- 1School of Engineering and Sciences, Tecnologico de Monterrey, Monterrey, Mexico
- 2Department of Biotechnology and Biochemistry, CINVESTAV Unidad Irapuato, Guanajuato, Mexico
- 3Mass Spectrometry Group, Max Planck Institute for Chemical Ecology, Jena, Germany
Peroxidases (PODs) have many biological functions during the plant life cycle. In maize kernels, endosperm PODs have been identified as biochemical contributors to resistance against Sitophilus zeamais, but their identities have not been determined. In this study, we identified these PODs and determined whether their contributions are basal or inducible. Semi-purification and LC-MS/MS analyses showed that the protein ZmPrx35 is the predominant soluble endosperm POD from kernels of Pob84-C3R. Subsequent time-course analyses after mechanical damage showed that POD activity was regulated in a fluctuating kinetics pattern and that zmprx35 mRNA expression levels reflected this pattern. After 48 h of infestation with S. zeamais or Prostephanus truncatus, soluble endosperm POD activities were 1.38- or 0.85-fold, respectively. Under the same conditions, zmprx35 expression was induced 1.61-fold (S. zeamais infestation) and 1.17-fold (P. truncatus infestation). These findings suggest that ZmPrx35 contributes to the protective responses of aleurone cells against wounding and pest attacks, which could be enhanced/repressed by insect factors. Our data also provide evidence that the mechanisms of resistance of maize Pob84-C3R kernels toward the insect pests S. zeamais and P. truncatus are independent.
Introduction
Maize (Zea mays L.) is the most cultivated cereal globally, with an estimated production of 1068 million tonnes per year (Cerquiglini et al., 2016). Postharvest losses due to insects, primarily Sitophilus zeamais and Prostephanus truncatus, represent 12–36% of the global yield, primarily affecting smallholders in vulnerable countries (Gitonga et al., 2013; Midega et al., 2016; Tefera et al., 2016).
Many strategies have been used to reduce insect-related postharvest losses, including chemical control and hermetic storage systems (Boyer et al., 2012; García-Lara et al., 2013; Gitonga et al., 2013; Mlambo et al., 2017). But these solutions are often unaffordable for smallholders (Midega et al., 2016). Thus, alternatives such as repellent ground powders (Midega et al., 2016) and local varieties and native landraces with natural pest resistance (García-Lara and Bergvinson, 2013; Gitonga et al., 2013; Mwololo et al., 2013; Mlambo et al., 2017) have been considered. As landraces often have comparatively low yields (Daiki et al., 2017), breeding programmes have been introduced globally to develop insect-resistant varieties with increased yields (Abebe et al., 2009; Tefera et al., 2011, 2016). For this purpose, the traits and their mechanisms of action associated with postharvest pest resistance need to be identified described. Antibiosis and antixenosis resistance mechanisms have been described in maize kernels (Arnason et al., 1994; Derera et al., 2001a, b; Bergvinson and García-Lara, 2004; Nwosu et al., 2015). Additionally, anatomical kernel traits, such as hardness, vitreousness, and pericarp thickness/toughness, have been considered as biophysical traits that confer resistance (Saulnier and Thibault, 1999; García-Lara et al., 2004; Mwololo et al., 2013; García-Lara and Bergvinson, 2014). Moreover, as biochemical sources of parasite resistance, phenolic compounds, amides, structural proteins, and endosperm peroxidases (PODs) have been identified (Classen et al., 1990; Arnason et al., 1994; Sen et al., 1994; Barros-Rios et al., 2015; López-Castillo et al., 2018a).
Correlations between soluble endosperm POD activity and resistance of maize kernels to the postharvest pest S. zeamais have been described previously (García-Lara et al., 2007; López-Castillo et al., 2018a). Recently, the PODs B6T173 and K7TID5 were shown to contribute 80% of the POD activity in maize kernels, and these are probably isoforms of the enzyme ZmPrx35 (López-Castillo et al., 2015). Hence, contributions of endosperm PODs and the associations of ZmPrx35 with resistance need to be determined.
Herein, we identify endosperm PODs that contribute resistance against postharvest pests and distinguish between their basal and inducible mechanisms. To this end, we performed activity-directed partial purification of endosperm PODs and then identified the enzymes using liquid chromatography–tandem mass spectrometry (LC-MS/MS). We also determined the expression levels of the zmprx35 gene and the related POD activities in Pob84-C3R endosperm tissues to determine whether ZmPrx35 is responsive to mechanical damage and attacks by the pests S. zeamais and P. truncatus.
Materials and Methods
Maize Genotypes
Population 84 is an open pollinated population that was developed at CIMMYT from 20 Caribbean accessions with natural resistance to the maize weevil S. zeamais and the larger grain borer P. truncatus. Details of the breeding methods used to improve this maize population were described previously (García-Lara and Bergvinson, 2014). We examined red kernels from the third cycle of selection (Pob84-C3R). These were harvested in increased quantities during 2014 at the CIMMYT experimental station at Agua Fria, Puebla, Mexico (19° N, 60 masl).
Enriched Endosperm Fractions
Endosperm samples were collected by hand dissection of Pob84-C3R kernels according to the methods described by López-Castillo et al. (2018a). Briefly, pedicels were removed from intact seeds by cutting kernel tip cap areas transversely. Seeds were then imbibed in distilled water for 10 min and pericarps were removed using tweezers. Finally, germ structures were carefully removed using a scalpel, dried and stored at 4°C until analysis.
Endosperm Milling
Corresponding endosperm-enriched tissue fractions were frozen in liquid nitrogen and were then milled in a pre-cooled coffee grinder (Krups, Solingen, Germany) in three steps of 1 min. The resulting flour was frozen again in a mortar, sifted through a pre-chilled 60-mesh sieve (Montinox, Mexico) and stored at −80°C until analysis.
Partial Purification of Endosperm PODs
Soluble endosperm PODs were extracted by homogenizing 250 g samples of endosperm flour in 750 mL of 25 mM Tris–HCl (pH 7.0), followed by incubation at 25°C for 1 h on a stirring plate at 300 rpm. Mixtures were then centrifuged at 10,000 × g for 30 min at 4°C. The resulting supernatants were recovered, filtered and partially purified using a three-step strategy. First, supernatants were loaded into a cationic-exchange chromatography HiTrap SP FF column (1 mL; GE Healthcare, Uppsala, Sweden) and were eluted with a linear gradient of 0–1 M NaCl in 25 mM Tris-HCl (pH 7.0). POD activities of fractions were then tested according to a method reported previously (López-Castillo et al., 2015). The fractions with POD activity were precipitated at −20°C overnight in 80% acetone. After centrifugation at 10,000 × g, supernatants were discarded, and pellets were resuspended in 25 mM Tris–HCl (pH 7.0) containing 1.5 M ammonium sulfate. In the second step, hydrophobic interaction chromatography was performed by loading 1 mL aliquots of the suspended protein into a Phenyl SP column (GE Healthcare). Proteins were eluted using a linear gradient of 1.5–0 M ammonium sulfate in 25 mM Tris–HCl (pH 7.0). Peroxidase-active fractions were then desalted and concentrated using ultrafiltration membranes with a molecular weight cut off of 10 kDa (Millipore, United States). Finally, these fractions were affinity-purified using a Concanavalin A-Sepharose 4B column (0.8 × 4 cm; SIGMA, United States). Proteins were eluted in a linear gradient of 0–1 M glucose in 25 mM Tris–HCl (pH 7.0) containing 1 mM CaCl2, 1 mM MnCl2, 1 mM MgCl2 and 500 mM NaCl. Fractions were tested again for POD activity. Protein bands with POD activity were separated using SDS-PAGE and were then excised from the gel and stored at 4°C until nano-electrospray ionisation (ESI)-LC-MS/MS identification.
Liquid Chromatography–Mass Spectrometry Analysis
Excised bands from the SDS-PAGE gel were trypsinised as described previously (Shevchenko et al., 2006). For LC-MS analysis, samples were reconstituted in 50 μL aqueous aliquots of 1% formic acid.
Peptide mixtures of 1 μL were injected onto an online ultra-performance liquid chromatography (UPLC) M-class system (Waters) coupled with a Synapt G2-si mass spectrometer equipped with a T-WAVE-IMS device (Waters). Samples were pre-concentrated online and were desalted using a UPLC M-Class Symmetry C18 trap column (100 Å, 180 μm × 20 mm, 5-μm particle size) at a flow rate of 15 μL/min in 0.1% aqueous formic acid. Peptides were then eluted onto an ACQUITY UPLC HSS T3 analytical column (100 Å, 75 μm × 200 mm, 1.8-μm particle size) at a flow rate of 350 nL/min using an increasing acetonitrile gradient from 2 to 90% B over 65 min (buffer A, 0.1% formic acid in water; buffer B, 100% acetonitrile in 0.1% formic acid).
Eluted peptides were transferred to a mass spectrometer, which was operated in V-mode with a resolving power of at least 20,000 full width at half maximum. All analyses were performed in the positive ESI mode. To compensate for mass shifts in MS and MS/MS fragmentation modes, human Glu-Fibrinopeptide B at 100 fmol/μL in 0.1% formic acid/acetonitrile (1:1 v/v) was infused through the reference sprayer every 45 s at a flow rate of 1 μL/min.
Data were acquired using data-dependent acquisition (DDA). The acquisition cycle for DDA analysis comprised a survey scan covering the range of 400–1800 m/z, followed by MS/MS fragmentation of the 10 most intense precursor ions, which were collected at 0.5-s intervals in the range of 50–2000 m/z. Dynamic exclusion was applied to minimize multiple fragmentations for the same precursor ions.
MS data were collected using MassLynx v4.1 software (Waters).
Data Processing and Protein Identification
Raw DDA data were processed and searched against a subdatabase containing common contaminants (human keratins and trypsin) using the ProteinLynx Global Server (PLGS), version 2.5.2 (Waters). The following search parameters were applied: fixed precursor ion mass tolerance of 10 ppm for the survey peptide, fragment ion mass tolerance of 0.02 Da, an estimated calibration error of 0.002 Da, one missed cleavage, fixed carbamidomethylation of cysteines and possible oxidation of methionine.
Spectra that remained unmatched in database searches were interpreted de novo to yield peptide sequences, and were subjected to homology-based searches using the MS BLAST program (Shevchenko et al., 2001) on a local server. MS BLAST searches were performed against a subdatabase containing plant proteins (downloaded on February 2, 2019). Concomitantly, pkl files of MS/MS spectra were generated and searched against plant protein subdatabases from NCBInr (downloaded on January 15, 2019, containing 7,456,519 sequences) using MASCOT software, version 2.6.2. The most representative proteins of Z. mays from each group with at least two peptide hits and supported by both identification strategies were chosen as candidates.
Mechanical Damage Kinetics
To induce mechanical damage, five holes of 1-mm diameter were bored into endosperms from Pob84-C3Red maize plants using a mini-drill (HER-201, Steren, Mexico). Perforated endosperms were then placed individually in a 48-well plate (COSTAR, 3548, Corning, CA, United States). For each sampling time, 48 drilled endosperms and 48 undamaged endosperms were tested. Endosperms were stored at 27 ± 2°C and 70 ± 5% relative humidity. Endosperm samples were collected at 0, 8, 16, 24, 48, 72, 96, 120, and 192 h after damage and were frozen and milled using liquid nitrogen as described in the previous sections. Samples were stored at −80°C until analysis.
Assays of Mechanical and Insect Damage
To induce mechanical damage, three replicates of 48 endosperms from Pob84-C3Red maize plants were drilled as described above and were placed individually into 48-well plates (COSTAR, 3548, Corning, CA, United States). To induce S. zeamais or P. truncatus damage, endosperms were placed in 48-well plates in triplicate. Single endosperms were placed in each well with three insects. Undamaged endosperms were used as controls. Plates were then incubated in darkness at 27 ± 2°C and 70 ± 5% relative humidity for 24 h. After removing insects, endosperms were frozen in liquid nitrogen as described above. Samples were stored at −80°C until analysis.
Determination of POD Activity
Peroxidases activity was measured using a method described previously (Bestwick et al., 1998), with modifications. Initially, 100-mg samples of endosperm flour were homogenized in 500 μL aliquots of 25-mM Tris–HCl (pH 6.8). Homogenates were incubated for 30 min at 25°C with continuous vortex shaking (3000 rpm) and were then centrifuged at 10,000 × g for 30 min at 4°C. Protein concentrations of supernatants were quantified using the Bradford method (Bradford, 1976) and a Coomassie dye binding protein assay kit (Sigma-Aldrich, St. Louis, MO, United States) according to the manufacturer’s instructions. POD activity was measured in 96-well plates (Costar®, 9017, Corning, CA, United States) at 470 nm using a Synergy HT microplate reader (BioTek®, Winooski, VT, United States). In these experiments, 200 μL of solution containing 20 mM guaiacol and 0.03% H2O2 in 25 mM Tris–HCl (pH 6.8) was added to each well. Reactions were started with the addition of 20 μL aliquots of extract. Immediately after protein addition, plates were read in the kinetics mode for 30 min with 1-min intervals between the reads. POD activity was calculated from changes in absorbance per minute using a 470 nm molar extinction coefficient for tetraguaiacol of 26,600 M–1 cm–1. Data were normalized to reaction volumes and protein quantities and POD activities in test samples were expressed relative to those in corresponding non-damaged controls.
Visualization of POD Activity
Peroxidases activity in endosperms was visualized using histological staining as described previously (López-Castillo et al., 2018a). Briefly, intact and damaged endosperms were incubated in 50-mM potassium phosphate buffer (pH 6.8) containing 10 mM guaiacol and 0.03% H2O2 for 15 min. Images were captured using a Leica Zoom 2000 Stereozoom Microscope (Leica, Germany) equipped with a Dino-Eye Digital Eye Piece Camera (5 MP, AnMo Electronics Corporation, Taiwan).
RNA Extraction and cDNA Preparation
RNA was extracted using a modification of the method reported by Gambino et al. (2008). All materials were autoclaved before use, and solutions were treated with 0.1% (v/v) diethylpyrocarbonate and were autoclaved before use. Endosperm RNA was obtained from 1-g samples of frozen flour and germ RNA was extracted from 50-mg flour samples. In both cases, flour samples were added to sterile tubes with six volumes of extraction buffer containing 100 mM Tris–HCl (pH 8.0), 2% CTAB, 2.5% PVP-40, 2-M NaCl, 25 mM EDTA and 2% (v/v) β-mercaptoethanol at 65°C. Samples were homogenized by vortex shaking and were incubated at 65°C in a water bath. Single volumes of chloroform:isoamyl alcohol (24:1) were then added to homogenates, vigorously mixed using vortex shaking (3000 rpm) and centrifuged at 12,000 rpm for 10 min at 4°C. Supernatants were recovered and two subsequent extractions were performed with chloroform:isoamyl alcohol. After extraction, supernatant volumes were carefully measured, and LiCl was added to a final concentration of 3 M. Samples were incubated at −20°C overnight and were centrifuged at 12,000 rpm for 20 min. The resulting pellet was resuspended in 500 μL of pre-heated (65°C) buffer containing 10-mM Tris–HCl (pH 8.0), 1-mM EDTA, 1% SDS and 1-M NaCl (SSTE buffer). Three extractions with one equal volume of chloroform:isoamyl alcohol were performed. Supernatants were then transferred to clean tubes and RNA was precipitated in 0.7 volumes of cold isopropanol by centrifuging at 12,000 rpm for 15 min at 4°C. Supernatants were discarded and pellets were washed with 70% ethanol and resuspended in 100-μL aliquots of nuclease-free water. RNA samples were cleaned using the RNA Clean & Concentrator-5TM kit (Zymo Research, United States) and were finally recovered in 30-μL aliquots of nuclease-free water.
DNA was removed from the samples by treating with DNase I (Thermo Fisher Scientific, United States), and cDNA was prepared from 1 μg of total RNA using M-MLV Reverse Transcriptase (Invitrogen, Carlsbad, CA, United States) and poly-dT primers. Reaction mixtures were incubated at 37°C for 1 h according to the manufacturer’s instructions. Reverse transcriptase reactions were stopped by heating at 70°C for 15 min. The resulting cDNA samples were stored at −20°C until use.
Gene Expression Analysis
Expression analyses of zmprx35 mRNA were performed using a quantitative polymerase chain reaction (qPCR)-based strategy. Primer sequences for zmprx35 expression analyses were 5′-GACCTTCGACAACCAGTACTTC-3′ and 5′-TTCCCTGATCTCTCCCTCATC-3′. The beta-tubulin gene was used as the control, with the primers reported by Lin et al. (2014). qPCR reactions were performed using PowerUpTM SYBRTM Green Master Mix (Applied Biosystems, United States) according to the manufacturer’ instructions in total reaction volumes of 20 μL containing 100 ng of cDNA and primers at 500 nM. Reactions were performed in quadruplicate in a Rotor Gene 3000 thermal cycler (Corbett Research, Australia) using the conditions prescribed by the manufacturer of the PowerUpTM SYBRTM Green Master Mix. Uracil-DNA glycosylase activation was performed at 50°C for 2 min and was followed by a denaturation step of 95°C for 2 min. PCRs were then performed with 40 cycles of 15-s denaturation at 95°C and 1-min annealing/extension at 60°C. After a melting curve analyses, the relative gene expression was calculated using the ΔΔCt method (Yuan et al., 2008) assuming equal efficiency for all samples.
Statistical Analysis
Analysis of variance was performed using the Statistix v.9 (Analytical Software, Tallahassee, FL, United States) statistical software program. Differences between means were compared using Tukey tests and were considered significant when P ≤ 0.05. POD activities and ZmPrx35 expression levels were assessed using Pearson correlation analyses.
Results and Discussion
Identification of Endosperm PODs That Contribute to Insect Resistance
In this work, we sought to identify endosperm PODs involved in the resistance of grain crops to attack by insects. According to the UniProt database, 727 proteins have been tagged as PODs. However, experimental evidence at the protein level has been reported only for three of them. In contrast, evidence at the transcript level has been reported for 208 sequences, whereas 387 have been inferred from homology and 129 have been annotated as predicted sequences (UniProtKB). Such enzymes have been described in studies of water-soluble (albumins/globulins) fractions of maize kernel proteins (García-Lara et al., 2007; Winkler and García-Lara, 2010; López-Castillo et al., 2015, 2018a). Yet, because these protein fractions represent less than 5% of the total grain protein (Wang et al., 2008; Díaz-Gómez et al., 2017), kernel PODs are not abundant (López-Castillo et al., 2015). Moreover, endosperm-soluble PODs are confined in aleurone layers (García-Lara et al., 2007; López-Castillo et al., 2018a). Thus, we investigated a strategy to enrich these enzymes and then semi-purified them according to enzyme activity. As shown in Figure 1 and Supplementary Figure S1, a single protein was identified as the prevailing POD in the endosperm, with an apparent molecular mass of approximately 35 kDa. Through the present purification steps, activity-directed staining was increased (Figures 1A,B) and the ensuing POD-active proteins were detected visually. However, in Coomassie R-250 staining analyses (Figure 1C), protein bands with POD activity were indistinguishable, suggesting that this protein is not abundant in endosperm tissues. Maize kernel PODs were previously considered rare but highly active enzymes (López-Castillo et al., 2015). Thus, we performed guaiacol staining of the prevailing bands from the last purification step and identified the protein using LC-MS/MS.
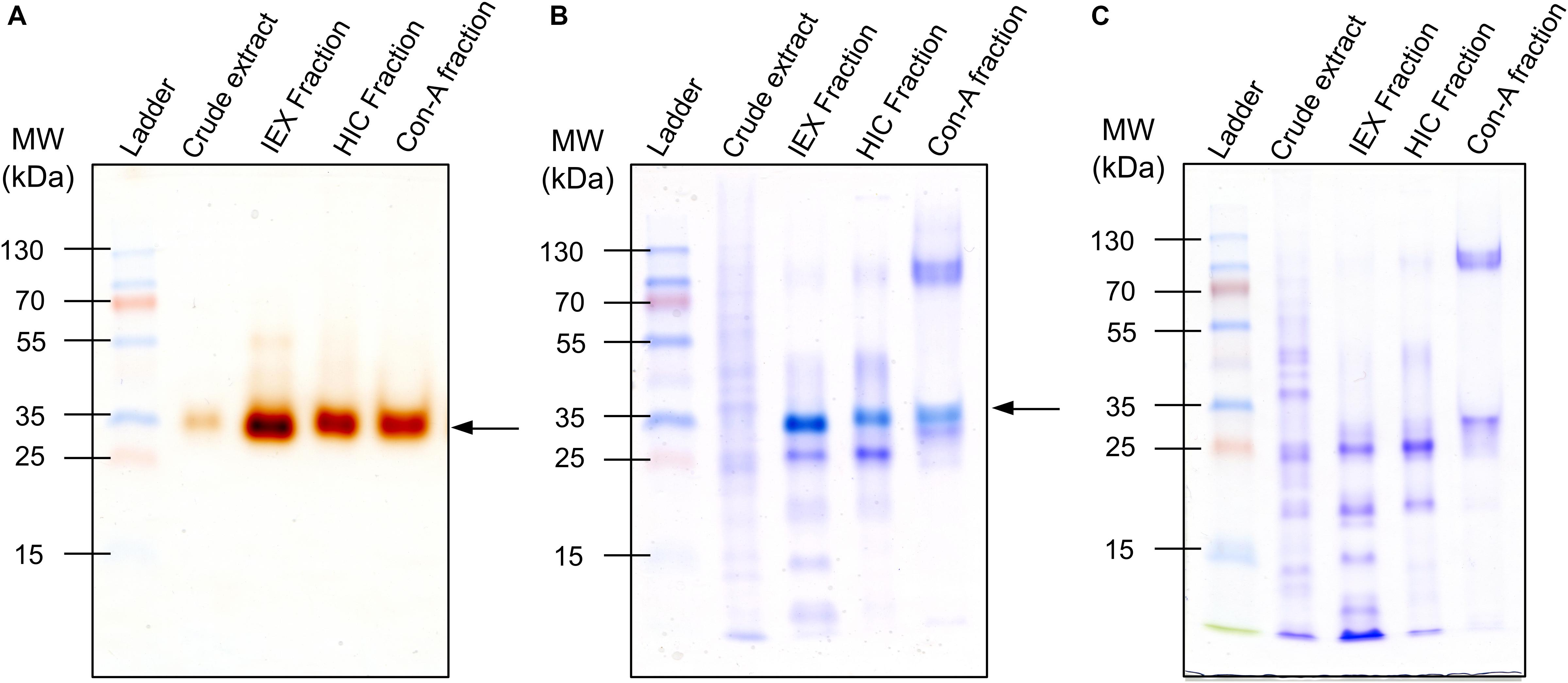
Figure 1. Purification of endosperm peroxidases (PODs) from insect-resistant maize P84-C3R. (A) Guaiacol–H2O2 staining of fractions from purification steps; active PODs are observed as brown-stained bands. (B) Sequential staining of POD-active fractions (guaiacol–H2O2 + Coomassie R-250); bands with POD activity are identified by the distinct bright bluish color. (C) Electrophoretic profile of POD-active fractions stained with Coomassie R-250; 10-μg samples of protein were loaded into each well. Arrows indicate proteins displaying POD activity.
Liquid chromatography–tandem mass spectrometry analyses of the guaiacol-active bands revealed multiple protein candidates, and the most representative Z. mays candidates identified using conventional database searching (MASCOT) and homology-based searching (de novo sequencing/MS BLAST) are displayed in Table 1 and Supplementary Table S1. Using this strategy, the protein K7TID5_MAIZE, which is a classical Class III POD, was predicted as the most probable POD. In addition, four proteins were identified: the globulin Q7M1Z8_MAIZE, the amine oxidase K7U2E4_MAIZE, the vicilin-like embryo storage protein C0PGM3_MAIZE and the acidic endochitinase A0A1D6HTN9_MAIZE.

Table 1. Identification of proteins from the semi-purified band with POD activity from the endosperm of P84-C3R maize using LC-MS/MS, conventional database searching (MASCOT) and homology-based searching (de novo sequencing/MS BLAST).
In BLAST searches of the K7TID5_MAIZE sequence on RedoxiBase (Fawal et al., 2013), this protein was 100% identical to the enzyme ZmPrx35, which was identified previously as the most active POD in whole kernels. The protein presents as the two possible isoforms B6T173_MAIZE and K7TID5_MAIZE, which only differ in ten amino acid residues (López-Castillo et al., 2015). With the identified peptides, we did not find evidence that suggested any of the isoforms of ZmPrx35 as the predominant POD in the endosperm of the insect-resistant maize genotype Pob84-C3R.
Modulation of Endosperm POD Activity and Expression of zmprx35 in Response to Mechanical and Insect Damage
After identifying ZmPrx35 as the prevailing endosperm POD in the insect-resistant maize strain Pob84-C3R, we determined whether this enzyme contributes to postharvest pest resistance. The following hypotheses for pest resistance have been discussed widely: the first establishes an indirect association of PODs in cell wall reinforcement via the oxidative coupling of feruloylated oligosaccharides, resulting in the formation of ester-linked diferulates (Saulnier and Thibault, 1999; Barros-Rios et al., 2015), with consequent increases in pericarp thickness and toughness (Saulnier and Thibault, 1999; García-Lara et al., 2004). The second hypothesis suggests a biochemical contribution through the catalysis of phenolic compounds in cells of the aleurone layer (Sen et al., 1994; García-Lara et al., 2007; López-Castillo et al., 2018a). Given the versatility of plant PODs (Hiraga et al., 2001; Passardi et al., 2005) and their induction following mechanical and insect damage, the second scenario is likely, as reported for species such as chickpea (Scalet et al., 1991), red oak, Allison and Schultz (2004), cotton, tomato, and cowpea (Singh et al., 2013).
Thus, to determine whether endosperm PODs are activated in response to mechanical damage, we performed histochemical staining of guaiacol–H2O2 in maize endosperms after scalpel or drill wounding (Figure 2). We observed POD activity in extracellular spaces of aleurone cells in the peripheral zones of endosperm wounds (Figures 2A,B). In particular, one kernel with naturally damaged aleurone cells had the same pattern of POD activity (Figure 2C), suggesting that aleurone PODs respond to mechanical damage and that their activities are compartmentalized in extracellular/apoplastic regions. These observations are congruent with the cell locations of ZmPrx35 (K7TID5_MAIZE or B6T173_MAIZE) that are predicted in the UniProt database (UniProtKB-Subcell), which locates proteins in extracellular regions and secreted proteins. In agreement, PODs were reportedly released into apoplasts of wheat roots as an early response to wounding (Minibayeva et al., 2009). Other studies show that the performance of a particular apoplastic enzyme depends on adequate gene transcription and protein synthesis, and on appropriate secretion and targeting to the required locations, in this case, wounded areas (Penel and Dunand, 2009; Minibayeva et al., 2015).
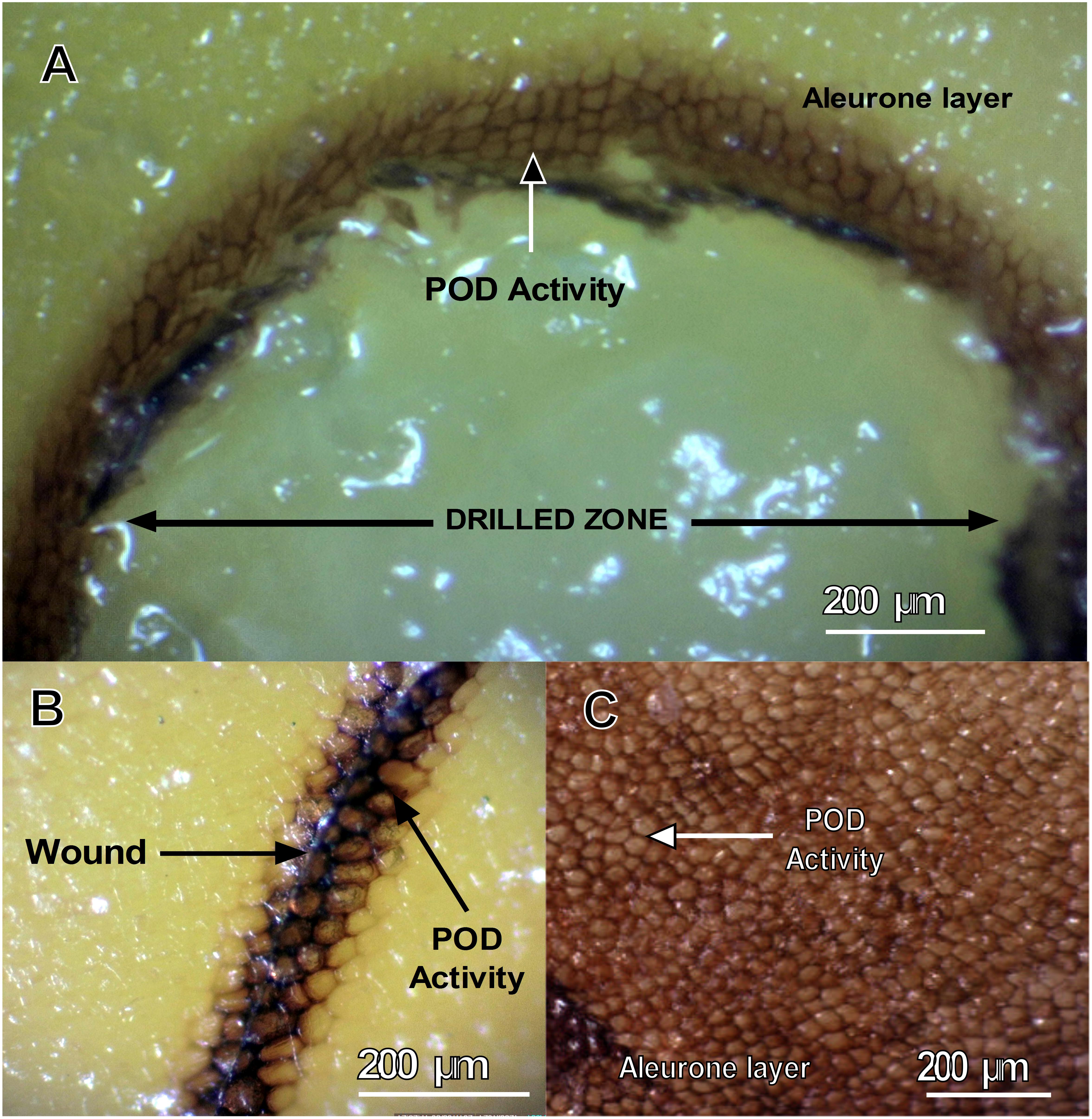
Figure 2. Induction of POD activity in aleurone layers of P84-C3R kernels. Guaiacol–H2O2 staining of a maize kernel after mechanical wounding by (A) drill perforation; (B) knife wounding; (C) naturally damaged aleurone; POD activity is visible as dark brown staining.
Apoplastic PODs have been generally associated with wounding responses, with data showing increased enzyme production and ROS scavenging (Minibayeva et al., 2015). Immediate increases in POD activity have been observed after wounding in multiple studies (Scalet et al., 1991; Holm et al., 2003; Bindschedler et al., 2006; Minibayeva et al., 2009; Roach et al., 2015), and these increases are sustained for several hours and even days (Scalet et al., 1991; Holm et al., 2003). In Asparagus spears, POD activity has also been associated with the accumulation of POD transcripts, suggesting that wound-induced activities reflect the release of PODs into apoplasts and the activation of enzymes by post-translational modifications (Holm et al., 2003; Minibayeva et al., 2015).
Therefore, to confirm that the activity of aleurone PODs and the expression of zmprx35 could be modulated by mechanical wounding, we performed time-course experiments for POD activity and zmprx35 expression levels. After wounding endosperms using a drill, as a model of P. truncatus mediated damage, we observed immediate increases in POD activity (199.31%) in comparison with undamaged endosperms (Figure 3A). However, these increases did not increase linearly during our time-course experiments. Instead, POD activities fluctuated in a ping-pong kinetic pattern (Figure 3A). Specifically, POD activity was increased at 16 (113.16%), 24 (114.56%), 72 (108.48%), 96 (131.94%), and 192 h (129.01%), but was decreased at 8 (90.68%), 48 (95.84), and 144 h (95.84%). These fluctuating kinetics has been described as a ping-pong bisubstrate mechanism for various PODs, including horseradish POD (Dunford, 1995; Deyhimi and Nami, 2011), tobacco anionic POD (Lagrimini et al., 1993) and Cistus multiflorus POD (Galende et al., 2015), and reflect the early release of water before the binding of an electron donor (Lagrimini et al., 1993; Deyhimi and Nami, 2011). These observations indicate that aleurone PODs could be subject to competitive substrate inhibition, as described above.
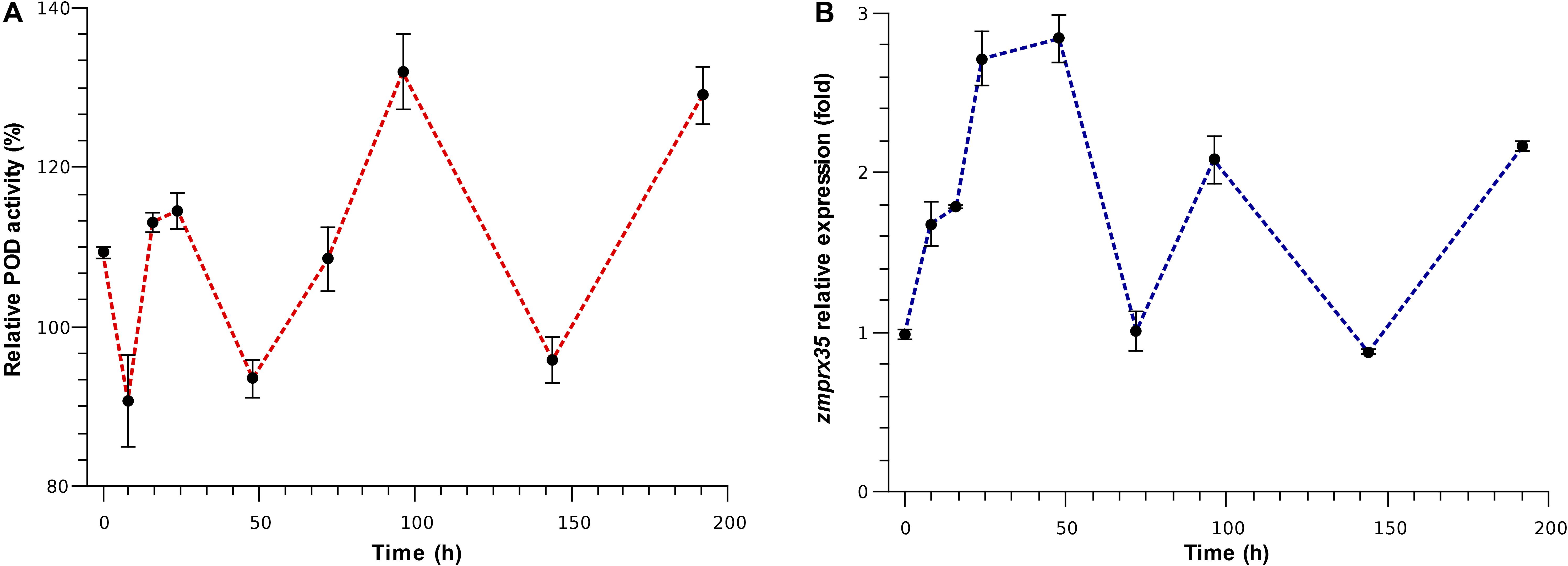
Figure 3. Modulation of POD activity and expression of zmprx35 mRNA over time. (A) Time course of POD activity after mechanical wounding (T0); (B) time course of zmprx35 expression after mechanical wounding (T0); relative POD activity and zmprx35 mRNA expression levels were calculated in comparison with those from non-damaged controls incubated under the same conditions. Error bars are standard deviations (SDs) of the mean.
To determine whether zmprx35 transcription is responsive to mechanical damage, we examined mRNA expression levels in samples from the same time course. Similar fluctuating expression patterns were observed in these experiments (Figure 3B), with fold changes in zmprx35 expression of 0.99-fold immediately after wounding, 1.67-fold at 8 h, 1.78-fold at 16 h, 2.71-fold at 24 h, 2.84-fold at 48 h, 2.08-fold at 96 h and 2.16-fold at 192 h. Between these time points, we observed baseline expression at 72 h (1.01-fold) and decreased expression at 144 h (0.87-fold). These changes in POD activity and zmprx35 expression suggest that zmprx35 could be modulated in response to mechanical damage. Pearson analysis did not display significant correlation between the expression of zmprx35 and measured endosperm POD activities (0.200, p = 0.604), suggesting that POD activity is independent of the transcription rate of zmprx35.
After examining the responses of aleurone PODs to mechanical wounding, we determined whether the contributions of endosperm PODs to postharvest pest resistance are induced in response to wounds or compounds from insects. To this end, we determined POD activities and zmprx35 expression levels following 24-h infestations with S. zeamais or P. truncatus (Figure 4). In the presence of S. zeamais, POD activity was higher than that in mechanically damaged kernels (133.02 vs. 114.03%). Similarly, zmprx35 transcription was increased by 1.61-fold in comparison with non-damaged controls.
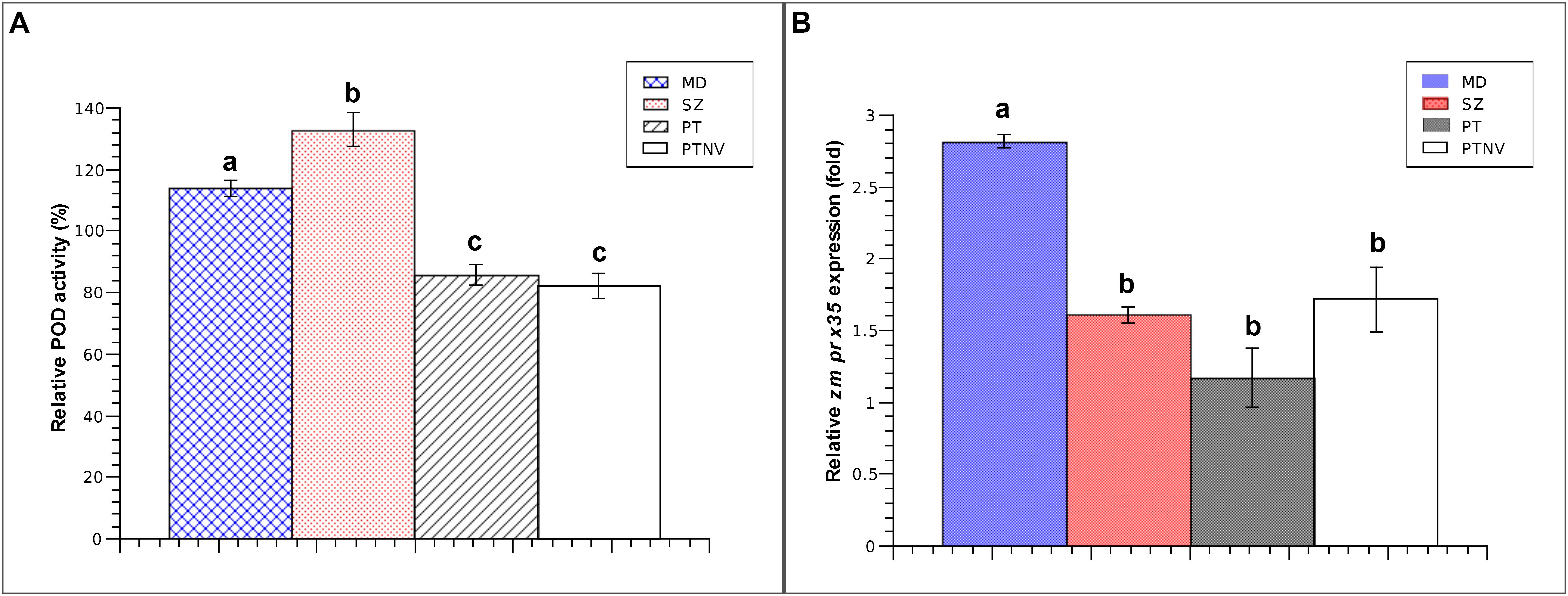
Figure 4. Endosperm responses to mechanical and insect damage after 24 h. (A) Relative POD activity in endosperms from Pob84C3R maize at 24 h after mechanical or insect damage; comparisons are made with non-damaged endosperms. (B) Relative expression of zmprx35 mRNA in endosperms from Pob84C3R maize at 24 h after mechanical or insect damage; comparisons are made with non-damaged endosperms. MD, mechanically wounded endosperms; SZ, S. zeamais-infested endosperms; PT, P. truncatus-damaged kernels; PTNV, P. truncatus exposed kernels with no apparent damage; comparisons were performed using Tukey tests and were considered significant when α = 0.05. Means with different letters indicate significant differences, p < 0.05; error bars are SDs.
In experiments with P. truncatus, endosperms had characteristic tunneling patterns at 24 h after exposure to pest-induced damage. We also identified endosperms that remained apparently undamaged. In comparison with the endosperms of untreated kernels, damaged endosperms, and exposed but intact endosperms had POD activities of 85.87 and 82.21%, respectively. The expression of zmprx35 mRNA was increased in both visually damaged endosperms of kernels (1.17-fold) and intact endosperms that had been in contact with the pest (1.72-fold). This finding is notable, because although POD activity decreased, the de novo synthesis of zmprx35 transcripts was clear and independent of the size of the wound. These results further suggest the transcriptional modulation of zmprx35 by signaling pathways that could be activated in response to stress factors, such as wounding or compounds from P. truncatus.
Implications of Endosperm PODs in Insect Resistance
Apoplastic class III PODs have been directly associated with the maintenance of cell wall integrity, performing catalytic roles in cross-linking, loosening, lignification and suberization (Barros-Rios et al., 2015; Shigeto and Tsutsumi, 2016; Černý et al., 2018). Beyond these structural functions, these enzymes have protective roles under stress conditions, as key regulators of extracellular H2O2 concentrations and as producers of reactive oxygen intermediates, such as hydroxyl radicals (•OH) and hydroperoxyl radicals (•OOH; Passardi et al., 2004; Bindschedler et al., 2006; Schweizer, 2008). In a recent study, PODs released from cell walls to apoplast were associated with protective responses to stress conditions and oxidative bursts (Podgórska and Burian, 2017). Hence, the regulation of POD activity may be achieved at transcriptional, translational (control of protein secretion to apoplasts) and post-translational levels (oxidative modifications, such as glutathionylation or nitrosylation) and at the level of in vivo substrate specificity (Minibayeva et al., 2015; Podgórska and Burian, 2017; Begara-Morales et al., 2019; Penel and Dunand, 2009).
The evidence presented in this study suggests that aleurone PODs, and particularly ZmPrx35, could be involved in wound-response signaling. The activity of these enzymes could be modulated by redox modifications, such as glutathionylation or nitrosylation (Beligni et al., 2002; Waszczak et al., 2015; Begara-Morales et al., 2019), or through inhibition by chemical compounds, such as ferulic acid, phytic acid, flavonoids, conjugated amines or hydrogen peroxide (Sen et al., 1994; Becraft, 2007; Ndolo et al., 2013). Our expression analysis of zmprx35 suggest that transcriptional activation follows wounding, and given the known quiescence of aleurone cells during dormancy (Schopfer et al., 2001; Becraft, 2007) and the magnitude of wounding, cell elongation and division for wound healing are unlikely. Thus, wound-induced signaling pathways and their consequent biochemical responses may remain active for long enough to induce de novo transcription in periodic pulses.
The increase in POD activity and zmprx35 mRNA expression observed after S. zeamais infestation is congruent with the oviposition habits of the insect females and the biochemical functions of aleurone cells. During oviposition, Sitophilus females merely puncture grain kernels and the subsequent grain weight losses are caused primarily by developing larvae (Sallam, 1999; Guedes et al., 2010). Thus, enhancements of POD activity and zmprx35 expression are likely associated with biochemical defense responses of aleurone cells to components of insect saliva. Previous research has identified saliva components from S. zeamais that induced the production of synomones in rice and wheat grains, which triggered an indirect defensive response by attracting females of the ectoparasitoid Theocolax elegans (Tang, 2016). This parasitoid has been suggested as a potential biocontrol agent for diverse Coleoptera that develops inside cereal grains or legume seeds, including S. zeamais (Dlamini and Amornsak, 2014; Adarkwah et al., 2019). Endosperm PODs and phenolic compounds have also been established as biochemical resistance factors (Arnason et al., 1992; Sen et al., 1994; García-Lara et al., 2007; López-Castillo et al., 2018b) that reinforce cell walls and improve pericarp thickness and toughness (Saulnier and Thibault, 1999; García-Lara et al., 2004). These associations have been determined in consideration of basal activities and concentrations of enzymes and metabolites. Yet, in models such as Nicotiana attenuata, defenses, including trypsin inhibitors and caffeoyl putrescine, are reportedly induced in response to Spodoptera exigua oviposition (Bandoly et al., 2015, 2016). Recently, hydroxycinnamic acid amides, such as diferuloyl putrescine, feruloyl putrescine, and cinnamoyl putrescine, have been detected in the pericarp and aleurone layers of various maize landraces (Burt et al., 2019). Thus, we suggest that defensive responses to S. zeamais are mediated by aleurone cells, in part through the activities of PODs such as ZmPrx35.
In the experiments with P. truncatus, the observed reduction in POD activity is consistent with the ability of this pest to metabolize lignocellulosic substrates, which allows their survival in non-agricultural hosts, including various tree species (Nansen and Meikle, 2002; Nansen et al., 2004). Collectively, these data suggest that the resistance of many maize strains, including Pob84-C3R, to P. truncatus is contributed by POD-independent mechanisms. Resistance to this pest has also been associated with kernel inhibitors of proteases and α-amylases (Blanco-Labra et al., 1995; Castro-Guillén et al., 2012), as shown in barley aleurone cells (Mundy and Rogers, 1986). Thus, quiescent but metabolically active maize aleurone cells (Becraft, 2007) likely play essential roles in the resistance against P. truncatus, presumably by playing protective roles in response to wounding and pest/pathogen attack during both germination and dormancy.
Conclusion
The protein ZmPrx35 was identified as the prevailing POD in endosperm cells of the insect-resistant maize Pob84-C3R. Although the immediate induction of aleurone POD activity was observed in response to mechanical wounding, these enzymes do not follow the canonical pattern of accumulation, and had fluctuating expression kinetics instead, potentially reflecting substrate inhibition. Moreover, zmprx35 transcript levels increased periodically, suggesting that the de novo synthesis of the enzyme is responsive to the mechanical wounding of aleurone cells.
Increases in both POD activity and zmprx35 expression in response to the maize weevil S. zeamais suggest defensive roles of ZmPrx35 in aleurone cells that could be enhanced by insect secretions.
In our experiments with the larger grain borer P. truncatus, POD activity was inhibited by pest attack but zmprx35 expression was not diminished, suggesting signaling roles of this gene in response to insect attacks. These data also confirm the presence of POD-independent resistance mechanism to the pest P. truncatus. Other chemical components of aleurone layers, such as amylase/protease inhibitors, are also plausible candidates that require further research. Beyond the digestive roles of aleurone layers during germination, these structures also play protective roles in response to wounding and pest attacks during seed dormancy.
Data Availability Statement
The datasets generated for this study can be found here: https://doi.org/10.5281/zenodo.3828063.
Author Contributions
LL-C, AG-L, MD-F-R, RW, and SG-L designed the research. LL-C, AG-L, and MD-F-R performed the research and analyzed the data. LL-C, AG-L, RW, NW, and SG-L wrote the manuscript.
Funding
This research was supported by the Research NutriOmics Chair Funds CAT-005 from Escuela de Ingenieria y Ciencias (Tecnologico de Monterrey). Publication fee was funded by Fondo Publicación Artículos Científicos (Escuela de Ingeniería y Ciencias, ITESM).
Conflict of Interest
The authors declare that the research was conducted in the absence of any commercial or financial relationships that could be construed as a potential conflict of interest.
Acknowledgments
The authors acknowledge Plinio A. Trinidad Calderón, Bernardo Martinez, César Villalobos, and Mariana Marrero for their contributions to this work.
Supplementary Material
The Supplementary Material for this article can be found online at: https://www.frontiersin.org/articles/10.3389/fpls.2020.00781/full#supplementary-material
References
Abebe, F., Tefera, T., Mugo, S., Beyene, Y., and Vidal, S. (2009). The resistance of maize varieties to the maize weevil Sitophilus zeamais (Motsch.) (Coleoptera: Curculionidae). Afr. J. Biotechnol. 8, 5937–5943. doi: 10.5897/ajb09.821
Adarkwah, C., Obeng-Ofori, D., Opuni-Frimpong, E., Ulrichs, C., and Schöller, M. (2019). Predator-parasitoid-host interaction: biological control of Rhyzopertha dominica and Sitophilus oryzae by a combination of Xylocoris flavipes and Theocolax elegans in stored cereals. Entomol. Exp. Appl. 167, 118–128.
Allison, S. D., and Schultz, J. C. (2004). Differential activity of peroxidase isozymes in response to wounding, gipsy moth, and plant hormones in Northern Red Oak (Quercus rubra L.). J. Chem. Ecol. 30, 1363–1379. doi: 10.1023/b:joec.0000037745.66972.3e
Arnason, J. T., Baum, B., Gale, J., Lambert, J. D. H., Bergvinson, D., Philogène, B. J. R., et al. (1994). Variation in resistance of Mexican landraces of maize to maize weevil Sitophilus zeamais, in relation to taxonomic and biochemical parameters. Euphytica 74, 227–236. doi: 10.1007/bf00040405
Arnason, J. T., Gale, J., Conilh de Beyssac, B., Sen, A., Miller, S. S., and Philogène, B. J. R. (1992). Role of phenolics in resistance of maize grain to the stored grain insects, Prostephanus truncatus (Horn) and Sitophilus zeamais (Motsch. J. Stored Prod. Res. 28, 119–126. doi: 10.1016/0022-474x(92)90019-m
Bandoly, M., Grichnik, R., Hilker, M., and Steppuhn, A. (2016). Priming of anti-herbivore defence in Nicotiana attenuata by insect oviposition: herbivore-specific effects. PlantCell Environ. 39, 848–859. doi: 10.1111/pce.12677
Bandoly, M., Hilker, M., and Steppuhn, A. (2015). Oviposition by Spodoptera exigua on Nicotiana attenuata primes induced plant defence against larval herbivory. Plant J. 83, 661–672. doi: 10.1111/tpj.12918
Barros-Rios, J., Santiago, R., Jung, H. J. G., and Malvar, R. A. (2015). Covalent cross-linking of cell-wall polysaccharides through esterified diferulates as a maize resistance mechanism against corn borers. J. Agric. Food Chem. 63, 2206–2214. doi: 10.1021/jf505341d
Becraft, P. W. (2007). “Aleurone cell development,” in Endosperm. Plant cell Monographs, ed. O. A. Olsen (Berlin: Springer), 45–56. doi: 10.1007/7089_2007_108
Begara-Morales, J. C., Chaki, M., Valderrama, R., Mata-Pérez, C., Padilla, M., and Barroso, J. B. (2019). “Transcriptional regulation of gene expression related to hydrogen peroxide (H2O2) and nitric oxide (NO),” in Nitric Oxide and Hydrogen Peroxide Signaling in Higher Plants, eds D. Gupta, J. Palma, and F. Corpas (Berlin: Springer), 69–90. doi: 10.1007/978-3-030-11129-8_4
Beligni, M. V., Fath, A., Bethke, P. C., Lamattina, L., and Jones, R. L. (2002). Nitric oxide acts as an antioxidant and delays programmed cell death in barley aleurone layers. Plant Physiol. 129, 1642–1650. doi: 10.1104/pp.002337
Bergvinson, D., and García-Lara, S. (2004). Genetic approaches to reducing losses of stored grain to insects and diseases. Curr. Opin. Plant Biol. 7, 480–485. doi: 10.1016/j.pbi.2004.05.001
Bestwick, C. S., Brown, I. R., and Mansfield, J. W. (1998). Localized changes in peroxidase activity accompany hydrogen peroxide generation during the development of a nonhost hypersensitive reaction in lettuce. Plant Physiol. 118, 1067–1078. doi: 10.1104/pp.118.3.1067
Bindschedler, L. V., Dewdney, J., Blee, K. A., Stone, J. M., Asai, T., Plotnikov, J., et al. (2006). Peroxidase-dependent apoplastic oxidative burst in Arabidopsis required for pathogen resistance. Plant J. 47, 851–863. doi: 10.1111/j.1365-313x.2006.02837.x
Blanco-Labra, A., Chagolla-López, A., Martínez-Gallardo, N. A., and Valdes-Rodríguez, S. (1995). Further characterization of the 12 kDa protease/alpha amylase inhibitor present in maize seeds. J. Food Biochem. 19, 27–41. doi: 10.1111/j.1745-4514.1995.tb00518.x
Boyer, S., Zhang, H., and Lempérière, G. (2012). A review of control methods and resistance mechanisms in stored-product insects. Bull. Entomol. Res. 102, 213–229. doi: 10.1017/s0007485311000654
Bradford, M. M. (1976). A rapid and sensitive method for the quantitation of microgram quantities of protein utilizing the principle of protein-dye binding. Anal. Biochem. 72, 248–254. doi: 10.1016/0003-2697(76)90527-3
Burt, A. J., Arnason, J. T., and García-lara, S. (2019). Natural variation of hydroxycinnamic acid amides in maize landraces. J. Cereal Sci. 88, 145–149. doi: 10.1016/j.jcs.2019.06.002
Castro-Guillén, J. L., Mendiola-Olaya, E., García-Gasca, T., and Blanco-Labra, A. (2012). Partial characterization of serine peptidases in larvae of Prostephanus truncatus (Horn) (Coleoptera: Bostrichidae), reveals insensitive peptidases to some plant peptidase inhibitors. J. Stored Prod. Res. 50, 28–35. doi: 10.1016/j.jspr.2012.04.002
Černý, M., Habánová, H., Berka, M., Luklová, M., and Brzobohatý, B. (2018). Hydrogen peroxide: its role in plant biology and crosstalk with signalling networks. Int. J. Mol. Sci. 19:2812. doi: 10.3390/ijms19092812
Cerquiglini, C., Claro, J., Giusti, A. M., Karumathy, G., Mancini, D., Marocco, E., et al. (2016). Food Outlook. Rome: Food and Agriculture Organization of the United Nations. 14.
Classen, D., Arnason, J. T., Serratos, J. A., Lambert, J. D. H., Nozzolillo, C., and Phylogène, B. J. R. (1990). Correlation of phenolic acid content of maize to resistance to Sitophilus zeamais, the maize weevil, in CIMMYT’s collections. J. Chem. Ecol. 16, 301–315.
Daiki, M., Tomoka, Y., Chandiona, M., Ephantus, N. B., Rodney, L. G., Jiwan, P. P., et al. (2017). Superiority of Malawian orange local maize variety in nutrients, cookability and storability. Afr. J. Agric. Res. 12, 1618–1628. doi: 10.5897/ajar2017.12138
Derera, J., Denash Giga, P., and Pixley, K. V. (2001a). Resistance of maize to the weevil: II. Non-Preference. Afr. Crop Sci. J. 9, 444–450.
Derera, J., Pixley, K. V., and Denash Giga, P. (2001b). Resistance of maize to the maize weevil: I. antibiosis. Afr. Crop Sci. J. 9, 431–440.
Deyhimi, F., and Nami, F. (2011). Peroxidase-catalyzed electrochemical assay of hydrogen peroxide: a ping–pong mechanism. Int. J. Chem. Kinetics 44, 699–704. doi: 10.1002/kin.20719
Díaz-Gómez, J. L., Castorena-Torres, F., Preciado-Ortiz, R. E., and García-Lara, S. (2017). Anti-cancer activity of maize bioactive peptides. Front. Chem. 5:44. doi: 10.3389/fchem.2017.00044
Dlamini, B. E., and Amornsak, W. (2014). Effect of host age on progeny production of Theocolax elegans (Westwood) (Hymenoptera: Pteromalidae) reared on Sitophilus zeamais (Motschulsky) (Coleoptera: Curculionidae). Kasetsart J. 48, 587–597.
Dunford, H. B. (1995). One-electron oxidations by peroxidases. Xenobiotica 25, 725–733. doi: 10.3109/00498259509061888
Fawal, N., Li, Q., Savelli, B., Brette, M., Passaia, G., Fabre, M., et al. (2013). PeroxiBase: a database for large-scale evolutionary analysis of peroxidases. Nucleic Acids Res. 41, 441–444.
Galende, P. P., Hidalgo, N., Ya, E., Roig, M. G., Villar, E., Shnyrov, V. L., et al. (2015). Kinetics of Spanish broom peroxidase obeys a Ping-Pong Bi – Bi mechanism with competitive inhibition by substrates. Int. J. Biol. Macromol. 81, 1005–1011. doi: 10.1016/j.ijbiomac.2015.09.042
Gambino, G., Perrone, I., and Gribaudo, I. (2008). A rapid and effective method for RNA extraction from different tissues of grapevine and other woody plants. Phytochem. Anal. 19, 520–525. doi: 10.1002/pca.1078
García-Lara, S., Arnason, J. T., Díaz-Pontones, D., Gonzalez, E., and Bergvinson, D. J. (2007). Soluble peroxidase activity in maize endosperm associated with maize weevil resistance. Crop Sci. 47, 1125–1130. doi: 10.2135/cropsci2006.10.0687
García-Lara, S., and Bergvinson, D. J. (2013). Identification of maize landraces with high level of resistance to storage pests Sitophilus zeamais Motschulsky and Prostephanus truncatus Horn in Latin America. Rev. Fitotecnia Mexicana 36, 347–356.
García-Lara, S., and Bergvinson, D. J. (2014). Phytochemical and nutraceutical changes during recurrent selection for storage pest resistance in tropical maize. Crop Sci. 54:2423.
García-Lara, S., Bergvinson, D. J., Burt, A. J., Ramputh, A. I., Díaz-Pontones, D. M., and Arnason, J. T. (2004). The role of pericarp cell wall components in maize weevil resistance. Crop Sci. 44, 1546–1552.
García-Lara, S., Ortíz-Islas, S., and Villers, P. (2013). Portable hermetic storage bag resistant to Prostephanus truncatus, Rhyzopertha dominica, and Callosobruchus maculatus. J. Stored Prod. Res. 54, 23–25.
Gitonga, Z. M., De Groote, H., Kassie, M., and Tefera, T. (2013). Impact of metal silos on households’ maize storage, storage losses and food security: an application of a propensity score matching. Food Policy 43, 44–55. doi: 10.1016/j.foodpol.2013.08.005
Guedes, N. M. P., Guedes, R. N. C., Campbell, J. F., and Throne, J. E. (2010). Contest behaviour of maize weevil larvae when competing within seeds. Anim. Behav. 79, 281–289. doi: 10.1016/j.anbehav.2009.10.022
Hiraga, S., Sasaki, K., Ito, H., Ohashi, Y., and Matsui, H. (2001). A large family of class III plant peroxidases. Plant Cell Physiol. 42, 462–468. doi: 10.1093/pcp/pce061
Holm, K. B., Andreasen, P. H., Eckloff, R. M. G., Kristensen, B. K., and Rasmussen, S. K. (2003). Three differentially expressed basic peroxidases from wound-lignifying Asparagus officinalis. J. Exp. Bot. 54, 2275–2284. doi: 10.1093/jxb/erg253
Lagrimini, L. M., Vaughn, J., Erb, W. A., and Miller, S. A. (1993). Peroxidase overproduction in tomato - wound-induced polyphenol deposition and disease resistance. HortScience 28, 218–221. doi: 10.21273/hortsci.28.3.218
Lin, Y., Zhang, C., Lan, H., Gao, S., Liu, H., Liu, J., et al. (2014). Validation of potential reference genes for qPCR in maize across abiotic stresses, hormone treatments, and tissue types. PLoS One 9:e95445. doi: 10.1371/journal.pone.0095445
López-Castillo, L. M., Díaz Flores-Rivera, M. F., Winkler, R., and García-Lara, S. (2018a). Increase of peroxidase activity in tropical maize after recurrent selection to storage pest resistance. J. Stored Prod. Res. 75, 47–55. doi: 10.1016/j.jspr.2017.11.007
López-Castillo, L. M., Silva-Fernández, S. E., Winkler, R., Bergvinson, D. J., Arnason, J. T., and García-Lara, S. (2018b). Postharvest insect resistance in maize. J. Stored Prod. Res. 77, 66–76.
López-Castillo, L. M., López-Arciniega, J. A. I., Guerrero-Rangel, A., Valdés-Rodríguez, S., Brieba, L. G., García-Lara, S., et al. (2015). Identification of B6T173 (ZmPrx35) as the prevailing peroxidase in highly insect-resistant maize (Zea mays, p84C3) kernels by activity-directed purification. Front. Plant Sci. 6:670. doi: 10.3389/fpls.2015.00670
Midega, C. A. O., Murage, A. W., Pittchar, J. O., and Khan, Z. R. (2016). Managing storage pests of maize: farmers’ knowledge, perceptions and practices in western Kenya. Crop Prot. 90, 142–149. doi: 10.1016/j.cropro.2016.08.033
Minibayeva, F., Beckett, R. P., and Kranner, I. (2015). Roles of apoplastic peroxidases in plant response to wounding. Phytochemistry 112, 122–129. doi: 10.1016/j.phytochem.2014.06.008
Minibayeva, F., Kolesnikov, O., Chasov, A., Beckett, R. P., LÜthje, S., Vylegzhanina, N., et al. (2009). Wound-induced apoplastic peroxidase activities: their roles in the production and detoxification of reactive oxygen species. Plant Cell Environ. 32, 497–508. doi: 10.1111/j.1365-3040.2009.01944.x
Mlambo, S., Mvumi, B. M., Stathers, T., Mubayiwa, M., and Nyabako, T. (2017). Field efficacy of hermetic and other maize grain storage options under smallholder farmer management. Crop Prot. 98, 198–210. doi: 10.1016/j.cropro.2017.04.001
Mundy, J., and Rogers, J. C. (1986). Selective expression of a probable amylase / protease inhibitor in barley aleurone cells: comparison to the barley amylase/subtilisin inhibitor. Planta 169, 51–63. doi: 10.1007/bf01369775
Mwololo, J., Mugo, S., Tefera, T., Sw, M., and Box, P. O. (2013). Evaluation of traits of resistance to postharvest insect pests in tropical maize. Int. J. Agric. Crop Sci. 6, 926–933.
Nansen, C., and Meikle, W. G. (2002). The biology of the larger grain borer, Prostephanus truncatus (Horn) (Coleoptera: Bostrichidae). Integrat. Pest Manag. Rev. 7, 91–104.
Nansen, C., Meikle, W. G., Tigar, B., Harding, S., and Tchabi, A. (2004). Nonagricultural hosts of Prostephanus truncatus (Coleoptera: Bostrichidae) in a West African forest. Ann. Entomol. Soc. Am. 97, 481–491. doi: 10.1603/0013-8746(2004)097[0481:nhoptc]2.0.co;2
Ndolo, V. U., Beta, T., and Fulcher, R. G. (2013). Ferulic acid fluorescence intensity profiles and concentration measured by HPLC in pigmented and non-pigmented cereals. Food Res. Int. 52, 109–118. doi: 10.1016/j.foodres.2013.02.031
Nwosu, L. C., Adedire, C. O., and Ogunwolu, E. O. (2015). Screening for new sources of resistance to Sitophilus zeamais motschulsky (Coleoptera: Curculionidae) infestation in stored maize genotypes. J. Crop Prot. 4, 277–229. doi: 10.12691/jfs-6-4-5
Passardi, F., Cosio, C., Penel, C., and Dunand, C. (2005). Peroxidases have more functions than a Swiss army knife. Plant Cell Rep. 24, 255–265. doi: 10.1007/s00299-005-0972-6
Passardi, F., Penel, C., and Dunand, C. (2004). Performing the paradoxical: how plant peroxidases modify the cell wall. Trends Plant Sci. 9, 534–540. doi: 10.1016/j.tplants.2004.09.002
Penel, C., and Dunand, C. (2009). “Signaling via plant peroxidases,” in Signaling in Plants, Signaling and Communication in Plants, eds F. Baluška and S. Mancuso (Heidelberg: Springer), 155–171. doi: 10.1007/978-3-540-89228-1_8
Podgórska, A., and Burian, M. (2017). Extra-cellular but extra-ordinarily important for cells: apoplastic reactive oxygen species metabolism. Front. Plant Sci. 8:1353. doi: 10.3389/fpls.2017.01353
Roach, T., Colville, L., Beckett, R. P., Minibayeva, F. V., Havaux, M., and Kranner, I. (2015). A proposed interplay between peroxidase, amine oxidase and lipoxygenase in the wounding-induced oxidative burst in Pisum sativum seedlings. Phytochemistry 112, 130–138. doi: 10.1016/j.phytochem.2014.06.003
Sallam, M. N. (1999). “. Insect damage. damage on post-harvest,” in InPho - Post-harvest Compendium, eds D. Mejia and B. Lewis (Nairobi, KE: Food and Agriculture Organization of the United Nations), 37.
Saulnier, L., and Thibault, J. F. (1999). Ferulic acid and diferulic acids as components of sugar-beet pectins and maize bran heteroxylans. J. Sci. Food Agric. 79, 396–402. doi: 10.1002/(sici)1097-0010(19990301)79:3<396::aid-jsfa262>3.0.co;2-b
Scalet, M., Federico, R., and Angelini, R. (1991). Time courses of diamine oxidase and peroxidase activities, and polyamine changes after mechanical injury of chick-pea seedlings. J. Plant Physiol. 137, 571–575. doi: 10.1016/s0176-1617(11)80701-7
Schopfer, P., Plachy, C., and Frahry, G. (2001). Release of reactive oxygen intermediates (superoxide radicals, hydrogen peroxide, and hydroxyl radicals) and peroxidase in germinating radish seeds controlled by light, gibberellin, and abscisic acid. Plant Physiol. 125, 1591–1602. doi: 10.1104/pp.125.4.1591
Schweizer, P. (2008). Tissue-specific expression of a defence-related peroxidase in transgenic wheat potentiates cell death in pathogen-attacked leaf epidermis. Mol. Plant Pathol. 9, 45–57. doi: 10.1111/j.1364-3703.2007.00446.x
Sen, A., Bergvinson, D., Miller, S. S., Atkinson, J., Fulcher, R. G., and Arnason, J. T. (1994). Distribution and microchemical detection of phenolic acids, flavonoids, and phenolic acid amides in maize kernels. J. Agric. Food Chem. 42, 1879–1883. doi: 10.1021/jf00045a009
Shevchenko, A., Sunyaev, S., Loboda, A., Shevchenko, A., Bork, P., Ens, W., et al. (2001). Charting the proteomes of organisms with unsequenced genomes by MALDI-quadrupole time-of-flight mass spectrometry and BLAST homology searching. Anal. Chem. 73, 1917–1926. doi: 10.1021/ac0013709
Shevchenko, A., Tomas, H., Havlis, J., Olsen, J. V., and Mann, M. (2006). In-gel digestion for mass spectrometric characterization of proteins and proteomes. Nat. Protoc. 1, 2856–2860. doi: 10.1038/nprot.2006.468
Shigeto, J., and Tsutsumi, Y. (2016). Diverse functions and reactions of class III peroxidases. New Phytol. 209, 1395–1402. doi: 10.1111/nph.13738
Singh, H., Dixit, S., Verma, P. C., and Singh, P. K. (2013). Differential peroxidase activities in three different crops upon insect feeding. Plant Signal. Behav. 8, 1–7.
Tang, Q. (2016). Sitophilus zeamais - induced rice grain volatiles: attractiveness towards the generalist parasitoid wasp. Theocolax elegans. Pakistan J. Zool. 48, 1817–1824.
Tefera, T., Mugo, S., and Beyene, Y. (2016). Developing and deploying insect resistant maize varieties to reduce pre-and post-harvest food losses in Africa. Food Secur. 8, 211–220. doi: 10.1007/s12571-015-0537-7
Tefera, T., Mugo, S., Likhayo, P., and Beyene, Y. (2011). Resistance of three-way cross experimental maize hybrids to post-harvest insect pests, the larger grain borer (Prostephanus truncatus) and maize weevil (Sitophilus zeamais). Int. J. Trop. Insect Sci. 31, 3–12. doi: 10.1017/s1742758411000075
Wang, L., Xu, C., Qu, M., and Zhang, J. (2008). Kernel amino acid composition and protein content of introgression lines from Zea mays ssp. mexicana into cultivated maize. J. Cereal Sci. 48, 387–393. doi: 10.1016/j.jcs.2007.09.014
Waszczak, C., Akter, S., Jacques, S., Huang, J., and Messens, J. (2015). Oxidative post-translational modifications of cysteine residues in plant signal transduction. J. Exp. Bot. 66, 2923–2934. doi: 10.1093/jxb/erv084
Winkler, R., and García-Lara, S. (2010). Activity-directed identification of maize kernel peroxidases associated with postharvest insect resistance. Mol. Bio Syst. 6, 1810–1812.
Keywords: aleurone, grain quiescence, insect damage, insect resistance, peroxidase, Zea mays
Citation: López-Castillo LM, González-Leyzaola A, Diaz-Flores-Rivera MF, Winkler R, Wielsch N and García-Lara S (2020) Modulation of Aleurone Peroxidases in Kernels of Insect-Resistant Maize (Zea mays L.; Pob84-C3R) After Mechanical and Insect Damage. Front. Plant Sci. 11:781. doi: 10.3389/fpls.2020.00781
Received: 14 September 2019; Accepted: 15 May 2020;
Published: 11 June 2020.
Edited by:
Wang Wei, Henan Agricultural University, ChinaReviewed by:
R. Glen Uhrig, University of Alberta, CanadaLyudmila Petrova Simova-Stoilova, Institute of Plant Physiology and Genetics (BAS), Bulgaria
Copyright © 2020 López-Castillo, González-Leyzaola, Diaz-Flores-Rivera, Winkler, Wielsch and García-Lara. This is an open-access article distributed under the terms of the Creative Commons Attribution License (CC BY). The use, distribution or reproduction in other forums is permitted, provided the original author(s) and the copyright owner(s) are credited and that the original publication in this journal is cited, in accordance with accepted academic practice. No use, distribution or reproduction is permitted which does not comply with these terms.
*Correspondence: Silverio García-Lara, c2dhcmNpYWxhcmFAdGVjLm14