- 1The Botanical Garden, School of Plant Sciences, Tel Aviv University, Tel Aviv, Israel
- 2Botanic Garden, Faculty of Biology, University of Warsaw, Warsaw, Poland
Floral color plays a key role as visual signaling and is therefore of great importance in shaping plant-pollinator interactions. Iris (Iridaceae), a genus comprising over 300 species and named after the Greek goddess of the colorful rainbow, is famous for its dazzling palette of flower colors and patterns, which vary considerably both within and among species. Despite the large variation of flower color in Iris, little is known about the phylogenetic and ecological contexts of floral color. Here, we seek to resolve the evolution of flower color in the genus Iris in a macroevolutionary framework. We used a phylogenetic analysis to reconstruct the ancestral state of flower color and other pollination-related traits (e.g., the presence of nectar and mating system), and also tracked the evolution of color variation. We further explored weather floral trait transitions are better explained by environmental or pollinator-mediated selection. Our study revealed that the most recent common ancestor likely had monomorphic, purple flowers, with a crest and a spot on the fall. The flowers were likely insect-pollinated, nectar-rewarding, and self-compatible. The diversity of floral traits we see in modern irises, likely represents a trade-off between conflicting selection pressures. Whether shifts in these flower traits result from abiotic or biotic selective agents or are maintained by neutral processes without any selection remains an open question. Our analysis serves as a starting point for future work exploring the genetic and physiological mechanisms controlling flower coloration in the most color-diverse genus Iris.
Introduction
Visual floral traits, flower color in particular, are important features that shape plant interactions with the surrounding environment (Schaefer and Ruxton, 2011; Willmer, 2017). Flowers show enormous variation in color among closely related taxa, and even between or within natural populations of the same species (i.e., flower color polymorphism) (Gigord et al., 2001; Warren and Mackenzie, 2001; Narbona et al., 2018; Souto-Vilarós et al., 2018). The factors that drive flower color evolution in highly diverse genera or families, particularly within a species, remain an open area of research.
Flower color is one of the most important characters for signaling to animal pollinators (Schiestl and Johnson, 2013). Therefore, pollinators are often perceived as one of the primary selective agents influencing flower color. Differences in the visual capabilities of pollinators can impose variable selective pressure on flower color, leading to variation (Wester and Lunau, 2017; de Camargo et al., 2019). In some plant species color transitions represent an adaptation to different, sometimes new, suites of pollinators (Armbruster et al., 2000; Fenster et al., 2004). For instance, in some plant genera, color is the best predictor of a transition between insect and bird pollination (Sutherland and Vickery, 1993; Roguz et al., 2018).
While flower color is a central visual cue to animal pollinators, the floral reward also plays a key role in shaping the interaction (Roy et al., 2017; Parachnowitsch et al., 2018; Roguz et al., 2018, 2019). Typically, plants provide a food reward of either pollen or nectar. Of these, nectar is perhaps the most important in an evolutionary sense (Simpson and Neff, 1983; Canto et al., 2011). Although it is a strong attractant, producing nectar is physiologically costly, and thus in several cases, the ability of flowers to produce nectar has been lost (Little, 1983; Dafni, 1984; Sletvold et al., 2016). In some plant families, e.g., Orchidaceae, the presence or absence of a nectar reward may be correlated with flower color. Non-rewarding, sexually deceptive orchids often have brightly colored flowers (Spaethe et al., 2010), while species with nectar often have green or white colored flowers (Duffy and Stout, 2011). The lack of a food reward might result in, or be the result of, the development of new rewarding characters that attract potential pollinators. These new attractants may include changes in flower color and size (Gigord et al., 2001; Vereecken and Schiestl, 2009). For example, night-sheltering reward systems without a food reward are often associated with large, dark flowers (Dafni et al., 1981; Sapir et al., 2005, 2006; Watts et al., 2013; Lavi and Sapir, 2015). Dark petals with their highly absorptive surfaces may result in higher temperatures inside the flower, which may benefit flower visitors (Sapir et al., 2006; Watts et al., 2013). As a result, in such systems flower size and color, but not food rewards, are typically under strong selection (Vereecken et al., 2013; Lavi and Sapir, 2015; Pellegrino et al., 2017).
Flower color changes might also be associated with a shift in plant mating system (Landis et al., 2018). The majority of flowering plants have both male and female reproductive parts, however, the mutualistic interactions with pollinators enable most of these plants to outcross (Goldberg et al., 2010). Shifts in flower color and pollination vectors may result in changes in mating system (i.e., selfing or outcrossing). Some species that rely on pollinators have lost the ability to self-fertilize, becoming self-incompatible, while in other cases the reverse occurred (Landis et al., 2018). Strong selection on floral traits, including color, is expected in self-incompatible taxa relying completely on animal-mediated pollination. Whereas in self-compatible taxa, this animal-mediated selection is expected to weaken since pollinator visitation may no longer be required for reproduction (Anderson and Busch, 2006). Thus, while pollinators often play a critical role in the evolution of flower color, this may not always be the case.
Plants experience a myriad of interactions with animals, both mutualistic and antagonistic. The strength and direction of selection that these agents exert on flower color may differ (Irwin et al., 2003). For example, flower color may have evolved as an adaptation against fungi and/or herbivores (Chalker-Scott, 1999; Chittka and Schürkens, 2001). Irwin et al. (2003) found that herbivores and pollinators exert opposing selection on flower color in Raphanus sativus. In this system, because both herbivores and pollinators prefer lighter flowers, dark flowers persist, thus maintaining a stable color polymorphism.
In some taxa, however, there is little or no association between color and interacting animals (Armbruster, 1996). Instead, variation in pigmentation within and among closely related taxa may be maintained by selection related to environmental heterogeneity (Warren and Mackenzie, 2001). The three main groups of pigments responsible for color in plants, flavonoids, carotenoids and betalains, play a functional role in plant physiology (Schaefer and Ruxton, 2011). Flavonoids, for example, are known to function as a response to plant stress caused by drought (Warren and Mackenzie, 2001), cold (Chalker-Scott, 1999; Harborne and Williams, 2000), and nitrogen deficiency (Bonguebartelsman and Phillips, 1995). They also protect plants against damage caused by UV radiation or visible light (Chalker-Scott, 1999; Tanaka et al., 2008). Flower color mediates plant interactions with both the abiotic and biotic environment, and thus exploring the drivers that lead to flower color diversity is of key importance to understand the evolution of plant diversity.
Transitions in flower color are common across the phylogenies of Angiosperm lineages (Streisfeld and Rausher, 2011; Wessinger and Rausher, 2012, 2014; Martins et al., 2016; Roguz et al., 2018). The diversity of flower color among and within these modern lineages suggests that most of these transitions must have been adaptive (Rausher, 2008). While the mutations causing flower color shifts are well understood at the biochemical level (Grotewold, 2006), the broader macro-evolutionary drivers of flower color diversity have only been studied in few plant groups (Landis et al., 2018; Ng and Smith, 2018). To understand these macro-evolutionary forces, we need to explore plant lineages exhibiting a diversity of flower colors.
The genus Iris is one of the most diversified genera in Asparagales. Iris comprises over 300 species (Royal Botanic and Gardens, 2020), which are widespread throughout the Northern Hemisphere (Goldblatt and Manning, 2008), with the greatest number of endemics occurring in the Mediterranean and Asia (Wilson, 2006). Although some irises are found in mesic or even wetland environments, most species grow in desert, semi-desert, or dry, rocky, montane habitats (Wilson, 2006). Members of this genus display a remarkable variety of flower colors, ranging from extremely dark, purple flowers, through violet and pink, to yellow and white flowers (Mathew, 1989; Sapir and Shmida, 2002; Sapir et al., 2002). This dazzling palette of colors and patterns seen in Iris flowers may be associated with their wide variety of life histories, pollination and mating systems, and habitats. Thus irises represent an outstanding model to study evolutionary biology and speciation in plants, especially in the context of flower color (Crespo et al., 2015).
To understand the unusual flower colors and color patterns in irises we investigated the evolution of flower color and several related traits, including pollinator type, nectar reward, and mating system, across the entire phylogeny and geographic range of the genus. By determining the ancestral state of Iris traits and comparing it to the traits of modern species, we should be able to shed light on which traits are the causes and which are the effects. To this end, we asked the following specific questions, first, what was the ancestral state of flower color and related traits in Iris, and second, are floral trait transitions from ancestral to modern states better explained by environmental or pollinator mediated selection?
Materials and Methods
Phylogenetic Tree
To set up our analyses on the evolution of flower color in irises, we first created a phylogenetic tree for the genera, based on a database of sequences. We created the database using six sequences, five plastid genomes (matK, trnL, trnK, NADPH, and rbcL) and one nuclear internal transcribed spacer (ITS). All of the gene sequences were acquired from GenBank (Accession numbers in Supplementary Material 1) and downloaded using the MatPhylobi program (Kranas et al., 2018), which is a command-line tool for constructing taxonomic data sets for phylogenetic inference based on NCBI data. The sequences downloaded represent 227 Iris taxa which include 215 species, 10 subspecies and two varieties (see full list and ranks in Supplementary Material 1). Our analysis included all taxa with data available in GenBank. This taxa sampling covers the floral and geographic diversity of the genus. To create the phylogenetic database in MatPhylobi, we seeded Iris pumila as the representative Iris species and selected Crocus vernus, Morea inclinata, and Dietes robinsoniana as outgroups, based on their previously established sister relationships (Goldblatt et al., 2002; Wilson, 2006). Overall, taxon sampling totalled 429 accessions and total gene coverage was approximately 53.7% (227 Iris taxa out of 431), with matK having the highest coverage (40.7%) and ITS the lowest coverage (9.95%).
To refine the database, all sequences were independently aligned using the multiple alignment program MAFFT (version 7; Kuraku et al., 2013; Katoh et al., 2017) (method = “localpair,” incorporating local pairwise alignment information, maxiterate = 1,000). Subsequently, we imported all of the alignments into Mesquite for visual inspection (version 3.6; Maddison and Maddison, 2018). Poorly aligned positions and divergent regions were eliminated using the Gblocks program (Version 0.91b, Castresana, 2000; Talavera and Castresana, 2007). The trimmed alignments were then concatenated with catfasta2phyml into a single aggregate alignment1. For each sequence, we selected the appropriate evolutionary model based on its specific characteristics using ModelTest-NG (version 0.1.3; Darriba et al., 2020; Supplementary Material 2).
Using our refined database of sequences, we used RAxML to generate our final phylogenetic tree. RAxML uses a series of maximum-likelihood (ML) tests to generate the tree (version 8.0; Stamatakis, 2014). To find the best phylogenetic tree, we used a bootstrap analysis with 1,000 replicates. Only bootstrap values (i.e., the probability that respective groups of taxa are present in the true phylogeny) higher than 50% were presented on the tree.
Floral and Habitat Characters
Once we had our phylogenetic tree, we prepared a database describing the diversity of irises, including 16 traits related to flower color, reproduction, habitat and distribution (Table 1). A majority of these traits were determined using Mathew (1989), which is a compilation of information about irises around the world. Additional sources included regional floras, the scientific literature, as well as internet sources2 3. Table with all data available in Supplementary Table 1.
Iris flowers have six sepals, which are usually divided into two types: three falls that droop downwards and three standards that are upright. These two sepal-types often have distinct characteristics (Figure 1).
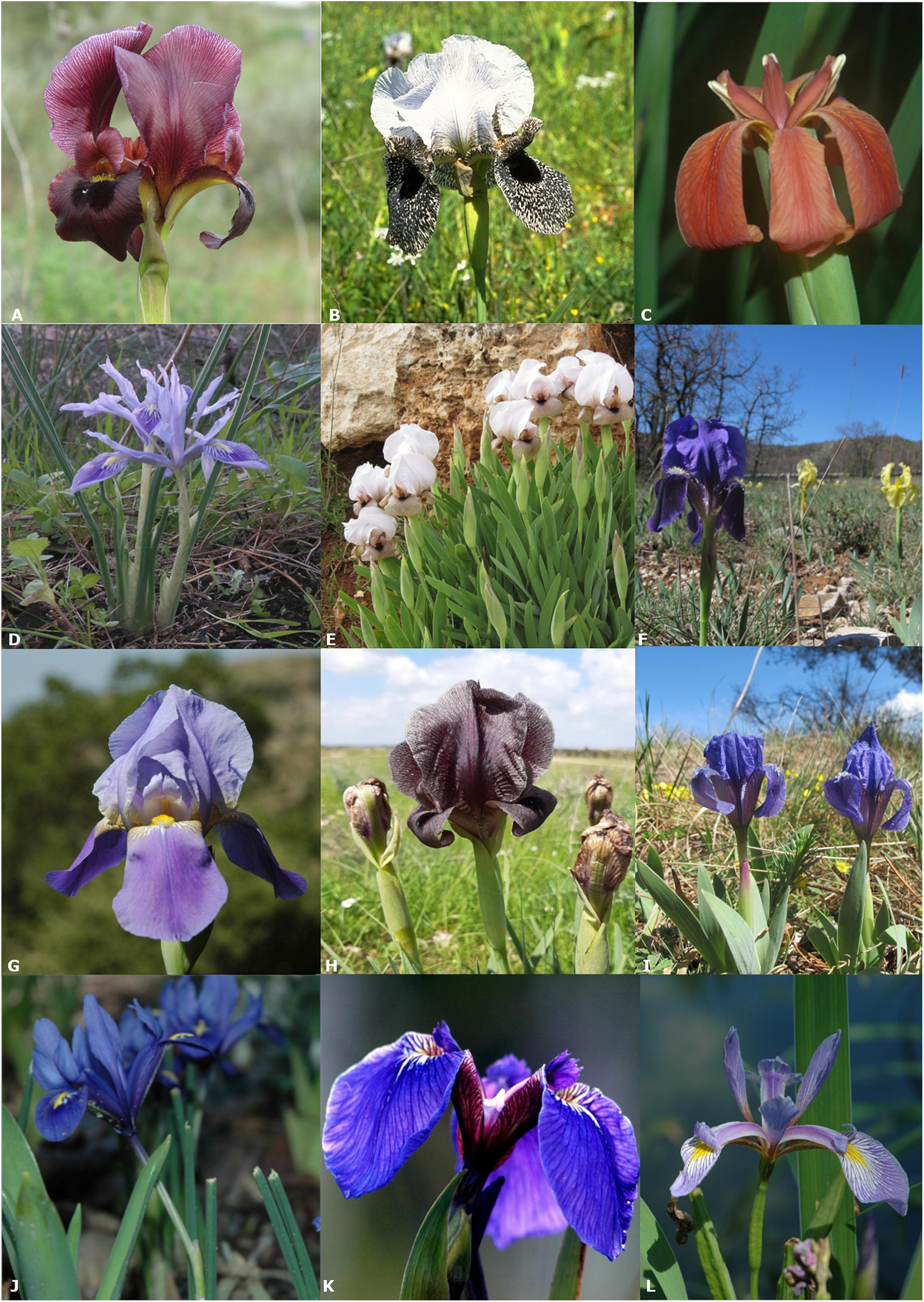
Figure 1. Flowers of selected Iris species [(A) I. atropurpurea, (B) I. bismarckiana, (C) I. fulva, (D) I. historio, (E) I. loretti, (F) I. lutescens, (G) I. mesopotamica, (H) I. petrana, (I) I. pumila, (J) I. reticulata, (K) I. setosa (L) I. virginica].
We assessed flower color and eight color-related traits. Because of the wide range of taxa and variation in literature sources regarding those taxa, our first color metric was a simple assessment of flower tepals color based on human perception. Although UV reflecting flower parts may play an important role in communication with pollinators, we were not able to include this information in our study due to a lack of relevant data. The availability of flower reflectance data of any sort is limited among Iris taxa and do not allow genus-level analysis. For each taxon, the color was assessed and categorized into seven flower color categories: maroon, orange, pink, purple, red, yellow and white. In irises the differences between blue, violet and purple flowers are vague, therefore we coded species with flowers in these colors as purple. Polymorphic taxa fell into several categories (e.g., I. lutescens with blue and yellow morphs). Taxa with bi-colored flowers (e.g., I. narbuttii with yellow standards and violet falls) were coded as representing two categories. Second, we assessed flower pigment. Taxa were categorized as having anthocyanins (pink, purple, or red flowers) or carotenoids (orange and yellow flowers) as the major pigment, or lacking anthocyanins flavonoids and carotenoids (white or creamy specimens). As in the previous flower color trait, polymorphic and bi-colored flowers were coded into multiple categories. Several previous studies have used these pigment categories, which allow comparisons among flower taxa while eliminating differences related to color perception or habitat influence (Arista et al., 2013; Smith, 2015; Ellis and Field, 2016; Landis et al., 2018).
Flower color polymorphic species with white-flowered morphs (i.e., lacking anthocyanins and carotenoids) were assigned based on the colored morph (with pigment) to a pigment trait category as has been done in previous studies (Smith, 2015; Ellis and Field, 2016; Landis et al., 2018). Therefore, to capture the presence of white morphs, we added a white/non-white binary trait category. We also had binary trait categories for bi-colored/not bi-colored flowers, poly/monomorphic flowers (not including white morphs), and continuous/non-continuous flower colors (e.g., where color morphs occur across a continuous color gradient instead of discrete color morphs, as is the case in I. petrana). All species with continuous flower colors were also described as having polymorphic flowers. Finally, we also assessed the presence or absence of three traits that contribute to the overall visual display of Iris flowers: beard, crest, and spot. In the centre of the fall, irises either have a hairy or bristly tuft called a beard, or a cockscomb-like crest. Additionally, many Iris taxa have a signal patch (hereafter spot) of a different or stronger color on the fall (Mathew, 1989).
We also assessed several traits related to flower attraction and reproduction that potentially played a role in the evolution of Iris flowers: corolla diameter, pollinator type, mating system (self-compatibility vs. self-incompatibility), and presence of nectar. Apart from diameter, the rest of these data were ascertained either from the published literature or from personal communication (Supplementary Table 1). In most cases, pollinator type was not described to taxon level, therefore to be conservative we included only two broad groups, insects or birds.
Finally, for all studied taxa with available information, we assessed habitat type, elevation (maximum height), and geographical range. There were 10 habitat categories, with some taxa falling into several categories (e.g., desert and stony slope). Irises are found across the entire Northern Hemisphere and we identified the specific regions in which each taxon can be found. To assess whether there is any pattern relating flower color to geographical range, we used the geographic data to overlay the proportion of taxa with different flower color categories onto a QGIS map using QGIS 3.10.5 (Szczepanek, 2012; QGIS Development Team, 2020). For this visualization, each pie chart is located in the center of the specific geographical region, as calculated by QGIS.
Overall, we have flower color data for all 227 taxa. Coverage of other traits is less complete: corolla diameter 92%, pollinator type 56%, mating system 51%, and nectar availability 59%. We were able to capture data about habitat type and geographical range for all taxa, but only found elevation data for 90% of taxa.
It is important to mention that our study is biased by the sources and quality of the data we were able to obtain to populate our trait table. Ideally, we would have liked to have more resources and resolution around some of these traits, particularly around the reproductive systems of the studied irises. Also, although flower scent and color may be derived from the same biosynthesis pathways (Delle-Vedove et al., 2017) and scent plays an important role in pollinators attraction (Dormont et al., 2019) we did not include scent in our analysis due to a general lack of data regarding iris floral volatiles. That said, we are confident that we captured what data is available for these taxa and have made our inferences on that basis.
Ancestral State Reconstruction and Phylogenetic Signal
We used our phylogenetic tree and trait databases to determine the ancestral state of flower color and related traits in Iris. The ancestral states were inferred from ultrametric tree, generated using the chronos function in “ape” package (with the age of the tree set to one, value of smoothing parameter lambda = 0 based on log-likelihoods; Paradis et al., 2004). For each trait, we first determined the appropriate transition probability model, choosing among ER—equal rate, SYM—symmetrical rate, and ARD—all-rates different, using a log likelihood ratio analysis. In all cases, the ARD transition probability model was chosen because it had the highest likelihood value. However, in our analyses testing the ancestral states of bi-color, continuous, and polymorphic flowers our results suggested that the ARD model was overfitted (number of detected trait changes with the ARD model was several million vs. a thousand in the ER model), therefore for these analyses we used the ER model instead. To compute the total number of character changes between all states of binary trait categories, we used the make.simmap function in the phytools package (100 simulations across the tree, Revell, 2012).
For polymorphic traits (i.e., flower color and pigment), we inferred the ancestral character states with the R package corHMM (Beaulieu et al., 2013) using the “rayDISC” function, which specifically accommodates polymorphic characters. For all of the binary trait categories, we estimated the ancestral character states using a continuous-time Markov chain model (Mk model, phytools package). For the ancestral state reconstruction of the continuous trait, diameter, we used the FastAnc function in the phytools package (version 0.5–20; Revell, 2012).
Testing the strength of the phylogenetic signal reveals a tendency for related taxa to resemble each other more than taxa drawn at random from the same tree. For all of the binary trait we measured a D-value (Fritz and Purvis, 2010), which is a measure of phylogenetic signal dedicated to this kind of dataset (caper package, “phylo.d” function, Orme et al., 2013). The strength of the phylogenetic signal on continuous data was calculated as the Blomgergs’s K (Blomberg et al., 2003) and Pagels λ (Pagel, 1999) (phytools package in R, version 0.5–20; Revell, 2012).
Results
Phylogenetic Tree
Most of the described large-scale phylogenetic relationships found in previous studies were recaptured in our final tree (Wilson, 2004, 2009; Wilson et al., 2016; Jiang et al., 2018). That said, we decided to exclude Iris darwasica from the analysis, because of conflicting nesting results between our study and others. Specifically, we found that I. darwasica was assigned to the subgenus Limniris, but according to previous studies and floral characters this taxon belongs to the subgenus Iris, section Regelia (Khassanov and Rakhimova, 2012). Leaving such a wrongly assigned taxon in our tree may have biased all subsequent analyses.
There were some differences in the topology observed between the six sequence-specific trees and the final summarizing tree. For example, in two of the six trees that were rooted on Crocus vernus (matK and ITS), the outgroup Dietes robinsoniana resolved as nested within the Iris subgenus Limniris (low bootstrap; hereafter Bp).
As in previous studies, the topology of our final phylogenetic tree has two major subgenera (Limniris and Iris) and five minor subgenera (Hermodactyloides, Nepalensis, Pardanthopsis, Scorpiris, and Xiphium). All but Limniris were resolved as monophyletic. Within Limniris, there were two sections, Limniris (71 Bp) and Lophiris, with the first section containing the majority of the taxa belonging to the genus. Within the subgenus Iris, there were six sections: Pardanthopsis (99 Bp), Psammiris (56 Bp), Pseudoregelia (99 Bp), Oncocyclus (86 Bp), Regelia (87 Bp), Hexapogon (86 Bp), and Pogon (Figure 2).
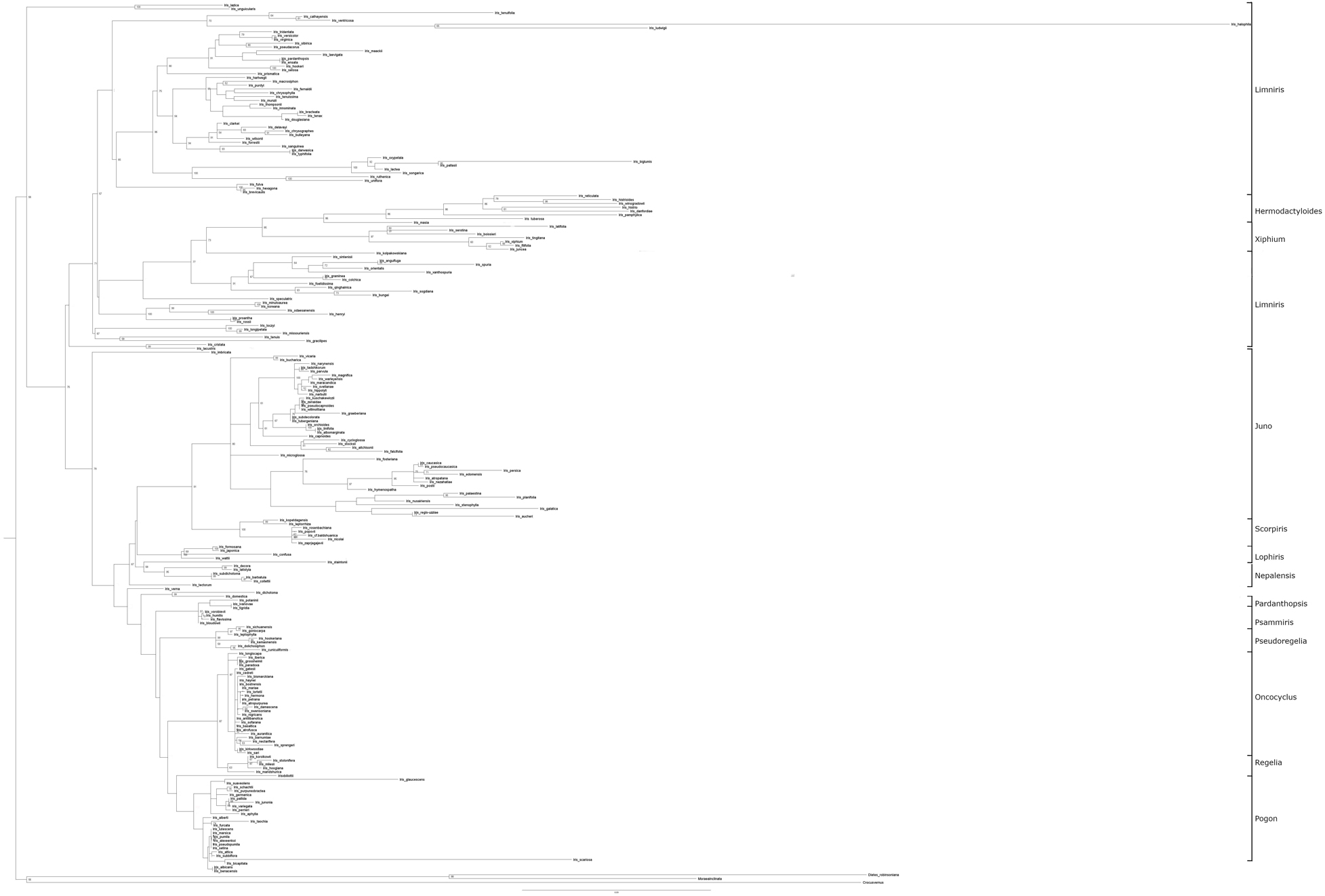
Figure 2. Maximum likelihood tree inferred from analysis of combined five plastid genomes (matK, trnL, trnK, NADPH and rbcL) and one nuclear internal transcribed spacer (ITS) sequences. The bootstrap values are given along the branches (only values > 50 presented).
A full discussion of the final tree and contributing sub-trees is presented in Supplementary Material 3.
Floral and Habitat Characters
Of the 226 Iris taxa included in this analysis, 49.3% had flowers in shades of purple and 24.5% had yellow flowers. The rest were distributed among maroon (14%), white (11%), pink (4%), orange (0.5%), red (0.5%). Most of the studied taxa were categorized as having anthocyanins (80%) and a third were characterized as having carotenoids (33%), with several taxa containing both pigments. Approximately 43% of the genus has white flower morphs or flower-parts (i.e., white standards or falls). There were 35 taxa categorized as having bi-colored flowers, 35 taxa categorized as having polymorphic flowers, and 23 taxa with continuous flower color variation. More than half of the Iris taxa have a crest (141 taxa), while over a third (85 taxa) have a beard; also, more than half of Iris taxa have a spot (143 taxa). Iris flowers range in diameter between 1.25 and 16.5 cm (MEAN ± SD: 6.2 ± 2.3 cm) (Figure 3 and Supplementary Table 1).
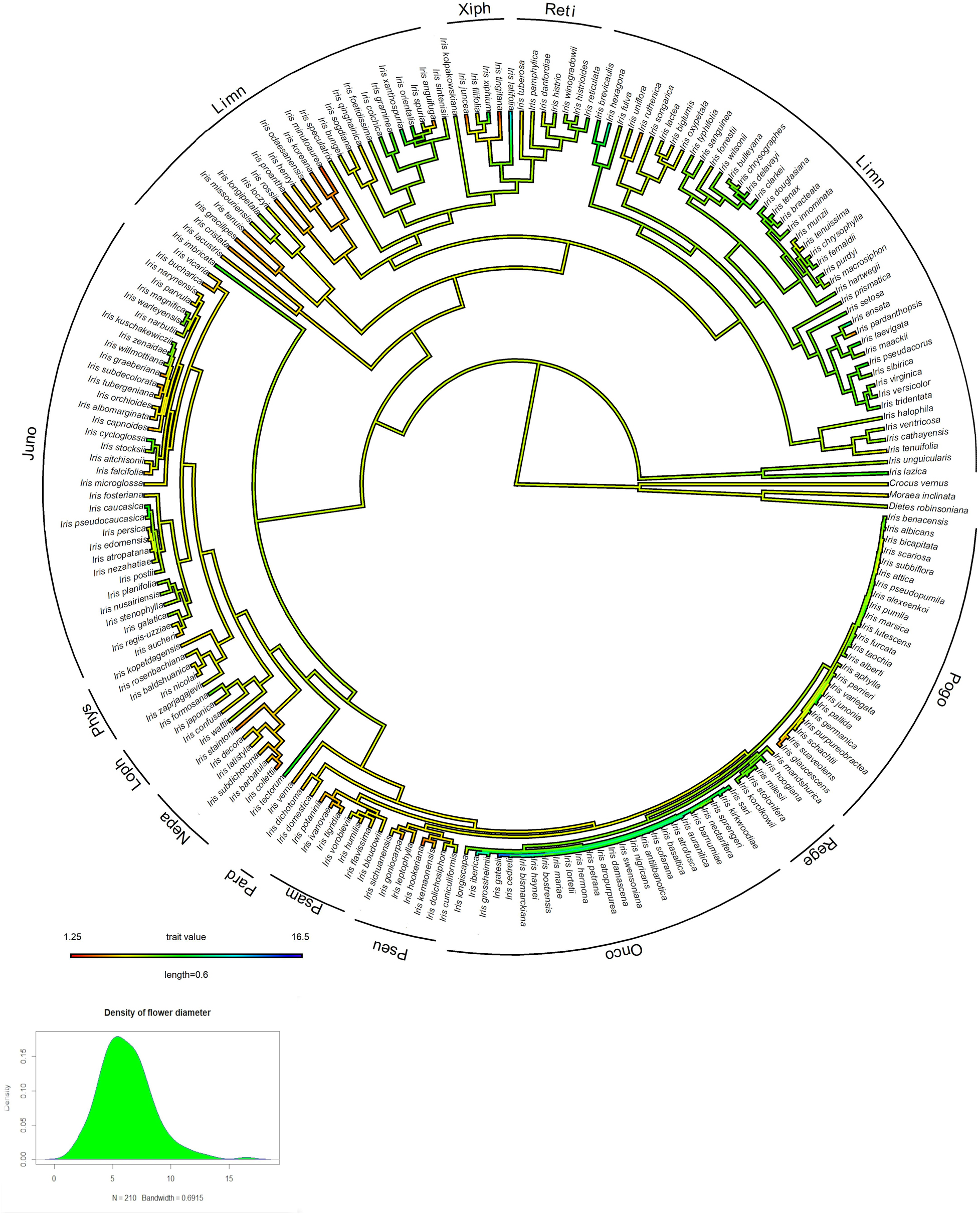
Figure 3. Maximum-likelihood ancestral state reconstruction of corolla diameter [A] and histogram showing representation of the distribution of the variables [B].
The majority of Iris taxa are pollinated by insects, primarily bees (members of Andrena, Anthophora, Apis, Colletes, Emphoropsis, Eucera, Hylaeus, Lasioglossum, Nombus, Osmia, Tetralonia, and Xylocopa). Several Iris taxa, however, are also pollinated by flies (e.g., I. bracteata, I. gracilipes, I. palaestina) and butterflies (e.g., I. fulva). Hummingbird pollination was observed in four Iris taxa: I. cristata, I. fulva, I. hexagona, and I. missouriensis. Most Iris taxa produce nectar and are self-compatible. The majority of taxa that do not produce nectar are also self-incompatible, have a beard, and belong to the Oncocyclus and Regalia sections.
Taxa with purple and yellow flowers are almost equally distributed on all continents, although yellow morphs are rare in South Asia. Polymorphic and bi-colored taxa are more prevalent in the Middle East than in other regions, and bi-colored purple-white species are completely absent from North America (Supplementary Figure 1).
Ancestral States of Floral Traits, Their Transitions, and Phylogenetic Signal
The ancestral flower color of Iris was most probably purple (Figure 4) and anthocyanin-based (Figure 5), without the ability to produce white flower morphs (Supplementary Figure 2). Most internal nodes also exhibited purple flowers with anthocyanin pigments (Figures 4, 5). Having color-monomorphic flowers, where all parts of the flower are the same color, was likely the ancestral state among Iris and remained so in the early nodes. Taxa with polymorphic, continuous, or bi-colored flowers all seem to be derived states that have arisen and been lost several times (Supplementary Figure 3). The most recent common ancestor of Iris likely had flowers with a crest and a spot (Supplementary Figure 4), and was self-compatible, insect-pollinated, and nectar-rewarding (Table 2A and Figure 6).
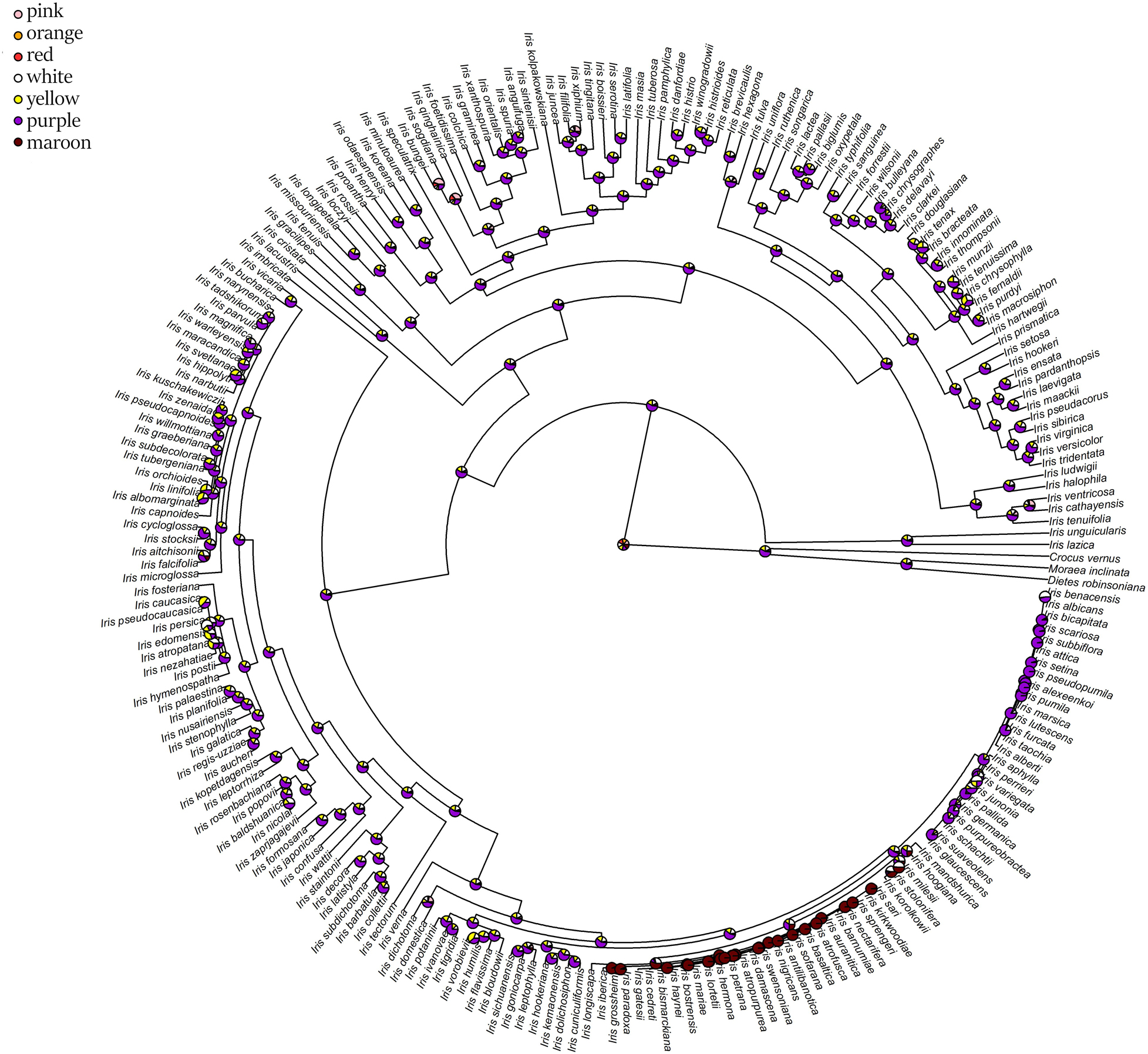
Figure 4. Estimation of ancestral states of flower colors among studied Iris taxa calculated using maximum likelihood across the posterior distribution.
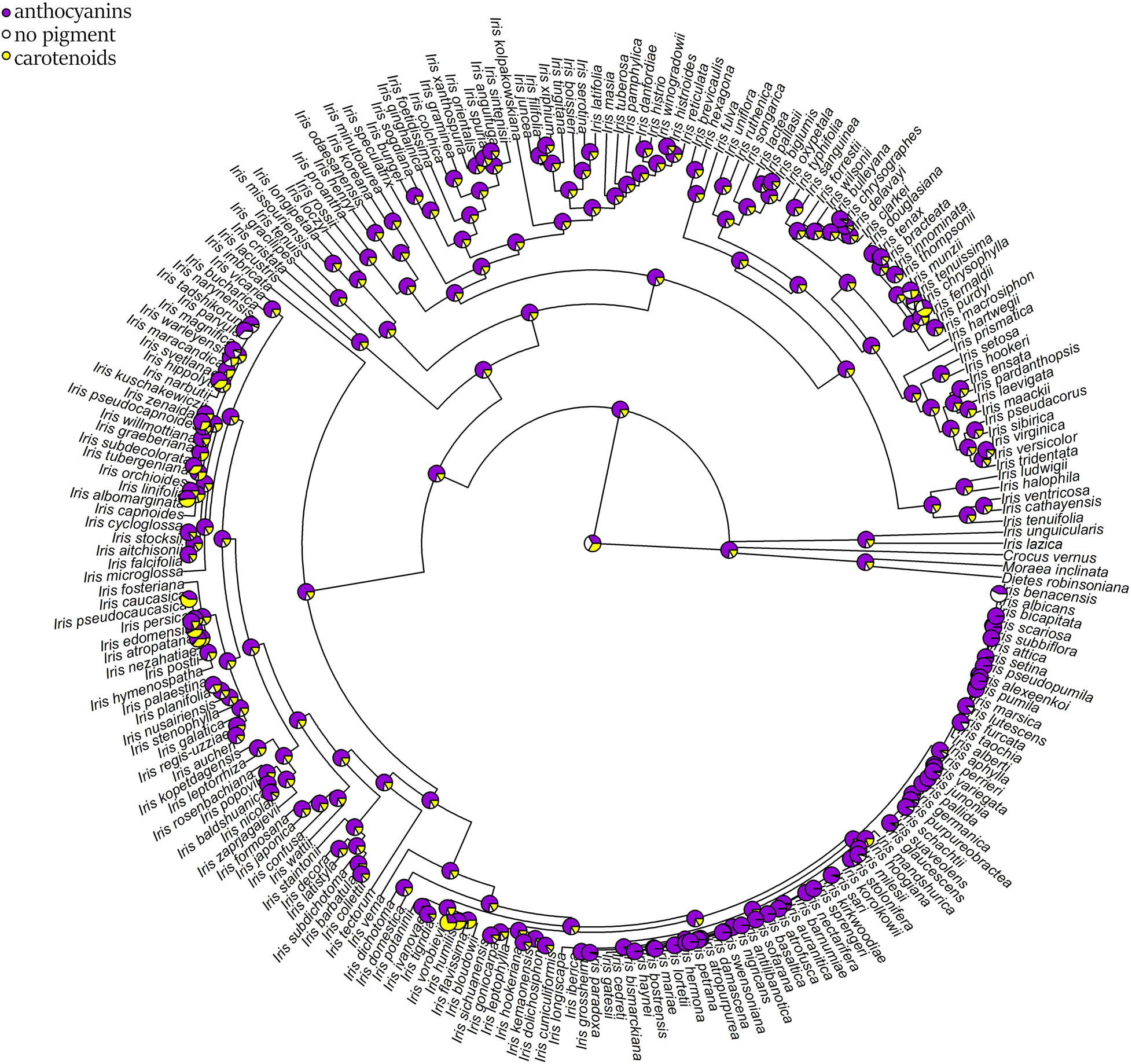
Figure 5. Estimation of ancestral states of flower pigments among studied Iris taxa calculated using maximum likelihood across the posterior distribution. White and creamy flowers were coded as lacking anthocyanins flavonoids and carotenoids and are represented as white; maroon, purple, pink, or red flowers were coded as having anthocyanins and are represented in purple; yellow or orange flowers were coded as having carotenoids and are represented in yellow.
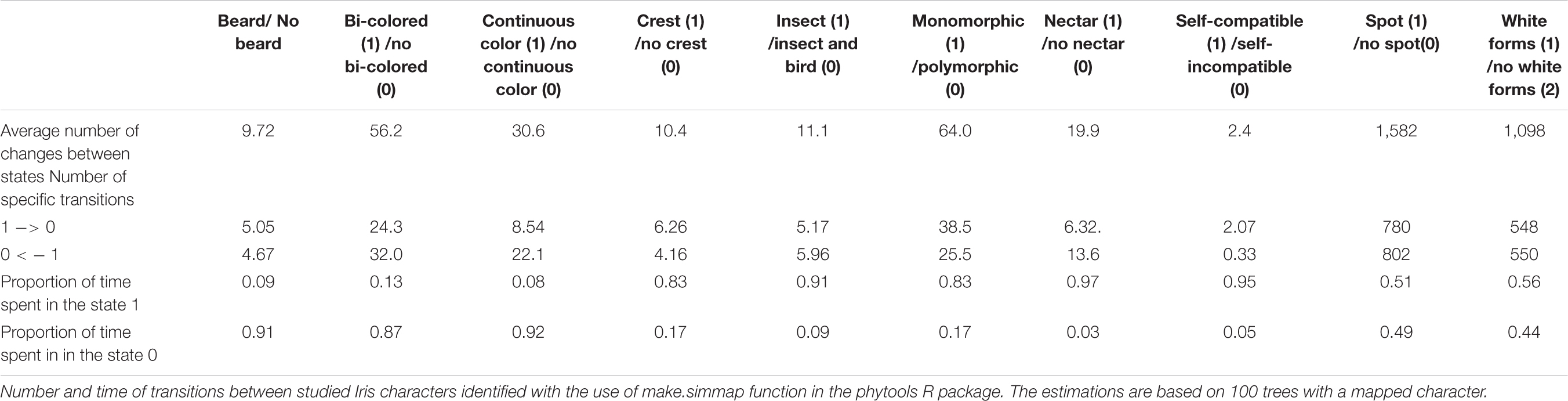
Table 2a. Results of simulated stochastic character mapping on Iris phylogenetic tree (all-rates different selected as transition probability model).
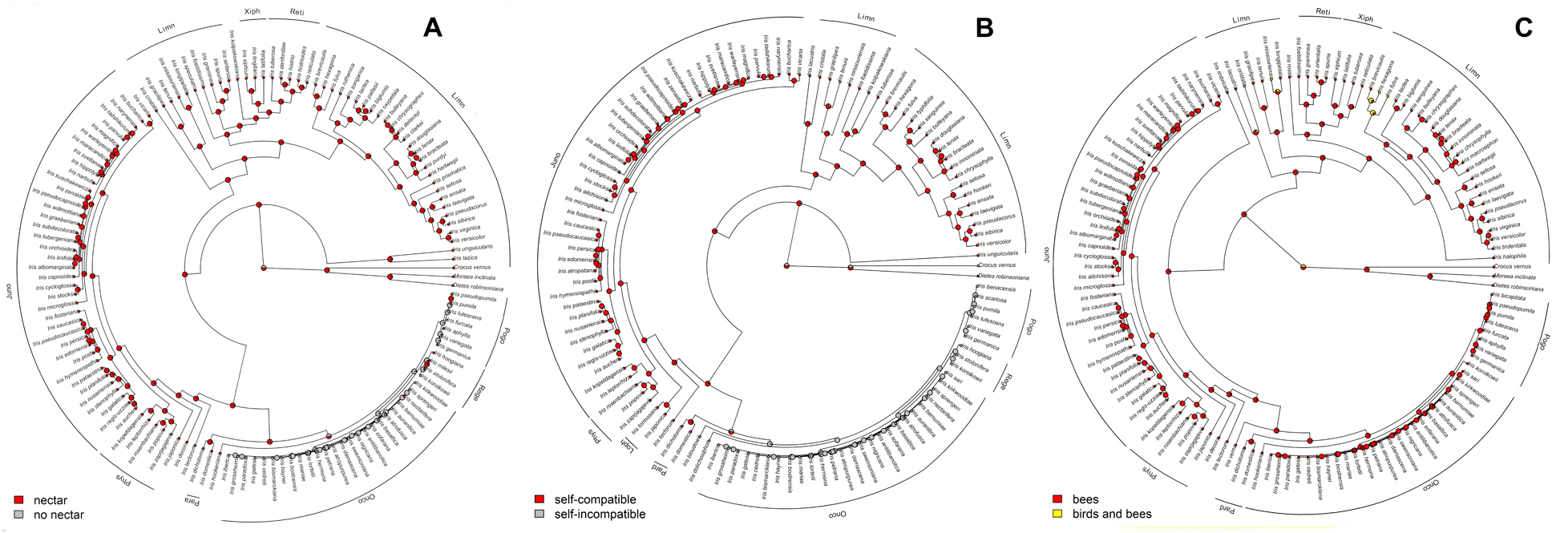
Figure 6. Summarized stochastic mapping of mating system (self-compatibility vs. self-incompatibility), (A) presence or absence of nectar (B), and pollinator type (insect vs. insect and bird) (C) in the genus Iris prepared using All Rates Different model with 1,000 iterations. Pie charts represent the proportion of the iterations showing either presence or absence of nectar at any given node.
Flower color and pigment are variable, with most of the color and pigment transitions being from yellow/carotenoid-dominated flowers to purple/anthocyanin-dominated flowers. The transitions between purple and white were also common. Of the taxa involved in these purple-white shifts, a majority represent bi-colored flowers, where either the fall or standard is white (Table 2B). The ability to produce white flower morphs is widespread across the phylogenetic tree (1,098 transitions on average; hereafter—average value based on 100 simulations), but labile, with several changes back and forth. In contrast, having monomorphic flowers with a crest persisted for most of the time. The small number of transitions between color monomorphic or polymorphic, and between having a crest or a beard suggests that these traits tend to be conserved. The proportion of time that Iris lineages have had a beard is comparatively short and this trait probably evolved once in a common ancestor of the subgenera Pogon, Oncocyclus, Pseudoregelia, Regelia, Psammiris, and Pardanthopsis, and two times more recently for I. falcifolia and I. imbricate. Unlike the crest and the beard, the presence of a spot varied more across taxa, with several changes among closely related taxa (Table 2A).
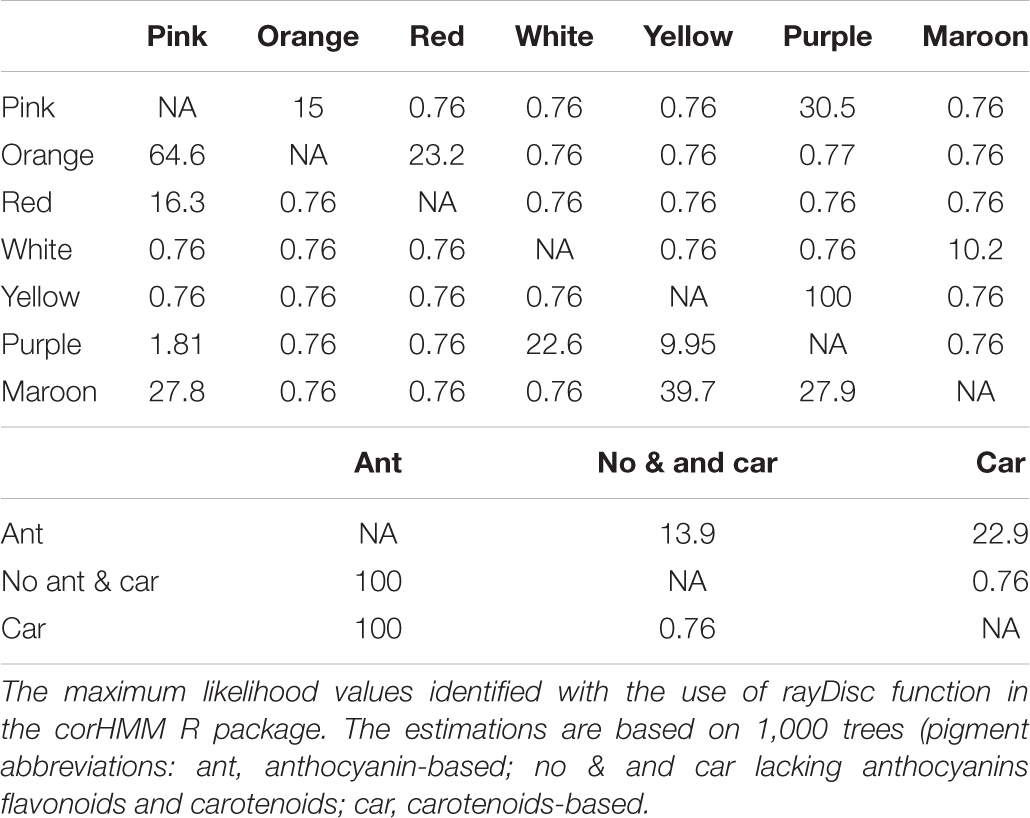
Table 2b. Matrix containing the maximum likelihood estimates of the transition rate of color and pigment on Iris phylogenetic tree (all-rates different selected as transition probability model).
The ability to self-pollinate and produce nectar was conserved for most of the time (Table 2A), and only lost once in the common ancestor of the subgenera Pogon and Oncocyclus. Both self-compatibility and the ability to produce nectar were regained several times. Even though an overwhelming majority of taxa are, and always have been, insect-pollinated, we found two independent shifts toward bird pollination (Figure 6), as well as several reversals from birds to insects (Table 2A).
Across the Iris lineage, we found all the studied binary traits to be phylogenetically conserved. Among them, the distribution of the states for the half of the traits (presence of bi-colored flowers, color polymorphism, continuous flower color and color spot on the falls) proofed to be distributed as expected under the Brownian motion model of evolution (0<D < 1). For the second half (presence of beard, crest, nectar, or mating system) the distribution of the states was more phylogenetically conserved than the Brownian expectation (D < 0). We also found a relatively strong phylogenetic signal for the flower diameter, however the significant value was obtained only for Pagel’s λ (Table 1).
Discussion
Iris flowers display a wide diversity of colors and color patterns among and within species. The diversity seen in modern irises is the result of several changes over evolutionary history in the genus. In this study, we reconstructed the traits of the most recent common ancestor of the Iris genus. This ancestor likely had monomorphic, one-colored purple flowers, with a crest and a spot on the falls. The flowers were likely insect-pollinated, nectar-rewarding, and self-compatible. Since then, the genus has diverged to include over 300 taxa that exhibit a wide range of colors and patterns, including polymorphic, continuous, and bi-colored flowers. Additionally, some taxa now have a beard, instead of a crest, have lost the spot (and sometimes regained it), and some are now self-incompatible and rewardless. There have been a few shifts from insect-pollination to bird-pollination, and occasionally back again. Thus, by comparing these derived states to the ancestral state, and placing them in the context of the Iris distribution and reproductive system, we can infer some of the drivers of floral color diversity in Iris.
Flower color is the most variable trait of those we studied in the genus Iris. Some of that diversity is likely the result of mutations, which can cause up- or down-regulation of specific genes, leading to differences in the amount of synthesized pigment and in color shades (Durbin et al., 2003). The correlation between flower color diversity and anthocyanin synthesis is common not just in Iris, but across many genera, e.g., Petunia and Ipomea (Durbin et al., 2003). Unlike some of the other groups, however, the flower color diversity in Iris is not just related to the presence or absence of anthocyanin pigments, but also the result of variation in colors produced by anthocyanins (Warren and Mackenzie, 2001). Moreover, in Iris we also observed many cases of bi-colored flowers, which may be the result of tissue-specific variation in anthocyanin production, or the loss of anthocyanins in the standard or the fall.
Color polymorphism, continuous coloration, and bi-colored flowers arose multiple times across the Iris phylogeny, and many are maintained to this day (Wang et al., 2016). The maintenance of color polymorphism is not common in other genera. Typically, one of the color morphs will eventually be lost and the taxa will again be monomorphic (Ellis and Field, 2016). The maintenance of stable color polymorphism, and other color variations, in Iris may be caused by an absence of selective disadvantage on color in these taxa or by stabilizing selection exerted by multiple agents (reviewed in Sapir et al., in review). For example most populations of I. lutescens and I. pumila are polymorphic and neutrally distributed in space (Wang et al., 2016), but in some populations color variation may be due to divergence (Souto-Vilarós et al., 2018). Several factors may maintain this neutral distribution, including environmental heterogeneity (Tucić et al., 1998). Additionally, while selection should lead to the fixation of one color morph, polymorphism may also be maintained over the long-term due to perennial life history and vegetative reproduction, which cause generation overlap in many Iris species (Gray and McKinnon, 2007).
Almost half of the studied Iris taxa produce white morphs, which is a common color-transition in many angiosperm taxa, resulting from shut-down of genes in the pigment biosynthesis pathway (Wessinger and Rausher, 2012). However, the modern Iris taxa with (only) white-colored flowers can be found in the subgenera Scorpiris and Limniris. For some Iris species white flowers may be an evolutionary dead-end, without possibility for reversal. Pigment loss may alter pollinator attractiveness (Waser and Price, 1981) or reduce the capacity to deal with environmental stress, such as drought (Ellis and Field, 2016), or a combination of the two (Warren and Mackenzie, 2001).
Like the high diversity of flower color and patterns seen across Iris taxa, corolla diameter seems to be fairly variable across the phylogeny. The visual attractiveness of flowers is substantially related to flower color and size. Although large flowers are costly (Teixido et al., 2016; Roddy, 2019), some of the species with large flowers in the genus Iris grow in desert or semi-arid habitats. This suggests that pollinator-mediated selection is more important in driving the evolution of large flowers (Lavi and Sapir, 2015; Souto-Vilarós et al., 2018). The strategy to produce big, showy, self-compatible flowers with lots of nectar is found in most Iris taxa and may increase mating success, especially when florivory is not a threat (Ghara et al., 2017). While positive pollinator-mediated directional selection on flower size may be a factor in some Iris taxa (Lavi and Sapir, 2015; Souto-Vilarós et al., 2018), this is not always the case (Ishii, 2005; Pellegrino, 2015).
Most Iris species are pollinated by insects, but in a few cases, shifts to bird pollination evolved. Several taxa of Iris have blue-violet or purple flowers, which tend to be associated with bee visitation because bees tend to have an innate preference for the blue range of wavelengths (Lunau and Maier, 1995; Dyer et al., 2015). Bees also have an innate preference for yellow flowers, and thus the common shift from purple to yellow flowers in Iris may be maintained by bee preferences (Giurfa et al., 1995; Lunau and Maier, 1995; Spaethe et al., 2001). This innate preference for both purple and yellow flowers may explain the equal seed set between color morphs in some polymorphic Iris populations (Imbert et al., 2014a, b; Souto-Vilarós et al., 2018).
Bird-pollinated flowers tend to be red or orange (Rodríguez-Gironés and Santamaría, 2004; Cronk and Ojeda, 2008), and these two colors have arisen several times in the Iris phylogeny. Red coloration may be a good predictor of bird-pollination in irises, but not vice-versa. There is only one taxon with red flowers, and it is bird-pollinated (I. fulva, Emms and Arnold, 2000; Martin et al., 2008), but other bird-pollinated species are not red (e.g., I. missouriensis, Lyon, 1973). This suggests that color is likely not the major trait driving pollinator-shifts from insects to birds in Iris. All the bird-pollinated taxa have wide, open and flat flowers that produce dilute nectar (Wesselingh and Arnold, 2000). Being able to access the flower (wide open, flat flowers) and obtain the preferred food reward (diluted nectar) seems likely to be the trigger for the transition to bird pollination. Shifting from purple to red flowers may be relatively easy because the anthocyanin biosynthesis pathway is relatively flexible (Warren and Mackenzie, 2001; Albert et al., 2011). Bird-driven transitions from purple to red flowers, however, would likely be countered by insect pollination because insects often prefer blue-violet (Lunau and Maier, 1995; Dyer et al., 2015) and yellow flowers (Giurfa et al., 1995; Lunau and Maier, 1995; Spaethe et al., 2001). This may explain why two out of the three extant bird-pollinated flowers are purple, and not red.
We found a strong signal for the association among floral structure, reward, and mating system. All taxa have either a crest or a beard, but never both. Although the ancestral Iris probably had a crest, the beard replaced the crest relatively early in the common ancestor of Oncocyclus, Pogon, and Regalia. Around the same time, this group of subgenera also lost the ability to produce nectar and became self-incompatible. Once the transition to a beard and self-incompatibility occurred in this section, it never changed back. In contrast, nectar production was regained several times. We also found, that white-purple and purple flower color, which are commonly found among members of these subgenera, are associated with self-incapability and a lack of nectar. While the association of these traits and their strong phylogenetic signal suggests the evolution of a monophyletic pollination syndrome, it is important to note the sampling of these traits was not similar across all species.
Nectar production requires regular and consistent water availability. In the dry regions inhabited by Oncocyclus, Pogon, and Regalia irises, water limitation could have been the agent of selection against nectar production, leading to the loss of this trait in their common ancestor. Once these irises became nectarless, the question becomes: how did they attract insect pollinators? Potentially, this is when the night-sheltering reward system, that is well-described in the Oncocyclus group, arose (Sapir et al., 2005; Monty et al., 2006; Vereecken et al., 2013; Watts et al., 2013). Oncocyclus irises are primarily pollinated by male Eucera bees (Apidae, Eucerini) that shelter in the flowers overnight (Sapir et al., 2005, 2006; Watts et al., 2013). Pollination occurs as the male bees visit multiple flowers before choosing the flower in which they will spend the night (Sapir et al., 2005; Monty et al., 2006). While it is possible that bees slept in the flowers before nectar was lost, after nectar-loss the sheltering component became the only reward. Thus, shelter may have become a replacement mechanism to attract pollinators.
The loss of nectar is associated with the transition from crest to beard. If a crest serves as a nectar guide, there will be no selection to maintain it in nectarless species. Instead, a beard might have selected by its advantage in alternative reward types, such as the derived sheltering-reward system. The role of the beard remains debatable, but it would be interesting to test whether the presence of a beard changes the airflow over the fall and impacts the rate of warming in these flowers, or whether it contributes for pollen deposition on stigma.
Another open question is why self-compatibility only arose in the closely related Oncocyclus, Pogon, and Regalia (except for I. tenuis). The loss of self-compatibility seems risky, but perhaps it was necessary to maintain genetic diversity. Most of the taxa in these subgenera occur in highly variable habitats in the Middle East and Western Asia. In a complex habitat mosaic, with patchy and extreme environmental conditions, being self-compatible may be maladaptive. Self-compatibility would naturally lead to selfing and inbreeding, thus reduce genetic diversity. Maintaining genetic diversity is particularly important for plants in highly variable and stressful conditions. It facilitates quick adaptation and increased population persistence, as a sort of insurance. Indeed, most of these taxa have highly variable flower traits, most notably in terms of color.
The nectarless Oncocyclus, Pogon, and Regalia, tend to have high levels of color polymorphism, continuous color variation, and bi-colored flowers. Within-species variation in flower color may be important in deceptive systems, since it impedes the learning ability of pollinators and leads to negative frequency-dependent selection (Smithson and MacNair, 1997; Gigord et al., 2001; Imbert et al., 2014a). Another strategy to maintain the attractiveness of no-food rewarding species is flowering early in the season and attracting naïve pollinators (Imbert et al., 2014a). In any case, it is surprising that more taxa in these subgenera did not regain nectar production. Comparative studies have shown that nectarless taxa in general (Aragon and Ackerman, 2004; Sletvold et al., 2016), and Iris taxa specifically (Lavi and Sapir, 2015; Souto-Vilarós et al., 2018), are much more pollen limited than nectar producing taxa.
Irises cover a broad geographic range across a mosaic of habitat types, environmental stresses and pollinator types, which is likely the ultimate cause of the observed flower diversity. In general, some Iris taxa seem to have strong pollinator-mediated selection on floral traits, such as color and size. In other taxa, however, the environment seems to play a stronger selective role in one or more of these traits. The diversity of flower colors we see in Iris, likely represents a trade-off between conflicting selection pressures (Souto-Vilarós et al., 2018). Whether changes in flower color are the result of neutral processes without any selection, or whether these changes are tightly maintained by abiotic or biotic selective agents, remains an open question.
Data Availability Statement
All datasets generated for this study are included in the article/Supplementary Material.
Author Contributions
YS and KR conceived and designed the research. KR and MG performed analyses. KR, MG, and YS wrote the manuscript, with contributions from all authors. All authors collected the data.
Funding
This work was supported by a grant from Israel Science Foundation to YS (ISF 336/16). KR was supported by scholarship from the Polish National Agency for Academic Exchange (NAWA; PPN/IWA/2018/1/00014). MG was supported by a Zuckerman STEM Leadership Postdoctoral Fellowship.
Conflict of Interest
The authors declare that the research was conducted in the absence of any commercial or financial relationships that could be construed as a potential conflict of interest.
Acknowledgments
This work inspired by discussions with Brian Mathew and Tony Hall of Royal Botanic Gardens, Kew. We thank Kenneth Walker and Tony Hall for providing data on the biology of many Iris species.
Dedication
This article is dedicated to Brian Mathew, leader in Iris taxonomy and ornamental bulb plants.
Supplementary Material
The Supplementary Material for this article can be found online at: https://www.frontiersin.org/articles/10.3389/fpls.2020.569811/full#supplementary-material
Supplementary Figure 1 | Map showing the total proportion of pigments among studied Iris taxa and the distribution of color morphs across the geographical range.
Supplementary Figure 2 | Summarized stochastic mapping of the presence of the white/no-white flowers in the genus Iris using All Rates Different model with 1,000 iterations. Pie charts represent the proportion of the iterations showing either presence or absence of the white/no-white flowers at any given node.
Supplementary Figure 3 | Summarized stochastic mapping of the presence of the poly/monomorphic (A), continuous/non-continuous color (B), bi-colored/not bi-colored (C) flowers in the genus Iris using All Rates Different model with 1,000 iterations. Pie charts represent the proportion of the iterations showing either presence or absence of the studied traits in flowers at any given node.
Supplementary Figure 4 | Summarized stochastic mapping of the crest (A), beard (B), or spot (C) presence/absence in the genus Iris using All Rates Different model with 1,000 iterations. Pie charts represent the proportion of the iterations showing either presence or absence of studied trait at any given node.
Supplementary Table 1 | Data table. Data include color and floral traits, habitat and source of data.
Supplementary Material 1 | Accession numbers of the gene sequences acquired from GenBank.
Supplementary Material 2 | Evolutionary models selected with the use of ModelTest-NG program.
Supplementary Material 3 | The contributing sub-trees of Iris genus; results and discussion of the phylogeny.
Footnotes
References
Albert, N. W., Lewis, D. H., Zhang, H., Schwinn, K. E., Jameson, P. E., and Davies, K. M. (2011). Members of an R2R3-MYB transcription factor family in Petunia are developmentally and environmentally regulated to control complex floral and vegetative pigmentation patterning. Plant J. 65, 771–784. doi: 10.1111/j.1365-313X.2010.04465.x
Anderson, I. A., and Busch, J. W. (2006). Relaxed pollinator-mediated selection weakens floral integration in self-compatible taxa of Leavenworthia (Brassicaceae). Am. J. Bot. 93, 860–867. doi: 10.3732/ajb.93.6.860
Aragon, S., and Ackerman, J. D. (2004). Does flower color variation matter in deception pollinated Psychilis monensis (Orchidaceae)? Oecologia 138, 405–413. doi: 10.1007/s00442-003-1443-9
Arista, M., Talavera, M., Berjano, R., and Ortiz, P. L. (2013). Abiotic factors may explain the geographical distribution of flower colour morphs and the maintenance of colour polymorphism in the scarlet pimpernel. J. Ecol. 101, 1613–1622. doi: 10.1111/1365-2745.12151
Armbruster, W. S. (1996). “Evolution of floral morphology and function: an integrative approach to adaptation, constraint, and compromise in Dalechampia (Euphorbiaceae),” in Floral Biology, eds D. G. Lloyd and S. C. H. Barrett (Boston, MA: Springer), 241–272. doi: 10.1007/978-1-4613-1165-2_9
Armbruster, S., Fenster, C., and Dudash, M. (2000). Pollination ‘principles’ revisited: specialization, pollination syndromes, and the evolution of flowers. Scandanav. Assoc. Pollinat. Ecol. 39, 179–200.
Beaulieu, J. M., O’Meara, B. C., and Donoghue, M. J. (2013). Identifying hidden rate changes in the evolution of a binary morphological character: the evolution of plant habit in campanulid angiosperms. Syst. Biol. 62, 725–737. doi: 10.1093/sysbio/syt034
Blomberg, S. P., Garland, T., and Ives, A. R. (2003). Testing for phylogenetic signal in comparative data: behavioral traits are more labile. Evolution 57, 717–745. doi: 10.1111/j.0014-3820.2003.tb00285.x
Bonguebartelsman, M., and Phillips, D. (1995). Nitrogen stress regulates gene-expression of enzymes in the flavonoid biosynthetic pathway of tomato. Plant Physiol. Biochem. 33, 539–546.
Canto, A., Herrera, C. M., García, I. M., Pérez, R., and Vaz, M. (2011). Intraplant variation in nectar traits in Helleborus foetidus (Ranunculaceae) as related to floral phase, environmental conditions and pollinator exposure. Flora 206, 668–675. doi: 10.1016/j.flora.2011.02.003
Castresana, J. (2000). Selection of conserved blocks from multiple alignments for their use in phylogenetic analysis. Mole. Biol. Evol. 17, 540–552. doi: 10.1093/oxfordjournals.molbev.a026334
Chalker-Scott, L. (1999). Environmental significance of anthocyanins in plant stress responses. Photochem. Photobiol 70, 1–9. doi: 10.1111/j.1751-1097.1999.tb01944.x
Chittka, L., and Schürkens, S. (2001). Successful invasion of a floral market. Nature 411, 653–653. doi: 10.1038/35079676
Crespo, M. B., Martínez-Azorín, M., and Mavrodiev, E. V. (2015). Can a rainbow consist of a single colour? A new comprehensive generic arrangement of the ‘ Iris sensu latissimo ’ clade (Iridaceae), congruent with morphology and molecular data. Phytotaxa 232, 1–78. doi: 10.11646/phytotaxa.232.1.1
Cronk, Q., and Ojeda, I. (2008). Bird-pollinated flowers in an evolutionary and molecular context. J. Exper. Bot. 59, 715–727. doi: 10.1093/jxb/ern009
Dafni, A. (1984). Mimicry and deception in pollination. Annu. Rev. Ecol. System. 15, 259–278. doi: 10.1016/bs.abr.2016.10.005
Dafni, A., Ivri, Y., and Brantjes, B. M. (1981). Pollination of Serapias vomeracea Briq. (Orchidaceae) by imitation of holes for sleeping solitary male bees (Hymenoptera). Acta Bot. Neerland. 30, 69–73. doi: 10.1111/j.1438-8677.1981.tb00388.x
Darriba, D., Posada, D., Kozlov, A. M., Stamatakis, A., Morel, B., and Flouri, T. (2020). ModelTest-NG: a new and scalable tool for the selection of DNA and protein evolutionary models. Mole. Biol. Evol. 37, 291–294. doi: 10.1101/612903
de Camargo, M. G. G., Lunau, K., Batalha, M. A., Brings, S., de Brito, V. L. G., and Morellato, L. P. C. (2019). How flower colour signals allure bees and hummingbirds: a community-level test of the bee avoidance hypothesis. New Phytol. 222, 1112–1122. doi: 10.1111/nph.15594
Delle-Vedove, R., Schatz, B., and Dufay, M. (2017). Understanding intraspecific variation of floral scent in light of evolutionary ecology. Anna. Bot. 120, 1–20. doi: 10.1093/aob/mcx055
Dormont, L., Joffard, N., and Schatz, B. (2019). Intraspecific variation in floral color and odor in orchids. Int. J. Plant Sci. 180, 1036–1058. doi: 10.1086/705589
Duffy, K. J., and Stout, J. C. (2011). Effects of conspecific and heterospecific floral density on the pollination of two related rewarding orchids. Plant Ecol. 212, 1397–1406. doi: 10.1007/s11258-011-99159911
Durbin, M. L., Lundy, K. E., Morrell, P. L., Torres-Martinez, C. L., and Clegg, M. T. (2003). Genes that determine flower color: the role of regulatory changes in the evolution of phenotypic adaptations. Mole. Phylogenet. Evol. 29, 507–518. doi: 10.1016/s1055-7903(03)00196-9
Dyer, A. G., Garcia, J. E., Shrestha, M., and Lunau, K. (2015). Seeing in colour: a hundred years of studies on bee vision since the work of the Nobel laureate Karl von Frisch. Proc. Royal Soc. Vict. 127, 66–72. doi: 10.1071/rs15006
Ellis, T. J., and Field, D. L. (2016). Repeated gains in yellow and anthocyanin pigmentation in flower colour transitions in the Antirrhineae. Anna. Bot. 117, 1133–1140. doi: 10.1093/aob/mcw043
Emms, S. K., and Arnold, M. L. (2000). Site-to-site differences in pollinator visitation patterns in a Louisiana Iris hybrid zone. Oikos 91, 568–578. doi: 10.1034/j.1600-0706.2000.910319.x
Felsenstein, J. (2012). A comparative method for both discrete and continuous characters using the threshold model. Am. Natur. 179, 145–156. doi: 10.1086/663681
Fenster, C. B., Armbruster, W. S., Wilson, P., Dudash, M. R., and Thomson, J. D. (2004). Pollination Syndromes and Floral Specialization. Annu. Rev. Ecol. Evol. System. 35, 375–403. doi: 10.1146/annurev.ecolsys.34.011802.132347
Fritz, S., and Purvis, A. (2010). Selectivity in Mammalian Extinction Risk and Threat Types: A New Measure of Phylogenetic Signal Strength in Binary Traits. Conservat. Biol. 24, 1042–1051. doi: 10.1111/j.1523-1739.2010.01455.x
Ghara, M., Ewerhardy, C., Yardeni, G., Matzliach, M., and Sapir, Y. (2017). Does floral herbivory reduce pollination-mediated fitness in shelter rewarding Royal Irises? BioRxiv doi: 10.1101/184382
Gigord, L. D. B., Macnair, M. R., and Smithson, A. (2001). Negative frequency-dependent selection maintains a dramatic flower color polymorphism in the rewardless orchid Dactylorhiza sambucina (L.) Soò. Proc. Natl. Acad. Sci. 98, 6253–6255. doi: 10.1073/pnas.111162598
Giurfa, M., Núñez, J., Chittka, L., and Menzel, R. (1995). Colour preferences of flower-naive honeybees. J. Comparat. Physiol. A 177, 247–259. doi: 10.1007/BF00192415
Goldberg, E. E., Kohn, J. R., Lande, R., Robertson, K. A., Smith, S. A., and Igic, B. (2010). Species selection maintains self-incompatibility. Science 330, 493–495. doi: 10.1126/science.1194513
Goldblatt, P., and Manning, J. C. (2008). The Iris family: Natural History and Classification. Portland: Timber Press.
Goldblatt, P., Savolainen, V., Porteous, O., Sostaric, I., Powell, M., Reeves, G., et al. (2002). Radiation in the Cape flora and the phylogeny of peacock irises Moraea (Iridaceae) based on four plastid DNA regions. Mole. Phylogenet. Evol. 25, 341–360. doi: 10.1016/S1055-7903(02)00235-X
Gray, S. M., and McKinnon, J. S. (2007). Linking color polymorphism maintenance and speciation. Trends Ecol. Evol. 22, 71–79. doi: 10.1016/j.tree.2006.10.005
Grotewold, E. (2006). The genetics and biochemistry of floral pigments. Annu. Rev. Plant Biol. 57, 761–780. doi: 10.1146/annurev.arplant.57.032905.105248
Harborne, J. B., and Williams, C. A. (2000). The phytochemical richness of the Iridaceae and its systematic significance. Annal Di Bot. 58, 43–50. doi: 10.4462/annbotrm-9061
Imbert, E., Wang, H., Anderson, B., Hervouet, B., Talavera, M., and Schatz, B. (2014a). Reproductive biology and colour polymorphism in the food-deceptive Iris lutescens (Iridaceae). Acta Bot. Gall. 161, 117–127. doi: 10.1080/12538078.2014.895419
Imbert, E., Wang, H., Conchou, L., Vincent, H., Talavera, M., and Schatz, B. (2014b). Positive effect of the yellow morph on female reproductive success in the flower colour polymorphic Iris lutescens (Iridaceae), a deceptive species. J. Evolut. Biol. 27, 1965–1974. doi: 10.1111/jeb.12451
Irwin, R. E., Strauss, S. Y., Storz, S., Emerson, A., and Guibert, G. (2003). The role of herbivores in the maintenance of a flower color polymorphism in wild radish. Ecology 84, 1733–1743. doi: 10.1890/0012-9658(2003)084[1733:trohit]2.0.co;2
Ishii, H. S. (2005). Analysis of bumblebee visitation sequences within single bouts: Implication of the overstrike effect on short-term memory. Behav. Ecol. Sociobiol. 57, 599–610. doi: 10.1007/s00265-004-0889-z
Jiang, Y. L., Huang, Z., Liao, J. Q., Song, H. X., Luo, X. M., Gao, S. P., et al. (2018). Phylogenetic analysis of Iris L. from China on chloroplast TRNL-F sequences. Biol. 73, 459–466. doi: 10.2478/s11756-018-006360
Katoh, K., Rozewicki, J., and Yamada, K. (2017). MAFFT online service: multiple sequence 149 alignment, interactive sequence choice and visualization. Brief. Bioinform. 20(4), 1160–1166. doi: 10.1093/bib/bbx108
Khassanov, F. O., and Rakhimova, N. (2012). Taxonomic revision of the genus Iris L. (Iridaceae Juss.) for the flora of Central Asia. Stapfia 97, 174–179.
Kuraku, S., Zmasek, C., Nishimura, O., and Katoh, K. (2013). Leaves facilitates on-demand exploration of metazoan gene family trees on MAFFT sequence alignment server with enhanced interactivity. Nucl. Acids Res. 41, 22–28.
Landis, J. B., Bell, C. D., Hernandez, M., Zenil-ferguson, R., McCarthy, E. W., Soltis, D. E., et al. (2018). Evolution of Floral Traits and Impact of Reproductive Mode on Diversification in the Phlox Family (Polemoniaceae). Mole. Phyl. Evol. 127, 878–890 doi: 10.1016/j.ympev.2018.06.035
Lavi, R., and Sapir, Y. (2015). Are pollinators the agents of selection for the extreme large size and dark color in Oncocyclus irises? New Phytol. 205, 369–377. doi: 10.1111/nph.12982
Little, R. J. (1983). “A review of floral food deception mimicries with comments on floral mutualism,” in Handbook of experimental pollination biology, eds C. E. Jones and R. J. Little, (New York: Van Nostrand Reinhold), 294–309.
Lunau, K., and Maier, E. J. (1995). Innate colour preferences of flower visitors. J. Comparat. Physiol. A 177, 1–19. doi: 10.1007/BF00243394
Lyon, D. L. (1973). Territorial and feeding activity of broad-tailed hummingbirds (Selasphorus platycercus) in Iris missouriensis. Condor 75, 346-349. doi: 10.2307/1366178
Maddison, W. P., and Maddison, D. R. (2018). Mesquite: a modular system for evolutionary analysis. Evolution 11:5.
Martin, N. H., Sapir, Y., and Arnold, M. L. (2008). The genetic architecture of reproductive isolation in Louisiana Irises: pollination syndromes and pollinator preferences. Evolution 62, 740–752. doi: 10.1111/j.1558-5646.2008.00342.x
Martins, T. R., Jiang, P., and Rausher, M. D. (2016). How petals change their spots: cis-regulatory re-wiring in Clarkia (Onagraceae). New Phytol. 216(2), 510–518. doi: 10.1111/nph.14163
Monty, A., Saad, L., and Mahy, G. G. (2006). Bimodal pollination system in rare endemic Oncocyclus irises (Iridaceae) of Lebanon. Canad. J. Bot. 84, 1327–1338. doi: 10.1139/b06-081
Narbona, E., Wang, H., Ortiz, P. L., Arista, M., and Imbert, E. (2018). Flower colour polymorphism in the Mediterranean Basin: occurrence, maintenance and implications for speciation. Plant Biol. 20, 8–20. doi: 10.1111/plb.12575
Ng, J., and Smith, S. D. (2018). Why are red flowers so rare? Testing the macroevolutionary causes of tippiness. J. Evolut. Biol. 31, 1863–1875. doi: 10.1111/jeb.13381
Orme, D., Freckleton, R., Thomas, G., Petzoldt, T., Fritz, S., Isaac, N., et al. (2013). Caper: comparative analyses of phylogenetics and evolution in R.R. package version 0.5.2.
Pagel, M. (1999). Inferring the historical patterns of biological evolution. Nature 40, 877–884. doi: 10.1038/44766
Parachnowitsch, A. L., Manson, J. S., and Sletvold, N. (2018). Evolutionary ecology of nectar. Anna. Bot. 123, 247–261. doi: 10.1093/aob/mcy132
Paradis, E., Claude, J., and Strimmer, K. (2004). APE: Analyses of Phylogenetics and Evolution in R language. Bioinformatics 20, 289–290. doi: 10.1093/bioinformatics/btg412
Pellegrino, G. (2015). Pollinator limitation on reproductive success in Iris tuberosa. AOB Plants 7:lu089. doi: 10.1093/aobpla/plu089
Pellegrino, G., Bellusci, F., and Palermo, A. M. (2017). Functional differentiation in pollination processes among floral traits in Serapias species (Orchidaceae). Ecol. Evolut. 7, 7171–7177. doi: 10.1002/ece3.3264
QGIS Development Team (2020). QGIS Geographic Information System. Open Source Geospatial Foundation Project. Available online at: http://qgis.osgeo.org
Rausher, M. D. (2008). Evolutionary Transitions in Floral Color. Int. J. Plant Sci. 169, 7–21. doi: 10.1086/523358
Revell, L. J. (2012). phytools: an R package for phylogenetic comparative biology (and other things). Methods Ecol. Evol. 3, 217–223. doi: 10.1111/j.2041-210X.2011.00169.x
Roddy, A. B. (2019). A physiological approach to the ecology and evolution of flowers. Int. J. Plant Sci. 9, 944–953. doi: 10.1101/539668
Rodríguez-Gironés, M. A., and Santamaría, L. (2004). Why are so many bird flowers red? PLoS Biol. 2:e350. doi: 10.1371/journal.pbio.0020350
Roguz, K., Bajguz, A., Golebiewska, A., Chmur, M., Hill, L., Kalinowski, P., et al. (2018). Functional Diversity of Nectary Structure and Nectar Composition in the Genus Fritillaria (Liliaceae). Front. Plant Sci. 9:1246. doi: 10.3389/fpls.2018.01246
Roguz, K., Bajguz, A., Chmur, M., Gołȩbiewska, A., Roguz, A., and Zych, M. (2019). Diversity of nectar amino acids in the Fritillaria (Liliaceae) genus: ecological and evolutionary implications. Sci. Rep. 9:15209. doi: 10.1038/s41598-019-5117051174
Roy, R., Schmitt, A. J., Thomas, J. B., and Carter, C. J. (2017). Review: Nectar biology: From molecules to ecosystems. Plant Sci. 262, 148–164. doi: 10.1016/j.plantsci.2017.04.012
Royal Botanic, and Gardens, K. (2020). World checklist of selected plant families. Peradeniya: Royal Botanic.
Sapir, Y., and Shmida, A. (2002). Species concepts and ecogeographical divergence of Oncocyclus irises. Isr. J. Plant Sci. 50, S119–S127.
Sapir, Y., Shmida, A., Fragman, O., and Comes, H. P. (2002). Morphological variation of the Oncocyclus irises (Iris: Iridaceae) in the southern Levant. Botan. J. Linn. Soc. 139, 369–382. doi: 10.1046/j.1095-8339.2002.00067.x
Sapir, Y., Shmida, A., and Ne’eman, G. (2005). Pollination of Oncocyclus irises (Iris: Iridaceae) by night-sheltering male bees. Plant Biol. 7, 417–424. doi: 10.1055/s-2005837709
Sapir, Y., Shmida, A., and Ne’eman, G. (2006). Morning floral heat as a reward to the pollinators of the Oncocyclus irises. Oecologia 147, 53–59. doi: 10.1007/s00442-005-0246-6
Schaefer, H., and Ruxton, G. (2011). “Floral communication and pollination,” in Plant-animal communication, eds H. Schaefer, and G. D. Ruxton, (Oxford: Oxford University Press).
Schiestl, F. P., and Johnson, S. D. (2013). Pollinator-mediated evolution of floral signals. Trends Ecol. Evol. 28, 307–315. doi: 10.1016/j.tree.2013.01.019
Simpson, B. B., and Neff, J. L. (1983). “Evolution and diversity of floral rewards,” in Handbook of Experimental Pollination Biology, eds C. E. Jones and R. J. Little (New York, NY: Van Nostrand Reinhold), 142–159.
Sletvold, N., Trunschke, J., Smit, M., Verbeek, J., and Agren, J. (2016). Strong pollinator-mediated selection for increased flower brightness and contrast in a deceptive orchid. Evolution 70, 716–724. doi: 10.1111/evo.12881
Smith, S. D. (2015). Tempo and mode of flower color evolution. Am. J. Bot. 102(7), 1014–25. doi: 10.3732/ajb.1500163
Smithson, A., and MacNair, M. R. (1997). Negative frequency- dependent selection by pollinators on artificial flowers with- out rewards. Evolution 51, 715–723. doi: 10.2307/2411148
Souto-Vilarós, D., Vuleta, A., Jovanović, S. M., Budečević, S., Wang, H., Sapir, Y., et al. (2018). Are pollinators the agents of selection on flower colour and size in irises? Oikos 127, 834–846. doi: 10.1111/oik.04501
Spaethe, J., Streinzer, M., and Paulus, H. F. (2010). Why sexually deceptive orchids have colored flowers. Commun. Integr. Biol. 3, 139–141. doi: 10.4161/cib.3.2.10333
Spaethe, J., Tautz, J., and Chittka, L. (2001). Visual constraints in foraging bumblebees: flower size and color affect search time and flight behavior. Proc. Natl. Acad. Sci. Unite. Stat. Am. 98, 3898–3903. doi: 10.1073/pnas.071053098
Stamatakis, A. (2014). RAxML version 8: a tool for phylogenetic analysis and post-analysis of large phylogenies. Bioinformatics 30, 1312–1313. doi: 10.1093/bioinformatics/btu033
Streisfeld, M. A., and Rausher, M. D. (2011). Poulation genetics, pleiotropy, and the preferential fixation of mutations during adaptive evolution. Evolution 65, 629–642. doi: 10.1111/j.1558-5646.2010.01165.x
Sutherland, S. D., and Vickery, R. K. Jr. (1993). On the relative importance of floral color, shape, and nectar rewards in attracting pollinators to Mimulus. Great Basin Natural. 53, 107–117.
Szczepanek, R. (2012). Quantum GIS – wolny i otwarty system informacji geograficznej. Czasopismo Techn. 4, 171–182.
Talavera, G., and Castresana, J. (2007). Improvement of phylogenies after removing divergent and ambiguously aligned blocks from protein sequence alignments. System. Biol. 56, 564–577. doi: 10.1080/10635150701472164
Tanaka, Y., Sasaki, N., and Ohmiya, A. (2008). Biosynthesis of plant pigments: anthocyanins, betalains and carotenoids. Plant J. 54, 733–749. doi: 10.1111/j.1365-313X.2008.03447.x
Teixido, A. L., Barrio, M., and Valladares, F. (2016). Size Matters: Understanding the Conflict Faced by Large Flowers in Mediterranean Environments. Botan. Rev. 82, 204–228. doi: 10.1007/s12229-016-91689168
Tucić, B., Tomic, V., Avramov, S., and Pemac, D. (1998). Testing the adaptive plasticity of Iris pumila leaf traits to natural light conditions using phenotypic selection analysis. Acta Oecol. 19, 473–481. doi: 10.1016/s1146-609x(99)80001-0
Vereecken, N. J., Dorchin, A., Dafni, A., Hötling, S., Schulz, S., and Watts, S. (2013). A pollinators’ eye view of a shelter mimicry system. Anna. Bot. 111, 1155–1165. doi: 10.1093/aob/mct081
Vereecken, N. J., and Schiestl, F. P. (2009). On the roles of colour and scent in a specialized floral mimicry system. Anna. Bot. 104, 1077–1084. doi: 10.1093/aob/mcp208
Wang, H., Talavera, M., Min, Y., Flaven, E., and Imbert, E. (2016). Neutral processes contribute to patterns of spatial variation for flower colour in the Mediterranean Iris lutescens (Iridaceae). Anna. Bot. 117, 995–1007. doi: 10.1093/aob/mcw036
Warren, J., and Mackenzie, S. (2001). Why are all colour combinations not equally represented as flower-colour polymorphisms? New Phytol. 151, 237–241. doi: 10.1046/j.1469-8137.2001.00159.x
Waser, N. M., and Price, M. V. (1981). Pollinator choice and stabilizing selection for flower color in Delphinium nelsonii. Evolution 35, 376–390. doi: 10.2307/2407846
Watts, S., Sapir, Y., Segal, B., and Dafni, A. (2013). The endangered Iris atropurpurea (Iridaceae) in Israel: honey-bees, night-sheltering male bees and female solitary bees as pollinators. Anna. Bot. 111, 395–407. doi: 10.1093/aob/mcs292
Wesselingh, R. A., and Arnold, M. L. (2000). Nectar production in Louisiana iris hybrids. Int. J. Plant Sci. 161, 245–251. doi: 10.1086/314252
Wessinger, C. A., and Rausher, M. D. (2012). Lessons from flower colour evolution on targets of selection. J. Experim. Bot. 63, 5741–9. doi: 10.1093/jxb/ers267
Wessinger, C. A., and Rausher, M. D. (2014). Predictability and irreversibility of genetic changes associated with flower color evolution in Penstemon barbatus. Evolution 68, 1058–1070. doi: 10.1111/evo.12340
Wester, P., and Lunau, K. (eds) (2017). “Plant-Pollinator Communication,” in How Plants Communicate with their Biotic Environment, (Netherland: Elseviere), 225–257. doi: 10.1016/bs.abr.2016.10.004
Wilson, C. (2006). Patterns in Evolution in Characters That Define Iris Subgenera and Sections. Aliso 22, 425–433. doi: 10.5642/aliso.20062201.34
Wilson, C. A. (2004). Phylogeny of Iris based on chloroplast matK gene and trnK intron sequence data. Mole. Phylogenet. Evolut. 33, 402–412. doi: 10.1016/j.ympev.2004.06.013
Wilson, C. A. (2009). Phylogenetic relationships among the recognized series in Iris section Limniris. System. Bot. 34, 277–284. doi: 10.1600/036364409788606316
Keywords: pollination syndrome, flower color evolution, color variation, mating system, pollinator shifts, shelter reward, nectar reward, ancestral trait reconstruction
Citation: Roguz K, Gallagher MK, Senden E, Bar-Lev Y, Lebel M, Heliczer R and Sapir Y (2020) All the Colors of the Rainbow: Diversification of Flower Color and Intraspecific Color Variation in the Genus Iris. Front. Plant Sci. 11:569811. doi: 10.3389/fpls.2020.569811
Received: 05 June 2020; Accepted: 15 September 2020;
Published: 13 October 2020.
Edited by:
Eduardo Narbona, Universidad Pablo de Olavide, SpainReviewed by:
Bertrand Schatz, Centre National de la Recherche Scientifique (CNRS), FranceCarolyn Wessinger, University of South Carolina, United States
Copyright © 2020 Roguz, Gallagher, Senden, Bar-Lev, Lebel, Heliczer and Sapir. This is an open-access article distributed under the terms of the Creative Commons Attribution License (CC BY). The use, distribution or reproduction in other forums is permitted, provided the original author(s) and the copyright owner(s) are credited and that the original publication in this journal is cited, in accordance with accepted academic practice. No use, distribution or reproduction is permitted which does not comply with these terms.
*Correspondence: Yuval Sapir, c2FwaXJ5QHRhdWV4LnRhdS5hYy5pbA==; U2FwaXJ5dXZhbEBnbWFpbC5jb20=
†These authors share first authorship