- 1Guangdong Key Laboratory of Ornamental Plant Germplasm Innovation and Utilization, Environmental Horticulture Research Institute, Guangdong Academy of Agricultural Sciences, Guangzhou, China
- 2College of Agronomy, Henan Agricultural University, Zhengzhou, China
- 3National Key Laboratory for Crop Genetics and Germplasm Enhancement, Nanjing Agricultural University, Nanjing, China
Versatile protoplast platforms greatly facilitate the development of modern botany. However, efficient protoplast-based systems are still challenging for numerous horticultural plants and crops. Orchids are globally cultivated ornamental and medicinal monocot plants, but few efficient protoplast isolation and transient expression systems have been developed. In this study, we established a highly efficient orchid protoplast isolation protocol by selecting suitable source materials and optimizing the enzymatic conditions, which required optimal D-mannitol concentrations (0.4–0.6 M) combined with optimal 1.2% cellulose and 0.6% macerozyme, 5 μM of 2-mercaptoethanol and 6 h digestion. Tissue- and organ-specific protoplasts were successfully isolated from young leaves [∼3.22 × 106/g fresh weight (FW)], flower pedicels (∼5.26 × 106/g FW), and young root tips (∼7.66 × 105/g FW) of Cymbidium orchids. This protocol recommends the leaf base tissues (the tender part of young leaves attached to the stem) as better source materials. High yielding viable protoplasts were isolated from the leaf base of Cymbidium (∼2.50 × 107/g FW), Phalaenopsis (1.83 × 107/g FW), Paphiopedilum (1.10 × 107/g FW), Dendrobium (8.21 × 106/g FW), Arundina (3.78 × 106/g FW) orchids, and other economically important monocot crops including maize (Zea mays) (3.25 × 107/g FW) and rice (Oryza sativa) (4.31 × 107/g FW), which showed marked advantages over previous mesophyll protoplast isolation protocols. Leaf base protoplasts of Cymbidium orchids were used for polyethylene glycol (PEG)-mediated transfection, and a transfection efficiency of more than 80% was achieved. This leaf base protoplast system was applied successfully to analyze the CsDELLA-mediated gibberellin signaling in Cymbidium orchids. We investigated the subcellular localization of the CsDELLA-green fluorescent protein fusion and analyzed the role of CsDELLA in the regulation of gibberellin to flowering-related genes via efficient transient overexpression and gene silencing of CsDELLA in Cymbidium protoplasts. This protoplast isolation and transient expression system is the most efficient based on the documented results to date. It can be widely used for cellular and molecular studies in orchids and other economically important monocot crops, especially for those lacking an efficient genetic transformation system in vivo.
Introduction
Protoplasts are plant cells from which the cell walls have been enzymatically removed (Eeckhaut et al., 2013). They are totipotent, sensitive, and versatile. Protoplast-based platforms allow creation of new plant species via protoplast fusion and regeneration (Melchers et al., 1978; Grosser and Gmitter, 1990). They explore the signal transduction and metabolic pathways transiently respond to hormones and stress factors (Sheen, 2001; Hirata et al., 2012), answer specific questions related to cell types (Petersson et al., 2015; Denyer et al., 2019), and determine the subcellular localization, transport, and interactions of tagged proteins (Goodman et al., 2004; Zhang et al., 2011). The versatility of plant protoplasts greatly facilitates modern botany development (Duarte et al., 2016).
Although a number of efficient protoplast isolation protocols for model plants and many crops are established (Table 1), it is still challenging for numerous horticultural plants and crops. Leaf tissues are the most commonly used source materials, and high yielding mesophyll protoplasts can be easily isolated from tobacco (Nagata and Takebe, 1971), Arabidopsis (Menczel, 1980), maize (Z. mays L.) (Kanai and Edwards, 1973), rice (Oryza sativa L.) (Toriyama and Hinata, 1985), and many other non-model plant species, such as Medicago sativa (Song et al., 1990), Panicum virgatum (Mazarei et al., 2008), Elaeis guineensis (Masani et al., 2014), Hevea brasiliensis (Zhang et al., 2016b), Phaseolus vulgaris (Nanjareddy et al., 2016), and Magnolia (Shen et al., 2017). However, it is not possible to isolate sufficient viable protoplasts from the mature leaves of many other plants due to species limitations. Hence, in vitro grown seedlings were tested, and mesophyll protoplasts have been isolated from Tanacetum (Keskitalo et al., 2001), grape (Vitis vinifera) (Mliki et al., 2003), pepper (Capsicum annuum L.) (Jeon et al., 2007), and pineapple (Priyadarshan et al., 2018). However, callus induction is time-consuming (several months), and flower petals have been selected as an alternative for protoplast isolation from ornamental plants, including Rosa rugosa (Borochov et al., 1976), Petunia hybrid (Oh and Kim, 1994), Hippeastrum, and Tulipa (Wagner, 1979). However, flowers withered within a short period, and petals are not sufficient to supply continuously for protoplast isolation.
Orchidaceae is one of the largest and the most evolved families of monocot plants on the planet, and more than 70,000 orchid species have been globally cultivated as ornamental and medicinal plants over the past 1500 years (Hsiao et al., 2006; Roberts and Dixon, 2008). Cymbidium orchids are important symbols of oriental culture. In the past few decades, extensive research on orchid tissue culture, protoplast fusion, and regeneration has been undertaken (Shrestha et al., 2007; Yam and Arditti, 2009). Nevertheless, few highly efficient protoplast isolation protocols are available for orchids. Orchid protoplasts were first successfully isolated from the leaves of Cymbidium (Capesius and Meyer, 1977) and various tissues of Renantanda, Dendrobium, and Paphiopedilum orchids (Teo and Neumann, 1978a, b). Leaf tissues of in vitro grown seedlings have been used most commonly (Table 1). Mesophyll protoplasts were successfully isolated from Dendrobium [∼3.97 × 105/g fresh weight (FW)] (Khentry et al., 2006), Cymbidium (maximum 1.1 × 107/g FW) (Pindel, 2007), and Phalaenopsis orchids (5.9 × 106/g FW) (Li et al., 2018). Additionally, flower petals were used for efficient protoplast isolation from Dendrobium (Hu et al., 1998) and Phalaenopsis [1.9 × 105 per petal (∼0.2 g/FW)] (Lin et al., 2018b), and Cymbidium orchids (∼3.50 × 107/g FW) (Ren et al., 2020). However, the protoplast isolation efficiency is relatively lower than that of Arabidopsis (∼3.0 × 107/g FW) (Wu et al., 2009), maize (1–5 × 106/g FW) (Cao et al., 2014), rice (∼1.0 × 107/g FW) (Zhang et al., 2011), and cassava (Manihot esculenta Crantz) (4.4 × 107/g FW) (Wu et al., 2017). Abundant calcium oxalate crystals accumulated in the ruptured protoplasts can puncture other protoplasts (Pindel, 2007), which is another limiting factor for high yielding protoplast isolation. Enzyme combinations and osmotic conditions influence the yield of viable protoplasts (Nagata and Takebe, 1971; Kanai and Edwards, 1973; Sheen, 2001; Chen et al., 2006; Yoo et al., 2007).
The protoplast-based transient expression system (PTES) provides an ideal platform for molecular, cellular, and functional identification of genes/proteins. It relies on the transient expression of exogenous genetic information via the introduction of nucleic acids vectors by polyethylene glycol (PEG) or electroporation (Krens et al., 1982; Hauptmann et al., 1987; Hayashimoto et al., 1990). Compared with stable transformation, PTES is fast, convenient and economical. High throughput PTES has been applied to screen transactivation of hundreds of transcription factors (Wehner et al., 2011), and highly efficient genome editing and gene silencing cassettes prior to the development of transgenic plants (Bart et al., 2006; Tang et al., 2016; Cao et al., 2014; Lin et al., 2018b; Page et al., 2019). Lin et al. (2018a) reported an efficient PTES to enable molecular, cellular, and functional studies for Phalaenopsis orchids. However, a versatile PTES has not been established for Cymbidium orchids.
In this study, we aimed to develop a simple and efficient protoplast isolation and transient expression system for Orchids. By selecting suitable source materials (including flower pedicels, young leaves, leaf base tissues, and young root tips) and optimizing the enzymatic conditions, high yielding protoplasts were successfully isolated from Cymbidium plants (Figure 1). This protocol recommends the leaf base tissues as suitable source materials that provide the highest yield of viable protoplasts, which is readily available, sustainable, and inexpensive. We obtained sufficient tissue- and organ-specific protoplasts form various tissues, including flower pedicels, young leaves, tender leaf bases, and young root tips, by determining the optimal enzymatic conditions. Additionally, this protocol has been applied to highly efficient leaf base protoplast isolation from Phalaenopsis, Paphiopedilum, Dendrobium, and Arundina orchids, and important monocot crops like maize and rice. Finally, the Cymbidium leaf base protoplasts were used to establish the PEG-mediated PTES, which is feasible for investigating protein subcellular localization, cellular signal transduction in response to phytohormones, and efficient gene transient overexpression and silencing. This versatile leaf base protoplast system would be useful for biological studies on orchids and many other monocot plant species.
Materials and Methods
Plant Materials and Growth Conditions
Five orchid species (Cymbidium, Phalaenopsis, Paphiopedilum, Dendrobium, and Arundina) were selected for this study. These orchids exhibited distinct morphological characteristics; for instance, the leaves of Phalaenopsis orchids were fleshy, whereas those of Cymbidium orchids were hard leather-like, and the leaves of Dendrobium and Paphiopedilum orchids were in between these. These orchid plants were obtained from the orchid breeding base of the Environmental Horticulture Research Institute, Guangdong Academy of Agricultural Sciences, China. They were grown in plastic pots (20 cm × 20 cm) in greenhouses under the exact growing conditions necessary for each species, as previously described (Yang et al., 2019). Maize and rice were also included, with maize and rice seeds obtained from the Rice Research Institute and Crops Research Institute of Guangdong Academy of Agricultural Sciences, respectively. The maize and rice seedlings were grown in plant growth rooms at 27°C under a 16 h light/8 h dark cycle and 60% humidity.
Protoplast Isolation
Protoplast isolation from the various plant species was conducted based on protocols established by Yoo et al. (2007) and Lin et al. (2018b) with some modifications. Various source materials collected from Cymbidium orchids, including flower pedicels, young leaves, leaf base tissues, and young root tips were tested for tissue- and organ-specific protoplast isolation. Additionally, leaf base tissues of Phalaenopsis, Paphiopedilum, Dendrobium, and Arundina orchids, and rice and maize plants were tested for protoplast isolation. Maize and rice plants were grown for 7 days after germination, and then the leaf bases of these seedlings were collected for protoplast isolation, whereas their young leaves served as controls.
The tissues were surface sterilized for 3 min in 75% ethyl alcohol (volume percentage, v/v), followed by five washes in sterilized water. The sterilized tissues were cut into 0.5–1.0 mm strips or slices using fresh surgical blades on sterile filter paper and were immediately transferred to a freshly prepared enzyme solution in a 100 mL sterile flask. The enzyme solution was prepared as follows: 20 mM KCl (Sigma, St. Louis, MO, United States), 20 mM 2-(N-morpholino)ethanesulfonic acid (pH 5.7) (Sigma) with different concentrations of D-mannitol (0.2, 0.3, 0.4, 0.5, 0.6, and 0.7 M) (Sigma), cellulose R-10 (0.6, 1.2 and 2.4, w/v), and macerozyme R-10 (0.3, 0.6, and 1.2%, w/v) (Yakult Pharmaceutical Industry Ltd., Nishinomiya, Japan). The solution was then warmed up to 55°C for 10 min, and cooled to room temperature (∼25°C). Then, 10 mM CaCl2 (Sigma), and 0.1% (w/v) bovine serum albumin (Sigma) were added to different concentrations of 2-mercaptoethanol (0, 1, 2, 5, and 10 mM) (Sigma). For each treatment, 10 mL enzyme solution was prepared for approximately 0.5 g fresh tissues. After incubation at 28°C in the dark with a rotation of 30 rpm for different periods (2, 4, 6, and 8 h), the released protoplasts were harvested. Generally, the enzyme mixture containing protoplasts was diluted with an equal volume of wash solution (W5) that contained 154 mM NaCl (Sigma), 125 mM CaCl2, 5 mM KCl, and 4 mM MES (pH = 5.7). The protoplast-containing solution was filtered through a 150 μm nylon mesh into a 50 mL round-bottomed centrifuge tube and centrifuged at 100 × g for 2 min to pellet the protoplasts (at room temperature ∼25°C). Subsequently, the supernatant was removed carefully as much as possible with a sterile syringe. The protoplast pellet was re-suspended in 20 mL of W5 solution (Negrutiu et al., 1986), and then the yield and viability of protoplasts were estimated.
Protoplast Yield and Viability Estimation
The protoplasts were counted and photographed using a Leica DM2500 microscope (Leica, Wetzlar, Germany) with a hemocytometer to calculate the estimated yield of protoplasts. The protoplast yield was calculated as the total number of protoplasts released in the enzyme mixture divided by the FW of tissues used for protoplast isolation (protoplasts/g FW). Fluorescein diacetate (FDA) (Sigma) staining was applied to determine the viability of protoplasts as follows: 9 μL of the protoplast-containing solution was placed onto a glass slide, followed by the addition of 1 μL of 0.2% FDA solution [dissolved in acetone (Solarbio Science and Technology Co., Ltd. Beijing, China)] and incubated at room temperature for 1–2 min. Viable protoplasts with green fluorescence were visualized and photographed using an LSM 710 confocal laser microscope (Carl Zeiss, Inc., Jena, Germany) with blue excitation block. Protoplast viability was measured as the number of protoplasts with green fluorescence divided by the total number of protoplasts ×100%. Three images were selected for the yield and viability measurements of each sample. Each experiment was repeated three times.
Plasmid Preparation
To test the feasibility of orchid leaf base protoplasts for protein subcellular localization and gene regulation analysis, vector plasmids expressing green fluorescent protein (GFP), GFP-protein fusions, proteins, and gene-specific target sequence were prepared for PEG-mediated protoplast transfection (Supplementary Figure 1A). The empty vector pAN580-GFP expressing GFP was used as a control for the protein subcellular localization. Plant organelle markers, including pGreenII62-SK-AtWAK2-GFP and pGreenII62-SK-AtPIP2A-GFP, were used in the present study. The two AtWAK2 and AtPIP2A signal peptides isolated from Arabidopsis thaliana were specifically accumulated in the endoplasmic reticulum and plasma membrane, respectively (Gomord et al., 1997; Cutler et al., 2000).
The recombinant plasmid pAN580-CsDELLA-GFP was obtained by inserting the full-length coding sequence (CDS) of CsDELLA (GenBank accession number: MK282635.1) without a termination codon between the dual cauliflower mosaic virus 35S promoter and the GFP gene of the vector pAN580-GFP. Meanwhile, the full-length CDS of CsDELLA was cloned into vector pAN580-GFP, and replaced with the GFP gene, resulting in the pAN580-CsDELLA plasmid (Supplementary Figure 1B). For the gene silencing vector pTCK303-CsDELLA-RNAi, the 243 base pair sequence of CsDELLA was cloned into the vector pTCK303-RNAi with an Ubiquitin promoter (Supplementary Figure 1C). Briefly, total RNA was extracted from freshly collected young leaves of Cymbidium sinense using an RNA Simple Total RNA Kit (Tiangen, Beijing, China). The DNA-free RNA was used for first-strand cDNA synthesis with oligo (dT) primers and a PrimeScriptTM 1st strand cDNA Synthesis Kit (Takara, Dalian, China), following the manufacturer’s instructions. Specific primers with overlapping homologous ends were designed using the Primer Premier 5.0 software (Premier, Palo Alto, CA, United States) according to the full-length CDS and gene-specific target sequence of CsDELLA (Supplementary Table 1). Subsequently, the fragment of CsDELLA was amplified using PrimerSTAR Max DNA Polymerase (Takara) with the cDNA and specific primers. The polymerase chain reaction (PCR) products were purified and cloned into certain vectors using a Seamless Assembly Cloning Kit (CloneSmarter, Houston, TX, United States) following the manufacturer’s instructions.
Vectors including pGreenII62-SK-AtWAK2-GFP, pGreenII62-SK-AtPIP2A-GFP, pAN580-GFP, pAN580-CsDELLA-GFP, pAN580-CsDELLA, and pTCK303-CsDELLA-RNAi were transformed into Escherichia coli DH5α competent cells (Tiangen) according to the manufacturer’s instructions, respectively. Each single E. coli colony was picked and inoculated in 50 mL Luria-Bertani broth [Tryptone (1.0%, w/v), Yeast extract (0.5%, w/v), and NaCl (1.0%, w/v), pH = 7.0] containing the appropriate antibiotic. Bacterial cells were pelleted by centrifuging cultures at 3,000 × g for 10 min following culturing at 37°C and 200 rpm shaking for 12–16 h. Following mass replication of the bacterium, plasmid DNA was extracted using an Endo-Free Plasmid Maxi Kit (Omega Bio-tek, Norcross, GA, United States). The concentrated plasmid DNA was prepared at different concentrations (up to 2.0 μg/μL), and was transformed into protoplasts.
PEG-Mediated Protoplast Transfection
Following yield and viability measurements, protoplasts were further purified before transfection and transient expression. The 20 mL protoplast-containing W5 solution was re-centrifuged at 100 × g for 2 min (at room temperature). Following resuspension in 20 mL W5 solution, protoplasts were placed on ice for 30 min. Viable protoplasts settled at the bottom of the tube by gravity. Finally, the supernatant was carefully removed as much as possible, and the purified protoplasts were adjusted to a density of 1 × 105 – 1 × 106/mL with the pre-chilled MMG solution (Negrutiu et al., 1987). The MMG solution comprised 0.5 M D-mannitol, 15 mM MgCl2, and 4 mM MES (pH = 5.7).
Protoplast transfection was conducted following a modified PEG-mediated protocol (Yoo et al., 2007). For each transfection, 100 μL of MMG-protoplast mixture was gently mixed with 10 μL of pre-chilled plasmid DNA in 2 mL round-bottomed centrifuge tubes. Then, an equal volume (110 μL) of freshly prepared PEG-CaCl2 solution was added and immediately mixed by gentle inversion. The PEG-CaCl2 solution was composed of 100 mM CaCl2, 0.2 M D-mannitol, and 25% PEG4000 (final concentration, w/v) (Sigma). The mixture was incubated at room temperature in the dark for 10 min. The transfection was stopped by adding two volumes of W5 solution (440 μL) followed by centrifugation at 100 × g for 2 min. The transfected protoplasts were then washed with W5 solution and re-suspended in 1 mL WI solution for each of the 6-well tissue culture plates. The WI solution was composed of 0.4 M D-mannitol, 20 mM KCl, and 4 mM MES (pH = 5.7). For transient expression of genes/proteins, the protoplast mixture was incubated in the dark at 23°C for 12–36 h.
Confocal Imaging of Transfected Protoplasts
After incubation in the dark at 23°C for 12–24 h, the fluorescence of GFP or GFP-protein fusions was viewed under an LSM710 confocal laser scanning microscope (Carl Zeiss, Inc.). Transformation efficiency of the protoplasts was determined based on the GFP-reporter expression using the transient expression vector pAN580-GFP. GFP fluorescence was observed and 3–5 images were taken randomly under an LSM780 fluorescent microscope (Carl Zeiss, Inc.) or LSM710 confocal laser scanning microscope. The transformation efficiency was measured as a bright green fluorescent protoplast number in view/total protoplast number in view (%). At least three photographs were taken for each sample, and these experiments were independently conducted at least three times. For subcellular localization analysis, plant organelle markers pGreenII62-SK-AtWAK2-GFP and pGreenII62-SK-AtPIP2A-GFP, and the control vector pGreenII62-SK-GFP were transfected into orchid leaf base protoplasts. Red chlorophyll fluorescence was used to indicate the intercellular location of chloroplasts.
Protoplast Treatment
Protoplast treatments were undertaken in W5 solution supplemented with different concentrations of gibberellin (GA3) (1, 10, and 100 μM). Approximately 107 protoplasts in 1 mL W5 solution were used for each treatment. W5 solution supplemented with water was used as the control treatment at each time point. Protoplasts were cultivated in 6-well plates in a growth chamber at 23°C in the dark for 6–24 h.
Quantitative Real-Time Polymerase Chain Reaction
Protoplasts treated with GA3 were harvested at 6, 12 and 24 hours post treatment (hpt), and transfected protoplasts were harvested at 12, 24 and 36 hours post transfection (hpt). First-strand cDNA was obtained from the total RNA of the harvested protoplasts. Gene-specific primers were designed according to the CDSs of CsDELLA, SUPPRESSOR OF OVEREXPRESSION OF CONSTANS1 (CsSOC1, MF474250.1), FLOWERING LOCUS T (CsFT, HM803115.1), SHORT VEGETATIVE PHASE3 (CsSVP3, MF462098.1), CsLFY (KC138806.1), and APETALA1 (CsAP1, JQ326260.1) using Primer Premier 5.0 software (Premier, Palo Alto, CA, United States). CsUbiquitin in Cymbidium (referred to as CsUBQ, AY907703) was used as an internal reference control to normalize the total amount of cDNA in each reaction (Supplementary Table 1).
The quantitative real-time polymerase chain reaction (qRT-PCR) was performed in a 20 μL reaction volume comprising 2.0 μL of 5× diluted first-strand cDNA (approximately 20 ng), 0.8 μL of each primer (10.0 μM), 10.0 μL of 2× SYBR Green I Master Mix (Takara), and 6.4 μL of sterile distilled H2O. All reactions were performed in 96-well reaction plates using a Bio-Rad CFX-96 Real-time PCR System (Bio-Rad, Hercules, Canada) with three technical replicates. The following PCR conditions were used: 95°C for 5 min, followed by 40 cycles at 95°C for 15 s, 60°C for 30 s, and 72°C for 30 s, and then at 68°C for 5 min. The expression of candidate genes was quantified using the relative quantification (2–ΔΔCT) method. Each sample was collected independently with three biological replicates.
Statistical Analysis
Statistical analysis was performed using one-way analysis of variance with SPSS version 18.0 software (SPSS Inc., Chicago, IL, United States). Data are presented as the mean ± standard error from three independent experiments. The least significant differences among treatments were determined at the 5% level of probability with Duncan’s multiple range tests.
Results
Selection of Source Materials for Cymbidium Protoplast Isolation
To establish an efficient protoplast isolation protocol for orchids, various source materials, including flower pedicels, young leaves, leaf base tissues, and young root tips of Cymbidium orchids were tested (Figure 2A). Viable protoplasts were successfully isolated from various tissues or organs of Cymbidium plants following the protocol described by Yoo et al. (2007) (Figure 2B). However, the yield and viability of released protoplasts were relatively low, and abundant calcium oxalate crystal raphides were observed in the protoplast-containing solution (Supplementary Figure 2).
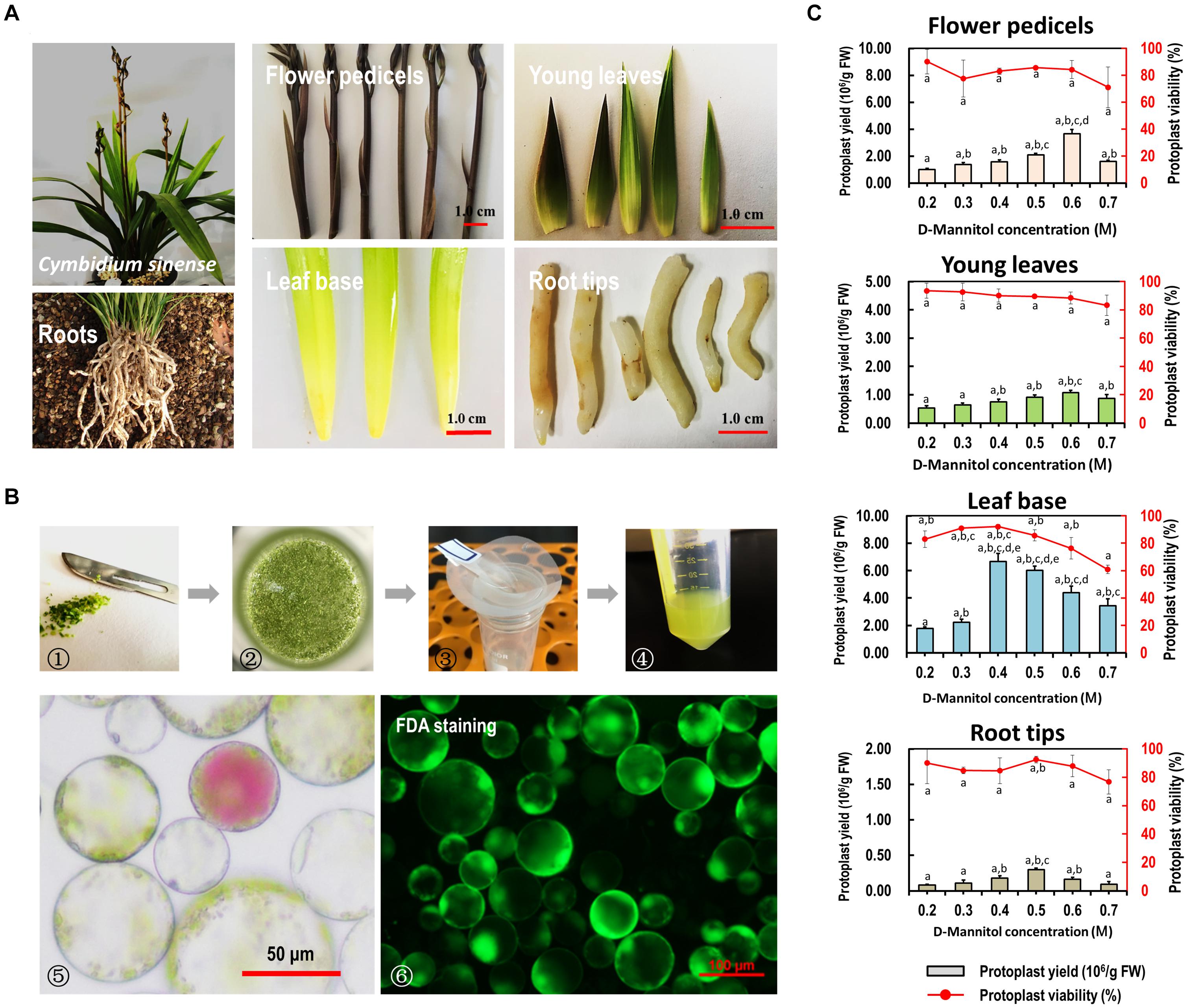
Figure 2. Protoplast isolation from various organs/tissues of Cymbidium orchids. (A) Various tissues including flower petals, flower pedicels, young leaves, leaf base tissues, and root tips collected from Cymbidium orchids were used for protoplast isolation. (B) Schematic illustration of protoplast isolation from Cymbidium orchid: (1) cut tissues into 0.5–1.0-mm strips or slices using fresh surgical blades on sterile filter papers; (2) transfer strips or slices quickly and gently into in a 100 mL flask; (3) enzymatic solution containing protoplasts were filter through a 150 μm nylon mesh into a 50 mL round-bottomed tube; (4) wash protoplasts twice with W5 solution; (5) check the morphology and yield of protoplasts under a microscope; (6) measure the protoplast viability using the FDA staining method. (C) Optimal D-mannitol concentrations in enzyme solutions were determined for different tissues. Data presented as means of three biological replicates with error bars indicating standard deviations (SD), and different letters (a–e) among treatments indicate statically significant differences at p < 0.05 based on Duncan’s multiple range test.
To reduce the protoplast rupture or collapse during enzymatic digestion, gradient concentrations of D-mannitol in the enzyme solution (0.2, 0.3, 0.4, 0.5, 0.6, and 0.7 M) were included for different tissues. We found that the D-mannitol concentration significantly affected the efficiency of protoplast isolation. Generally, increasing D-mannitol concentrations resulted in increased protoplast yields, whereas exceeding osmotic pressures resulted in decreased yields. The optimal D-mannitol concentrations for various tissues gave the highest yield and viability simultaneously. For flower pedicels, leaf base, young leaves, and root tips of Cymbidium orchids, the optimal D-mannitol concentrations were determined as 0.6, 0.6, 0.4, and 0.5 M, respectively. With the optimal D-mannitol concentrations, the orchid leaf base gave the highest yield of viable protoplasts (∼6.67 × 106/g FW), followed by the flower pedicels (∼3.67 × 106/g FW), young leaves (∼1.07 × 106/g FW), and root tips (∼2.97 × 105/g FW) (Figure 2C). In addition, protoplasts isolated from young leaves and root tips remained intact for more than 24 h in the W5 solution (Supplementary Figure 2), whereas that of flower pedicels ruptured within 6 h post-isolation. Most protoplasts isolated from the leaf base tissues (>70%) remained intact for up to 3 days in the WI solution (Nagy and Maliga, 1976), which was stronger and longer-term healthy than that of the other tissues/organs. Therefore, the leaf base was a better source material for protoplast isolation from Cymbidium orchids.
Optimization of Enzymatic Conditions for Cymbidium Protoplast Isolation
More factors affecting protoplast isolation were investigated to increase the yield and viability of protoplasts isolated from the leaf base of Cymbidium orchids. An increase in total enzyme concentrations increased the protoplast yield, whereas excess enzymes resulted in a decrease in protoplast yield and viability. The optimal combination of enzymes was 1.2% (weight/volume, w/v) of cellulose and 0.6% (w/v) of macerozyme, which gave the highest yield (6.97 × 106/g FW) and viability (∼88.49%) of leaf base protoplasts (Figure 3A). Moreover, insufficient digestion time led to incomplete release of protoplasts, whereas excess incubation resulted in the rupture of protoplasts. With optimal D-mannitol concentration and enzyme combination, the highest protoplast yield (6.40 × 106/g FW) and viability (∼92.76%) were obtained after 6 h of enzymatic digestion (Figure 3B). Further optimization revealed that the addition of 2-mercaptoethanol contributed to decreased calcium oxalate raphides and increased yield and viability of protoplasts. Given the combination of optimal D-mannitol concentration (0.4–0.6 M), enzyme mixture (1.2% cellulose and 0.6% macerozyme), digestion time (6 h), and 2-mercaptoethanol (5 μM), the highest Cymbidium protoplast yield (∼2.50 × 107/g FW) and viability (∼92.09%) were achieved (Figure 3C).
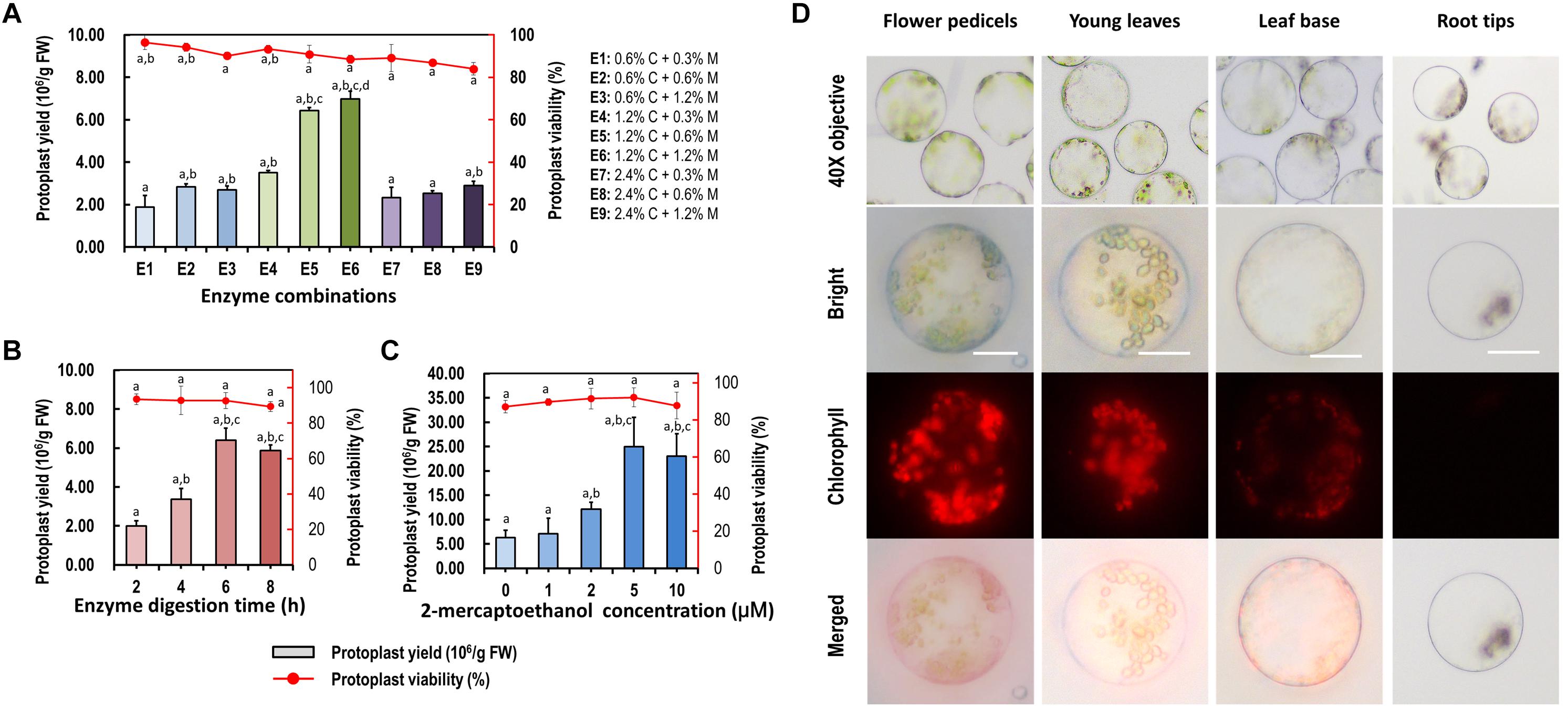
Figure 3. Optimization of Cymbidium protoplast isolation conditions. Factors including (A) enzyme combination, (B) enzyme digestion time, and (C) 2-mercaptoethanol concentration were investigated to optimize the yield and viability of protoplasts isolated from the Cymbidium leaf base; data presented as means of three biological replicates with error bars indicating standard deviations (SD), and different letters (a–e) among treatments indicate statically significant differences at p < 0.05 based on Duncan’s multiple range test. (D) Protoplasts were isolated from Cymbidium flower pedicels, young leaves, leaf base, and root tips. Protoplasts were photographed using an LSM 710 confocal laser microscope with green excitation block; Bar, 20 μm.
With this protocol, higher yields of viable protoplasts were isolated form young leaves (∼3.22 × 106/g FW), flower pedicels (∼5.26 × 106/g FW), and young root tips (∼7.66 × 105/g FW) (Figure 3D). The isolated protoplasts ranged from 20 to 100 μm in diameter, and a large proportion of protoplasts isolated from the young leaves and flower pedicels were rich in cytoplasm and anthocyanidin. Protoplasts isolated from the leaf base and root tips were almost translucent with few chloroplast auto-fluorescent signals. Therefore, our protoplast isolation protocol is suitable for investigating intracellular processes in tissue- and organ-specific protoplasts of Cymbidium orchids.
Suitability of the Leaf Base Protoplast Isolation Protocol for Other Orchids and Monocot Crops
To test the suitability of our protoplast isolation protocol for other species, leaf base tissues collected from Phalaenopsis, Paphiopedilum, Dendrobium, and Arundina orchids were tested with different D-mannitol concentrations (0.2–0.7 M) in enzyme solution (Figure 4A). High yielding protoplasts was obtained from these orchids, and optimal D-mannitol concentrations were determined to be 0.4–0.6 M (Figure 4B). The maximum yields of viable protoplasts were isolated from Phalaenopsis (1.83 × 107/g FW) and Paphiopedilum (1.10 × 107/g FW), which were higher than those of Dendrobium (8.21 × 106/g FW) and Arundina (3.78 × 106/g FW) (Figure 4B). Protoplasts isolated from Phalaenopsis and Paphiopedilum (ranged from 40 to 100 μm in diameter) were larger than those isolated from Dendrobium and Arundina (approximately 20–60 μm in diameter) (Figure 4C). Thus, our protocol exhibits broad suitability in efficient protoplast isolation for orchids exhibiting distinct morphological characteristics.
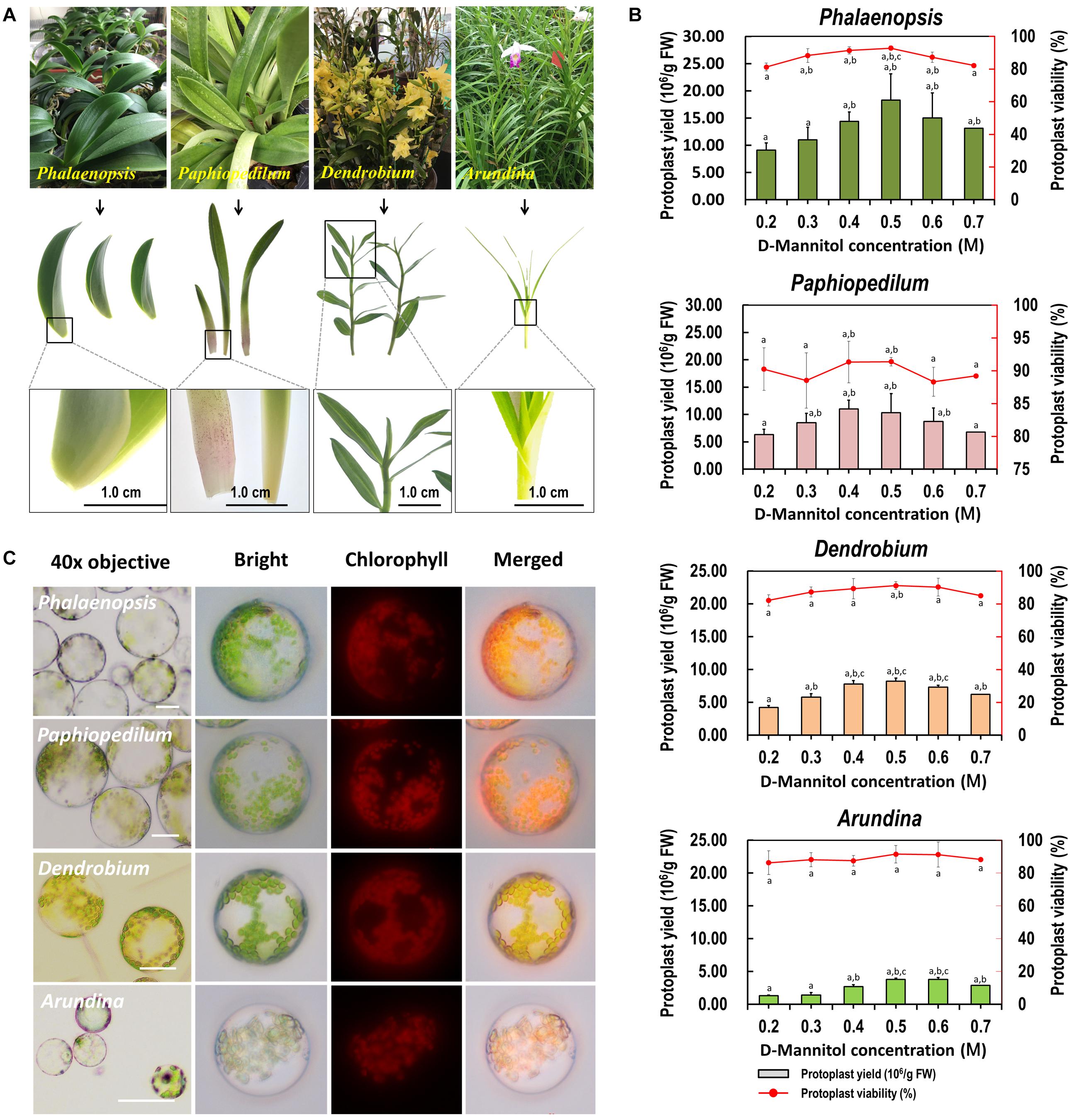
Figure 4. Suitability of the optimized protoplast isolation protocol for other orchid species. (A) The leaf base of Phalaenopsis and Paphiopedilum, and young leaves of Dendrobium and Arundina were collected for orchid protoplast isolation. (B) Different D-mannitol concentrations in the enzyme solutions (0.2, 0.3, 0.4, 0.5, 0.6, and 0.7 M) were established for different orchid species, data presented as means of three biological replicates with error bars indicating the SD values, and different letters (a–e) among treatments indicate statically significant differences at p < 0.05 based on Duncan’s multiple range test. (C) Protoplasts were isolated from the leaf base of Phalaenopsis and Paphiopedilum, and from the young leaves of Dendrobium and Arundina orchids. Protoplasts were photographed using an LSM 710 confocal laser microscope with green excitation block. Bar, 20 μm.
Sharing similar anatomical characteristics with orchids, economically important monocot crops maize and rice were also tested for the suitability of our protoplast isolation protocol. Leaf base tissues and young leaves were all used, and leaf base protoplast isolation method showed marked advantages over previous mesophyll protoplast isolation protocols (Table 1). High yielding viable protoplasts were released from the leaf base of maize (3.25 × 107/g FW) and rice (4.31 × 107/g FW), and their optimal D-mannitol concentrations were both determined to be 0.5 M (Figure 5). The leaf base protoplasts isolated from maize (ranging from 20 to 60 μm in diameter) were smaller than those of rice (20–90 μm) (Supplementary Figure 3). The majority of rice protoplasts were transparent and highly viable. The efficiency of protoplast isolation from the leaf base tissues was higher than that of the leaves (Figure 5 and Table 1). It indicated that our leaf base protoplast isolation protocol enables highly efficient protoplast isolation from many other monocot plants.
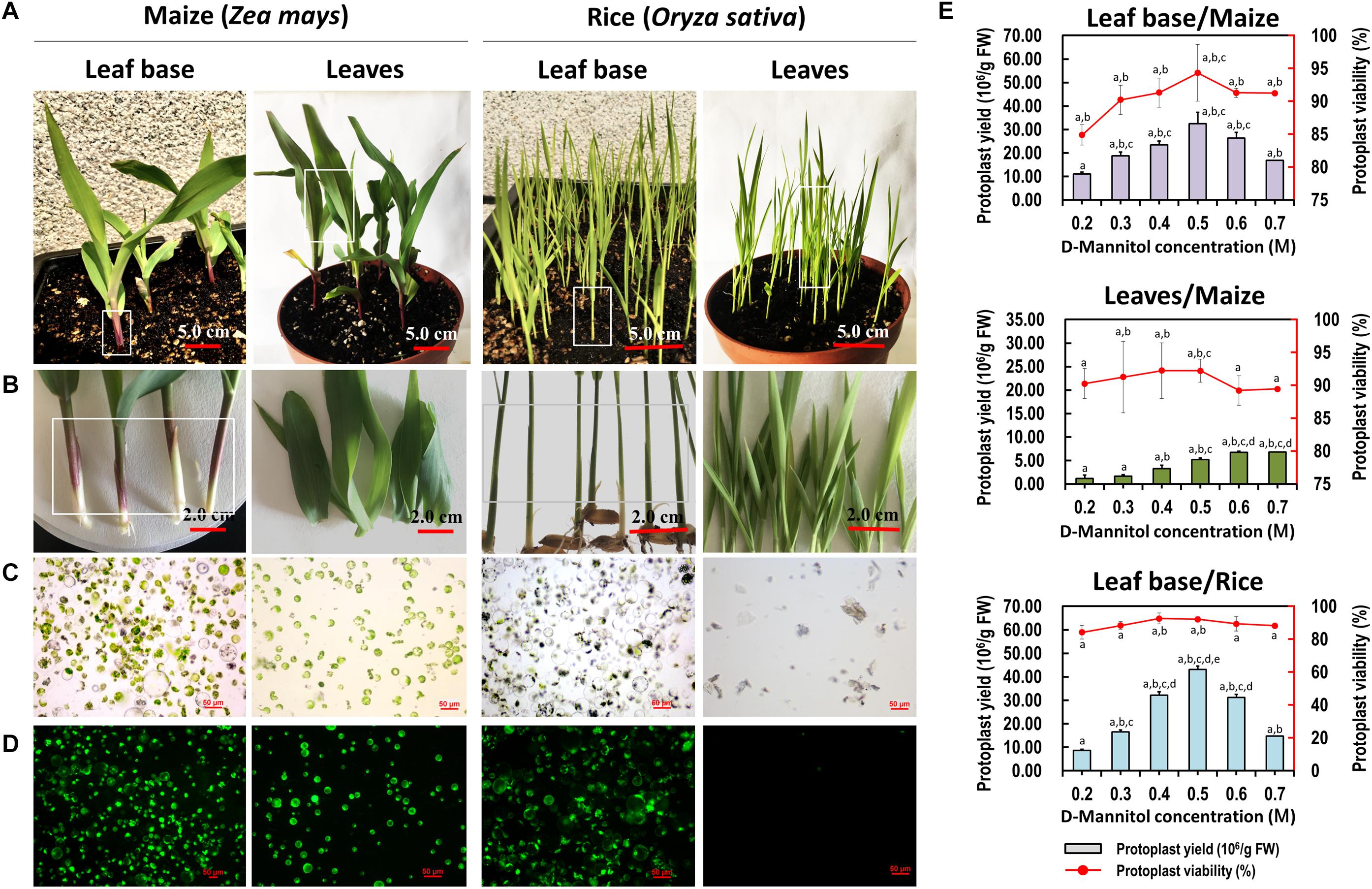
Figure 5. Protoplasts isolated from the leaf base and young leaves of maize and rice. (A) Ten-days-old maize and rice seedlings were used for protoplast isolation. (B) The leaf base and young leaves of maize and rice were collected for protoplast isolation, respectively. (C) Protoplasts were successfully isolated from the leaf base of maize and rice, which was better than that of isolated from the young leaves. (D) Viability test of protoplasts isolated from maize and rice using FDA staining. (E) Different D-mannitol concentrations in the enzyme solutions (0.2, 0.3, 0.4, 0.5, 0.6, and 0.7 M) were established for protoplast isolation. Data presented as means of three biological replicates with error bars indicating the SD values, and different letters (a–e) among treatments indicate statically significant differences at p < 0.05 based on Duncan’s multiple range test.
PEG-Mediated Transient Expression and Protein Subcellular Localization
To test the feasibility of the leaf base protoplasts for gene transient expression, PEG-mediated Cymbidium protoplast transfection was performed using the vector pAN580-GFP. According to the GFP expression in the transfected protoplasts, a maximum transfection efficiency of more than 80% was obtained by optimizing factors affecting PEG-mediated transfection, including incubation time, final PEG4000 concentration, and amount of plasmid DNA (Figure 6A). In addition, most of the transfected protoplasts maintained integrity up to 36 hpt. These results indicate that leaf base protoplasts of orchids are suitable for transient gene/protein expression.
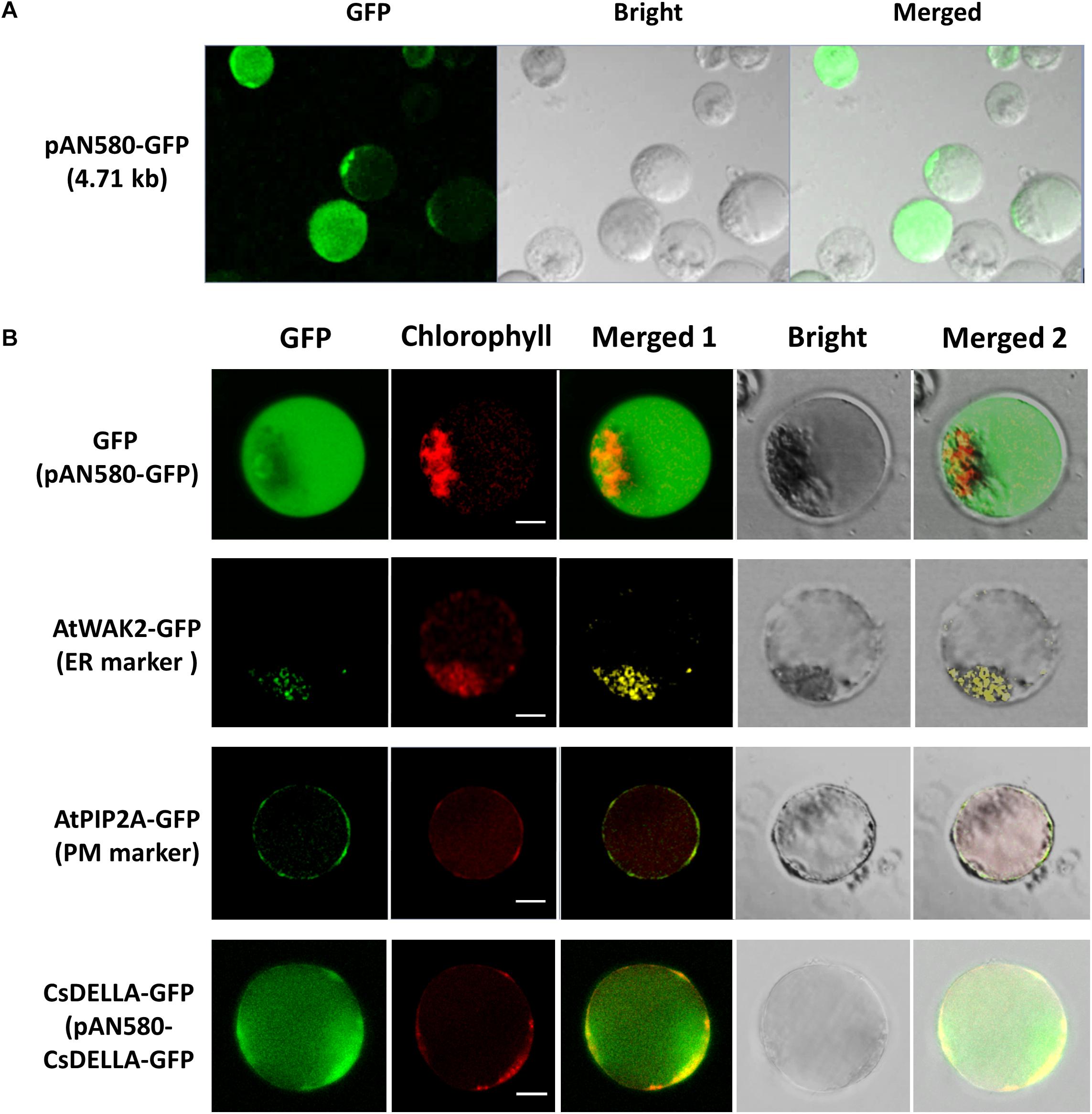
Figure 6. Transient expression and protein subcellular localization studies using Cymbidium leaf base protoplasts. (A) Transient expression of GFP in the Cymbidium leaf base protoplasts transfected with empty control vector pAN580-GFP (expressing GFP). (B) Empty control vector, endoplasmic reticulum marker AtWAK2-GFP, plasma membrane marker AtPIP2A-GFP, and CsDELLA-GFP vectors were transformed into Cymbidium protoplasts to test the feasibility of the PTES for protein subcellular localization. Bar, 10 μm.
The efficient transient expression system established in the present study was tested further for the feasibility of the subcellular localization of proteins. Empty control vector pAN580-GFP, the two plant intercellular organelle markers (pGreenII62-SK-AtWAK2-GFP and pGreenII62-SK-AtPIP2A-GFP), and the recombined vector pAN580-CsDELLA-GFP were transfected into Cymbidium leaf base protoplasts, respectively. A total of 12–16 h later, green fluorescence was observed in the intracellular compartments of the transfected protoplasts. Fusion proteins AtWAK2-GFP and AtPIP2A-GFP were detected specifically in the endoplasmic reticulum and plasma membrane, respectively, whereas the fusion protein CsDELLA-GFP and vector control were distributed throughout the entire cell (Figure 6B). Thus, the leaf base protoplasts are suitable for molecular and cellular characterization of genes/proteins.
The Protoplast System Enables Cellular and Molecular Investigations in Cymbidium Orchids
To test the feasibility of the Cymbidium protoplast system in cellular and molecular studies, functional identification of CsDELLA was conducted in Cymbidium protoplast. As DELLA-mediated GA signaling is known to affect flowering (Yamaguchi et al., 2014; Bao et al., 2020), we analyzed the role of CsDELLA in the regulation of GA to flowering-related genes. To determine the optimum concentration, the Cymbidium protoplasts were treated with different concentrations of GA3 (1, 10, and 100 μM), and the expression of CsDELLA in Cymbidium protoplasts was examined by qRT-PCR. CsDELLA expression was suppressed by the addition of GA3, and 10 μM GA3 was the best concentration for protoplast treatment (Figure 7A). To determine the reliability and repeatability, the viability of protoplasts post the treatment was estimated with FDA staining, and most protoplasts remained viable up to 24 hpt (Figure 7B).
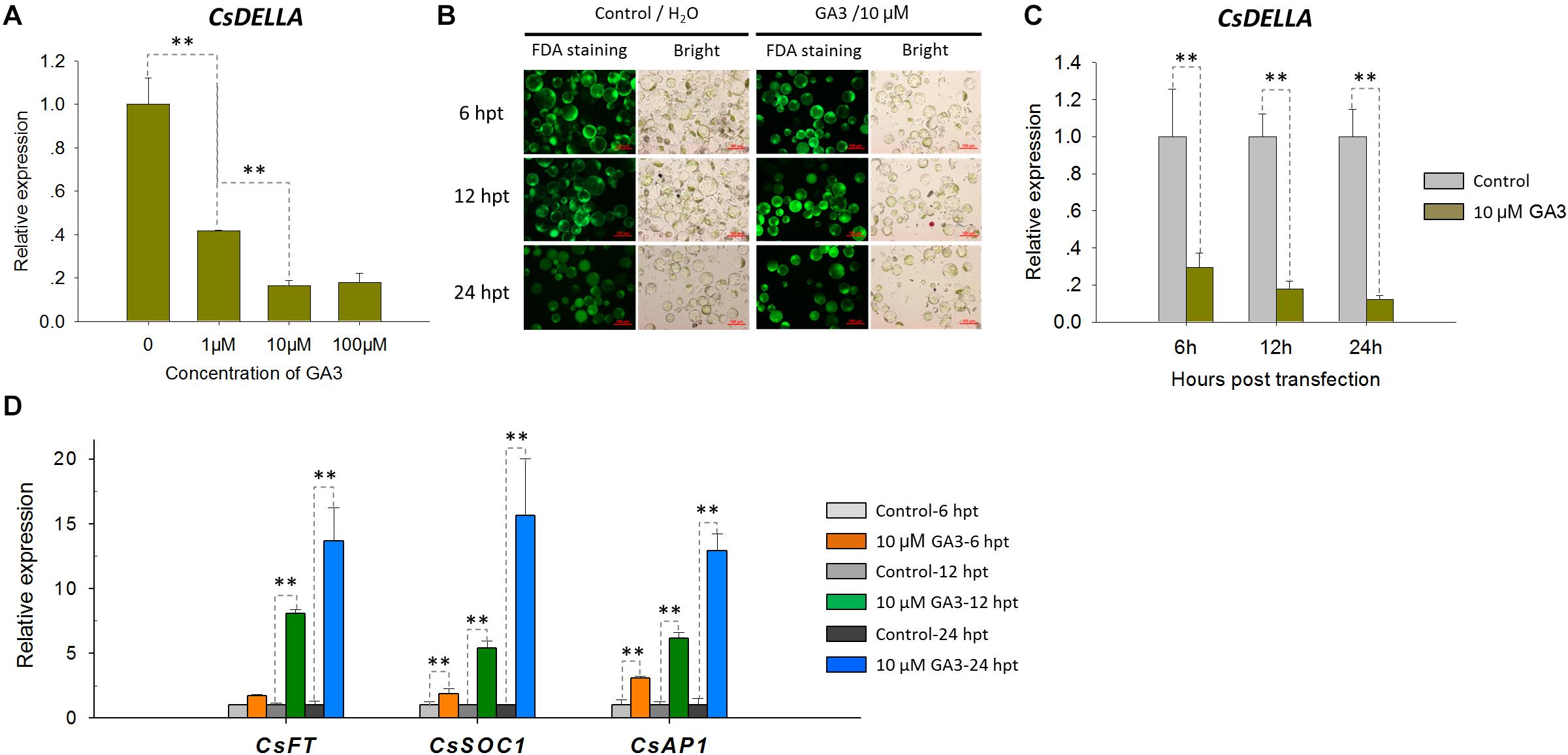
Figure 7. Expression analysis of CsDELLA, CsFT, CsSOC1, and CsAP1 genes in response to GA3 induction with qRT-PCR. (A) Relative expression levels of the CsDELLA gene induced by different concentrations of GA3 were measured with qRT-PCR. (B) Viability test of Cymbidium protoplasts treated with GA3 using FDA staining. (C) Relative expression levels of the CsDELLA gene induced by 10 μM GA3 were measured by qRT-PCR. (D) Relative expression levels of CsFT, CsSOC1, and CsAP1 genes in response to GA3 induction by qRT-PCR. Y-axes indicate the relative expression levels; significant difference was assessed by Mann–Whitney U-test and indicated by asterisks; single asterisk (*) represents p ≤ 0.05, double asterisk (**) represents p ≤ 0.01; data are expressed as the mean of three biological replicates, with error bars indicating the SD values.
Protoplasts treated with 10 μM GA3 and H2O control were collected at 6, 12, and 24 hpt, and the expressions of CsDELLA and flowering-related genes were analyzed by qRT-PCR. It was resulted that CsDELLA expression was significantly downregulated 3–6-fold from 12 to 24 hpt in the presence of 10 μM GA3 compared with controls at each time point (Figure 7C). However, the three key flowering-related genes CsFT, CsSOC1, and CsAP1 were all significantly upregulated 1.3–15-fold from 12 to 24 hpt in response to 10 μM GA3 (Figure 7D). Whether GA promotes the expression of flowering related genes by suppressing the expression of CsDELLA remains unclear, and the role of CsDELLA in the regulation of GA to flowering-related genes needs further investigation. These results indicate the potential of our protoplast system for investigating cellular and molecular behaviors in response to phytohormones and other inducing factors.
Efficient Transient Overexpression and RNA Interference for Gene Regulation Analysis in Cymbidium Protoplasts
To analyze the regulation of CsDELLA to flowering-related genes, CsDELLA was transiently over-expressed or knocked-down in Cymbidium protoplasts. The expression of flowering-related genes was examined with qRT-PCR. The recombinant plasmid pAN580-CsDELLA was transfected into Cymbidium protoplasts, and CsDELLA was successfully overexpressed 90–350-fold from 12 to 24 hpt (Figure 8A). The expressions of CsSOC1 and LEAFY (CsLFY) were significantly downregulated 5–10-fold from 12 to 24 hpt, while those of CsFT, CsSVP3, and CsAP1 were slightly downregulated. It indicated that the transient overexpression of CsDELLA suppressed the expression of CsSOC1 and CsLFY (Figure 8B).
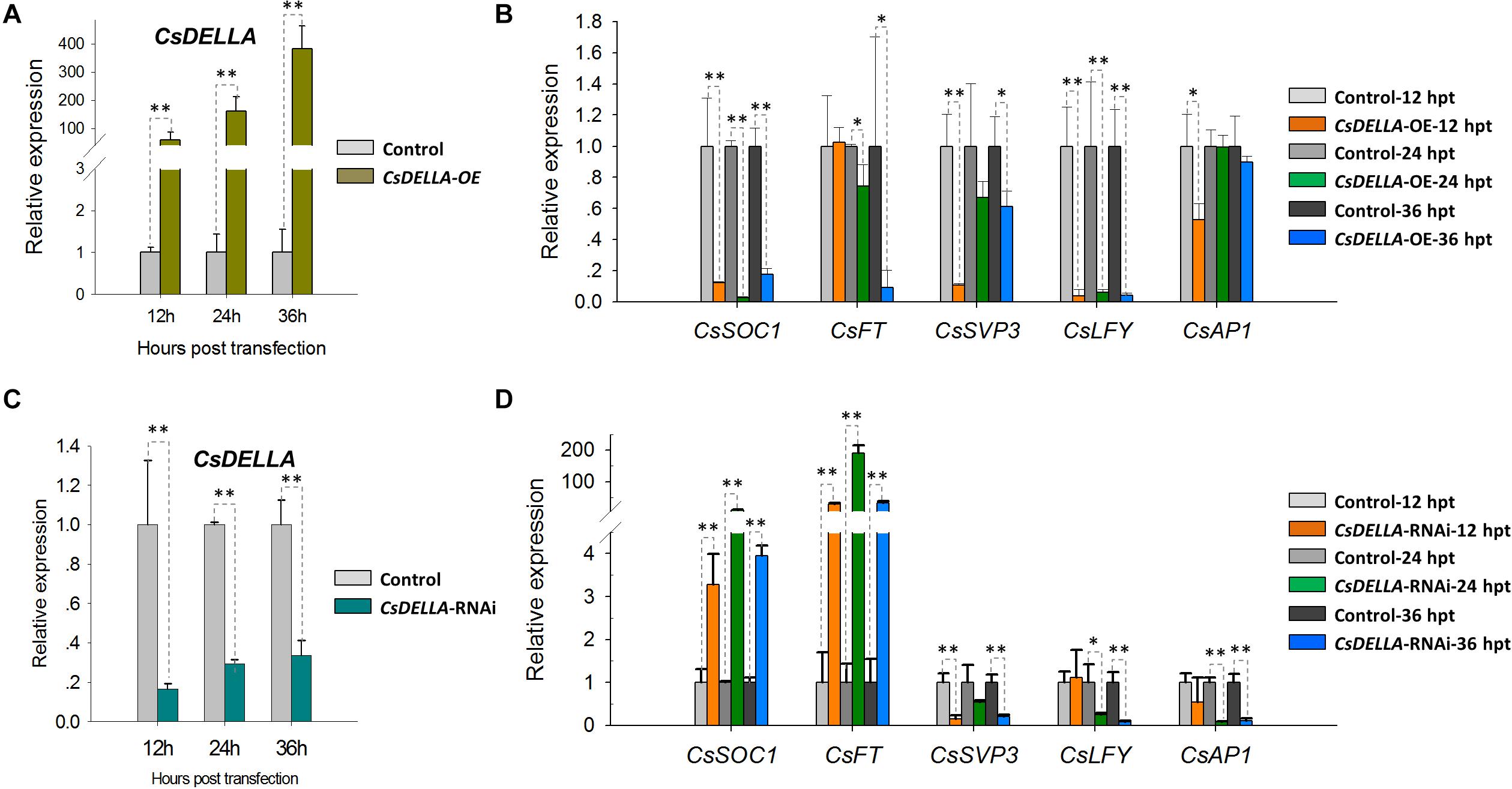
Figure 8. Regulation analysis of CsDELLA protein to the expression of flowering-related genes with qRT-PCR. (A) CsDELLA were transiently overexpressed in Cymbidium protoplasts and (B) the expression of CsSOC1, CsFT, CsSVP3, CsLFY, and CsAP1 genes at 12, 24, and 36 hpt were examined with qRT-PCR. (C) CsDELLA expression was downregulated using RNAi and (D) the expression of CsSOC1, CsFT, CsSVP3, CsLFY, and CsAP1 genes in the transfected protoplasts were examined with qRT-PCR. Y-axes indicate the relative expression levels; significant difference was assessed by Mann–Whitney U-test and indicated by asterisks; single asterisk (*) represents p ≤ 0.05, double asterisk (**) represents p ≤ 0.01. Data are expressed as the mean of three biological replicates, with error bars indicating the SD values.
To identify the regulation relationships, CsDELLA was silenced with the RNA interference (RNAi) technology. The 243-bp CsDELLA-specific sequence was inserted into the RNAi vector pTCK303, and the vector pTCK303-CsDELLA-RNAi was transfected into Cymbidium protoplasts. It was resulted that the vector pTCK303-CsDELLA-RNAi (approximately 14.8 kb) was expressed in Cymbidium protoplasts, and CsDELLA was silenced to almost 70–80% (Figure 8C). The expression of CsSOC1 was significantly upregulated 3–30-fold, and those of CsFT was greatly upregulated 50–160-fold from 12 to 24 hpt. However, CsSVP, CsLFY, and CsAP1 were significantly downregulated (Figure 8D). These results indicated that silenced CsDELLA promoted the expression of CsSOC1 and CsFT, CsDELLA probably positively regulates the expression of CsSOC1 and CsFT. Our leaf base protoplast transfection system was confirmed to conveniently analyze gene expression regulation relationships, and to screen efficient vectors before genetic transformation.
Discussion
Orchids are important ornamental and medicinal plants that are cultivated worldwide. Orchid biologists have placed great effort in protoplast, meristem, and tissue culturing for virus-free, hybrid, and rapid propagation of orchids (Lim et al., 1993; Shrestha et al., 2007; Yam and Arditti, 2009; Panattoni et al., 2013). With the release of Phalaenopsis (Cai et al., 2015), Dendrobium (Zhang et al., 2016a), and Apostasia (Zhang et al., 2017) whole genome sequences, highly efficient measures for rapid functional genomic studies are required. However, stable transgenes in orchids remain challenging because of their long-term growth cycles and difficulties in callus induction and plant regeneration (Kobayashi et al., 1993; Yam and Arditti, 2009). Accordingly, versatile protoplast systems have provoked attention. Herein, we performed a comprehensive parallel study to compare various tissues/organs of different orchid species as source materials for protoplast isolation and transfection.
Although appropriate optimizations have been determined for higher yield and viability of protoplasts isolated from different tissues of numerous species, the basic procedures for protoplast isolation have undergone little change since the first report (Nagata and Takebe, 1971; Kanai and Edwards, 1973; Sheen, 2001; Chen et al., 2006; Yoo et al., 2007). Source material is the most critical factor affecting protoplast release (Jeon et al., 2007; Pindel, 2007; Yoo et al., 2007; Zhang et al., 2016b). Various tissues of in vitro grown plantlets, including leaf tissues, flower pedicel, and column (Pindel, 2007), and ex vitro grown leaves and flower petals (Nanjareddy et al., 2016) as well as suspension cultured cells (Mazarei et al., 2008; Wang et al., 2015; Yao et al., 2016) have been used, and protoplasts have been isolated from various plant species (Table 1). In the present study, protoplasts were successfully isolated from various ex vitro tissues of orchids via the selection of suitable source materials and the optimization of enzymatic conditions. Using the improved method based on past reliable protocols, the tender leaf base gave higher yields (∼2.50 × 107/g FW) compared to young leaves (∼3.22 × 106/g FW), flower pedicels (∼5.26 × 106/g FW), and root tips (∼7.66 × 105/g FW) of Cymbidium orchids under optimal conditions (Figure 2). In addition to high yielding protoplasts isolated from Cymbidium flower petals (3.3 × 107/g FW) (Ren et al., 2020), we successfully obtained sufficient tissue- and organ-specific protoplasts. Because orchids bloom only once a year and flowering lasts less than 1 month, the leaf base is the most suitable source material as it is available all year round for the isolation of high yielding viable orchid protoplasts. Moreover, leaf base protoplasts are of long-term health, which was mostly on non-differentiated stem cells. Since the ultimate goal of a protoplast isolation and transfection system is for plant regeneration, the totipotency of leaf base protoplast improved their probability of developing into mature plants.
The major factors affecting protoplast preparation are osmotic pressure, enzymatic concentration, and their combination in the enzyme solution, as well as the enzymolysis time (Kantharajah and Dodd, 1990; Davey et al., 2005). Herein, a concentration gradient of D-mannitol was used. Appropriate D-mannitol concentration can maintain the balance of interior and exterior osmotic pressures of protoplasts to prevent them from rupture or collapse (Huang et al., 2013). The optimum values for various tissues differed (Figure 2). Additionally, concentration gradients of cellulose-R10 combined with macerozyme-R10 were included. The increase in total enzyme concentrations resulted in increased protoplast yield and viability, whereas excess enzymes led to the phytotoxicity of enzymes on the membrane of protoplasts (Zhu et al., 1997; Raikar et al., 2008). The optimum concentrations of cellulose-R10 combined with macerozyme-R10 were determined to be 1.2% (w/v) and 0.6% (w/v), respectively (Table 1). Abundant calcium oxalate crystals accumulated in the ruptured protoplasts, which could puncture other protoplasts (Pindel, 2007; Lin et al., 2018b), and were observed in the protoplast solution (Supplementary Figure 1). Peroxides released by the ruptured protoplasts could also damage the protoplasts (de Marco and Roubelakis-Angelakis, 1996; Yasuda et al., 2007). To address these problems and protect protoplasts from rupturing, a suitable concentration of the reducing agent 2-mercaptoethanol was added to the enzyme solution. The protoplast yields markedly increased with the addition of 2-mercaptoethanol (Figure 3C). With this optimized protocol, high yielding viable protoplasts were obtained from the leaf base tissues of Phalaenopsis (1.83 × 107/g FW) and Paphiopedilum (1.10 × 107/g FW) (Figure 4) orchids, and other economically important monocot crops, including maize (3.25 × 107/g FW) and rice (4.31 × 107/g FW) (Figure 5). The isolation efficiency was higher or comparable to that of previous results (Table 1). This indicated the wide application of this protoplast isolation protocol for other orchids and monocot crops.
Protoplast-based transient expression system enables the molecular, cellular, and functional identification of genes/proteins in plants (Lin et al., 2018b). The emerging advantages of PTES have led to establishing a robust transient expression system using the leaf base protoplasts of Cymbidium orchids. Protoplast transfection efficiency greater than 50% is required for reliable and reproducible experimental data (Yoo et al., 2007). Hence, plasmid NDA expressing GFP was transfected into the protoplasts using a PEG-mediated method. Factors affecting PEG-mediated transfection, including incubation time, final PEG4000 concentration, and amount of plasmid DNA were optimized (Huang et al., 2013; Li et al., 2018; Page et al., 2019). A maximum transfection efficiency greater than 80% was obtained (Figure 6A), which is equivalent to or greater than that reported previously (Wu et al., 2017; Li et al., 2018; Lin et al., 2018b). Although most of the transfected protoplasts were almost translucent with few auto-fluorescent signals (Figure 3D), this PTES was successfully used for protein subcellular localization (Figure 6C). Moreover, we analyzed the induction of GA3 to the expression of CsDELLA and flowering-related genes in Cymbidium protoplasts (Figure 7). Moreover, we found that transient overexpression of CsDELLA significantly downregulated the expression of CsSOC1, CsFT, and other flowering-related genes (Figure 8A), whereas the silencing of CsDELLA resulted in the upregulated expression of CsSOC1 and CsFT (Figure 8B). Thus, GA3 promotes the expression of flowering-related genes by suppressing CsDELLA expression (Supplementary Figure 4). Taken together, the leaf base protoplast isolation and transfection system described in the present study is robust, reliable, and sustainable for molecular and cellular characterization of genes/proteins.
Data Availability Statement
The original contributions presented in the study are included in the article/Supplementary Material, further inquiries can be directed to the corresponding author/s.
Author Contributions
RR and JG carried out all the experiments and drafted the manuscript. CL, YW, and JJ contributed to the data analyses. DY, KL, SA, GZ, and FY were involved in co-ordination and supervision of the work and review of the manuscript. All authors have read and agreed to the published version of the manuscript.
Funding
This work was supported by grants from National natural science foundation of China (31872151 and 31672184), National Key Technologies R&D Program (2019YFD1001003 and 2018YFD1000404), R&D plan in key areas of Guangdong Province (2018B020202001), Natural Science Foundation of Guangdong Province (2017A030312004), Guangdong Modern Agricultural Industrial Technology System Flower Innovation Team Expert Project (2019KJ121), Guangzhou Scientific Research Project (201904020026 and 201707010307), and Guangdong Academy of Agricultural Sciences Project (201612TD, R2016PY-QF015, and 2017A070702008).
Conflict of Interest
The authors declare that the research was conducted in the absence of any commercial or financial relationships that could be construed as a potential conflict of interest.
Acknowledgments
We thank Sek-Man Wong of National University of Singapore for his critical review of this manuscript and Liang G. from Xishuangbanna Tropical Botanical Garden, Chinese Academy of Sciences for giving us the marker vectors.
Supplementary Material
The Supplementary Material for this article can be found online at: https://www.frontiersin.org/articles/10.3389/fpls.2021.626015/full#supplementary-material
Supplementary Figure 1 | Schematic of recombined vectors. (A) Schematic of GFP control, endoplasmic reticulum marker AtWAK2-GFP, plasma membrane marker AtPIP2A-GFP. (B) Schematic of recombined vector expressing CsDELLA-GFP fusion protein for subcellular localization analysis, and CsDELLA protein for transient over-expression (OE). (C) Schematic of the pTCK303-CsDELLA-RNAi vector construction.
Supplementary Figure 2 | Protoplasts isolated from the young leaves of Cymbidium orchids. Most of the protoplasts maintained integrity not only at (A) 3 h post releasing, but also at (B) 24 h post isolation. Arrows indicated the of calcium oxalate raphides.
Supplementary Figure 3 | Cellular morphology comparison of protoplasts isolated from maize and rice. Bar, 20 μm.
Supplementary Figure 4 | Conserved putative genetic regulatory networks of flowering in plants. Genes are represented by ovals. Lines with an arrow represent promotion of mRNA transcript, and those with a perpendicular bar represent repression. Red lines indicate regulation analyzed in this study.
Supplementary Table 1 | Primer sequences used in this study.
References
Aqeel, R., Zehra, M., Kazmi, S., Khan, S., Kayani, H., and Mirbahar, A. (2016). A study on the isolation of protoplasts from mesophyll cells of Dendrobium queen pink. Pak. J. Bot. 48, 693–697. doi: 10.1186/s13007-016-0142-6
Bao, S., Hua, C., Shen, L., and Yu, H. (2020). New insights into gibberellin signaling in regulating flowering in Arabidopsis. J. Integr. Plant Biol. 62, 118–131. doi: 10.1111/jipb.12892
Bart, R., Chern, M., Park, C. J., Bartley, L., and Ronald, P. C. (2006). A novel system for gene silencing using siRNAs in rice leaf and stem-derived protoplasts. Plant Methods 2:13. doi: 10.1186/1746-4811-2-13
Borochov, A., Halevy, A. H., and Shinitzky, M. (1976). Increase in microviscosity with ageing in protoplast plasmalemma of rose petals. Nature 263, 158–159. doi: 10.1186/1746-4811-2-13
Cai, J., Liu, X., Vanneste, K., Proost, S., Tsai, W. C., Liu, K. W., et al. (2015). The genome sequence of the orchid Phalaenopsis equestris. Nat. Genet. 47, 65–72. doi: 10.1038/ng.3149
Cao, J., Yao, D., Lin, F., and Jiang, M. (2014). PEG-mediated transient gene expression and silencing system in maize mesophyll protoplasts: a valuable tool for signal transduction study in maize. Acta Physiol. Plant 36, 1271–1281. doi: 10.1007/s11738-014-1508-x
Capesius, I., and Meyer, Y. (1977). Isolation of nuclei from protoplasts of orchids. Cytobiologie 15, 485–490.
Chen, S., Tao, L., Zeng, L., Vega-Sanchez, M. E., Umemura, K., and Wang, G. (2006). A highly efficient transient protoplast system for analyzing defence gene expression and protein-protein interactions in rice. Mol. Plant Path. 7, 417–427. doi: 10.1111/j.1364-3703.2006.00346.x
Cutler, S. R., Ehrhardt, D. W., Griffitts, J. S., and Somerville, C. R. (2000). Random, GFP::cDNA fusions enable visualization of subcellular structures in cells of Arabidopsis at a high frequency. Proc. Natl. Acad. Sci. U.S.A. 97, 3718–3723. doi: 10.1073/pnas.97.7.3718
Davey, M. R., Anthony, P., Power, J. B., and Lowe, K. C. (2005). Plant protoplast technology: current status. Acta Physiol. Plant 27, 117–130. doi: 10.1007/s11738-005-0044-0
de Marco, A., and Roubelakis-Angelakis, K. A. (1996). The complexity of enzymic control of hydrogen peroxide concentration may affect the regeneration potential of plant protoplasts. Plant Physiol. 110, 137–145. doi: 10.2307/4276973
Denyer, T., Ma, X., Klesen, S., Scacchi, E., Nieselt, K., and Timmermans, M. C. (2019). Spatiotemporal developmental trajectories in the Arabidopsis root revealed using high-throughput single-cell RNA sequencing. Dev. Cell 48, 840–852. doi: 10.2139/ssrn.3300042
Duarte, P., Ribeiro, D., Carqueijeiro, I., Bettencourt, S., and Sottomayor, M. (2016). “Protoplast transformation as a plant-transferable transient expression system,” in Biotechnology of Plant Secondary Metabolism, ed. A. G. Fett-Neto (New York, NY: Humana Press), 137–148. doi: 10.1007/978-1-4939-3393-8_13
Eeckhaut, T., Lakshmanan, P. S., Deryckere, D., Van Bockstaele, E., and Van Huylenbroeck, J. (2013). Progress in plant protoplast research. Planta 238, 991–1003. doi: 10.1111/j.1399-3054.1992.tb04754.x
Gomord, V., Denmat, L. A., Fitchette-Lainé, A. C., Satiat-Jeunemaitre, B., Hawes, C., and Faye, L. (1997). The C-terminal HDEL sequence is sufficient for retention of secretory proteins in the endoplasmic reticulum (ER) but promotes vacuolar targeting of proteins that escape the ER. Plant J. 11, 313–325. doi: 10.1046/j.1365-313X.1997.11020313.x
Goodman, C. D., Casati, P., and Walbot, V. (2004). A multidrug resistance-associated protein involved in anthocyanin transport in Zea mays. Plant Cell 16, 1812–1826. doi: 10.1105/tpc.022574
Grosser, J. W., and Gmitter, J. F. G. (1990). Protoplast fusion and citrus improvement. Plant Breed. Rev. 8, 339–374. doi: 10.1002/9781118061053.ch10
Guo, J., Morrell-Falvey, J. L., Labbé, J. L., Muchero, W., Kalluri, U. C., Tuskan, G. A., et al. (2012). Highly efficient isolation of Populus mesophyll protoplasts and its application in transient expression assays. PLoS One 7:e44908. doi: 10.1371/journal.pone.0044908
Hauptmann, R. M., Ozias-Akins, P., Vasil, V., Tabaeizadeh, Z., Rogers, S. G., Horsch, R. B., et al. (1987). Transient expression of electroporated DNA in monocot and dicotyledonous species. Plant Cell Rep. 6, 265–270. doi: 10.1007/BF00271995
Hayashimoto, A., Li, Z., and Murai, N. (1990). A polyethylene glycol-mediated protoplast transformation system for production of fertile transgenic rice plants. Plant Physiol. 93, 857–863. doi: 10.1104/pp.93.3.857
Hirata, H., Ohnishi, T., Ishida, H., Tomida, K., Sakai, M., and Hara, M. (2012). Functional characterization of aromatic amino acid aminotransferase involved in 2-phenylethanol biosynthesis in isolated rose petal protoplasts. J. Plant Physiol. 169, 444–451. doi: 10.1016/j.jplph.2011.12.005
Hsiao, Y., Tsai, W., Kuoh, C., Huang, T., Wang, H., Wu, T., et al. (2006). Comparison of transcripts in Phalaenopsis bellina and Phalaenopsis equestris (Orchidaceae) flowers to deduce monoterpene biosynthesis pathway. BMC Plant Biol. 6:14. doi: 10.1186/1471-2229-6-14
Hu, W. W., Wong, S. M., Loh, C. S., and Goh, C. J. (1998). Synergism in replication of cymbidium mosaic potexvirus (CymMV) and odontoglossum ringspot tobamovirus (ORSV) RNA in orchid protoplasts. Arch. Virol. 143, 1265–1275. doi: 10.1007/s007050050374
Huang, H., Wang, Z., Cheng, J., Zhao, W., Li, X., Wang, H., et al. (2013). An efficient cucumber (Cucumis sativus L.) protoplast isolation and transient expression system. Sci. Hortic. Amsterdam 150, 206–212. doi: 10.1016/j.scienta.2012.11.011
Huo, A., Chen, Z., Wang, P., Yang, L., Wang, G., Wang, D. et al. (2017). Establishment of transient gene expression systems in protoplasts from Liriodendron hybrid mesophyll cells. Plos One 12:e0172475. doi: 10.1371/journal.pone.0172475
Jeon, J. M., Ahn, N. Y., Son, B. H., Kim, C. Y., Han, C., Kim, G. D., et al. (2007). Efficient transient expression and transformation of PEG-mediated gene uptake into mesophyll protoplasts of pepper (Capsicum annuum L.). Plant Cell Tiss. Org. Cult. 88, 225–232. doi: 10.1007/s11240-006-9194-z
Jia, N., Zhu, Y., and Xie, F. (2018). An efficient protocol for model legume root protoplast isolation and transformation. Front. Plant Sci. 9:670. doi: 10.3389/fpls.2018.00670
Jia, X., Zhang, X., Qu, J., and Han, R. (2016). Optimization conditions of wheat mesophyll protoplast isolation. Agricul. Sci. 7, 850–858. doi: 10.4236/as.2016.712077
Kanai, R., and Edwards, G. E. (1973). Separation of mesophyll protoplasts and bundle sheath cells from maize leaves for photosynthetic studies. Plant Physiol. 51, 1133–1137. doi: 10.2307/4263286
Kantharajah, A. S., and Dodd, W. A. (1990). Factors that influence the yield and viability of Cucumber (Cucumis sativus L.) cotyledon protoplasts. Aust. J. Bot. 38, 169–175. doi: 10.1071/BT9900169
Keskitalo, M., Pehu, E., and Simon, J. E. (2001). Variation in volatile compounds from tansy (Tanacetum vulgare L.) related to genetic and morphological differences of genotypes. Biochem. Syst. Ecol. 29, 267–285. doi: 10.1016/S0305-1978(00)00056
Khentry, Y., Paradornuvat, A., Tantiwiwat, S., Phansiri, S., and Thaveechai, N. (2006). Protoplast isolation and culture of Dendrobium Sonia “Bom 17”. Kasetsart J. (Nat. Sci.) 40, 361–369.
Kobayashi, S., Kameya, T., and Ichihashi, S. (1993). Plant regeneration from protoplasts derived from callus of Phalaenopsis. Plant Tiss. Cult. Lett. 10, 267–270. doi: 10.5511/plantbiotechnology1984.10.267
Krens, F. A., Molendijk, L., Wullems, G. J., and Schilperoort, R. A. (1982). In vitro transformation of plant protoplasts with Ti-plasmid DNA. Nature 296:72. doi: 10.1038/296072a0
Li, J., Liao, X., Zhou, S., Liu, S., Jiang, L., and Wang, G. (2018). Efficient protoplast isolation and transient gene expression system for Phalaenopsis hybrid cultivar ‘Ruili Beauty’. Vitro Cell Dev. Biol. Plant 54, 87–93. doi: 10.1007/s11627-017-9872-z
Lim, S. T., Wong, S. M., and Goh, C. J. (1993). Elimination of Cymbidium mosaic virus and Odontoglossum ringspot virus from orchids by meristem culture and thin section culture with chemotherapy. Ann. Appl. Biol. 122, 289–297. doi: 10.1111/j.1744-7348.1993.tb04034.x
Lin, C., Hsu, C., Yang, L., Lee, L., Fu, J., Cheng, Q., et al. (2018a). Application of protoplast technology to CRISPR/Cas9 mutagenesis: from single-cell mutation detection to mutant plant regeneration. Plant Biotech. J. 16, 1295–1310. doi: 10.1111/pbi.12870
Lin, H., Chen, J., and Fang, S. (2018b). A protoplast transient expression system to enable molecular, cellular, and functional studies in Phalaenopsis orchids. Front. Plant Sci. 9:843. doi: 10.3389/fpls.2018.00843
Machmudi, M., Purnobasuki, H., and Utami, E. S. W. (2019). The optimization mesophyll protoplast isolation for Phalaenopsis amboinensis. Bulgarian J. Agricul. Sci. 25, 737–743.
Masani, M. Y. A., Noll, G. A., Parveez, G. K. A., Sambanthamurthi, R., and Prüfer, D. (2014). Efficient transformation of oil palm protoplasts by PEG-mediated transfection and DNA microinjection. PLoS One 9:e96831. doi: 10.1371/journal.pone.0096831
Mazarei, M., Al-Ahmad, H., Rudis, M. R., and Stewart, J. C. N. (2008). Protoplast isolation and transient gene expression in switchgrass. Panicum virgatum L. Biotech. J. 3, 354–359. doi: 10.1002/biot.200700189
Melchers, G., Sacristán, M. D., and Holder, A. A. (1978). Somatic hybrid plants of potato and tomato regenerated from fused protoplasts. Carlsberg Res. Commun. 43:203. doi: 10.1007/BF02906548
Menczel, L. (1980). Improved protoplast culture and plant regeneration from protoplast-derived callus in Arabidopsis thaliana. Z. Pflanzenphysiol. 96, 77–80. doi: 10.1016/S0044-328X(80)80102-4
Mliki, A., Jardak, R., Reustle, G. M., and Ghorbel, A. (2003). Isolation and culture of leaf protoplasts from Tunisian grapes. OENO One 37, 145–153. doi: 10.1080/0963748031000148718
Nagata, T., and Takebe, I. (1971). Plating of isolated tobacco mesophyll protoplasts on agar medium. Planta 99, 12–20. doi: 10.1007/BF00392116
Nagy, J. I., and Maliga, P. (1976). Callus induction and plant regeneration from mesophyll protoplasts of Nicotiana sylvestris. Z. Pflanzenphysiol. 78, 453–455. doi: 10.1016/S0044-328X(76)80093-1
Nanjareddy, K., Arthikala, M. K., Blanco, L., Arellano, E. S., and Lara, M. (2016). Protoplast isolation, transient transformation of leaf mesophyll protoplasts and improved Agrobacterium-mediated leaf disc infiltration of Phaseolus vulgaris: tools for rapid gene expression analysis. BMC Biotech. 16:53. doi: 10.1186/s12896-016-0283-8
Negrutiu, I., de Brouwer, D., Watts, J. W., Sidorov, V. I., Dirks, R., and Jacobs, M. (1986). Fusion of plant protoplasts: a study using auxotrophic mutants of Nicotiana plumbaginifolia. Viviani. Theor. Appl. Genet. 72, 279–286. doi: 10.1007/BF00267005
Negrutiu, I., Shillito, R., Potrykus, I., Biasini, G., and Sala, F. (1987). Hybrid genes in the analysis of transformation conditions. Plant Mol. Biol. 8, 363–373. doi: 10.1007/BF00015814
Oh, M. H., and Kim, S. G. (1994). Plant regeneration from petal protoplast culture of Petunia hybrida. Plant Cell Tiss. Org. Cult. 36, 275–283. doi: 10.1007/BF00046084
Page, M. T., Parry, M. A., and Carmo-Silva, E. (2019). A high-throughput transient expression system for rice. Plant Cell Environ. 42, 2057-2064. doi: 10.1111/pce.13542
Panattoni, A., Luvisi, A., and Triolo, E. (2013). Elimination of viruses in plants: twenty years of progress. Span J. Agri. Res. 1, 173–188. doi: 10.1080/08832323.1934.9956380
Petersson, S. V., Lindén, P., Moritz, T., and Ljung, K. (2015). Cell-type specific metabolic profiling of Arabidopsis thaliana protoplasts as a tool for plant systems biology. Metabolomics 11, 1679–1689. doi: 10.1007/s11306-015-0814-7
Pindel, A. (2007). Optimization of isolation conditions of Cymbidium protoplasts. Folia Hort. 19, 79–88.
Priyadarshan, S. V. G. N., Hu, B., Li, W., Ali, H., Jia, H., Zhao, L., et al. (2018). Simple protoplast isolation system for gene expression and protein interaction studies in pineapple (Ananas comosus L.). Plant Methods 14:95. doi: 10.1186/s13007-018-0365-9
Raikar, S. V., Braun, R. H., Bryant, C., Conner, A. J., and Christey, M. C. (2008). Efficient isolation, culture and regeneration of Lotus corniculatus protoplasts. Plant Biotech. Rep. 2:171. doi: 10.1007/s11816-008-0058-3
Ren, R., Gao, J., Lu, C., Wei, Y., Jin, J., Wong, S. M., et al. (2020). Highly Efficient protoplast isolation and transient expression system for functional characterization of flowering related genes in Cymbidium orchids. Int. J. Mol. Sci. 21:2264. doi: 10.3390/ijms21072264
Roberts, D. L., and Dixon, K. W. (2008). Orchids. Curr. Biol. 18, 325–329. doi: 10.1016/j.cub.2008.02.026
Sheen, J. (2001). Signal transduction in maize and Arabidopsis mesophyll protoplasts. Plant Physiol. 127, 1466–1475. doi: 10.1104/pp.010820
Shen, Y., Meng, D., McGrouther, K., Zhang, J., and Cheng, L. (2017). Efficient isolation of Magnolia protoplasts and the application to subcellular localization of MdeHSF1. Plant Methods 13:44. doi: 10.1186/s13007-017-0193-3
Shrestha, B. R., Tokuhara, K., and Mii, M. (2007). Plant regeneration from cell suspension-derived protoplasts of Phalaenopsis. Plant Cell Rep. 26, 719–725. doi: 10.1007/s00299-006-0286-3
Song, J., Sorensen, E. L., and Liang, G. H. (1990). Direct embryogenesis from single mesophyll protoplasts in alfalfa (Medicago sativa L.). Plant Cell Rep. 9, 21–25. doi: 10.1007/BF00232128
Sun, B., Zhang, F., Xiao, N., Jiang, M., Yuan, Q., Xue S., et al. (2018). An efficient mesophyll protoplast isolation, purification and peg-mediated transient gene expression for subcellular localization in Chinese kale. Sci. Hortic. 241, 187–193. doi: 10.1016/j.scienta.2018.07.001
Tan, B., Xu, M., Chen, Y., and Huang, M. (2013). Transient expression for functional gene analysis using Populus protoplasts. Plant Cell Tiss. Organ Cult. 114, 11–18. doi: 10.1007/s11240-013-0299-x
Tang, X., Zheng, X., Qi, Y., Zhang, D., Cheng, Y., Tang, A., et al. (2016). A single transcript CRISPR-Cas9 system for efficient genome editing in plants. Mol. Plant 9, 1088–1091. doi: 10.1016/j.molp.2016.05.001
Teo, C. K. H., and Neumann, K. H. (1978b). The culture of protoplasts isolated from Renantanda Rosalind Cheok. Orchid. Rev. 86, 156–158.
Teo, C. K. H., and Neumann, K. H. (1978a). Gewinnung, Kultur and Fusion von Orchideen protoplasten. Orchidee 29, 90–92.
Toriyama, K., and Hinata, K. (1985). Cell suspension and protoplast culture in rice. Plant Sci. 41, 179–183. doi: 10.1016/0168-9452(85)90086-X
Wagner, G. J. (1979). Content and vacuole/extravacuole distribution of neutral sugars, free amino acids, and anthocyanin in protoplasts. Plant Physiol. 64, 88–93. doi: 10.1104/pp.64.1.88
Wang, H., Wang, W., Zhan, J., Huang, W., and Xu, H. (2015). An efficient peg-mediated transient gene expression system in grape protoplasts and its application in subcellular localization studies of flavonoids biosynthesis enzymes. Sci. Hortic. 191, 82–89. doi: 10.1016/j.scienta.2015.04.039
Wehner, N., Hartmann, L., Ehlert, A., Böttner, S., Oñate-Sánchez, L., and Dröge-Laser, W. (2011). High-throughput protoplast transactivation (PTA) system for the analysis of Arabidopsis transcription factor function. Plant J. 68, 560–569. doi: 10.1111/j.1365-313X.2011.04704.x
Wu, F., Shen, S., Lee, L., Lee, S., Chan, M., and Lin, C. (2009). Tape-Arabidopsis Sandwich-a simpler Arabidopsis protoplast isolation method. Plant Methods 5, 16. doi: 10.1186/1746-4811-5-16
Wu, J., Liu, Q., Geng, X., Li, K., Luo, L., and Liu, J. (2017). Highly efficient mesophyll protoplast isolation and PEG-mediated transient gene expression for rapid and large-scale gene characterization in cassava (Manihot esculenta Crantz). BMC Biotechnol. 17:29. doi: 10.1186/s12896-017-0349-2
Yam, T. W., and Arditti, J. (2009). History of orchid propagation: a mirror of the history of biotechnology. Plant Biotech. Rep. 3:1. doi: 10.1007/s11816-008-0066-3
Yamaguchi, N., Winter, C. M., Wu, M. F., Kanno, Y., Yamaguchi, A., Seo, M., et al. (2014). Gibberellin acts positively then negatively to control onset of flower formation in Arabidopsis. Science 344, 638–641. doi: 10.1126/science.1250498
Yang, F., Zhu, G., Wei, Y., Gao, J., Liang, G., Peng, L., et al. (2019). Low-temperature-induced changes in the transcriptome reveal a major role of CgSVP genes in regulating flowering of Cymbidium goeringii. BMC Genom. 20:53. doi: 10.1186/s12864-019-5425-7
Yao, L., Liao, X., Gan, Z., Peng, X., Wang, P., Li, S., et al. (2016). Protoplast isolation and development of a transient expression system for sweet cherry (Prunus avium L.). Sci. Hortic. Amsterdam 209, 14–21. doi: 10.1016/j.scienta.2016.06.003
Yasuda, K., Watanabe, Y., and Watanabe, M. (2007). Generation of intracellular reactive oxygen species during the isolation of Brassica napus leaf protoplasts. Plant Biotechnol. 24, 361–366. doi: 10.5511/plantbiotechnology.24.361
Yoo, S. D., Cho, Y. H., and Sheen, J. (2007). Arabidopsis mesophyll protoplasts: a versatile cell system for transient gene expression analysis. Nat. Prot. 2, 1565–1572. doi: 10.1038/nprot.2007.199
Zhang, G., Liu, K., Li, Z., Lohaus, R., Hsiao, Y. Y., Niu, S. C., et al. (2017). The Apostasia genome and the evolution of orchids. Nature 549, 379–383. doi: 10.1038/nature23897
Zhang, G., Xu, Q., Bian, C., Tsai, W., Yeh, C., Liu, K., et al. (2016a). The Dendrobium catenatum Lindl. genome sequence provides insights into polysaccharide synthase, floral development and adaptive evolution. Sci. Rep. 6:19029. doi: 10.1038/srep19029
Zhang, X., Wang, L., He, C., and Luo, H. (2016b). An efficient transient mesophyll protoplast system for investigation of the innate immunity responses in the rubber tree (Hevea brasiliensis). Plant Cell Tiss. Org. Cult. 126, 281–290. doi: 10.1007/s11240-016-0997-2
Zhang, Y., Su, J., Duan, S., Ao, Y., Dai, J., Liu, J., et al. (2011). A highly efficient rice green tissue protoplast system for transient gene expression and studying light/chloroplast-related processes. Plant Methods 7, 30–38. doi: 10.1186/1746-4811-7-30
Zhao, F., Li, Y., Hu, Y., Gao, Y., Zang, X., Ding, Q., et al. (2016). A highly efficient grapevine mesophyll protoplast system for transient gene expression and the study of disease resistance proteins. Plant Cell Tiss. Org. Cult. 125, 43–57. doi: 10.1007/s11240-015-0928-7
Zhu, Y., Hoshino, Y., Nakano, M., Takahashi, E., and Mii, M. (1997). Highly efficient system of plant regeneration from protoplasts of grapevine (Vitis vinifera L.) through somatic embryogenesis by using embryogenic callus culture and activated charcoal. Plant Sci. 123, 151–157. doi: 10.1016/S0168-9452(96)04557-8
Keywords: CsDELLA-mediated gibberellin signaling, gene silencing, leaf base, orchids, protoplast isolation, transient expression
Citation: Ren R, Gao J, Yin D, Li K, Lu C, Ahmad S, Wei Y, Jin J, Zhu G and Yang F (2021) Highly Efficient Leaf Base Protoplast Isolation and Transient Expression Systems for Orchids and Other Important Monocot Crops. Front. Plant Sci. 12:626015. doi: 10.3389/fpls.2021.626015
Received: 04 November 2020; Accepted: 25 January 2021;
Published: 15 February 2021.
Edited by:
Vladimir Orbovic, University of Florida, United StatesReviewed by:
Hong-Hwa Chen, National Cheng Kung University, TaiwanAli Parsaeimehr, Delaware State University, United States
Copyright © 2021 Ren, Gao, Yin, Li, Lu, Ahmad, Wei, Jin, Zhu and Yang. This is an open-access article distributed under the terms of the Creative Commons Attribution License (CC BY). The use, distribution or reproduction in other forums is permitted, provided the original author(s) and the copyright owner(s) are credited and that the original publication in this journal is cited, in accordance with accepted academic practice. No use, distribution or reproduction is permitted which does not comply with these terms.
*Correspondence: Fengxi Yang, eWFuZ2Zlbmd4aUBnZGFhcy5jbg==; Genfa Zhu, Z2VuZmF6aHVAMTYzLmNvbQ==