- 1Rijk Zwaan, De Lier, Netherlands
- 2Institute for Plant Sciences, University of Cologne, Cologne, Germany
- 3Max Planck Institute for Plant Breeding Research, Cologne, Germany
- 4Cluster of Excellence on Plant Sciences, “SMART Plants for Tomorrow’s Needs,” Heinrich Heine University Düsseldorf, Düsseldorf, Germany
Most temperate species require prolonged exposure to winter chilling temperatures to flower in the spring. In the Brassicaceae, the MADS box transcription factor FLOWERING LOCUS C (FLC) is a major regulator of flowering in response to prolonged cold exposure, a process called vernalization. Winter annual Arabidopsis thaliana accessions initiate flowering in the spring due to the stable silencing of FLC by vernalization. The role of FLC has also been explored in perennials within the Brassicaceae family, such as Arabis alpina. The flowering pattern in A. alpina differs from the one in A. thaliana. A. alpina plants initiate flower buds during vernalization but only flower after subsequent exposure to growth-promoting conditions. Here we discuss the role of FLC in annual and perennial Brassicaceae species. We show that, besides its conserved role in flowering, FLC has acquired additional functions that contribute to vegetative and seed traits. PERPETUAL FLOWERING 1 (PEP1), the A. alpina FLC ortholog, contributes to the perennial growth habit. We discuss that PEP1 directly and indirectly, regulates traits such as the duration of the flowering episode, polycarpic growth habit and shoot architecture. We suggest that these additional roles of PEP1 are facilitated by (1) the ability of A. alpina plants to form flower buds during long-term cold exposure, (2) age-related differences between meristems, which enable that not all meristems initiate flowering during cold exposure, and (3) differences between meristems in stable silencing of PEP1 after long-term cold, which ensure that PEP1 expression levels will remain low after vernalization only in meristems that commit to flowering during cold exposure. These features result in spatiotemporal seasonal changes of PEP1 expression during the A. alpina life cycle that contribute to the perennial growth habit. FLC and PEP1 have also been shown to influence the timing of another developmental transition in the plant, seed germination, by influencing seed dormancy and longevity. This suggests that during evolution, FLC and its orthologs adopted both similar and divergent roles to regulate life history traits. Spatiotemporal changes of FLC transcript accumulation drive developmental decisions and contribute to life history evolution.
Plants Have Different Ways of Using Winter Cold to Synchronize Flowering in the Spring
Winter annuals, biennials and perennial species overwinter as seedlings or plants and flower in the spring when favorable environmental conditions return. Annual and biennial species follow a monocarpic life strategy and die after setting seeds once, whereas the majority of perennials are polycarpic and are able to reproduce several times during their life time (Albani and Coupland, 2010; Bratzel and Turck, 2015). Low temperatures during the winter are important to enable synchronous flowering in the spring but generally regulate different stages of the flowering process in monocarpic compared to polycarpic species.
Winter annuals and biennials require prolonged exposure to cold to accelerate or to enable flowering, a process called vernalization. Prolonged cold exposure is effective when it is applied to imbibed seeds or young seedlings (of winter annual species), or to older plants (of winter annual and biennial species) (Lang, 1952; Chouard, 1960). The requirement of cold exposure for flowering is common in plants adapted to temperate climates and ensures that they will not flower before the winter (Chouard, 1960). Vernalization is considered as a preparatory process that has to take place before a plant can initiate flowering in response to increased daylength in the spring (Chouard, 1960). The effectiveness of vernalization is usually estimated by the reduction of days that plants take to flower after returning to growth-promoting conditions (Chouard, 1960). Thus, vernalization aligns with the life cycle of winter annual and biennial species that initiate flowering in the spring (Figure 1A; Chouard, 1960). In A. thaliana, vernalization has also a quantitative effect on flowering and the duration of cold that is required for the acceleration of flowering can vary between accessions (Shindo et al., 2006; Li et al., 2014). Plants can be vernalized at different temperatures ranging from 0 to 16°C (Wollenberg and Amasino, 2012; Duncan et al., 2015). In some habitats, this temperature range can be achieved during summer or autumn, suggesting that plants can be vernalized before the winter (Billings and Mooney, 1968; Duncan et al., 2015; O’Neill et al., 2019). In addition, older plants show a stronger response to vernalization than young seedlings, suggesting that the developmental stage/age of the plant influences its ability to respond to prolonged cold treatment (Chouard, 1960). The effect of cold exposure is also reversible and plants can devernalize when cold exposure is followed by high temperatures (Chouard, 1960; Périlleux et al., 2013). This suggests that the flower-promoting role of vernalization can be lost when plants are exposed to exceptionally high spring temperatures.
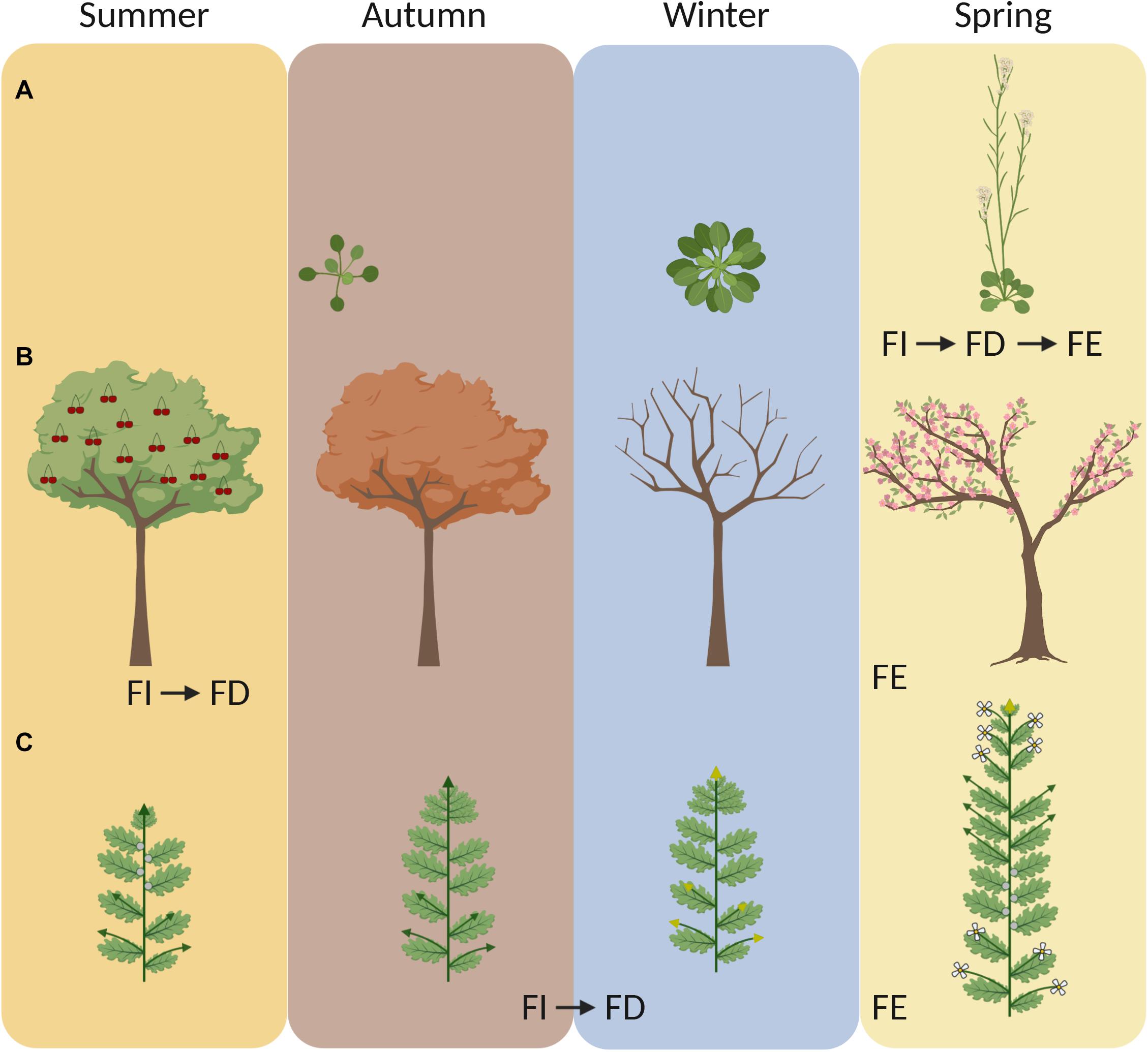
Figure 1. Annuals and perennials initiate floral buds at different times during the year and use winter to synchronize flowering. Cartoons depict the yearly life cycle of (A) a winter annual (e.g., A. thaliana), (B) a woody perennial (e.g., cherry tree) and (C) an herbaceous alpine perennial (e.g., A. alpina). Winter annual A. thaliana accessions induce flowering in the spring. After floral induction (FI), flowering is initiated and floral buds develop (FD) and emerge (FE). In A. thaliana, all steps from floral induction to emergence are very rapid (FI, FD and FE). In perennials, the stages of floral induction (FI) and floral emergence (FE) are temporarily separated. Many woody perennials induce and initiate flowering during summer (FI and FD) but flower buds emerge (FE) in the spring of the next year. In A. alpina, flowering is induced and initiated (FI and FD) during cold exposure in the late autumn, but flowers emerge (FE) after plants return to warm temperatures. In panel (C), green arrows represent vegetative branches and yellow arrows represent flowering branches. Image created with BioRender.com.
Polycarpic perennials do not commit all meristems to flowering and are able to maintain growth from one year to the next by keeping some meristems in a vegetative state (Albani and Coupland, 2010). Maintenance of vegetative growth after flowering is determined by the coordinated action of age-related factors, which determine whether individual meristems are sensitive to flower-inductive stimuli, and seasonal changes in expression patterns of environmentally-regulated flowering time genes (Wang et al., 2009; Bergonzi and Albani, 2011; Koskela et al., 2012; Hyun et al., 2017, 2019; Zhou et al., 2021). Perennials, however, still have to synchronize their annual cycle in order to flower and set fruits during favorable environmental conditions in spring and summer (Falavigna et al., 2019). The environmental conditions that induce flowering in temperate perennials vary greatly between species, although most of them concentrate their flowering season in the spring. For example, grapevine, sweet cherry and peach trees initiate flower buds during the summer (Figure 1B; Engin and Ünal, 2007; Carmona et al., 2008; Vimont et al., 2019). Therefore, spring flowering in these perennials occurs when the flower buds, which were initiated the previous year, grow out. Interestingly, the observed variation in spring flowering between cultivars is not associated with differences in flower bud initiation but rather with the requirement of prolonged cold exposure that is needed for plants to exit the endodormant state that they enter in autumn (Vimont et al., 2019). In general, bud dormancy can be divided into three phases: paradormancy, endodormancy and ecodormancy. Paradormancy (also referred as latency) is the stage during which the growth of a bud is inhibited by surrounding organs (Lang et al., 1987). The other stages of dormancy are environmentally regulated, with endodormancy being induced and ecodormancy being maintained by environmental cues (Lang et al., 1987). Buds enter endodormancy during the autumn and throughout this phase their growth is inhibited by internal signals (Lang et al., 1987). Prolonged exposure to low temperatures in the winter is required for endodormancy release, and once the chilling requirement is met the buds transition into ecodormancy (reviewed in Falavigna et al., 2019). Bud growth during ecodormancy is inhibited by unfavorable environmental conditions and can be reactivated again when plants experience growth-promoting conditions (Lang et al., 1987). Overall, prolonged cold exposure is important for bud dormancy release and synchronized resumption of growth the following spring (referred to as budbreak). Exposure to chilling temperatures for dormancy breaking is considered to be a distinct process from vernalization (Chouard, 1960). Specifically, because low temperatures during dormancy breaking do not induce a phase change that leads to the formation of new kinds of organs but cause the regrowth of already existing organs (Chouard, 1960). However, there are obvious similarities between both processes, which both require long-term cold to synchronize spring flowering (Or, 2009; Atkinson et al., 2013) and share a considerable overlap in their molecular pathways (reviewed in Horvath, 2009).
There is also a third variant by which low temperatures lead to flowering. This variant has been observed in several Brassicaceae species, including winter oil seed rape, A. alpina, Arabidopsis lyrata, pak choi, and Brussels sprouts (Chouard, 1960; Wang et al., 2009; Kemi et al., 2019; O’Neill et al., 2019). In these species, flower buds initiate and develop during cold exposure, suggesting that vernalization is not only a preparatory process for flowering but also directly regulates the initiation and formation of floral buds (Figure 1C; Chouard, 1960; Wang et al., 2009; Kemi et al., 2019; O’Neill et al., 2019). In the perennial A. alpina, flowering is initiated during vernalization under short photoperiods (Figures 1C, 2; Wang et al., 2009; Lazaro et al., 2018). Similarly, field studies in winter oil seed rape demonstrated that inflorescences are initiated during the autumn in response to low temperatures (O’Neill et al., 2019). In both species, the length of the cold exposure is also important for the outgrowth of flower buds after the return to warm temperatures (Lazaro et al., 2018; O’Neill et al., 2019). Flower buds may still enter dormancy during the winter or grow more slowly due to low temperatures. In field studies using oil seed rape plants, dissection of shoot apical meristems showed that inflorescence meristems gradually grow during the winter and thus may not be dormant (O’Neill et al., 2019). Although flower buds in such species develop during autumn, daylength is still important for flowering. As shown in A. lyrata, long photoperiods are important for inflorescence bolting in the spring (Kemi et al., 2019). Ecological studies in alpine species suggest that flower bud development during cold exposure can be an advantageous adaptive trait in harsh environments characterized by prolonged snow coverage and short growing seasons (Diggle, 1997; Meloche and Diggle, 2001). This modification of the role of vernalization on flowering does not seem to be related to the plant life strategy as both annual and perennial species have been reported to form flower buds during cold treatment (Chouard, 1960; Wang et al., 2009; Kemi et al., 2019; O’Neill et al., 2019).
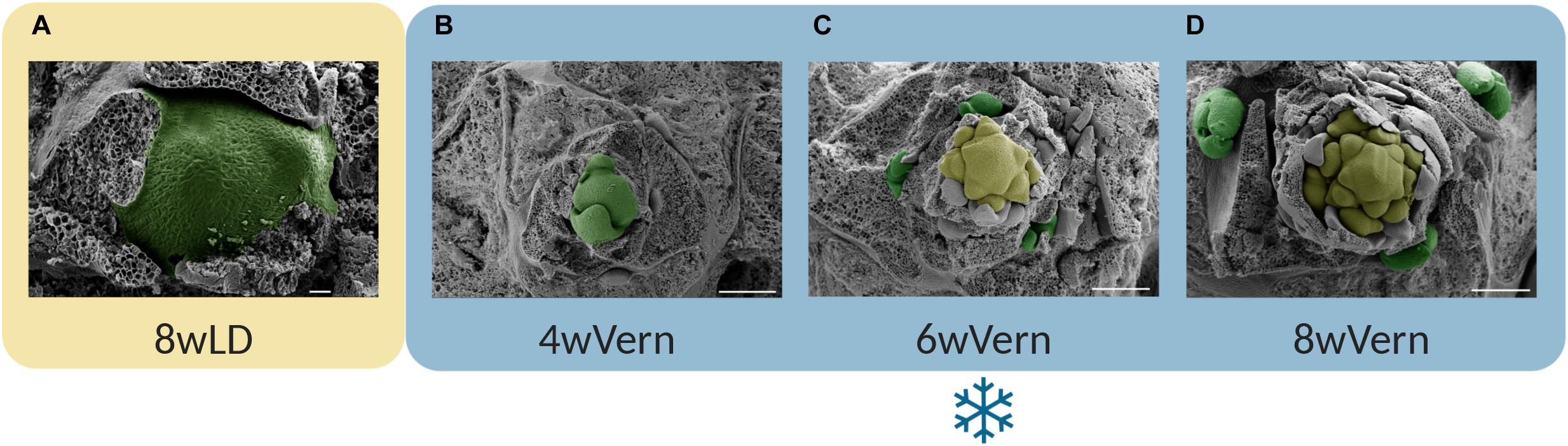
Figure 2. Flower buds in A. alpina are initiated during vernalization. Progression of the shoot apical meristem of A. alpina accession Pajares imaged by scanning electron microscopy (SEM) in (A) plants were grown for 8 weeks in a long day greenhouse, and subsequently transferred to vernalization for (B) 4 weeks (4wVern), (C) 6 weeks (6wVern) or (D) 8 weeks (8wVern). The main shoot apex is vegetative in plants grown in greenhouse conditions or vernalized only for 4 weeks (4wVern) (A,B, highlighted in green). The main shoot apex initiates flowering after 6 weeks in vernalization (C, highlighted in yellow) and inflorescences develop during vernalization (D, highlighted in yellow). In the axils of leaf primordia close to the shoot apical meristem, new vegetative buds are also formed (C,D, highlighted in green). Scale bars correspond to 20 μm (A) or to 200 μm (B–D). Colored boxes represent different growth conditions: the yellow box a long day greenhouse; and the blue box the vernalization at 4°C and short days (8 h light: 16 h dark). SEM pictures were modified from the Ph.D. dissertation of Zhou (2019). Image was composed using BioRender.com.
The Role and Regulation of FLOWERING LOCUS C (FLC) in A. thaliana
Several studies in A. thaliana demonstrated that the major regulator of flowering through the vernalization pathway is the MADS box transcription factor FLOWERING LOCUS C (FLC) (Michaels and Amasino, 1999; Sheldon et al., 2000). In temperate grasses, which also require prolonged cold exposure to flower, and also in biennials such as sugar beet, the regulation of vernalization is mediated by pathways independent of FLC (reviewed in Bouché et al., 2017). FLC is a floral repressor that is highly expressed in A. thaliana accessions that require vernalization to accelerate flowering (Shindo et al., 2006; Li et al., 2014). Prolonged exposure to low temperatures regulates FLC in two ways: (1) by quantitatively repressing FLC transcript accumulation and (2) by ensuring that FLC will remain stably silenced after plants have returned to warm conditions. The major mechanism that drives FLC transcriptional regulation by long-term cold consists of chromatin modifications (reviewed in Berry and Dean, 2015). In A. thaliana accessions, non-coding cis polymorphisms at the FLC locus underlie variation in the length of cold required to achieve stable silencing of FLC (Coustham et al., 2012; Qüesta et al., 2020). FLC is transcriptionally repressed by long non-coding RNAs (lncRNAs), expressed at the locus in response to cold, which play an early role in the epigenetic silencing of FLC (Heo and Sung, 2011; Csorba et al., 2014). A parallel pathway that interprets long-term exposure to low temperatures involves the plant homeodomain (PHD) protein, VERNALIZATION INSENSITIVE 3 (VIN3) (Sung and Amasino, 2004; Wood et al., 2006; De Lucia et al., 2008; Swiezewski et al., 2009). VIN3 expression increases gradually during cold by a mechanism that is mediated by the NAC transcription factor NTM1-LIKE 8 (NTL8) (Sung and Amasino, 2004; Bond et al., 2009; Zhao et al., 2020). NTL8 protein accumulates during cold exposure, due to growth retardation and reduced number of cell divisions that occur at low temperatures, and upregulates VIN3 transcription (Zhao et al., 2020). Subsequently, VIN3 forms a complex with its homologous PHD protein VERNALIZATION5 (VIN5) and the Polycomb Repressive Complex 2 (PRC2) proteins VERNALIZATION2 (VRN2), MSI1, FIE/EED, and the E(z) homologs SWINGER (SWN) and CURLY LEAF (CLF) (reviewed in Berry and Dean, 2015). This PHD-PRC2 complex is recruited to the FLC locus at 3 nucleosomes, covering exon 1 and part of the first intron (which is called the nucleation region), and methylates H3 lysine 27 residues resulting in a gradual cell-autonomous FLC silencing (Angel et al., 2011). The B3-binding transcription factor VP1/ABI3-LIKE 1 (VAL1) is required for the PHD-PRC2 action at FLC (Qüesta et al., 2016; Yuan et al., 2016). After cold exposure, the H3K27me3 mark spreads over the entire FLC locus and ensures maintenance of long-term silencing of FLC (Finnegan and Dennis, 2007; De Lucia et al., 2008). This spreading of H3K27me3 is enabled by the PHD-PRC2 complex and requires the action of the Polycomb protein LIKE HETEROCHROMATIN PROTEIN1 (LHP1) that specifically binds to H3K27me3 marks (reviewed in Costa and Dean, 2019).
Similar to other MADS-domain proteins, FLC regulates its downstream genes by binding to conserved CArG-box motifs in their promoters or introns (Deng et al., 2011; Mateos et al., 2017). FLC target genes act in different pathways throughout development, of which several are implicated in flowering (Deng et al., 2011; Mateos et al., 2017). These include the floral promoter FLOWERING LOCUS T (FT), that regulates flowering through the photoperiod pathway; SUPPRESSOR OF OVEREXPRESSION OF CONSTANS 1 (SOC1), that regulates several genes involved in floral transition at the shoot apex; the SQUAMOSA BINDING PROTEIN LIKE (SPL) family member SPL15, that contributes to reproductive competence and floral transition; and SEPALLATA3 (SEP3), that regulates flower development (reviewed in Madrid et al., 2020). Stable FLC silencing after vernalization is, therefore, a key feature to explain the flowering pattern of winter annual A. thaliana accessions. This is because the reduced mRNA levels of FLC allow the activation of floral promoting pathways that ensure the initiation of flowering in the spring. For the life cycle of an annual plant it is important that FLC expression is restored in the next generation. In A. thaliana this occurs during embryo development and by the end of embryogenesis all H3K27me3 marks have been removed from the FLC locus so that the gene is fully reactivated (Tao et al., 2019; Luo et al., 2020).
Arabidopsis thaliana plants that carry weak FLC alleles can initiate flowering in the autumn due to low starting FLC mRNA levels (Hepworth et al., 2020). These genotypes bolt precociously and show higher mortality in the field, which emphasizes the importance of FLC in ensuring spring flowering in A. thaliana (Hepworth et al., 2020). In oil seed rape plants, which initiate flowering during cold exposure, the starting levels of BnaFLC also negatively correlate with the timing of flower bud initiation in the autumn (O’Neill et al., 2019). Cultivars with lower BnaFLC mRNA levels initiate flowering earlier compared to cultivars with higher starting BnaFLC mRNA levels (O’Neill et al., 2019). Interestingly, although oil seed rape plants do not bolt precociously, there is a penalty on plant yield if flowering is initiated earlier in the autumn (Brown et al., 2019).
The Role of FLC in the Regulation of Flowering in Perennial Brassicaceae Species
The role of FLC has been explored in perennial Brassicaceae species such as A. alpina (Wang et al., 2009; Albani et al., 2012), A. lyrata (Kemi et al., 2013), Arabidopsis halleri (Aikawa et al., 2010), Arabidopsis arenosa (Baduel et al., 2016), and Boechera stricta (Lee et al., 2018). At regions, syntenic to the FLC locus in A. thaliana, some of these species contain tandem FLC copies derived from duplication events after their divergence from A. thaliana (Nah and Chen, 2010; Albani et al., 2012). A. lyrata has two tandemly duplicated FLC genes (FLC1 and FLC2), whereas A. arenosa contains one partial and two complete FLC copies (Nah and Chen, 2010). In A. alpina a region of ∼2 kb at the FLC ortholog, PERPETUAL FLOWERING 1 (PEP1), has been tandemly duplicated (Albani et al., 2012). This tandem duplication includes the first exon of PEP1 (AaFLC) and parts of its promoter and first intron (Albani et al., 2012). Interestingly, this tandem duplication leads to the production of two overlapping PEP1 transcripts (PEP1a and PEP1b) that have different transcriptional start sites, using the two tandemly duplicated first exons (Albani et al., 2012). This finding could suggest that duplication events in FLC orthologs may give rise to diverse functions or specialized forms of the protein. Nevertheless, tandem duplications in FLC do not contribute to the perennial life cycle. For example, several FLC orthologs in both annual and perennial Arabis species contain duplicated regions (Kiefer et al., 2017). In addition, even within A. alpina not all accessions contain the partial duplication at PEP1 (AaFLC) (Albani et al., 2012).
The role of FLC in flowering in perennial Brassicaceae is similar to that reported in A. thaliana and other annual Brassicas. FLC1 mRNA levels in A. lyrata are down regulated in response to vernalization and FLC genes are co-localized with QTLs determining flowering time differences between late and early flowering A. lyrata accessions (Kemi et al., 2013). Similarly, A. alpina accessions carrying lesions in PEP1 (AaFLC) do not require vernalization to flower (Albani et al., 2012). The A. alpina pep1 mutant also flowers without vernalization compared to its wild type accession Pajares, that has an obligate vernalization requirement to flower (Wang et al., 2009). An interesting feature in perennial Brassicaceae is that FLC is not stably silenced after vernalization, resembling FLC expression patterns of A. thaliana accessions that require extended vernalization to flower (Wang et al., 2009; Kemi et al., 2013; Baduel et al., 2016; Qüesta et al., 2020). Unstable silencing of FLC orthologs by vernalization has been observed in plants grown in controlled environmental conditions (Wang et al., 2009; Kemi et al., 2013; Lazaro et al., 2018) and also in plants experiencing winter chilling temperatures in the field (Aikawa et al., 2010; Nishio et al., 2020). For instance, transcript accumulation of the A. halleri FLC (AhgFLC) is reduced during winter and is upregulated again the following spring (Aikawa et al., 2010). Interestingly, the accumulation of the H3K27me3 mark mirrors the expression patterns of AhgFLC (Nishio et al., 2020). In A. alpina plants vernalized for 12 weeks, PEP1 (AaFLC) is also temporarily silenced during cold exposure but it is transcriptionally activated again after plants return to growth-promoting conditions (Wang et al., 2009). On the contrary, FLC of the annual close relative Arabis montbretiana (AmFLC) is stably silenced by vernalization (Kiefer et al., 2017). Plants carrying introgressions of genomic segments containing the AmFLC into A. alpina, indicated that, in the same genetic background, PEP1 and AmFLC are differentially silenced after vernalization (Kiefer et al., 2017; Hyun et al., 2019). The major difference between the A. alpina and A. montbretiana FLC orthologs lies in polymorphisms within non-coding regions suggesting that, similar to A. thaliana FLC, non-coding polymorphisms may confer differences between PEP1 and AmFLC in stable silencing by vernalization (Coustham et al., 2012; Kiefer et al., 2017). Within the Arabideae, the annual life strategy arose several times during evolution (Kiefer et al., 2017). Phylogenetic studies in different taxa suggest that annuals were derived from perennial ancestors, although in some genera annuals seem to have switched back to the perennial growth habit (Friedman and Rubin, 2015). We can, therefore, hypothesize that Brassicaceae annual species may have gained independently cis polymorphisms at FLC non-coding regions to ensure stable silencing, which is important for the spring-flowering habit.
In addition to the seasonal cycling of PEP1 mRNA levels, the perennial behavior in A. alpina is also characterized by a strong regulation of age-related factors that determine whether individual meristems will initiate flowering in response to vernalization or not (Wang et al., 2009; Bergonzi et al., 2013; Hyun et al., 2019; Lazaro et al., 2019; Zhou et al., 2021). Interestingly, the degree of PEP1 (AaFLC) stable silencing by vernalization has been demonstrated to vary between meristems, depending on whether they initiated flowering during the cold exposure (Lazaro et al., 2018). In juvenile meristems that fail to initiate flowering during cold treatment, PEP1 (AaFLC) mRNA levels are reduced during vernalization and upregulated again after plants return to warm temperatures (Wang et al., 2011; Lazaro et al., 2018). In this way, PEP1 (AaFLC) ensures maintenance of vegetative growth after vernalization even when meristems develop further and acquire competence to flower. The underlying cause of the difference in PEP1 (AaFLC) regulation by prolonged cold exposure between juvenile and adult meristems is not known. In A. thaliana, DNA replication has been proposed to be essential for the maintenance of FLC silencing after vernalization (Finnegan and Dennis, 2007; Qüesta et al., 2020). On the same lines, differences in cell division and DNA replication between meristems may explain the variation observed in PEP1 (AaFLC) stable silencing by cold. In A. alpina, PEP1 (AaFLC) reactivation after vernalization has been shown to be facilitated by PERPETUAL FLOWERING2 [PEP2, the A. alpina ortholog of APETALA2 (AP2)], a role not previously reported for AP2 in A. thaliana (Bergonzi et al., 2013; Lazaro et al., 2019). The mechanism for this role of PEP2 (AaAP2) is not known. AP2 in A. thaliana interacts with chromatin remodeling factors such as HISTONE DEACETYLASE 19 (HDA19) (Krogan et al., 2012), but has never been associated with histone demethylases which could facilitate the upregulation of PEP1 (AaFLC) mRNA levels after vernalization.
Flowering in adult/competent meristems is initiated at about 6 weeks after the start of vernalization (Figure 2; Wang et al., 2009; Lazaro et al., 2018). For plants to flower, however, exposure to a minimum of 12 weeks of vernalization is required as inflorescence meristems should develop further during vernalization (Wang et al., 2009; Lazaro et al., 2018). The molecular mechanisms enabling floral development during vernalization involve the floral integrator AaSPL15 and increased sensitivity to the growth regulator gibberellin (GA) (Hyun et al., 2019; Tilmes et al., 2019). PEP1 expression is still not stably silenced after 12 weeks of vernalization, which leads to floral reversion phenotypes, such as the presence of bracts or flower to inflorescence reversion (Wang et al., 2009; Lazaro et al., 2018). Exposure to a minimum of 18 weeks of cold treatment is required to inhibit floral reversion and to stably silence PEP1 (Lazaro et al., 2018). Floral reversion phenotypes are also observed in A. halleri plants, although in this species it has not been reported whether flower buds are formed the previous year (Aikawa et al., 2010; Nishio et al., 2020).
Interestingly, A. alpina genes that act in the age pathway to regulate the age-dependent response to vernalization also influence the duration of vernalization required for flowering. For example, PEP2, TARGET OF EAT2 (AaTOE2), TERMINAL FLOWER1 (AaTFL1), and AaSPL15 determine the age at which plants become competent to flower in response to cold treatment, but also control the duration of vernalization required for flowering (Wang et al., 2011; Lazaro et al., 2018; Hyun et al., 2019; Zhou et al., 2021). The pep2 and Aatoe2 mutants and transgenic lines with reduced function of AaTFL1 (DsRNAi AaTFL1) flower when vernalized at a young age (at 3 weeks old compared to 5 weeks for wild type plants) and require less than 8 weeks of vernalization to flower (compared to 12 weeks for wild type plants) (Wang et al., 2011; Lazaro et al., 2019; Zhou et al., 2021).
In woody perennials, bud dormancy is regulated by a cluster of tandemly duplicated genes, DORMANCY ASSOCIATED MADS-BOX 1-6 (DAM1-6) (reviewed in Falavigna et al., 2019). Similar to FLC, DAM genes encode MADS box transcription factors, but these are more closely related to A. thaliana SHORT VEGETATIVE PHASE (SVP) and AGAMOUS-like 24 (AGL24) (reviewed in Falavigna et al., 2019). The expression of DAM genes is upregulated during dormancy induction and downregulated during the transition from endo- to ecodormancy (Maurya and Bhalerao, 2017; Vimont et al., 2019). DAM genes are also regulated by epigenetic mechanisms such as histone modifications (Leida et al., 2012; de la Fuente et al., 2015; Saito et al., 2015) and DNA methylation (Rothkegel et al., 2017). In A. thaliana, SVP is a floral repressor that interacts with other transcription factors to regulate floral development and flowering time (De Folter et al., 2005; Gregis et al., 2006; Lee et al., 2007; Balanzà et al., 2014; Mateos et al., 2015). FLC is an interactor of SVP in A. thaliana and both proteins act together to regulate flowering time by repressing FT and SOC1 or GA-related genes (Lee et al., 2007; Andrés et al., 2014; Mateos et al., 2015). These data suggest that SVP or other MADS box genes might have taken the role of FLC in woody perennials to regulate developmental traits related to prolonged cold exposure.
Pleiotropic Roles of FLC in Perennial Brassicaceae
In addition to the role of FLC in flowering, studies in A. thaliana and other Brassicaceae species demonstrated that FLC has pleiotropic effects on other developmental traits. Pleiotropic phenotypes may be an indirect effect caused by differences in flowering behavior adopted by different species and/or may be due to direct roles of FLC on other traits. Examples of direct and indirect pleiotropic effects of FLC are reported below.
Polycarpic Growth Habit and Shoot Architecture in the Perennial A. alpina
PERPETUAL FLOWERING 1 (AaFLC) contributes to the polycarpic growth habit and ensures that A. alpina plants maintain vegetative growth after flowering (Wang et al., 2009). In pep1 mutants all axillary branches flower, suggesting that PEP1 (AaFLC) regulates the fate of axillary meristems (Wang et al., 2009; Vayssières et al., 2020). This role of PEP1 (AaFLC) is facilitated by the ability of A. alpina plants to initiate flowering during vernalization (Figure 2; Wang et al., 2009; Lazaro et al., 2018). Flower bud development during vernalization is also coupled with the formation of axillary meristems in the leaf axils close to the shoot apical meristem (Wang et al., 2009; Ponraj and Theres, 2020; Vayssières et al., 2020). These newly formed axillary meristems develop into buds in a basipetal sequence and do not commit to reproductive development during cold exposure (green in Figures 2C,D; Wang et al., 2009; Ponraj and Theres, 2020; Vayssières et al., 2020). A. alpina mutants that carry lesions in floral repressors in the age pathway (AaTOE2 and AaAP2/PEP2) but also transgenic lines with altered levels of SPLs (e.g., microRNA cleavage-resistant forms of SPL15, rSPL15) have axillary branches at these subapical nodes which develop and become reproductive (Hyun et al., 2019; Lazaro et al., 2019; Zhou et al., 2021). A closer look at the A. alpina toe2 mutant demonstrated that these subapical buds initiate flowering already during vernalization (Figure 3B; Zhou et al., 2021). This result suggests that the fate of the subapical buds is determined during vernalization by the age pathway.
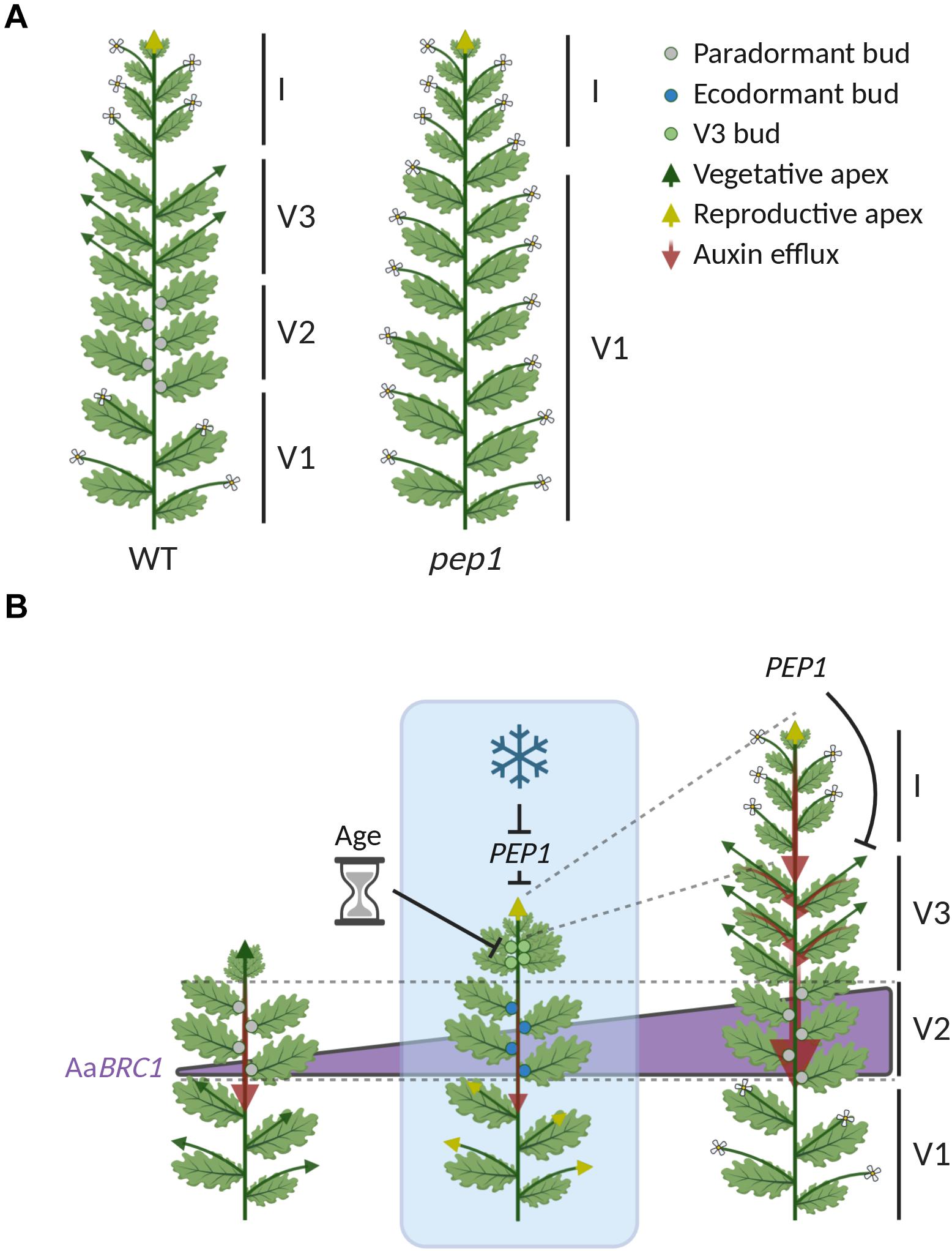
Figure 3. Shoot architecture in A. alpina is shaped by vernalization and PEP1. (A) Representation of wild type A. alpina (WT) and pep1 mutant plants after flowering. Wild type plants require exposure to long-term cold to flower, whereas pep1 mutants do not and can flower directly in greenhouse conditions. Flowering wild type plants have a complex shoot architecture, which is organized in differentiated zones of basal flowering axillary branches (V1) that partially senesce; dormant buds (V2); vegetative axillary branches (V3); and the inflorescence (I). The pep1 mutant lacks this zonation and has only V1 axillary branches and the inflorescence. (B) Representation of the shoot architecture of a wild type plant during its life cycle before, during and after vernalization. Blue box represents exposure to prolonged cold, whereas the time before and after cold exposure is not colored. During long-term cold, PEP1 (AaFLC) transcript levels are reduced and both the main shoot apex and the apices of V1 axillary branches initiate flowering (yellow arrowheads). In addition, new buds (V3 buds, green circles) are formed in those leaf primordia close to the main shoot apex. These V3 buds (green circles) do not initiate flowering during cold exposure due to the repressive action of the age pathway. After cold exposure, V3 buds give rise to V3 axillary branches, which grow vegetatively because PEP1 (AaFLC) transcript levels are upregulated and repress flowering. The outgrowth of V2 buds is inhibited by paradormancy (or apical dominance) before vernalization (gray circles), by ecodormancy during cold exposure (blue circles) and by paradormancy (or latency) after plants return to growth-promoting conditions (gray circles). The dormancy status of V2 buds increases during and after vernalization, and is regulated by a mechanism that is independent of PEP1 (AaFLC). Increased dormancy in V2 buds correlates with the upregulation of BRANCHED 1 (AaBRC1), a repressor of bud outgrowth (illustrated with the purple triangle). After vernalization, increased dormancy in V2 buds is correlated with increased endogenous IAA levels in the stem and auxin transport (red arrows). Image created using BioRender.com.
Wild type A. alpina flowering plants exhibit a complex shoot architecture in which axillary meristems behave in different ways and are organized in different zones (Figure 3A; Lazaro et al., 2018; Ponraj and Theres, 2020; Vayssières et al., 2020). Zonation patterns of differential bud activity and fate have also been reported in other perennials (Costes et al., 2014). The main stem of A. alpina plants consist of zones of basal axillary flowering branches (V1), axillary vegetative branches (V3) and dormant buds (V2) (Figure 3A; Lazaro et al., 2018; Ponraj and Theres, 2020; Vayssières et al., 2020). PEP1 (AaFLC) contributes to this complex shoot architecture considering that the pep1 mutant consists only of V1 axillary branches and lacks the V2 dormant buds and V3 vegetative branches (Figure 3A; Wang et al., 2009; Vayssières et al., 2020). In wild type plants, V1 axillary branches behave similarly to the main shoot apex and initiate flowering during vernalization, when PEP1 mRNA levels are reduced (yellow arrows in Figure 3B; Wang et al., 2009; Vayssières et al., 2020). V3 axillary branches arise after vernalization from buds formed at the subapical nodes during cold (green in Figures 2C,D; green circles in Figure 3B; Wang et al., 2009; Lazaro et al., 2018; Ponraj and Theres, 2020; Vayssières et al., 2020; Zhou et al., 2021). PEP1 determines the fate of these buds after vernalization (Wang et al., 2009; Lazaro et al., 2018). Specifically, PEP1 mRNA levels are upregulated after vernalization to repress flowering and ensure that these branches will maintain vegetative development (V3 green arrows in Figure 3B; Wang et al., 2009; Lazaro et al., 2018). V2 buds follow a different developmental path, in which their growth is repressed before and after vernalization by paradormancy (or latency) and their activity is influenced by their position on the shoot (gray circles in Figure 3B; Vayssières et al., 2020). During cold exposure, V2 buds become ecodormant (blue circles in Figure 3B; Vayssières et al., 2020). The activity of V2 buds is not directly regulated by PEP1 (AaFLC) but rather by the A. alpina ortholog of BRANCHED 1 (AaBRC1), whose expression increases during and after vernalization (Purple triangle in Figure 3B; Vayssières et al., 2020). Flowering time genes such as FT in A. thaliana and SPL15 (OsSPL14) in rice have been reported to influence bud activity (Miura et al., 2010; Tsuji et al., 2015). PEP1 (AaFLC) might indirectly inhibit the outgrowth of buds in the nodes within the V2 zone. Specifically, PEP1 (AaFLC), by repressing flowering in V3 axillary branches, ensures the continuous formation of young leaves that may act as an auxin source to inhibit the outgrowth of V2 buds (Red arrows in Figure 3B; Vayssières et al., 2020). Many studies in A. thaliana support the theory that auxin saturation in the transport stream of the main stem can inhibit bud outgrowth by blocking auxin transport from lateral sources (Prusinkiewicz et al., 2009). Dormant buds are always located at the axils of leaves below the zone of V3 vegetative branches, supporting the hypothesis that V3 branches may become the new auxin source that represses the outgrowth of V2 buds (Red arrows in Figure 3B; Vayssières et al., 2020). Nevertheless, mutants in which V3 branches flower still have a dormant bud zone (Hyun et al., 2019; Zhou et al., 2021). This phenotype can be explained by the fact that flowering V3 branches still maintain vegetative development through secondary or tertiary branching and can still act as auxin sources (Zhou et al., 2021).
Overall, the complex architecture in A. alpina is a result of the effect of prolonged cold exposure on the regulation of flowering and on bud dormancy, which differs between meristems. These roles of cold are partially controlled by PEP1 (AaFLC).
Duration of the Flowering Season in the Perennial A. alpina
Many temperate perennials restrict the duration of the flowering season to spring and summer. In A. alpina, pep1 mutants and accessions that carry inactive PEP1 alleles flower perpetually (continuously) (Wang et al., 2009; Albani et al., 2012). In the field, perpetual flowering genotypes with non-functional PEP1 alleles show extended and asynchronous flowering (Hughes et al., 2019). Interestingly, these genotypes also exhibit reduced survival in the field, suggesting that PEP1 contributes to plant fitness (Hughes et al., 2019). The role of PEP1 on the duration of the flowering season is a result of the upregulation of PEP1 mRNA levels after vernalization. PEP1 restricts the duration of flowering season by ensuring that no further meristems will initiate flowering after the return to warm temperatures.
So far, there are only a few studies exploring the regulation of flowering duration in other perennials. In the Rosaceace, there is natural variation for this trait. Genotypes that follow either the seasonal flowering habit (and restrict the duration of the flowering episode) or the perpetual flowering habit (and flower continuously) can be found (Brown and Wareing, 1965; Crespel et al., 2002; Albani et al., 2004). In the wild strawberry Fragaria vesca and rose, the perpetual flowering habit arose from loss of function mutations in the floral repressor TERMINAL FLOWER1 (TFL1) (Iwata et al., 2012; Koskela et al., 2012). Interestingly, all perpetual flowering F. vesca accessions contain the same 2 bp deletion in FvTFL1, suggesting that in F. vesca (but not in A. alpina and rose) the perpetual flowering habit arose only once during evolution (Albani et al., 2012; Iwata et al., 2012; Koskela et al., 2012). It is also worth noting that the perpetual flowering habit in most genotypes is linked to a loss of environmental sensitivity for flowering-promoting conditions. F. vesca accessions that flower seasonally require exposure to short photoperiods to induce flowering, whereas perpetual flowering accessions are day neutral (Koskela et al., 2012). Similarly, seasonal flowering A. alpina accessions require vernalization to flower, whereas perpetual flowering accessions can flower without being exposed to vernalization (Wang et al., 2009; Albani et al., 2012).
Leaf Traits in the Annual Cardamine hirsuta
FLOWERING LOCUS C has been detected in QTL studies to underlie differences in leaf size and complexity in the annual Brassicaceae Cardamine hirsuta (Cartolano et al., 2015). Accessions with reduced expression of the C. hirsuta FLC ortholog (ChFLC) flower early and have leaves with more leaflets (Cartolano et al., 2015). Interestingly, cis polymorphisms in the nucleation region of ChFLC seem to be responsible for the differences in leaf complexity and flowering time (Cartolano et al., 2015). This effect of ChFLC on leaf shape cannot be uncoupled from flowering (Cartolano et al., 2015), suggesting that the leaf phenotype in C. hirsuta may be associated with flowering time.
Pleiotropic Roles of FLC in Seed Traits in A. thaliana and A. alpina
Plant fitness can be influenced by seed traits such as seed dormancy and longevity. Seed dormancy is defined as the incapacity of seeds to germinate under favorable conditions and enables seeds to prevent germination outside the favorable growth season. Seed longevity determines how long seeds remain viable in the seed bank. Monocarpic plants are usually characterized by seeds with higher seed longevity, as seeds constitute their only option to persist in a certain habitat (Thompson et al., 1998).
In A. thaliana, several studies using the (low dormant) accessions Landsberg erecta (Ler) and Columbia (Col) provided evidence that FLC enhances germination (Figure 4; Chiang et al., 2009; Auge et al., 2017; Blair et al., 2017). However, this positive effect of FLC on germination has not always been observed. Other studies (also using the Ler and Col accessions) indicated that FLC plays a negative role in germination by enhancing seed dormancy (Figure 4; Chen et al., 2014; Chen and Penfield, 2018) or that it does not influence dormancy (Liu et al., 2011). These different results suggest a role of FLC in germination and dormancy in A. thaliana that could depend on environmental conditions and genetic backgrounds. This is also suggested by the observation that the germination phenotype of FLC depends on the dormancy level of seeds (Blair et al., 2017). In addition, these different observations could be explained by the function of other genes, such as FT, that control FLC expression during germination and independently affect seed germination (Auge et al., 2019). To further enhance the complexity by which these flowering time genes regulate seed traits, FT has also been shown to either enhance or reduce dormancy in different studies (Chiang et al., 2009; Chen et al., 2014; Chen and Penfield, 2018). Although complex, the observed phenotypes indicate a role for FLC and other flowering time genes in the control of germination. The exact role and mechanism of FLC in germination control is still unclear, but it seems likely to be influenced by environmental factors (in particular temperature) that vary between experiments. This is consistent with the observation that FLC is maternally controlled in its influence on dormancy (Chiang et al., 2009; Chen and Penfield, 2018). FLC is therefore likely to have a role in the translation of environmental conditions experienced by the mother plant into the dormancy level of its progeny seeds.
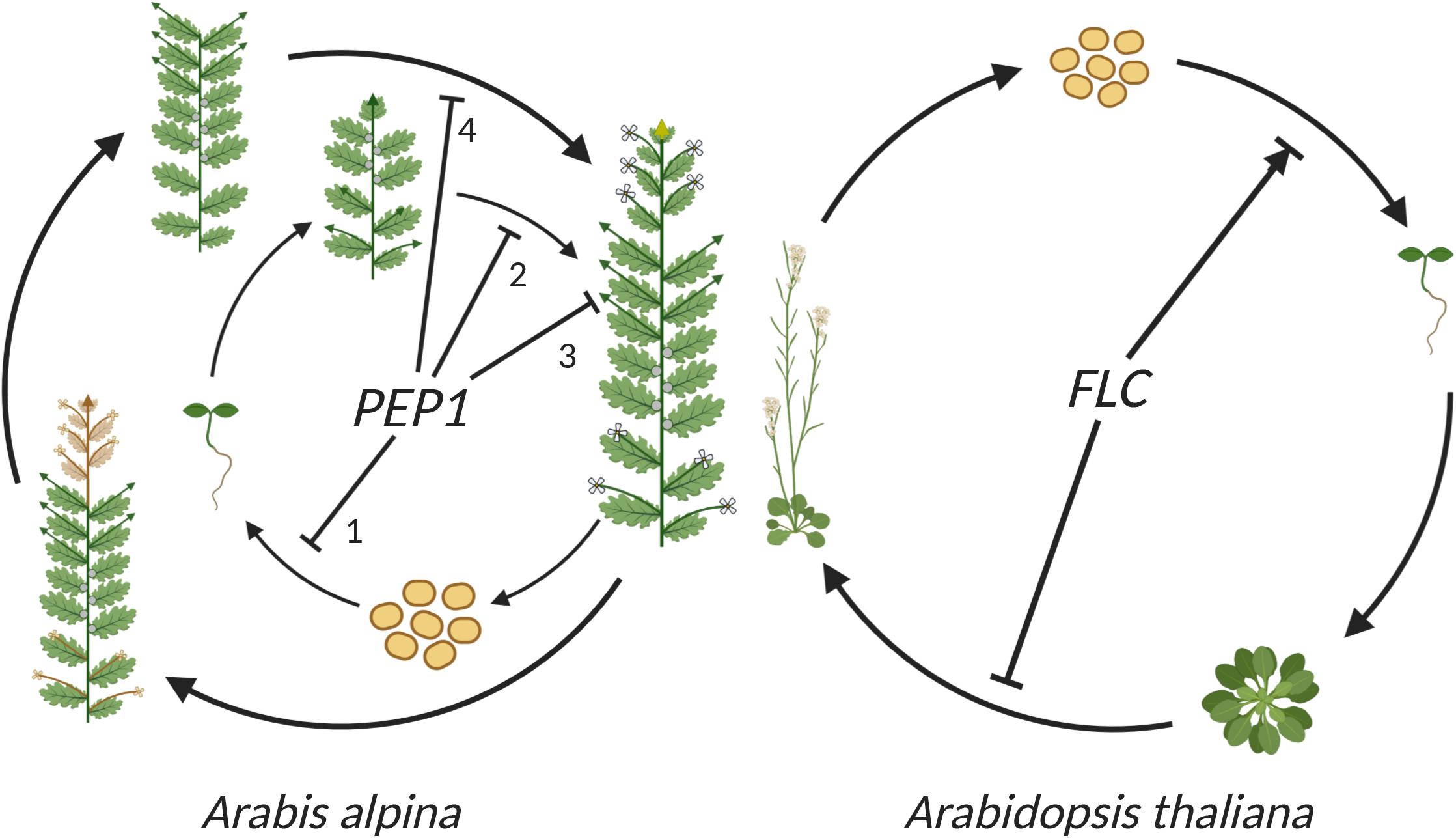
Figure 4. The role of FLC in the life cycle of perennial A. alpina and annual A. thaliana. In A. alpina, PEP1 (AaFLC) regulates flowering in response to vernalization and contributes to the perennial life cycle by acting at different developmental stages and tissues. Starting from seeds, PEP1 acts as a negative regulator of germination and an enhancer of seed longevity (1). Later on, PEP1 represses flowering and ensures that plants will synchronize anthesis with favorable environmental conditions in the spring (2). PEP1 also ensures that V3 axillary branches will remain vegetative after flowering (3). The role of PEP1 in flowering and maintenance of vegetative development are repeated every year (4). In Arabidopsis, FLC acts as a floral repressor in accessions that require vernalization to flower. FLC also influences germination in A. thaliana, either positively or negatively depending on the environmental conditions and genetic background. Lines with (→) indicate positive influence and lines with (⊥) indicate negative influence. As in Figures 1, 3, green arrows represent vegetative branches, yellow arrows represent flowering branches and gray circles represent dormant V2 buds. Image created using BioRender.com.
Although both flowering and germination should be properly timed to ensure reproductive success, the suppressive role of FLC on flowering seems to be more consistent among experiments and species, compared to its role in seed traits. An interesting difference between these two developmental transitions is that seed germination can be arrested and postponed for a year if conditions are unfavorable, which is not possible for flowering in monocarpic plants. Seed dormancy induction on the mother plant is also more sensitive to subtle differences in ambient temperature compared to flowering induction. Small differences in temperature before and during seed development can have a strong impact on seed dormancy (Springthorpe and Penfield, 2015). If FLC has a role in translating these subtle temperature differences to dormancy differences, it could explain the varying phenotypes between experiments. Another difference is that exposure to long-term low temperatures (below 10°C) downregulates FLC transcript accumulation and leads to flowering, whereas exposure of plants to mildly low temperatures (16 versus 22°C) before seeds are fully ripe can enhance FLC expression (Chen and Penfield, 2018). This suggests a different mechanism by which temperature regulates FLC expression, which could be related with its more flexible role in germination compared to flowering.
In annuals, FLC regulates both flowering time and germination traits and therefore constitutes a connecting factor between both traits. Different scenarios for this connection can be hypothesized which could be advantageous in different climates. The combination of late flowering and low dormancy could cause seeds to germinate in autumn and enable the plant to survive in a vegetative state during winter. Late flowering and high dormancy would delay germination till the next spring and enable plants to grow vegetatively during the year and flower the following spring. Early flowering and low dormancy would be advantageous in a climate with humid summers and enable two generations within one year. Early flowering and high dormancy would enable flowering and seed set in spring and delay germination until the next spring. In perennials, all these different scenarios are less relevant as plants can persist longer in their ecosystems.
In A. alpina, PEP1 (AaFLC) also influences seed dormancy and seed longevity. The pep1 mutant alleles have low dormancy and reduced longevity, suggesting that PEP1 positively regulates these traits (Figure 4; Hughes et al., 2019). The influence of PEP1 on seed dormancy seems more consistent in A. alpina compared to that of FLC in A. thaliana. Although there has only been a single study of this trait in A. alpina until now, it showed a consistent role of PEP1 in greenhouse and garden experiments. The existence of many A. alpina accessions with non-functional PEP1 alleles suggests an advantage of the combination of perpetual flowering and low dormancy in certain environments.
Overall, the role of FLC in the two major developmental transitions of plants and its adjustment to temperature give it an important regulatory role to determine the life cycle of Brassicaceae species. Additional functions of FLC as described in this review also suggest a link between the regulation of these developmental transitions and the architecture of the plant. This could have selective advantages in nature but also implies that breeding for altered flowering time in Brassicaceae species could have unexpected effects on seed traits and/or plant architecture or other still unknown trade-offs. Therefore, functional studies of this link can provide new insights in the relation between these traits and support breeding for crop adaptation to local and changing climates. Whereas FLC has been demonstrated to affect different traits in Brassicaceae, other genes are expected to fulfill its function in other plant families. For instance, in cereals the VRN genes have a similar role in the regulation of vernalization as FLC in the Brassicaceae. It would be of great interest to find out whether these genes also have similar roles in other traits that are regulated by FLC. Conservation of this link would indicate its general importance for survival and adaptation of plants to their environment.
Author Contributions
All authors developed the concept and wrote the manuscript. NVdIT prepared the figures.
Funding
This work was supported by the Cluster of Excellence on Plant Sciences (CEPLAS) funded by the Deutsche Forschungsgemeinschaft (DFG, German Research Foundation) under Germany’s Excellence Strategy – EXC 2048/1 – Project ID: 390686111.
Conflict of Interest
WJJS was employed by company Rijk Zwaan.
The remaining authors declare that the writing of this review was conducted in the absence of any commercial or financial relationships that could be construed as a potential conflict of interest.
Acknowledgments
We thank Margaret Kox for critical reading of the manuscript and Alice Vayssières for contributing in the design of the A. alpina model.
References
Aikawa, S., Kobayashi, M. J., Satake, A., Shimizu, K. K., and Kudoh, H. (2010). Robust control of the seasonal expression of the Arabidopsis FLC gene in a fluctuating environment. Proc. Natl. Acad. Sci. U.S.A. 107, 11632–11637. doi: 10.1073/pnas.0914293107
Albani, M. C., Battey, N. H., and Wilkinson, M. J. (2004). The development of ISSR-derived SCAR markers around the SEASONAL FLOWERING LOCUS (SFL) in Fragaria vesca. Theor. Appl. Genet. 109, 571–579. doi: 10.1007/s00122-004-1654-4
Albani, M. C., Castaings, L., Wötzel, S., Mateos, J. L., Wunder, J., Wang, R., et al. (2012). PEP1 of Arabis alpina is encoded by two overlapping genes that contribute to natural genetic variation in perennial flowering. PLoS Genet. 8:e1003130. doi: 10.1371/journal.pgen.1003130
Albani, M. C., and Coupland, G. (2010). Comparative analysis of flowering in annual and perennial plants. Curr. Top. Dev. Biol. 91, 323–348. doi: 10.1016/S0070-2153(10)91011-9
Andrés, F., Porri, A., Torti, S., Mateos, J., Romera-Branchat, M., García-Martínez, J. L., et al. (2014). SHORT VEGETATIVE PHASE reduces gibberellin biosynthesis at the Arabidopsis shoot apex to regulate the floral transition. Proc. Natl. Acad. Sci. U.S.A. 111, E2762–E2769. doi: 10.1073/pnas.1409567111
Angel, A., Song, J., Dean, C., and Howard, M. (2011). A Polycomb-based switch underlying quantitative epigenetic memory. Nature 476, 105–109. doi: 10.1038/nature10241
Atkinson, C. J., Brennan, R. M., and Jones, H. G. (2013). Declining chilling and its impact on temperate perennial crops. Environ. Exp. Bot. 91, 48–62. doi: 10.1016/j.envexpbot.2013.02.004
Auge, G. A., Blair, L. K., Neville, H., and Donohue, K. (2017). Maternal vernalization and vernalization-pathway genes influence progeny seed germination. New Phytol. 216, 388–400. doi: 10.1111/nph.14520
Auge, G. A., Penfield, S., and Donohue, K. (2019). Pleiotropy in developmental regulation by flowering-pathway genes: is it an evolutionary constraint? New Phytol. 224, 55–70. doi: 10.1111/nph.15901
Baduel, P., Arnold, B., Weisman, C. M., Hunter, B., and Bomblies, K. (2016). Habitat-Associated life history and stress-tolerance variation in Arabidopsis arenosa. Plant Physiol. 171, 437–451. doi: 10.1104/pp.15.01875
Balanzà, V., Martínez-Fernández, I., and Ferrándiz, C. (2014). Sequential action of FRUITFULL as a modulator of the activity of the floral regulators SVP and SOC1. J. Exp. Bot. 65, 1193–1203. doi: 10.1093/jxb/ert482
Bergonzi, S., and Albani, M. C. (2011). Reproductive competence from an annual and a perennial perspective. J. Exp. Bot. 62, 4415–4422. doi: 10.1093/jxb/err192
Bergonzi, S., Albani, M. C., Van Themaat, E. V. L., Nordström, K. J. V., Wang, R., Schneeberger, K., et al. (2013). Mechanisms of age-dependent response to winter temperature in perennial flowering of Arabis alpina. Science 340, 1094–1097. doi: 10.1126/science.1234116
Berry, S., and Dean, C. (2015). Environmental perception and epigenetic memory: mechanistic insight through FLC. Plant J. 83, 133–148. doi: 10.1111/tpj.12869
Billings, W. D., and Mooney, H. A. (1968). The ecology of arctic and Alpine plants. Biol. Rev. Camb. Philos. Soc. 43, 481–529.
Blair, L., Auge, G., and Donohue, K. (2017). Effect of FLOWERING LOCUS C on seed germination depends on dormancy. Funct. Plant Biol. 44, 493–506. doi: 10.1071/FP16368
Bond, D. M., Dennis, E. S., Pogson, B. J., and Finnegan, E. J. (2009). Histone acetylation, VERNALIZATION INSENSITIVE 3, FLOWERING LOCUS C, and the vernalization response. Mol. Plant 2, 724–737. doi: 10.1093/mp/ssp021
Bouché, F., Woods, D. P., and Amasino, R. M. (2017). Winter memory throughout the plant kingdom: different paths to flowering. Plant Physiol. 173, 27–35. doi: 10.1104/pp.16.01322
Bratzel, F., and Turck, F. (2015). Molecular memories in the regulation of seasonal flowering: from competence to cessation. Genome Biol. 16, 1–14. doi: 10.1186/s13059-015-0770-6
Brown, J. K. M., Beeby, R., and Penfield, S. (2019). Yield instability of winter oilseed rape modulated by early winter temperature. Sci. Rep. 9, 1–9. doi: 10.1038/s41598-019-43461-7
Brown, T., and Wareing, P. F. (1965). The genetical control of the everbearing habit and three other characters in varieties of Fragaria vesca. Euphytica 14, 97–112. doi: 10.1007/BF00032819
Carmona, M. J., Chaïb, J., Martínez-Zapater, J. M., and Thomas, M. R. (2008). A molecular genetic perspective of reproductive development in grapevine. J. Exp. Bot. 59, 2579–2596. doi: 10.1093/jxb/ern160
Cartolano, M., Pieper, B., Lempe, J., Tattersall, A., Huijser, P., Tresch, A., et al. (2015). Heterochrony underpins natural variation in Cardamine hirsuta leaf form. Proc. Natl. Acad. Sci. U.S.A. 112, 10539–10544. doi: 10.1073/pnas.1419791112
Chen, M., MacGregor, D. R., Dave, A., Florance, H., Moore, K., Paszkiewicz, K., et al. (2014). Maternal temperature history activates Flowering Locus T in fruits to control progeny dormancy according to time of year. Proc. Natl. Acad. Sci. U.S.A. 111, 18787–18792. doi: 10.1073/pnas.1412274111
Chen, M., and Penfield, S. (2018). Feedback regulation of COOLAIR expression controls seed dormancy and flowering time. Science 12, 1014–1017.
Chiang, G. C. K., Barua, D., Kramer, E. M., Amasino, R. M., and Donohue, K. (2009). Major flowering time gene, FLOWERING LOCUS C, regulates seed germination in Arabidopsis thaliana. Proc. Natl. Acad. Sci. U.S.A. 106, 11661–11666.
Chouard, P. (1960). Vernalization and its relations to dormancy. Annu. Rev. Plant Physiol. 11, 191–238. doi: 10.1146/annurev.pp.11.060160.001203
Costa, S., and Dean, C. (2019). Storing memories: the distinct phases of Polycomb-mediated silencing of Arabidopsis FLC. Biochem. Soc. Trans. 47, 1187–1196. doi: 10.1042/BST20190255
Costes, E., Crespel, L., Denoyes, B., Morel, P., Demene, M.-N., Lauri, P.-E., et al. (2014). Bud structure, position and fate generate various branching patterns along shoots of closely related Rosaceae species: a review. Front. Plant Sci. 5:666. doi: 10.3389/fpls.2014.00666
Coustham, V., Li, P., Strange, A., Lister, C., Song, J., and Dean, C. (2012). Quantitative modulation of polycomb silencing underlies natural variation in vernalization. Science 337, 584–587. doi: 10.1126/science.1221881
Crespel, L., Chirollet, M., Durel, C. E., Zhang, D., Meynet, J., and Gudin, S. (2002). Mapping of qualitative and quantitative phenotypic traits in Rosa using AFLP markers. Theor. Appl. Genet. 105, 1207–1214. doi: 10.1007/s00122-002-1102-2
Csorba, T., Questa, J. I., Sun, Q., and Dean, C. (2014). Antisense COOLAIR mediates the coordinated switching of chromatin states at FLC during vernalization. Proc. Natl. Acad. Sci. U.S.A. 111, 16160–16165. doi: 10.1073/pnas.1419030111
De Folter, S., Immink, R. G. H., Kieffer, M., Pařenicová, L., Henz, S. R., Weigel, D., et al. (2005). Comprehensive interaction map of the Arabidopsis MADS box transcription factors. Plant Cell 17, 1424–1433. doi: 10.1105/tpc.105.031831
de la Fuente, L., Conesa, A., Lloret, A., Badenes, M. L., and Ríos, G. (2015). Genome-wide changes in histone H3 lysine 27 trimethylation associated with bud dormancy release in peach. Tree Genet. Genomes 11:45. doi: 10.1007/s11295-015-0869-7
De Lucia, F., Crevillen, P., Jones, A. M. E., Greb, T., and Dean, C. (2008). A PHD-polycomb repressive complex 2 triggers the epigenetic silencing of FLC during vernalization. Proc. Natl. Acad. Sci. U.S.A. 105, 16831–16836. doi: 10.1073/pnas.0808687105
Deng, W., Ying, H., Helliwell, C. A., Taylor, J. M., Peacock, W. J., and Dennis, E. S. (2011). FLOWERING LOCUS C (FLC) regulates development pathways throughout the life cycle of Arabidopsis. Proc. Natl. Acad. Sci. U.S.A. 108, 6680–6685. doi: 10.1073/pnas.1103175108
Diggle, P. K. (1997). Extreme preformation in alpine Polygonum viviparum: an architectural and developmental analysis. Am. J. Bot. 84, 154–169. doi: 10.2307/2446077
Duncan, S., Holm, S., Questa, J., Irwin, J., Grant, A., and Dean, C. (2015). Seasonal shift in timing of vernalization as an adaptation to extreme winter. eLife 4:e06620. doi: 10.7554/elife.06620
Engin, H., and Ünal, A. (2007). Examination of flower bud initiation and differentiation in sweet cherry and peach by scanning electron microscope. Turk. J. Agric. For. 31, 373–379. doi: 10.3906/tar-0707-1
Falavigna, V. D. S., Guitton, B., Costes, E., and Andrés, F. (2019). I want to (Bud) break free: the potential role of DAM and SVP-Like genes in regulating dormancy cycle in temperate fruit trees. Front. Plant Sci. 9:1990. doi: 10.3389/fpls.2018.01990
Finnegan, E. J., and Dennis, E. S. (2007). Vernalization-induced Trimethylation of histone H3 Lysine 27 at FLC is not maintained in mitotically quiescent cells. Curr. Biol. 17, 1978–1983. doi: 10.1016/j.cub.2007.10.026
Friedman, J., and Rubin, M. J. (2015). All in good time: understanding annual and perennial strategies in plants. Am. J. Bot. 102, 497–499. doi: 10.3732/ajb.1500062
Gregis, V., Sessa, A., Colombo, L., and Kater, M. M. (2006). AGL24, SHORT VEGETATIVE PHASE, and APETALA1 redundantly control AGAMOUS during early stages of flower development in Arabidopsis. Plant Cell 18, 1373–1382. doi: 10.1105/tpc.106.041798
Heo, J. B., and Sung, S. (2011). Vernalization-mediated epigenetic silencing by a long intronic noncoding RNA. Science 331, 76–79. doi: 10.1126/science.1197349
Hepworth, J., Antoniou-kourounioti, R. L., Berggren, K., Selga, C., and Tudor, E. H. (2020). Natural variation in autumn expression is the major adaptive determinant distinguishing Arabidopsis FLC haplotypes. eLife 9:e57671.
Horvath, D. (2009). Common mechanisms regulate flowering and dormancy. Plant Sci. 177, 523–531. doi: 10.1016/j.plantsci.2009.09.002
Hughes, P. W., Soppe, W. J. J., and Albani, M. C. (2019). Seed traits are pleiotropically regulated by the flowering time gene PERPETUAL FLOWERING 1 (PEP1) in the perennial Arabis alpina. Mol. Ecol. 28, 1183–1201. doi: 10.1111/mec.15034
Hyun, Y., Richter, R., and Coupland, G. (2017). Competence to flower: age-controlled sensitivity to environmental cues. Plant Physiol. 173, 36–46. doi: 10.1104/pp.16.01523
Hyun, Y., Vincent, C., Tilmes, V., Bergonzi, S., Kiefer, C., Richter, R., et al. (2019). A regulatory circuit conferring varied flowering response to cold in annual and perennial plants. Science 363, 409–412. doi: 10.1126/science.aau8197
Iwata, H., Gaston, A., Remay, A., Thouroude, T., Jeauffre, J., Kawamura, K., et al. (2012). The TFL1 homologue KSN is a regulator of continuous flowering in rose and strawberry. Plant J. 69, 116–125. doi: 10.1111/j.1365-313X.2011.04776.x
Kemi, U., Leinonen, P. H., Savolainen, O., and Kuittinen, H. (2019). Inflorescence shoot elongation, but not flower primordia formation, is photoperiodically regulated in Arabidopsis lyrata. Ann. Bot. 124, 91–102. doi: 10.1093/aob/mcz035
Kemi, U., Niittyvuopio, A., Toivainen, T., Pasanen, A., Quilot-Turion, B., Holm, K., et al. (2013). Role of vernalization and of duplicated FLOWERING LOCUS C in the perennial Arabidopsis lyrata. New Phytol. 197, 323–335. doi: 10.1111/j.1469-8137.2012.04378.x
Kiefer, C., Severing, E., Karl, R., Bergonzi, S., Koch, M., Tresch, A., et al. (2017). Divergence of annual and perennial species in the Brassicaceae and the contribution of cis-acting variation at FLC orthologues. Mol. Ecol. 26, 3437–3457. doi: 10.1111/mec.14084
Koskela, E. A., Mouhu, K., Albani, M. C., Kurokura, T., Rantanen, M., Sargent, D. J., et al. (2012). Mutation in TERMINAL FLOWER1 reverses the photoperiodic requirement for flowering in the wild strawberry Fragaria vesca. Plant Physiol. 159, 1043–1054. doi: 10.1104/pp.112.196659
Krogan, N. T., Hogan, K., and Long, J. A. (2012). APETALA2 negatively regulates multiple floral organ identity genes in Arabidopsis by recruiting the co-repressor TOPLESS and the histone deacetylase HDA19. Development 139, 4180–4190. doi: 10.1242/dev.085407
Lang, G. A., Early, J. D., Martin, G. C., and Darnell, R. L. (1987). Endo-, para-, and ecodormancy: physiological terminology and classification for dormancy research. Hortscience 22, 371–377.
Lazaro, A., Obeng-Hinneh, E., and Albani, M. C. (2018). Extended vernalization regulates inflorescence fate in Arabis alpina by stably silencing PERPETUAL FLOWERING1. Plant Physiol. 176, 2819–2833. doi: 10.1104/pp.17.01754
Lazaro, A., Zhou, Y., Giesguth, M., Nawaz, K., Bergonzi, S., Pecinka, A., et al. (2019). PERPETUAL FLOWERING2 coordinates the vernalization response and perennial flowering in Arabis alpina. J. Exp. Bot. 70, 995–1004. doi: 10.1093/jxb/ery423
Lee, C. R., Hsieh, J. W., Schranz, M. E., and Mitchell-Olds, T. (2018). The functional change and deletion of FLC homologs contribute to the evolution of rapid flowering in Boechera stricta. Front. Plant Sci. 9:1078. doi: 10.3389/fpls.2018.01078
Lee, H. L., Seong, J. Y., Soo, H. P., Hwang, I., Jong, S. L., and Ji, H. A. (2007). Role of SVP in the control of flowering time by ambient temperature in Arabidopsis. Genes Dev. 21, 397–402. doi: 10.1101/gad.1518407
Leida, C., Conesa, A., Llácer, G., Badenes, M. L., and Ríos, G. (2012). Histone modifications and expression of DAM6 gene in peach are modulated during bud dormancy release in a cultivar-dependent manner. New Phytol. 193, 67–80. doi: 10.1111/j.1469-8137.2011.03863.x
Li, P., Filiault, D., Box, M. S., Kerdaffrec, E., van Oosterhout, C., Wilczek, A. M., et al. (2014). Multiple FLC haplotypes defined by independent cis-regulatory variation underpin life history diversity in Arabidopsis thaliana. Genes Dev. 28, 1635–1640. doi: 10.1101/gad.245993.114
Liu, Y., Geyer, R., van Zanten, M., Carles, A., Li, Y., Hörold, A., et al. (2011). Identification of the Arabidopsis REDUCED DORMANCY 2 gene uncovers a role for the polymerase associated factor 1 complex in seed dormancy. PLoS One 6:e0022241. doi: 10.1371/journal.pone.0022241
Luo, X., Ou, Y., Li, R., and He, Y. (2020). Maternal transmission of the epigenetic ‘memory of winter cold’ in Arabidopsis. Nat. Plants 6, 1211–1218. doi: 10.1038/s41477-020-00774-0
Madrid, E., Chandler, J. W., and Coupland, G. (2020). Gene regulatory networks controlled by FLOWERING LOCUS C that confer variation in seasonal flowering and life history. J. Exp. Bot. 72, 4–14. doi: 10.1093/jxb/eraa216
Mateos, J. L., Madrigal, P., Tsuda, K., Rawat, V., Richter, R., Romera-Branchat, M., et al. (2015). Combinatorial activities of SHORT VEGETATIVE PHASE and FLOWERING LOCUS C define distinct modes of flowering regulation in Arabidopsis. Genome Biol. 16, 1–23. doi: 10.1186/s13059-015-0597-1
Mateos, J. L., Tilmes, V., Madrigal, P., Severing, E., Richter, R., Rijkenberg, C. W. M., et al. (2017). Divergence of regulatory networks governed by the orthologous transcription factors FLC and PEP1 in Brassicaceae species. Proc. Natl. Acad. Sci. U.S.A. 114, E11037–E11046. doi: 10.1073/pnas.1618075114
Maurya, J. P., and Bhalerao, R. P. (2017). Photoperiod- and temperature-mediated control of growth cessation and dormancy in trees: a molecular perspective. Ann. Bot. 120, 351–360. doi: 10.1093/aob/mcx061
Meloche, C. G., and Diggle, P. K. (2001). Preformation, architectural complexity, and developmental flexibility in Acomastylis rossii (Rosaceae). Am. J. Bot. 88, 980–991. doi: 10.2307/2657079
Michaels, S. D., and Amasino, R. M. (1999). FLOWERING LOCUS C encodes a novel MADS domain protein that acts as a repressor of flowering. Plant Cell 11:949. doi: 10.2307/3870827
Miura, K., Ikeda, M., Matsubara, A., Song, X. J., Ito, M., Asano, K., et al. (2010). OsSPL14 promotes panicle branching and higher grain productivity in rice. Nat. Genet. 42, 545–549. doi: 10.1038/ng.592
Nah, G., and Chen, Z. J. (2010). Tandem duplication of the FLC locus and the origin of a new gene in Arabidopsis related species and their functional implications in allopolyploids. New Phytol. 186, 228–238. doi: 10.1111/j.1469-8137.2009.03164.x
Nishio, H., Buzas, D. M., Nagano, A. J., Iwayama, K., Ushio, M., and Kudoh, H. (2020). Repressive chromatin modification underpins the long-term expression trend of a perennial flowering gene in nature. Nat. Commun. 11, 1–12. doi: 10.1038/s41467-020-15896-4
O’Neill, C. M., Lu, X., Calderwood, A., Tudor, E. H., Robinson, P., Wells, R., et al. (2019). Vernalization and floral transition in autumn drive winter annual life history in oilseed rape. Curr. Biol. 29, 4300–4306. doi: 10.1016/j.cub.2019.10.051
Or, E. (2009). “Grape bud dormancy release-the molecular aspect,” in Grapevine Molecular Physiology & Biotechnology, ed. K. A. Roubelakis-Angelakis (Cham: Springer), 1–29. doi: 10.1007/978-90-481-2305-6
Périlleux, C., Pieltain, A., Jacquemin, G., Bouché, F., Detry, N., D’Aloia, M., et al. (2013). A root chicory MADS box sequence and the Arabidopsis flowering repressor FLC share common features that suggest conserved function in vernalization and de-vernalization responses. Plant J. 75, 390–402. doi: 10.1111/tpj.12208
Ponraj, U., and Theres, K. (2020). Keep a distance to be different: axillary buds initiating at a distance from the shoot apical meristem are crucial for the perennial lifestyle of Arabis alpina. New Phytol. 227, 116–131. doi: 10.1111/nph.16512
Prusinkiewicz, P., Crawford, S., Smith, R. S., Ljung, K., Bennett, T., Ongaro, V., et al. (2009). Control of bud activation by an auxin transport switch. Proc. Natl. Acad. Sci. U.S.A. 106, 17431–17436. doi: 10.1073/pnas.0906696106
Qüesta, J. I., Antoniou-Kourounioti, R. L., Rosa, S., Li, P., Duncan, S., Whittaker, C., et al. (2020). Noncoding SNPs influence a distinct phase of Polycomb silencing to destabilize long-term epigenetic memory at Arabidopsis FLC. Genes Dev. 34, 446–461. doi: 10.1101/gad.333245.119
Qüesta, J. I., Song, J., Geraldo, N., An, H., and Dean, C. (2016). Arabidopsis transcriptional repressor VAL1 triggers Polycomb silencing at FLC during vernalization. Science 353, 485–488. doi: 10.1126/science.aaf7354
Rothkegel, K., Sánchez, E., Montes, C., Greve, M., Tapia, S., Bravo, S., et al. (2017). DNA methylation and small interference RNAs participate in the regulation of MADS-box genes involved in dormancy in sweet cherry (Prunus avium L.). Tree Physiol. 37, 1739–1751. doi: 10.1093/treephys/tpx055
Saito, T., Bai, S., Imai, T., Ito, A., Nakajima, I., and Moriguchi, T. (2015). Histone modification and signalling cascade of the dormancy-associated MADS-box gene, PpMADS13-1, in Japanese pear (Pyrus pyrifolia) during endodormancy. Plant Cell Environ. 38, 1157–1166. doi: 10.1111/pce.12469
Sheldon, C. C., Rouse, D. T., Finnegan, E. J., Peacock, W. J., and Dennis, E. S. (2000). The molecular basis of vernalization: the central role of FLOWERING LOCUS C (FLC). Proc. Natl. Acad. Sci. U.S.A. 97, 3753–3758. doi: 10.1073/pnas.060023597
Shindo, C., Lister, C., Crevillen, P., Nordborg, M., and Dean, C. (2006). Variation in the epigenetic silencing of FLC contributes to natural variation in Arabidopsis vernalization response. Genes Dev. 20, 3079–3083. doi: 10.1101/gad.405306
Springthorpe, V., and Penfield, S. (2015). Flowering time and seed dormancy control use external coincidence to generate life history strategy. eLife 2015:e05557. doi: 10.7554/eLife.05557
Sung, S., and Amasino, R. M. (2004). Vernalization in Arabidopsis thaliana is mediated by the PHD finger protein VIN3. Nature 427, 159–164. doi: 10.1038/nature02195
Swiezewski, S., Liu, F., Magusin, A., and Dean, C. (2009). Cold-induced silencing by long antisense transcripts of an Arabidopsis Polycomb target. Nature 462, 799–802. doi: 10.1038/nature08618
Tao, Z., Hu, H., Luo, X., Jia, B., Du, J., and He, Y. (2019). Embryonic resetting of the parental vernalized state by two B3 domain transcription factors in Arabidopsis. Nat. Plants 5, 424–435. doi: 10.1038/s41477-019-0402-3
Thompson, K., Bakker, J. P., Bekker, R. M., and Hodgson, J. G. (1998). Ecological correlates of seed persistence in soil in the North-West European Flora. J. Ecol. 86, 163–169.
Tilmes, V., Mateos, J. L., Madrid, E., Vincent, C., Severing, E., Carrera, E., et al. (2019). Gibberellins act downstream of Arabis PERPETUAL FLOWERING1 to accelerate floral induction during vernalization. Plant Physiol. 180, 1549–1563. doi: 10.1104/pp.19.00021
Tsuji, H., Tachibana, C., Tamaki, S., Taoka, K. I., Kyozuka, J., and Shimamoto, K. (2015). Hd3a promotes lateral branching in rice. Plant J. 82, 256–266. doi: 10.1111/tpj.12811
Vayssières, A., Mishra, P., Roggen, A., Neumann, U., Ljung, K., and Albani, M. C. (2020). Vernalization shapes shoot architecture and ensures the maintenance of dormant buds in the perennial Arabis alpina. New Phytol. 227, 99–115. doi: 10.1111/nph.16470
Vimont, N., Fouché, M., Campoy, J. A., Tong, M., Arkoun, M., Yvin, J. C., et al. (2019). From bud formation to flowering: transcriptomic state defines the cherry developmental phases of sweet cherry bud dormancy. BMC Genomics 20:974. doi: 10.1186/s12864-019-6348-z
Wang, R., Albani, M. C., Vincent, C., Bergonzi, S., Luan, M., Bai, Y., et al. (2011). Aa TFL1 confers an age-dependent response to vernalization in perennial Arabis alpina. Plant Cell 23, 1307–1321. doi: 10.1105/tpc.111.083451
Wang, R., Farrona, S., Vincent, C., Joecker, A., Schoof, H., Turck, F., et al. (2009). PEP1 regulates perennial flowering in Arabis alpina. Nature 459, 423–427. doi: 10.1038/nature07988
Wollenberg, A. C., and Amasino, R. M. (2012). Natural variation in the temperature range permissive for vernalization in accessions of Arabidopsis thaliana. Plant Cell Environ. 35, 2181–2191. doi: 10.1111/j.1365-3040.2012.02548.x
Wood, C. C., Robertson, M., Tanner, G., Peacock, W. J., Dennis, E. S., and Helliwell, C. A. (2006). The Arabidopsis thaliana vernalization response requires a polycomb-like protein complex that also includes VERNALIZATION INSENSITIVE 3. Proc. Natl. Acad. Sci. U.S.A. 103, 14631–14636. doi: 10.1073/pnas.0606385103
Yuan, W., Luo, X., Li, Z., Yang, W., Wang, Y., Liu, R., et al. (2016). A cis cold memory element and a trans epigenome reader mediate Polycomb silencing of FLC by vernalization in Arabidopsis. Nat. Genet. 48, 1527–1534. doi: 10.1038/ng.3712
Zhao, Y., Antoniou-kourounioti, R. L., Calder, G., Dean, C., and Howard, M. (2020). Temperature-dependent growth contributes to long-term cold sensing. Nature 583, 825–829. doi: 10.1038/s41586-020-2485-4
Zhou, Y. (2019). Identification and Characterisation of Genes that Regulate Flowering and Perennial Traits in Arabis alpina. Köln: Universität zu Köln.
Keywords: Arabis alpina, FLC, flowering, PEP1, perennial, perpetual flowering, polycarpic growth habit, vernalization
Citation: Soppe WJJ, Viñegra de la Torre N and Albani MC (2021) The Diverse Roles of FLOWERING LOCUS C in Annual and Perennial Brassicaceae Species. Front. Plant Sci. 12:627258. doi: 10.3389/fpls.2021.627258
Received: 08 November 2021; Accepted: 25 January 2021;
Published: 15 February 2021.
Edited by:
Caroline Dean, John Innes Centre, United KingdomReviewed by:
David Horvath, Edward T. Schafer Agricultural Research Center (USDA-ARS), United StatesJulia Qüesta, Centre for Research in Agricultural Genomics (CRAG), Spain
Copyright © 2021 Soppe, Viñegra de la Torre and Albani. This is an open-access article distributed under the terms of the Creative Commons Attribution License (CC BY). The use, distribution or reproduction in other forums is permitted, provided the original author(s) and the copyright owner(s) are credited and that the original publication in this journal is cited, in accordance with accepted academic practice. No use, distribution or reproduction is permitted which does not comply with these terms.
*Correspondence: Maria C. Albani, bWFsYmFuaUB1bmkta29lbG4uZGU=; YWxiYW5pQG1waXB6Lm1wZy5kZQ==