- 1Department of Biology, Universitat de les Illes Balears, INAGEA, Palma de Mallorca, Spain
- 2Centre for Sustainable Ecosystem Solutions, School of Earth, Atmosphere and Life Sciences, University of Wollongong, Wollongong, NSW, Australia
- 3School of Biological Sciences, The University of Adelaide, Adelaide, SA, Australia
- 4Manchester Metropolitan University, Manchester, United Kingdom
- 5Laboratorio de Ecofisiología Vegetal y Cambio Climático y Núcleo de Estudios Ambientales (NEA), Facultad de Recursos Naturales, Universidad Católica de Temuco, Temuco, Chile
- 6Facultad de Química y Biología, Universidad de Santiago de Chile, Santiago, Chile
- 7Global Challenges Program, University of Wollongong, Wollongong, NSW, Australia
The terrestrial flora of Antarctica’s frozen continent is restricted to sparse ice-free areas and dominated by lichens and bryophytes. These plants frequently battle sub-zero temperatures, extreme winds and reduced water availability; all influencing their ability to survive and grow. Antarctic mosses, however, can have canopy temperatures well above air temperature. At midday, canopy temperatures can exceed 15°C, depending on moss turf water content. In this study, the optimum temperature of photosynthesis was determined for six Antarctic moss species: Bryum pseudotriquetrum, Ceratodon purpureus, Chorisodontium aciphyllum, Polytrichastrum alpinum, Sanionia uncinata, and Schistidium antarctici collected from King George Island (maritime Antarctica) and/or the Windmill Islands, East Antarctica. Both chlorophyll fluorescence and gas exchange showed maximum values of electron transport rate occurred at canopy temperatures higher than 20°C. The optimum temperature for both net assimilation of CO2 and photoprotective heat dissipation of three East Antarctic species was 20–30°C and at temperatures below 10°C, mesophyll conductance did not significantly differ from 0. Maximum mitochondrial respiration rates occurred at temperatures higher than 35°C and were lower by around 80% at 5°C. Despite the extreme cold conditions that Antarctic mosses face over winter, the photosynthetic apparatus appears optimised to warm temperatures. Our estimation of the total carbon balance suggests that survival in this cold environment may rely on a capacity to maximize photosynthesis for brief periods during summer and minimize respiratory carbon losses in cold conditions.
Introduction
Antarctica is considered the coldest continent on Earth, since the surface air temperature can reach annual means of -23°C (-45°C in interior regions higher than 1500 m a. s. l.) (Fortuin and Oerlemans, 1990). However, outside the Antarctic circle the meteorological conditions have been reported to be relatively milder. For instance, in the South Shetlands Islands of Maritime Antarctica the daytime mean air temperatures vary between -5°C and 13°C in the summer and only reach -30°C in winter (Convey and Smith, 2005; Pearce, 2008). In these southern latitudes, terrestrial vegetation – mainly lichens and bryophytes – is restricted to ice-free areas (Peat et al., 2007; Ochyra et al., 2008). Soil surface temperatures have been recorded to be much warmer than the ~2 m air temperatures reported by meteorological stations, with maximum differences of 10.7°C (Schenker and Block, 1986), 25°C (Smith, 1996) or even 27°C (Matsuda, 1968) in summer. In winter, Antarctic mosses will normally be in a dormant state protected by a thick, insulating layer of snow.
Likewise, the microclimate of mosses has been described to be radically different from air temperature recorded in Antarctica (Longton, 1974; Walton, 1982; Edwards and Smith, 1988; Smith, 1988; Hovenden et al., 1994; Melick and Seppelt, 1994; Davey and Rothery, 1997; Green et al., 2005; Pannewitz et al., 2005; Block et al., 2009; Bramley-Alves et al., 2014; Zúñiga, 2016; Convey et al., 2018). Block et al. (2009) reported daily cycles of temperature ranging from 0°C to 44.4°C during the day and then to -2.2°C at night in the moss Andreaea regularis on a rock surface at Signy Island (60°S). Such a variation between the plant surface and air temperatures has been attributed to radiation, the angle of its incidence and wind speed in polar (Wilson, 1957) and alpine (Körner, 2003) environments. The fact that daily temperature variation is so high raises questions about the actual period during which Antarctic mosses have optimum conditions for physiological processes, such us carbon fixation.
Several researchers have reported a high optimum temperature in Antarctic mosses for both CO2 uptake (Longton, 1988b; Kappen et al., 1989; Davey and Rothery, 1997; Green et al., 2000; Pannewitz et al., 2005; Block et al., 2009) and O2 evolution (Rastorfer, 1970; Ino, 1990; Wilson, 1990; Smith, 1999; Newsham, 2010), suggesting that the studied species were not truly psychrophilic. Longton et al. (1988a; 1988b) observed high optimum temperatures for Antarctic mosses but with a broad curve resulting in a positive intercept at 0°C. In contrast, some authors have considered bryophytes to generally have lower temperature optima for photosynthesis at about 5–15°C (He et al., 2016 and references therein). Although, Ino (1990) pointed out that only mosses inhabiting locations that were frequently submersed in cold water had low optimum temperatures for photosynthesis. So far, very few researchers have addressed the effect of the high specific heat capacity of water on the maximum temperatures experienced by mosses during a daily cycle (Block et al., 2009). Water availability has been suggested to be more relevant than temperature to biology (Kennedy, 1993) and to be the main factor ruling species distribution in Antarctica (Davey and Rothery, 1997; Convey et al., 2014). However, the interactive effect that water and temperature have in providing a favourable environment for positive carbon balance in Antarctica remains unknown.
The effect of temperature on photosynthesis has been thoroughly studied (Sage and Kubien, 2007; Flexas et al., 2014; von Caemmerer and Evans, 2015). Several biochemical and biophysical processes involved in photosynthesis are affected by temperature: (1) thylakoid membrane fluidity (Hirano et al., 1981), (2) kinetics of electron transport and the Calvin-Benson cycle (Holaday et al., 1992; Sage, 2002; Walker et al., 2013), (3) stomatal aperture, and (4) CO2 diffusivity in membranes and aqueous/wall phase of mesophyll (as a sum, termed mesophyll conductance, gm). gm is one of the more relevant limiting factors of photosynthesis, especially in bryophytes, which present the lowest values of gm of the plant kingdom (Flexas et al., 2012; Carriquí et al., 2019; Gago et al., 2019). The short-term response of gm to temperature is considered species-specific (Bunce, 2008; von Caemmerer and Evans, 2015), although most of the studied species have shown a decrease of gm at low temperatures (Bernacchi et al., 2002; Warren and Dreyer, 2006; Scafaro et al., 2011; Ubierna et al., 2017; but see also Qiu et al., 2017). The response of gm to temperature, however, is unknown for bryophytes.
As a consequence of reduced photosynthetic rates at low temperatures is that the capacity to use light energy in carbon assimilation will decrease and saturation will occur at lower irradiances (Huner et al., 1993; Ensminger et al., 2006). Many photoprotection mechanisms have been described in both vascular plants and bryophytes to avoid photodamage of photosynthetic apparatus in this situation (Robinson and Waterman, 2014). One of the more short-term dynamic mechanisms of photoprotection is the regulated heat dissipation of excess energy (estimated by a chlorophyll fluorescence parameter termed non-photochemical quenching, NPQ), which is dependent on the build-up of a gradient in pH across the thylakoid membrane and has been reported to broadly increase during acclimation to low temperatures (Hendrickson et al., 2004; Míguez et al., 2015; Yang et al., 2018). To our knowledge, despite its relevance to understanding the limitations of photosynthesis at low, variable temperatures in Antarctic mosses, neither the temperature response of photoprotective heat dissipation nor gm has so far been reported for bryophytes (with the exception of unsteady-state NPQ for one Mediterranean moss species in Deltoro et al., 1999).
Thus, the objectives of this study were (1) to model the daily carbon balance of Antarctic mosses during summer based on canopy surface temperature and its effect on photosynthesis, (2) to test the interspecific differences and the possible buffering effect of water content on moss canopy temperature, and (3) to determine the temperature responses of net CO2 assimilation, electron transport rate, gm and photoprotection mechanisms assessed by NPQ.
Materials and Methods
Study Site and Plant Material
Two Antarctic locations were included in this study: Casey station (66°16′57″S, 110°31′36″E) on Bailey Peninsula (Windmill Islands region, East Antarctica) and Fildes Peninsula (62°12′05″S, 58°57′44″W) on King George Island (South Shetland Islands) (Figure 1). According to the Australian Bureau of Meteorology, mean maximum and minimum air temperature at Casey Station during the hottest month of the Antarctic summer (January) is 2.3 and -2.5°C, respectively, whereas in winter (July) mean maximum temperatures can drop to -10.8°C (data from 1989 to 2019). Fildes Peninsula in Maritime Antarctica present similar air temperatures in summer with mean max/min temperatures of 2.8/0.1°C (data from 1969–2012, for Bellingshausen Station, consistent with data reported for Frei Montalva Station by Carrasco and González, 2007).
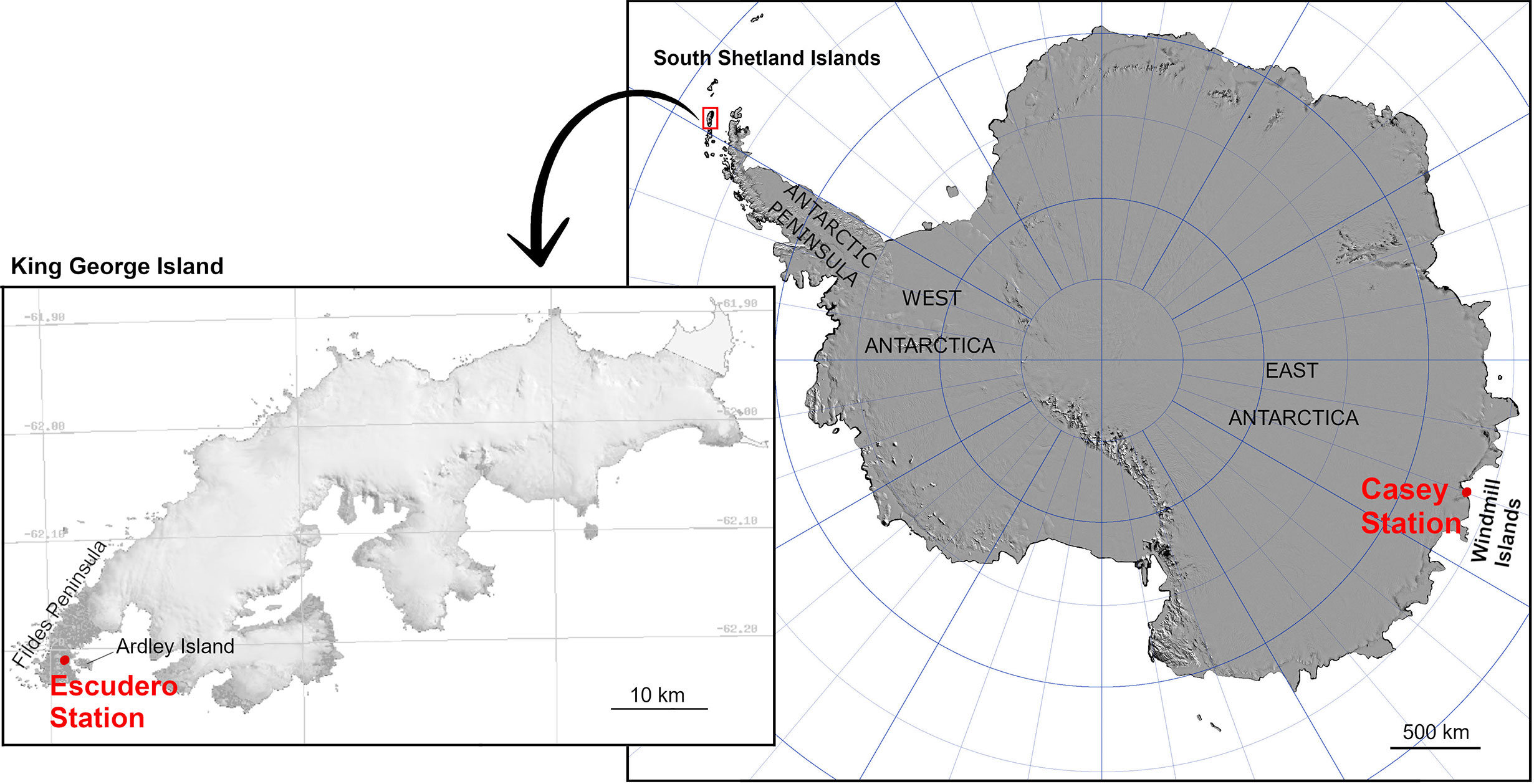
Figure 1 Location of the South Shetland Islands and Windmill Islands (Casey Station), Antarctica with inset of King George Island showing location of Escudero Station (original source: the Scientific Committee on Antarctic Research).
Six species of bryophytes were studied during different Antarctic campaigns (Table 1). Bryum pseudotriquetrum, Ceratodon purpureus and Schistidium antarctici were found on Bailey Peninsula near Casey Station (see Robinson et al., 2018 and King et al., 2020 for detailed maps and site descriptions). B. pseudotriquetrum, S. antarctici, Chorisodontium aciphyllum, Polytrichastrum alpinum and Sanionia uncinata were located at Fildes Peninsula and Ardley Island near Escudero Station. Mosses were identified to species by ACK and MJW (King George Island) and SR, JBA, and JDT (Windmill Islands). Specimen vouchers of each species were deposited in either the Janet Cosh Herbarium (University of Wollongong, Australia) or the CONC Herbarium (Universidad de Concepción, Chile).
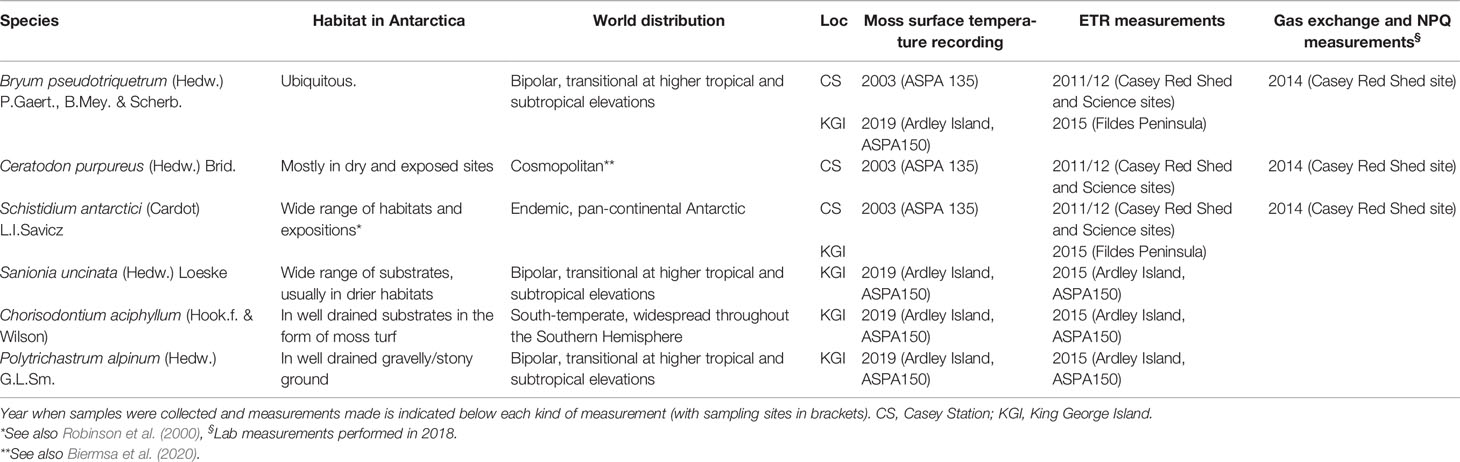
Table 1 Description of studied species with their habitat in Antarctica and phytogeography according to Ochyra et al., 2008.
Microclimatic Conditions and Moisture Effect
Daily Moss Surface Temperature Near Casey Station, East Antarctica
The surface temperature of East Antarctic B. pseudotriquetrum, C. purpureus, and S. antarctici was recorded during the Antarctic summer of 2003 (from 16/01/2003 to 28/01/2003) at six locations around Casey Station (ASPA 135). At each location a polyurethane sealed iBCod temperature sensor (Thermodata Pty. Ltd., Brisbane, Australia) was placed on the moss surface for 13 days of continuous recording. The frequency at which mosses experienced a determined interval of temperature (intervals of 2°C from -4 to 28°C) was calculated as: Time (%) = 100 · t/T, where t is the number of records of each interval of temperature and T is the total of records for each species.
For the same species and locations, additional spot measurements of photosynthetic photon flux density (PPFD), wind gust speed, moss surface temperature, and air temperature were collected at midday (local time, UTC +13) for a wider period (22 days distributed from 9/11/2002 to 01/02/2003). Moss surface temperature was measured with an infrared thermometer (Scotchtrack T Heat tracer IR1600L; 3M, Austin TX, USA). A high correlation (r = 0.914) was found between iBCod and infrared thermometer recordings for four random days when samples were measured with both sensors at midday (Supplemental Figure 1). PPFD was measured with a LS-C mini quantum sensor attached to the leaf clip holder of a Walz MINI-PAM Photosynthesis Yield Analyser (WALZ, Effeltrich, Germany) placed at moss surface level during measurement. Wind gust speed and air temperature were obtained from the Australian Bureau of Meteorology at Casey station.
Daily Moss Surface Temperature on Fildes Peninsula and Ardley Island, Maritime Antarctica
Daily air and moss surface temperature of maritime Antarctic B. pseudotriquetrum, C. aciphyllum, P. alpinum, and S. uncinata were recorded during the Antarctic summer of 2019 (from 08/01/2019 to 30/01/2019) at seven locations around Ardley Island (ASPA150). In this case, one HOBO 4-channel thermocouple datalogger (UX120-014M, Onset Computer Corporation, Bourne, MA, USA) was placed at each location, so that the moss surface temperature of three specimens and air temperature could be recorded simultaneously. The frequency with which mosses experienced a particular temperature interval was calculated as in Daily Moss Surface Temperature Near Casey Station, East Antarctica.
Environmental Moisture Effect on Moss Surface Temperature
In order to test the effect of water on the seasonal shift in moss surface temperature, 90 iBcod and iButton sensors (Maxim Integrated, San Jose, USA) were deployed across water gradients on top of cushions of S. antarctici at the Red Shed site at Casey Station. Three environments were described: wet, dry and intermediate. Wet environments were located adjacent to a meltwater stream (Figure 2); dry environments were located 2 m from the water edge, with intermediate environment located in the middle. Moss turf water content (TWC) of each environment was estimated after Lucieer et al. (2014) and King (2017) by submerging sponges within the moss turf for 24 h. Significantly different values of TWC were obtained for each environment (SupplementalFigure 2), partially published at Bramley-Alves et al. (2015). Moss surface temperatures were logged continuously from 29/11/2011 to 26/01/2012 and from 13/01/2013 to 31/01/2013. Frequencies of time at which mosses experienced a particular temperature interval was also calculated as in Daily Moss Surface Temperature Near Casey Station, East Antarctica.
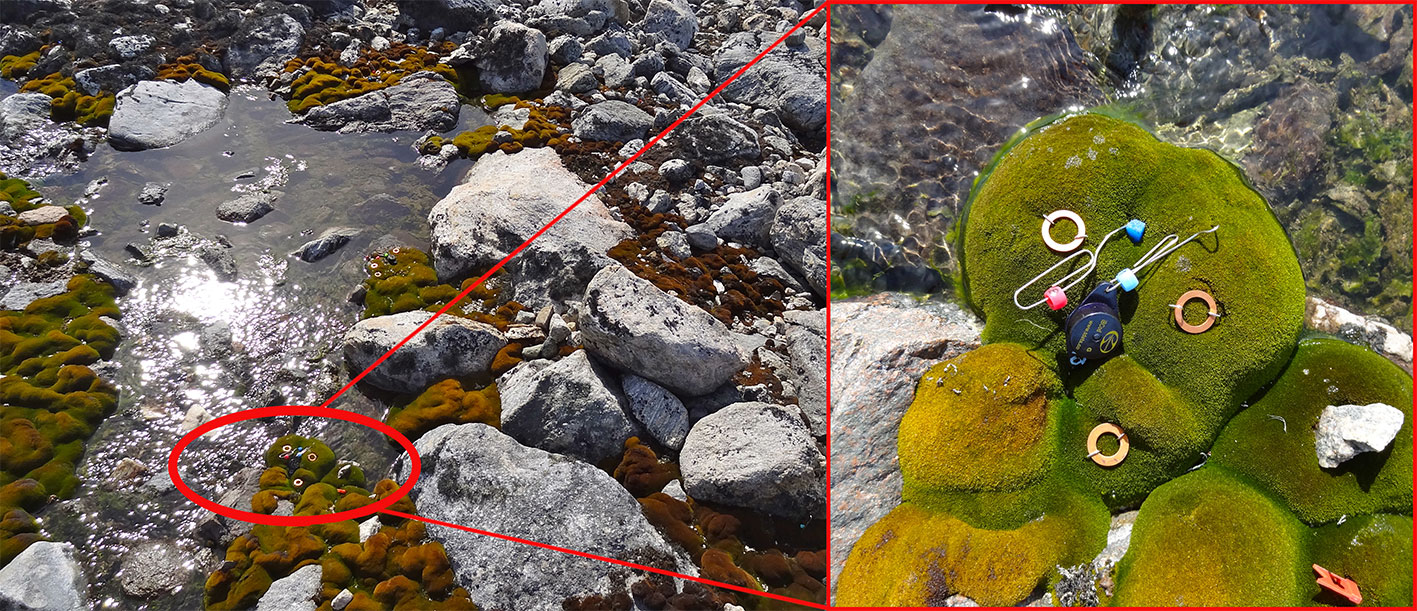
Figure 2 A typical wet site abutting a melt stream at the Red Shed site at Casey (left). Close up (right) shows iBCod sensor setup on a moss cushion.
Temperature Responses of Chlorophyll Fluorescence and Gas Exchange
Electron Transport Rate at Casey and Escudero Station
In order to determine the optimum temperatures for electron transport rate (ETR) of the moss species, measurements of chlorophyll fluorescence were performed under lab conditions with a Walz MINI-PAM Photosynthesis Yield Analyser fitted to a Walz external halogen lamp (FL 400). Fresh samples of moss tissue were collected from sunny microhabitats on Fildes Peninsula, King George Island (January 2015) and Casey Station, Antarctica (December 2011) and measured within 2 days. Moss samples were maintained under natural sunlight and temperature levels prior to measurement. Moss cushions were divided into moss plugs of 1–2 cm2 diameter and subjected randomly to temperatures from 5 to 40°C in groups of 5 replicates per temperature curve (from 4 to 28°C for Casey measurements) in the laboratory. Replicate plugs were maintained in aluminum cups in a water bath set to the target temperature and moss surface temperature were monitored. The aluminum cups allowed heat transfer within the water bath but prevented submergence and ensured the photosynthetic surface of the moss remained exposed to air. At each temperature, the specimens were pre-illuminated at PPFD of ~100 μmol photons m-2 s-1 while moss temperature equilibrated with the water temperature. They were then maintained at temperature for 5 min before a rapid light response curve was performed. Thermocouples were used to measure temperature of the photosynthetic tissue before and after each light response curve. ETR was calculated according to Krall and Edwards (1992): ETR = ϕPSII · PPFD · αβ, where ϕPSII is the yield of PSII and αβ is the product of absorbance and the partitioning of absorbed quanta between PSI and PSII. ϕPSII was calculated according to Genty et al. (1989): ϕPSII = (Fm’-Fs)/Fm’, where Fs and Fm’ are the steady-state and maximal fluorescence at light adapted conditions, respectively.
Since αβ was unknown for this set of measurements, a provisional value of 0.42 was used (Maxwell and Johnson, 2000) and was assumed to remain constant with temperature. The maximum light-saturated ETR (ETRmax) was obtained by fitting each light curve to a rational model (Smith, 1936) or to the waiting-in-line model (Ritchie, 2008) by using the Microsoft Excel Solver tool (adapted to ETR light curves from Lobo et al., 2013). The lowest square sum errors of a non-photoinhibited light curve were obtained with rational model (eqn 1), while the waiting-in-line model (eqn 2) was used for photoinhibited curves.
where AQE is the Apparent Quantum Efficiency, also fitted by the model.
Mesophyll Conductance and NPQ Measurement
In order to determine optimum temperature for CO2 assimilation (AN) at saturating light (Asat) and gm, 6-7 samples of approximately 3 cm2 of three study species – S. antarctici, C. purpureus and B. pseudotriquetrum – were collected near the Red Shed at Casey Station in 2014. Each sample was air-dried and stored at -20°C until analysis. In 2018, samples were thawed and rehydrated with distilled water for 10-16 h in dark conditions at 4°C prior to measurement under laboratory conditions. Prior to further measurements moss health was assessed by chlorophyll fluorescence, with high maximum quantum yield of PSII (Fv/Fm) indicating full recovery.
All gas exchange measurements were performed with a LiCOR 6800 system (LiCOR Biosciences, Lincoln, NE, USA). Between 40 and 47 dark-adapted sub-samples of each species were introduced into a custom-made cuvette consisting of a gasket affixed to a piece of thin polyester stocking fabric (Supplementary Figure 3). The size of these gaskets was equal to the chamber size to ensure proper closure of the chamber and achieve a minimum CO2 leakage (Supplementary Figure 4). CO2 concentration was standardized at 400 μmol CO2·mol-1 air, relative humidity at 60%–75% and the flow rate within the chamber was 700 µmol·s-1. The temperature of the chamber was varied between 5-35°C in steps of 5°C (n = 6–7 for each temperature of S. antarctici, and B. pseudotriquetrum; n = 4–5 for C. purpureus). After 5 min inside the chamber in dark conditions, dark respiration (RD) was measured and a saturating pulse was applied in order to measure basal and maximum chlorophyll fluorescence (F0 and Fm, respectively) and to calculate Fv/Fm = (Fm-F0)/Fm. Then, the sample was exposed to saturating red light, with a maximum emission at 625 nm (800 µmol·m-2·s-1, which was determined with partial light curves performed a priori). Asat at minimum saturating light was recorded at steady-state conditions, when diffusion limitations due to interstitial water were null and biochemistry was fully light-adapted (Supplementary Figure 5). At this stage, a second saturating pulse was applied to determine ϕPSII (as explained above) and non-photochemical quenching (NPQ) at each temperature, the latter calculated according to Bilger and Björkman (1990): NPQ = (Fm – Fm’)/Fm’. ETR was calculated as explained in Daily Moss Surface Temperature on Fildes Peninsula and Ardley Island, Maritime Antarctica without assuming a constant αβ. For the samples where Asat was also measured, αβ was determined as 4/slope of the relationship between ϕPSII and ϕCO2 ((AN + light respiration)/PPFD) obtained by varying PPFD under non‐photorespiratory conditions in an atmosphere containing <1% O2 (Valentini et al., 1995) (n = 3–5 light curves per temperature and species). Since the ratios ϕPSII and ϕCO2 increased significantly at higher PPFD, the αβ of the highest PPFD (the same used for measuring Asat) was chosen for calculating ETR. Light respiration (RL) was calculated from the initial light-limited portion of the low-O2-light curves as the negative intercept of the relationship between AN and (ϕPSII · PPFD)/4 according to Yin et al. (2011). In order to avoid desiccation, if required, the sample was fully rehydrated before the low-O2-light curves were measured; by immersing in distilled water for 1–2 min with excess water removed gently with a paper tissue before placing the sample back in the chamber.
gm was estimated according to Harley et al. (1992) with the modifications of Carriquí et al. (2019):
where Γ* is the chloroplastic hypothetical CO2 compensation point in the absence of respiration and Ca is the atmospheric CO2 concentration. Since stomata are absent in gametophytes of bryophytes, stomatal CO2 concentration (Ci) is substituted by Ca in Harley original formula. Γ* was calculated from the Rubisco specificity factor (SC/O) as:
SC/O was averaged from the bryophytes species reported by Font et al. (Font and Galmés, 2016). The temperature coefficient Q10 was calculated for intervals of linear gm-temperature as follow (Van’t Hoff, 1884):
The relative mesophyll (lm) and biochemical (lb) limitations to photosynthesis were calculated according to Grassi and Magnani (2005) with the modifications of Carriquí et al. (2019):
As a proxy to δA/δCc the quotient AN/Cc at 400 µmol CO2 · mol-1 air was calculated.
Estimation of Carbon Gain
Estimations of the carbon gain of East Antarctic B. pseudotriquetrum, C. purpureus, and S. antarctici were made by combining the surface temperature recorded at Casey during the summer of 2003 (see Daily Moss Surface Temperature Near Casey Station, East Antarctica) and the temperature response curves of Asat measured in lab conditions for the same species collected from the same location during the summer of 2014 (see Mesophyll Conductance and NPQ Measurement). Surface temperature values of S. antarctici at different moist environments at Casey during the summer of 2011/12 and 2013 were also analyzed. Each interval of temperature experienced by mosses was assigned a corresponding mean value of Asat (calculated from the polynomial curve fitting of the corresponding temperature response of Asat) and its contribution to the total net CO2 assimilation over the study period (Asat,T) was estimated as: Asat,T = Asat · f, where f is the frequency of time at which this interval of temperature was recorded. The balance between net carbon fixation (sum of positive Asat,T) and carbon lost (sum of negative Asat,T) during the studied period was calculated per species as:
These calculations were done by assuming that: (1) all high temperatures are experienced under high light conditions, (2) Asat at the lowest temperatures (<4°C) is negative (as a conservative worst case scenario) and similar to a ratio of RD experienced at 5°C (three scenarios were modeled based on 50%, 33.3%, 25% and 5% of RD at 5°C), and (3) the water content of the measured specimens allowed optimum gas exchange.
Statistical Analysis
All analyses were performed using the R statistical software (R Core Team, 2015). The packets used were: plyr (Wickham, 2011), ggplot2 (Wickham, 2016), nmle (Pinheiro et al., 2019), and agricolae packages (de Mendiburu, 2009).
Microclimate Data
Differences between species in microclimate data (see Daily Moss Surface Temperature Near Casey Station, East Antarctica and Daily Moss Surface Temperature on Fildes Peninsula and Ardley Island, Maritime Antarctica) were tested after logarithmic transformation of daily mean, maximum, and minimum temperatures by analysing a mixed ANOVA where localization of the sensors and date were considered as random variables and species as fixed factor. One-way ANOVA was performed to test the effect of the water content on the moss surface temperature (daily mean, maximum and minimum). The relationships between PPFD or wind gust speed and the difference between moss surface temperature and air temperature were tested by Pearson correlation test.
Chlorophyll Fluorescence and Gas Exchange Data
ETRmax/temperature curves were fitted to a 3-degree polynomial equation. Optimum temperatures for ETRmax were obtained for each sample of the studied species by determining where the 1st derivative of fitted polynomials was zero. Then, one-way ANOVA was used to test differences between species in optimum temperature for ETRmax. The effect of temperature on gas exchange derived parameters in each species was tested by two-way ANOVA. The relationships between the parameters lb, lm, αβ, and Fv/Fm with temperature were tested by Pearson correlation test.
Results
Microclimatic Conditions and Moisture Effect
Daily Moss Surface Temperature Near Casey Station, East Antarctica
Microclimate data of B. pseudotriquetrum, C. purpureus, and S. antarctici recorded during the summer of 2002/3 near Casey Station (ASPA 135) are shown in Figure 3. No significant effect of species on daily mean, maximum, and minimum temperatures was observed (P = 0.564, 0.995, and 0.795, respectively). The highest mean temperatures of the mosses surface were obtained between 10:00 and 13:00 (local time, UTC +13) and reached values around 11°C in the three species. Although maximum mean temperatures were only on average 2.3°C higher than mean surface temperatures, absolute maximum temperatures of 19, 18, and 17°C were recorded at midday for B. pseudotriquetrum, C. purpureus, and S. antarctici, respectively. At night, mean surface temperatures remained positive in the three studied species and only absolute minimum temperatures declined to -1°C. At midday, absolute daily minimum moss surface temperature was never below +4°C. During 13 days of measurements over the peak Antarctic summer (January), the mosses experienced temperatures below +4°C for 56.6% of the time. Moss temperatures exceeded 14°C for an average of just 2.5% of the time.
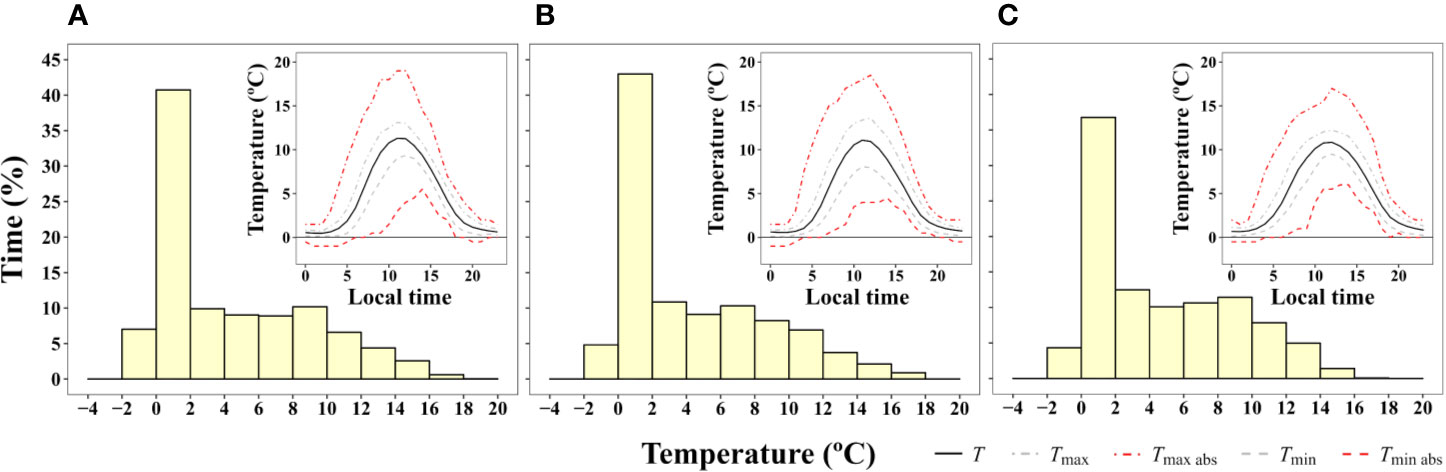
Figure 3 Frequency of time (% of hours) during which a certain two degree interval of surface temperature was experienced by (A) B. pseudotriquetrum, (B) C. purpureus, and (C) S. antarctici averaged over 13 days during the 2003 summer season (from 16/01/2003 to 28/01/2003). Moss temperature was recorded with iBcod sensors placed in moss beds at six locations within 100 m of Casey Station. Inset graph shows diurnal course of surface temperature.
The difference between moss surface temperature and air temperature at midday was predominantly driven by solar radiation (Figure 4A, P<0.05, R2 = 0.546) and in a weaker but significant way by maximum speed of wind gust (Figure 4B, P<0.05, R2 = 0.165). On clear days, when irradiation exceeded 1,000 µmol·m-2·s-1, air temperature and moss surface temperature reached a mean maximum difference of 16.2°C for all three species, with the highest absolute maximum difference recorded for C. purpureus (22.3°C).
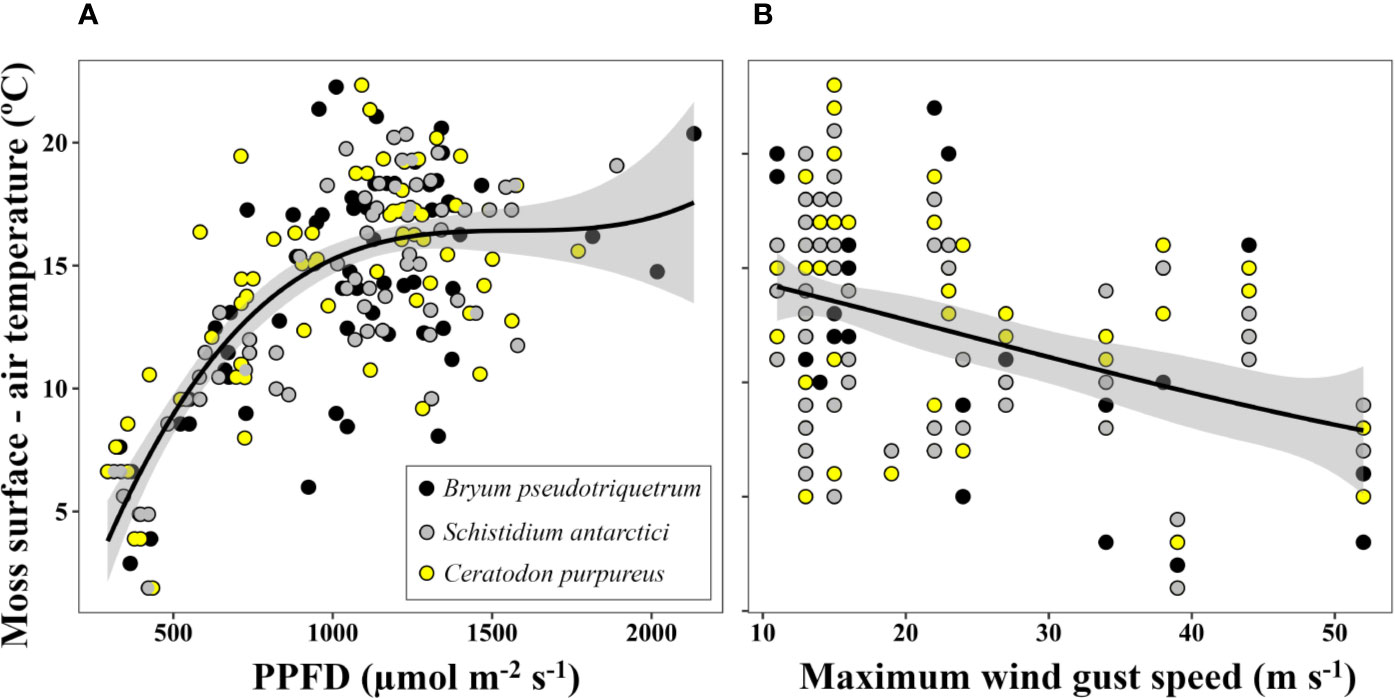
Figure 4 Relationship between the increase of moss surface temperature relative to air temperature and (A) solar photosynthetic photon flux density (PPFD) (P<0.05, R2 = 0.546 for logarithmic transformed data) and (B) daily maximum wind gust speed (P<0.05, R2 = 0.165). Lines represent quadratic polynomial fittings of temperature with their respective 95% confidence intervals (shaded areas). Moss surface temperature and PPFD were measured at 12:00 (UTC +13) with an infrared thermometer and a LS-C mini quantum sensor, respectively. Air temperature at this time and daily maximum wind gust speed were obtained from the Australian Bureau of Meteorology at Casey station. Data represent eight days of measurement within a period of 22 days (from 09/11/2002 to 01/02/2003) at three locations (one daily measurement per species and location).
Daily Moss Surface Temperature on Fildes Peninsula and Ardley Island, Maritime Antarctica
Microclimate data for the maritime Antarctic species studied during summer 2019 on Fildes Peninsula and Ardley Island are shown in Figure 5. No significant differences between species surface temperatures were found (P = 0.785, 0.461, and 0.972 for mean, maximum and minimum moss surface temperature, respectively). As in the Windmill Islands, the highest mean moss surface temperatures were obtained at midday and reached values around 8.6°C. Absolute maximum temperatures of 29.7 and 34.2°C were measured in C. aciphyllum and S. uncinata, respectively, meanwhile B. pseudotriquetrum and P. alpinum showed absolute maximums around 20.4°C. In the coldest hours of night, mean surface temperatures dropped to +0.1°C and absolute minimum temperatures declined to -3°C on average. Absolute minimum temperatures remained close to 0°C at midday. As in Windmill Islands, the studied mosses experienced temperatures below +4°C most of the time (66.7%) and moss surface temperature only exceeded 14°C for 3% of the time.
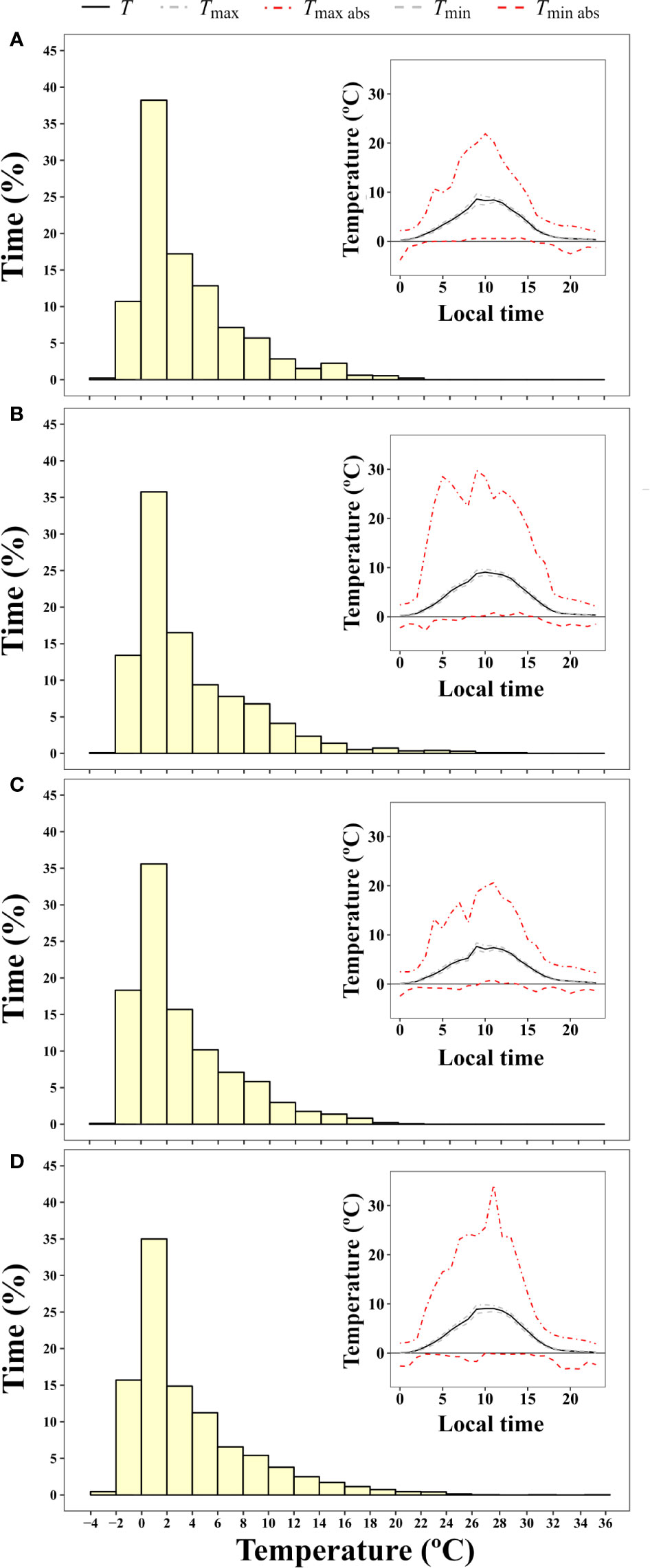
Figure 5 Frequency of time (% of hours) during which a certain two degree interval of surface temperature was experienced by (A) B. pseudotriquetrum, (B) C. aciphyllum, (C) P. alpinum, and (D) S. uncinata averaged for 20 days over the 2019 summer season (from 07/01/2019 to 29/01/2019). Thermocouple dataloggers were placed in moss beds at three to nine locations per species around Ardley Island. Inset graphs show diurnal course of surface temperature.
Environmental Moisture Effect on Moss Surface Temperature
Mean maximum and minimum surface temperature of S. antarctici were significantly affected by hydration status (Figure 6). Thus, dry canopy temperatures reached significantly higher maximum (15.0 ± 0.9 and 21.9 ± 0.9°C for data of 2011/12 and 2013, respectively) and lower minimum (-3.0 ± 0.4 and -3.1 ± 0.4°C) daily mean temperatures. While in intermediate and wet environments, extreme temperatures were more buffered. Mean temperatures were also significantly higher in dry sites during the summer of 2013 but not in the previous summer. The percentage of time when moss surface temperatures exceeded 14°C was 10.2%–19.5% for dry moss, 10.1%–4.8% for intermediate sites and only 2.4%–1.6% of time for wet sites (data not shown).
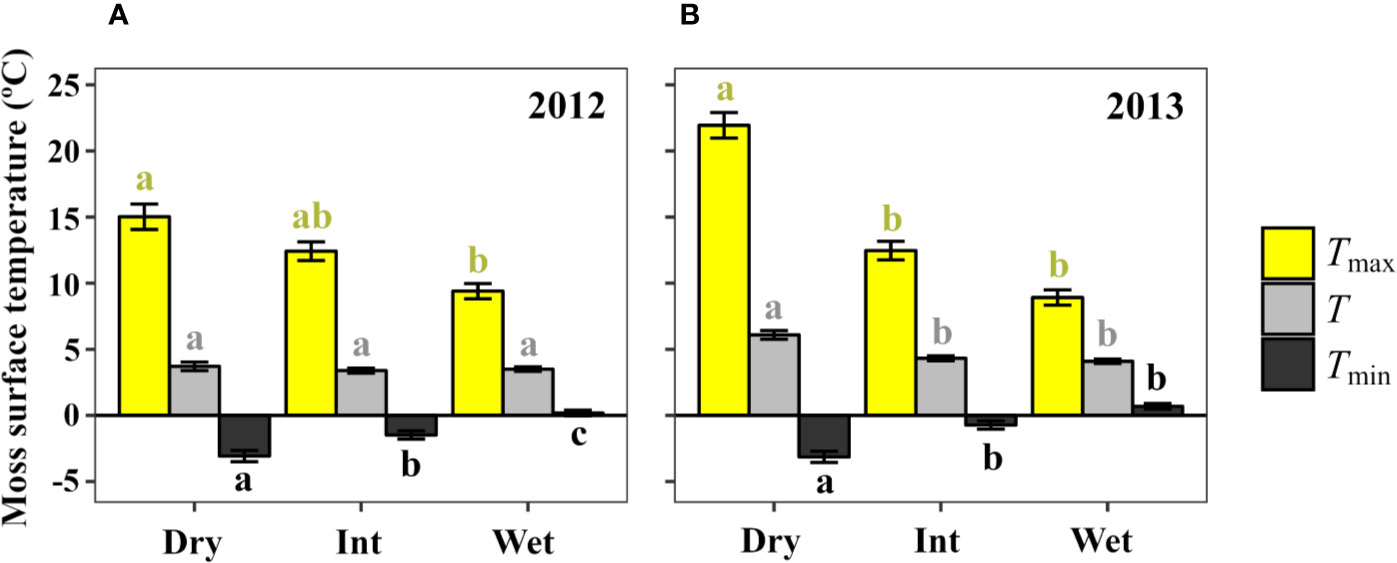
Figure 6 Daily moss surface temperature mean (T), maximum (Tmax) and minimum (Tmin) in dry, intermediate and wet environments (3 sensors each) across a S. antarctici moss bed near Casey station (East Antarctica) during two summer seasons: data from (A) 29/12/2011 to 26/01/2012 (87 days), and (B) 13/01/2013 to 31/01/2013 (54 days). Data are means ± se. Different letters denote significant differences between environments by Duncan post-hoc (P < 0.05).
Temperature Responses of Chlorophyll Fluorescence and Gas Exchange
ETR light curves at each studied temperature for species from East and maritime Antarctic locations are shown in Supplementary Figures 6 and 7. The ETRmax from rational and waiting-in-line light curve models (Supplementary Figure 8) gave maximum values at temperatures between 19–26.3°C (Table 2). The only endemic species studied, S. antarctici, had the lowest optimum temperature for ETR (19.00 ± 0.9°C), followed by C. purpureus (21.3 ± 1.9°C) a cosmopolitan species. Polytrichastrum alpinum, which is associated with polar and alpine habitats, showed the highest optimum temperature (26.3 ± 0.7°C). No significant difference was found between optimum temperatures of B. pseudotriquetrum measured at the two study sites.
Figure 7 shows the change in gas exchange and associated fluorescence parameters with temperature. The highest Asat were recorded at 25–30°C by B. pseudotriquetrum and C. purpureus, meanwhile S. antarctici showed its optimum Asat at 20–25°C (Figure 7A). Interestingly, B. pseudotriquetrum could not maintain a positive carbon balance at 5 or 10°C. RD was also strongly inhibited at low temperatures, showing reductions of around 80% at 5°C in the three studied species. Conversely maximum values were found at the highest tested temperature, 35°C (Figure 7B).
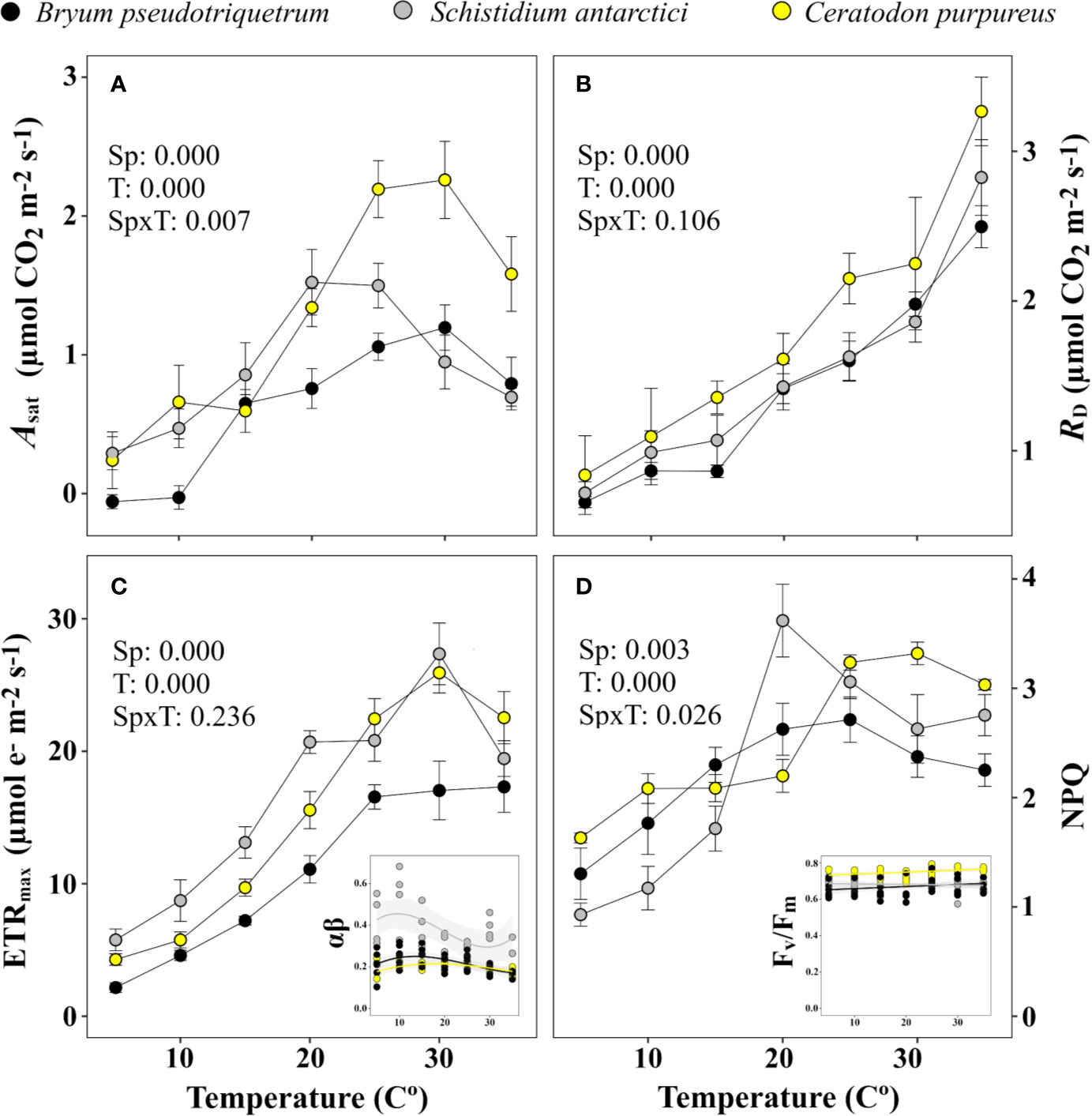
Figure 7 Temperature response curve of three East Antarctic moss species for (A) saturating net CO2 assimilation (Asat), (B) dark respiration (RD), (C) maximum electron transport rate (ETRmax), and (D) non-photochemical quenching (NPQ). Inset graph of (C) shows variation in the product of absorbance and partitioning of photons (αβ) used for calculation of ETR across temperature (R2 = 0.068, 0.063, and 0.095 for C. purpureus, S. antarctici, and B. pseudotriquetrum, respectively). Inset graph of (D) shows stability of Fv/Fm across temperature (R2 = 0.086, 0.028 and 0.046 for C. purpureus, S. antarctici and B. pseudotriquetrum, respectively). P values results from two-way ANOVA are shown in the upper-left corner of each graph. Mean ± se (n = 4-6, except for the light curve derived parameter αβ, where n = 3-5).
The optimum temperatures for electron transport were also above 25°C in the three species studied (Figure 7C). The absorbance of PSII used for calculating ETR did not vary significantly across temperature (R2 < 0.1 in all species). Maximum NPQ occurred at 20°C or higher temperatures (Figure 7D), whereas Fv/Fm varied between 0.574 and 0.794 independent of the temperatures and the species (R2 < 0.09 in all cases).
The estimated mesophyll conductance also showed maximum values at high temperatures – B. pseudotriquetrum, C. purpureus and S. antarctici showed optimum gm values at >30, 30, and 15–35°C, respectively (Figure 8). gm values at 5 and 10°C were not significantly different from zero. At low temperatures the diffusional limitation due to the mesophyll (lm) increased significantly in opposition to biochemical limitation (lb, P < 0.001 in B. pseudotriquetrum, P = 0.017 and 0.008 in S. antarctici and C. purpureus, respectively). Q10 of gm in B. pseudotriquetrum, C. purpureus, and S. antarctici were calculated as 1.38, 6.6, and 1.31, respectively (using mean values of gm between 15 and 25°C).
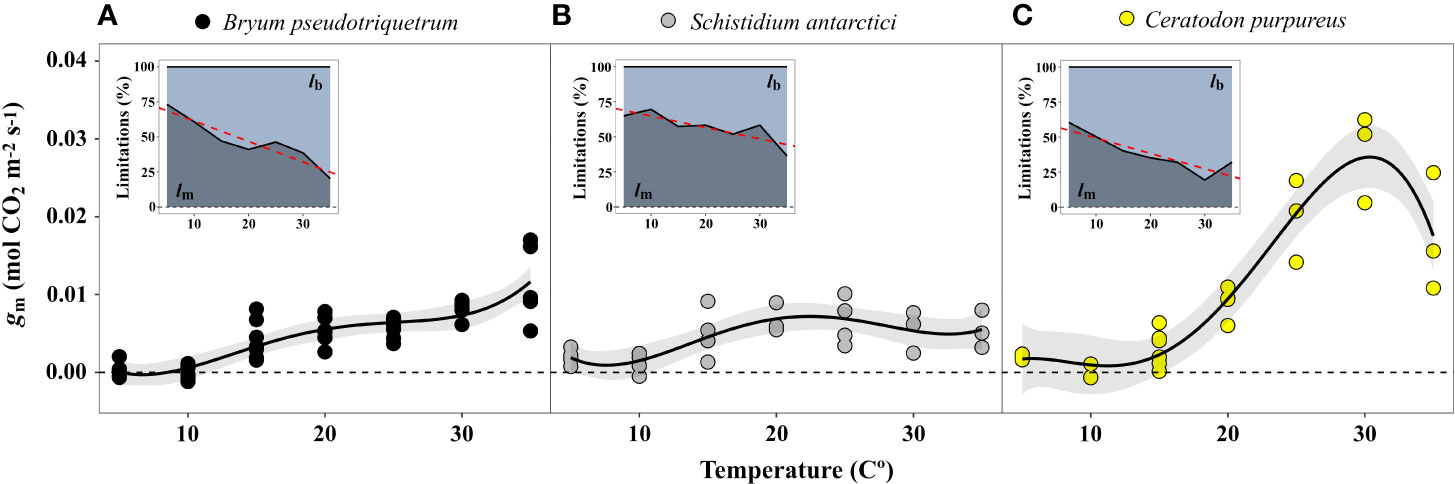
Figure 8 Temperature response of mesophyll conductance (gm) of (A) B. pseudotriquetrum, (B) S. antarctici, and (C) C. purpureus. Lines represent quadratic polynomial fittings of gm with their respective 95% confidence intervals (shaded areas, n = 3–5). Inset graphs show the temperature relationship of the percentage of biochemical (lb) vs mesophyll limitations (lm) to photosynthesis. Linear regression of lm-temperature relation is represented by a red dashed line (P < 0.05).
Estimation of Carbon Gain
Based on the temperature data from the field (Figure 3), Windmill Island moss surface temperatures only exceeded 14°C for 2.5% of the time during midsummer. However, modeling of carbon gain using temperature and gas exchange data shown in Figure 7A indicate that 36.7%, 12%, and 8.4% of total positive net CO2 was fixed during this short period in B. pseudotriquetrum, C. purpureus, and S. antarctici, respectively (data not shown). The total balance of positive and negative net CO2 exchanged (lost vs fixed) is shown in Table 3. A positive carbon balance –i.e. when carbon fixation exceeds 50% of the CO2 exchanged– was only obtained when inhibition of respiration is high for temperatures below 4°C (33.3% and 25% of the RD at 5°C in C. purpureus and S. antarctici and 5% in B. pseudotriquetrum).
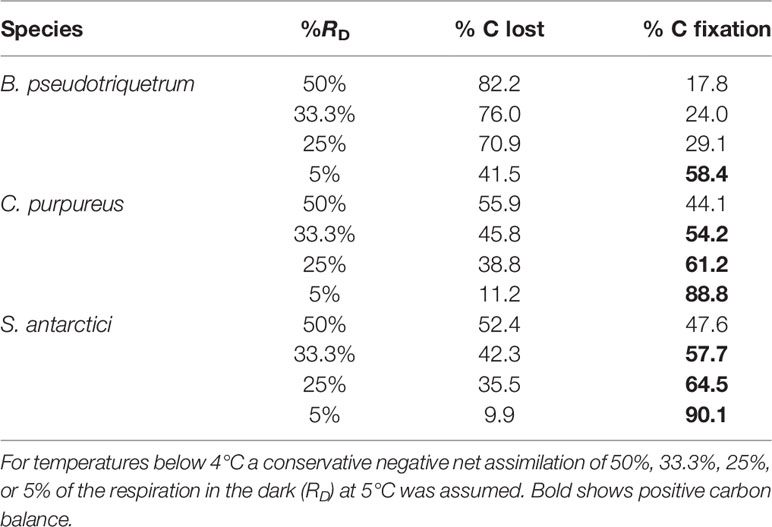
Table 3 Estimated carbon balance for three East Antarctic species calculated from surface temperature data (see Daily Moss Surface Temperature Near Casey Station, East Antarctica) and temperature response of Asat (see Mesophyll Conductance and NPQ Measurement).
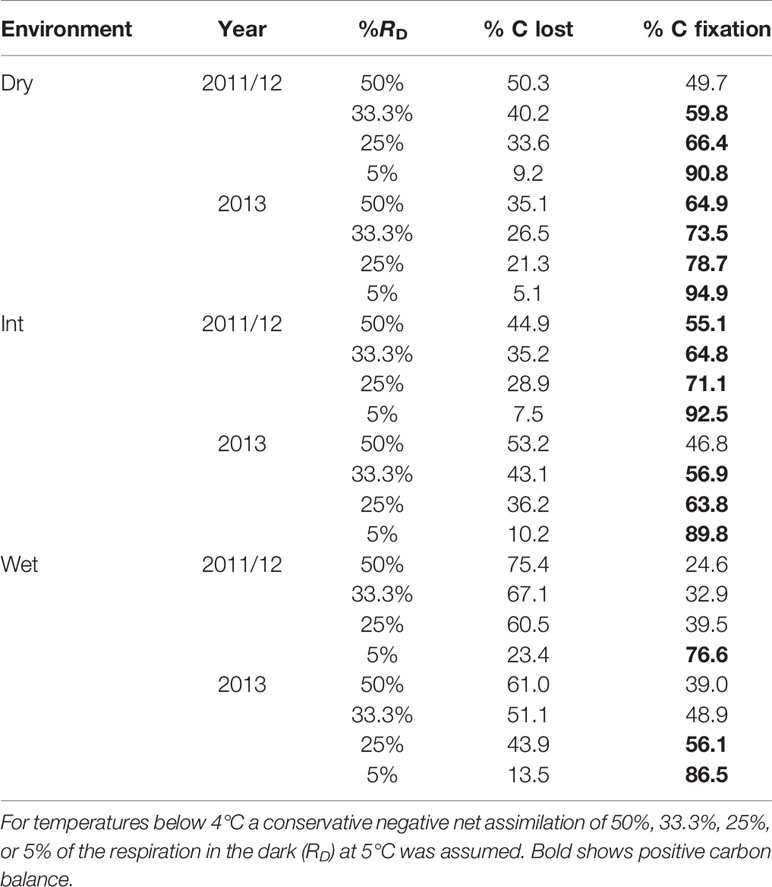
Table 4 Estimated carbon balance for East Antarctic S. antarctici calculated from surface temperature data (see Environmental Moisture Effect on Moss Surface Temperature) and temperature response of Asat (see Mesophyll Conductance and NPQ Measurement).
When carbon balance was modeled for S. antarctici across a hydrological gradient during the summer of 2011/12 and 2013 in East Antarctica (Figure 6), positive carbon balance was obtained for dry and intermediate environments under most RD scenarios (except when 50% of RD at 5°C was modeled for dry environment in 2011/12 and intermediate moist environment in 2013) (Table 4). On the contrary, the wet environments only presented positive carbon balance when the inhibition of RD was the highest modeled (5% of RD at 5°C in 2011/12 and below 25% in 2013).
Discussion
In line with previous research, our study verified that Antarctic bryophytes are not psychrophilic plants, since all the measured species presented optimum temperatures for ETRmax and Asat between 19 and 26.3°C, as has been reported for mesic and tropical bryophytes (Dilks and Proctor, 1975; Furness and Grime, 1982; Glime, 2011; Wagner et al., 2013; He et al., 2016). Despite the relationship between mean temperatures experienced during the growing season and temperature optima of net photosynthesis reported from polar, alpine, temperate, desert and tropical ecosystems (Wagner et al., 2013), high specific plasticity can be observed for Antarctic species (Table 5). The lowest optimum temperature has been found in the endemic species S. antarctici between 0 and 10°C (Kappen et al., 1989; Davey and Rothery, 1997; Block et al., 2009), although in the present study ETRmax of this species was obtained between temperatures of 19–30°C, also previously reported for O2 evolution (30°C, Wilson, 1990). High optima for photosynthesis have also been found in non-endemic species with polar distributions, such as Hennediella heimii (previously Bryum antarcticum) (19°C, Rastorfer, 1970), or species such as P. alpinum which show a moderate bipolar distribution with transitional populations in alpine environments, (Topt = 26.3°C here), but note (Topt = 10°C) in a previous study by Davey and Rothery (1997). This suggests that possessing low optimum temperatures for photosynthesis is not a strict requirement for surviving in Antarctic environments (at least until 63–64°S), even in species that restrict their distributions to these habitats (e.g., S. antarctici).
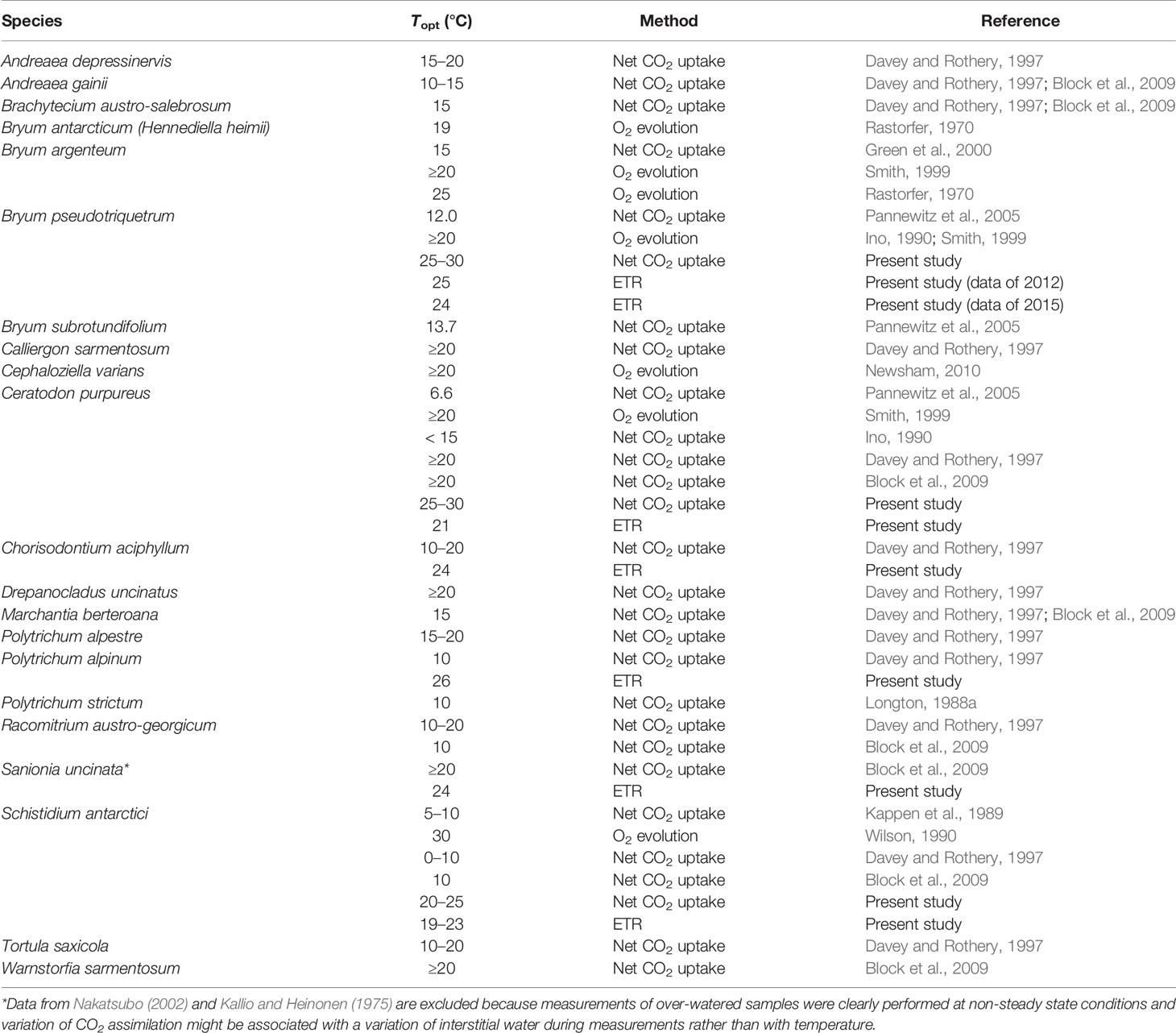
Table 5 Temperature optimum (Topt) for photosynthesis for a range of Antarctic bryophytes measured under field and laboratory conditions and as CO2 assimilation, O2 evolution, or ETR.
The fact that all Antarctic mosses measured showed such high temperature optima for photosynthesis even when summer mean maximum temperatures are much cooler (2.3°C in January at Casey Station) suggests that moss surface temperatures must regularly exceed air temperatures. This was evidenced in our microclimate analysis where the temperature experienced by mosses was measured with iBCods, infrared thermometers and/or thermocouples. Ecological researchers have pointed out the importance of a deep description of microclimate in understanding and modeling present and future species distribution and ecosystem functioning, specially in small-stature species (Convey et al., 2018; Lembrechts et al., 2019; Lembrechts and Lenoir, 2020). In our study, around midday moss surfaces were elevated above mean air temperatures by 16.2(22.3)°C (Figure 4) enhanced by high irradiation and low wind speed, as has been described for Arctic and alpine ecosystems (Wilson, 1957; Körner, 2003). However, most of the time Antarctic mosses experienced suboptimal conditions for photosynthesis (during the night or cloudy/windy days) such that their surface temperatures exceed 14°C only 2.5%–3% of the time. This is a lower percentage of time than reported by Smith (1988), where moss temperatures exceeded 20°C 24% of the time. Even so, the percentage of time at which the temperature of mosses allows a positive net CO2 assimilation must be enough to compensate for loss of carbon by respiration in order to achieve the very low growth rates (average 1.33 mm per year) reported for these Antarctic mosses (Clarke et al., 2012). In future, under climate change, Antarctic mosses are also expected to experience an increase in air temperatures and this would be expected to lead to an increase in the percentage of time they spend at optimal temperatures (Robinson et al., 2020). Our current estimation of carbon balance suggests that carbon balance can only be positive if a large reduction of carbon loss by respiration is assumed for the lowest temperatures (Figure 3). In environments with high nocturnal temperatures, such as tropical regions, bryophytes can lose more than 50% of the CO2 fixed during the daytime each night (Zotz et al., 1997). So, moss survival in Antarctica may be more related to an ability to inhibit respiration at low temperatures, rather than having lower optimum temperatures for photosynthesis.
Another factor that affects carbon gain of many bryophytes is water availability (Proctor, 1982). Both dehydration and an excessive interstitial water content can inhibit photosynthesis (Smith, 1982; Rice et al., 2011; Wagner et al., 2013; Supplementary Figure 5). Our study confirms that water content also influences the temperatures experienced by Antarctic mosses, with excess water buffering the extremes (decreasing their maximum and increasing their minimum temperatures) and, therefore, reducing the time when mosses experience temperatures higher than 14°C. This can be explained by the high specific heat capacity of water, as has been suggested previously by Pannewitz et al. (2005) and Block et al. (2009) for Antarctic mosses and by soil researchers (Campbell et al., 1995). Thus, provided the moss cells remain hydrated and the drier the interstitial environment, the wider the window for positive net CO2 assimilation, since maximum temperatures are closer to optimum for photosynthesis and minimum temperatures are enough to substantially inhibit the loss of CO2 by respiration (see Figure 4 for estimation of carbon balance). However, the optimum water content for maximum carbon gain of these Antarctic mosses is still unknown, and the interaction of limitations by both temperature and water content should be analyzed in the future.
The effect of low temperatures on photosynthesis in the Antarctic species studied here was mainly driven by diffusional limitations, rather than biochemical ones, as has been reported for Antarctic vascular plants (Sáez et al., 2018) and by other important environmental stresses such as water stress (Flexas et al., 2018; Nadal and Flexas, 2018). Variable responses of gm to temperature have been reported for vascular plants (Bernacchi et al., 2002; von Caemmerer and Evans, 2015; Xiong et al., 2015; Huang et al., 2017). In our study, B. pseudotriquetrum, S. antarctici, and C. purpureus showed increasing gm with temperature and only in the latter was a decline at supra-optimal temperatures observed. The components of mesophyll conductance that rule its response to temperature are not well understood (Shrestha et al., 2019). Since the temperature coefficient reported for CO2 diffusion in pure water (Q10 = 1.25, Jähne et al., 1987) is lower than that reported for gm in vascular plants (1.8-2.2) (Bernacchi et al., 2002; Yamori et al., 2006), physical diffusion alone cannot explain the variation of gm with temperature. Instead, it has been hypothesised that CO2 diffusion through both liquid phase (cell wall, cytosol and chloroplast stroma) and membranes (plasmic and chloroplastic, facilitated by protein transporters, i.e., aquaporins) is affected by temperature (Bernacchi et al., 2002; Evans and Von Caemmerer, 2013; Walker et al., 2013; von Caemmerer and Evans, 2015). The Q10 of C. purpureus, B. pseudotriquetrum, and S. antarctici was calculated as 6.6, 1.38, and 1.31, respectively. This suggests that the role of any facilitated process for CO2 diffusion is highly variable and is enhanced more in C. purpureus than in either the latter two species or the reported vascular plants.
At suboptimal temperatures photosynthesis in these mosses is unlikely to be able to utilise all the absorbed light producing an energy imbalance. Despite this, photoprotective heat dissipation (here estimated by NPQ) decreased when saturating light was combined with short-term exposure to suboptimal temperatures (optimum temperatures for NPQ = 20°C for S. antarctici and 25°C for C. purpureus and B. pseudotriquetrum). Lovelock et al. (1995) reported similar results for S. antarctici, which decreased NPQ (expressed as qN) after 2 h of 5–0°C. Only when temperatures were below the freezing point of -7°C (Melick and Seppelt, 1992), was de-epoxidation-independent (dithiothreitol insensitive) NPQ significantly increased, as has been observed for Antarctic lichens (Barták et al., 2007). Previous research into short-term changes of NPQ with suboptimal temperature (both cold and heat stress) in mesic and Antarctic species has reported various results including: (1) an increase in maximum steady-state NPQ (Xu et al., 1999; Hendrickson et al., 2004; Sinsawat et al., 2004; D’Ambrosio et al., 2006; Savitch et al., 2009; Sharkey and Zhang, 2010), (2) a decrease of NPQ, as in our study, (Bilger and Björkman, 1991; Fracheboud and Leipner, 2003; Corcuera et al., 2005; Lambrev et al., 2007; Pérez-Torres et al., 2007; Wang et al., 2009), or (3) invariable NPQ (Pérez-Torres et al., 2007). No correlation between de-epoxidase state or zeaxanthin concentration and NPQ at low temperatures (Xu et al., 1999; D’Ambrosio et al., 2006) and a decrease in the percentage of NPQ inhibited by dithiothreitol (Xu et al., 1999) suggest that the NPQ that is enhanced in the short-term at low temperatures could consist of zeaxanthin-independent heat dissipation (Johnson et al., 2009) and/or photoinactivated PSII reaction centre heat dissipation (Krause and Weis, 1988; Lee et al., 2001; Ivanov et al., 2003). This would be consistent with the fact that violaxanthin de-epoxidase is inhibited at low temperatures (Bilger and Björkman, 1991; Szilágyi et al., 2007) and the associated lower electron transport rates will compromise the generation of ΔpH, which is required for activation of violaxanthin de-epoxidase and inhibition of zeaxanthin epoxidase (Gilmore, 1997; Goss et al., 2008). The decrease of NPQ at low temperatures in our study could be a direct consequence of the inhibitory processes described above and not/less related with zeaxanthin-independent or photoinactivated PSII heat dissipation. Furthermore, maintenance of a constitutive zeaxanthin/lutein pool, which is a common mechanism for enhancing NPQ during cold-hardening (Haldimann et al., 1996; Leipner et al., 1997; Faria et al., 1998; Venema et al., 2000; Caffarri et al., 2005; Ivanov et al., 2006; Sáez et al., 2019; but see also Savitch et al., 2002), is less likely to be present in these short-term experiments. East Antarctic S. antarctici, C. purpureus, and B. pseudotriquetrum exhibit high zeaxanthin content in the field as a result of this cold hardening (Lovelock and Robinson, 2002). However, the presence of zeaxanthin alone without the generation of a pH gradient is insufficient to induce fluorescence quenching (Bilger and Björkman, 1991; Hurry et al., 1997; Hwang et al., 2003; Goss et al., 2008). Thus, questions remain about the photoprotection role of high zeaxanthin levels in Antarctic mosses at low temperatures, given they are not associated with the build-up of a transthylakoidal ΔpH which normally induces regulated heat dissipation. Screening and/or antioxidant roles of zeaxanthin may need to be considered (Havaux et al., 2007; Solovchenko, 2010). However, at high temperatures and high light levels, a high and sustained concentration of zeaxanthin could help to enable a rapid photoprotection response of heat dissipation at temperatures close to the photosynthetic optima.
Conclusion
We conclude that Antarctic mosses are not psychrophilic plants, since their photosynthetic optima occur at relatively high temperatures. A positive carbon gain can be maintained providing respiration is strongly inhibited at low temperatures. However, the interaction of limitations by both temperature and water require further study, since the moisture of the moss environment influences the temperatures at which they metabolize. At low temperatures, NPQ was not enhanced and the decline in photosynthesis was largely caused by an increase of diffusional limitations, which also suggests the existence of facilitated and variable processes for CO2 diffusion in these mosses.
Data Availability Statement
All data associated with this manuscript is available in Australian Antarctic Data Centre (https://data.aad.gov.au/metadata/records/AAS_4046_TempOptima_Frontiers_Perera-Castro).
Author Contributions
AP-C designed and conducted all photosynthetic experiments in the laboratory in Australia and wrote the first draft of the manuscript. MW, SR, AC-K, and GZ collected samples and conducted experiments at the Instituto Antártico Chileno Profesor Julio Escudero Station. EM, JW, JT, and JB-A collected samples and performed Windmill Island experiments. AP-C, JF, and MA performed analyses. All authors contributed to the article and approved the submitted version.
Funding
The Spanish Ministry of Education, Culture and Sport (MECD) supported pre-doctoral fellowship (FPU-02054) awarded to AP-C. Research on King George Island was supported by the Instituto Antártico Chileno (INACH Grant RT-2716 to AC-K) and the National Fund for Scientific and Technological Development (FONDECYT grant 118745 to AC-K). Research at Casey was supported by Australian Antarctic Science Grant 4046 (SR). Research was funded by ARC DP110101714 (SR) and DP180100113 (SR) and a Global Challenges Project Grant ECO-Antarctica (GC Project ID 91 to MW).
Conflict of Interest
The authors declare that the research was conducted in the absence of any commercial or financial relationships that could be construed as a potential conflict of interest.
Acknowledgments
We are grateful for Station logistic support provided at both Profesor Julio Escudero Station (INACH) and at Casey Station (Australian Antarctic Division). We also thank Dr. Elena Baraza for her help with statistical analysis.
Supplementary Material
The Supplementary Material for this article can be found online at: https://www.frontiersin.org/articles/10.3389/fpls.2020.01178/full#supplementary-material.
References
Barták, M., Váczi, P., Hájek, J., Smykla, J. (2007). Low-temperature limitation of primary photosynthetic processes in Antarctic lichens Umbilicaria antarctica and Xanthoria elegans. Polar Biol. 31, 47–51. doi: 10.1007/s00300-007-0331-x
Bernacchi, C. J., Portis, A. R., Nakano, H., Von Caemmerer, S., Long, S. P. (2002). Temperature response of mesophyll conductance. Implications for the determination of Rubisco enzyme kinetics and for limitations to photosynthesis in vivo. Plant Physiol. 130, 1992–1998. doi: 10.1104/pp.008250
Biermsa, M. E., Convey, P., Wyber, R., Robinson, S. A, Dowton, M., Vijver, B. V., et al (2020). Latitudinal biogeographic structuring in the globally distributed moss Ceratodon purpureus. Front. Plant Sci.
Bilger, W., Björkman, O. (1990). Role of the xanthophyll cycle in photoprotection elucidated by measurements of light-induced absorbance changes, fluorescence and photosynthesis in leaves of Hedera canariensis. Photosynth. Res. 25, 173–185. doi: 10.1007/BF00033159
Bilger, W., Björkman, O. (1991). Temperature dependence of violaxanthin de-epoxidation and non-photochemical fluorescence quenching in intact leaves of Gossypium hirsutum L. and Malva parviflora L. Planta 184, 226–234. doi: 10.1007/BF00197951
Block, W., Smith, R. I. L., Kennedy, A. D. (2009). Strategies of survival and resource exploitation in the Antarctic fellfield ecosystem. Biol. Rev. 84, 449–484. doi: 10.1111/j.1469-185X.2009.00084.x
Bramley-Alves, J., King, D. H., Robinson, S. A., Miller, R. E. (2014). “Dominating the Antarctic environment: bryophytes in a time of change,” in Photosynthesis in bryophytes and early land plants. Eds. Hanson, D. T., Rice, S. K. (Dordrecht: Springer), 309–324.
Bramley-Alves, J., Wanek, W., French, K., Robinson, S. A. (2015). Moss δ13C: an accurate proxy for past water environments in polar regions. Glob. Change Biol. 21, 2454–2464. doi: 10.1111/gcb.12848
Bunce, J. A. (2008). Acclimation of photosynthesis to temperature in Arabidopsis thaliana and Brassica oleracea. Photosynthetica 46, 517–524. doi: 10.1007/s11099-008-0088-7
Caffarri, S., Frigerio, S., Olivieri, E., Righetti, P. G., Bassi, R. (2005). Differential accumulation of Lhcb gene products in thylakoid membranes of Zea mays plants grown under contrasting light and temperature conditions. Proteomics 5, 758–768. doi: 10.1002/pmic.200402008
Campbell, G. S., Jungbauer, J. D., Jr., Bristow, K. L., Hungerford, R. D. (1995). Soil temperature and water content beneath a surface fire. Soil Sci. 159, 363–374. doi: 10.1097/00010694-199506000-00001
Carrasco, J., González, M. (2007). Climatología de la Península Antártica y de la base presidente Eduardo Frei Montalva (Santiago: Dirección Meteorológica de Chile).
Carriquí, M., Roig-Oliver, M., Brodribb, T. J., Coopman, R., Gill, W., Mark, K., et al. (2019). Anatomical constraints to nonstomatal diffusion conductance and photosynthesis in lycophytes and bryophytes. New Phyt. 222, 1256–1270. doi: 10.1111/nph.15675
Clarke, L. J., Robinson, S. A., Hua, Q., Ayre, D. J., Fink, D. (2012). Radiocarbon bomb spike reveals biological effects of Antarctic climate change. Global Change Biol. 18, 301–310. doi: 10.1111/j.1365-2486.2011.02560.x
Convey, P., Smith, R. I. L. (2005). “Responses of terrestrial Antarctic ecosystems to climate change,” in Plants and Climate Change. Eds. Rozema, J., Aerts, R., Cornelissen, H. (Dordrecht: Springer), 1–12.
Convey, P., Chown, S. L., Clarke, A., Barnes, D. K., Bokhorst, S., Cummings, V., et al. (2014). The spatial structure of Antarctic biodiversity. Ecol. Monogr. 84, 203–244. doi: 10.1890/12-2216.1
Convey, P., Coulson, S. J., Worland, M. R., Sjöblom, A. (2018). The importance of understanding annual and shorter-term temperature patterns and variation in the surface levels of polar soils for terrestrial biota. Polar Biol. 41, 1587–1605. doi: 10.1007/s00300-018-2299-0
Corcuera, L., Morales, F., Abadia, A., Gil-Pelegrín, E. (2005). The effect of low temperatures on the photosynthetic apparatus of Quercus ilex subsp. ballota at its lower and upper altitudinal limits in the Iberian peninsula and during a single freezing-thawing cycle. Trees 19, 99–108. doi: 10.1007/s00468-004-0368-1
Davey, M. C., Rothery, P. (1997). Interspecific variation in respiratory and photosynthetic parameters in Antarctic bryophytes. New Phyt. 137, 231–240. doi: 10.1046/j.1469-8137.1997.00805.x
de Mendiburu, F. (2009). Una herramienta de análisis estadístico para la investigación agrícola. [Thesis] (Lima: Universidad Nacional de Ingeniería).
Deltoro, V. I., Calatayud, Á., Morales, F., Abadía, A., Barreno, E. (1999). Changes in net photosynthesis, chlorophyll fluorescence and xanthophyll cycle interconversions during freeze-thaw cycles in the Mediterranean moss Leucodon sciuroides. Oecologia 120, 499–505. doi: 10.1007/s004420050883
Dilks, T. J. K., Proctor, M. C. F. (1975). Comparative experiments on temperature responses of bryophytes: assimilation, respiration and freezing damage. J. Bryol. 8, 317–336. doi: 10.1179/jbr.1975.8.3.317
D’Ambrosio, N., Arena, C., De Santo, A. V. (2006). Temperature response of photosynthesis, excitation energy dissipation and alternative electron sinks to carbon assimilation in Beta vulgaris L. Environ. Exp. Bot. 55, 248–257. doi: 10.1016/j.envexpbot.2004.11.006
Edwards, J. A., Smith, R. I. (1988). Photosynthesis and respiration of Colobanthus quitensis and Deschampsia antarctica from the maritime Antarctic. Brit. Antarct. Surv. B. 81, 43–63.
Ensminger, I., Busch, F., Huner, N. P. (2006). Photostasis and cold acclimation: sensing low temperature through photosynthesis. Physiol. Plantarum 126, 28–44. doi: 10.1111/j.1399-3054.2006.00627.x
Evans, J. R., Von Caemmerer, S. (2013). Temperature response of carbon isotope discrimination and mesophyll conductance in tobacco. Plant Cell Environ. 36, 745–756. doi: 10.1111/j.1365-3040.2012.02591.x
Faria, T., Silvério, D., Breia, E., Cabral, R., Abadia, A., Abadia, J., et al. (1998). Differences in the response of carbon assimilation to summer stress (water deficits, high light and temperature) in four Mediterranean tree species. Physiol. Plantarum 102, 419–428. doi: 10.1034/j.1399-3054.1998.1020310.x
Flexas, J., Barbour, M. M., Brendel, O., Cabrera, H. M., Carriquí, M., Diaz-Espejo, A., et al. (2012). Mesophyll diffusion conductance to CO2: an unappreciated central player in photosynthesis. Plant Sci. 193, 70–84. doi: 10.1016/j.plantsci.2012.05.009
Flexas, J., Diaz-Espejo, A., Gago, J., Gallé, A., Galmés, J., Gulías, J., et al. (2014). Photosynthetic limitations in Mediterranean plants: a review. Environ. Exp. Bot. 103, 12–23. doi: 10.1016/j.envexpbot.2013.09.002
Flexas, J., Carriquí, M., Nadal, M. (2018). Gas exchange and hydraulics during drought in crops: who drives whom? J. Exp. Bot. 69, 3791–3795. doi: 10.1093/jxb/ery235
Font, M., Galmés, J. (2016). Temperature response of in vitro Rubisco kinetics in bryophytes and ferns. [Poster]. 17th International Congress on Photosynthesis Research, Maastricht.
Fortuin, J. P. F., Oerlemans, J. (1990). Parameterization of the annual surface temperature and mass balance of Antarctica. Ann. Glaciol. 14, 78–84. doi: 10.3189/S0260305500008302
Fracheboud, Y., Leipner, J. (2003). “The application of chlorophyll fluorescence to study light, temperature, and drought stress,” in Practical applications of chlorophyll fluorescence in plant biology. Eds. DeEll, J. R., Toivonen, P. M. A. (Boston: Springer), 125–150.
Furness, S. B., Grime, J. P. (1982). Growth rate and temperature responses in bryophytes: II. A comparative study of species of contrasted ecology. J. Ecol. 1, 525–536. doi: 10.2307/2259920
Gago, J., Carriquí, M., Nadal, M., Clemente-Moreno, M. J., Coopman, R. E., Fernie, A. R., et al. (2019). Photosynthesis optimized across land plant phylogeny. Trends Plant Sci. 24, 947–958. doi: 10.1016/j.tplants.2019.07.002
Genty, B., Briantais, J. M., Baker, N. R. (1989). The relationship between the quantum yield of photosynthetic electron transport and quenching of chlorophyll fluorescence. BBA-Gen. Subj. 990, 87–92. doi: 10.1016/S0304-4165(89)80016-9
Gilmore, A. M. (1997). Mechanistic aspects of xanthophyll cycle-dependent photoprotection in higher plant chloroplasts and leaves. Physiol. Plantarum 99, 197–209. doi: 10.1111/j.1399-3054.1997.tb03449.x
Glime, J. M. (2011). “Ecological and physiological effects of changing climate on aquatic bryophytes,” in Bryophyte Ecology and Climate Change. Eds. Tuba, Z., Slack, N. G., Stark, L. R. (Cambridge: Cambridge University Press), 93–114.
Goss, R., Opitz, C., Lepetit, B., Wilhelm, C. (2008). The synthesis of NPQ-effective zeaxanthin depends on the presence of a transmembrane proton gradient and a slightly basic stromal side of the thylakoid membrane. Planta 228, 999–1009. doi: 10.1007/s00425-008-0800-7
Grassi, G., Magnani, F. (2005). Stomatal, mesophyll conductance and biochemical limitations to photosynthesis as affected by drought and leaf ontogeny in ash and oak trees. Plant Cell Environ. 28, 834–849. doi: 10.1111/j.1365-3040.2005.01333.x
Green, T. G. A., Schroeter, B., Seppelt, R. D. (2000). “Effect of temperature, light and ambient UV on the photosynthesis of the moss Bryum argenteum Hedw., in continental Antarctica,” in Antarctic Ecosystems: Models for Wider Ecological Understanding. Eds. Davidson, W., Howard-Williams, C., Broady, P. (Christchurch: The Caxton Press), 165–170.
Green, T. A., Kulle, D., Pannewitz, S., Sancho, L. G., Schroeter, B. (2005). UV-A protection in mosses growing in continental Antarctica. Polar Biol. 28, 822–827. doi: 10.1007/s00300-005-0011-7
Haldimann, P., Fracheboud, Y., Stamp, P. (1996). Photosynthetic performance and resistance to photoinhibition of Zea mays L. leaves grown at sub-optimal temperature. Plant Cell Environ. 19, 85–92. doi: 10.1111/j.1365-3040.1996.tb00229.x
Harley, P. C., Loreto, F., Di Marco, G., Sharkey, T. D. (1992). Theoretical considerations when estimating the mesophyll conductance to CO2 flux by analysis of the response of photosynthesis to CO2. Plant Physiol. 98, 1429–1436. doi: 10.1104/pp.98.4.1429
Havaux, M., Dall’Osto, L., Bassi, R. (2007). Zeaxanthin has enhanced antioxidant capacity with respect to all other xanthophylls in Arabidopsis leaves and functions independent of binding to PSII antennae. Plant Physiol. 145, 1506–1520. doi: 10.1104/pp.107.108480
He, X., He, K. S., Hyvönen, J. (2016). Will bryophytes survive in a warming world? Perspect. Plant Ecol. 19, 49–60. doi: 10.1016/j.ppees.2016.02.005
Hendrickson, L., Förster, B., Furbank, R. T., Chow, W. S. (2004). Processes contributing to photoprotection of grapevine leaves illuminated at low temperature. Physiol. Plantarum 12, 272–281. doi: 10.1111/j.0031-9317.2004.0324.x
Hirano, M., Satoh, K., Katoh, S. (1981). The effect on photosynthetic electron transport of temperature-dependent changes in the fluidity of the thylakoid membrane in a thermophilic blue-green alga. BBA-Bioenergetics 635, 476–487. doi: 10.1016/0005-2728(81)90107-9
Holaday, A. S., Martindale, W., Alred, R., Brooks, A. L., Leegood, R. C. (1992). Changes in activities of enzymes of carbon metabolism in leaves during exposure of plants to low temperature. Plant Physiol. 98, 1105–1114. doi: 10.1104/pp.98.3.1105
Hovenden, M. J., Jackson, A. E., Seppelt, R. D. (1994). Field photosynthetic activity of lichens in the Windmill Islands oasis, Wilkes Land, continental Antarctica. Physiol. Plantarum 90, 567–576. doi: 10.1111/j.1399-3054.1994.tb08816.x
Huang, G., Zhang, Q., Wei, X., Peng, S., Li, Y. (2017). Nitrogen can alleviate the inhibition of photosynthesis caused by high temperature stress under both steady-state and flecked irradiance. Front. Plant Sci. 8:945:945. doi: 10.3389/fpls.2017.00945
Huner, N. P., Öquist, G., Hurry, V. M., Krol, M., Falk, S., Griffith, M. (1993). Photosynthesis, photoinhibition and low temperature acclimation in cold tolerant plants. Photosynth. Res. 37, 19–39. doi: 10.1007/BF02185436
Hurry, V., Anderson, J. M., Chow, W. S., Osmond, C. B. (1997). Accumulation of zeaxanthin in abscisic acid-deficient mutants of Arabidopsis does not affect chlorophyll fluorescence quenching or sensitivity to photoinhibition in vivo. Plant Physiol. 113, 639–648. doi: 10.1104/pp.113.2.639
Hwang, H. J., Xu, C. C., Moon, B. Y., Lee, C. H. (2003). Recovery from low-temperature photoinhibition is related to dephosphorylation of phosphorylated CP29 rather than zeaxanthin epoxidation in rice leaves. J. Plant Biol. 46, 122–129. doi: 10.1007/BF03030441
Ino, Y. (1990). Field measurement of net photosynthesis of mosses at Langhovde, East Antarctica. Ecol. Res. 5, 195–205. doi: 10.1007/BF02346991
Ivanov, A. G., Sane, P., Hurry, V., Król, M., Sveshnikov, D., Huner, N. P., et al. (2003). Low-temperature modulation of the redox properties of the acceptor side of photosystem II: photoprotection through reaction centre quenching of excess energy. Physiol. Plantarum 119, 376–383. doi: 10.1034/j.1399-3054.2003.00225.x
Ivanov, A. G., Krol, M., Sveshnikov, D., Malmberg, G., Gardeström, P., Hurry, V., et al. (2006). Characterization of the photosynthetic apparatus in cortical bark chlorenchyma of Scots pine. Planta 223, 1165–1177. doi: 10.1007/s00425-005-0164-1
Jähne, B., Heinz, G., Dietrich, W. (1987). Measurement of the diffusion coefficients of sparingly soluble gases in water. J. Geophys. Res.-Oceans 92, 10767–10776. doi: 10.1029/JC092iC10p10767
Johnson, M. P., Pérez-Bueno, M. L., Zia, A., Horton, P., Ruban, A. V. (2009). The zeaxanthin-independent and zeaxanthin-dependent qE components of nonphotochemical quenching involve common conformational changes within the photosystem II antenna in Arabidopsis. Plant Physiol. 149, 1061–1075. doi: 10.1104/pp.108.129957
Kallio, P., Heinonen, S. (1975). “CO2 exchange and growth of Rhacomitrium lanuginosum and Dicranum elongatum,” in Fennoscandian Tundra Ecosystems Ecological Studies (Analysis and Synthesis). Ed. Wiegolaski, F. E. (Berlin: Springer), 138–148.
Kappen, L., Smith, R. L., Meyer, M. (1989). Carbon dioxide exchange of two ecodemes of Schistidium antarctici in continental Antarctica. Polar Biol. 9, 415–422. doi: 10.1007/BF00443227
Kennedy, A. D. (1993). Water as a limiting factor in the Antarctic terrestrial environment: a biogeographical synthesis. Arctic Alpine Res. 25, 308–315. doi: 10.2307/1551914
King, D. H. (2017). “Monitoring Antarctic bryophyte communities in a time of change. [Ph D Thesis],” (Wollongong: University of Wollongong).
King, D. H., Wasley, J., Ashcroft, M. B., Ryan-Colton, E., Lucieer, A., Chisholm, L. A., Robinson, S. A. (2020). Semi-automated analysis of digital photographs for monitoring East Antarctic vegetation. Front. Plant Sci. doi: 10.3389/fpls.2020.00766
Körner, C. (2003). Alpine plant life. Functional Plant Ecology of High Mountain Ecosystems (Berlin: Springer).
Krall, J. P., Edwards, G. E. (1992). Relationship between photosystem II activity and CO2 fixation in leaves. Physiol. Plantarum 86, 180–187. doi: 10.1111/j.1399-3054.1992.tb01328.x
Krause, G. H., Weis, E. (1988). “The photosynthetic apparatus and chlorophyll fluorescence. An introduction,” in Applications of chlorophyll fluorescence in photosynthesis research, stress physiology, hydrobiology and remote sensing. Ed. Lichtenthaler, H. K. (Dordrecht: Springer), 3–11.
Lambrev, P. H., Tsonev, T., Velikova, V., Georgieva, K., Lambreva, M. D., Yordanov, I., et al. (2007). Trapping of the quenched conformation associated with non-photochemical quenching of chlorophyll fluorescence at low temperature. Photosynth. Res. 94, 321. doi: 10.1007/s11120-007-9216-7
Lee, H. Y., Hong, Y. N., Chow, W. S. (2001). Photoinactivation of photosystem II complexes and photoprotection by non-functional neighbours in Capsicum annuum L. leaves. Planta 212, 332–342. doi: 10.1007/s004250000398
Leipner, J., Fracheboud, Y., Stamp, P. (1997). Acclimation by suboptimal growth temperature diminishes photooxidative damage in maize leaves. Plant Cell Environ. 20, 366–372. doi: 10.1046/j.1365-3040.1997.d01-76.x
Lembrechts, J. J., Lenoir, J. (2020). Microclimatic conditions anywhere at any time! Global Change Biol. 26, 337–339. doi: 10.1111/gcb.14942
Lembrechts, J. J., Lenoir, J., Roth, N., Hattab, T., Milbau, A., Haider, S., et al. (2019). Comparing temperature data sources for use in species distribution models: From in-situ logging to remote sensing. Global Ecol. Biogeogr. 28, 1578–1596. doi: 10.1111/geb.12974
Lobo, F. D. A., De Barros, M. P., Dalmagro, H. J., Dalmolin, Â. C., Pereira, W. E., De Souza, E. C., et al. (2013). Fitting net photosynthetic light-response curves with Microsoft Excel—a critical look at the models. Photosynthetica 51, 445–456. doi: 10.1007/s11099-013-0045-y
Longton, R. E. (1974). Microclimate and biomass in communities of the Bryum association on Ross Island, Continental Antarctica. Bryologist 77, 109–127. doi: 10.2307/3241549
Longton, R. E. (1988a). Adaptations and strategies of polar bryophytes. Bot. J. Linn. Soc 98, 253–268. doi: 10.1111/j.1095-8339.1988.tb02429.x
Longton, R. E. (1988b). Biology of polar bryophytes and lichens (Melbourne: Cambridge University Press).
Lovelock, C. E., Robinson, S. A. (2002). Surface reflectance properties of Antarctic moss and their relationship to plant species, pigment composition and photosynthetic function. Plant Cell Environ. 25, 1239–1250. doi: 10.1046/j.1365-3040.2002.00916.x
Lovelock, C. E., Jackson, A. E., Melick, D. R., Seppelt, R. D. (1995). Reversible photoinhibition in Antarctic moss during freezing and thawing. Plant Physiol. 109, 955–961. doi: 10.1104/pp.109.3.955
Lucieer, A., Turner, D., King, D. H., Robinson, S. A. (2014). Using an Unmanned Aerial Vehicle (UAV) to capture micro-topography of Antarctic moss beds. Int. J. Appl. Earth Obs. 27, 53–62. doi: 10.1016/j.jag.2013.05.011
Matsuda, T. (1968). Ecological study of the moss community and microorganisms in the vicinity of Syowa Station, Antarctica. JARE Sci. Rep. Ser. E Biol. 29, 1–58.
Maxwell, K., Johnson, G. N. (2000). Chlorophyll fluorescence—a practical guide. J. Exp. Bot. 51, 659–668. doi: 10.1093/jexbot/51.345.659
Melick, D. R., Seppelt, R. D. (1992). Loss of soluble carbohydrates and changes in freezing point of Antarctic bryophytes after leaching and repeated freeze-thaw cycles. Antarc. Sci. 4, 399–404. doi: 10.1017/S0954102092000592
Melick, D. R., Seppelt, R. D. (1994). Seasonal investigations of soluble carbohydrates and pigment levels in Antarctic bryophytes and lichens. Bryologist 1, 13–19. doi: 10.2307/3243343
Míguez, F., Fernández-Marín, B., Becerril, J. M., García-Plazaola, J. I. (2015). Activation of photoprotective winter photoinhibition in plants from different environments: a literature compilation and meta-analysis. Physiol. Plantarum 155, 414–423. doi: 10.1111/ppl.12329
Nadal, M., Flexas, J. (2018). “Mesophyll conductance to CO2 diffusion: effects of drought and opportunities for improvement,” in Water Scarcity and Sustainable Agriculture in Semiarid Environment. Eds. García-Tejero, I. F., Durán-Zuazo, V. H. (London: Elsevier), 403–438.
Nakatsubo, T. (2002). Predicting the impact of climatic warming on the carbon balance of the moss Sanionia uncinata on a maritime Antarctic island. J. Plant Res. 115, 99–106. doi: 10.1007/s102650200014
Newsham, K. K. (2010). The biology and ecology of the liverwort Cephaloziella varians in Antarctica. Antarc. Sci. 22, 131–143. doi: 10.1017/S0954102009990630
Ochyra, R., Lewis, S., Bednarek-Ochyra, H. (2008). The illustrated moss flora of Antarctica (Cambridge: Cambridge University Press).
Pannewitz, S., Green, T. A., Maysek, K., Schlensog, M., Seppelt, R., Sancho, L. G., et al. (2005). Photosynthetic responses of three common mosses from continental Antarctica. Antarc. Sci. 17, 341–352. doi: 10.1017/S0954102005002774
Pearce, D. A. (2008). Climate change and the microbiology of the Antarctic Peninsula region. Sci. Pro. 91, 203–217. doi: 10.3184/003685008X332534
Peat, H. J., Clarke, A., Convey, P. (2007). Diversity and biogeography of the Antarctic flora. J. Biogeog. 34, 132–146. doi: 10.1111/j.1365-2699.2006.01565.x
Pérez-Torres, E., Bravo, L. A., Corcuera, L. J., Johnson, G. N. (2007). Is electron transport to oxygen an important mechanism in photoprotection? Contrasting responses from Antarctic vascular plants. Physiol. Plantarum 130, 185–194. doi: 10.1111/j.1399-3054.2007.00899.x
Pinheiro, J., Bates, D., DebRoy, S., Sarkar, D., R Core Team (2019). nlme: Linear and Nonlinear Mixed Effects Models. R package version 3, 1–143.
Proctor, M. C. F. (1982). “Physiological ecology: water relations, light and temperature responses, carbon balance,” in Bryophyte ecology. Ed. Smith, A. J. E. (Dordrecht: Springer), 333–381.
Qiu, C., Ethier, G., Pepin, S., Dubé, P., Desjardins, Y., Gosselin, A. (2017). Persistent negative temperature response of mesophyll conductance in red raspberry (Rubus idaeus L.) leaves under both high and low vapour pressure deficits: a role for abscisic acid? Plant Cell Environ. 40, 1940–1959. doi: 10.1111/pce.12997
R Core Team (2015). R: A language and environment for statistical computing (Vienna, Austria.: R Foundation for Statistical Computing). Available at: http://www.R-project.org/ (Accessed 24 May 2016).
Rastorfer, J. R. (1970). Effects of light intensity and temperature on photosynthesis and respiration of two East Antarctic mosses, Bryum argenteum and Bryum antarcticum. Bryologist 1, 544–556. doi: 10.2307/3241493
Rice, S. K., Neal, N., Mango, J., Black, K. (2011). “Modeling bryophyte productivity across gradients of water availability using canopy form-function relationships,” in Bryophyte ecology and climate change. Eds. Tuba, Z., Slack, N. G., Stark, L. R. (Cambridge: Cambridge University Press), 441–457.
Ritchie, R. J. (2008). Fitting light saturation curves measured using modulated fluorometry. Photosynth. Res. 96, 201–215. doi: 10.1007/s11120-008-9300-7
Robinson, S. A., Waterman, M. J. (2014). “Sunsafe bryophytes: photoprotection from excess and damaging solar radiation,” in Photosynthesis in bryophytes and early land plants. Eds. Hanson, D., Rice, S. (Dordrecht: Springer), 113–130.
Robinson, S. A., Wasley, J., Popp, M., Lovelock, C. E. (2000). Desiccation tolerance of three moss species from continental Antarctica. Funct. Plant Biol. 27, 379–388. doi: 10.1071/PP99133
Robinson, S. A., King, D. H., Bramley-Alves, J., Waterman, M. J., Ashcroft, M. B., Wasley, J., et al. (2018). Rapid change in East Antarctic terrestrial vegetation in response to regional drying. Nat. Clim. Change 8, 879–884. doi: 10.1038/s41558-018-0280-0
Robinson, S. A., Klekociuk, A. R., King, D. H., Rojas, M. P., Zúñiga, G. E., Bergstrom, D. M. (2020). The 2019/2020 summer of Antarctic heatwaves. Glob. Change Biol. 26, 3178–3180. doi: 10.1111/gcb.15083
Sáez, P. L., Galmés, J., Ramírez, C. F., Poblete, L., Rivera, B. K., Cavieres, L. A., et al. (2018). Mesophyll conductance to CO2 is the most significant limitation to photosynthesis at different temperatures and water availabilities in Antarctic vascular species. Environ. Exp. Bot. 156, 279–287. doi: 10.1016/j.envexpbot.2018.09.008
Sáez, P. L., Rivera, B. K., Ramírez, C. F., Vallejos, V., Cavieres, L. A., Corcuera, L. J., et al. (2019). Effects of temperature and water availability on light energy utilization in photosynthetic processes of Deschampsia antarctica. Physiol. Plantarum 165, 511–523. doi: 10.1111/ppl.12739
Sage, R. F., Kubien, D. S. (2007). The temperature response of C3 and C4 photosynthesis. Plant Cell Environ. 30, 1086–1106. doi: 10.1111/j.1365-3040.2007.01682.x
Sage, R. F. (2002). Variation in the k cat of Rubisco in C3 and C4 plants and some implications for photosynthetic performance at high and low temperature. J. Exp. Bot. 53, 609–620. doi: 10.1093/jexbot/53.369.609
Savitch, L. V., Leonardos, E. D., Krol, M., Jansson, S., Grodzinski, B., Huner, N. P. A., et al. (2002). Two different strategies for light utilization in photosynthesis in relation to growth and cold acclimation. Plant Cell Environ. 25, 761–771. doi: 10.1046/j.1365-3040.2002.00861.x
Savitch, L. V., Ivanov, A. G., Gudynaite-Savitch, L., Huner, N. P., Simmonds, J. (2009). Effects of low temperature stress on excitation energy partitioning and photoprotection in Zea mays. Funct. Plant Biol. 36, 37–49. doi: 10.1071/FP08093
Scafaro, A. P., Von Caemmerer, S., Evans, J. R., Atwell, B. J. (2011). Temperature response of mesophyll conductance in cultivated and wild Oryza species with contrasting mesophyll cell wall thickness. Plant Cell Environ. 34, 1999–2008. doi: 10.1111/j.1365-3040.2011.02398.x
Schenker, R., Block, W. (1986). Micro-arthropod activity in three contrasting terrestrial habitats on Signy Island, maritime Antarctic. Brit. Antarct. Surv. B. 71, 31–43.
Sharkey, T. D., Zhang, R. (2010). High temperature effects on electron and proton circuits of photosynthesis. J. Integr. Plant Biol. 52, 712–722. doi: 10.1111/j.1744-7909.2010.00975.x
Shrestha, A., Song, X., Barbour, M. M. (2019). The temperature response of mesophyll conductance, and its component conductances, varies between species and genotypes. Photosynth. Res. 15, 1–18. doi: 10.1007/s11120-019-00622-z
Sinsawat, V., Leipner, J., Stamp, P., Fracheboud, Y. (2004). Effect of heat stress on the photosynthetic apparatus in maize (Zea mays L.) grown at control or high temperature. Environ. Exp. Bot. 52, 123–129. doi: 10.1016/j.envexpbot.2004.01.010
Smith, E. L. (1936). Photosynthesis in relation to light and carbon dioxide. P. Natl. Acad. Sci. U.S.A. 22, 504–511. doi: 10.1073/pnas.22.8.504
Smith, R. I. L. (1988). “Recording bryophyte microclimate in remote and severe environments,” in Methods in bryology. Ed. Glime, J. M. (Mainz: The Hattori Botanical Laboratory), 275–284.
Smith, R. I. L. (1996). “Terrestrial and freshwater biotic components of the western Antarctic Peninsula,” in Foundations for Ecological Research West of the Antarctic Peninsula. Eds. Ross, R. M., Hoffmann, E., II, Quetin, L. B. (Washington, D.C.: American Geophysical Union), 15–59.
Smith, R. I. L. (1999). Biological and environmental characteristics of three cosmopolitan mosses dominant in continental Antarctica. J. Veg. Sci. 10, 231–242. doi: 10.2307/3237144
Solovchenko, A. (2010). “Screening pigments: general questions,” in Photoprotection in Plants. Ed. Aolovchenko, A. (Berlin: Springer), 9–31.
Szilágyi, A., Sommarin, M., Åkerlund, H. E. (2007). Membrane curvature stress controls the maximal conversion of violaxanthin to zeaxanthin in the violaxanthin cycle—influence of α-tocopherol, cetylethers, linolenic acid, and temperature. BBA-Biomembranes 1768, 2310–2318. doi: 10.1016/j.bbamem.2007.06.001
Ubierna, N., Gandin, A., Boyd, R. A., Cousins, A. B. (2017). Temperature response of mesophyll conductance in three C4 species calculated with two methods: 18O discrimination and in vitro Vpmax. New Phytol. 214, 66–80. doi: 10.1111/nph.14359
Valentini, R., Epron, D., De Angelis, P., Matteucci, G., Dreyer, E. (1995). In situ estimation of net CO2 assimilation, photosynthetic electron flow and photorespiration in Turkey oak (Q. cerris L.) leaves: diurnal cycles under different levels of water supply. Plant Cell Environ. 18, 631–640. doi: 10.1111/j.1365-3040.1995.tb00564.x
Venema, J. H., Villerius, L., van Hasselt, P. R. (2000). Effect of acclimation to suboptimal temperature on chilling-induced photodamage: comparison between a domestic and a high-altitude wild Lycopersicon species. Plant Sci. 152, 153–163. doi: 10.1016/S0168-9452(99)00228-9
von Caemmerer, S., Evans, J. R. (2015). Temperature responses of mesophyll conductance differ greatly between species. Plant Cell Environ. 38, 629–637. doi: 10.1111/pce.12449
Wagner, S., Zotz, G., Salazar Allen, N., Bader, M. Y. (2013). Altitudinal changes in temperature responses of net photosynthesis and dark respiration in tropical bryophytes. Ann. Bot. 111, 455–465. doi: 10.1093/aob/mcs267
Walker, B., Ariza, L. S., Kaines, S., Badger, M. R., Cousins, A. B. (2013). Temperature response of in vivo Rubisco kinetics and mesophyll conductance in Arabidopsis thaliana: comparisons to Nicotiana tabacum. Plant Cell Environ. 36, 2108–2119. doi: 10.1111/pce.12166
Walton, D. W. H. (1982). The Signy Island terrestrial reference sites. XV. Microclimate monitoring 1972-74. Brit. Antarct. Surv. B. 55, 111–126.
Wang, L. J., Loescher, W., Duan, W., Li, W. D., Yang, S. H., Li, S. H. (2009). Heat acclimation induced acquired heat tolerance and cross adaptation in different grape cultivars: relationships to photosynthetic energy partitioning. Funct. Plant Biol. 36, 516–526. doi: 10.1071/FP09008
Warren, C. R., Dreyer, E. (2006). Temperature response of photosynthesis and internal conductance to CO2: results from two independent approaches. J. Exp. Bot. 57, 3057–3067. doi: 10.1093/jxb/erl067
Wickham, H. (2011). The Split-Apply-Combine Strategy for Data Analysis. J. Stat. Software 40, 1–29. http://www.jstatsoft.org/v40/i01/. doi: 10.18637/jss.v040.i01
Wilson, J. W. (1957). Observations on the temperatures of arctic plants and their environment. J. Ecol. 1, 499–531. doi: 10.2307/2256933
Wilson, M. E. (1990). Morphology and photosynthetic physiology of Grimmia antarctici from wet and dry habitats. Polar Biol. 10, 337–341. doi: 10.1007/BF00237820
Xiong, D., Liu, X. I., Liu, L., Douthe, C., Li, Y., Peng, S., et al. (2015). Rapid responses of mesophyll conductance to changes of CO2 concentration, temperature and irradiance are affected by N supplements in rice. Plant Cell Environ. 38, 2541–2550. doi: 10.1111/pce.12558
Xu, C. C., Jeon, Y. A., Lee, C. H. (1999). Relative contributions of photochemical and non-photochemical routes to excitation energy dissipation in rice and barley illuminated at a chilling temperature. Physiol. Plantarum 107, 447–453. doi: 10.1034/j.1399-3054.1999.100411.x
Yamori, W., Noguchi, K., Hanba, Y. T., Terashima, I. (2006). Effects of internal conductance on the temperature dependence of the photosynthetic rate in spinach leaves from contrasting growth temperatures. Plant Cell Physiol. 47, 1069–1080. doi: 10.1093/pcp/pcj077
Yang, Y. J., Zhang, S. B., Huang, W. (2018). Chloroplastic ATP synthase alleviates photoinhibition of photosystem I in tobacco illuminated at chilling temperature. Front. Plant Sci. 9:1648:1648. doi: 10.3389/fpls.2018.01648
Yin, X., Sun, Z., Struik, P. C., Gu, J. (2011). Evaluating a new method to estimate the rate of leaf respiration in the light by analysis of combined gas exchange and chlorophyll fluorescence measurements. J. Exp. Bot. 62, 3489–3499. doi: 10.1093/jxb/err038
Zotz, G., Büde, B., Meyer, A., Zellner, H., Lange, O. L. (1997). Water relations and CO2 exchange of tropical bryophytes in a lower montane rain forest in Panama. Bot. Acta 110, 9–17. doi: 10.1111/j.1438-8677.1997.tb00605.x
Keywords: Antarctica, bryophytes, carbon balance, electron transport rate, mesophyll conductance, net CO2 assimilation, non-photochemical quenching, respiration
Citation: Perera-Castro AV, Waterman MJ, Turnbull JD, Ashcroft MB, McKinley E, Watling JR, Bramley-Alves J, Casanova-Katny A, Zuniga G, Flexas J and Robinson SA (2020) It Is Hot in the Sun: Antarctic Mosses Have High Temperature Optima for Photosynthesis Despite Cold Climate. Front. Plant Sci. 11:1178. doi: 10.3389/fpls.2020.01178
Received: 20 January 2020; Accepted: 21 July 2020;
Published: 07 August 2020.
Edited by:
Jeffrey Graham Duckett, Natural History Museum, United KingdomReviewed by:
Peter Convey, British Antarctic Survey (BAS), United KingdomJeffrey William Bates, Imperial College London, United Kingdom
Copyright © 2020 Perera-Castro, Waterman, Turnbull, Ashcroft, McKinley, Watling, Bramley-Alves, Casanova-Katny, Zuniga, Flexas and Robinson. This is an open-access article distributed under the terms of the Creative Commons Attribution License (CC BY). The use, distribution or reproduction in other forums is permitted, provided the original author(s) and the copyright owner(s) are credited and that the original publication in this journal is cited, in accordance with accepted academic practice. No use, distribution or reproduction is permitted which does not comply with these terms.
*Correspondence: Alicia V. Perera-Castro, cGVyZXJhYWxpY2lhMTFAZ21haWwuY29t