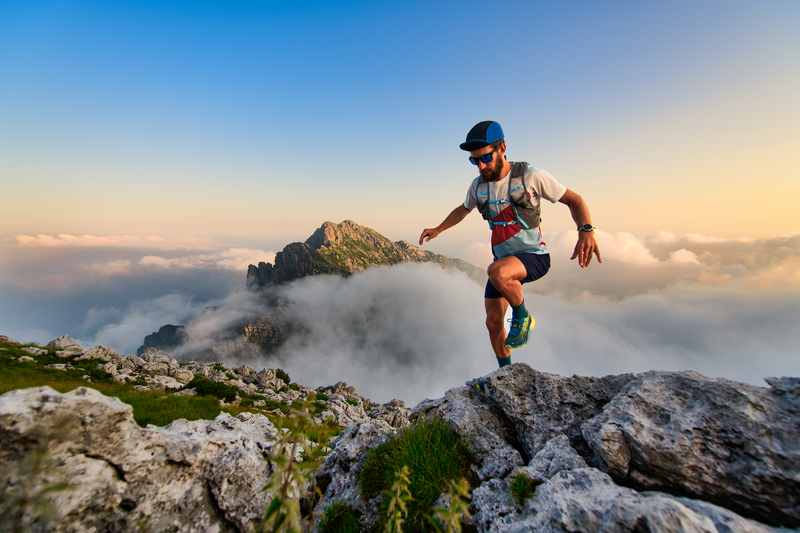
94% of researchers rate our articles as excellent or good
Learn more about the work of our research integrity team to safeguard the quality of each article we publish.
Find out more
METHODS article
Front. Plant Sci. , 04 September 2020
Sec. Technical Advances in Plant Science
Volume 11 - 2020 | https://doi.org/10.3389/fpls.2020.01302
A correction has been applied to this article in:
Corrigendum: Membrane Inlet Mass Spectrometry: A Powerful Tool for Algal Research
Since the first great oxygenation event, photosynthetic microorganisms have continuously shaped the Earth’s atmosphere. Studying biological mechanisms involved in the interaction between microalgae and cyanobacteria with the Earth’s atmosphere requires the monitoring of gas exchange. Membrane inlet mass spectrometry (MIMS) has been developed in the early 1960s to study gas exchange mechanisms of photosynthetic cells. It has since played an important role in investigating various cellular processes that involve gaseous compounds (O2, CO2, NO, or H2) and in characterizing enzymatic activities in vitro or in vivo. With the development of affordable mass spectrometers, MIMS is gaining wide popularity and is now used by an increasing number of laboratories. However, it still requires an important theory and practical considerations to be used. Here, we provide a practical guide describing the current technical basis of a MIMS setup and the general principles of data processing. We further review how MIMS can be used to study various aspects of algal research and discuss how MIMS will be useful in addressing future scientific challenges.
Photosynthetic microoganisms are major actors shaping the Earth’s atmosphere and limiting global warming by fixing CO2. We hereby describe practical and theoretical state of the art of the most polyvalent technique to measure gas exchange in microorganisms.
Since its formation, the Earth’s atmosphere has continuously been shaped by living organisms. Among all biological processes, oxygenic photosynthesis has dramatically changed the atmosphere composition by massively capturing CO2 and producing O2 during the Great Oxygenation Event that began 2.4 billion years ago with the emergence of cyanobacteria (Hohmann-Marriott and Blankenship, 2011). Nowadays, microalgae and cyanobacteria account for more than 50% of global photosynthesis (Field et al., 1998) and have a great importance in the regulation of atmospheric CO2 levels and global warming. Understanding biological mechanisms underlying CO2 capture or production of other greenhouse gases such as nitrous oxide (N2O) by microbial photosynthesis is of utmost relevance to better assess the impact of global changes on oceanic carbon sinks. It is also crucial to explore the limits of biomass productivity of algae and give some hints to assess the impact of microalgae-based biofuels on the environment (Burlacot et al., 2020a).
In the early 60s, Hoch and Kok (1963) designed a membrane inlet system coupled to a mass spectrometer (MIMS), which allowed the direct measurement of concentrations of dissolved CO2 and O2 in a microalgal suspension. In the MIMS setup they developed, a thin plastic membrane (polyethylene or Teflon) permeable to gases allowed part of the dissolved gases to pass from the microalgal suspension to the mass spectrometer. Coupled to the use of 18O-labeled O2, MIMS allowed in situ measurement of O2 uptake processes occurring during microalgal and cyanobacterial photosynthesis (Hoch and Kok, 1963; Gerster et al., 1974; Radmer and Kok, 1976; Gerster et al., 1977; Radmer and Ollinger, 1980b; Peltier and Thibault, 1985a; Peltier and Thibault, 1985b). The use of isotope-labeled water has also been an important tool for the study of water oxidation mechanisms by photosystem II (PSII) (Radmer and Ollinger, 1980a; Radmer and Ollinger, 1986; Shevela et al., 2011; Koroidov et al., 2014), which is not reviewed here and readers are referred to a recent review (Shevela and Messinger, 2013).
Later on, MIMS has been used to monitor various biological gases in the context of: i, inorganic carbon transport (Badger et al., 1994; Yu et al., 1994; Sültemeyer et al., 1998); ii, hydrogen production (van der Oost and Cox, 1988; Redding et al., 1999; Cournac et al., 2002; Tamburic et al., 2011); or iii, ethylene production (Zavřel et al., 2016). With the development of genetics tools, MIMS has been widely used to characterize various microalgae or cyanobacteria mutants and contributed to the understanding of molecular mechanisms involved in photosynthetic gas exchange (So et al., 1998; Hanson et al., 2003; Helman et al., 2003; Cournac et al., 2004; Jans et al., 2008). It has also been used for in vitro studies of enzymes using gases as a substrate or products, like hydrogenases (Leroux et al., 2008), carbonic anhydrases (Northrop and Simpson, 1998) and the Fatty Acid Photodecarboxylase (Sorigué et al., 2017). In addition, it has also been used to study artificial catalysts for water oxidation (Poulsen et al., 2005; Koroidov et al., 2015) and to follow biogeochemical cycles in the oceans (Chua et al., 2016). Taken together, MIMS has made significant contributions to the fields of photosynthesis research, enzymology, biofuel research, and earth science which was recently reviewed (Burlacot et al., 2020a).
MIMS is nowadays a mature technique implicated in a growing number of applications in the field of algal research. With mass spectrometers being more affordable in the past years, MIMS is increasingly available in many laboratories. However, mounting and running a MIMS setup is technically and theoretically challenging. Previous reviews have focused on some specific aspects of MIMS in the context of photosynthesis research (Degn, 1992; Kotiaho and Lauritsen, 2002; Konermann et al., 2008; Beckmann et al., 2009; Shevela and Messinger, 2013; Cheah et al., 2014; Shevela et al., 2018). The current review intends to provide a guide covering theoretical, technical, and data processing aspects required for a broad usage of MIMS in algal research.
A typical MIMS setup is composed of five parts (Figure 1): (i), a reaction vessel containing the liquid medium/algal culture where gas exchange reactions take place; (ii), a membrane separating the liquid phase of the reaction vessel from the high vacuum line; (iii), a vacuum line connecting the reaction vessel to the mass spectrometer; (iv), a cold trap protecting the mass spectrometer from water leakage; and (v), a mass spectrometer for gas analysis. MIMS allows measurement of any volatile compound, with a molar mass up to a hundred gram per mole, dissolved in liquid phase (Kotiaho and Lauritsen, 2002). MIMS operation requires proper material setup and appropriate data processing. Each of the technical parts needs to be optimized to ensure reliable and stable measurement.
Figure 1 Schematic setup of a Membrane Inlet Mass Spectrometry. The biological sample (cell suspension, cell free extract, or enzyme containing medium) is introduced inside a thermo-regulated reaction vessel equipped with a gas permeable membrane. Dissolved gases contained in the liquid medium pass through the membrane to a high vacuum tubing. After passing through a cold trap protecting the mass spectrometer from water leaks, gases enter the ion source of a mass spectrometer. Upon ionization, charged molecules are accelerated by an electric field (E), deviated by a magnetic field (B), and collected by a detector. An auxiliary vacuum pump allows for making the vacuum on the line with the closed inlet to the mass spectrometer. B, magnetic field; E, electric field.
The membrane is gas permeable and serves as a physical barrier between the liquid phase and the ion source of the mass spectrometer connected by a vacuum line. In the setup described in Figure 1 the membrane is held at the bottom of the reaction vessel supported by a stainless-steel grid. Note that different membrane inlet setups like microprobes can be used (Kotiaho and Lauritsen, 2002; Konermann et al., 2008). The gas leak through the membrane occurs through a three-step process called permeation: adsorption at the membrane surface, diffusion through the membrane material, and desorption on the other side of the membrane. In this process, diffusion is the limiting factor (Konermann et al., 2008) and is a temperature-dependent process. Thus, membrane permeability is highly influenced by temperature (Figure 2), and the reaction vessel must be precisely thermo-regulated (Figure 1). The gas leak is crucial since it defines the sensitivity of the method, especially it should remain small compared to the measured reaction rates to limit noise. Gas leakage depends on the membrane properties and is linearly related to the gas concentration inside the vessel (Methods S1). Various porous plastic films can be used as a membrane, although polytetrafluoroethylene (PTFE, also known as Teflon) and silicon are the most commonly used (Beckmann et al., 2009; Shevela et al., 2018). The permeability to gases depends on the nature of the membrane. Silicon membranes are more permeable to gases than PTFE membranes (Lloyd et al., 1983) (PTFE membranes can be found on www.hansatech-instruments.com/ or www.ysi.com). The gas leak depends on both membrane surface and thickness, which must be chosen depending on the required sensitivity and on the duration of the experiments to be carried out. The ion source is overly sensitive to water that interferes with ionization and therefore quantification. In this respect, a PTFE membrane has the advantage over silicone of being seven times less permeable to water relatively to N2 (Jensen and Cox, 1988), resulting in less noise. A magnetic stirrer is used to maintain algae in suspension and homogenize the gas content of the liquid medium (Lundsgaard et al., 1978). In a case where the stirring occurs on the membrane, the stirring speed must be adjusted to ensure a proper homogenization without compromising membrane integrity. Likewise, a smooth PTFE coated stirring bar can be used to achieve optimal stirring while limiting membrane wear.
Figure 2 The influence of temperature on the MIMS signal strength illustrated for N2, O2, and CO2. Reaction vessel is filled with C. reinhardtii minimal growing medium buffered at pH = 7.2 using 3-(N-morpholino)propane sulfonic acid (MOPS, 20 mM final concentration) and is flushed with air until reaching steady state. A PTFE membrane (13 µm) is used. To avoid solubility issues, the signal shown is normalized by the Bunsen coefficient (see Methods 1). The mass spectrometer signal is given in amperes (A).
The vacuum line connecting the space below the membrane to the ion source of the mass spectrometer is, together with the membrane, a key element to consider when optimizing the response time of the experimental setup. Because of high vacuum (10−5–10−8 mbar) in the tubing, gases do not flow like a fluid but perform a random walk inside the tube towards the ion source of the mass spectrometer where gases are ionized. During their random walk, gases can adsorb and desorb at the surface of the tubing, thus slowing down the gas flow. The tubing length and cross-section should be optimized to increase the time response without compromising output signal of the mass spectrometer. Limiting length of the tubing decreases the response time of the system both because of limited inner tubing volume and decreased surface adsorption effects of the tubing. On the other hand, while small tubing cross-section favors the time response by limiting the tubing volume, this effect is counter-balanced by an increased probability of molecule absorption on the tubing surface, a too small cross-section decreasing the signal/noise ratio of the setup. We found an inner diameter of ¼ inch to be a good compromise for the type of experiments shown in this paper although smaller tubing down to inch can be suitably used (Konermann et al., 2008).
To limit water vapor entering the mass spectrometer ion source, the vacuum line tubing passes through a cold trap where the water vapor is condensed. Since the cold trap can also condense other gases, the choice of the trap temperature depends on the gas species to be analyzed. For example, cooling the vacuum line with liquid nitrogen (77 K) allows efficiently trapping water but also CO2 (Figure 3). The condensation points of other main gases [N2, O2, and argon (Ar)] are much lower than that of water and are usually hard to selectively trap (Figure 3). A temperature of 200 K allows selective trapping of water and can be obtained using a mix of ethanol and dry ice in the absence of a cooling unit (Bailleul et al., 2017). The cold trap should be situated as close as possible to the vacuum pump of the mass spectrometer in order to keep its internal pressure as low as possible and limit unintended trapping. For further information on optimization of the membrane inlet system, readers are referred to (Konermann et al., 2008; Beckmann et al., 2009; Shevela et al., 2018).
Figure 3 Phase diagram of some gas components of the Earth’s atmosphere. Shown are graphical views of the vapor–liquid and vapor–solid border H2O, N2, O2, Ar, CO2, N2O, and NO. Dots represent triple points. Letters are placed on the side of the phase diagram, representing gas phase (G), solid phase (S), and liquid phase (L). Shown are data from the gas encyclopedia, Air Liquide, 1976 more data can be found at https://encyclopedia.airliquide.com/.
Mass spectrometry is a technique that allows separation and detection of charged molecules in a gas phase depending on their mass over charge (m/z) ratio. During ionization, most reactions produce a single positively charged ion. But most often, the molecule is broken down by ionization, and seldom, double ionization can occur; these effects give rise to two or more fragments. The fragmentation pattern is a signature of the molecule and depends on the ionization method used. In the case of small molecules like gases, the main ion (molecular ion) results from the loss of an electron, other fragments representing a minor part in the fragmentation pattern (Table 1). The mass spectrometer, by measuring the signal intensity of detected ions for m/z of interest, enables the determination of gas amounts (Methods S1). Any mass spectrometer can be used in a MIMS setup with various ion sources (e.g. pulsed ionization, glow discharged ionization) and analyzers (e.g. Time Of Flight, Ion Trap) (Johnson et al., 2000). However, magnetic sector or quadrupole mass spectrometers are the most commonly used for gas analysis in a MIMS setup, although quadrupole has the advantage of portability and low price (Ferrón et al., 2016; Bailleul et al., 2017; Chatton et al., 2017).
Because the mass spectrometer is consuming gases, measured variations of gas concentrations must be corrected from the mass spectrometer consumption to determine actual gas exchange rates between the biological sample and the extracellular medium (Methods S2). Although this effect has been well described (Berlier et al., 1985; Kotiaho and Lauritsen, 2002; Konermann et al., 2008) its correction has been overlooked in the recent literature. After calculating gas concentrations inside the reaction vessel, gas exchange rates are calculated by correcting from the mass spectrometer consumption. Fluctuations of physical properties of the setup can result in variations of the flux of gas to the mass spectrometer and be an important source of noise. An additional normalization of gas exchange rates to a non-reactive gas (like N2 or Ar) can advantageously correct such signal shifts or noise. These artifacts are therefore highly limited although biggest ones remain (Figure S2). Note that prior to any experiment, one must ensure the absence of gas leakage in the system (tubing, connections, inlets), which can be done by flushing helium outside the setup and following the m/z = 4 with the mass spectrometer. Details of the calculations have been described in Methods S1 and S2, and we have developed easy-to-run software that allows real time calculation and visualization of MIMS data (Downloadable at: https://github.com/francoisBurlacot/MIMS_Analysis).
MIMS has been initially developed and is still widely used to measure oxygen exchange during photosynthesis. Its usage has been extended to the study of other cellular mechanisms, such as hydrogen production, carbon concentrating mechanisms, and more recently, nitric oxide (NO) photoreduction into N2O. We hereby provide some examples of applications of the MIMS in the field of algal biology to measure gas exchanges in the model species Chlamydomonas reinhardtii (Figure 4).
Figure 4 Schematic view of major reactions involving gas exchange in microalgae illustrated for C. reinhardtii. Arrows indicate the subcellular localization of the different reactions. For each reaction, gas species that can be measured by MIMS are in red. ADP, adenosine diphosphate; acyl-CoA, acyl-Coenzyme A; e−, reducing equivalent; trans-2-enoyl-CoA, trans-2,3-dehydroacyl-Coenzyme A.
By using 18O-enriched O2 in illuminated microalgal suspension, Hoch and Kok (Hoch and Kok, 1963) could show that O2 can be both produced and consumed during photosynthesis. While O2 is produced by PSII from water splitting, O2 is simultaneously consumed by different cellular processes (Figures 4 and 5A). Practically, the use of highly enriched O2 (usually around 99% 18O) allows neglecting 18O16O species, thus measurements of 16O16O (m/z = 32) and 18O18O (m/z = 36) are used to determine rates of gross O2 evolution (O2 Evolution) and O2 uptake (O2 Uptake), net O2 production rate (Net O2) being the end result of O2 Evolution and Uptake. Considering that water splitting only produces 16O2 (the natural abundance of 16O being 99.8%, O2 is produced from H2O at 99.6% as 16O2) and neglecting isotopic discrimination between 18O2 and 16O2 by uptake mechanisms, the following equations modified from (Radmer and Kok, 1976; Peltier and Thibault, 1985b) can be used:
Figure 5 In vivo measurements of photosynthetic O2 exchange in the presence of 18O-labeled O2. (A). Schematic view of oxygen exchange illustrated in C. reinhardtii. While photosystem II (PSII) produces unlabeled O2 from the photolysis of H2O, oxygen uptake mechanisms consume both 18O-labeled and unlabeled O2 (B) 16O2 and 18O2 concentrations measured in C. reinhardtii cells during dark–light transients. (C, D). Calculated cumulated O2 exchanges (C) and the corresponding O2 exchange rates (D) for the same experiment. Cells were grown photoautotrophically in air, centrifuged and resuspended in fresh medium at a concentration of 20 µg Chl ml−1. Upon addition of 5mM HCO3−, 18O2 was injected inside the cell suspension, and the reaction vessel was closed. After 5 min of dark adaptation, green light was turned on (500 µmol photon m−2 s−1) for 10 min. Levels of 16O2 and 18O2 were recorded at respective m/z = 32 and 36. O2 Uptake (red), O2 Evolution (blue), and Net O2 production (black) were calculated as described; cumulated gas exchange were calculated by directly integrating obtained exchange rates. To limit noise on the exchange rates graphic, data shown in (D) are integrated with a sliding average of 30 s wide.
where and are the gas exchange rates of 18O2 and 16O2 respectively; and represent the gas concentrations of 18O2 and 16O2 respectively (see Methods S1 and S2). Typical patterns of 18O2 and 16O2 concentration, gross O2 evolution, O2 uptake, and O2 net production rates as well as cumulated O2 exchanges measured in C. reinhardtii cells during a dark to light transient are shown in Figures 5B–D.
Measuring O2 exchange by MIMS in microalgae and cyanobacteria allowed dissecting molecular players involved in the O2 uptake process, initially by using various inhibitors or characterizing the dependency on O2 or CO2 concentrations (Badger et al., 2000). (Radmer and Kok, 1976) early proposed that a highly efficient O2 photoreduction process was present in cyanobacteria and microalgae. The persistence of mitochondrial respiration in the light was shown to contribute (Peltier and Thibault, 1985b), and the existence of a CO2-dependent O2 uptake component was evidenced (Badger, 1985; Sültemeyer et al., 1987). With the development of genetic approaches, the nature and contribution of the different players were better characterized. For example, the contribution of mitochondrial respiration to O2 uptake is enhanced in the absence of the proton gradient regulation like 1 (PGRL1)-mediated cyclic electron flow (CEF), thus showing the functional complementarity between these pathways in the production of intra-cellular ATP (Dang et al., 2014). Redox communication between chloroplast and peroxisome (Kong et al., 2018) or with mitochondria in diatoms (Bailleul et al., 2015) was also evidenced from O2 exchange measurements. The involvement of Flavodiiron proteins (Flvs) in light-dependent O2 uptake was further established in cyanobacteria (Helman et al., 2003; Allahverdiyeva et al., 2013), and more recently in microalgae (Chaux et al., 2017). MIMS is nowadays widely used to understand the fate of the photosynthetic electron flow in various environmental conditions and mutant of cyanobacteria (Ermakova et al., 2016; Boatman et al., 2018; Luimstra et al., 2019), microalgae (Fisher and Halsey, 2016), or coral reef symbiosis (Einbinder et al., 2016).
If laboratory studies performed on model species allowed recognizing O2 photoreduction as a major alternative photosynthetic electron sink crucial for cell acclimation to various environmental conditions (Curien et al., 2016), the relative contribution of different O2 uptake mechanisms in natural environments remains largely unexplored (Bailleul et al., 2017). Despite its performance in measuring gross photosynthesis, MIMS has long remained a cumbersome technique, not suitable for field experiments. On the other hand, chlorophyll fluorescence measurements using pulse-amplitude modulated (PAM) fluorimeter have been widely used for estimating PSII yield in natural environments such as in the ocean (Falkowski and Kolber, 1995). Chlorophyll fluorescence however, faces some limitations when used to determine gross O2 production since the estimation of electron transport rates requires the measurement of cell absorbance (Genty et al., 1989; Godaux et al., 2015), which is difficult to realize in outdoor conditions. Recently, the miniaturization of mass spectrometers allowed in situ measurements of O2 exchange in phytoplankton from the north Pacific (Ferrón et al., 2016) or in planktonic blooms from the north Atlantic (Bailleul et al., 2017), starting thus a new era for expanding research obtained in the laboratory on model species to species in their natural environments.
Indeed, fluorescence measurements have often been used together with MIMS CO2 and O2 exchange measurements to study the link between CO2 fixation and non-photochemical quenching of chlorophyll fluorescence (Sültemeyer et al., 1989; Fratamico et al., 2016; Ware et al., 2020). Furthermore, in conditions where the use of 18O2 is not possible (e.g. anaerobiosis), coupling PSII quantum yield with gas exchanges has recently allowed determining gross O2 production and inferring the existence of an O2 uptake process (Burlacot et al., 2018).
In microalgae and cyanobacteria, hydrogenases (H2ases) catalyze the reversible formation of hydrogen (H2) by direct reduction of protons (H+). In microalgae, the electron donor to the [Fe–Fe] H2ase is ferredoxin (Florin et al., 2001), which can be reduced by the photosynthetic electron transport chain or by fermentative pathways (Catalanotti et al., 2013). Experimentally, H2 production can be measured by different techniques, including modified O2 electrodes (Wang, 1980; Godaux et al., 2015), gas chromatography (Hunt and Smith, 1961; Nagy et al., 2018), nuclear magnetic resonance spectroscopy (NMR) (Xu et al., 2016; Manz et al., 2017), or MIMS. Among these techniques, MIMS and modified O2 electrodes allow in vivo quantitative measurement of hydrogen in a time-resolved manner. Under natural conditions, hydrogen photoproduction by microalgae is a transient phenomenon, generally considered as a safety valve avoiding over-reduction of PSI electron acceptors under anaerobiosis (Ghysels et al., 2013). Hydrogen photoproduction is limited by the O2 sensitivity of H2ase, O2 being produced by PSII during illumination (Erbes et al., 1979). When using experimental conditions maintaining anaerobic conditions (thus limiting the H2ase inhibition) like sulfur deprivation (Melis et al., 2000), low illumination (Liran et al., 2016) or O2 quenchers like glucose and glucose oxidase/catalase (Godaux et al., 2015), a limitation of the supply of electrons to the H2ase can be evidenced. The use of MIMS and of various C. reinhardtii mutants allowed identifying biological bottlenecks limiting the supply of electrons to the H2ase (Tóth and Yacoby, 2019; Burlacot et al., 2020a). Lately, MIMS was used in the development of a very promising H2 photoproducing protocol using flashing light as the light source in photoautotrophic self-anaerobic conditions (Kosourov et al., 2018).
MIMS has early been used for in vitro and in vivo measurements of H2ase activity (Jouanneau et al., 1980; Vignais et al., 1982; Berlier et al., 1985). In the presence of H2, H2ase spontaneously splits H2 (Hoberman and Rittenberg, 1943; Rittenberg and Krasna, 1955), forming one proton with the reversible reaction:
where Hyd is the binding site of H2ase. When supplying deuterium (D2), HD is formed during the back reaction (6) in the presence of protons in the reaction mixture:
This reaction directly depends on the turnover rate of H2ases (i.e. H2ase activity) (Vignais, 2005). In the absence of H2 production or uptake, following kinetics of D2, HD and H2 with MIMS allows measuring the H2ase activity (H2ase activity) by the H/D exchange rate (Vexch):
with
where , and are the concentrations of D2, H2 and HD respectively and , being the gas exchange rates of H2 and HD, respectively (see Methods S1 and S2) (Cournac et al., 2004). A few microalgal species such as C. reinhardtii harbor H2ases (Burlacot and Peltier, 2018) under anaerobic conditions. Measuring the H/D exchange allowed monitoring of H2ase induction or inhibition in vivo (Vignais et al., 2002; Tolleter et al., 2011; Burlacot et al., 2018). Typical patterns of D2, H2 and HD exchange measured upon injection of D2 before and after induction of H2ase in C. reinhardtii are shown on Figure 6. Note that in conditions where H2ase produces H2 during illumination the H2ase activity, needs to be corrected from the increase in total hydrogen species (H2, HD, D2) (Cournac et al., 2004). Although gas chromatography or NMR has also the potential to differentiate D2, HD and H2 (Hunt and Smith, 1961; Xu et al., 2016), MIMS allows performing such measurements in vitro and in vivo in a time resolved manner. If H/D exchange measurements using a MIMS allow determining the catalytic constant of H2ase, it can also be used to determine the resistance of gas diffusion between the active H2ase site and the reaction medium in vitro (Leroux et al., 2008). In vitro H/D exchange measurements have been used to study enzymatic properties of native H2ase (Abou Hamdan et al., 2012; Gauquelin et al., 2018), including O2-tolerant H2ases (Liebgott et al., 2011), as well as H2ases modified by site-directed mutagenesis in order to limit O2 diffusion to the active site (Cano et al., 2014). H2 photoproduction by microorganisms has recently regained huge interest for biofuel production due to recent improvements in strains and experimental protocols (Tóth and Yacoby, 2019). The use of MIMS should help in evaluating the upcoming combination of newly developed H2 production protocols (Kosourov et al., 2018; Nagy et al., 2018) and previously characterized mutants photo-producing more H2 (Tolleter et al., 2011; Eilenberg et al., 2016; Burlacot et al., 2018; Ben-Zvi et al., 2019). This has recently started using flv mutants (Jokel et al., 2019) and is promising for future bio H2 production developments.
Figure 6 In vivo measurement of the hydrogenase activity by H/D exchange (A). Principle of H/D exchange. In the presence of labeled hydrogen (D2), the labeled deuterium (D+) is exchanged with protons (H+) at the catalytic site of H2ase (-Hyd). (B). Cumulated gas exchange of D2, HD, and H2 in wild type cells after 1 h anaerobiosis with induced hydrogenase. (C). Cumulated gas exchange of D2, HD, and H2 in wild type cells after 1 min of anaerobiosis without induction of hydrogenase. For (B, C), cell suspension of C. reinhardtii was maintained in anaerobiosis for 1 min (B) or 1 h (C) before t = 0. At t = 1 min (black arrow), D2 was bubbled for a few seconds before the reaction vessel was closed and H/D exchange recorded.
On Earth, 6% of the radiative forcing is due to N2O (IPCC, 2013), whose greenhouse effect is 300 times that of CO2. N2O is produced from the reduction of nitric oxide (NO) by bacteria (Goreau et al., 1980), fungi (Maeda et al., 2015), and microalgae (Guieysse et al., 2013). The measurement of N2O in a time resolved manner can help understand the dynamics of N2O formation in the environment or in isolated organisms. In green microalgae, MIMS has recently been used to dissect the molecular mechanisms involved in the conversion of NO into N2O (Figure 7) (Burlacot et al., 2020b). In practical terms, the detection of N2O requires specific calculations because its mass spectrum overlaps that of CO2 (see Methods S1). However, due to a relatively high detection limit for N2O (around 18 µM) (Chatton et al., 2017), MIMS is not suitable for detecting low N2O amounts, such as in water in equilibrium with ambient air (4.3 µM) (IPCC, 2013).
Figure 7 In vivo measurement of NO reduction into N2O. (A). Schematic N2O production mechanisms illustrated in C. reinhardtii. Nitric oxide (NO) is reduced in the chloroplast both in a light dependent and independent manner (Burlacot et al., 2020b). (B). Cumulated gas exchange of NO and N2O in C. reinhardtii cells grown autotrophically (100 µg chlorophyll. ml−1) during a dark to light transition. Glucose oxidase/catalase and glucose are added to the algal suspension to reach anaerobiosis. After injection of a NO-saturated water solution in the cell suspension (black arrow), NO and N2O exchange is measured as described in (Burlacot et al., 2020b).
NO is an important intracellular signaling molecule in algae like in most living organisms. While NO is crucial for growth of C. reinhardtii under anaerobiosis and for its acclimation to nitrogen, sulfur or phosphate deprivation (Hemschemeier et al., 2013; Wang and Spalding, 2014; De Mia et al., 2019; Filina et al., 2019), mechanisms of NO production remain blurry. The use of MIMS in algae fed with nitrates or nitrites, allowed to evidence the role of NO reduction mechanisms in the regulation of NO homeostasis (Burlacot et al., 2020b). Following NO production can also be based on imaging fluorescent chemical probes specifically reacting with NO (Li and Wan, 2015) or on the use of specific electrodes (Csonka et al., 2015). However, MIMS by supplying quantitative, time resolved, and stable measurements of NO (Bethke et al., 2004; Conrath et al., 2004), with further simultaneous measurement of NO reduction products such as N2O, should help deciphering mechanisms participating in NO homeostasis.
When grown under low CO2 concentration, many microalgae or cyanobacteria induce an active import of inorganic carbon (Ci = CO2 and HCO3−) (Figure 8A) from the extracellular medium to the active site of CO2 fixation (Badger et al., 1980; Badger and Andrews, 1982; Sültemeyer et al, 1991; Giordano et al., 2005; Reinfelder, 2011). This mechanism, called Carbon Concentrating Mechanism (CCM), principally operates by concentrating HCO3− inside cells (Price et al., 2007). In microalgae, HCO3− is converted into CO2 inside the pyrenoid, at the vicinity of the carbon-fixing enzyme (RuBisCO) thus increasing the local CO2 concentration (Figure 8A) (Mackinder, 2018). The CCM ensures high Ci fixation rates by photosynthesis under low Ci concentration (Badger et al., 1980). When active, the CCM results in an increased apparent affinity of photosynthesis for Ci, which can be assessed by measuring O2 production rates at various Ci concentrations, either using an O2 electrode (Badger et al., 1980) or a MIMS (Sültemeyer et al., 1993). MIMS has the advantage of simultaneously measuring O2 and CO2. Since the CO2 decreases during the time course of the experiment due to the activity of photosynthesis, it is possible to determine in one single experiment net O2 or CO2 exchange rates at different CO2 concentrations ( or ) during CO2 fixation by photosynthesis (Douchi et al., 2019). Figures 8B, C show typical experiments in which CO2 uptake and O2 production rates have been determined as a function of the CO2 concentration in air-grown and CO2-grown cells of C. reinhardtii. Note that in such experiments the cell concentration must be kept low enough to ensure that photosynthetic gas exchange kinetics are sufficiently slow as compared to the response time of the MIMS setup. Using this technique (Figure 8), the apparent affinity for Ci of photosynthetic O2 production of cells with an active CCM is about 10 times higher than in cells with no active CCM as previously reported (Badger et al., 1980; Sültemeyer et al., 1998).
Figure 8 In vivo measurement of the apparent affinity of photosynthesis for CO2 during the induction of the Carbon Concentrating Mechanisms. (A). Schematic view of the carbon transport mechanism in C. reinhardtii. In the presence of a CCM, inorganic carbon (Ci) is actively transported, thus increasing the CO2 concentration at the CO2 fixation site. (B). Ci uptake rates measured at different Ci concentrations during its depletion in C. reinhardtii cells grown in 2% CO2 in air (in red) or in air levels of CO2 (in black). (C). Net O2 production rates depending on Ci during the same experiments as in (B). C. reinhardtii cells were grown either at air level of CO2 or 2% CO2, growing medium; cell sampling was the same as described in Figure 2. After sampling and resuspention in fresh medium, green saturating light (3,000 µmol photon m−2 s−1) was turned on, and O2 production and Ci uptake were recorded during the depletion of Ci. With this technique, the apparent affinity for Ci of photosynthesis measured by net O2 production in cells with an active CCM (K1/2 = 5 µM) is 10 times higher than the one measured when the CCM is not induced (K1/2 = 50 µM).
During the induction of CCM, carbonic anhydrases (CAs) are also induced and catalyze the reversible hydration of CO2 by water which is summarized in reaction (8):
In microalgae, different CA isoforms are present in the different cellular compartments, thus limiting the disequilibrium between transported and consumed Ci species (Figure 4) (Sültemeyer et al., 1990; Mackinder et al., 2017). Inside the pyrenoid, CA rapidly converts HCO3− into CO2, the substrate of RuBisCO, thus ensuring a high CO2 concentration at the catalytic site of the enzyme (Mackinder, 2018).
When their CCM is active, cyanobacteria and microalgae can take both CO2 and HCO3− in the medium (Sültemeyer et al., 1991; Badger et al., 1994). A way to assess the net flux of both species is to use the disequilibrium between inorganic species (see equation 8) induced by the preferential uptake of one species. Note that this approach is not possible in the presence of extracellular CA, which has limited its usage in microalgae (Badger et al., 1994; Palmqvist et al., 1994). By comparing net O2 production (proportional to the overall net Ci uptake) to the net CO2 uptake and knowing the uncatalyzed rate of CO2 and HCO3− interconversion (8), it is possible to calculate the net HCO3− uptake (Badger et al., 1994). Using the disequilibrium method with MIMS has shown that HCO3− was preferentially taken during CCM in cyanobacteria (Sültemeyer et al., 1998).
In the presence of doubly 18O-labeled CO2, the CA activity which catalyzes the exchange of oxygen isotopes between CO2 and H2O results in a progressive dilution of 18O from CO2 (Gerster, 1971) (Figure 9A). To limit the background level of m/z = 44 (12CO2) due to naturally present CO2 in the algal suspension, the assay can be done using 18O-enriched 13CO2 (Radmer and Kok, 1976), following the m/z = 45 (13C16O2), 47 (13C18O16O) and 49 (13C18O2). After injection of 13C18O2 (supplied as H13C18O3− in a buffered reaction medium) a typical pattern of the progressive unlabeling of 13CO2 in C. reinhardtii cells is shown (Figures 9B–E). The isotopic content of 18O in 13CO2 during the progressive unlabeling is given by:
where and are the concentrations of 13C18O2, 13C18O16O and 13C16O2 respectively (Methods 1). On purified CA, the isotopic enrichment decays exponentially:
where θ is the rate constant of the exchange of 18O with water (Gerster, 1971; Silverman, 1982). Note that in some cases like a concomitant use of 18O-labeled O2, the presence of 18O and C16O2 in the ion source of the mass spectrometer can spontaneously generate C18O16O which needs to be corrected (Cournac et al., 1993). In vivo, a CA isotope exchange assay measures the global contribution of all CAs present in the biological sample with an additional effect due to diffusion/transport of Ci through the membranes (Sültemeyer and Rinast, 1996; Tolleter et al., 2017). When a periplasmic CA is present, as it is the case in C. reinhardtii cells with an active CCM, its activity dominates the exchange kinetics (Sültemeyer and Rinast, 1996). MIMS is so far the most reliable method for CA activity measurements in biological samples and is particularly suitable for in vivo measurements on algae (Dang et al., 2014; Benlloch et al., 2015; Tolleter et al., 2017), cyanobacteria (Whitehead et al., 2014), corals (Tansik et al., 2015), and plants (Peltier et al., 1995; Clausen et al., 2005; Burén et al., 2011; Benlloch et al., 2015). In vitro, the use MIMS and H13CO3− has also allowed unraveling the existence of a light-induced CO2 production by the PSII (Koroidov et al., 2014; Shevela et al., 2020).
Figure 9 In vivo measurement of carbonic anhydrase (CA) activity. (A). Cascade of reactions leading to the unlabeling of 18O-enriched CO2 in solution in H2O. (B, C). Concentrations of 13C16O2, 13C18O16O, 13C18O2 and total 13C-labeled carbon form (Σ) upon injection of 13C18O2 in a suspension of C. reinhardtii cells cultured in 2% CO2 (B) or at air level of CO2 (C). (D, E). Isotopic 18O content (α) of 13CO2 during experiments of (B, C) respectively. 13C and 18O-enriched CO2 was used to avoid the mass spectrometric background on m/z = 44 due to naturally present CO2, therefore enhancing the signal to background ratio (Radmer and Kok, 1976). 13C18O2 was injected after 1 min of darkness at 0.1 mM final concentration (vertical dotted line).
Although MIMS is more than 50 years old, its usage has only recently become popular (Ketola and Lauritsen, 2016). The recent development in setups and analytical protocols and its popularity helped pushed the limits of our knowledge in the biology of photosynthetic microorganisms at various scales (Burlacot et al., 2020a).
Gas exchange measurements, chlorophyll fluorescence (Maxwell and Johnson, 2000), and electrochromism measurements (Bailleul et al., 2010) are the three main tools available to measure photosynthetic activity in microalgae and cyanobacteria on intact organisms. Simultaneous measurements of gas exchange by MIMS and chlorophyll fluorescence have allowed for correlating energy dissipation processes with CO2 and O2 photoreduction occurring during photosynthesis (Sültemeyer et al., 1989; Burlacot and Peltier, 2018; Ware et al., 2020). However, our understanding is still limited by the functional redundancy of many mechanisms and their interaction with cryptic mechanisms such as cyclic electron flow, which are not easily experimentally accessible. Further coupling of these methods, allowing for instance parallel measurement of gas exchange by MIMS and cyclic electron flow by electrochromism, together with an increased accessibility to genetic resources, should provide in the future new insights on how the main photosynthetic processes are regulated and interact during acclimation to various environmental situations.
In the perspective of large-scale biofuel production by microalgae or cyanobacteria, recent research has focused on the design and use of photobioreactors coupled to MIMS for the analysis of volatile compounds of interest. These setups have been used to measure real time productions of H2 (Tamburic et al., 2011; Zhang et al., 2015) or ethylene (Zavřel et al., 2016) during medium and long-term cultivation of microalgae or cyanobacteria. In the future, MIMS could be used to study and optimize the production in photobioreactors of volatile hydrocarbons by engineered photosynthetic cells.
Further miniaturization and decreasing prices of mass spectrometers should enable an even larger number of laboratories to have access to MIMS in the future, thus accelerating our understanding of how photosynthetic microorganisms impact the atmosphere of our planet (Burlacot et al., 2020a). The use or application of MIMS in the field opens a new era of evaluating the occurrence and ecological relevance of molecular mechanisms in natural environment.
All datasets presented in this study are included in the article/Supplementary Material.
AB and GP designed the illustrating experiments. AB performed the experiments. AB and FB designed the software. FB developed the software with supervision from AB. AB, YL-B and GP wrote the manuscript.
This work was supported by the French Agence Nationale de la Recherche (ANR) projects OTOLHYD and PHOTOALKANE. AB is a recipient of a CEA international PhD studentship (Irtelis).
The authors declare that the research was conducted in the absence of any commercial or financial relationships that could be construed as a potential conflict of interest.
The authors thank Dr. Solène Moulin for drawings used in Figures 4, 5, 7, and 8 and Dr. Bernard Genty for stimulating discussions. The authors acknowledge the European Union Regional Developing Fund, the Region Provence-Alpes-Côte d’Azur, the French Ministry of Research, and the CEA for funding the HelioBiotec platform.
The Supplementary Material for this article can be found online at: https://www.frontiersin.org/articles/10.3389/fpls.2020.01302/full#supplementary-material
Abou Hamdan, A., Dementin, S., Liebgott, P.-P., Gutierrez-Sanz, O., Richaud, P., De Lacey, A. L., et al. (2012). Understanding and Tuning the Catalytic Bias of Hydrogenase. J. Am. Chem. Soc 134, 8368–8371. doi: 10.1021/ja301802r
Allahverdiyeva, Y., Mustila, H., Ermakova, M., Bersanini, L., Richaud, P., Ajlani, G., et al. (2013). Flavodiiron proteins Flv1 and Flv3 enable cyanobacterial growth and photosynthesis under fluctuating light. Proc. Natl. Acad. Sci. U. S. A. 110, 4111–4116. doi: 10.1073/pnas.1221194110
Badger, M. R., Andrews, T. J. (1982). Photosynthesis and inorganic carbon usage by the marine cyanobacterium, Synechococcus sp. Plant Physiol. 70, 517–523. doi: 10.1104/pp.70.2.517
Badger, M. R., Kaplan, A., Berry, J. A. (1980). Internal inorganic carbon pool of Chlamydomonas reinhardtii. Plant Physiol. 66, 407–413. doi: 10.1104/pp.66.3.407
Badger, M. R., Palmqvist, K., Yu, J.-W. (1994). Measurement of CO2 and HCO3– fluxes in cyanobacteria and microalgae during steady-state photosynthesis. Physiol. Plant. 90, 529–536. doi: 10.1111/j.1399-3054.1994.tb08811.x
Badger, M. R., von Caemmerer, S., Ruuska, S., Nakano, H. (2000). Electron flow to oxygen in higher plants and algae: rates and control of direct photoreduction (Mehler reaction) and rubisco oxygenase. Phil. Trans. R. Soc B. 355, 1433–1446. doi: 10.1098/rstb.2000.0704
Badger, M. R. (1985). Photosynthetic oxygen exchange. Ann. Rev. Plant Physiol. 36, 27–53. doi: 10.1146/annurev.pp.36.060185.000331
Bailleul, B., Cardol, P., Breyton, C., Finazzi, G. (2010). Electrochromism: a useful probe to study algal photosynthesis. Photosynth. Res. 106, 179. doi: 10.1007/s11120-010-9579-z
Bailleul, B., Berne, N., Murik, O., Petroutsos, D., Prihoda, J., Tanaka, A., et al. (2015). Energetic coupling between plastids and mitochondria drives CO2 assimilation in diatoms. Nature 524, 366. doi: 10.1038/nature14599
Bailleul, B., Park, J., Brown, C. M., Bidle, K. D., Lee, S. H., Falkowski, P. G. (2017). Direct measurements of the light dependence of gross photosynthesis and oxygen consumption in the ocean. Limnol. Oceanogr. 62, 1066–1079. doi: 10.1002/lno.10486
Beckmann, K., Messinger, J., Badger, M. R., Wydrzynski, T., Hillier, W. (2009). On-line mass spectrometry: membrane inlet sampling. Photosynth. Res. 102, 511–522. doi: 10.1007/s11120-009-9474-7
Benlloch, R., Shevela, D., Hainzl, T., Grundström, C., Shutova, T., Messinger, J., et al. (2015). Crystal structure and functional characterization of photosystem II-associated carbonic anhydrase CAH3 in Chlamydomonas reinhardtii. Plant Physiol. 167, 950–962. doi: 10.1104/pp.114.253591
Ben-Zvi, O., Dafni, E., Feldman, Y., Yacoby, I. (2019). Re-routing photosynthetic energy for continuous hydrogen production in vivo. Biotechnol. Biofuel 12, 266. doi: 10.1186/s13068-019-1608-3
Berlier, Y. M., Dimon, B., Fauque, G., Lespinat, P. A. (1985). “Direct mass-spectrometric monitoring of the metabolism and isotope exchange in enzymic and microbiological investigations,” in Gas Enzymology. Eds. Degn, H., Cox, R. P., Toftlund, H. (Springer Netherlands: Dordrecht), pp 17–35.
Bethke, P. C., Badger, M. R., Jones, R. L. (2004). Apoplastic synthesis of nitric oxide by plant tissues. Plant Cell 16, 332–341. doi: 10.1105/tpc.017822
Boatman, T. G., Davey, P. A., Lawson, T., Geider, R. J. (2018). CO2 modulation of the rates of photosynthesis and light-dependent O2 consumption in Trichodesmium. J. Exp. Bot. 70, 589–597. doi: 10.1093/jxb/ery368
Burén, S., Ortega-Villasante, C., Blanco-Rivero, A., Martínez-Bernardini, A., Shutova, T., Shevela, D., et al. (2011). Importance of post-translational modifications for functionality of a chloroplast-localized carbonic anhydrase (CAH1) in Arabidopsis thaliana. PLoS One 6, e21021. doi: 10.1371/journal.pone.0021021
Burlacot, A., Peltier, G. (2018). “Photosynthetic electron transfer pathways during hydrogen photoproduction in green algae: mechanisms and limitations,” in Microalgal Hydrogen Production: Achievements and Perspectives. Eds. Seibert, M., Torzillo, G. (London, U. K.: The Royal Society of Chemistry), pp 189–212. doi: 10.1039/9781849737128-00189
Burlacot, A., Sawyer, A., Cuiné, S., Auroy-Tarrago, P., Blangy, S., Happe, T., et al. (2018). Flavodiiron-mediated O2 photoreduction links H2 production with CO2 fixation during the anaerobic induction of photosynthesis. Plant Physiol. 177, 1639–1649.
Burlacot, A., Li-Beisson, Y., Peltier, G. (2020a). Membrane inlet mass spectrometry at the crossroads of photosynthesis, biofuel and climate research. Plant Physiol. 183, 451–45. doi: 10.1104/pp.20.00368
Burlacot, A., Richaud, P., Gosset, A., Li-Beisson, Y., Peltier, G. (2020b). Algal photosynthesis converts nitric oxide into nitrous oxide. Proc. Nat. Acad. Sci. U. S. A. 117 (5), 2704–2709. doi: 10.1073/pnas.1915276117
Cano, M., Volbeda, A., Guedeney, G., Aubert-Jousset, E., Richaud, P., Peltier, G., et al. (2014). Improved oxygen tolerance of the Synechocystis sp. PCC 6803 bidirectional hydrogenase by site-directed mutagenesis of putative residues of the gas diffusion channel. Int. J. Hyd. Energ. 39, 16872–16884. doi: 10.1016/j.ijhydene.2014.08.030
Catalanotti, C., Yang, W., Posewitz, M., Grossman, A. (2013). Fermentation metabolism and its evolution in algae. Front. Plant Sci. 4, 150. doi: 10.3389/fpls.2013.00150
Chatton, E., Labasque, T., de La Bernardie, J., Guihéneuf, N., Bour, O., Aquilina, L. (2017). Field continuous measurement of dissolved gases with a CF-MIMS: applications to the physics and biogeochemistry of groundwater flow. Environ. Sci. Technol. 51, 846–854. doi: 10.1021/acs.est.6b03706
Chaux, F., Burlacot, A., Mekhalfi, M., Auroy, P., Blangy, S., Richaud, P., et al. (2017). Flavodiiron proteins promote fast and transient O2 photoreduction in Chlamydomonas. Plant Physiol. 174, 1825–1836. doi: 10.1104/pp.17.00421
Cheah, M. H., Millar, A. H., Myers, R. C., Day, D. A., Roth, J., Hillier, W., et al. (2014). Online oxygen kinetic isotope effects using membrane inlet mass spectrometry can differentiate between oxidases for mechanistic studies and calculation of their contributions to oxygen consumption in whole tissues. Anal. Chem. 86, 5171–5178. doi: 10.1021/ac501086n
Chua, E. J., Savidge, W., Short, R. T., Cardenas-Valencia, A. M., Fulweiler, R. W. (2016). A review of the emerging field of underwater mass spectrometry. Front. Mar. Sci. 3, 209. doi: 10.3389/fmars.2016.00209
Clausen, J., Beckmann, K., Junge, W., Messinger, J. (2005). Evidence that bicarbonate is not the substrate in photosynthetic oxygen evolution. Plant Physiol. 139, 1444–1450. doi: 10.1104/pp.105.068437
Conrath, U., Amoroso, G., Köhle, H., Sültemeyer, D. F. (2004). Non-invasive online detection of nitric oxide from plants and some other organisms by mass spectrometry. Plant J. 38, 1015–1022. doi: 10.1111/j.1365-313X.2004.02096.x
Cournac, L., Dimon, B., Peltier, G. (1993). Evidence for 18O labeling of photorespiratory CO2 in photoautotrophic cell cultures of higher plants illuminated in the presence of 18O2. Planta 190, 407–414. doi: 10.1007/BF00196970
Cournac, L., Mus, F., Bernard, L., Guedeney, G., Vignais, P., Peltier, G. (2002). Limiting steps of hydrogen production in Chlamydomonas reinhardtii and Synechocystis PCC 6803 as analysed by light-induced gas exchange transients. Int. J. Hydrog. Energy 27, 1229–1237. doi: 10.1016/S0360-3199(02)00105-2
Cournac, L., Guedeney, G., Peltier, G., Vignais, P. M. (2004). Sustained photoevolution of molecular hydrogen in a mutant of Synechocystis sp strain PCC 6803 deficient in the type I NADPH-dehydrogenase complex. J. Bacteriol. 186, 1737–1746. doi: 10.1128/JB.186.6.1737-1746.2003
Csonka, C., Páli, T., Bencsik, P., Görbe, A., Ferdinandy, P., Csont, T. (2015). Measurement of NO in biological samples. Brit. J. Pharmacol. 172, 1620–1632. doi: 10.1111/bph.12832
Curien, G., Flori, S., Villanova, V., Magneschi, L., Giustini, C., Forti, G., et al. (2016). The Water to Water Cycles in Microalgae. Plant Cell Physiol. 57, 1354–1363. doi: 10.1093/pcp/pcw048
Dang, K. V., Plet, J., Tolleter, D., Jokel, M., Cuine, S., Carrier, P., et al. (2014). Combined increases in mitochondrial cooperation and oxygen photoreduction compensate for deficiency in cyclic electron flow in Chlamydomonas reinhardtii. Plant Cell 26, 3036–3050. doi: 10.1105/tpc.114.126375
De Mia, M., Lemaire, S. D., Choquet, Y., Wollman, F.-A. (2019). Nitric oxide remodels the photosynthetic apparatus upon S-starvation in Chlamydomonas reinhardtii. Plant Physiol. 179, 718–731. doi: 10.1104/pp.18.01164
Degn, H. (1992). Membrane inlet mass spectrometry in pure and applied microbiology. J. Microbiol. Methods 15, 185–197. doi: 10.1016/0167-7012(92)90039-7
Douchi, D., Liang, F., Cano, M., Xiong, W., Wang, B., Maness, P.-C., et al. (2019). Membrane-Inlet Mass Spectrometry enables a quantitative understanding of inorganic carbon uptake flux and carbon concentrating mechanisms in metabolically engineered cyanobacteria. Front. Microbiol. 10, 1356–1356. doi: 10.3389/fmicb.2019.01356
Eilenberg, H., Weiner, I., Ben-Zvi, O., Pundak, C., Marmari, A., Liran, O., et al. (2016). The dual effect of a ferredoxin-hydrogenase fusion protein in vivo: successful divergence of the photosynthetic electron flux towards hydrogen production and elevated oxygen tolerance. Biotechnol. Biofuels 9, 182. doi: 10.1186/s13068-016-0601-3
Einbinder, S., Gruber, D. F., Salomon, E., Liran, O., Keren, N., Tchernov, D. (2016). Novel adaptive photosynthetic characteristics of mesophotic symbiotic microalgae within the reef-building coral, Stylophora pistillata. Front. Marine Sci. 3, 195. doi: 10.3389/fmars.2016.00195
Erbes, D. L., King, D., Gibbs, M. (1979). Inactivation of hydrogenase in cell-free extracts and whole cells of Chlamydomonas reinhardi by oxygen. Plant Physiol. 63, 1138–1142. doi: 10.1104/pp.63.6.1138
Ermakova, M., Huokko, T., Richaud, P., Bersanini, L., Howe, C. J., Lea-Smith, D. J., et al. (2016). Distinguishing the roles of thylakoid respiratory terminal oxidases in the cyanobacterium Synechocystis sp. PCC 6803. Plant Physiol. 171, 1307–1319. doi: 10.1104/pp.16.00479
Falkowski, P., Kolber, Z. (1995). Variations in chlorophyll fluorescence yields in phytoplankton in the world oceans. Func. Plant Biol. 22, 341–355. doi: 10.1071/PP9950341
Ferrón, S., del Valle, D. A., Björkman, K. M., Quay, P. D., Church, M. J., Karl, D. M. (2016). Application of membrane inlet mass spectrometry to measure aquatic gross primary production by the 18O in vitro method. Limnol. Oceanogr. Methods 14, 610–622. doi: 10.1002/lom3.10116
Field, C. B., Behrenfeld, M. J., Randerson, J. T., Falkowski, P. (1998). Primary production of the biosphere: integrating terrestrial and oceanic components. Science 281, 237–240. doi: 10.1126/science.281.5374.237
Filina, V., Grinko, A., Ermilova, E. (2019). Truncated hemoglobins 1 and 2 are implicated in the modulation of phosphorus deficiency-induced nitric oxide levels in Chlamydomonas. Cells 8, 947. doi: 10.3390/cells8090947
Fisher, N. L., Halsey, K. H. (2016). Mechanisms that increase the growth efficiency of diatoms in low light. Photosynth. Res. 129, 183–197. doi: 10.1007/s11120-016-0282-6
Florin, L., Tsokoglou, A., Happe, T. (2001). A novel type of iron hydrogenase in the green alga Scenedesmus obliquus is linked to the photosynthetic electron transport chain. J. Biol. Chem. 276, 6125–6132. doi: 10.1074/jbc.M008470200
Fratamico, A., Tocquin, P., Franck, F. (2016). The chlorophyll a fluorescence induction curve in the green microalga Haematococcus pluvialis: further insight into the nature of the P–S–M fluctuation and its relationship with the “low-wave” phenomenon at steady-state. Phot. Res. 128, 271–285. doi: 10.1007/s11120-016-0241-2
Gauquelin, C., Baffert, C., Richaud, P., Kamionka, E., Etienne, E., Guieysse, D., et al. (2018). Roles of the F-domain in [FeFe] hydrogenase. Biochim. Biophys. Acta Bioenerg. 1859, 69–77. doi: 10.1016/j.bbabio.2017.08.010
Genty, B., Briantais, J. M., Baker, N. R. (1989). The relationship between the quantum yield of photosynthetic electron transport and quenching of chlorophyll fluorescence. Biochim. Biophys. Acta 990, 87–92. doi: 10.1016/S0304-4165(89)80016-9
Gerster, R., Dimon, B., Peybernes, A. (1974). “The fate of oxygen in photosynthesis,” in Proceeding of the third international congress on photosynthesis. (Amsterdam: Elsevier Publishing Co. Ltd.), pp 1589–1600.
Gerster, R. H., Dimon, B., Tournier, P., Peybernes, A. (1977). “Metabolism of oxygen during photorespiration,” in Stable isotopes in the life of sciences. (Vienna Austria: IAEA), pp 293–301.
Gerster, R. (1971). An attempt to interpret the kinetics of isotope exchange between C18O2 and the water of a leaf: Experiments in the dark. Planta 97, 155–172. doi: 10.1007/BF00386763
Ghysels, B., Godaux, D., Matagne, R. F., Cardol, P., Franck, F. (2013). Function of the chloroplast hydrogenase in the microalga Chlamydomonas: The role of hydrogenase and state transitions during photosynthetic activation in anaerobiosis. PLoS One 8, e64161. doi: 10.1371/journal.pone.0064161
Giordano, M., Beardall, J., Raven, J. A. (2005). CO2 concentration mechanisms in algae: mechanisms, environmental modulation, and evolution. Annu. Rev. Plant Biol. 56, 99–131. doi: 10.1146/annurev.arplant.56.032604.144052
Godaux, D., Bailleul, B., Berne, N., Cardol, P. (2015). Induction of photosynthetic carbon fixation in anoxia relies on hydrogenase activity and Proton-Gradient Regulation-Like1-mediated cyclic electron flow in Chlamydomonas reinhardtii. Plant Physiol. 168, 648–658. doi: 10.1104/pp.15.00105
Goreau, T. J., Kaplan, W. A., Wofsy, S. C., McElroy, M. B., Valois, F. W., Watson, S. W. (1980). Production of NO2- and N2O by nitrifying bacteria at reduced concentrations of oxygen. Appl. Environ. Microbiol. 40, 526–532. doi: 10.1128/AEM.40.3.526-532.1980
Guieysse, B., Plouviez, M., Coilhac, M., Cazali, L. (2013). Nitrous Oxide (N2O) production in axenic Chlorella vulgaris microalgae cultures: evidence, putative pathways, and potential environmental impacts. Biogeosciences 10, 6737–6746. doi: 10.5194/bg-10-6737-2013
Hanson, D. T., Franklin, L. A., Samuelsson, G., Badger, M. R. (2003). The Chlamydomonas reinhardtii cia3 mutant lacking a thylakoid lumen-localized carbonic anhydrase is limited by CO2 supply to rubisco and not photosystem II function in vivo. Plant Physiol. 132, 2267–2275. doi: 10.1104/pp.103.023481
Helman, Y., Tchernov, D., Reinhold, L., Shibata, M., Ogawa, T., Schwarz, R., et al. (2003). Genes encoding a-type flavoproteins are essential for photoreduction of O2 in cyanobacteria. Curr. Biol. 13, 230–235. doi: 10.1016/S0960-9822(03)00046-0
Hemschemeier, A., Düner, M., Casero, D., Merchant, S. S., Winkler, M., Happe, T. (2013). Hypoxic survival requires a 2-on-2 hemoglobin in a process involving nitric oxide. Proc. Nat. Acad. Sci. U. S. A. 110, 10854–10859. doi: 10.1073/pnas.1302592110
Hoberman, H. D., Rittenberg, D. (1943). Biological catalysis of the exchange reaction between water and hydrogen. J. Biol. Chem. 147, 211–227.
Hoch, G., Kok, B. (1963). A mass spectrometer inlet system for sampling gases dissolved in liquid phases. Arch. Biochem. Biophys. 101, 160–170. doi: 10.1016/0003-9861(63)90546-0
Hohmann-Marriott, M. F., Blankenship, R. E. (2011). Evolution of photosynthesis. Annu. Rev. Plant Biol. 62, 515–548. doi: 10.1146/annurev-arplant-042110-103811
Hunt, P. P., Smith, H. A. (1961). The separation of hydrogen, deuterium and hydrogen deuteride mixtures by gas chromatography. J. Phys. Chem. 65, 87–89. doi: 10.1021/j100819a027
IPCC (2013). Climate Change 2013: The Physical Science Basis. Contribution of Working Group I to the Fifth Assessment Report of the Intergovernmental Panel on Climate Change (Cambridge, United Kingdom and New York, NY, USA: Cambridge University Press).
Jans, F., Mignolet, E., Houyoux, P. A., Cardol, P., Ghysels, B., Cuine, S., et al. (2008). A type II NAD(P) H dehydrogenase mediates light-independent plastoquinone reduction in the chloroplast of Chlamydomonas. Proc. Natl. Acad. Sci. U. S. A. 105, 20546–20551. doi: 10.1073/pnas.0806896105
Jensen, B. B., Cox, R. P. (1988). “Measurement of hydrogen exchange and nitrogen uptake by mass spectrometry,” in Methods in Enzymology, vol. 167. (San Diego, USA: Academic Press), pp 467–474.
Johnson, R. C., Cooks, R. G., Allen, T. M., Cisper, M. E., Hemberger, P. H. (2000). Membrane introduction mass spectrometry: trends and applications. Mass Spectom. Rev. 19, 1–37. doi: 10.1002/(SICI)1098-2787(2000)19:1<1::AID-MAS1>3.0.CO;2-Y
Jokel, M., Nagy, V., Tóth, S. Z., Kosourov, S., Allahverdiyeva, Y. (2019). Elimination of the flavodiiron electron sink facilitates long-term H2 photoproduction in green algae. Biotechnol. Biofuels 12, 280. doi: 10.1186/s13068-019-1618-1
Jouanneau, Y., Kelley, B. C., Berlier, Y., Lespinat, P. A., Vignais, P. M. (1980). Continuous monitoring, by mass spectrometry, of H2 production and recycling in Rhodopseudomonas capsulata. J. Bacteriol. 143, 628–636. doi: 10.1128/JB.143.2.628-636.1980
Ketola, R. A., Lauritsen, F. R. (2016). “Membrane inlet mass spectrometry (MIMS) in historical perspective,” in The Encyclopedia of Mass Spectrometry. Eds. Gross, M. L., Caprioli, R. M. (Boston: Elsevier), pp 143–148.
Konermann, L., Messinger, J., Hillier, W. (2008). “Mass spectrometry-based methods for studying kinetics and dynamics in biological systems,” in Biophysical Techniques in Photosynthesis. Eds. Aartsma, T. J., Matysik, J. (Springer Netherlands: Dordrecht), pp 167–190.
Kong, F., Burlacot, A., Liang, Y., Légeret, B., Alseekh, S., Brotman, Y., et al. (2018). Interorganelle communication: peroxisomal MALATE DEHYDROGENASE2 connects lipid catabolism to photosynthesis through redox coupling in Chlamydomonas. Plant Cell 30, 1824–1847. doi: 10.1105/tpc.18.00361
Koroidov, S., Shevela, D., Shutova, T., Samuelsson, G., Messinger, J. (2014). Mobile hydrogen carbonate acts as proton acceptor in photosynthetic water oxidation. Proc. Acad. Nat. Sci. U. S. A. 111, 6299–6304. doi: 10.1073/pnas.1323277111
Koroidov, S., Anderlund, M. F., Styring, S., Thapper, A., Messinger, J. (2015). First turnover analysis of water-oxidation catalyzed by Co-oxide nanoparticles. Energ. Environ. Sci. 8, 2492–2503. doi: 10.1039/C5EE00700C
Kosourov, S., Jokel, M., Aro, E.-M., Allahverdiyeva, Y. (2018). A new approach for sustained and efficient H2 photoproduction by Chlamydomonas reinhardtii. Energy Environ. Sci. 11, 1431–1436. doi: 10.1039/C8EE00054A
Kotiaho, T., Lauritsen, F. R. (2002). “Membrane inlet mass spectrometry,” in Comprehensive Analytical Chemistry, vol. 37. (Netherlands: Elsevier), pp 531–557.
Leroux, F., Dementin, S., Burlat, B., Cournac, L., Volbeda, A., Champ, S., et al. (2008). Experimental approaches to kinetics of gas diffusion in hydrogenase. Proc. Nat. Acad. Sci. U. S. A. 105, 11188–11193. doi: 10.1073/pnas.0803689105
Li, H., Wan, A. (2015). Fluorescent probes for real-time measurement of nitric oxide in living cells. Analyst 140, 7129–7141. doi: 10.1039/C5AN01628B
Liebgott, P.-P., de Lacey, A. L., Burlat, B., Cournac, L., Richaud, P., Brugna, M., et al. (2011). Original design of an oxygen-tolerant [NiFe] hydrogenase: major effect of a valine-to-cysteine mutation near the active site. J. Am. Chem. Soc. 133, 986–997. doi: 10.1021/ja108787s
Liran, O., Semyatich, R., Milrad, Y., Eilenberg, H., Weiner, I., Yacoby, I. (2016). Microoxic niches within the thylakoid stroma of air-grown Chlamydomonas reinhardtii protect [FeFe]-hydrogenase and support hydrogen production under fully aerobic environment. Plant Physiol. 172, 264–271. doi: 10.1104/pp.16.01063
Lloyd, D., Scott, R. I., Williams, T. N. (1983). Membrane inlet mass spectrometry — measurement of dissolved gases in fermentation liquids. Trends Biotechnol. 1, 60–63. doi: 10.1016/0167-7799(83)90071-9
Luimstra, V. M., Schuurmans, J. M., de Carvalho, C. F. M., Matthijs, H. C. P., Hellingwerf, K. J., Huisman, J. (2019). Exploring the low photosynthetic efficiency of cyanobacteria in blue light using a mutant lacking phycobilisomes. Photosynth. Res. 141, 291–301. doi: 10.1007/s11120-019-00630-z
Lundsgaard, J. S., Grønlund, J., Degn, H. (1978). Error in oxygen measurements in open systems owing to oxygen consumption in unstirred layer. Biotechnol. Bioeng. 20, 809–819. doi: 10.1002/bit.260200604
Mackinder, L. C. M., Chen, C., Leib, R. D., Patena, W., Blum, S. R., Rodman, M., et al. (2017). A spatial interactome reveals the protein organization of the algal CO2-concentrating mechanism. Cell 171, 133–147.e114. doi: 10.1016/j.cell.2017.08.044
Mackinder, L. C. M. (2018). The Chlamydomonas CO2-concentrating mechanism and its potential for engineering photosynthesis in plants. New Phytol. 217, 54–61. doi: 10.1111/nph.14749
Maeda, K., Spor, A., Edel-Hermann, V., Heraud, C., Breuil, M.-C., Bizouard, F., et al. (2015). N2O production, a widespread trait in fungi. Sci. Rep. 5, 9697. doi: 10.1038/srep09697
Manz, D.-H., Duan, P.-C., Dechert, S., Demeshko, S., Oswald, R., John, M., et al. (2017). Pairwise H2/D2 exchange and H2 substitution at a bimetallic dinickel(II) complex featuring two terminal hydrides. J. Am. Chem. Soc 139, 16720–16731. doi: 10.1021/jacs.7b08629
Maxwell, K., Johnson, G. N. (2000). Chlorophyll fluorescence—a practical guide. J. Exp. Bot. 51, 659–668. doi: 10.1093/jexbot/51.345.659
Melis, A., Zhang, L. P., Forestier, M., Ghirardi, M. L., Seibert, M. (2000). Sustained photobiological hydrogen gas production upon reversible inactivation of oxygen evolution in the green alga Chlamydomonas reinhardtii. Plant Physiol. 122, 127–135. doi: 10.1104/pp.122.1.127
Nagy, V., Podmaniczki, A., Vidal-Meireles, A., Tengölics, R., Kovács, L., Rákhely, G., et al. (2018). Water-splitting-based, sustainable and efficient H2 production in green algae as achieved by substrate limitation of the Calvin–Benson–Bassham cycle. Biotechnol. Biofuels 11, 69. doi: 10.1186/s13068-018-1069-0
Northrop, D. B., Simpson, F. B. (1998). Kinetics of enzymes with isomechanisms: britton induced transport catalyzed by bovine carbonic anhydrase II, measured by rapid-flow mass spectrometry. Arch. Biochem. Biophys. 352, 288–292. doi: 10.1006/abbi.1997.0589
Palmqvist, K., Yu, J.-W., Badger, M. R. (1994). Carbonic anhydrase activity and inorganic carbon fluxes in low- and high-Ci cells of Chlamydomonas reinhardtii and Scenedesmus obliquus. Physiol. Plantarum 90, 537–547. doi: 10.1111/j.1399-3054.1994.tb08812.x
Peltier, G., Thibault, P. (1985a). Light-dependent oxygen uptake, glycolate, and ammonia release in L-Methionine Sulfoximine-treated Chlamydomonas. Plant Physiol. 77, 281–284. doi: 10.1104/pp.77.2.281
Peltier, G., Thibault, P. (1985b). Oxygen uptake in the light in Chlamydomonas. Evidence for persistent mitochondrial respiration. Plant Physiol. 79, 225–230. doi: 10.1104/pp.79.1.225
Peltier, G., Cournac, L., Despax, V., Dimon, B., Fina, L., Genty, B., et al. (1995). Carbonic anhydrase activity in leaves as measured in vivo by 18O exchange between carbon dioxide and water. Planta 196, 732–739. doi: 10.1007/BF01106768
Poulsen, A. K., Rompel, A., McKenzie, C. J. (2005). Water oxidation catalyzed by a dinuclear Mn complex: a functional model for the oxygen-evolving center of photosystem II. Angewandte Chemie 44, 6916–6920. doi: 10.1002/anie.200502114
Price, G. D., Badger, M. R., Woodger, F. J., Long, B. M. (2007). Advances in understanding the cyanobacterial CO2-concentrating-mechanism (CCM): functional components, Ci transporters, diversity, genetic regulation and prospects for engineering into plants. J. Exp. Bot. 59, 1441–1461. doi: 10.1093/jxb/erm112
Radmer, R. J., Kok, B. (1976). Photoreduction of O2 pimes and replaces CO2 assimilation. Plant Physiol. 58, 336–340. doi: 10.1104/pp.58.3.336
Radmer, R., Ollinger, O. (1980a). Isotopic composition of photosynthetic O2 flash yields in the presence of H218O and HC18O–3. FEBS Lett. 110, 57–61. doi: 10.1016/0014-5793(80)80022-6
Radmer, R., Ollinger, O. (1980b). “Measurement of the oxygen cycle : the mass spectrometric analysis of gases dissolved in a liquid phase,” in Methods in Enzymology, vol. 69 . Ed. San Pietro, A. (San Diego, USA: Academic Press), pp 547–560.
Radmer, R., Ollinger, O. (1986). Do the higher oxidation states of the photosynthetic O2-evolving system contain bound H2O? FEBS Lett. 195, 285–289. doi: 10.1016/S0076-6879(80)69054-5
Redding, K., Cournac, L., Vassiliev, I. R., Golbeck, J. H., Peltier, G., Rochaix, J. D. (1999). Photosystem I is indispensable for photoautotrophic growth, CO2 fixation, and H2 photoproduction in Chlamydomonas reinhardtii. J. Biol. Chem. 274, 10466–10473. doi: 10.1074/jbc.274.15.10466
Reinfelder, J. R. (2011). Carbon concentrating mechanisms in eukaryotic marine phytoplankton. Annu. Rev. Mar. Sci. 3, 291–315. doi: 10.1146/annurev-marine-120709-142720
Rittenberg, D., Krasna, A. I. (1955). Interaction of hydrogenase with hydrogen. Discuss. Faraday Soc 20, 185–189. doi: 10.1039/DF9552000185
Shevela, D., Messinger, J. (2013). Studying the oxidation of water to molecular oxygen in photosynthetic and artificial systems by time-resolved membrane-inlet mass spectrometry. Front. Plant Sci. 4, 473. doi: 10.3389/fpls.2013.00473
Shevela, D., Beckmann, K., Clausen, J., Junge, W., Messinger, J. (2011). Membrane-inlet mass spectrometry reveals a high driving force for oxygen production by photosystem II. Proc. Nat. Acad. Sci. U. S. A. 108, 3602–3607. doi: 10.1073/pnas.1014249108
Shevela, D., Schröder, W. P., Messinger, J. (2018). “Liquid-phase measurements of photosynthetic oxygen evolution,” in Photosynthesis: Methods and Protocols. Ed. Covshoff, S. (New York, NY: Springer New York), pp. 197–211. doi: 10.1007/978-1-4939-7786-4_11
Shevela, D., Do, H.-N., Fantuzzi, A., Rutherford, A. W., Messinger, J. (2020). Bicarbonate-mediated CO2 formation on both sides of photosystem II. BIochem. 59, 2442–2449. doi: 10.1021/acs.biochem.0c00208
Silverman, D. N. (1982). “Carbonic anhydrase: oxygen-18 exchange catalyzed by an enzyme with rate-contributing proton-transfer steps,” in Methods in Enzymology, vol. 87 . Ed. Purich, D. L. (San Diego, USA: Academic Press), pp 732–752.
So, A. K. C., Van Spall, H. G. C., Coleman, J. R., Espie, G. S. (1998). Catalytic exchange of 18O from 13C18O-labelled CO2 by wild-type cells and ecaA, ecaB, and ccaA mutants of the cyanobacteria Synechococcus PCC7942 and Synechocystis PCC6803. Can. J. Bot. 76, 1153–1160. doi: 10.1139/b98-063
Sorigué, D., Légeret, B., Cuiné, S., Blangy, S., Moulin, S., Billon, E., et al. (2017). An algal photoenzyme converts fatty acids to hydrocarbons. Science 357, 903–907. doi: 10.1126/science.aan6349
Sültemeyer, D., Rinast, K.-A. (1996). The CO2 permeability of the plasma membrane of Chlamydomonas reinhardtii: mass-spectrometric 18O-exchange measurements from 13C18O2 in suspensions of carbonic anhydrase-loaded plasma-membrane vesicles. Planta 200, 358–368. doi: 10.1007/BF00200304
Sültemeyer, D. F., Klug, K., Fock, H. P. (1987). Effect of dissolved inorganic carbon on oxygen evolution and uptake by Chlamydomonas reinhardtii suspensions adapted to ambient and CO2-enriched air. Photosynth. Res. 12, 25–33. doi: 10.1007/BF00019148
Sültemeyer, D. F., Miller, A. G., Espie, G. S., Fock, H. P., Canvin, D. T. (1989). Active CO2 transport by the green alga Chlamydomonas reinhardtii. Plant Physiol. 89, 1213–1219. doi: 10.1104/pp.89.4.1213
Sültemeyer, D. F., Fock, H. P., Canvin, D. T. (1990). Mass spectrometric measurement of intracellular carbonic anhydrase activity in high and low Ci cells of Chlamydomonas. Plant Physiol. 94, 1250–1257. doi: 10.1104/pp.94.3.1250
Sültemeyer, D. F., Fock, H. P., Canvin, D. T. (1991). Active uptake of inorganic carbon by Chlamydomonas reinhardtii: evidence for simultaneous transport of HCO3– and CO2 and characterization of active CO2 transport. Can. J. Bot. 69, 995–1002. doi: 10.1139/b91-128
Sültemeyer, D., Biehler, K., Fock, H. P. (1993). Evidence for the contribution of pseudocyclic photophosphorylation to the energy requirement of the mechanism for concentrating inorganic carbon in Chlamydomonas. Planta 189, 235–242. doi: 10.1007/BF00195082
Sültemeyer, D., Klughammer, B., Badger, M. R., Dean Price, G. (1998). Fast induction of high-affinity HCO3- transport in cyanobacteria. Plant Physiol. 116, 183–192. doi: 10.1104/pp.116.1.183
Tamburic, B., Zemichael, F. W., Crudge, P., Maitland, G. C., Hellgardt, K. (2011). Design of a novel flat-plate photobioreactor system for green algal hydrogen production. Int. J. Hydrogen Energy 36, 6578–6591. doi: 10.1016/j.ijhydene.2011.02.091
Tansik, A. L., Fitt, W. K., Hopkinson, B. M. (2015). External carbonic anhydrase in three Caribbean corals: quantification of activity and role in CO2 uptake. Coral Reefs 34, 703–713. doi: 10.1007/s00338-015-1289-8
Tolleter, D., Ghysels, B., Alric, J., Petroutsos, D., Tolstygina, I., Krawietz, D., et al. (2011). Control of hydrogen photoproduction by the proton gradient generated by cyclic electron flow in Chlamydomonas reinhardtii. Plant Cell 23, 2619–2630. doi: 10.1105/tpc.111.086876
Tolleter, D., Chochois, V., Poiré, R., Price, G. D., Badger, M. R. (2017). Measuring CO2 and HCO3– permeabilities of isolated chloroplasts using a MIMS-18O approach. J. Exp. Bot. 68, 3915–3924. doi: 10.1093/jxb/erx188
Tóth, S. Z., Yacoby, I. (2019). Paradigm shift in algal H2 production: bypassing competitive processes. Trends Biotechnol. 37, 1159–1163. doi: 10.1016/j.tibtech.2019.05.001
van der Oost, J., Cox, R. P. (1988). Hydrogenase activity in nitrate-grown cells of the unicellular cyanobacterium Cyanothece PCC 7822. Arch. Microbiol. 151, 40–43. doi: 10.1007/BF00444666
Vignais, P. M., Henry, M.-F., Berlier, Y., Lespinat, P. A. (1982). Effect of pH on H-2H exchange, H2 production and H2 uptake, catalysed by the membrane-bound hydrogenase of Paracoccus denitrificans. Biochim. Biophys. Acta Bioenerg. 681, 519–529. doi: 10.1016/0005-2728(82)90195-5
Vignais, P. M., Cournac, L., Hatchikian, E. C., Elsen, S., Serebryakova, L., Zorin, N., et al. (2002). Continuous monitoring of the activation and activity of [NiFe]-hydrogenases by membrane-inlet mass spectrometry. Int. J. Hyd. Energ. 27, 1441–1448. doi: 10.1016/S0360-3199(02)00114-3
Vignais, P. M. (2005). H/D exchange reactions and mechanistic aspects of the hydrogenases. Coord. Chem. Rev. 249, 1677–1690. doi: 10.1016/j.ccr.2005.01.026
Wang, Y., Spalding, M. H. (2014). Acclimation to very low CO2: contribution of limiting CO2 inducible proteins, LCIB and LCIA, to inorganic carbon uptake in Chlamydomonas reinhardtii. Plant Physiol. 166, 2040–2050. doi: 10.1104/pp.114.248294
Wang, R. T. (1980). “Amperometric hydrogen electrode,” in Methods in Enzymology, vol. 69 . Ed. San Pietro, A. (San Diego, USA: Academic Press), pp 409–413.
Ware, M. A., Hunstiger, D., Cantrell, M., Peers, G. (2020). A chlorophyte alga utilizes alternative electron transport for primary photoprotection. Plant Physiol. 183, 1735–1748. doi: 10.1104/pp.20.00373
Whitehead, L., Long, B. M., Price, G. D., Badger, M. R. (2014). Comparing the in vivo function of α-carboxysomes and β-carboxysomes in two model cyanobacteria. Plant Physiol. 165, 398–411. doi: 10.1104/pp.114.237941
Xu, T., Yin, C.-J. M., Wodrich, M. D., Mazza, S., Schultz, K. M., Scopelliti, R., et al. (2016). A functional model of [Fe]-hydrogenase. J. Am. Chem. Soc 138, 3270–3273. doi: 10.1021/jacs.5b12095
Yu, J., Price, G., Badger, M. (1994). Characterisation of CO2 and HCO3- uptake during steady-state photosynthesis in the cyanobacterium Synechococcus PCC7942. Funct. Plant Biol. 21, 185–195. doi: 10.1071/PP9940185
Zavřel, T., Knoop, H., Steuer, R., Jones, P. R., Červený, J., Trtílek, M. (2016). A quantitative evaluation of ethylene production in the recombinant cyanobacterium Synechocystis sp. PCC 6803 harboring the ethylene-forming enzyme by membrane inlet mass spectrometry. Biores. Technol. 202, 142–151. doi: 10.1016/j.biortech.2015.11.062
Keywords: gas exchange, photosynthesis, carbonic anhydrase, CO2 concentrating mechanism, O2 evolution, H2 production, microalgae, cyanobacteria
Citation: Burlacot A, Burlacot F, Li-Beisson Y and Peltier G (2020) Membrane Inlet Mass Spectrometry: A Powerful Tool for Algal Research. Front. Plant Sci. 11:1302. doi: 10.3389/fpls.2020.01302
Received: 18 May 2020; Accepted: 11 August 2020;
Published: 04 September 2020.
Edited by:
Pietro Franceschi, Fondazione Edmund Mach, ItalyReviewed by:
Giuseppe Pieraccini, University of Florence, ItalyCopyright © 2020 Burlacot, Burlacot, Li-Beisson and Peltier. This is an open-access article distributed under the terms of the Creative Commons Attribution License (CC BY). The use, distribution or reproduction in other forums is permitted, provided the original author(s) and the copyright owner(s) are credited and that the original publication in this journal is cited, in accordance with accepted academic practice. No use, distribution or reproduction is permitted which does not comply with these terms.
*Correspondence: Gilles Peltier, Z2lsbGVzLnBlbHRpZXJAY2VhLmZy
†ORCID: Adrien Burlacot, orcid.org/0000-0001-7434-6416
François Burlacot, orcid.org/0000-0001-9783-6848
Yonghua Li-Beisson, orcid.org/0000-0003-1064-1816
Gilles Peltier, orcid.org/0000-0002-2226-3931
Disclaimer: All claims expressed in this article are solely those of the authors and do not necessarily represent those of their affiliated organizations, or those of the publisher, the editors and the reviewers. Any product that may be evaluated in this article or claim that may be made by its manufacturer is not guaranteed or endorsed by the publisher.
Research integrity at Frontiers
Learn more about the work of our research integrity team to safeguard the quality of each article we publish.