- 1Department of Agricultural Chemistry and Edaphology, University of Córdoba, Córdoba, Spain
- 2Departamento de Química, Universidade Federal de São Carlos, São Carlos, Brazil
- 3Department d’Hortofruticultura, Botànica i Jardineria, Agrotecnio, Universitat de Lleida, Lleida, Spain
- 4Department of Plant Breeding, Institute for Sustainable Agriculture, CSIC (IAS-CSIC), Córdoba, Spain
Different Lolium species, common weeds in cereal fields and fruit orchards in Chile, were reported showing isolated resistance to the acetyl CoA carboxylase (ACCase), acetolactate synthase (ALS) and 5-enolpyruvylshikimate-3-phosphate synthase (EPSPS) inhibiting herbicides in the late 1990s. The first case of multiple resistance to these herbicides was Lolium multiflorum found in spring barley in 2007. We hypothesized that other Lolium species may have evolved multiple resistance. In this study, we characterized the multiple resistance to glyphosate, diclofop-methyl and iodosulfuron-methyl-sodium in Lolium rigidum, Lolium perenne and Lolium multiflorum resistant (R) populations from Chile collected in cereal fields. Lolium spp. populations were confirmed by AFLP analysis to be L. rigidum, L. perenne and L. multiflorum. Dose-response assays confirmed multiple resistance to glyphosate, diclofop-methyl and iodosulfuron methyl-sodium in the three species. Enzyme activity assays (ACCase, ALS and EPSPS) suggested that the multiple resistance of the three Lolium spp. was caused by target site mechanisms, except the resistance to iodosulfuron in the R L. perenne population. The target site genes sequencing revealed that the R L. multiflorum population presented the Pro-106-Ser/Ala (EPSPS), Ile-2041-Asn++Asp-2078-Gly (ACCase), and Trp-574-Leu (ALS) mutations; and the R L. rigidum population had the Pro-106-Ser (EPSPS), Ile-1781-Leu+Asp-2078-Gly (ACCase) and Pro-197-Ser/Gln+Trp-574-Leu (ALS) mutations. Alternatively, the R L. perenne population showed only the Asp-2078-Gly (ACCase) mutation, while glyphosate resistance could be due to EPSPS gene amplification (no mutations but high basal enzyme activity), whereas iodosulfuron resistance presumably could involve non-target site resistance (NTSR) mechanisms. These results support that the accumulation of target site mutations confers multiple resistance to the ACCase, ALS and EPSPS inhibitors in L. multiflorum and L. rigidum from Chile, while in L. perenne, both target and NTSR could be present. Multiple resistance to three herbicide groups in three different species of the genus Lolium in South America represents a significant management challenge.
Introduction
Lolium grasses are problematic weeds around the world (Salas et al., 2012; Menegat et al., 2016), causing yield losses in small grains, orchards, and vineyards, as well as in non-agricultural lands (Travlos et al., 2018). There are 17 Lolium species described, but the most problematic ones in agricultural producing systems are L. multiflorum (Lam.) Husnot (Italian ryegrass), L. perenne L. (perennial ryegrass) and L. rigidum Gaudin (rigid ryegrass) (Menegat et al., 2016). The last species is considered the most economically important weed in Australia, while L. multiflorum and L. perenne are frequents weeds in crops of North and South America and the Mediterranean region (Heap, 2020).
Over the past three decades, control of Lolium grasses has been carried out using almost exclusively PRE- and POST-emergence herbicides, exerting high selection pressure that has resulted in resistance to most herbicide sites of action (SoAs) (Tehranchian et al., 2018). The first case of resistance in L. rigidum was found in canola, spring barley, and wheat fields in Australia in 1982. Since then, herbicide resistance cases in Lolium species have increased substantially over time (Heap, 2020). There are currently 67, 9, and 48 cases of herbicide resistance in L. multiflorum, L. perenne, and L. rigidum, respectively, recorded in the database of the International Survey of Herbicide Resistant Weeds. At least half of these are cases of cross and/or multiple resistance (Heap, 2020).
Lolium grasses are difficult to control chemically because they have the capacity to evolve different herbicide resistance mechanisms very quickly, depending on the distribution of resistant individuals within the cultivated areas, seed viability, obligated cross-pollination, genetic variability and high phenotypic plasticity (Travlos et al., 2018). For example, L. rigidum has evolved resistance to 14 different herbicide SoAs, and L. multiflorum has evolved resistant to 9 SoAs (Heap, 2020). In addition, because Lolium weed species generally are diploids (Dalton et al., 1999; Busi and Powles, 2013), they are capable of hybridizing with each other, resulting in populations with homozygous and heterozygous individuals that can carry multiple resistance alleles (Menegat et al., 2016). In the long term, this situation reduces the options for diversification of alternative herbicides for the integrated management of these weeds (Fernández et al., 2017). In addition, resistance alleles are generally not linked and accumulate independently, i.e., Lolium species are able to accumulate different resistance mechanisms, so different combinations of resistance alleles can be found resulting in complex multiple herbicide resistance profiles (Tan et al., 2007; Martins et al., 2014). The most serious multiple herbicide resistance cases are those including resistance to glyphosate (5-enolpyruvylshikimate-3-phosphate synthase [EPSPS] inhibitor) (Fernández-Moreno et al., 2017a). Populations of L. multiflorum showing resistance to glyphosate, sethoxydim (acetyl CoA carboxylase [ACCase] inhibitor), and paraquat have been found in the United States (Tehranchian et al., 2018); L. rigidum populations resistant to glyphosate, paraquat, ACCase and acetolactate synthase (ALS) inhibitors have been found in Australia (Yu et al., 2007, 2008). Additionally, different populations of Lolium species with multiple resistance to up to four herbicide SoAs have been reported throughout the Mediterranean region in Europe (Collavo and Sattin, 2014; Fernández et al., 2017; Travlos et al., 2018).
In Chile, herbicide resistance is not recent, and of the 19 known cases, nine involve Lolium species with single and/or multiple resistance occurring in small grain fields (barley, canola, cereals, lupins, spring and winter wheat) and fruit orchards (Heap, 2020). In Chile, L. rigidum, L. multiflorum and L. perenne were found with resistance to ACCase inhibitors in small grains fields in 1997, 1998 and 2001, respectively (Heap, 2020). Lolium multiflorum was the first species to show resistance to glyphosate, and it was found in fruit orchards in 2001 (Perez and Kogan, 2003). The first case of resistance to ALS inhibitors was also L. multiflorum, found in wheat fields in 2002, and this case was the first one of multiple resistance, since this species also showed resistance to glyphosate (Heap, 2020). Because ACCase- and ALS-inhibiting herbicides became recurring alternatives to manage glyphosate resistance and vice versa, we hypothesized that populations of Lolium species from Chile may have had multiple resistance to these three herbicide groups, since they can select herbicide resistance in few generations, even when exposed to subdoses (Busi et al., 2012). However, the resistance mechanisms were characterized only for L. multiflorum resistance to glyphosate (Perez and Kogan, 2003; Michitte et al., 2007). In addition, herbicide resistance in Lolium species is widely dispersed in neighboring Latin American countries (Heap, 2020), and only in Brazil is it estimated that L. multiflorum affects more than 4.2 million ha (Alcántara-de la Cruz et al., 2020). However, with the exception of the recent characterization of the resistance mechanisms to ACCase inhibitors in L. perenne from Argentina (Yanniccari and Gigón, 2020), there are no studies of resistance mechanisms in Lolium species from South America (L. multiflorum and L. perenne) other than for glyphosate (Yanniccari et al., 2017; Barroso et al., 2018).
In this work, the multiple resistances to glyphosate, diclofop-methyl and iodosulfuron-methyl-sodium (inhibiting herbicides of EPSPS, ACCase and ALS enzymes, respectively) were characterized in three putative resistant (R) populations of L. rigidum, L. perenne and L. multiflorum, collected in spring barley and winter wheat fields in the Regions VIII (San Bernardo and Olivar) and IX (Vilcún) in Chile. The possible target-site resistance (TSR) mechanisms involved were studied in these three populations, being a one-of-a-kind study for South America.
Materials and Methods
Chemicals
Trade formulations of glyphosate (Roundup 480, Monsanto Europe), diclofop-methyl (Firelo, Dupont Spain) and iodosulfuron-methyl-sodium (Hussar, Bayer CropScience Spain) were used for the dose-response assays. Glyphosate, diclofop-acid and iodosulfuron of analytical grade (Sigma-Aldrich, Spain) with a purity of 99% were used for enzymatic assays.
Plant Material
Seeds of the different R Lolium spp. (L. rigidum, L. perenne, and L. multiflorum) were supplied in 2010 by Dr. Nelson Espinoza (INIA of Carillanca, Chile). Mature seeds were collected from different fields of spring barley (Region VIII) and winter wheat (Region IX) grown under direct drilling systems for several years (Espinoza et al., 2009). Spring weeds were removed in pre-sowing with glyphosate, and during the crop season, ALS- (iodosulfuron) and/or ACCase- (diclofop, haloxyfop, and others) inhibiting herbicides were used for weed control. A screening test was conducted on the R populations to eliminate susceptible individuals from the field-collected seed, which consisted of germinating the seeds of the putative R populations and treating the seedlings at field doses with diclofop-methyl (900 g ai ha–1), glyphosate (720 g ae ha–1) and iodosulfuron (5 g ai ha–1). Individuals surviving these applications were allowed to grow to maturity to collect the purified seeds used in this study. Seeds of populations of each Lolium species that were susceptible (S) to these herbicides were collected in areas near these crops.
Seeds of the R and S Lolium spp. populations were placed for germination in 9-cm Petri dishes containing moistened filter paper. Petri dishes were placed in a growth chamber at 26/18°C (day/night), with relative humidity of 60% and a photoperiod of 16 h at a light density of 850 mmol m–2 s–1. Seedlings were transplanted into plastic pots in 250 mL substrate (sand and peat 1:1). Pots were returned to the growth chamber, and the plants were irrigated as necessary until ready for use (plants having 3–4 true leaves).
Molecular Characterisation of Lolium Species
One population of each Lolium species, i.e., L. multiflorum, L. rigidum (harvested in southern Spain) and L. perenne (North of Portugal) were included as reference populations in the molecular analysis with amplified fragment length polymorphism (AFLP) markers. The molecular identity of the reference populations has been previously confirmed. Twelve plants of each Lolium spp. population were used for DNA extraction from leaf tissue (50 mg plant–1) by using the Speedtool DNA Extraction Plant kit (Biotools, Madrid, Spain). DNA was quantified in a NanoDrop ND-1000 spectrophotometer. AFLP analysis was carried out using the fluorescent AFLP IRDye kit for Large Plant Genome Analysis (LI-COR Biosciences) following the manufacturer’s instructions. Twelve primer pairs [E36-M48 (E-ACC MCAC)/E36-M60 (E-ACC MCTC)/E37-M49 (E-ACG MCAG)/E38-M50 (E-ACT MCAT)/E40-M61 (E-AGC MCTG)/E35-M49 (E-ACA MCAG)/E36-M49 (E-ACC MCAG)/E35-M61 (E-ACA MCTG)/E40-M62 (E-AGC MCTT)/E32-M60 (E-AAC MCTC)/E33-M50 (E-AAG MCAT)/E35-M48 (E-ACA MCAC)] were used for selective amplification (Fernández et al., 2017; Fernández-Moreno et al., 2017a,b). AFLP products were separated in a polyacrylamide electrophoresis using an automated sequencer (LICOR 4300). Polymorphic AFLP markers (12) and primers were identified, and individuals were scored for presence (1) or absence (0) of AFLP fragments using the software package SAGAMX 2 GENERATION. Genetic distances were calculated using Jaccard’s coefficients of similarity. Grouping of the genotypes was determined by using the unweighted pair group method with arithmetic mean (UPGMA). The analysis was performed with AFLP marker data in the program NTSYSpc 2.2.
Dose–Response Assays
R and S Lolium spp. plants were treated with different doses of diclofop (0, 100, 200, 400, 800, 1,600, 3,200, 4,000 g ai ha–1), glyphosate (0, 31.25, 62.5, 125, 250, 500, 1,000, 1,500, 2,000, 4,000 g ae ha–1) and iodosulfuron (0, 1, 2, 4, 8, 16, 32, 64, 128 g ai ha–1) in a laboratory chamber (SBS-060 De Vries Manufacturing, Hollandale, MN, United States) equipped with 8002 flat fan nozzles and delivering 200 L ha–1 at 250 KPa. The experiments were based on a completely random design with eight replications by dose; and experiments were repeated. Plant mortality and above-ground fresh weight were evaluated 28 days after treatment (DAT), and data were expressed as the percentage of the untreated control. Herbicide rates reducing the plant growth (GR50) or causing plant mortality by 50% (LD50) with respect to the untreated control were determined for each Lolium spp. population and herbicide.
Enzyme Activity Assays
EPSPS Activity
The extraction and activity of the EPSPS enzyme was carried out following the methodology described by Dayan et al. (2015). Five grams of foliar tissue from the different Lolium spp. populations were ground with liquid N2 to a fine powder. Samples were transferred to 50 mL Falcon tubes containing 25 mL of extraction buffer (100 mM MOPS, 5 mM EDTA, 10% glycerol, 50 mM KCl, and 0.5 mM benzamidine) with 70 μL of ß-mercaptoethanol (10 mM) and 1% in polyvinylpolypyrrolidone (PVPP) to extract the EPSPS. Samples were vortexed for 5 min, avoiding foaming, and then centrifuged (18,000 g, 30 min, 4°C). Supernatant was filtered using a cheesecloth in a cold beaker and then slowly added (NH4)2SO4, was then slowly added while supernatant was under continuous stirring until a 45% solution of (NH4)2SO4 (w/v) was obtained. After addition of (NH4)2SO4 the sample was stirred for 30 min and then centrifuged (15,000 g, 30 min, 4°C). This step was repeated once more to obtain a 70% (NH4)2SO4 (w/v) solution to precipitate the fraction that contained the EPSPS activity. Supernatants were discarded, and pellets were resuspended in 1–2 mL assay buffer (100 mM MOPS, 1 mM MgCl2, 10% glycerol (v/v), 2 mM sodium molybdate, 200 mM NaF). Samples were dialysed using Slide-A-Lyzer dialysis cassettes (1000-MWC, Thermo Scientific, Meridian, IL, United States) overnight in 2 L of dialysis buffer (100 mM MOPS and 5 mM EDTA) at 4°C on a stir plate. The final pH of the buffers was adjusted to 7.0 with HCl or NaOH. The concentration of total protein soluble (TPS) was determined by Bradford assay (Bradford, 1976). Basal and enzyme EPSPS activities were determined in a continuous assay quantifying the inorganic phosphate (Pi) released from shikimate-3-phosphate with the EnzCheck phosphate assay kit (Invitrogen, Carlsbad, CA, United States) following the manufacturer’s instructions. The glyphosate concentrations used were: 0, 0.1, 1, 10, 100 and 1,000 μM. The amount Pi released was measured for 10 min at 360 nm in a spectrophotometer (DU-640, Beckman Coulter Inc. Fullerton, CA, United States). The EPSPS activity was calculated by determining the amount of Pi (μmol) released in μg of TSP–1 min–1. EPSPS enzyme activity was expressed as percentage of enzyme activity in presence of glyphosate with respect to the control (basal activity without glyphosate). The experiment was repeated for all populations, and each glyphosate concentration had three technical replicates.
ACCase Activity
The in vitro ACCase enzyme activity was performed following the protocol described by De Prado et al. (2005). Six grams of fresh weight were taken from new leaves of the different Lolium spp. populations for the extraction step. The samples were ground using liquid N2 and then added to 24 mL extraction buffer (0.1 M Hepes-KOH at pH 7.5, 0.5 M glycerol, 2 mM EDTA and 0.32 mM PMSF). After mixing for 3 min with a magnetic stirrer and then filtered using a cheesecloth, the samples were centrifuged (24,000 g, 30 min, 4°C). the supernatant was fractioned with (NH4)2SO4 and centrifuged (12,000 g, 10 min at 4°C). The pellets were resuspended in 1 mL S400 buffer (0.1 M Tricine-KOH at pH 8.3, 0.5 M glycerol, 0.05 M KCl, 2 mM EDTA and 0.5 mM DTT). The homogenate was applied to a desalting column (PD-10 columns, Sephadex G-25 M, Amersham Biosciences AB, SE-751 84, Uppsala, Sweden) and eluted in 2 mL of S400 buffer. The specific protein concentrations were determined by Bradford assay (Bradford, 1976). The enzyme activity was measured through the ATP-dependent incorporation of NaH[14C]O3 into [14C]malonyl-CoA. The reaction was conducted at 34°C in 7 mL scintillation vials with 0.1 M Tricine-KOH at pH 8.3, 0.5 M glycerol, 0.05 M KCl, 2 mM EDTA, 0.5 mM DTT, 1.5 mM MgCl2, 15 mM NaH[14C]O3 (1.22 MBq μmol–1), 50 μL of enzyme extract, 5 mM acetyl-CoA in a final volume of 0.2 mL. The reaction was stopped after 5 min by adding 30 μL of HCl 4N. After the drying step, 0.5 mL of ethanol-water solution (1:1, v/v) was added to the vial, followed by 5 mL of scintillation cocktail (Ultima Gold, Perkin-Elmer, BV BioScience Packard). Radioactivity was determined by liquid scintillation spectrometry. One unit of ACCase activity was defined as 1 μmol malonyl CoA formed min–1. The diclofop-acid concentrations were: 0, 0.1, 1, 10, 100, 1,000 and 10,000 μM. The experiment was repeated with three replicates for each herbicide concentration.
ALS Activity
ALS activity in presence of different iodosulfuron concentrations was determined in vitro following the protocol described by Rojano-Delgado et al. (2015). Three grams of young leaf tissue were powdered using liquid N2. Polyvinylpyrrolidone (0.5 g) was added to the fine powder as well as extraction buffer [1M K-phosphate buffer solution (pH 7.5), 10 mM sodium pyruvate, 5 mM MgCl2, 50 mM thiamine pyrophosphate, 100 μM flavin adenine dinucleotide, 12 mM dithiothreitol and glycerol–water (1:9, v/v)] in a proportion of 1:2 tissue–buffer. Samples were agitated for 10 min at 4°C, filtered through a cheesecloth, and centrifuged (20,000 g for 20 min). The supernatant was immediately used for the enzyme assays. For the ALS enzyme activity, 90 μL of enzyme extract was used with 110 μL of freshly prepared assay buffer [0.08 M K-phosphate buffer solution (pH 7.5), 0.5 M sodium pyruvate, 0.1 M MgCl2, 0.5 mM thiamine pyrophosphate and 1,000 μM flavin adenine dinucleotide]. The iodosulfuron concentrations assayed were: 0, 0.1, 1, 10, 50, 100, 500, 1,000 and 5,000 μM. Aliquots of 250 μL of a solution 0.04 M K2HPO4 at pH 7.0 were added. This mixture was incubated for 1 h at 37°C. Afterward, the reaction was stopped by adding 50 μL of H2SO4/water 1:10 (v/v). To decarboxylate acetolactate to acetoin, the tubes with the mixture were heated for 15 min at 60°C. A colored complex (λ 520 nm) formed after the addition of 250 μL of creatine (5 g L–1 freshly prepared in water) and 250 μL of 1-naphthol (50 g L–1 freshly prepared in 5 N NaOH) prior to incubation at 60°C for 15 min. Total protein content was determined by the Bradford method (Bradford, 1976), based on measurement of the absorbance at 595 nm of an acidic solution of Coomassie Brilliant Blue G-250 after binding to proteins. The experiment was repeated and with three replicates for each concentration of the herbicide.
RNA Extraction and Target-Site Genes Sequencing
Samples (∼100 mg) of foliar tissue were taken from 20 seedlings from the R and S Lolium spp. populations. Then, both R plants were treated with glyphosate, diclofop and iodosulfuron at a rate of 720 (g ae ha–1), 400 and 5 g ai ha–1, respectively, as was done in the dose-response assays. Only R plants that survived these application rates 21 DAT were considered for molecular analyses (Cruz-Hipolito et al., 2015). Total RNA was isolated from leaves using TRIzol reagent (Invitrogen, Carlsbad, CA, United States) following the manufacturer’s instructions. RNA was treated with TURBO DNase (RNase-Free; Ambion, Warrington, United Kingdom) to eliminate any DNA contamination and stored at −80°C. First strand complementary DNA (cDNA) synthesis was carried out with 2 μg RNA per sample using an iScript cDNA Synthesis Kit (Bio-Rad Laboratories, Inc. CA, United States). The primers to amplify the ACCase (two fragments), ALS (two fragments), EPSPS genes and the PCR conditions are described in Table 1. Each PCR reaction was conducted in a total volume of 25 μL [50 ng of cDNA, 0.2 μM of each primer, 0.2 mM dNTP mix (PE Applied Biosystems; Life Technologies S.A., Spain), 2 mM MgCl2, 1X buffer, and 0.625 units of a 100:1 enzyme mixture of non-proofreading (Thermus thermophilus) and proofreading (Pyrococcus furiosus) polymerases (BIOTOOLS, Madrid, Spain)]. Each cDNA sample, i.e., cDNA of each plant, was amplified in triplicate. An aliquot of the PCR product (10 μL) was loaded in a 1% agarose gel to check the correct band amplification. The rest of the PCR product (15 μL) was purified using ExoSAP-IT® for PCR Product Clean-Up (USB, Cleveland, OH, United States) as indicated by the manufacturers and sequenced. Sanger sequencing was carried out by the SCAI DNA sequencing service of the University of Córdoba, Spain. Gene sequences of the R and S Lolium species were verified and assembled using the Geneious 8.1.8 (Biomatters Ltd, Auckland, New Zealand) software, to identify mutations responsible for conferring herbicide resistance.
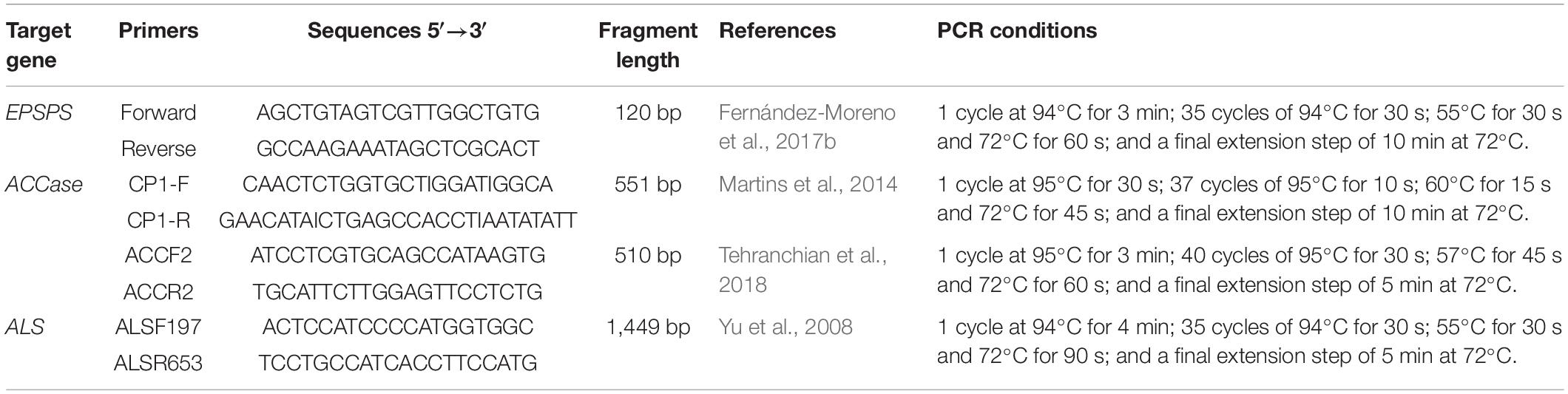
Table 1. Primers and PCR conditions used to amplify the 5-enolpiruvylshikimate-3-phosphate synthase (EPSPS), acetyl-coenzyme A carboxylase (ACCase, two fragments) and acetolactate synthase (ALS) genes to identify potential mutations responsible for herbicide resistance in Lolium species in Chile.
Statistical Analysis
Dose-response and enzyme activity data were subjected to non-linear regression analysis using the log-logistic equation Y = d/1+(x/g)b); where Y represents the percentage of fresh weight reduction, plant survival and enzyme activity inhibition with respect to the control, d is the limits of the upper asymptote, b is the slope of the curve, g is the herbicide rate at the inflection point (i.e., GR50, LD50 or I50), and x (independent variable) is the herbicide dose. Using this equation, the GR50, LD50 and I50 (herbicide concentration to inhibit the ACCase, EPSPS and/or ALS activity by 50%) of each population of each species were calculated. Regression analyses were conducted using the ‘drc’ package with program R version 3.2.5 (Ritz et al., 2015). Resistance factors (RF = R/S) were calculated as the ratio of R to-S GR50, LD50 or I50.
Specific enzyme activity data were submitted to one-way ANOVA (for P < 0.05). The Tukey test was used for mean comparison (95% confidence level) to test for significant differences between populations within each species and for each enzyme.
Results
Molecular Characterisation of Lolium Species
The UPGMA analysis clustered the Lolium spp. populations into a large group and two subgroups. In the larger group, the Jaccard index indicated a 62% similarity between L. rigidum, L. perenne, and L. multiflorum. In the first subgroup, the putative R and S L. rigidum populations from Chile were confirmed to belong to this species, because they were grouped together to the reference population from Spain. In the second subgroup, L. multiflorum and L. perenne populations were found to have 80% similarity. The putative R and S populations from Chile of each of these two Lolium species were grouped with their respective reference population from Portugal (L. perenne) and Spain (L. multiflorum), confirming their identities at molecular level (Figure 1).
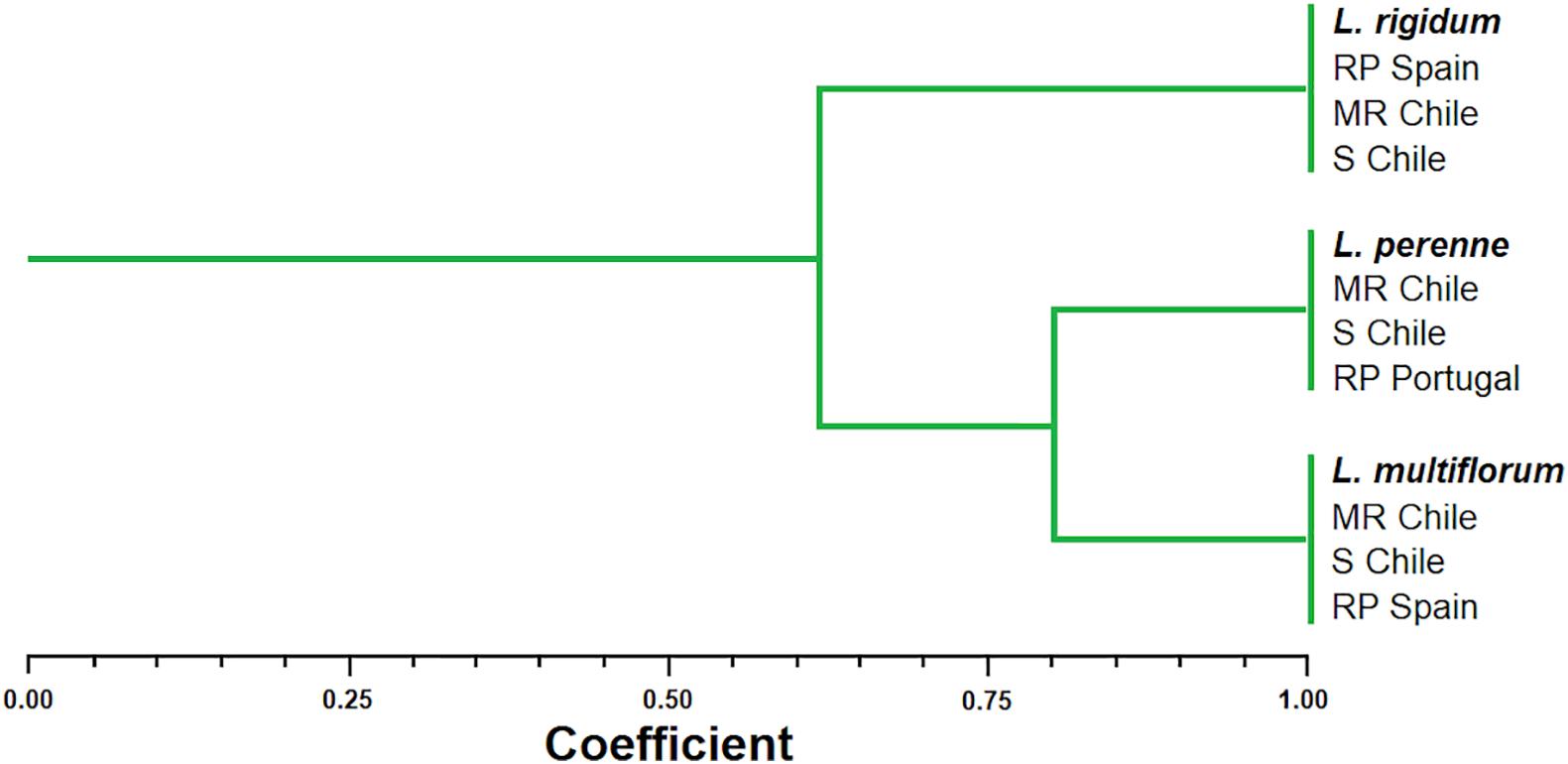
Figure 1. Dendrogram of the genetic similarities among multiple herbicide resistant (MR) and susceptible (S) populations of Lolium species from Chile in comparison to reference’s populations (RP) from Portugal and Spain after the UPGMA analysis performed with AFLP marker data. Twelve plants of each putative population were used for the molecular analysis.
Glyphosate Dose–Response Assays
The fresh weight of the S Lolium spp. populations was reduced by 50% with glyphosate doses close to 100 g ae ha–1 (Figures 2A,B). The R populations of L. perenne, L. rigidum, and L. multiflorum were 5- (GR50 = 453 g ae ha–1), 6.6- (GR50 = 689 g ae ha–1) and 12.5- (GR50 = 937 g ae ha–1) times more resistant to glyphosate, respectively, in relation to their respective S counterparts. Regarding survival (LD50), the RF values of the R populations of L. perenne, L. rigidum and L. multiflorum were 5.6, 7.1, and 14.1, respectively. These RF values were determined according to the LD50 (210, 258 and 290 g ae ha–1 glyphosate) values of the S populations following the same species order (Table 2).
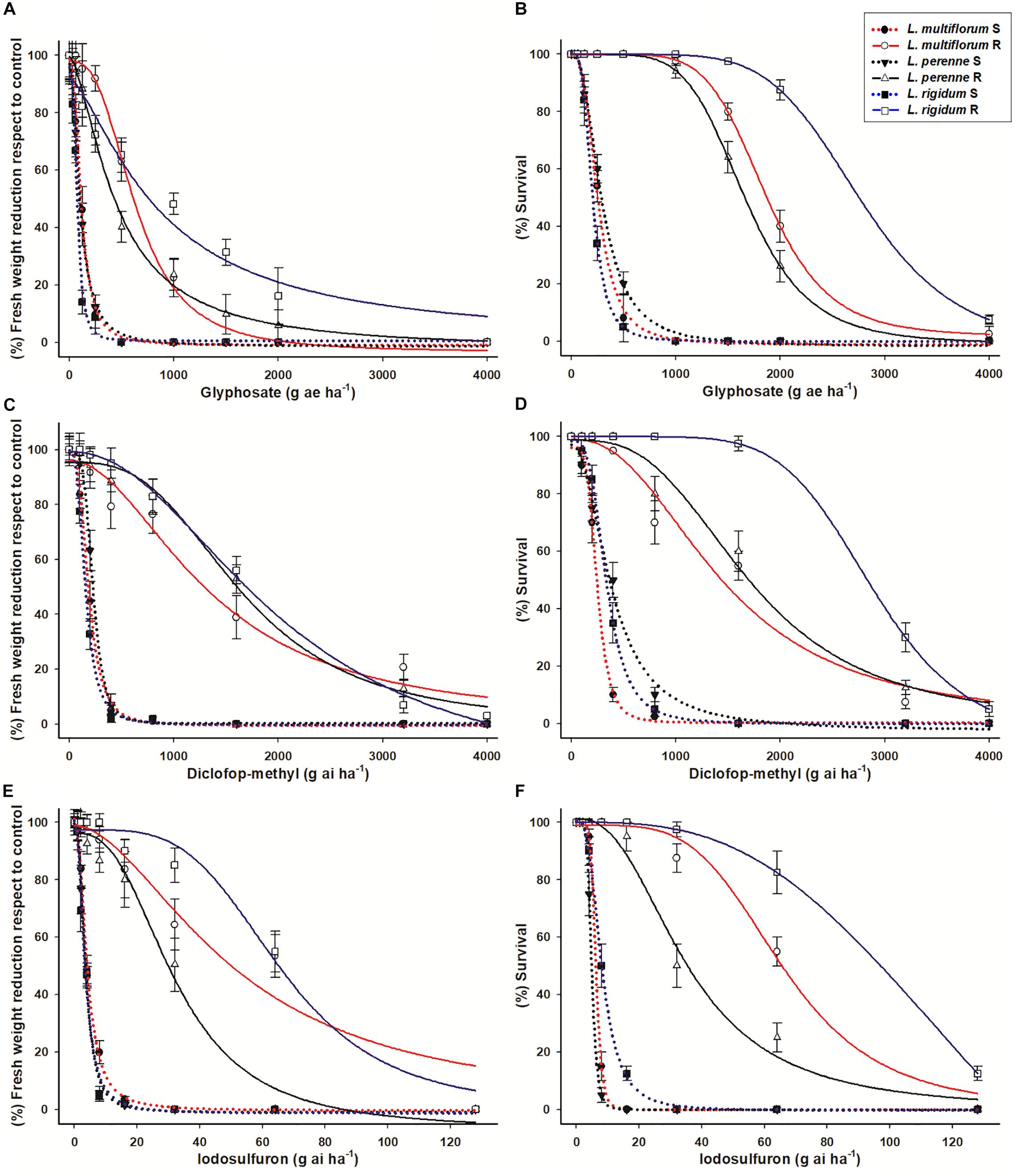
Figure 2. Dose-response curves (fresh weight reduction and survival rates) of glyphosate (A,B), diclofop-methyl (C,D) and iodosulfuron (E,F) in resistant (R) and susceptible (S) populations of Lolium species from Chile. Vertical bars ± standard error (n = 8).
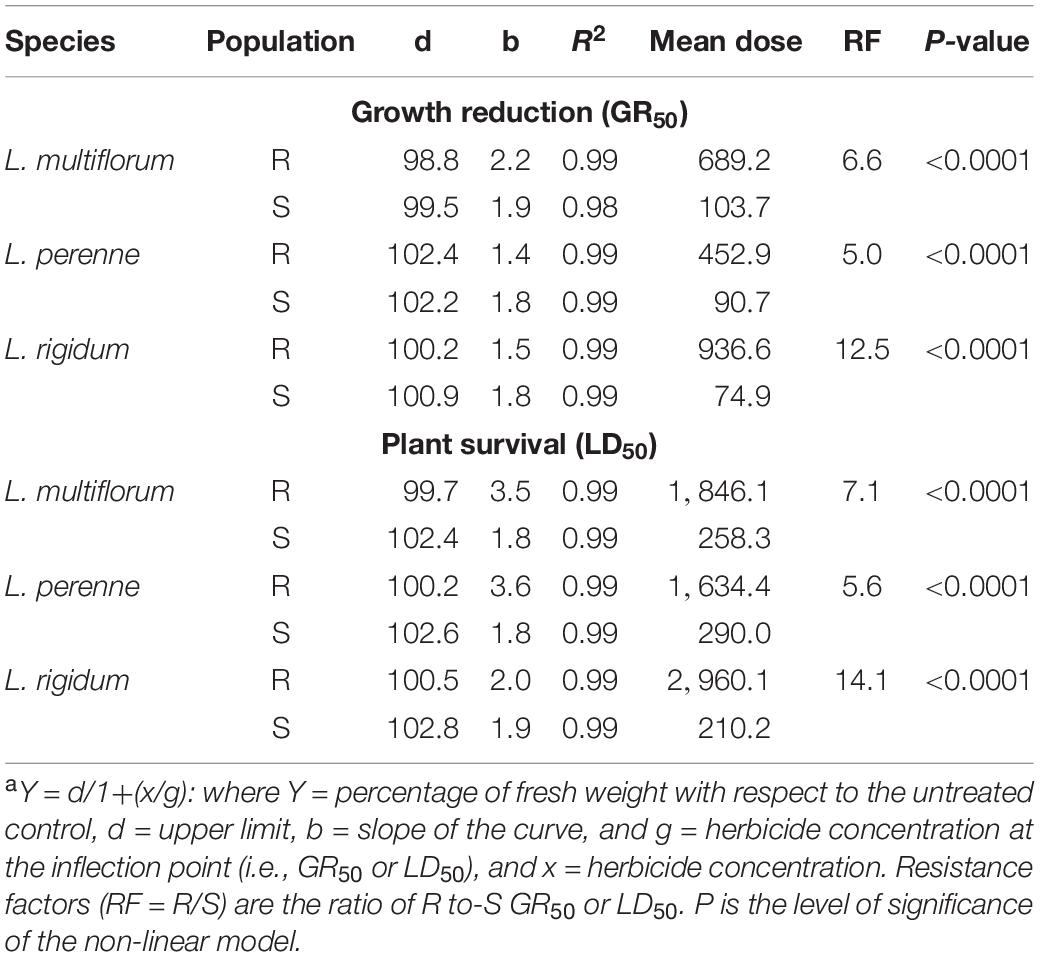
Table 2. Parameters of the sigmoidal equationa used to estimate the effective mean dose of glyphosate (EPSPS inhibitor) required to reduce the fresh weight (GR50) and plant survival (LD50) by 50% in multiple-resistant (R) and -susceptible (S) populations of Lolium species in Chile.
Diclofop Dose–Response Assays
Diclofop resistance of the R Lolium spp. populations followed the same trend as glyphosate resistance, i.e., the R population of L. perenne had the lowest resistance level, followed by L. multiflorum (intermediate resistance level), while L. rigidum was the most resistant species, both in relation to the percentage of fresh weight reduction as well the plant survival rate by 50% (Figures 2C,D). The GR50 values of the S populations ranged from 148 to 254 g ai ha–1 diclofop, and the LD50 values were between 269 and 388 g ai ha–1. The RF of the R populations ranged from 6.4 to 11.4 and from 4.4 to 9.4 in relation to the GR50 and LD50 values, respectively, of the S populations (Table 3).
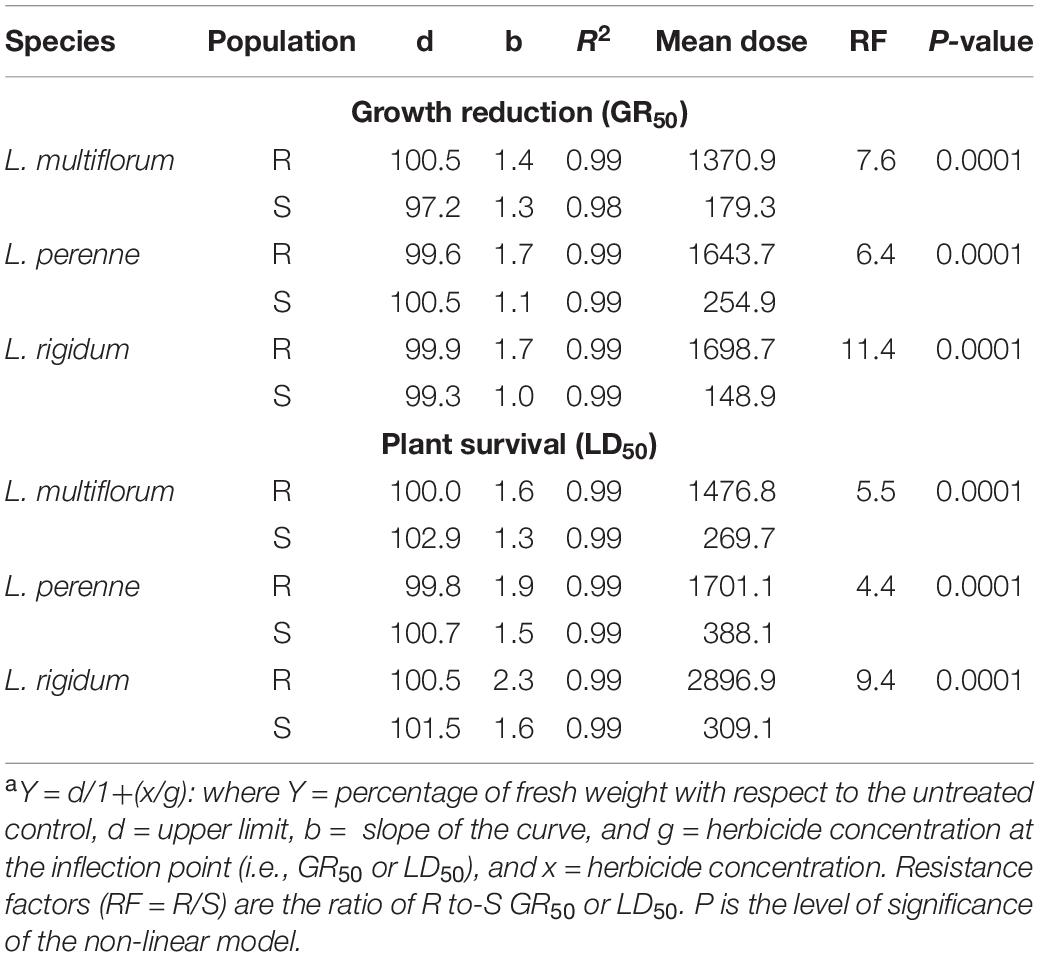
Table 3. Parameters of the sigmoidal equationa used to estimate the effective mean dose of diclofop-methyl (ACCase inhibitor) required to reduce the fresh weight (GR50) and plant survival (LD50) by 50% in multiple-resistant (R) and -susceptible (S) populations of Lolium species in Chile.
Iodosulfuron Dose–Response Assays
The R populations of the three Lolium species survived iodosulfuron rates higher than the recommended field dose (3.5–5 g ai ha–1) (Figures 2E,F). Based on weight reduction, the least resistant species was L. perenne (GR50 = 27.0 g ai ha–1), followed by L. multiflorum (GR50 = 50.2 g ai ha–1). Lolium rigidum (GR50 = 66.8 g ai ha–1) was the most resistant species. The R populations were between 9 and 24 times more resistant to iodosulfuron than the S populations. These levels of resistance decreased in relation to the survival rate, where the LD50 values of the S populations ranged from 4.9 to 6.2 g ai ha–1 and those of R populations from 36 to 82.8 g ai ha–1. The RF of the R populations in relation to the S ones were 7.3, 11.2, and 13.4 for L. perenne, L. multiflorum, and L. rigidum, respectively (Table 4).
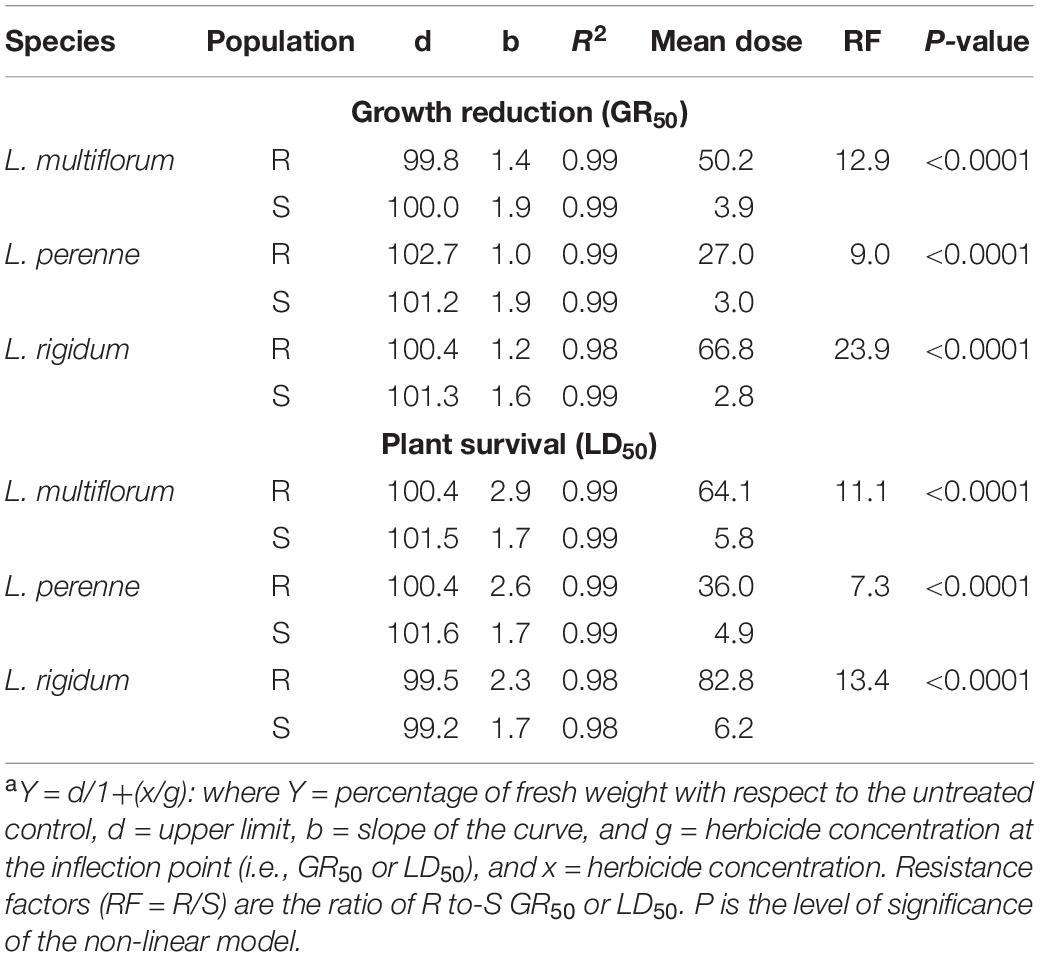
Table 4. Parameters of the sigmoidal equationa used to estimate the effective mean dose of iodosulfuron-methyl-sodium (ALS inhibitor) required to reduce the fresh weight (GR50) and plant survival (LD50) by 50% in multiple-resistant (R) and -susceptible (S) populations of Lolium species in Chile.
Enzyme Activity Assays
The specific activity of the EPSPS was similar between R and S populations of L. multiflorum (0.037 μmol Pi μg–1 TSP min–1) and L. rigidum (0.026 μmol Pi μg–1 TSP min–1); however, differences of such activity were observed between L. perenne populations [0.032 (S) vs 0.133 (R) μmol Pi μg–1 TSP min–1]. The specific ACCase activity, that ranged from 10.3 to 17.2 nmol HCO3– mg protein–1 min–1, was lower in the R populations of the three Lolium species. In the case of the specific ALS activity, there were differences between species, but not among R and S populations within each Lolium species. The averages were 1,511, 1,752, and 1,293 nmol acetoin mg–1 protein h–1 for L. multiflorum, L. perenne, and L. rigidum, respectively (Figures 3A,C,E).
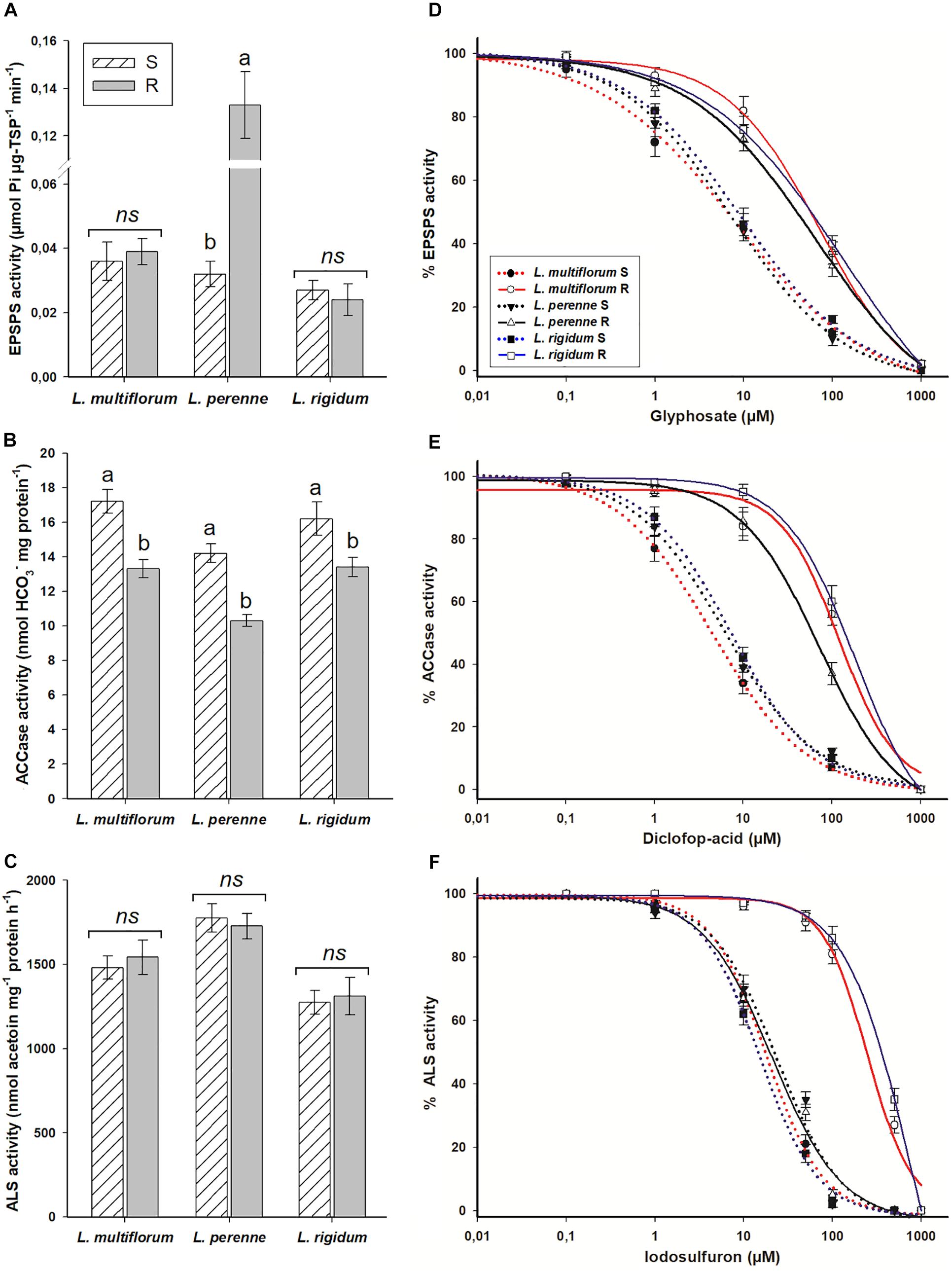
Figure 3. Enzyme activity of resistant (R) and susceptible (S) populations of Lolium species in Chile, without and with presence of EPSPS (A,D), ACCase (B,E) and ALS (C,F) inhibitors. Different letters above bars indicate differences between populations within species according to the Tukey test at 95%. NS, not significant. Vertical bars represent ± SE (n = 3).
The R populations of L. multiflorum, L. perenne, and L. rigidum required 14.2, 8.4, and 12.3 times, respectively, more glyphosate to inhibit EPSPS activity by 50%; and 27.7, 11.1, and 29.6 times more diclofop to inhibit ACCase in the same proportion in relation to their respective S counterparts. The ALS activities of the R populations of L. multiflorum and L. rigidum in response to iodosulfuron were 13.4 and 23.2 times lower, respectively, than the S populations; however, no differences in the sensitivity of this enzyme were observed between the R and S populations of L. perenne (Figures 3B,D,F and Table 5).
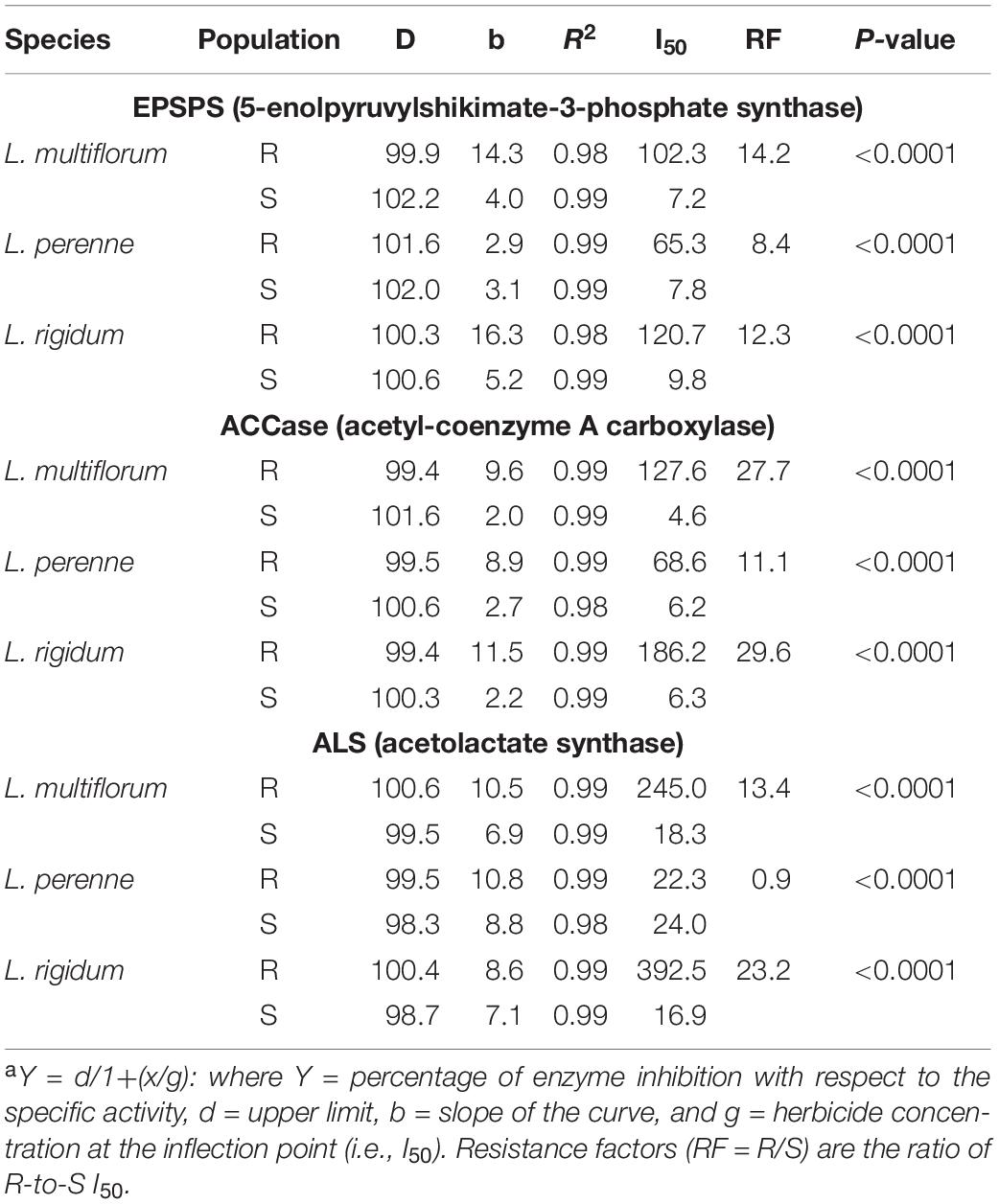
Table 5. Parameters of the sigmoidal equationa used to estimate the concentrations (μM) of glyphosate, diclofop-methyl and iodosulfuron-methyl-sodium to inhibit the enzymatic activity of the EPSPS, ACCase and ALS by 50% (I50), respectively, in multiple-resistant (R) and -susceptible (S) populations of Lolium species in Chile.
Target Site Changes
Nucleotide substitutions were found at the 106-CCA codon, which naturally encodes to proline, in the EPSPS gene, in the R L. multiflorum and L. rigidum populations (Table 6). The most frequent codon substitution was TCA (serine) found in 8 and 12 resistant individuals of L. multiflorum and L. rigidum, respectively. In addition, five R individuals of L. multiflorum presented the ACA (alanine) nucleotide combination. Regarding the ACCase gene, the three R populations of Lolium species showed different combinations of amino acid substitutions. Four and five R individuals of L. multiflorum presented the mutations Ile-2041-Asn and Asp-2078-Gly, respectively; L. perenne presented only the Asp-2078-Gly mutation (7 individuals); and L. rigidum presented the mutations Ile-1781-Leu and Asp-2078-Gly (5 and 8 individuals, respectively). Finally, the most frequent amino acid substitution found in the ALS gene of the R L. multiflorum and L. rigidum populations was Trp-574-Leu (11 and 6 individuals, respectively). In addition, two different amino acid substitutions were found at the Pro-197 position of the ALS gene in four (Ser) and three (Gln) individuals of the R L. rigidum population. No nucleotide changes were detected in the R L. perenne population (Table 6).
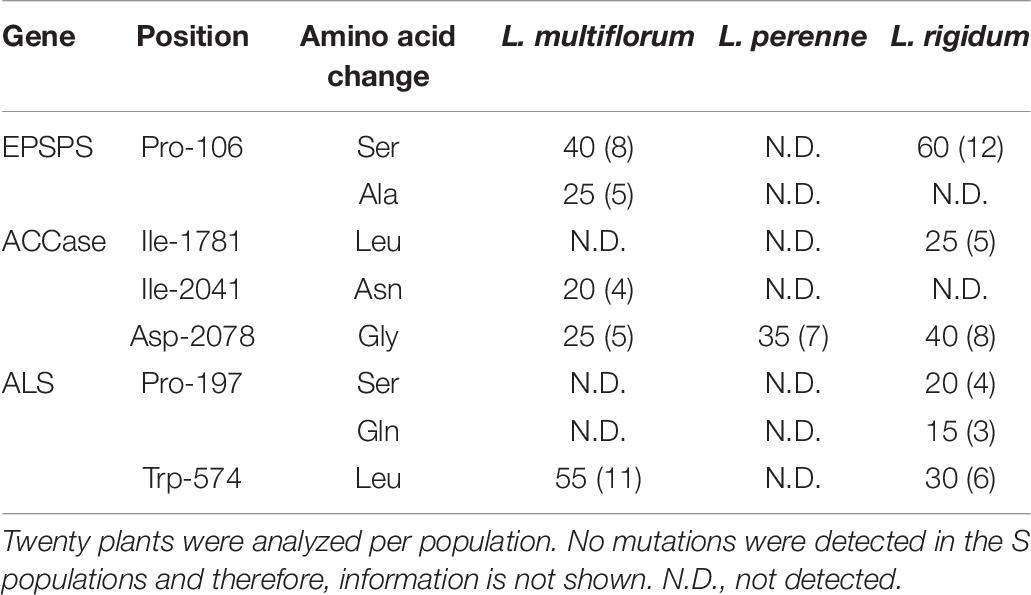
Table 6. Frequency of amino acid substitutions (% and number of plants in parenthesis) in the genes of the target enzymes 5-enolpyruvylshikimate-3-phosphate synthase (EPSPS), acetyl-coenzyme A carboxylase (ACCase) and acetolactate synthase (ALS) of multiple-resistant (R) Lolium spp. populations from Chile.
Discussion
Differentiation between Lolium species on the basis of phenotypic characteristics is difficult (Menegat et al., 2016), therefore, a molecular characterisation was required to establish the molecular identities between the three populations studied. AFLP analyses make it possible to differentiate which populations belong to each Lolium species based on their molecular relationships (>90% similarity), by comparing them with their respective reference species of L. multiflorum, L. perenne and L. rigidum. However, this molecular tool did not allow differentiating the susceptibility or resistance status to herbicides since the genetic similarity between R and S populations within each species was equal to or greater than 95%. Due to the lack of homology and size homoplasy of fragments between individuals and populations, AFLP analyses may give biased results (Caballero et al., 2008; Fernández-Moreno et al., 2017a). For example, glyphosate R and S populations of Amaranthus palmeri presented high similarity levels of genetic variation (Chandi et al., 2013), as well as populations of Spartina alterniflora from different coastal regions of China that showed a weak genetic differentiation (Li et al., 2019). Therefore, AFLPs analyses are important to understand genetic diversity between and within weed populations, but these studies are not so crucial to optimize weed management programs (Sterling et al., 2004).
Resistance to EPSPS Inhibitor
Among grasses worldwide, Lolium species have evolved the greatest number of cases of selection for glyphosate resistance (Heap, 2020). In Chile, the first case of glyphosate resistance was found in L. multiflorum in 2001 in fruit orchards in Region VIII (San Bernardo and Olivar) (Perez and Kogan, 2003), where two R populations presented RF 2- to 4-fold higher in relation to a S population. Subsequently, glyphosate resistant populations of the same species were also found in wheat fields of Region IX (Vilcún) with RF of 7.3 (Michitte et al., 2005). Glyphosate resistance levels of the R L. multiflorum, L. perenne, and L. rigidum populations included in this study, which varied between 6- and 11-fold based on growth reduction, and 4- to 9-fold according to plant mortality, were closer to those observed in the R L. multiflorum population of Vilcún, as well as to those reported for other glyphosate-resistant populations of Lolium spp. (Chandi et al., 2013; Fernández et al., 2017; Fernández-Moreno et al., 2017a,b; Yanniccari et al., 2017).
Herbicide resistance can be conferred by target-site resistance (TSR) and non-target site resistance (NTSR) mechanisms (Gaines et al., 2020). In this study we did not evaluate NTSR mechanisms; however, this does not mean that they cannot be involved in the herbicide resistance observed in the three R Lolium populations. Target site mutations at the Pro-106 position of the EPSPS gene in Lolium species, with Pro substituted by Ser, Thr, or Ala, impart low to intermediate glyphosate resistance levels, with RF ranging from 2 to 15 (Preston et al., 2009). Mutations in this position (Pro-106) narrow the cavity of the glyphosate binding site with EPSPS but maintain the affinity of this enzyme with its substrate (phosphoenolpyruvate), which allows plants exposed to glyphosate field doses to survive (Funke et al., 2006). The mutations found in the EPSPS gene of the R populations of L. multiflorum (Pro-106-Ser/Ala) and L. rigidum (Pro-106-Ser) explain their glyphosate resistance levels, as corroborated in the enzyme activity assays. The occurrence of multiple mutations among R individuals of L. multiflorum suggests a complex evolutionary history of the resistance traits, as observed in R L. multiflorum populations of northwest California that showed high nucleotide diversity at Pro-106 position between and within populations (Karn and Jasieniuk, 2017). Although L. perenne did not present any mutation, its glyphosate resistance could also be explained at target site level, since the R population presented an EPSPS specific activity four times higher than the S population. The high EPSPS specific activity in this R population suggests that there was an overproduction of the EPSPS enzyme, which could be due to increased EPSPS gene copy number, or alternatively EPSPS activity could be higher in R populations due to post-translational modifications of the enzyme (Gherekhloo et al., 2017; Gaines et al., 2019). Thus, although the EPSPS of R plants is glyphosate sensitive, higher concentrations of herbicide are required to completely inhibit it (Gaines et al., 2019). EPSPS gene amplification was reported as the main TSR mechanism in glyphosate-resistant populations of L. multiflorum in the United States (Salas et al., 2012), and L. perenne in Argentina (Yanniccari et al., 2017), conferring varying levels of resistance depending on the numbers of copies of the EPSPS gene of each population.
Resistance to ACCase Inhibitors
Resistance to ACCase inhibitors based on ACCase gene overexpression is unusual, and it has only been found to confer resistance in Digitaria sanguinalis in Canada (Laforest et al., 2017). In our case we can rule out this mechanism, since the ACCase specific activity of the three R Lolium spp. populations was slightly lower than in the S populations. This lower specific activity could be due to the Asp-2078-Gly mutation that was found in some individuals of the R populations of L. multiflorum, L. perenne, and L. rigidum. Unlike other mutations, which also confer resistance to ACCase inhibitors such as those found in another R Lolium individual (Ile-1781-Leu and Ile-2041-Asn), the Asp-2078-Gly mutation has a fitness penalty for the plants that carry it, which reduces the speed in catalyzing the formation of malonyl-CoA at the expense of natural substrates (acetyl-CoA, ATP, and HCO3) (Vila-Aiub et al., 2015). This fitness penalty was observed in Alopecurus myosuroides and L. rigidum resistant to ACCase-inhibitors, where the specific activity of the ACCase of homozygous R plants of these weeds was reduced by ∼30% in relation to S plants (Vila-Aiub et al., 2015). The mutation Asp-2078-Gly has been reported to confer cross and different levels of ACCase resistance in L. multiflorum and L. rigidum around the world (Yu et al., 2007). In L. perenne, this mutation was found in R populations in Argentina (Yanniccari and Gigón, 2020). The selection of the Asp-2078-Gly mutation in the ACCase gene may fully explain the resistance to diclofop in L. perenne, and partially those observed in L. multiflorum and L. rigidum in dose-response and ACCase activity assays, since some individuals presented other mutations that also confer resistance to ACCase inhibitors.
The Ile-1781-Leu and Ile-2041-Asn combinations found in some R individuals of L. multiflorum and L. rigidum, respectively, contributed to an increase in the resistance to diclofop, but also showed that the R populations of these three Lolium species were not homogeneous. As already noted, because Lolium species are weeds of obligated cross-pollination, they may carry different alleles for both cross and multiple target-site resistance within populations (Malone et al., 2013; Martins et al., 2014), and in some case, in the same individual (Yu et al., 2007), as observed in clethodim-resistant L. rigidum in Australia, where different individuals of nine populations presented multiple ACCase mutations (one population presented 5 mutations), and two ACCase resistant alleles were found in single L. rigidum plants of two populations (Saini et al., 2015). The amino acid substitution Ile-1781-Leu is the most common mutation in resistant grass weed species and confers resistance to all classes of ACCase inhibitors (Kaundun, 2014), and does not have a fitness cost (Vila-Aiub et al., 2015). The Ile-2041-Asn mutation may or not confer resistance to ACCase inhibitors, therefore its presence does not imply it. For example, this mutation was reported to confer resistance to clethodim in L. rigidum (Yu et al., 2007), and Phalaris paradoxa (Hochberg et al., 2009), but not in A. myosuroides (Délye et al., 2008). When the Ile-2041-Asn contributes to ACCase resistance, it is responsible for moderate to high resistance levels to ariloxyphenoxypropionates (FOPs) and low to moderate to phenylpyrazolines (DENs) (Ghanizadeh et al., 2019).
Resistance to ALS Inhibitors
Like for the ACCase gene, overexpression of the ALS gene is also rare (Zhao et al., 2018). In addition, ALS gene overexpression may be insufficient to provide resistance to ALS inhibitors (Wang et al., 2019). Since the specific activity of the ALS was similar between S and R populations within each Lolium species, the possible involvement of ALS gene overexpression in the resistance to iodosulfuron was ruled out. ALS gene sequencing revealed target site mutations in L. multiflorum (Trp-574-Leu) and L. rigidum (Pro-197-Ser/Gln + Trp-574-Leu). Although multiple mutations that confer resistance to ALS inhibitors in the same individual can be found (Singh et al., 2019), it is important to note that the two mutations found in L. rigidum occurred in different individuals. Mutations at Pro-197 (resistance to sulfonylureas) and Trp-574 (cross resistance to imidazolines and sulfonylureas) positions have been found in more than 20 weed species. These are the most common mutations conferring resistance to ALS inhibitors (Moss, 2017), since mutations at these points do not represent a major fitness cost (Yu and Powles, 2014). In Lolium species, six different substitutions have been found at these amino acid positions (Pro-197-Ala/Arg/Gln/Leu/Ser/Thr and Trp-574-Leu), conferring moderate to high resistance levels to ALS inhibitors in L. multiflorum and L. rigidum (Yu et al., 2008; Liu et al., 2014), as well as in others weeds such as Descurainia sophia (Deng et al., 2017), and Rapistrum rugosum (Ntoanidou et al., 2019).
The Asp-376-Glu and Trp-574-Leu mutations conferred resistance to ALS inhibitors in L. perenne (Menegat et al., 2016); however, we found no evidence of their participation in the iodosulfuron resistance of the R L. perenne population, which was confirmed by means of the dose-response assays, i.e., TSR mechanisms were not involved in such resistance in this species. Therefore, L. perenne resistance to iodosulfuron could presumably be governed by NTSR mechanisms, mainly enhanced herbicide metabolism mediated by major enzyme families of plant-degrading routes such as cytochrome P450, glutathione-S-tranferase and/or glycosyltransferase (Jugulam and Shyam, 2019). Considering that the occurrence of both TSR and NTSR mechanisms (mainly herbicide metabolism) in ALS- and ACCase-resistant weeds is widespread (Han et al., 2016; Fang et al., 2019), we cannot rule out the participation of NTSR mechanisms in the resistance of L. multiflorum and L. rigidum. In addition, some of the mutations found in the ALS and ACCase genes of the R Lolium populations from Chile have been reported to confer cross resistance (Murphy and Tranel, 2019). Therefore, future research will be focused in characterizing the NTSR-based iodosulfuron resistance in L. perenne, the potential contribution of these mechanisms in the R populations of L. rigidum and L. multiflorum, as well as possible cross resistance within chemical families of ALS and ACCase inhibitors.
Conclusion
An accumulation of target site mutations that confer resistance to ALS, ACCase and EPSPS inhibiting herbicides was found in R populations of L. rigidum and L. multiflorum collected in barley and wheat fields in Chile. The resistance profile to glyphosate, diclofop and iodosulfuron of L. perenne differed in relation to the other two species of Lolium (annual weeds), possibly due to its perennial habit facilitating vegetative reproduction instead of sexual reproduction. Therefore, in addition to including non-chemical methods in the management of these resistant Lolium species, the characterisation of the possible participation of NTSR-based herbicide resistance is essential for the establishment of a properly integrated weed management program. The Chilean farmers should implement crop rotation, the use of mechanical control, sowing variation, and others.
This is the first study in which herbicide resistance mechanisms were characterized in L. rigidum from South America. In addition, it is also the first time that multiple resistance to ACCase, ALS and EPSPS inhibiting herbicides have been reported in L. multiflorum and L. perenne worldwide.
Data Availability Statement
The raw data supporting the conclusions of this article will be made available by the authors, without undue reservation.
Author Contributions
JV-G, CP-B, AR-D, HC-H, and RD performed the dose-response and enzyme activity assays. RA, JT, and FB carried out the molecular analysis. RD performed funding project administration and supervision. All authors wrote, corrected and approved the submitted version of this manuscript.
Funding
This work was funded by the Asociación de Agroquímicos y Medioambiente. The Spanish Ministry of Science and Innovation (Project PID2019-110847RB-I00) and the European Regional Development Fund (FEDER) supported this research. We acknowledge support of the publication fee by the CSIC Open Access Publication Support Initiative through its Unit of Information Resources for Research (URICI). JT acknowledges support from the Spanish Ministry of Science, Innovation and Universities (grant Ramon y Cajal RYC2018-023866-I) and RA thanks support to the Fundação de Amparo à Pesquisa do Estado de São Paulo (FAPESP 2018/15910-6).
Conflict of Interest
The authors declare that the research was conducted in the absence of any commercial or financial relationships that could be construed as a potential conflict of interest.
Supplementary Material
The Supplementary Material for this article can be found online at: https://www.frontiersin.org/articles/10.3389/fpls.2020.553948/full#supplementary-material
References
Alcántara-de la Cruz, R., Oliveira, G. M., Carvalho, L. B., and Silva, M. F. G. F. (2020). “Herbicide resistance in Brazil: status, impacts, and future challenges,” in Herbicides–Current Research and Case Studies in Use, 2nd Edn, ed. K. M. Ferreira (Rijeka: IntechOpen), 1–25. doi: 10.5772/intechopen.91236
Barroso, A. A. M., Costa, M. G. S., Neto, N. J., Dos Santos, J., Balbuena, T. S., Carbonari, C. A., et al. (2018). Protein identification before and after glyphosate exposure in Lolium multiflorum genotypes. Pest. Manag. Sci. 74, 1125–1133. doi: 10.1002/ps.4831
Bradford, M. M. (1976). A rapid and sensitive method for the quantitation of microgram quantities of protein utilizing the principle of protein-dye binding. Anal. Biochem. 72, 248–254. doi: 10.1016/0003-2697(76)90527-3
Busi, R., Neve, P., and Powles, S. (2012). Evolved polygenic herbicide resistance in Lolium rigidum by low-dose herbicide selection within standing genetic variation. Evol. Appl. 6, 231–242. doi: 10.1111/j.1752-4571.2012.00282.x
Busi, R., and Powles, S. (2013). Cross-resistance to prosulfocarb and triallate in pyroxasulfone-resistant Lolium rigidum. Pest. Manag. Sci. 69, 1379–1384. doi: 10.1002/ps.3516
Caballero, A., Quesada, H., and Rolán-Alvarez, E. (2008). Impact of amplified fragment length polymorphism size homoplasy on the estimation of population genetic diversity and the detection of selective loci. Genetics 179, 539–554. doi: 10.1534/genetics.107.083246
Chandi, A., Milla-Lewis, S. R., Jordan, D. L., York, A. C., Burton, J. D., Zuleta, M. C., et al. (2013). Use of AFLP markers to assess genetic diversity in palmer amaranth (Amaranthus palmeri) populations from North Carolina and Georgia. Weed Sci. 61, 136–145. doi: 10.1614/ws-d-12-00053.1
Collavo, A., and Sattin, M. (2014). First glyphosate-resistant Lolium spp. biotypes found in a European annual arable cropping system also affected by ACCase and ALS resistance. Weed Res. 54, 325–334. doi: 10.1111/wre.12082
Cruz-Hipolito, H., Fernandez, P., Alcantara, R., Gherekhloo, J., Osuna, M., and De Prado, R. (2015). Ile-1781-Leu and Asp-2078-Gly mutations in ACCase gene, endow cross-resistance to APP, CHD, and PPZ in Phalaris minor from Mexico. Int. J. Mol. Sci. 16, 21363–21377. doi: 10.3390/ijms160921363
Dalton, S., Bettany, A., Timms, E., and Morris, P. (1999). Co-transformed, diploid Lolium perenne (perennial ryegrass), Lolium multiflorum (Italian ryegrass) and Lolium temulentum (darnel) plants produced by microprojectile bombardment. Plant Cell Rep. 18, 721–726. doi: 10.1007/s002990050649
Dayan, F. E., Owens, D. K., Corniani, N., Silva, F. M. L., Watson, S. B., Howell, J., et al. (2015). Biochemical markers and enzyme assays for herbicide mode of action and resistance studies. Weed Sci. 63, 23–63. doi: 10.1614/WS-D-13-00063.1
De Prado, J. L., Osuna, M. D., Heredia, A., and De Prado, R. (2005). Lolium rigidum, a pool of resistance mechanisms to ACCase inhibitor herbicides. J. Agric. Food Chem. 53, 2185–2191. doi: 10.1021/jf049481m
Délye, C., Matéjicek, A., and Michel, S. (2008). Cross-resistance patterns to ACCase-inhibiting herbicides conferred by mutant ACCase isoforms in Alopecurus myosuroides Huds. (black-grass), re-examined at the recommended herbicide field rate. Pest Manag. Sci. 64, 1179–1186. doi: 10.1002/ps.1614
Deng, W., Yang, Q., Zhang, Y., Jiao, H., Mei, Y., Li, X., et al. (2017). Cross-resistance patterns to acetolactate synthase (ALS)-inhibiting herbicides of flixweed (Descurainia sophia L.) conferred by different combinations of ALS isozymes with a Pro-197-Thr mutation or a novel Trp-574-Leu mutation. Pestic. Biochem. Physiol. 136, 41–45. doi: 10.1016/j.pestbp.2016.08.006
Espinoza, N., Díaz, J., Galdames, R., Rodríguez, C., Gaete, N., and De Prado, R. (2009). “Status of herbicide resistance in wheat and others extensive crops in south of chile,” in Seminario Internacional “Diagnóstico y Manejo de la Resistencia a Herbicidas”, ed. N. Espinoza (Chile: Instituto Nacional de Investigación Agropecuaria, Serie de Actas de INIA N° 44), 92–105.
Fang, J., Zhang, Y., Liu, T., Yan, B., Li, J., and Dong, L. (2019). Target-site and metabolic resistance mechanisms to penoxsulam in barnyardgrass (Echinochloa crus-galli (L.) P. Beauv). J. Agric. Food Chem. 67, 8085–8095. doi: 10.1021/acs.jafc.9b01641
Fernández, P., Alcántara, R., Osuna, M. D., Vila-Aiub, M. M., and De Prado, R. (2017). Forward selection for multiple resistance across the non-selective glyphosate, glufosinate and oxyfluorfen herbicides in Lolium weed species. Pest Manag. Sci. 73, 936–944. doi: 10.1002/ps.4368
Fernández-Moreno, P. T., Alcántara-de la Cruz, R., Smeda, R. J., and De Prado, R. (2017a). Differential resistance mechanisms to glyphosate result in fitness cost for Lolium perenne and L. multiflorum. Front. Plant Sci. 8:1796. doi: 10.3389/fpls.2017.01796
Fernández-Moreno, P. T., Travlos, I., Brants, I., and De Prado, R. (2017b). Different levels of glyphosate-resistant Lolium rigidum L. among major crops in southern Spain and France. Sci. Rep. 7:13116. doi: 10.1038/s41598-017-13384-2
Funke, T., Han, H., Healy-Fried, M. L., Fischer, M., and Schonbrunn, E. (2006). Molecular basis for the herbicide resistance of Roundup Ready crops. Proc. Natl. Acad. Sci. U.S.A. 103, 13010–13015. doi: 10.1073/pnas.0603638103
Gaines, T. A., Duke, S. O., Morran, S., Rigon, C. A., Tranel, P. J., Küpper, A., et al. (2020). Mechanisms of evolved herbicide resistance. J. Biol. Chem. 295, 10307–10330. doi: 10.1074/jbc.REV120.013572
Gaines, T. A., Patterson, E. L., and Neve, P. (2019). Molecular mechanism of adaptive evolution revealed by global selection for glyphosate resistance. New Phytol. 223, 1770–1775. doi: 10.1111/nph.15858
Ghanizadeh, H., Harrington, K. C., and Mesarich, C. H. (2019). The target site mutation Ile-2041-Asn is associated with resistance to ACCase-inhibiting herbicides in Lolium multiflorum. New Zeal. J. Agric. Res. 63, 416–429. doi: 10.1080/00288233.2019.1620296
Gherekhloo, J., Fernández-Moreno, P. T., Alcántara-de la Cruz, R., Sánchez-González, E., Cruz-Hipolito, H. E., Domínguez-Valenzuela, J. A., et al. (2017). Pro-106-Ser mutation and EPSPS overexpression acting together simultaneously in glyphosate-resistant goosegrass (Eleusine indica). Sci. Rep. 7:6702. doi: 10.1038/s41598-017-06772-1
Han, H., Yu, Q., Owen, M. J., Cawthray, G. R., and Powles, S. B. (2016). Widespread occurrence of both metabolic and target-site herbicide resistance mechanisms in Lolium rigidum populations. Pest Manag. Sci. 72, 255–263. doi: 10.1002/ps.3995
Heap, I. (2020). International Survey of Herbicide Resistant Weeds. Available at: http://www.weedscience.org (accessed April 08, 2020)
Hochberg, O., Sibony, M., and Rubin, B. (2009). The response of ACCase-resistant Phalaris paradoxa populations involves two different target site mutations. Weed Res. 49, 37–46. doi: 10.1111/j.1365-3180.2008.00677.x
Jugulam, M., and Shyam, C. (2019). Non-target-site resistance to herbicides: recent developments. Plants 8:417. doi: 10.3390/plants8100417
Karn, E., and Jasieniuk, M. (2017). Nucleotide diversity at site 106 of EPSPS in Lolium perenne L. ssp. multiflorum from California indicates multiple evolutionary origins of herbicide resistance. Front. Plant Sci. 8:777. doi: 10.3389/fpls.2017.00777
Kaundun, S. S. (2014). Resistance to acetyl-CoA carboxylase-inhibiting herbicides. Pest Manag. Sci. 70, 1405–1417. doi: 10.1002/ps.3790
Laforest, M., Soufiane, B., Simard, M.-J., Obeid, K., Page, E., and Nurse, R. E. (2017). Acetyl-CoA carboxylase overexpression in herbicide-resistant large crabgrass (Digitaria sanguinalis). Pest Manag. Sci. 73, 2227–2235. doi: 10.1002/ps.4675
Li, F.-F., Gong, L., Li, J.-S., Liu, X.-Y., and Zhao, C.-Y. (2019). Low genetic differentiation yet high phenotypic variation in the invasive populations of Spartina alterniflora in Guangxi, China. PLoS One 14:e0222646. doi: 10.1371/journal.pone.0222646
Liu, M., Hulting, A. G., and Mallory-Smith, C. A. (2014). Characterization of multiple-herbicide-resistant Italian ryegrass (Lolium perenne spp. multiflorum). Pest. Manag. Sci. 70, 1145–1150. doi: 10.1002/ps.3665
Malone, J. M., Boutsalis, P., Baker, J., and Preston, C. (2013). Distribution of herbicide-resistant acetyl-coenzyme A carboxylase alleles in Lolium rigidum across grain cropping areas of South Australia. Weed Res. 54, 78–86. doi: 10.1111/wre.12050
Martins, B. A. B., Sánchez-Olguín, E., Perez-Jones, A., Hulting, A. G., and Mallory-Smith, C. (2014). Alleles contributing to ACCase-resistance in an Italian Ryegrass (Lolium perenne ssp. multiflorum) population from Oregon. Weed Sci. 62, 468–473. doi: 10.1614/WS-D-13-00169.1
Menegat, A., Bailly, G. C., Aponte, R., Heinrich, G. M. T., Sievernich, B., and Gerhards, R. (2016). Acetohydroxyacid synthase (AHAS) amino acid substitution Asp376Glu in Lolium perenne: effect on herbicide efficacy and plant growth. J. Plant Dis. Prot. 123, 145–153. doi: 10.1007/s41348-016-0023-2
Michitte, P., De Prado, R., Espinosa, N., and Gauvrit, C. (2005). Glyphosate resistance in a Chilean Lolium multiflorum. Commun. Agric. Appl. Biol. Sci. 70, 507–513.
Michitte, P., De Prado, R., Espinoza, E., Ruiz-Santaella, J. P., and Gauvrit, C. (2007). Mechanisms of resistance to glyphosate in a Ryegrass (Lolium multiflorum) biotype from Chile. Weed Sci. 55, 435–440. doi: 10.1614/WS-06-167.1
Moss, S. (2017). “Herbicide resistance in weeds,” in Weed Research: Expanding Horizons, eds P. E. Hatcher, and R. J. Froud-Williams (Chichester: John Wiley & Sons, Ltd), 181–214. doi: 10.1002/9781119380702.ch7
Murphy, B. P., and Tranel, P. J. (2019). Target-site mutations conferring herbicide resistance. Plants 8:e382. doi: 10.3390/plants8100382
Ntoanidou, S., Madesis, P., and Eleftherohorinos, I. (2019). Resistance of Rapistrum rugosum to tribenuron and imazamox due to Trp574 or Pro197 substitution in the acetolactate synthase. Pestic. Biochem. Physiol. 154, 1–6. doi: 10.1016/j.pestbp.2018.12.001
Perez, A., and Kogan, M. (2003). Glyphosate-resistant Lolium multiflorum in Chilean orchards. Weed Res. 43, 12–19. doi: 10.1046/j.1365-3180.2003.00311.x
Preston, C., Wakelin, A. M., Dolman, F. C., Bostamam, Y., and Boutsalis, P. (2009). A decade of glyphosate-resistant Lolium around the world: mechanisms, genes, fitness, and agronomic management. Weed Sci. 57, 435–441. doi: 10.1614/WS-08-181.1
Ritz, C., Baty, F., Streibig, J. C., and Gerhard, D. (2015). Dose-response analysis using R. PLoS One 10:e0146021. doi: 10.1371/journal.pone.0146021
Rojano-Delgado, A. M., Priego-Capote, F., Luque de Castro, M. D., and De Prado, R. (2015). Mechanism of imazamox resistance of the Clearfield® wheat cultivar for better weed control. Agron. Sustain. Dev. 35, 639–648. doi: 10.1007/s13593-014-0232-7
Saini, R. K., Malone, J., Preston, C., and Gill, G. (2015). Target enzyme-based resistance to clethodim in Lolium rigidum populations in Australia. Weed Sci. 63, 946–953. doi: 10.1614/WS-D-14-00176.1
Salas, R. A., Dayan, F. E., Pan, Z., Watson, S. B., Dickson, J. W., Scott, R. C., et al. (2012). EPSPS gene amplification in glyphosate-resistant Italian ryegrass (Lolium perenne ssp. multiflorum) from Arkansas. Pest. Manag. Sci. 68, 1223–1230. doi: 10.1002/ps.3342
Singh, S., Singh, V., Salas-Perez, R. A., Bagavathiannan, M. V., Lawton-Rauh, A., and Roma-Burgos, N. (2019). Target-site mutation accumulation among ALS inhibitor-resistant Palmer amaranth. Pest. Manag. Sci. 75, 1131–1139. doi: 10.1002/ps.5232
Sterling, T. M., Thompson, D. C., and Abbott, L. B. (2004). Implications of invasive plant variation for weed management. Weed Technol. 18, 1319–1324. doi: 10.1614/0890-037x(2004)018[1319:ioipvf]2.0.co;2
Tan, M. K., Preston, C., and Wang, G. X. (2007). Molecular basis of multiple resistance to ACCase-inhibiting and ALS-inhibiting herbicides in Lolium rigidum. Weed Res. 47, 534–541. doi: 10.1111/j.1365-3180.2007.00591.x
Tehranchian, P., Nandula, V., Jugulam, M., Putta, K., and Jasieniuk, M. (2018). Multiple resistance to glyphosate, paraquat and ACCase-inhibiting herbicides in Italian ryegrass populations from California: confirmation and mechanisms of resistance. Pest. Manag. Sci. 74, 868–877. doi: 10.1002/ps.4774
Travlos, I., Cheimona, N., De Prado, R., Jhala, A., Chachalis, D., and Tani, E. (2018). First case of glufosinate-resistant rigid ryegrass (Lolium rigidum Gaud.) in Greece. Agronomy 8:35. doi: 10.3390/agronomy8040035
Vila-Aiub, M. M., Yu, Q., Han, H., and Powles, S. B. (2015). Effect of herbicide resistance endowing Ile-1781-Leu and Asp-2078-Gly ACCase gene mutations on ACCase kinetics and growth traits in Lolium rigidum. J. Exp. Bot. 66, 4711–4718. doi: 10.1093/jxb/erv248
Wang, H., Zhang, L., Li, W., Bai, S., Zhang, X., Wu, C., et al. (2019). Isolation and expression of acetolactate synthase genes that have a rare mutation in shepherd’s purse (Capsella bursa-pastoris (L.) Medik.). Pestic. Biochem. Physiol. 155, 119–125. doi: 10.1016/j.pestbp.2019.01.013
Yanniccari, M., and Gigón, R. (2020). Cross-resistance to acetyl-CoA carboxylase–inhibiting herbicides conferred by a target-site mutation in perennial ryegrass (Lolium perenne) from Argentina. Weed Sci. 68, 116–124. doi: 10.1017/wsc.2020.1
Yanniccari, M., Gómez-Lobato, M. E., Istilart, C., Natalucci, C., Giménez, D. O., and Castro, A. M. (2017). Mechanism of resistance to glyphosate in Lolium perenne from Argentina. Front. Ecol. Evol. 5:123. doi: 10.3389/fevo.2017.00123
Yu, Q., Collavo, A., Zheng, M.-Q., Owen, M., Sattin, M., and Powles, S. B. (2007). Diversity of Acetyl-Coenzyme A carboxylase mutations in resistant Lolium populations: evaluation using clethodim. Plant Physiol. 145, 547–558. doi: 10.1104/pp.107.105262
Yu, Q., Han, H., and Powles, S. B. (2008). Mutations of the ALS gene endowing resistance to ALS-inhibiting herbicides in Lolium rigidum populations. Pest. Manag. Sci. 64, 1229–1236. doi: 10.1002/ps.1624
Yu, Q., and Powles, S. B. (2014). Resistance to AHAS inhibitor herbicides: current understanding. Pest. Manag. Sci. 70, 1340–1350. doi: 10.1002/ps.3710
Zhao, N., Yan, Y., Wang, H., Bai, S., Wang, Q., Liu, W., et al. (2018). Acetolactate synthase overexpression in mesosulfuron-methyl-resistant shortawn foxtail (Alopecurus aequalis Sobol.): reference gene selection and herbicide target gene expression analysis. J. Agric. Food Chem. 66, 9624–9634. doi: 10.1021/acs.jafc.8b03054
Keywords: glyphosate, diclofop-methyl, iodosulfuron methyl-sodium, italian ryegrass, perennial ryegrass, rigid ryegrass
Citation: Vázquez-García JG, Alcántara-de la Cruz R, Palma-Bautista C, Rojano-Delgado AM, Cruz-Hipólito HE, Torra J, Barro F and De Prado R (2020) Accumulation of Target Gene Mutations Confers Multiple Resistance to ALS, ACCase, and EPSPS Inhibitors in Lolium Species in Chile. Front. Plant Sci. 11:553948. doi: 10.3389/fpls.2020.553948
Received: 21 April 2020; Accepted: 08 October 2020;
Published: 28 October 2020.
Edited by:
Joshua Widhalm, Purdue University, United StatesReviewed by:
Bryan Young, Purdue University, United StatesDean E. Riechers, University of Illinois at Urbana-Champaign, United States
Todd Gaines, Colorado State University, United States
Copyright © 2020 Vázquez-García, Alcántara-de la Cruz, Palma-Bautista, Rojano-Delgado, Cruz-Hipólito, Torra, Barro and De Prado. This is an open-access article distributed under the terms of the Creative Commons Attribution License (CC BY). The use, distribution or reproduction in other forums is permitted, provided the original author(s) and the copyright owner(s) are credited and that the original publication in this journal is cited, in accordance with accepted academic practice. No use, distribution or reproduction is permitted which does not comply with these terms.
*Correspondence: Francisco Barro, ZmJhcnJvQGlhcy5jc2ljLmVz