., Leung, C. -H., Nie, S. -P., et al. (2016). Structure characterization and immunomodulating effects of polysaccharides isolated from dendrobium officinale. J. Agric. Food Chem. 64, 881–889. doi: 10.1021/acs.jafc.5b05180
Xie, X. B., Li, S., Zhang, R. F., Zhao, J., Chen, Y. C., Zhao, Q., et al. (2012). The bHLH transcription factor MdbHLH3 promotes anthocyanin accumulation and fruit colouration in response to low temperature in apples. Plant Cell Environ. 35, 1884–1897. doi: 10.1111/j.1365-3040.2012.02523.x
Xu, W., Dubos, C., and Lepiniec, L. (2015). Transcriptional control of flavonoid biosynthesis by MYB–bHLH–WDR complexes. Trends Plant Sci. 20, 176–185. doi: 10.1016/j.tplants.2014.12.001
Young, M. D., Wakefield, M. J., Smyth, G. K., and Oshlack, A. (2010). Gene ontology analysis for RNA-seq: accounting for selection bias. Genome Biol. 11:R14. doi: 10.1186/gb-2010-11-2-r14
Zhang, Y., Butelli, E., and Martin, C. (2014). Engineering anthocyanin biosynthesis in plants. Curr. Opin. Plant Biol. 19, 81–90. doi: 10.1016/j.pbi.2014.05.011
Zhang, F., Gonzalez, A., Zhao, M., Payne, C. T., and Lloyd, A. (2003). A network of redundant bHLH proteins functions in all TTG1-dependent pathways of Arabidopsis. Development 130, 4859–4869. doi: 10.1242/dev.00681
Zhang, H. N., Li, W. C., Wang, H. C., Shi, S. Y., Shu, B., Liu, L. Q., et al. (2016). Transcriptome profiling of light-regulated anthocyanin biosynthesis in the pericarp of litchi. Front. Plant Sci. 7:963. doi: 10.3389/fpls.2016.00963
Zhang, Y., Xu, S., Cheng, Y., Peng, Z., and Han, J. (2018). Transcriptome profiling of anthocyanin-related genes reveals effects of light intensity on anthocyanin biosynthesis in red leaf lettuce. PeerJ 6:e4607. doi: 10.7717/peerj.4607
Zhao, J., and Dixon, R. A. (2009). MATE transporters facilitate vacuolar uptake of epicatechin 3′-O-glucoside for proanthocyanidin biosynthesis in Medicago truncatula and Arabidopsis. Plant Cell 21, 2323–2340. doi: 10.1105/tpc.109.067819
Keywords: Dendrobium candidum, DcTTG1, anthocyanin biosynthesis, transcriptional regulation, transcription factor
Citation: Jia N, Wang J, Wang Y, Ye W, Liu J, Jiang J, Sun J, Yan P, Wang P, Wang F and Fan B (2021) The Light-Induced WD40-Repeat Transcription Factor DcTTG1 Regulates Anthocyanin Biosynthesis in Dendrobium candidum. Front. Plant Sci. 12:633333. doi: 10.3389/fpls.2021.633333
Edited by:
Cristina Garcia-Viguera, Consejo Superior de Investigaciones Científicas (CSIC), SpainReviewed by:
Shenghui Jiang, Qingdao Agricultural University, ChinaKate Warpeha, University of Illinois at Chicago, United States
Copyright © 2021 Jia, Wang, Wang, Ye, Liu, Jiang, Sun, Yan, Wang, Wang and Fan. This is an open-access article distributed under the terms of the Creative Commons Attribution License (CC BY). The use, distribution or reproduction in other forums is permitted, provided the original author(s) and the copyright owner(s) are credited and that the original publication in this journal is cited, in accordance with accepted academic practice. No use, distribution or reproduction is permitted which does not comply with these terms.
*Correspondence: Bei Fan, ZmFuYmVpNTE3QDE2My5jb20=; Fengzhong Wang, d2FuZ2Zlbmd6aG9uZ0BzaW5hLmNvbQ==