- 1College of Tobacco Science, Henan Agricultural University, Zhengzhou, China
- 2Sanmenxia Tobacco Company, Sanmenxia, China
- 3College of Life Science, Henan Agricultural University, Zhengzhou, China
Drought and heat stresses are two major environmental stress factors that severely threaten crop growth and productivity. Plant delay of germination 1-like (DOG1L) family genes play important roles in various developmental processes and stress responses. In our previous study, a tobacco DOG1L gene (NtDOG1L-T) was found to regulate seedling growth and drought response. Unfortunately, the role of DOG1L genes in heat stress response is yet to be studied. Here, we present data supporting the role of DOG1L genes in heat stress and possible underlying molecular mechanisms. Transcript levels of NtDOG1L-T were rapidly induced by heat or abscisic acid (ABA) treatment. Furthermore, NtDOG1L-T promoter activity was markedly activated by ABA or heat stress, as revealed by histochemical staining in transgenic tobacco seedlings. Overexpression of NtDOG1L-T in transgenic lines improved heat stress tolerance. The NtDOG1L-T-transgenic plants exhibited lower levels of reactive oxygen species (ROS) and lipid peroxidation but higher antioxidant enzyme activities in response to heat stress. Furthermore, transcript abundance of some defense-, heat-, and ABA-responsive marker genes was significantly upregulated, as shown by reverse transcription quantitative PCR (qPCR) in these transgenic plants. In conclusion, NtDOG1L-T positively regulates heat stress tolerance possibly by modulation of antioxidant capability and defense-, heat-, and ABA-related gene expression in tobacco. This study may provide valuable resource for the potential exploitation of DOG1Ls in genetic improvement of heat stress tolerance in crops.
Introduction
With aggravation of global warming, heat stress caused by high temperature has become an increasingly serious problem in agricultural production worldwide (Tubiello et al., 2007). Heat stress leads to excessive reactive oxygen species (ROS) accumulations, membrane lipid peroxidation, and metabolic disturbance, even cell death in the plant (Iba et al., 2002; Choudhury et al., 2017). Thus, heat stress adversely affects development, yield, and quality of crops. Over the long time of evolution, plants have developed multifaceted adaptive mechanisms, in which numerous stress defense genes (or proteins) are activated to cope with the heat stress (Ohama et al., 2017). Such functional genes mainly encode signaling molecules such as mitogen activated protein kinase (MAPK) cascades, cellular protective enzymes such as peroxidase (POD), transcription factors such as heat shock transcription factors (HSFs), and cellular stress proteins such as heat shock proteins (HSPs). Some of these genes have been reported to protect plant cells from damage through sustaining cellular ROS homeostasis or regulating downstream signaling pathway under heat stress (Jacob et al., 2017; Raja et al., 2017; Zhang et al., 2018). It was reported that the abscisic acid (ABA)-deficient and ABA-insensitive Arabidopsis mutants were susceptible to heat stress, whereas overexpression of the ABA-responsive element-binding protein (AREB) enhanced thermotolerance (Suzuki et al., 2016), demonstrating that ABA signaling plays a positive role in heat stress response. Nowadays, majority of the genes identified have great potential to improve heat stress tolerance in crop plants (Ul Haq et al., 2019). Thus, it is pivotal for crop genetic improvement to identify more stress-tolerant genes and explore their exact roles.
Delay of germination 1 (DOG1) is a plant-specific protein, which contains an AHG-binding motif and a heme-binding domain, but has unknown biochemical function. The first DOG1 gene was identified by genetic analysis of a major quantitative trait locus for increased seed dormancy in Arabidopsis (Bentsink et al., 2006). Since then, a great number of studies have been focused on regulatory mechanisms of the DOG1 in seed dormancy and germination in Arabidopsis. It is reported that Arabidopsis DOG1 regulates seed germination in a temperature- and gibberellin-dependent manner (Graeber et al., 2014). Interestingly, several reports have shown that the DOG1 controls seed dormancy via formation of DOG1-PP2C phosphatase complex in the ABA signaling pathway (Née et al., 2017; Nishimura et al., 2018). Most recently, the Arabidopsis DOG1 has been found to be involved in controlling seed dormancy and germination through ethylene (ET) signaling (Li et al., 2019). Also, Bryant et al. (2019) have found that Arabidopsis DOG1 is activated by the basic LEUCINE ZIPPER TRANSCRIPTION FACTOR67 (bZIP67) during seed dormancy establishment (Bryant et al., 2019). These results suggest that DOG1 is an important regulator in seed dormancy and germination in Arabidopsis.
In recent years, increasing evidence has shown that DOG1 is required for multiple developmental processes and stress responses beyond seed dormancy in Arabidopsis (Teng et al., 2008; Dekkers et al., 2016; Huo et al., 2016; Yatusevich et al., 2017). For instances, Huo et al. (2016) showed that Arabidopsis DOG1 could regulate seed dormancy and flowering time by affecting levels of miR156 and miR172 (Huo et al., 2016). That year, Dekkers et al. (2016) also reported a new role for DOG1 in seed development using genetic analyses of ABSCISIC ACID INSENSITIVE 5 (ABI5) and ABI3 mutants in Arabidopsis (Dekkers et al., 2016). Apart from developmental processes, DOG1 also participates in stress responses in Arabidopsis. It was evidenced that decrease of antisense DOG1 RNA (asDOG1) resulted in high levels of DOG1 expression and enhanced drought tolerance in Arabidopsis (Yatusevich et al., 2017). In addition, Arabidopsis DOG1 has shown to be involved in ABA-mediated sugar signaling pathway by affecting ABI4 expression (Teng et al., 2008; Vishwakarma et al., 2017). These findings indicate that DOG1 plays vital roles in developmental and stress responses in Arabidopsis.
In crop plants, functional studies of DOG1-like (DOG1L) genes are still in infancy. It was reported that cereal DOG1L genes shared similar structural features among rice, wheat, barley, maize, and sorghum and had conserved roles in seed dormancy control in transgenic plants (Ashikawa et al., 2013, 2014). Common tobacco (Nicotiana tabacum, genome TTSS) is an allotetraploid species, which originates from interspecific hybridization of Nicotiana sylvestris (2n = 24, genome SS) and Nicotiana tomentosiformis (2n = 24, genome TT) about 200,000 years ago (Sierro et al., 2014). More importantly, tobacco is a global commodity with great economic value (Sierro et al., 2014). Nowadays, environmental stresses such as drought, heat, and chilling are still major limiting factors for productivity and quality of tobacco (Su et al., 2017). Recently, we have discovered a novel drought-responsive gene (named NtDOG1L), which encodes a DOG1-like protein by transcriptome analyses. Further evidence showed that the tobacco DOG1L was involved in drought tolerance via transgenic overexpression approach (Zhang et al., 2019). Unfortunately, the role of DOG1L genes in heat stress response is yet to be studied. Here, we further investigate its heat stress response and possible function mechanisms using the transgenic tobacco lines.
Materials and Methods
Plant Materials, Growth Conditions, and Stress Treatments
Nicotiana tabacum cv. Ws38 [wild type (WT)] and NtDOG1L-T transgenic lines (background Ws38) were used in this study. The DOG1L-T transgenic tobacco lines were produced as described previously (Zhang et al., 2019). Briefly, the CDS fragment of the NtDOG1L-T was subcloned into the binary vector pWM101 downstream of the CaMV 35S promoter. The resulting construct (pWM101-35S:NtDOG1L-T) was introduced into Agrobacterium tumefaciens strain GV3101, which was transformed into the tobacco cv. WS38 via the Agrobacterium-mediated leaf disc transformation method. Positive transgenic lines were screened and further identified by PCR and quantitative PCR (qPCR). Three independent lines (OE-5, OE-6, and OE-9) with high transcript levels were used for further analysis.
Tobacco seeds were germinated and grown on MS medium in a culture chamber at 25°C with a photoperiod of 16/8 h light/dark. After 10 days, these seedlings were transplanted into pots with a mixture of vermiculite and soddy soil (1:1) for cultivation in the growth room. The seedlings were irrigated weekly with Hoagland’s nutrient solution as described previously (Zhang et al., 2019). For heat and ABA treatments, 2-week-old tobacco seedlings were maintained at 45°C for heat stress (65% relative humidity) or were sprayed by 50 μM ABA at 25°C in growth chambers, and seedlings were sampled separately at various time points after each treatment. During heat stress, the pots with the nutrient soil were covered with nylon membranes to reduce water loss and avoid a dehydration stress of the seedlings. Harvested samples were immediately frozen in liquid nitrogen and then stored at −85°C for gene expression analyses.
Tobacco DOG1L-T promoter:GUS Fusion Vector Construction and Transient GUS Expression Assays Under ABA or Heat Stress
The NtDOG1L-T promoter fragment (about 1.4 kb) was amplified by PCR with corresponding primers (Supplementary Table S1). Subsequently, the fragment was subcloned into the pCAMBIA1381 vector containing a β-glucuronidase (GUS) reporter with EcoRI/HindIII restriction sites and confirmed by sequencing.
Transformation and culture of the Agrobacterium harboring the recombinant plasmid (pDOG1L-T-Pro:GUS) were conducted as described by Xu et al. (2019). Then, the sterile cultured tobacco seedlings at 5- or 6-leaf stage were infiltrated by A. tumefaciens with the promoter:GUS construct. After 12 h the infiltrated seedlings were exposed to heat (45°C) or ABA (50 μM) treatment for 2 h, and then 6–8 seedlings for each treatment were collected for GUS histochemical staining. The infiltrated seedlings in MS plates under normal conditions were treated as controls. The transient GUS expression assays were carried out using a protocol described previously (Xu et al., 2019).
Histochemical GUS Staining, Imaging, and Quantification
GUS staining assay was conducted as previously described (Xu et al., 2019). Briefly, tobacco seedlings were firstly incubated in GUS staining buffer (100 mM sodium phosphate, 10 mM EDTA, 1 mM 5-bromo-4-chloro-3-indolyl-β-D-glucuronic acid, and 0.5 mM potassium ferrocyanide) at 37°C for 12 h. Then, the seedlings were destained in 70% ethanol for 12 h, and imaged using a ZEISS upright light microscope equipped with a camera. Finally, quantification of GUS signal in aerial parts of the stained seedlings was done using ImageJ 1.41 software1 as described previously (Béziat et al., 2017).
Heat Stress Tolerance Analysis of Tobacco DOG1L-T Transgenic Plants
The WT and transgenic tobacco lines (OE-5, OE-6, and OE-9) were cultured in MS plates under a 16/8 h light/dark cycle at 25°C in culture chamber for 2 weeks, and then the seedlings were transferred to pots filled with nutrient soil in a growth room where they were regularly cultivated for additional 6 weeks. And then, 8-week-old WT and transgenic lines were transferred to a culture chamber and subjected to a heat stress treatment (45°C) for 24 h. The corresponding WT and transgenic lines grown in the other chamber at 25°C were treated as controls. After that, phenotypes of treated and control plants were photographed, and electrolyte leakage and chlorophyll content in stressed or control plants were determined following the procedure described previously (Zhang et al., 2019). These experiments were performed in three biological replicates.
Determination of Stress-Related Physiological and Biochemical Parameters
Eight-week-old transgenic lines and WT tobacco plants were transferred to a growth chamber and exposed to heat stress (45°C) for 24 h. Meanwhile, the corresponding WT and transgenic plants were grown in the other chamber under normal conditions as controls. After treatments, leaf samples were immediately taken for measurements of H2O2 and malondialdehyde (MDA) contents and major antioxidant enzyme activities, including superoxide dismutase (SOD), catalase (CAT), and POD in stressed and control plants, as described by Zhang et al. (2019). Accumulations of O2− and H2O2 in leaf discs from treated or control plants were detected by nitro blue tetrazolium (NBT) and 3,3'-diaminobenzidine (DAB) staining, respectively as described by Sun et al. (2019).
Reverse Transcription Quantitative PCR
The reverse transcription qPCR (RT-qPCR) was employed to examine transcript levels of some heat-responsive and defense genes. Total RNA extraction, first-strand cDNA synthesis, and qPCR assay were performed as described previously (Su et al., 2017). The tobacco Actin2 was used as internal reference genes, and relative transcript levels were calculated as described by us (Zhang et al., 2019). Three biological replicates and three technical replicates were applied for the whole assays. The primes used for qPCR are listed in Supplementary Table S1.
Statistical Analysis
The data were expressed as the means ± SE and subjected to statistical analysis using the SPSS (version 17.0, SPSS Inc., United States). Data were analyzed by one-way ANOVA, and means were compared by Duncan’s multiple range test at a significance level of p < 0.05. Histograms were plotted using the GraphPad Prism (v 8.01).
Results
Transcript Profiles of Two Tobacco DOG1L Genes in Response to ABA or Heat Stress
In our previous study, two homologs of the DOG1L genes (NtDOG1L-T and NtDOG1L-S) from the tetraploid tobacco N. tabacum (Nt, TTSS) were identified. Moreover, the two DOG1L genes shared high identity (98.7%) at amino acid level (Zhang et al., 2019). To further explore their responses to ABA or heat stress, time-course analyses of transcript levels of the two DOG1L genes in tobacco were conducted by RT-qPCR (Figure 1). Upon heat stress, the transcripts of both DOG1L genes increased significantly and peaked at 2 h until the end of the stress and then gradually decreased during 6 h of the heat stress (Figures 1A,B). Particularly, the NtDOG1L-T showed remarkable upregulation response (about 15-fold increase at 2 h of the stress). Similarly, both DOG1L genes responded alike during 24 h of the ABA treatment. Noticeably, the NtDOG1L-T expression increased rapidly after 1 h, and reached a peak at 6 h (about 5-fold increase), then gradually decreased, and ultimately maintained higher levels during the whole treatment (Figures 1C,D). These data imply that two DOG1L genes showed similar response pattern upon ABA or heat stress. Moreover, transcriptional response of the NtDOG1L-T was much stronger than that of the NtDOG1L-S during heat stress.
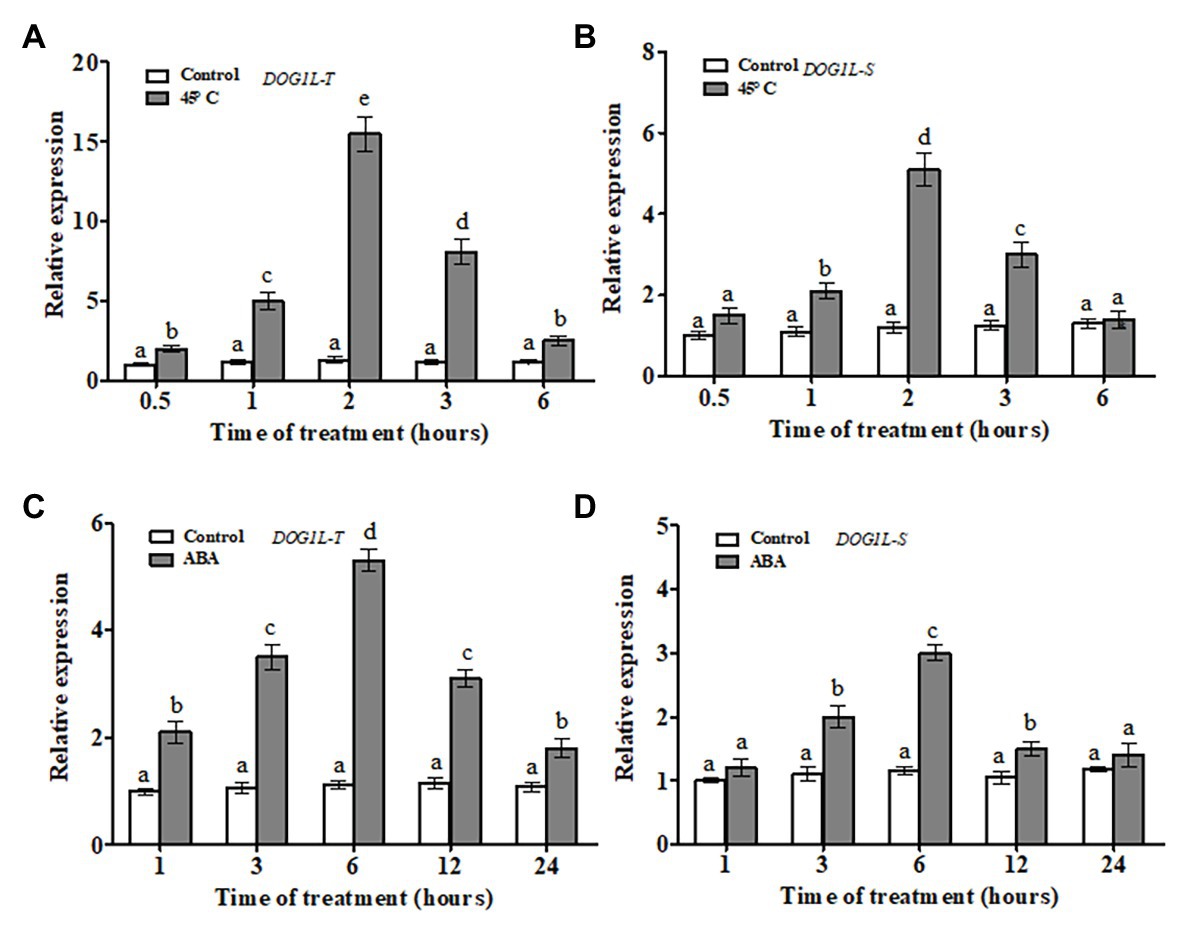
Figure 1. Transcript profiles of tobacco delay of germination 1-like (DOG1L) genes in response to abscisic acid (ABA) or heat stress. Two-week-old tobacco seedlings were treated with 45°C heat or 50 μM ABA, and seedlings were sampled at various time points after each treatment to extract RNA, and then transcript levels of both DOG1L-T and DOG1L-S were checked by quantitative PCR (qPCR) under heat stress (A,B) or ABA treatment (C,D). For each reverse transcription quantitative PCR (RT-qPCR), the transcript levels of tobacco reference gene Actin2 were also evaluated in various samples. For each experiment, three technical replicates were conducted. Data shown are Mean ± SE of three independent experiments. Statistical analysis was performed using ANOVA test (p < 0.05) and significant differences are indicated by different letters.
Promoter Activity of the NtDOG1L-T in Response to ABA or Heat Stress
To further understand its transcriptional responses to ABA or heat stress, we first isolated NtDOG1L-T promoter fragment (about 1.4 kb) and analyzed its regulatory elements using PlantCARE database.2 As shown in Figure 1A, in addition to a number of core cis-acting elements, such as TATA-box and CAAT-box elements, some known elements involved in hormone and abiotic stress responses were found in this region. Particularly, these elements contain two ABA response elements (ABRE,ACGTG; for ABA response), one heat shock response element (HSE,GAAXTTC; for heat response), one MYB binding site (MBS, CAACTG; for drought response), and one TCA-element (CAGAAAAGGA, for salicylic acid response; Figure 2A).
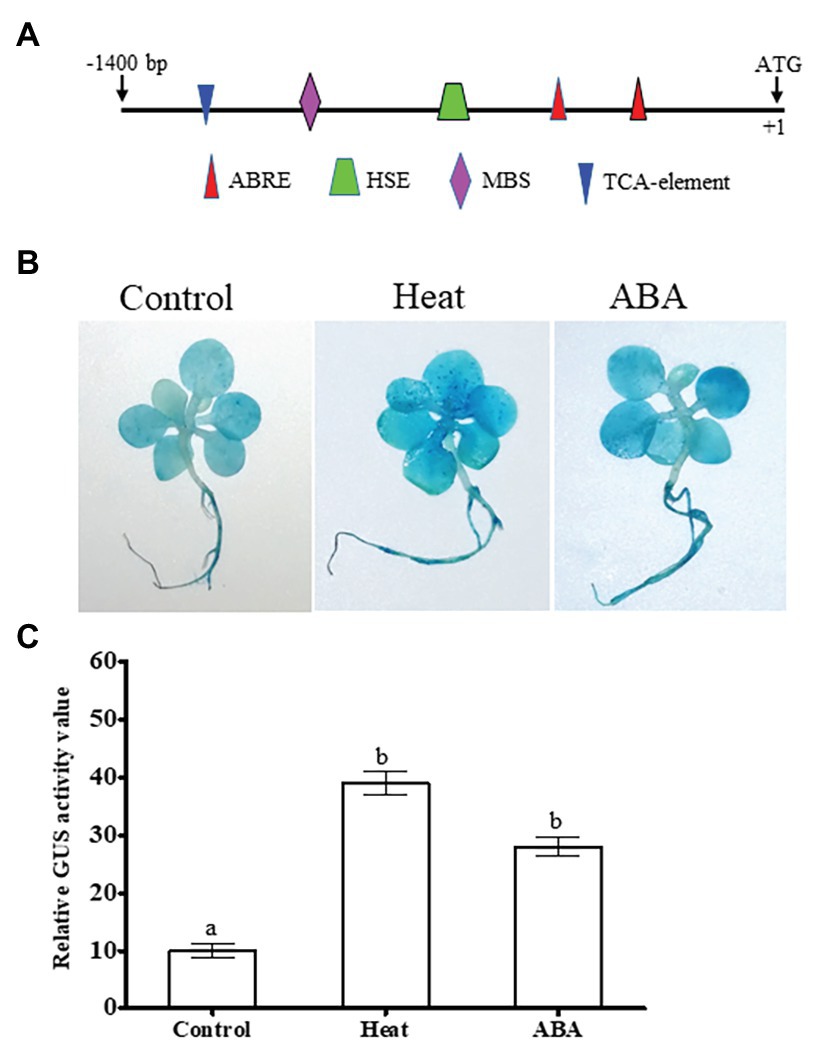
Figure 2. Promoter activity analysis of the tobacco DOG1L-T by histochemical staining under ABA or heat stress. (A) Promoter cis-regulatory elements of the tobacco DOG1L-T. Putative cis-elements in the promoter region of the DOG1L-T were predicted by PlantCARE. (B) β-glucuronidase (GUS) histochemical staining of tobacco seedlings transiently expressing the DOG1L-T promoter upon heat or ABA treatment. (C) Relative quantitative analysis of GUS activity by ImageJ. In (B) sterile cultured tobacco seedlings at 5- or 6-leaf stage were infiltrated by Agrobacterium tumefaciens harboring DOG1L-T-Pro:GUS construct. After 12 h the infiltrated seedlings were exposed to heat (45°C) or ABA (50 μM) treatment for 2 h, and then 6–8 seedlings for each treatment were collected for GUS histochemical staining. The seedlings grown in the solid MS medium were treated as controls. In (C) error bars represented SE of three replicates. Statistical analysis was performed using ANOVA test (p < 0.05) and significant differences are indicated by different letters.
Histochemical detection of the NtDOG1L-T promoter activity was conducted with GUS staining in these heat- or ABA-treated seedlings. As shown in Figure 2B, transiently expressed NtDOG1L-T-Pro:GUS tobacco seedlings showed much stronger GUS staining upon heat or ABA treatment than control conditions (Figure 2B). These significant differences were also observed from the quantitative values in GUS staining intensity in these transgenic tobacco seedlings under heat, ABA, and control conditions (Figure 2C). These results indicate that transcription of the NtDOG1L-T is regulated by ABA or heat stress.
Responses of Transgenic NtDOG1L-T Overexpression Lines to Heat Stress
We next examined the function of NtDOG1L-T in heat stress tolerance using the transgenic tobacco lines overexpressing NtDOG1L-T cDNA under 35S cauliflower mosaic virus (CaMV) constitutive promoter. Eight-week-old transgenic lines (OE-5, OE-6, and OE-9), along with WT tobacco plants were transferred to a culture chamber and subjected to a heat stress treatment (45°C). Meanwhile, the corresponding WT and transgenic lines grown in the other chamber at 25°C were treated as controls. After 24 h of treatment, the WT plants showed relative higher yellowing than these transgenic lines (Figure 3A). In contrast, under normal conditions, there were no obvious phenotypic differences between WT and transgenic lines (Figure 3A). Accordingly, remaining chlorophyll contents in these transgenic lines were significantly higher than that in WT plants (Approximately 56% on the average; Figure 3B). Furthermore, the electrolyte leakage rate in WT plants was about 45% higher than those in these transgenic lines (Figure 3C). These results evidenced that overexpression of NtDOG1L-T in transgenic overexpression lines improved tolerance to heat stress.
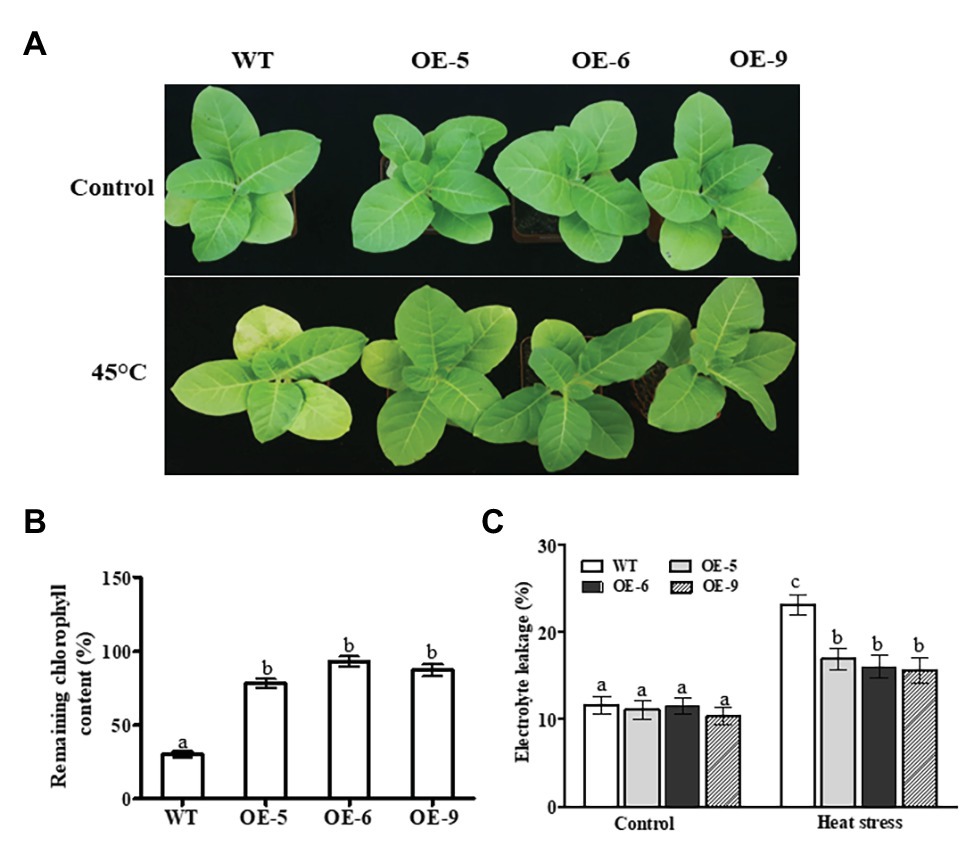
Figure 3. Phenotypic analyses of DOG1L-T transgenic tobacco lines under heat stress. (A) Phenotypes of 8-week-old wild type (WT) and transgenic lines subjected to a heat stress treatment (45°C) for 24 h. (B) Remaining chlorophyll contents in WT and transgenic lines after 24 h-heat stress. (C) Electrolyte leakage rates in transgenic lines and WT after 24 h-heat stress. The WT and transgenic lines (OE-5, OE-6, and OE-9) were cultured in MS plates under a 16/8 h light/dark cycle at 25°C in culture chamber for 2 weeks, and then the seedlings were transferred to pots filled with nutrient soil in growth room where they were regularly cultivated for additional 6 weeks. And then, 8-week-old WT and transgenic lines were transferred to a culture chamber and subjected to a heat stress treatment (45°C) for 24 h. The corresponding WT and transgenic lines grown in the other chamber at 25°C were treated as controls. In both (B,C) data are presented as means ± SE. Statistical analysis was performed using ANOVA test (p < 0.05) and significant differences are indicated by different letters.
Changes in Lipid Peroxidation and Antioxidant Enzyme Activities in NtDOG1L-T Transgenic Overexpression Lines Under Heat Stress
To uncover potential physiological mechanisms by which NtDOG1L-T improved heat stress tolerance, we measured MDA content and several antioxidant enzyme activities in NtDOG1L-T transgenic and WT plants under heat stress. MDA, the final product of membrane lipid peroxidation in plants, is an important parameter to reflect the potential antioxidant capacity of plant cells. Under control conditions, both transgenic and WT plants showed no significant differences in MDA content (Figure 4A). After heat stress, the MDA content in both transgenic and WT plants was significantly elevated, but the average increase magnitude of the MDA content in these transgenic lines was much lower than that in the WT (Figure 4A). This result indicated that these NtDOG1L-T transgenic overexpression lines showed less membrane damage than the WT upon heat stress.
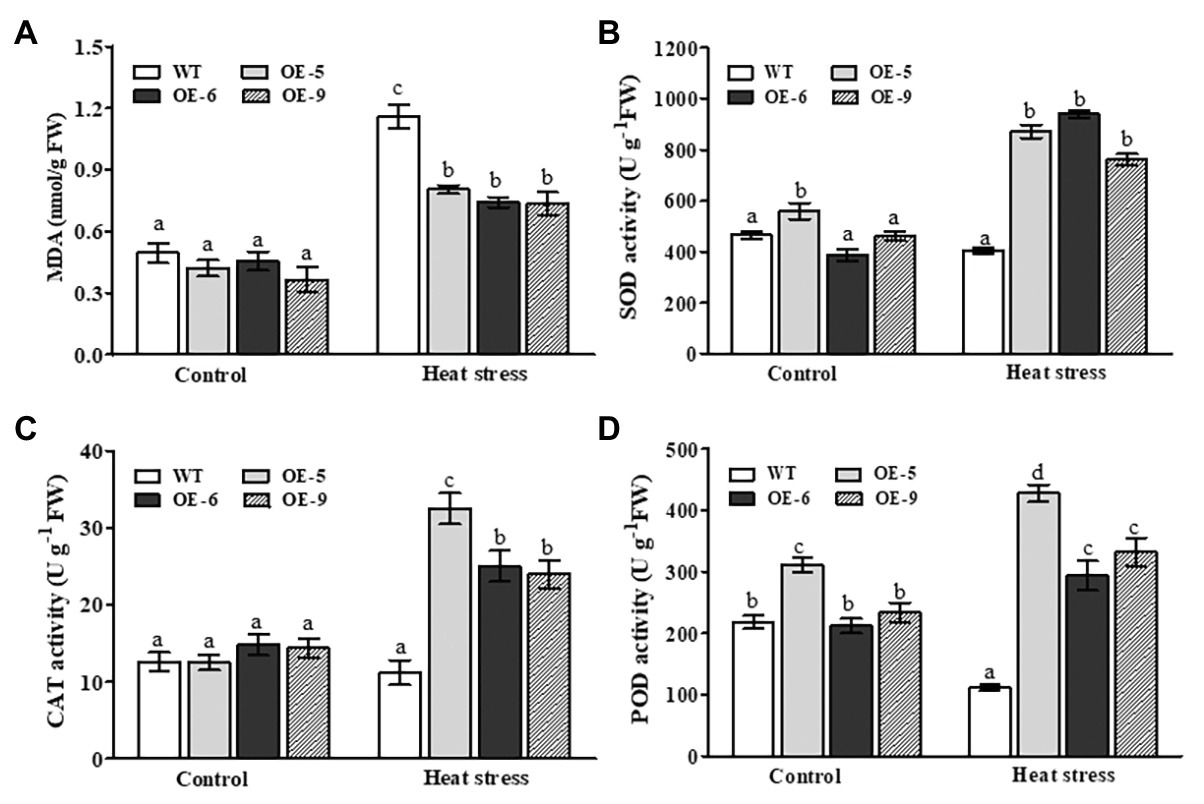
Figure 4. Changes in membrane peroxidation and antioxidant enzymes in the WT and DOG1L-T transgenic tobacco lines under heat stress. The malondialdehyde (MDA) content (A) and activity levels of antioxidant enzymes superoxide dismutase (SOD; B), catalase (CAT; C), and peroxidase (POD; D) in transgenic lines and WT plants upon 24 h-heat stress exposure. Statistical analysis was performed using ANOVA test (p < 0.05) and significant differences are indicated by different letters.
We next assayed the activities of three antioxidant enzymes, SOD, CAT, and POD between NtDOG1L-T transgenic and WT plants upon heat stress. As shown in Figures 4B–D, after 24 h of heat stress, compared to their corresponding controls, activities of the three antioxidant enzymes in NtDOG1L-T transgenic lines markedly increased, but not in the WT plants. Moreover, these transgenic lines had more magnitudes of increases than the WT plants (Figures 4B–D). This result, along with fluctuations of MDA content, suggested that NtDOG1L-T overexpression activated several antioxidant enzymes and attenuated membrane damage upon heat stress in tobacco plants.
Changes in Reactive Oxygen Species Levels in NtDOG1L-T Transgenic Overexpression Lines Under Heat Stress
To test the ROS scavenging activity of transgenic plants overexpressing NtDOG1L-T, accumulations of O2− and H2O2 were examined upon heat stress. The 8-week-old WT and transgenic overexpression lines were exposed to heat stress (45°C) for 24 h, and then leaf discs from these stressed plants were sampled for DAB or NBT staining. As shown in Figures 5A,B, upon heat stress these three transgenic lines showed lower accumulations of O2− and H2O2 than the WT in the leaf discs, as observed by NBT staining (brown pigment) and DAB staining (blue pigment; Figures5A,B). In contrast, under control conditions, there were no significant differences in O2− or H2O2 accumulation between WT and transgenic lines, except for the line OE-6 (Figures5A,B). Furthermore, we quantified the differences in H2O2 content between transgenic lines and WT plants under heat or control conditions. As shown in Figure 5C, the three transgenic lines accumulated much lower H2O2 than the WT when exposed to 24 h of heat stress (Figure 5C). In addition, under control conditions, as shown in the Figure 5C, H2O2 content in the OE-6 was obviously lower than that in the WT (Figure 5C). These results, together with changes of antioxidant enzyme activities, indicated that NtDOG1L-T overexpression improved antioxidant enzyme activities, thereby reducing ROS accumulations and alleviating oxidative damage in transgenic overexpression lines under heat stress.
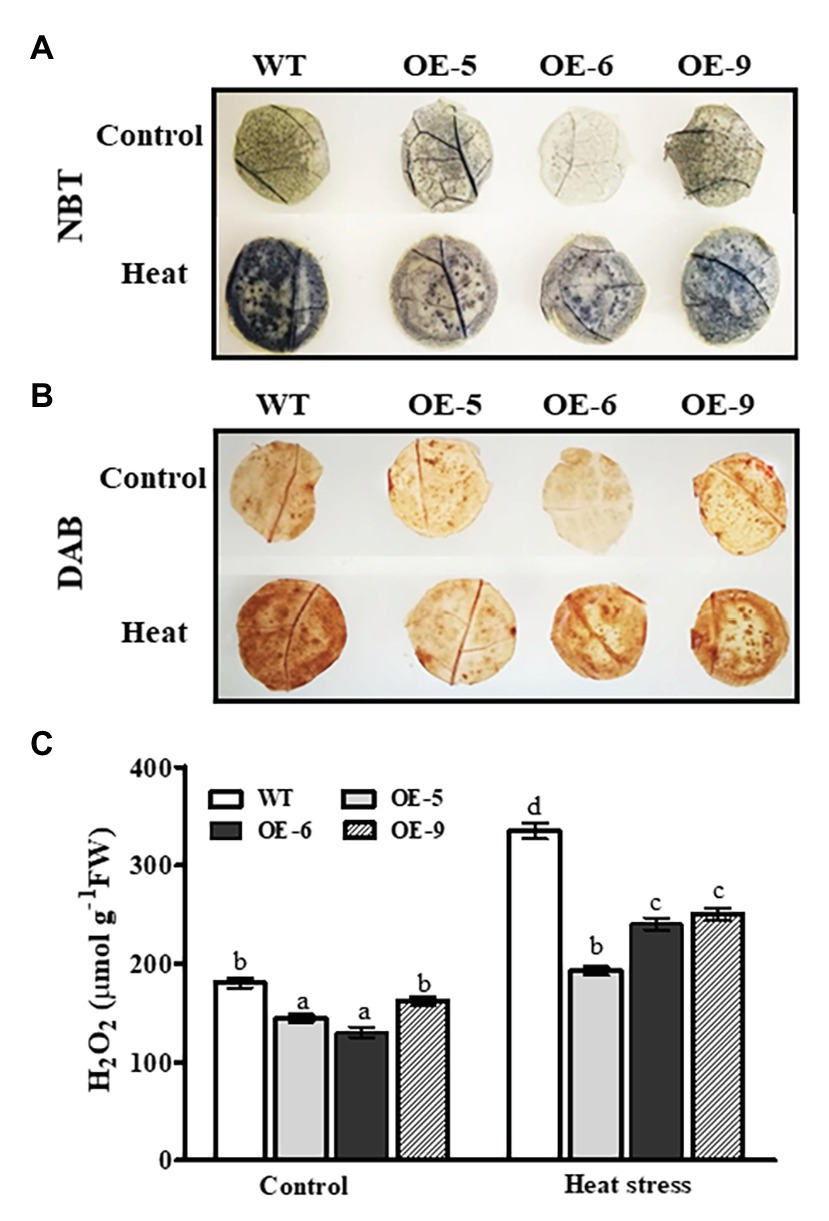
Figure 5. O2− and H2O2 levels in the WT and DOG1L-T transgenic tobacco lines under heat stress. (A) O2− production in leaf discs of WT and transgenic lines upon heat exposure. (B) H2O2 accumulation in leaf discs of WT and transgenic lines upon heat exposure. (C) Quantitative measurement of total H2O2 content in WT and transgenic lines upon heat exposure. The 8-week-old WT and transgenic overexpression lines were exposed to heat stress (45°C) for 24 h, and then, leaf discs from these stressed plants were taken for 3,3'-diaminobenzidine (DAB) and nitro blue tetrazolium (NBT) staining as described in the experimental procedures. Data are presented as means ± SE. Statistical analysis was performed using ANOVA test (p < 0.05) and significant differences are indicated by different letters.
Transcriptional Changes of Antioxidant- and Defense-Related Genes in NtDOG1L-T Transgenic Tobacco Lines Under Heat Stress
To elucidate possible molecular mechanisms of NtDOG1L-T-conferred heat tolerance, we used RT-qPCR assays to check transcript levels of several representative antioxidant- and defense-related genes between transgenic lines and WT plants under control or heat stress conditions. These genes included three antioxidant genes (superoxide dismutase-encoding gene NtSOD1, catalase-encoding gene NtCAT1, and cytoplasmic peroxidase NtPOD1) and three stress defense genes (early response protein 10-encoding genes NtERD10C and NtERD10D, and late embryogenesis abundant 5-encoding gene NtLEA5), which have been evidenced to play pivotal roles in protecting tobacco plants from abiotic stresses (Wang et al., 2019; Zhang et al., 2019). As shown in Figure 6-upper lanes, under heat stress transcript levels of the three antioxidant genes in the NtDOG1L-T transgenic lines were significantly upregulated, compared with those in the WT plants (Figure 6, upper lanes). In particular, the average expression level of the NtPOD1 in these transgenic lines was about three times that of the WT plants (Figure 6, upper lanes). In contrast, under control conditions no clear differences were observed in these antioxidant genes, except for the NtPOD1, between WT and transgenic lines (Figure 6, upper lanes). Thereafter, we examined changes in expression levels of the three defense genes NtERD10C, NtERD10D, and NtLEA5 between WT and NtDOG1L-T transgenic lines under heat stress. As shown in Figure 6-lower lanes, upon heat stress all the three defense-related genes in the transgenic lines showed significantly more transcripts than those in the WT plants (Figure 6, lower lanes). Noticeably, under control conditions these transgenic lines had significantly higher transcript levels of the NtERD10D than the WT plants (Figure 6, lower lanes). These data suggested that NtDOG1L-T overexpression upregulated the antioxidant- and defense-related gene expression upon heat stress.
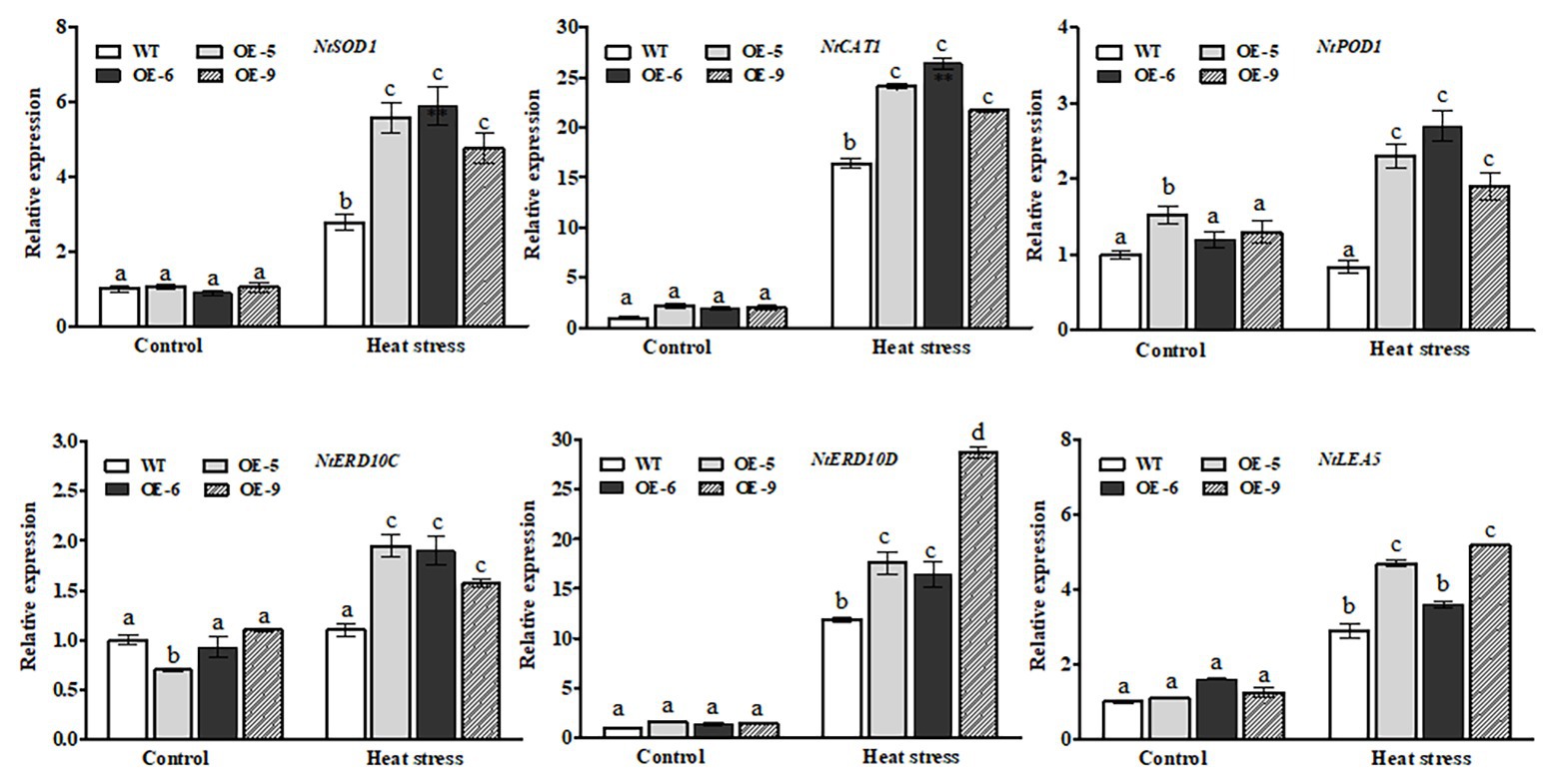
Figure 6. Transcriptional expression of antioxidant- and defense-related genes in WT and transgenic tobacco lines under heat stress. Eight-week-old WT and transgenic overexpression lines were exposed to heat stress (45°C) for 24 h, and leaves were sampled for RNA extraction, cDNA synthesis, and qPCR analysis. For each RT-qPCR, the transcript levels of tobacco reference gene Actin2 were also evaluated in various samples. For each experiment, three technical replicates were conducted. Data shown are mean ± SE of three independent experiments. Statistical analysis was performed using ANOVA test (p < 0.05) and significant differences are indicated by different letters.
Transcriptional Changes of Heat- or ABA-Responsive Genes in NtDOG1L-T Transgenic Tobacco Lines Under Heat Stress
It is well known that HSPs, such as HSP70, HSP90, and HSP101, act as important molecular chaperones to protect many functional proteins from misfolding and aggregation during adaptation of plants to heat stress (von Koskull et al., 2007). Therefore, we performed RT-qPCR to examine the expression of these tobacco HSP genes between the NtDOG1L-T transgenic lines and WT plants upon heat stress. As shown in Figure 7-upper lanes, NtDOG1L-T transgenic lines exhibited significantly higher levels of transcripts for NtHSP70, NtHSP90, and NtHSP101 than the WT upon 24 h of heat stress (Figure 7, upper lanes). In contrast, no significant differences were detected in expression levels of these three HSPs between WT and transgenic tobacco lines under control conditions (Figure 7, upper lanes).
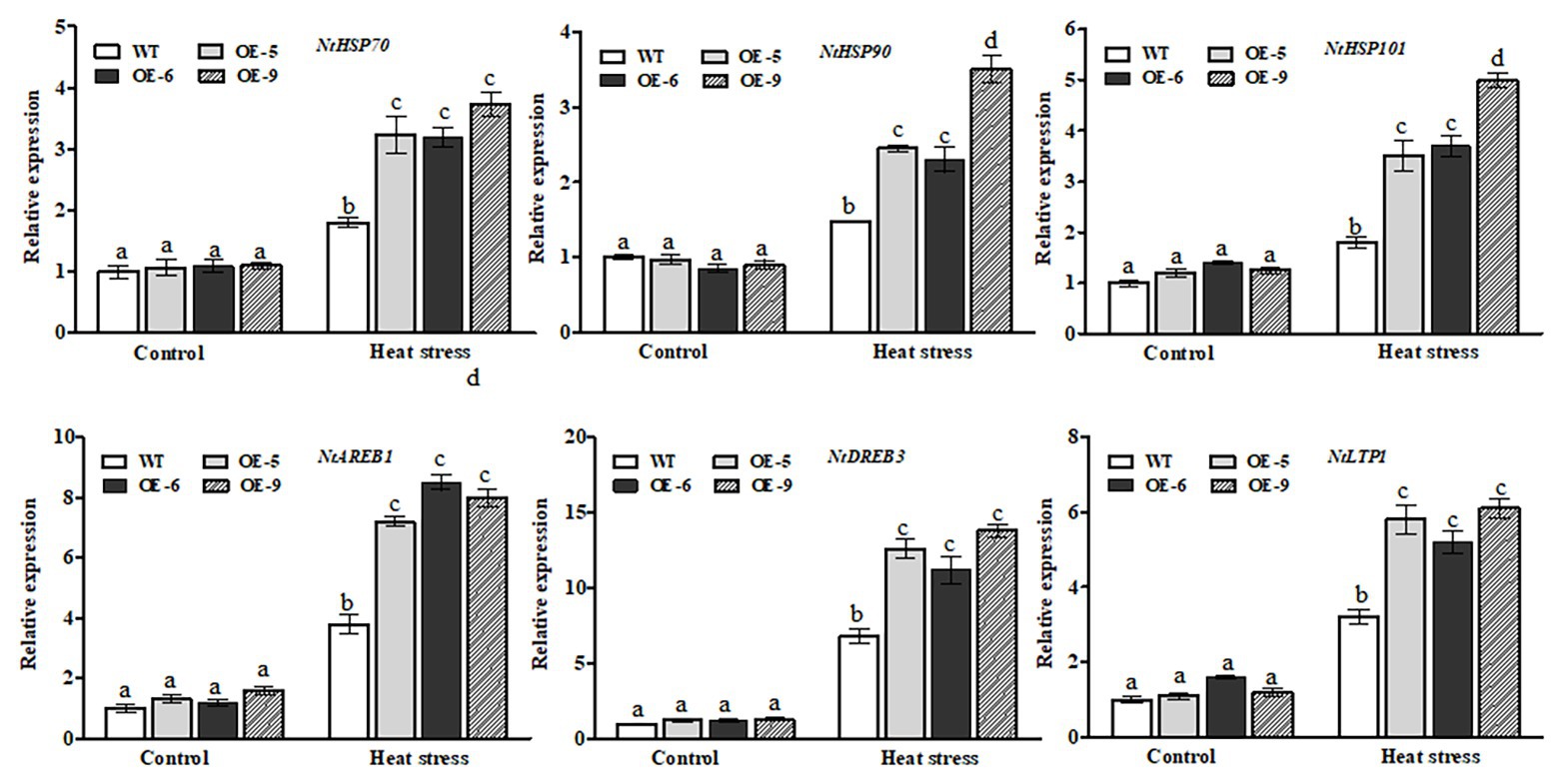
Figure 7. Transcriptional expression of heat- or ABA-responsive genes by qPCR in WT and transgenic tobacco lines under heat stress. Eight-week-old WT and transgenic overexpression lines were exposed to heat stress (45°C) for 24 h, and leaf samples were taken for RNA extraction, cDNA synthesis, and qPCR analysis. For each RT-qPCR, the transcript levels of tobacco reference gene Actin2 were also evaluated in various samples. For each experiment, three technical replicates were conducted. Data shown are mean ± SE of three independent experiments. Statistical analysis was performed using ANOVA test (p < 0.05) and significant differences are indicated by different letters.
Expression of the NtDOG1L-T was upregulated by exogenous ABA (Figures 1, 2), which promoted us to examine transcriptional changes in ABA-responsive genes between NtDOG1L-T transgenic lines and WT plants upon heat stress. These ABA-responsive marker genes included AREB1 (encoding ABA response element-binding protein 1), DREB3 (encoding dehydration-responsive element-binding protein 3), and LTP1 (encoding lipid transfer protein 1), which have been shown to be involved in ABA or heat stress response (Xia et al., 2013, 2016; He et al., 2017). As shown in Figure 7-lower lanes, transcript levels of all the three ABA response genes in these NtDOG1L-T transgenic lines exhibited greater degrees of upregulation than the WT upon heat stress (Figure 7, lower lanes). These results indicate that NtDOG1L-T overexpression activated expression of the heat- and ABA-responsive genes under heat stress.
Discussion
To date, most of the studies have been focused on roles of Arabidopsis DOG1 family genes in important developmental processes, including seed dormancy and germination, flowering, and seed maturation (Bentsink et al., 2006; Dekkers et al., 2016; Huo et al., 2016). Recently, through transcriptome analyses, we have discovered a novel drought-responsive gene NtDOG1L-T, which encodes a DOG1-like protein and is involved in drought tolerance in tobacco (Zhang et al., 2019). In this study, we report molecular and physiological responses of the NtDOG1L-T transgenic overexpression plants under heat stress. Our results have demonstrated that NtDOG1L-T overexpression improves heat stress tolerance by regulating antioxidant metabolism and defense and heat stress response genes.
There are five DOG1Ls in Arabidopsis and six DOG1Ls in rice (Ashikawa et al., 2013). In tobacco, DOG1Ls are encoded by two genes (NtDOG1L-S and NtDOG1L-T) in the genome, which are highly homologous to the Arabidopsis DOG1-like 4 (DOGL4; Zhang et al., 2019). Previous studies have shown that DOG1 family proteins play important roles in various developmental processes, such as seed dormancy and germination, flowering, and seed development. In our study, we did not observe significant changes in seed dormancy and germination, and flowering time in NtDOG1L-T transgenic plants. Unexpectedly, we found that NtDOG1L-T overexpression promoted tobacco seedling growth (Zhang et al., 2019). In accordance with our observations, Sall et al. (2019) reported that reduced seed dormancy and longevity phenotypes were found in dog1 seeds but not in dogl4 mutants (Sall et al., 2019), suggesting that DOG1L genes might have functional divergence in seed dormancy function in Arabidopsis. In addition to developmental cues, Arabidopsis DOG1 has been suggested to regulate drought tolerance (Yatusevich et al., 2017). Similarly, our recent research has found that overexpression of the NtDOG1L-T enhanced tolerance to drought stress in transgenic tobacco (Zhang et al., 2019). Furthermore, we uncovered the potential of NtDOG1L-T in heat stress tolerance in tobacco in this study. These data indicate that plant DOG1Ls have functional diversification.
Heat stress can induce excessive ROS accumulations, which are harmful and result in cell death. Thus, ROS homeostasis is tightly controlled in plants. CAT, POD, and SOD are three ROS-scavenging enzymes, which are necessary for ROS detoxification (Baxter et al., 2014). In our present study, NtDOG1L-T transgenic overexpression lines exhibited higher antioxidant enzyme activities and less H2O2 and O2− accumulations than the WT during heat stress (Figures 4, 5). Furthermore, the transcripts of antioxidant-related genes NtPOD1, NtSOD1, and NtCAT1 were significantly upregulated in the NtDOG1L-T transgenic plants during heat stress (Figure 6). These data suggested that NtDOG1L-T might enhance antioxidant capability for excess ROS scavenging by activating these antioxidant enzymes, thereby improving heat stress tolerance. Consistent with our conclusion, it was reported that overexpression of the SOD and APX genes in transgenic potato plants enhanced tolerance to heat and oxidative stresses (Kim et al., 2010). Similarly, Guan et al. (2013) evidenced that Arabidopsis copper/zinc superoxide dismutase 1 (SOD1) and SOD2 functioned as ROS scavengers to regulate thermotolerance (Guan et al., 2013). By contrast, impairment of APX or CAT decreased heat stress tolerance in Arabidopsis (Vanderauwera et al., 2011). Together, NtDOG1L-T conferred thermotolerance by enhancing antioxidant capability that efficiently maintained ROS homeostasis and reduced membrane damage under heat stress. In further study, it is needed to dissect the precise role of NtDOG1L-T in ROS homeostasis by multiple-omics approaches using these transgenic tobacco lines during heat stress.
Emerging evidence has shown that the phytohormone ABA plays a positive role in plant thermotolerance response (Larkindale et al., 2005; Huang et al., 2016; Suzuki et al., 2016). Our results indicate that NtDOG1L-T may be involved in ABA-mediated thermotolerance response in tobacco. This inference is based on the following three aspects: firstly, transcript levels of the NtDOG1L-T were rapidly induced by heat or ABA treatment, as revealed by qPCR (Figure 1). Secondly, there are two ABREs for ABA response and one HSE for heat stress response in promoter region of the NtDOG1L-T (Figure 2A). Furthermore, histochemical staining of the NtDOG1L-T-Pro:GUS transgenic tobacco seedlings showed that promoter activity of the NtDOG1L-T was markedly activated by ABA or heat stress (Figures 2B,C). These data suggest that transcription of the NtDOG1L-T gene is regulated by ABA or heat stress. Thirdly, transcript levels of ABA signaling genes NtAREB1, NtDREB3, and NtLTP1 and heat-responsive genes NtHSP70, NtHSP90, and NtHSP101 were significantly elevated in NtDOG1L-T transgenic lines upon heat stress (Figure 7), suggesting that the enhanced heat tolerance in the NtDOG1L-T transgenic plants might be associated with ABA signaling and HSP expression. In support of this notion, it was found that ABA conferred heat tolerance by inducing accumulations of several HSPs, including HSP70, HSP 90, and HSP101 in plants (Li et al., 2014). Interestingly, the Arabidopsis DOG1 gene was also found to regulate seed development through genetically interacting with ABI3 and modulating ABI5 expression. Furthermore, transcriptome analysis revealed that hundreds of genes including LEAs and HSPs were altered in the dog1 mutant (Dekkers et al., 2016). In addition, the transcription factors ABI3 and ABI5 have been evidenced to be involved in plant development, abiotic stress responses, and phytohormone crosstalk (Skubacz et al., 2016). Based on above reports plus our results, it is reasonable to speculate that NtDOG1L-T regulates thermotolerance response possibly by affecting ABA signaling, thereby activating downstream defense and heat stress-responsive gene expression in tobacco. Thus, it is of interest to explore exact role of the NtDOG1L-T in ABA signaling pathway during heat stress via proper tobacco mutants such as abi3 and abi5.
Together, our data have evidenced that NtDOG1L-T positively regulates heat stress tolerance at least partly due to increases of antioxidant capability and upregulation of the expression of defense-, heat-, and ABA-related genes in tobacco (Figure 8). This study provides valuable resource for the potential exploitation of DOG1Ls in genetic improvement of heat stress tolerance in crops.
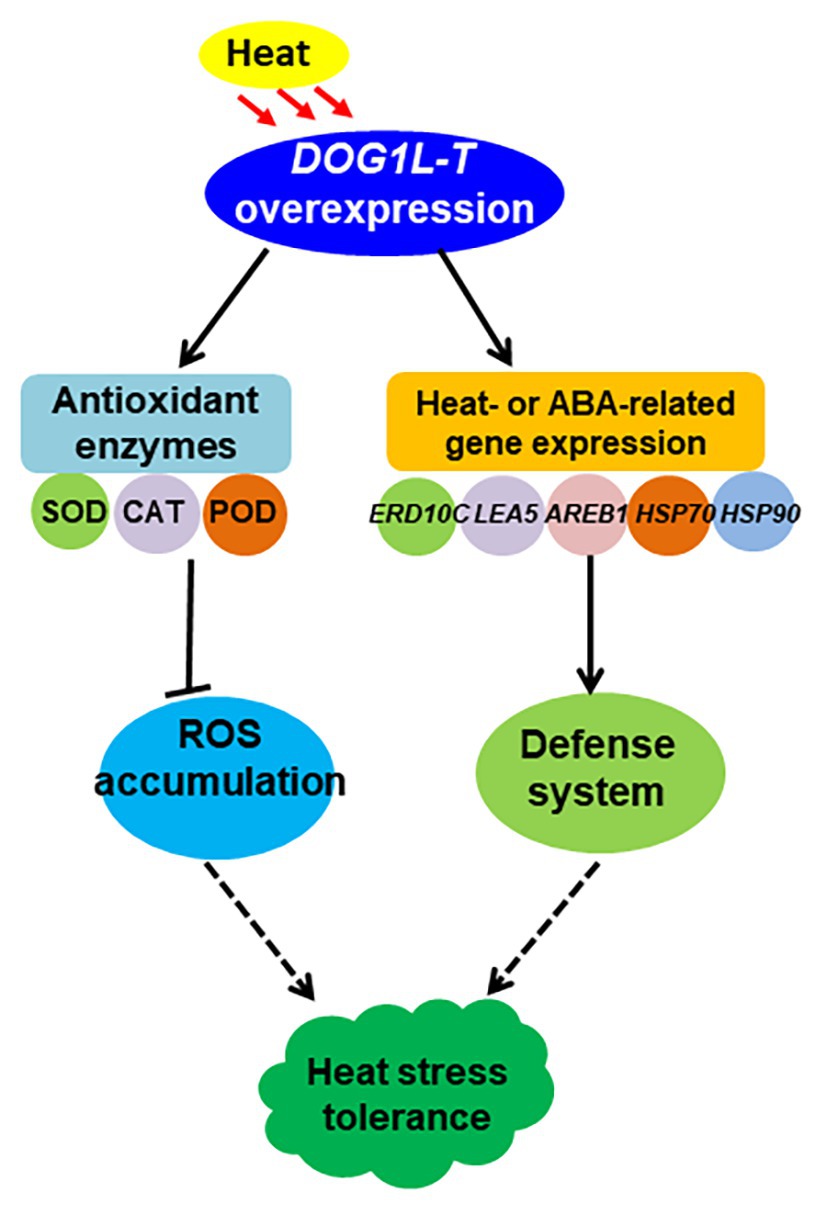
Figure 8. A model for the role of tobacco DOG1L-T under heat stress. NtDOG1L-T improves antioxidant machinery by activating the transcription of SOD, CAT, and POD when the plants are exposed to heat stress; meanwhile it upregulates expression of several heat- or ABA-responsive genes (HSP70, HSP90, HSP101, LEA5, and ABRE1). Thus, the NtDOG1L-T may play a protective role under heat stress in tobacco.
Data Availability Statement
The raw data supporting the conclusions of this article will be made available by the authors, without undue reservation.
Author Contributions
ZX: Conceptualization, funding acquisition, supervision, and writing. YW, XD, SX, HL, and XZ: Investigation. XS, YC, TY, and ZX: Project administration. All authors contributed to the article and approved the submitted version.
Funding
This work was partially funded by project of R&D of science and technology from Sanmenxia Tobacco Company (2019411200200094) and major project of science and technology from Henan Tobacco Company (2018410000270035).
Conflict of Interest
XS, YC, and HL were employed by Sanmenxia Tobacco Company.
The remaining authors declare that the research was conducted in the absence of any commercial or financial relationships that could be construed as a potential conflict of interest.
Supplementary Material
The Supplementary Material for this article can be found online at: https://www.frontiersin.org/articles/10.3389/fpls.2020.568489/full#supplementary-material
Footnotes
References
Ashikawa, I., Abe, F., and Nakamura, S. (2013). DOG1-like genes in cereals: investigation of their function by means of ectopic expression in Arabidopsis. Plant Sci. 208, 1–9. doi: 10.1016/j.plantsci.2013.03.011
Ashikawa, I., Mori, M., Nakamura, S., and Abe, F. (2014). A transgenic approach to controlling wheat seed dormancy level by using triticeae DOG1-like genes. Transgenic Res. 23, 621–629. doi: 10.1007/s11248-014-9800-5
Baxter, A., Mittler, R., and Suzuki, N. (2014). ROS as important players in plant stress signalling. J. Exp. Bot. 65, 1229–1240. doi: 10.1093/jxb/ert375
Bentsink, L., Jowett, J., Hanhart, C. J., and Koornneef, M. (2006). Cloning of DOG1, a quantitative trait locus controlling seed dormancy in Arabidopsis. Proc. Natl. Acad. Sci. U. S. A. 103, 17042–17047. doi: 10.1073/pnas.0607877103
Béziat, C., Kleine-Vehn, J., and Feraru, E. (2017). Histochemical staining of β-glucuronidase and its spatial quantification. Methods Mol. Biol. 1497, 73–80. doi: 10.1007/978-1-4939-6469-7_8
Bryant, F. M., Hughes, D., Hassani-Pak, K., and Eastmond, P. J. (2019). Basic LEUCINE ZIPPER TRANSCRIPTION FACTOR67 transactivates delay of germination1 to establish primary seed dormancy in Arabidopsis. Plant Cell 31, 1276–1288. doi: 10.1105/tpc.18.00892
Choudhury, F. K., Rivero, R. M., Blumwald, E., and Mittler, R. (2017). Reactiveoxygen species, abiotic stress and stress combination. Plant J. 90, 856–867. doi: 10.1111/tpj.13299
Dekkers, J., He, H., Hanson, J., Willems, L. A., Jamar, D. C., Cueff, G., et al. (2016). The Arabidopsis DELAY OF GERMINATION 1 gene affects ABSCISIC ACID INSENSITIVE 5 (ABI5) expression and genetically interacts with ABI3 during Arabidopsis seed development. Plant J. 85, 451–465. doi: 10.1111/tpj.13118
Graeber, K., Linkies, A., Steinbrecher, T., Mummenhoff, K., Tarkowská, D., Turečková, V., et al. (2014). DELAY OF GERMINATION 1 mediates a conserved coat-dormancy mechanism for the temperature- and gibberellin-dependent control of seed germination. Proc. Natl. Acad. Sci. U. S. A. 111, E3571–E3580. doi: 10.1073/pnas.1403851111
Guan, Q., Lu, X., Zeng, H., Zhang, Y., and Zhu, J. (2013). Heat stress induction of miR398 triggers a regulatory loop that is critical for thermotolerance in Arabidopsis. Plant J. 74, 840–851. doi: 10.1111/tpj.12169
He, Y., Zhang, Y., Chen, L., Wu, C., Luo, Q., Zhang, F., et al. (2017). A member of the 14-3-3 gene family in Brachypodium distachyon, BdGF14d, confers salt tolerance in transgenic tobacco plants. Front. Plant Sci. 8:340. doi: 10.3389/fpls.2017.00340
Huang, Y. C., Niu, C. Y., Yang, C. R., and Jinn, T. L. (2016). The heat stress factor HSFA6b connects ABA signaling and ABA-mediated heat responses. Plant Physiol. 172, 1182–1199. doi: 10.1104/pp.16.00860
Huo, Y., Wang, M., Wei, Y., and Xia, Z. (2016). Overexpression of the maize psbA gene enhances drought tolerance through regulating antioxidant system, photosynthetic capability, and stress defense gene expression in tobacco. Front. Plant Sci. 6:1223. doi: 10.3389/fpls.2015.01223
Huo, H., Wei, S., and Bradford, K. J. (2016). DELAY OF GERMINATION1 (DOG1) regulates both seed dormancy and flowering time through microRNA pathways. Proc. Natl. Acad. Sci. U. S. A. 113, E2199–E2206. doi: 10.1073/pnas.1600558113
Iba, K. (2002). Acclimative response to temperature stress in higher plants: approaches of gene engineering for temperature tolerance. Annu. Rev. Plant Biol. 53, 225–245. doi: 10.1146/annurev.arplant.53.100201.160729
Jacob, P., Hirt, H., and Bendahmane, A. (2017). The heat-shock protein/chaperone network and multiple stress resistance. Plant Biotechnol. J. 15, 405–414. doi: 10.1111/pbi.12659
Kim, M. D., Kim, Y. H., Kwon, S. Y., Yun, D. J., and Kwak, S. S. (2010). Enhanced tolerance to methyl viologen-induced oxidative stress and high temperature in transgenic potato plants overexpressing the CuZnSOD, APX and NDPK2 genes. Physiol. Plant. 140, 153–162. doi: 10.1111/j.1399-3054.2010.01392.x
Larkindale, J., Hall, J. D., Knight, M. R., and Vierling, E. (2005). Heat stress phenotypes of Arabidopsis mutants implicate multiple signaling pathways in the acquisition of thermotolerance. Plant Physiol. 138, 882–897. doi: 10.1104/pp.105.062257
Li, X., Chen, T., Li, Y., Wang, Z., Cao, H., Chen, F., et al. (2019). ETR1/RDO3 regulates seed dormancy by relieving the inhibitory effect of the ERF12-TPL complex on DELAY OF GERMINATION1 expression. Plant Cell 31, 832–847. doi: 10.1105/tpc.18.00449
Li, H., Liu, S. S., Yi, C. Y., Wang, F., Zhou, J., Xia, X. J., et al. (2014). Hydrogen peroxide mediates abscisic acid-induced HSP70 accumulation and heat tolerance in grafted cucumber plants. Plant Cell Environ. 37, 2768–2780. doi: 10.1111/pce.12360
Née, G., Kramer, K., Nakabayashi, K., Yuan, B., Xiang, Y., Miatton, E., et al. (2017). DELAY OF GERMINATION1 requires PP2C phosphatases of the ABA signaling pathway to control seed dormancy. Nat. Commun. 8:72. doi: 10.1038/s41467-017-00113-6
Nishimura, N., Tsuchiya, W., Moresco, J. J., Hayashi, Y., Satoh, K., Kaiwa, N., et al. (2018). Control of seed dormancy and germination by DOG1-AHG1 PP2C phosphatase complex via binding to heme. Nat. Commun. 9:2132. doi: 10.1038/s41467-018-04437-9
Ohama, N., Sato, H., Shinozaki, K., and Yamaguchi-Shinozaki, K. (2017). Transcriptional regulatory network of plant heat stress response. Trends Plant Sci. 22, 53–65. doi: 10.1016/j.tplants.2016.08.015
Raja, V., Majeed, U., Kang, H., Andrabi, K. I., and John, R. (2017). Abiotic stress: interplay between ROS, hormones and MAPKs. Environ. Exp. Bot. 137, 142–157. doi: 10.1016/j.envexpbot.2017.02.010
Sall, K., Dekkers, B. J. W., Nonogaki, M., Katsuragawa, Y., Koyari, R., Hendrix, D., et al. (2019). DELAY OF GERMINATION 1-LIKE 4 acts as an inducer of seed reserve accumulation. Plant J. 100, 7–19. doi: 10.1111/tpj.14485
Sierro, N., Battey, J. N., Ouadi, S., Bakaher, N., Bovet, L., Willig, A., et al. (2014). The tobacco genome sequence and its comparison with those of tomato and potato. Nat. Commun. 5:3833. doi: 10.1038/ncomms4833
Skubacz, A., Daszkowska-Golec, A., and Szarejko, I. (2016). The role and regulation of ABI5 (ABA-insensitive 5) in plant development, abiotic stress responses and phytohormone crosstalk. Front. Plant Sci. 7:1884. doi: 10.3389/fpls.2016.01884
Su, X., Wei, F., Huo, Y., and Xia, Z. (2017). Comparative physiological and molecular analyses of two contrasting flue-cured tobacco genotypes under progressive drought stress. Front. Plant Sci. 8:827. doi: 10.3389/fpls.2017.00827
Sun, K., Wang, H., and Xia, Z. (2016). The maize bHLH transcription factor bHLH105 confers manganese tolerance in transgenic tobacco. Plant Sci. 280, 97–109.
Suzuki, N., Bassil, E., Hamilton, J. S., Inupakutika, M. A., Zandalinas, S. I., Tripathy, D., et al. (2016). ABA is required for plant acclimation to a combination of salt and heat stress. PLoS One 11:e0147625. doi: 10.1371/journal.pone.0147625
Teng, S., Rognoni, S., Bentsink, L., and Smeekens, S. (2008). The Arabidopsis GSQ5/DOG1 Cvi allele is induced by the ABA-mediated sugar signaling pathway and enhances sugar sensitivity by stimulating ABI4 expression. Plant J. 55, 372–381. doi: 10.1111/j.1365-313X.2008.03515.x
Tubiello, F. N., Soussana, J. F., and Howden, S. M. (2007). Crop and pasture response to climate change. Proc. Natl. Acad. Sci. U. S. A. 104, 19686–19690. doi: 10.1073/pnas.0701728104
Ul Haq, S., Khan, A., Ali, M., Khattak, A. M., Gai, W. X., Zhang, H. X., et al. (2019). Heat shock proteins: dynamic biomolecules to counter plant biotic and abiotic stresses. Int. J. Mol. Sci. 20:5321. doi: 10.3390/ijms20215321
Vanderauwera, S., Suzuki, N., Miller, G., van de Cotte, B., Morsa, S., Ravanat, J. L., et al. (2011). Extranuclear protection of chromosomal DNA from oxidative stress. Proc. Natl. Acad. Sci. U. S. A. 108, 1711–1716. doi: 10.1073/pnas.1018359108
Vishwakarma, K., Upadhyay, N., Kumar, N., Yadav, G., Singh, J., Mishra, R., et al. (2017). Abscisic acid signaling and abiotic stress tolerance in plants: a review on current knowledge and future prospects. Front. Plant Sci. 8:161. doi: 10.3389/fpls.2017.00161
von Koskull, D. P., Scharf, K. D., and Nover, L. (2007). The diversity of plant heat stress transcription factors. Trends Plant Sci. 12, 452–457. doi: 10.1016/j.tplants.2007.08.014
Wang, H., Wang, M., and Xia, Z. (2019). Overexpression of a maize SUMO conjugating enzyme gene (ZmSCE1e) increases sumoylation levels and enhances salt and drought tolerance in transgenic tobacco. Plant Sci. 281, 113–121. doi: 10.1016/j.plantsci.2019.01.020
Xia, Z., Huo, Y., Wei, Y., Chen, Q., Xu, Z., and Zhang, W. (2016). The Arabidopsis Lyst interacting protein 5 acts in regulating abscisic acid signaling and drought eesponse. Front. Plant. Sci. 7:758.
Xia, Z., Wei, Y., Sun, K., Wu, J., Wang, Y., and Wu, K. (2013). The maize AAA-type protein SKD1 confers enhanced salt and drought stress tolerance in transgenic tobacco by interacting with Lyst-interacting protein 5. PLoS One. 8:e69787.
Xu, Z., Wang, M., Guo, Z., Zhu, X., and Xia, Z. (2019). Identification of a 119-bp promoter of the maize sulfite oxidase gene (ZmSO) that confers high-level gene expression and ABA or drought inducibility in transgenic plants. Int. J. Mol. Sci. 20:3326.
Yatusevich, R., Fedak, H., Ciesielski, A., Krzyczmonik, K., Kulik, A., Dobrowolska, G., et al. (2017). Antisense transcription represses Arabidopsis seed dormancy QTL DOG1 to regulate drought tolerance. EMBO Rep. 18, 2186–2196. doi: 10.15252/embr.201744862
Zhang, M., Su, J., Zhang, Y., Xu, J., and Zhang, S. (2018). Conveying endogenous and exogenous signals: MAPK cascades in plant growth and defense. Curr. Opin. Plant Biol. 45, 1–10. doi: 10.1016/j.pbi.2018.04.012
Keywords: heat stress, promoter activity, antioxidant enzyme, gene expression, transgenic tobacco, DOG1
Citation: Dai X, Wang Y, Chen Y, Li H, Xu S, Yang T, Zhang X, Su X and Xia Z (2020) Overexpression of NtDOG1L-T Improves Heat Stress Tolerance by Modulation of Antioxidant Capability and Defense-, Heat-, and ABA-Related Gene Expression in Tobacco. Front. Plant Sci. 11:568489. doi: 10.3389/fpls.2020.568489
Edited by:
Randy D. Allen, Oklahoma State University, United StatesReviewed by:
Ulrike Bechtold, University of Essex, United KingdomAkiyoshi Kawaoka, Akita Jujo Chemicals Co, Ltd., Japan
Vijaykumar Veerappan, Eastern Connecticut State University, United States
Copyright © 2020 Dai, Wang, Chen, Li, Xu, Yang, Zhang, Su and Xia. This is an open-access article distributed under the terms of the Creative Commons Attribution License (CC BY). The use, distribution or reproduction in other forums is permitted, provided the original author(s) and the copyright owner(s) are credited and that the original publication in this journal is cited, in accordance with accepted academic practice. No use, distribution or reproduction is permitted which does not comply with these terms.
*Correspondence: Xiaoquan Zhang, zxq013415@sina.com; Xinhong Su, xhsu@163.com; Zongliang Xia, xiazl08@163.com