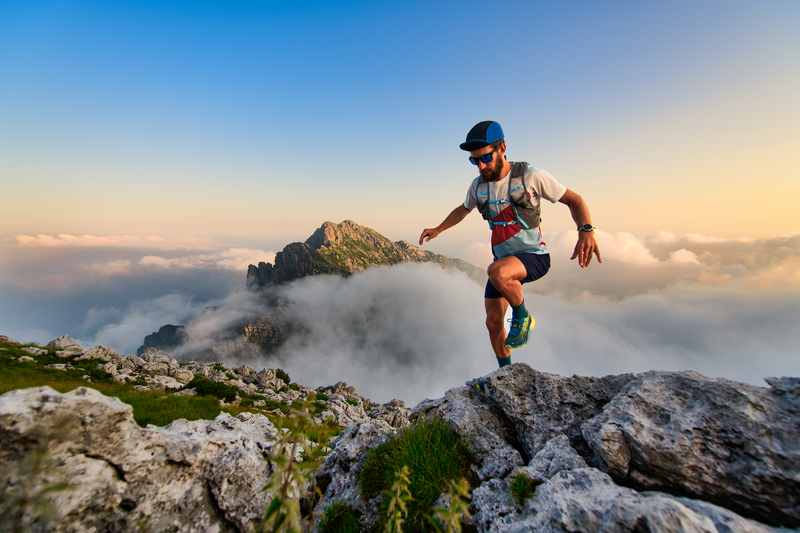
95% of researchers rate our articles as excellent or good
Learn more about the work of our research integrity team to safeguard the quality of each article we publish.
Find out more
REVIEW article
Front. Plant Sci. , 16 December 2020
Sec. Plant Systematics and Evolution
Volume 11 - 2020 | https://doi.org/10.3389/fpls.2020.574616
This article is part of the Research Topic The Evolutionary and Population Genomics of Plant Adaptation to Extreme Habitats View all 7 articles
Serpentine barrens are among the most challenging settings for plant life. Representing a perfect storm of hazards, serpentines consist of broadly skewed elemental profiles, including abundant toxic metals and low nutrient contents on drought-prone, patchily distributed substrates. Accordingly, plants that can tolerate the challenges of serpentine have fascinated biologists for decades, yielding important insights into adaptation to novel ecologies through physiological change. Here we highlight recent progress from studies which demonstrate the power of serpentine as a model for the genomics of adaptation. Given the moderate – but still tractable – complexity presented by the mix of hazards on serpentine, these venues are well-suited for the experimental inquiry of adaptation both in natural and manipulated conditions. Moreover, the island-like distribution of serpentines across landscapes provides abundant natural replicates, offering power to evolutionary genomic inference. Exciting recent insights into the genomic basis of serpentine adaptation point to a partly shared basis that involves sampling from common allele pools available from retained ancestral polymorphism or via gene flow. However, a lack of integrated studies deconstructing complex adaptations and linking candidate alleles with fitness consequences leaves room for much deeper exploration. Thus, we still seek the crucial direct link between the phenotypic effect of candidate alleles and their measured adaptive value – a prize that is exceedingly rare to achieve in any study of adaptation. We expect that closing this gap is not far off using the promising model systems described here.
Local adaptation optimizes fitness to the environment, often at the scale of meters. The resultant spatially varying selection leads to between-population genomic divergence that, depending on the intensity of gene flow, may maintain intraspecific adaptive diversity or lead to ecological speciation (Rundle and Nosil, 2005; Savolainen et al., 2013). In sessile plants, heterogeneous landscape mosaics, such as mountains or patchy soils, can trigger dramatic cases of local adaptation, especially in the presence of a steep gradient in the selective agent (Jain and Bradshaw, 1966). However, despite recent progress in the genomics of adaptation, there are still a few empirical inquiries into spatially varying selection (e.g., Hämälä et al., 2018; Hämälä and Savolainen, 2019) that provide empirical verification of the theory concerning adaptation under migration scenarios via finding correlation between fitness and environmental factors underlying local selection in natural populations (Yeaman and Whitlock, 2011). Only few studies assess the fine-scale genomic architecture of complex adaptive traits adequately (Holliday et al., 2016).
Serpentine barrens (Figure 1) represent powerful models to understand genome modification to local conditions because their extreme chemical and physical properties act as strong, quantifiable selective pressures. Derived from ultramafic rocks, serpentine soils are highly skewed in their content of many elements, being typically: (i) low in macronutrients such as Ca, K, N, and P, (ii) high in metals Co, Cr, and Ni, and (iii) greatly reduced in Ca relative to Mg. Worldwide, it is this highly skewed Ca/Mg ratio that defines serpentines (O’Dell and Rajakaruna, 2011), despite considerable diversity in other qualities. To add insult to injury, serpentine soils are typically very porous, with low water holding capacity and, due to their dark color, are frequently prone to substrate over-heating (Proctor and Woodell, 1975; Brady et al., 2005). These multifarious chemical and physical characteristics together have been termed “the serpentine syndrome” (Jenny, 1980), a state that results in very low ecosystem productivity with low competition and frequent endemism (Kruckeberg, 1954; Whittaker, 1954; Brady et al., 2005; Harrison and Rajakaruna, 2011). Moreover, the island-like distribution of serpentines provides abrupt edaphic contrasts that are replicated frequently across landscapes, triggering parallel adaptation (Roberts and Proctor, 1992). Such natural replicates can be leveraged to discern consistent trends in mechanisms and genetic bases of adaptation, as well as ecological speciation (Rundle and Nosil, 2005; Losos, 2011).
Figure 1. Extreme environment of serpentine barrens, illustrating sharp boundaries to adjacent land and low productivity (Pindos Mountains, Greece). Photos: F. Kolář.
The distinctive floristic composition of serpentines attracted botanists as early as the beginning of the twentieth century. What started as a general fascination with floristic peculiarities (Pančić, 1859; Lämmermayr, 1927; Novák, 1928; reviewed by Whittaker, 1954; Eggler, 1955) continued with experiments testing local adaptation (Kruckeberg, 1951, 1954) and targeted genetic investigations (Bradshaw, 2005; Bratteler et al., 2006). Contributions have emerged from a broad diversity of species, including Achillea, Cerastium, Collinsia, Gilia, Helianthus, Knautia, Mimulus, Silene, and Streptanthus (reviewed by Brady et al., 2005; O’Dell and Rajakaruna, 2011; Rajakaruna, 2018), significantly contributing to our understanding of the importance of local adaptation and ecotypic differentiation in plants.
As historical context is well summarized elsewhere (e.g., Brady et al., 2005; Anacker, 2014), we here focus on recent advances providing context for genomic studies. We first highlight advances in our understanding of serpentine adaptation at the phenotypic, physiological, and genomic levels. We discuss: (i) advances in knowledge of the selective factors imposed by serpentines, (ii) progress in experimental verification of local adaptation to serpentine soils, and (iii) plant responses to serpentines: the “phenotype” of serpentine adaptation. Finally, (iv) we summarize the first genomic studies that have very recently been built on previous insights and outline ways forward to integrate the study of serpentine adaptation.
Given the heterogeneity between various serpentine sites, in order to understand the mechanistic basis of serpentine adaptation it is first necessary to define the exact selective agents in play for any given case. While serpentine syndrome represents a complex set of selection pressures that vary from site to site, since the 1950’s there has been broad evidence that Ca availability plays a leading role (Vlamis and Jenny, 1948; Vlamis, 1949; Kruckeberg, 1954; Walker et al., 1955). More recently, the advent of high-throughput inductively coupled plasma mass spectrometry (ICP-MS; “ionomics” – Salt et al., 2008; Huang and Salt, 2016) has accelerated the characterization of that ‘hidden half’ of the plant environment: the underground soil matrix. Ionomics has allowed the rapid characterization of elemental accumulation in plant tissues in common garden experiments in diverse soil types from serpentines, to toxic mines, to saline soils (e.g., Arnold et al., 2016; Stein et al., 2017; Busoms et al., 2018; Preite et al., 2019). This and other recent advances in soil profiling have supported the salient role of distorted Ca/Mg ratio on serpentines (Palm and Van Volkenburgh, 2014), but has also identified other players, such as elevated heavy metal concentrations (Co, Cr, Ni, and/or Zn), for example in Arabidopsis lyrata (Veatch-Blohm et al., 2017) or Knautia serpentinicola (Čertner et al., 2018). These elevated metal levels are sometimes accompanied by lower concentrations of nutrients K, P, and S, as seen in Helianthus exilis (Sambatti and Rice, 2006), Cerastium alpinum (Berglund et al., 2004), and Arabidopsis arenosa (Arnold et al., 2016). Additional factors, such as drought, can interact with major chemical factors contributing to local adaptation to specific stresses, especially in drought-prone areas (e.g., Salehi Eskandari et al., 2017). The roles of biotic interactions, such as with bacteria, archaea, or mycorrhiza, are largely unknown with the little available evidence suggesting highly diverse effects (Mengoni et al., 2001; Pal and Paul, 2004; Davoodian et al., 2012; Doubková et al., 2012; reviewed by Schechter and Branco, 2014 and Southworth et al., 2014). For instance, higher diversity of arbuscular mycorrhizal fungi (AMF) was observed in serpentine populations of Collinsia sparsiflora compared to non-serpentine ones (Schechter and Bruns, 2008). Further, AMF more efficiently promoted growth and P uptake in serpentine K. serpentinicola (Doubková et al., 2012). On the other hand, the bacterial communities from serpentine and non-serpentine soils in Northern California were not different from each other (Oline, 2006).
To add to this complexity, there commonly exists fine-scale, site-specific differences in the serpentine-defining factors themselves. Indeed, Berglund et al. (2004) leveraged such variability among different serpentine sites occupied by C. alpinum to demonstrate that strength of tolerance to Mg and Ni was related particularly to effective concentrations of these elements in soil at each site. Similarly, the relative roles of other components depend on particular species – or even the site – studied. For example, variation in B, Ca, Fe, Na, and Zn is observed between serpentine barrens harboring Mimulus guttatus (Selby and Willis, 2018). In line with this, differences in physical properties also seem to be regionally specific rather than a universal property of all serpentines. For example, while drought and erosion characterize serpentines in drought-prone regions such as California or the Middle East (Kruckeberg, 1984; Salehi-Eskandari et al., 2018), they do not distinguish serpentine and non-serpentine sites of otherwise similar geomorphology in Central and Northern Europe (Novák, 1928; Rune, 1953), (Teptina et al., 2018), and tropical regions in South and Southeast Asia (Galey et al., 2017).
In summary, the study of serpentine adaptation requires an initial decision: one must choose whether to address serpentine adaptation holistically (including physical properties, biotic interactions, and site-specific soil chemistry) or to instead focus on a universally dominating parameter (such as altered Ca/Mg ratio or elevated Ni content).
A long tradition of reciprocal transplant experiments since the 1950’s (e.g., Kruckeberg, 1950, 1951, 1954, 1967) provided broad evidence of local adaptation to serpentine soils. These approaches have recently been expanded to diverse species, e.g., Helianthus exilis (Sambatti and Rice, 2006), Collinsia sparsiflora (Wright et al., 2006), Achillea millefolium (O’Dell and Claassen, 2006), Mimulus guttatus (reviewed by Selby et al., 2014; Selby and Willis, 2018), and Arabidopsis arenosa (Figure 2). The observed adaptive differences were compromised of a wide range of fitness proxies, from the extent of juvenile mortality in Mimulus, to higher biomass production in Achillea, and seed production in Helianthus.
Figure 2. Transplant experiment demonstrating parallel fitness response of two lineages of Arabidopsis arenosa to serpentine soil, depending on the substrate of origin. Two pairs of originally serpentine (S) and non-serpentine (N) populations representing different genetic lineages were cultivated in their native and foreign (native to the other member of the pair) soil, illustrative photo of representatives from all populations and treatments are depicted. Note the considerably smaller plants of non-serpentine origin when cultivated in serpentine soil but lack of such response for serpentine population in the non-serpentine soil suggesting the absence of the substrate-related trade-offs for serpentine plants. Photos by V. Konečná.
Taking our understanding of adaptive differences a step further, specific deconstruction of serpentine tolerance to individual elements has been performed in several contexts. Because serpentine chemical stress can be simply modeled, researchers have modulated the cardinal factors: Ca/Mg ratios and Ni levels in hydroponics and custom growth media (Proctor, 1971; Gabbrielli and Pandolfini, 1984; O’Dell and Rajakaruna, 2011). In Knautia arvensis and Cerastium alpinum, higher tolerance of serpentine populations to elevated Mg and Ni is evidenced by greater root growth (Berglund et al., 2004; Kolář et al., 2014). A specific effect of Ca/Mg ratio on both total biomass and photosynthetic rates was shown in M. guttatus (Palm et al., 2012). Furthermore, the effect of specific elements (Cr, Ni, and Ca/Mg ratio) on seed germination has been examined in Arabidopsis lyrata (Veatch-Blohm et al., 2013, 2017). There, while Ni caused a slower seedling growth, especially in non-serpentine accessions, there was no differential response to elevated Cr and Mg that could be related to fitness. Therefore, we can conclude that mechanisms reducing the Ni and/or Mg toxicity evolved in serpentine populations of many species; their phenotypes, such as length of the root, however, differ.
The origins of these phenotypes have been probed with genetic investigations of population history, which have documented striking manifold parallel colonizations of sites for a majority of the sufficiently sampled species or species groups (e.g., Alyssum serpyllifolium, Mengoni et al., 2003; Sobczyk et al., 2017; C. alpinum, Berglund et al., 2004; Lasthenia californica complex, Rajakaruna et al., 2003a; M. guttatus, Selby and Willis, 2018; Minuartia verna complex, Nunvářová Kabátová et al., 2019; Solidago virgaurea, Sakaguchi et al., 2017; Streptanthus glandulosus complex, Mayer and Soltis, 1994). In fact, single origins of serpentine populations are very rarely documented among species growing both on and off multiple serpentines (e.g., Picris hieracioides, Sakaguchi et al., 2018), and remains rather a property of genuine serpentine endemics (e.g., K. serpentinicola, Kolář et al., 2012, Halacsya sendtneri, Cecchi and Selvi, 2009). Unfortunately, only in a few cases have such genetic investigations been coupled with reciprocal transplants (Sakaguchi et al., 2017, 2019; Selby and Willis, 2018) or hydroponic experiments (Rajakaruna et al., 2003b,c; Berglund et al., 2004), ultimately demonstrating parallel substrate adaptation across multiple serpentine populations. Such rare cases provide particularly valuable naturally replicated model systems for further, finer-scale investigations of serpentine adaptation.
In other cases, serpentine adaptation is a constitutive trait present in all populations of a species regardless of their native soil chemistry. Such constitutive tolerance towards Ni has been reported for instance in Silene dioica (Westerbergh, 1994), Noccaea goesingense (Reeves and Baker, 1984), and Noccaea montana (Peer et al., 2006). Constitutive tolerance to both high Ni and low Ca/Mg ratios, at least as indicated by root growth, was documented for Galium valdepilosum, a likely “pre-adapted” species that colonized nearly all scattered serpentine outcrops throughout its overall species range (Kolář et al., 2014). Moreover, even plants that do not occur on serpentines but grow on dry and nutrient-poor habitats, such as granite outcrops, can tolerate extremely low Ca/Mg ratios, such as Phacelia dubia (Taylor and Levy, 2002). Overall, such cases of constitutive tolerance are only rarely documented (reviewed by O’Dell and Rajakaruna, 2011), potentially due to a publication bias towards “positive” results (i.e., where clear within-species local adaptation is evident).
Experimental studies have revealed a range of life-history and physiological mechanisms potentially underlying serpentine adaptation (Brady et al., 2005; Harrison and Rajakaruna, 2011; O’Dell and Rajakaruna, 2011). Drought stress adaptations include slower growth rate, reduced height, higher root/shoot biomass ratios, early flowering and specific modification of flowers (O’Dell and Rajakaruna, 2011; von Wettberg et al., 2014), and reduced leaf size and sclerophylly observed in some serpentine plants (Brady et al., 2005). In terms of chemistry, selective uptake of some micronutrients and macronutrients such as Ca and exclusion (or regulated accumulation and storage) of different metals are major adaptive mechanisms (Brady et al., 2005; Kazakou et al., 2008). Modulating the status of particular nutrients linked with monitoring uptake thus allows for valuable insight. For example, Kolář et al. (2014) compared serpentine populations of Knautia serpentinicola with non-serpentine populations of closely related Knautia arvensis in hydroponics with different concentrations of Ni and Mg. Interestingly, serpentine-origin plants accumulated less Ni when cultivated in a high Mg solution. On the contrary, non-serpentine plants accumulated approximately the same concentration of Ni regardless of the Mg concentration in media. Concordantly, in A. lyrata under Ni treatment, serpentine-origin plants had lower shoot and root Ni levels compared to non-serpentine plants (Veatch-Blohm et al., 2017).
Given that serpentine is a multi-hazard environment, it is only natural that serpentine adaptation results in physiological changes touching on a range of chemical challenges. A high-throughput approach to assessing this mixture is represented by performing common garden experiments incorporating a broad natural variation in a given species and to measure the relative accumulation of a panel of mineral nutrients. In this way, Arnold et al. (2016) assayed 20 elements in plants from 29 A. arenosa populations, after growing them in common conditions. This matrix was contrasted with data from source soil samples, providing a direct comparison of specific natural genetic variation across the ionome. Plants of serpentine origin accumulated the highest levels of K and S, excluded Ni, and exhibited the highest Ca/Mg ratios. These changes indicate a suite of specific, refined physiological adaptations in serpentine A. arenosa, which has a genetic basis. In this way, ionomics brings a key tool for understanding the actual “adaptive phenotype” distinguishing serpentine-adapted plants. Such approach, combined with common garden or transplant experiments, should become a standard practice in the characterization of the genetic basis of edaphic adaptation.
Adding to the complexity of multi-hazard adaptation, the rich experimental literature documents multiple solutions to identical environmental triggers (reviewed by Palm and Van Volkenburgh, 2014). For example, plants react to a skewed Ca/Mg ratio with a stunning diversity of mechanisms: either by selective translocation of Ca from roots to shoots (e.g., A. millefolium, O’Dell and Claassen, 2006), restriction of Mg uptake (e.g., Gilia capitata, Kruckeberg, 1951), or tolerance to higher concentrations of Mg in shoots (e.g., Streptanthus polygaloides, Boyd et al., 2009). Analogously, serpentine-adapted plants respond to elevated levels of heavy metals such as Ni in soil either by restricted uptake to shoots (Doubková et al., 2012; Kolář et al., 2014; Salehi Eskandari et al., 2017) or tolerance to high Ni levels in tissues (particularly in metal hyperaccumulators, e.g., Assunção et al., 2003; Galardi et al., 2007; Leigh Broadhurst et al., 2009).
While we have learned much about different adaptation mechanisms at a species level, considerably less is known about the variation in these traits at a population level within species. Experiments leveraging multiple cases of repeated within-species colonization of serpentine patches have demonstrated a broad variation in the strength of responses in a handful of studies (e.g., Berglund et al., 2004; Galardi et al., 2007; Veatch-Blohm et al., 2017). Together with variation in the soil chemistry across serpentine barrens, this suggests that there may be independent solutions even within a species, each fine-tuned to particular conditions at each site.
In summary, because serpentines produce clear challenges that can be experimentally dissected into specific factors (and moreover, which are replicated within species), decades of research have prepared a solid foundation for studies of their genomic basis.
Given the multi-challenge nature of serpentines and the diverse adaptive phenotypes generated in response, we may expect a highly complex, polygenic basis. Further, the variation in elemental soil composition between serpentine barrens suggests no single “basis” of serpentine adaptation. On the other hand, as compared to other multi-hazard “extreme” environments (coastal, alpine, or high-arctic sites), serpentines are fairly well-defined, dominated by the effect of few major elements. This, together with strong selective pressures, makes discovery of major-effect candidates seem likely, similar to what has been found for other soils such as metal contaminated and saline sites (e.g., in Arabidopsis halleri, Courbot et al., 2007; Willems et al., 2007; in Noccaea caerulescens, Deniau et al., 2006; and in Mimulus guttatus, Wright et al., 2013). In addition, theory suggests that specific aspects of serpentine colonization – such as abrupt fitness differences and patchily-distributed habitats – favor the emergence of large-effect alleles (Dittmar et al., 2016; Gilbert and Whitlock, 2017). Theory also suggests that adaptation in the face of gene flow, such as that from nearby non-serpentine sites, may promote fixation of smaller numbers of large-effect loci (Yeaman and Whitlock, 2011).
In line with this, the few quantitative trait locus (QTL) studies applied to serpentine ecotypes so far have provided evidence for a simple genetic architecture of single traits (e.g., Ni tolerance in Silene vulgaris, Bratteler et al., 2006 and Caulanthus amplexicaulis, Burrell et al., 2012). Additionally, studies focused on particular genes known to have an ion homeostasis effect show strong natural differentiation in its sequence variation and/or in associated phenotypic responses (e.g., A. serpyllifolium, Sobczyk et al., 2017; Arabidopsis thaliana, Bradshaw, 2005; Agrawal et al., 2012). While such hypothesis-driven studies bring valuable insights into the basis of a particular gene or trait, they capture neither genetic architecture nor the complexity of the full serpentine syndrome. Accordingly, neither classical QTL studies nor candidate gene-targeted inquiries can alone provide a picture of the broad genomic remodeling which we speculate is required for robust establishment in such a multi-hazard environment.
In contrast to QTL studies, high-density genomic divergence scans detect signatures of directional selection in a purely natural system from a holistic perspective, both in terms of genes (the entire genome) and parameters screened (the entire serpentine syndrome in nature: both known and unknown factors). In other words, genome scans can be both genetically and phenotypically agnostic. Such scans have been performed in two wild, outcrossing Arabidopsis species – A. arenosa (Arnold et al., 2016) and A. lyrata (Turner et al., 2008, 2010). Both indicate a highly polygenic basis of serpentine adaptation. The study by Turner et al. (2010), notably one of the first truly genome-scale scans for selection used sequencing of pooled samples to reveal outlier differentiated SNPs at loci involved in ion transmembrane transport, metal tolerance, and calcium ion binding. Clearer detection of candidate loci was achieved by individual-level genome resequencing by Arnold et al. (2016), discriminating approximately 160 genes exhibiting multiple signatures of selection in a serpentine-adapted population of A. arenosa. These included genes related to Ca signaling, ion homeostasis, metal transport, root macronutrient transport, and dehydration tolerance. These works provided a broad view on the genetic basis of serpentine adaptation and have served as hypothesis generators that can now guide functional assessment of particular alleles in natural conditions.
A complementary study in M. guttatus innovatively combined field and genomic approaches by assessing survival differences of F2 mapping populations grown on serpentines by bulk segregant analysis (Selby and Willis, 2018). This enabled the identification of a major QTL (containing several 100 candidate genes) that contributed to the survival of plants by 33%. However, because of the limited size of the survivor pool, the study was underpowered to detect smaller effect loci. Indeed, mapping approaches commonly fail to identify small effect loci and tend to overestimate the influence of large effect loci due to the linkage (Rockman, 2012). Nevertheless, this study was an important step towards linking genomic variation and fitness consequences and demonstrates that the Mimulus system has a strong potential to reveal more refined results in the future (Selby et al., 2014).
Ecological speciation can occur when strong divergent selection is associated with the rise of reproductive incompatibilities between ecologically distinct populations (Nosil et al., 2009). Such reproductive incompatibilities may emerge in association with serpentine either as a direct consequence of selection against maladaptive gene flow (e.g., reinforcement to avoid hybrids that are unfit in either environment) or as a by-product of local adaptation, e.g., through physical linkage (as observed in copper-tolerant Mimulus, Rajakaruna and Whitton, 2004; Wright et al., 2013) or shifts in flowering time (found in serpentine Solidago, Sakaguchi et al., 2018, 2019). Indeed, serpentine barrens provide intriguing candidate cases of incipient – and even parallel – ecological speciation (e.g., Rajakaruna and Whitton, 2004; Moyle et al., 2012; reviewed by Ostevik et al., 2012). The genomic basis of these incompatibilities remains unknown. Aeschbacher et al. (2017) used coalescent modeling on pooled sequence data to detect genome-wide signals of selection against maladaptive gene flow from non-serpentine M. guttatus to serpentine populations. Whether such selection promotes accumulation of reproductive barriers through reinforcement or is directly linked with between ecotype-specific reproductive incompatibilities is unknown. However, given experimental evidence for the accumulation of reproductive incompatibilities associated with colonization of serpentines across plant taxa (reviewed by Rajakaruna and Whitton, 2004; Rajakaruna, 2018) the genomics of ecological speciation in serpentine plants promises to be a fruitful area for future research.
The patchy, island-like distribution of serpentines provides convenient natural replicates to study repeated evolution both within species (parallelism) and between them (convergence). Given the many independent evolutionary paths that species (and indeed populations within species) have taken to serpentine-adaptive phenotypes, it is reasonable to expect reuse of common pathways, or even proximal molecular actors to the common serpentine challenges.
While phenotypic parallelism and convergence are obvious from a rich literature, only recently has genetic parallelism and convergence been tested. Genetic parallelism has been indicated in M. guttatus, where two serpentine populations share a major QTL important for serpentine adaptation (Selby and Willis, 2018). Some indication of genetic parallelism has also been provided by Turner et al. (2010) in European and North American serpentine populations of A. lyrata, which shared the same non-synonymous mutation that codes for a change in the TPC1 locus encoding Ca-ion channel. This is almost fixed in three serpentine populations in two continents. Genetic convergence was described in A. arenosa and A. lyrata by Arnold et al. (2016) in nine gene coding loci with serpentine-relevant predicted functions, such as Ca, K, and Ni homeostasis (Table 1). Importantly, studies of evolutionary history and fitness responses of several other plant systems, such as C. alpinum (Scandinavia – Berglund et al., 2004), L. californica complex (California – Rajakaruna et al., 2003a,b,c), and Solidago virgaurea (Japan – Sakaguchi et al., 2017, 2019), suggest parallel evolution of serpentine ecotypes, which may be a frequent phenomenon (see also Figure 2). Further leveraging such truly non-model systems for genomic studies should bring novel vital insights into the basis of local adaptation.
Table 1. Candidate convergent loci mediating serpentine adaptation in Alyssum serpyllifolium, Arabidopsis arenosa, Arabidopsis lyrata, and Mimulus guttatus.
An intriguing open question concerns the origin of the variants that repeatedly exhibit signatures of selection in different serpentine populations. Such repeated signatures may arise in three major ways (Stern, 2013; Lee and Coop, 2019): (i) the causal variants might have been repeatedly sampled from the standing variation present in the ancestral population; (ii) they may have been transferred between adapted populations by gene flow; or (iii) they may have arisen by independent de novo mutations. That adaptive gene flow may be involved in serpentine adaptation has been indicated in A. arenosa and A. lyrata despite overall weak genome-wide signal for introgression between these two species (Arnold et al., 2016). Yet, the relative contribution of these pathways to repeated adaptation remains unknown, particularly in closely related populations and thus represents a fruitful area for future research.
Given the perfect storm of challenges that serpentine presents, a comprehensive understanding of serpentine adaptation is a challenging task that requires multidisciplinary investigation (Wright and Von Wettberg, 2009). However, recent progress in quantitative genetic, ionomic, and population genomic study has shown that understanding the basis for the interacting phenotypes which constitute serpentine adaptation is well worth the effort. Specifically, recent inquiries in the Arabidopsis and Mimulus genera have provided proof-of-concept that the genetic basis of serpentine adaptation is accessible, and the time is now ripe for synthetic studies (Figure 3). Here, we sketched gaps in our understanding of serpentine adaptation and considered integrative approaches to link candidate adaptive alleles with fitness effects as a promising avenue to make progress.
Figure 3. Approaches to study the basis of serpentine adaptation: Integration of holistic (orange) and one factor/hypothesis driven approaches (blue) to investigate links between genotype, phenotype, fitness, and serpentine environments. The right side of the triangle depicts ionomic analysis of Arabidopsis arenosa (Arnold et al., 2016), the bottom side is accompanied by the scheme of bulk segregant analysis applied in the recent study of Mimulus guttatus (Selby and Willis, 2018), and the left side is supplemented with allele frequency difference graph (AFD) of S and N population for TPC1 locus Arnold et al. (2016). Line thickness scales with the quantity of empirical studies available. S – serpentine adapted plant, N – non-serpentine plant. The scheme is inspired by Sork et al. (2013) and Rellstab et al. (2015). Illustrations by V. Konečná.
We suggest that ongoing ecological and population genomic studies of serpentine adaptation hold strong potential to contribute to our understanding of fundamental evolutionary principles, similar to the contributions of early eco-evolutionary studies achieved in the 1950s and 1960s (Kruckeberg, 1950, 1951, 1954, 1967). Aside from the obvious benefit of improved knowledge about soil-stress adaptations for rational crop breeding, serpentine models can efficiently address our understanding of environmentally driven adaptation and ecological speciation. Specifically, serpentine soils represent a strong selective pressure leading to well-tractable adaptive phenotypes. Yet, in contrast to similarly poised mine sites, serpentines provide fully natural setups with longer time frames on which selection has acted. Frequent reports of serpentine endemism and the reproductive isolation experienced by edaphic ecotypes also suggest serpentine plants as attractive, yet still too rarely leveraged models for ecological speciation. Beyond this, serpentine is a highly defined environment characterized primarily by a limited number of soil chemistry components (also with potentially linked biotic interactions). Such “intermediate complexity” makes serpentine an attractive model of adaptation towards a suite of naturally relevant factors, which can still be experimentally manipulated using, e.g., reciprocal transplants. Finally, the repeated origins of serpentine adaptation allow leveraging natural replicates empowering genomic and experimental inference. Genome-wide studies of serpentine populations combined with ecological genomic experiments and/or forward genetic validation may thus bring significant contribution to our general knowledge on the adaptation mechanisms towards environmental challenges.
All authors listed have made a substantial, direct and intellectual contribution to the work and approved it for publication.
The study was supported by the Charles University, project GA UK No. 410120 to VK and the European Research Council (ERC) under the European Union’s Horizon 2020 Research and Innovation Programme (grant number ERC-StG 679056 HOTSPOT), via a grant to LY. Additional support was provided by Charles University (Primus/SCI/35 to FK) and by long-term research development project No. RVO 67985939 of the Czech Academy of Sciences.
The authors declare that the research was conducted in the absence of any commercial or financial relationships that could be construed as a potential conflict of interest.
Aeschbacher, S., Selby, J. P., Willis, J. H., and Coop, G. (2017). Population-genomic inference of the strength and timing of selection against gene flow. Proc. Natl. Acad. Sci. U. S. A. 114, 7061–7066. doi: 10.1073/pnas.1616755114
Agrawal, B., Lakshmanan, V., Kaushik, S., and Bais, H. P. (2012). Natural variation among Arabidopsis accessions reveals malic acid as a key mediator of nickel (Ni) tolerance. Planta 236, 477–489. doi: 10.1007/s00425-012-1621-2
Anacker, B. L. (2014). The nature of serpentine endemism. Am. J. Bot. 101, 219–224. doi: 10.3732/ajb.1300349
Arnold, B. J., Lahner, B., DaCosta, J. M., Weisman, C. M., Hollister, J. D., Salt, D. E., et al. (2016). Borrowed alleles and convergence in serpentine adaptation. Proc. Natl. Acad. Sci. U. S. A. 113, 8320–8325. doi: 10.1073/pnas.1600405113
Assunção, A. G. L., Bookum, W. M., Nelissen, H. J. M., Vooijs, R., Schat, H., and Ernst, W. H. O. (2003). Differential metal-specific tolerance and accumulation patterns among Thlaspi caerulescens populations originating from different soil types. New Phytol. 159, 411–419. doi: 10.1046/j.1469-8137.2003.00819.x
Berglund, A. N., Dahlgren, S., and Westerbergh, A. (2004). Evidence for parallel evolution and site-specific selection of serpentine tolerance in Cerastium alpinum during the colonization of Scandinavia. New Phytol. 161, 199–209. doi: 10.1046/j.1469-8137.2003.00934.x
Boyd, R. S., Wall, M. A., Santos, S. R., and Davis, M. A. (2009). Variation of morphology and elemental concentrations in the California nickel hyperaccumulator Streptanthus polygaloides (Brassicaceae). Northeast. Nat. 16, 21–38. doi: 10.1656/045.016.0503
Bradshaw, H. D. (2005). Mutations in CAX1 produce phenotypes characteristic of plants tolerant to serpentine soils. New Phytol. 167, 81–88. doi: 10.1111/j.1469-8137.2005.01408.x
Brady, K. U., Kruckeberg, A. R., and Bradshaw Jr., H. D. (2005). Evolutionary ecology of plant adaptation to serpentine soils. Annu. Rev. Ecol. Evol. Syst. 36, 243–266. doi: 10.1146/annurev.ecolsys.35.021103.105730
Bratteler, M., Baltisberger, M., and Widmer, A. (2006). QTL analysis of intraspecific differences between two Silene vulgaris ecotypes. Ann. Bot. 98, 411–419. doi: 10.1093/aob/mcl113
Burrell, M. A., Hawkins, A. K., and Pepper, A. E. (2012). Genetic analyses of nickel tolerance in a north American serpentine endemic plant, Caulanthus amplexicaulis var. barbarae (Brassicaceae). Am. J. Bot. 99, 1875–1883. doi: 10.3732/ajb.1200382
Busoms, S., Paajanen, P., Marburger, S., Bray, S., Huang, X. Y., Poschenrieder, C., et al. (2018). Fluctuating selection on migrant adaptive sodium transporter alleles in coastal Arabidopsis thaliana. Proc. Natl. Acad. Sci. U. S. A. 115, E12443–E12452. doi: 10.1073/pnas.1816964115
Cecchi, L., and Selvi, F. (2009). Phylogenetic relationships of the monotypic genera Halacsya and Paramoltkia and the origins of serpentine adaptation in circummediterranean Lithospermeae (Boraginaceae): insights from ITS and matK DNA sequences. Taxon 58, 700–714. doi: 10.1002/tax.583002
Čertner, M., Sudová, R., Weiser, M., Suda, J., and Kolář, F. (2018). Ploidy-altered phenotype interacts with local environment and may enhance polyploid establishment in Knautia serpentinicola (Caprifoliaceae). New Phytol. 221, 1117–1127. doi: 10.1111/nph.15426
Courbot, M., Willems, G., Motte, P., Arvidsson, S., Roosens, N., Saumitou-laprade, P., et al. (2007). A major quantitative trait locus for cadmium tolerance in Arabidopsis halleri colocalizes with HMA4, a gene encoding a heavy metal ATPase 1 [OA]. Plant Physiol. 144, 1052–1065. doi: 10.1104/pp.106.095133
Davoodian, N., Bosworth, J., and Rajakaruna, N. (2012). Mycorrhizal colonization of Hypericum perforatum L. (Hypericaceae) from serpentine and granite outcrops on the deer isles, Maine. Northeast. Nat. 19, 517–526. doi: 10.1656/045.019.0312
Deniau, A. X., Pieper, B., Ten Bookum, W. M., Lindhout, P., Aarts, M. G. M., and Schat, H. (2006). QTL analysis of cadmium and zinc accumulation in the heavy metal hyperaccumulator Thlaspi caerulescens. Theor. Appl. Genet. 113, 907–920. doi: 10.1007/s00122-006-0350-y
Dittmar, E. L., Oakley, C. G., Conner, J. K., Gould, B. A., and Schemske, D. W. (2016). Factors influencing the effect size distribution of adaptive substitutions. Proc. R. Soc. B Biol. Sci. 283:20153065. doi: 10.1098/rspb.2015.3065
Doubková, P., Suda, J., and Sudová, R. (2012). The symbiosis with arbuscular mycorrhizal fungi contributes to plant tolerance to serpentine edaphic stress. Soil Biol. Biochem. 44, 56–64. doi: 10.1016/j.soilbio.2011.09.011
Eggler, J. (1955). Ein Beitrag zur Serpentinvegetation in der Gulsen bei Kraubath in Obersteiermark. Mitt Naturw Ver Steiermark 85, 27–72.
Gabbrielli, R., and Pandolfini, T. (1984). Effect of Mg2+ and Ca2+ on the response to nickel toxicity in a serpentine endemic and nickel-accumulating species. Physiol. Plant. 62, 540–544. doi: 10.1111/j.1399-3054.1984.tb02796.x
Galardi, F., Corrales, I., Mengoni, A., Pucci, S., Barletti, L., Barzanti, R., et al. (2007). Intra-specific differences in nickel tolerance and accumulation in the Ni-hyperaccumulator Alyssum bertolonii. Environ. Exp. Bot. 60, 377–384. doi: 10.1016/j.envexpbot.2006.12.011
Galey, M. L., van der Ent, A., Iqbal, M. C. M., and Rajakaruna, N. (2017). Ultramafic geoecology of south and Southeast Asia. Bot. Stud. 58:18. doi: 10.1186/s40529-017-0167-9
Gilbert, K. J., and Whitlock, M. C. (2017). The genetics of adaptation to discrete heterogeneous environments: frequent mutation or large-effect alleles can allow range expansion. J. Evol. Biol. 30, 591–602. doi: 10.1111/jeb.13029
Hämälä, T., Mattila, T. M., and Savolainen, O. (2018). Local adaptation and ecological differentiation under selection, migration, and drift in Arabidopsis lyrata. Evolution 72, 1373–1386. doi: 10.1111/evo.13502
Hämälä, T., and Savolainen, O. (2019). Genomic patterns of local adaptation under gene flow in Arabidopsis lyrata. Mol. Biol. Evol. 36, 2557–2571. doi: 10.1093/molbev/msz149
Harrison, S., and Rajakaruna, N. (2011). Serpentine: The evolution and ecology of a model system. University of California Press.
Holliday, J. A., Zhou, L., Bawa, R., Zhang, M., and Oubida, R. W. (2016). Evidence for extensive parallelism but divergent genomic architecture of adaptation along altitudinal and latitudinal gradients in Populus trichocarpa. New Phytol. 209, 1240–1251. doi: 10.1111/nph.13643
Huang, X. Y., and Salt, D. E. E. (2016). Plant ionomics: from elemental profiling to environmental adaptation. Mol. Plant 9, 787–797. doi: 10.1016/j.molp.2016.05.003
Jain, S. K., and Bradshaw, A. D. (1966). Evolutionary divergence among adjacent plant populations I. the evidence and its theoretical analysis. Heredity 21, 407–441. doi: 10.1038/hdy.1966.42
Kazakou, E., Dimitrakopoulos, P. G., Baker, A. J. M., Reeves, R. D., and Troumbis, A. Y. (2008). Hypotheses, mechanisms and trade-offs of tolerance and adaptation to serpentine soils: from species to ecosystem level. Biol. Rev. Camb. Philos. Soc. 83, 495–508. doi: 10.1111/j.1469-185X.2008.00051.x
Kolář, F., Dortová, M., Lepš, J., Pouzar, M., and Krejčová, A. (2014). Serpentine ecotypic differentiation in a polyploid plant complex: shared tolerance to Mg and Ni stress among di‐ and tetraploid serpentine populations of. Plant Soil 374, 435–447. doi: 10.1007/s11104-013-1813-y
Kolář, F., Fér, T., Štech, M., Trávníček, P., Dušková, E., Schönswetter, P., et al. (2012). Bringing together evolution on serpentine and polyploidy: spatiotemporal history of the diploid-tetraploid complex of Knautia arvensis (Dipsacaceae). PLoS One 7:e39988. doi: 10.1371/journal.pone.0039988
Kruckeberg, A. R. (1950). An experimental inquiry into the nature of endemism on serpentine soils. Berkeley: University of California.
Kruckeberg, A. R. (1951). Intraspecific variability in the response of certain native plant species to serpentine soil. Am. J. Bot. 38, 408–419. doi: 10.1002/j.1537-2197.1951.tb14842.x
Kruckeberg, A. R. (1954). The ecology of serpentine soils: a symposium. III. Plant species in relation to serpentine soils. Ecology 35, 267–274.
Kruckeberg, A. R. (1967). Ecotypic response to ultramafic soils by some plant species of northwestern United States. Brittonia 19, 133–151. doi: 10.2307/2805271
Kruckeberg, A. R. (1984). California serpentines: Flora, vegetation, geology, soils and management problems. University of California Press.
Lämmermayr, L. (1927). Materialien zur Systematik und Ökologie der Serpentinflora. II. Das Problem der, Serpentinpflanzen “–Eine kritische ökologische Studie, Sitzungsber. Akad. Wiss. Wien, math.-naturw. Kl., Abt., 25–69.
Lee, K. M., and Coop, G. (2019). Population genomics perspectives on convergent adaptation. Philos. Trans. R Soc. Lond. B Biol. Sci. 374, 1–19. doi: 10.1098/rstb.2018.0236
Leigh Broadhurst, C., Tappero, R. V., Maugel, T. K., Erbe, E. F., Sparks, D. L., and Chaney, R. L. (2009). Interaction of nickel and manganese in accumulation and localization in leaves of the Ni hyperaccumulators Alyssum murale and Alyssum corsicum. Plant Soil 314, 35–48. doi: 10.1007/s11104-008-9703-4
Losos, J. B. (2011). Convergence, adaptation, and constraint. Evolution 65, 1827–1840. doi: 10.1111/j.1558-5646.2011.01289.x
Mayer, M., and Soltis, P. S. (1994). The evolution of serpentine endemics : a chloroplast DNA phylogeny of the Streptanthus glandulosus complex (Cruciferae). Syst. Bot. 19, 557–574. doi: 10.2307/2419777
Mengoni, A., Baker, A. J. M., Bazzicalupo, M., Reeves, R. D., Adigüzel, N., Chianni, E., et al. (2003). Evolutionary dynamics of nickel hyperaccumulation in alyssum revealed by ITS nrDNA analysis. New Phytol. 159, 691–699. doi: 10.1046/j.1469-8137.2003.00837.x
Mengoni, A., Barzanti, R., Gonnelli, C., Gabbrielli, R., and Bazzicalupo, M. (2001). Characterization of nickel-resistant bacteria isolated from serpentine soil. Environ. Microbiol. 3, 691–698. doi: 10.1046/j.1462-2920.2001.00243.x
Moyle, L. C., Levine, M., Stanton, M. L., and Wright, J. W. (2012). Hybrid sterility over tens of meters between ecotypes adapted to serpentine and non-serpentine soils. Evol. Biol. 39, 207–218. doi: 10.1007/s11692-012-9180-9
Nosil, P., Funk, D. J., and Ortiz-Barrientos, D. (2009). Divergent selection and heterogeneous genomic divergence. Mol. Ecol. 18, 375–402. doi: 10.1111/j.1365-294X.2008.03946.x
Nunvářová Kabátová, K., Kolář, F., Jarolímová, V., Krak, K., and Chrtek, J. (2019). Does geography, evolutionary history or ecology drive ploidy and genome size variation in the Minuartia verna group (Caryophyllaceae) across Europe? Plant Syst. Evol. 305, 1019–1040. doi: 10.1007/s00606-019-01621-2
O’Dell, R. E., and Claassen, V. P. (2006). Serpentine and nonserpentine Achillea millefolium accessions differ in serpentine substrate tolerance and response to organic and inorganic amendments. Plant Soil 279, 253–269. doi: 10.1007/s11104-005-2360-y
O’Dell, R. E., and Rajakaruna, N. (2011). “Intraspecific variation, adaptation, and evolution” in Serpentine: Evolution and ecology in a model system. eds. S. Harrison and N. Rajakaruna (University of California Press), 97–137.
Oline, D. K. (2006). Phylogenetic comparisons of bacterial communities from serpentine and nonserpentine soils. Appl. Environ. Microbiol. 72, 6965–6971. doi: 10.1128/AEM.00690-06
Ostevik, K. L., Moyers, B. T., Owens, G. L., and Rieseberg, L. H. (2012). Parallel ecological speciation in plants? Int. J. Ecol. 2012, 1–17. doi: 10.1155/2012/939862
Pal, A., and Paul, A. K. (2004). Aerobic chromate reduction by chromium-resistant bacteria isolated from serpentine soil. Microbiol. Res. 159, 347–354. doi: 10.1016/j.micres.2004.08.001
Palm, E., Brady, K., and Van Volkenburgh, E. (2012). Serpentine tolerance in Mimulus guttatus does not rely on exclusion of magnesium. Funct. Plant Biol. 39, 679–688. doi: 10.1071/FP12059
Palm, E. R., and Van Volkenburgh, E. (2014). “Physiological adaptation of plants to serpentine soil” in Plant ecology and evolution in harsh environments. eds. N. Rajakaruna, R. S. Boyd, and T. B. Harris (New York: Nova Science Publishers), 129–148.
Pančić, J. (1859). Die Flora der Serpentinberge in Mittel-Serbien. Verhandl. Zool.-Bot. Gesell. Wien 9, 139–150.
Peer, W. A., Mahmoudian, M., Freeman, J. L., Lahner, B., Richards, E. L., Reeves, R. D., et al. (2006). Assessment of plants from the Brassicaceae family as genetic models for the study of nickel and zinc hyperaccumulation. New Phytol. 172, 248–260. doi: 10.1111/j.1469-8137.2006.01820.x
Preite, V., Sailer, C., Syllwasschy, L., Bray, S., Kraemer, U., and Yant, L. (2019). Convergent evolution in Arabidopsis halleri and Arabidopsis arenosa on calamine metalliferous soils. Philos. Trans. R. Soc. B 374:20180243. doi: 10.1098/rstb.2018.0243
Proctor, J., and Woodell, S. R. (1975). The ecology of serpentine soils. Adv. Ecol. Res. 9, 255–366.
Rajakaruna, N. (2018). Lessons on evolution from the study of edaphic specialization. Bot. Rev. 84, 39–78. doi: 10.1007/s12229-017-9193-2
Rajakaruna, N., Baldwin, B. G., Chan, R., Desrochers, A. M., Bohm, B. A., and Whitton, J. (2003a). Edaphic races and phylogenetic taxa in the Lasthenia californica complex (Asteraceae: Heliantheae): an hypothesis of parallel evolution. Mol. Ecol. 12, 1675–1679. doi: 10.1046/j.1365-294x.2003.01843.x
Rajakaruna, N., Bradfield, G. E., Bohm, B. A., and Whitton, J. (2003b). Adaptive differentiation in response to water stress by edaphic races of Lasthenia californica (Asteraceae). Int. J. Plant Sci. 164, 371–376. doi: 10.1086/368395
Rajakaruna, N., Siddiqi, M. Y., Whitton, J., Bohm, B. A., and Glass, A. D. M. (2003c). Differential responses to Na+/K+ and Ca2+/Mg2+ in two edaphic races of the Lasthenia californica (Asteraceae) complex: a case for parallel evolution of physiological traits. New Phytol. 157, 93–103. doi: 10.1046/j.1469-8137.2003.00648.x
Rajakaruna, N., and Whitton, J. (2004). “Trends in the evolution of edaphic specialists with an example of parallel evolution in the Lasthenia californica complex” in Plant adaptation: Molecular genetics and ecology. NRC Research Press, 103–110.
Reeves, R. D., and Baker, A. J. M. (1984). Studies on metal uptake by plants from serpentine and non-serpentine populations of Thlaspi goesingense Hálácsy (Crycuferae). New Phytol. 98, 191–204. doi: 10.1111/j.1469-8137.1984.tb06108.x
Rellstab, C., Gugerli, F., Eckert, A. J., Hancock, A. M., and Holderegger, R. (2015). A practical guide to environmental association analysis in landscape genomics. Mol. Ecol. 24, 4348–4370. doi: 10.1111/mec.13322
Roberts, B. A., and Proctor, J. (1992). The ecology of areas with Serpentinized rocks. A World View. Dordrecht: Kluwer Academic Press.
Rockman, M. V. (2012). The QTN program and the alleles that matter for evolution: all that’s gold does not glitter. Evolution 66, 1–17. doi: 10.1111/j.1558-5646.2011.01486.x
Rundle, H. D., and Nosil, P. (2005). Ecological speciation. Ecol. Lett. 8, 336–352. doi: 10.1111/j.1461-0248.2004.00715.x
Rune, O. (1953). Plant life on serpentines and related rocks in the north of Sweden. Sv. växtgeografiska sällsk.
Sakaguchi, S., Horie, K., Ishikawa, N., Nagano, A. J., Yasugi, M., Kudoh, H., et al. (2017). Simultaneous evaluation of the effects of geographic, environmental and temporal isolation in ecotypic populations of Solidago virgaurea. New Phytol. 216, 1268–1280. doi: 10.1111/nph.14744
Sakaguchi, S., Horie, K., Ishikawa, N., Nishio, S., Worth, J. R. P., Fukushima, K., et al. (2019). Maintenance of soil ecotypes of Solidago virgaurea in close parapatry via divergent flowering time and selection against immigrants. J. Ecol. 107, 418–435. doi: 10.1111/1365-2745.13034
Sakaguchi, S., Horie, K., Kimura, T., Nagano, A. J., Isagi, Y., and Ito, M. (2018). Phylogeographic testing of alternative histories of single-origin versus parallel evolution of early flowering serpentine populations of Picris hieracioides L. (Asteraceae) in Japan. Ecol. Res. 33, 537–547. doi: 10.1007/s11284-017-1536-2
Salehi Eskandari, B., Ghaderian, S. M., and Schat, H. (2017). The role of nickel (Ni) and drought in serpentine adaptation: contrasting effects of Ni on osmoprotectants and oxidative stress markers in the serpentine endemic, Cleome heratensis, and the related non-serpentinophyte, Cleome foliolosa. Plant Soil 417, 183–195. doi: 10.1007/s11104-017-3250-9
Salehi-Eskandari, B., Ghaderian, S. M., and Schat, H. (2018). Differential interactive effects of the Ca/Mg quotient and PEG-simulated drought in Alyssum inflatum and Fortuynia garcinii. Plant Soil 428, 213–222. doi: 10.1007/s11104-018-3649-y
Salt, D. E., Baxter, I., and Lahner, B. (2008). Ionomics and the study of the plant Ionome. Annu. Rev. Plant Biol. 59, 709–733. doi: 10.1146/annurev.arplant.59.032607.092942
Sambatti, J. B. M., and Rice, K. J. (2006). Local adaptation, patterns of selection, and gene flow in the Californian serpentine sunflower (Helianthus exilis). Evolution 60, 696–710. doi: 10.1111/j.0014-3820.2006.tb01149.x
Savolainen, O., Lascoux, M., and Merilä, J. (2013). Ecological genomics: genes in ecology and ecology in genes. Nat. Rev. Genet. 14, 807–820. doi: 10.1038/nrg3522
Schechter, S., and Branco, S. (2014). “The ecology and evolution of mycorrhizal fungi in extreme soils” in Plant ecology and evolution in harsh environments. eds. N. Rajakaruna, R. S. Boyd, and T. B. Harris (New York: Nova Science Publishers), 33–52.
Schechter, S. P., and Bruns, T. D. (2008). Serpentine and non-serpentine ecotypes of Collinsia sparsiflora associate with distinct arbuscular mycorrhizal fungal assemblages. Mol. Ecol. 17, 3198–3210. doi: 10.1111/j.1365-294X.2008.03828.x
Selby, J. P. (2014). The genetic basis of local adaptation to serpentine soils in Mimulus guttatus. Dissertation, Duke University.
Selby, J. P., Jeong, A. L., Toll, K., Wright, K. M., and Lowry, D. B. (2014). “Methods and discoveries in the pursuit of understanding the genetic basis of adaptation to harsh environments in Mimulus” in Plant ecology and evolution in harsh environments. eds. N. Rajakaruna, R. S. Boyd, and T. B. Harris (New York: Nova Science Publishers), 243–266.
Selby, J. P., and Willis, J. H. (2018). Major QTL controls adaptation to serpentine soils in Mimulus guttatus. Mol. Ecol. 27, 5073–5087. doi: 10.1111/mec.14922
Sobczyk, M. K., Smith, J. A. C., Pollard, A. J., and Filatov, D. A. (2017). Evolution of nickel hyperaccumulation and serpentine adaptation in the Alyssum serpyllifolium species complex. Heredity 118, 31–41. doi: 10.1038/hdy.2016.93
Sork, V. L., Aitken, S. N., Dyer, R. J., Eckert, A. J., Legendre, P., and Neale, D. B. (2013). Putting the landscape into the genomics of trees: approaches for understanding local adaptation and population responses to changing climate. Tree Genet. Genomes 9, 901–911. doi: 10.1007/s11295-013-0596-x
Southworth, D., Tackaberry, L. E., and Massicotte, H. B. (2014). Mycorrhizal ecology on serpentine soils. Plant Ecol. Diversity 7, 445–455. doi: 10.1080/17550874.2013.848950
Stein, R. J., Höreth, S., de Melo, J. R. F., Syllwasschy, L., Lee, G., Garbin, M. L., et al. (2017). Relationships between soil and leaf mineral composition are element-specific, environment-dependent and geographically structured in the emerging model Arabidopsis halleri. New Phytol. 213, 1274–1286. doi: 10.1111/nph.14219
Stern, D. L. (2013). The genetic causes of convergent evolution. Nat. Rev. Genet. 14, 751–764. doi: 10.1038/nrg3483
Taylor, S. I., and Levy, F. (2002). Responses to soils and a test for preadaptation to serpentine in Phacelia dubia (Hydrophyllaceae). New Phytol. 155, 437–447. doi: 10.1046/j.1469-8137.2002.00478.x
Teptina, A., Paukov, A., and Rajakaruna, N. (2018). Ultramafic vegetation and soils in the circumboreal region of the northern hemisphere. Ecol. Res. 33, 609–628. doi: 10.1007/s11284-018-1577-1
Turner, T. L., Bourne, E. C., Von Wettberg, E. J., Hu, T. T., and Nuzhdin, S. V. (2010). Population resequencing reveals local adaptation of Arabidopsis lyrata to serpentine soils. Nat. Genet. 42, 260–263. doi: 10.1038/ng.515
Turner, T. L., von Wettberg, E. J., and Nuzhdin, S. V. (2008). Genomic analysis of differentiation between soil types reveals candidate genes for local adaptation in Arabidopsis lyrata. PLoS One 3:e3183. doi: 10.1371/journal.pone.0003183
Veatch-Blohm, M. E., Roche, B. M., and Campbell, M. J. (2013). Evidence for cross-tolerance to nutrient deficiency in three disjunct populations of Arabidopsis lyrata ssp. lyrata in response to substrate calcium to magnesium ratio. PLoS One 8:e63117. doi: 10.1371/journal.pone.0063117
Veatch-Blohm, M. E., Roche, B. M., and Dahl, E. E. (2017). Serpentine populations of Arabidopsis lyrata ssp. lyrata show evidence for local adaptation in response to nickel exposure at germination and during juvenile growth. Environ. Exp. Bot. 138, 1–9. doi: 10.1016/j.envexpbot.2017.02.017
Vlamis, J. (1949). Growth of lettuce and barley as influenced by degree of calcium-saturation of soil. Soil Sci. 67:453–466. doi: 10.1097/00010694-194906000-00005
Vlamis, J., and Jenny, H. (1948). Calcium deficiency in serpentine soils as revealed by adsorbent technique. Science 107:549. doi: 10.1126/science.107.2786.549
von Wettberg, E. J. B., Ray-Mukherjee, J., D’Adesky, N., Nesbeth, D., and Sistla, S. (2014). “The Evoltionary ecology and genetics of stress resistance syndrome (SRS) traits: revisiting chapin, autumn and pugnaire (1993)” in Plant ecology and evolution in harsh environments. eds. N. Rajakaruna, R. S. Boyd, and T. B. Harris (New York: Nova Science Publishers), 201–226.
Walker, R. B., Walker, H. M., and Ashworth, P. (1955). Calcium-magnesium nutrition with special reference to serpentine soils. Plant Physiol. 30, 214–221. doi: 10.1104/pp.30.3.214
Westerbergh, A. (1994). Serpentine and non-serpentine Silene dioica plants do not differ in nickel tolerance. Plant Soil 167, 297–303. doi: 10.1007/BF00007956
Willems, G., Dräger, D. B., Courbot, M., and Godé, C. (2007). The genetic basis of zinc tolerance in the metallophyte Arabidopsis halleri ssp. halleri (Brassicaceae): an analysis of quantitative trait loci. Genetics 176, 659–674. doi: 10.1534/genetics.106.064485
Wright, K. M., Lloyd, D., Lowry, D. B., Macnair, M. R., and Willis, J. H. (2013). Indirect evolution of hybrid lethality due to linkage with selected locus in Mimulus guttatus. PLoS Biol. 11:e1001497. doi: 10.1371/journal.pbio.1001497
Wright, J. W., Stanton, M. L., and Scherson, R. (2006). Local adaptations to serpentine and non-serpentine soils in Collinsia sparsiflora. Evol. Ecol. Res. 8, 1–21.
Wright, J. W., and Von Wettberg, E. (2009). ‘Serpentinomics’ ‐ an emerging new field of study. Northeast. Nat. 16, 285–296. doi: 10.1656/045.016.0521
Keywords: serpentine, adaptation, population genomics, edaphic extremes, ionomics
Citation: Konečná V, Yant L and Kolář F (2020) The Evolutionary Genomics of Serpentine Adaptation. Front. Plant Sci. 11:574616. doi: 10.3389/fpls.2020.574616
Received: 20 June 2020; Accepted: 23 November 2020;
Published: 16 December 2020.
Edited by:
Thomas L. P. Couvreur, IRD UMR232 Diversité, adaptation, développement des plantes (DIADE), FranceReviewed by:
Nishanta Rajakaruna, California Polytechnic State University, United StatesCopyright © 2020 Konečná, Yant and Kolář. This is an open-access article distributed under the terms of the Creative Commons Attribution License (CC BY). The use, distribution or reproduction in other forums is permitted, provided the original author(s) and the copyright owner(s) are credited and that the original publication in this journal is cited, in accordance with accepted academic practice. No use, distribution or reproduction is permitted which does not comply with these terms.
*Correspondence: Filip Kolář, ZmlsaXAua29sYXJAbmF0dXIuY3VuaS5jeg==; ZmlsaXAua29sYXJAZ21haWwuY29t; Levi Yant, bGV2aS55YW50QG5vdHRpbmdoYW0uYWMudWs=
Disclaimer: All claims expressed in this article are solely those of the authors and do not necessarily represent those of their affiliated organizations, or those of the publisher, the editors and the reviewers. Any product that may be evaluated in this article or claim that may be made by its manufacturer is not guaranteed or endorsed by the publisher.
Research integrity at Frontiers
Learn more about the work of our research integrity team to safeguard the quality of each article we publish.