- 1Department of Physiology and Cell Biology, Leibniz Institute of Plant Genetics and Crop Plant Research, Gatersleben, Germany
- 2Division of Molecular Biology, Centre of the Region Hana for Biotechnological and Agriculture Research, Faculty of Science, Palacký University, Olomouc, Czechia
- 3Department of Biology, Payame Noor University, Teheran, Iran
- 4Instituto de Biología Molecular y Celular de Rosario (IBR-UNR/CONICET), Facultad de Ciencias Bioquímicas y Farmacéuticas, Universidad Nacional de Rosario, Rosario, Argentina
Chloroplasts, the sites of photosynthesis in higher plants, have evolved several means to tolerate short episodes of drought stress through biosynthesis of diverse metabolites essential for plant function, but these become ineffective when the duration of the stress is prolonged. Cyanobacteria are the closest bacterial homologs of plastids with two photosystems to perform photosynthesis and to evolve oxygen as a byproduct. The presence of Flv genes encoding flavodiiron proteins has been shown to enhance stress tolerance in cyanobacteria. In an attempt to support the growth of plants exposed to drought, the Synechocystis genes Flv1 and Flv3 were expressed in barley with their products being targeted to the chloroplasts. The heterologous expression of both Flv1 and Flv3 accelerated days to heading, increased biomass, promoted the number of spikes and grains per plant, and improved the total grain weight per plant of transgenic lines exposed to drought. Improved growth correlated with enhanced availability of soluble sugars, a higher turnover of amino acids and the accumulation of lower levels of proline in the leaf. Flv1 and Flv3 maintained the energy status of the leaves in the stressed plants by converting sucrose to glucose and fructose, immediate precursors for energy production to support plant growth under drought. The results suggest that sugars and amino acids play a fundamental role in the maintenance of the energy status and metabolic activity to ensure growth and survival under stress conditions, that is, water limitation in this particular case. Engineering chloroplasts by Flv genes into the plant genome, therefore, has the potential to improve plant productivity wherever drought stress represents a significant production constraint.
Introduction
Drought poses a major constraint over crop productivity, both directly and through its aggravation of the impact of other stress factors (Wang et al., 2011; Gómez et al., 2019). Biochemical mechanisms of drought stress response have been presented to explain the carbon catabolite changes on primary metabolites utilization (Fàbregas and Fernie, 2019) and secondary metabolite production (Hodaei et al., 2018). In general, plants respond to water restriction by closing their stomata, which in turn decreases the supply of the CO2 needed for carbon assimilation via the Calvin-Benson cycle (CBC) and ultimately, starch synthesis (Lawlor and Tezara, 2009). A limitation in carbon assimilation results in down-regulation of carbohydrate metabolism, which serves as an immediate precursor for the production of e.g., amino acids and/or energy donors such as nucleotides. Thus, the balancing of biochemical processes, especially carbohydrate and nitrogen metabolisms and the concomitant pathways including glycolysis and the TCA cycle during stress is of great importance for plants to tolerate adverse conditions. Knowledge gained on the nature of plant stress responses allowed the development of various experimental strategies to improve drought tolerance (Pires et al., 2016; Fàbregas et al., 2018).
Limitations in the fixation of atmospheric CO2, whether caused by internal or external factors, will result in over-reduction of the photosynthetic electron transport chain (PETC) in chloroplasts, leading to inhibition of both PSI and PSII activities (Haupt-Herting and Fock, 2002). Once the availability of terminal electron acceptors becomes limiting, the PETC begins to leak electrons, resulting in the reduction of oxygen to detrimental compounds such as peroxides, superoxide and hydroxyl radicals, commonly classified as reactive oxygen species (ROS; Takagi et al., 2016). The photorespiratory pathway of C3 plants represents a major sink for electrons under conditions of either limited CO2 availability or drought stress (Cruz de Carvalho, 2008). Also, the plastid terminal oxidase (PTOX) can extract electrons from plastoquinone (PQ), which are used to reduce oxygen to water, thereby maintaining the oxidation status of PSII during stress episodes (Sun and Wen, 2011).
To overcome the restriction of photosynthesis and thus the limitation of carbohydrate and metabolite production for better growth, we have been pursuing an alternative strategy by expressing specific cyanobacterial electron shuttles in chloroplasts (Tognetti et al., 2006; Zurbriggen et al., 2009). This strategy has never been employed to the important crop plant barley so that the proof of concept is a straightforward step towards sustainable food security. Among alternative electron sinks, flavodiiron proteins (Flvs) represent a class of electron carriers able to reduce oxygen directly to water without ROS formation (Saraiva et al., 2004). Flavodiiron proteins have been found in many prokaryotic species (Wasserfallen et al., 1998) as well as in anaerobic protozoa, green algae, and most plant lineages, with the major exception being angiosperms (Zhang et al., 2009; Peltier et al., 2010; Allahverdiyeva et al., 2015b).
In photosynthetic organisms, Flvs protect against photoinhibition by reducing oxygen in the non-heme diiron active site of their metallolactamase-like domain. The flavin mononucleotide (FMN) present in the C-terminal flavodoxin-like domain acts as a co-factor for this reaction, enabling electron transfer to the Fe–Fe center (Silaghi-Dumitrescu et al., 2005). The genome of the cyanobacterium Synechocystis sp. PCC 6803 (hereafter Synechocystis) encodes four distinct Flvs, Flv1 through Flv4 (Allahverdiyeva et al., 2011). Flv1 and Flv3 may form part of a single operon or be interspersed with 1–5 open reading frames (ORFs), whereas Flv2 and Flv4 are organized as an Flv4-ORF-Flv2 operon. Flv1 and Flv3 have been proposed to form a heterodimer able to protect PSI under fluctuating light conditions by preventing the accumulation of ROS at the level of PSI (Helman et al., 2003; Allahverdiyeva et al., 2013, 2015a; Sétif et al., 2020). Flvs can mediate Mehler-like reactions and therefore complement cyclic electron transfer pathways in relieving the excess of excitation energy on the PETC (Dang et al., 2014; Gerotto et al., 2016), a phenomenon recently also observed in Arabidopsis thaliana plants expressing the Flv1/Flv3 orthologues from the moss Physcomitrella patens (Yamamoto et al., 2016). When Gómez et al. (2018) introduced the Synechocystis Flv1/Flv3 genes into tobacco, the proton motive force of dark-adapted leaves was enhanced, while the chloroplasts’ photosynthetic performance under steady-state illumination remained comparable to that of wild-type (WT) siblings. The heterologous expression of P. patens Flv1 and Flv3 in two rice mutants defective in cyclic electron transport was shown to restore biomass accumulation to WT levels (Wada et al., 2018). Recently, we demonstrated that the co-expression of Synechocystis Flv1 and Flv3 in A. thaliana enhanced the efficiency of light utilization, boosting the plant’s capacity to accumulate biomass as the growth light intensity was raised (Tula et al., 2020).
Plants expressing Flv1 and Flv3 have been assayed for their photosynthetic performance and light responses (Yamamoto et al., 2016; Gómez et al., 2018; Wada et al., 2018; Tula et al., 2020), but the effects of this additional chloroplast electron sink on stress tolerance and yield were not reported. The present study, therefore, aimed to create for the first time an additional dissipating electron sink downstream of PSI in the chloroplasts of barley, achieved by co-expressing Synechocystis Flv1 and Flv3, and to determine the benefits that the presence of such transgenes could bring to the plant response to drought stress with respect to the production of carbohydrates and accompanying intermediates. Barley is the fourth most important cereal as a source for food and fodder and considered a model crop to investigate the influence of Flv1 and Flv3 expression on productivity traits such as biomass and yield. The focus was to investigate whether metabolic activity through photosynthesis can improve drought stress tolerance, thereby supporting the growth of plants exposed to this commonly occurring constraint over crop productivity.
Materials and Methods
Barley Transformation and Growth
The methods used to transform barley followed those reported by Marthe et al. (2015). Briefly, the Synechocystis Flv1 and Flv3 genes were PCR-amplified, integrated into the pUBI-AB-M plasmid and subsequently cloned via the SfiI restriction sites into the binary vector p6i-2x35S-TE9 (Figure 1A), This generic vector harbors hygromycin phosphotransferase (hpt) as a plant selectable marker gene containing the potato LS1 intron and driven by a doubled-enhanced Cauliflower Mosaic Virus (CaMV) 35S promoter, the Sm/Sp (Streptomycin/Spectinomycin) bacterial selection marker gene and T-DNA borders derived from the p6i plasmid (DNA-Cloning-Service, Hamburg, Germany). Each Flv gene was placed between the maize Polyubiquitin-1 promoter including 5′-untranslated region and first intron and the Agrobacterium tumefaciens nos terminator, with its coding region being fused in-frame at its 5′-end to a DNA fragment encoding the pea ferredoxin-NADP+ reductase (FNR) transit peptide for chloroplast targeting. The individual constructs harboring either Flv1 or Flv3 were transformed into the barley cultivar “Golden Promise” using A. tumefaciens AGL-1 (a hypervirulent succinamopine strain with C58 background) by electroporation. Putative transgenic calli were kept for 12 h at 24°C in the light (mean relative humidity 50%) and for additional 12 h at 18°C in the dark (mean relative humidity 80%) until the formation of plantlets following shoot and root development. Thereafter, plantlets were transferred to soil and maintained at 80% humidity for 7–10 days by covering with a plastic hood. Plants were grown in a greenhouse providing a 12-h photoperiod at 250 μmol photons m–2 s–1 and a day/night temperature of 16°C/12°C (ambient conditions) until maturity, and grains were harvested for further experiments.
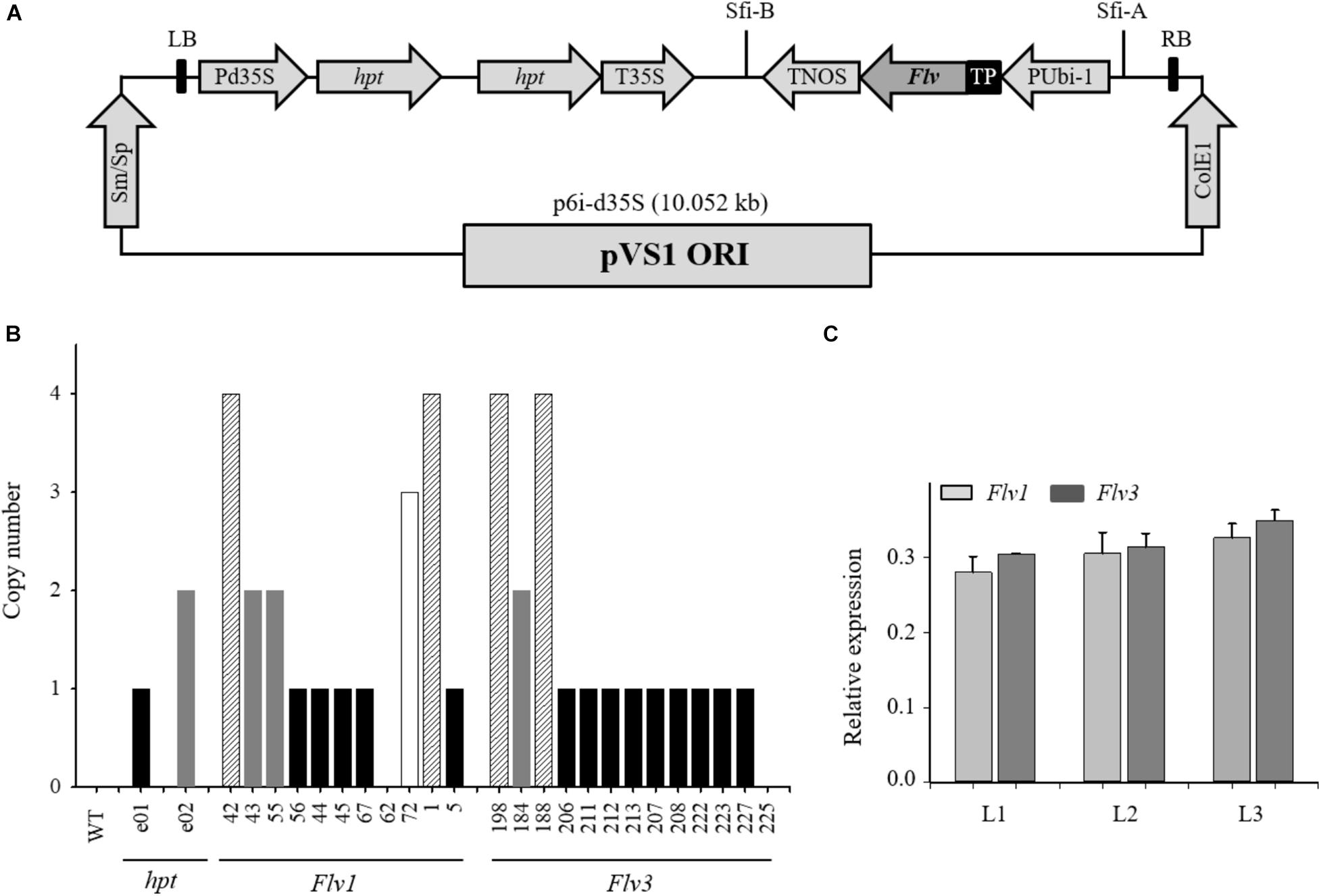
Figure 1. Expression of cyanobacterial Flv1-Flv3 genes in barley plants. (A) Schematic representation of the p6i-2x35S-TE9 binary vector used to clone Flv1 and Flv3 genes. The vector harbors the hpt plant selectable marker gene containing the potato LS1 intron and driven by a double-enhanced CaMV 35S promoter (Pd35S) and terminator (T35S) as well as the Sm/Sp bacterial selection marker gene. A sequence encoding the chloroplast-targeting FNR transit peptide (TP) was fused in-frame to the 5′-termini of Flv1 and Flv3 coding regions and placed under the control of the maize Ubi-1 promoter with its 5′-untranslated regions and the first intron (PUbi-1) and the nos terminator (TNOS) between T-DNA borders (LB and RB) derived from the p6i plasmid. (B) Determination of the copy number of individual Flv genes harbored by transgenic barley plants, as estimated using quantitative real-time PCR. T1 plants containing a single-locus of Flv1 and Flv3 (black bars) which could be identified by a 3:1 presence/absence were chosen as progenitors of the subsequently analyzed transgenic plants. WT barley represented the negative control and two plants (e01, e02) known to harbor one and two copies of the hpt gene, respectively, as positive controls. (C) Determination of Flv transcript levels in leaves from transgenic barley lines L1, L2, and L3 co-expressing Flv1 and Flv3 genes. Data are shown in the form of means ± SE (n = 3). Single-locus T2 homozygotes displaying similar expression levels were inter-crossed (44 × 211, 67 × 207, and 5 × 206) by two rounds of self-pollination to produce double-homozygous L1, L2, and L3 plants, respectively.
T1 generation grains were sown in 96-well trays containing substrate 2 (Klasmann-Deilmann GmbH, Geeste, Germany), compost and sand (2:2:1), held at 4°C for 14 days, then exposed to a 16-h photoperiod at a day/night temperature of 18°C/12°C. Seedlings at the four-leaf stage were potted into a 3:2:1 compost, vermiculite and sand mixture and grown to maturity in a greenhouse under ambient conditions.
Transgene Copy Number Determination
An estimate of the number of Flv transgene copies present in leaves of barley T1 individuals was obtained using a quantitative real-time PCR assay as described by Song et al. (2002) and Kovalchuk et al. (2013). Briefly, DNA was extracted from the second leaf of each plant following the method of Saghai-Maroof et al. (1984) and was serially diluted in sterile deionized water to give solutions containing between 12.5 and 200 ng μL–1 DNA. For the calculation of transgene copy number from unknown DNA samples, a serial dilution (400, 200, 100, 50, and 25 ng) of genomic DNA extracted from an available plant known to contain 1-2 copies of the hpt gene was used as the target sequence. Primers and PCR conditions are listed in Supplementary Table 1. For template loading normalization, the PCR reactions included a dual-labeled sequence 5′-CAL fluor Gold 540-ATGGTGGAAGGGCGGCTGTGABHQ1 as a probe complementary to a portion of the barley orthologue of the wheat Pin-b gene (Kovalchuk et al., 2013). The PCR efficiency for each primer set was determined from an analysis of the Ct values obtained from the serial dilution. Transgene copy numbers were determined by applying the 2–Δ Δ CT method (Li et al., 2004; Figure 1B). For each single-locus transgene construct harboring either Flv1 or Flv3, 16 T1 individuals were then self-pollinated. Homozygotes were selected by segregation analysis as determined by PCR amplification with primers Flv1 F/R and Flv3 F/R given in Supplementary Table 1. Only those behaving as having a single major gene (exhibiting a 3:1 segregation) in the T2 generation were retained as illustrated in Supplementary Figure 1. Siblings lacking Flv fragments, confirmed by PCR amplification, were used as “azygous” control plants.
To produce double-homozygous plants harboring Flv1/Flv3, single-locus T2 homozygotes (Figure 1B) with nearly the same expression level were then inter-crossed (44 × 211, 67 × 207 and 5 × 206) to render double-homozygous L1, L2, and L3 plants, respectively after two generations of self-pollination (Supplementary Figure 2).
Expression Analysis of the Flv1/Flv3 Genes
To monitor the expression of the Flv1/Flv3 genes in the three independent lines L1–L3 (Figure 1C), total RNA was extracted from young leaves according to Logemann et al. (1987). RNA was subjected to DNase treatment (Thermo Fischer Scientific, Dreieich, Germany) and converted to single-stranded cDNA using a RevertAid first-strand cDNA synthesis kit (Life Technologies, Darmstadt, Germany) with a template of 1 μg total RNA and oligo primer. The reaction was run at 42°C for 60 min. Quantitative reverse transcription-PCR (qRT-PCR) was performed in a CFX384 touch real-time system (Bio-Rad, United States) using the SYBR Green Master Mix Kit (Bio-Rad, Feldkirchen, Germany). Primers employed to amplify Flv1 (Flv1-RT F/R) and Flv3 (Flv3-RT F/R), along with those amplifying the reference sequence gene ubiquitin-conjugating enzyme 2 (E2 F/R), that was stably expressed under the experimental conditions tested for barley, are listed in Supplementary Table 1. Relative transcript abundances were determined using the Schmittgen and Livak (2008) method. Each qRT-PCR result relied upon three biological replicates per line, each of which being represented by three technical replicates.
Quantifying the Barley Response to Drought Stress
A representative set of barley plants harboring Flv1/Flv3 transgenes (L1–L3) were selected along with sibling azygous plants. A set of 24 plants of each of the Flv1/Flv3 transgenic lines (F3), non-transgenic barley cultivar “Golden Promise” (referred as WT) and azygous controls were grown for 28 days under a well-watered regime in a chamber providing ambient conditions. Twelve of the seedlings were then transferred into 5-cm pots with 50 g of soil (one seedling per pot) for the drought stress treatment at the vegetative stage and were allowed to recover for 3 days after being transferred. The other 12 seedlings were planted in larger pots (20-cm diameter and 200 g of soil, one seedling per pot) to assess the effect of stress at the reproductive stage. For the stress experiment at the seedling stage, six plants were kept under well-watered, ambient conditions, maintaining a soil moisture level of 65-70% of field capacity (FC; Supplementary Figure 3A). The remaining six plants were subjected to the drought treatment by withholding water for 3–4 days until the soil moisture level in the pots falls to 10–12% FC, and this state was maintained for five days (Supplementary Figure 3B). Subsequently, the 12 treated plants were transferred to the glasshouse and grown under well-watered conditions until maturity (∼90 days) to determine growth parameters such as days to heading.
For the reproductive stage stress experiment, plants were kept well-watered (65–70% FC) under ambient conditions until the emergence of the first spike in 90% of the plants. Drought stress treatment was imposed five days post-anthesis by withholding water until FC fell to 10–12% and leaf wilting was observed. Thereafter, each pot was given 200 mL water every fourth day to maintain the soil moisture level at 10–12% FC over 21 days. Control plants (n = 6) were kept fully watered throughout. Flag leaves were collected 10 days after stress had been initiated, and the fresh weight (FW) of each leaf was measured immediately before it was placed into a collection tube. The relative water content (RWC) was calculated using six individuals each of WT and transgenic plants applying the following equation: RWC (%) = [(FW - DW)/(TW - DW)] × 100, where FW is the fresh weight at harvest time, TW is the total weight at maximal turgor estimated after 24 h of imbibition, and DW is the dry weight after 48 h at 85°C (Marchetti Cintia et al., 2019).
Phenotypic Effects of Drought
The effect of drought stress on barley plants was assessed by measuring the following traits: days to heading, defined as the number of days from sowing to the time when 50% of the spikes had emerged from the flag leaf sheath, using Zadoks scale 55 (Zadoks et al., 1974); plant height (the height from the soil surface to the tip of the longest spike, excluding awns); above-ground plant biomass at maturity measured after the plants had been oven-dried at 60°C for 72 h; the number of spikes produced per plant; the grain number per plant and the total grain weight per plant (g). The latter two traits were quantified using a Marvin-universal seed analyser (GTA Sensorik GmbH, Neubrandenburg, Germany).
Metabolite Measurements
Flag leaves of two spikes per plant (n = 6) with the same developmental stage (head emergence) were sampled when a completed leaf rolling as the primary visible symptom of drought stress occurred. The leaves were pooled, grinded and 50 mg of each sample were used for the measurement of the metabolites in WT, azygous and transgenic L1–L3 individuals. The contents of amino acids, including the stress marker proline, were quantified as described by Mayta et al. (2018), whereas extraction and analysis of soluble sugars were essentially performed according to Ahkami et al. (2013).
Adenine nucleotides were quantified employing a UPLC-based method developed from that described by Haink and Deussen (2003). Prior to the separation step, a 50-μL aliquot of the sample and a mixture of ATP, ADP, and AMP were derivatized by the addition of 25 μL of 10% (v/v) chloracetaldehyde and 425 μL of 62 mM sodium citrate/76 mM KH2PO4, pH 5.2, followed by a 40-min incubation at 80°C, cooling on ice, and centrifugation at 20,000 g for 1 min. The separation was achieved using an ultra-pressure reversed-phase chromatography system (AcQuity H-Class, Waters GmbH, Eschborn, Germany) consisting of a quaternary solvent manager, a sample manager-FTN, a column manager and a fluorescent detector (PDA eλ Detector). The gradient was established using eluents A (TBAS/KH2PO4: 5.7 mM tetrabutylammonium bisulfate/30.5 mM KH2PO4, pH 5.8) and B (a 2:1 mixture of acetonitrile and TBAS/KH2PO4); the Roti C Solv HPLC reagents were purchased from Roth (Karlsruhe, Germany). The 1.8-μm, 2.1 mm × 50 mm separation column was a Luna Omega C18, (Phenomenex, Aschaffenburg, Germany). The column was pre-equilibrated for at least 30 min in a 9:1 mixture of eluents A and B. During the first two min of the run, the column contained 9:1 A:B, changed thereafter to 2:3 A:B for 2 min followed by a change to 1:9 A:B for 1 min and set to initial values of 9:1 A:B for 2 min. The flow rate was 0.5 mL min–1 and the column temperature was maintained at 45°C. The excitation and emission wavelengths were 280 and 410 nm, respectively. Chromatograms were integrated using Empower Pro software (Waters, Eschborn, Germany). Energy charge was calculated from the expression ([ATP] + 0.5 [ADP])/([ATP] + [ADP] + [AMP]) (Atkinson and Walton, 1967).
Statistical Analyses
Descriptive statistics (means and SE) and data analysis were carried out using SigmaPlot (Systat Software, San Jose, CA, United States). The Student’s t-test was applied for evaluating statistically significant differences between means of individual transgenic lines versus the wild-type.
Results
Flv Expression Influences Plant Growth Under Drought Stress at the Seedling Stage
Homozygous plants containing a single copy of the transgenes and co-expressing high levels of Flv1 and Flv3 (L1–L3) were used to assess drought tolerance (Figure 1). When grown under ambient conditions, Flv1/Flv3-expressing plants were taller than their WT and azygous siblings (Figure 2A and Supplementary Figure 3A), without significant differences in aboveground biomass dry weight (Figure 2B). Height differences between WT and transgenic plants were maintained under drought stress applied at the seedling stage (Figure 2A and Supplementary Figure 3B). The treatment caused a major decrease (up to 40%) of total biomass in non-transformed and azygous plants, which was reduced to less than 10% in their transgenic siblings (Figure 2B). Compared to WT plants, up to 1.5-fold, more biomass was accumulated by Flv1/Flv3-expressing lines under drought (Figure 2B). In the absence of stress, Flv1/Flv3 transgenic plants generally reached heading 2–3 days sooner than non-transformed and azygous counterparts, with these differences becoming more pronounced (5–7 days) under drought (Figure 2C).
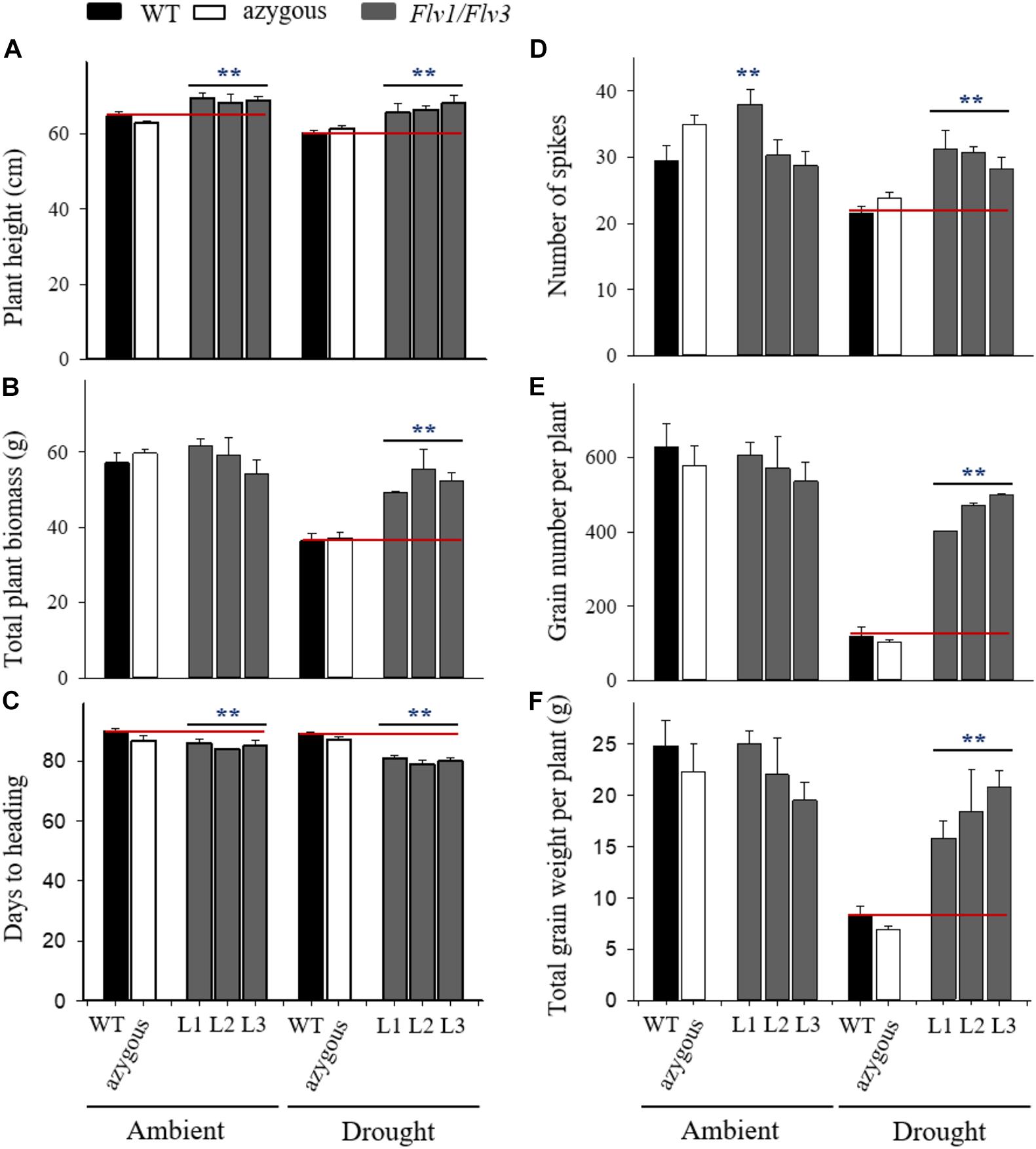
Figure 2. Effect of heterologously expressing Flv1/Flv3 genes on productivity-associated traits of barley plants grown either under ambient conditions or exposed to drought stress for 5 days at the seedling stage. Measurements were carried out at maturity (∼90 days). Other experimental details are given in Materials and Methods. (A) Plant height, (B) total plant biomass, (C) days to heading, (D) the number of spikes per plant, (E) the total number of grains per plant, (F) the total grain weight per plant. Lines L1–L3 co-express Flv1 and Flv3 genes. Data are shown as means ± SE (n = 6). **means differed significantly (P ≤ 0.01) from those of non-transgenic plants.
Plants expressing both transgenes were the least compromised by drought stress with respect to the number of spikes produced (Figure 2D). Compared to WT and azygous plants, there was also significant preservation in the number of grains set per plant by drought-challenged Flv1/Flv3 transgenic lines. The stress treatment decreased grain number by as much as 4-fold in WT and azygous plants while the three transgenic lines displayed less than 20% reduction (Figure 2E), setting at least 3.7-fold more grain than their non-transgenic controls in drought-stressed conditions (Figure 2E). A similar trend was observed for the total grain weight per plant, which was reduced up to 3-fold in WT and azygous plants upon drought stress, but only up to 30% in the transformants (Figure 2F). Indeed, the total grain weight per plant of Flv1/Flv3 transgenic plants from lines L2 and L3 appeared not to be affected by the adverse condition. The total grain weight per plant was up to 3-fold higher in the Flv1/Flv3-expressing lines subjected to drought stress than that achieved by the non-transgenic plants (Figure 2F).
Flv Expression Influences Plant Performance Under Drought Stress at the Reproductive Stage
The increased height of the Flv1/Flv3 transgenic plants under non-stressed conditions was maintained as plants entered the reproductive stage (Figure 3A). While the RWC measured at this stage decreased upon drought stress, it did not differ significantly between WT and transgenic plants grown under ambient conditions (about 78%) nor in plants exposed to drought stress (about 47%). The height increase driven by Flv1/Flv3 presence was lost upon drought exposure at the reproductive stage (Figure 3A). In contrast, drought-dependent reduction in aboveground biomass was similar to that observed upon stress application at the seedling stage and was equally protected by Flv1/Flv3 (Figure 3B). The imposition of drought stress at the reproductive stage advanced heading only in line L3 of Flv transgenic plants by about three days (Figure 3C).
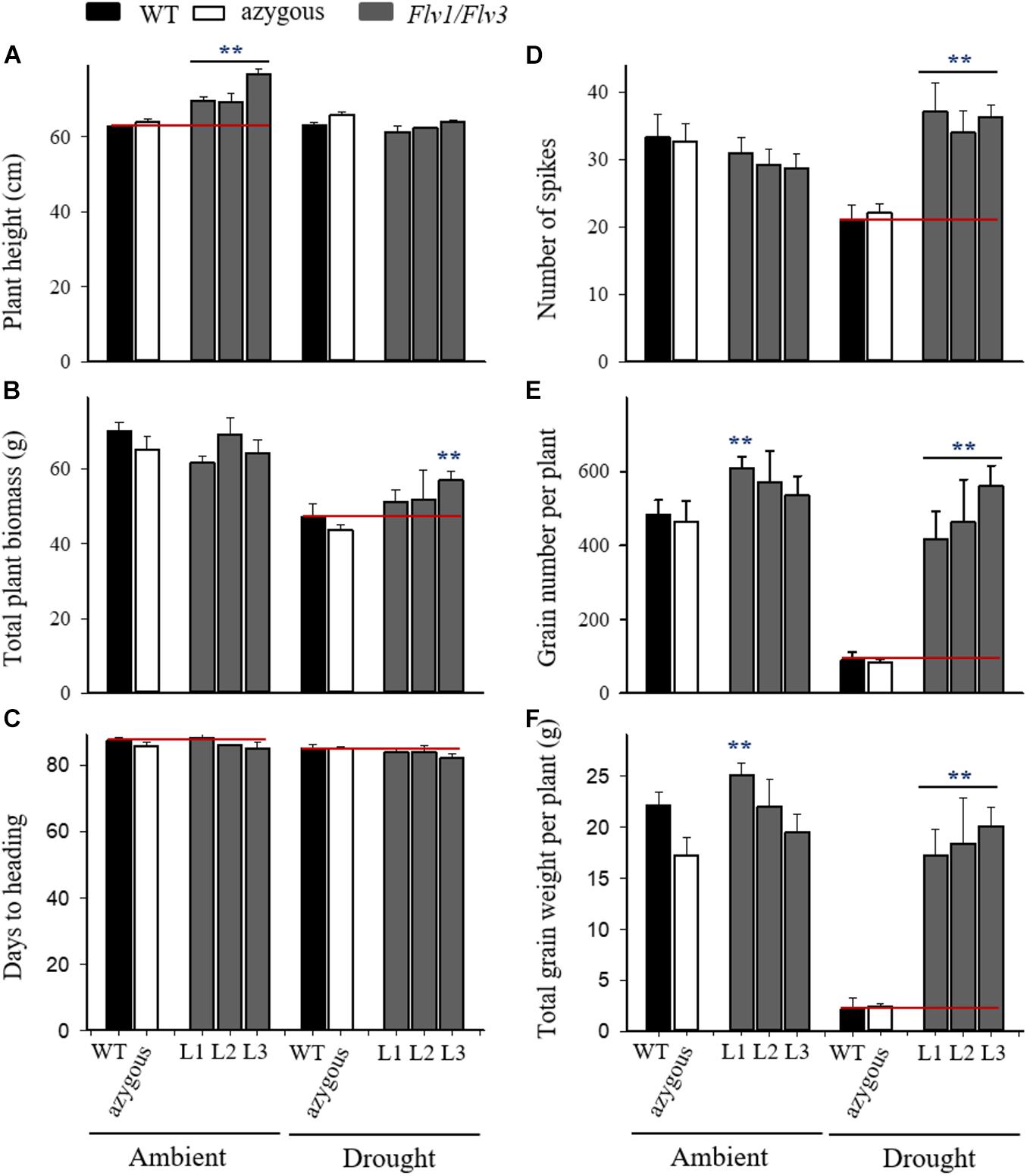
Figure 3. Effect of heterologously expressing Flv1/Flv3 genes on productivity-associated traits of barley plants grown either under ambient conditions or exposed to drought stress for 21 days at the reproductive stage. Measurements were carried out at the end of the 21-day drought treatment. Other experimental details are given in section “Materials and Methods.” (A) Plant height, (B) total plant biomass, (C) days to heading, (D) the number of spikes per plant, (E) the total number of grains per plant, (F) the total grain weight per plant. Lines L1–L3 harbor both Flv1 and Flv3 genes. Data are shown as means ± SE (n = 6). **means differed significantly (P ≤ 0.01) from those of non-transgenic plants.
With respect to the number of spikes produced per plant, the Flv1/Flv3 transgenic plants were notable for the protective effect exerted under drought, while there was no variation between lines in the absence of stress (Figure 3D). Drought also had a devastating effect on yield when applied at the reproductive stage, but Flv1/Flv3 transgenic plants were able to set ∼2- to 3-fold more grain per plant than their WT siblings (Figure 3E), and their total grain weight per plant was 8- to 9.5-fold greater (Figure 3F). Under these conditions, the total grain weight per plant of lines L2 and L3 were unaffected by the stress treatment. In summary, expression of Flv1/Flv3 preserved major productivity traits such as the number of spikes, grain number and total grain weight per plant in transgenic barley plants exposed to drought treatments applied at either the seedling or the reproductive stages (Figures 2, 3).
Flv Expression Influences Carbohydrate Contents and Amino Acid Levels Under Drought Stress
Under ambient conditions, flag leaf glucose and fructose were not detectable in control plants used for drought stress experiments applied at seedling stage or in a low amount at the reproductive stage, with no significant differences between WT, azygous and transgenic plants (Figures 4A,B,D,E). Sucrose also failed to display differences between lines, although their levels increased ∼5-fold in plants challenged at the reproductive stage (Figures 4C,F).
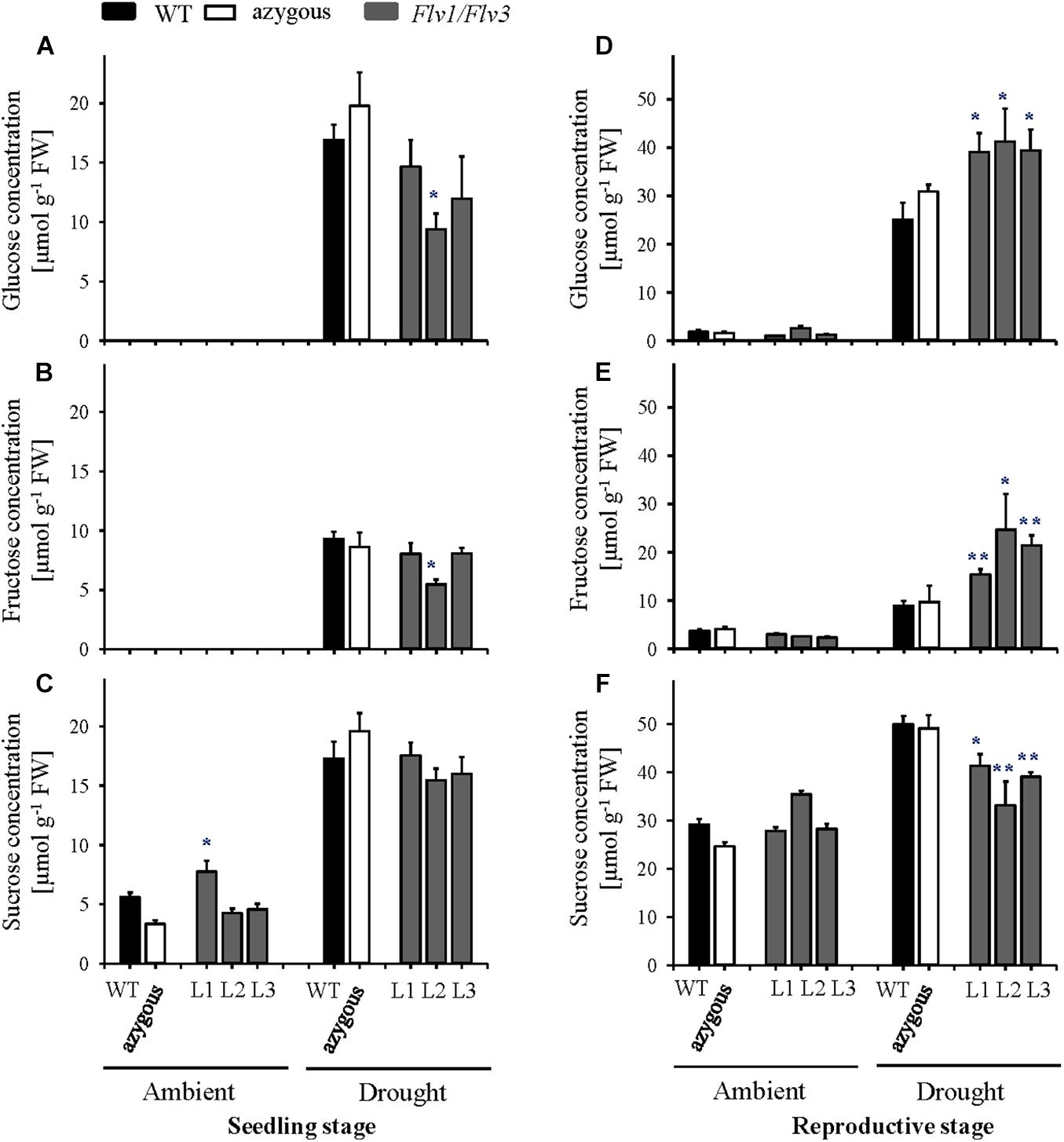
Figure 4. Effect of heterologously expressing Flv1/Flv3 genes on sugar contents in flag leaves of barley plants grown either under ambient conditions or exposed to drought stress at the seedling stage (A–C) and the reproductive stage (D–F). Samples were collected at the leaf rolling stage. Other details are given in section “Materials and Methods.” (A,D) Glucose, (B,E) fructose, (C,F) sucrose. Lines L1–L3 co-express Flv1 and Flv3 genes. Data are shown as means ± SE (n = 6). **; *means differed significantly (P ≤ 0.01 and P ≤ 0.05, respectively) from those of non-transgenic plants. FW, fresh weight.
Application of the drought treatment at the seedling stage led to major increases in all soluble sugars, irrespective of the genotype (Figures 4A–C). Significant differences between lines became instead apparent when the stress treatment was assayed at the reproductive stage, with higher leaf glucose and fructose contents (Figures 4D,E) and lower sucrose levels in transgenic plants compared to their WT siblings (Figure 4F).
Flag leaf amino acid contents were not affected by Flv1/Flv3 expression in plants grown under ambient conditions except for the case of glutamate, whose levels were up to 1.6-fold higher in the transformants relative to WT counterparts (Figure 5A and Supplementary Table 2). Drought treatment had little effect on the amounts of free amino acids in WT and azygous plants, but for significant increases in glycine and proline (Figure 5). In contrast, an increased pool of histidine, asparagine, serine, glutamine, glutamate, asparagine, threonine, and alanine was observed in Flv1/Flv3 transgenics under stress conditions (Figures 5A–E and Supplementary Table 2). Leaf contents of proline increased strongly (up to 60-fold) in drought-exposed WT and azygous plants, which is in line with its recognized role as a stress marker. By contrast, proline levels increased significantly less in stress-treated Flv transformants, despite their higher proline levels under ambient conditions (Figure 5F, inset).
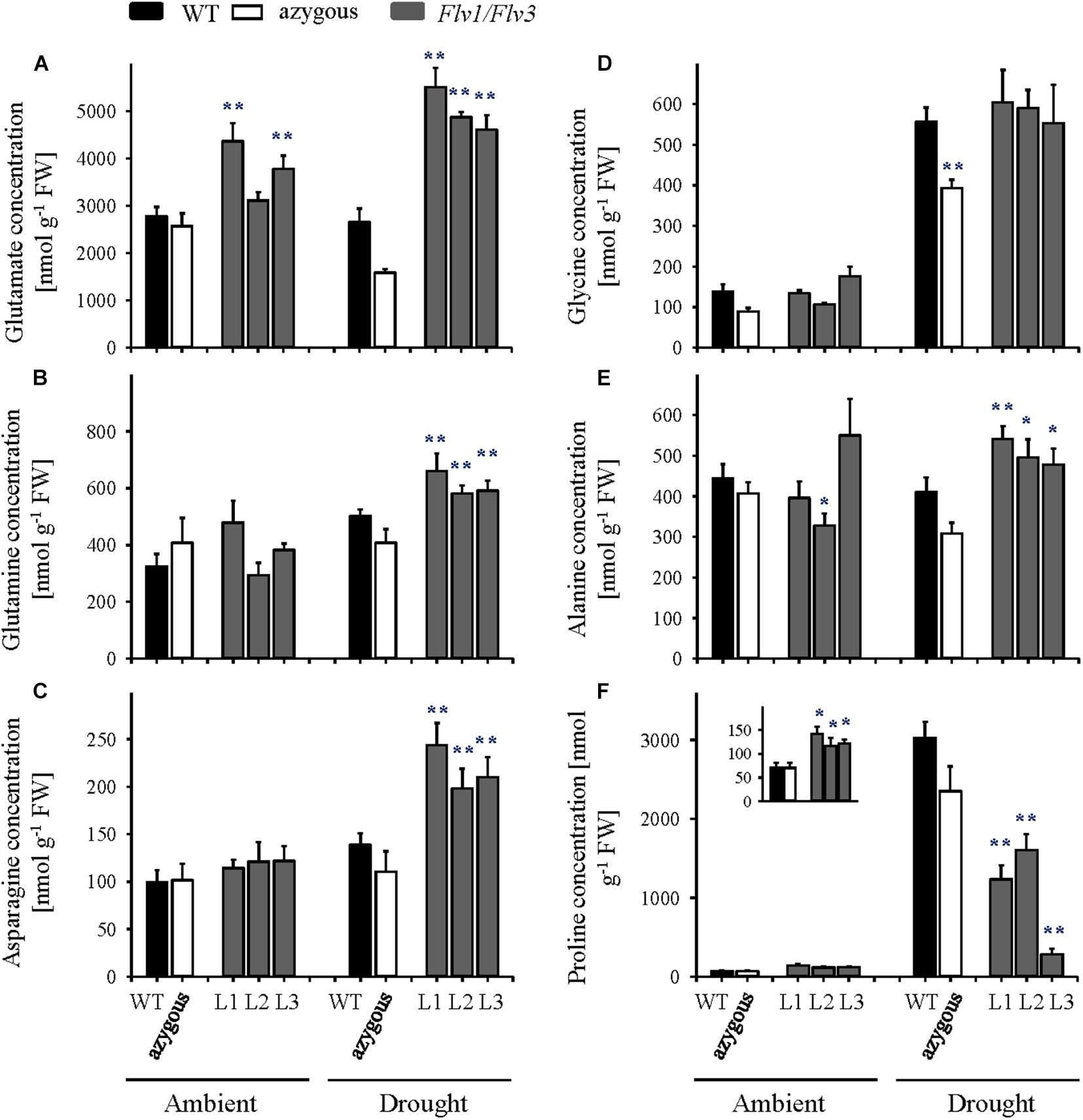
Figure 5. Effect of heterologously expressing Flv1/Flv3 genes on free amino acid contents in flag leaves of barley plants grown either under ambient conditions or exposed to drought stress at the seedling stage. Amino acid levels were measured in the same samples used for carbohydrate determinations. (A) Glutamate, (B) glutamine, (C) asparagine, (D) glycine, (E) alanine, and (F) proline. Lines L1–L3 harbor both Flv1 and Flv3 genes. Data are shown as means ± SE (n = 5–7). **; *means differed significantly (P ≤ 0.01 and P ≤ 0.05, respectively) from those of non-transgenic plants. FW, fresh weight.
Under ambient conditions, the flag leaf contents of free amino acids increased significantly as the plants entered the reproductive stage (Figure 6 and Supplementary Table 3), with no major differences between lines except for proline and glutamine, which accumulated to lower levels in Flv-expressing plants (Figures 6B,F). Drought exposure increased the amounts of several amino acids (most conspicuously proline) in WT and azygous plants, (Figure 6 and Supplementary Table 3). Noteworthy, the stress condition did not affect the amounts of specific amino acids derived from the glycolytic metabolism, such as glutamate, glutamine, asparagine, aspartate, and serine (Figures 6A–E), as well as glycine and threonine (Supplementary Table 3) in leaves of the Flv transformants. Proline levels were up-regulated by drought in Flv1/Flv3 plants, but significantly less than in their WT and azygous counterparts (Figure 6F). No clear differences were observed for other amino acids following exposure to drought as compared to non-stressed plants (Supplementary Table 3).
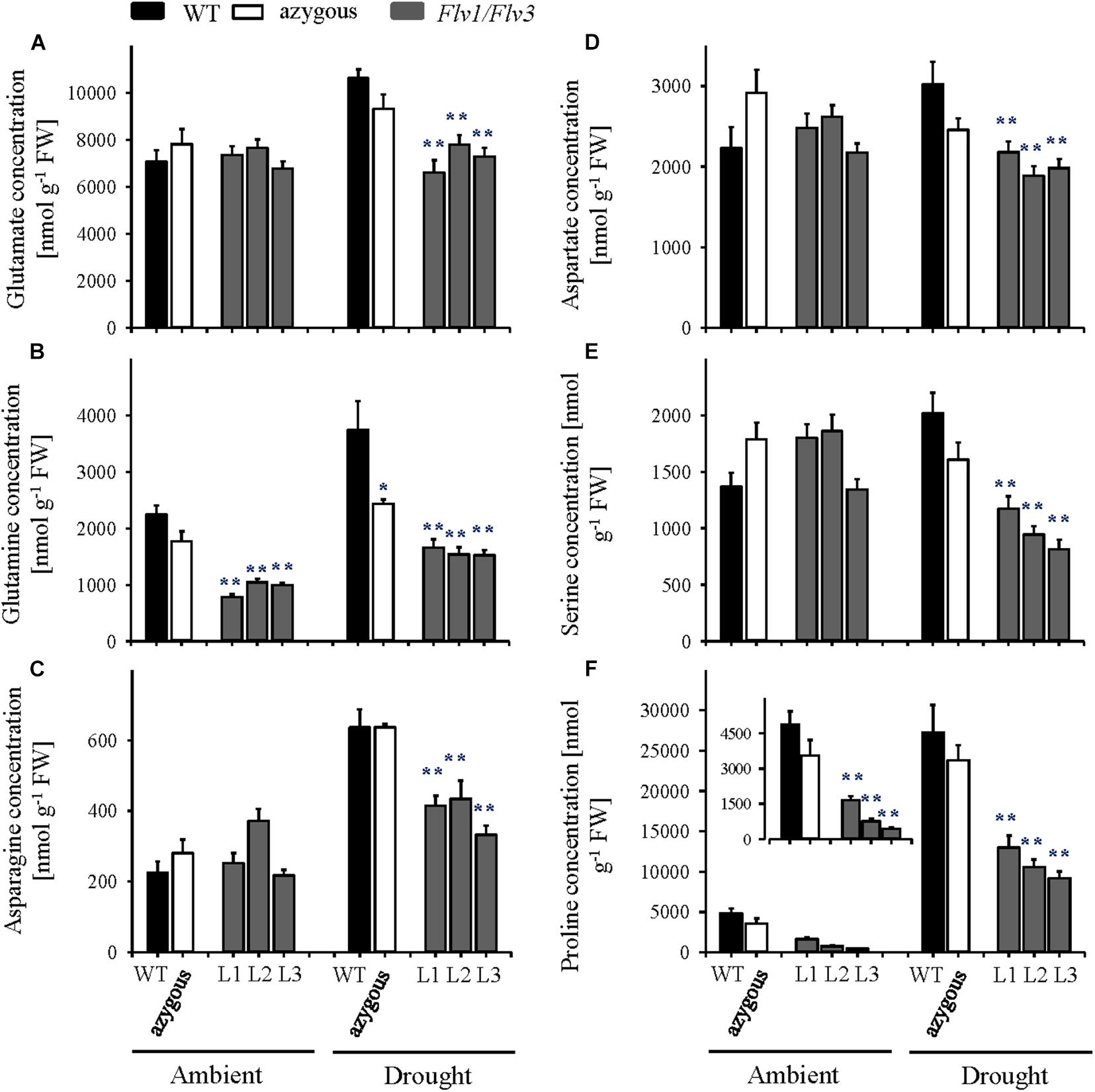
Figure 6. Influence of heterologously expressing Flv1/Flv3 genes on free amino acid contents in flag leaves of barley plants grown either under ambient conditions or exposed to drought stress at the reproductive stage. Amino acid levels were measured in the same samples used for carbohydrate determinations. (A) Glutamate, (B) glutamine, (C) asparagine, (D) aspartate, (E) serine, and (F) proline. Lines L1–L3 co-express Flv1 and Flv3 genes. Data are shown as means ± SE (n = 6–7 for WT and azygous, and n = 8–14 for transgenic lines). **; *means differed significantly (P ≤ 0.01 and P ≤ 0.05, respectively) from those of non-transgenic plants. FW, fresh weight.
Flv Expression Influences the Energy Status of Drought-Stressed Barley Plants
At the seedling stage, ATP and ADP contents were similar in leaves from WT, azygous and transgenic plants under ambient conditions while there was a decrease of AMP levels up to 1.7-fold in Flv-expressing lines compared to WT and azygous siblings (Supplementary Figures 4A–C). The contents of all adenylates strongly increased in drought-stressed WT and azygous plants and the transgenic line L1, whereas lines L2 and L3 maintained ATP and ADP at ambient levels (Supplementary Figures 4A–C).
Upon reaching the reproductive stage, adenylate contents increased 3- to 8-fold in WT and azygous plants under ambient conditions, but significantly less in the transformants (Supplementary Figures 4D–F). Accordingly, adenine nucleotide levels were as much as 3-fold (AMP), 1.8-fold (ADP), and 2.1-fold (ATP) lower in the leaves of Flv-expressing plants compared to WT and azygous counterparts (Supplementary Figures 4D–F). Drought stress, in turn, led to a moderate decline in adenylate contents (especially ADP and AMP) in WT and azygous plants but increased those of Flv transformants, resulting in similar levels for the three nucleotides in all lines (Supplementary Figures 4D–F).
As a consequence of these effects of Flv1/Flv3 expression on adenylate levels, the ATP/ADP ratio and the energy charge were largely similar between lines under both ambient and drought conditions applied at either the seedling or reproductive stages, with only a few exceptions illustrated in Supplementary Figure 5.
Discussion
This is the first study to show that the introduction of the cyanobacterial Flv1 and Flv3 gene products into the chloroplast improves the productivity of barley under drought through maintenance of metabolic activity and increasing carbohydrate and amino acid utilization.
The Heterologous Expression of Flv1/Flv3 in Barley Improves Plant Productivity Under Drought Stress
Crops frequently encounter drought as transient or terminal stress (Alegre, 2004) and indeed, terminal-drought stress is the most serious constraint to cereal production worldwide (Lonbani and Arzani, 2011). Plant survival under these unfavorable conditions depends on their duration and intensity. When exposed to moderate stress, plants survive by adaptation or acclimation strategies and by repair mechanisms. To cope with chronic drought conditions causing severe damage or death, they evolve resistance mechanisms further classified into drought avoidance and drought tolerance (Price et al., 2002). A typical response of cereals such as barley to drought or high-temperature stress is to slow down their vegetative growth, followed by progressive leaf wilting if the adverse condition is prolonged. When these stresses occur around anthesis, the plant response may include premature leaf senescence, which results in a decline in photosynthesis and assimilates production as well as an acceleration of physiological maturation (Gan, 2003). Drought stress diminished grain yield by reducing the number of tillers, grains and spikes per plant and individual grain weight in barley (Farooq et al., 2009). Here, when barley plants were exposed to drought at the seedling stage, the heterologous expression of Flv1/Flv3 resulted in the acceleration of heading time and flowering (Figure 2C). For such plants, one likely consequence is that they are less prone to experience terminal drought stress because they earlier reach maturity. The presence of the Flv1/Flv3 transgenes was thus associated with the production of more spikes and a significantly higher grain number and yield under drought stress conditions applied at both the seedling and reproductive stages (Figures 2, 3).
Under conditions of drought stress, the barley Flv1/Flv3 transgenic plants out-performed their non-transgenic controls in the accumulation of aboveground biomass, the number of grains set and the total grain weight per plant (Figures 2, 3). These observations suggest that heterodimeric Flvs are also functional in a monocotyledonous species, acting to maintain growth in a situation where surplus electrons are produced. Additional support for this contention is also provided by the reduced accumulation of proline (a marker of drought stress, see Szabados and Savouré, 2010) in leaves of the transgenic plants (Figures 5, 6).
The Heterologous Expression of Flv1/Flv3 Resulted in a Distinct Response of Carbohydrates, Amino Acids and Energy Status at Various Developmental Stages in Drought-Stressed Barley Plants
Drought stress suppresses the production of carbohydrates either by restricting CO2 fixation following to stomatal closure (Quick et al., 1992; Brestic et al., 1995) or via limiting the supply of ATP as a result of inhibition of ATP synthase (Tezara et al., 1999). Sucrose synthesized during photosynthesis represents the major feedstock for starch production (Counce and Gravois, 2006), but in drought-stressed plants it also acts as an osmolyte, helping to maintain turgor pressure and to mitigate membrane damage (Couée et al., 2006).
The response of plants with respect to sugar accumulation under drought conditions depends on the species and even on the intraspecific lines within a given species, as reported for wheat by Guo et al. (2018). The comparison of drought-sensitive and -tolerant wheat varieties revealed that soluble sugars such as sucrose or fructose displayed opposite stress behavior, that is, they are reduced in the sensitive and increased in the tolerant plants under drought (Guo et al., 2018). In the present study, drought treatments applied at either the vegetative or reproductive stages resulted in a strong accumulation of soluble sugars including glucose, fructose and sucrose in WT and transgenic plants (Figure 4). This indicates that these metabolites play important roles in the delivery of assimilates to sink organs for further growth (Fàbregas and Fernie, 2019, and references therein) or as osmoprotectants (Singh et al., 2015), and as such are highly sensitive markers of environmental adversities. Sugar accumulation is a general response to drought stress in different plant species, as demonstrated in the current study and several other reports (Singh et al., 2015; Das et al., 2017; Fàbregas et al., 2018; Fàbregas and Fernie, 2019). Remarkably, transgenic lines expressing Flv1/Flv3 genes exhibited even higher glucose and fructose contents and a slightly lower sucrose content compared to WT and azygous plants under drought conditions (Figure 5), suggesting a higher activity of downstream pathways including glycolysis to keep pace with the environmental changes.
Improved metabolic activity exerted by chloroplast-expressed Flv1/Flv3 is also reflected by the differential drought response of amino acid turnover. A schematic model describing metabolic fluxes in WT and Flv1/Flv3-transgenic plants is shown in Figure 7. At the vegetative stage, several amino acids such as Glu, Gln, Asp and Ala increased in the flag leaves of transgenic plants under drought with respect to those in WT siblings. By contrast, at the reproductive stage, most amino acids including Glu, Gln, Ser, Asp, and Asn decreased while being maintained at the levels found in the absence of stress (Figure 5, 6 and Supplementary Tables 2, 3). This contrasting effect of drought on amino acid accumulation (Figure 7) might be because at the vegetative stage, barley plants invest available assimilates into defense mechanisms to resist the stress condition for better growth. Improved assimilate production in transgenic plants might result from a better performance of photosynthetic activity exerted by the presence of Flv1/Flv3 proteins as demonstrated in several studies (Yamamoto et al., 2016; Gómez et al., 2018; Wada et al., 2018).
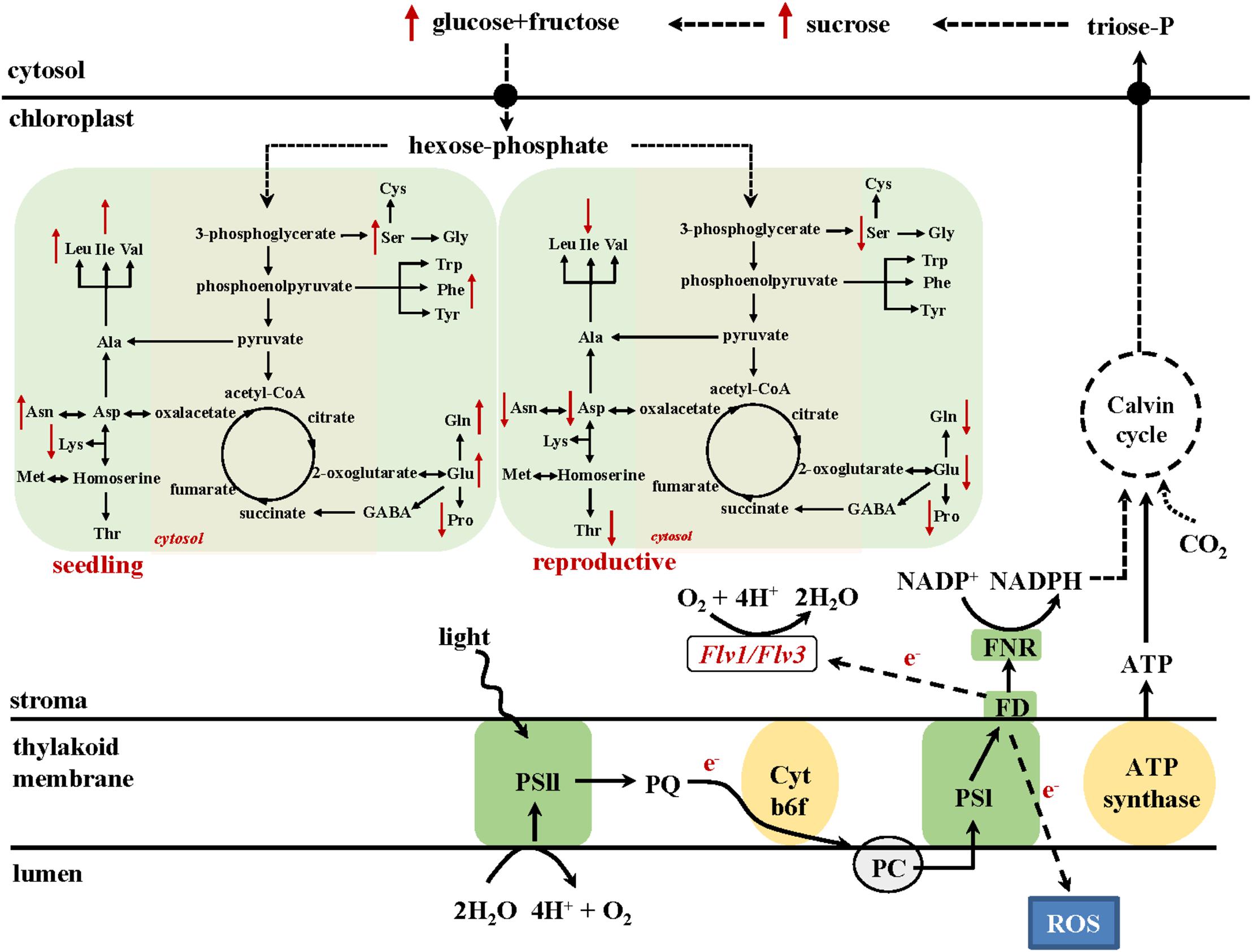
Figure 7. A model describing the metabolic consequences of heterologously expressing Flv1/Flv3 genes in the chloroplasts of barley plants exposed to drought stress at the seedling (left) and the reproductive stage (right). The presence of Flv gene products generates an electron sink and balances the electron pressure generated under stress by delivering the surplus of reducing equivalents to oxygen, which is converted to water. Based on the results, we propose that this activity is acting as a valve to relieve the excess of electrons and does not affect NADPH production, allowing CO2 assimilation through the Calvin-Benson cycle to form triose-phosphates. Sucrose produced from triose-phosphates is cleaved to soluble sugars glucose and fructose. The resulting hexose-phosphates are incorporated into amino acids, or used for energy production through glycolysis. As a consequence, these intermediates are preferentially employed to maintain the energy source necessary to support the growth of plants exposed to stress. PQ, plastoquinone; Cytb6/f, cytochrome b6/f complex; PC, plastocyanin; FD, ferredoxin; FNR, ferredoxin-NADP+ reductase.
At the reproductive stage, water limitation led to a strong increase in amino acid levels in WT flag leaves compared to non-stressed conditions (Figures 5, 7 and Supplementary Table 2). However, in the flag leaves of transgenic plants, the same amino acids were maintained at the levels found under non-stressed conditions or decreased in comparison to the contents of WT plants (Figures 6, 7 and Supplementary Table 3). At this stage, a stable metabolic activity is crucial for the maintenance of assimilates translocation from the flag leaves to the growing sink tissues, in this particular case the grains that are highly dependent on the delivery of the assimilates from the source organs. Thus, most likely WT plants use the produced sugars to synthesize amino acids such as Glu that serve as a key hub for the production of defense compounds such as proline, a sensitive marker of drought stress (Fàbregas and Fernie, 2019). However, due to a better performance of the metabolic activity, transgenic barley plants may compensate the loss of nitrogen-containing amino acids including a reduced production of proline (Figures 5, 6). The saving of nitrogen-containing compounds such as glutamate, the immediate precursor of proline synthesis, might be used for further assimilation and translocation to sink organs (Rai and Sharma, 1991; Hildebrandt et al., 2015). This fact; however, does not refer to any specific role of proline in the stress tolerance displayed by Flv1/Flv3 plants. Indeed, proline accumulation is a universal non-specific response to many sources of abiotic stress including drought and salt whose function is still a matter of debate. The contribution of proline in stress tolerance depends on various factors such as genetic background of the species, the intensity of abiotic stress, the physiological state of the plants and the environment, as outlined by Ebrahim et al. (2020). Proline is proposed to contribute to stabilization of sub-cellular structures, scavenging free radicals, and buffering cellular redox potential rather than to be used as energy source (Kaur and Asthir, 2015). Ebrahim et al. (2020) reported lower proline build-up in salt-tolerant barley cultivars compared to salt-sensitive varieties. This is basically the same phenomenon we observed with drought in our study: proline, unlike other amino acids, was down-regulated in our stress-tolerant Flv plants. A possible explanation for these observations is that proline accumulation is rendered less necessary in plants displaying other mechanisms of stress tolerance, such as expression of additional electron sinks.
Recent publications have demonstrated that high levels of energy and sugars improve plant development and tolerance to drought stress (Guo et al., 2018; Fàbregas and Fernie, 2019). This is also a fundamental basis for an active metabolism with increased pools of intermediates such as amino acids. Furthermore, amino acids have been reported to contribute to both membrane permeability and ion transport in the leaves of Vicia faba (Rai and Sharma, 1991). Indeed, Flv-expressing plants showed significant drought-associated increases in specific amino acids such as alanine, glutamate, serine and aspartate (Figure 7) which are derived from precursors of the glycolytic metabolism and serve as immediate primary substrates to build up nitrogen sources like glutamine and asparagine or antioxidative compounds like glutathione or polyamines. Thus, the metabolite profiling supports the idea that carbohydrates and amino acid metabolism help maintain the fitness of plants under drought stress, which is also in agreement with previously reported results of drought-tolerant varieties in other species (Guo et al., 2018).
Following exposure to drought, ATP levels were found to increase (relative to ambient conditions) in the leaves of Flv transgenic plants at both the seedling and reproductive stages (Supplementary Figure 4), indicating that Flv1/Flv3 were able to maintain linear electron flow and thereby support ATP synthesis under the adverse condition. Sustaining cellular metabolism and ensuring growth and survival under stress rely heavily on a continuous supply of ATP (Sharkey and Badger, 1982). By improving the availability of electron acceptors at PSI, Flv1/Flv3 can prevent ROS build-up (Rutherford et al., 2012), which may, in turn, inhibit both PSI and PSII activity and compromise the function of the ATP synthase complex (Lawlor, 1995).
Conclusion
Data presented here show how integrating additional electron sinks to the PETC can boost the level of drought tolerance in a monocotyledonous crop species, irrespective of whether the drought condition was applied at the seedling stage or post-flowering. The heterologous expression of both Flv1 and Flv3 in barley had the effect of allowing efficient utilization of produced assimilates including sugars and amino acids, thereby supporting plant growth in the face of either early- or late-onset drought and ultimately supporting the conversion of assimilates into biomass and yield (Figure 7). Overall, the experiments have confirmed that adopting this genetic manipulation approach has substantial potential to enhance the level of stress tolerance exerted by crop plants.
Data Availability Statement
The original contributions presented in the study are included in the article/Supplementary Material, further inquiries can be directed to the corresponding author.
Author Contributions
FS and MH have made substantial contributions to conception and design, interpretation of the results, and preparation of the manuscript. FS conducted the experiments and analyzed the data. ST, GH, and JK supported producing of transgenic plants. NR and NN helped in the phenotypic evaluation of the plants. RG, AL, and NC have been involved in the interpretation of the data and editing the manuscript. NC reviewed the manuscript. All authors read and approved the final manuscript for publication.
Funding
This project was supported by funds of the Bundesministerium für Bildung und Forschung (BMBF), Germany, to FS, ST, and MH, by grant PICT 2015-3828 from the National Agency for the Promotion of Science and Technology (ANPCyT, Argentina) to NC, and by grants PICT 2017-3080 from ANPCyT, Argentina and IO 212-2017 from the Santa Fe Agency for Science, Technology and Innovation to AL.
Conflict of Interest
The authors declare that the research was conducted in the absence of any commercial or financial relationships that could be construed as a potential conflict of interest.
Acknowledgments
We wish to thank Melanie Ruff, Nicole Schäfer, Sabine Sommerfeld, Heike Büchner and Heike Nierig for their excellent technical assistances at the IPK. RG was a Fellow and AL and NC are Staff Researchers from CONICET, Argentina. AL and NC are Faculty members of the Facultad de Ciencias Bioquímicas y Farmacéuticas, Universidad Nacional de Rosario, Argentina. This manuscript has been released as a pre-print at bioRxiv 2020.09.29.318394 (Shahinnia et al., 2020).
Supplementary Material
The Supplementary Material for this article can be found online at: https://www.frontiersin.org/articles/10.3389/fpls.2020.613731/full#supplementary-material
Supplementary Figure 1 | A representative segregation analysis of the Flv1 transgene in a set of barley T2 individuals (lanes 1-16), as determined by PCR amplification. The selection of single-locus transgenic plants was made based on a monogenic (3:1) ratio for both Flv1 and Flv3. M: 1 kbp DNA ladder, WT: wild-type, P: empty plasmid control, N: no-template negative control. The size of the target amplicon was 1.8 kbp. Plants lacking the Flv1 amplicon (i.e., lanes 1, 3, 5, 9, 14) were used to produce azygous individuals.
Supplementary Figure 2 | A model describing the steps for producing double-homozygous plants harboring Flv1/Flv3 to conduct drought experiments.
Supplementary Figure 3 | The appearance of typical barley plants heterologously expressing Flv1/Flv3 genes at the seedling stage under ambient (A) and drought-stressed (B) conditions. Lines L1-L3 harbor both Flv1 and Flv3 genes. Images captured seven days after rewatering from a soil maintained at 10-12% FC for 5 days. Growth performance of WT, azygous and transgenic plants in ambient condition (A). Seven days after re-watering, WT barley plants exposed to severe drought exhibited retarded growth and leaf wilting, while leaves of the three transgenic lines retained turgor (albeit turning slightly yellowish). Numerals on the left indicate height in cm.
Supplementary Figure 4 | Influence of heterologously expressing Flv genes on adenosine nucleotides in the flag leaves of barley plants grown either under ambient conditions or exposed to drought stress at the seedling stage (A–C) and the reproductive stage (D–F). Adenine nucleotide levels were measured in the same samples used for carbohydrate determinations (Figure 5). (A,D) ATP, (B,E) ADP, (C,F) AMP. Lines L1-L3 harbor both Flv1 and Flv3 genes. Data are shown as means ± SE (n = 5-6). ∗∗; ∗: means differed significantly (P ≤ 0.01 or P ≤ 0.05, respectively) from those of non-transgenic plants. FW, fresh weight.
Supplementary Figure 5 | Effect of heterologously expressing Flv genes on the energy status of flag leaves of barley plants grown either under ambient conditions or exposed to drought stress at the seedling stage (A,B) and the reproductive stage (C,D). (A,C) ATP to ADP ratio, (B,D) energy charge. Lines L1-L3 co-express Flv1 and Flv3 genes. Data are shown as means ± SE (n = 5-6). ∗∗; ∗: means differed significantly (P ≤ 0.01 or P ≤ 0.05, respectively) from the performance of non-transgenic plants.
Supplementary Table 1 | Sequence-specific forward (F) and reverse (R) primers, annealing temperature and extension time used for PCR and qRT-PCR analysis.
Supplementary Table 2 | Effect of heterologously expressing Flv genes on amino acid contents in flag leaves of barley plants grown either under ambient conditions or exposed to drought stress at the vegetative stage. Lines L1-L3 harbor both Flv1 and Flv3 genes. Data are shown in nmol g–1 FW and as means ± SE (n = 6-7 for WT and azygous and n = 8-14 for transgenic lines). Yellow and blue shading: means differed significantly (P ≤ 0.01 and P ≤ 0.05, respectively) from those of non-transgenic plants. FW, fresh weight.
Supplementary Table 3 | Effect of heterologously expressing Flv genes on amino acid contents in flag leaves of barley plants grown either under ambient conditions or exposed to drought stress at the reproductive stage. Lines L1-L3 harbor both Flv1 and Flv3 genes. Data are shown in nmol g–1 FW and as means ± SE (n = 6-7 for WT and azygous and n = 8-14 for transgenic lines). Yellow and blue shading: means differed significantly (P ≤ 0.01 and P ≤ 0.05, respectively) from those of non-transgenic plants. FW, fresh weight.
References
Ahkami, A. H., Melzer, M., Ghaffari, M. R., Pollmann, S., Ghorbani Javid, M., Shahinnia, F., et al. (2013). Distribution of indole-3-acetic acid in Petunia hybrida shoot tip cuttings and relationship between auxin transport, carbohydrate metabolism and adventitious root formation. Planta 238, 499–517. doi: 10.1007/s00425-013-1907-z
Alegre, L. (2004). Review: Die and let live: leaf senescence contributes to plant survival under drought stress. Funct. Plant Biol. 31, 203–216. doi: 10.1071/fp03236
Allahverdiyeva, Y., Ermakova, M., Eisenhut, M., Zhang, P., Richaud, P., Hagemann, M., et al. (2011). Interplay between flavodiiron proteins and photorespiration in Synechocystis sp. PCC 6803. J. Biol. Chem. 286, 24007–24014. doi: 10.1074/jbc.M111.223289
Allahverdiyeva, Y., Isojärvi, J., Zhang, P., and Aro, E. M. (2015a). Cyanobacterial oxygenic photosynthesis is protected by flavodiiron proteins. Life 5, 716–743. doi: 10.3390/life5010716
Allahverdiyeva, Y., Mustila, H., Ermakova, M., Bersanini, L., Richaud, P., Ajlani, G., et al. (2013). Flavodiiron proteins Flv1 and Flv3 enable cyanobacterial growth and photosynthesis under fluctuating light. Proc. Natl. Acad. Sci. U. S. A. 110, 4111–4116. doi: 10.1073/pnas.1221194110
Allahverdiyeva, Y., Suorsa, M., Tikkanen, M., and Aro, E. M. (2015b). Photoprotection of photosystems in fluctuating light intensities. J. Exp. Bot. 66, 2427–2436. doi: 10.1093/jxb/eru463
Atkinson, D. E., and Walton, G. M. (1967). Adenosine triphosphate conservation in metabolic regulation. Rat liver citrate cleavage enzyme. J. Biol. Chem. 242, 3239–3241.
Brestic, M., Cornic, G., Fryer, M. J., and Baker, N. R. (1995). Does photorespiration protect the photosynthetic apparatus in French bean leaves from photoinhibition during drought stress? Planta 196, 450–457. doi: 10.1007/BF00203643
Couée, I., Sulmon, C., Gouesbet, G., and Amrani, A. E. I. (2006). Involvement of soluble sugars in reactive oxygen species balance and responses to oxidative stress in plants. J. Expt. Bot. 57, 449–459. doi: 10.1093/jxb/erj027
Counce, P. A., and Gravois, K. A. (2006). Sucrose synthase activity as a potential indicator of high rice grain yield. Crop Sci. 46, 1501–1507. doi: 10.2135/cropsci2005.0240
Cruz de Carvalho, M. H. (2008). Drought stress and reactive oxygen species: production, scavenging and signalling. Plant Signal. Behav. 3, 156–165. doi: 10.4161/psb.3.3.5536
Dang, K. V., Plet, J., Tolleter, D., Jokel, M., Cuiné, S., Carrier, P., et al. (2014). Combined increases in mitochondrial cooperation and oxygen photoreduction compensate for deficiency in cyclic electron flow in Chlamydomonas reinhardtii. Plant Cell 26, 3036–3035. doi: 10.1105/tpc.114.126375
Das, A., Rushton, P. J., and Rohila, J. S. (2017). Metabolomic profiling of soybeans (Glycine max L.) reveals the importance of sugar and nitrogen metabolism under drought and heat stress. Plants 6:21. doi: 10.3390/plants6020021
Ebrahim, F., Arzani, A., and Peng, J. (2020). Salinity tolerance of wild barley Hordeum vulgare ssp. Spontaneum. Plant Breed. 139, 304–316. doi: 10.1111/pbr.12770
Fàbregas, N., and Fernie, A. R. (2019). The metabolic response to drought. J. Exp. Bot. 70, 1077–1085. doi: 10.1093/jxb/ery437
Fàbregas, N., Lozano-Elena, F., and Blasco-Escámez, D. (2018). Overexpression of the vascular brassinosteroid receptor BRL3 confers drought resistance without penalizing plant growth. Nat. Commun. 9:4680. doi: 10.1038/s41467-018-06861-3
Farooq, M., Wahid, A., Kobayashi, N., Fujita, D., and Basra, S. (2009). Plant drought stress: effects, mechanisms and management. Agron. Sust. Devel. 29, 185–212. doi: 10.1051/agro:2008021
Gan, S. (2003). Mitotic and postmitotic senescence in plants. Sci. Aging Knowl. Environ. 24:RE7. doi: 10.1126/sageke.2003.38.re7
Gerotto, C., Alboresi, A., Meneghesso, A., Jokel, M., Suorsa, M., Aro, E. M., et al. (2016). Flavodiiron proteins act as safety valve for electrons in Physcomitrella patens. Proc. Natl. Acad. Sci. U. S. A. 113, 12322–12327. doi: 10.1073/pnas.1606685113
Gómez, R., Carrillo, N., Morelli, M. P., Tula, S., Shahinnia, F., Hajirezaei, M. R., et al. (2018). Faster photosynthetic induction in tobacco by expressing cyanobacterial flavodiiron proteins in chloroplasts. Photosynth. Res. 136, 129–138. doi: 10.1007/s11120-017-0449-9
Gómez, R., Vicino, P., Carrillo, N., and Lodeyro, A. F. (2019). Manipulation of oxidative stress responses as a strategy to generate stress–tolerant crops. From damage to signaling to tolerance. Crit. Rev. Biotechnol. 39, 693–708. doi: 10.1080/07388551.2019.1597829
Guo, R., Shi, L. X., Jiao, Y., Li, M. X., Zhong, X. L., Gu, F. X., et al. (2018). Metabolic responses to drought stress in the tissues of drought-tolerant and drought–sensitive wheat genotype seedlings. AoB Plants 10:ly016. doi: 10.1093/aobpla/ply016
Haink, G., and Deussen, A. (2003). Liquid chromatography method for the analysis of adenosine compounds. J. Chromatogr. 784, 189–193. doi: 10.1016/s1570-0232(02)00752-3
Haupt-Herting, S., and Fock, H. P. (2002). Oxygen exchange in relation to carbon assimilation in water-stressed leaves during photosynthesis. Ann. Bot. 89, 851–859. doi: 10.1093/aob/mcf023
Helman, Y., Tchernov, D., Reinhold, L., Shibata, M., Ogawa, T., Schwarz, R., et al. (2003). Genes encoding A-type flavoproteins are essential for photoreduction of O2 in cyanobacteria. Curr. Biol. 13, 230–235. doi: 10.1016/S0960-9822(03)00046-0
Hildebrandt, T. M., Nunes Nesi, A., Araujo, W. L., and Braun, H. P. (2015). Amino acid catabolism in plants. Mol. Plant. 8, 1563–1579. doi: 10.1016/j.molp.2015.09.005
Hodaei, M., Rahimmalek, M., Arzani, A., and Talebi, M. (2018). The effect of water stress on phytochemical accumulation, bioactive compounds and expression of key genes involved in flavonoid biosynthesis in Chrysanthemum morifolium L. Ind. Crops Prod. 120, 295–304. doi: 10.1016/j.indcrop.2018.04.073
Kaur, G., and Asthir, B. (2015). Proline: a key player in plant abiotic stress tolerance. Biol. Plant. 59, 609–619. doi: 10.1007/s10535-015-0549-3
Kovalchuk, N., Jia, W., Eini, O., Morran, S., Pyvovarenko, T., Fletcher, S., et al. (2013). Optimization of TaDREB3 gene expression in transgenic barley using cold-inducible promoters. Plant Biotechnol. J. 11, 659–670. doi: 10.1111/pbi.12056
Lawlor, D. W. (1995). “Effects of water deficit on photosynthesis,” in Environment and Plant Metabolism: Flexibility and Acclimation, ed. N. Smirnoff, (Oxford:BIOS Scientific Publishers Limited), 129–160.
Lawlor, D. W., and Tezara, W. (2009). Causes of decreased photosynthetic rate and metabolic capacity in water-deficient leaf cells: a critical evaluation of mechanisms and integration of processes. Annals Bot. 103, 561–579. doi: 10.1093/aob/mcn244
Li, Z., Hansen, J. L., Liu, Y., Zemetra, R. S., and Berger, P. H. (2004). Using real−time PCR to determine transgene copy number in wheat. Plant Mol. Biol. Rep. 22, 179–188. doi: 10.1007/BF02772725
Logemann, J., Schell, J., and Willmitzer, L. (1987). Improved method for the isolation of RNA from plant tissues. Anal. Biochem. 163, 16–20. doi: 10.1016/0003-2697(87)90086-8
Lonbani, M., and Arzani, A. (2011). Morpho-physiological traits associated with terminal droughtstress tolerance in triticale and wheat. Agron. Res. 9, 315–329.
Marchetti Cintia, F., Ugena, L., Humplík Jan, F., Polák, M., Ćavar Zeljković, S., Podlešáková, K., et al. (2019). A novel image-based screening method to study water-deficit response and recovery of barley populations using canopy dynamics phenotyping and simple metabolite profiling. Front. Plant Sci. 10:1252. doi: 10.3389/fpls.2019.01252
Marthe, C., Kumlehn, J., and Hensel, G. (2015). Barley (Hordeum vulgare L.) transformation using immature embryos. Methods Mol. Biol. 1223, 71–83. doi: 10.1007/978-1-4939-1695-5_6
Mayta, M. L., Lodeyro, A. F., Guiamet, J. J., Tognetti, V. B., Melzer, M., Hajirezaei, M. R., et al. (2018). Expression of a plastid-targeted flavodoxin decreases chloroplast reactive oxygen species accumulation and delays senescence in aging tobacco leaves. Front. Plant Sci. 9:1039. doi: 10.3389/fpls.2018.01039
Peltier, G., Tolleter, D., Billon, E., and Cournac, L. (2010). Auxiliary electron transport pathways in chloroplasts of microalgae. Photosynth. Res. 106, 19–31. doi: 10.1007/s11120-010-9575-3
Pires, M. V., Pereira Júnior, A. A., Medeiros, D. B., Daloso, D. M., Pham, P. A., and Barros, K. A. (2016). The influence of alternative pathways of respiration that utilize branched-chain amino acids following water shortage in Arabidopsis. Plant Cell Environ. 39, 1304–1319. doi: 10.1111/pce.12682
Price, A. H., Cairns, J. E., Horton, P., Jones, H. G., and Griffiths, H. (2002). Linking drought-resistance mechanisms to drought avoidance in upland rice using a QTL approach: progress and new opportunities to integrate stomatal and mesophyll responses. J. Exp. Bot. 53, 989–1004. doi: 10.1093/jexbot/53.371.989
Quick, W. P., Chaves, M. M., Wendler, R., David, M., Rodrigues, M. L., Passaharinho, J. A., et al. (1992). The effect of water stress on photosynthetic carbon metabolisms in four species grown under field conditions. Plant Cell Environ. 15, 25–35. doi: 10.1111/j.1365-3040.1992.tb01455.x
Rai, V. K., and Sharma, U. D. (1991). Amino acids can modulate ABA induced stomatal closure, stomatal resistance and K+ fluxes in Vicia faba leaves. Beitr. Biol. Pflanz. 66, 393–405. doi: 10.1016/S0015-3796(89)80057-5
Rutherford, A. W., Osyczka, A., and Rappaport, F. (2012). Back-reactions, short-circuits, leaks and other energy wasteful reactions in biological electron transfer: redox tuning to survive life in O2. FEBS Lett. 586, 603–616. doi: 10.1016/j.febslet.2011.12.039
Saghai-Maroof, M. A., Soliman, K. M., Jorgensen, R. A., and Allard, R. W. (1984). Ribosomal DNA spacer-length polymorphisms in barley: Mendelian inheritance, chromosomal location and population dynamics. Proc. Natl. Acad. Sci. 81, 8014–8018. doi: 10.1073/pnas.81.24.8014
Saraiva, L. M., Vicente, J. B., and Teixeira, M. (2004). The role of the flavodiiron proteins in microbial nitric oxide detoxification. Adv. Microb. Physiol. 49, 77–129. doi: 10.1016/S0065-2911(04)49002-X
Schmittgen, T. D., and Livak, K. J. (2008). Analyzing real-time PCR data by the comparative C(T) method. Nat. Protoc. 3, 1101–1108. doi: 10.1038/nprot.2008.73
Sétif, P., Shimakawa, G., Krieger-Liszkay, A., and Miyake, C. (2020). Identification of the electron donor to flavodiiron proteins in Synechocystis sp. PCC6803 by in vivo spectroscopy. Biochim. Biophys. Acta Bioenerg. 1861:148256. doi: 10.1016/j.bbabio.2020.148256
Shahinnia, F., Tula, S., Hensel, G., Reiahisamani, N., Nasr, N., Kumlehn, J., et al. (2020). Integrating cyanobacterial flavodiiron proteins within the chloroplast photosynthetic electron transport chain maintains carbohydrate turnover and enhances drought stress tolerance in barley. bioRxiv doi: 10.1101/2020.09.29.318394
Sharkey, T. D., and Badger, M. R. (1982). Effects of water stress on photosynthetic electron transport, photophosphorylation and metabolite levels of Xanthium strumarium cells. Planta 156, 199–206. doi: 10.1007/BF00393725
Silaghi-Dumitrescu, R., Kurtz, D. M. Jr., Ljungdahl, L. G., and Lanzilotta, W. N. (2005). X-ray crystal structures of Moorella thermoacetica FprA. Novel diiron site structure and mechanistic insights into a scavenging nitric oxide reductase. Biochemistry 44, 6492–6501. doi: 10.1021/bi0473049
Singh, M., Kumar, J., Singh, S., Singh, V. P., and Prasad, S. M. (2015). Roles of osmoprotectants in improving salinity and drought tolerance in plants: a review. Environ. Sci. Biotechnol. 14, 407–426. doi: 10.1007/s11157-015-9372-8
Song, P., Cai, C. Q., Skokut, M., Kosegi, B., and Petolino, J. (2002). Quantitative real-time PCR as a screening tool for estimating transgene copy number in WHISKERSTM-derived transgenic maize. Plant Cell Rep. 20, 948–954. doi: 10.1007/s00299-001-0432-x
Sun, X., and Wen, T. (2011). Physiological roles of plastid terminal oxidase in plant stress responses. J. Biosci. 36, 951–956. doi: 10.1007/s12038-011-9161-7
Szabados, L., and Savouré, A. (2010). Proline: a multifunctional amino acid. Trends Plant Sci. 15, 89–97. doi: 10.1016/j.tplants.2009.11.009
Takagi, D., Takumi, S., Hashiguchi, M., Sejima, T., and Miyake, C. (2016). Superoxide and singlet oxygen produced within the thylakoid membranes both cause photosystem I photoinhibition. Plant Physiol. 171, 1626–1634. doi: 10.1104/pp.16.00246
Tezara, W., Mitchell, V. J., Driscoll, S. D., and Lawlor, D. W. (1999). Water stress inhibits plant photosynthesis by decreasing coupling factor and ATP. Nature 401, 914–917. doi: 10.1038/44842
Tognetti, V. B., Palatnik, J. F., Fillat, M. F., Melzer, M., Hajirezaei, M. R., Valle, E. M., et al. (2006). Functional replacement of ferredoxin by a cyanobacterial flavodoxin in tobacco confers broad-range stress tolerance. Plant Cell 18, 2035–2050. doi: 10.1105/tpc.106.042424
Tula, S., Shahinnia, F., Melzer, M., Rutten, T., Gómez, R., Lodeyro, A. F., et al. (2020). Providing an additional electron sink by the introduction of cyanobacterial flavodiirons enhances growth of A. thaliana under various light intensities. Front. Plant Sci. 11, 1–12. doi: 10.3389/fpls.2020.00902
Wada, S., Yamamoto, H., Suzuki, Y., Yamori, W., Shikanai, T., and Makino, A. (2018). Flavodiiron protein substitutes for cyclic electron flow without competing CO2 assimilation in rice. Plant Physiol. 176, 1509–1518. doi: 10.1104/pp.17.01335
Wang, X., Cai, J., Jiang, D., Liu, F., Dai, T., and Cao, W. (2011). Pre-anthesis high-temperature acclimation alleviates damage to the flag leaf caused by post-anthesis heat stress in wheat. J. Plant Physiol. 168, 585–593. doi: 10.1016/j.jplph.2010.09.016
Wasserfallen, A., Ragettli, S., Jouanneau, Y., and Leisinger, T. (1998). A family of flavoproteins in the domains Archaea and Bacteria. Eur. J. Biochem. 254, 325–332. doi: 10.1046/j.1432-1327.1998.2540325.x
Yamamoto, H., Takahashi, S., Badger, M. R., and Shikanai, T. (2016). Artificial remodelling of alternative electron flow by flavodiiron proteins in Arabidopsis. Nat. Plants 2:16012. doi: 10.1038/nplants.2016.12
Zadoks, J. C., Chang, T. T., and Konzak, C. F. (1974). A decimal code for the growth stages of cereals. Weed Res. 14, 415–421. doi: 10.1111/j.1365-3180.1974.tb01084.x
Zhang, P., Allahverdiyeva, Y., Eisenhut, M., and Aro, E. M. (2009). Flavodiiron proteins in oxygenic photosynthetic organisms: photoprotection of photosystem II by Flv2 and Flv4 in Synechocystis sp. PCC 6803. PLoS One 4:e5331. doi: 10.1371/journal.pone.0005331
Zurbriggen, M. D., Carrillo, N., Tognetti, V. B., Melzer, M., Peisker, M., Hause, B., et al. (2009). Chloroplast-generated reactive oxygen species play a major role in localized cell death during the non-host interaction between tobacco and Xanthomonas campestris pv. vesicatoria. Plant J. 60, 962–973. doi: 10.1111/j.1365-313X.2009.04010.x
Keywords: biomass, Hordeum vulgare L., metabolites, photosynthesis, plastid biotechnology, yield
Citation: Shahinnia F, Tula S, Hensel G, Reiahisamani N, Nasr N, Kumlehn J, Gómez R, Lodeyro AF, Carrillo N and Hajirezaei MR (2021) Plastid-Targeted Cyanobacterial Flavodiiron Proteins Maintain Carbohydrate Turnover and Enhance Drought Stress Tolerance in Barley. Front. Plant Sci. 11:613731. doi: 10.3389/fpls.2020.613731
Received: 03 October 2020; Accepted: 18 December 2020;
Published: 13 January 2021.
Edited by:
Patricia León, National Autonomous University of Mexico, MexicoReviewed by:
Ahmad Arzani, Isfahan University of Technology, IranDiego H. Sanchez, CONICET Instituto de Investigaciones Fisiológicas y Ecologicas Vinculadas a la Agricultura (IFEVA), Argentina
Copyright © 2021 Shahinnia, Tula, Hensel, Reiahisamani, Nasr, Kumlehn, Gómez, Lodeyro, Carrillo and Hajirezaei. This is an open-access article distributed under the terms of the Creative Commons Attribution License (CC BY). The use, distribution or reproduction in other forums is permitted, provided the original author(s) and the copyright owner(s) are credited and that the original publication in this journal is cited, in accordance with accepted academic practice. No use, distribution or reproduction is permitted which does not comply with these terms.
*Correspondence: Mohammad R. Hajirezaei, mohammad@ipk-gatersleben.de
†Present address: Goetz Hensel, Centre of Plant Genome Engineering, Institute of Plant Biochemistry, Heinrich-Heine-University, Duesseldorf, Germany Rodrigo Gómez, Dipartimento di Biotecnologie, Università di Verona, Verona, Italy