- 1Department of Forest Genetics and Plant Physiology, Umeå Plant Science Centre, Swedish University of Agricultural Sciences, Umeå, Sweden
- 2Department of Plant Physiology, Umeå Plant Science Centre, Umeå University, Umeå, Sweden
Malectin domain (MD) is a ligand-binding protein motif of pro- and eukaryotes. It is particularly abundant in Viridiplantae, where it occurs as either a single (MD, PF11721) or tandemly duplicated domain (PF12819) called malectin-like domain (MLD). In herbaceous plants, MD- or MLD-containing proteins (MD proteins) are known to regulate development, reproduction, and resistance to various stresses. However, their functions in woody plants have not yet been studied. To unravel their potential role in wood development, we carried out genome-wide identification of MD proteins in the model tree species black cottonwood (Populus trichocarpa), and analyzed their expression and co-expression networks. P. trichocarpa had 146 MD genes assigned to 14 different clades, two of which were specific to the genus Populus. 87% of these genes were located on chromosomes, the rest being associated with scaffolds. Based on their protein domain organization, and in agreement with the exon-intron structures, the MD genes identified here could be classified into five superclades having the following domains: leucine-rich repeat (LRR)-MD-protein kinase (PK), MLD-LRR-PK, MLD-PK (CrRLK1L), MLD-LRR, and MD-Kinesin. Whereas the majority of MD genes were highly expressed in leaves, particularly under stress conditions, eighteen showed a peak of expression during secondary wall formation in the xylem and their co-expression networks suggested signaling functions in cell wall integrity, pathogen-associated molecular patterns, calcium, ROS, and hormone pathways. Thus, P. trichocarpa MD genes having different domain organizations comprise many genes with putative foliar defense functions, some of which could be specific to Populus and related species, as well as genes with potential involvement in signaling pathways in other tissues including developing wood.
Introduction
Plant cells are surrounded by cell walls made of cellulose, hemicelluloses, pectins and structural proteins, with lignin being present in cell types specialized for mechanical support (sclerenchyma) and water transport (xylem). Cell wall biosynthesis needs to be regulated so that its mechanical properties can be adapted to different circumstances according to the signals perceived. It is becoming generally accepted that there is constant feedback from the wall to the protoplast, mediated by different molecular pathways commonly termed cell wall integrity (CWI) signaling (Hématy et al., 2007; reviewed by Wolf and Höfte, 2014; Hamann, 2015; Voxeur and Höfte, 2016; Wolf, 2017; Rui and Dinneny, 2020). Perception of signals external to the protoplast is usually mediated by plasmalemma-localized proteins with various ectodomains. One large group of ectodomain-containing proteins is the receptor-like kinases (RLKs) that allow the plant cells to perceive external cues and transduce them, using a phosphorylation relay, into signals to initiate cellular responses (Gish and Clark, 2011; Engelsdorf and Hamann, 2014). Plant RLKs belong to the RLK/Pelle kinase family, one of the largest gene families in plants with more than 600 members in Arabidopsis (Shiu and Bleecker, 2001, 2003). It comprises both RLKs and receptor-like cytoplasmic kinases (RLCKs), and has been divided into 45 subfamilies, including wall-associated kinases, extensin-like RLKs, lectin RLKs, and leucine-rich repeat RLKs. RLCKs are cytoplasmic kinases without a transmembrane domain (TMD) and they recognize signaling molecules intracellularly. The RLKs usually function as heterodimers: one subunit with a large extracellular domain interacts with a ligand, and the other, which has a smaller extracellular domain, stabilizes this interaction and enhances signal transduction (Xi et al., 2019).
Among the different clades of plant RLKs, the Catharanthus roseus receptor-like kinase 1-like proteins (CrRLK1Ls) have received significant attention as mediators of CWI (reviewed by Wolf and Höfte, 2014; Li et al., 2016; Franck et al., 2018). The family is conserved in all Streptophytes analyzed so far, including moss and liverwort, indicating its ancient origin (Galindo-Trigo et al., 2016). CrRLK1Ls are characterized by two malectin ectodomains (MDs) forming a malectin-like domain (MLD), a transmembrane helix and a C-terminal intracellular Ser and Thr kinase domain. The Arabidopsis genome contains 17 CrRLK1L genes and the majority of them have been functionally analyzed. THESEUS1 (THE1) was the first member to be identified as a mediator of dwarfism and ectopic lignification induced by defects in cellulose biosynthesis (Hématy et al., 2007; Merz et al., 2017). Other members of CrRLK1L family including CURVY1 (CVY1), FERONIA (FER) and ANXUR1 (ANX1) are required for polar cell growth in different cell types. FER, ANX1/2 and BUDDHA’S PAPER SEAL1 and 2 (BUPS1 and 2) participate in sexual reproduction. FER mediates signaling by reactive oxygen species (ROS) and Ca2+ during pollen tube reception at the filiform apparatus (Escobar-Restrepo et al., 2007), whereas ANX1/2 together with BUPS1/2 form a receptor complex for RAPID ALKALINIZATION FACTOR (RALF) 4 or 19 in the growing tip of pollen tube and regulate ROS and Ca2+ gradients essential for its growth and CWI (Ge et al., 2017). In addition, CrRLK1L proteins are involved in immune responses. FER positively regulates pathogen-associated molecular pattern (PAMP)-triggered immunity (PTI) by facilitating the formation of a receptor complex composed of BAK1-FLS2-FER or BAK1-EFR-FER (Stegmann et al., 2017), whereas ANX1 functions antagonistically in PTI and inhibits effector-triggered immunity (ETI) (Mang et al., 2017). The downstream responses of CrRLK1Ls are diverse and include Rho-GTPases activating NADPH oxidases involved in the production of apoplastic ROS (Foreman et al., 2003; Duan et al., 2010; Denness et al., 2011; Boisson-Dernier et al., 2013), RLCKs (Boisson-Dernier et al., 2015; Du et al., 2016), inhibition of the proton pump AHA1 (Haruta et al., 2014), Ca2+ signaling mediated by MLO proteins (Kessler et al., 2010; Meng et al., 2020), as yet unknown Ca2+ channels and a signaling cascade via intracellular kinases that eventually activate or repress gene transcription (Franck et al., 2018).
The MLD, which is characteristic of CrRLK1L proteins, and the MD are also found in other types of plant RLKs (Zhang et al., 2016; Bellande et al., 2017). The MD was first identified in the protein called malectin residing in the endoplasmic reticulum of Xenopus laevis and other animals, where it monitors protein glycosylation by binding diglucose motifs with α-1,4-, α-1,3- and α-1,2-linkage in glycosylated proteins (Schallus et al., 2008, 2010). However, the crystal structure of MLD in ANX1, ANX2, and FER indicated an absence of the aromatic residues that interact with diglucosides in animal MDs, and suggested different ligand specificities and/or functions of the MLDs in these proteins (Du et al., 2018; Moussu et al., 2018; Xiao et al., 2019). Several peptides from the RALF family have been demonstrated to bind to ectodomains of CrRLK1L proteins in Arabidopsis: RALF34 to THE1 (Gonneau et al., 2018), RALF1/17/23/32/33 to FER (Haruta et al., 2014; Stegmann et al., 2017), and RALF4/19 to the ANX1/2-BUPS1/2 receptor complex (Ge et al., 2017). Recently it has been shown that the binding of RALF23 to FER is stabilized by interaction with LORELEI-like-GPI-ANCHORED PROTEINS (LLGs) and the formation of such a heterocomplex is required for PTI signaling (Xiao et al., 2019). Moreover, the ectodomain of FER has been shown to bind to the leucine-rich repeat (LRR) domain of LRR-extensin 1 (LRX1) (Dünser et al., 2019) and to pectin (Feng et al., 2018).
Malectin domain is classified as CBM57 in the CAZy database1. Interestingly, the CBM57 family is greatly expanded in the model tree species Populus trichocarpa compared to the herbaceous model plant Arabidopsis thaliana (Kumar et al., 2019). Moreover, transcript of the CBM57 family members are highly upregulated in developing wood tissues of Populus tremula (Kumar et al., 2019) and Eucalyptus grandis (Pinard et al., 2015). These data suggest that MD/MLD-containing proteins (subsequently called MD proteins) have important functions in trees. We hypothesize that MD proteins are involved in the regulation of cell wall formation during secondary growth via pathways analogous to those reported for primary growth (Wolf and Höfte, 2014; Hamann, 2015; Li et al., 2016; Wolf, 2017), and that they participate in signaling cascades related to stress responses and developmental processes in trees. To find candidates for receptors active during secondary growth, we first carried out genome-wide identification of P. trichocarpa genes with predicted MD and MLD. Second, we used expression datasets from different organs (Sundell et al., 2015; Immanen et al., 2016) and high-resolution expression data for wood developmental zones in P. tremula (Sundell et al., 2017) to identify those MD proteins that are expressed during wood biosynthesis, and to classify them according to expression at specific stages of xylogenesis. Finally, we identified co-expression networks for the MD proteins expressed during secondary wall deposition, which include their putative interactors. Our analyses provide a framework to identify CWI monitoring, stress response, and other signaling pathways operating during wood development.
Materials and Methods
Identification of P. trichocarpa Proteins With Malectin and Malectin-Like Domains
The MD proteins of black cottonwood (P. trichocarpa Torr. and A. Gray) were identified by Basic Local Alignment Search Tool (BLAST) (Altschul et al., 1990) searches in the genome browser of the PopGenIE database2 containing P. trichocarpa genome assembly v3.0, using as baits the P. trichocarpa proteins containing Pfam domains 11721 and 12819, corresponding to MD and MLD, respectively, retrieved from the Pfam database3 (El-Gebali et al., 2019). The same approach was applied to A. thaliana using the TAIR database (v10.0) for BLAST searches4. The BLASTP tool of a high-performance sequence aligner DIAMOND was used in –unal 0, –evalue 1e-05, –max-target-seqs 4000, and –more-sensitive mode, other parameters were kept as default (Buchfink et al., 2015). All other web-based tools were used in default mode as per developers’ recommendations. The presence of MDs/MLDs in the proteins selected for both P. trichocarpa and A. thaliana was confirmed using the CDvist web tool5 (Adebali et al., 2015), which also served to identify other conserved domains in these proteins. The amino acid sequence lengths, molecular weights, isoelectric points and indices of protein stability of the putative proteins were calculated using the ProtParam tool provided on the ExPASy website6. The presence of signal peptides and subcellular localization were predicted with the SignalP 4.1 server7 (Petersen et al., 2011) and DeepLoc-1.0 server8 (Armenteros et al., 2017), respectively. The exon-intron organization of the PtMD genes was determined using the PopGenIE GBrowse tool9 and their localization was mapped to P. trichocarpa chromosomes using the chromosome-diagram tool10. Assignment of a gene to a gene cluster on each chromosome was based on the definition of Holub (2001).
Phylogenetic Analysis and Classification of the MD Proteins of P. trichocarpa
All PtMD proteins identified were classified into clades based on phylogenetic analysis with A. thaliana. The amino acid sequences were aligned by MUSCLE11 and phylogenetic trees were constructed using the neighbor-joining (NJ) method in the MEGA7 software package with a bootstrap test with 1000 replicates (Kumar et al., 2016).
To identify the conserved residues in MD and MLD regions of poplar MD proteins, these regions were aligned with reference sequences using Jalview Version 2 (Waterhouse et al., 2009) with the MAFFT option (Katoh et al., 2005).
To evaluate evolutionary conservation of MD genes across tree species, we have extracted protein sequences for Eucalyptus grandis v2.0, Malus domestica v1.0, Salix purpurea v1.0, Theobroma cacao v2.1, Citrus sinensis v1.1, Prunus persica v1.0 and Betula pendula v1.0 from Phytozome genome portal12 using BLAST (with same parameters as stated in the section above) and PtMDs as query sequences. The protein sequences of resulting hits and the MD proteins of P. trichocarpa and A. thaliana were used to generate a phylogenetic tree using one click method described in https://ngphylogeny.fr (Lemoine et al., 2019). The phylogenetic tree and other detailed method descriptions can be found at ftp://plantgenie.org/Publications/Kumar2020/Phylogeny.
Expression Analysis of PtMDs in Developing Leaves and Wood
Developing leaves (leaf number 8, 11, 21 and 23) and developing wood including cambium/phloem and xylem depositing secondary walls were collected from 10 weeks old hybrid aspen (Populus tremula L. x tremuloides Michx.) grown in the greenhouse. The cultivation conditions and RNA extraction protocols were as described in Ratke et al. (2018). Between five and ten biological replicates of each sample were sequenced using Illumina HiSeq-PE150 platforms of Novogene Bioinformatics Technology Co., Ltd. (Beijing). Quality control and mapping to P. trichocarpa transcriptome v3.0 of leaf 8 and 11 samples were performed by Novogene. Other samples had RNA-Seq raw data filtered using FastQC (v0.10.113). rRNA reads were removed using SortMeRNA v1.8 (Kopylova et al., 2012). Low-quality reads were removed using Trimmomatic v0.27 (Lohse et al., 2012) with a sliding window of 5 bp, minimum quality score of 20, minimum read length of 50 bp, minimum leading read quality of 20 and a custom clipping file containing all Illumina adapters. The preprocessed reads were mapped to v3.0 of the P. trichocarpa transcriptome (retrieved from PopGenIE see footnote 2) using Kallisto (v0.43.1) with default parameters (Bray et al., 2016). The raw counts were normalized separately for each experiment using Variance Stabilizing Transformation (VST) in R (v3.4.0; R Core Team, 2014) using the Bioconductor (v3.4; Gentleman et al., 2004) DESeq2 package (v1.16.1; Love et al., 2014). Then the VST data were merged together using a sample-based median centering approach as described by Kumar et al. (2019; the R scripts are available at https://github.com/UPSCb/UPSCb/tree/master/manuscripts/Kumar2018). Mean VST data for PtMDs were displayed using ComplexHeatmap with default parameters and using the tissue with a peak of expression for each gene as a categorical variable for clustering (Gu et al., 2016). The tissue/organ specificity score tau - a score ranging from 0 (ubiquitous expression) to 1 (tissue/organ specific expression), as detailed in Yanai et al. (2005) was calculated for each PtMD gene. The customized R scripts used to calculate tau are available at https://github.com/UPSCb/UPSCb/tree/master/manuscripts/Kumar2018. The raw RNA-Seq data for this study have been deposited in the European Nucleotide Archive (ENA) at EMBL-EBI under accession number PRJEB4117014.
Expression of PtMDs in Different Organs of Populus
RNA-Seq datasets of expression values in different tissues/organs of outdoor and greenhouse grown aspen (P. tremula L.) and hybrid aspen (P. tremula L. x tremuloides Michx., clone T89) are available from the PlantGenIE website (Sundell et al., 2015). Data for secondary tissues of greenhouse grown T89 hybrid aspen are detailed by Immanen et al. (2016). Raw data for all biological replicates of each sample (min. = 3) were preprocessed as described in the section above except that the reads were aligned to the Populus trichocarpa genome using STAR and quantified using HTSeq. Other steps of quality assessment and filtering are explained above and available at: http://www.epigenesys.eu/en/protocols/bio-informatics/1283-guidelines-for-rna-seq-data-analysis. The VST values were median-centered for each sample, and means for all biological replicates were used for hierarchical clustering and calculating the tau tissue/organ specificity score, as described above.
PtMDs Involved in Wood Biosynthesis
The AspWood high-spatial-resolution RNA-Seq dataset (Sundell et al., 2017) was used for analysis of expression of PtMDs during wood biosynthesis. The database provides VST expression values for four trees. Identity of wood developmental zones was based on the expression of marker genes (Sundell et al., 2017). A heatmap of PtMD expression in wood developmental zones was constructed for one representative tree (tree 1) using the AspWood server15.
Co-expression Analysis
PtMD genes from the selected expression clusters were used as ‘Guide Genes’ to obtain co-expression networks for developing secondary tissues, using the AspWood program (see text footnote 14). The AspWood calculates co-expression networks utilizing mutual information and context likelihood of relatedness as explained by Sundell et al. (2017). The corresponding GraphML files were generated using the ExNet tool16 with a Z-score threshold of 5.0, and visualized using Cytoscape 3.4.0 (Shannon et al., 2003).
Results and Discussion
Identification of MD Proteins in P. trichocarpa and Their Classification
Searches of the P. trichocarpa and A. thaliana genomes for MD proteins resulted in the identification of 146 and 87 gene models, respectively (Supplementary Tables 1, 2). Previous analyses identified 62 MD genes in strawberry (Zhang et al., 2016), 74 in A. thaliana (Bellande et al., 2017; Sultana et al., 2020), and 84 in rice (Jing et al., 2020).
The P. trichocarpa proteins identified were analyzed for sequence similarity using protein sequence alignment and phylogenetic analysis, revealing the presence of 12 clades supported by at least 87 % of bootstrap replicates, and three ungrouped sequences, two of which had orthologous sequences in A. thaliana, and were therefore considered to be two single member clades III and XI (Figures 1, 2). The sequences were numbered PtMD1 to PtMD146 according to their sequential appearance in the intraspecific phylogenetic tree (Figure 1). The predicted protein properties and probable subcellular localizations of PtMD proteins are listed in Supplementary Table 1. The deduced sequence lengths ranged from 274 to 1192 amino acids, and isoelectric points (pIs) ranged from 4.55 to 9.49. Seventy-six out of the 146 PtMD proteins had a signal peptide (SP) cleavage site. The SP was not found in any members of clades I and XIV. Thirteen of the PtMDs were predicted to be soluble proteins, with the predicted localization of six of them being extracellular, six - including all members of clade XIV - being cytoplasmic and one being peroxisomal. Out of 133 membrane proteins, one was predicted to localize in the endoplasmic reticulum.
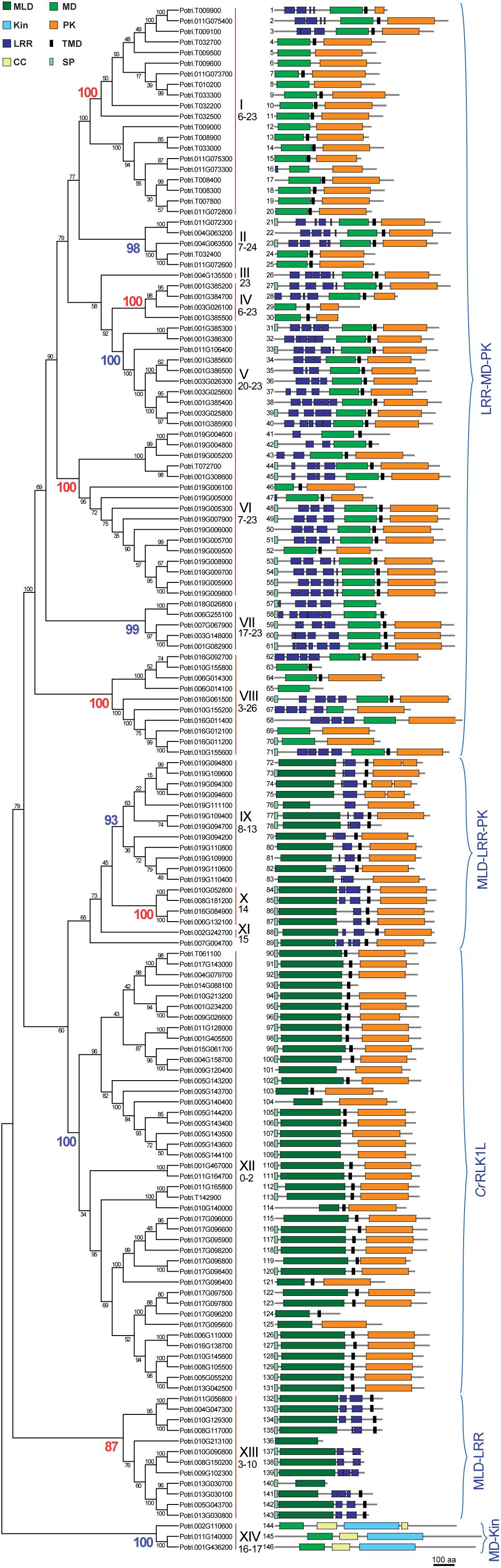
Figure 1. Phylogenetic tree of poplar malectin/malectin-like domain-containing proteins (PtMDs) showing their domain structures. The tree was constructed from MUSCLE-aligned amino acid sequences using the neighbor-joining method in MEGA 7.0 with 1000 bootstrap replicates and bootstrap support is displayed beside the nodes as percentages. PtMDs are identified by the number shown next to each protein structure. Domain abbreviations are: CC, coiled coil; Kin, kinesin; LRR, leucine-rich repeat; MD, malectin domain; MLD, malectin-like domain; PK, protein kinase; SP, signal peptide; TMD, transmembrane domain. Main clades are numbered with Roman numerals and their corresponding bootstrap values are colored in the phylogenetic tree. Numbers below the Roman numerals correspond to the number of introns observed within a clade. Five groups containing clades with similar protein domain structures are identified by the blue brackets: LRR-MD-PK (also known as poplar LRR-RLK XIII; Zan et al., 2013), MLD-LRR-PK (known as poplar LRR-RLK I; Zan et al., 2013), CrRLK1L, MLD-LRR, and MD-Kin.
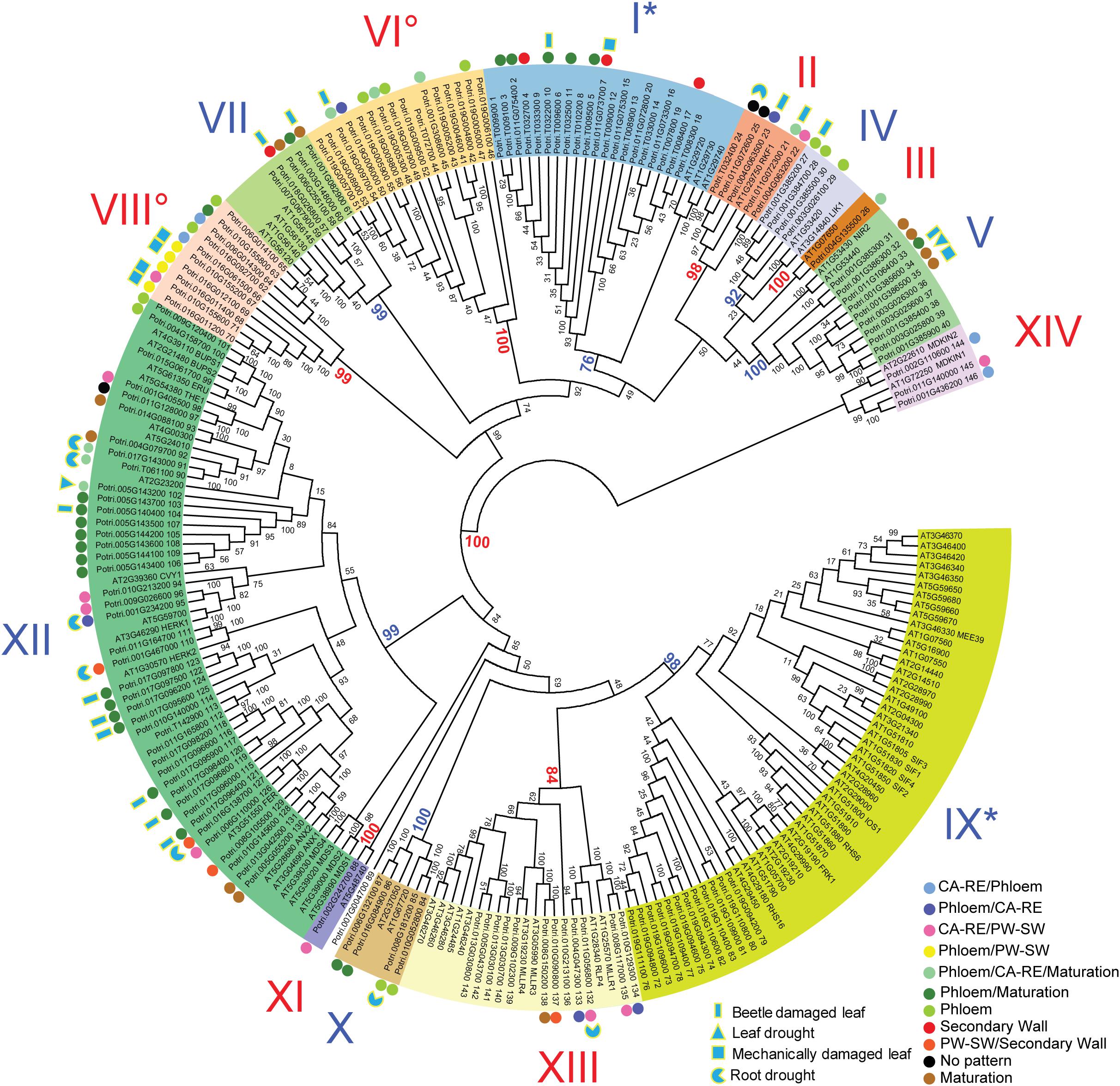
Figure 2. Phylogenetic tree of malectin/malectin-like domain-containing proteins in P. trichocarpa and A. thaliana. Each protein ID is followed by the name (A. thaliana) or PtMD protein number (P. trichocarpa). The phylogenetic tree was constructed based on MUSCLE-aligned amino acid sequences using the neighbor-joining method in MEGA 7.0 using 1000 bootstrap replicates, and the bootstrap support is displayed in percentages. Main clades are numbered with Roman numerals, and their supporting bootstrap values are shown in color. Colored dots beside PtMDs identify genes expressed in secondary vascular tissues based on the AspWood (http://aspwood.popgenie.org/aspwood-v3.0/) database and showing maximum expression in different developmental zones as indicated by colors. CA-RE, cambium-radial expansion zone; PW-SW, primary to secondary wall transition zone. Blue shapes with yellow outlines show stress-related expression based on the aspen expression atlas available at http://popgenie.org. Degree symbols and asterisks beside Roman numerals indicate clades that are represented by only one species or are significantly expanded in one species (χ2-test, P ≤ 0.05), respectively.
Domain analysis (Figure 1 and Supplementary Table 1) revealed the presence of two major groups, one with MD (clades I–VIII, and XIV) and the other with MLD (clades IX to XIII). There was relatively little conservation in the amino acid sequence between the two domains (Figure 3), and in many cases, the proteins having MLD were not classified as members of the CBM57 family (Supplementary Table 1; Kumar et al., 2019). Nevertheless, similarity between MD and each of the two sub-domains of MLD has previously been shown by comparisons of their 3D structures (Moussu et al., 2018). Among the conserved residues of MD, which were proposed to interact with diglucose in Xenopus laevis malectin (Y67, Y89, Y116, F117, and D186) (Schallus et al., 2008, 2010), only Y67 and F117 were conserved in poplar MD (Figure 3 and Supplementary Figure 1). In contrast, the residues proposed to interact with ligands in the MLD of ANX1, Y77, R102, E150, E182, R215, L232, and R234 (Moussu et al., 2018) were to a large extent conserved in poplar MLD (Figure 3 and Supplementary Figures 2A,B).
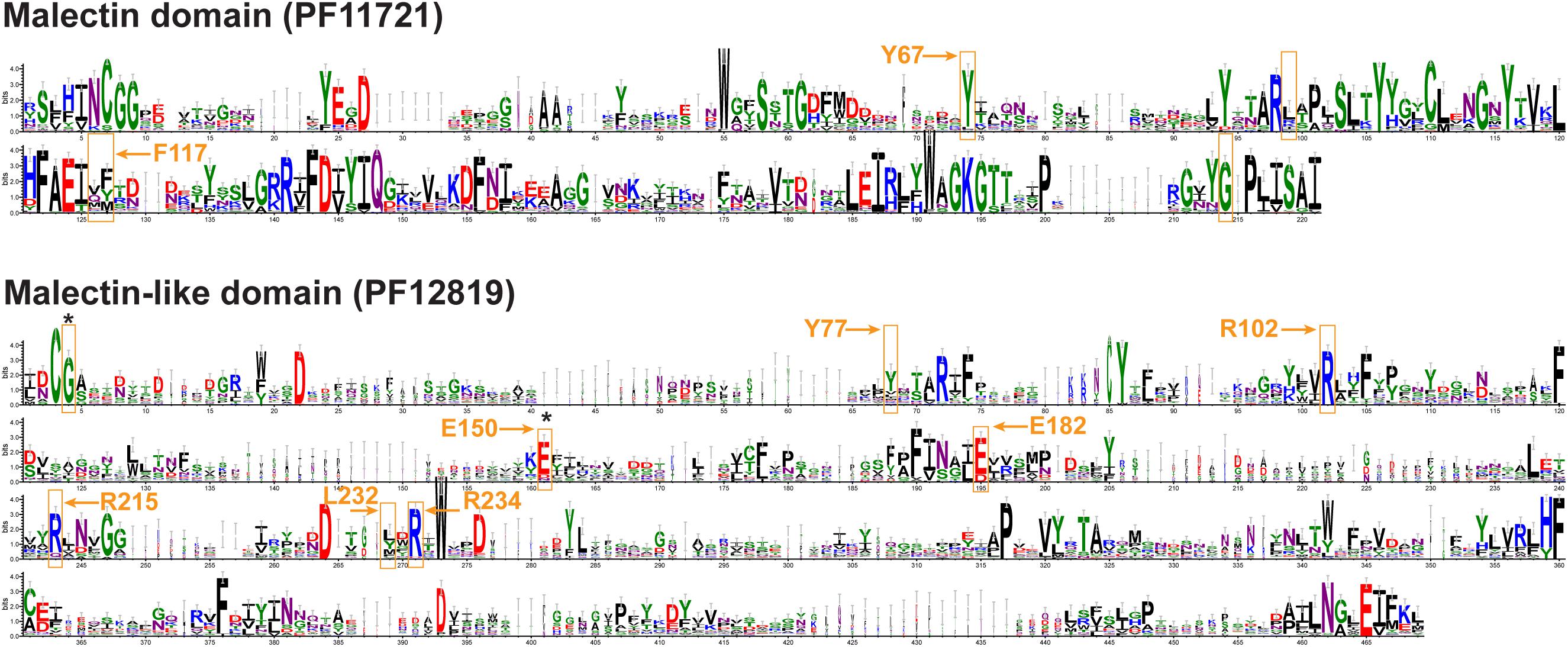
Figure 3. Conserved motifs in malectin (top) and malectin-like (bottom) domains of P. trichocarpa. The residues involved in interaction with ligands according to Schallus et al. (2008) and Moussu et al. (2018) are shown in orange boxes. The amino acids conserved in P. trichocarpa are marked by arrows. The mutated residues in the THE1 protein in the the1 mutants studied (Hématy et al., 2007) are indicated by black asterisks. The logos were created based on P. trichocarpa MD/MLD amino acid sequence alignments by using the WebLogo 3.7.4 online tool (http://weblogo.threeplusone.com, Crooks et al., 2004). The conservation of amino acid residues is represented in bits and residues are colored according to chemical properties. The associated multiple sequence alignments are shown in Supplementary Figures 1, 2.
Clades I-XII had MD or MLD followed by a transmembrane helix preceding the protein kinase domain, in agreement with the typical topology of RLKs (Figure 1 and Supplementary Table 1). Protein kinase domains were frequently of the Tyr kinase type. Some MD proteins with a kinase domain had extracellular localization predicted for this domain (Supplementary Table 1). Clade V was the only one in which all kinase domains were predicted to be intracellular. Extracellular kinase domains were also predicted for the majority of G- and L-type lectin RLKs in poplar (Yang et al., 2016) but experimental validation of such predictions is currently lacking.
In all clades but XII and XIV, several LRR domains were found in tandem repeats. The LRR domain forms a horseshoe-like structure that functions in protein-protein or protein-ligand interactions (Bella et al., 2008). LRRs are known to occur in LRR-RLKs, receptor-like proteins (RLPs), resistance (R) proteins, LRR extensins (LRX), and other families (Wang et al., 2008; Draeger et al., 2015; Choi et al., 2016; Song et al., 2018). Poplar MD proteins had various types of LRR domains, most frequently LRR_4 and LRR_8 (Supplementary Table 1). The proteins from clade XIII had unique combinations of LRR domains; PtMD134 and PtMD135 had the sd00031 LRR domain, PtMD141 had the LRR_1 domain, and PtMD137, -138, and -139 had the LRRNT2 domain. The LRR domains found in the poplar MD family either preceded MD (clades I-VIII) or followed MLD (clades IX-XI and XIII) (Figure 1). Thus, the placement of LRR domains correlated with the presence of either MD or MLD. In clades with members containing LRRs, there were also several members devoid of any LRRs. This probably indicates domain loss due to unequal crossing over. A previous study on poplar LRR-RLKs (Zan et al., 2013) identified and classified some of the MD proteins studied here; MD clades I-VIII were previously classified as LRR-RLK group XIII, and MD clades IX-XI as LRR-RLK group I (Supplementary Table 1).
Clade XI and clade XII members exhibited an unusual TMD, CD12087, which is typical of epidermal growth factor receptors of animals where it functions in receptor dimerization (Mineev et al., 2010). Whether it can carry out such a function in plant MD proteins remains to be investigated.
Clade XIV domain structure and topology was unique in having a kinesin domain (Kin) along with an N-terminal MD (Figure 1). This clade has not been previously included in the surveys of MD genes in A. thaliana (Bellande et al., 2017) or rice (Jing et al., 2020). Recently, one of the A. thaliana clade XIV members, MDKIN2, was found to function in pollen and seed development (Galindo-Trigo et al., 2020). Orthologs of the clade XIV genes could be identified in many species of Viridiplantae, including moss, lower vascular plants, dicots and monocots.
Based on domain composition and domain order, poplar MD gene clades could be grouped into a higher order organization with five superclades characterized by the following domain patterns: 1) LRR-MD-PK (LRR-RLK group XIII, Zan et al., 2013), 2) MLD-LRR-PK (LRR-RLK group I, Zan et al., 2013), 3) MLD-PK (CrRLK1L), 4) MLD-LRR (RLPs) and 5) MD-Kin (Figure 1).
Chromosomal Distribution of MD Genes in P. trichocarpa
127 out of the 146 poplar MD gene models were mapped to chromosomes, while 19 gene models were located on five different scaffolds (Figure 4). The majority of chromosomal genes (79) were present in clusters comprising between two and eleven genes (Figure 4 and Supplementary Table 3). Clusters were also present on the scaffolds. The clusters consisted of tandem repeats having the same or reverse orientations. This large number of tandem duplications strongly suggests that the main mechanism of MD family expansion in P. trichocarpa is via local gene duplication, rather than whole genome duplications. Gene multiplication at a given locus could occur via an unequal crossing over mechanism, which after multiple rounds would result in large numbers of tandemly repeated sequences. Such a mechanism was proposed as featuring particularly in various LRR gene families (Schaper and Anisimova, 2015) including LRR-RLK (Shiu and Bleecker, 2001; Zan et al., 2013; Zulawski et al., 2014; Zhang et al., 2016; Wang et al., 2019) and R genes (Choi et al., 2016). Indeed, 11 out of our16 clusters of PtMD genes had members with LRR domain(s) (Supplementary Table 3).
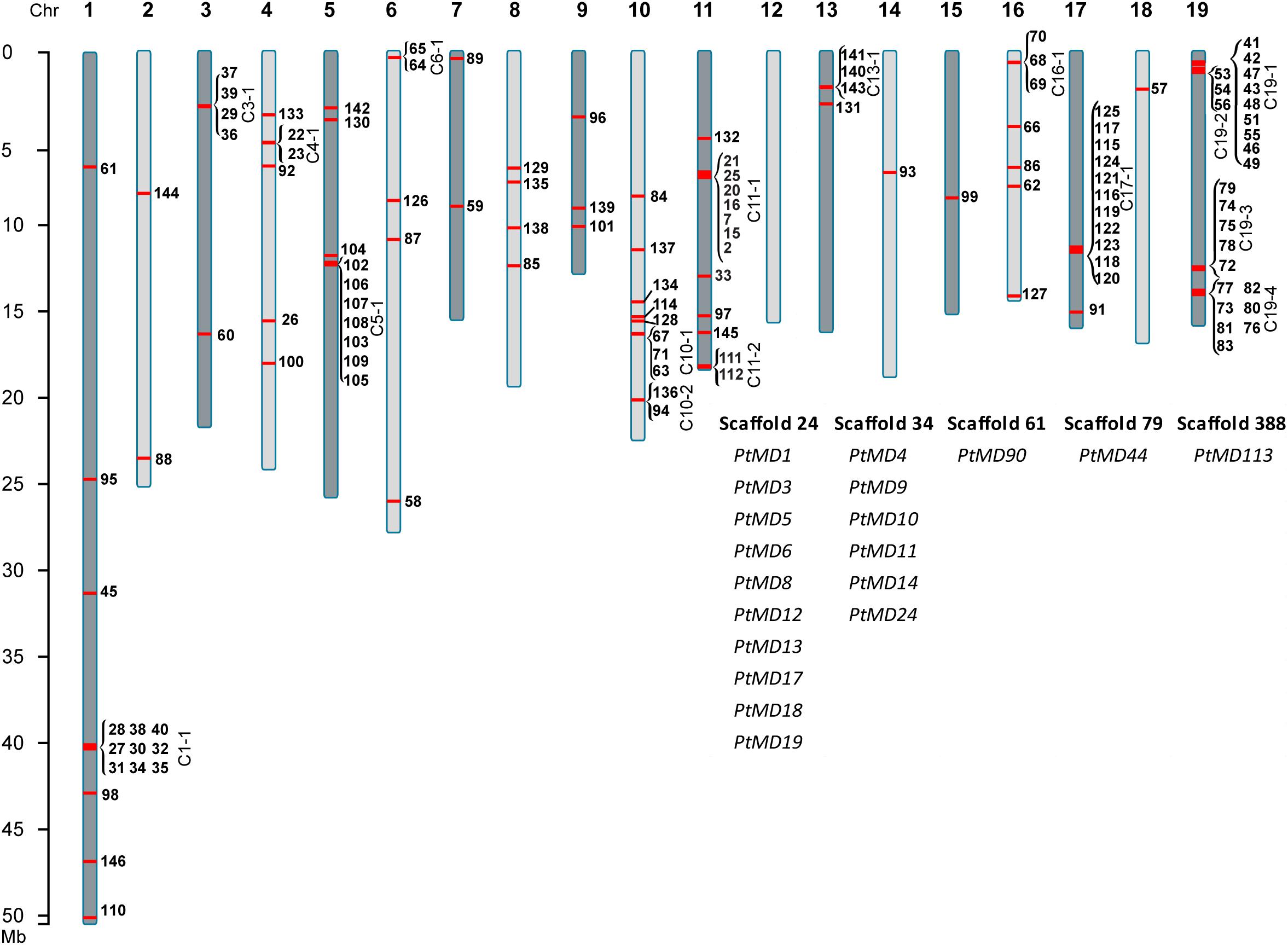
Figure 4. Chromosomal localization of the PtMD genes. The PtMD ID numbers are indicated beside chromosomes. Several PtMDs are found in clusters, shown by parentheses and labeled with a letter “C” followed by the chromosome number and the cluster number. Some PtMDs are on scaffolds, shown below the chromosomes.
Tandem duplications allow rapid gene family expansion and the creation of novel alleles are thought to be particularly important for the co-evolution of R and Avr genes in hosts and their parasites (Holub, 2001; Choi et al., 2016). Partial duplications with omission of some domains form a key mechanism for neofunctionalization. Such a process apparently characterized the poplar MD family, since there were seven out of 16 clusters that included genes with LRR and kinase domains along with closely related members without LRR domains (Figure 4 and Supplementary Table 3).
Analysis of Exon-Intron Structures of PtMD Genes
Exon-intron structure reflects the evolutionary history of genes; hence we analyzed the exon-intron organization of PtMDs. Although the majority of clades displayed very diverse numbers of introns (Supplementary Table 4 and Figure 1), the maximum number of introns for clades within a superclade was similar. The superclade LRR-MD-PK, comprising clades I-VIII, had genes with very large numbers of introns (maximum between 23 and 26); superclade MLD-LRR-PK (clades IX-XI) had at most 15 introns; superclade MLD-PK (clade XII or the CrRLK1L group) contained genes with up to two, but typically without any introns; and superclades MLD-LRR (clade XIII) and MD-Kin (clade XIV) had at most 10 and 17 introns, respectively (Supplementary Table 4 and Figure 1). Lack, or low frequency, of introns in CrRLK1L genes has also been observed in other species including strawberry, Arabidopsis and rice (Zhang et al., 2016; Bellande et al., 2017; Jing et al., 2020). Thus, the exon-intron organization of poplar MD genes supported their grouping into superclades, which represent ancestral diversification of plant MD genes.
Comparison of P. trichocarpa and A. thaliana MD Proteins
The phylogenetic tree of MD proteins was generally consistent between P. trichocarpa and A. thaliana with bootstrap values of greater than 76 % for the main clades (Figure 2). Three exceptions were noted, however: one orphan protein PtMD89, clade VI that included PtMD41-PtMD56, and clade VIII with PtMD62-PtMD71. These poplar genes apparently did not have orthologs in A. thaliana. To address a hypothesis that these genes represent tree-specific functions, we analyzed the MD gene families in other tree species with the whole genome data available (Supplementary Figure 3). Close homologs to PtMD89 were found in other tree species including Salix purpurea, Eucalyptus grandis, Theobroma cacao, Malus domestica, Prunus persica, and Citrus sinensis (Supplementary Figure 3). Each of these species had only one putative PtMD89 ortholog indicating that PtMD89 function is conserved in angiosperm trees belonging to different families. Clades VI and VIII include tandemly repeated genes and were not well resolved by the phylogenetic analysis (Figure 4 and Supplementary Table 3). The clear homologs to P. trichocarpa tandemly replicated genes of clade VI were present in Salix purpurea although we cannot exclude that genes with less supported association to clade VI are present in Prunus persica, Malus domestica, Theobroma cacao and Betula pendula (Supplementary Figure 3). Clade VIII included only P. trichocarpa and Salix purpurea genes, but this clade had a weak bootstrap support (Supplementary Figure 3). Thus, the genes of clades VI and VIII had undergone recent tandem duplication in the P. trichocarpa lineage after its separation from that of A. thaliana that could be conserved in other members of Salicaceae. It is therefore possible that they represent specialized genes, such as R genes important for immunity, that co-evolved with poplar symbionts and/or pathogens of the Salicaceae family (Holub, 2001).
Besides identifying clades not represented in Arabidopsis, we found that the relative clade sizes (number of genes per clade relative to genome size) show some differences between the two species (Figure 2). Clade IX was expanded in A. thaliana, whereas clade I was expanded in P. trichocarpa (χ2-test at P ≤ 0.05). The phylogenetic analysis of MD genes including different tree species confirmed the expansion of clade IX in A. thaliana and clade I in P. trichocarpa (Supplementary Figure 3).
Domain composition and organization were consistent between P. trichocarpa and A. thaliana in clades present in both species (Supplementary Tables 1, 2). Previous studies in A. thaliana classified LRR-RLKs (Shiu and Bleecker, 2001) and assigned them putative receptor or co-receptor functions based on the sizes of ectodomains (Xi et al., 2019). Many MD proteins identified in the current study were among the previously classified LRR-RLKs (Supplementary Table 2). The group of clades I-VIII, except for clades VI and VIII, which were not represented in A. thaliana, have been classified as being of the LRR-VIII-2 class (Shiu and Bleecker, 2001). This group had large ectodomains including several LRR motifs followed by MD, TMD and internal kinase domains (LRR-MD-PK) (Supplementary Table 2 and Figure 1). A. thaliana proteins of clades IX-XI belong to class LRR-I (Shiu and Bleecker, 2001), having a large MLD ectodomain terminated with a short LRR repeat, TMD, and an internal protein kinase domain (MLD-LRR-PK), as was observed for poplar (Supplementary Table 2 and Figure 1). A. thaliana clade XII proteins correspond to the CrRLK1L group, which is characterized by a large MLD ectodomain followed by TMD and the internal protein kinase domain, whereas clade XIII in A. thaliana, as in P. trichocarpa, was characterized by a large ectodomain including MLD and LRR domains. Such proteins are classified as RLPs (Wang et al., 2008).
Only four out of the 14 clades identified contained members that have been functionally analyzed in A. thaliana. In addition to clade XII (CrRLK1L), which has been the most extensively studied, with members involved in CWI sensing, polar growth, fertilization, and immune responses (Franck et al., 2018), the members of clades IV, V, and IX have been functionally characterized. Clade IV includes LYSM RLK1-INTERACTING KINASE 1 (LIK1), an RLK interacting with the chitin receptor formed by the CERK1-LYSM RLK1 complex, which signals the presence of chitin and activates PTI (Le et al., 2014). Clade IX includes several RLKs involved in both immunity and development. For example, IMPAIRED OOMYCETE SUSCEPTIBILITY 1 (IOS1) acts as a co-receptor of flagellin, EF-Tu and chitin, interacting with FLS2, EFR, and CERK1, respectively (Yeh et al., 2016). STRESS INDUCED FACTORs 1-4 (SIF1, SIF2, SIF3 and SIF4) have been characterized as RLKs involved in biotic and abiotic stress responses (Yuan et al., 2018). SIF2 was found to interact with BAK1 and mediate PTI during pathogen attack. FLG22-INDUCED RECEPTOR-LIKE KINASE 1 (FRK1) is known to be an early-induced PTI gene (Asai et al., 2002). ROOT HAIR SPECIFIC 6 and 16 (RHS6 and RHS16) were found to be specifically expressed in root hairs and RHS16 overexpression dramatically altered root hair morphology, indicating an important function in root hair growth (Won et al., 2009), whereas MATERNAL EFFECT EMBRYO ARREST 39 (MEE39) was found to be essential for embryo development based on the mutant phenotype (Pagnussat et al., 2005).
Expression of PtMDs in Different Organs of Populus
RNA sequencing strategy was adopted to examine the expression of 146 PtMD genes in developing leaves and wood tissues (Figure 5 and Supplementary Table 5). Datasets were centered by median expression in each sample and the variance-stabilized transformation (VST) expression values were clustered considering the tissue with maximum expression as a covariate. Majority of PtMD genes had the highest expression in the leaves, especially the fully mature ones (leaf 23). Relatively large number of PtMD genes was highly expressed in expanding leaves (leaf 8), and many of these genes were also highly expressed in the cambium. There were 12 and 13 PtMD genes with maximum expression in the cambium-phloem and developing xylem tissues, respectively. Majority of these genes showed generally high expression in leaves.
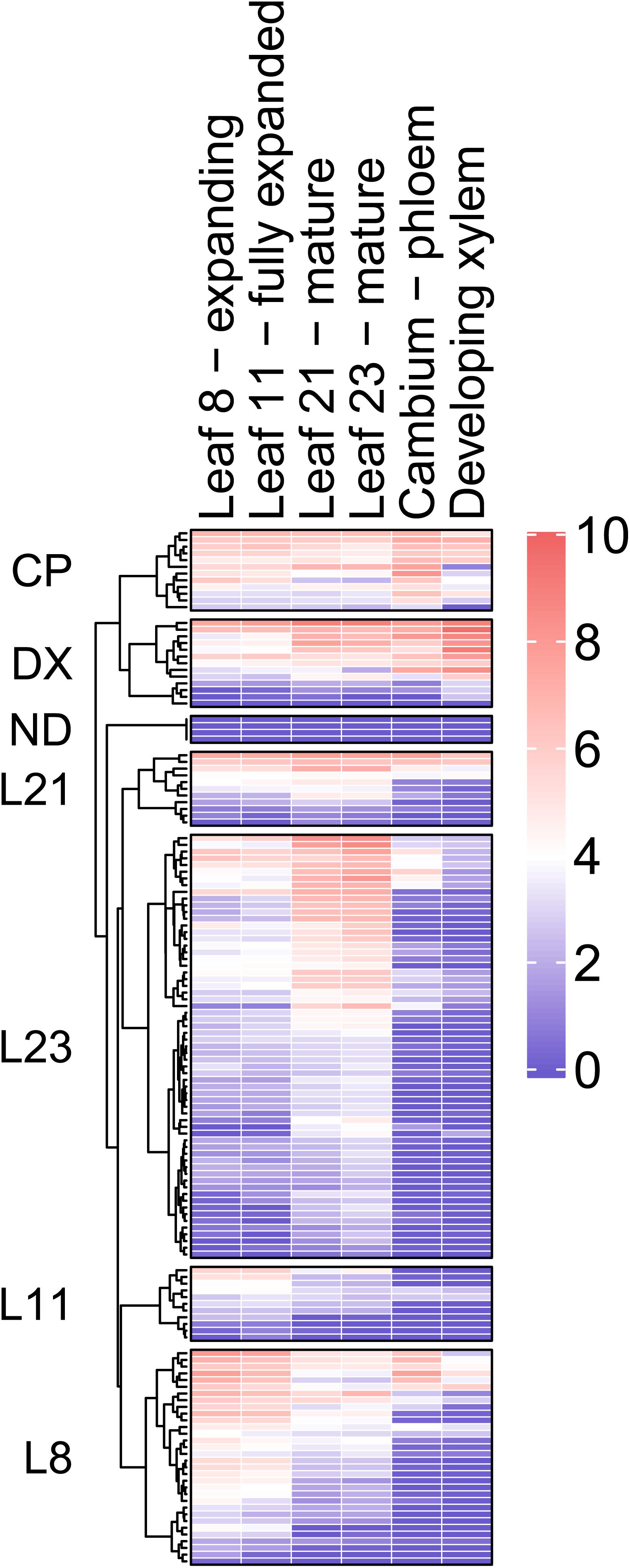
Figure 5. Heatmap of PtMD gene expression patterns in developing leaves and wood of hybrid aspen based on RNA sequencing. Details about the expression of each PtMD gene in the different tissues are listed in Supplementary Table 5. CP, cambium-phloem; DX, developing xylem; ND, not detected.
High expression in mature leaves suggests function in foliar defenses and homeostasis for majority of the PtMD genes. To investigate it, we also examined the RNA sequencing datasets available for different organs and tissues subjected to variety of stress and growth conditions in the greenhouse and in the field, and calculated VST expression values (Figure 6 and Supplementary Table 6) using the same approach as used for our leaf and wood developmental series. Considering all datasets examined (Figures 5, 6 and Supplementary Tables 5, 6), out of the 146 genes, 145 were expressed at least in one of the organs and tissues tested. Similar to our datasets, the majority of PtMD genes (99) showed maximum expression in leaves, especially the mature ones. Moreover, many of them (51) showed the highest expression in leaves exposed to abiotic/biotic stress, such as beetle, drought or mechanical damage. The genes highly expressed in mature and stress-exposed leaves usually exhibited high expression specificity as determined by the tau specificity score (Supplementary Table 6). Interestingly, genes belonging to the clades missing in Arabidopsis (VI and VIII) were found expressed, indicating that they are functional, and many of them showed a peak of expression in the mature and beetle or mechanically damaged leaves (Figure 2 and Supplementary Tables 5, 6) pointing to their involvement in stress responses. These observations support an important role of the leaf-expressed PtMD genes in foliar defense responses, some of which could be species-specific, as suggested by the phylogenetic analyses revealing differences in the presence and size of certain clades of MD genes (Figure 2 and Supplementary Figure 3) as well as by the expression analyses in different species. For example, almost all MD genes of strawberry (Fragaria vesca) were upregulated upon exposure to low temperature or drought stress (Zhang et al., 2016), whereas in rice (Oryza sativa), the expression levels of many MD genes greatly increased upon salt and drought stress, but not in response to low temperature (Jing et al., 2020).
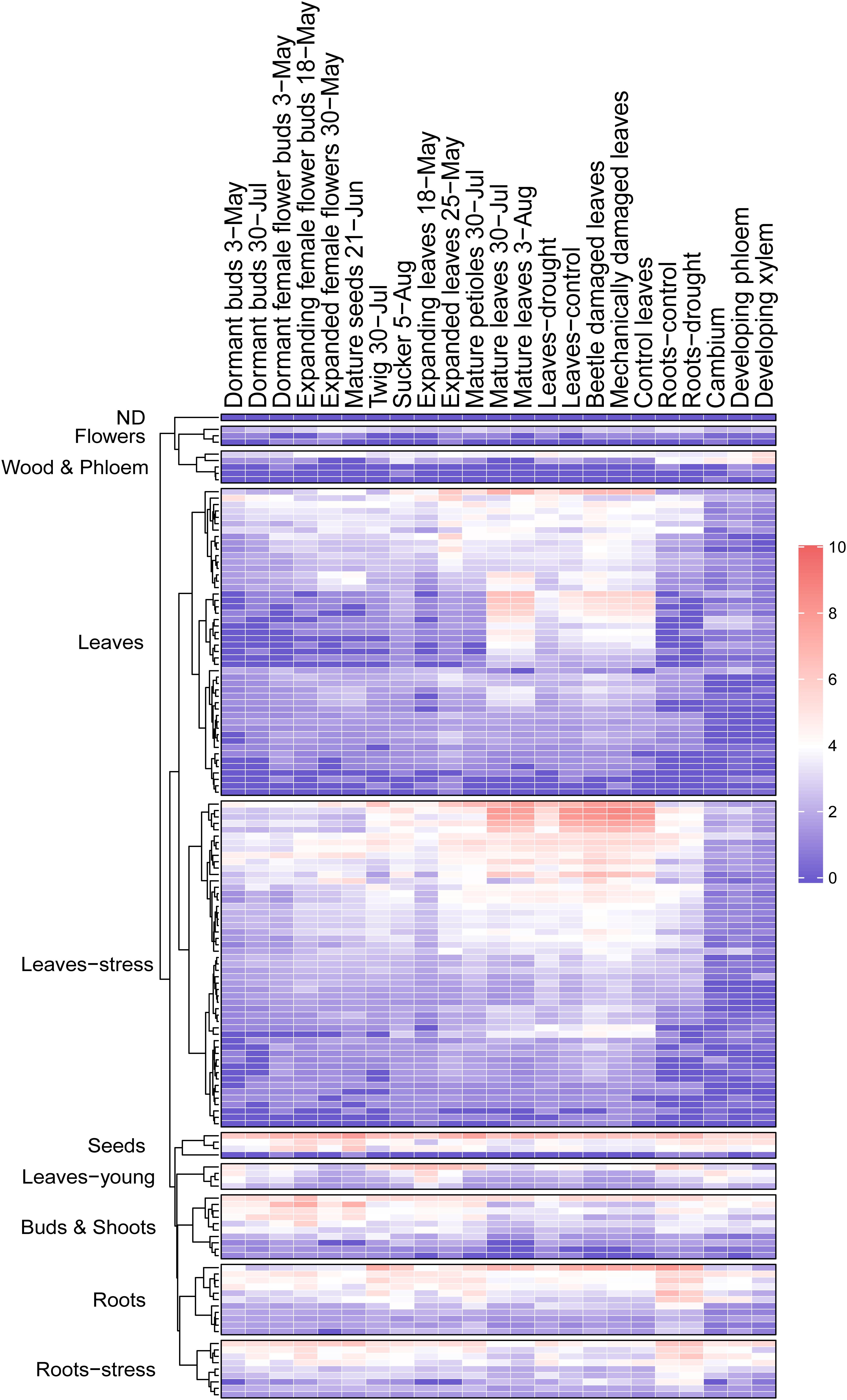
Figure 6. Heatmap of PtMD gene expression patterns in different organs of aspen. Data were retrieved from repositories described by Sundell et al. (2015) and Immanen et al. (2016), and normalized expression values are listed in Supplementary Table 6.
Twenty PtMD genes were most highly expressed in roots of which nine showed highest expression in roots exposed to drought. Genes with maximal expression values detected in stressed organs were distributed among clades I, II, IV, V, VI, VII, VIII, X, XII, and XIII (Figure 2), suggesting stress response functions for these clades. Interestingly, no gene that was maximally expressed in stressed organs was found in clades III, IX, XI or XIV, suggesting their involvement in other types of signaling. Several PtMD genes were most highly expressed in the vegetative growing organs: young roots or leaves (Figures 5, 6 and Supplementary Tables 5, 6). Eight genes, all from clades XII and XIII, were most highly expressed in female flowers at various developmental stages, and four in mature seeds. The genes highly expressed in expanding female flower buds or in mature seeds were in many cases also highly expressed in developing secondary tissues, vascular cambium or developing secondary xylem and phloem (Figure 6 and Supplementary Table 6).
PtMDs Involved in Wood Biosynthesis
Since many PtMD genes were found expressed in developing wood (Figure 5 and Supplementary Table 5), we wanted to determine at which wood developmental stage these genes are active. For that, we used the AspWood database (see text footnote 14), which provides data on high-spatial-resolution transcript abundance in developing secondary xylem and phloem tissues of aspen (Sundell et al., 2017). Only 89 PtMDs (61%) were found to be expressed in developing secondary vascular tissues (Supplementary Table 7), with the majority exhibiting distinct patterns of expression, clustering in ten expression groups (Supplementary Table 7 and Figure 7). This clustering indicates that certain sets of PtMDs have specific functions at certain stages of secondary vascular development. Some of the PtMD genes expressed in secondary vascular tissue also exhibited high expression under diverse stress conditions in leaves or roots (Supplementary Table 6 and Figure 2).
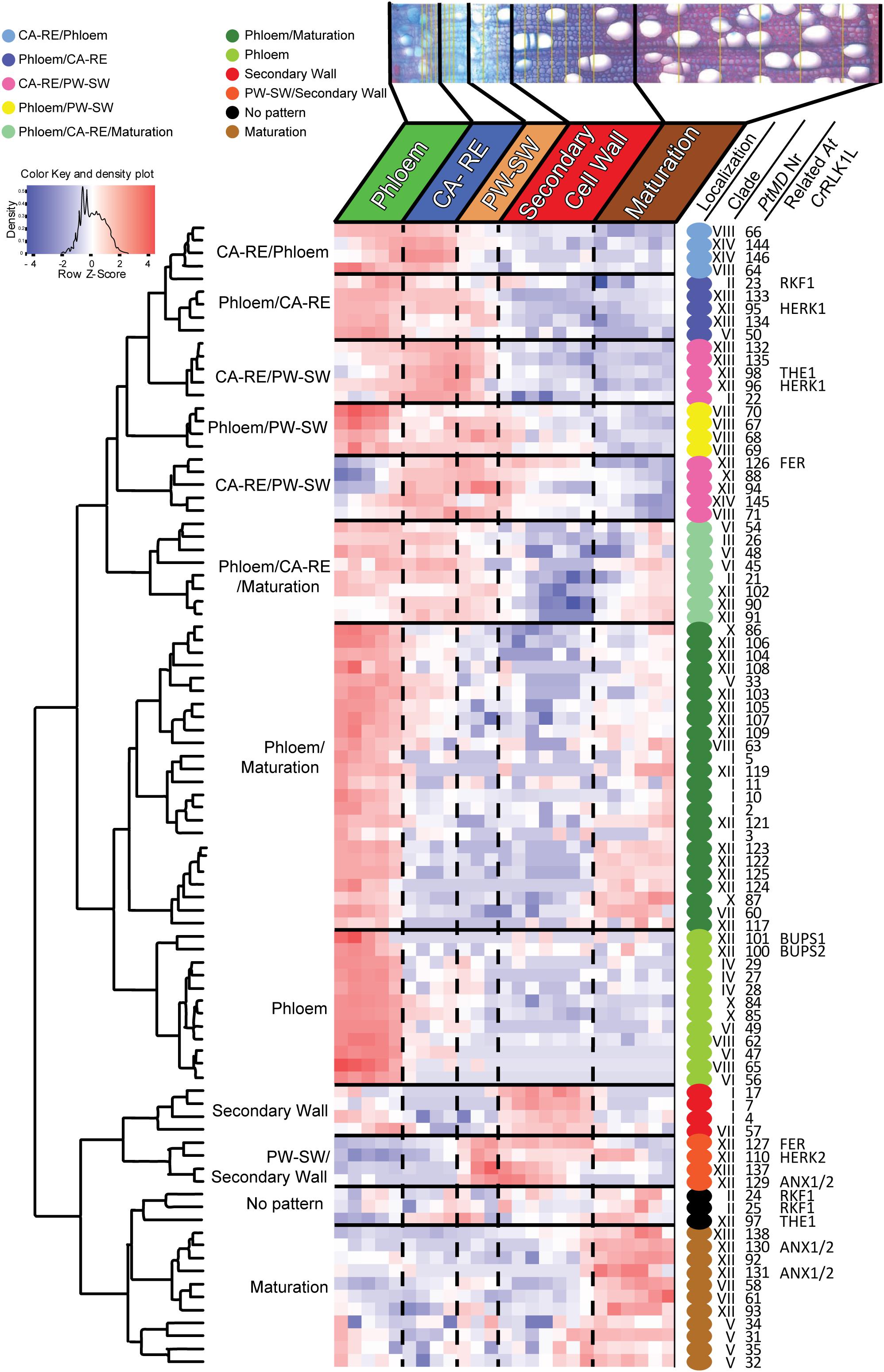
Figure 7. Heatmap of scaled PtMD expression patterns in developing secondary vascular tissues based on the AspWood database (http://aspwood.popgenie. org/aspwood-v3.0/). The majority of PtMD genes show maximum expression in the phloem and in the cambium-radial expansion zone (CA-RE). Smaller clusters of genes are expressed in the developing xylem including the secondary wall formation zone, the transition between the primary and secondary wall zone (PW-SW), or the maturation zone. Specific wood developmental stages are defined based on the patterns of expression of marker genes (Sundell et al., 2017). Colored dots beside PtMDs identify groups with maximum expression in different developmental zones.
The largest group of PtMD genes (50) that were expressed in secondary vascular tissue showed a peak of expression in the phloem (Supplementary Table 7 and Figure 7). These genes were mostly from superclades LRR-MD-PK including many members of clade VI and VIII without orthologs in Arabidopsis, MLD-PK (CrRLK1L), and MLD-LRR-PK. Cambium and radial expansion zones were the zones characterized by the greatest variety of PtMD transcripts including members of superclades MD-Kin, LRR-MD-PK, MLD-LRR, and MLD-PK (CrRLK1L). In contrast, PtMD genes having a peak of expression at the transition between primary and secondary wall deposition were mostly from the MLD-PK (CrRLK1L) group. Intriguingly, the genes with maximum expression during secondary wall deposition were expressed at relatively low levels and many of them belonged to clade I of PtMDs, which lacks LRR. PtMD genes with the highest expression in the maturation zone were mostly from clades V and XII.
Networks of Xylogenesis-Related PtMD Genes
To find putative partners involved in signaling pathways together with the xylogenesis-related PtMD genes, we analyzed co-expression networks of PtMD genes identified as being expressed during xylogenesis. Ten PtMD genes forming two clusters with a peak of expression in the cambium-radial expansion zone and primary to secondary transition zone (CA-RE/PW-SW), and eight genes from clusters PW-SW/Secondary Wall and Secondary Wall (Figure 7 and Supplementary Table 7), representing, respectively, the early and main stages of secondary wall deposition were used as baits for network analyses.
The baits for the CA-RE/PW-SW zones formed five separate networks (Figure 8A and Supplementary Table 8), the largest being that of PtMD126 -one of the two poplar orthologs of AtFER. It included several candidates for functioning in signaling by phosphorylation relay and ROS, and for regulation of cell wall development. Apoplastic ROS in wood forming tissues could have a double role, in signaling and in regulation of lignin polymerization. Thus, the PtMD126 network included a homolog of PBS1-LIKE 19 (AtPBL19), encoding a RLCK of subfamily VII-4, which signals a response to chitin perceived by CHITIN ELICITOR RECEPTOR KINASE 1 (AtCERK1) through a phosphorylation relay (Bi et al., 2018), and ROS production (Rao et al., 2018). Homologs of AtTGA1 and AtTGA7, which encode basic leucine zipper transcription factors involved in oxidative stress-mediated responses to biotrophic and necrotrophic pathogens (reviewed by Gatz, 2013), were, respectively, positively and negatively correlated with PtMD126 (Figure 8A and Table 1). The oxidation state of AtTGA1 is regulated by a glutaredoxin, AtROXY19 (Li et al., 2019), the homolog of which has been found to respond to altered secondary wall xylan in aspen (Ratke et al., 2018), suggesting that the PtMD126 network might include candidates for sensing secondary wall integrity. AtTGA1 interacts with the BLADE-ON-PETIOLE 1 and 2 (AtBOP1/2) transcription factors (Wang et al., 2019), which are known to regulate xylem fiber differentiation (Liebsch et al., 2014). Moreover, the network includes a homolog of the BEL1-LIKE HOMEODOMAIN 8 (AtBLH8) transcription factor, which controls expression of BOP1 (Khan et al., 2015; Figure 8A and Table 1). The network also includes a homolog of the gene encoding GROWTH-REGULATING FACTOR 9 (AtGRF9), a 14-3-3 protein that regulates developmental programs and stress signaling by binding phosphoproteins and regulating their activities (Mayfield et al., 2007; Liu et al., 2014; Omidbakhshfard et al., 2018). The presence of a homolog of IMPORTIN-BETA 4 (AtIMB4), which is required to transport GRF-INTERACTING FACTOR 1 (AtGIF1) to the nucleus (Liu et al., 2019; Figure 8A and Table 1) further supports the involvement of GRF/14-3-3 genes in the PtMD126 network.
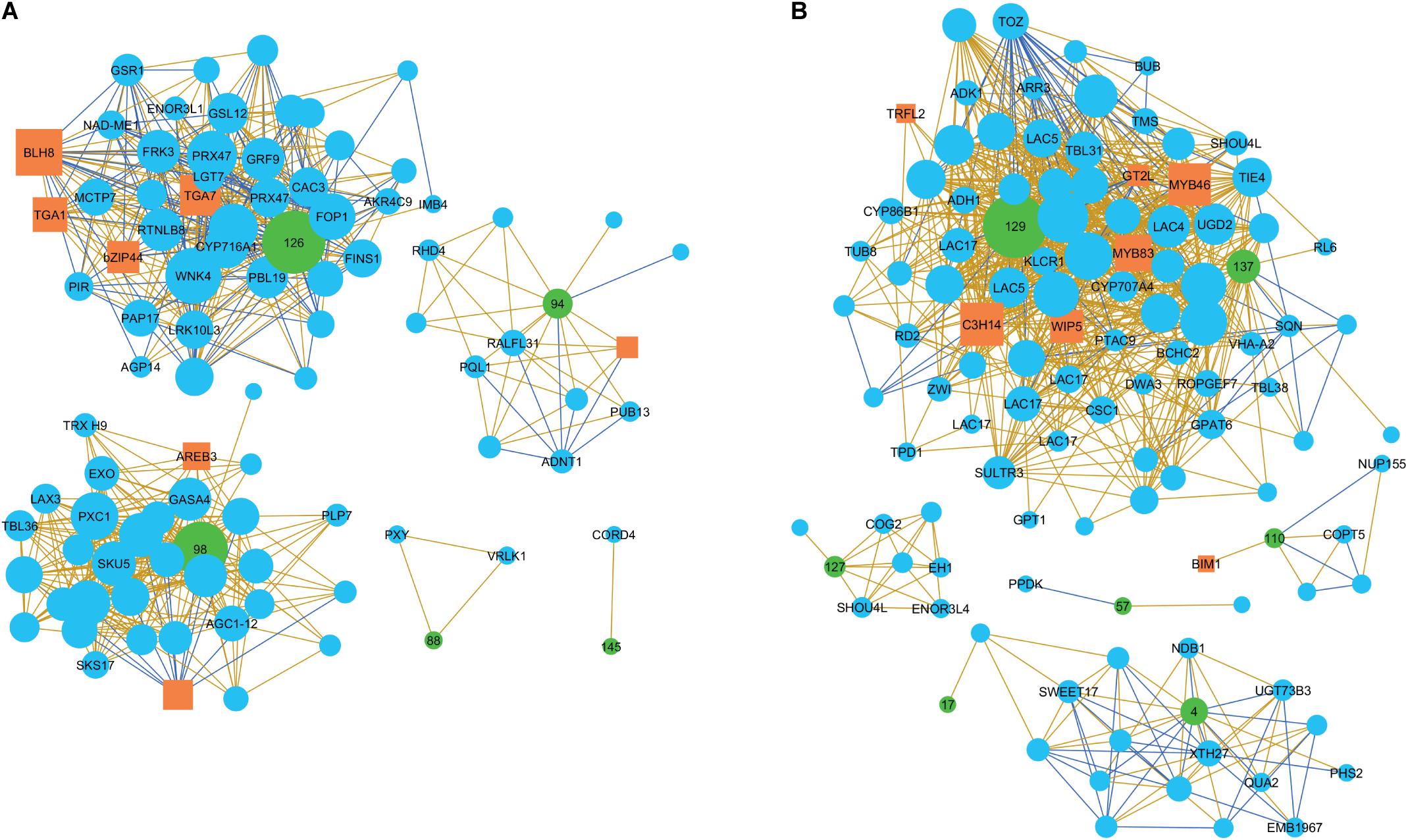
Figure 8. Co-expression networks of PtMDs in developing wood of aspen. The networks of genes expressed during the early (A) and main (B) stages of secondary wall deposition. The co-expressed genes were selected with threshold = 5 from the AspWood database (http://aspwood.popgenie.org/aspwood-v3.0/) and visualized in Cytoscape v 3.4.0. The transcription factors are marked by orange rectangles and the PtMD genes, used as baits, by green circles with PtMD ID numbers. PtMD7, 22, 71, 96, 132 and 135 did not have any co-expressed genes (not shown). The corresponding Arabidopsis gene names are used in the figures. Positive and negative correlations are shown by beige and blue lines, respectively. The size of each marker corresponds to the number of correlations associated with it.
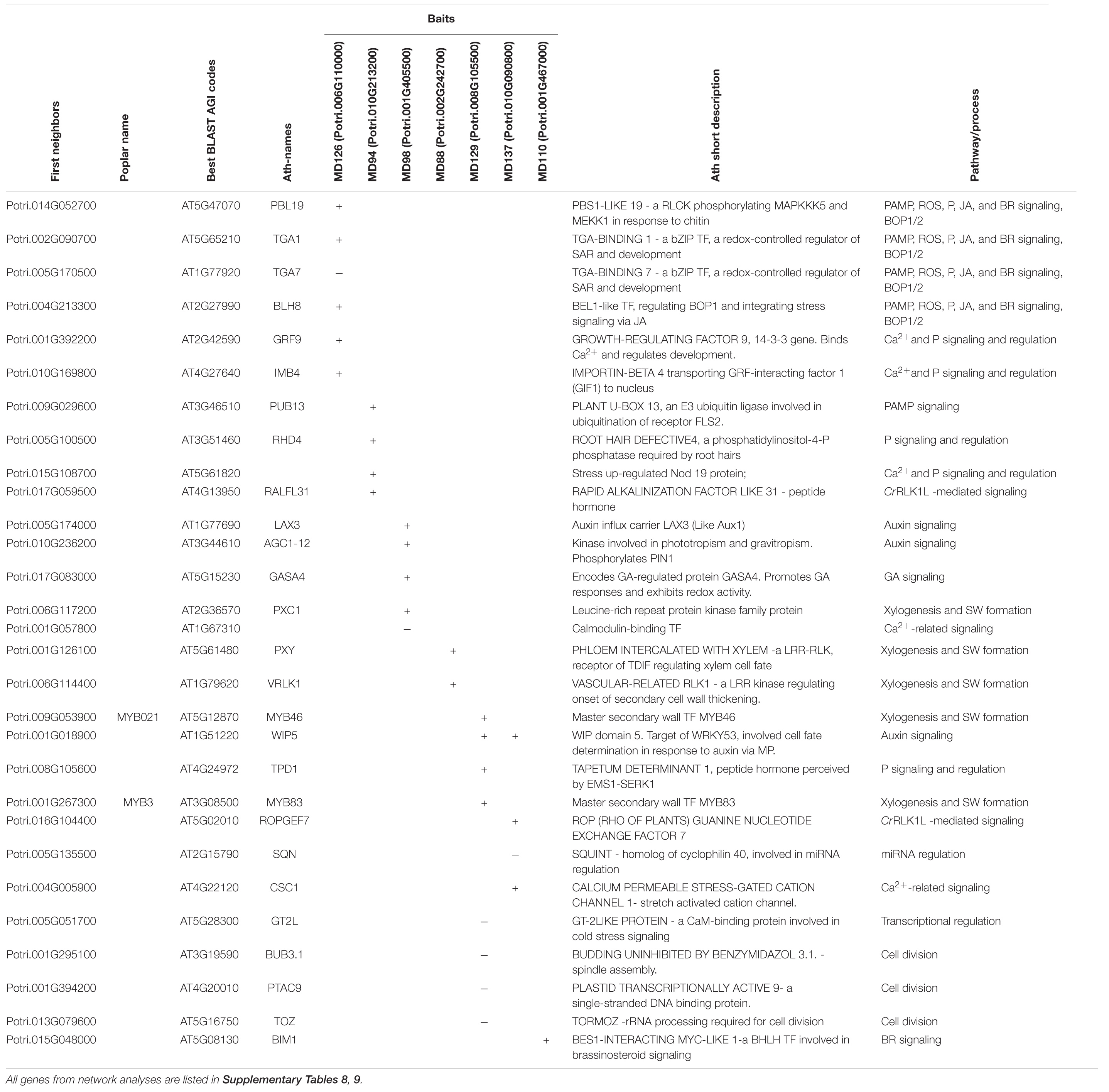
Table 1. Genes co-regulated with poplar MD genes expressed during secondary wall formation that were discussed in the text.
A separate large network was formed by neighbors of PtMD98 -one of the two poplar orthologs of AtTHE1 (Figure 8A, Table 1 and Supplementary Table 8). This network comprised genes related to hormonal signaling by IAA and GA, and to the regulation of xylogenesis. One example is a homolog of AtLAX3, which encodes an auxin influx carrier (Swarup et al., 2008). Another is a homolog of AtAGC1-12, encoding a kinase phosphorylating the auxin efflux carrier AtPIN1 (Haga et al., 2018). We have also identified a homolog of AtGASA4 involved in GA responses and redox regulation (Rubinovich and Weiss, 2010). It is noteworthy that GA responses and GASA genes were also found to be upregulated in response to a secondary wall xylan defect in aspen (Ratke et al., 2018). Moreover, the co-expression network included an LRR-RLK homologous to AtPXY-CORRELATED 1 (AtPXC1), which is required for secondary wall deposition (Wang et al., 2013).
The network of PtMD94 -the clade XII member related to AtHERK1 and AtCVY1 -included a homolog of AtRALFL31 (Figure 8A, Table 1 and Supplementary Table 8). RALF genes encode hormone peptides that signal developmental processes and stress responses by interacting with CrRLK1Ls. AtRALFL31 belongs to subfamily IIIA which includes as yet uncharacterized members, but both AtRALFL31 and Potri.017G059500 have the conserved YISY motif essential for interaction with AtFER (Campbell and Turner, 2017). Thus, Potri.017G059500 could potentially encode a peptide hormone recognized by PtMD94. The PtMD94 network also included other candidates for signaling. For example, there was a homolog of PLANT U-BOX 13 (AtPUB13), which encodes an E3 ligase involved in signal-activated ubiquitination and subsequent degradation of different receptors including ABA INSENSITIVE 1 (AtABI1) (Kong et al., 2015), BRASSINOSTEROID INSENSITIVE 1 (AtBRI1) (Zhou et al., 2018), LYSM-CONTAINING RECEPTOR-LIKE KINASE 5 (AtLYK5) (Liao et al., 2017), and FLAGELLIN-SENSITIVE 2 (AtFLS2) (Liu et al., 2012; Antignani et al., 2015). The ubiquitination of flg22-bound AtFLS2 by AtPUB13 depends on its interactor protein RAB GTPASE HOMOLOG A 4B (AtRABA4B) (Antignani et al., 2015). Interestingly, a homolog to another PtMD94 network member encodes ROOT HAIR DEFECTIVE 4 (AtRHD4) which mediates polar localization of AtRABA4B (Thole et al., 2008). Consequently, it seems likely that these PtMD94 network members are indeed functionally linked within the same network.
Two other PtMD genes expressed during early secondary wall biosynthesis, PtMD88 and PtMD145, formed small networks, which included important regulatory genes in xylem cell differentiation (Figure 8A, Table 1 and Supplementary Table 8). One of them was the homolog of the master spatial regulator of vascular differentiation, PHLOEM INTERCALATED WITH XYLEM (AtPXY), encoding an LRR-RLK that promotes cell division in the cambium upon binding the small CLE peptide AtTDIF, which is essential for xylem differentiation (Fisher and Turner, 2007). The other was a homolog of VASCULAR-RELATED RECEPTOR-LIKE KINASE 1 (AtVRLK1) (Huang et al., 2018) which is probably responsible for the switch between xylem cell expansion and secondary wall deposition.
The late secondary wall-expressed baits formed five networks (Figure 8B, Table 1 and Supplementary Table 9). The largest of these was associated with two PtMD genes, PtMD129, a clade XII member related to AtANX1 and AtANX2, and PtMD137, which encodes an LRR-RLK, from clade XIII. Orthologs of key signaling-related genes were included within this network. One of them was CALCIUM PERMEABLE STRESS-GATED CATION CHANNEL 1 (AtCSC1). Stretch-activated Ca2+ channels have been predicted to be important players in CWI (Engelsdorf and Hamann, 2014). AtCSC1 belongs to a newly characterized family of stretch-activated Ca2+ channels conserved in eukaryotes (Hou et al., 2014; Liu et al., 2018). In addition, we found Potri.015G108700/AT5G61820, encoding an uncharacterized NOD19-like protein, which has been implicated in responses to cold stress downstream of mechanosensitive Ca2+ channels (Mori et al., 2018). The aspen homolog of AtCSC1 is thus a promising candidate for a secondary wall damage sensor. Another important signaling-related homolog is TAPETUM DETERMINANT 1 (AtTPD1), which encodes a small peptide hormone that is recognized by an RLK complex consisting of AtEMS1 and AtSERK1/2 to activate transcription factors of the BES1 family (Chen et al., 2019). Moreover, a homolog of Arabidopsis ROP (RHO OF PLANTS) GUANINE NUCLEOTIDE EXCHANGE FACTOR 7 (AtROPGEF7) was among the hits for PtMD137. AtROPGEF7 interacts with the kinase domain of AtFER, mediating downstream NADPH oxidase-dependent ROS signaling which is needed for polarized cell growth (Duan et al., 2010). Finally, we identified a homolog of Arabidopsis GT-2LIKE PROTEIN (AtGT2L), which encodes a Ca2+-dependent calmodulin (CaM)-binding trihelix transcription factor involved in plant abiotic stress signaling (Xi et al., 2012). In addition to signaling-related genes, the PtMD129-PtMD137 network included some key cell fate regulator proteins (Figure 8B, Table 1 and Supplementary Table 9). One of these was the homolog of WIP DOMAIN PROTEIN 5 (AtWIP5), which encodes a zinc-finger protein involved in root patterning downstream of auxin (Crawford et al., 2015) and ROS signaling (Miao et al., 2004). Another was a homolog of Arabidopsis SQUINT (AtSQN), which encodes a cyclophilin 40-like protein that promotes the accumulation of miRNAs miR156 and miR172, targeting master regulatory genes in organ development (Smith et al., 2009; Prunet et al., 2015). The network also included orthologs of two genes encoding master transcriptional regulators, AtMYB46 and AtMYB83, which activate the secondary wall program (Zhong and Ye, 2012). Both these genes showed positive correlation with PtMD129. In contrast, orthologs of three genes with roles in cell division were negatively correlated with PtMD129 (Table 1). This supports the hypothesis that secondary wall integrity signaling results in coordination between cell division and secondary wall formation activities in developing wood (Ratke et al., 2018).
The network for PtMD110, which together with PtMD111 forms a pair orthologous to AtHERK2, included a homolog of the Arabidopsis gene encoding the transcription factor BES1-INTERACTING MYC-LIKE1 (AtBIM1) (Figure 8B, Table 1 and Supplementary Table 9), which mediates brassinosteroid signaling (Chandler et al., 2009). Several other genes discussed above can be linked to BR-dependent or BES-related BR-independent signaling (Table 1). Intriguingly, a secondary wall xylan defect induced transcriptomic changes suggesting stimulation of BR signaling in aspen (Ratke et al., 2018), supporting the involvement of the AtBIM1 homolog in sensing secondary wall integrity.
Conclusion
Malectin and malectin-like domains (MD/MLD) are lectin-like motifs found in proteins (MD proteins) of pro- and eukaryotes; they are particularly abundant in plants, where they carry out essential signaling functions in defense and development (Bellande et al., 2017; Franck et al., 2018). This has been shown by studies on MD genes from herbaceous plants such as Arabidopsis (Bellande et al., 2017; Sultana et al., 2020), strawberry (Zhang et al., 2016) and rice (Jing et al., 2020). However, no such comprehensive study has been available for MD genes in trees. Here we carried out a census of MD genes in the model woody species P. trichocarpa (Supplementary Table 1) and expanded the set for A. thaliana (Supplementary Table 2).
In total, 146 MD genes were found in P. trichocarpa and they were assigned to fourteen clades based on sequence similarity, and to five superclades based on predicted protein domain organization and intron-exon structures (Figures 1, 2). The variety of MD protein structures reflects their range of different functions in plants.
Additional genome-wide analysis by using available sequence data from different woody species revealed, that certain MD genes appeared to be specific either to trees or to the Populus lineage and absent from Arabidopsis (Supplementary Figure 3). The prevalence of tandem duplications within the MD gene family, which apparently led to family expansion, may have created conditions conducive to gene neofunctionalization and rapid evolution (Schaper and Anisimova, 2015; Choi et al., 2016).
The majority of the poplar MD genes were found to be highly expressed in mature leaves, particularly those subjected to biotic and abiotic stress conditions (Figure 6), supporting their role in stress signaling. Detailed analysis of expression in wood forming tissues revealed subsets upregulated in xylem cells during secondary wall deposition (Figure 7). These genes, not unexpectedly, include candidates for the sensing of cell wall integrity. We identified their co-expression networks revealing potential molecular pathways in which these MD genes might participate to ensure the coordination of secondary wall formation (Table 1).
The current study provides an extensive analysis of Populus MD genes and opens the possibility to better understand their role in essential physiological pathways related to stress signaling and the regulation of wood formation in trees.
Data Availability Statement
The datasets presented in this study can be found in online repositories. The names of the repository/repositories and accession number(s) can be found in the article/Supplementary Material.
Author Contributions
VK identified MD genes and their chromosomal clustering, and wrote the first draft. VK and FB identified protein domains and the main clades of MD genes. VK and SK analyzed exon-intron structures. ED analyzed gene expression in leaves and wood. VK and ED analyzed in silico gene expression in different organs. ED, JU, and VK analyzed conserved regions in MD and MLD of poplar. VK and FB analyzed co-expression networks. VK and CM analyzed the phylogeny across the tree species. EM conceived and coordinated the project, and finalized the manuscript with contributions from all authors. All authors contributed to the article and approved the submitted version.
Funding
This work was supported by Swedish Research Council, the Kempe Foundation, the Knut and Alice Wallenberg Foundation, and the SSF program ValueTree RBP14-0011.
Conflict of Interest
The authors declare that the research was conducted in the absence of any commercial or financial relationships that could be construed as a potential conflict of interest.
Acknowledgments
We thank Dr. Nicolas Delhomme and the Bioinformatics Facility at UPSC for allowing us to use their server during the work. This manuscript has been released as a pre-print at Research Square, Kumar et al. (2020).
Supplementary Material
The Supplementary Material for this article can be found online at: https://www.frontiersin.org/articles/10.3389/fpls.2020.588846/full#supplementary-material
Footnotes
- ^ http://www.cazy.org/
- ^ http://popgenie.org
- ^ https://pfam.xfam.org
- ^ http://www.arabidopsis.org/
- ^ http://cdvist.zhulinlab.org
- ^ https://web.expasy.org/protparam/
- ^ www.cbs.dtu.dk/services/SignalP/
- ^ http://www.cbs.dtu.dk/services/DeepLoc/
- ^ http://popgenie.org/gbrowse
- ^ http://popgenie.org/chromosome-diagram
- ^ http://phylogeny.lirmm.fr/phylo_cgi/index.cgi
- ^ https://genome.jgi.doe.gov/portal/pages/dynamicOrganismDownload.jsf?organism=Phytozome
- ^ www.bioinformatics.babraham.ac.uk/projects/fastqc/
- ^ https://www.ebi.ac.uk/ena/browser/view/PRJEB41170
- ^ http://aspwood.popgenie.org/aspwood-v3.0/
- ^ http://popgenie.org/exnet
References
Adebali, O., Ortega, D. R., and Zhulin, I. B. (2015). CDvist: a webserver for identification and visualization of conserved domains in protein sequences. Bioinformatics 31, 1475–1477. doi: 10.1093/bioinformatics/btu836
Altschul, S. F., Gish, W., Miller, W., Myers, E. W., and Lipman, D. J. (1990). Basic local alignment search tool. J. Mol. Biol. 215, 403–410. doi: 10.1016/S0022-2836(05)80360-2
Antignani, V., Klocko, A. L., Bak, G., Chandrasekaran, S. D., Dunivin, T., and Nielsen, E. (2015). Recruitment of PLANT U-BOX13 and the PI4Kβ1/β2 phosphatidylinositol-4 kinases by the small GTPase RabA4B plays important roles during salicylic acid-mediated plant defense signaling in Arabidopsis. Plant Cell 27, 243–261. doi: 10.1105/tpc.114.134262
Armenteros, A. J. J., Sønderby, C. K., Sønderby, S. K., Nielsen, H., and Winther, O. (2017). DeepLoc: prediction of protein subcellular localization using deep learning. Bioinformatics 33, 3387–3395. doi: 10.1093/bioinformatics/btx431
Asai, T., Tena, G., Plotnikova, J., Willmann, M. R., Chiu, W.-L., Gomez-Gomez, L., et al. (2002). MAP kinase signalling cascade in Arabidopsis innate immunity. Nature 415, 977–983. doi: 10.1038/415977a
Bella, J., Hindle, K. L., McEwan, P. A., and Lovell, S. C. (2008). The leucine-rich repeat structure. Cell. Mol. Life Sci. 65, 2307–2333. doi: 10.1007/s00018-008-8019-0
Bellande, K., Bono, J.-J., Savelli, B., Jamet, E., and Canut, H. (2017). Plant lectins and lectin receptor-like kinases: how do they sense the outside? Intern. J. Mol. Sci. 18:1164. doi: 10.3390/ijms18061164
Bi, G., Zhou, Z., Wang, W., Li, L., Rao, S., Wu, Y., et al. (2018). Receptor-like cytoplasmic kinases directly link diverse pattern recognition receptors to the activation of mitogen-activated protein kinase cascades in Arabidopsis. Plant Cell 30, 1543–1561. doi: 10.1105/tpc.17.00981
Boisson-Dernier, A., Franck, C. M., Lituiev, D. S., and Grossniklaus, U. (2015). Receptor-like cytoplasmic kinase MARIS functions downstream of CrRLK1L-dependent signaling during tip growth. Proc. Natl. Acad. Sci. U.S.A. 112, 12211–12216. doi: 10.1073/pnas.1512375112
Boisson-Dernier, A., Lituiev, D. S., Nestorova, A., Franck, C. M., Thirugnanarajah, S., and Grossniklaus, U. (2013). ANXUR receptor-like kinases coordinate cell wall integrity with growth at the pollen tube tip via NADPH oxidases. PLoS Biol. 11:e1001719. doi: 10.1371/journal.pbio.1001719
Bray, N. L., Pimentel, H., Melsted, P., and Pachter, L. (2016). Near-optimal probabilistic RNA-seq quantification. Nat. Biotechnol. 34, 525–527. doi: 10.1038/nbt.3519
Buchfink, B., Xie, C., and Huson, D. H. (2015). Fast and sensitive protein alignment using DIAMOND. Nat. Methods 12, 59–60. doi: 10.1038/nmeth.3176
Campbell, L., and Turner, S. R. (2017). A comprehensive analysis of RALF proteins in green plants suggests there are two distinct functional groups. Front. Plant Sci. 8:37. doi: 10.3389/fpls.2017.00037
Chandler, J. W., Cole, M., Flier, A., and Werr, W. (2009). BIM1, a bHLH protein involved in brassinosteroid signalling, controls Arabidopsis embryonic patterning via interaction with DORNRÖSCHEN and DORNRÖSCHEN-LIKE. Plant Mol. Biol. 69, 57–68. doi: 10.1007/s11103-008-9405-6
Chen, W., Lv, M., Wang, Y., Wang, P.-A., Cui, Y., Li, M., et al. (2019). BES1 is activated by EMS1-TPD1-SERK1/2-mediated signaling to control tapetum development in Arabidopsis thaliana. Nat. Commun. 10:4164. doi: 10.1038/s41467-019-12118-4
Choi, K., Reinhard, C., Serra, H., Ziolkowski, P. A., Underwood, C. J., Zhao, X., et al. (2016). Recombination rate heterogeneity within Arabidopsis disease resistance genes. PLoS Genet. 12:e1006179. doi: 10.1371/journal.pgen.1006179
Crawford, B. C. W., Sewell, J., Golembeski, G., Roshan, C., Long, J. A., and Yanofsky, M. F. (2015). Genetic control of distal stem cell fate within root and embryonic meristems. Science 347, 655–659. doi: 10.1126/science.aaa0196
Crooks, G. E., Hon, G., Chandonia, J.-M., and Brenner, S. E. (2004). WebLogo: a sequence logo generator. Genome Res. 14, 1188–1190. doi: 10.1101/gr.849004
Denness, L., McKenna, J. F., Segonzac, C., Wormit, A., Madhou, P., Bennett, M., et al. (2011). Cell wall damage-induced lignin biosynthesis is regulated by a reactive oxygen species- and jasmonic acid-dependent process in Arabidopsis. Plant Physiol. 156, 1364–1374. doi: 10.1104/pp.111.175737
Draeger, C., Ndinyanka Fabrice, T., Gineau, E., Mouille, G., Kuhn, B. M., Moller, I., et al. (2015). Arabidopsis leucine-rich repeat extensin (LRX) proteins modify cell wall composition and influence plant growth. BMC Plant Biol. 15:155. doi: 10.1186/s12870-015-0548-8
Du, C., Li, X., Chen, J., Chen, W., Li, B., Li, C., et al. (2016). Receptor kinase complex transmits RALF peptide signal to inhibit root growth in Arabidopsis. Proc. Natl. Acad. Sci. U.S.A. 113, E8326–E8334. doi: 10.1073/pnas.1609626113
Du, S., Qu, L.-J., and Xiao, J. (2018). Crystal structures of the extracellular domains of the CrRLK1L receptor-like kinases ANXUR1 and ANXUR2. Protein Sci. 27, 886–892. doi: 10.1002/pro.3381
Duan, Q., Kita, D., Li, C., Cheung, A. Y., and Wu, H.-M. (2010). FERONIA receptor-like kinase regulates RHO GTPase signaling of root hair development. Proc. Natl. Acad. Sci. U.S.A. 107, 17821–17826. doi: 10.1073/pnas.1005366107
Dünser, K., Gupta, S., Herger, A., Feraru, M. I., Ringli, C., and Kleine-Vehn, J. (2019). Extracellular matrix sensing by FERONIA and leucine-rich repeat extensins controls vacuolar expansion during cellular elongation in Arabidopsis thaliana. EMBO J. 38:e100353. doi: 10.15252/embj.2018100353
El-Gebali, S., Mistry, J., Bateman, A., Eddy, S. R., Luciani, A., Potter, S. C., et al. (2019). The Pfam protein families database in 2019. Nucleic Acids Res. 47, D427–D432. doi: 10.1093/nar/gky995
Engelsdorf, T., and Hamann, T. (2014). An update on receptor-like kinase involvement in the maintenance of plant cell wall integrity. Ann. Bot. 114, 1339–1347. doi: 10.1093/aob/mcu043
Escobar-Restrepo, J.-M., Huck, N., Kessler, S., Gagliardini, V., Gheyselinck, J., Yang, W.-C., et al. (2007). The FERONIA receptor-like kinase mediates male-female interactions during pollen tube reception. Science 317, 656–660. doi: 10.1126/science.1143562
Feng, W., Kita, D., Peaucelle, A., Cartwright, H. N., Doan, V., Duan, Q., et al. (2018). The FERONIA receptor kinase maintains cell-wall integrity during salt stress through Ca2 + signaling. Curr. Biol. 28, 666–675.e665. doi: 10.1016/j.cub.2018.01.023
Fisher, K., and Turner, S. (2007). PXY, a receptor-like kinase essential for maintaining polarity during plant vascular-tissue development. Curr. Biol. 17, 1061–1066. doi: 10.1016/j.cub.2007.05.049
Foreman, J., Demidchik, V., Bothwell, J. H. F., Mylona, P., Miedema, H., Torres, M. A., et al. (2003). Reactive oxygen species produced by NADPH oxidase regulate plant cell growth. Nature 422, 442–446. doi: 10.1038/nature01485
Franck, C. M., Westermann, J., and Boisson-Dernier, A. (2018). Plant malectin-like receptor kinases: from cell wall integrity to immunity and beyond. Ann. Rev. Plant Biol. 69, 301–328. doi: 10.1146/annurev-arplant-042817-40557
Galindo-Trigo, S., Grand, T. M., Voigt, C. A., and Smith, L. M. (2020). A malectin domain kinesin functions in pollen and seed development in Arabidopsis. J. Exper. Bot. 71, 1828–1841. doi: 10.1093/jxb/eraa023
Galindo-Trigo, S., Gray, J. E., and Smith, L. M. (2016). Conserved roles of CrRLK1L receptor-like kinases in cell expansion and reproduction from algae to angiosperms. Front. Plant Sci. 7:1269. doi: 10.3389/fpls.2016.01269
Gatz, C. (2013). From pioneers to team players: TGA transcription factors provide a molecular link between different stress pathways. Mol. Plant Microb. Interact. 26, 151–159. doi: 10.1094/mpmi-04-12-0078-ia
Ge, Z., Bergonci, T., Zhao, Y., Zou, Y., Du, S., Liu, M.-C., et al. (2017). Arabidopsis pollen tube integrity and sperm release are regulated by RALF-mediated signaling. Science 358, 1596–1600. doi: 10.1126/science.aao3642
Gentleman, R. C., Carey, V. J., Bates, D. M., Bolstad, B., Dettling, M., Dudoit, S., et al. (2004). Bioconductor: open software development for computational biology and bioinformatics. Genome Biol. 5:R80. doi: 10.1186/gb-2004-5-10-r80
Gish, L. A., and Clark, S. E. (2011). The RLK/Pelle family of kinases. Plant J. 66, 117–127. doi: 10.1111/j.1365-313X.2011.04518.x
Gonneau, M., Desprez, T., Martin, M., Doblas, V. G., Bacete, L., Miart, F., et al. (2018). Receptor kinase THESEUS1 is a rapid alkalinization factor 34 receptor in Arabidopsis. Curr. Biol. 28, 2452–2458.e2454. doi: 10.1016/j.cub.2018.05.075
Gu, Z., Eils, R., and Schlesner, M. (2016). Complex heatmaps reveal patterns and correlations in multidimensional genomic data. Bioinformatics 32, 2847–2849. doi: 10.1093/bioinformatics/btw313
Haga, K., Frank, L., Kimura, T., Schwechheimer, C., and Sakai, T. (2018). Roles of AGCVIII kinases in the hypocotyl phototropism of Arabidopsis seedlings. Plant Cell Physiol. 59, 1060–1071. doi: 10.1093/pcp/pcy048
Hamann, T. (2015). The plant cell wall integrity maintenance mechanism - A case study of a cell wall plasma membrane signaling network. Phytochemistry 112, 100–109. doi: 10.1016/j.phytochem.2014.09.019
Haruta, M., Sabat, G., Stecker, K., Minkoff, B. B., and Sussman, M. R. (2014). A peptide hormone and its receptor protein kinase regulate plant cell expansion. Science 343, 408–411. doi: 10.1126/science.1244454
Hématy, K., Sado, P.-E., Van Tuinen, A., Rochange, S., Desnos, T., Balzergue, S., et al. (2007). A receptor-like kinase mediates the response of Arabidopsis cells to the inhibition of cellulose synthesis. Curr. Biol. 17, 922–931. doi: 10.1016/j.cub.2007.05.018
Holub, E. B. (2001). The arms race is ancient history in Arabidopsis, the wildflower. Nat. Rev. Genet. 2, 516–527. doi: 10.1038/35080508
Hou, C., Tian, W., Kleist, T., He, K., Garcia, V., Bai, F., et al. (2014). DUF221 proteins are a family of osmosensitive calcium-permeable cation channels conserved across eukaryotes. Cell Res. 24, 632–635. doi: 10.1038/cr.2014.14
Huang, C., Zhang, R., Gui, J., Zhong, Y., and Li, L. (2018). The receptor-like kinase AtVRLK1 regulates secondary cell wall thickening. Plant Physiol. 177, 671–683. doi: 10.1104/pp.17.01279
Immanen, J., Nieminen, K., Smolander, O.-P., Kojima, M., Alonso Serra, J., Koskinen, P., et al. (2016). Cytokinin and auxin display distinct but interconnected distribution and signaling profiles to stimulate cambial activity. Curr. Biol. 26, 1990–1997. doi: 10.1016/j.cub.2016.05.053
Jing, X.-Q., Shalmani, A., Zhou, M.-R., Shi, P.-T., Muhammad, I., Shi, Y., et al. (2020). Genome-wide identification of malectin/malectin-like domain containing protein family genes in rice and their expression regulation under various hormones, abiotic stresses, and heavy metal treatments. J. Plant Growth Regul. 39, 492–506. doi: 10.1007/s00344-019-09997-8
Katoh, K., Kuma, K.-I., Toh, H., and Miyata, T. (2005). MAFFT version 5: improvement in accuracy of multiple sequence alignment. Nucleic Acids Res. 33, 511–518. doi: 10.1093/nar/gki198
Kessler, S. A., Shimosato-Asano, H., Keinath, N. F., Wuest, S. E., Ingram, G., Panstruga, R., et al. (2010). Conserved molecular components for pollen tube reception and fungal invasion. Science 330, 968–971. doi: 10.1126/science.1195211
Khan, M., Ragni, L., Tabb, P., Salasini, B. C., Chatfield, S., Datla, R., et al. (2015). Repression of lateral organ boundary genes by PENNYWISE and POUND-FOOLISH is essential for meristem maintenance and flowering in Arabidopsis. Plant Physiol. 169, 2166–2186. doi: 10.1104/pp.15.00915
Kong, L., Cheng, J., Zhu, Y., Ding, Y., Meng, J., Chen, Z., et al. (2015). Degradation of the ABA co-receptor ABI1 by PUB12/13 U-box E3 ligases. Nat. Commun. 6:8630. doi: 10.1038/ncomms9630
Kopylova, E., Noé, L., and Touzet, H. (2012). SortMeRNA: fast and accurate filtering of ribosomal RNAs in metatranscriptomic data. Bioinformatics 28, 3211–3217. doi: 10.1093/bioinformatics/bts611
Kumar, S., Stecher, G., and Tamura, K. (2016). MEGA7: molecular evolutionary genetics analysis version 7.0 for bigger datasets. Mol. Biol. Evol. 33, 1870–1874. doi: 10.1093/molbev/msw054
Kumar, V., Barbut, F., Kushwah, S., Donev, E. N., Urbancsok, J., and Mellerowicz, E. J. (2020). Genome-wide identification of poplar malectin/malectin-like domain-containing proteins and in-silico expression analyses find novel candidates for signaling and regulation of wood development. Research Square [Preprint], doi: 10.21203/rs.3.rs-28356/v1
Kumar, V., Hainaut, M., Delhomme, N., Mannapperuma, C., Immerzeel, P., Street, N. R., et al. (2019). Poplar carbohydrate-active enzymes: whole-genome annotation and functional analyses based on RNA expression data. Plant J. 99, 589–609. doi: 10.1111/tpj.14417
Le, M. H., Cao, Y., Zhang, X.-C., and Stacey, G. (2014). LIK1, a CERK1-interacting kinase, regulates plant immune responses in Arabidopsis. PLoS One 9:e102245. doi: 10.1371/journal.pone.0102245
Lemoine, F., Correia, D., Lefort, V., Doppelt-Azeroual, O., Mareuil, F., Cohen-Boulakia, S., et al. (2019). NGPhylogeny.fr: new generation phylogenetic services for non-specialists. Nucleic Acids Res. 47, W260–W265. doi: 10.1093/nar/gkz303
Li, C., Wu, H.-M., and Cheung, A. Y. (2016). FERONIA and her pals: functions and mechanisms. Plant Physiol. 171, 2379–2392. doi: 10.1104/pp.16.00667
Li, N., Muthreich, M., Huang, L.-J., Thurow, C., Sun, T., Zhang, Y., et al. (2019). TGACG-BINDING FACTORs (TGAs) and TGA-interacting CC-type glutaredoxins modulate hyponastic growth in Arabidopsis thaliana. New Phytol. 221, 1906–1918. doi: 10.1111/nph.15496
Liao, D., Cao, Y., Sun, X., Espinoza, C., Nguyen, C. T., Liang, Y., et al. (2017). Arabidopsis E3 ubiquitin ligase PLANT U-BOX13 (PUB13) regulates chitin receptor LYSIN MOTIF RECEPTOR KINASE5 (LYK5) protein abundance. New Phytol. 214, 1646–1656. doi: 10.1111/nph.14472
Liebsch, D., Sunaryo, W., Holmlund, M., Norberg, M., Zhang, J., Hall, H. C., et al. (2014). Class I KNOX transcription factors promote differentiation of cambial derivatives into xylem fibers in the Arabidopsis hypocotyl. Development 141, 4311–4319. doi: 10.1242/dev.111369
Liu, H.-H., Xiong, F., Duan, C.-Y., Wu, Y.-N., Zhang, Y., and Li, S. (2019). Importin β4 mediates nuclear import of GRF-interacting factors to control ovule development in Arabidopsis. Plant Physiol. 179, 1080–1092. doi: 10.1104/pp.18.01135
Liu, J., Li, W., Ning, Y., Shirsekar, G., Cai, Y., Wang, X., et al. (2012). The U-Box E3 ligase SPL11/PUB13 is a convergence point of defense and flowering signaling in plants. Plant Physiol. 160, 28–37. doi: 10.1104/pp.112.199430
Liu, J., Rice, J. H., Chen, N., Baum, T. J., and Hewezi, T. (2014). Synchronization of developmental processes and defense signaling by growth regulating transcription factors. PLoS One 9:e98477. doi: 10.1371/journal.pone.0098477
Liu, X., Wang, J., and Sun, L. (2018). Structure of the hyperosmolality-gated calcium-permeable channel OSCA1.2. Nat. Commun. 9:5060. doi: 10.1038/s41467-018-07564-5
Lohse, M., Bolger, A. M., Nagel, A., Fernie, A. R., Lunn, J. E., Stitt, M., et al. (2012). RobiNA: a user-friendly, integrated software solution for RNA-Seq-based transcriptomics. Nucleic Acids Res. 40, W622–W627. doi: 10.1093/nar/gks540
Love, M. I., Huber, W., and Anders, S. (2014). Moderated estimation of fold change and dispersion for RNA-seq data with DESeq2. Genome Biol. 15:550. doi: 10.1186/s13059-014-0550-8
Mang, H., Feng, B., Hu, Z., Boisson-Dernier, A., Franck, C. M., Meng, X., et al. (2017). Differential regulation of two-tiered plant immunity and sexual reproduction by ANXUR receptor-like kinases. Plant Cell 29, 3140–3156. doi: 10.1105/tpc.17.00464
Mayfield, J. D., Folta, K. M., Paul, A.-L., and Ferl, R. J. (2007). The 14-3-3 proteins μ and υ influence transition to flowering and early phytochrome response. Plant Physiol. 145, 1692–1702. doi: 10.1104/pp.107.108654
Meng, J.-G., Liang, L., Jia, P.-F., Wang, Y.-C., Li, H.-J., and Yang, W.-C. (2020). Integration of ovular signals and exocytosis of a Ca2 + channel by MLOs in pollen tube guidance. Nat. Plants 6, 143–153. doi: 10.1038/s41477-020-0599-1
Merz, D., Richter, J., Gonneau, M., Sanchez-Rodriguez, C., Eder, T., Sormani, R., et al. (2017). T-DNA alleles of the receptor kinase THESEUS1 with opposing effects on cell wall integrity signaling. J. Exper. Bot. 68, 4583–4593. doi: 10.1093/jxb/erx263
Miao, Y., Laun, T., Zimmermann, P., and Zentgraf, U. (2004). Targets of the WRKY53 transcription factor and its role during leaf senescence in Arabidopsis. Plant Mol. Biol. 55, 853–867. doi: 10.1007/s11103-004-2142-6
Mineev, K. S., Bocharov, E. V., Pustovalova, Y. E., Bocharova, O. V., Chupin, V. V., and Arseniev, A. S. (2010). Spatial structure of the transmembrane domain heterodimer of ErbB1 and ErbB2 receptor tyrosine kinases. J. Mol. Biol. 400, 231–243. doi: 10.1016/j.jmb.2010.05.016
Mori, K., Renhu, N., Naito, M., Nakamura, A., Shiba, H., Yamamoto, T., et al. (2018). Ca2 + -permeable mechanosensitive channels MCA1 and MCA2 mediate cold-induced cytosolic Ca2 + increase and cold tolerance in Arabidopsis. Sci. Rep. 8:550. doi: 10.1038/s41598-017-17483-y
Moussu, S., Augustin, S., Roman, A. O., Broyart, C., and Santiago, J. (2018). Crystal structures of two tandem malectin-like receptor kinases involved in plant reproduction. Acta Crystallogr. 74(Pt 7), 671–680. doi: 10.1107/s205979831800774x
Omidbakhshfard, M. A., Fujikura, U., Olas, J. J., Xue, G.-P., Balazadeh, S., and Mueller-Roeber, B. (2018). GROWTH-REGULATING FACTOR 9 negatively regulates Arabidopsis leaf growth by controlling ORG3 and restricting cell proliferation in leaf primordia. PLoS Genet. 14:e1007484. doi: 10.1371/journal.pgen.1007484
Pagnussat, G. C., Yu, H.-J., Ngo, Q. A., Rajani, S., Mayalagu, S., Johnson, C. S., et al. (2005). Genetic and molecular identification of genes required for female gametophyte development and function in Arabidopsis. Development 132, 603–614. doi: 10.1242/dev.01595
Petersen, T. N., Brunak, S., von Heijne, G., and Nielsen, H. (2011). SignalP 4.0: discriminating signal peptides from transmembrane regions. Nat. Methods 8, 785–786. doi: 10.1038/nmeth.1701
Pinard, D., Mizrachi, E., Hefer, C. A., Kersting, A. R., Joubert, F., Douglas, C. J., et al. (2015). Comparative analysis of plant carbohydrate active enZymes and their role in xylogenesis. BMC Genom. 16:402. doi: 10.1186/s12864-015-1571-8
Prunet, N., Morel, P., Champelovier, P., Thierry, A.-M., Negrutiu, I., Jack, T., et al. (2015). SQUINT promotes stem cell homeostasis and floral meristem termination in Arabidopsis through APETALA2 and CLAVATA signalling. J. Exper. Bot. 66, 6905–6916. doi: 10.1093/jxb/erv394
R Core Team (2014). R: A Language and Environment for Statistical Computing. Vienna: R Foundation for Statistical Computing.
Rao, S., Zhou, Z., Miao, P., Bi, G., Hu, M., Wu, Y., et al. (2018). Roles of receptor-like cytoplasmic kinase VII members in pattern-triggered immune signaling. Plant Physiol. 177, 1679–1690. doi: 10.1104/pp.18.00486
Ratke, C., Terebieniec, B. K., Winestrand, S., Derba-Maceluch, M., Grahn, T., Schiffthaler, B., et al. (2018). Downregulating aspen xylan biosynthetic GT43 genes in developing wood stimulates growth via reprograming of the transcriptome. New Phytol. 219, 230–245. doi: 10.1111/nph.15160
Rubinovich, L., and Weiss, D. (2010). The Arabidopsis cysteine-rich protein GASA4 promotes GA responses and exhibits redox activity in bacteria and in planta. Plant J. 64, 1018–1027. doi: 10.1111/j.1365-313X.2010.04390.x
Rui, Y., and Dinneny, J. R. (2020). A wall with integrity: surveillance and maintenance of the plant cell wall under stress. New Phytol. 225, 1428–1439. doi: 10.1111/nph.16166
Schallus, T., Fehér, K., Sternberg, U., Rybin, V., and Muhle-Goll, C. (2010). Analysis of the specific interactions between the lectin domain of malectin and diglucosides. Glycobiology 20, 1010–1020. doi: 10.1093/glycob/cwq059
Schallus, T., Jaeckh, C., Fehér, K., Palma, A. S., Liu, Y., Simpson, J. C., et al. (2008). Malectin: a novel carbohydrate-binding protein of the endoplasmic reticulum and a candidate player in the early steps of protein N-glycosylation. Mol. Biol. Cell 19, 3404–3414. doi: 10.1091/mbc.e08-04-0354
Schaper, E., and Anisimova, M. (2015). The evolution and function of protein tandem repeats in plants. New Phytol. 206, 397–410. doi: 10.1111/nph.13184
Shannon, P., Markiel, A., Ozier, O., Baliga, N. S., Wang, J. T., Ramage, D., et al. (2003). Cytoscape: a software environment for integrated models of biomolecular interaction networks. Genome Res. 13, 2498–2504. doi: 10.1101/gr.1239303
Shiu, S.-H., and Bleecker, A. B. (2001). Receptor-like kinases from Arabidopsis form a monophyletic gene family related to animal receptor kinases. Proc. Natl. Acad. Sci. U.S.A. 98, 10763–10768. doi: 10.1073/pnas.181141598
Shiu, S.-H., and Bleecker, A. B. (2003). Expansion of the receptor-like kinase/Pelle gene family and receptor-like proteins in Arabidopsis. Plant Physiol. 132, 530–543. doi: 10.1104/pp.103.021964
Smith, M. R., Willmann, M. R., Wu, G., Berardini, T. Z., Möller, B., Weijers, D., et al. (2009). Cyclophilin 40 is required for microRNA activity in Arabidopsis. Proc. Natl. Acad. Sci. U.S.A. 106, 5424–5429. doi: 10.1073/pnas.0812729106
Song, H., Guo, Z., Chen, T., Sun, J., and Yang, G. (2018). Genome-wide identification of LRR-containing sequences and the response of these sequences to nematode infection in Arachis duranensis. BMC Plant Biol. 18:279. doi: 10.1186/s12870-018-1508-x
Stegmann, M., Monaghan, J., Smakowska-Luzan, E., Rovenich, H., Lehner, A., Holton, N., et al. (2017). The receptor kinase FER is a RALF-regulated scaffold controlling plant immune signaling. Science 355, 287–289. doi: 10.1126/science.aal2541
Sultana, M. M., Hachiya, T., Dutta, A. K., Nishimura, K., Suzuki, T., Tanaka, A., et al. (2020). Expression analysis of genes encoding malectin-like domain (MLD)- and leucine-rich repeat (LRR)- containing proteins in Arabidopsis thaliana. Biosci. Biotechnol. Biochem. 84, 154–158. doi: 10.1080/09168451.2019.1661769
Sundell, D., Mannapperuma, C., Netotea, S., Delhomme, N., Lin, Y.-C., Sjödin, A., et al. (2015). The plant genome integrative explorer resource: plantGenIE.org. New Phytol. 208, 1149–1156. doi: 10.1111/nph.13557
Sundell, D., Street, N. R., Kumar, M., Mellerowicz, E. J., Kucukoglu, M., Johnsson, C., et al. (2017). AspWood: high-spatial-resolution transcriptome profiles reveal uncharacterized modularity of wood formation in Populus tremula. Plant Cell 29, 1585–1604. doi: 10.1105/tpc.17.00153
Swarup, K., Benková, E., Swarup, R., Casimiro, I., Péret, B., Yang, Y., et al. (2008). The auxin influx carrier LAX3 promotes lateral root emergence. Nat. Cell Biol. 10, 946–954. doi: 10.1038/ncb1754
Thole, J. M., Vermeer, J. E. M., Zhang, Y., Gadella, T. W. J., and Nielsen, E. (2008). ROOT HAIR DEFECTIVE4 encodes a phosphatidylinositol-4-phosphate phosphatase required for proper root hair development in Arabidopsis thaliana. Plant Cell 20, 381–395. doi: 10.1105/tpc.107.054304
Voxeur, A., and Höfte, H. (2016). Cell wall integrity signaling in plants: “To grow or not to grow that’s the question”. Glycobiology 26, 950–960. doi: 10.1093/glycob/cww029
Wang, G., Ellendorff, U., Kemp, B., Mansfield, J. W., Forsyth, A., Mitchell, K., et al. (2008). A genome-wide functional investigation into the roles of receptor-like proteins in Arabidopsis. Plant Physiol. 147, 503–517. doi: 10.1104/pp.108.119487
Wang, J., Kucukoglu, M., Zhang, L., Chen, P., Decker, D., Nilsson, O., et al. (2013). The Arabidopsis LRR-RLK, PXC1, is a regulator of secondary wall formation correlated with the TDIF-PXY/TDR-WOX4 signaling pathway. BMC Plant Biol. 13:94. doi: 10.1186/1471-2229-13-94
Wang, Y., Salasini, B. C., Khan, M., Devi, B., Bush, M., Subramaniam, R., et al. (2019). Clade I TGACG-motif binding basic leucine zipper transcription factors mediate BLADE-ON-PETIOLE-dependent regulation of development. Plant Physiol. 180, 937–951. doi: 10.1104/pp.18.00805
Wang, J., Hu, T., Wang, W., Hu, H., Wei, Q., and Bao, C. (2019). Investigation of evolutionary and expressional relationships in the function of the leucine-rich repeat receptor-like protein kinase gene family (LRR-RLK) in the radish (Raphanus sativus L.). Sci. Rep. 9:6937. doi: 10.1038/s41598-019-43516-9
Waterhouse, A. M., Procter, J. B., Martin, D. M. A., Clamp, M., and Barton, G. J. (2009). Jalview Version 2 - a multiple sequence alignment editor and analysis workbench. Bioinformatics 25, 1189–1191. doi: 10.1093/bioinformatics/btp033
Wolf, S. (2017). Plant cell wall signalling and receptor-like kinases. Biochem. J. 474, 471–492. doi: 10.1042/bcj20160238
Wolf, S., and Höfte, H. (2014). Growth control: a saga of cell walls, ROS, and peptide receptors. Plant Cell 26, 1848–1856. doi: 10.1105/tpc.114.125518
Won, S.-K., Lee, Y.-J., Lee, H.-Y., Heo, Y.-K., Cho, M., and Cho, H.-T. (2009). cis-Element- and transcriptome-based screening of root hair-specific genes and their functional characterization in Arabidopsis. Plant Physiol. 150, 1459–1473. doi: 10.1104/pp.109.140905
Xi, J., Qiu, Y., Du, L., and Poovaiah, B. W. (2012). Plant-specific trihelix transcription factor AtGT2L interacts with calcium/calmodulin and responds to cold and salt stresses. Plant Sci. 185–186, 274–280. doi: 10.1016/j.plantsci.2011.11.013
Xi, L., Wu, X. N., Gilbert, M., and Schulze, W. X. (2019). Classification and interactions of LRR receptors and co-receptors within the Arabidopsis plasma membrane - an overview. Front. Plant Sci. 10:472. doi: 10.3389/fpls.2019.00472
Xiao, Y., Stegmann, M., Han, Z., DeFalco, T. A., Parys, K., Xu, L., et al. (2019). Mechanisms of RALF peptide perception by a heterotypic receptor complex. Nature 572, 270–274. doi: 10.1038/s41586-019-1409-7
Yanai, I., Benjamin, H., Shmoish, M., Chalifa-Caspi, V., Shklar, M., Ophir, R., et al. (2005). Genome-wide midrange transcription profiles reveal expression level relationships in human tissue specification. Bioinformatics 21, 650–659. doi: 10.1093/bioinformatics/bti042
Yang, Y., Labbé, J., Muchero, W., Yang, X., Jawdy, S. S., Kennedy, M., et al. (2016). Genome-wide analysis of lectin receptor-like kinases in Populus. BMC Genom. 17:699. doi: 10.1186/s12864-016-3026-2
Yeh, Y.-H., Panzeri, D., Kadota, Y., Huang, Y.-C., Huang, P.-Y., Tao, C.-N., et al. (2016). The Arabidopsis malectin-like/LRR-RLK IOS1 is critical for BAK1-dependent and BAK1-independent pattern-triggered immunity. Plant Cell 28, 1701–1721. doi: 10.1105/tpc.16.00313
Yuan, N., Yuan, S., Li, Z., Zhou, M., Wu, P., Hu, Q., et al. (2018). STRESS INDUCED FACTOR 2, a leucine-rich repeat kinase regulates basal plant pathogen defense. Plant Physiol. 176, 3062–3080. doi: 10.1104/pp.17.01266
Zan, Y., Ji, Y., Zhang, Y., Yang, S., Song, Y., and Wang, J. (2013). Genome-wide identification, characterization and expression analysis of Populus leucine-rich repeat receptor-like protein kinase genes. BMC Genom. 14:318. doi: 10.1186/1471-2164-14-318
Zhang, Q., Jia, M., Xing, Y., Qin, L., Li, B., and Jia, W. (2016). Genome-wide identification and expression analysis of MRLK family genes associated with strawberry (Fragaria vesca) fruit ripening and abiotic stress responses. PLoS One 11:e0163647. doi: 10.1371/journal.pone.0163647
Zhong, R., and Ye, Z.-H. (2012). MYB46 and MYB83 bind to the SMRE sites and directly activate a suite of transcription factors and secondary wall biosynthetic genes. Plant Cell Physiol. 53, 368–380. doi: 10.1093/pcp/pcr185
Zhou, J., Liu, D., Wang, P., Ma, X., Lin, W., Chen, S., et al. (2018). Regulation of Arabidopsis brassinosteroid receptor BRI1 endocytosis and degradation by plant U-box PUB12/PUB13-mediated ubiquitination. Proc. Natl. Acad. Sci. U.S.A. 115, E1906–E1915. doi: 10.1073/pnas.1712251115
Keywords: Populus, cell wall integrity, malectin domain, malectin-like domain, CBM57, receptor-like protein kinases, CrRLK1L
Citation: Kumar V, Donev EN, Barbut FR, Kushwah S, Mannapperuma C, Urbancsok J and Mellerowicz EJ (2020) Genome-Wide Identification of Populus Malectin/Malectin-Like Domain-Containing Proteins and Expression Analyses Reveal Novel Candidates for Signaling and Regulation of Wood Development. Front. Plant Sci. 11:588846. doi: 10.3389/fpls.2020.588846
Received: 11 August 2020; Accepted: 18 November 2020;
Published: 22 December 2020.
Edited by:
Seonghoe Jang, World Vegetable Center Korea, South KoreaReviewed by:
Hanyao Zhang, Southwest Forestry University, ChinaMelis Kucukoglu, University of Helsinki, Finland
Nathalie Leblanc-Fournier, Université Clermont Auvergne, France
Copyright © 2020 Kumar, Donev, Barbut, Kushwah, Mannapperuma, Urbancsok and Mellerowicz. This is an open-access article distributed under the terms of the Creative Commons Attribution License (CC BY). The use, distribution or reproduction in other forums is permitted, provided the original author(s) and the copyright owner(s) are credited and that the original publication in this journal is cited, in accordance with accepted academic practice. No use, distribution or reproduction is permitted which does not comply with these terms.
*Correspondence: János Urbancsok, amFub3MudXJiYW5jc29rQHNsdS5zZQ==