- 1Institute of Crop and Nuclear Technology Utilization, Zhejiang Academy of Agricultural Sciences, Hangzhou, China
- 2Huzhou Keao Seed Co., Ltd., Huzhou, China
Sunflower seed storage is accompanied by the loss of seed vigor. Seed drying is a key link between seed harvest and seed storage; however, to date, the effect of seed drying on sunflower seed deterioration during storage remains unclear. The present study performed hot air drying for sunflower seeds with an initial moisture content of 30% to examine the manner in which drying temperature (35, 40, 45, 50, and 55°C) affects the drying performance and seed vigor following storage process (6 and 12 months). A drying temperature of 40°C was evidently safe for sunflower seeds, whereas the high drying temperatures (HTD, 45, 50, and 55°C) significantly lowered sunflower seed vigor by regulating the fatty acid metabolism, glycometabolism, and abscisic acid (ABA)/gibberellin (GA) balance. HDT significantly increased the seed damage rate and accelerated sunflower seed deterioration during natural and artificial aging process. Further biochemical analysis indicated that HDT significantly increased lipoxygenase and dioxygenase activities, leading to malonaldehyde and reactive oxygen species over-accumulation during storage. During early seed germination, HDT significantly inhibited fatty acid hydrolysis and glycometabolism by decreasing triacylglycerol lipase, CoA-SH oxidase, and invertase activities. Moreover, HDT remarkably increased ABA levels but reduced GA levels by regulating gene expressions and metabolic enzyme activities during early imbibitions. Cumulatively, the seed drying effect on sunflower seed vigor deterioration during the storage process may be strongly related to fatty acid oxidation and hydrolysis metabolism, toxic substance accumulation, and ABA/GA balance.
Introduction
Sunflower (Helianthus annuus L.), originally native to North America, represents an important oilseed crop in the world (Buti et al., 2011). Further, sunflower is the third most important oil crop in China, with over 1.15 million hm2 planting area and 299 t overall yield (Zhang and Zhang, 2018). Sunflower has recently been gaining substantial attention because of its highly edible oil production and ornamental values.
Seed vigor is indicated by more rapid germination or greater tolerance of stressful conditions (Rajjou et al., 2012). Furthermore, seed longevity plays a crucial role in seed vigor, determined by seed physiological, genetic characteristics and storage conditions (Dang et al., 2014). High seed vigor is strongly related with excellent field emergence and productivity, whereas low seed vigor results in decreased output. Seed moisture can affect seed vigor in stored seeds; thus, it should be kept in an appropriate range for seed storage (Akpinar, 2005; Bie et al., 2007). Normally, sunflower seed are usually harvested with lower initial content (<25%) in main sunflower producing areas in northern China. However, harvested sunflower seeds have a high initial moisture content (IMC) ranging from 20 to 35% in southern China, especially in Zhejiang province, mainly due to the influence of the rainy season. A high IMC may result in microbial proliferation, thereby causing deteriorated seed vigor. Ideally, a moisture content of 9.0% is considered appropriate for sunflower seeds (Zhen et al., 2020). Therefore, it is important to conduct research on seed drying for the reproduction of high-vigor sunflower seeds.
Seed drying involves a non-linear process as it is delayed for a long term and is extremely complicated. IMC, drying temperature and drying environment are the key parameters that affect seed response to hot air drying. Drying temperature regulated seed vigor, and regulates the seed temperature and drying speed. Drying at high temperatures may lead to seed stress cracks, enzyme activity dysregulation, and seed vigor loss (Igathinathane et al., 2008). Moreover, it may decrease seed vigor via suppressing storage substance catabolism in the seed endosperm. Besides, the impact of drying temperature on seed germination depends on the IMC, the higher the IMC the higher the effect (Huang et al., 2020b). Seeds with high IMC are likely to cause seed vigor degradation with a high drying temperature (Thakur and Gupta, 2006). A lower drying temperature should be applied for high IMC seeds to ensure seed vigor (Zhou et al., 2018).
Seed vigor deterioration routinely occurs during the seed storage process, closely associated with seed property, storage conditions, and storage duration (Faligowska et al., 2012; Zhao et al., 2017). However, there is no report on the mechanism via which drying temperature affects seed vigor deterioration during the crop seed storage process, particularly in oilseeds. Oilseeds are rich in unsaturated fatty acid, and the oxidation of unsaturated fatty acids can easily cause cell membrane permeability. Lipoxygenases (LOXs) play a vital role in lipid peroxidation of the seeds of rice (Oryza sativa L.) (Xu et al., 2015), soybean (Glycine max L.) (Lima et al., 2010), sweet lupin (Lupinus L.) (Stephany et al., 2015), and canola (Brassica napus L.) (Terp et al., 2006). LOX catalyzes oxygenation of polyunsaturated fatty acids (PUFAs), including linoleic and linolenic acids, to form conjugated diene hydroperoxides. Furthermore, lipid oxidation produces peroxides and free radicals, which cause damage to DNA, proteins, membrane structures, and cell tissues, thereby inducing seed vigor loss (Chen et al., 2010). Zhou et al. (2019) reported that the processes of triacylglycerol and fatty acid hydrolysis were blocked during the early germination stage of the aging soybean seeds, and it was the primary cause for the reduced soybean seed vigor following storage.
Seeds contain a large amount of macromolecules nutrients such as fat, starch, and protein. Such macromolecules can be gradually decomposed and used in germination (Xiong et al., 2019). Triacylglycerol is the primary storage lipid in oilseeds, and its decomposition plays a vital role in oilseed germination, including sunflower and soybean seed. In early germination process, triacylglycerol undergoes direct hydrolysis to form glycerin and fatty acids via lipase. Fatty acids generate CoA-SH via theβ-oxidation pathway, which further produces oxaloacetic acid via the glyoxylate or tricarboxylic acid cycle and is finally reversely converted to sucrose via glycolysis (Goepfert and Poirier, 2007). Glycerin is another triacylglycerol hydrolysis product that can produce phosphoglyceride catalyzed byglycerol-3-P dehydrogenase (GPDH) and subsequently generate dihydroxyacetone phosphate (DHAP) via dehydrogenation. DHAP is eventually converted to glucose and used in seed germination (Quettier et al., 2008).
Gibberellin (GA) and abscisic acid (ABA) are two vital phytohormones involved in the seed dormancy and germination. GA is responsible for seed dormancy breaking and seed germination induction and the subsequent activation of seed endogenous hydrolase activity that results in the promotion of storage substance cleavage (Rajjou et al., 2012). GA biosynthesis in higher plants is primarily catalyzed by GA20-oxidase (GA20ox) and GA3-oxidase (GA3ox), whereas GA catabolism is catalyzed by GA2-oxidase (GA2ox) (Hedden and Thomas, 2012). In comparison, ABA plays a major role in seed dormancy. ABA accumulation leads to seed dormancy, whereas its deficiency provokes non-dormancy phenotypes. Zeaxanthin epoxidase (ZEP), abscisic aldehyde oxidase (AAO), and 9-cis-epoxycarotenoid dioxygenase (NCED) represent three main enzymes responsible for ABA biosynthesis in higher plants, and ABA-8′-hydroxylase (ABA8ox) is responsible for ABA catabolism (Vishwakarma et al., 2017).
To date, research regarding the optimal sunflower seed drying temperature is scarce. Moreover, the regulatory mechanism of drying temperature on sunflower seeds vigor during storage and germination has not yet been thoroughly examined. In the present study, the effects of air drying temperature (35, 40, 45, 50, and 55°C) on drying performance and seed vigor of sunflower seeds during storage and early germination were investigated. In addition, the reactive oxygen species (ROS) metabolism, fatty acid metabolism, glycometabolism, and plant hormone metabolism of sunflower seeds during storage and germination was assessed. The present research may provide a future reference to design of a drying technology for sunflower seeds and enrich the regulatory mechanism underlying the effect of drying temperature on crop seed vigor.
Materials and Methods
Materials
Seeds of the sunflower (Helianthus annuus L.) cultivar “Aidatou” were used in the present study. Seeds with an IMC of 30% were harvested on October 13, 2018. The IMC was measured using a corn moisture apparatus (GAC-2100AGRI, Tuopu, Hangzhou, China), with ten biological replications. The weather conditions during harvest are shown in Supplementary Table 1. Sunflower seeds were dried with the Sanjiu low-temperature dryer (NEW PRO-120 H).
Drying Experiment
Sunflower seeds with an IMC of 30% were dried until a moisture content of 9% was achieved at 35, 40, 45, 50, and 55°C. Seed samples were taken every 6 h during the drying process. Subsequently, the damaged seed number was calculated. Seeds with visually observable cracks were considered as damaged seeds. Each drying experiment was performed in triplicate under every drying temperature.
Seed Storage
The dried sunflower seeds were stored in a dry, airtight container at room temperature for 1, 6, and 12 months. Seed samples were collected and used for the germination test and further determinations.
Accelerated Aging Test
The accelerated aging test (AAT) was performed with the method of Missaoui and Hill (2015). Briefly, 100 sunflower seeds were added into a seed aging box for a 48-h incubation under 45°C and 100% relative humidity conditions (AAT conditions were decided based on preliminary experiments). Thereafter, seeds were dried at room temperature for 2 days before the germination tests. The accelerated aging test was performed with four biological replicates.
Seed Germination Test
Seed germination test was conducted after disinfection of the seeds with 0.1% sodium hypochlorite solution for 15 min. Sunflower seeds were added into rolled towels (with 50 seeds in each towel) and subjected to 5 days of incubation within the germination chamber under 25°C and 12-h/12-h light/dark cycle conditions. The germinated seed number was calculated on days 3 and 5, and seed samples were sampled on days 1 and 3 during germination. The seed germination test was performed with four biological replicates.
H2O2, O2–, and Malondialdehyde Analysis
H2O2 content was analyzed with the method of Huang et al. (2011) based on absorbance measurement of the supernatant-measured as its optical density (OD) at 390 nm. In addition, O2– level was determined with the method of Jiang and Zhang (2003) based on supernatant OD at 530 nm. Malondialdehyde (MDA) level was detected according to the method reported by Gao et al. (2009) and calculated based on supernatant OD at 532 and 600 nm. The analysis of H2O2, O2–, and malondialdehyde was performed with four biological replicates.
Quantification of Various Sugars
Anthrone-H2SO4colorimetry was applied for determining the concentration of total soluble sugar in sunflower seeds according to Zhu et al. (2015). In addition, sucrose concentration was measured using the resorcinol approach based on OD at 480 nm (Shi et al., 2016). The fructose concentration was measured using a previously reported approach (Cai et al., 2016). Glucose level was analyzed with high performance liquid chromatography (HPLC) according to Bailly et al. (2010). The quantification of various sugars was performed with four biological replicates.
ATP and Energy Charge Analysis
High performance liquid chromatography was performed to determine ATP and energy charge according to Liu et al. (2006). Data of ATP and energy charge analysis were expressed as means of four replicate determinations. The analysis of ATP and energy charge was performed with four biological replicates.
Fatty Acid Concentration Measurement
Sunflower seed samples were grinded to a powder form for fatty acid extraction. Briefly, 2 mL of n-hexane was added to 50 mg sunflower seed powder in each tube, followed by 15 min of ultrasonic extraction (40 kHz) and 3 h of incubation at room temperature. Thereafter, the mixed solution was subjected to 10 min of centrifugation under 4°C and 10,000 rpm. The supernatants were collected and subjected to vortex oscillation for 30 s after the addition of 3 mL methanolic potassium hydroxide solution (0.6 M). Thereafter, the supernatant was collected and added to a 5-mL bottle, and n-hexane was used to dilute the solution to a total volume of 5 mL. Subsequently, a 0.45-μm filter (organic phase) was used to add the extract into the gas chromatography–mass spectrometry (GC-MS) system. Finally, fatty acid concentration was test with the method described by Yang et al. (2017). The fatty acid concentration measurement was performed with four biological replicates.
ABA and GA Level Determination
The ABA and GA extraction from sunflower seeds was performed using the method of Huang et al. (2017). Typically, ABA and GA concentrations within extracting solution were determined using the HPLC system using a reversed phase column (C18, 6.0 mm × 120 mm, particle size 5 mm; Shim-Pack CLC-ODS) and an ultraviolet detector. Meanwhile, the mobile phase composed of methanol/water (64:36, v/v) was adopted with a flow rate of 1 mL⋅min–1. ABA and GA levels were determined from four biological replicates.
Assay of Fatty Acid Metabolism-, Glycometabolism-, and ABA and GA Metabolism-Related Enzyme Activity
The activities of lipoxygenase (LOX), dioxygenase (DOX), triacylglycerol lipase (LIPG), GPDH, CoA-SH oxidase (ACX), phosphoenolpyruvate carboxykinase (PCK), invertase (INV), NCED, ZEP, AAO, ABA8ox, GA3ox, GA20ox, and GA2ox were detected using an enzyme-linked immune assay kit (Mlbio, Shanghai, China) according to the kit introduction. Following the chromogenic reaction, an enzyme mark instrument was used to spectrophotometrically measure the color change at 450 nm. The activities of enzymes related to fatty acid metabolism, glycometabolism, and ABA and GA metabolism were determined according to the OD of the samples relative to the standard curve. The enzyme activity analysis was performed with four biological replicates.
Gene Expression Analysis
The PrimeScriptTM RT Reagent Kit (Vazyme, Nanjing, China) was used for RNA extraction from seed samples and cDNA synthesis via reverse transcription. The CFX96 Touch Real-Time PCR machine (Bio-Rad) was adopted for quantitative polymerase chain reaction (qPCR) procedure using the ChamQ SYBR qPCR Master Mix (Vazyme). Supplementary Table 2 presents all primers used in the present study; 18srRNA was used as the endogenous reference. The fold change (FC) in expression was calculated as follows: FC = EΔCt, where E indicates the mean gene amplification efficacy and ΔCt stands for the difference in mean Ct values (obtained from all duplicates). The values were presented in the manner of mean SD after normalization. The gene expression analysis was performed with four biological replicates, and each was made in three technical replicates.
Statistical Analyses
Data were statistically analyzed with one-way ANOVA using the Statistical Analysis System software. Further, the least significant difference of p value of < 0.05 (LSD0.05) was adopted for multiple comparisons. Percentage data were converted using the arcsine transformation before statistical comparison as follows: ŷ = arcsine[sqrt (x/100)].
Results
HDT Decreased the Moisture Content and Increased the Seed Deterioration Rate in Sunflower
The sunflower seed drying rate and equilibrium temperature showed substantial increase with the increase in drying temperature. Consequently, the drying time significantly declined with the increase of drying temperature (Table 1). The drying time required for reaching the target seed moisture content of 9% was 36.1, 30.3, 26.2, 22.0, and 18.2 h at drying temperatures of 35, 40, 45, 50, and 55°C, respectively (Figure 1A).

Table 1. High drying temperature decreased drying time and increased drying rate and seeds temperature of sunflower seeds.
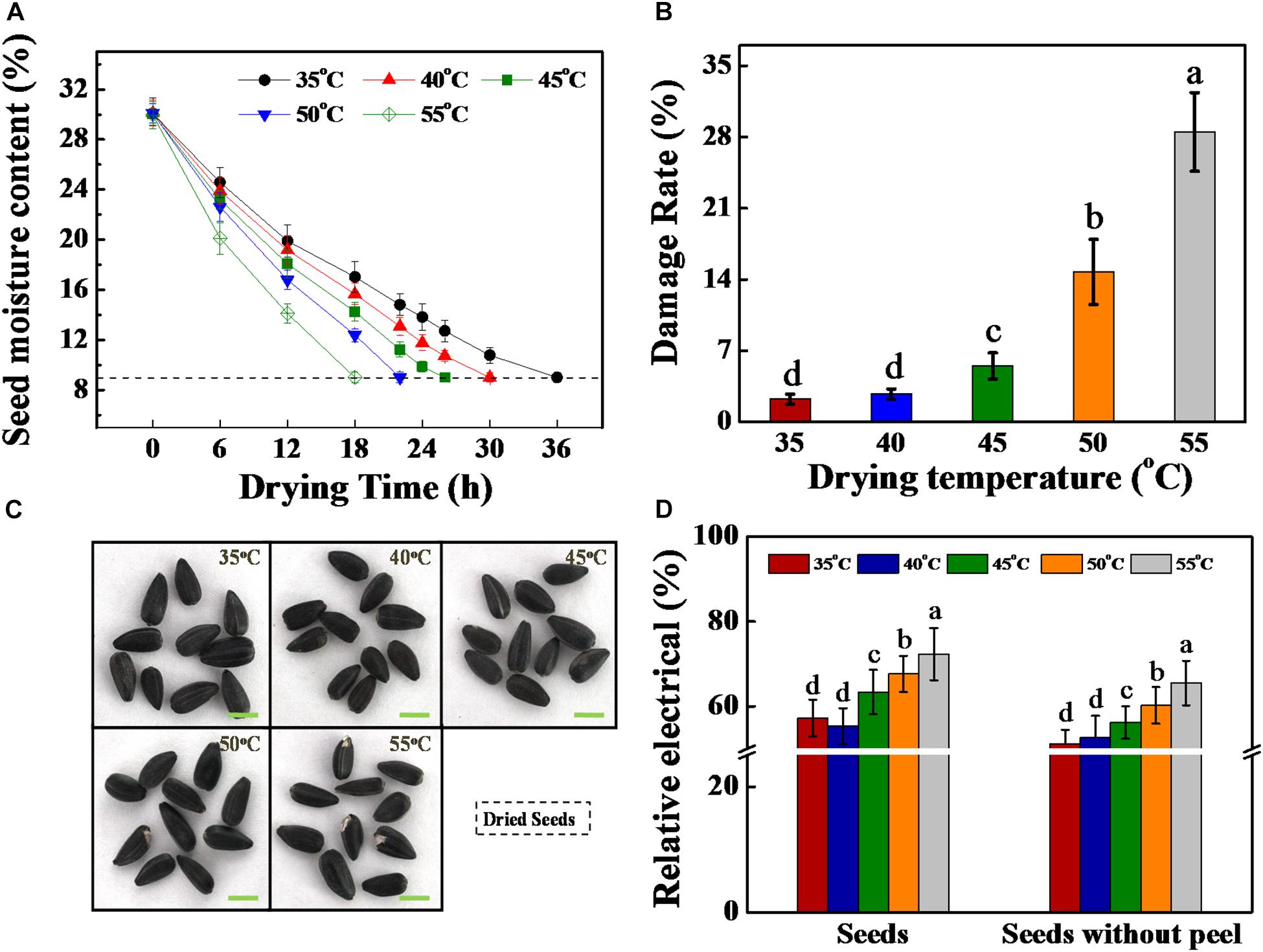
Figure 1. High drying temperature decreased the moisture content and increased the damage rate of sunflower seeds. (A) Seed moisture content during drying stage. (B) Damage rate of dried sunflower seeds after drying. (C) Photographs of dried sunflower seeds after drying. (D) Relative electrical of dried sunflower seeds. Sunflower seeds were dried to a target moisture content of 9% at temperatures of 35, 40, 45, 50, and 55°C. Percentages represent the means from four experiments ± SE. The diverse small letter(s) on top of the bars indicate significant differences (p < 0.01, LSD) between treatments. Each drying experiment was performed in three biological replicates.
Thereafter, in the present study, the drying effect on the damage rate and relative electrical in sunflower seeds were further analyzed. The seed damage rate remarkably enhanced with the increase in drying temperature. This rate was found to be < 5% under temperatures of 35, 40, and 45°C. By contrast, the damage rates were 14.8 and 28.5% under temperatures of 50 and 55°C, respectively (Figures 1B,C). Consistent with the above result, the relative electrical of sunflower seeds with or without peel were significantly increased with the increase in drying temperature (Figure 1D).
HDT Inhibited the Germination of Sunflower Seeds During Storage
After storage for 0, 6, and 12 months, the germinability of sunflower seeds was determined (Figure 2). HDT significantly decreased the germination rate of sunflower seeds after storage for different time periods. For sunflower seeds stored for 0 month, the germination rates of seeds dried at 50 and 55°C were significantly lower than those of seeds dried at 35, 40, and 45°C. After 6 months of storage, sunflower seeds dried at 35 and 40°C maintained a high germination rate (90%), whereas those dried at 45, 50, and 55°C showed significantly decreased germination rates (82, 70, and 53%, respectively). Moreover, the germination rate of 45°C-dried sunflower seeds was remarkably lower than those in 40°C-dried seeds after 6 months of storage, although no significant difference in germination rate was observed between the 40- and 45°C-dried seeds at 0 month of storage. A similar finding was observed in sunflower seeds stored for 12 months. Furthermore, the radicle weight and length data were also consistent with the germination results (Supplementary Figure 1). To further confirm the drying effect on sunflower seed vigor, we used AAT to obtain the artificially aged sunflower seeds. The germination rate of AAT-aged sunflower seeds significantly decreased with the increase in drying temperature (Supplementary Figure 2). In general, drying temperatures higher than 40°C reduced sunflower seed vigor during the natural and artificial aging process. The drying temperature of 40°C was evidently safe for sunflower seeds with an IMC of 30%. On clarifying the role of drying temperature (35, 40, 45, 50, and 55°C) in germination deterioration of stored sunflower seeds, only seed samples dried at 40, 45, and 50°C were used in subsequent experiments.
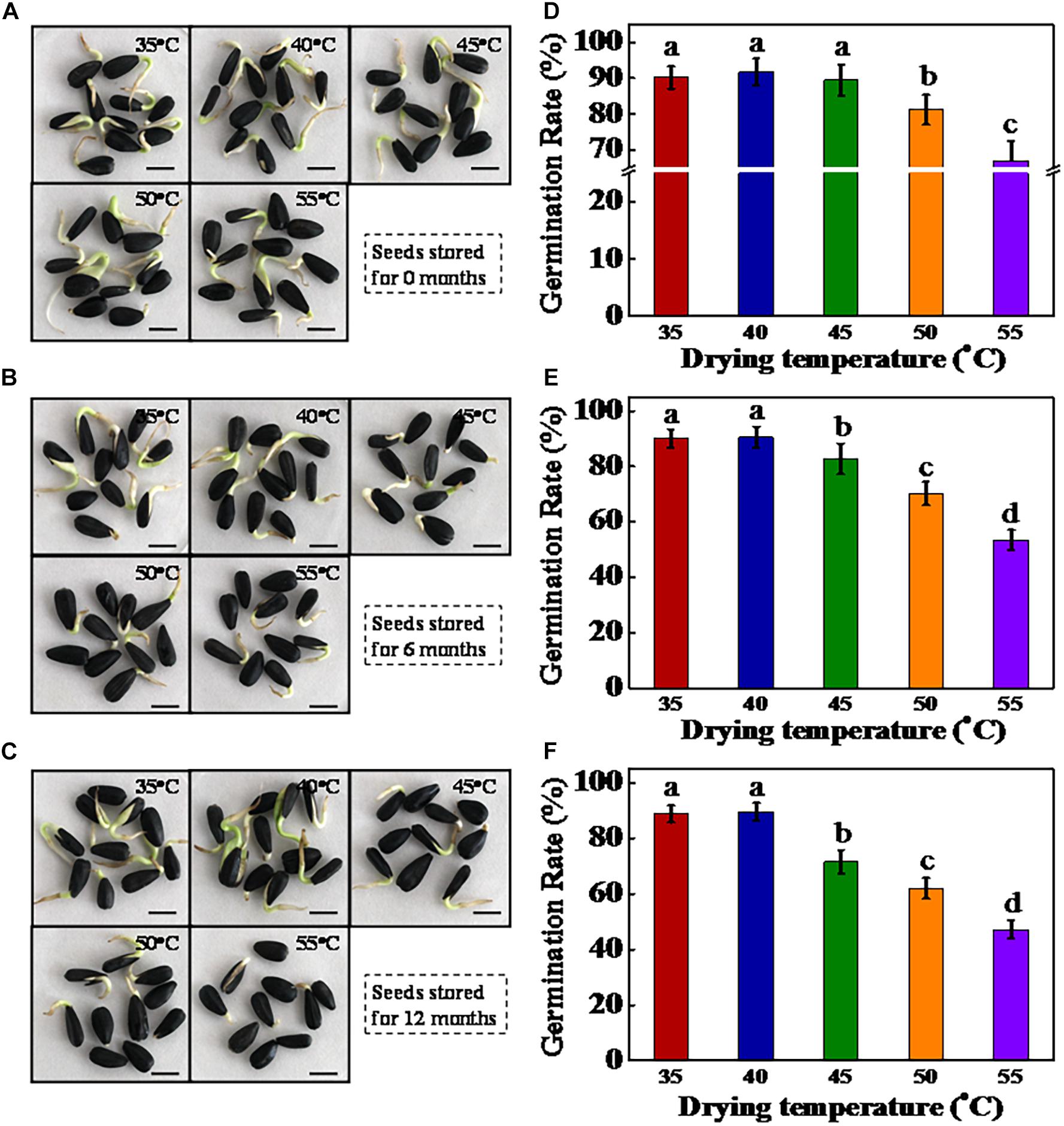
Figure 2. High drying temperature inhibited the germination of sunflower seeds during storage. (A–C) Representative photographs of sunflower seeds during the imbibition process (2 days). The dried sunflower seeds were subjected to 1, 6, or 12 months of storage before analysis. Scale bar = 10 mm. (D–F) Quantitative analysis on the germination rates across diverse samples (A–C) (on day 7 of sowing). Sunflower seeds were dried to a target moisture content of 9% at temperatures of 35, 40, 45, 50, and 55°C. Percentages represent the means from four experiments ± SE. The diverse small letter(s) on top of the bars indicate significant differences (p < 0.01, LSD) between treatments. Seed germination test was performed with four biological replicates.
HDT Induced H2O2, O2–, and MDA Over-Accumulation in Sunflower Seeds During Storage and Germination
The respiration-generated ROS contributed to seed aging. ROS over-accumulation induced lipid peroxidation by LOX, thus causing damage to cell membranes and further deteriorating seed vigor (Sharma et al., 2012; Ahmed et al., 2016). To further investigate the physiological and molecular mechanisms of drying temperature on sunflower seed deterioration, we analyzed H2O2, O2–, and MDA concentrations during storage and early germination of sunflower seeds. As is shown in Figure 3, HDT significantly elevated H2O2, O2–, and MDA levels in sunflower seeds during storage and early germination. At 0, 6, and 12 months of storage, significantly higher H2O2, O2–, and MDA levels were detected in 50°C-dried seeds compared with those in 40- and 45°C-dried sunflower seeds. Moreover, the drying temperature of 50°C significantly increased the H2O2, O2–, and MDA levels of sunflower seeds on days 1 and 3 of germination compared with temperatures of 40 and 45°C.
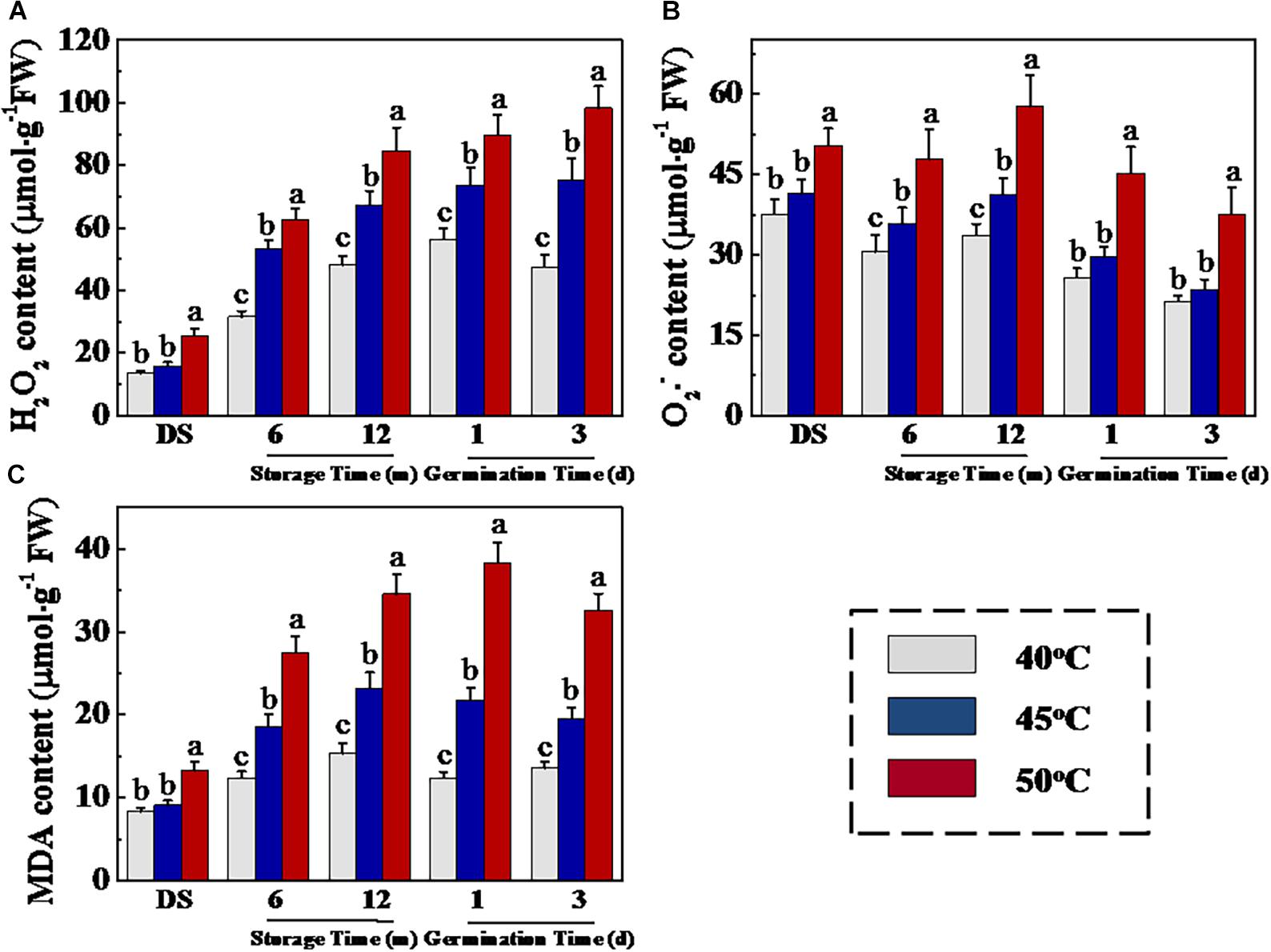
Figure 3. High drying temperature increased H2O2 (A), O2– (B), and MDA (C) levels in sunflower seeds during storage and germination. Sunflower seeds were dried to a target moisture content of 9% at temperatures of 40, 45, and 50°C. The dried sunflower seeds were subjected to 1, 6, or 12 months of storage before analysis. Sunflower seeds stored for 12 months were used for germination test. Percentages represent the means from four experiments ± SE. The diverse small letter(s) on top of the bars indicate significant differences (p < 0.01, LSD) between treatments. The analysis of H2O2, O2–, and malondialdehyde was performed with four biological replicates.
HDT Increased LOX and α-Dioxygenase Activities and Transcription of Corresponding Genes in Sunflower Seeds During Storage and Germination
Fatty acid oxidation during storage is an important cause of toxic substance accumulation in the aging seeds, such as ROS and MDA. LOXs and dioxygenases (DOXs) represent the most frequently fatty acid oxidases (Andreou and Feussner, 2009), and we further analyzed the impact of drying temperature on the activities of these two enzymes as well as transcription of their corresponding genes (Figure 4). The LOX activity of sunflower seeds was remarkably increased with the increase of drying temperature during storage and early germination (Figure 4A). In addition, the DOS activity of 50°C-dried sunflower seeds during storage and early germination were remarkably higher than that of 40°C-dried sunflower seeds (Figure 4B). Consistently, the qPCR analysis revealed that HDT significantly up-regulated HaLOX2, HaLOX3, and HaDX2 transcription in 6- and 12-month-stored sunflower seeds (Figure 4C).
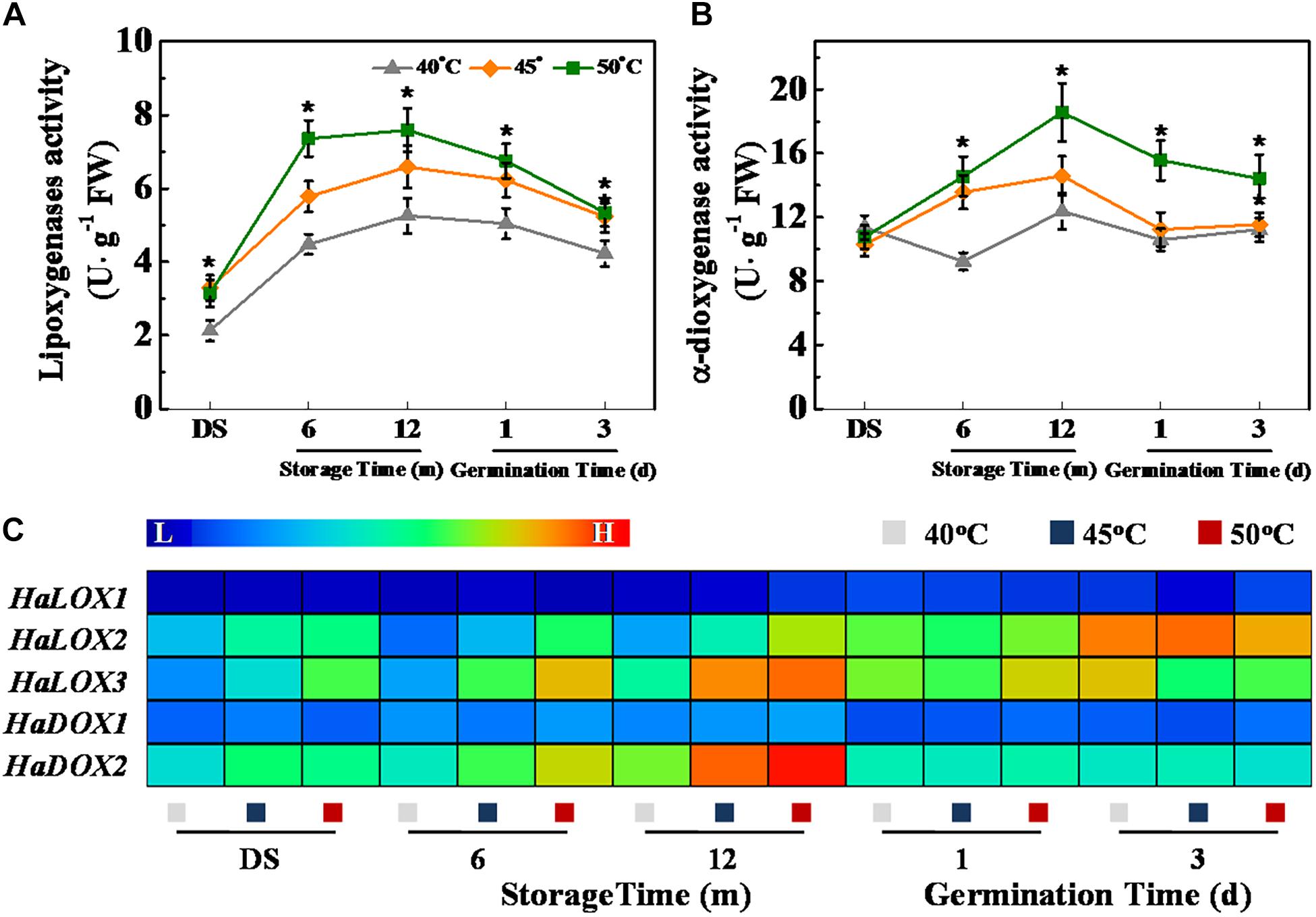
Figure 4. High drying temperature increased lipoxygenases (A) and α-dioxygenase (B) activities and transcription of corresponding genes (C) in sunflower seeds during storage and germination. Sunflower seeds were dried to a target moisture content of 9% at temperatures of 40, 45, and 50°C. The dried sunflower seeds were subjected to 1, 6, or 12 months of storage before analysis. Sunflower seeds stored for 12 months were used for germination test. LOX: lipoxygenases; DOS: α-dioxygenase. Percentages are the means of four experiments ± SE. The asterisk (*) indicates significant differences (p < 0.01, LSD) across treatments. The Illustrator software was used for creating the heat map. Gene expression levels from low (L) to high (H) indicated the lowest and highest levels in the entire database. The enzyme activity analysis was performed with four biological replicates. The gene expression analysis was performed with four biological replicates, and each was made in three technical replicates.
HDT Decreased Soluble Sugar Concentrations and ATP Level and Energy Charge in Sunflower Seeds During Storage and Imbibition Stages
The seed storage substances are the major sources of energy during early seed germination and seedling emergence, and soluble sugar is the main nutrient produced by the decomposition of storage substances. To further analyze the mechanism of drying temperature in affecting sunflower seed vigor, we then determined soluble sugar, fructose, and sucrose contents in sunflower seeds (Figure 5). The results showed that HTD significantly decreased the soluble sugar, fructose, and sucrose levels in sunflower seeds at 12 months of storage. Moreover, significant higher levels of soluble sugar, fructose, and sucrose were observed in 40°C-dried sunflower seeds compared with those in 45- and 50°C-dried sunflower seeds at days 1 and 3 of germination. In addition, similar drying effects on ATP level and energy charge were shown in sunflower seeds during early germination process (Supplementary Figure 3). The above findings revealed that drying temperature affected sunflower seed germination after storage via the regulation of soluble sugar and ATP levels.
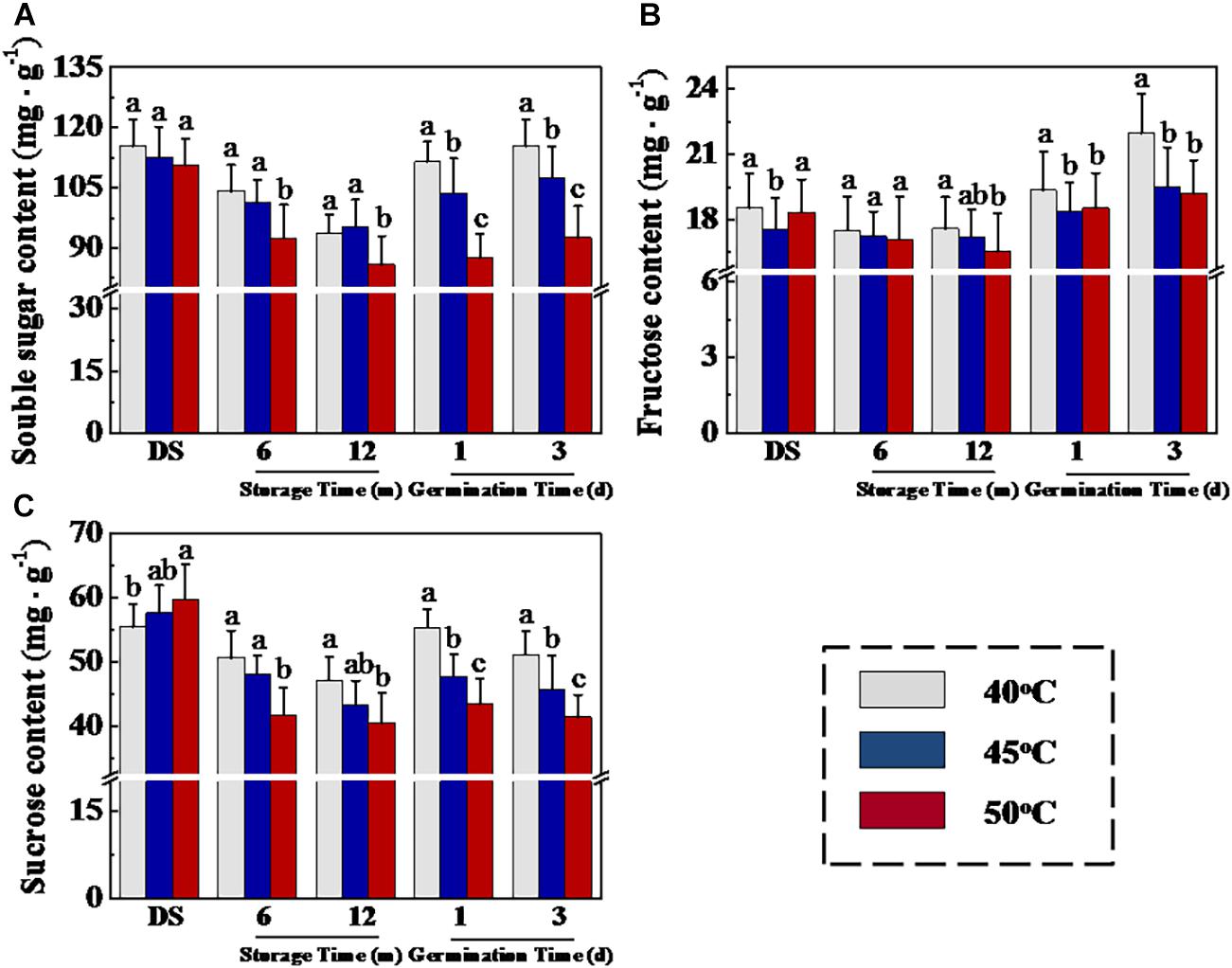
Figure 5. High drying temperature decreased soluble sugar (A), fructose (B), and sucrose (C) levels in sunflower seeds during storage and germination. Sunflower seeds were dried to a target moisture content of 9% at temperatures of 40, 45, and 50°C. The dried sunflower seeds were subjected to 1, 6, or 12 months of storage before analysis. Sunflower seeds stored for 12 months were used for germination test. Percentages represent the means from four experiments ± SE. The diverse small letter(s) on top of the bars indicate significant differences (p < 0.01, LSD) between treatments. The quantification of various sugars was performed with four biological replicates.
It is well known that triacylglycerol hydrolysis in germinated oilseeds generated fatty acids and glycerol, which are subsequently transformed to different soluble sugars by gluconeogenesis (Eastmond, 2007; Quettier and Eastmond, 2009). For elucidating the reasons responsible for the effect of drying temperature on soluble sugar levels in sunflower seeds during early germination, we measured fatty acid concentration during sunflower seed storage and germination (Figure 6). In the GC-MS analysis, drying temperature demonstrated no effect on total fatty acid, unsaturated fatty acid, and saturated fatty acid concentrations in stored sunflower seeds. Whereas, on days 1 and 3 of germination, total fatty acid and unsaturated fatty acid concentrations in 40°C-dried sunflower seeds were evidently higher than those in 45- and 50°C-dried sunflower seeds. Moreover, the drying temperature of 50°C caused a significant decrease in saturated fatty acid concentration on day 3 of germination.
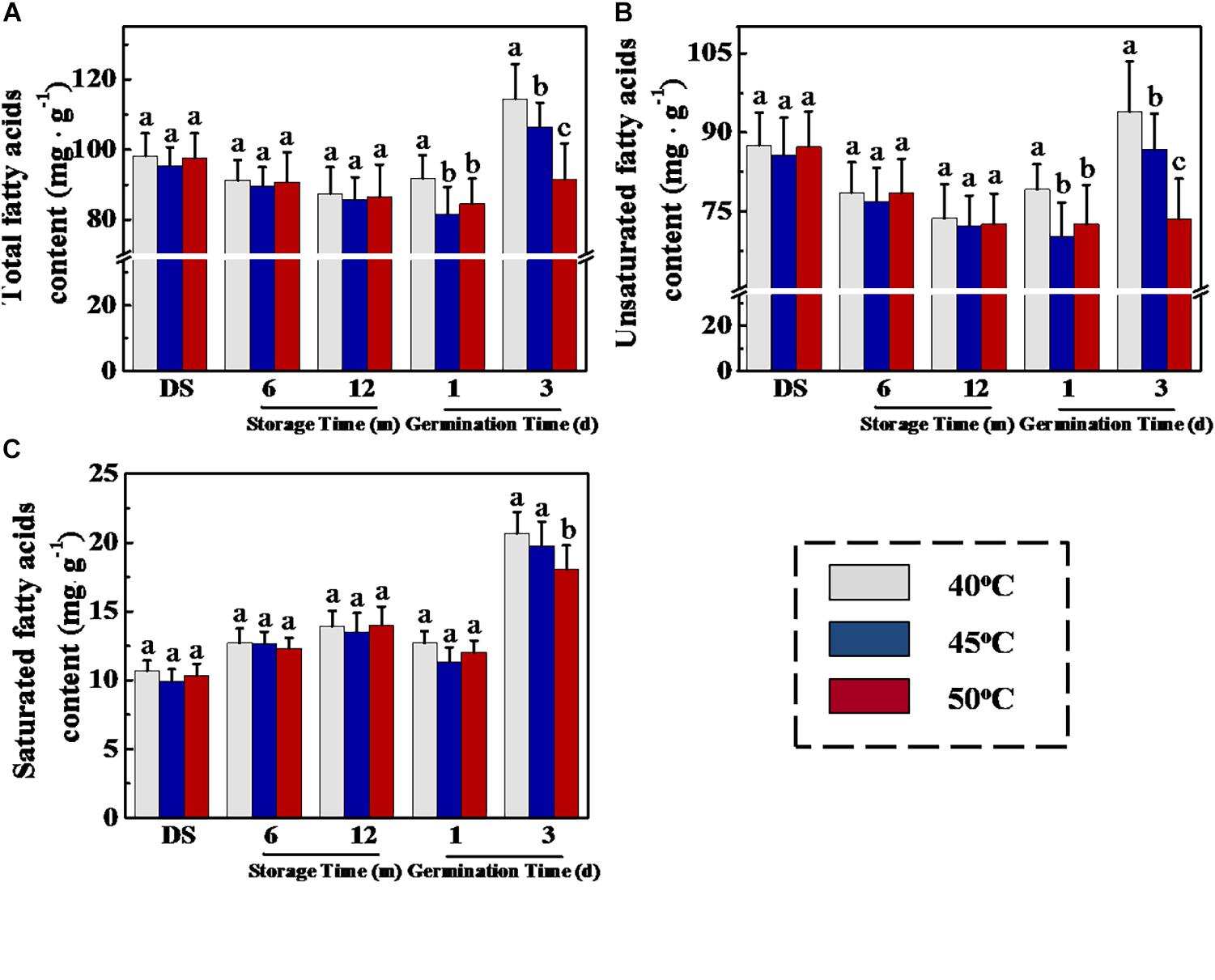
Figure 6. Effect of drying temperature on total fatty acid (A), unsaturated fatty acid (B), and saturated fatty acid (C) level in sunflower seeds during storage and germination. Sunflower seeds were dried to a target moisture content of 9% at temperatures of 40, 45, and 50°C. The dried sunflower seeds were subjected to 1, 6, or 12 months of storage before analysis. Sunflower seeds stored for 12 months were used for germination test. Percentages represent the means from four experiments ± SE. The diverse small letter(s) on top of the bars indicate significant differences (p < 0.01, LSD) between treatments. The fatty acid analysis was performed with four biological replicates.
Drying Temperature Regulated Several Key Enzyme Activities Involved in Fatty Acid Metabolism and Glycometabolism in Sunflower Seeds During Storage and Germination
Considering that HDT decreased soluble sugar and fatty acid levels during sunflower seed imbibition, the drying effect on activities of several critical enzymes related to triacylglycerol conversion into fatty acids and sugars was examined (Figure 7). No significant difference in LIPG, ACX, and INV activities was observed in 6- and 12-month-stored sunflower seeds between different treatments. However, LIPG, ACX, and INV activities of imbibition seeds (days 1 and 3) dried at 40°C were apparently higher than that of 45- and 50°C-dried seeds. Drying temperature exerted no effect on GPDH and PCK activities during storage and early germination. These results were consistent with the drying effect on fatty acid and soluble sugar concentrations in sunflower seeds during early germination process.
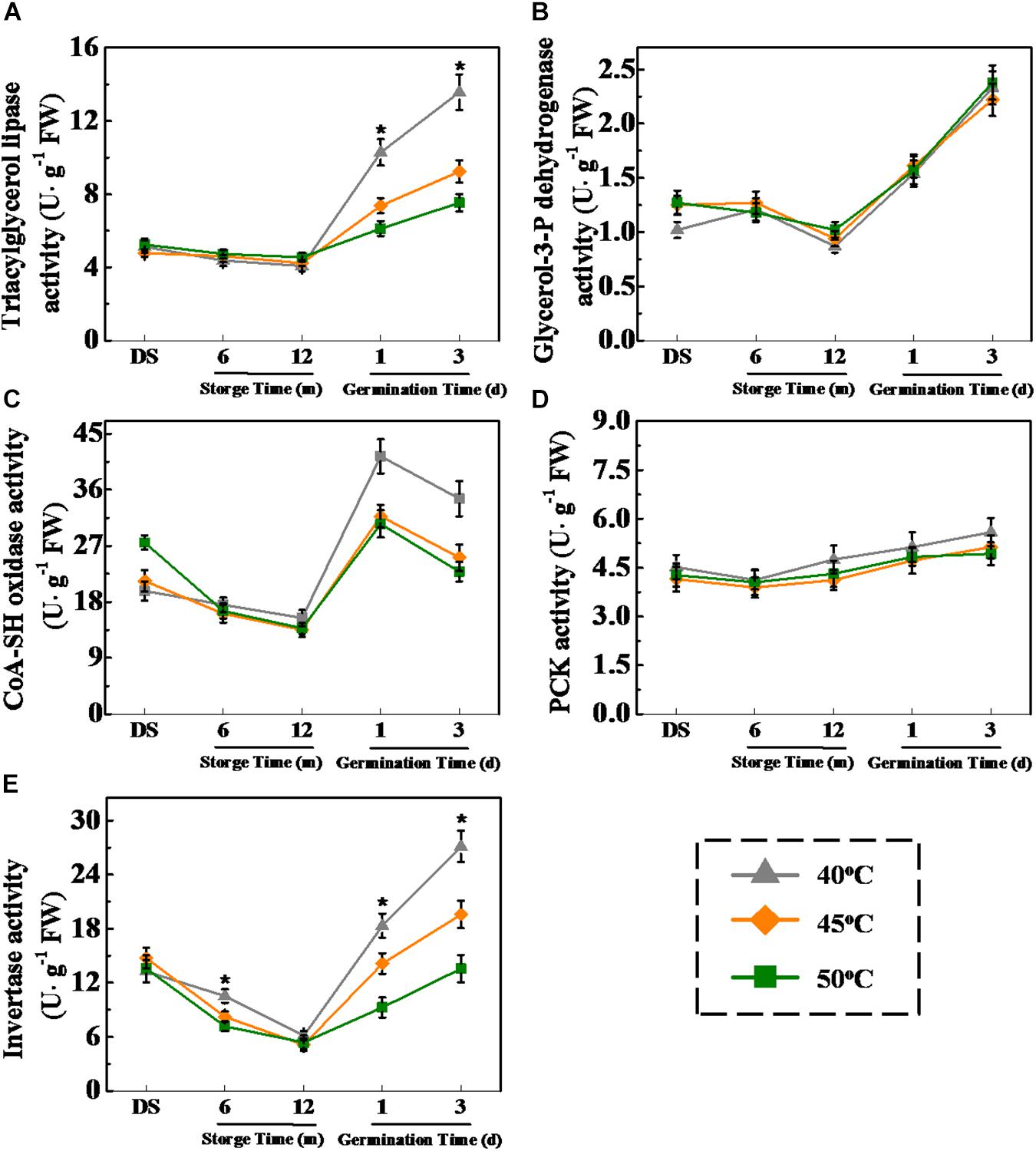
Figure 7. Effect of drying temperature on the activities of several key enzymes related to fatty acid metabolism and glycometabolism in sunflower seeds during storage and germination. (A) Triacylglycerol lipase activity; (B) glycerol-3-P dehydrogenase activity; (C) CoA-SH oxidase activity; (D) phosphoenolpyruvate carboxykinase (PCK) activity; and (E) invertase activity. Fresh sunflower seeds were dried to a target moisture content of 9% at temperatures of 40, 45, and 50°C. The dried sunflower seeds were subjected to 1, 6, or 12 months of storage before analysis. Sunflower seeds stored for 12 months were used for germination test. Percentages represent the means from four experiments ± SE. The diverse small letter(s) on top of the bars indicate significant differences (p < 0.01, LSD) between treatments. The enzyme activity analysis was performed with four biological replicates.
HDT Decreased the Transcription Levels of Fatty Acid Metabolism- and Glycometabolism-Related Genes in Sunflower Seeds During Storage and Germination
The qPCR analysis revealed that the transcriptional levels of HaLIPG1-3, HaACX1-3, and HaINV1-5 were lowly expressed during storage process and highly expressed during the early germination period. The transcription of HaLIPG1, HaLIPG3, HaACX2, and HaACX4 was significantly declined with the increase of drying temperature at days 1 and 3 of germination. Similarly, the drying temperatures of 45 and 50°C significantly lowered the transcription of HaINV3 and HaINV4 during early germination compared with the drying temperature of 40°C (Figure 8).
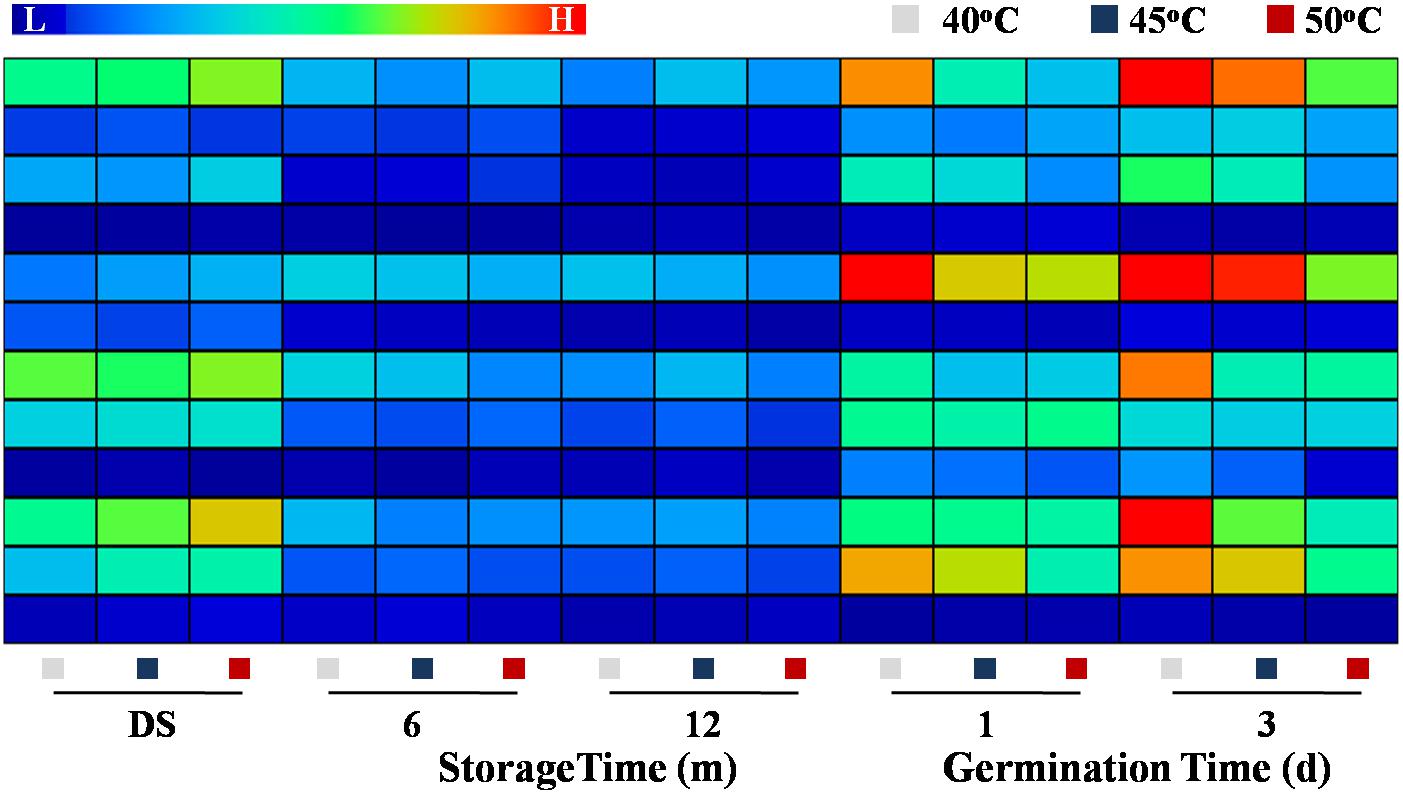
Figure 8. Effect of drying temperature on the transcription levels of fatty acid metabolism- and glycometabolism-related genes in sunflower seeds during storage and germination. Sunflower seeds were dried to a target moisture content of 9% at temperatures of 40, 45, and 50°C. The dried sunflower seeds were subjected to 1, 6, or 12 months of storage before analysis. Sunflower seeds stored for 12 months were used for germination test. LIPG: triacylglycerol lipase; ACX: CoA-SH oxidase; INV: invertase. The Illustrator software was used for creating the heat map. Gene expression levels from low (L) to high (H) indicated the lowest and highest levels in the entire database. The gene expression analysis was performed with four biological replicates, and each was made in three technical replicates.
HaLIPG encodes LIPG, a critical enzyme related to triacylglycerol hydrolysis. HaACX encodes ACX associated with the β-oxidation of fatty acid. HaINV is responsible for encoding INV, which catalyze the transformation of sucrose to fructose. As found in our study, drying temperature exerted certain effect on triacylglycerol hydrolysis and fatty acid transformation to sugars via regulating the key pathway-related gene expression and enzyme activities. Fructose and sucrose, the triacylglycerol hydrolysis end-products, generated ATP and energy essential to support the germination of stored sunflower seeds.
HDT Increased ABA Level and Decreased GA Level by Affecting the Corresponding Enzyme Activities in Sunflower Seeds During Storage and Germination
Considering the important role of GA and ABA in plant seed dormancy and germination (Shu et al., 2016, 2018), the relationship between drying effect on seed vigor and the metabolic pathways of GA and ABA during storage and early germination of sunflower seeds was investigated (Figures 9, 10). The results showed that ABA level was significantly increased with the increase of drying temperature during sunflower seed storage and early germination (Figure 9A). The drying temperature of 50°C remarkably enhanced NCED and AAO activities in sunflower seeds during storage and germination. By contrast, ABA8ox activity in 50°C-dried sunflower seeds was apparently lower than those in sunflower seeds dried at 40 and 45°C (Figure 9E).
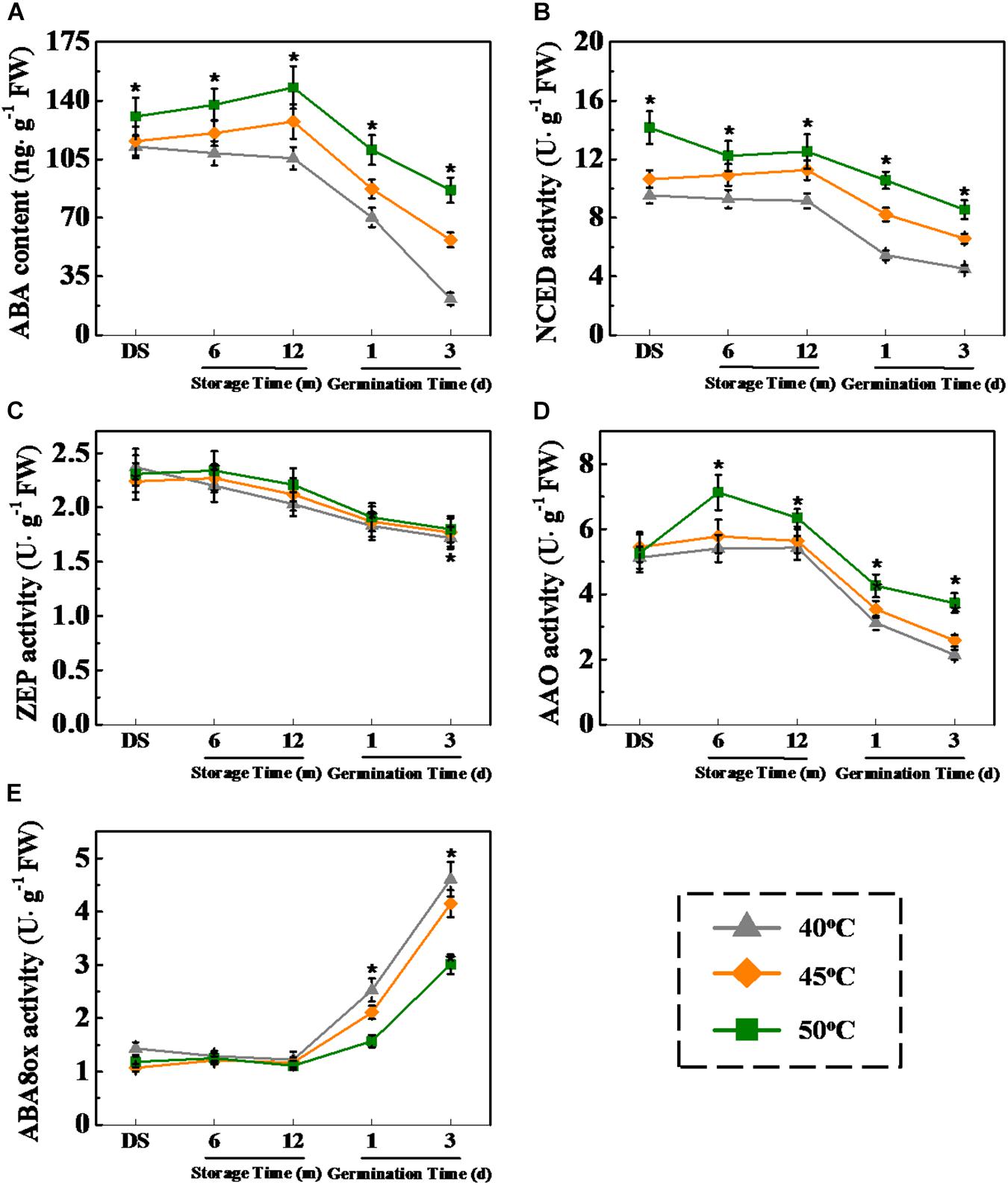
Figure 9. High drying temperature increased abscisic acid (ABA) level and affected corresponding enzyme activities in sunflower seeds during storage and germination. (A) ABA level; (B) 9-cis-epoxycarotenoid dioxygenase (NCED) activity; (C) Zeaxanthin epoxidase (ZEP) activity; (D) abscisic aldehyde oxidase (AAO) activity; and (E) ABA-8′-hydroxylases (ABA8ox) activity. Sunflower seeds were dried to a target moisture content of 9% at temperatures of 40, 45, and 50°C. The dried sunflower seeds were subjected to 1, 6, or 12 months of storage before analysis. Sunflower seeds stored for 12 months were used for germination test. Percentages represent the means from four experiments ± SE. The diverse small letter(s) on top of the bars indicates significant differences (p < 0.01, LSD) between treatments. The analysis of ABA and enzyme activity was performed with four biological replicates.
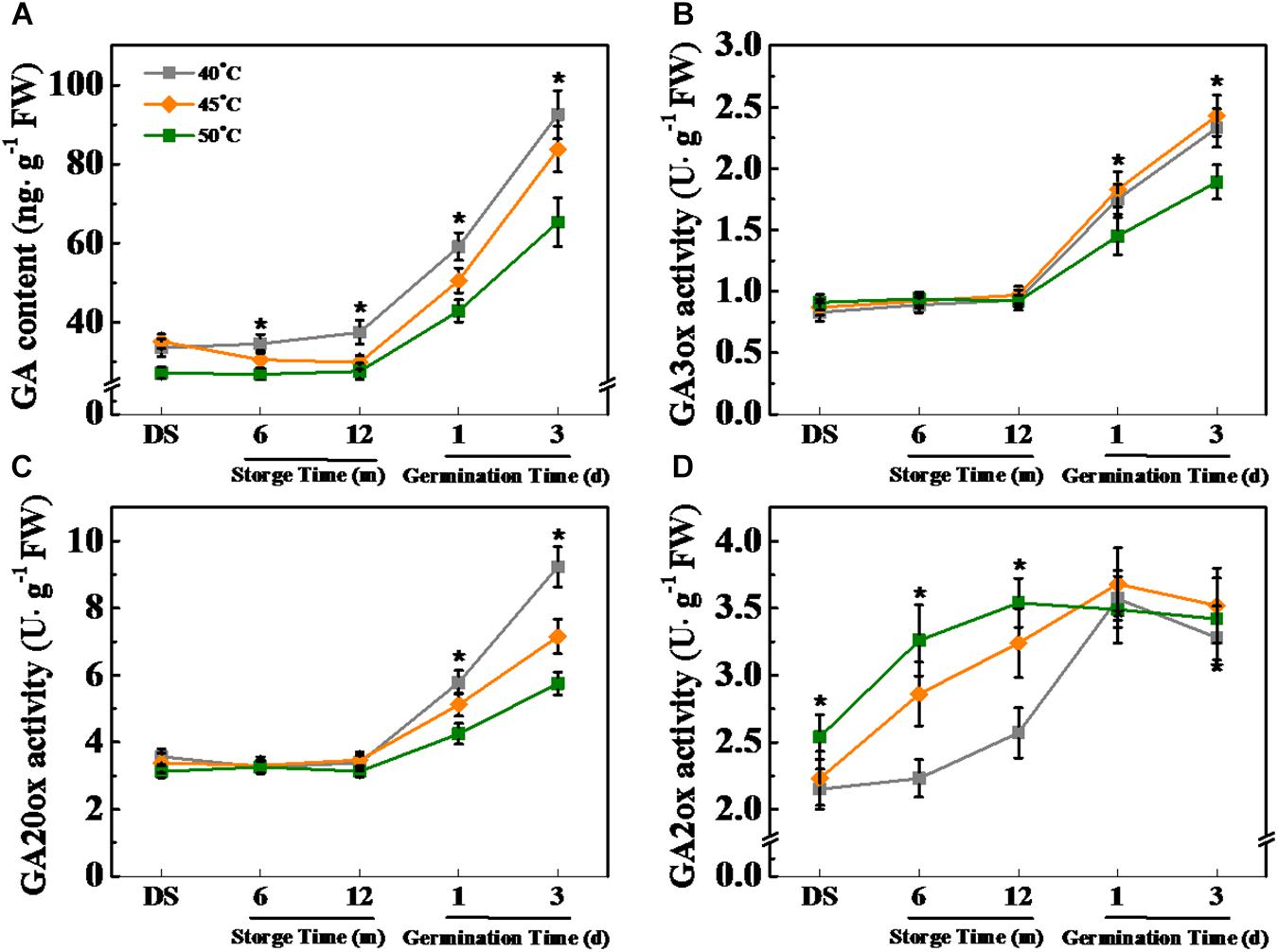
Figure 10. High drying temperature decreased gibberellin (GA) level and affected corresponding enzyme activities in sunflower seeds during storage and germination. (A) GA level; (B) GA3-oxidase (GA3ox) activity; (C) GA20-oxidase (GA20ox) activity; and (D) GA2-oxidase (GA2ox) activity. Sunflower seeds were dried to a target moisture content of 9% at temperatures of 40, 45, and 50°C. The dried sunflower seeds were subjected to 1, 6, or 12 months of storage before analysis. Sunflower seeds stored for 12 months were used for germination test. Percentages represent the means from four experiments ± SE. The asterisk (*) indicates significant differences (p < 0.01, LSD) across treatments. The analysis of GA and enzyme activity was performed with four biological replicates.
Gibberellin level was significantly lowered by HDT (45 and 50°C) during sunflower seed storage and imbibition stages. Differently, the accumulation of GA in 40°C-dried sunflower seeds was significantly higher than that in sunflower seeds dried at 45and 50°C on days 1 and 3 of imbibitions (Figure 10A). GA3ox and GA20ox activities remained unaffected by drying temperature during sunflower seed storage and were down-regulated by HDT during early germination (Figures 10B,C). GA2ox activity was remarkably elevated with the increase of drying temperature during seed storage but remained unchanged on days 1 and 3 of imbibition (Figure 10D).
Drying Temperature Affected the Transcription of ABA and GA Metabolism-Related Genes in Sunflower Seeds During Storage and Germination
The qPCR analysis revealed that the expressions of ABA synthesis genes HaNCED1, HaNCED3, and HaAAO were remarkably increased in 50°C-dried sunflower seeds at 0 and 6 months of storage; whereas no significant effect of drying temperature on those genes expression was detected during sunflower seed early germination. The transcription levels of ABA catabolic genes HaABA8ox1 and HaABA8ox4 was significantly decreased with the increase of drying temperature on days 1 and 3 of imbibition but remained unchanged during storage. On days 1 and 3 of imbibition, the transcription levels of GA synthesis genes HaGA3ox and HaGA20ox2 in 40°C-dried sunflower seeds was significantly elevated compared with those observed in 45- and 50°C-dried seeds. Moreover, the expression of the GA catabolic gene HaGA2ox2 in sunflower seeds was significantly increased with the increase of drying temperature at 0 month of storage (Figure 11). The above findings revealed that drying temperature affected ABA and GA metabolism via the regulation of the critical enzyme activities and gene transcription levels associated with the corresponding pathways.
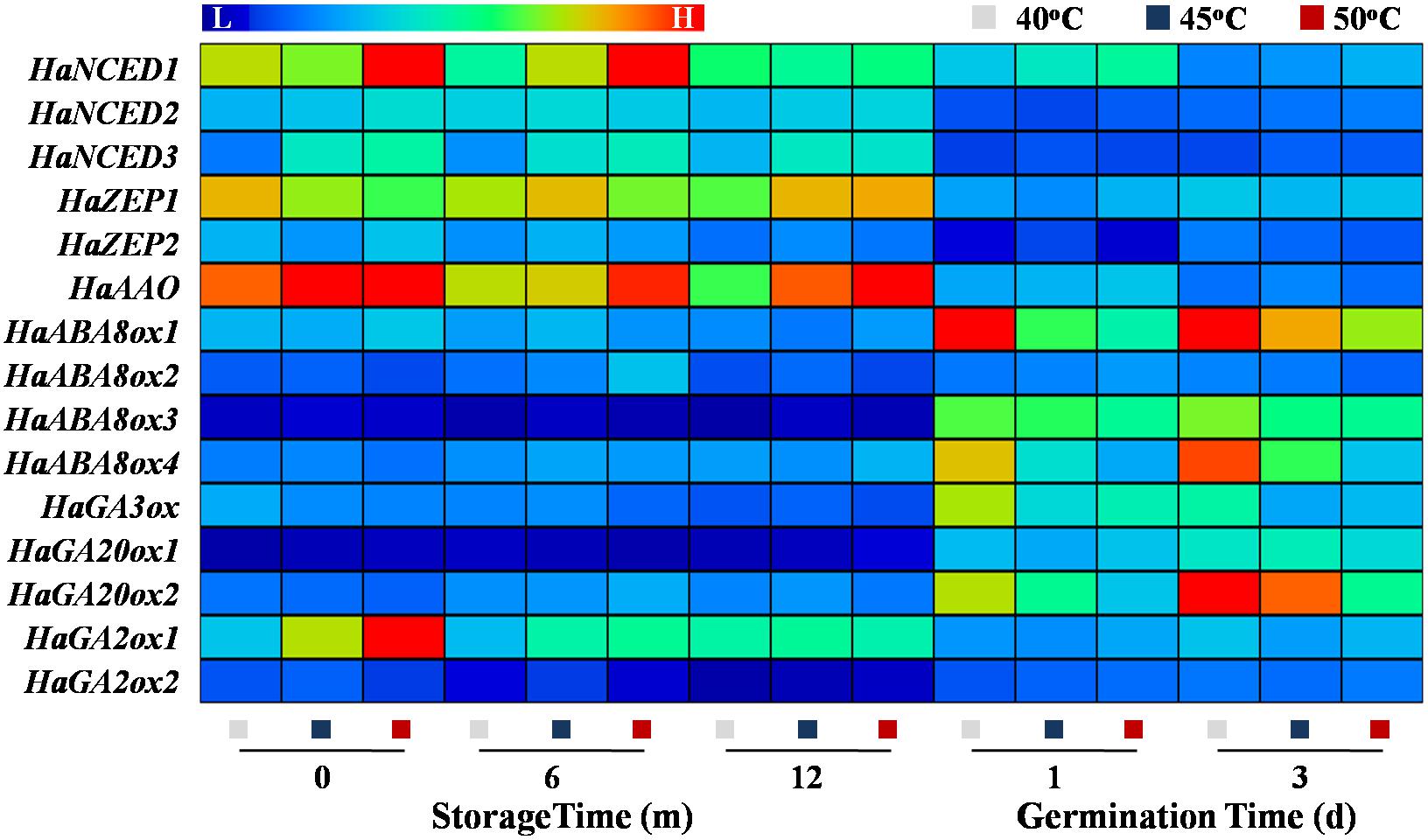
Figure 11. Effect of drying temperature on the transcription levels of abscisic acid (ABA) and gibberellin (GA) metabolism-related genes in sunflower seeds during storage and germination. Sunflower seeds were dried to a target moisture content of 9% at temperatures of 40, 45, and 50°C. The dried sunflower seeds were subjected to 1, 6, or 12 months of storage before analysis. Sunflower seeds stored for 12 months were used for germination test. NCED: 9-cis-epoxycarotenoid dioxygenase; ZEP: zeaxanthin epoxidase; AAO: abscisic aldehyde oxidase; ABA8ox: abscisic acid-8′-hydroxylase; GA3ox: GA3-oxidase; GA20ox: GA20-oxidase; GA2ox: GA2-oxidase. The Illustrator software was used for creating the heat map. Gene expression levels from low (L) to high (H) indicated the lowest and highest levels in the entire database. The gene expression analysis was performed with four biological replicates, and each was made in three technical replicates.
Discussion
Seeds represent the foundation of agriculture, and high seed vigor is critical for crop growth and productivity (Ellis, 2011). Mechanical seed drying is among the key steps for controlling seed quality in seed production (Li et al., 2017). The present study found that HDT increased the damage rate of dried sunflower seeds and aggravated the seed deterioration during storage process, which is accompanied by the regulation of fatty acid oxidation and hydrolysis metabolism, toxic substance accumulation, and ABA/GA balance.
Drying Temperature Exerted an Effect on Drying Performance and Sunflower Seed Characteristics
The drying rate and seed temperature are closely involved in the drying effect on crop seed vigor (Huang et al., 2020a). HDT leads to an accelerated drying rate, thereby reducing the drying time. In the present study, seed temperature and drying rate showed significant increases with the increase of drying temperature, indicating a significant positive correlation between drying temperature and seed temperature and drying rate. These findings were consistent with those reported by Hasan et al. (2014), suggesting that HDT possibly elevated drying rate by increasing the internal diffusion and transfer rates of seed water. Furthermore, drying temperature exerted a consistent effect on the drying rate and seed temperature in other crops including wheat (Ueno, 2003), table olives (Ongen et al., 2005), green chili (Hossain and Bala, 2002), strawberry (Doymaz, 2008), and tomatoes (Doymaz, 2007). Besides, HDT lead to a significant increased of damage rate and relative electrical in sunflower seeds, suggesting that HDT made a mechanical damage to the peel and seed coat of sunflower. Consistently, Igathinathane et al. (2008) reported that high-temperature drying can cause seed damage such as stress cracks and mechanical damage.
HDT Accelerated Sunflower Seed Deterioration During Storage
The effect of mechanical drying on seed vigor has been reported in crops, such as rice (Huang et al., 2020a,b), corn (Jittanit et al., 2010), soybean (Souza et al., 2015), and wheat (Ueno, 2003). While the studies mentioned above primarily focused on the seed vigor after drying but did not monitor the dynamic process of seed vigor deterioration during storage. In the present study, it was observed that HDT of 50 and 55°C significantly reduced the sunflower seed germination rate after drying, whereas drying temperatures of 35, 40, and 45°C did not lead to any significant difference in germination rate after drying, with all seeds achieving a rate of 90%. Remarkably, seed germination rate after drying at 45°C was significantly decreased following natural aging and artificial aging process, which remained high after drying at 35 and 40°C. Therefore, it was proposed that drying temperature exerted an important effect on sunflower seed vigor. For sunflower seeds with an IMC of 30%, drying temperature higher than 40°C accelerated sunflower seed deterioration during the natural storage and artificial aging process. However, we previously reported that the upper limits of drying temperature for rice seeds increased with decreasing seed IMC (Huang et al., 2020b). It was worth noting that seed harvested with lower IMC will show less impact of HTD (Hasan et al., 2014; Souza et al., 2015).
HDT Induced ROS and MDA Over-Accumulation in Aged Sunflower Seeds
Seed storage leads to seed deterioration, which is specifically manifested as a decrease in seed vigor. Oilseeds are rich in lipids, and lipid oxidation may be an important cause of seed degradation during storage (Kibinza et al., 2011). PUFAs, which are abundant in oilseeds, show particular susceptibility to oxidation. LOXs and dioxygenase (DOX) are the vital enzymes oxidizing PUFA (Feussner and Wasternack, 2002). The role of LOX-catalyzed lipid oxidation during seed aging has been reported in rice, which showed that the loss of LOX activity resulted in enhanced seed storage stability and reduced ROS and MDA accumulation (Gayen et al., 2014; Huang et al., 2014; Xu et al., 2015). Moreover, Devaiah et al. (2007) reported that the level of MDA, the PUFA oxidation end-product, was negatively correlated with seed vigor during natural and artificial seed aging processes in Arabidopsis. Excessive ROS level aggravated lipid peroxidation via LOX, thereby inducing cell membrane damage and accelerating seed deterioration (Sharma et al., 2012; Ahmed et al., 2016). Consistently, our study revealed that HDT significantly enhanced H2O2, O2–, and MDA levels in sunflower seeds during storage and early germination. Moreover, LOX and DOS activities and HaLOX2, HaLOX3, and HaDOX2 transcription levels in sunflower seeds during storage was remarkably enhanced with the increase of drying temperature. It was proposed that HDT remarkably aggravated lipid oxidation, leading to ROS and MDA over-accumulation in sunflower seeds during storage. Changes in membrane lipids due to peroxidation may be involved in the increase in membrane permeability and cellular leakage that is associated with seed aging (Ma et al., 2015). HDT might increased the microfractures of sunflower pericarp and seed coat, which interrupting the impermeability to the oxygen favoring the formation of peroxide radicals in the endosperm of the seed.
HDT Inhibited Triacylglycerol Hydrolysis to Sugars in Stored Sunflower Seeds
Soluble sugars, particularly fructose and sucrose, are the main energy sources for plant seeds during early germination (Quettier et al., 2008). In oilseeds, such as sunflower and soybean, triacylglycerol catabolism plays a vital role in providing energy for seed germination and seedling emergence. Triacylglycerol is catalyzed by LIPG to form fatty acids and glycerin; thereafter, fatty acids and glycerin are converted to sucrose via glycolysis, tricarboxylic acid cycle, or glyoxylate cycle, thereby providing nutrients for seed germination (Kaymak, 2014; Basnet et al., 2016). We confirmed that HDT significantly suppressed LIPG, ACX, and INV activities along with their associated gene expression in 12-month-stored sunflower seeds during the early germination stage. Consistently, total fatty acid, unsaturated fatty acid, soluble sugar, and ATP levels in HDT-dried sunflower seeds showed a significant decrease. The above results suggested that HDT suppressed the conversion of triacylglycerol and fatty acid into sucrose and decreased the sunflower seed vigor.
HDT Affected the ABA/GA Balance in Stored Sunflower Seeds
The GA and ABA metabolism is closely associated with seed aging and germination (An and Lin, 2011; Zhang et al., 2011; Arc et al., 2013; Boccaccini et al., 2016). During storage, seeds with a low seed vigor showed high ABA level but low GA level (Lin et al., 2017). Moreover, the decrease in ABA level and increase in GA level were crucial for normal seed germination during the early imbibition process (Jia et al., 2012; Liu et al., 2016). However, whether the drying effect on sunflower seed vigor during storage and germination is associated with the ABA and GA metabolism has not yet been studied. In this study, HDT significantly increased the ABA level in sunflower seeds during storage and early germination. The transcriptions of HaNCED1, HaNCED3, and HaAAO increased, and the transcriptions of HaABA8ox1 and HaABA8ox4 decreased, thereby increasing NCED activity and lowering ABA8ox activity in sunflower seeds after HDT treatment. This result was consistent with the drying effect on ABA level in aged sunflower seeds. Moreover, HDT significantly increased the GA2ox activity in sunflower seeds during storage and decreased GA3ox and GA20ox activities during early germination, thereby suppressing GA accumulation in sunflower seeds. Consistently, Lin et al. (2017) reported that natural aging remarkably suppressed rice seed germination by increasing GA2ox activity and OsGA2ox5 expression. It was proposed that GA2ox might play crucial role in the regulation of GA on seed deterioration during seed storage. The above findings suggested that HDT exerts an effect on the ABA and GA metabolism via changes in enzyme activities and transcription levels during seed storage and germination.
Conclusion
Cumulatively, the present study revealed that drying temperature exerted a substantial effect on sunflower seed vigor deterioration. A drying temperature of 40°C was evidently safe for sunflower seeds with an IMC of 30%, whereas HDT of 45, 50, and 55°C remarkably reduced sunflower seed vigor during storage and AAT process. Furthermore, HDT made a mechanical damage to the peel and seed coat of sunflower, and this may explain the significant aggravated lipid oxidation and ROS over-accumulation in sunflower seeds during storage process. During early germination, HDT remarkably suppressed triglyceride and fatty acid hydrolysis in sunflower seeds after storage. Moreover, HDT increased the ABA level and decreased the GA level by regulating enzyme activities and gene expressions in the corresponding pathways (Figure 12).
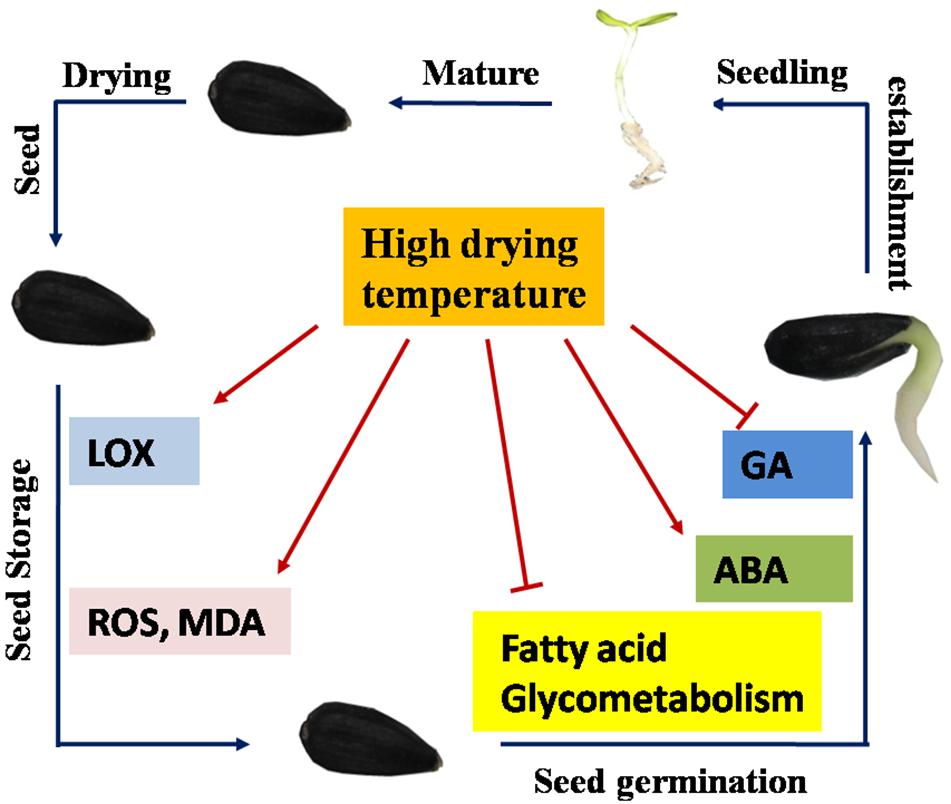
Figure 12. Proposed scheme for the role of drying temperature in sunflower seed vigor. Seed drying reduced the moisture of newly harvested sunflower seeds to a safe level (9%), and the dried seeds entered the storage stage. The low drying temperatures of 35 and 40°C suppressed seed deterioration during storage. However, higher drying temperatures enhanced LOX and DOX activities during seed storage and induced ROS and MDA over-accumulation. During early germination, sunflower seeds dried at a high temperature exhibited hindered conversion from triacylglycerol to fatty acid and glycerol and subsequently to soluble sugars. Moreover, a high drying temperature elevated abscisic acid (ABA) levels but reduced gibberellin (GA) levels in sunflower seeds via modulation of metabolism-related gene expression and enzyme activities. In conclusion, this model suggested that high drying temperature inhibited the sunflower seed vigor via modulation of the fatty acid oxidation and hydrolysis metabolism, toxic substance accumulation, and ABA/GA balance.
Data Availability Statement
The original contributions presented in the study are included in the article/Supplementary Material, further inquiries can be directed to the corresponding author/s.
Author Contributions
YH designed and performed most of the experiments. ML and HW performed the experiments of qRT-PCR analyses. TZ and PW contributed to the enzymes and HPLC analysis. DC reviewed and edited the whole manuscript. All authors read and approved the final version of the manuscript.
Funding
This research was supported by the Key Research and Development Program of Zhejiang Province (No. 2019C02004).
Conflict of Interest
ML, HW, TZ, and PW were employed by the company Huzhou Keao Seed Co., Ltd.
The remaining authors declare that the research was conducted in the absence of any commercial or financial relationships that could be construed as a potential conflict of interest.
Acknowledgments
We thank Shuyu Cai for the fruitful conversation and revising the manuscript.
Supplementary Material
The Supplementary Material for this article can be found online at: https://www.frontiersin.org/articles/10.3389/fpls.2021.628251/full#supplementary-material
References
Ahmed, Z., Yang, H., and Fu, Y. B. (2016). The associative changes in scutellum nuclear content and morphology with viability loss of naturally aged and accelerated aging wheat (Triticum aestivum) seeds. Front. Plant Sci. 7:1474. doi: 10.3389/fpls.2016.01474
Akpinar, E. K. (2005). Determination of suitable thin layer drying curve model for some vegetables and fruits. J. Food Eng. 73, 75–84. doi: 10.1016/j.jfoodeng.2005.01.007
An, Y. Q., and Lin, L. (2011). Transcriptional regulatory programs underlying barley germination and regulatory functions of gibberellin and abscisic acid. BMC Plant Biol. 11:105. doi: 10.1186/1471-2229-11-105
Andreou, A., and Feussner, I. (2009). Lipoxygenases-structure and reaction mechanism. Phytochemistry 70, 1504–1510. doi: 10.1016/j.phytochem.2009.05.008
Arc, E., Sechet, J., Corbineau, F., Rajjou, L., and Marion-Poll, A. (2013). ABA crosstalk with ethylene and nitric oxide in seed dormancy and germination. Front. Plant Sci. 4:63. doi: 10.3389/fpls.2013.00063
Bailly, C., Audigier, C., Ladonne, F., Wagner, M. H., Coste, F., Corbineau, F., et al. (2010). Changes in oligosaccharide content and antioxidant enzyme activities in developing bean seeds as related to acquisition of drying tolerance and seed quality. J. Exp. Bot. 52, 701–708. doi: 10.1093/jexbot/52.357.701
Basnet, R. K., Carpio, D. P. D., Xiao, D., Bucher, J., Jin, M., Boyle, K., et al. (2016). A systems genetics approach identifies gene regulatory networks associated with fatty acid composition in Brassica rapa seed. Plant Physiol. 170, 568–585. doi: 10.1104/pp.15.00853
Bie, W. B., Srzednick, G., and Driscoll, R. H. (2007). Study of temperature and moisture distribution in paddy in a triangular spouted bed dryer. Dry Technol. 25, 177–183. doi: 10.1080/07373930601161062
Boccaccini, A., Lorrai, R., Ruta, V., Frey, A., Mercey-Boutet, S., Marion-Poll, A., et al. (2016). The DAG1 transcription factor negatively regulates the seed-to-seedling transition in Arabidopsis acting on ABA and GA levels. BMC Plant Biol. 16:198. doi: 10.1186/s12870-016-0890-5
Buti, M., Giordani, T., Cattonaro, F., Cossu, R. M., Pistelli, L., Vukich, M., et al. (2011). Temporal dynamics in the evolution of the sunflower genome as revealed by sequencing and annotation of three large genomic regions. Theor. Appl. Genet. 123, 779–791. doi: 10.1007/s00122-011-1626-4
Cai, Y., Shao, L., Li, X., Liu, G., and Chen, S. (2016). Gibberellin stimulates regrowth after defoliation of sheepgrass (Leymus chinensis) by regulating expression of fructan-related genes. J. Plant Res. 129, 935–944. doi: 10.1007/s10265-016-0832-1
Chen, X. J., Qi, K. C., Pu, H. M., Zhang, J. F., Chen, S., Gao, J. Q., et al. (2010). Effects of storage period and temperature on seed fatty acid and vigor of Brassica napus L. Chin. J. Oil Crop Sci. 32, 491–494.
Dang, X. J., Thi, T. G. T., Dong, G. S., Wang, H., Edzesi, W. M., and Hong, D. L. (2014). Genetic diversity and association mapping of seed vigor in rice (Oryza sativa. L.). Planta 239, 1309–1319. doi: 10.1007/s00425-014-2060-z
Devaiah, S. P., Pan, X., Hong, Y., Roth, M., Welti, R., and Wang, X. (2007). Enhancing seed quality and viability by suppressing phospholipase D in Arabidopsis. Plant J. 50, 950–957. doi: 10.1111/j.1365-313x.2007.03103.x
Doymaz, I. (2007). Air-drying characteristics of tomatoes. J. Food Eng. 78, 1291–1297. doi: 10.1016/j.jfoodeng.2005.12.047
Doymaz, I. (2008). Convective drying kinetics of strawberry. Chem. Eng. Process. 47, 914–919. doi: 10.1016/j.cep.2007.02.003
Eastmond, P. J. (2007). MONODEHYROASCORBATE REDUCTASE4 is required for seed storage oil hydrolysis and postgerminative growth in Arabidopsis. Plant Cell. 19, 1376–1387. doi: 10.1105/tpc.106.043992
Ellis, R. H. (2011). Rice seed quality development and temperature during late development and maturation. Seed Sci. Res. 21, 95–101. doi: 10.1017/s0960258510000425
Faligowska, A., Bartosspychala, M., and Panasiewicz, K. (2012). The effect of storage period on sowing value and vigor of narrow-leaved lupin dressed seed. Prog. Plant Prot. 52, 1151–1155.
Feussner, I., and Wasternack, C. (2002). The lipoxygenase pathway. Annu. Rev. Plant Biol. 53, 275–297.
Gao, C. H., Hu, J., Zhang, S., Zheng, Y. Y., and Knapp, A. (2009). Association of polyamines in governing the chilling sensitivity of maize genotypes. Plant Growth Regul. 57, 31–38. doi: 10.1007/s10725-008-9315-2
Gayen, D., Ali, N., Ganguly, M., Paul, S., Datta, K., and Datta, S. K. (2014). RNAi mediated silencing of lipoxygenase gene to maintain rice grain quality and viability during storage. Plant Cell Tiss. Org. 118, 229–243. doi: 10.1007/s11240-014-0476-6
Goepfert, S., and Poirier, Y. (2007). β-Oxidation in fatty acid degradation and beyond. Curr. Opin. Plant Biol. 10, 245–251. doi: 10.1016/j.pbi.2007.04.007
Hasan, A. A. M., Bala, B. K., and Rowshon, M. K. (2014). Thin layer drying of hybrid rice seed. Eng. Agric. Environ. Food. 7, 169–175.
Hedden, P., and Thomas, S. G. (2012). Gibberellin biosynthesis and its regulation. Biochem. J. 444, 11–25. doi: 10.1042/bj20120245
Hossain, M. A., and Bala, B. K. (2002). Thin layer drying characteristics for green chilli. Dry Technol. 20, 489–505. doi: 10.1081/drt-120002553
Huang, J., Cai, M., Long, Q., Liu, L., Lin, Q., Jiang, L., et al. (2014). OsLOX2, a rice type I lipoxygenase, confers opposite effects on seed germination and longevity. Transgenic Res. 23, 643–655. doi: 10.1007/s11248-014-9803-2
Huang, Y. T., Lin, C., He, F., Li, Z., Guan, Y. J., Hu, Q. J., et al. (2017). Exogenous spermidine improves seed germination of sweet corn via involvement in phytohormone interactions, H2O2 and relevant gene expression. BMC Plant Biol. 17, 1–16. doi: 10.1186/s12870-016-0951-9
Huang, Y. T., Wu, W., Zhao, T. Y., Lu, M., Wu, H. P., and Cao, D. D. (2020a). Drying temperature regulates vigor of high moisture rice seeds via involvement in phytohormone, ROS, and relevant gene expression. J. Sci. Food Agric. 2:614. doi: 10.1002/jsfa.10837
Huang, Y. T., Wu, W., Zou, W. X., Wu, H. P., and Cao, D. D. (2020b). Drying temperature affects rice seed vigor via gibberellin, abscisic acid, and antioxidant enzyme metabolism. J. Zhejiang Univ. SCI B. 21, 796–810. doi: 10.1631/jzus.b2000297
Igathinathane, C., Chattopadhyay, P. K., and Pordesimo, L. O. (2008). Moisture diffusion modeling of parboiled paddy accelerated tempering process with extended application to multi-pass drying simulation. J. Food Eng. 88, 239–253. doi: 10.1016/j.jfoodeng.2008.02.014
Jia, L., Wu, Q., Ye, N., Liu, R., Shi, L., Xu, W., et al. (2012). Proanthocyanidins inhibit seed germination by maintaining a high level of abscisic acid in Arabidopsis thaliana. J. Integr. Plant Biol. 54, 663–673. doi: 10.1111/j.1744-7909.2012.01142.x
Jiang, M., and Zhang, J. (2003). Effect of abscisic acid on active oxygen species, antioxidative defence system and oxidative damage in leaves of maize seedlings. Plant Cell Physiol. 42, 1265–1273. doi: 10.1093/pcp/pce162
Jittanit, W., Srzednicki, G., and Driscoll, R. (2010). Corn, rice, and wheat seed drying by two-stage concept. Dry Technol. 28, 807–815. doi: 10.1080/07373937.2010.485081
Kaymak, H. (2014). Potential effect of seed fatty acid profile of pepper (Capsicum annuum L.) cultivars on germination at various temperatures. Zemdirbyste 101, 321–326. doi: 10.13080/z-a.2014.101.041
Kibinza, S., Bazin, J., Bailly, C., Farrant, J. M., Corbineau, F., and El-Maarouf-Bouteau, H. (2011). Catalase is a key enzyme in seed recovery from aging during priming. Plant Sci. 181, 309–315. doi: 10.1016/j.plantsci.2011.06.003
Li, M., Wu, Y. Y., and Guan, Z. Q. (2017). Effect of physical osmosis methods on quality of tilapia fillets processed by heat pump drying. Pol. J. Food Nutr. Sci. 67, 145–150. doi: 10.1515/pjfns-2016-0016
Lima, W. A. A., Borem, A., Dias, D., Moreira, M. A., and Dias, L. A. S. (2010). Lipoxygenase and physiological quality of soybean seeds during storage. Seed Sci. Technol. 38, 767–771. doi: 10.15258/sst.2010.38.3.23
Lin, C., Shen, H. Q., Guan, Y. J., An, J. Y., Hu, W. M., and Hu, J. (2017). Changes of physiological, biochemistry and gene expression related to ABA and GA3 in hybrid rice seeds stored at different moisture contents and packing methods. Plant Physiol. J. 53, 1077–1086.
Liu, H., Jiang, Y. M., Luo, Y. B., and Jiang, W. B. (2006). A simple and rapid determination of ATP. ADP and AMP concentrations in pericarp tissue of litchi fruit by high performance liquid chromatography. Food Technol. Biotech. 4, 531–534.
Liu, X., Hu, P., Huang, M., Tang, Y., Li, Y., Li, L., et al. (2016). The NF-YC-RGL2 module integrates GA and ABA signaling to regulate seed germination in Arabidopsis. Nat. Commun. 7:12768.
Ma, L., Zhu, F. G., Li, Z. W., Zhang, J. F., Li, X., Dong, J. L., et al. (2015). TALEN-based mutagenesis of lipoxygenase LOX3 enhances the storage tolerance of rice (Oryza sativa) seeds. PLoS One. 10:e0143877. doi: 10.1371/journal.pone.0143877
Missaoui, A. M., and Hill, N. S. (2015). Use of accelerated aging as a surrogate phenotyping approach to improve endophyte survival during storage of tall fescue seed. Field Crop Res. 183, 43–49. doi: 10.1016/j.fcr.2015.07.016
Ongen, G., Sargin, S., Tetik, D., and Kose, T. (2005). Hot air drying of green table olives. Food Technol. Biotech. 43, 181–187.
Quettier, A. L., and Eastmond, P. J. (2009). Storage oil hydrolysis during early seedling growth. Plant Physiol. Biochem. 47, 485–490. doi: 10.1016/j.plaphy.2008.12.005
Quettier, A. L., Shaw, E., and Eastmond, P. J. (2008). SUGAR-DEPENDENT6 encodes a mitochondrial flavin adenine dinucleotide-dependent glycerol-3-P dehydrogenase, which is required for glycerol catabolism and postgerminative seedling growth in Arabidopsis. Plant Physiol. 148, 519–528. doi: 10.1104/pp.108.123703
Rajjou, L., Duval, M., Gallardo, K., Catusse, J., Bally, J., Job, C., et al. (2012). Seed germination and vigor. Ann. Rev. Plant Biol. 63, 507–533.
Sharma, P., Jha, A. B., Dubey, R. S., and Pessarakli, M. (2012). Reactive oxygen species, oxidative damage, and antioxidative defense mechanism in plants under stressful conditions. J. Bot. 2012:e217037.
Shi, H., Wang, B., Yang, P., Li, Y., and Miao, F. (2016). Differences in sugar accumulation and mobilization between sequential and non-sequential senescence wheat cultivars under natural and drought conditions. PLoS One. 11:e0166155. doi: 10.1371/journal.pone.0166155
Shu, K., Liu, X. D., Xie, Q., and He, Z. H. (2016). Two faces of one seed: hormonal regulation of dormancy and germination. Mol. Plant 9, 34–45. doi: 10.1016/j.molp.2015.08.010
Shu, K., Zhou, W., and Yang, W. (2018). APETALA 2-domain-containing transcription factors: focusing on abscisic acid and gibberellins antagonism. New Phytol. 217, 977–983. doi: 10.1111/nph.14880
Souza, G. F. M. V., Miranda, R. F., and Barrozo, M. A. A. (2015). Soybean (Glycine max L. Merrill) seed drying in fixed bed: process heterogeneity and seed quality. Dry Technol. 33, 1779–1787. doi: 10.1080/07373937.2015.1039542
Stephany, M., Bader-Mittermaier, S., Schweiggert-Weisz, U., and Carle, R. (2015). Lipoxygenase activity in different species of sweet lupin (Lupinus L.) seeds and flakes. Food Chem. 174, 400–406. doi: 10.1016/j.foodchem.2014.11.029
Terp, N., Göbel, C., Brandt, A., and Feussner, I. (2006). Lipoxygenases during Brassica napus seed germination. Phytochemistry 67, 2030–2040. doi: 10.1016/j.phytochem.2006.06.023
Thakur, A. K., and Gupta, A. K. (2006). Two stage drying of high moisture paddy with intervening rest period. Energ. Convers. Manage 47, 3069–3083. doi: 10.1016/j.enconman.2006.03.008
Ueno, K. (2003). Effects of temperature during drying of immature wheat seed on germination. Seed Sci. Technol. 31, 587–595. doi: 10.15258/sst.2003.31.3.08
Vishwakarma, K., Upadhyay, R. G., Pandey, M., and Sharma, S. (2017). Abscisic acid signaling and abiotic stress tolerance in plants: a review on current knowledge and future prospects. Front. Plant Sci. 8:161. doi: 10.3389/fpls.2017.00161
Huang, A. X., She, X. P., Cao, B. H., and Ren, Y. (2011). Distribution of hydrogen peroxide during adventitious roots initiation and development in mung bean hypocotyls cuttings. Plant Growth Regul. 64, 109–118. doi: 10.1007/s10725-010-9545-y
Xiong, Y. F., Ren, Y., Li, W., Wu, F. S., Yang, W. J., Huang, X. L., et al. (2019). NF-YC12 is a key multi-functional regulator of accumulation of seed storage substances in rice. J. Exp. Bot. 70:15.
Xu, H., Wei, Y., Zhu, Y., Lian, L., Xie, H., Cai, Q., et al. (2015). Antisense suppression of LOX3 gene expression in rice endosperm enhances seed longevity. Plant Biotechnol. J. 13, 526–539. doi: 10.1111/pbi.12277
Yang, C., Iqbal, N., Hu, B., Zhang, Q., Wu, H., Liu, X., et al. (2017). Targeted metabolomics analysis of fatty acids in soybean seeds using GC-MS to reveal the metabolic manipulation of shading in the intercropping system. Anal. Methods UK. 9, 2144–2152. doi: 10.1039/c7ay00011a
Zhang, Y., and Zhang, W. L. (2018). Analysis of changes and trends in world sunflower production and trade structure. World Agric. 9, 119–126.
Zhang, Z. L., Ogawa, M., Fleet, C. M., Zentella, R., Hu, J. H., Heo, J., et al. (2011). SCARECROW-LIKE 3 promotes gibberellin signaling by antagonizing master growth repressor DELLA in Arabidopsis. Proc. Natl. Acad. Sci. U. S. A. 108, 2160–2165. doi: 10.1073/pnas.1012232108
Zhao, S., Huang, W., Jiang, H., Sun, J. Y., Yin, L. Y., and Li, W. (2017). Hydrocharis dubia seeds maintain high seed vigor in ambient wet storage condition through scavenging hydrogen peroxide by antioxidant systems. Aquat Bot. 143, 18–24. doi: 10.1016/j.aquabot.2017.08.001
Zhen, H. Y., Liu, H. H., Jia, H. B., and Ren, P. (2020). Sunflower cultivation in northern China. Beijing: China Agriculture Press.
Zhou, W. G., Chen, F., Zhao, S. H., Yang, C. Q., Meng, Y. J., Shuai, H. W., et al. (2019). DA-6 promotes germination and seedling emergence from aged soybean seeds by mediating fatty acid metabolism and glycometabolism. J. Exp. Bot. 70, 101–114. doi: 10.1093/jxb/ery247
Zhou, X. X., Liu, L., Fu, P. C., Lyu, F., Zhang, J. Y., Gu, S. Q., et al. (2018). Effects of infrared radiation drying and heat pump drying combined with tempering on the quality of long-grain paddy rice. Int. J. Food Sci. Tech. 53, 2448–2456. doi: 10.1111/ijfs.13834
Keywords: drying temperature, fatty acid, phytohormones, ROS, seed germination, seed storage, sunflower
Citation: Huang Y, Lu M, Wu H, Zhao T, Wu P and Cao D (2021) High Drying Temperature Accelerates Sunflower Seed Deterioration by Regulating the Fatty Acid Metabolism, Glycometabolism, and Abscisic Acid/Gibberellin Balance. Front. Plant Sci. 12:628251. doi: 10.3389/fpls.2021.628251
Received: 11 November 2020; Accepted: 03 May 2021;
Published: 28 May 2021.
Edited by:
Luis A. N. Aguirrezabal, National University of Mar del Plata, ArgentinaReviewed by:
Catello Di Martino, University of Molise, ItalyDiego Batlla, University of Buenos Aires, Argentina
Copyright © 2021 Huang, Lu, Wu, Zhao, Wu and Cao. This is an open-access article distributed under the terms of the Creative Commons Attribution License (CC BY). The use, distribution or reproduction in other forums is permitted, provided the original author(s) and the copyright owner(s) are credited and that the original publication in this journal is cited, in accordance with accepted academic practice. No use, distribution or reproduction is permitted which does not comply with these terms.
*Correspondence: Dongdong Cao, amVsbHljYW9AMTYzLmNvbQ==