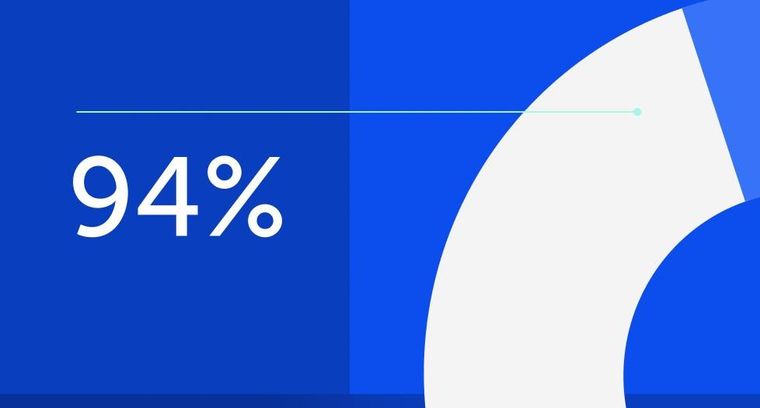
94% of researchers rate our articles as excellent or good
Learn more about the work of our research integrity team to safeguard the quality of each article we publish.
Find out more
REVIEW article
Front. Plant Sci., 13 August 2020
Sec. Plant Pathogen Interactions
Volume 11 - 2020 | https://doi.org/10.3389/fpls.2020.01237
This article is part of the Research TopicRNAi Based PesticidesView all 15 articles
Canonical RNAi, one of the so-called RNA-silencing mechanisms, is defined as sequence-specific RNA degradation induced by long double-stranded RNA (dsRNA). RNAi occurs in four basic steps: (i) processing of long dsRNA by RNase III Dicer into small interfering RNA (siRNA) duplexes, (ii) loading of one of the siRNA strands on an Argonaute protein possessing endonucleolytic activity, (iii) target recognition through siRNA basepairing, and (iv) cleavage of the target by the Argonaute’s endonucleolytic activity. This basic pathway diversified and blended with other RNA silencing pathways employing small RNAs. In some organisms, RNAi is extended by an amplification loop employing an RNA-dependent RNA polymerase, which generates secondary siRNAs from targets of primary siRNAs. Given the high specificity of RNAi and its presence in invertebrates, it offers an opportunity for highly selective pest control. The aim of this text is to provide an introductory overview of key mechanistic aspects of RNA interference for understanding its potential and constraints for its use in pest control.
RNA interference (RNAi) is one of the pathways, collectively named RNA silencing pathways, that employ small RNAs as guides for sequence-specific silencing [reviewed in (Ketting, 2011)]. RNAi was discovered in C. elegans and defined as sequence-specific mRNA degradation induced by long double-stranded RNA (dsRNA) (Fire et al., 1998). Although some authors use the term RNAi as a synonym for RNA silencing [e.g., (Ketting, 2011)], this review will adhere to the original definition as formulated by Fire et al.
The primary aim of this contribution is to provide an overview of RNA interference mechanism with focus on selected aspects concerning RNAi targeting and off-targeting in animals as these would be most relevant features for discussing the use of RNAi for pest control. Therefore, I will purposefully not go into the details. Interested readers should check out referenced reviews or original articles. For a thorough overview of RNAi, readers are welcome to refer to a comprehensive compilation of information on RNAi and related pathways in different animal taxons and plants, which we assembled with colleagues for the European Food and Safety Authority (Paces et al., 2017).
Some kind of RNA silencing pathway (Figure 1A) exists in almost every eukaryotic organism with some notable exceptions among fungi and protists (Nakayashiki et al., 2006; Matveyev et al., 2017). RNA silencing pathways utilize 20-30 nucleotide long RNAs loaded on Argonaute proteins, which guide sequence-specific repression through basepairing with target RNAs. RNA silencing pathways differ in the origin and biogenesis of small RNAs, mechanisms leading to target repression, and biological roles [reviewed in (Ketting, 2011)].
Figure 1 RNA silencing pathways. (A) General concept of RNA silencing. (B) General RNAi pathway overview, and (C) miRNA pathway (animal set up).
RNA substrates giving rise to small RNA guides in RNA silencing pathways vary in structure. They include double-stranded RNA (dsRNA) with blunt ends, small and long RNA hairpins with perfect and less-than-perfect complementarity, sense and antisense RNA (basepaired or not), or single-stranded “aberrant” RNA that would be converted to dsRNA by an RNA-dependent RNA polymerases (RdRP) or converted directly to small RNAs. Substrates can be converted to a small RNA either by Dicer, an RNase III cleaving dsRNA and/or canonical microRNA (miRNA) precursors, or by some Dicer-independent mechanism [reviewed in (Kim et al., 2009)].
Target repression can be post-transcriptional or transcriptional. Post-transcriptional RNA silencing could have a form of endonucleolytic cleavage of cognate RNA (traditionally associated with RNAi), or translational repression coupled with mRNA destabilization (historically associated with animal miRNAs). Transcriptional RNA silencing is common in plants but rare among animals [reviewed in (Wassenegger, 2005; Malik and Svoboda, 2012)]. It may involve de novo DNA methylation or transcriptionally repressive histone modifications.
Common biological roles of RNA silencing pathways include regulation of endogenous gene expression, antiviral immunity, and genome protection against transposable elements [summarized in (Ketting, 2011)]. During evolution, RNA silencing could evolve into a complex system of interconnected pathways [exemplified by plants, reviewed for example in (Borges and Martienssen, 2015)] or into a relatively simple set up (mammalian soma). The following text will focus on RNAi but includes also the miRNA pathway because of its close mechanistic relationship to RNAi.
The canonical RNAi pathway (Figure 1B) is initiated by cleavage of long dsRNA into small interfering RNAs (siRNAs). One siRNA strand then becomes loaded onto an Argonaute protein possessing endonucleolytic activity (e.g., AGO2 in vertebrates and arthropods). A complementary mRNA is cleaved by the Argonaute in the middle of the siRNA:mRNA duplex. In some taxons (e.g., plants or C. elegans), RNAi pathways employ the above-mentioned RdRPs, which can provide an amplification loop synthesizing small RNAs or dsRNA on targeted RNA templates [reviewed in (Maida and Masutomi, 2011)]. C. elegans employs so-called “transitive RNAi” where RdRP produces secondary siRNAs extending upstream of the targeted sequence (Sijen et al., 2001). Plants also exhibit transitive silencing (Vaistij et al., 2002); the transitivity may even spread downstream of the targeted sequence (Moissiard et al., 2007).
Canonical RNAi is traditionally viewed as a defense pathway providing antiviral innate immunity in invertebrates and plants against viruses that produce dsRNA (Ding and Voinnet, 2007). However, RNAi could evolve additional roles, such as maintenance of genome integrity through suppression of transposable elements or control of gene expression. In plants, for example, the basic RNAi mechanism has been integrated into a complex pathway system of post-transcriptional and transcriptional silencing, which employs multiple Dicer, Argonaute and RdRP proteins and functions in antiviral defense, protection of genome integrity, and regulation of gene expression [reviewed for example in (Bologna and Voinnet, 2014; Borges and Martienssen, 2015)]. In C. elegans. RNAi exists as a complex of antiviral RNAi, endo-RNAi controlling endogenous genes, and exo-RNAi responding to dsRNA in the environment [reviewed in (Billi et al., 2014)]. RNAi is functional in insects (Dowling et al., 2016) and other arthropod subphyla, including Chelicerata [ticks and mites (Kurscheid et al., 2009; Schnettler et al., 2014; Hoy et al., 2016)] and Crustacea [shrimps (Chen et al., 2011; Huang and Zhang, 2013; Yang et al., 2014)]; genomic data suggest that Myriapoda arthropods also have functional RNAi (Palmer and Jiggins, 2015). In vertebrates, the RNAi pathway has become vestigial; protein factors for siRNA biogenesis and target repression serve the miRNA pathway [reviewed in (Svoboda, 2014)]. This is presumably a consequence of the innate immunity system evolving an array of protein sensors detecting pathogen markers such as dsRNA, which trigger the so-called interferon response [reviewed in (Gantier and Williams, 2007)]. An important limiting factor for functional RNAi in somatic mammalian cells seems to be inefficient siRNA production due to the low processivity of mammalian Dicer, which is adapted for non-processive miRNA biogenesis (Demeter et al., 2019).
While the miRNA pathway (Figure 1C) can share some components with the RNAi pathway, it differs in several fundamental aspects. miRNAs are genome-encoded repressors of gene expression with defined sequences (i.e., can be precisely annotated). While RNAi employs a population of siRNAs stochastically generated from dsRNA to destroy a pool of RNAs with the complementary sequence, one specific miRNA sequence can guide repression of many different mRNAs through imperfect miRNA:mRNA basepairing.
Animal miRNA biogenesis [reviewed in (Kim et al., 2009)] starts with a primary miRNA (pri-miRNA), a long Pol II transcript carrying one or more local hairpins, which can be cut out from the pri-miRNA by RNase III activity of the nuclear Microprocessor complex. The resulting miRNA precursor (pre-miRNA) is transported to the cytoplasm, where it is cleaved by Dicer. One strand of the resulting duplex is loaded onto an AGO protein similarly to the RNAi pathway. Vertebrates have usually four AGO paralogs; teleost fish acquired an additional AGO3 paralogue through a fish-specific genome duplication event (Mcfarlane et al., 2011). All four mammalian AGO proteins accommodate miRNAs equally well (Meister et al., 2004), including AGO2, which is the only one with “slicing” endonucleolytic activity. All four mouse AGO proteins seem to be functionally redundant in the miRNA pathway, as shown by rescue experiments in embryonic stem cells lacking all four Ago genes (Su et al., 2009).
Typical miRNA:mRNA interaction in animals occurs with partial complementarity (described in detail further below) and results in translational repression, which is associated with substantial mRNA degradation. Plant miRNA biogenesis [reviewed in (Jones-Rhoades et al., 2006)] employs one of the Dicer paralogs (DCL1), which processes both pri-miRNA and pre miRNA. Plant miRNAs often have higher sequence complementarity resulting in RNAi-like cleavage of their targets but also frequently repress translation (Brodersen et al., 2008; Lanet et al., 2009). In animals, miRNAs can also mediate RNAi-like cleavage, as demonstrated by reporters designed to have full complementarity to a specific miRNA (Schmitter et al., 2006), but naturally occurring RNAi-like endonucleolytic cleavage of targets is rare (Yekta et al., 2004). The experimental approach to knocking down gene expression in mammalian cells by delivering a siRNA (either as an in vitro synthesized RNA or expressed from a plasmid vector) is commonly called RNAi. Mechanistically, however, the approach hijacks the miRNA pathway and its aforementioned ability to produce RNAi-like cleavage.
While there is an apparent mechanistic overlap, there is functional divergence of RNAi and miRNA pathways, which likely influenced the co-existence of the two pathways in different model systems during evolution (Figure 2). One is represented by Drosophila, where both pathways genetically diverged such that each pathway has a dedicated Dicer and AGO protein, while the crosstalk between the two pathways is minimal. Dicer in the RNAi pathway is phylogenetically more derived, which would be consistent with its engagement in dsRNA-based antiviral defense and host-pathogen evolutionary arms race (Murphy et al., 2008; Obbard et al., 2009). C. elegans employs a single Dicer in production of miRNAs and siRNAs, but has a complex system of Argonaute proteins and RdRP amplification, which contributes to the separation of the pathways. Mammals have a single Dicer mainly serving for miRNA biogenesis; canonical RNAi was functionally replaced by the interferon response, which allows for sensing more structural features of replicating RNA viruses. Functional RNAi in mammalian cells requires high Dicer activity, enough dsRNA substrate, and suppression of the interferon response (Kennedy et al., 2015; Maillard et al., 2016; Kennedy et al., 2017; Van Der Veen et al., 2018; Demeter et al., 2019). However, these three conditions are rarely met—a unique example occurs in the mouse oocyte [reviewed in (Svoboda, 2014)].
Interestingly, in one of the plant RNA silencing mechanisms, RNAi essentially serves as an amplifier of miRNA silencing where miRNA-mediated cleavage of mRNA targets is followed by RdRP-mediated production of long dsRNA, which is processed by Dicer into so-called phased siRNAs (phasiRNA). PhasiRNAs themselves are a complex small RNA category as they can be generated by different Dicers and mediate target cleavage as well as transcriptional silencing. (reviewed in (Komiya, 2017; Deng, 2018).
RNase III Dicer (reviewed in detail in [Jaskiewicz and Filipowicz, 2008; Svobodova et al., 2016)] is the enzyme producing small RNAs in canonical RNAi and miRNA pathways. Dicer is a large (~200 kDa) multidomain protein (Figure 3A). Structural and biochemical analyses (mainly in mammals but also in the protozoan Giardia intestinalis) uncovered how canonical Dicer generates small RNAs of defined length from long dsRNA substrates (Provost et al., 2002; Zhang et al., 2002; Zhang et al., 2004; Macrae et al., 2006; Macrae et al., 2007). Dicer preferentially cleaves dsRNA at the termini (Figure 3B). A dsRNA terminus is bound by the PAZ domain, which has high affinity to 3’ protruding overhangs, typical termini of canonical miRNA precursors and of processive cleavage of long dsRNA (Lingel et al., 2003; Song et al., 2003; Yan et al., 2003; Ma et al., 2004). A canonical Dicer functions as a molecular ruler defining the length of a small RNA by the distance between the PAZ domain and RNase III cleavage sites (Macrae et al., 2006). Dicer has two RNase III domains, which form a single processing center containing two catalytic “half sites” (Zhang et al., 2004; Macrae et al., 2006). Each of them cleaves one strand of the dsRNA, producing a small RNA duplex with two nucleotide 3’ overhangs and 5’ monophosphate and 3’ hydroxyl groups at the RNA termini (Zhang et al., 2004). The length of the product depends on the specific Dicer. A typical length of an animal Dicer product is 22 nucleotides although 20-22 nt siRNAs was reported for different insects (Santos et al., 2019). Giardia produces 25 nt small RNAs, plants, which utilize several Dicer paralogs (Figure 3A), produce shorter (21/22 nt) and longer (24 nt) small RNAs (Jaskiewicz and Filipowicz, 2008).
Figure 3 Dicer and production of small RNAs. (A) Domain composition of Dicer proteins in a common multicellular model system. (B) Schematic organization of Dicer and its interaction with dsRNA [based on the mammalian Dicer structure (Lau et al., 2012)]. (C) Model examples of different types of Dicer substrates and products. Production of phased small interfering RNAs (siRNAs) requires a double-stranded (dsRNA) terminus where Dicer will initiate processive cleavage. It could be produced by an RdRP, typically during viral replication. In specific cases, such as plant phased siRNAs (phasiRNA), also by a cellular RdRp [reviewed in (Komiya, 2017)]. However, not all RdRP-produced dsRNAs result in the formation of phased RNAs.
Dicer can process structurally different dsRNA substrates—e.g., small hairpins of pre-miRNAs, dsRNA with blunt ends, or dsRNA with long single-strand overhangs or loops (Figure 3C). As mentioned above, Dicer structure implies that Dicer preferentially cleaves dsRNA at the termini. However, as shown for human Dicer, it can also cleave the dsRNA stem internally, albeit with low efficiency (Provost et al., 2002; Zhang et al., 2002). The type of dsRNA processing determines the composition of a small RNA population produced from each type of the template (Figure 3C). miRNAs are precisely defined because precursors have a uniform structure and there is just a single Dicer cleavage event. Long blunt-end dsRNA, which is cleaved processively from its ends, generates phased siRNAs produced by consecutive cleavage. In this case, there may be some variability/shifts as the termini are not as precisely defined as 2nt overhangs of miRNA precursors. Dicers with low processivity, exemplified by mammalian Dicers, generate siRNAs mainly from dsRNA termini—RNAi efficiency in this case thus depends on the efficiency of the first siRNAs at the termini (Demeter et al., 2019). When Dicer cannot initiate cleavage from a terminus because it is, for example obstructed by longer overhangs, dsRNA processing is initiated by an internal cleavage; the resulting siRNA population appear random and there would be no evidence of phasing [e.g., (Tam et al., 2008; Watanabe et al., 2008)].
Loading of a small RNA onto an Argonaute protein is the key step in formation of the RNAi effector complex also known as RNA-induced silencing complex (RISC). While Argonaute proteins interact with many other proteins [reviewed in (Meister, 2013)], the minimal RNAi effector complex, the holo-RISC, is a specific Argonaute loaded with a siRNA. Loading is an important step for selecting the targeting strand and sorting small RNAs into distinct RNA silencing pathways. As shown for animal Argonautes, loading a specific strand of the small RNA duplex produced by Dicer, exhibits a thermodynamic bias where the strand whose 5′-end is less thermodynamically stable is preferentially loaded onto AGO as the guide strand (Khvorova et al., 2003; Schwarz et al., 2003). This feature is important for designing effective siRNAs for experimental repression.
Loading is assisted by a family of proteins with tandemly organized dsRNA binding domains (dsRBDs), which interact with Dicer and AGO proteins to form the RISC loading complex (RLC). Sorting through RLC varies among animal taxons. For example, C. elegans employs a single Dicer protein, but evolved an extreme diversity of Argonaute proteins among common model systems [25-27 Argonaute family members (Buck and Blaxter, 2013)]. Together with RdRPs, RNA silencing in C. elegans is a complex system of biogenesis and sorting of primary and secondary cytoplasmic and nuclear small RNAs in soma and germline (Yigit et al., 2006; Buck and Blaxter, 2013). The exo-RNAi pathway in C. elegans involves loading of AGO protein RDE-1 with primary siRNAs with the assistance of dsRBP RDE-4 (Tabara et al., 1999; Parrish and Fire, 2001; Tabara et al., 2002; Lu et al., 2005; Wilkins et al., 2005). This is followed by biogenesis of secondary siRNAs (22G RNAs) loaded on AGO protein CSR-1 (Aoki et al., 2007). C. elegans miRNAs are exclusively loaded on ALG-1/2 AGO proteins (Correa et al., 2010). Drosophila employs dedicated Dicer and Argonaute proteins for RNAi (DCR-2 and AGO2) and miRNA pathways (DCR-1 and AGO1). Loading of each AGO is assisted by two dsRBPs: R2D2 [its orthologs exist in winged insects (Dowling et al., 2016)] is coupled with the RNAi pathways and Loquacious (LOQS) primarily with the miRNA pathway; these two dsRBPs thus bridge processing of specific substrates by both Dicers and their loading onto specific AGO proteins, although the separation is not complete (Forstemann et al., 2007; Tomari et al., 2007; Okamura et al., 2008; Czech et al., 2009; Okamura et al., 2009; Ghildiyal et al., 2010). Mammals, in contrast, have minimal if any sorting of small RNAs and load them onto all four AGO proteins equally well (Meister et al., 2004; Burroughs et al., 2011; Dueck et al., 2012). This is presumably because the mammalian RNAi pathway is vestigial and the silencing machinery primarily serves the miRNA pathway.
Recognition of targets is coupled with the loaded Argonaute structure (Figures 4A, B). The human AGO2 has a bilobed composition with a central cleft for binding guide and target RNAs (Elkayam et al., 2012; Schirle and Macrae, 2012; Schirle et al., 2014; Schirle et al., 2015). AGO2 binds both ends of a siRNA. The 5’ end is buried in a pocket between MID and PIWI domains, while the 3’ end is anchored in the PAZ domain (Ma et al., 2004). The PIWI domain has an RNase H-like fold and provides the endonucleolytic “slicer” activity (Song et al., 2004; Yuan et al., 2005).
Figure 4 Argonaute protein and target repression. (A) Domain composition of human AGO2. (B) Schematic organization of domains in AGO2. Magnified is the 5’ end of a small RNA and its A-like form. (C) Division of a small RNA into five different modules as described by Wee at al. (Wee et al., 2012). (D) Schematic depiction of miRNA-like and RNAi-like silencing effects. An RNAi-like effect requires extensive sequence complementarity and AGO2.
A small RNA loaded onto an animal AGO protein has five distinct sequence modules: the anchor, seed, central, 3’ supplementary, and tail (Figure 4C) (Wee et al., 2012). The 5’ end nucleotides 2 to 6 are positioned in an A-form (Figure 4B - inset) conformation facilitating basepairing with the target (Schirle and Macrae, 2012). Structural analysis of the human AGO2 suggested a stepwise mechanism for interaction with cognate RNAs, where AGO2 exposes nucleotides 2 to 5 for initial target pairing, which then promotes conformational changes that expose nucleotides 2 to 8 and 13 to 16 for further target recognition (Schirle et al., 2014). Structural data were corroborated by kinetic data and single molecule analyses, which support the idea that different regions of the siRNA play distinct roles in the cycle of target recognition, cleavage, and product release (Haley and Zamore, 2004; Li et al., 2012; Wee et al., 2012; Zander et al., 2014; Salomon et al., 2015). The seed sequence disproportionately contributes to target RNA-binding energy, whereas base pairs formed by the central and 3’ regions of the siRNA provide a helical geometry required for catalysis (Haley and Zamore, 2004). Because of the A conformation of the seed, a loaded AGO2 exhibits kinetic properties more typical of RNA-binding proteins and does not follow the rules by which sole oligonucleotides find, bind, and dissociate from complementary nucleic acid sequences (Salomon et al., 2015). Importantly, the concept of the seed sequence is fundamental for understanding one of the main causes of off-targeting.
For the Argonaute function in RNAi, a “two-state” model was proposed (Tomari and Zamore, 2005), where the seed guides binding to the target, while pairing of the 3’ end requires dislodging of the 3’ end from the PAZ domain in order to cleave the cognate RNA. Efficient cleavage requires full complementarity in the middle of the basepaired sequence, in order to be cleaved by the PIWI domain (Figure 4B). Mismatches in the central part of the small RNA interfere with the cleavage and explain the high specificity of RNAi (i.e., endonucleolytic cleavage by the AGO2 slicer activity) (Figure 4D).
Single-molecule experiments with the loaded AGO2 showed that target binding starts at the seed region of the small RNA (Chandradoss et al., 2015; Jo et al., 2015a; Jo et al., 2015b). AGO2 initially scans for target sites with complementarity to nucleotides 2–4 of the miRNA. This initial interaction propagates into stable association when the target complementarity extends to nucleotides 2–8. The recognition process is coupled to lateral diffusion of AGO2 along the target RNA, which promotes the target search by enhancing the retention of AGO2 on the RNA (Chandradoss et al., 2015). RISC binding with the seed match can thus be established, which is consistent with the seed-match rule of miRNA target selection (Chandradoss et al., 2015; Jo et al., 2015a; Jo et al., 2015b). An important conclusion from the kinetic analysis by Wee et al. is that low-abundant miRNAs are unlikely to contribute biologically meaningful regulations, because they are present at concentrations below their KD for seed-matching targets, which are in a picomolar range (3.7 pM for mouse AGO2 and 20 pM for Drosophila AGO2) (Wee et al., 2012). Importantly, accessibility of the target for seed sequence binding is another important factor for efficient targeting. It was shown that the accessibility of the target site correlates directly with the efficiency of cleavage, recognition of inaccessible sequences is impaired because RISC does not unfold structured RNA (Ameres et al., 2007).
siRNA-mediated target recognition is highly specific. However, discrimination of RNAi between two sequences differing by a single nucleotide depends on the position and type of the mismatch (Du et al., 2005; Holen et al., 2005; Haley et al., 2010). Analysis of minimal siRNA complementarity in Drosophila showed that perfect complementarity at positions 2–17 is sufficient for RNAi (Haley et al., 2010). G:U wobble basepairs are surprisingly well tolerated; target sites containing such mismatches were silenced almost as efficiently as with full complementarity (Du et al., 2005). Tolerated can also be A:C mismatches (Du et al., 2005).
Of note is that consensus basepairing rules for functional plant miRNA-target interactions differ from those for animals: there is little tolerance of mismatches at nucleotides 2–13, with especially little tolerance of mismatches at nucleotides 9–11, and more tolerance of mismatches at nucleotides 1 and 14–21 (Wang et al., 2015). Furthermore, the perfect complementarity is not as prevalent as usually thought among plant miRNAs, as most of the identified miRNA targets in plant cells have some imperfect basepairing [summarized in (Jones-Rhoades et al., 2006)].
RdRPs can contribute to RNAi by converting single-stranded RNA to dsRNA or by synthesizing short RNAs that could be loaded onto AGO proteins. Importantly, all RdRPs identified so far seem to come from one ancestral RdRP, whose orthologs were found in plants, fungi and some animals (Cerutti and Casas-Mollano, 2006; Murphy et al., 2008). Homologs of RdRPs exist in numerous metazoan taxons, including Nematoda (e.g., Caenorhabditis elegans), Cnidaria (hydra), Chelicerata (tick), Hemichordata (acorn worm), Urochordata (sea squirt), but appear absent in the genomes of others, including Platyhelminthes (planaria), Hexapoda (Drosophila), or Craniata (vertebrates). Consequently, transitive RNAi generating secondary sequences upstream of the region targeted by siRNAs was not observed in Drosophila or mouse (Schwarz et al., 2002; Roignant et al., 2003; Stein et al., 2003). Therefore, the absence of an RdRP gene in the genome can help as an indicator of the absence of the amplification loop.
It was shown in pioneering experiments in C. elegans that RNAi can be induced by simply soaking the worm into dsRNA solution (Tabara et al., 1998) or feed it bacteria expressing dsRNA (Timmons and Fire, 1998). These spectacular effects combined two distinct phenomena: (i) environmental RNAi where cells can uptake long dsRNA or small RNAs from the environment, and (ii) systemic RNAi where silencing can spread across cellular boundaries. While both phenomena can co-exist in one species, they might be distinct because the RNAi mediator spreading across cellular boundaries can be a different RNA molecule that the original inducing RNA molecule taken up from the environment. As the biology of systemic and environmental RNAi is complex and beyond the scope of this contribution, readers can look for more details into reviews on this topic, such as (Whangbo and Hunter, 2008; Huvenne and Smagghe, 2010; Ivashuta et al., 2015).
dsRNA can be taken up via specific transmembrane channel mediated uptake (e.g., C. elegans or flower beetle) or through alternative endocytosis [e.g., in Drosophila, reviewed in more detail in (Whangbo and Hunter, 2008)]. Non-cell autonomous RNAi has been reported from parasitic nematodes (Geldhof et al., 2007), hydra (Chera et al., 2006), planaria (Newmark et al., 2003; Orii et al., 2003), some insects (Tomoyasu et al., 2008; Xu and Han, 2008), and plants (Himber et al., 2003). Some of the molecular mechanisms underlying systemic and environmental RNAi have been identified, such as dsRNA-transporting channels encoded by sid-1 and sid-2 genes (systemic RNAi-deficient), which function in systemic and environmental RNAi in C. elegans (Winston et al., 2002). Sid-1 encodes a conserved transmembrane protein that forms a dsRNA channel and has homologs (but not necessarily orthologs) in a wide range of animals, including mammals (Feinberg and Hunter, 2003; Tomoyasu et al., 2008; Shih et al., 2009; Shih and Hunter, 2011; Cappelle et al., 2016). In contrast, Sid-2, which encodes a transmembrane protein, has only been found in several Caenorhabditis species (Winston et al., 2007; Dalzell et al., 2011).
In organisms displaying environmental and systemic RNAi, delivery of dsRNA could be used to intervene or harm. This phenomenon underlies strategies for crop protection [reviewed in more detail, for example, in (Cai et al., 2018)] and further discussed in the section Horizontal Transfer of Small RNAs and RNAi Across Kingdoms.
Notably, dsRNA itself has a potential to be used directly without producing a transgenic plant – as shown, for example, by topical application of dsRNA, which protected Nicotiana benthamiana and cowpea against infection with the potyvirus bean common mosaic virus (Worrall et al., 2019) and other cases [e.g., (Konakalla et al., 2019; Namgial et al., 2019)]. On a large scale, dsRNA feeding was used, for example, to protect bees against acute paralysis virus (Hunter et al., 2010), and spraying dsRNA solution was used to protect plants against fungus Fusarium graminearum (Koch et al., 2016).
One of the frequently raised questions is how specific and selective gene targeting by RNAi is. There is not a simple answer to that question, because there are several different strategies to induce RNAi and each of them has different potential for inducing off-targeting, i.e., downregulating an unintended target. Off-targeting was typically discussed as non-specific effects within one experimental model system [e.g., (Echeverri et al., 2006; Svoboda, 2007)]. In case of RNAi-mediated pest control, off-targeting would mainly consider effects on gene expression in other species than the targeted pest. There are two possible general effects on non-targeted species: (i) RNAi (typically siRNA-based) would induce miRNA-like repression of genes whose transcripts have complementarity to the seed sequence (wrong genes silenced in wrong species), (ii) RNAi would target gene(s) with high sequence similarity to dsRNA/siRNA (right gene (or its homologs) silenced in wrong species).
In addition, off-targeting in mammalian cells was also linked with a sequence-independent interferon response induced by long dsRNA. Although it is not clear whether environmental exposure to doses of dsRNA used for pest control would induce the interferon response in humans or other mammals, it is a testable and resolvable issue.
miRNA-like off-target repression is a common off-targeting issue, particularly troubling RNAi experiments in mammalian cells, where it was shown that the off-target gene repression depends on the siRNA concentration and seed sequence (Jackson et al., 2003; Jackson et al., 2006). Several strategies have been proposed for achieving more selective RNAi in mammalian cells, including good experimental design (e.g., using the lowest effective siRNA concentration and employing specificity controls) or using RNAi-inducing agents with increased specificity—these include (i) chemical modifications eliminating activity of the “passenger”(non-targeting) siRNA strand or affecting seed pairing (Jackson et al., 2006; Chen et al., 2008; Fluiter et al., 2009; Snead et al., 2013; Seok et al., 2016), and (ii) pools of more different siRNAs with the molarity of each seed sequence proportionally diluted. Accordingly, when considering this “miRNA-like” type of off-targeting, the two key factors are the mechanism of RNAi induction and the concentration of the RNAi-inducing molecule (leaving aside additional issues like small RNA sorting into different RNA silencing pathways and a varying crosstalk between miRNA and RNAi in different organisms). In general, a long dsRNA, which is converted into a siRNA pool or a pool of chosen siRNAs, principally represents a low if any risk of miRNA-like off-targeting in contrast to a single targeting siRNA (Stein et al., 2005; Hannus et al., 2014). However, exceptions may emerge: an RNAi screen with long dsRNA in Drosophila showed that some long dsRNA sequences yielded off-targeting, which stemmed from short tandem repeat sequences in the dsRNA (Ma et al., 2006).
This issue is represented by targeting a homologous gene because of existing sequence similarity. This off-target effect is most likely to appear in closely related species in the environment treated with RNAi-based pest control. However, it is difficult to predict at which point the sequence divergence will render RNAi non-effective. As discussed above, a single nucleotide mismatch may be sufficient to prevent targeting, but this depends on the position and type of the mismatch (Du et al., 2005). Given the inhibitory effects of mismatches in the seed sequence and in the central part, 90% sequence identity with evenly distributed mismatches of an off-target homologous gene could be sufficiently diverged to lack perfect complementarity regions >17 nt. The effects on the off-target homolog would also depend on the concentration of RNAi-inducing agent; in one case in C. elegans, an 80% sequence identity of two genes yielded cross-interference, which was remedied by reducing concentration of microinjected dsRNA from 1 mg/ml to 100 μg/ml (Tabara et al., 1998). In a study of targeting a V-ATPase gene in the western corn rootworm gene with dsRNA, a silencing of its ortholog in the Colorado potato beetle (80% sequence identity) was observed but LC50 values showed a ten-fold difference in activity (Baum et al., 2007). Analysis of ten insect families in four different orders showed that the dsRNA targeting the Snf7 gene in western corn rootworm was only active in a subset of species in the Chrysomelidae family (leaf beetles) whose Snf7 genes had >90% identity with the dsRNA sequence (Bachman et al., 2013). While percentage of the sequence identity may be an arbitrary factor as the distribution of mismatches in the sequence is also important, these numbers imply that 80%–90% sequence identity is around threshold for functional RNAi.
An additional important factor is how large the off-target gene downregulation will manifest as a biologically relevant off-target phenotype. RNAi-mediated silencing of gene homologs in other species will likely be less efficient than downregulation of the desired target, because the same amount of dsRNA will produce less functional siRNAs in non-targeted species. Therefore, while off-targeting may be detectable by qPCR, it could be tolerated without adverse effects.
In 2012, a study suggested that miRNAs from ingested plants could traverse into the mammalian bloodstream and suppress genes in the liver (Zhang et al., 2012). The report received a lot of attention and spurred a major debate because of implications these data could have. We reviewed this issue in detail in the aforementioned report (Paces et al., 2017), including three problematic areas that lacked strong experimental support: (i) the mechanism of transport from the digestive system through the bloodstream to the cells, (ii) the effector complex structure, particularly its loading with single-stranded methylated plant miRNA, (iii) the targeting stoichiometry consistent with the above-mentioned picomolar range of miRNA KD. Some of the follow-up studies supported the existence of functionally relevant “xenomiRs” in humans and other mammals, while other studies questioned or rejected the idea (Paces et al., 2017). A recent survey of 824 datasets from human tissue and body fluids argues that human xenomiRs are likely artifacts (Kang et al., 2017). Among the strong arguments against biologically relevant dietary xenomiRs in humans were: the minimal fraction of xenomiRs (0.001% of host human miRNA counts), apparent batch effects of xenomiRs, no significant enrichment in sequencing data from tissues and body fluids exposed to dietary intake (e.g., liver), no significant depletion in tissues and body fluids that are relatively separated from the main bloodstream (e.g., brain and cerebro-spinal fluid), and, remarkably, the observation that the majority (81%) of body fluid xenomiRs would stem from rodents, an unlikely dietary source but common experimental material. These data argue that miRNAs from the diet are not uptaken by mammals and integrated into their miRNA pathways. At the same time, organisms with environmental and systemic RNAi can be susceptible to dietary uptake of dsRNA or small RNA. This was already shown in the pioneering RNAi experiments mentioned above – soaking in dsRNA or feeding dsRNA-expressing bacteria could suppress gene expression in C. elegans (Tabara et al., 1998; Timmons and Fire, 1998).
Consequently, trans-kingdom RNAi potential could be exploited in plants expressing dsRNA and selectively targeting RNAi-sensitive pests with an outcome of choice, e.g., repelling the pest, immobilizing it, sterilizing it (Bhatia et al., 2012), or killing it (Baum et al., 2007; Mao et al., 2007; Bhatia et al., 2012; Zhang et al., 2015; Kola et al., 2016). Processing of expressed dsRNA by plant’s RNA silencing machinery, which could reduce amount of dsRNA ingested by a pest or cause off-targeting of plant genes, can be prevented by localizing dsRNA expression into chloroplasts (Zhang et al., 2015). Given the genome sequence diversity and relatively high sequence specificity of RNAi, an RNAi-based pesticide could represent a biodegradable, highly selective pesticide with an adjustable selectivity for the pest control [reviewed, for example, in (Kunte et al., 2020)].
Every new technology brings safety concerns. If the small RNAs can spread, could an RNAi-inducing transgene in a plant or topical application of dRNA/siRNA also affect a non-targeted organisms? What could be the consequences? In principle, the off-targeting risk is inherent to the RNAi approach, but it can be monitored and significantly reduced by a proper experimental design. Furthermore, if RNAi were induced transiently (i.e., through dsRNA or siRNA), the transient nature of RNAi would allow recovery from the off-targeting within days in the species lacking an RdRP amplification loop producing secondary siRNAs. It could take longer if the off-targeting triggered transitive RNAi in the species with an RdRP and/or could induce transcriptional silencing. Transgenerational silencing [reviewed in (Rechavi and Lev, 2017)] has variable duration. In C. elegans, RNAi targeting genes expressed in the soma typically affects only the F1 progeny, although exceptional transgenerational silencing for up to 13 generations was also reported (Minkina and Hunter, 2017). Importantly, the probability of inducing a long transgenerational off-target effect in an organism other than the targeted one is negligible for dsRNA sequences with good sequence divergence from closely related species.
There is always a risk of resistance to RNAi. In the case of an RNAi-based pesticide, one could expect selection for mutations affecting RNAi efficiency rendering the RNAi-based pesticide ineffective. This could either involve accumulation of mutations within the sequence of the pest target gene (rather unlikely for long dsRNA), mutations within RNAi pathway factors of the pest (including uptake mechanisms), or evolution of bona fide RNAi suppressor proteins, which are known defense strategy against RNAi used by viruses (Roth et al., 2004; Haasnoot et al., 2007; Nayak et al., 2010).
Animals lacking RNAi may be viable and fertile, as shown in an rde-1 mutant in C. elegans (Tabara et al., 1999). In fact, wild type isolates of C. elegans vary in the RNAi response and may exhibit different degrees of resistance to RNAi (Tijsterman et al., 2002; Elvin et al., 2011; Felix et al., 2011) despite the fact that some of the mutations could make them more susceptible to infection (Felix et al., 2011). A similar scenario could be expected for pests targeted by RNAi that would acquire some mutations in the RNAi pathway. Since most mutations would be recessive, the manifestation of resistance (and strong positive selection) would require homozygosity. Evolved resistance against dsRNA was reported in western corn rootworm (Khajuria et al., 2018). It was a single locus recessive mutation resulting in impaired luminal uptake of dsRNA (Khajuria et al., 2018). Therefore, one should consider the reproduction and life cycle of the targeted pest to develop an optimal treatment regimen to reduce (or not facilitate) the probability of occurrence of homozygous RNAi pathway mutants.
RNAi offers selective gene targeting in a species-specific manner. RNAi induced by long dsRNA or unmodified siRNA offers a species-specific biodegradable pesticide. RNAi can be a particularly potent tool against pests that display environmental and systemic RNAi. The risk of potential off-targeting effects can be minimized when selecting the target and its sequence. Off-targeting effects can be monitored in closely related species and targets and, if identified, they would disappear after termination of the RNAi treatment.
The author confirms being the sole contributor of this work and has approved it for publication.
This work was supported by the Ministry of Education, Youth, and Sports project NPU1 LO1419. The content is based on a presentation given at the OECD Conference on RNAi-based Pesticides, which was sponsored by the OECD Co-operative Research Programme: Biological Resource Management for Sustainable Agricultural Systems whose financial support made it possible for the author to participate in the workshop.
The author declares that the research was conducted in the absence of any commercial or financial relationships that could be construed as a potential conflict of interest.
This manuscript summarizes PS’s contribution during the OECD Conference on RNAi-based Pesticides, which was sponsored by the OECD Co-operative Research Programme: Biological Resource Management for Sustainable Agricultural Systems whose financial support made it possible for the author to participate in the conference.
Ameres, S. L., Martinez, J., Schroeder, R. (2007). Molecular basis for target RNA recognition and cleavage by human RISC. Cell 130, 101–112. doi: 10.1016/j.cell.2007.04.037
Aoki, K., Moriguchi, H., Yoshioka, T., Okawa, K., Tabara, H. (2007). In vitro analyses of the production and activity of secondary small interfering RNAs in C. elegans. EMBO J. 26, 5007–5019. doi: 10.1038/sj.emboj.7601910
Bachman, P. M., Bolognesi, R., Moar, W. J., Mueller, G. M., Paradise, M. S., Ramaseshadri, P., et al. (2013). Characterization of the spectrum of insecticidal activity of a double-stranded RNA with targeted activity against Western Corn Rootworm (Diabrotica virgifera virgifera LeConte). Transgenic Res. 22, 1207–1222. doi: 10.1007/s11248-013-9716-5
Baum, J. A., Bogaert, T., Clinton, W., Heck, G. R., Feldmann, P., Ilagan, O., et al. (2007). Control of coleopteran insect pests through RNA interference. Nat. Biotechnol. 25, 1322–1326. doi: 10.1038/nbt1359
Bhatia, V., Bhattacharya, R., Uniyal, P. L., Singh, R., Niranjan, R. S. (2012). Host Generated siRNAs Attenuate Expression of Serine Protease Gene in Myzus persicae. PloS One 7, e46343–e46343. doi: 10.1371/journal.pone.0046343
Billi, A. C., Fischer, S. E., Kim, J. K. (2014). Endogenous RNAi pathways in C. elegans. WormBook, ed. The C. elegans Research Community, WormBook 1–49. doi: 10.1895/wormbook.1.170.1
Bologna, N. G., Voinnet, O. (2014). The diversity, biogenesis, and activities of endogenous silencing small RNAs in Arabidopsis. Annu. Rev. Plant Biol. 65, 473–503. doi: 10.1146/annurev-arplant-050213-035728
Borges, F., Martienssen, R. A. (2015). The expanding world of small RNAs in plants. Nat. Rev. Mol. Cell Biol. 16, 727–741. doi: 10.1038/nrm4085
Brodersen, P., Sakvarelidze-Achard, L., Bruun-Rasmussen, M., Dunoyer, P., Yamamoto, Y. Y., Sieburth, L., et al. (2008). Widespread translational inhibition by plant miRNAs and siRNAs. Science 320, 1185–1190. doi: 10.1126/science.1159151
Buck, A. H., Blaxter, M. (2013). Functional diversification of Argonautes in nematodes: an expanding universe. Biochem. Soc. Trans. 41, 881–886. doi: 10.1042/BST20130086
Burroughs, A. M., Ando, Y., De Hoon, M. J. L., Tomaru, Y., Suzuki, H., Hayashizaki, Y., et al. (2011). Deep-sequencing of human argonaute-associated small RNAs provides insight into miRNA sorting and reveals argonaute association with RNA fragments of diverse origin. RNA Biol. 8, 158–177. doi: 10.4161/rna.8.1.14300
Cai, Q., He, B., Kogel, K. H., Jin, H. (2018). Cross-kingdom RNA trafficking and environmental RNAi-nature’s blueprint for modern crop protection strategies. Curr. Opin. Microbiol. 46, 58–64. doi: 10.1016/j.mib.2018.02.003
Cappelle, K., De Oliveira, C. F., Van Eynde, B., Christiaens, O., Smagghe, G. (2016). The involvement of clathrin-mediated endocytosis and two Sid-1-like transmembrane proteins in double-stranded RNA uptake in the Colorado potato beetle midgut. Insect Mol. Biol. 25, 315–323. doi: 10.1111/imb.12222
Cerutti, H., Casas-Mollano, J. A. (2006). On the origin and functions of RNA-mediated silencing: from protists to man. Curr. Genet. 50, 81–99. doi: 10.1007/s00294-006-0078-x
Chandradoss, S. D., Schirle, N. T., Szczepaniak, M., Macrae, I. J., Joo, C. (2015). A Dynamic Search Process Underlies MicroRNA Targeting. Cell 162, 96–107. doi: 10.1016/j.cell.2015.06.032
Chen, P. Y., Weinmann, L., Gaidatzis, D., Pei, Y., Zavolan, M., Tuschl, T., et al. (2008). Strand-specific 5 ‘-O-methylation of siRNA duplexes controls guide strand selection and targeting specificity. Rna-a Publ. RNA Soc. 14, 263–274. doi: 10.1261/rna.789808
Chen, Y. H., Jia, X. T., Zhao, L., Li, C. Z., Zhang, S. A., Chen, Y. G., et al. (2011). Identification and functional characterization of Dicer2 and five single VWC domain proteins of Litopenaeus vannamei. Dev. Comp. Immunol. 35, 661–671. doi: 10.1016/j.dci.2011.01.010
Chera, S., De Rosa, R., Miljkovic-Licina, M., Dobretz, K., Ghila, L., Kaloulis, K., et al. (2006). Silencing of the hydra serine protease inhibitor Kazal1 gene mimics the human SPINK1 pancreatic phenotype. J. Cell Sci. 119, 846–857. doi: 10.1242/jcs.02807
Correa, R. L., Steiner, F. A., Berezikov, E., Ketting, R. F. (2010). MicroRNA-directed siRNA biogenesis in Caenorhabditis elegans. PloS Genet. 6, e1000903. doi: 10.1371/journal.pgen.1000903
Czech, B., Zhou, R., Erlich, Y., Brennecke, J., Binari, R., Villalta, C., et al. (2009). Hierarchical Rules for Argonaute Loading in Drosophila. Mol. Cell 36, 445–456. doi: 10.1016/j.molcel.2009.09.028
Dalzell, J. J., Mcveigh, P., Warnock, N. D., Mitreva, M., Bird, D. M., Abad, P., et al. (2011). RNAi effector diversity in nematodes. PloS Negl. Trop. Dis. 5, e1176. doi: 10.1371/journal.pntd.0001176
Demeter, T., Vaskovicova, M., Malik, R., Horvat, F., Pasulka, J., Svobodova, E., et al. (2019). Main constraints for RNAi induced by expressed long dsRNA in mouse cells. Life Sci. Alliance 2, 1–13. doi: 10.26508/lsa.201800289
Deng, P., Muhammad, S., Wu, L. (2018). Biogenesis and regulatory hierarchy of phased small interfering RNAs in plants. Plant Biotechnol. J. 16 (5), 965–975. doi: 10.1111/pbi.12882
Ding, S. W., Voinnet, O. (2007). Antiviral immunity directed by small RNAs. Cell 130, 413–426. doi: 10.1016/j.cell.2007.07.039
Dowling, D., Pauli, T., Donath, A., Meusemann, K., Podsiadlowski, L., Petersen, M., et al. (2016). Phylogenetic Origin and Diversification of RNAi Pathway Genes in Insects. Genome Biol. Evol. 8, 3784–3793. doi: 10.1093/gbe/evw281
Du, Q., Thonberg, H., Wang, J., Wahlestedt, C., Liang, Z. (2005). A systematic analysis of the silencing effects of an active siRNA at all single-nucleotide mismatched target sites. Nucleic Acids Res. 33, 1671–1677. doi: 10.1093/nar/gki312
Dueck, A., Ziegler, C., Eichner, A., Berezikov, E., Meister, G. (2012). microRNAs associated with the different human Argonaute proteins. Nucleic Acids Res. 40, 9850–9862. doi: 10.1093/nar/gks705
Echeverri, C. J., Beachy, P. A., Baum, B., Boutros, M., Buchholz, F., Chanda, S. K., et al. (2006). Minimizing the risk of reporting false positives in large-scale RNAi screens. Nat. Methods 3, 777–779. doi: 10.1038/nmeth1006-777
Elkayam, E., Kuhn, C. D., Tocilj, A., Haase, A. D., Greene, E. M., Hannon, G. J., et al. (2012). The Structure of Human Argonaute-2 in Complex with miR-20a. Cell 150, 100–110. doi: 10.1016/j.cell.2012.05.017
Elvin, M., Snoek, L. B., Frejno, M., Klemstein, U., Kammenga, J. E., Poulin, G. B. (2011). A fitness assay for comparing RNAi effects across multiple C. elegans genotypes. BMC Genomics 12, 510. doi: 10.1186/1471-2164-12-510
Feinberg, E. H., Hunter, C. P. (2003). Transport of dsRNA into cells by the transmembrane protein SID-1. Science 301, 1545–1547. doi: 10.1126/science.1087117
Felix, M. A., Ashe, A., Piffaretti, J., Wu, G., Nuez, I., Belicard, T., et al. (2011). Natural and experimental infection of Caenorhabditis nematodes by novel viruses related to nodaviruses. PloS Biol. 9, e1000586. doi: 10.1371/journal.pbio.1000586
Fire, A., Xu, S., Montgomery, M. K., Kostas, S. A., Driver, S. E., Mello, C. C. (1998). Potent and specific genetic interference by double-stranded RNA in Caenorhabditis elegans. Nature 391, 806–811. doi: 10.1038/35888
Fluiter, K., Mook, O. R., Baas, F. (2009). The therapeutic potential of LNA-modified siRNAs: reduction of off-target effects by chemical modification of the siRNA sequence. Methods Mol. Biol. 487, 189–203. doi: 10.1007/978-1-60327-547-7_9
Forstemann, K., Horwich, M. D., Wee, L., Tomari, Y., Zamore, P. D. (2007). Drosophila microRNAs are sorted into functionally distinct argonaute complexes after production by dicer-1. Cell 130, 287–297. doi: 10.1016/j.cell.2007.05.056
Gantier, M. P., Williams, B. R. (2007). The response of mammalian cells to double-stranded RNA. Cytokine Growth Factor Rev. 18, 363–371. doi: 10.1016/j.cytogfr.2007.06.016
Geldhof, P., Visser, A., Clark, D., Saunders, G., Britton, C., Gilleard, J., et al. (2007). RNA interference in parasitic helminths: current situation, potential pitfalls and future prospects. Parasitology 134, 609–619. doi: 10.1017/S0031182006002071
Ghildiyal, M., Xu, J., Seitz, H., Weng, Z. P., Zamore, P. D. (2010). Sorting of Drosophila small silencing RNAs partitions microRNA* strands into the RNA interference pathway. Rna-a Publ. RNA Soc. 16, 43–56. doi: 10.1261/rna.1972910
Haasnoot, J., De Vries, W., Geutjes, E. J., Prins, M., De Haan, P., Berkhout, B. (2007). The Ebola virus VP35 protein is a suppressor of RNA silencing. PloS Pathog. 3, e86. doi: 10.1371/journal.ppat.0030086
Haley, B., Zamore, P. D. (2004). Kinetic analysis of the RNAi enzyme complex. Nat. Struct. Mol. Biol. 11, 599–606. doi: 10.1038/nsmb780
Haley, B., Foys, B., Levine, M. (2010). Vectors and parameters that enhance the efficacy of RNAi-mediated gene disruption in transgenic Drosophila. Proc. Natl. Acad. Sci. U.S.A. 107, 11435–11440. doi: 10.1073/pnas.1006689107
Hannus, M., Beitzinger, M., Engelmann, J. C., Weickert, M. T., Spang, R., Hannus, S., et al. (2014). siPools: highly complex but accurately defined siRNA pools eliminate off-target effects. Nucleic Acids Res. 42, 8049–8061. doi: 10.1093/nar/gku480
Himber, C., Dunoyer, P., Moissiard, G., Ritzenthaler, C., Voinnet, O. (2003). Transitivity-dependent and -independent cell-to-cell movement of RNA silencing. EMBO J. 22, 4523–4533. doi: 10.1093/emboj/cdg431
Holen, T., Moe, S. E., Sorbo, J. G., Meza, T. J., Ottersen, O. P., Klungland, A. (2005). Tolerated wobble mutations in siRNAs decrease specificity, but can enhance activity in vivo. Nucleic Acids Res. 33, 4704–4710. doi: 10.1093/nar/gki785
Hoy, M. A., Waterhouse, R. M., Wu, K., Estep, A. S., Ioannidis, P., Palmer, W. J., et al. (2016). Genome sequencing of the phytoseiid predatory mite Metaseiulus occidentalis reveals completely atomised Hox genes and super-dynamic intron evolution. Genome Biol. Evol. 8 (6), 1762–1775. doi: 10.1093/gbe/evw048
Huang, T. Z., Zhang, X. B. (2013). Host defense against DNA virus infection in shrimp is mediated by the siRNA pathway. Eur. J. Immunol. 43, 137–146. doi: 10.1002/eji.201242806
Hunter, W., Ellis, J., Vanengelsdorp, D., Hayes, J., Westervelt, D., Glick, E., et al. (2010). Large-scale field application of RNAi technology reducing Israeli acute paralysis virus disease in honey bees (Apis mellifera, Hymenoptera: Apidae). PloS Pathog. 6, e1001160. doi: 10.1371/journal.ppat.1001160
Huvenne, H., Smagghe, G. (2010). Mechanisms of dsRNA uptake in insects and potential of RNAi for pest control: a review. J. Insect Physiol. 56, 227–235. doi: 10.1016/j.jinsphys.2009.10.004
Ivashuta, S., Zhang, Y., Wiggins, B. E., Ramaseshadri, P., Segers, G. C., Johnson, S., et al. (2015). Environmental RNAi in herbivorous insects. RNA 21, 840–850. doi: 10.1261/rna.048116.114
Jackson, A. L., Bartz, S. R., Schelter, J., Kobayashi, S. V., Burchard, J., Mao, M., et al. (2003). Expression profiling reveals off-target gene regulation by RNAi. Nat. Biotechnol. 21, 635–637. doi: 10.1038/nbt831
Jackson, A. L., Burchard, J., Schelter, J., Chau, B. N., Cleary, M., Lim, L., et al. (2006). Widespread siRNA “off-target” transcript silencing mediated by seed region sequence complementarity. Rna 12, 1179–1187. doi: 10.1261/rna.25706
Jaskiewicz, L., Filipowicz, W. (2008). Role of Dicer in posttranscriptional RNA silencing. Curr. Top. Microbiol. Immunol. 320, 77–97. doi: 10.1007/978-3-540-75157-1_4
Jo, M. H., Shin, S., Jung, S. R., Kim, E., Song, J. J., Hohng, S. (2015a). Human Argonaute 2 Has Diverse Reaction Pathways on Target RNAs. Mol. Cell 59, 117–124. doi: 10.1016/j.molcel.2015.04.027
Jo, M. H., Song, J.-J., Hohng, S. (2015b). Single-molecule fluorescence measurements reveal the reaction mechanisms of the core-RISC, composed of human Argonaute 2 and a guide RNA. Bmb Rep. 48, 643–644. doi: 10.5483/BMBRep.2015.48.12.235
Jones-Rhoades, M. W., Bartel, D. P., Bartel, B. (2006). “MicroRNAs and their regulatory roles in plants,”. Annu. Rev. Plant Biol.) 19–53. doi: 10.1146/annurev.arplant.57.032905.105218
Kang, W., Bang-Berthelsen, C. H., Holm, A., Houben, A. J., Muller, A. H., Thymann, T., et al. (2017). Survey of 800+ data sets from human tissue and body fluid reveals xenomiRs are likely artifacts. RNA 23, 433–445. doi: 10.1261/rna.059725.116
Kennedy, E. M., Whisnant, A. W., Kornepati, A. V., Marshall, J. B., Bogerd, H. P., Cullen, B. R. (2015). Production of functional small interfering RNAs by an amino-terminal deletion mutant of human Dicer. Proc. Natl. Acad. Sci. U.S.A. 112, E6945–E6954. doi: 10.1073/pnas.1513421112
Kennedy, E. M., Kornepati, A. V., Bogerd, H. P., Cullen, B. R. (2017). Partial reconstitution of the RNAi response in human cells using Drosophila gene products. RNA 23, 153–160. doi: 10.1261/rna.059345.116
Ketting, R. F. (2011). The many faces of RNAi. Dev. Cell 20, 148–161. doi: 10.1016/j.devcel.2011.01.012
Khajuria, C., Ivashuta, S., Wiggins, E., Flagel, L., Moar, W., Pleau, M., et al. (2018). Development and characterization of the first dsRNA-resistant insect population from western corn rootworm, Diabrotica virgifera virgifera LeConte. PloS One 13, e0197059. doi: 10.1371/journal.pone.0197059
Khvorova, A., Reynolds, A., Jayasena, S. D. (2003). Functional siRNAs and miRNAs exhibit strand bias. Cell 115, 209–216. doi: 10.1016/S0092-8674(03)00801-8
Kim, V. N., Han, J., Siomi, M. C. (2009). Biogenesis of small RNAs in animals. Nat. Rev. Mol. Cell Biol. 10, 126–139. doi: 10.1038/nrm2632
Koch, A., Biedenkopf, D., Furch, A., Weber, L., Rossbach, O., Abdellatef, E., et al. (2016). An RNAi-Based Control of Fusarium graminearum Infections Through Spraying of Long dsRNAs Involves a Plant Passage and Is Controlled by the Fungal Silencing Machinery. PloS Pathog. 12, e1005901. doi: 10.1371/journal.ppat.1005901
Kola, V. S., Renuka, P., Padmakumari, A. P., Mangrauthia, S. K., Balachandran, S. M., Ravindra Babu, V., et al. (2016). Silencing of CYP6 and APN Genes Affects the Growth and Development of Rice Yellow Stem Borer, Scirpophaga incertulas. Front. Physiol. 7:20. doi: 10.3389/fphys.2016.00020
Komiya, R. (2017). Biogenesis of diverse plant phasiRNAs involves an miRNA-trigger and Dicer-processing. J. Plant Res. 130, 17–23. doi: 10.1007/s10265-016-0878-0
Konakalla, N. C., Kaldis, A., Masarapu, H., Voloudakis, A. E. (2019). Topical application of double stranded RNA molecules deriving from Sesbania mosaic virus (SeMV) CP and MP genes protects Sesbania plants against SeMV. Eur. J. Plant Pathol. 155, 1345–1352. doi: 10.1007/s10658-019-01821-z
Kunte, N., Mcgraw, E., Bell, S., Held, D., Avila, L. A. (2020). Prospects, challenges and current status of RNAi through insect feeding. Pest Manag. Sci. 76, 26–41. doi: 10.1002/ps.5588
Kurscheid, S., Lew-Tabor, A. E., Valle, M. R., Bruyeres, A. G., Doogan, V. J., Munderloh, U. G., et al. (2009). Evidence of a tick RNAi pathway by comparative genomics and reverse genetics screen of targets with known loss-of-function phenotypes in Drosophila. BMC Mol. Biol. 10, 26–26. doi: 10.1186/1471-2199-10-26
Lanet, E., Delannoy, E., Sormani, R., Floris, M., Brodersen, P., Crete, P., et al. (2009). Biochemical Evidence for Translational Repression by Arabidopsis MicroRNAs. Plant Cell 21, 1762–1768. doi: 10.1105/tpc.108.063412
Lau, P. W., Guiley, K. Z., De, N., Potter, C. S., Carragher, B., Macrae, I. J. (2012). The molecular architecture of human Dicer. Nat. Struct. Mol. Biol. 19, 436–440. doi: 10.1038/nsmb.2268
Li, F., Li, P., Yang, L. M., Tang, B. (2012). Simple and sensitive fluorescence detection of the RNA endonuclease activity of mammalian argonaute2 protein based on an RNA molecular beacon. Chem. Commun. 48, 12192–12194. doi: 10.1039/c2cc36404b
Lingel, A., Simon, B., Izaurralde, E., Sattler, M. (2003). Structure and nucleic-acid binding of the Drosophila Argonaute 2 PAZ domain. Nature 426, 465–469. doi: 10.1038/nature02123
Lu, R., Maduro, M., Li, F., Li, H. W., Broitman-Maduro, G., Li, W. X., et al. (2005). Animal virus replication and RNAi-mediated antiviral silencing in Caenorhabditis elegans. Nature 436, 1040–1043. doi: 10.1038/nature03870
Ma, J. B., Ye, K., Patel, D. J. (2004). Structural basis for overhang-specific small interfering RNA recognition by the PAZ domain. Nature 429, 318–322. doi: 10.1038/nature02519
Ma, Y., Creanga, A., Lum, L., Beachy, P. A. (2006). Prevalence of off-target effects in Drosophila RNA interference screens. Nature 443, 359–363. doi: 10.1038/nature05179
Macrae, I. J., Zhou, K., Li, F., Repic, A., Brooks, A. N., Cande, W. Z., et al. (2006). Structural basis for double-stranded RNA processing by Dicer. Science 311, 195–198. doi: 10.1126/science.1121638
Macrae, I. J., Zhou, K., Doudna, J. A. (2007). Structural determinants of RNA recognition and cleavage by Dicer. Nat. Struct. Mol. Biol. 14, 934–940. doi: 10.1038/nsmb1293
Maida, Y., Masutomi, K. (2011). RNA-dependent RNA polymerases in RNA silencing. Biol. Chem. 392, 299–304. doi: 10.1515/bc.2011.035
Maillard, P. V., Van Der Veen, A. G., Deddouche-Grass, S., Rogers, N. C., Merits, A., Reis E Sousa, C. (2016). Inactivation of the type I interferon pathway reveals long double-stranded RNA-mediated RNA interference in mammalian cells. EMBO J. 35, 2505–2518. doi: 10.15252/embj.201695086
Malik, R., Svoboda, P. (2012). “Nuclear RNA silencing and related phenomena in animals,” in Toxicology and Epigenetics. Ed. Sahu, S. C. (Chichester, West Sussex, United Kingdom: John Wiley & Sons).
Mao, Y. B., Cai, W. J., Wang, J. W., Hong, G. J., Tao, X. Y., Wang, L. J., et al. (2007). Silencing a cotton bollworm P450 monooxygenase gene by plant-mediated RNAi impairs larval tolerance of gossypol. Nat. Biotechnol. 25, 1307–1313. doi: 10.1038/nbt1352
Matveyev, A. V., Alves, J. M., Serrano, M. G., Lee, V., Lara, A. M., Barton, W. A., et al. (2017). The Evolutionary Loss of RNAi Key Determinants in Kinetoplastids as a Multiple Sporadic Phenomenon. J. Mol. Evol. 84, 104–115. doi: 10.1007/s00239-017-9780-1
Mcfarlane, L., Svingen, T., Braasch, I., Koopman, P., Schartl, M., Wilhelm, D. (2011). Expansion of the Ago gene family in the teleost clade. Dev. Genes Evol. 221, 95–104. doi: 10.1007/s00427-011-0363-7
Meister, G., Landthaler, M., Patkaniowska, A., Dorsett, Y., Teng, G., Tuschl, T. (2004). Human Argonaute2 mediates RNA cleavage targeted by miRNAs and siRNAs. Mol. Cell 15, 185–197. doi: 10.1016/j.molcel.2004.07.007
Meister, G. (2013). Argonaute proteins: functional insights and emerging roles. Nat. Rev. Genet. 14, 447–459. doi: 10.1038/nrg3462
Minkina, O., Hunter, C. P. (2017). Stable Heritable Germline Silencing Directs Somatic Silencing at an Endogenous Locus. Mol. Cell 65659-670, e655. doi: 10.1016/j.molcel.2017.01.034
Moissiard, G., Parizotto, E. A., Himber, C., Voinnet, O. (2007). Transitivity in Arabidopsis can be primed, requires the redundant action of the antiviral Dicer-like 4 and Dicer-like 2, and is compromised by viral-encoded suppressor proteins. RNA 13, 1268–1278. doi: 10.1261/rna.541307
Murphy, D., Dancis, B., Brown, J. R. (2008). The evolution of core proteins involved in microRNA biogenesis. BMC Evol. Biol. 8, 92. doi: 10.1186/1471-2148-8-92
Nakayashiki, H., Kadotani, N., Mayama, S. (2006). Evolution and diversification of RNA silencing proteins in fungi. J. Mol. Evol. 63, 127–135. doi: 10.1007/s00239-005-0257-2
Namgial, T., Kaldis, A., Chakraborty, S., Voloudakis, A. (2019). Topical application of double-stranded RNA molecules containing sequences of Tomato leaf curl virus and Cucumber mosaic virus confers protection against the cognate viruses. Physiol. Mol. Plant Pathol. 108, 1–9. doi: 10.1016/j.pmpp.2019.101432
Nayak, A., Berry, B., Tassetto, M., Kunitomi, M., Acevedo, A., Deng, C., et al. (2010). Cricket paralysis virus antagonizes Argonaute 2 to modulate antiviral defense in Drosophila. Nat. Struct. Mol. Biol. 17, 547–554. doi: 10.1038/nsmb.1810
Newmark, P. A., Reddien, P. W., Cebria, F., Sanchez Alvarado, A. (2003). Ingestion of bacterially expressed double-stranded RNA inhibits gene expression in planarians. Proc. Natl. Acad. Sci. U.S.A. 100 Suppl 1, 11861–11865. doi: 10.1073/pnas.1834205100
Obbard, D. J., Gordon, K. H., Buck, A. H., Jiggins, F. M. (2009). The evolution of RNAi as a defence against viruses and transposable elements. Philos. Trans. R. Soc. Lond. B. Biol. Sci. 364, 99–115. doi: 10.1098/rstb.2008.0168
Okamura, K., Chung, W. J., Ruby, J. G., Guo, H. L., Bartel, D. P., Lai, E. C. (2008). The Drosophila hairpin RNA pathway generates endogenous short interfering RNAs. Nature 453, 803–U808. doi: 10.1038/nature07015
Okamura, K., Liu, N., Lai, E. C. (2009). Distinct Mechanisms for MicroRNA Strand Selection by Drosophila Argonautes. Mol. Cell 36, 431–444. doi: 10.1016/j.molcel.2009.09.027
Orii, H., Mochii, M., Watanabe, K. (2003). A simple “soaking method” for RNA interference in the planarian Dugesia japonica. Dev. Genes Evol. 213, 138–141. doi: 10.1007/s00427-003-0310-3
Paces, J., Nic, M., Novotny, T., Svoboda, P. (2017). Literature review of baseline information to support the risk assessment of RNAi-based GM plants. EFSA Support. Publ. 14, 1246E. doi: 10.2903/sp.efsa.2017.EN-1246
Palmer, W. J., Jiggins, F. M. (2015). Comparative Genomics Reveals the Origins and Diversity of Arthropod Immune Systems. Mol. Biol. Evol. 32, 2111–2129. doi: 10.1093/molbev/msv093
Parrish, S., Fire, A. (2001). Distinct roles for RDE-1 and RDE-4 during RNA interference in Caenorhabditis elegans. Rna 7, 1397–1402.
Provost, P., Dishart, D., Doucet, J., Frendewey, D., Samuelsson, B., Radmark, O. (2002). Ribonuclease activity and RNA binding of recombinant human Dicer. EMBO J. 21, 5864–5874. doi: 10.1093/emboj/cdf578
Rechavi, O., Lev, I. (2017). Principles of Transgenerational Small RNA Inheritance in Caenorhabditis elegans. Curr. Biol. 27, R720–R730. doi: 10.1016/j.cub.2017.05.043
Roignant, J. Y., Carre, C., Mugat, B., Szymczak, D., Lepesant, J. A., Antoniewski, C. (2003). Absence of transitive and systemic pathways allows cell-specific and isoform-specific RNAi in Drosophila. RNA 9, 299–308. doi: 10.1261/rna.2154103
Roth, B. M., Pruss, G. J., Vance, V. B. (2004). Plant viral suppressors of RNA silencing. Virus Res. 102, 97–108. doi: 10.1016/j.virusres.2004.01.020
Salomon, W. E., Jolly, S. M., Moore, M. J., Zamore, P. D., Serebrov, V. (2015). Single-Molecule Imaging Reveals that Argonaute Reshapes the Binding Properties of Its Nucleic Acid Guides. Cell 162, 84–95. doi: 10.1016/j.cell.2015.06.029
Santos, D., Mingels, L., Vogel, E., Wang, L., Christiaens, O., Cappelle, K., et al. (2019). Generation of Virus- and dsRNA-Derived siRNAs with Species-Dependent Length in Insects. Viruses 11, 1–15. doi: 10.3390/v11080738
Schirle, N. T., Macrae, I. J. (2012). The Crystal Structure of Human Argonaute2. Science 336, 1037–1040. doi: 10.1126/science.1221551
Schirle, N. T., Sheu-Gruttadauria, J., Macrae, I. J. (2014). Structural basis for microRNA targeting. Science 346, 608–613. doi: 10.1126/science.1258040
Schirle, N. T., Sheu-Gruttadauria, J., Chandradoss, S. D., Joo, C., Macrae, I. J. (2015). Water-mediated recognition of t1-adenosine anchors Argonaute2 to microRNA targets. Elife 4, 1–16. doi: 10.7554/eLife.07646
Schmitter, D., Filkowski, J., Sewer, A., Pillai, R. S., Oakeley, E. J., Zavolan, M., et al. (2006). Effects of Dicer and Argonaute down-regulation on mRNA levels in human HEK293 cells. Nucleic Acids Res. 34, 4801–4815. doi: 10.1093/nar/gkl646
Schnettler, E., Tykalova, H., Watson, M., Sharma, M., Sterken, M. G., Obbard, D. J., et al. (2014). Induction and suppression of tick cell antiviral RNAi responses by tick-borne flaviviruses. Nucleic Acids Res. 42, 9436–9446. doi: 10.1093/nar/gku657
Schwarz, D. S., Hutvagner, G., Haley, B., Zamore, P. D. (2002). Evidence that siRNAs function as guides, not primers, in the Drosophila and human RNAi pathways. Mol. Cell 10, 537–548. doi: 10.1016/S1097-2765(02)00651-2
Schwarz, D. S., Hutvagner, G., Du, T., Xu, Z., Aronin, N., Zamore, P. D. (2003). Asymmetry in the assembly of the RNAi enzyme complex. Cell 115, 199–208. doi: 10.1016/S0092-8674(03)00759-1
Seok, H., Jang, E.-S., Chi, S. W. (2016). Rationally designed siRNAs without miRNA-like off-target repression. Bmb Rep. 49, 135–136. doi: 10.5483/BMBRep.2016.49.3.019
Shih, J. D., Hunter, C. P. (2011). SID-1 is a dsRNA-selective dsRNA-gated channel. RNA 17, 1057–1065. doi: 10.1261/rna.2596511
Shih, J. D., Fitzgerald, M. C., Sutherlin, M., Hunter, C. P. (2009). The SID-1 double-stranded RNA transporter is not selective for dsRNA length. RNA 15, 384–390. doi: 10.1261/rna.1286409
Sijen, T., Fleenor, J., Simmer, F., Thijssen, K. L., Parrish, S., Timmons, L., et al. (2001). On the role of RNA amplification in dsRNA-triggered gene silencing. Cell 107, 465–476. doi: 10.1016/S0092-8674(01)00576-1
Snead, N. M., Escamilla-Powers, J. R., Rossi, J. J., Mccaffrey, A. P. (2013). 5 ‘ Unlocked Nucleic Acid Modification Improves siRNA Targeting. Mol. Therapy-Nucleic Acids 2, e103–e103. doi: 10.1038/mtna.2013.36
Song, J. J., Liu, J., Tolia, N. H., Schneiderman, J., Smith, S. K., Martienssen, R. A., et al. (2003). The crystal structure of the Argonaute2 PAZ domain reveals an RNA binding motif in RNAi effector complexes. Nat. Struct. Biol. 10, 1026–1032. doi: 10.1038/nsb1016
Song, J. J., Smith, S. K., Hannon, G. J., Joshua-Tor, L. (2004). Crystal structure of Argonaute and its implications for RISC slicer activity. Science 305, 1434–1437. doi: 10.1126/science.1102514
Stein, P., Svoboda, P., Anger, M., Schultz, R. M. (2003). RNAi: mammalian oocytes do it without RNA-dependent RNA polymerase. Rna 9, 187–192. doi: 10.1261/rna.2860603
Stein, P., Zeng, F., Pan, H., Schultz, R. M. (2005). Absence of non-specific effects of RNA interference triggered by long double-stranded RNA in mouse oocytes. Dev. Biol. 286, 464–471. doi: 10.1016/j.ydbio.2005.08.015
Su, H., Trombly, M. I., Chen, J., Wang, X. Z. (2009). Essential and overlapping functions for mammalian Argonautes in microRNA silencing. Genes Dev. 23, 304–317. doi: 10.1101/gad.1749809
Svoboda, P. (2007). Off-targeting and other non-specific effects of RNAi experiments in mammalian cells. Curr. Opin. Mol. Ther. 9, 248–257.
Svoboda, P. (2014). Renaissance of mammalian endogenous RNAi. FEBS Lett. 588, 2550–2556. doi: 10.1016/j.febslet.2014.05.030
Svobodova, E., Kubikova, J., Svoboda, P. (2016). Production of small RNAs by mammalian Dicer. Pflugers Arch. 468, 1089–1102. doi: 10.1007/s00424-016-1817-6
Tabara, H., Grishok, A., Mello, C. C. (1998). RNAi in C. elegans: soaking in the genome sequence. Science 282, 430–431. doi: 10.1126/science.282.5388.430
Tabara, H., Sarkissian, M., Kelly, W. G., Fleenor, J., Grishok, A., Timmons, L., et al. (1999). The rde-1 gene, RNA interference, and transposon silencing in C. elegans. Cell 99, 123–132. doi: 10.1016/S0092-8674(00)81644-X
Tabara, H., Yigit, E., Siomi, H., Mello, C. C. (2002). The dsRNA binding protein RDE-4 interacts with RDE-1, DCR-1, and a DExH-box helicase to direct RNAi in C. elegans. Cell 109, 861–871. doi: 10.1016/S0092-8674(02)00793-6
Tam, O. H., Aravin, A. A., Stein, P., Girard, A., Murchison, E. P., Cheloufi, S., et al. (2008). Pseudogene-derived small interfering RNAs regulate gene expression in mouse oocytes. Nature 453, 534–538. doi: 10.1038/nature06904
Tijsterman, M., Okihara, K. L., Thijssen, K., Plasterk, R. H. (2002). PPW-1, a PAZ/PIWI protein required for efficient germline RNAi, is defective in a natural isolate of C. elegans. Curr. Biol. 12, 1535–1540. doi: 10.1016/S0960-9822(02)01110-7
Timmons, L., Fire, A. (1998). Specific interference by ingested dsRNA. Nature 395, 854. doi: 10.1038/27579
Tomari, Y., Zamore, P. D. (2005). Perspective: machines for RNAi. Genes Dev. 19, 517–529. doi: 10.1101/gad.1284105
Tomari, Y., Du, T., Zamore, P. D. (2007). Sorting of Drosophila small silencing RNAs. Cell 130, 299–308. doi: 10.1016/j.cell.2007.05.057
Tomoyasu, Y., Miller, S. C., Tomita, S., Schoppmeier, M., Grossmann, D., Bucher, G. (2008). Exploring systemic RNA interference in insects: a genome-wide survey for RNAi genes in Tribolium. Genome Biol. 9, R10. doi: 10.1186/gb-2008-9-1-r10
Vaistij, F. E., Jones, L., Baulcombe, D. C. (2002). Spreading of RNA targeting and DNA methylation in RNA silencing requires transcription of the target gene and a putative RNA-dependent RNA polymerase. Plant Cell 14, 857–867. doi: 10.1105/tpc.010480
Van Der Veen, A. G., Maillard, P. V., Schmidt, J. M., Lee, S. A., Deddouche-Grass, S., Borg, A., et al. (2018). The RIG-I-like receptor LGP2 inhibits Dicer-dependent processing of long double-stranded RNA and blocks RNA interference in mammalian cells. EMBO J. 37, 1–14. doi: 10.15252/embj.201797479
Wang, F., Polydore, S., Axtell, M. J. (2015). More than meets the eye? Factors that affect target selection by plant miRNAs and heterochromatic siRNAs. Curr. Opin. Plant Biol. 27, 118–124. doi: 10.1016/j.pbi.2015.06.012
Wassenegger, M. (2005). The role of the RNAi machinery in heterochromatin formation. Cell 122, 13–16. doi: 10.1016/j.cell.2005.06.034
Watanabe, T., Totoki, Y., Toyoda, A., Kaneda, M., Kuramochi-Miyagawa, S., Obata, Y., et al. (2008). Endogenous siRNAs from naturally formed dsRNAs regulate transcripts in mouse oocytes. Nature 453, 539–543. doi: 10.1038/nature06908
Wee, L. M., Flores-Jasso, C. F., Salomon, W. E., Zamore, P. D. (2012). Argonaute Divides Its RNA Guide into Domains with Distinct Functions and RNA-Binding Properties. Cell 151, 1055–1067. doi: 10.1016/j.cell.2012.10.036
Whangbo, J. S., Hunter, C. P. (2008). Environmental RNA interference. Trends Genet. 24, 297–305. doi: 10.1016/j.tig.2008.03.007
Wilkins, C., Dishongh, R., Moore, S. C., Whitt, M. A., Chow, M., Machaca, K. (2005). RNA interference is an antiviral defence mechanism in Caenorhabditis elegans. Nature 436, 1044–1047. doi: 10.1038/nature03957
Winston, W. M., Molodowitch, C., Hunter, C. P. (2002). Systemic RNAi in C-elegans requires the putative transmembrane protein SID-1. Science 295, 2456–2459. doi: 10.1126/science.1068836
Winston, W. M., Sutherlin, M., Wright, A. J., Feinberg, E. H., Hunter, C. P. (2007). Caenorhabditis elegans SID-2 is required for environmental RNA interference. Proc. Natl. Acad. Sci. U.S.A. 104, 10565–10570. doi: 10.1073/pnas.0611282104
Worrall, E. A., Bravo-Cazar, A., Nilon, A. T., Fletcher, S. J., Robinson, K. E., Carr, J. P., et al. (2019). Exogenous Application of RNAi-Inducing Double-Stranded RNA Inhibits Aphid-Mediated Transmission of a Plant Virus. Front. Plant Sci. 10:265. doi: 10.3389/fpls.2019.00265
Xu, W., Han, Z. (2008). Cloning and phylogenetic analysis of sid-1-like genes from aphids. J. Insect Sci. 8, 1–6. doi: 10.1673/031.008.3001
Yan, K. S., Yan, S., Farooq, A., Han, A., Zeng, L., Zhou, M. M. (2003). Structure and conserved RNA binding of the PAZ domain. Nature 426, 468–474. doi: 10.1038/nature02129
Yang, L. S., Li, X. L., Jiang, S., Qiu, L. H., Zhou, F. L., Liu, W. J., et al. (2014). Characterization of Argonaute2 gene from black tiger shrimp (Penaeus monodon) and its responses to immune challenges. Fish Shellfish Immunol. 36, 261–269. doi: 10.1016/j.fsi.2013.11.010
Yekta, S., Shih, I. H., Bartel, D. P. (2004). MicroRNA-directed cleavage of HOXB8 mRNA. Science 304, 594–596. doi: 10.1126/science.1097434
Yigit, E., Batista, P. J., Bei, Y., Pang, K. M., Chen, C. C., Tolia, N. H., et al. (2006). Analysis of the C. elegans Argonaute family reveals that distinct Argonautes act sequentially during RNAi. Cell 127, 747–757. doi: 10.1016/j.cell.2006.09.033
Yuan, Y. R., Pei, Y., Ma, J. B., Kuryavyi, V., Zhadina, M., Meister, G., et al. (2005). Crystal structure of A-aeolicus Argonaute, a site-specific DNA-guided endoribonuclease, provides insights into RISC-mediated mRNA cleavage. Mol. Cell 19, 405–419. doi: 10.1016/j.molcel.2005.07.011
Zander, A., Holzmeister, P., Klose, D., Tinnefeld, P., Grohmann, D. (2014). Single-molecule FRET supports the two-state model of Argonaute action. RNA Biol. 11, 45–56. doi: 10.4161/rna.27446
Zhang, H., Kolb, F. A., Brondani, V., Billy, E., Filipowicz, W. (2002). Human Dicer preferentially cleaves dsRNAs at their termini without a requirement for ATP. EMBO J. 21, 5875–5885. doi: 10.1093/emboj/cdf582
Zhang, H., Kolb, F. A., Jaskiewicz, L., Westhof, E., Filipowicz, W. (2004). Single processing center models for human Dicer and bacterial RNase III. Cell 118, 57–68. doi: 10.1016/j.cell.2004.06.017
Zhang, L., Hou, D. X., Chen, X., Li, D. H., Zhu, L. Y., Zhang, Y. J., et al. (2012). Exogenous plant MIR168a specifically targets mammalian LDLRAP1: evidence of cross-kingdom regulation by microRNA. Cell Res. 22, 107–126. doi: 10.1038/cr.2011.158
Keywords: RNAi, dicer, argonaute, miRNA, dsRNA, off-targeting
Citation: Svoboda P (2020) Key Mechanistic Principles and Considerations Concerning RNA Interference. Front. Plant Sci. 11:1237. doi: 10.3389/fpls.2020.01237
Received: 02 September 2019; Accepted: 28 July 2020;
Published: 13 August 2020.
Edited by:
Azeddine Si Ammour, Fondazione Edmund Mach, ItalyReviewed by:
Matthew R. Willmann, Cornell University, United StatesCopyright © 2020 Svoboda. This is an open-access article distributed under the terms of the Creative Commons Attribution License (CC BY). The use, distribution or reproduction in other forums is permitted, provided the original author(s) and the copyright owner(s) are credited and that the original publication in this journal is cited, in accordance with accepted academic practice. No use, distribution or reproduction is permitted which does not comply with these terms.
*Correspondence: Petr Svoboda, c3ZvYm9kYXBAaW1nLmNhcy5jeg==
Disclaimer: All claims expressed in this article are solely those of the authors and do not necessarily represent those of their affiliated organizations, or those of the publisher, the editors and the reviewers. Any product that may be evaluated in this article or claim that may be made by its manufacturer is not guaranteed or endorsed by the publisher.
Research integrity at Frontiers
Learn more about the work of our research integrity team to safeguard the quality of each article we publish.