- 1Horticulture and Product Physiology, Department of Plant Sciences, Wageningen University, Wageningen, Netherlands
- 2Centre for Crop Systems Analysis, Department of Plant Sciences, Wageningen University, Wageningen, Netherlands
- 3Molecular Plant Physiology, Institute of Environmental Biology, Utrecht University, Utrecht, Netherlands
- 4Plant Ecophysiology, Institute of Environmental Biology, Utrecht University, Utrecht, Netherlands
Due to their slow movement and closure upon shade, partially closed stomata can be a substantial limitation to photosynthesis in variable light intensities. The abscisic acid deficient flacca mutant in tomato (Solanum lycopersicum) displays very high stomatal conductance (gs). We aimed to determine to what extent this substantially increased gs affects the rate of photosynthetic induction. Steady-state and dynamic photosynthesis characteristics were measured in flacca and wildtype leaves, by the use of simultaneous gas exchange and chlorophyll fluorometry. The steady-state response of photosynthesis to CO2, maximum quantum efficiency of photosystem II photochemistry (Fv/Fm), as well as mesophyll conductance to CO2 diffusion were not significantly different between genotypes, suggesting similar photosynthetic biochemistry, photoprotective capacity, and internal CO2 permeability. When leaves adapted to shade (50 µmol m−2 s−1) at 400 µbar CO2 partial pressure and high humidity (7 mbar leaf-to-air vapour pressure deficit, VPD) were exposed to high irradiance (1500 µmol m−2 s−1), photosynthetic induction was faster in flacca compared to wildtype leaves, and this was attributable to high initial gs in flacca (~0.6 mol m−2 s−1): in flacca, the times to reach 50 (t50) and 90% (t90) of full photosynthetic induction were 91 and 46% of wildtype values, respectively. Low humidity (15 mbar VPD) reduced gs and slowed down photosynthetic induction in the wildtype, while no change was observed in flacca; under low humidity, t50 was 63% and t90 was 36% of wildtype levels in flacca. Photosynthetic induction in low CO2 partial pressure (200 µbar) increased gs in the wildtype (but not in flacca), and revealed no differences in the rate of photosynthetic induction between genotypes. Effects of higher gs in flacca were also visible in transients of photosystem II operating efficiency and non-photochemical quenching. Our results show that at ambient CO2 partial pressure, wildtype gs is a substantial limitation to the rate of photosynthetic induction, which flacca overcomes by keeping its stomata open at all times, and it does so at the cost of reduced water use efficiency.
Introduction
In the leaves of higher plants, stomata balance carbon uptake against water loss. They achieve this balance by dynamically regulating stomatal aperture in response to intrinsic and extrinsic factors. Typically, stomatal aperture decreases in low irradiance or darkness, and increases in high irradiance. Stomatal opening after sudden increases in irradiance is slow compared to changes in Calvin cycle metabolism (McAusland et al., 2016), with time constants in the range of 4 to 29 min (Vico et al., 2011). Due to the slow opening of stomata, the increase of stomatal conductance (gs, mol m−2 s−1) from a low initial value is assumed to be one of the three main limitations of net photosynthesis rate (A, µmol m−2 s−1) in response to increases in irradiance (e.g., Urban et al., 2008; Kaiser et al., 2016; Li et al., 2016; Kaiser et al., 2017a; Zhang et al., 2018; Zhang et al., 2020). Given that solar irradiance incident on a leaf often fluctuates, these dynamic limitations of photosynthesis decrease photosynthetic irradiance use efficiency (Morales et al., 2018). Improving gs, including its dynamics, is an attractive means with which to improve both irradiance and water use efficiency (Lawson and Blatt, 2014; Vialet-chabrand et al., 2017). Increases in the limitation imposed upon A by gs can be identified via transient decreases of leaf internal CO2 partial pressure (Ci, µbar). The other two main limitations during photosynthetic induction arise from slow rates of change in the activity of enzymes involved in ribulose-1,5-bisphosphate (RuBP) regeneration, and from slow activation of Rubisco (Pearcy et al., 1996; Way and Pearcy, 2012; Kaiser et al., 2015; Kaiser et al., 2018). These limitations occur additionally to the limitations at steady state due to, e.g., the rate of electron transport, Calvin cycle metabolism, sucrose metabolism, or mesophyll conductance (gm, mol m−2 s−1).
Disentangling stomatal and other limitations during photosynthetic induction is difficult. Many treatments affecting gs also affect other transient limitations of photosynthetic induction, such as leaf temperature (Kaiser et al., 2017a) and salt stress (Zhang et al., 2018). Similarly, some genetic mutations affecting gs, such as the abscisic acid (ABA) deficient aba2-1 mutant in Arabidopsis thaliana, showed enhanced A/Ci responses compared to its wildtype, Col-0 (Kaiser et al., 2016), suggesting pleiotropic effects, which may confound effects of altered gs. Also, models estimating transient stomatal limitation have often been based on linear – not curvilinear – A/Ci relationships (Woodrow and Mott, 1989; Tinoco-Ojanguren and Pearcy, 1993), or are based on steady-state A/Ci responses (Kaiser et al., 2017a). In fact, the maximum rate of carboxylation (Vcmax), for example, increases strongly during photosynthetic induction (Soleh et al., 2016; Taylor and Long, 2017). In another approach, the limitation during induction was attributed to gs alone up to the point where A reached 95% of the steady-state value (McAusland et al., 2016), entirely ignoring limitations by RuBP regeneration and Rubisco activation kinetics. Tools to better separate stomatal from other limitations are thus warranted, and mutants or transformants with substantially altered stomatal characteristics but similar photosynthetic biochemistry (Raissig et al., 2017; Papanatsiou et al., 2019; Tomimatsu et al., 2019; Kimura et al., 2020; Yamori et al., 2020) can be counted among such tools.
The tomato (Solanum lycopersicum L.) flacca mutant has a 80% to 90% lower ABA content than its wildtype (Tal and Nevo, 1973; Sagi et al., 2002). Flacca leaves exhibit a very high gs, without affecting the A/Ci response, suggesting that photosynthetic capacity is independent of ABA (Bradford et al., 1983). Lack of ABA has not been found to affect gm in the aba-1 mutant of Nicotiana plumbaginifolia (Mizokami et al., 2015) but to our knowledge has not been determined in flacca. The aim of this study is to determine the dynamic limitations of photosynthetic induction due to gs in tomato leaves. For this, steady-state and dynamic photosynthesis characteristics were measured in the ABA-deficient flacca mutant and its wildtype, by the use of simultaneous gas exchange and chlorophyll fluorometry.
Materials and Methods
Plant Material
Seeds of tomato cv. Rheinlands Ruhm wildtype (LA0535) and flacca (LA0673) were obtained from the Tomato Genetics Resource Center (University of California, Davis, USA). Seeds were germinated in stonewool plugs (Grodan, Roermond, NL). A week after sowing, they were transferred to stonewool cubes (10 cm × 10 cm × 7 cm; Grodan). Plants were grown in a climate chamber under a day/night cycle of 16/8 h (day/night), 20/18°C temperature, ambient CO2 partial pressure, 70% relative air humidity, and 154 µmol m−2 s−1 photosynthetically active radiation (PAR; measured 10 cm above table height), which was provided by fluorescent tubes (Master TL-D 58W/840 Reflex Eco; Philips, Eindhoven, the Netherlands). Stonewool cubes were standing in a layer (height, 1–2 cm) of nutrient solution (Yara Benelux B.V., Vlaardingen, the Netherlands), which was replenished every 1 to 2 days and contained 12.4 mM NO3−, 7.2 mM K+, 4.1 mM Ca2+, 3.3 mM SO42−, 1.8 mM Mg2+, 1.2 mM NH4+, 1.1 mM PO43−, 30 μM BO33−, 25 μM Fe3+, 10 μM Mn2+, 5 μM Zn2+, 0.75 μM Cu+, and 0.5 μM MoO42− (EC 2.1 dS m−1, pH 5.5). Between 1 and 4 weeks after sowing, flacca plants were sprayed daily with a solution containing 10 μM ABA, 0.01% (w/v) Triton-X, and 0.1% (v/v) ethanol (Bradford et al., 1983), using commercially available horticultural hand sprayers. Wildtype plants were sprayed with a control solution containing 0.01% Triton-X and 0.1% ethanol. Untreated flacca plants are smaller and show much higher transpiration rates than the wildtype, together with leaf epinasty and strong root formation along the stem (Tal, 1966). Growing flacca with application of ABA causes plants to grow similarly well as the wildtype (Imber and Tal, 1970). When the application of ABA is stopped, flacca reverts to its mutant phenotype within days, including always-open stomata (Imber and Tal, 1970). All chemicals were purchased from Sigma (St. Louis, MO, USA).
Measurements
When plants were between 5 and 6 weeks old, the fourth leaf, counting from the bottom, was selected for measurements. ABA spraying was stopped seven days before the start of measurements, to allow the high gs phenotype of flacca to reassert itself. Measurements were performed in a lab, using the LI-6400 XT photosynthesis system (LI-COR Biosciences, Lincoln, Nebraska, USA) equipped with a fluorescence chamber (leaf area: 2 cm2). Conditions inside the leaf chamber during measurements were: 25°C chamber temperature, 7 mbar leaf-to-air vapour pressure deficit (VPD; except when stated otherwise) and a flow rate of 500 µmol s−1. Irradiance was provided by LEDs in a 90/10 red/blue irradiance mixture, with peak intensities at wavelengths of 635 and 465 nm, respectively. For all measurements, five plants per genotype were used (n = 5).
All measurements were performed on the same spot of a leaf, to reduce measurement noise caused by spatial variation (Lawson and Weyers, 1999; Matthews et al., 2017): (a) dark-adapted Fv/Fm, (b) A/PAR curves at 2% oxygen, (c) A/Ci curves at 2% oxygen, (d) A/Ci curves at 21% oxygen, (e-g) photosynthetic induction under three different environmental conditions (described below). While measurements a-d were performed in the same sequence, the order of photosynthetic induction measurements was randomized for each plant. Values of A were corrected for CO2 leakage based on the manufacturers' suggestions. Measurements were started at 7:30 in the morning and took 8 to 9 h to complete per leaf.
Dark-Adapted Fv/Fm and Net CO2 Exchange in Darkness
Leaves were dark-adapted for 20 minutes. Then, net CO2 exchange in darkness (Adark) was logged, after which a weak measuring beam was turned on to measure Fo. Then, Fm was determined by exposing the leaf to a single-pulse saturating flash of ~9.000 µmol m−2 s−1 intensity and 1-s duration. Dark-adapted Fv/Fm was calculated as Fv/Fm = (Fm − Fo)/Fm.
A/PAR Curves at 2% Oxygen
A gas mixture containing 2% oxygen and 98% nitrogen was fed to the inlet of the LI-6400 XT. Leaf external CO2 partial pressure (Ca) was set to 2000 µbar, and irradiance was set to 200 µmol m−2 s−1. After reaching steady-state A, irradiance was decreased in steps of 150, 100, 70, 50, and 30 µmol m−2 s−1, and A was logged for 30 s after reaching steady-state A, at steps of 5 s. Values were later averaged at each step to reduce measurement noise.
A/Ci Curves at 2% Oxygen
A gas mixture containing 2% oxygen and 98% nitrogen was fed to the inlet of the LI-6400 XT. Irradiance was set to 1,500 µmol m−2 s−1, and Ca was set to 150 µbar. After reaching steady-state A, Ca was decreased in steps of 130, 100, 70, and 50 µbar. A and Ci were logged as described above. At each Ca, the infrared gas analysers were matched.
A/Ci Curves at 21% Oxygen
Irradiance was set to 1,500 µmol m−2 s−1, and Ca was set to 400 µbar. After reaching steady-state A, Ca was decreased in steps of 300, 200, 130, 100, 70 and 50 µbar. Then, Ca was raised to 400 µbar and after reaching steady-state A, Ca was increased in steps of 600, 750, 900, 1,100, 1,400, 1,700, and 2,000 µbar. A and Ci were logged after reaching steady-state (3–5 min per step) as described above. At each Ca, the infrared gas analysers were matched. Parameters describing maximum rate of carboxylation (Vcmax), rate of linear electron transport at the measuring irradiance (J1500) and triose phosphate utilization capacity (TPU) were determined using the excel solver tool by Sharkey (2016). Additionally, operating and maximal fluorescence in light-adapted leaves (F and Fm′, respectively) were determined at each Ca by using a multi-phase flash protocol (MPF; Loriaux et al., 2013). The maximum intensity of the MPF was ~9.000 µmol m−2 s−1, the durations of the three phases were 0.3, 0.7 and 0.4 s respectively, and the percentage decrease of flash intensity during phase two was 60%. These MPF settings were found to yield the most accurate results in pilot experiments (data not shown). Photosystem II operating efficiency (ΦPSII) was calculated as ΦPSII = (Fm′ − F)/Fm′. Mesophyll conductance (gm) was determined following the variable J method proposed by Harley et al. (1992); the variables A, Ci, and ΦPSII to calculate gm were determined at a Ca of 400 µbar and an irradiance of 1500 µmol m−2 s−1. Parameters to calculate gm, namely Rd, Γ* and s, were determined from A/Ci and A/PAR measurements at 2 and 21% oxygen following Yin et al. (2009).
Photosynthetic Induction
Leaves were adapted to 50 µmol m−2 s−1 until gs was constant (40–60 minutes). Irradiance was then increased to 1500 µmol m−2 s−1 in a step change, and gas exchange values were logged every 2 s for 60 min. These measurements were performed at Ca and air humidity close to the plant’s growth conditions (400 µbar Ca, 7 mbar VPD), termed “control” hereafter. Photosynthetic induction was additionally assessed under two other conditions: “high VPD” (15 mbar) and “low CO2” (200 µbar), keeping all other conditions the same. During photosynthetic induction, chlorophyll fluorescence was measured using a saturating MPF (described above) once every minute during the first ten minutes, and once every two minutes thereafter. Photosynthetic induction (PI, %) was calculated as a percentage of the total change between initial A (Ai) and final A (Af) of each transient: PI = (A-Ai)/(Af-Ai)*100. Intrinsic water use efficiency (WUEi) was calculated as WUEi = A/gs. Non-photochemical quenching (NPQ) during photosynthetic induction was calculated as NPQ = (Fm − Fm′)/Fm′. The coefficient of photochemical quenching (qP) and the efficiency of open photosystem II traps (Fv′/Fm′) were calculated after Oxborough and Baker (1997), as qP = (Fm′ − F)/(Fm′ − Fo′) and Fv′/Fm′ = (Fm′ − Fo′)/Fm′, where Fo′ is minimal fluorescence from irradiance- adapted leaves. Fo′ was calculated after Oxborough and Baker (1997).
Statistical Analysis
All statistical tests were performed at P=0.05 as threshold for significance. Where appropriate, a two-sided Student’s t-test was used to determine significant differences between genotypes. For photosynthetic induction under different environmental conditions, a two-way analysis of variance (ANOVA) was performed, and interaction means were separated based on Fisher’s least significant difference test. Residuals were tested for normal distribution (Shapiro-Wilk test) and equal variances were assumed for treatment groups. If the requirement for normal distribution was not fulfilled, the procedure was repeated on log-transformed data. If after log transformation residuals still did not show normality, Kruskal-Wallis one-way ANOVA, considering six treatments (three environmental conditions times two genotypes), was performed using the original data. In case of significant treatment effects, Dunn’s test of multiple comparisons was performed to identify differences between the six treatments. All statistical tests were performed in Genstat (VSN international, Hempstead, UK) except for Dunn’s test, which was performed in R (R Core Team, 2020) using the dunn.test package (Dinno, 2017).
Results
Steady-State CO2 and Irradiance Responses of Photosynthesis
Wildtype and flacca leaves showed very similar responses of A and ΦPSII to Ci (Figure 1). In the CO2 range 50 to 300 µbar, A increased near-linearly, then peaked at ~500 µbar and with further increases in Ci, A declined in both genotypes. Compared to A, ΦPSII peaked at lower Ci (~300 µbar) and exhibited a stronger decline with further increases in Ci. Parameters describing photosynthetic capacity, i.e., Vcmax, J1500 and TPU, were not significantly different between genotypes (Figure 1, insert). Mesophyll conductance and its components were not significantly different between genotypes (except Ci, which was significantly greater in flacca, Figure 2C), although flacca tended to show greater values for A, J, Rd, and Cc (Figure 2). In dark-adapted leaves, A was −1.2 ± 0.1 µmol m−2 s−1 in wildtype and −1.9 ± 0.1 µmol m−2 s−1 in flacca leaves (p=0.008). At low irradiance (50 µmol m−2 s−1), on the other hand, A was similar between genotypes (Table 1).
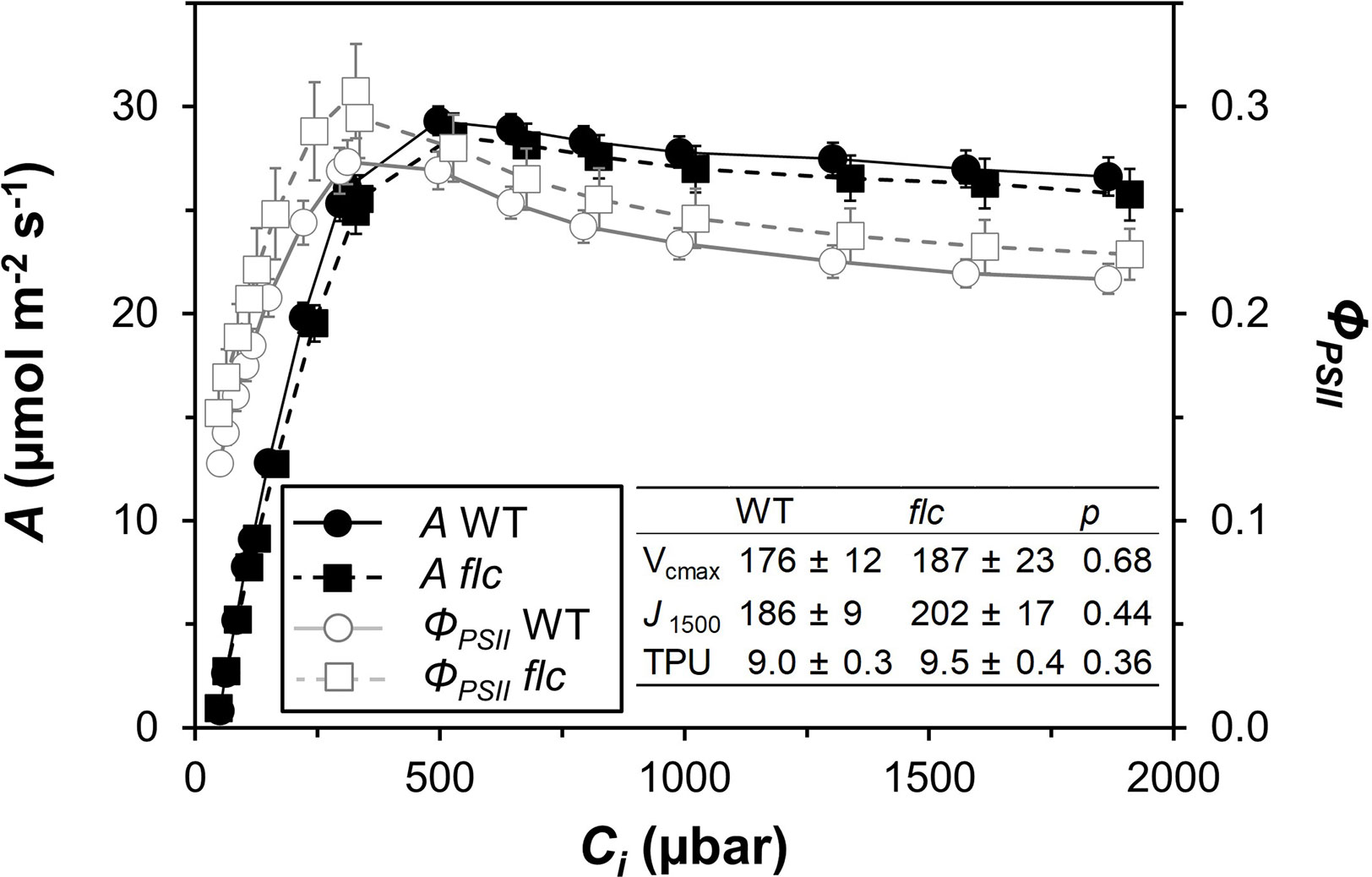
Figure 1 Steady-state responses of net photosynthesis rate (A) and photosystem II operating efficiency (ΦPSII) to leaf internal CO2 partial pressure (Ci), in wildtype (WT) and flacca (flc) leaves, measured at 21% oxygen. Insert: parameters derived from curve fitting (after Sharkey, 2016) to A/Ci response (unit for all: μmol m−2 s−1), namely maximum rate of carboxylation (Vcmax), rate of linear electron transport at 1500 μmol m−2 s−1 PAR (J1500) and triose phosphate utilisation capacity (TPU); P values for comparisons between WT and flc based on two-sided t-test. Symbols and numbers represent averages ± SEM, n = 5.
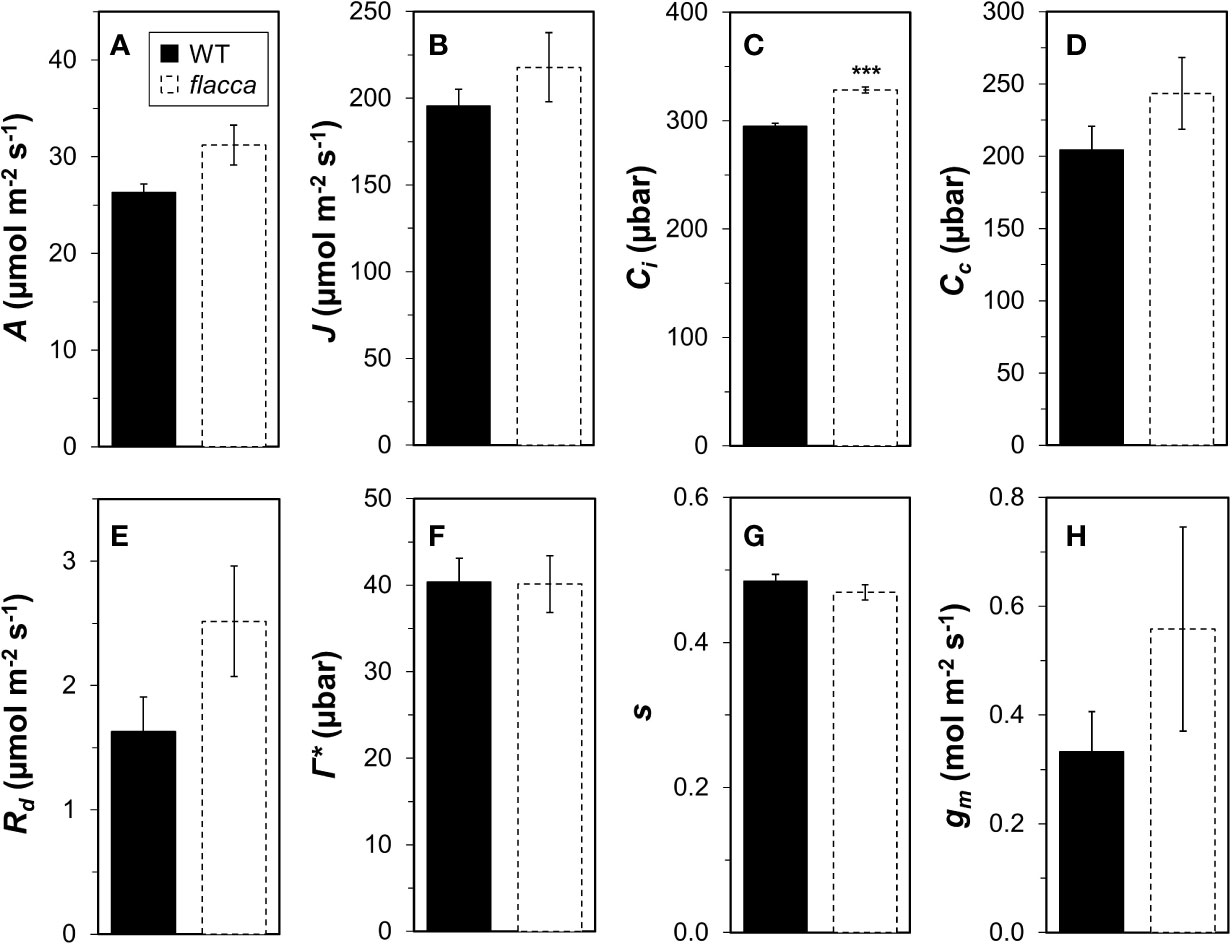
Figure 2 Analysis of mesophyll conductance (gm) and its components in wildtype (WT) and flacca leaves. (A) net photosynthesis rate (A); (B), linear electron transport rate (J); (C) substomatal CO2 partial pressure (Ci); (D) chloroplast CO2 partial pressure (Cc); (E) mitochondrial respiration (Rd); (F) CO2 compensation point in the absence of mitochondrial respiration (Γ*); (G) lumped parameter to convert ΦPSII to J (s); (H), gm. A, J and Ci were determined under 1000 μmol m−2 s−1 PAR, 400 μbar CO2 atmospheric partial pressure and 25°C chamber temperature. Rd, Γ*, and s were determined from A/Ci and A/PAR curves under photorespiratory and non-photorespiratory conditions after Yin et al. (2009). Bars and error bars represent averages ± SEM, n = 5. ***P<0.01.
Response of Photosynthetic Gas Exchange to a Stepwise Irradiance Increase
Next, we tested how gas exchange in wildtype and flacca leaves that had been adapted to low irradiance (50 µmol m−2 s−1) reacted to a stepwise increase to high irradiance (1500 µmol m−2 s−1). In wildtype leaves, the rate of photosynthetic induction was slower at high VPD, compared to the other two treatments (low CO2 or high VPD; Figure 3A), while in flacca, there was no difference between control and high VPD treatments (Figure 3B). However, while in wildtype leaves the rate of photosynthetic induction was the same in the control and low CO2 treatments, in flacca, induction at low CO2 was slower than in the control treatment (Figures 3A, B). The flacca mutation had significant effects on the times to reach 50 (t50) and 90% (t90) of full photosynthetic induction: under control conditions, t50 was 91% and t90 was 46% of wildtype values in flacca, while under high VPD, t50 was 63% and t90 was 36% of wildtype values in flacca (Figure 4). Both indices were not significantly different between genotypes under low CO2 (Figure 4). Transient A was higher in flacca than in wildtype leaves, and in both genotypes was slightly higher in control than in high VPD, as well as substantially reduced at low CO2 (insets in Figures 3A, B; Table 1).
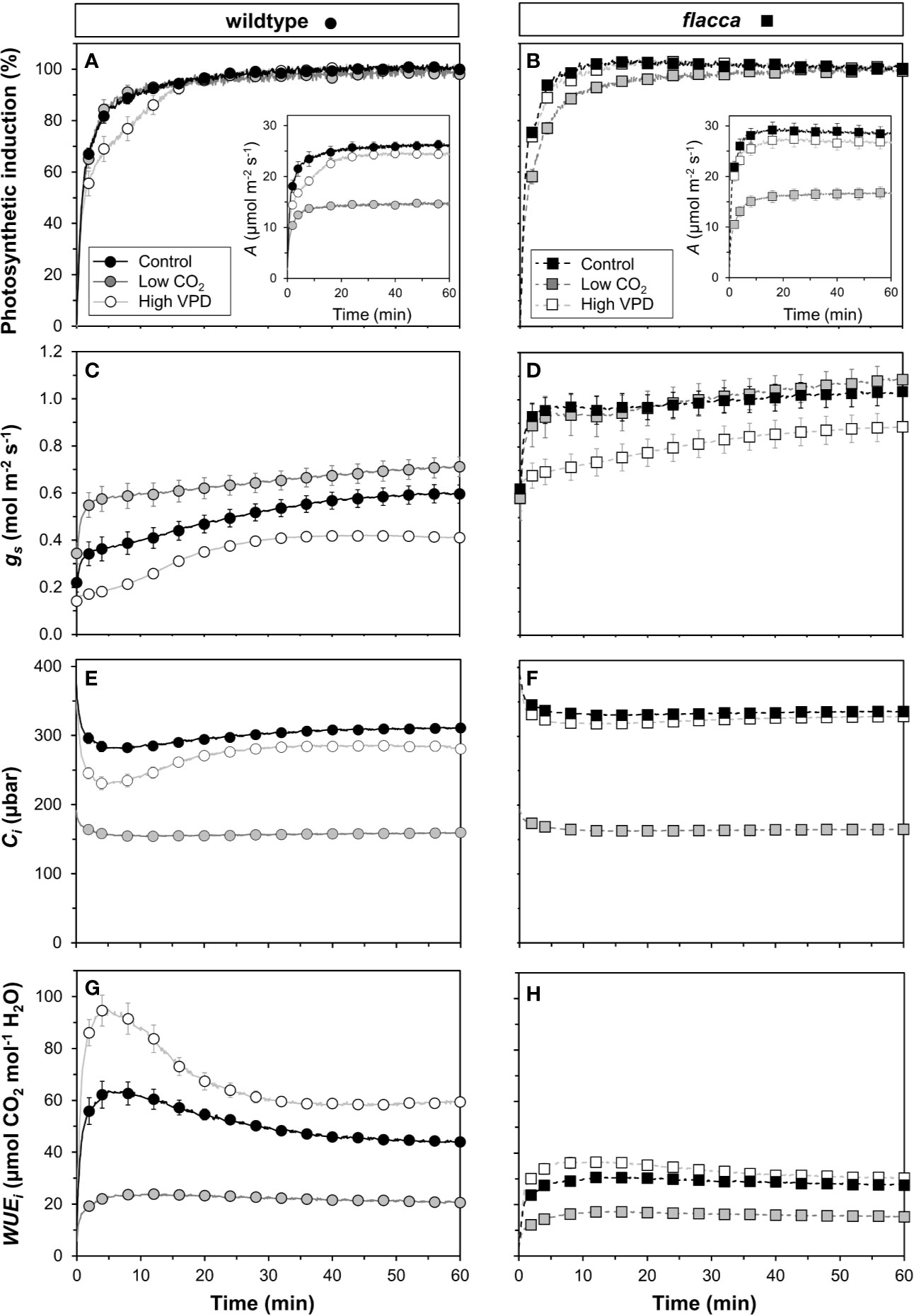
Figure 3 Time courses of gas exchange in wildtype (left panel) and flacca leaves (right panel) after a transition from low to high irradiance: (A, B) photosynthetic induction; (C, D) stomatal conductance (gs); (E, F) leaf internal CO2 partial pressure (Ci); (G, H) intrinsic water use efficiency (WUEi). Insets in (A, B) show net photosynthesis rates (A). Leaves initially adapted to 50 µmol m−2 s−1 PAR were exposed to 1500 µmol m−2 s−1 PAR at time = 0 min. Data were logged at 400 µbar CO2 and 7 mbar leaf-to-air VPD (Control), 200 instead of 400 µbar (Low CO2) and 15 instead of 7 mbar (High VPD). Lines and symbols represent averages ± SEM, n = 5.
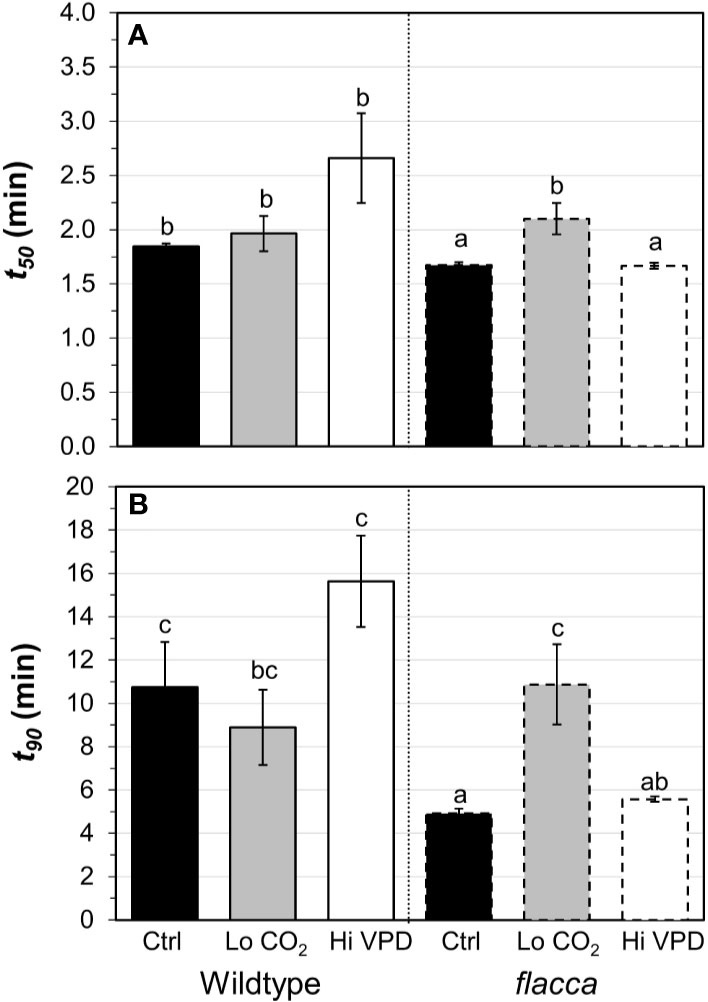
Figure 4 Time to reach 50 (A, t50) and 90% (B, t90) full photosynthetic induction of wildtype and flacca leaves after a step change from low to high irradiance. Experimental conditions identical to those described under Figure 3. Different letters depict significant differences (P<0.05) between ranks, as determined by Dunn’s test. Bars and error bars represent averages ± SEM, n = 5.
In flacca, A showed a small decrease between ~1.5 and 2.0 min after the stepwise increase in irradiance under control and high VPD conditions (Figure S1B). These dynamics are very similar to those previously seen in shade-adapted wildtype tomato leaves undergoing photosynthetic induction under high CO2 partial pressure (Kaiser et al., 2017b), and in the present study were observed neither at low CO2 (Figure S1B), nor in wildtype leaves (Figure S1A). The most likely explanation for this phenomenon is a transient mismatch between the rate of CO2 fixation in the Calvin cycle and downstream sucrose metabolism, which would transiently limit the availability of free phosphate in the chloroplast (Prinsley and Leegood, 1986; Stitt and Grosse, 1988; Stitt and Quick, 1989).
During the complete trajectory of photosynthetic induction, stomatal conductance (gs) in wildtype leaves was strongly increased by lowering CO2, and substantially reduced by increasing VPD (Figure 3C; Table 1). In flacca, gs was lower in the high VPD treatment compared to both other treatments during photosynthetic induction (Figure 3D; Table 1). Intriguingly, while the low irradiance adapted (initial) gs in wildtype leaves reacted to the treatment levels in a predictable way, i.e., decreasing under high VPD and increasing under low CO2 relative to control (Figure 3C), initial gs in flacca did not (Figure 3D; Table 1). Also, gs in flacca was substantially higher than that in wildtype leaves in all cases, as would be expected of an ABA mutant.
In control conditions, Ci in wildtype leaves showed an initial decrease in the first 10 minutes of photosynthetic induction, after which it gradually increased as stomata opened (Figure 3E). This decrease was exacerbated in the high VPD treatment. Even after partial recovery of Ci due to stomatal opening, Ci did not reach control values when approaching steady state (Figure 3E; Table 1). In flacca leaves, Ci decreased less strongly under both control and high VPD conditions, and did not increase much during the remainder of photosynthetic induction (Figure 3F). Also, Ci time courses in both of these treatments were virtually indistinguishable in flacca, which is explained by the diminished reduction of gs under high VPD (Figure 3D). Under low CO2, Ci was similar in wildtype and flacca, displaying only small decreases in the beginning of photosynthetic induction without subsequent recovery (Figures 3E, F).
Intrinsic water use efficiency (WUEi) was roughly twice as high in wildtype compared to flacca leaves (Figures 3G, H). In the wildtype, a high VPD resulted in large increases (+31%), and a low CO2 in large decreases (−56%), of WUEi relative to control conditions (Figure 3G). In flacca, WUEi was markedly reduced (−50%) under low CO2 relative to the other two conditions (Figure 3H). While WUEi showed strong dynamics in the first 30 minutes after exposure to high irradiance under high VPD and control conditions in the wildtype, it plateaued early after an initial increase (<5 min) in all other cases (Figures 3G, H).
Chlorophyll Fluorescence Dynamics During Photosynthetic Induction
In wildtype leaves, an initial rapid increase in ΦPSII within the first ~8 min was followed by a slower, more gradual increase towards a steady state under control and high VPD conditions until ~40 min (Figure 5A). In flacca leaves in control and high VPD conditions, a gradual decrease was observed after the initial increase in ΦPSII (Figure 5B). In both genotypes, ΦPSII under low CO2 stabilized quickly at lower values and then plateaued. Dark-adapted Fv/Fm was ~0.82 in both genotypes (n.s., Figure 5B, inset). A rapid increase in NPQ in the first five minutes was followed by a decrease until 10 to 20 min, which was followed by a slower increase until the final measurement after 60 minutes (Figures 5C, D). Under control and high VPD conditions, NPQ initially rose to much higher values in wildtype (1.6–1.7) compared to flacca leaves (1.4–1.5). The subsequent decrease to a local minimum showed a greater amplitude in wildtype (~0.1) compared to flacca leaves (~0.05). Under low CO2, NPQ tended to be greater in both genotypes compared to the other treatments. The coefficient of photochemical quenching (qP) showed dynamics similar to those of ΦPSII (Figures 5E, F). qP and ΦPSII were highly correlated in all treatments (R2 >0.99). The efficiency of open photosystem II traps (Fv′/Fm′) showed dynamics that were the inverse of those of NPQ (Figures 5G, H); NPQ and Fv′/Fm′ were highly correlated (R2 >0.99). These correlations suggest that ΦPSII dynamics were largely due to changes in qP rather than changes in Fv′/Fm′ or NPQ (Baker et al., 2007).
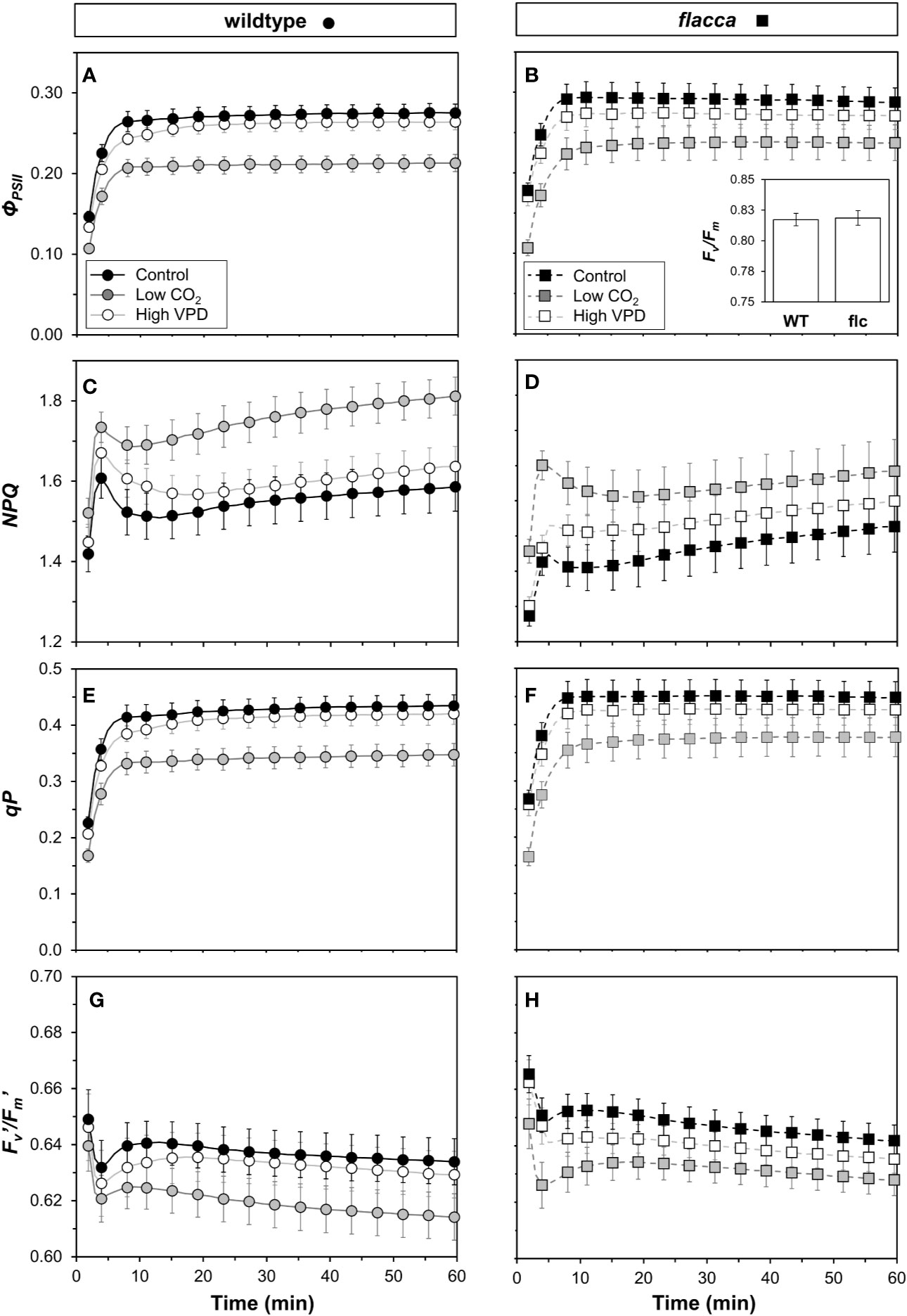
Figure 5 Time courses of chlorophyll fluorescence in wildtype (WT, left panel) and flacca leaves (flc, right panel) after a transition from low to high irradiance. (A, B) photosystem II operating efficiency (ΦPSII); (C, D) non-photochemical chlorophyll fluorescence quenching (NPQ); (E, F) coefficient of photochemical quenching (qP); (G, H) efficiency of open photosystem II traps (Fv′/Fm′). Experimental conditions identical to those described under Figure 3. Lines and symbols represent averages ± SEM, n = 5.
Stomatal Effects on Rate of Photosynthetic Induction
Next, we explored several ways to visualize the effects of (partially) closed stomata on photosynthetic induction. First, initial, low-irradiance adapted gs was plotted against t90 (Figure 6A). Across both genotypes, there was a consistent threshold-type relationship between initial gs and t90: at initial gs <0.4 mol m−2 s−1, t90 increased strongly with decreases in initial gs, reaching values of ~15 min at an initial gs of 0.11 mol m−2 s−1. At initial gs >0.4 mol m−2 s−1, the value of t90 (~5 min) was unaffected by further increases in initial gs. Roughly, a similar threshold was visible between t50 and initial gs, as initial gs <0.2 mol m−2 s−1 tended to increase t50, while t50 was unaffected by differences in initial gs in the range 0.2 to 0.8 (Figure 6A, inset). Initial gs versus t50 or t90 did not show a similar relationship at low CO2 (Figure S2) and was therefore omitted from Figure 6A.
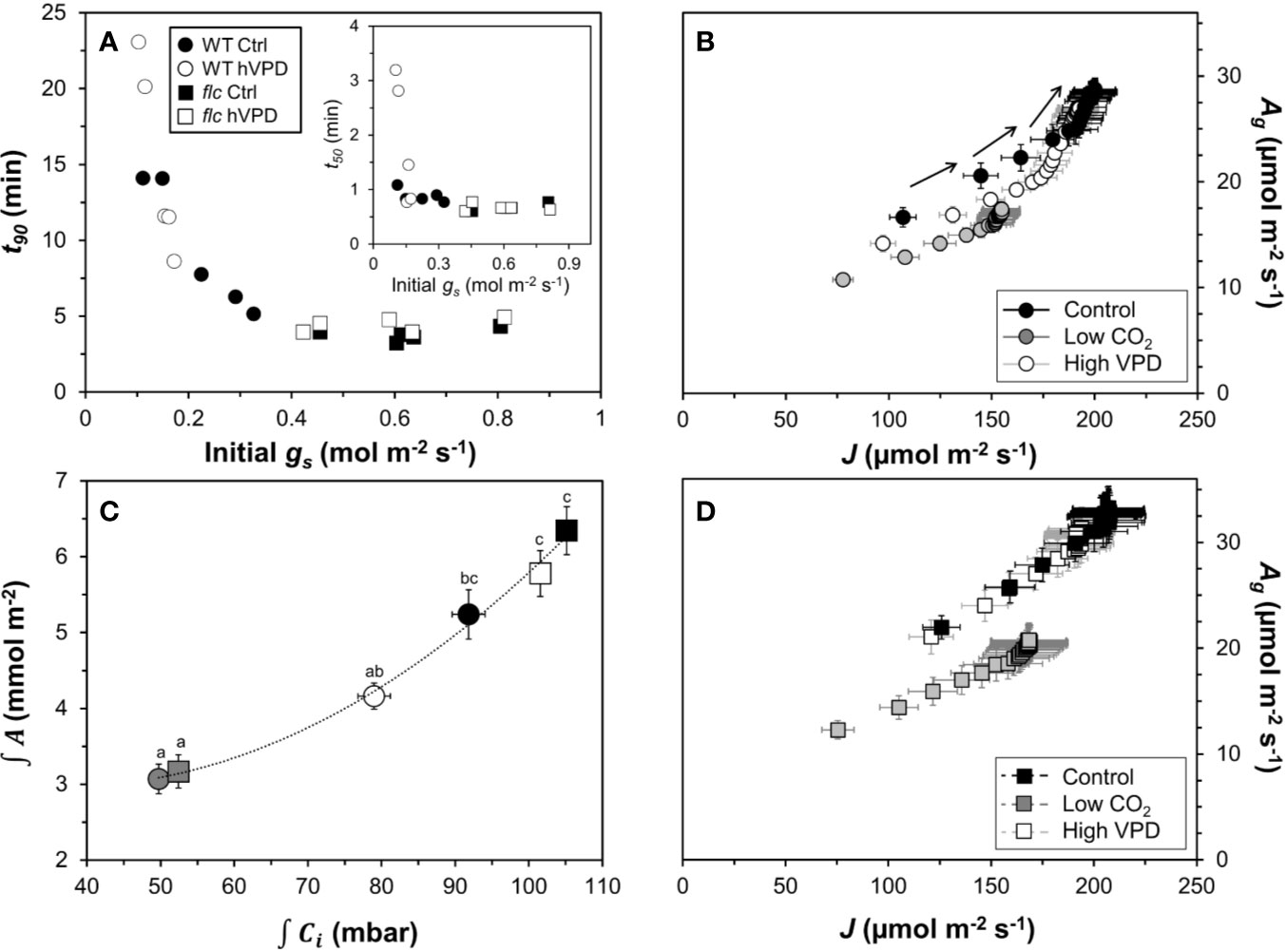
Figure 6 Effects of stomatal conductance on photosynthetic induction. (A) relationship between stomatal conductance of leaves adapted to 50 µmol m−2 s−1 PAR (initial gs) and time to reach 90% of full photosynthetic induction (t90) after a switch to high irradiance, inset: relationship between initial gs and t50; (B) relationship between electron transport rate (J) and gross photosynthesis rate (Ag) in the wildtype, arrows show sequence in which data were logged; (C) relationship between integrated net photosynthesis rate (∫A) and integrated leaf internal CO2 partial pressure (∫Ci) during the first five minutes of photosynthetic induction, different letters indicate statistically significant (P<0.05) differences between treatments; (D) relationship between J and Ag in flacca. Experimental conditions identical to those described under Figure 3. Symbols represent averages ± SEM, n = 5.
Photosynthesis integrated over the initial five minutes of photosynthetic induction scaled well with Ci integrated over the same period (Figure 6C). This suggests that A was affected by Ci, while the change in Ci was due to treatment and/or genotype effects on gs. Finally, plotting J vs. gross photosynthesis rates (Ag, calculated as A plus Rd) produced very similar results between control and high VPD conditions in flacca leaves (Figure 6D), while in wildtype leaves Ag showed higher values for the same J in control compared to high VPD conditions (Figure 6B). Under low CO2, both genotypes showed decreased Ag for a given J. Also, while in flacca leaves the plots of Ag vs. J were highly linear (Figure 6D), in wildtype leaves they showed an upwards curvature at higher Ag/J values, i.e., Ag increased more strongly than J (Figure 6B). This upwards curvature is indicative of an increase in the rate of carboxylation relative to the rate of oxygenation, most likely caused by an increase in Ci due to increased stomatal opening (Kaiser et al., 2017a; Zhang et al., 2018).
Discussion
In recent years, the dynamic responses of photosynthesis to fluctuating light, and their limitations, have received more attention. It is now widely recognised that increasing photosynthesis is a pathway to increasing crop productivity (Ort et al., 2015), that crops frequently encounter light intensity fluctuations (Kaiser et al., 2018), and that alleviating some of the limitations acting on photosynthesis transients can strongly increase biomass (Kromdijk et al., 2016). Indeed, research looking into reductions of limitations acting on dynamic photosynthesis currently receives a lot of attention (Tanaka et al., 2019; Acevedo-Siaca et al., 2020; Kimura et al., 2020; Yamori et al., 2020).
Greater Stomatal Conductance Increases the Rate of Photosynthetic Induction
Responses of A to Ci, as well as mesophyll conductance, were similar between the flacca mutant and the wildtype (Figures 1 and 2), while stomatal conductance was strongly enhanced in flacca leaves (Figures 3C, D). This confirms our hypothesis that the flacca mutant is a useful system for strongly reducing stomatal limitations and, via their reduction, better understanding their effects. This is similar to earlier reports using genotypes with “always-open” stomata (Tomimatsu and Tang, 2012; Tomimatsu et al., 2019; Kimura et al., 2020; Yamori et al., 2020). Our results further suggest that the faster photosynthetic induction observed in flacca compared to wildtype leaves under control and high VPD conditions (Figures 3A, B and 4) was indeed due to much higher stomatal conductance (difference between genotypes in initial gs was ~0.4 mol m−2 s−1). Initial gs in leaves adapted to darkness or shade strongly impacts on rates of photosynthetic induction upon illumination with high irradiance. It has been reported previously that parameters such as the time needed to reach 50 or 90% of full photosynthetic induction (t50 and t90, respectively) show a strong bimodal relationship with initial gs (Valladares et al., 1997; Allen and Pearcy, 2000; Kaiser et al., 2016), similar as shown in the present study (Figure 6A).
The decrease in initial gs in the wildtype upon high VPD (Figure 3C) translated into a marked decrease in Ci during photosynthetic induction (Figures 3E and 6C). This decrease was less strong in control conditions and was barely visible in flacca under control or high VPD (Figure 3F), as initial gs in flacca did not react to high VPD (Figure 3D). The reduced transient availability of Ci decreased the rate of photosynthetic induction in the wildtype under high VPD (Figure 3A). This reduction, in turn, fed back on dynamic ΦPSII (which was slowed down; first 15 minutes in Figure 5A), NPQ (which initially increased to higher levels and then relaxed less quickly than under control conditions; Figure 5C) and the relationship between gross photosynthesis and electron transport (Figure 6B). Unlike initial gs, stomatal opening (difference between initial and final gs) was not different between wildtype and flacca leaves under control (~0.4 mol m−2 s−1) and high VPD conditions (~0.3 mol m−2 s−1; Table 1).
Perhaps surprisingly, photosynthetic induction was not different between genotypes under low CO2 partial pressure (Figures 3 and 4). This may be explained in two ways: firstly, initial gs in the wildtype increased, from 0.22 mol m−2 s−1 at 400 µbar to 0.34 mol m−2 s−1 at 200 µbar, while that in flacca did not (Table 1). This gs increase in wildtype leaves almost halved the difference in initial gs between genotypes (0.4 → 0.24 mol m−2 s−1). Secondly, any positive effect that the remaining difference in initial gs may have had on photosynthetic induction in flacca was probably additionally decreased by low CO2 availability.
Initial gs in Leaves Lacking ABA Does Not React to Low CO2 or High VPD
A striking finding of the present study was that while gs in wildtype leaves (as expected) increased upon reductions in CO2 partial pressure (Figure 3C, Table 1), gs in flacca was virtually unchanged (Figure 3D). This confirms that ABA is part of the CO2 signalling pathway in stomatal regulation (reviewed in Engineer et al., 2016). Under high VPD (15 mbar), gs in flacca leaves adapted to low irradiance was similar to that at low VPD (7 mbar; Figure 3D). In wildtype leaves, stomata again responded as expected, by reducing their aperture under increased VPD (Figure 3C). At high irradiance, however, gs in flacca did respond to the increase in VPD, as its value was reduced by ~0.15 mol m−2 s−1 compared to that at 7 mbar (Table 1). While there is ongoing controversy about the role of ABA in stomatal sensing of humidity, Merilo et al. (2018) showed that a number of genotypes that are either ABA deficient or ABA insensitive closed their stomata when exposed to an increase in VPD (at 150–500 µmol m−2 s−1 PAR). The authors explained this phenomenon (which is at variance with Mcadam et al., 2015; McAdam et al., 2016) as most likely being a hydropassive response, resulting from much higher initial gs in ABA mutants, and thus a greater drop in humidity in the substomatal cavity (Merilo et al., 2018).
Limitations of the Study
In this study, we only used one mutant (flacca, LA0673) to examine the effects of open stomata on the rate of photosynthetic induction. Ideally, a larger number of mutants, each resulting in differential gs relative to its wildtype, should be used; this to make sure that the observed effects on photosynthetic induction were truly caused by gs rather than some other putative effects of the flacca mutation. Secondly, during growth flacca plants were regularly sprayed with ABA, following the recommendations of Imber and Tal (1970). It may be that this ABA application triggered unwanted responses in the plants, although based on all results presented here it seems that photosynthesis was fully functional in flacca.
Conclusions and Outlook
The current study suggests that in wildtype leaves, gs exerts a substantial limitation on non-steady state photosynthesis. The flacca mutant can partially overcome this limitation through stomata that remain open in low light, resulting in substantially reduced t90 in ambient CO2 partial pressure (t90 was 36–46% in in flacca relative to wildtype values). Nevertheless, while flacca is a good system for testing (dynamic) stomatal limitation in the laboratory, breeding for a similar stomatal behaviour will not be useful for most crops (except possibly for production in wetland areas), as this improvement of photosynthesis in fluctuating light will come with a significant reduction in water use efficiency and a major fitness disadvantage. A more promising approach may be to improve stomatal responsiveness to light intensity fluctuations, as this can potentially increase both light and water use efficiency under fluctuating light intensities. Recent, promising examples of increased gs responsiveness are the BLINK1 transformant (Papanatsiou et al., 2019) and the PATROL1 overexpressor (Kimura et al., 2020).
Data Availability Statement
All datasets presented in this study are included in the article/Supplementary Material.
Author Contributions
EK and AM designed the study with input from all other authors. EK performed measurements and data analysis. EK wrote the manuscript with input from all other authors.
Conflict of Interest
The authors declare that the research was conducted in the absence of any commercial or financial relationships that could be construed as a potential conflict of interest.
Acknowledgments
We thank the C.M. Rick Tomato Genome Resource Center (TGRC) for kindly supplying us with flacca and wildtype seeds.
Supplementary Material
The Supplementary Material for this article can be found online at: https://www.frontiersin.org/articles/10.3389/fpls.2020.01317/full#supplementary-material
Abbreviations
A, net photosynthesis rate; Ag, gross photosynthesis rate; ABA, abscisic acid; ANOVA, analysis of variance; Ca, leaf external CO2 partial pressure; Cc, chloroplast CO2 partial pressure; Ci, substomatal CO2 partial pressure; F, fluorescence emission from leaves under actinic irradiance; Fm, maximal fluorescence in dark-adapted leaves; Fm’, maximal fluorescence in light-adapted leaves; Fo, minimal fluorescence in dark-adapted leaves; Fv/Fm, maximum quantum efficiency of PSII photochemistry; Fv′/Fm′, efficiency of open PSII traps; gm, mesophyll conductance; gs, stomatal conductance; J, rate of linear electron transport; MPF, multi-phase flash protocol; PAR, photosynthetically active radiation; qP, coefficient of photochemical quenching; Rd, mitochondrial respiration; s, lumped parameter used to convert ΦPSII to J; TPU, triose phosphate utilization capacity; WUEi, intrinsic water use efficiency; Vcmax, maximum rate of carboxylation; VPD, leaf-to-air vapour pressure deficit; Γ*, CO2 compensation point in the absence of mitochondrial respiration; ΦPSII, photosystem II operating efficiency.
References
Acevedo-Siaca, L. G., Coe, R., Wang, Y., Kromdijk, J., Quick, W. P., Long, S. P. (2020). Variation in photosynthetic induction between rice accessions and its potential for improving productivity. New Phytol. 227, 1097–1108. doi: 10.1111/nph.16454. 10.1111/np.
Allen, M. T., Pearcy, R. W. (2000). Stomatal behavior and photosynthetic performance under dynamic light regimes in a seasonally dry tropical rain forest. Oecologia 122, 470–478. doi: 10.1007/s004420050968
Baker, N. R., Harbinson, J., Kramer, D. M. (2007). Determining the limitations and regulation of photosynthetic energy transduction in leaves. Plant Cell Environ. 30, 1107–1125. doi: 10.1111/j.1365-3040.2007.01680.x
Bradford, K. J., Sharkey, T. D., Farquhar, G. D. (1983). Gas exchange, stomatal behavior, and delta13C values of the flacca tomato mutant in relation to abscisic acid. Plant Physiol. 72, 245–250. doi: 10.1104/pp.72.1.245
Dinno, A. (2017).Dunn’s Test of Multiple Comparisons Using Rank Sums. Available at: https://cran.r-project.org/web/packages/dunn.test/dunn.test.pdf (Accessed last accessed June 7, 2020).
Engineer, C. B., Hashimoto-Sugimoto, M., Negi, J., Israelsson-Nordström, M., Azoulay-Shemer, T., Rappel, W.-J., et al. (2016). CO2 sensing and CO2 regulation of stomatal conductance: advances and open questions. Trends Plant Sci. 21, 16–30. doi: 10.1016/j.tplants.2015.08.014
Harley, P. C., Loreto, F., Di Marco, G., Sharkey, T. D. (1992). Theoretical considerations when estimating the mesophyll conductance to CO2 flux by analysis of the response of photosynthesis to CO2. Plant Physiol. 98, 1429–1436. doi: 10.1104/pp.98.4.1429
Imber, D., Tal, M. (1970). Phenotypic reversion of flacca, a wilty mutant of tomato, by abscisic acid. Sci. (80-. ). 169, 592–593. doi: 10.1126/science.169.3945.592
Kaiser, E., Morales, A., Harbinson, J., Kromdijk, J., Heuvelink, E., Marcelis, L. F. M. (2015). Dynamic photosynthesis in different environmental conditions. J. Exp. Bot. 66, 2415–2426. doi: 10.1093/jxb/eru406
Kaiser, E., Morales, A., Harbinson, J., Heuvelink, E., Prinzenberg, A. E., Marcelis, L. F. M. (2016). Metabolic and diffusional limitations of photosynthesis in fluctuating irradiance in Arabidopsis thaliana. Sci. Rep. 6 (31252), 1–13 .doi: 10.1038/srep31252. 10.1038/sr.
Kaiser, E., Kromdijk, J., Harbinson, J., Heuvelink, E., Marcelis, L. F. M. (2017a). Photosynthetic induction and its diffusional, carboxylation and electron transport processes as affected by CO2 partial pressure, temperature, air humidity and blue irradiance. Ann. Bot. 119, 191–205. doi: 10.1002/dvdy
Kaiser, E., Zhou, D., Heuvelink, E., Harbinson, J., Morales, A., Marcelis, L. F. M. (2017b). Elevated CO2 increases photosynthesis in fluctuating irradiance regardless of photosynthetic induction state. J. Exp. Bot. 68, 5629–5640. doi: 10.1093/jxb/erx357
Kaiser, E., Morales, A., Harbinson, J. (2018). Fluctuating light takes crop photosynthesis on a rollercoaster ride. Plant Physiol. 176, 977–989. doi: 10.1104/pp.17.01250
Kimura, H., Hashimoto-Sugimoto, M., Iba, K., Terashima, I., Yamori, W. (2020). Improved stomatal opening enhances photosynthetic rate and biomass production in fluctuating light. J. Exp. Bot. 71, 2339–2350. doi: 10.1093/jxb/eraa090
Kromdijk, J., Glowacka, K., Leonelli, L., Gabilly, S. T., Iwai, M., Niyogi, K. K., et al. (2016). Improving photosynthesis and crop productivity by accelerating recovery from photoprotection. Sci. (80-.). 354, 857–861. doi: 10.1126/science.aai8878
Lawson, T., Blatt, M. R. (2014). Stomatal size, speed, and responsiveness impact on photosynthesis and water use efficiency. Plant Physiol. 164, 1556–1570. doi: 10.1104/pp.114.237107
Lawson, T., Weyers, J. (1999). Spatial and temporal variation in gas exchange over the lower surface of Phaseolus vulgaris L. primary leaves. J. Exp. Bot. 50, 1381–1391. doi: 10.1093/jxb/50.337.1381
Li, T., Kromdijk, J., Heuvelink, E., van Noort, F. R., Kaiser, E., Marcelis, L. F. M. (2016). Effects of diffuse light on radiation use efficiency of two Anthurium cultivars depend on the response of stomatal conductance to dynamic light intensity. Front. Plant Sci. 7, 56. doi: 10.3389/fpls.2016.00056
Loriaux, S. D., Avenson, T. J., Welles, J. M., McDermitt, D. K., Eckles, R. D., Riensche, B., et al. (2013). Closing in on maximum yield of chlorophyll fluorescence using a single multiphase flash of sub-saturating intensity. Plant Cell Environ. 36, 1755–1570. doi: 10.1111/pce.12115
Matthews, J. S. A., Vialet-Chabrand, S. R. M., Lawson, T. (2017). Diurnal variation in gas exchange: the balance between carbon fixation and water loss. Plant Physiol. 174, 614–623. doi: 10.1104/pp.17.00152
Mcadam, S. A. M., Sussmilch, F. C., Brodribb, T. J., Ross, J. J. (2015). Molecular characterization of a mutation affecting abscisic acid biosynthesis and consequently stomatal responses to humidity in an agriculturally important species. AoB Plants 7, 1–11. doi: 10.1093/aobpla/plv091
McAdam, S. A. M., Sussmilch, F. C., Brodribb, T. J. (2016). Stomatal responses to vapour pressure deficit are regulated by high speed gene expression in angiosperms. Plant Cell Environ. 39, 485–491. doi: 10.1111/pce.12633
McAusland, L., Vialet-Chabrand, S., Davey, P., Baker, N. R., Brendel, O., Lawson, T. (2016). Effects of kinetics of light-induced stomatal responses on photosynthesis and water-use efficiency. New Phytol. 211, 1209–1220. doi: 10.1111/nph.14000
Merilo, E., Yarmolinsky, D., Jalakas, P., Parik, H., Tulva, I., Rasulov, B., et al. (2018). Stomatal VPD response: there is more to the story than ABA. Plant Physiol. 176, 851–864. doi: 10.1104/pp.17.00912
Mizokami, Y., Noguchi, K., Kojima, M., Sakakibara, H., Terashima, I. (2015). Mesophyll conductance decreases in the wild type but not in an ABA-deficient mutant (aba1) of Nicotiana plumbaginifolia under drought conditions. Plant Cell Environ. 38, 388–398. doi: 10.1111/pce.12394
Morales, A., Kaiser, E., Yin, X., Harbinson, J., Molenaar, J., Driever, S. M., et al. (2018). Dynamic modelling of limitations on improving leaf CO2 assimilation under fluctuating irradiance. Plant Cell Environ. 41, 589–604. doi: 10.1111/pce.13119
Ort, D. R., Merchant, S. S., Alric, J., Barkan, A., Blankenship, R. E., Bock, R., et al. (2015). Redesigning photosynthesis to sustainably meet global food and bioenergy demand. Proc. Natl. Acad. Sci. 112, 8529–8536. doi: 10.1073/pnas.1424031112
Oxborough, K., Baker, N. R. (1997). Resolving chlorophyll a fluorescence images of photosynthetic efficiency into photochemical and non-photochemical components - Calculation of qP and Fv’/Fm’ without measuring Fo’. Photosynth. Res. 54, 135–142. doi: 10.1023/A:1005936823310
Papanatsiou, M., Petersen, J., Henderson, L., Wang, Y., Christie, J. M., Blatt, M. R. (2019). Optogenetic manipulation of stomatal kinetics improves carbon assimilation,water use, and growth. Sci. (80-. ). 363, 1456–1459. doi: 10.1126/science.aaw0046
Pearcy, R. W., Krall, J. P., Sassenrath-Cole, G. F. (1996). “Photosynthesis in fluctuating light environments,” in Photosynthesis and the Environment. Ed. Baker, N. R. (Dordrecht, the Netherlands: Kluwer Academic), 321–346. doi: 10.1007/0-306-48135-9_13
Prinsley, R. T., Leegood, R. C. (1986). Factors affecting photosynthetic induction in spinach leaves. Biochim. Biophys. Acta 849, 244–253. doi: 10.1016/0005-2728(86)90031-9
R Core Team. (2020). R: A language and environment for statistical computing. (Vienna, Austria: R Foundation for Statistical Computing). Available at: https://www.R-project.org/.
Raissig, M. T., Matos, J. L., Gil, M. X. A., Kornfeld, A., Bettadapur, A., Abrash, E., et al. (2017). Mobile MUTE specifies subsidiary cells to build physiologically improved grass stomata. Sci. (80-. ). 355, 1215–1218. doi: 10.1126/science.aal3254
Sagi, M., Scazzocchio, C., Fluhr, R. (2002). The absence of molybdenum cofactor sulfuration is the primary cause of the flacca phenotype in tomato plants. Plant J. 31, 305–317. doi: 10.1046/j.1365-313X.2002.01363.x
Sharkey, T. D. (2016). What gas exchange data can tell us about photosynthesis. Plant Cell Environ. 39, 1161–1163. doi: 10.1111/pce.12641
Soleh, M. A., Tanaka, Y., Nomoto, Y., Iwahashi, Y., Nakashima, K., Fukuda, Y., et al. (2016). Factors underlying genotypic differences in the induction of photosynthesis in soybean [Glycine max (L.) Merr.]. Plant Cell Environ. 131, 305–315 .doi: 10.1111/pce.12674
Stitt, M., Grosse, H. (1988). Interactions between sucrose synthesis and CO2 fixation I. Secondary kinetics during photosynthetic induction are related to a delayed activation of sucrose synthesis. J. Plant Physiol. 133, 129–137. doi: 10.1016/S0176-1617(88)80127-5
Stitt, M., Quick, W. P. (1989). Photosynthetic carbon partitioning: its regulation and posibilities for manipulation. Physiol. Plant 77, 633–641. doi: 10.1111/j.1399-3054.1989.tb05402.x
Tal, M., Nevo, Y. (1973). Abnormal stomatal behavior and root resistance, and hormonal imbalance in three wilty mutants of tomato. Biochem. Genet. 8, 291–300. doi: 10.1007/BF00486182
Tal, M. (1966). Abnormal Stomatal Behavior in Wilty Mutants of Tomato. Plant Physiol. 41, 1387–1391. doi: 10.1104/pp.41.8.1387
Tanaka, Y., Adachi, S., Yamori, W. (2019). Natural genetic variation of the photosynthetic induction response to fluctuating light environment. Curr. Opin. Plant Biol. 49, 52–59. doi: 10.1016/j.pbi.2019.04.010
Taylor, S. H., Long, S. P. (2017). Slow induction of photosynthesis on shade to sun transitions in wheat may cost at least 21% of productivity. Philos. Trans. R. Soc B 372, 1–9. doi: 10.1098/rstb.2016.0543
Tinoco-Ojanguren, C., Pearcy, R. W. (1993). Stomatal dynamics and its importance to carbon gain in two rainforest Piper species. II. Stomatal versus biochemical limitations during photosynthetic induction. Oecologia 94, 395–402.
Tomimatsu, H., Tang, Y. (2012). Elevated CO2 differentially affects photosynthetic induction response in two Populus species with different stomatal behavior. Oecologia 169, 869–878. doi: 10.1007/s00442-012-2256-5
Tomimatsu, H., Sakata, T., Fukayama, H., Tang, Y. (2019). Short-term effects of high CO2 accelerate photosynthetic induction in Populus koreana × trichocarpa with always-open stomata regardless of phenotypic changes in high CO2 growth conditions. Tree Physiol. 39, 474–483. doi: 10.1093/treephys/tpy078
Urban, O., Šprtová, M., Košvancová, M., Lichtenthaler, H. K., Marek, M. V. (2008). Comparison of photosynthetic induction and transient limitations during the induction phase in young and mature leaves from three poplar clones. Tree Physiol. 28, 1189–1197. doi: 10.1093/treephys/28.8.1189
Valladares, F., Allen, M. T., Pearcy, R. W. (1997). Photosynthetic responses to dynamic light under field conditions in six tropical rainforest shrubs occuring along a light gradient. Oecologia 111, 505–514. doi: 10.1007/s004420050264
Vialet-chabrand, S. R. M., Matthews, J. S. A., McAusland, L., Blatt, M. R., Griffiths, H., Lawson, T. (2017). Temporal dynamics of stomatal behavior: Modeling and implications for photosynthesis and water use. Plant Physiol. 174, 603–613. doi: 10.1104/pp.17.00125
Vico, G., Manzoni, S., Palmroth, S., Katul, G. (2011). Effects of stomatal delays on the economics of leaf gas exchange under intermittent light regimes. New Phytol. 192, 640–652. doi: 10.1111/j.1469-8137.2011.03847.x
Way, D. A., Pearcy, R. W. (2012). Sunflecks in trees and forests: from photosynthetic physiology to global change biology. Tree Physiol. 32, 1066–1081. doi: 10.1093/treephys/tps064
Woodrow, I. E., Mott, K. A. (1989). Rate limitation of non-steady-state photosynthesis by Ribulose-1,5-bisphosphate Carboxylase in spinach. Aust. J. Plant Physiol. 16, 487–500. doi: 10.1071/PP9890487
Yamori, W., Kusumi, K., Iba, K., Terashima, I. (2020). Increased stomatal conductance induces rapid changes to photosynthetic rate in response to naturally fluctuating light conditions in rice. Plant Cell Environ. 43, 1230–1240. doi: 10.1111/pce.13725
Yin, X., Struik, P. C., Romero, P., Harbinson, J., Evers, J. B., Van Der Putten, P. E. L., et al. (2009). Using combined measurements of gas exchange and chlorophyll fluorescence to estimate parameters of a biochemical C3 photosynthesis model: a critical appraisal and a new integrated approach applied to leaves in a wheat (Triticum aestivum) canopy. Plant Cell Environ. 32, 448–464. doi: 10.1111/j.1365-3040.2009.01934.x
Zhang, Y., Kaiser, E., Zhang, Y., Yang, Q., Li, T. (2018). Short-term salt stress strongly affects dynamic photosynthesis, but not steady-state photosynthesis, in tomato (Solanum lycopersicum). Environ. Exp. Bot. 149, 109–119. doi: 10.1016/j.envexpbot.2018.02.014
Keywords: abscisic acid, air humidity, CO2 concentration, fluctuating irradiance, dynamic photosynthesis, stomatal conductance
Citation: Kaiser E, Morales A, Harbinson J, Heuvelink E and Marcelis LFM (2020) High Stomatal Conductance in the Tomato Flacca Mutant Allows for Faster Photosynthetic Induction. Front. Plant Sci. 11:1317. doi: 10.3389/fpls.2020.01317
Received: 07 June 2020; Accepted: 11 August 2020;
Published: 25 August 2020.
Edited by:
Diana Santelia, ETH Zürich, SwitzerlandReviewed by:
Christine Helen Foyer, University of Birmingham, United KingdomThomas D. Sharkey, Michigan State University, United States
Copyright © 2020 Kaiser, Morales, Harbinson, Heuvelink and Marcelis. This is an open-access article distributed under the terms of the Creative Commons Attribution License (CC BY). The use, distribution or reproduction in other forums is permitted, provided the original author(s) and the copyright owner(s) are credited and that the original publication in this journal is cited, in accordance with accepted academic practice. No use, distribution or reproduction is permitted which does not comply with these terms.
*Correspondence: Elias Kaiser, ZWxpYXMua2Fpc2VyQHd1ci5ubA==
†Present address: Jeremy Harbinson, Laboratory of Biophysics, Wageningen University, Wageningen, Netherlands