- 1Laboratory of Plant Pathology, Multidisciplinary Institute for Environmental Studies Ramon Margalef, University of Alicante, Alicante, Spain
- 2Department of Marine Sciences and Applied Biology, Laboratory of Plant Pathology, University of Alicante, Alicante, Spain
- 3Department of Agrochemistry and Biochemistry, Multidisciplinary Institute for Environmental Studies Ramon Margalef, University of Alicante, Alicante, Spain
- 4Department of Plant Biology (Plant Physiology), University of Murcia, Murcia, Spain
- 5Department of Organic Chemistry, Chemistry Faculty, University of Seville, Seville, Spain
- 6Department of Mathematics, University of Alicante, Alicante, Spain
- 7Plant Physiology Laboratory, Faculty of Biosciences, Universidad Autonoma de Barcelona, Bellaterra, Spain
In this work, we use electrophysiological and metabolomic tools to determine the role of chitosan as plant defense elicitor in soil for preventing or manage root pests and diseases sustainably. Root exudates include a wide variety of molecules that plants and root microbiota use to communicate in the rhizosphere. Tomato plants were treated with chitosan. Root exudates from tomato plants were analyzed at 3, 10, 20, and 30 days after planting (dap). We found, using high performance liquid chromatography (HPLC) and excitation emission matrix (EEM) fluorescence, that chitosan induces plant hormones, lipid signaling and defense compounds in tomato root exudates, including phenolics. High doses of chitosan induce membrane depolarization and affect membrane integrity. 1H-NMR showed the dynamic of exudation, detecting the largest number of signals in 20 dap root exudates. Root exudates from plants irrigated with chitosan inhibit ca. twofold growth kinetics of the tomato root parasitic fungus Fusarium oxysporum f. sp. radicis-lycopersici. and reduced ca. 1.5-fold egg hatching of the root-knot nematode Meloidogyne javanica.
Introduction
Chitosan is a linear polymer of β-(1-4)-linked N-acetyl-2-amino-2-deoxy-D-glucose (acetylated) and 2-amino-2-deoxy-D-glucose (deacetylated) (Kaur and Dhillon, 2014). It is generally obtained by partial deacetylation of chitin (Kumar, 2000), which is the second most abundant polysaccharide in nature after cellulose (Elieh-Ali-Komi and Hamblin, 2016). Chitin is a major component of the cuticle of insects, exoskeleton of crustaceans and fungal cell walls. Chitosan has been described as elicitor of plant defenses (Yin et al., 2016) and hormones of food security crops such as tomato (Iriti and Faoro, 2008; El-Tantawy, 2009). Chitosan also propitiates accumulation of auxin [mainly indoleacetic acid (IAA)] in the apex of plant roots (Lopez-Moya et al., 2017). Since most studies have been carried out in the phylloplane, we devised experiments to test the effect of chitosan on tomato rhizodeposition in both hydroponic and solid plant substrate systems.
Electrophysiology can monitor the response of plant roots to stress (Rodrigo-Moreno et al., 2013). Membrane potential reflects the action of all pumps in the cell membrane to maintain ion gradients (Alberts et al., 2002). Root cell membranes detect changes in their environment and respond starting metabolic cascade reactions (Fürstenberg-Hägg et al., 2013; Matzke and Matzke, 2013). Those reactions could lead to new compounds of agronomic and ecological interest.
Rhizodeposition is the process of releasing organic compounds from roots to the external medium. Plants exude a wide variety of low molecular weight organic compounds (e.g., amino acids and small peptides, organic acids, hormones, sugars, phenolics, and other secondary metabolites) (Flores et al., 1996). Resolving this complex mixture requires the use of diverse metabolomics technologies (Escudero et al., 2014; van Dam and Bouwmeester, 2016). Root exudates are paramount in plant–microbe interactions in the rhizosphere, including beneficial and pathogenic microbes and play a key role in signaling (Hirsch et al., 2003; Bais et al., 2006; Walker et al., 2009; Zhang et al., 2019; Valette et al., 2020). Metabolomics can help us to understand the chemical interactions between organisms in the rhizosphere, as well as the importance to uncover toxic compounds (Baldrian, 2019). Metabolomics allows detection of phenolic compounds by fluorescence (Hupp et al., 2019). Phenolics have diverse functions in plants, but one of the most important is their role in plant defense and signaling (Mandal et al., 2010). Hormones are also signaling molecules that can also be found using metabolomics (Street and Schenk, 1981; Li et al., 2009; Yang et al., 2015). Auxin (e.g., IAA), salicylic acid (SA), jasmonic acid (JA), and abscisic acid (ABA) are produced in response to physiologic or metabolic changes (Asami and Nakagawa, 2018). Phytomelatonin is considered a master regulator in plant stress conditions (Arnao and Hernández-Ruiz, 2019) and also involved in the regulation of several plant hormones (Arnao and Hernández-Ruiz, 2018). Phytomelatonin promotes growth and root appearance (Hernández-Ruiz et al., 2005; Arnao and Hernández-Ruiz, 2007).
Fungal (Fusarium oxysporum f. sp. radicis-lycopersici) and nematode (Meloidogyne javanica) root pathogens are used in this work. These pathogens threaten food security worldwide (FAO, 2017). Root-knot nematodes cause 25–100% tomato yield losses (Seid et al., 2015) and Fusarium wilt causes up to 80% of crop losses (Singh et al., 2017).
The aim of this work is to determine the effect of chitosan on plant rhizodeposition. Root electrophysiology allows us to monitor the effect of chitosan on membrane functionality. In addition, metabolomic techniques are used to determine how chitosan modulates the composition of root exudates. Finally, we test these exudates against root pathogens which threaten food security worldwide. This will allow us to validate the role of chitosan as a plant defense inducer in soil for preventing or managing root pests and diseases sustainably.
Materials and Methods
Chitosan, Plants, Fungi, and Nematodes
Chitosan with 70 kDa molecular weight and 80.5% deacetylation degree was used in all experiments. Chitosan was obtained from Marine BioProducts GmbH (Bremerhaven, Germany) and made as in Palma-Guerrero et al. (2007). Tomato plants (Solanum lycopersicum cv. Marglobe) were used in all experiments. Pochonia chlamydosporia, isolate number 123 (ATCC no. MYA-4875; CECT no. 20929; PRJNA68669, NCBI txid1052797) was isolated from Heterodera avenae eggs in south west Spain (Olivares and López-Llorca, 2002). Fusarium oxysporum f. sp. radicis-lycopersici (Strain 4287, CBS 123668, FGSC 9935, NRRL 34396) was obtained from CBS-KNAW culture collection. Meloidogyne javanica was obtained from a field population and maintained in susceptible tomato plants. Nematode egg masses were dissected from root-knot nematode infested roots and stored at 4°C. Egg masses were hand-picked and surface-sterilized as in McClure et al. (1973) with modifications.
Root Electrophysiology Experiments
Tomato seeds were surface sterilized using 1% sodium hypochlorite and washed three times 1 min each with sterile distilled water (SDW). Tomato seeds were then placed on germination medium (GM, Glucose 10 g L–1, Yeast Extract 0.1 g L–1, Bactopeptone 0.1 g L–1, Technical Agar 12 g L–1). GM plates with tomato seeds were placed at 4°C for 2 days for stratification and incubated at 24°C, 65% relative humidity (RH), in the dark for 5 days and in a photoperiod (16:8) for further 5 days. Plantlets were then placed individually in a holder chamber filled with Gamborgs B5 1:10 (Sigma; Gamborg et al., 1968). Glass microcapillaries filled with 0.5M KCl (tip diameter < 1 μm) were inserted into root cortical cells until a stable basal potential was obtained (Gunsé et al., 2016). Roots were exposed to increasing concentrations of chitosan (0.1, 1, and 2 mg mL–1 in Gamborgs B5 1:10 medium) and membrane potentials were recorded. No chitosan was used for control treatments. Between each change of chitosan concentration, root medium was replaced for Gamborgs B5 1:10 to check the physiological status of the cell. At least three biological replicates and four technical replicates were performed per treatment.
Root Vital Staining
Tomato plantlets were placed in Gamborgs B5 1:10 liquid medium 1 day for acclimatization. Chitosan was then added at 0.1, 1, and 2 mg mL–1. Plants exposed only to Gamborgs B5 1:10 were used as controls. Plantlets with treatments were incubated for 24 h. Roots were then stained with fluorescein diacetate (FDA), 5 mg mL–1 in acetone diluted 1:250 in Dulbecco’s phosphate-buffered saline (DPBS, Thermo Fisher) and propidium iodide (PI, 20 mg mL–1 in DPBS; Jones and Senft, 1985). Roots were visualized using a Nikon Optiphot microscope using 10X objective with an attached Nikon DS–5 M camera system using epifluorescence (Ex: 450–490 nm, Em: 520 nm). Non-damaged cells show green FDA fluorescence and nuclei of damaged cells show red PI fluorescence.
Plant Growth Conditions
Two experiments were carried out: in vitro and in cups. This allowed us to progressively approach to a realistic system.
Tomato plantlets (Experiment 1) were placed in 200 mL expanded polystyrene sterile cups each containing 100 cm3 of sterilized sand. They were then incubated in a culture chamber (SANYO MLR-351H) at 65% RH, 24°C with a 16:8 h (light:dark) photoperiod. Plants were irrigated for 20 days with Gamborgs B5 basal mixture 1:10 keeping moisture to field capacity. Plants were then removed, washed and introduced individually in Magenta Boxes (575 mL, Sigma). Each Box was filled with 50 mL of Gamborgs B5 1:10 (control) or Gamborgs B5 1:10 amended with chitosan (0.1, 1, 2 mg mL–1). Nine biological replicates were made per treatment. Plants were incubated for 3 days as described above. Exudates collected in Experiment 1 are root exudates accumulated over 3 days. This experiment was performed two times independently.
In Experiment 2, tomato plantlets were grown as for Experiment 1. Plants in cups were then irrigated with 1:10 Gamborgs B5 basal mixture on its own or amended with 0.1 mg mL–1 chitosan. Plants were incubated as before. Ten biological replicates per treatment were made. Root Exudates were sampled 10, 20 and 30 days after planting (dap) for further analyses. Exudates collected in Experiment 2 are active root exudates (24 h collection, see section “Collection of Root Exudates”). This experiment was performed two times independently.
Collection of Root Exudates
In Experiment 1, root exudates accumulated for 3 days in Magenta Boxes were collected and filtered through Miracloth (Calbiochem). Pools were made with the exudates of three plants each, so we worked with three biological replicates per treatment. This was performed twice. Root exudate pools were filtered by 0.22 μm (Q-MAX) and frozen at −20°C for further use.
In Experiment 2, whole plants were removed, and their root systems washed in SDW. De novo root exudates from these plants were collected by placing individual whole plants in sterile plastic containers with 20 mL SDW per gram of root. Plants were incubated in the dark at 24°C, 65% RH for 24 h. Plants were then removed, and root exudates from the 10 biological replicates were collected by 0.22 μm (Q-MAX) filtration and stored frozen at −20°C until used. This was performed twice.
Emission Excitation Matrix (EEM) Fluorescence Analysis
Two mL of each root exudate (Experiments 1 and 2) were collected and excitation emission matrix (EEM) Fluorescence spectra were obtained with a spectrofluorometer (Jasco FP-6500) equipped with a 150W Xenon lamp. Contour maps of EEM fluorescence spectra were obtained from water extracts of whole root exudates (10 samples) or pools. The emission (Em) wavelength range was fixed from 220 to 460 nm in 5 nm steps, whereas the excitation (Ex) wavelength was fixed from 220 to 350 nm in 2 nm steps. The slit width was 5 nm and the root exudates were placed in a 1 cm path length fused quartz cell (Hellma). The UV-visible spectra of samples were acquired (SHIMADZU UV-160 spectrophotometer, 200–800 nm, 1 cm quartz cuvette). Absorbance was always lower than 0.1 (ODunits) at 254 nm in order to reduce the absorbance of the solution to eliminate potential inner filter effects (Mobed et al., 1996). EEM fluorescence spectra of root exudates were analyzed using parallel factor analysis (PARAFAC) as in Ohno and Bro (2006). PARAFAC model Components were calculated for each treatment and time. These analyses have been performed twice with 9 or 10 biological replicates each (Experiment 1 or Experiment 2 respectively).
Plant Hormone Analysis
Root exudate pools (Experiment 1) were lyophilized to analyze indoleacetic acid (IAA), abscisic acid (ABA), salicylic acid (SA) and jasmonic acid (JA) by ultra performance liquid chromatography-mass spectrometry (UPLC-MS). Material was extracted with 80% Methanol-1% Acetic acid. Deuterium-labeled hormones (purchased from Prof. L. Mander-Canberra, OlChemim Ltd. -Olomouc): [2H5] IAA, [2H4] SA, and [2H6] ABA were added as internal standards. For quantification of JA, dhJA was used instead. For collecting the fractions containing SA, ABA, and JA; extracts were passed consecutively through HLB (reverse phase), MCX (cationic exchange) and WAX (ionic exchange) columns (Oasis 30 mg, Waters), as described in Seo et al. (2011). The final residue was dissolved in 5% Acetonitrile – 1% Acetic acid and separated by reverse phase UPLC chromatography (2.6 μm Accucore RP-MS column, 100 mm length × 2.1 mm i.d.; Thermo Fisher Scientific) with a 5 to 50% acetonitrile gradient. Hormones were analyzed by electrospray ionization and targeted-SIM using a Q-Exactive spectrometer (Orbitrap detector, Thermo Fisher Scientific). Concentrations of hormones in extracts were determined using embedded calibration curves and the Xcalibur 4.1 SP1 build 48 and TraceFinder programs. We thank Dr. Esther Carrera for hormone quantification carried out at the Plant Hormone Quantification Service, Valencia, Spain1.
Phytomelatonin Detection
Phytomelatonin was also tested in both exudates and tissues. Ten-day old seedlings were grown with chitosan (0.1, 1, 2 mg mL–1) in Gamborgs B5 1:10 for 3 days. Plants exposed to Gamborgs B5 1:10 only were used as control. For each treatment, 0.2–0.3 g of roots were dried onto sterile paper and placed in a 4 mL polypropylene tube with 3 mL ethyl acetate. Three biological replicates were made. Samples were shaken at 120 rpm and 4°C in the dark overnight. Roots were removed and solvent evaporated under vacuum. The dry residue was resuspended in 1 mL acetonitrile and 0.22 μm filtered. Phytomelatonin from root tissues was quantified by HPLC with fluorescence detection with Ex/Em wavelength pair of 280/348 nm, as in Hernández-Ruiz and Arnao (2008). For root exudates, three biological replicates of 1 mL of Experiment 1 pools were used. Phytomelatonin was extracted and analyzed as described above.
Nuclear Magnetic Resonance (1H NMR)
Root exudates from Experiment 2 were pooled (5 replicates/pool), lyophilized and resuspended in 1 mL of D2O (deuterated water). Two pools were obtained from each treatment and time. Six hundred microliters of filtered pools were placed in a 5 mm NMR tube with 0.75% 3-(trimethylsilyl)propionic-2,2,3,3-d4 acid sodium salt (TSP) and 0.002 g sodium azide.
1H NMR experiments were performed on a Bruker AVIII 700 MHz (CITIUS, University of Sevilla, Spain). The number of scans was 256 and the experiments were carried out at 298 K. 1H chemical shifts were internally referenced to the TSP at δ 0.00. 1H NMR spectra were aligned using TopSpinTM (Bruker).
Sensitivity of NMR is different in each region. For region I (organic-acid and amino-acid region), the threshold was set in 0.1. For II (sugars/polyalcohols region) and III (phenolics/aromatic compounds region) peaks showed less intensity, consequently the threshold was set in 0.01 and 0.001 respectively.
High Performance Liquid Chromatography Electrospray Ionization Tandem Mass Spectrometry (HPLC-ESI-MS)
HPLC-ESI-MS analyses were performed with a High-Performance Liquid Chromatography system with an Agilent 1100 Series model coupled to a UV-visible variable wavelength detector and a mass spectrometer with ion trap analyser Series LC/MSD Trap SL (Agilent, Santa Clara, CA, United States). The mass spectrometer was operated in the positive and negative ESI modes, and the ion spray voltage was set at 4 kV. Mass range was set from 50 to 350 atomic mass units. Nitrogen was used as carrier gas (70 psi), and the ion transfer capillary heated to 350°C. Injections were carried out using an HTC Pal autosampler (CTC Analytics, Zwingen, Switzerland) equipped with a 20 μL sample loop.
Pools of 20 dap tomato root exudates (Experiment 2) were infused into the flow of the HPLC system (10 μL) through a T connection under the following conditions: flow rate, 1 mL min–1. Ultrapure water with 0.1% Formic Acid was used as Solvent A, whereas MeOH with 0.1% Formic Acid was used as Solvent B. From 0 to 15 min Solvent B was kept at 10% and afterward a gradient to 90% was established during 20 min and decreased again to 10% until minute 25. The LC separations were carried out with a Poroshell 120 EC-C18 column (4.6 mm × 100 mm, 2.7 μm -Agilent Technologies-). Runs were performed at 25°C. Raw data were transformed as explained in Marhuenda-Egea et al. (2013).
Partial least squares regression discriminant analyses (PLSLDA) show that m/z intensities depend on treatment. Loading values lower than 0 indicate that m/z intensity is higher in chitosan-treated plant root exudates. A threshold was set at ± 0.2 to identify m/z with significant variations.
Significant m/z values after PLSLDA analyses were selected for further analysis by HPLC-ESI-MS/MS using a LC-MSD-Trap-SL (Agilent). The temperature was 350°C and the pressure 70.00 psi with a flow of 12 L min–1. Selected masses are shown in the dataset (Supplementary Material).
After obtaining data on the fragmentation of the ions of interest, using MS\MS, we sought to identify the molecules that generated the fractionation patterns. We used two different strategies to identify the molecules. In the first strategy, we searched the databases by means of the mass of the ions, using the LC–MS search tool, using the positive (adduct type M+H) or negative (adduct type M-H) mode, with a tolerance of 0.1 Da. Once the molecules with masses similar to that searched were obtained, we checked the spectra, both real and predicted, collected in the databases that were most suitable for the MS\MS spectra we had obtained. The second strategy was based on searching different fragments generated by an ion in MS\MS fractionation using the LC–MS\MS search tool and comparing the spectra, in the same way as it was done previously. The signals from NMR and HPLC-MS were assigned, putatively, with different databases, such as Human Metabolome Database2, MassBank3 and Biological Magnetic Resonance Data Bank4.
Evaluation of Tomato Root Exudates on Fungi and Nematode Eggs
Bioassays were performed in 96-well plates (Thermo Scientific) using 20 dap root exudate pools obtained in Experiment 2. These exudates were used because higher doses of chitosan would not allow the correct development of the plant and could not be used in a realistic experiment. Three technical replicates of each exudate were made. For each treatment, there were two biological replicates (pools of exudates containing 10 biological replicates initially). For fungal experiments, 200 μL of exudate (pool of 5 plant root exudates each) and conidia were added per well to reach a concentration of 106 conidia⋅mL–1. For both, FORL and Pc, OD490 was calculated after 4 and 8-days respectively using a microplate reader (Tecan SPECTRAfluor). Results were handled with XFluor SoftwareTM. For time series with FORL and Pc, the relation between two variables is not linear. In both cases, Smoother Model Lowess (“Locally weighted regression”) was applied (Cleveland, 1979).
To evaluate the effects of root exudates on root-knot nematode eggs, experiments were performed with 200 μl of exudate or water (control) containing 100 M. javanica eggs each. Hatching percentage was scored using an inverted microscope after 72 h incubation at 30°C. All experiments were performed twice with similar results.
Statistical Analyses
Excitation emission matrix Fluorescence data was analyzed by PARAFAC, as described above, and the contribution of the Components 1, 2, and 3 analyzed by ANOVA tests. The level of significance in all cases was 95%. All statistical analyses were performed using GraphPad Prism version 7.00 (GraphPad Software, La Jolla, CA, United States5).
HPLC-ESI-MS data were processed using a Partial Least Square (PLS) regression model (Verboven and Hubert, 2005; Marhuenda-Egea et al., 2013). Classical PCA were also performed to display and group data (Verboven and Hubert, 2005). This data analysis was carried out using the LIBRA toolbox6.
Results
Chitosan Depolarizes Plasma Membrane and Reduces Tomato Root Cell Viability
Chitosan (1 and 2 mg mL–1) depolarizes (p < 0.001) plasma membrane of tomato root cells (Figure 1A). This is reflected in loss of cell viability (PI red staining, Figure 1B). Roots incubated with 2 mg mL–1 chitosan show a curved morphology and dark precipitates. Conversely, a low dose of chitosan (0.1 mg mL–1) does not alter plasma membrane potential. However, these roots show both viable (FDA green staining) and non-viable cells, indicating some chitosan damage. Untreated tomato roots show mostly viable cells, stained with FDA. The slight red staining is due to natural senescence of root epidermic cells (Figure 1B, arrow).
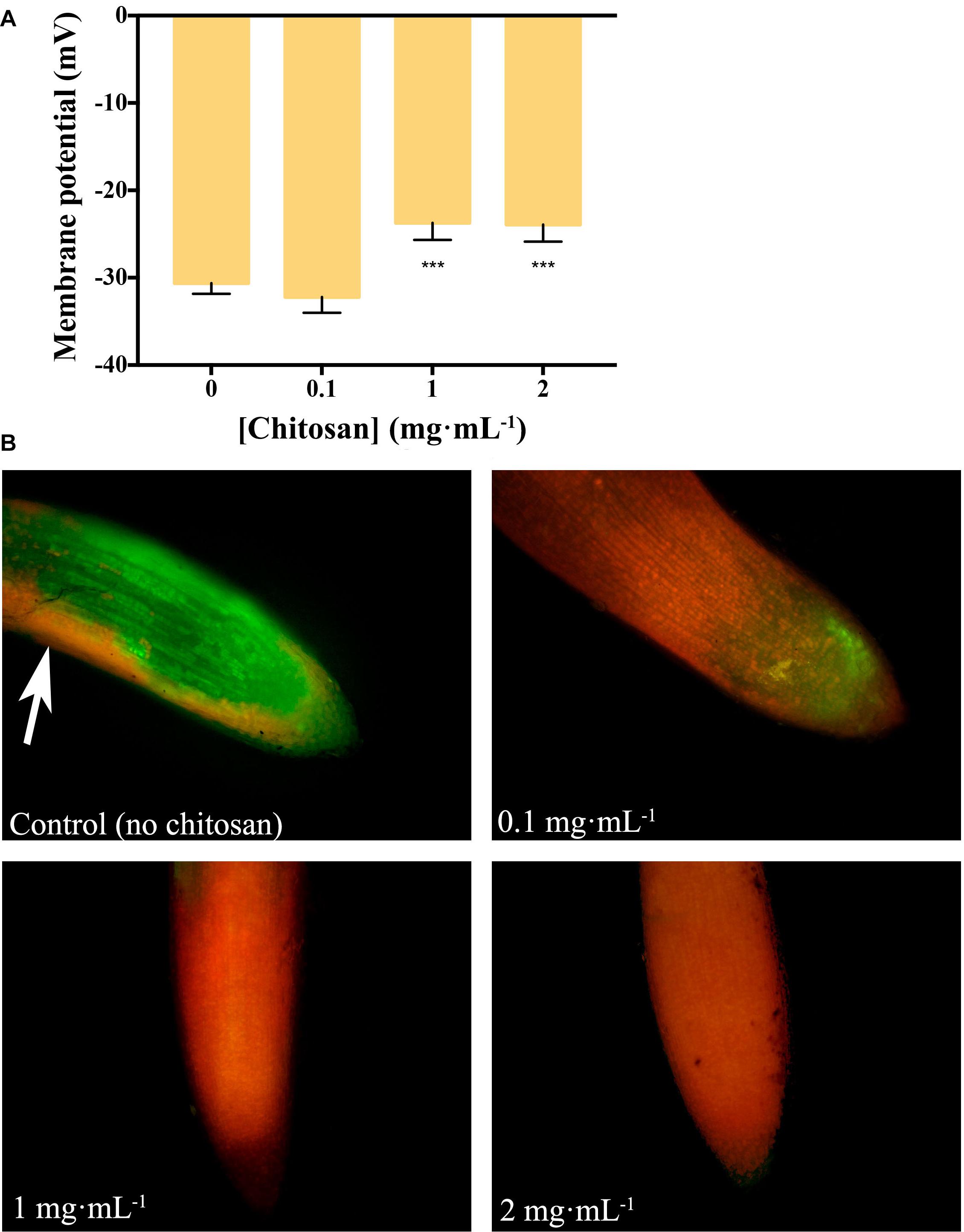
Figure 1. Chitosan depolarizes plasma membrane and damages tomato root cells. (A) Variation in membrane potential of root cells with chitosan. High doses (1 and 2 mg mL– 1) of chitosan significantly reduce membrane potential. (B) High doses of chitosan damage root cells after 24 h. Red staining labels damaged cells while green staining labels living ones. Three biological replicates were made for each treatment. Multifactorial ANOVA was used to compare treatments [p-values 0.05 (*), 0.01 (**), 0.001 (***), and 0.0001 (****)].
Chitosan Induces Hormones and Phenolic Compounds in Root Exudates
Excitation emission matrix Fluorescence analysis of root exudates resulted in three components (Figure 2 and Supplementary Table 1). Components 1 and 3 are the most induced (p < 0.01) by chitosan. Component 1 includes a putative fluorophore with Ex/Em wavelength pair of 315/430 nm, which could correspond to SA (Street and Schenk, 1981). Component 3 includes Ex/Em wavelength pairs of 245/384 and 265/384 nm, which may correspond to aromatic amino acids and peptides (Yang et al., 2015; Table 1).
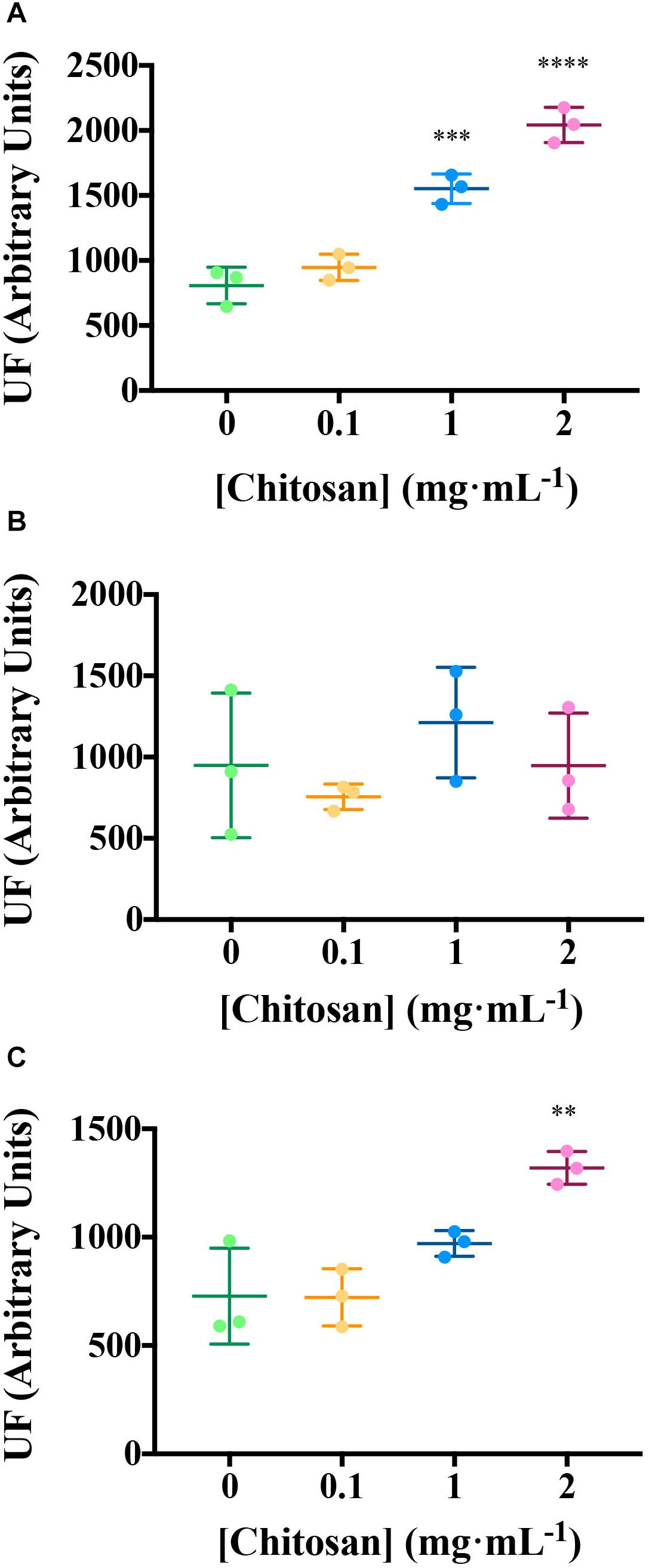
Figure 2. Chitosan increases EEM fluorescence of tomato root exudates. (A) Component 1 (salicylic acid); (B) Component 2 (phenolics and salicylic acid derivatives); (C) Component 3 (aromatic aa and peptides). For Ex/Em coordinates of Components see Table 1. UF, fluorescence units; EEM, emission excitation matrix. Mean and Standard Deviation of three biological replicates (three pools containing root exudates from three individuals each) is represented. Multifactorial ANOVA was used to compare treatments [p-values 0.05 (*), 0.01 (**), 0.001 (***), and 0.0001 (****)].
Chitosan induces other hormones in tomato root exudates (HPLC–MS; Figure 3). High doses of chitosan (1 and 2 mg mL–1) induce (p < 0.01) IAA accumulation in root exudates (Figure 3A). Plant defense hormones (SA, JA, and ABA) are also significantly induced (p < 0.05) by chitosan (1 mg mL–1) in tomato root exudates (Figures 3B–D). This effect is lost at 2 mg mL–1 chitosan. This could be the result of a root systemic damage caused by large chitosan concentrations. In view of the effect of chitosan on plant hormone homeostasis, endogenous phytomelatonin in roots was evaluated. Chitosan (1 mg mL–1) causes a slight rise on phytomelatonin content in tomato root cells (Figure 3E). Phytomelatonin levels detected in tomato root exudates are close to nil (Supplementary Figure 1).
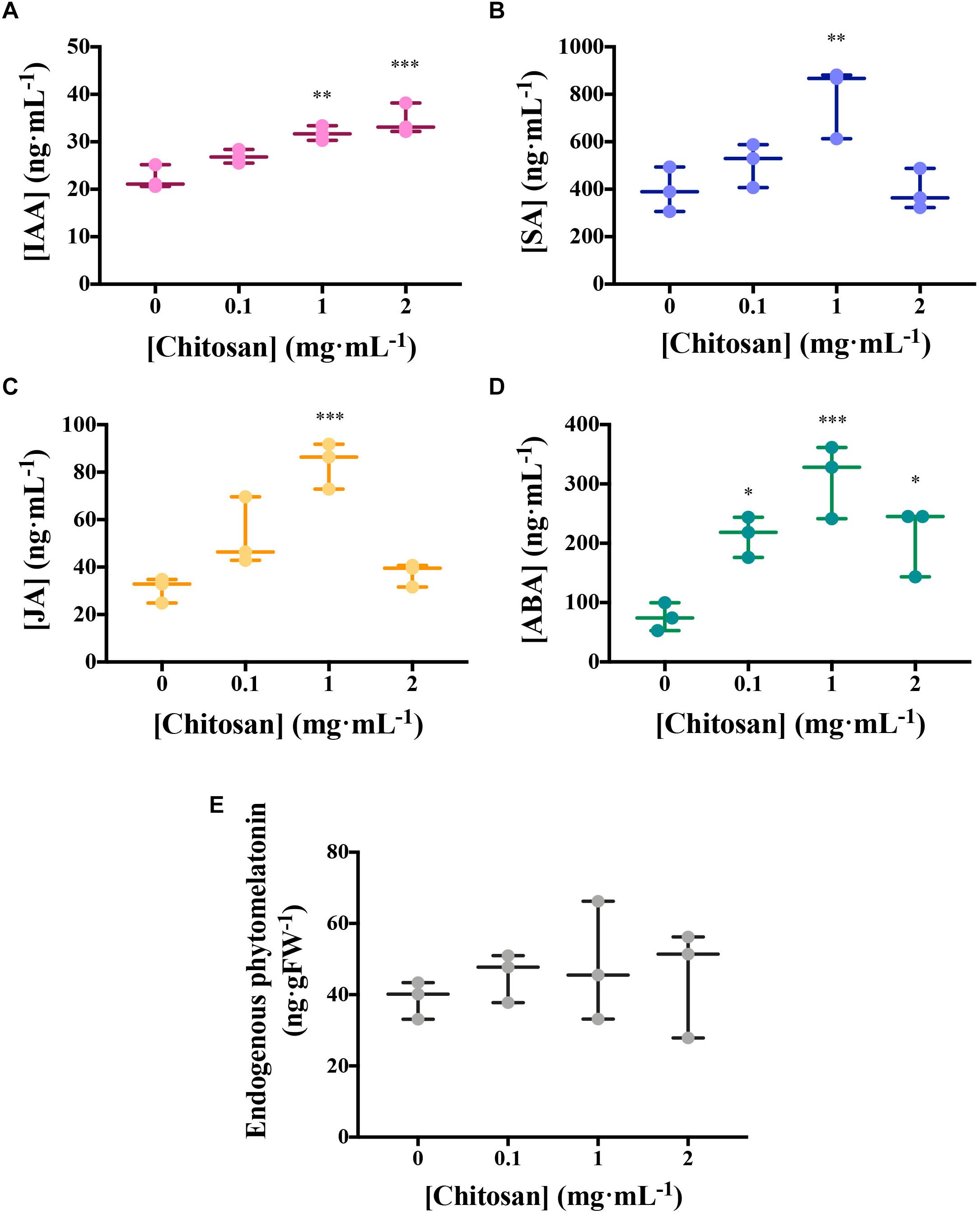
Figure 3. Chitosan induces plant hormones. (A–D) Figures correspond to hormone quantitation of accumulated tomato root exudates in three biological replicates over 3 days. (A) Indole acetic acid; (B) salicylic acid; (C) jasmonic acid; (D) abscisic acid; (E) Phytomelatonin quantitation in tomato roots. There is a maximum level of hormones present in exudates from plants treated with 1 mg mL−1 chitosan. Multifactorial ANOVA was used to compare treatments [p-values 0.05 (*), 0.01 (**), 0.001 (***), and 0.0001 (****)].
Chitosan Induces de novo Exudation of SA and Phenolics in Roots
In view of the results obtained with tomato roots elicited for 3 days with chitosan, we irrigated tomato plants with a low dose of chitosan (0.1 mgmL–1) during 10, 20, and 30 days and analyzed de novo exudation in roots. Ten days after planting (dap), chitosan increases de novo (p < 0.01) exudation of a fluorescence signature putatively belonging to SA (Figure 4 and Table 1). Twenty dap, chitosan significantly increases (p < 0.001) fluorescence intensity of Component 3, putatively assigned to phenolics (Mostofa et al., 2013; Parri et al., 2020) and SA derivatives (Street and Schenk, 1981). This tendency is also found for Components 1 (putatively aromatic amino acids and peptides) and 2 (putatively IAA; Li et al., 2009), although differences are not significant. In late root exudates (30 dap), fluorescence intensity decreases respect to early root exudates. This could be due to root aging and lignification. At this time, EEM Fluorescence spectrum is separated into 2 Components only (Supplementary Table 1 and Supplementary Figure 2) with no differences respect to controls.
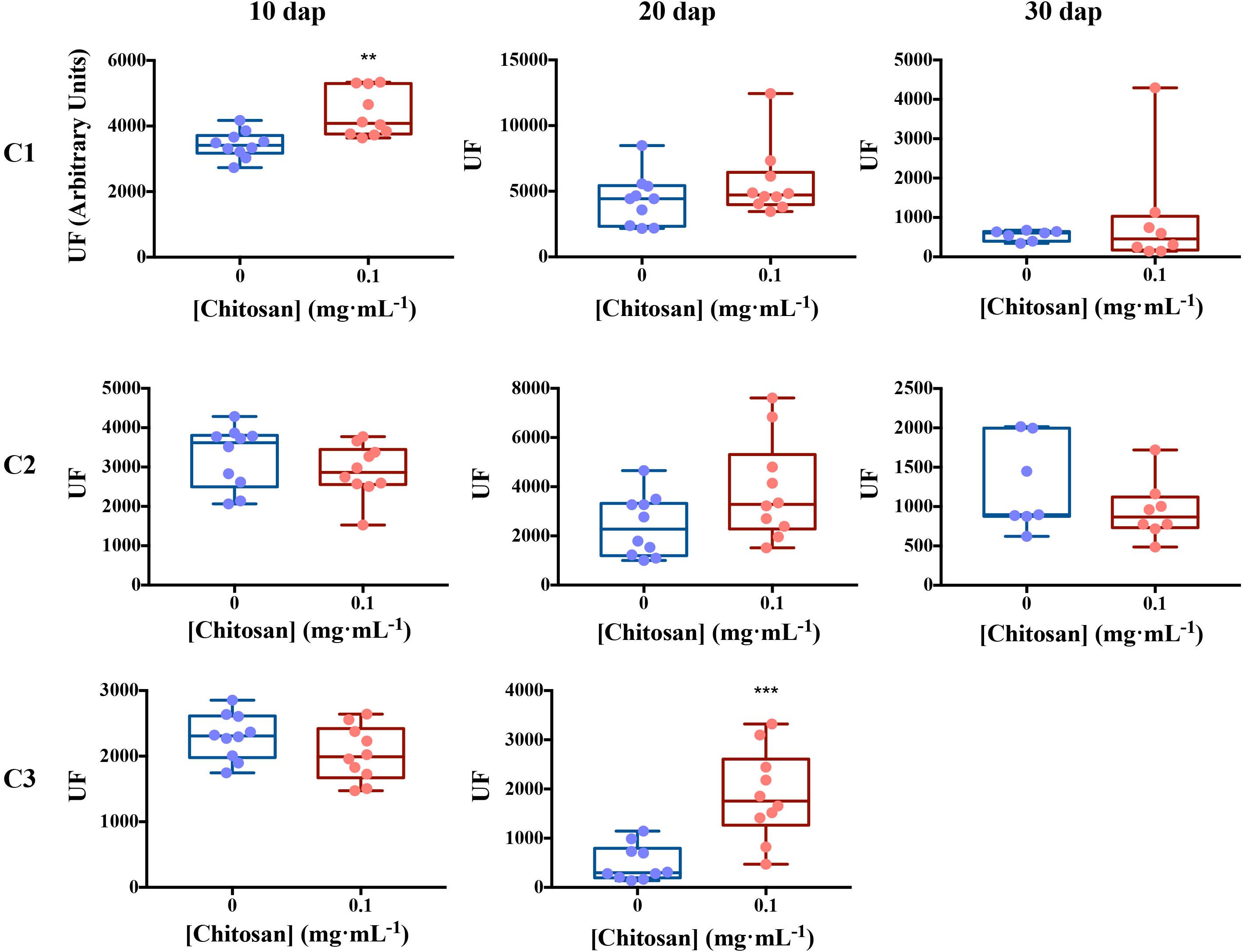
Figure 4. Excitation emission matrix fluorescence dynamics of tomato root exudates. For Ex/Em coordinates of Components see Table 1. Ten biological replicates per treatment were used. EEM, emission excitation matrix; dap, days after planting; C1, component 1; C2, component 2; C3, component 3; UF, fluorescence units. Mann–Whitney test was used for not normal distribution treatments. Welch’s parametric test was used for the remaining treatments [p-values 0.05 (*), 0.01 (**), 0.001 (***), and 0.0001 (****)].
Metabolomic Diversity of Tomato Root Exudates Varies With Time
Pools were made to concentrate root exudates and avoid sample variability (Yuan et al., 2015; Yang et al., 2016). Root exudates from 20 dap tomato plants (chitosan treated and controls) displayed most NMR peaks (Figure 5). This may be because older plants (30 dap) are more lignified (Cervilla et al., 2009) and display less rhizodeposition. Manual curation of 1H NMR profiles of root exudates from plants 10, 20, and 30 dap from chitosan treatments and controls show no qualitative differences and contain 31, 123, and 18, peaks respectively. Ninety-three peaks are specific to 20 dap root exudates. Nine 20 dap specific peaks were identified as (Table 2): leucine/isoleucine (13), acetate (53), raffinose (99, 103), glucose (100), uracil (105 and 120), cinnamic acid (109, 119, 121), fumaric acid (109), p-aminobenzoic acid (112 and 122) and trigonelline (125, 130, 131). Chitosan decreases (p < 0.05) acetate (peak 53) content in 20 dap root exudates. Six peaks, among them methanol (80) and formic acid (129), are found 20 and 30 dap. Lactate (peak 36) and malate (peaks 62 and 64) have been detected in all time points evaluated. All detected peaks are listed in Supplementary Table 2. A representative 1H NMR profile of tomato root exudates is shown in Supplementary Figure 3.
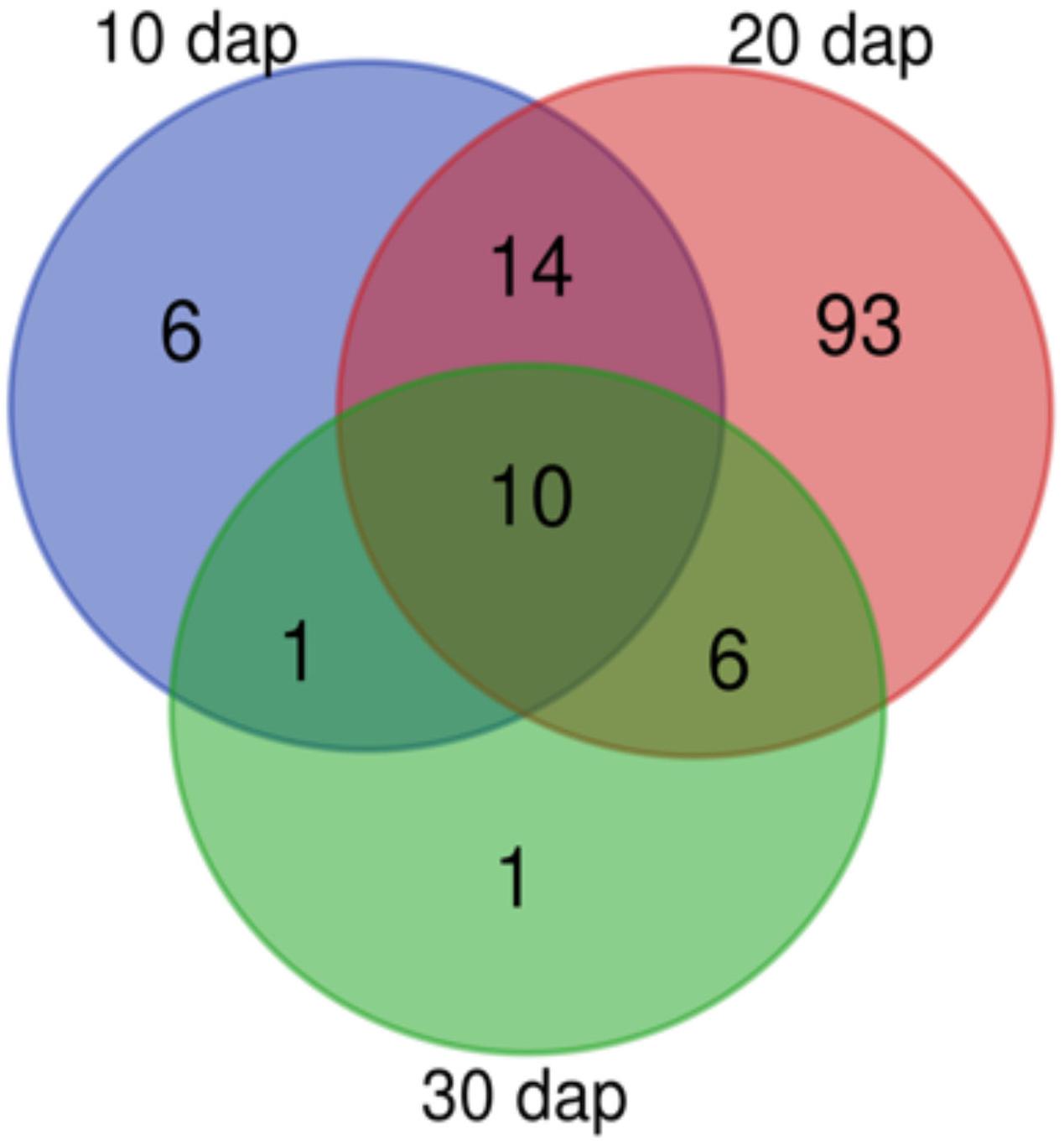
Figure 5. Tomato rhizodeposition varies with time. Venn-diagram of 1H Nuclear Magnetic Resonance analyses peaks detected in tomato root exudates at 10, 20, and 30 days after planting. In all times, plants were treated with 0.1 mg mL– 1 chitosan.
Chitosan Induces Lipid Signaling and Defense Compounds in Tomato Root Exudates
Lipid signaling and defense compounds are putatively identified in 20 dap root exudates (Figure 6 and Supplementary Table 4, Supplementary Database HPLC-MS/MS). Putative oxidized fatty acid (FA18:4+1O, m/z 293.5+) and other fatty acids (Ethyl stearate/Arachidic acid, m/z 313.7+) are increased with chitosan. On the contrary, FA18:2+3O (m/z 327.3–) is reduced with chitosan (m/z 290.5+). Stress response metabolites such as -putatively- Atropine / Hyoscyamine (A/H, m/z 290.5+) and putative citric acid (CA, m/z 191.0–) are increased with chitosan. On the contrary, 3-O′-Methyladenosine (3OM, m/z 282.7+), is slightly reduced. N-Fructosyl tyrosine (m/z 344.3+) is slightly decreased with chitosan. Putative Fructose-1,6-bisphosphate (FB, m/z 327.7–), involved in the glycolysis pathway, is increased with chitosan.
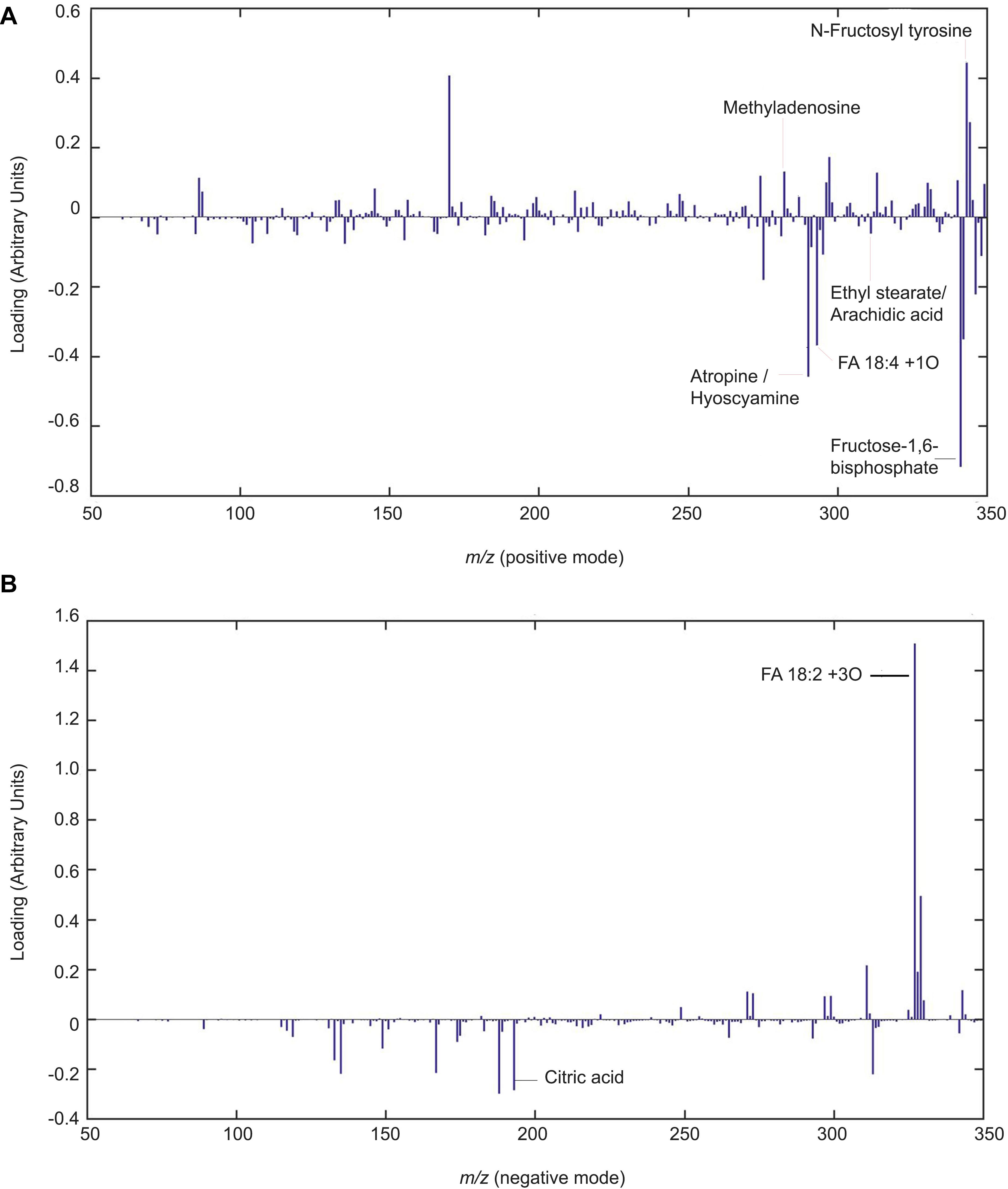
Figure 6. Chitosan induces lipid signaling and defense compounds in tomato root exudates. Partial least squares regression discriminant analysis (PLSLDA) of HPLC-ESI-MS analysis of pools of 20 days after planting tomato root exudates in modes positive (A) and negative (B). Four biological replicates per treatment are represented. Blue bars indicate a particular mass that differs from the control treatment. The larger the bar size, the more noticeable the difference in intensity of the mass compared to the control.
Root Exudates From Plants Treated With Chitosan Inhibit Soil-Borne Pathogens
Soil-borne pathogens (fungi and nematodes) are inhibited by root exudates from plants treated with chitosan. Root exudates from chitosan-treated plants cause ca. 1.5-fold reduction (p < 0.05) on hatching of the root-knot nematode Meloydogine javanica eggs after 72 h respect to tomato control root exudates (Figure 7A). These exudates also inhibit growth of Fusarium oxysporum f.sp. radicis-lycopersici (FORL) ca. twofold respect to controls (Figure 7B). The chitosan resistant fungus Pochonia chlamydosporia strain 123 (Pc) does not show significant differences in growth with both exudates over time (Figure 7C).
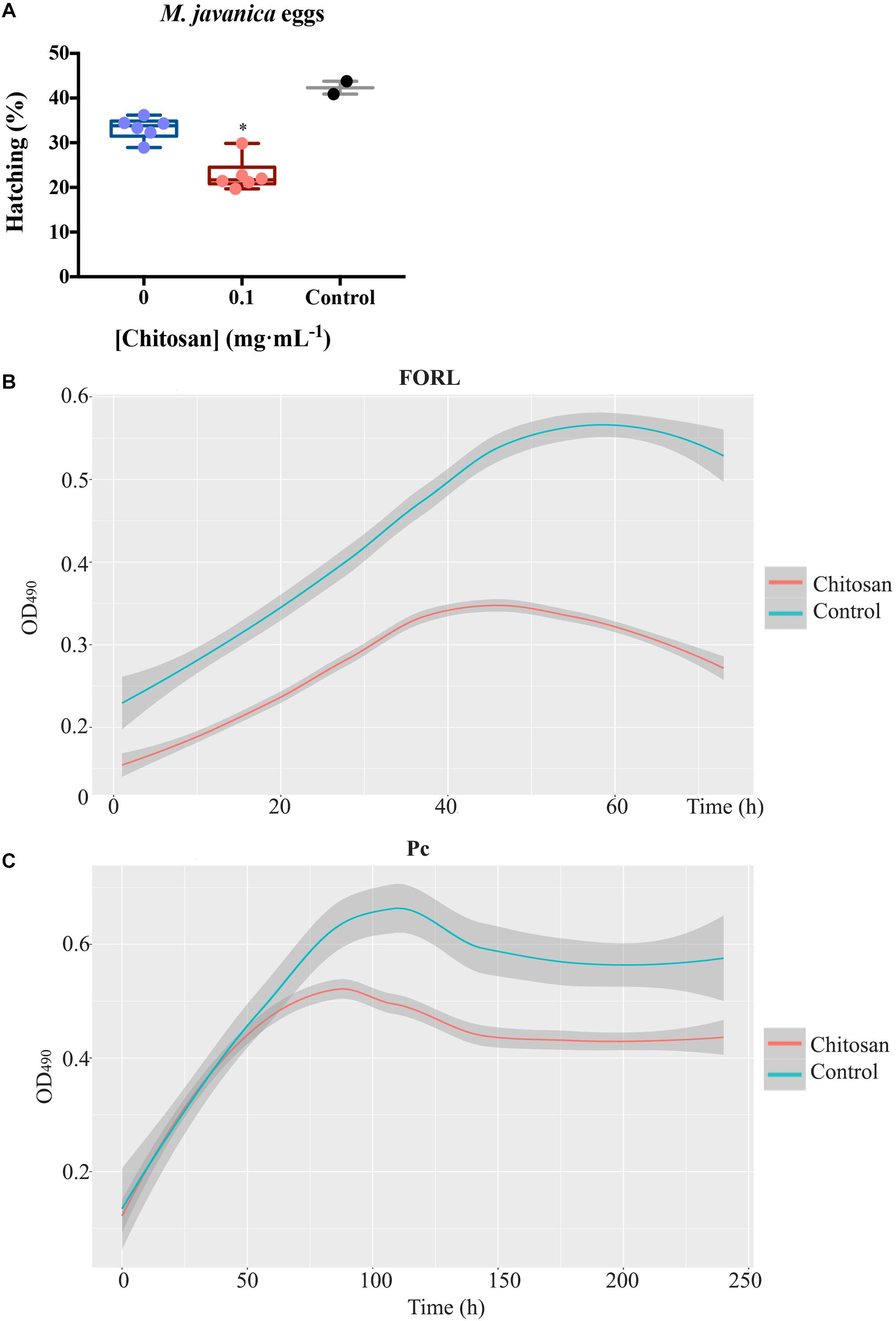
Figure 7. Root exudates from plants treated with chitosan inhibit soil-borne pathogens. (A) Effect on hatching of Meloidogyne javanica eggs in tomato root exudates. Egg hatching is reduced ca. 1.5-fold. Multifactorial analysis Kruskal–Wallis was performed to compare treatments [p-values 0.05 (*), 0.01 (**), 0.001 (***), and 0.0001 (****)] Control is egg hatching in water, 0 corresponds to untreated tomato root exudates. (B) Growth kinetics of FORL in root exudates, chitosan inhibits ca. twofold growth. C, Growth kinetics of P. chlamydosporia strain 123 in tomato root exudates. Smoother Models were adjusted with band 0.75 and degree 2. Shadow area stablishes the prediction confidence intervals at 95%.
Discussion
Chitosan has a well stablished role as a plant defense activator (El Hadrami et al., 2010). In this study we give evidences that chitosan induces plant defenses in tomato root exudates. Plasma membranes are likely to be a main target of this molecule (Palma-Guerrero et al., 2010; Meisrimler et al., 2011; Jaime et al., 2012). In this work, we show that increasing chitosan concentrations depolarize tomato root cell plasma membrane. He et al. (2009) proposed chitosan as a potential cell penetration enhancer. This fact has been demonstrated using vital staining in fungi (Palma-Guerrero et al., 2010) and, in this work, in root apices. These effects of chitosan on roots are likely to modify rhizodeposition (Pitta-Alvarez and Giulietti, 1999). Chitosan membrane depolarization may trigger an increase of reactive oxygen species (ROS) in the plant cell, generating secondary metabolites (Pandey, 2017). ROS are known to alter chemical components of the cell and lipids in particular. We have found putative FA18:4+1O and Ethyl stearate/Arachidic acid increase in exudates from roots treated with chitosan. These fatty acids could have been released from cell membranes by the oxidative stress produced by chitosan in plants (Lopez-Moya et al., 2017). These long chain fatty acids could be involved in the production of oxylipins (Noverr et al., 2003). Some oxylipins, such as oxophytodienoic acid (OPDA), are JA precursors (Dave and Graham, 2012). This could explain our finding of JA increase in root exudates from chitosan treated plants. Oxylipins other than jasmonates are probably also essential for the resistance of plants to pathogens (Blée, 2002). Putative alkaloids and CA are also increased with chitosan. These molecules have been shown to mediate stress in plant cell responses (Moharrami et al., 2017; Mallhi et al., 2019). The increase of fructose-1,6-bisphosphate in root exudates from plants treated with chitosan could reflect an extra consumption of glucose in root cells in response to chitosan induced ROS increase (Cao et al., 2020). Finally, the slight reduction of Methyladenosine in root exudates from chitosan-treated plants, could indicate that this polymer is perhaps affecting gene expression in roots (Zheng et al., 2020).
Importance of chitosan in plant hormone production and systemic acquired resistance has been widely demonstrated (Mika et al., 2010; Colman et al., 2019; Fooladi vanda et al., 2019; Iglesias et al., 2019; Ma et al., 2019). We have found that increasing chitosan leads to the accumulation of fluorescence compounds corresponding to phenolics, as well as hormones related to plant growth and defense (JA, SA, ABA, and IAA). This correlation between chitosan and phenolics has been previously studied (Pitta-Alvarez and Giulietti, 1999; Park et al., 2019; Jaisi and Panichayupakaranant, 2020; Samari et al., 2020). Chitosan enhances metabolic pathways (e.g., phenylpropanoid) involved in the biosynthesis of phenolic compounds (Asgari-Targhi et al., 2018; Fooladi vanda et al., 2019; Singh et al., 2020). A low dose of chitosan enhances plant immunity via plant hormone (JA and SA, mainly) accumulation in root tissues (Lopez-Moya et al., 2017; Iglesias et al., 2019; Singh et al., 2020). However, this effect has not yet been detected in tomato root exudates. In addition, there is evidence that chitosan could be used as a substitute for commonly used growth factors such as methyl jasmonate, auxins, or cytokinins (Cui et al., 2012; Sivanandhan et al., 2012; Ahmad et al., 2019; Acemi, 2020) due to its elicitor effects (Salachna and Zawadzińska, 2014; Malerba and Cerana, 2016). Phytomelatonin has been recently considered a plant master regulator involved in abiotic and biotic stress responses. In our work, exposure of tomato roots to increasing chitosan doses accumulates phytomelatonin and hormones in roots and root exudates in a typical biotic stress response (Arnao and Hernández-Ruiz, 2019; Moustafa-Farag et al., 2019). The decrease in levels of some plant hormones at toxic chitosan doses has also been found for phenolics (Park et al., 2019). EEM Fluorescence signatures corresponding to IAA (Li et al., 2009) and SA (Miles and Schenk, 1970) are increased by chitosan in tomato root exudates. These hormones are known to be increased by chitosan (Lopez-Moya et al., 2017; Fooladi vanda et al., 2019). SA and JA are related to plant responses to stress (War et al., 2011). Therefore, chitosan can be an elicitor of plant defenses (Benhamou, 1996) in root exudates. Our bioassays show that chitosan induces root exudates inhibitory to root pathogenic fungus FORL and root-knot nematode eggs without significantly affecting the growth and development of a biocontrol fungus (Pc). The toxic effect of chitosan-derived exudates may be related to the overproduction of SA which, in combination with chitosan induce systemic acquired resistance and reduce infection by root-knot nematodes (Vasyukova et al., 2003; Singh et al., 2020). Chitosan, moreover, by itself, is toxic to fungi such as Fusarium spp. (Palma-Guerrero et al., 2010; Al-Hetar et al., 2011). Other studies show that plants treated with chitosan are protected from FORL infection (Benhamou and Thériault, 1992).
Chitosan depolarizes plasma membrane of tomato root cells, causing the secretion of hormones, lipid signaling and plant defense compounds, including phenolics. This process affects cell viability and rhizodeposition, so plant age must be considered before applying chitosan, as well as the duration of applications. Further studies should test chitosan derived exudates under in vivo conditions to determine their efficacy to prevent the proliferation of root pathogens and subsequently reduce disease severity. Overall, our results have proven that root exudates of chitosan-treated plants are capable to reduce soil-borne pathogens growth by in vitro. This makes chitosan a promising agent to prevent and manage pests and diseases in a sustainable way.
Data Availability Statement
The datasets presented in this study can be found in online repositories. The names of the repository/repositories and accession number(s) can be found below: https://datadryad.org/stash/share/iQ4Fh9XTzpF5Rbsgz9dakjCP2BfvpSigHxhhuy57GY.
Author Contributions
LVL-L conceived the original screening and research plans and wrote the manuscript. MS-F performed the main experiments and wrote the manuscript. FCM-E analyzed the fluorescence, 1H NMR and HPLC-MS data. FL-M supervised the experiments and writing. MBA provided technical assistance to phytomelatonin detection experiments. FC-E provided technical assistance to 1H NMR experiments. MJN performed the time series analyses. BG performed electrophysiological and vital staining experiments. MS-F agrees to serve as the author responsible for contact and ensures communication. All authors contributed to the article and approved the submitted version.
Funding
This work was supported by AGL 2015 66833-R Grant from the Spanish Ministry of Economy and Competitiveness and H2020 MUSA 727624 European Project.
Conflict of Interest
The authors declare that the research was conducted in the absence of any commercial or financial relationships that could be construed as a potential conflict of interest.
Acknowledgments
Thanks are due to members of the Plant Pathology Laboratory of the University of Alicante, for their help and support. Authors also wish to thank Mr. Arnau Hernández Royo for his help conducting electrophysiological and microscopy experiments. This manuscript has been released as a pre-print at bioRXiv (Suarez-Fernandez et al., 2020).
Supplementary Material
The Supplementary Material for this article can be found online at: https://www.frontiersin.org/articles/10.3389/fpls.2020.572087/full#supplementary-material
Footnotes
- ^ www.ibmcp.upv.es
- ^ https://hmdb.ca/
- ^ https://massbank.eu/MassBank/
- ^ http://www.bmrb.wisc.edu/metabolomics/
- ^ https://www.graphpad.com/
- ^ http://wis.kuleuven.be/stat/robust/software
References
Acemi, A. (2020). Chitosan versus plant growth regulators: a comparative analysis of their effects on in vitro development of Serapias vomeracea (Burm.f.) Briq. Plant Cell Tiss Organ. Cult. 141, 327–338. doi: 10.1007/s11240-020-01789-3
Ahmad, Z., Shahzad, A., and Sharma, S. (2019). Chitosan versus yeast extract driven elicitation for enhanced production of fragrant compound 2-hydroxy-4-methoxybenzaldehyde (2H4MB) in root tuber derived callus of Decalepis salicifolia (Bedd. ex Hook.f.) Venter. Plant Cell Tiss Organ. Cult. 136, 29–40. doi: 10.1007/s11240-018-1488-4
Alberts, B., Johnson, A., Lewis, J., Raff, M., Roberts, K., and Walter, P. (2002). Ion Channels and the Electrical Properties of Membranes. Molecular Biology of the Cell, 4th Edn. Available online at: https://www.ncbi.nlm.nih.gov/books/NBK26910/ (accessed March 19, 2020)
Al-Hetar, M. Y., Zainal Abidin, M. A., Sariah, M., and Wong, M. Y. (2011). Antifungal activity of chitosan against Fusarium oxysporum f. sp. cubense. J. Appl. Polym. Sci. 120, 2434–2439. doi: 10.1002/app.33455
Arnao, M. B., and Hernández-Ruiz, J. (2018). Melatonin and its relationship to plant hormones. Ann. Bot. 121, 195–207. doi: 10.1093/aob/mcx114
Arnao, M. B., and Hernández-Ruiz, J. (2019). Melatonin: a new plant hormone and/or a plant master regulator? Trends Plant Sci. 24, 38–48. doi: 10.1016/j.tplants.2018.10.010
Arnao, M. B., and Hernández-Ruiz, J. (2007). Melatonin promotes adventitious- and lateral root regeneration in etiolated hypocotyls of Lupinus albus L. J. Pineal Res. 42, 147–152. doi: 10.1111/j.1600-079X.2006.00396.x
Asami, T., and Nakagawa, Y. (2018). Preface to the special issue: brief review of plant hormones and their utilization in agriculture. Pestic. Sci. 43, 154–158. doi: 10.1584/jpestics.M18-02
Asgari-Targhi, G., Iranbakhsh, A., and Ardebili, Z. O. (2018). Potential benefits and phytotoxicity of bulk and nano-chitosan on the growth, morphogenesis, physiology, and micropropagation of Capsicum annuum. Plant Physiol. Biochem. 127, 393–402. doi: 10.1016/j.plaphy.2018.04.013
Bais, H. P., Weir, T. L., Perry, L. G., Gilroy, S., and Vivanco, J. M. (2006). The role of root exudates in rhizosphere interactions with plants and other organisms. Annu. Rev. Plant Biol. 57, 233–266. doi: 10.1146/annurev.arplant.57.032905.105159
Baldrian, P. (2019). The known and the unknown in soil microbial ecology. FEMS Microbiol. Ecol. 95:fiz005. doi: 10.1093/femsec/fiz005
Benhamou, N. (1996). Elicitor-induced plant defence pathways. Trends Plant Sci. 1, 233–240. doi: 10.1016/1360-1385(96)86901-9
Benhamou, N., and Thériault, G. (1992). Treatment with chitosan enhances resistance of tomato plants to the crown and root rot pathogen Fusarium oxysporum f. sp. radicis-lycopersici. Physiol. Mol. Plant Pathol. 41, 33–52. doi: 10.1016/0885-5765(92)90047-Y
Blée, E. (2002). Impact of phyto-oxylipins in plant defense. Trends Plant Sci. 7, 315–322. doi: 10.1016/S1360-1385(02)02290-2
Cao, B., Ma, Q., and Xu, K. (2020). Silicon restrains drought-induced ROS accumulation by promoting energy dissipation in leaves of tomato. Protoplasma 257, 537–547. doi: 10.1007/s00709-019-01449-0
Cervilla, L. M., Rosales, M. A., Rubio-Wilhelmi, M. M., Sánchez-Rodríguez, E., Blasco, B., Ríos, J. J., et al. (2009). Involvement of lignification and membrane permeability in the tomato root response to boron toxicity. Plant Sci. 176, 545–552. doi: 10.1016/j.plantsci.2009.01.008
Cleveland, W. S. (1979). Robust locally weighted regression and smoothing scatterplots. J. Am. Stat. Assoc. 74, 829–836. doi: 10.1080/01621459.1979.10481038
Colman, S. L., Salcedo, M. F., Mansilla, A. Y., Iglesias, M. J., Fiol, D. F., Martín-Saldaña, S., et al. (2019). Chitosan microparticles improve tomato seedling biomass and modulate hormonal, redox and defense pathways. Plant Physiol. Biochem. 143, 203–211. doi: 10.1016/j.plaphy.2019.09.002
Cui, L., Wang, Z.-Y., and Zhou, X.-H. (2012). Optimization of elicitors and precursors to enhance valtrate production in adventitious roots of Valeriana amurensis Smir. ex Kom. Plant Cell Tiss. Organ. Cult. 108, 411–420. doi: 10.1007/s11240-011-0052-2
Dave, A., and Graham, I. A. (2012). Oxylipin signaling: a distinct role for the jasmonic acid precursor cis-(+)-12-Oxo-phytodienoic acid (cis-OPDA). Front. Plant Sci. 3:42. doi: 10.3389/fpls.2012.00042
El Hadrami, A., Adam, L. R., El Hadrami, I., and Daayf, F. (2010). Chitosan in plant protection. Mar. Drugs 8, 968–987. doi: 10.3390/md8040968
Elieh-Ali-Komi, D., and Hamblin, M. R. (2016). Chitin and chitosan: production and application of versatile biomedical nanomaterials. Int. J. Adv. Res. 4, 411–427.
El-Tantawy, E. M. (2009). Behavior of tomato plants as affected by spraying with chitosan and aminofort as natural stimulator substances under application of soil organic amendments. Pak. J. Biol. Sci. 12, 1164–1173. doi: 10.3923/pjbs.2009.1164.1173
Escudero, N., Marhuenda-Egea, F. C., Ibanco-Cañete, R., Zavala-Gonzalez, E. A., and Lopez-Llorca, L. V. (2014). A metabolomic approach to study the rhizodeposition in the tritrophic interaction: tomato, Pochonia chlamydosporia and Meloidogyne javanica. Metabolomics 10, 788–804. doi: 10.1007/s11306-014-0632-3
FAO (2017). Good Agricultural Practices for Greenhouse Vegetable Production in the South East European Countries. Rome: FAO. ISSN 2070-2515. Plant Production and Protection Paper 230.
Flores, H. E., Weber, C., and Puffett, J. (1996). “Underground metabolism: the biosynthetic potential of roots,” in Roots: The Hidden Half 2nd edn., ed. Y. Waisel (New York: Marcel Dekker Inc.), 931–956.
Fooladi vanda, G., Shabani, L., and Razavizadeh, R. (2019). Chitosan enhances rosmarinic acid production in shoot cultures of Melissa officinalis L. through the induction of methyl jasmonate. Bot. Stud. 60:26. doi: 10.1186/s40529-019-0274-x
Fürstenberg-Hägg, J., Zagrobelny, M., and Bak, S. (2013). Plant defense against insect herbivores. Int. J. Mol. Sci. 14, 10242–10297. doi: 10.3390/ijms140510242
Gamborg, O. L., Miller, R. A., and Ojima, K. (1968). Nutrient requirements of suspension cultures of soybean root cells. Exp. Cell Res. 50, 151–158. doi: 10.1016/0014-4827(68)90403-5
Gunsé, B., Poschenrieder, C., Rankl, S., Schröeder, P., Rodrigo-Moreno, A., and Barceló, J. (2016). A highly versatile and easily configurable system for plant electrophysiology. MethodsX 3, 436–451. doi: 10.1016/j.mex.2016.05.007
He, W., Guo, X., Xiao, L., and Feng, M. (2009). Study on the mechanisms of chitosan and its derivatives used as transdermal penetration enhancers. Int. J. Pharm. 382, 234–243. doi: 10.1016/j.ijpharm.2009.07.038
Hernández-Ruiz, J., and Arnao, M. B. (2008). Distribution of melatonin in different zones of lupin and barley plants at different ages in the presence and absence of light. J. Agric. Food Chem. 56, 10567–10573. doi: 10.1021/jf8022063
Hernández-Ruiz, J., Cano, A., and Arnao, M. B. (2005). Melatonin acts as a growth-stimulating compound in some monocot species: melatonin as growth promoter in plants. J. Pineal Res. 39, 137–142. doi: 10.1111/j.1600-079X.2005.00226.x
Hirsch, A. M., Bauer, W. D., Bird, D. M., Cullimore, J., Tyler, B., and Yoder, J. I. (2003). Molecular signals and receptors: controlling rhizosphere interactions between plants and other organisms. Ecology 84, 858–868.
Hupp, S., Rosenkranz, M., Bonfig, K., Pandey, C., and Roitsch, T. (2019). Noninvasive phenotyping of plant–pathogen interaction: consecutive In Situ Imaging of Fluorescing Pseudomonas syringae, plant phenolic fluorescence, and chlorophyll fluorescence in Arabidopsis leaves. Front. Plant Sci. 10:1239. doi: 10.3389/fpls.2019.01239
Iglesias, M. J., Colman, S. L., Terrile, M. C., París, R., Martín-Saldaña, S., Chevalier, A. A., et al. (2019). Enhanced properties of chitosan microparticles over bulk chitosan on the modulation of the auxin signaling pathway with beneficial impacts on root architecture in plants. J. Agric. Food Chem. 67, 6911–6920. doi: 10.1021/acs.jafc.9b00907
Iriti, M., and Faoro, F. (2008). Abscisic acid is involved in chitosan-induced resistance to tobacco necrosis virus (TNV). Plant Physiol. Biochem. 46, 1106–1111. doi: 10.1016/j.plaphy.2008.08.002
Jaime, M. D., Lopez-Llorca, L., Conesa, A., Lee, A. Y., Proctor, M., Heisler, L. E., et al. (2012). Identification of yeast genes that confer resistance to chitosan oligosaccharide (COS) using chemogenomics. BMC Genomics 13:267. doi: 10.1186/1471-2164-13-267
Jaisi, A., and Panichayupakaranant, P. (2020). Enhanced plumbagin production in Plumbago indica root culture by simultaneous and sequential dual elicitations using chitosan with L-alanine and methyl-β-cyclodextrin. Bioresour. Bioprocess. 7:10. doi: 10.1186/s40643-020-0298-9
Jones, K. H., and Senft, J. A. (1985). An improved method to determine cell viability by simultaneous staining with fluorescein diacetate-propidium iodide. J. Histochem Cytochem. 33, 77–79. doi: 10.1177/33.1.2578146
Kaur, S., and Dhillon, G. S. (2014). The versatile biopolymer chitosan: potential sources, evaluation of extraction methods and applications. Crit. Rev. Microbiol. 40, 155–175. doi: 10.3109/1040841X.2013.770385
Kumar, M. N. V. (2000). A review of chitin and chitosan applications. React. Funct. Polym. 46, 1–27. doi: 10.1016/S1381-5148(00)00038-9
Li, Y.-N., Wu, H.-L., Zhu, S.-H., Nie, J.-F., Yu, Y.-J., Wang, X.-M., et al. (2009). Determination of indole-3-acetic acid in soil using excitation-emission matrix fluorescence with trilinear decomposition-based calibration methods. Anal. Sci. 25, 83–88. doi: 10.2116/analsci.25.83
Lopez-Moya, F., Escudero, N., Zavala-Gonzalez, E. A., Esteve-Bruna, D., Blázquez, M. A., Alabadí, D., et al. (2017). Induction of auxin biosynthesis and WOX5 repression mediate changes in root development in Arabidopsis exposed to chitosan. Sci. Rep. 7:16813. doi: 10.1038/s41598-017-16874-5
Ma, B., Wang, J., Liu, C., Hu, J., Tan, K., Zhao, F., et al. (2019). Preventive effects of fluoro-substituted benzothiadiazole derivatives and chitosan oligosaccharide against the rice seedling blight induced by Fusarium oxysporum. Plants 8:538. doi: 10.3390/plants8120538
Malerba, M., and Cerana, R. (2016). Chitosan effects on plant systems. Int. J. Mol. Sci. 17:996. doi: 10.3390/ijms17070996
Mallhi, Z. I., Rizwan, M., Mansha, A., Ali, Q., Asim, S., Ali, S., et al. (2019). Citric acid enhances plant growth, photosynthesis, and phytoextraction of lead by alleviating the oxidative stress in castor beans. Plants 8:525. doi: 10.3390/plants8110525
Mandal, S. M., Chakraborty, D., and Dey, S. (2010). Phenolic acids act as signaling molecules in plant-microbe symbioses. Plant Signal. Behav. 5, 359–368.
Marhuenda-Egea, F. C., Gonsálvez-Álvarez, R. D., Lledó-Bosch, B., Ten, J., and Bernabeu, R. (2013). New approach for chemometric analysis of mass spectrometry data. Anal. Chem. 85, 3053–3058. doi: 10.1021/ac303255h
Matzke, A. J. M., and Matzke, M. (2013). Membrane “potential-omics”: toward voltage imaging at the cell population level in roots of living plants. Front. Plant Sci. 4:311. doi: 10.3389/fpls.2013.00311
McClure, M. A., Kruk, T. H., and Misaghi, I. (1973). A method for obtaining quantities of clean meloidogyne eggs. J. Nematol. 5:230.
Meisrimler, C.-N., Planchon, S., Renaut, J., Sergeant, K., and Lüthje, S. (2011). Alteration of plasma membrane-bound redox systems of iron deficient pea roots by chitosan. J. Proteomics 74, 1437–1449. doi: 10.1016/j.jprot.2011.01.012
Mika, A., Boenisch, M. J., Hopff, D., and Lüthje, S. (2010). Membrane-bound guaiacol peroxidases from maize (Zea mays L.) roots are regulated by methyl jasmonate, salicylic acid, and pathogen elicitors. J. Exp. Bot. 61, 831–841. doi: 10.1093/jxb/erp353
Miles, C. I., and Schenk, G. H. (1970). Fluorescence of acetylsalicylic acid in solution and its measurement in presence of salicylic acid. Anal. Chem. 42, 656–659. doi: 10.1021/ac60288a032
Mobed, J. J., Hemmingsen, S. L., Autry, J. L., and McGown, L. B. (1996). Fluorescence characterization of IHSS humic substances: total luminescence spectra with absorbance correction. Environ. Sci. Technol. 30, 3061–3065. doi: 10.1021/es960132l
Moharrami, F., Hosseini, B., Sharafi, A., and Farjaminezhad, M. (2017). Enhanced production of hyoscyamine and scopolamine from genetically transformed root culture of Hyoscyamus reticulatus L. elicited by iron oxide nanoparticles. In Vitro Cell Dev. Biol. Plant 53, 104–111. doi: 10.1007/s11627-017-9802-0
Mostofa, K. M. G., Yoshioka, T., Mottaleb, A., and Vione, D. (eds) (2013). Photobiogeochemistry of Organic Matter: Principles and Practices in Water Environments. Berlin: Springer-Verlag, doi: 10.1007/978-3-642-32223-5
Moustafa-Farag, M., Almoneafy, A., Mahmoud, A., Elkelish, A., Arnao, M. B., Li, L., et al. (2019). Melatonin and its protective role against biotic stress impacts on plants. Biomolecules 10:54. doi: 10.3390/biom10010054
Noverr, M. C., Erb-Downward, J. R., and Huffnagle, G. B. (2003). Production of eicosanoids and other oxylipins by pathogenic eukaryotic microbes. Clin. Microbiol. Rev. 16, 517–533. doi: 10.1128/cmr.16.3.517-533.2003
Ohno, T., and Bro, R. (2006). Dissolved organic matter characterization using multiway spectral decomposition of fluorescence landscapes. Soil Sci. Soc. Am. J. 70, 2028–2037. doi: 10.2136/sssaj2006.0005
Olivares, C. M., and López-Llorca, L. V. (2002). Fungal egg-parasites of plant-parasitic nematodes from Spanish soils. Rev. Iberoam. Micol. 19, 104–110.
Palma-Guerrero, J., Jansson, H.-B., Salinas, J., and Lopez-Llorca, L. V. (2007). Effect of chitosan on hyphal growth and spore germination of plant pathogenic and biocontrol fungi. J. Appl. Microbiol 104, 541–553. doi: 10.1111/j.1365-2672.2007.03567.x
Palma-Guerrero, J., Lopez-Jimenez, J. A., Pérez-Berná, A. J., Huang, I.-C., Jansson, H.-B., Salinas, J., et al. (2010). Membrane fluidity determines sensitivity of filamentous fungi to chitosan. Mol. Microbiol. 75, 1021–1032. doi: 10.1111/j.1365-2958.2009.07039.x
Pandey, G. K. (2017). Mechanism of Plant Hormone Signaling Under Stress. Hoboken, NJ: John Wiley & Sons.
Park, C. H., Yeo, H. J., Park, Y. E., Chun, S. W., Chung, Y. S., Lee, S. Y., et al. (2019). Influence of chitosan, salicylic acid and jasmonic acid on phenylpropanoid accumulation in germinated buckwheat (Fagopyrum esculentum Moench). Foods 8:153. doi: 10.3390/foods8050153
Parri, E., Santinami, G., and Domenici, V. (2020). Front-face fluorescence of honey of different botanic origin: a case study from tuscany (Italy). Appl. Sci. 10:1776. doi: 10.3390/app10051776
Pitta-Alvarez, S. I., and Giulietti, A. M. (1999). Influence of chitosan, acetic acid an citric acid on growth and tropane alkaloid production in transformed roots of Brugmansia candida – Effect of medium pH and growth phase. Plant Cell Tiss. Org. 59, 31–38. doi: 10.1023/A:1006359429830
Rodrigo-Moreno, A., Andrés-Colás, N., Poschenrieder, C., Gunsé, B., Peñarrubia, L., and Shabala, S. (2013). Calcium- and potassium-permeable plasma membrane transporters are activated by copper in Arabidopsis root tips: linking copper transport with cytosolic hydroxyl radical production: copper transport and hydroxyl radical production. Plant Cell Environ. 36, 844–855. doi: 10.1111/pce.12020
Salachna, P., and Zawadzińska, A. (2014). Effect of chitosan on plant growth, flowering and corms yield of potted. J. Ecol. Eng. 15, 97–102. doi: 10.12911/22998993.1110223
Samari, E., Sharifi, M., Ghanati, F., Fuss, E., and Ahmadian Chashmi, N. (2020). Chitosan-induced phenolics production is mediated by nitrogenous regulatory molecules: NO and PAs in Linum album hairy roots. Plant Cell Tiss. Organ. Cult. 140, 563–576. doi: 10.1007/s11240-019-01753-w
Seid, A., Fininsa, C., Mekete, T., Decraemer, W., and Wesemael, W. M. L. (2015). Tomato (Solanum lycopersicum) and root-knot nematodes (Meloidogyne spp.) – a century-old battle. Nematology 17, 995–1009. doi: 10.1163/15685411-00002935
Seo, M., Jikumaru, Y., and Kamiya, Y. (2011). “Profiling of hormones and related metabolites in seed dormancy and germination studies,” in Seed Dormancy Methods in Molecular Biology, ed. A. R. Kermode (Totowa, NJ: Humana Press), 99–111. doi: 10.1007/978-1-61779-231-1_7
Singh, M., Poddar, N. K., Singh, D., and Agrawal, S. (2020). Foliar application of elicitors enhanced the yield of withanolide contents in Withania somnifera (L.) Dunal (variety. Poshita). 3 Biotech 10:157. doi: 10.1007/s13205-020-2153-2
Singh, V. K., Singh, A. K., and Kumar, A. (2017). Disease management of tomato through PGPB: current trends and future perspective. 3 Biotech 7:255. doi: 10.1007/s13205-017-0896-1
Sivanandhan, G., Arun, M., Mayavan, S., Rajesh, M., Mariashibu, T. S., Manickavasagam, M., et al. (2012). Chitosan enhances withanolides production in adventitious root cultures of Withania somnifera (L.) Dunal. Ind. Crop. Prod. 37, 124–129. doi: 10.1016/j.indcrop.2011.11.022
Street, K. W., and Schenk, G. H. (1981). Spectrofluorometric determination of acetylsalicylic acid, salicylamide, and salicyclic acid as an impurity in pharmaceutical preparations. J. Pharm. Sci. 70, 641–646. doi: 10.1002/jps.2600700617
Suarez-Fernandez, M., Marhuenda-Egea, F. C., Lopez-Moya, F., Arnao, M. B., Cabrera-Escribano, F., Nueda, M. J., et al. (2020). Chitosan induces plant hormones and defences in tomato root exudates. bioRxiv [Preprint]. doi: 10.1101/2020.06.09.142653
Valette, M., Rey, M., Gerin, F., Comte, G., and Wisniewski-Dyé, F. (2020). A common metabolomic signature is observed upon inoculation of rice roots with various rhizobacteria. J. Integr. Plant Biol. 62, 228–246. doi: 10.1111/jipb.12810
van Dam, N. M., and Bouwmeester, H. J. (2016). Metabolomics in the rhizosphere: tapping into belowground chemical communication. Trends Plant Sci. 21, 256–265. doi: 10.1016/j.tplants.2016.01.008
Vasyukova, N. I., Zinov’eva, S. V., Udalova, Z. V., Panina, Y. S., Ozeretskovskaya, O. L., and Sonin, M. D. (2003). The role of salicylic acid in systemic resistance of tomato to nematodes. Dokl. Biol. Sci. 391, 343–345. doi: 10.1023/A:1025158702692
Verboven, S., and Hubert, M. (2005). LIBRA: a MATLAB library for robust analysis. Chemom. Intell. Lab. Syst. 75, 127–136. doi: 10.1016/j.chemolab.2004.06.003
Walker, T. S., Bais, H. P., Halligan, K. M., Stermitz, F. R., and Vivanco, J. M. (2009). Correction to metabolic profiling of root exudates of Arabidopsis thaliana. J. Agric. Food Chem. 57, 9346–9346. doi: 10.1021/jf902670b
War, A. R., Paulraj, M. G., War, M. Y., and Ignacimuthu, S. (2011). Role of salicylic acid in induction of plant defense system in chickpea (Cicer arietinum L.). Plant Signal. Behav. 6, 1787–1792. doi: 10.4161/psb.6.11.17685
Yang, G., Zhou, B., Zhang, X., Zhang, Z., Wu, Y., Zhang, Y., et al. (2016). Effects of tomato root exudates on Meloidogyne incognita. PLoS One 11:e0154675. doi: 10.1371/journal.pone.0154675
Yang, H., Xiao, X., Zhao, X., and Wu, Y. (2015). “Intrinsic fluorescence spectra of tryptophan, tyrosine and phenyloalanine,” in 5th International Conference on Advanced Design and Manufacturing Engineering, Amsterdam: Atlantis Press, doi: 10.2991/icadme-15.2015.46
Yin, H., Du, Y., and Dong, Z. (2016). Chitin oligosaccharide and chitosan oligosaccharide: two similar but different plant elicitors. Front. Plant Sci. 7:522. doi: 10.3389/fpls.2016.00522
Yuan, J., Zhang, N., Huang, Q., Raza, W., Li, R., Vivanco, J. M., et al. (2015). Organic acids from root exudates of banana help root colonization of PGPR strain Bacillus amyloliquefaciens NJN-6. Sci. Rep. 5:13438. doi: 10.1038/srep13438
Zhang, B., Weston, P. A., Gu, L., Zhang, B., Li, M., Wang, F., et al. (2019). Identification of phytotoxic metabolites released from Rehmannia glutinosa suggest their importance in the formation of its replant problem. Plant Soil 441, 439–454. doi: 10.1007/s11104-019-04136-4
Keywords: chitosan, root exudates, membrane potential, lipid signaling, plant defenses, plant hormones, soil-borne pathogens
Citation: Suarez-Fernandez M, Marhuenda-Egea FC, Lopez-Moya F, Arnao MB, Cabrera-Escribano F, Nueda MJ, Gunsé B and Lopez-Llorca LV (2020) Chitosan Induces Plant Hormones and Defenses in Tomato Root Exudates. Front. Plant Sci. 11:572087. doi: 10.3389/fpls.2020.572087
Received: 12 June 2020; Accepted: 08 October 2020;
Published: 04 November 2020.
Edited by:
Jens Rohloff, Norwegian University of Science and Technology, NorwayReviewed by:
Dayakar Venkata Badri, Hill’s Pet Nutrition, Inc., United StatesBerin A. Boughton, The University of Melbourne, Australia
Copyright © 2020 Suarez-Fernandez, Marhuenda-Egea, Lopez-Moya, Arnao, Cabrera-Escribano, Nueda, Gunsé and Lopez-Llorca. This is an open-access article distributed under the terms of the Creative Commons Attribution License (CC BY). The use, distribution or reproduction in other forums is permitted, provided the original author(s) and the copyright owner(s) are credited and that the original publication in this journal is cited, in accordance with accepted academic practice. No use, distribution or reproduction is permitted which does not comply with these terms.
*Correspondence: Marta Suarez-Fernandez, bWFydGEuc3VhcmV6QHVhLmVz; c3VhcmV6ZmVybmFuZGV6bWFydGFAZ21haWwuY29t