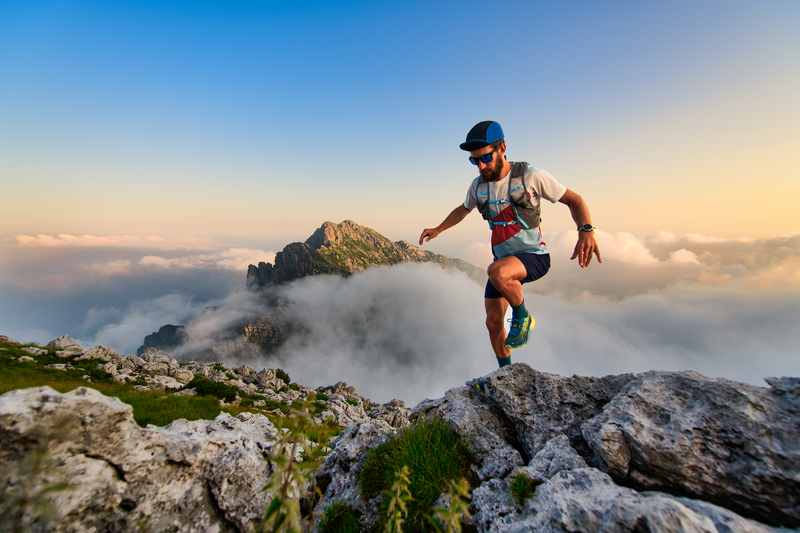
95% of researchers rate our articles as excellent or good
Learn more about the work of our research integrity team to safeguard the quality of each article we publish.
Find out more
ORIGINAL RESEARCH article
Front. Plant Sci. , 26 February 2021
Sec. Plant Metabolism and Chemodiversity
Volume 12 - 2021 | https://doi.org/10.3389/fpls.2021.623742
The MYB gene family is one of the largest groups of transcription factors (TFs) playing diverse roles in several biological processes. Hedychium coronarium (white ginger lily) is a renowned ornamental plant both in tropical and subtropical regions due to its flower shape and strong floral scent mainly composed of terpenes and benzenoids. However, there is no information available regarding the role of the MYB gene family in H. coronarium. In the current study, the MYB gene family was identified and extensively analyzed. The identified 253 HcMYB genes were unevenly mapped on 17 chromosomes at a different density. Promoter sequence analysis showed numerous phytohormones related to cis-regulatory elements. The majority of HcMYB genes contain two to three introns and motif composition analysis showed their functional conservation. Phylogenetic analysis revealed that HcMYBs could be classified into 15 distinct clades, and the segmental duplication events played an essential role in the expansion of the HcMYB gene family. Tissue-specific expression patterns of HcMYB genes displayed spatial and temporal expression. Furthermore, seven HcMYB (HcMYB7/8/75/79/145/238/248) were selected for further investigation. Through RT-qPCR, the response of candidates HcMYB genes toward jasmonic acid methyl ester (MeJA), abscisic acid (ABA), ethylene, and auxin was examined. Yeast one-hybrid (Y1H) assays revealed that candidate genes directly bind to the promoter of bottom structural volatile synthesis genes (HcTPS1, HcTPS3, HcTPS10, and HcBSMT2). Moreover, yeast two-hybrid (Y2H) assay showed that HcMYB7/8/75/145/248 interact with HcJAZ1 protein. In HcMYB7/8/79/145/248-silenced flowers, the floral volatile contents were decreased and downregulated the expression of key structural genes, suggesting that these genes might play crucial roles in floral scent formation in H. coronarium by regulating the expression of floral scent biosynthesis genes. Collectively, these findings indicate that HcMYB genes might be involved in the regulatory mechanism of terpenoids and benzenoid biosynthesis in H. coronarium.
Transcription factors (TFs) are proteins typically comprising of two functional domains involved in DNA-binding and transcriptional activation or repression of gene expression in response to internal or external stimuli (Riechmann et al., 2000; Amoutzias et al., 2007). Identification and deciphering the molecular function of TFs in regulating the gene expression provide insight into the signal transduction pathways and stress responses in different crops. TFs can be divided into different families based on DNA-binding domains that regulate the specific target genes. Among different TFs families, MYB is one of the largest and functionally diverse TFs superfamily found in nearly all eukaryotes. They are involved in a variety of critical processes, such as controlling plant growth and development, metabolism, physiological activities, cell morphology, and responses to environmental stresses (Baumann et al., 2007; Jung et al., 2008; Rawat et al., 2009; Dubos et al., 2010; Cao et al., 2020). MYB gene was first identified in avian myeloblastosis virus (AMV) followed by their identification in slime mold, fungi, animals, and plants (Klempnauer et al., 1982; Paz-Ares et al., 1987; Rosinski and Atchley, 1998; Stracke et al., 2001). COLORED1 (C1) was the first functionally characterized MYB gene from Zea mays in the plant kingdom and was associated with the regulation of anthocyanin biosynthesis (Paz-Ares et al., 1987). Although MYB genes have been extensively studied (Du et al., 2009; Dubos et al., 2010), the role of several MYB TFs remains ambiguous.
MYB proteins contain conserved MYB DNA-binding domains approximately ∼50–53 amino acids in length at the N-terminus and three α-helices tryptophan residues capable of forming a helix-turn-helix (HLH) to stabilize DNA-binding domain. In contrast, the C-terminal has diverse activation domains that play pivotal regulatory roles in these TFs (Ogata et al., 1996). Based on the number of repeats, MYB TFs are classified into four different groups: 1R, R2R3, 3R, and 4R-MYB (Stracke et al., 2001; Dubos et al., 2010). Unlike in animals, R2R3-MYB domain proteins are prevalent in plants (Martin and Paz-Ares, 1997; Dubos et al., 2010). The evolution of R2R3-MYB genes from R1R2R3-MYB genes by the loss of R1 repeat or duplication of R1 repeat from the 1R-MYB genes following expansion in plants has been proposed (Rosinski and Atchley, 1998; Jiang et al., 2004).
MYB proteins have been reported to be involved in controlling or regulating a wide range of processes, such as growth and development, flavonoid/phenylpropanoid metabolism, anthocyanin biosynthetic pathway, sugar signaling, secondary wall biosynthesis, and resistance to biotic and abiotic stresses (McCarthy et al., 2010; Medina-Puche et al., 2014; Tuan et al., 2015; Chen Y.-S. et al., 2017). In Arabidopsis thaliana, the overexpression of AtMYB96 increases drought tolerance via coordinating auxin and ABA signaling pathway as well as by regulating the Lipid transfer protein 3 (LTP3) target gene (Seo et al., 2009). AtMYB44 and AtMYB60 were involved in drought stress by regulating the stomatal movement (Jung et al., 2008). Gossypium barbadense MYB5 promotes drought tolerance in transgenic tobacco and cotton plants (Chen et al., 2015). Several MYB genes play roles in induced resistance toward insect herbivores or against mechanical wounding, such as AtMYB15, AtMYB34, AtMYB51, and AtMYB75. However, rice OsLTR1 regulates jasmonic acid (JA)-dependent defense responses (Johnson and Dowd, 2004; Liu et al., 2010). Jasmonate ZIM domain protein (JAZ) is a key component of the JA signaling pathway and plays a crucial role in plant responses to environmental stimuli. The suppressor proteins JAZ is the main component in the crosstalk as it interacts with other hormone signaling pathways, such as auxin, ABA, salicylic acid (SA), and ethylene (Yang et al., 2019a). JAZ proteins interact with the other TFs, such as MYB/MYC/bHLH/WRKY/AP2/ERF/NAC to suppress the expression of jasmonate-responsive genes. Through the interaction between the MYB and JAZ, this process governs plant growth and development, abiotic stresses, defense resistance, and regulates various secondary metabolites (Zhou and Memelink, 2016). Moreover, AtMYB125 regulates male germ cell division and differentiation (Brownfield et al., 2009), while AtMYB33 and AtMYB65 facilitate carpel development and ensure the production of viable pollen in anther (Millar and Gubler, 2005). AtMYB115/AtMYB118 plays an essential role in embryogenesis (Wang et al., 2009). Likewise, AtMYBL2 acts as a transcriptional repressor and inhibits the accumulation of proanthocyanin content in Arabidopsis (Dubos et al., 2008). Meanwhile, the grape VvMYB4-like gene and the soybean GmMYB100 negatively regulate flavonoid biosynthesis in plants (Yan et al., 2015; Pérez-Díaz et al., 2016). Similarly, R2R3-MybA TFs from Muscari armeniacum was involved in the biosynthesis of anthocyanin and can be used in flower color modification (Chen et al., 2019). Similarly, the characterization of novel litchi R2R3-MYB revealed its involvement in tissue acidification and anthocyanin biosynthesis (Lai et al., 2019). AtMYBL2/4/7 plays a crucial role in the regulation of flavonoid biosynthesis in Arabidopsis (Jin et al., 2000; Dubos et al., 2008). In apple, MdMYB3 regulates the biosynthesis of anthocyanin through activating the numerous flavonoid pathway genes and is involved in flower development (Vimolmangkang et al., 2013). GmMYB176 plays a pivotal role in flavonoid biosynthesis in Glycine max (Yi et al., 2010). ODORANT1 (ODO1), EMISSION OF BENZENOID II (EOBII), EOBI, and LATE ELONGATED HYPOCOTYL (LHY) regulate flower scent production via activating scent-related genes in petunia (Petunia hybrida) (Verdonk et al., 2005; Spitzer-Rimon et al., 2010, 2012; Fenske et al., 2015). In Pinus pinaster, MYB8 and ELONGATED HYPOCOTYL (HY5) controls the formation of phenylalanine and its metabolic channeling for lignin biosynthesis (El-Azaz et al., 2020). The MYB10 and FaEOBII from strawberry (Fragaria × ananassa) were involved in the regulation of eugenol (Medina-Puche et al., 2014, 2015). PpMYB15 and PpMYBF1 from Prunus persica had flower-specific expression and involved in the regulation of flavanol biosynthesis (Cao et al., 2018).
H. coronarium (white ginger lily or butterfly ginger) is a perennial ornamental flowering plant native to the Eastern Himalayas region and southern China. Floral fragrance is one of the main characteristics of ornamental plants, which improves their economic and aesthetic values (Dudareva et al., 2013; Muhlemann et al., 2014; Abbas et al., 2017). H. coronarium flower emits a strong scent, which is a complex mixture of volatiles terpenes including β-ocimene, 1,8-cineole, linalool, eucalyptol (monoterpenes), α-farnesene, β-caryophyllene (sesquiterpenes), and benzenoids (Fan et al., 2003, 2007; Báez et al., 2011; Yue et al., 2014, 2015; Ke et al., 2019). However, there is no information about the role of MYB TFs in H. coronarium.
Previously, we identified key structural genes involved in volatile biosynthetic pathways, such as benzoic/salicylic acid methyltransferase gene (BSMT) HcBSMT2 and the terpene synthases (TPSs) HcTPS1, HcTPS3, and HcTPS10. HcBSMT2, HcTPS1, HcTPS3, and HcTPS10 are among the key structural genes involved in the biosynthetic pathway of floral scent formation (Yue et al., 2015). Functional characterization of HcTPS1 and HcTPS3 revealed their role in the formation of monoterpenes eucalyptol and β-ocimene, respectively. HcTPS10 performs a dual function by interacting with both GPP and FPP to generates ocimene and α-farnesene, respectively. Furthermore, HcTPS1 and HcTPS3 showed a positive correlation with the emission of monoterpenes, a similar trend was observed for HcTPS10 with the emission of sesquiterpene α-farnesene. On the other hand, HcBSMT2 was specifically expressed in flowers and was involved in methyl benzoate formation (Yue et al., 2015). However, the transcriptional regulation of these genes is not elucidated. Regulatory proteins are important as they control the expression of structural genes and many structural genes are efficiently targeted for crop improvement (Bovy et al., 2007). In the current study, HcMYB genes were comprehensively analyzed. Moreover, Seven R2R3-HcMYB genes were functionally characterized and their role toward floral scent emission has been elucidated. This research will provide insights and will assist the scientists to further elucidate the biological roles of MYB genes in H. coronarium.
H. coronarium was planted in the growth chamber under conditions: 26 ± 2°C and 13/11 h light/dark photoperiod. For tissue-specific expression analysis, three different plant parts; flowers at full bloom, green leaves, and rhizomes were used. For the flowers, different flower developmental stages including D1; squaring stage, D2; half-open stage, D3; full-bloom, D4; senescence stage were selected. For different hormone treatments, the flower stems were shortly cut into 40 cm and then placed in sterilized water containing 100 μM IAA, ABA (200 μM), and MeJA (100 μM). For ethylene treatment, flowers were incubated with 10 μl/L of ethylene for 12 h in a sealed bottle. After that, the flowers were placed in an artificial growth chamber with a 14/10 h day/light photoperiod at 25°C. The floral volatile compound analysis was performed at the full-bloom stage of the treated flowers and immediately frozen in liquid nitrogen and stored at −80°C. Nicotiana benthamiana and Arabidopsis thaliana plants were grown under conditions: 24°C temperature with a photoperiod of 12/12 h (day/night).
To identify HcMYB proteins in H. coronarium, 125 Arabidopsis (TAIR)1, and 127 tomato MYB protein sequences (SOL genome)2 were used as a query sequence in the H. coronarium genomic data (unpublished) and transcriptome data (Yue et al., 2015). A total of 286 candidate MYB proteins were obtained. Thereafter, SMART3 (Letunic et al., 2015) and NCBI Conserved Domain Database (Marchler-Bauer et al., 2015) with default parameters were used to confirm the presence of MYB domains. The reductant and false predicted sequences were excluded. A total of 253 HcMYB genes were finally identified in the H. coronarium genome and subsequently comprehensively analyzed. Through the ProtParam tool4, the physical and chemical parameters of HcMYB genes were measured. The N-terminal sequences of HcMYB genes were analyzed using prediction software (WoLF PSORT)5.
Using the Clustal Ω program (Sievers et al., 2011), multiple sequence alignment of the putative H. coronarium MYB genes was analyzed with the default parameters. To construct an unrooted neighborhood phylogenetic tree of MYB TFs, the aligned protein sequences of AtMYBs, SlMYBs, OsMYBs, and HcMYBs were submitted to MEGA X (Kumar et al., 2018) with bootstraps set at 1,000 values. The syntenic relationship between H. coronarium, banana, pineapple, and rice was assessed using the MCScanX program (Tang et al., 2008).
The distribution of exon/intron in HcMYB genes was visualized by submitting the corresponding coding and genomic sequences to the Gene Structure Display Server (GSDS)6 (Hu et al., 2015). Multiple Expectation Maximization for Motif Elicitation (MEME)7 (Bailey et al., 2015) was used to predict the conserved motifs in HcMYB proteins sequences. The MEME program was set at the following parameters: the maximum number of motifs at 10, while the other parameters were kept at default and then visualized in the web logo server8.
For promoters analysis, the 2 kb upstream genomic DNA sequences of HcMYB genes were retrieved from the H. coronarium genome and submitted to the PlantCARE database9 (Lescot et al., 2002) and verified in the PLACE databases10 (Higo et al., 1999).
For subcellular localization of selected MYB genes, HcMYB7, HcMYB8, HcMYB145, and HcMYB248 coding sequences with SpeI and NcoI restriction sites were fused with a green fluorescent protein (GFP) into the vector 35Spro:GFP (Wang et al., 2002). The transformation of Arabidopsis thaliana with 35Spro:GFP vectors and protoplasts isolation was performed following Yoo et al. (2007). The protoplasts were visualized 16–18 h after transformation through a laser scanning confocal microscope (LSCM). The primers used for GFP vector construction are listed in Supplementary Table 2.
Transcriptional activity was determined using the yeast-one-hybrid system (Clontech, Takara) as described in the manufacturer’s protocol. The promoters of HcTPS1, HcTPS3, HcTPS10, and HcBSMT2 were cloned into the vector pAbAi act as baits (HcTPS1-pAbAi, HcTPS3-pAbAi, HcTPS10-pAbAi, and HcBSMT2-pAbAi). The full-length of HcMYB7, HcMYB8, HcMYB75, HcMYB79, HcMYB145, HcMYB238, and HcMYB248 were fused into the pGAL4 activation vector (pGADT7-AD) to generate pGADT7-HcMYB7, pGADT7-HcMYB8, pGADT7-HcMYB75, pGADT7-HcMYB79, pGADT7-HcMYB145, pGADT7-HcMYB238, and pGADT7-HcMYB248 as preys. The transformation of yeast cells and confirmation of positive interactions were performed as described in the Y1H Gold yeast strain manufacturer’s protocol (Clontech Takara).
The full-length sequences of HcMYBs (HcMYB7, HcMYB8, HcMYB75, HcMYB79, HcMYB145, HcMY238, and HcMYB248) were ligated into the vector pGADT7 (AD), and the coding sequences of HcJAZ1 was cloned into the vector pGBKT7 (BD). For the Y2H assay, the AH109 cells containing both BD and AD were placed on the SD/-Leu/-Trp medium for 3 days at 30°C. The empty vector pGADT7 was used as blank control. The transactivation activity was affirmed via yeast growth on the aforementioned plates. Yeast colonies expressing MEL1 turn blue with the addition of X-α-Gal substrate (Clontech, TaKaRa) because MEL-1 encodes α-galactosidase. Primers used are listed in Supplementary Table 2.
VIGS was conducted in the barley stripe mosaic virus (BSMV) system (Renner et al., 2009; Yuan et al., 2011). A 250- to 300-bp amplicon of HcMYB7, HcMYB8, HcMYB145, HcMYB238, and HcMYB248 genes were inserted in pCaBSγ vector at ApaI restriction site making pCaBSγ:HcMYB7, pCaBSγ:HcMYB8, pCaBSγ:HcMYB145, pCaBSγ:HcMYB238, and pCaBSγ:HcMYB248 constructs for corresponding genes supersession. The constructs were then transformed into Agrobacterium tumefaciens strain EHA105. For infiltration assays, the A. tumefaciens culture was suspended in infiltration buffer (10 mM MES; pH 5.6, 10 mM MgCl2, and 0.1 mM acetosyringone) at OD600 of 1. The solution was applied to the flowers at the D1 stage (bud stage). Vacuum infiltration was performed by immersing the flowers in the bacterial suspension. After the release of the vacuum, the flowers were washed in deionized water, placed into an MS medium liquid culture, and then maintained with a 12/12 h light/dark cycle at 16°C for 4–5 days. The total floral volatile compounds were collected and analyzed at the full-bloom stage by GC-MS. The experiment was performed in three to five biological replicates.
The whole flower was placed in a glass bottle (500 ml) with the addition of an internal standard. After 30 min, a PDMS fiber was injected for 30 min to trap volatile followed by insertion into a GC-MS system (Agilent) for volatile analysis as described previously (Ke et al., 2019). The floral volatiles were measured at the full-bloom stage via GC-MS as described previously (Yue et al., 2014; Abbas et al., 2019, 2020).
Total RNA extracted and cDNA was synthesized as described previously (Abbas et al., 2020). For RT-qPCR, 20 μl reaction system comprising 10 μl iTaqTM Universal SYBR Green Supermix (BIO-RAD), 2 μl of cDNA, 0.4 μl of forward and reverse primers each, and 7.2 μl of ddH2O was performed in an ABI 7500 Fast Real-Time PCR System (Applied Biosystems, United States). GAPDH was used for normalization of data and the 2–ΔΔCT method was used for relative expression analysis (Livak and Schmittgen, 2001). All the reactions were performed in triplicate. The primers used for RT-qPCR are listed in Supplementary Table 2.
SPSS 19.0 program (SPSS Inc., Chicago, IL, United States) was used for statistical analysis and Student’s t-test. Data are presented as the mean ± SD and p < 0.05 (n = 3).
A total of 253 potential candidate HcMYB genes were identified in H. coronarium genome data. All genes were designated as HcMYB1-HcMYB253 based on the chromosomal location. Among them, 27 HcMYB genes belong to 1R, six 3R, one 4R, and the rest are all R2R3 type. The HcMYB protein size and molecular mass range from 100 aa/11.37 kDa (HcMYB100) to 1,749 aa/192.53 kDa (HcMYB10). Similarly, the pI also varies greatly from 4.51 (HcMYB170) to 12.2 (HcMYB252), indicating their functional diversity in microenvironments. A similar pattern was observed in Prunus persica and Phyllostachys edulis (Zhang C. et al., 2018; Yang et al., 2019b). Moreover, in silico analysis revealed that HcMYB proteins were predicted to localize in the nucleus. The detailed information of HcMYB genes is provided in Supplementary Table 1.
The domain structure analysis showed that all HcMYB genes contain highly conserved typical SANT DNA-binding domains in their sequences, which is essential for their different regulatory interaction mechanism. The multiple sequence alignment of mostly HcMYB genes revealed that they possess 2R and 3R repeat signatures at the N-terminus, and seven candidate HcMYB genes were selected for multiple sequence alignment (Supplementary Figure 1). The seven candidate HcMYB proteins were selected for further characterization based on their expression pattern with flower scent emission. To assess the phylogenetic relationship among MYB genes in H. coronarium (253 HcMYBs), rice (66 OsMYBs), Arabidopsis (125 AtMYBs), and tomato (127 SlMYBs), an unrooted NJ phylogenetic tree was generated. All MYB proteins were divided into 15 distinct clades designed as G1–G15. Among them, subgroup G13 constitutes the largest group containing 64 MYB members of four different species followed by G15 (63), G1 (56), G7 (54), G8 (48), G6 (48), G7 (40), G11 (39), G5 (30), G12 (28), G2 (26), G10 (26), G4 (25), G14 (8), and G3 (6), respectively. Similarly, the maximum number of HcMYB genes were clustered into subgroup G15 (29) followed by G11 (27), G8 (27), G1 (25), G7 (23), G8 (23), G13 (20), G2, (15), G10 (15), G5 (13), G9 (12), G12 (10), G14 (7), G4 (5), and G3 (2), respectively. Interestingly, subgroup G14 contains eight MYBs from which seven belong to H. coronarium (Figure 1). Overall, all HcMYBs with AtMYB, OsMYB, and SlMYBs were unevenly clustered into all groups indicating their evolutionary divergence. The group of MYB genes in the same subclade may have a similar function. The phylogenetic tree analysis of only MYB proteins from H. coronarium revealed that 253 HcMYB proteins were distinctly grouped into 12 different clades (Supplementary Figure 2). Moreover, three groups (G3, G13, and G15) from four genome phylogeny, were missing. Moreover, the phylogenetic tree of 253 HcMYBs was also constructing with the previously identified scent-related MYB TFs (Supplementary Figure 3). The data showed that all HcMYBs along with previously identified scent-related MYBs were clustered into five distinct clades (G I-G V). The maximum number of scent-related MYB proteins were clustered in G II (11) followed by G V (5), G III (2), and G I (1), respectively. Furthermore, the selected seven-candidate HcMYB proteins were also grouped in different clades. HcMYB79 was clustered in G I, which included AtMYBL2, and HcMYB75/145/238 was found in G II, which contains the majority of scent-related MYBs (AtMYB2/21/24, FaEOBII, PhEOBI, PhEOBII, FvEOBII, PsMYB26, AmMYB305/340, and NiMYB3005). Similarly, HcMYB248 was found in G III, which includes AtMYB42 and PhODOI, while HcMYB7/8 was clustered in G V which includes AtMYB4/7, FaMYB1/10, PhMYB4, and PtMYB14 (Supplementary Figure 3).
Figure 1. Phylogenetic analysis of MYB proteins among H. coronarium, Arabidopsis, tomato, and rice. Full-length amino acid sequences were aligned using ClustalX 2.1 program and unrooted NJ phylogenetic tree was generated using MEGA X with 1,000 bootstrap values. The bootstrap values of less than 50 were omitted. All MYB proteins are clustered into 15 subgroups (G1–G15) indicated by different colors.
To investigate the functional diversification of HcMYB proteins, 10 conserved motifs (motifs 1–10) were identified in the MEME server (Supplementary Figure 4). The majority of the HcMYB proteins within the subclade had a similar composition of motifs, while variation was observed among different subclades. The motifs 1, 2, and 3 were the most conserved and appear in most of the HcMYB proteins. All the HcMYB proteins contain two or more than two motifs except HcMYB185 which only has motif 2 (Supplementary Figure 5). HcMYB23, HcMYB40, HcMYB47, HcMYB55, HcMYB110, HcMYB126, HcMYB168, and HcMYB253 proteins contained motif 3 and motif 8; HcMYB31, HcMYB67, HcMYB91, HcMYB121, and HcMYB179 proteins contained motif 1 and motif 2. HcMYB proteins containing motif 9 and motif 10 belong to subgroup G10 and G9, respectively. Interestingly, some HcMYB proteins contain the repetition of motif 3 and motif 8 and fall into subgroup G2 indicating their involvement in a specific function (Supplementary Figures 2, 5B).
Gene structure analysis was performed to better understand the expansion of HcMYB genes in H. coronarium. The number of exon/intron ranges from 0 to 21. Gene structure analysis revealed that five HcMYB (HcMYB27, HcMYB87, HcMYB185, HcMYB217, and HcMYB249) genes were intron-less, while HcMYB10 have a maximum of 21 introns (Supplementary Figure 5C). Moreover, HcMYB89 has the longest intron, and the majority of them (82%) were disrupted by two or three introns. In General, the HcMYB genes with the same number of introns were grouped into a similar subclade.
The chromosomal location of HcMYBs was performed to investigate the genomic distribution of the MYB gene family in H. coronarium. The results revealed an uneven distribution of HcMYB genes in the chromosomes (Figure 2A). In total, 6 HcMYB genes were found on chromosomes 1 and 13, 7 on chromosomes 8 and 16, 9 on chromosomes 3 and 15, 11 on chromosomes 10 and 12, 12 on chromosome 6, 14 on chromosomes 7 and 11, 15 on chromosomes 5 and 9, 16 on chromosome 17, 18 on chromosome 14, and 19 on chromosome 2. The maximum number of HcMYB genes (20) were presented on chromosome 4. The majority of HcMYB genes were observed on the top and bottom of the chromosomes, while rarely found in the middle of the chromosome.
Figure 2. Synteny and collinearity analysis of MYB gene family. (A) Circos plot of MYB genes in H. coronarium genome. Segmental duplication of MYB gene pairs is indicated by red color in H. coronarium. (B) Colinearity plot of HcMYB genes between Musa acuminata and three other representative plant species. Gray lines in the background showed collinear blocks within H. coronarium and other plant genomes, while red lines indicate syntenic MYB gene pairs.
Gene duplications play a crucial role in the evolution and expansion of gene families in plants. It was found that H. coronarium genome contained 62 HcMYB segmental duplications (Figure 2A). The maximum number of segmental duplication events was observed on chromosomes 14 and 17, while a low number of duplication events were found on chromosome 3. Moreover, three tandem duplication pairs were also identified on chromosome 17 in H. coronarium genome. The Ka/Ks (synonymous/non-synonymous) values of all segmentally and tandemly duplicated HcMYB gene pairs had less than 1, indicating that they evolved under the pressure of purifying selection (Figure 2A and Supplementary Table 3). The average Ka/Ks value of tandem duplication genes (0.57) was higher than that segmented duplication genes (0.27). Moreover, the segmental and tandem duplication gene events occurred about 47 Mya (million years ago), implying that duplication events play a crucial role in functional and evolutional divergence.
To determine the evolutionary mechanism of HcMYB genes, comparative synteny maps of H. coronarium associated with rice, pineapple, Arabidopsis, and banana were constructed (Figure 2B). A total of 7 ortholog MYB gene pairs between H. coronarium and Arabidopsis, 19 orthologs between H. coronarium and rice, 44 orthologs between H. coronarium and pineapple, and 193 orthologs between H. coronarium and banana were identified (Figure 2B and Supplementary Tables 4–7). The number of HcMYB-MaMYB was greater than HcMYB-AtMYB, HcMYB-OsMYB, and HcMYB-AcMYB, indicating the closer evolutionary relationship between H. coronarium and Musa accuminata and their divergence from the common monocot ancestor. Interestingly, HcMYB29 and HcMYB194 collinear gene pairs were present among four species. HcMYB29 was homologous pair of AT5G65230.1 (A. thaliana), LOC_Os02g51799.1 (O. sativa), Aco002802.1 (A. comosus), and GSMUA_Achr10T24560 (M. acuminata). Similarly, HcMYB194 was collinear gene pair of AT4G37780.1, LOC_Os03g56090.1, Aco004978.1, and GSMUA_Achr5T01930. In short, the aforementioned two collinear gene pairs were found among four species genomes, implying their evolutionary mechanism and specific function in H. coronarium.
A 2-kb upstream promoter region of HcMYB genes was scanned for cis-regulatory elements analysis. The data showed that MeJA responsiveness elements are the most common ones, which were found in 210 HcMYB gene promoters. Other cis-acting regulatory elements found in the promoter region of HcMYB genes were ABARE (208), ERE (180), GARE (144), MBS (128), Auxin responsive (120), SARE (99), and low-temperature responsiveness elements (96) (Supplementary Figure 6). The detailed information regarding the cis-elements present in the promoter region of HcMYB genes is given in Supplementary Table 8. Previous studies showed that miRNA play key roles in plant secondary metabolism. In A. thaliana, miR858 encodes regulatory peptides and controls flavonoid biosynthesis and development (Sharma et al., 2020). Similarly, miR156 was involved in the regulation of anthocyanin biosynthesis in poplar (Wang et al., 2020). The miRNA prediction analysis showed that HcMYB genes were targeted by different families of miRNA. About 37 HcMYB genes were mainly targeted by miR156, miR157, miR158, miR160, miR167, miR168, miR169, miR172, miR319, miR854, and miR858. The HcMYB genes were commonly targeted by miR156, miR167, and miR319 (Supplementary Table 9). Interestingly, HcMYB187, HcMYB166, and HcMYB157 were targeted by both miR167 and miR319. The majority of HcMYB genes (42) were targeted by miR858.
The differential expression patterns of HcMYB genes in different organs and flower developmental stages were analyzed using the RNA sequencing data (Yue et al., 2015; Supplementary Figure 7). The expression pattern of HcMYB genes was grouped into three clusters. The cluster I showed a group of HcMYB genes, which had preferential expression pattern in flowers. Similarly, cluster II and cluster III represent the member of HcMYBs, which showed their high expression levels in rhizome and leaves, respectively (Supplementary Figure 7A). Interestingly, HcMYB7, HcMYB8, HcMYB75, HcMYB145, HcMYB238, and HcMYB248 from cluster I had the highest expression level in the flower. Some members of the cluster I also showed their spatial expression both in flower and rhizome including HcMYB79, HcMYB128, HcMYB162, HcMYB130, HcMYB108, HcMYB199, and HcMYB208. The members of HcMYB genes in cluster II showed high expression in the rhizome, while a few genes from cluster III showed high expression both in flowers and leaves and some in leaves and rhizomes. However, the majority of genes from cluster III showed their high expression in leaves. The amount of volatile contents released from flowers was maximum, while less quantity was observed in leaves and rhizomes (Figure 3A). Previous studies showed that tissue-specific expression is important for gene functioning (Sonawane et al., 2017). The data indicate the group of HcMYBs present in cluster I might be involved in floral scent formation, while cluster II and cluster III in the functioning of vegetative organs (rhizome and leaves).
Figure 3. The total volatile contents and expression levels of candidates HcMYB genes. (A) The total volatile contents emitted from flower, leaf, and rhizome. (B) The total floral volatiles released during different flower developmental stages (D1: squaring stage; D2: half open stage; D3: full-bloom; D4: senescence stage). (C) Differential expression patterns of selected candidate HcMYB genes during four different flower developmental stages. The heat map was generated with the relative expression RT-qPCR data of candidate HcMYB genes. The red (upregulated expression levels) and green (downregulated expression levels) color represent the log2 transformed expression values.
In H. coronarium, the process of floral scent formation is associated with flower development. The emission quantity of floral volatiles was low at the bud stage and substantially increase with the flower development peak at the blooming period and declined at the senescence stage (Figure 3B). To clarify the functioning of HcMYBs during flower development stages, their expression level at three stages was divided into three clades via a heat map (Supplementary Figure 7B). Several HcMYB genes showed high expression levels at the D1 stage followed by D4 and D6, respectively. However, some HcMYBs (HcMYB34, HcMYB51, HcMYB181, and HcMYB232), which belong to cluster I, showed a substantial increase in expression level with the developmental stages. The members of HcMYB genes from cluster II showed diverse expression levels. The majority of genes showed a high expression level at the D4 stage only, although HcMYB5, HcMYB62, HcMYB106, HcMYB111, HcMYB240, and HcMYB245 from cluster II showed high expression both at D1 and D4 stage. Likewise, within-cluster II, some HcMYBs (HcMYB16, HcMYB94, HcMYB203, HcMYB208, HcMYB218, and HcMYB221) showed specific high expression both at D4 and D6 stage only (Supplementary Figure 7B). Interestingly, HcMYB22, HcMYB55, and HcMYB112, within-cluster III, showed a high expression at the D1 stage, with no expression at the D4 stage and dramatically peak at the D6 stage.
Based on transcriptome data, seven HcMYB genes were selected and their spatial expression pattern was validated via RT-qPCR at four different flower development stages (Figure 3C). The expression level of HcMYB79 increased with the flower development and peak at the senescence stage, while HcMYB8 and HcMYB75 showed a high expression level at the D2 stage (half-open). However, HcMYB7, HcMYB145, HcMYB238, and HcMYB248 showed a dramatic increase in the expression level from D2 to D3 stage. Moreover, the expression of candidate HcMYB genes correlates with the emission of total volatile contents (Supplementary Figure 8). The expression level of HcMYB genes significantly altered with the flower developmental process, suggesting their potential role during the development of flower and floral scent emission.
Plant hormones play several functions in growth and development and are involved in different signaling pathways. Auxin, abscisic acid, ethylene, and jasmonates are the key hormones playing an essential role in flower development and senescence. The amount of floral volatile contents was increased by 27.6, 23.9, 25.5, and 33.1% under IAA, ABA, ethylene, and MeJA treatment, respectively (Figure 4A). The expression levels of selected HcMYBs were analyzed via RT-qPCR under the abovementioned treatments. The data showed that mRNA levels of HcMYB75, HcMYB79, HcMYB145, and HcMYB238 were upregulated under auxin treatments, while HcMYB248 was downregulated (Figure 4C). Under ABA treatment, HcMYB145 expression level was increased by 19-fold followed by HcMYB75, HcMYB79, and HcMYB238, respectively. However, the expression level of HcMYB248 was reduced (Figure 4D). For ethylene treatment, HcMYB79 and HcMYB145 were significantly upregulated; however, HcMYB7, HcMYB8, and HcMYB248 were downregulated (Figure 4E). The expression levels of HcMYB75, HcMYB79, HcMYB145, and HcMYB238 were upregulated when treated with MeJA, while HcMYB248 was downregulated (Figure 4F). Moreover, mRNA levels of key structural biosynthesis genes (HcTPS1, HcTPS3, HcTPS10, and HcBSMT2) were also upregulated upon phytohormones stresses (Figure 4B). These results indicate that HcMYB genes respond to various stresses and play crucial roles through crosstalk with different hormones.
Figure 4. Relative expression level of candidate HcMYB genes and emission of total floral volatiles under phytohormone stresses. (A) Total floral volatiles contents emitted during phytohormone treatments. (B) mRNA levels of key structural volatile biosynthesis genes upon phytohormone stresses. (C) IAA: (Inode-3-acetic acid), (D) ABA: (abscisic acid), (E) ethylene, (F) MeJA: jasmonic acid methyl ester. Error bars indicate SD of three biological replicates and asterisk represents significant differences (∗p < 0.05).
In silico subcellular localization of HcMYB genes revealed that all H. corornarium MYB genes are predicted to localize in the nucleus except HcMYB126, which is predicted to localize in the chloroplast (Supplementary Table 1). To experimentally validate the predicted localization, four HcMYB genes (HcMYB7, HcMYB8, HcMYB145, and HcMYB248) were selected for analysis in Arabidopsis protoplast. The NLS-mCherry was applied in each transformed design to act as a marker for nuclear localization. The results revealed that HcMYB7, HcMYB8, HcMYB145, and HcMYB248 proteins were localized to the nucleus (Figure 5).
Figure 5. Nuclear localization of HcMYB7/8/145/248 proteins in Arabidopsis protoplasts. GFP; green fluorescent protein, mCheyy; nuclear marker, merged; combined mCheryy and GFP and bright field. Bars, 10 μm.
To understand the potential involvement of HcMYB genes in the floral scent formation, a virus-induced gene silencing system was performed for HcMYB7, HcMYB8, HcMYB79, HcMYB145, and HcMYB248. The data showed that the mRNA expression level of HcMYB7, HcMYB8, HcMYB79, HcMYB145, and HcMYB248 was decreased 41.4, 47.8, 78.7, 75.8, and 85.3%, respectively (Figure 6A), relative to the control flowers. The silencing of HcMYB genes in flowers also downregulated the expression level of key structural volatile biosynthesis genes. In the HcMYB7-silenced flower, the expression level of HcTPS1, HcTPS3, HcTPS10, and HcBSMT2 was downregulated up to 59.7, 23.1, 65.7, and 58.3%, respectively, compared to control. The silencing of HcMYB8 in H. coronarium flowers, downregulated the expression level of HcTPS1, HcTPS3, HcTPS10, and HcBSMT2 by 76.5, 54.5, 82.8, and 87.7%, respectively. Alike, silencing of HcMYB79 significantly downregulated the expression levels of HcTPS1 (74.4%), HcTPS10 (87.6%), and HcBSMT2 (87.4%). The expression level of HcTPS1 (68.3%), HcTPS3 (57.7%), HcTPS10 (52.6%), and HcBSMT2 (48.3%) was significantly suppressed in the HcMYB145-silenced flowers. Furthermore, the mRNA levels of HcTPS1 (66.5%), HcTPS3 (38.8%), HcTPS10 (72.1%), and HcBSMT2 (81.4%) were significantly changed in the HcMYB248-silenced flowers (Figure 6B).
Figure 6. HcMYB7/8/79/145/248 silencing changes the amount of main floral volatile contents and expression of key structure genes in H. coronarium flowers. (A) RT-qPCR analysis of HcMYB7/8/79/145/248 mRNA levels in BSMV silenced and control flowers. (B) mRNA levels of bottom structural volatile synthesis genes in BSMV silenced flowers compared with control. (C) GC-MS analysis of volatile contents of eucalyptol, (D) ocimene, (E) methyl benzoate, and (F) linalool after silencing of HcMYB7/8/79/145/248 genes. Data are presented as the mean ± SEM (n = 3). Asterisk indicates significant differences [Student’s t-test, ∗(P ≤ 0.05)] between treatments and control.
The effect of silencing of HcMYB genes on the floral volatile profile of H. coronarium was assessed. It was observed that eucalyptol contents were decreased 42.2, 40.8, 22.6, 34.6, and 39.8% in the HcMYB7, HcMYB8, HcMYB79, HcMYB145, and HcMYB248-silenced flowers, respectively (Figure 6C). Similarly, the silencing of HcMYB7, HcMYB8, and HcMYB79 significantly decreased the accumulation of ocimene by 23.3, 24.4, and 27.1%, respectively. However, no significant change in volatile contents was found in the HcMYB145 and HcMYB248-silenced flowers (Figure 6D). The volatile contents of methyl benzoate were significantly decreased by 27.6 and 31.9% in the HcMYB8 and HcMYB248-silenced flowers, respectively, while silencing of HcMYB145 did not affect the level of methyl benzoate (Figure 6E). Furthermore, the contents of linalool were decreased by 16.9, 26, 13.9, 26.2, and 15.1% in the HcMYB7, HcMYB8, HcMYB79, HcMYB145, and HcMYB248-silenced flowers, respectively (Figure 6F). The data showed that these HcMYB genes play a crucial role in the floral scent formation in H. coronarium.
Promoter sequences analysis of HcTPS1 (1,131), HcTPS3 (1,557), HcTPS10 (867), and HcBSMT2 (1,131) revealed that they encompass 6, 4, 3, and 13 copies of MYB-core binding motifs, respectively (Supplementary Table 10). To examine that the identified HcMYB regulators directly bind or not to the promoters of key volatile floral scent biosynthesis genes (HcTPS1, HcTPS3, HcTPS10, and HcBSMT2) in H. coronarium, a Y1H assay was conducted. It was observed that the bait strains harboring proHcTPS1 and proHcTPS3 grow well on gained aureobasidin A antibiotic SD-Leu medium co-expression with HcMYB7, HcMYB8, and HcMYB145. Moreover, bait strains harboring proHcTPS10 gained aureobasidin A antibiotic resistance upon expression of HcMYB8, HcMYB75, HcMYB79, and HcMYB238. Furthermore, bait strains harboring proHcBSMT2 gained aureobasidin A antibiotic resistance upon expression of HcMYB7, HcMYB8, HcMYB79, HcMYB145, and HcMYB248 (Figure 7A).
Figure 7. Candidate HcMYB proteins binds to the promoters of bottom structural genes. (A) The interaction was determined on SD medium lacking leucine (Leu) in the presence of aureobasidin A (-Leu + AbA). AD was used as a control. (B) HcMYB7/8/75/79/145/238/248 interacts with HcJAZ1 protein by yeast two-hybrid assay. The vectors were transformed into yeast strain Y2HGold followed by the screening of transformants via SD/-Trp/-His/-Ade + X-α-gal media. Yeast cells transformed with pGBKT7–53 + pGADT7-T, pGBKT7-Lamin + pGADT7-T, or pGADT7-T- pGBKT7-HcJAZ1 were included as negative or positive controls.
To further unravel the molecular mechanism of the involvement of HcMYBs in the regulation of floral aroma biosynthesis, a yeast-two-hybrid (Y2H) assay was performed. It was observed that pGADT7-T + pGBKT7-53 (positive control) along with yeast cells, which co-transformed with pGADT7-HcMYB7 + pGBKT7-HcJAZ1, pGADT7-HcMYB8 + pGBKT7-HcJAZ1, pGADT7-HcMYB75 + pGBKT7-HcJAZ1, pGADT7-HcMYB145 + pGBKT7-HcJAZ1, and pGADT7-HcMYB7248 + pGBKT7-HcJAZ1, grew well on SD plates lacking Trp, His, Ade, and Leu but in the existence of 5 mM 3-AT. On the other hand, yeast cells harboring pGBKT7-Lam + pGADT7-T (negative control), pGADT7-HcMYB79 + pGBKT7-HcJAZ1, and pGADT7-HcMYB238 + pGBKT7-HcJAZ1 did not grow (Figure 7B). These results indicated that HcJAZ1 may interact with five HcMYBs (HcMYB7, HcMYB8, HcMYB75, HcMYB145, and HcMYB248) in yeast.
H. coronarium is an important industrial, medicinal, and ornamental plant. The snowy white flowers emit a strong scent mainly composed of terpenes, benzoates, and some phenylpropanoids (Matsumoto et al., 1993; Fan et al., 2003, 2007; Báez et al., 2011). The MYB gene family is one of the biggest TF families playing key physiological and biochemical roles in the plant. The role of this family in H. coronarium is unknown. Taking the advantage of this and the availability of H. coronarium in our group, we performed a genome-wide analysis of the MYB gene family and its potential role in floral aroma production.
The number of MYB genes varies among different species including 204 MYB TFs in Arabidopsis thaliana, 218, 244, 256, 127, 116, 122, and 85 MYBs from Oryza sativa, Glycine max, Prunus persica, Solanum lycopersicum, Physcomitrella patens, Brachypodium distachyon, and Phyllostachys edulis, respectively (Du et al., 2012; Katiyar et al., 2012; Li Z. et al., 2016; Chen S. et al., 2017; Zhang C. et al., 2018; Yang et al., 2019b; Pu et al., 2020). However, a total of 253 HcMYB genes were identified in H. coronarium. The HcMYB genes can be divided into 4 families, including 27 1R-MYB proteins, 219 R2R3-MYB proteins, 6 3R-proteins, and 1 4R-MYB protein. The presence of one 4R-MYB protein identified in H. coronarium was in line with previous findings in Arabidopsis, peach, pear, and Chinese jujube (Dubos et al., 2010; Lan et al., 2013; Li X. et al., 2016; Zhang C. et al., 2018). However, in some plants, more than one 4R-MYB protein have also been reported (Saha et al., 2016; Salih et al., 2016). In the current study, six 3R-MYB proteins were identified, which is inconsistent with other findings: 11 3R-MYB proteins in Chinese cabbage (Saha et al., 2016), 15 in cotton (Salih et al., 2016), and four in tomato and peach (Li Z. et al., 2016; Zhang C. et al., 2018). The differences in numbers are probably due to the differences in the evolution of plants.
Gene duplication plays a crucial role in the expansion and evolution of genes and accelerates the expansion of gene families (Davidson et al., 2013; Chen S. et al., 2017). The data showed that segmental and tandem duplication events occur unevenly on all chromosomes (Figure 2A). Sixty two HcMYB genes were identified as segmentally duplicated, while three HcMYB genes were observed as tandemly duplicated genes. Similar trends of MYB gene duplication pairs were observed in Arabidopsis thaliana (Cannon et al., 2004), Solanum tuberosum L. (Sun et al., 2019), Citrus sinensis (Liu et al., 2014), and Ananas comosus (Liu et al., 2017). The results indicate that segmental duplication events play key roles in the expansion of MYB genes compared to tandemly duplicated genes. The Ka/Ks ratios of 65 HcMYB replications suggested that this gene family undergo purifying selection, a clear indication of highly conserved evolution.
To gain more insight into the evolutionary relationship of HcMYB genes, a phylogenetic tree was built including MYB proteins from Arabidopsis thaliana, Oryza sativa, and Solanum lycopersicum. As shown in Figure 1, all MYB genes were distinctly grouped into 15 different clades from G1–G15. HcMYB7 and HcMYB8 genes fell into subgroup G11, which contains AtMYB32, AtMYB3, AtMYB4, AtMYB6, AtMYB7, and AtMYB8, which are involved in anthocyanin and flower development (Jin et al., 2000; Vimolmangkang et al., 2013; Fornalé et al., 2014). HcMYB75 and HcMYB145 with AtMYB21, AtMYB24, and AtMYB78 were grouped in subclade G6, which had flower-specific expression and involved in plant secondary metabolism (Shin et al., 2002; Spitzer-Rimon et al., 2010; Medina-Puche et al., 2015). Similarly, HcMYB79 was clustered in subgroup G1 with AtMYB1, AtMYB44, AtMYB70, and AtMYB77. The functional characterization of the abovementioned TFs revealed that they were involved in different abiotic stress responses (Jung et al., 2008). HcMYB238 and HcMYB248 were grouped into subclades G5 and G15, respectively. The results showed that the tandemly or segmentally duplicated genes were grouped into the same clade (Supplementary Figure 2). The prediction results showed that all HcMYB genes include the conserved MYB DNA-binding domain; however, some HcMYB genes also possess additional domains, such as HcMYB6 containing a CS domain, which is involved in recruiting heat-shock proteins to multi-protein assemblies (Lee et al., 2004). Similarly, HcMYB10 includes additional VHS-ENTH-ANTH, GAT, and Med15 superfamily (Supplementary Figure 6B). Moreover, the exon/intron structure and motif analysis of HcMYB genes was also performed. The data showed that the majority of HcMYB genes contained motif 1, motif 2, and motif 3. Furthermore, HcMYB genes (∼82%) had two to three number of introns, which is consistent with previous findings (Du et al., 2012; Liu et al., 2020). In General, the number of motifs and intron in the same clade were similar, while variations were observed in a few clades, implying the functional redundancy of these genes. The colinearity relationship of HcMYB genes revealed high levels of collinearity with Musa acuminata MYB genes followed by Ananas comosus, Oryza sativa, and Arabidopsis thaliana implying that these MYB genes probably come from a common ancestor (Figure 2B). Two colinear genes (HcMYB29 and HcMYB194) were observed, which exist in all the plant’s genomes, indicating that they are probably associated with the evolutionary processes.
MYB transcription factors play key roles in regulating plant secondary metabolites. The production, as well as emission of floral volatile compounds, is developmentally regulated and peak at the bloom stage (Muhlemann et al., 2014; Abbas et al., 2017). Likewise, in H. coronairum, the maximum floral volatile contents were emitted at the full-bloom flower (Figure 3A). As shown in Figure 5, the group of HcMYB genes in cluster I showed their preferential expression in flowers. Moreover, the RNA-seq data of different flower development stages revealed their higher expression pattern with the flower development stage, implying their potential involvement in the production and emission of floral aroma. In Syringa oblata, two R2R3-MYB transcription factors showed a high expression pattern during the flower development, which is similar in strawberry and petunia (Van Moerkercke et al., 2011; Medina-Puche et al., 2015). The expression pattern of selected HcMYB genes at four different flower development stages showed a substantial increase in the mRNA level consistent with the flower development stage (Figure 3). Interestingly, the key structural genes (HcTPS1, HcTPS3, HcTPS10, and HcBSMT2) also showed flower-specific expression consistent with the production of high volatile contents at the full-bloom stage (Yue et al., 2015). Moreover, the formation of floral volatile compounds was higher in the flower than in leaves and rhizome. Likewise, Pinus taeda MYB14 (PtMYB14) regulates the isoprenoid-oriented response, which encourages the accumulation of sesquiterpene (Bedon et al., 2010). The FaEOBII, FaEOBI, and ODO1 from strawberry also showed flower specific expression pattern and was involved in the regulation of eugenol (Verdonk et al., 2005; Spitzer-Rimon et al., 2010; Van Moerkercke et al., 2011, 2012; Medina-Puche et al., 2015). PpMYB15 and PpMYBF1 from Prunus persica had flower-specific expression and involved in the regulation of flavanol biosynthesis (Cao et al., 2018). The data indicate that the HcMYB genes potentially regulate the floral aroma production during flower development.
HcTPS1, HcTPS3, HcTPS10, and HcBSMT2 are the bottom structural genes that are involved in the majority of the floral aroma production in H. coronarium (Yue et al., 2015) and contained MYB-binding elements in the promoter regions (Supplementary Table 10). The volatile organic compounds are diverse and play significant roles throughout the plant lifespan. The yeast-one-hybrid assay results showed that HcMYB7/145 can transactivate the promoter of HcTPS1, HcTPS3, and HcBSMT2, while HcMYB75/238 activates the promoter of HcTPS10. Moreover, HcMYB8 transactivates the promoter of HcTPS1, HcTPS3, HcTPS10, and HcBSMT2, while HcMYB248 activates the promoter of HcBSMT2. Furthermore, HcMYB79 binds to the promoters of HcTPS10 and HcBSMT2 (Figure 7A). ODO1, EOBI, and EOBII from Petunia regulate the biosynthetic pathway of benzenoids by regulating the transcript level of several key structure genes [CHORISMATE MUTASE (CM), PHENYLALANINE AMMONIA LYASE (PAL), 3-deoxy-d-arabino-heptulosonate-7-phosphate synthase (DAHPS), and S-adenosyl Met Synthase (SAMS)]. EOBI not only directly binds but also activates the promoters of PAL, ODO1, and ISOEUGENOL SYNTHASE (IGS) to regulate aroma production (Spitzer-Rimon et al., 2010, 2012; Saha et al., 2016). In strawberry, FaMYB10 regulates several structural genes and is involved in the biosynthesis of flavonoids and phenylpropanoids (Medina-Puche et al., 2014). Our findings indicate that HcMYB7/8/75/79/145/238/248 proteins directly activate these structural genes and are localized to the nucleus (Figures 5, 7B). Notably, the HcMYB8 protein was capable of activating the promoters of different volatile biosynthesis genes. AtMYB21 and AtMYB24 from Arabidopsis were involved in the production of sesquiterpenes (Tholl et al., 2005). In spearmint, MsMYB binds to the promoter region of geranyl diphosphate synthase (GPPS) and negatively regulates the production of monoterpenes by suppressing the activity of GPPS (Amarr et al., 2017). Moreover, to investigate the involvement of HcMYB7/8/79/145/248 in floral scent formation, the activity of these genes was suppressed via gene silencing (Figure 6). There was a significant decrease in the floral volatile contents and suppressed the expression of scent-related key genes in the HcMYB7/8/79/145/248-silenced flowers (Figure 6B). Likewise, in petunia, suppression of ODO1 downregulated the expression of numerous scent-related genes (Spitzer-Rimon et al., 2012). Overexpression of Vitis vinifera VvMYB5b in tomato encourages the accumulation of β-carotene (Mahjoub et al., 2009). LcMYB5 directly activated the expression of key structural genes and was involved in anthocyanin biosynthesis in litchi (Lai et al., 2019). Less information is available regarding the transcriptional regulatory mechanism of MYB genes in the floral biosynthetic pathway in non-model fragrance plants, such as H. coronarium.
The transcriptional complexes formed by protein–protein interactions between MYBs and other proteins are necessary to control gene expression (Ali and Baek, 2020). In Arabidopsis, AtJAZ proteins interact with MYBs, modulating the expression of key enzyme genes, such as C4H, in the anthocyanin biosynthesis pathway (Qi et al., 2011). Similarly, AtJAZ protein interacts with MYB21/24 to affect jasmonate-regulated stamen development (Xie et al., 2016). Further studies also revealed that JAZ proteins interact with the WD-repeat/bHLH/MYB complexes to regulate anthocyanin accumulation (Payne et al., 2000). In Fagopyrum tataricum, four MYB transcription factors (FtMYB13/14/15/16) interact with FtJAZ1 to regulate the accumulation of rutin (Zhang K. et al., 2018). In S. lycopersicum, application of JA induces defense protein accumulation and volatile emissions in plants (Degenhardt et al., 2010). Several JA-responsive genes are involved in the biosynthesis of numerous clades of secondary metabolism and responses to wounding and herbivores (Wu and Baldwin, 2010). In lima bean, it has been reported that the production of volatile compounds is regulated by JA (Dicke et al., 1999). In our study, the Y2H assay revealed that HcMYB7/8/75/145/248 interact with HcJAZ1 protein (Figure 7B). The volatile organic compounds are diverse and play significant roles in the plant lifespan. Our results will provide new insight into the mechanism of floral aroma production and role of MYB TFs in JA signaling transduction in non-model fragrance plants.
Phytohormones including auxin, ABA, ethylene, and MeJA are key hormones that regulate various plant developmental processes including flower development and senescence (Zubo et al., 2011; Wils and Kaufmann, 2017; Ke et al., 2019). Several cis-regulatory elements related to hormone response were predicted in the promoters of HcMYB genes, implying that HcMYB genes respond to hormones. The data showed that MeJA responsive elements were the most common in the promoter sequences of HcMYB genes followed by ABARE, ERE, GARE, auxin, SARE, MBS, and LTR (Supplementary Table 8 and Figure S6C). Likewise, the expression of AtMYB21 and AtMYB24 was rapidly induced under MeJA (Stracke et al., 2001). In B. distachyon, the expression of BdMYB78/BdMYB89 and Oryza sativa MYB91 was upregulated by ethylene, MeJA, and ABA treatment (Zhu et al., 2015; Chen S. et al., 2017). Similarly, in Tamarix hispida, the expression of the majority of MYB genes was significantly upregulated under ABA and MeJA hormone treatments (Zhang T. et al., 2018). It was observed that the total floral volatile contents were significantly increased under these hormone treatments. The expression level of HcMYB75/79/145/238 was significantly induced under auxin, MeJA, and ABA treatments, while HcMYB248 was significantly downregulated (Figure 4). Similarly, the expression of OsMYB511 and CMYB1 was induced by ABA treatment (Duan et al., 2014; Huang et al., 2015). Moreover, the expression of HcMYB79/145 was dramatically induced, while HcMYB7/8/248 was significantly downregulated under ethylene treatment. Our findings support the fact that MYB transcription factors regulate the floral aroma via controlling the key structural genes. The data indicate that under hormone treatments, the expression of HcMYB genes change, and the amount of floral volatile increased via upregulating the expression of key structural volatile biosynthesis genes, implying that the process of floral scent formation is associated with hormone signal transduction.
In this study, a total of 253 HcMYB genes were identified from H. coronarium genome. Gene structure analysis, chromosomal location, evolutionary relationship, and expression pattern were comprehensively analyzed. Moreover, functional characterization of important candidates HcMYB genes revealed that they play a key role in the biosynthesis and emission of floral aroma. Furthermore, subcellular localization and protein interaction analysis revealed that candidate HcMYB genes transactivate the promoters of key structural volatile synthesis genes. Overall, our findings will facilitate further investigation related to floral scent formation, which is very important for aromatic plant breeding.
All datasets generated for this study are included in the article/Supplementary Material and in Zenodo database doi: 10.5281/zenodo.4387064 (Repository link), further inquiries can be directed to the corresponding author/s.
FA, YF, and RY conceived and designed the concept and revised and finalized the manuscript. FA, YK, and YZ performed the experiments. FA, MW, YZ, and UA analyzed the data. CW, XW, YyY, XL, and YcY did the formal analysis. FA and YK drafted the manuscript. All authors endorsed the final version of the manuscript.
This work was supported by the National Natural Science Foundation of China (Grant no. 31770738), Key-Areas Research and Development Program of Guangdong Province (Grant no. 2020B0202022007), People’s Livelihood Science and Technology Projects of Guangzhou (Grant no. 201903010054) to YF, and National Natural Science Foundation of China to RY (Grant no. 31870690).
The authors declare that the research was conducted in the absence of any commercial or financial relationships that could be construed as a potential conflict of interest.
The Supplementary Material for this article can be found online at: https://www.frontiersin.org/articles/10.3389/fpls.2021.623742/full#supplementary-material
AbA, Aureobasidin A; ABA, Abscisic acid; Aux/IAA, Auxin/Indole-3-Acetic Acid; BSMT, Salicylic acid/benzoic acid methyltransferase; cDNA, Complementary DNA; GC-MS, Gas chromatography-mass spectrometer; GFP, Green fluorescent protein; NLS, Nuclear localization signal; TPS, Terpene synthase; DBD, DNA-binding domain; ERE, Ethylene responsive elements; GO, Gene Ontology; JA, Jasmonic acid; MeJA, methyl jasmonate; MEME, Multiple Em for Motif Elicitation; NJ, Neighbor-Joining; ORF, Open reading frame; PlantTFDB, Plant Transcription Factor Database; RNA-seq, RNA-sequencing; SA, Salicylic acid; SMART, Simple Modular Architecture Research Tool; TAIR, The Arabidopsis Information Resource; ABRE, ABA-responsive element; CDS, coding domain sequence; Ka, non-synonymous substitution rate; Ks, synonymous substitution rate; Mya, million years ago; qRT-PCR, quantitative real-time PCR.
Abbas, F., Ke, Y., Yu, R., and Fan, Y. (2019). Functional characterization and expression analysis of two terpene synthases involved in floral scent formation in Lilium ‘Siberia’. Planta 249, 71–93. doi: 10.1007/s00425-018-3006-7
Abbas, F., Ke, Y., Yu, R., Yue, Y., Amanullah, S., Jahangir, M. M., et al. (2017). Volatile terpenoids: multiple functions, biosynthesis, modulation and manipulation by genetic engineering. Planta 246, 803–816. doi: 10.1007/s00425-017-2749-x
Abbas, F., Ke, Y., Zhou, Y., Waseem, M., Yu, Y., Ashraf, U., et al. (2020). Cloning, functional characterization and expression analysis of LoTPS5 from Lilium ‘Siberia’. Gene 756:144921. doi: 10.1016/j.gene.2020.144921
Ali, M., and Baek, K. H. (2020). Jasmonic acid signaling pathway in response to abiotic stresses in plants. Int. J. Mol. Sci. 21:621. doi: 10.3390/ijms21020621
Amarr, V., Nori, P., and Zhu, H. (2017). Spearmint R2R3â MYB transcription factor MsMYB negatively regulates monoterpene production and suppresses the expression of geranyl diphosphate synthase large subunit (MsGPPS.LSU). Plant Biotechnol. J. 15, 1105–1119. doi: 10.1111/pbi.12701
Amoutzias, G., Veron, A., Weiner, J. III, Robinson-Rechavi, M., Bornberg-Bauer, E., Oliver, S., et al. (2007). One billion years of bZIP transcription factor evolution: conservation and change in dimerization and DNA-binding site specificity. Mol. Biol. Evol. 24, 827–835. doi: 10.1093/molbev/msl211
Báez, D., Pino, J. A., and Morales, D. (2011). Floral scent composition in Hedychium coronarium J. Koenig analyzed by SPME. J. Essent. Oil Res. 23, 64–67. doi: 10.1080/10412905.2011.9700460
Bailey, T. L., Johnson, J., Grant, C. E., and Noble, W. S. (2015). The MEME suite. Nucleic Acids Res. 43, W39–W49. doi: 10.1093/nar/gkv416
Baumann, K., Perez-Rodriguez, M., Bradley, D., Venail, J., Bailey, P., Jin, H., et al. (2007). Control of cell and petal morphogenesis by R2R3 MYB transcription factors. Development 134, 1691–1701. doi: 10.1242/dev.02836
Bedon, F., Bomal, C., Caron, S., Levasseur, C., Boyle, B., Mansfield, S. D., et al. (2010). Subgroup 4 R2R3-MYBs in conifer trees: gene family expansion and contribution to the isoprenoid-and flavonoid-oriented responses. J. Exp. Bot. 61, 3847–3864. doi: 10.1093/jxb/erq196
Bovy, A., Schijlen, E., and Hall, R. D. (2007). Metabolic engineering of flavonoids in tomato (Solanum lycopersicum): the potential for metabolomics. Metabolomics 3, 399–412. doi: 10.1007/s11306-007-0074-2
Brownfield, L., Hafidh, S., Borg, M., Sidorova, A., Mori, T., and Twell, D. (2009). A plant germline-specific integrator of sperm specification and cell cycle progression. PLoS Genet. 5:e1000430. doi: 10.1371/journal.pgen.1000430
Cannon, S. B., Mitra, A., Baumgarten, A., Young, N. D., and May, G. (2004). The roles of segmental and tandem gene duplication in the evolution of large gene families in Arabidopsis thaliana. BMC Plant Biol. 4:10. doi: 10.1186/1471-2229-4-10
Cao, Y., Li, K., Li, Y., Zhao, X., and Wang, L. (2020). MYB transcription factors as regulators of secondary metabolism in plants. Biology 9:61. doi: 10.3390/biology9030061
Cao, Y., Xie, L., Ma, Y., Ren, C., Xing, M., Fu, Z., et al. (2018). PpMYB15 and PpMYBF1 transcription factors are involved in regulating flavonol biosynthesis in peach fruit. J. Agric. Food Chem. 67, 644–652. doi: 10.1021/acs.jafc.8b04810
Chen, K., Du, L., Liu, H., and Liu, Y. (2019). A novel R2R3-MYB from grape hyacinth, MaMybA, which is different from MaAN2, confers intense and magenta anthocyanin pigmentation in tobacco. BMC Plant Biol. 19:390. doi: 10.1186/s12870-019-1999-0
Chen, S., Niu, X., Guan, Y., and Li, H. (2017). Genome-wide analysis and expression profiles of the MYB genes in Brachypodium distachyon. Plant Cell Physiol. 58, 1777–1788. doi: 10.1093/pcp/pcx115
Chen, T., Li, W., Hu, X., Guo, J., Liu, A. and Zhang, B. (2015). A cotton MYB transcription factor, GbMYB5, is positively involved in plant adaptive response to drought stress. Plant Cell Physiol. 56, 917–929. doi: 10.1093/pcp/pcv019
Chen, Y.-S., Chao, Y.-C., Tseng, T.-W., Huang, C.-K., Lo, P.-C., and Lu, C.-A. (2017). Two MYB-related transcription factors play opposite roles in sugar signaling in Arabidopsis. Plant Mol. Biol. 93, 299–311. doi: 10.1007/s11103-016-0562-8
Davidson, C. J., Guthrie, E. E., and Lipsick, J. S. (2013). Duplication and maintenance of the Myb genes of vertebrate animals. Biol. Open 2, 101–110. doi: 10.1242/bio.20123152
Degenhardt, D. C., Refi-Hind, S., Stratmann, J. W., and Lincoln, D. E. (2010). Systemin and jasmonic acid regulate constitutive and herbivore-induced systemic volatile emissions in tomato, Solanum lycopersicum. Phytochemistry 71, 2024–2037. doi: 10.1016/j.phytochem.2010.09.010
Dicke, M., Gols, R., Ludeking, D., and Posthumus, M. A. (1999). Jasmonic acid and herbivory differentially induce carnivore-attracting plant volatiles in lima bean plants. J. Chem. Ecol. 25, 1907–1922. doi: 10.1023/A:1020942102181
Du, H., Yang, S.-S., Liang, Z., Feng, B.-R., Liu, L., Huang, Y.-B., et al. (2012). Genome-wide analysis of the MYB transcription factor superfamily in soybean. BMC Plant Biol. 12:106. doi: 10.1186/1471-2229-12-106
Du, H., Zhang, L., Liu, L., Tang, X.-F., Yang, W.-J., Wu, Y.-M., et al. (2009). Biochemical and molecular characterization of plant MYB transcription factor family. Biochemistry (Mosc.) 74, 1–11. doi: 10.1134/s0006297909010015
Duan, M., Huang, P., Yuan, X., Chen, H., Huang, J., and Zhang, H. (2014). CMYB1 encoding a MYB transcriptional activator is involved in abiotic stress and circadian rhythm in rice. Sci. World J. 2014:178038. doi: 10.1155/2014/178038
Dubos, C., Le Gourrierec, J., Baudry, A., Huep, G., Lanet, E., Debeaujon, I., et al. (2008). MYBL2 is a new regulator of flavonoid biosynthesis in Arabidopsis thaliana. Plant J. 55, 940–953. doi: 10.1111/j.1365-313X.2008.03564.x
Dubos, C., Stracke, R., Grotewold, E., Weisshaar, B., Martin, C., and Lepiniec, L. (2010). MYB transcription factors in Arabidopsis. Trends Plant Sci. 15, 573–581. doi: 10.1016/j.tplants.2010.06.005
Dudareva, N., Klempien, A., Muhlemann, J. K., and Kaplan, I. (2013). Biosynthesis, function and metabolic engineering of plant volatile organic compounds. New Phytol. 198, 16–32. doi: 10.1111/nph.12145
El-Azaz, J., de la Torre, F., Pascual, M. B., Debille, S., Canlet, F., Harvengt, L., et al. (2020). Transcriptional analysis of arogenate dehydratase genes identifies a link between phenylalanine biosynthesis and lignin biosynthesis. J. Exp. Bot. 71, 3080–3093. doi: 10.1093/jxb/eraa099
Fan, Y., Yu, R., Huang, Y., and Chen, Y. (2003). Studies on the essential constituent of Hedychium flavum and H. coronarium. Acta Horti. Sin. 30:475. doi: 10.16420/j.issn.0513-353x.2003.04.030
Fan, Y.-p, Wang, X.-r, Yu, R.-c, and Yang, P. (2007). Analysis on the aroma components in several species of Hedychium. Acta Horti. Sin. 34, 231–234. doi: 10.16420/j.issn.0513-353x.2007.01.049
Fenske, M. P., Hazelton, K. D. H., Hempton, A. K., Shim, J. S., Yamamoto, B. M., Riffell, J. A., et al. (2015). Circadian clock gene LATE ELONGATED HYPOCOTYL directly regulates the timing of floral scent emission in Petunia. Proc. Natl. Acad. Sci. U.S.A. 112, 9775–9780. doi: 10.1073/pnas.1422875112
Fornalé, S., Lopez, E., Salazar-Henao, J. E., Fernández-Nohales, P., Rigau, J., and Caparros-Ruiz, D. (2014). AtMYB7, a new player in the regulation of UV-sunscreens in Arabidopsis thaliana. Plant Cell Physiol. 55, 507–516. doi: 10.1093/pcp/pct187
Higo, K., Ugawa, Y., Iwamoto, M., and Korenaga, T. (1999). Plant cis-acting regulatory DNA elements (PLACE) database: 1999. Nucleic Acids Res. 27, 297–300. doi: 10.1093/nar/27.1.297
Hu, B., Jin, J., Guo, A.-Y., Zhang, H., Luo, J., and Gao, G. (2015). GSDS 2.0: an upgraded gene feature visualization server. Bioinformatics 31, 1296–1297. doi: 10.1093/bioinformatics/btu817
Huang, P., Chen, H., Mu, R., Yuan, X., Zhang, H., and Huang, J. (2015). OsMYB511 encodes a MYB domain transcription activator early regulated by abiotic stress in rice. Genet. Mol. Res. 14, 9506–9517. doi: 10.4238/2015.August.14.14
Jiang, C., Gu, J., Chopra, S., Gu, X., and Peterson, T. (2004). Ordered origin of the typical two-and three-repeat Myb genes. Gene 326, 13–22. doi: 10.1016/j.gene.2003.09.049
Jin, H., Cominelli, E., Bailey, P., Parr, A., Mehrtens, F., Jones, J., et al. (2000). Transcriptional repression by AtMYB4 controls production of UV−protecting sunscreens in Arabidopsis. EMBO J. 19, 6150–6161. doi: 10.1093/emboj/19.22.6150
Johnson, E. T., and Dowd, P. F. (2004). Differentially enhanced insect resistance, at a cost, in Arabidopsis thaliana constitutively expressing a transcription factor of defensive metabolites. J. Agric. Food Chem. 52, 5135–5138. doi: 10.1021/jf0308049
Jung, C., Seo, J. S., Han, S. W., Koo, Y. J., Kim, C. H., Song, S. I., et al. (2008). Overexpression of AtMYB44 enhances stomatal closure to confer abiotic stress tolerance in transgenic Arabidopsis. Plant Physiol. 146, 623–635. doi: 10.1104/pp.107.110981
Katiyar, A., Smita, S., Lenka, S. K., Rajwanshi, R., Chinnusamy, V., and Bansal, K. C. (2012). Genome-wide classification and expression analysis of MYB transcription factor families in rice and Arabidopsis. BMC Genomics 13:544. doi: 10.1186/1471-2164-13-544
Ke, Y., Abbas, F., Zhou, Y., Yu, R., Yue, Y., Li, X., et al. (2019). Genome-wide analysis and characterization of the Aux/IAA family genes related to floral scent formation in Hedychium coronarium. Int. J. Mol. Sci. 20:3235. doi: 10.3390/ijms20133235
Klempnauer, K.-H., Gonda, T. J., and Bishop, J. M. (1982). Nucleotide sequence of the retroviral leukemia gene v-myb and its cellular progenitor c-myb: the architecture of a transduced oncogene. Cell 31, 453–463. doi: 10.1016/0092-8674(82)90138-6
Kumar, S., Stecher, G., Li, M., Knyaz, C., and Tamura, K. (2018). MEGA X: molecular evolutionary genetics analysis across computing platforms. Mol. Biol. Evol. 35, 1547–1549. doi: 10.1093/molbev/msy096
Lai, B., Du, L.-N., Hu, B., Wang, D., Huang, X.-M., Zhao, J.-T., et al. (2019). Characterization of a novel litchi R2R3-MYB transcription factor that involves in anthocyanin biosynthesis and tissue acidification. BMC Plant Biol. 19:62. doi: 10.1186/s12870-019-1658-5
Lan, J.-b, Yu, R.-c, Yu, Y.-y, and Fan, Y.-p (2013). Molecular cloning and expression of Hedychium coronarium farnesyl pyrophosphate synthase gene and its possible involvement in the biosynthesis of floral and wounding/herbivory induced leaf volatile sesquiterpenoids. Gene 518, 360–367. doi: 10.1016/j.gene.2013.01.007
Lee, Y.-T., Jacob, J., Michowski, W., Nowotny, M., Kuznicki, J., and Chazin, W. J. (2004). Human Sgt1 binds HSP90 through the CHORD-Sgt1 domain and not the tetratricopeptide repeat domain. J. Biol. Chem. 279, 16511–16517. doi: 10.1074/jbc.M400215200
Lescot, M., Déhais, P., Thijs, G., Marchal, K., Moreau, Y., Van de Peer, Y., et al. (2002). PlantCARE, a database of plant cis-acting regulatory elements and a portal to tools for in silico analysis of promoter sequences. Nucleic Acids Res. 30, 325–327. doi: 10.1093/nar/30.1.325
Letunic, I., Doerks, T., and Bork, P. (2015). SMART: recent updates, new developments and status in 2015. Nucleic Acids Res. 43, D257–D260. doi: 10.1093/nar/gku949
Li, X., Xue, C., Li, J., Qiao, X., Li, L., and Yu, L. a, et al. (2016). Genome-wide identification, evolution and functional divergence of MYB transcription factors in Chinese white pear (Pyrus bretschneideri). Plant Cell Physiol. 57, 824–847. doi: 10.1093/pcp/pcw029
Li, Z., Peng, R., Tian, Y., Han, H., Xu, J., and Yao, Q. (2016). Genome-wide identification and analysis of the MYB transcription factor superfamily in Solanum lycopersicum. Plant Cell Physiol. 57, 1657–1677. doi: 10.1093/pcp/pcw091
Liu, C., Hao, J., Qiu, M., Pan, J., and He, Y. (2020). Genome-wide identification and expression analysis of the MYB transcription factor in Japanese plum (Prunus salicina). Genomics 112, 4875–4886. doi: 10.1016/j.ygeno.2020.08.018
Liu, C., Wang, X., Xu, Y., Deng, X., and Xu, Q. (2014). Genome-wide analysis of the R2R3-MYB transcription factor gene family in sweet orange (Citrus sinensis). Mol. Biol. Rep. 41, 6769–6785. doi: 10.1007/s11033-014-3563-1
Liu, C., Xie, T., Chen, C., Luan, A., Long, J., Li, C., et al. (2017). Genome-wide organization and expression profiling of the R2R3-MYB transcription factor family in pineapple (Ananas comosus). BMC Genomics 18:503. doi: 10.1186/s12864-017-3896-y
Liu, R., Lü, B., Wang, X., Zhang, C., Zhang, S., Qian, J., et al. (2010). Thirty-seven transcription factor genes differentially respond to a hairpin protein and affect resistance to the green peach aphid in Arabidopsis. J. Biosci. 35, 435–450. doi: 10.1007/s12038-010-0049-8
Livak, K. J., and Schmittgen, T. D. (2001). Analysis of relative gene expression data using real-time quantitative PCR and the 2−ΔΔCT method. Methods 25, 402–408. doi: 10.1006/meth.2001.1262
Mahjoub, A., Hernould, M., Joubès, J., Decendit, A., Mars, M., Barrieu, F., et al. (2009). Overexpression of a grapevine R2R3-MYB factor in tomato affects vegetative development, flower morphology and flavonoid and terpenoid metabolism. Plant Physiol. Biochem. 47, 551–561. doi: 10.1016/j.plaphy.2009.02.015
Marchler-Bauer, A., Derbyshire, M. K., Gonzales, N. R., Lu, S., Chitsaz, F., Geer, L. Y., et al. (2015). CDD: NCBI’s conserved domain database. Nucleic Acids Res. 43, D222–D226. doi: 10.1093/nar/gku1221
Martin, C., and Paz-Ares, J. (1997). MYB transcription factors in plants. Trends Genet. 13, 67–73. doi: 10.1016/s0168-9525(96)10049-4
Matsumoto, F., Idetsuki, H., Harada, K., Nohara, I., and Toyoda, T. (1993). Volatile components of Hedychium coronarium Koenig flowers. J. Essent. Oil Res. 5, 123–133. doi: 10.1080/10412905.1993.9698190
McCarthy, R. L., Zhong, R., Fowler, S., Lyskowski, D., Piyasena, H., Carleton, K., et al. (2010). The poplar MYB transcription factors, PtrMYB3 and PtrMYB20, are involved in the regulation of secondary wall biosynthesis. Plant Cell Physiol. 51, 1084–1090. doi: 10.1093/pcp/pcq064
Medina-Puche, L., Cumplido-Laso, G., Amil-Ruiz, F., Hoffmann, T., Ring, L., Rodríguez-Franco, A., et al. (2014). MYB10 plays a major role in the regulation of flavonoid/phenylpropanoid metabolism during ripening of Fragaria× ananassa fruits. J. Exp. Bot. 65, 401–417. doi: 10.1093/jxb/ert377
Medina-Puche, L., Molina-Hidalgo, F. J., Boersma, M., Schuurink, R. C., López-Vidriero, I., Solano, R., et al. (2015). An R2R3-MYB transcription factor regulates eugenol production in ripe strawberry fruit receptacles. Plant Physiol. 168, 598–614. doi: 10.1104/pp.114.252908
Millar, A. A., and Gubler, F. (2005). The Arabidopsis GAMYB-like genes, MYB33 and MYB65, are microRNA-regulated genes that redundantly facilitate anther development. Plant Cell 17, 705–721. doi: 10.1105/tpc.104.027920
Muhlemann, J. K., Klempien, A., and Dudareva, N. (2014). Floral volatiles: from biosynthesis to function. Plant Cell Environ. 37, 1936–1949. doi: 10.1111/pce.12314
Ogata, K., Kanei-Ishii, C., Sasaki, M., Hatanaka, H., Nagadoi, A., Enari, M., et al. (1996). The cavity in the hydrophobic core of Myb DNA-binding domain is reserved for DNA recognition and trans-activation. Nat. Struct. Mol. Biol. 3, 178–187. doi: 10.1038/nsb0296-178
Payne, C. T., Zhang, F., and Lloyd, A. M. (2000). GL3 encodes a bHLH protein that regulates trichome development in arabidopsis through interaction with GL1 and TTG1. Genetics 156, 1349–1362.
Paz-Ares, J., Ghosal, D., Wienand, U., Peterson, P., and Saedler, H. (1987). The regulatory c1 locus of Zea mays encodes a protein with homology to myb proto−oncogene products and with structural similarities to transcriptional activators. EMBO J. 6, 3553–3558. doi: 10.1002/j.1460-2075.1987.tb02684.x
Pérez-Díaz, J. R., Pérez-Díaz, J., Madrid-Espinoza, J., González-Villanueva, E., Moreno, Y., and Ruiz-Lara, S. (2016). New member of the R2R3-MYB transcription factors family in grapevine suppresses the anthocyanin accumulation in the flowers of transgenic tobacco. Plant Mol. Biol. 90, 63–76. doi: 10.1007/s11103-015-0394-y
Pu, X., Yang, L., Liu, L., Dong, X., Chen, S., Chen, Z., et al. (2020). Genome-wide analysis of the MYB transcription factor superfamily in Physcomitrella patens. Int. J. Mol. Sci. 21:975. doi: 10.3390/ijms21030975
Qi, T., Song, S., Ren, Q., Wu, D., Huang, H., Chen, Y., et al. (2011). The Jasmonate-ZIM-domain proteins interact with the WD-Repeat/bHLH/MYB complexes to regulate Jasmonate-mediated anthocyanin accumulation and trichome initiation in Arabidopsis thaliana. Plant Cell 23, 1795–1814. doi: 10.1105/tpc.111.083261
Rawat, R., Schwartz, J., Jones, M. A., Sairanen, I., Cheng, Y., Andersson, C. R., et al. (2009). REVEILLE1, a Myb-like transcription factor, integrates the circadian clock and auxin pathways. Proc. Natl. Acad. Sci. U.S.A. 106, 16883–16888. doi: 10.1073/pnas.0813035106
Renner, T., Bragg, J., Driscoll, H. E., Cho, J., Jackson, A. O., and Specht, C. D. (2009). Virus-induced gene silencing in the culinary ginger (Zingiber officinale): an effective mechanism for down-regulating gene expression in tropical monocots. Mol. Plant 2, 1084–1094. doi: 10.1093/mp/ssp033
Riechmann, J. L., Heard, J., Martin, G., Reuber, L., Jiang, C.-Z., Keddie, J., et al. (2000). Arabidopsis transcription factors: genome-wide comparative analysis among eukaryotes. Science 290, 2105–2110. doi: 10.1126/science.290.5499.2105
Rosinski, J. A., and Atchley, W. R. (1998). Molecular evolution of the Myb family of transcription factors: evidence for polyphyletic origin. J.Mol. Evol. 46, 74–83. doi: 10.1007/pl00006285
Saha, G., Park, J.-I., Ahmed, N. U., Kayum, M. A., Kang, K.-K., and Nou, I.-S. (2016). Characterization and expression profiling of MYB transcription factors against stresses and during male organ development in Chinese cabbage (Brassica rapa ssp. pekinensis). Plant Physiol. Biochem. 104, 200–215. doi: 10.1016/j.plaphy.2016.03.021
Salih, H., Gong, W., He, S., Sun, G., Sun, J., and Du, X. (2016). Genome-wide characterization and expression analysis of MYB transcription factors in Gossypium hirsutum. BMC Genet. 17:129. doi: 10.1186/s12863-016-0436-8
Seo, P. J., Xiang, F., Qiao, M., Park, J.-Y., Lee, Y. N., Kim, S.-G., et al. (2009). The MYB96 transcription factor mediates abscisic acid signaling during drought stress response in Arabidopsis. Plant Physiol. 151, 275–289. doi: 10.1104/pp.109.144220
Sharma, A., Badola, P. K., Bhatia, C., Sharma, D. and Trivedi, P. K. (2020). Primary transcript of miR858 encodes regulatory peptide and controls flavonoid biosynthesis and development in Arabidopsis. Nat. Plants 6, 1262–1274. doi: 10.1038/s41477-020-00769-x
Shin, B., Choi, G., Yi, H., Yang, S., Cho, I., Kim, J., et al. (2002). AtMYB21, a gene encoding a flower−specific transcription factor, is regulated by COP1. Plant J. 30, 23–32. doi: 10.1046/j.1365-313x.2002.01264.x
Sievers, F., Wilm, A., Dineen, D., Gibson, T. J., Karplus, K., Li, W., et al. (2011). Fast, scalable generation of high−quality protein multiple sequence alignments using Clustal Omega. Mol. Syst. Biol. 7:539. doi: 10.1038/msb.2011.75
Sonawane, A. R., Platig, J., Fagny, M., Chen, C.-Y., Paulson, J. N., Lopes-Ramos, C. M., et al. (2017). Understanding tissue-specific gene regulation. Cell Rep. 21, 1077–1088. doi: 10.1016/j.celrep.2017.10.001
Spitzer-Rimon, B., Farhi, M., Albo, B., Cna’ani, A., Zvi, M. M. B., Masci, T., et al. (2012). The R2R3-MYB–like regulatory factor EOBI, acting downstream of EOBII, regulates scent production by activating ODO1 and structural scent-related genes in Petunia. Plant Cell 24, 5089–5105. doi: 10.1105/tpc.112.105247
Spitzer-Rimon, B., Marhevka, E., Barkai, O., Marton, I., Edelbaum, O., Masci, T., et al. (2010). EOBII, a gene encoding a flower-specific regulator of phenylpropanoid volatiles’ biosynthesis in Petunia. Plant Cell 22, 1961–1976. doi: 10.1105/tpc.109.067280
Stracke, R., Werber, M., and Weisshaar, B. (2001). The R2R3-MYB gene family in Arabidopsis thaliana. Curr. Opin. Plant Biol. 4, 447–456. doi: 10.1016/s1369-5266(00)00199-0
Sun, W., Ma, Z., Chen, H., and Liu, M. (2019). MYB gene family in potato (Solanum tuberosum L.): genome-wide identification of hormone-responsive reveals their potential functions in growth and development. Int. J. Mol. Sci. 20:4847. doi: 10.3390/ijms20194847
Tang, H., Bowers, J. E., Wang, X., Ming, R., Alam, M., and Paterson, A. H. (2008). Synteny and collinearity in plant genomes. Science 320, 486–488. doi: 10.1126/science.1153917
Tholl, D., Chen, F., Petri, J., Gershenzon, J., and Pichersky, E. (2005). Two sesquiterpene synthases are responsible for the complex mixture of sesquiterpenes emitted from Arabidopsis flowers. Plant J. 42, 757–771. doi: 10.1111/j.1365-313X.2005.02417.x
Tuan, P. A., Bai, S., Yaegaki, H., Tamura, T., Hihara, S., Moriguchi, T., et al. (2015). The crucial role of PpMYB10.1 in anthocyanin accumulation in peach and relationships between its allelic type and skin color phenotype. BMC Plant Biol. 15:280. doi: 10.1186/s12870-015-0664-5
Van Moerkercke, A., Haring, M. A., and Schuurink, R. C. (2011). The transcription factor EMISSION OF BENZENOIDS II activates the MYB ODORANT1 promoter at a MYB binding site specific for fragrant Petunias. Plant J. 67, 917–928. doi: 10.1111/j.1365-313X.2011.04644.x
Van Moerkercke, A., Haring, M. A., and Schuurink, R. C. (2012). A model for combinatorial regulation of the Petunia R2R3-MYB transcription factor ODORANT1. Plant Signal. Behav. 7, 518–520. doi: 10.4161/psb.19311
Verdonk, J. C., Haring, M. A., van Tunen, A. J., and Schuurink, R. C. (2005). ODORANT1 regulates fragrance biosynthesis in Petunia flowers. Plant Cell 17, 1612–1624. doi: 10.1105/tpc.104.028837
Vimolmangkang, S., Han, Y., Wei, G., and Korban, S. S. (2013). An apple MYB transcription factor, MdMYB3, is involved in regulation of anthocyanin biosynthesis and flower development. BMC Plant Biol. 13:176. doi: 10.1186/1471-2229-13-176
Wang, J., Fuguang, L. I., Qianru, L. I., and Universi, Z. (2002). Construction of a screening vector by cloning the green fluorescent protein gene into the plasmid pUC18. J. Henan Med. Univ. 37, 621–624. doi: 10.13705/j.issn.1671-6825.2002.05.029
Wang, X., Niu, Q.-W., Teng, C., Li, C., Mu, J., Chua, N.-H., et al. (2009). Overexpression of PGA37/MYB118 and MYB115 promotes vegetative-to-embryonic transition in Arabidopsis. Cell Res. 19, 224–235. doi: 10.1038/cr.2008.276
Wang, Y., Liu, W., Wang, X., Yang, R., Wu, Z., Wang, H., et al. (2020). MiR156 regulates anthocyanin biosynthesis through SPL targets and other microRNAs in poplar. Hortic. Res. 7:118. doi: 10.1038/s41438-020-00341-w
Wils, C. R., and Kaufmann, K. (2017). Gene-regulatory networks controlling inflorescence and flower development in Arabidopsis thaliana. Biochim. Biophys. Acta Gene Regul. Mech. 1860, 95–105. doi: 10.1016/j.bbagrm.2016.07.014
Wu, J., and Baldwin, I. T. (2010). New insights into plant responses to the attack from insect herbivores. Ann. Rev. Genet. 44, 1–24. doi: 10.1146/annurev-genet-102209-163500
Xie, Y., Tan, H., Ma, Z., and Huang, J. (2016). DELLA proteins promote anthocyanin biosynthesis via sequestering MYBL2 and JAZ suppressors of the MYB/bHLH/WD40 complex in Arabidopsis thaliana. Mol. Plant 9, 711–721. doi: 10.1016/j.molp.2016.01.014
Yan, J., Wang, B., Zhong, Y., Yao, L., Cheng, L., and Wu, T. (2015). The soybean R2R3 MYB transcription factor GmMYB100 negatively regulates plant flavonoid biosynthesis. Plant Mol. Biol. 89, 35–48. doi: 10.1007/s11103-015-0349-3
Yang, J., Duan, G., Li, C., Liu, L., Han, G., Zhang, Y., et al. (2019a). The crosstalks between jasmonic acid and other plant hormone signaling highlight the involvement of jasmonic acid as a core component in plant response to biotic and abiotic stresses. Front. Plant Sci. 10:1349. doi: 10.3389/fpls.2019.01349
Yang, K., Li, Y., Wang, S., Xu, X., Sun, H., Zhao, H., et al. (2019b). Genome-wide identification and expression analysis of the MYB transcription factor in moso bamboo (Phyllostachys edulis). PeerJ 6:e6242. doi: 10.7717/peerj.6242
Yi, J., Derynck, M. R., Li, X., Telmer, P., Marsolais, F., and Dhaubhadel, S. (2010). A single−repeat MYB transcription factor, GmMYB176, regulates CHS8 gene expression and affects isoflavonoid biosynthesis in soybean. Plant J. 62, 1019–1034. doi: 10.1111/j.1365-313X.2010.04214.x
Yoo, S. D., Cho, Y. H., and Sheen, J. (2007). Arabidopsis mesophyll protoplasts: a versatile cell system for transient gene expression analysis. Nat. Protoc. 2, 1565–1572. doi: 10.1038/nprot.2007.199
Yuan, C., Li, C., Yan, L., Jackson, A. O., Liu, Z., Han, C., et al. (2011). A high throughput barley stripe mosaic virus vector for virus induced gene silencing in monocots and dicots. PLoS One 6:e26468. doi: 10.1371/journal.pone.0026468
Yue, Y., Yu, R., and Fan, Y. (2014). Characterization of two monoterpene synthases involved in floral scent formation in Hedychium coronarium. Planta 240, 745–762. doi: 10.1007/s00425-014-2127-x
Yue, Y., Yu, R., and Fan, Y. (2015). Transcriptome profiling provides new insights into the formation of floral scent in Hedychium coronarium. BMC Genomics 16:470. doi: 10.1186/s12864-015-1653-7
Zhang, C., Ma, R., Xu, J., Yan, J., Guo, L., Song, J., et al. (2018). Genome-wide identification and classification of MYB superfamily genes in peach. PLoS One 13:e0199192. doi: 10.1371/journal.pone.0199192
Zhang, K., Logacheva, M. D., Meng, Y., Hu, J., Wan, D., Li, L., et al. (2018). Jasmonate-responsive MYB factors spatially repress rutin biosynthesis in Fagopyrum tataricum. J. Exp. Bot. 69, 1955–1966. doi: 10.1093/jxb/ery032
Zhang, T., Zhao, Y., Wang, Y., Liu, Z., and Gao, C. (2018). Comprehensive analysis of MYB gene family and their expressions under abiotic stresses and hormone treatments in Tamarix hispida. Front. Plant Sci. 9:1303. doi: 10.3389/fpls.2018.01303
Zhou, M., and Memelink, J. (2016). Jasmonate-responsive transcription factors regulating plant secondary metabolism. Biotechnol. Adv. 34, 441–449. doi: 10.1016/j.biotechadv.2016.02.004
Zhu, N., Cheng, S., Liu, X., Du, H., Dai, M., Zhou, D.-X., et al. (2015). The R2R3-type MYB gene OsMYB91 has a function in coordinating plant growth and salt stress tolerance in rice. Plant Sci. 236, 146–156. doi: 10.1016/j.plantsci.2015.03.023
Keywords: Hedychium coronarium, MYB, terpenes, floral scent, structural genes
Citation: Abbas F, Ke Y, Zhou Y, Yu Y, Waseem M, Ashraf U, Wang C, Wang X, Li X, Yue Y, Yu R and Fan Y (2021) Genome-Wide Analysis Reveals the Potential Role of MYB Transcription Factors in Floral Scent Formation in Hedychium coronarium. Front. Plant Sci. 12:623742. doi: 10.3389/fpls.2021.623742
Received: 02 November 2020; Accepted: 11 January 2021;
Published: 26 February 2021.
Edited by:
Supaart Sirikantaramas, Chulalongkorn University, ThailandReviewed by:
Wajid Waheed Bhat, Michigan State University, United StatesCopyright © 2021 Abbas, Ke, Zhou, Yu, Waseem, Ashraf, Wang, Wang, Li, Yue, Yu and Fan. This is an open-access article distributed under the terms of the Creative Commons Attribution License (CC BY). The use, distribution or reproduction in other forums is permitted, provided the original author(s) and the copyright owner(s) are credited and that the original publication in this journal is cited, in accordance with accepted academic practice. No use, distribution or reproduction is permitted which does not comply with these terms.
*Correspondence: Yanping Fan, ZmFueWFucGluZ0BzY2F1LmVkdS5jbg==
†These authors have contributed equally to this work
Disclaimer: All claims expressed in this article are solely those of the authors and do not necessarily represent those of their affiliated organizations, or those of the publisher, the editors and the reviewers. Any product that may be evaluated in this article or claim that may be made by its manufacturer is not guaranteed or endorsed by the publisher.
Research integrity at Frontiers
Learn more about the work of our research integrity team to safeguard the quality of each article we publish.