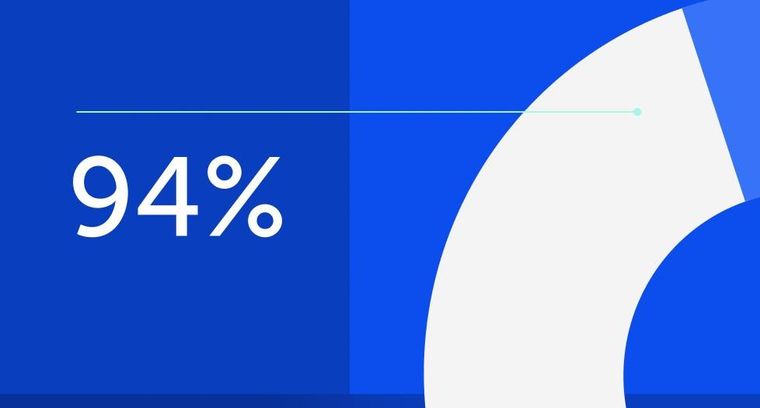
94% of researchers rate our articles as excellent or good
Learn more about the work of our research integrity team to safeguard the quality of each article we publish.
Find out more
ORIGINAL RESEARCH article
Front. Plant Sci., 03 June 2020
Sec. Plant Systematics and Evolution
Volume 11 - 2020 | https://doi.org/10.3389/fpls.2020.00719
This article is part of the Research TopicChromosomal Evolution in PlantsView all 18 articles
The tribe Aethionemeae is sister to all other crucifers, making it a crucial group for unraveling genome evolution and phylogenetic relationships within the crown group Brassicaceae. In this study, we extend the analysis of Brassicaceae genomic blocks (GBs) to Aethionema whereby we identified unique block boundaries shared only with the tribe Arabideae. This was achieved using bioinformatic methods to analyze synteny between the recently updated genome sequence of Aethionema arabicum and other high-quality Brassicaceae genome sequences. We show that compared to the largely conserved genomic structure of most non-polyploid Brassicaceae lineages, GBs are highly rearranged in Aethionema. Furthermore, we detected similarities between the genomes of Aethionema and Arabis alpina, in which also a high number of genomic rearrangements compared to those of other Brassicaceae was found. These similarities suggest that tribe Arabideae, a clade showing conflicting phylogenetic position between studies, may have diverged before diversification of the other major lineages, and highlight the potential of synteny information for phylogenetic inference.
The Brassicaceae is an economically important plant family, containing the Brassica crops, rapeseed and several ornamental taxa (e.g., Aubrieta, Iberis). Due to the availability of abundant genomic resources, such as the high-quality reference genome for model plant Arabidopsis thaliana, the family has become a model system for studying plant trait, genome and chromosomal evolution. The Brassicaceae family diverged from its sister-family, the Cleomaceae, ∼43 mya (million years ago) (Schranz and Mitchell-Olds, 2006; Edger et al., 2018). Divergence of tribe Aethionemeae, sister lineage to all other Brassicaceae, with its single genus Aethionema occurred ∼32 mya (Hohmann et al., 2015). The subsequent diversification of the rest of the family, or “crown-group,” started ∼23 mya (Hohmann et al., 2015). The crown-group includes ∼3,900 species in 350 genera, grouped into 51 monophyletic tribes1 (BrassiBase; Koch et al., 2018). These tribes are further grouped into either three or five major lineages, termed I–III or A–E (Koch and Al-Shehbaz, 2009; Franzke et al., 2011; Huang et al., 2016; Nikolov et al., 2019).
Despite the wealth of sequence information used for recent phylogenetic reconstructions, the deeper nodes of the crown group Brassicaceae, including between lineages, are still not fully resolved. All data show Aethionemeae as the first diverging lineage. However, differing branching orders of the crown-group lineages have been reported. This is largely due to conflicting signals between plastome and nuclear data. Recent phylogenies based on extensive nuclear genome data support lineage III/E, including for example Euclidium syriacum, as sister to lineages I/A, including model species A. thaliana, and II/B, including the Brassica crops (Huang et al., 2016; Kiefer et al., 2019; Nikolov et al., 2019; Figure 1A). Plastome sequence based phylogenies on the other hand consistently place lineage II/B and III/E as sister to lineage I/A (Guo et al., 2017; Mabry et al., 2019; Nikolov et al., 2019; Figure 1B). Additionally, tribe Arabideae, including the important model plant Arabis alpina, is placed either as sister to lineages I/A and II/B (Kiefer et al., 2019; Nikolov et al., 2019; Figure 1A) or within lineage II/B (e.g., Hohmann et al., 2015; Guo et al., 2017; Figure 1B). Given the importance of Brassicaceae as a model system, a resolved and reliable backbone phylogeny is a crucial prerequisite for understanding genome and trait evolution on a family-wide scale.
Figure 1. Three potential evolutionary scenarios for the backbone phylogeny of Brassicaceae. (A) Nuclear data places lineage III/E as sister to the rest of the family, with Arabideae branching off next and outside of lineage II/B (Kiefer et al., 2019; Nikolov et al., 2019). (B) Phylogenetic reconstruction based on plastome data consistently places lineage I/A as sister to lineages II/B and III/E, and Arabideae in lineage II/B or extended lineage II (e.g., Hohmann et al., 2015; Guo et al., 2017). (C) Genomic blocks of Arabis alpina and Aethionema arabicum show some similarities indicating that Arabideae may be the first branching clade in crown group Brassicaceae (this study). The At-α WGD occurred sometime before divergence of Brassicaceae. Note that these three scenarios are not a comprehensive summary of all Brassicaceae phylogenies – other tree topologies have been published (e.g., Beilstein et al., 2010), however, here we only show topologies from recent genomic studies based on a high number of genes. Species names are representatives of their evolutionary lineages and were not necessarily included in the cited studies.
To facilitate comparative genomics and studies of genome evolution, a reference system of genomic blocks (GBs) was established for Brassicaceae genomes (Schranz et al., 2006). Originally inferred from genetic maps and the first available whole genome sequences (A. thaliana, Arabidopsis lyrata, Capsella rubella and Brassica rapa), this resulted in the description of the ACK (ancestral crucifer karyotype) with eight chromosomes termed AK1–AK8 and 24 GBs (A–X). Despite its name, the ACK was not the ancestral genome of the Brassicaceae, but rather it can be seen as the hypothetical genome of the MRCA (most recent common ancestor) of lineages I/A and II/B. Since its description, the release of additional whole genome sequences as well as comparative cytogenetic analyses have led to the family-wide expansion of the genomic-block concept and reduction to 22 conserved GBs (Lysak et al., 2016). The PCK (Proto-Calepineae Karyotype, n = 7) was described as the ancestral karyotype for clades of lineage II/B (Mandáková and Lysak, 2008), and the CEK (Clade E Karyotype, n = 7) as the ancestral karyotype of lineage III/E (Mandáková et al., 2017a). The CEK genome bears some resemblance to the organization of GBs in the A. alpina genome (Willing et al., 2015). A high number of within-GB breakpoints compared to the ACK has been observed in both the CEK and A. alpina genomes, thus raising the question whether these clades may indeed be more closely related than supported by plastome data. Analysis of GBs can be conducted either with cytogenetic methods, or using bioinformatics. Cytogenetic methods usually rely on Bacterial Artifical Chromosome (BAC) clones, often from A. thaliana when studying Brassicaceae species, for chromosome painting. This method has the advantage that even species with little genomic information available can be studied; however, it is limited by the need for BAC clones from more or less closely related taxa. In contrast, bioinformatic methods can be applied even to distantly related species, but high-quality genomic information is required to identify genomic collinearity (syntenic blocks). The evolutionary distance between Aethionema and Arabidopsis limits the success of chromosome painting, and a high-quality reference genome was so far unavailable. Thus, no species from the more distantly related tribe Aethionemeae has been analyzed in the context of the GBs using either method.
The genus Aethionema comprises 57 species (BrassiBase, see footnote 1; Koch et al., 2018). It most likely originated in the Anatolian Diagonal, and dispersed throughout the Irano-Turanian region and large parts of the Mediterranean (Mohammadin et al., 2017). Over the past few years, the genus has been used to study fruit dimorphism (Lenser et al., 2016, 2018; Wilhelmsson et al., 2019) and seed germination (Mérai et al., 2019), in particular using the species Aethionema arabicum (e.g., the ERA-CAPS SeedAdapt project). Its divergence occurred sometime after the Brassicaceae-specific At-α WGD (whole genome duplication) (Figure 1). Ancient WGDs are thought to be associated with diversification (Tank et al., 2015), and duplicated genes originating from these events may play an important role for evolving new traits (Hofberger et al., 2013). Remnants of repeated ancient WGDs are found in all land plants (One Thousand Plant Transcriptomes Initiative, 2019), with some of them shared between orders and families, and some family-specific. In addition to At-α, the At-β event specific to the core Brassicales (Edger et al., 2015) is of particular importance to the Brassicaceae. The evolution of glucosinolates, secondary compounds involved in herbivore defense, and the family’s coevolution with Pieridae butterflies is most likely associated with gene family expansion due to WGD following At-β and At-α (Hofberger et al., 2013; Edger et al., 2015). Following polyploidization, genomes often undergo genome size reduction eventually leading to diploidization, a process also referred to as genome fractionation. Genome downsizing is generally accompanied by chromosomal rearrangements and gene loss. The phylogenetic position of Aethionema as sister to all other Brassicaceae makes this lineage a crucial link that is needed to understand genome evolution after WGD in Brassicaceae.
The observation that diversifications after WGDs often occur after a considerable time lag and exclude a species-poor sister lineage that shares the WGD has been formalized in the “WGD radiation lag-time model” (Schranz et al., 2012). Diversification of the species-rich and successfully diversifying Brassicaceae crown group contrasted by species-poor tribe Aethionemeae follows this pattern (Schranz et al., 2012). Fractionation and unequal gene loss may be responsible, but this hypothesis still needs to be tested (Schranz et al., 2012). An updated and substantially improved reference genome for Ae. arabicum was recently released (Ae. arabicum genome v3.0; Nguyen et al., 2019). Its chromosome-level assembly of the eleven linkage groups corresponding to the chromosomes (n = 11) allows us to apply the concept of GBs to Aethionema, and analyze the genomic structure of the sister clade to all other Brassicaceae in more detail.
Phylogenetics have so far failed to resolve the deeper nodes within Brassicaceae, even using ever larger transcriptome data sets. Instead of relying on nucleotide sequences, using genomic features such as synteny and/or chromosomal rearrangements could therefore prove to be a useful tool to resolve such problematic nodes and disentangle phylogenetic placement of Brassicaceae lineages. Here, we present the syntenic blocks in the genome of Ae. arabicum and explore open questions concerning genome evolution and phylogenetics in Brassicaceae: Given the early divergence of Aethionema and its position as the species-poor sister group, is its genomic structure similar to the ACK and the largely conserved genomic structure of crown group Brassicaceae? Are the same breakpoints observed between Aethionema, Arabis and CEK genomes, and can synteny be used to obtain evidence for the phylogenetic position of early diverging lineages? We show that compared to the ACK, the syntenic blocks in the genome of Aethionema are broken into a high number of sub-blocks across its linkage groups. Among the high number of breakpoints, we observed, some are shared with A. alpina and E. syriacum representing the ancestral CEK genome. Our results suggest that Arabideae may have diverged before diversification of lineages I–III.
Our analysis revealed 13,719 syntenic genes between Aethionema and A. thaliana that are in syntenic blocks. These blocks are defined as regions sharing at least 20 collinear genes (our chosen threshold for the detection of GBs) when disregarding syntenic regions originating from the At-α, At-β, and segmental duplication events. The duplicated regions could easily be identified using Ks values; orthologous blocks generally had median Ks values of around 0.77 (purple colored in Figure 2A), while mean Ks values for blocks derived from At-α and older duplication events (WGD-derived paralogs) were higher, around 1.37 (blue and turquoise colored in Figure 2A). The average length of the syntenic blocks was 199 ± 183 (mean ± SD) syntenic genes, ranging from 23 to 833 genes. Using the same analysis and parameters on the Arabis genome resulted in the detection of 16,588 syntenic genes with an average block length of 313 ± 373 genes, ranging from 25 to 1,507 genes in a block. Here, Ks was lower for orthologous blocks (0.41) and At-α derived blocks (1.01). This difference is most likely the result of the additive effect of lineage-specific substitution, leading to a higher number of substitutions in the pairwise comparison of the more divergent species. The difference in syntenic block length is also reflected by the number of syntenic blocks: 69 were detected in the eleven linkage groups of the Aethionema genome (Figure 2B), compared to 53 in Arabis. All 22 GBs from the ACK (following the updated definition by Lysak et al. (2016) were present in Aethionema. However, genomic block G was not detected when setting the minimum number of genes in a syntenic block to 20. When not restricting block size, G was detected (with six syntenic genes) on LG9 between F4 and H, the same position this block has in the ACK.
Figure 2. Aethionema arabicum genomic blocks. (A) Syntenic dotplot of Ae. arabicum and Arabidopsis lyrata, which closely resembles the ACK. The dotplot was generated using SynMap implemented in CoGe. Syntenic genes are colored by Ks values to help differentiate between orthologs and At-α, At-β or segmental duplication derived paralogs. Assignment to genomic blocks is given on the left for Aethionema and below for Arabidopsis. Red boxes highlight At-α derived blocks syntenic to contiguous blocks detected in the Aethionema/Arabis comparison, blue boxes their orthologous counterparts. (B) Genomic blocks on the eleven linkage groups of Ae. arabicum. Up to eight sub-blocks were detected and blocks are ordered relative to the ACK. Inversions are indicated by upside-down block names. For reasons of consistency, genomic block G is shown despite its size below our chosen 20 genes threshold. (C) Histogram of synonymous substitution rate Ks. Color scheme corresponds to that used in panel (A).
Analysis of the syntenic regions in the genome of Ae. arabicum revealed the duplicated regions originating from gene and ancient whole genome duplications. In reciprocal analyses (self–self comparisons), Ks values of around 0.8 are generally indicative of duplicates retained from At-α (Schranz et al., 2012). Higher Ks values are characteristic for duplicated genes originating from the At-β and older WGDs (Tang and Lyons, 2012). In pairwise analyses, like we conducted here, these characteristic Ks values are increased relative to the divergence of the selected species, as each lineage accumulates their own mutations after divergence, thus median Ks for orthologs was between 0.58 and 0.94 (Figures 2A,C).
Notably, in Ks histograms of Aethionema vs. other Brassicaceae the peak from orthologs is almost indistinguishable from that resulting from At-α duplicates. However, the origin of syntenic regions can clearly be distinguished in the syntenic dotplots (Figure 2A and Supplementary Figures 1–3). Median Ks in all histograms of pairwise comparisons involving Aethionema is around 0.8 (Supplementary Figure 4). This is not the case when comparing other Brassicaceae species, where Ks values are between 0.44 and 0.52. The similar Ks values between orthologs and paralogs (At-α derived) in Aethionema are consistent with only a relatively short time passing between At-α and divergence of Aethionema from the rest of Brassicaceae, but diversification of the crown group having occurred with some delay. Nevertheless, the small differences between orthologs and paralogs in Aethionema are sufficient to differentiate between the two in the syntenic dotplots (Figure 2A), as well as using median Ks of syntenic blocks. In addition, gene content between orthologs is more similar compared to paralogs, due to unequal fractionation, with paralogs generally containing more syntenic genes.
Most GBs in crown group diploid Brassicaceae species are conserved, i.e., they are not broken into sub-blocks. Interestingly, when within-block breaks and rearrangements are observed, this most often involved AK6 and AK8 [PCK; (Mandáková and Lysak, 2008)] and additionally AK4 [Arabideae; (Mandáková et al., 2020)], with a maximum number of three sub-blocks. This is, however, not the case in Aethionema. GBs from all eight chromosomes of the ACK show multiple within-block breakpoints, with the exception of three GBs (G, H, and K–L). The three GBs that did not break into sub-blocks in Aethionema are also conserved as a unit throughout most Brassicaceae lineages. Both G and H are present as a single block each on AK3 of the ACK, and they are also conserved in the PCK, CEK, Arabis and in the more rearranged A. thaliana genome. K-L is conserved as a unit in most lineages of Brassicaceae, but split into K and L in A. thaliana, and bioinformatic analysis also detected a small segment (35 genes) of K-L on A. alpina chromosome 3, while the largest part of this GB was detected on chromosome 5.
Apart from conserved blocks, also conserved shared GB associations can be observed across the family. While in crown group species only few new GB associations were created through rearrangements (e.g., translocations, inversions), this is the case for almost all blocks in Aethionema. Only four GB associations are shared between ACK, PCK, CEK, Arabideae, and Aethionema. The A-B association on AK1 can be found on LG-7 (A4-B1), the F-G association on AK3 on LG-8 (F4-G), the G–H association on AK3 is located on LG-8 as well, and the I–J association on AK4 is located on LG-5 (I2–J1). Notably, the I–J association is not found in Arabis, but a recent analysis of Arabideae revealed that this association is conserved in Pseudoturritis turrita, the sister to all other Arabideae, while it is not retained in later diverging Arabideae genera (Mandáková et al., 2020) and therefore the lack of this association can be considered the derived state in Arabis.
To explore whether genomic features could help resolve the deeper nodes of the Brassicaceae phylogeny, we searched for shared breakpoints and boundaries between blocks and sub-blocks in the genome sequences of Aethionema and Arabis that are not present in the ACK. As no chromosome-level assembly from any species of lineage III/E are yet available, we could not extend our analysis to this lineage. Instead, we searched the E. syriacum genome for the syntenic regions of interest identified in the Aethionema–Arabis analysis. Three regions of interest were identified that represent shared block and sub-block boundaries or similar breakpoints.
We identified three shared unique boundaries between Aethionema and Arabis: J1-V2-O; U3-B5; and V1-O2-P. First, the association J1-V2-O1 from LG-5 (Figure 3A) corresponds to the Ja-V-O association on chromosome Ar6 across Arabideae (Willing et al., 2015; Mandáková et al., 2020). In Euclidium, the genome segment containing the J-V-O segment is assembled in one fragment, but contains only partial and inverted V and O orthologs. Comparative chromosome painting did not detect V on chromosome Es3 in E. syriacum (Mandáková et al., 2017a). Interestingly, on chromosome Ct6 in Chorispora tenella (also lineage III/E), V and a small fragment of neighboring block W have inserted between Ja and Oa (Mandáková et al., 2017a). However, the genome sequence of the species would be required to determine if the breakpoints are identical with those identified in Aethionema and Arabideae genomes. Second, the boundary U3-B5 on LG-6 (Figure 3B) is found on chromosome 7 of the Arabis genome. The B segment was not detected using chromosome painting on this chromosome in Arabis (Willing et al., 2015; Mandáková et al., 2020), but could be detected using SynMap (Supplementary Figure 1A). In Euclidium, this fragment of the genome was not assembled contiguously, therefore no conclusions on lineage III/E can be drawn until a chromosome-level assembly becomes available. Finally, the association V1-O2-P1 on LG-9 (Figure 3C) is also detected on chromosome 6 in the Arabis genome using bioinformatic methods. In contrast to the other three regions, this association is also shared with Euclidium according to our analysis of its genome sequence. However, cytogenetic analyses did not show evidence of block V in vicinity of O in the Euclidium genome (Mandáková et al., 2017a), potentially due to the small size of the respective blocks (in Arabis, the V sub-block was only 73 syntenic genes long). Detailed figures of the three genomic regions in all pairwise comparisons are shown in Supplementary Figures 5–7.
Figure 3. Three syntenic regions in Aethionema and Arabis. (A) Sub-blocks O1, V2 and J1 are present as a unit on LG5 of Aethionema and chromosome 6 of Arabis, but not contiguous in A. lyrata. In Euclidium, large parts of O and parts of V and J are missing, and the O-V fragment is inverted relative to Aethionema and Arabis. (B) U3 and B5 are contiguous in Aethionema on LG6 and Arabis on chromosome 7, but not in A. lyrata. (C) V1 and O2 on LG9 of Aethionema and chromosome 6 of Arabis are associated, which is also detected in Euclidium, but not in Arabidopsis. More detailed figures of the three genomic regions in all pairwise comparisons are shown in Supplementary Figures 5–7.
In support of the potential “ancestral state” of the three aforementioned shared breakpoints between Aethionema and Arabis, the older At-α derived paralogous blocks are highly syntenic to all three regions (highlighted in red boxes in Figure 2A and Supplementary Figures 1–3; the respective orthologs are highlighted in blue). The J1-V2-O1 block detected in Aethionema and Arabis is syntenic to a part of A on the AK1, the U3-B5 block is syntenic to a part of O on AK6, and the V1-O2-P1 block from Aethionema, Arabis and Euclidium is syntenic to a part of U on AK7. This similarity of At-α blocks and continuous blocks in Aethionema and Arabis can be seen as strong evidence for the ancestral status of these genomic regions in the two species, with subsequent rearrangements leading to the blocks building up the ACK.
In genomes of lineage III/E and Arabis, blocks from AK4, AK6, AK7, and AK8 are subject to extensive rearrangements and within-block breaks. The association of GBs P and V in particular is observed in Arabis on chromosome Ar6 and conserved across Arabideae (Mandáková et al., 2020). Interestingly, the association V3-P2 on LG-9 is also detected in Aethionema. However, different block borders are associated, suggesting multiple independent origins. In Euclidium, this genomic region was again not assembled contiguously, and no conclusion on lineage III/E can be drawn from genome sequence data. However, cytogenetic analyses have not shown evidence for an association of blocks V and P in any species from this lineage (Mandáková et al., 2017a).
Here, we analyzed GBs in the genome of Ae arabicum; comparison with A. alpina and E. syriacum provide evidence for a new placement of Arabis within the Brassicaceae. The phylogenetic position of Aethionema as sister of all other Brassicaceae lineages makes this genus particularly interesting in the context of crucifer genome evolution. Due to the earlier availability of genomes and genetic maps of species from lineages I/A and II/B, comparative genomics in Brassicaceae was traditionally conducted relative to the ACK (n = 8). The recent update and improvement of the Ae arabicum genome sequence (Nguyen et al., 2019) to chromosome-level assembly of the eleven linkage groups has allowed us to apply the system of GBs previously used for other Brassicaceae lineages to tribe Aethionemeae. Compared to other Brassicaceae species, the GBs of the ACK are broken into multiple sub-blocks in Aethionema. Interestingly, the genomes of tribe Arabideae (Mandáková et al., 2020) and lineage III/E (Mandáková et al., 2017a) also contain a higher number of sub-blocks than “diploid” genomes from lineage I/A and II/B. A high number of within-block breaks is also observed in diploidized mesopolyploid genomes (e.g., Lysak et al., 2016; Mandáková et al., 2017b) or meso-neopolyploid ones, such as allohexaploid genomes of Camelina sativa (Mandáková et al., 2019) and B. rapa (Cheng et al., 2013) from lineage I/A and II/B, respectively. However, mesopolyploidization has not been observed in any tribe belonging to lineage III/E (Mandáková et al., 2017a, b), and the Arabideae have also not undergone a WGD post-dating the At-alpha (Willing et al., 2015; Mandáková et al., 2020). Thus, there seem to be two different reasons for elevated fractionation of GBs. While homeologous and ectopic recombination between the duplicated GBs, accompanying post-polyploid diploidization of the mesopolyploid genomes, explains the high number of within-block breakpoints in these genomes, the very definition of ACK and 22 building blocks (Schranz et al., 2006; Lysak et al., 2016) does not reflect the structure of more ancestral genomes of lineage III/E, Arabideae and Aethionema. The ACK represents a diploidized genome derived from a more ancestral paleotetraploid genome most likely resembling that of Ae. arabicum and to lesser extent those of Arabideae and lineage III/E tribes. With the caveat that phylogenetic position of some crucifer genera and clades remains unresolved as evident by low support values at deeper nodes (Nikolov et al., 2019) and conflicting topologies between studies (e.g., Beilstein et al., 2010; Huang et al., 2016; Nikolov et al., 2019), the phylogenetic placement of ACK is revisited as an ancestral genome of lineage I/A and lineage II/B (Mandáková et al., 2017a).
The backbone phylogeny of Brassicaceae has been a subject of debate in recent years, with conflicting signals from plastome and nuclear genome data, and low resolution at deeper nodes despite large data sets. The use of non-nucleotide genomic data, such as patterns of synteny, may thus help in resolving these nodes. Tools to reconstruct phylogenetic trees based on genome rearrangement patterns have been developed recently (Drillon et al., 2020; Zhao et al., 2020), and first angiosperm-wide phylogenetic reconstructions based on synteny data have shown that these methods may provide alternative phylogenetic evidence for controversial nodes (Zhao et al., 2020). Our analysis of the genome structure of Aethionema and Arabis in the context of the entire Brassicaceae family was aimed at identifying evidence for the phylogenetic position of Arabideae relative to lineage II/B, where the tribe is consistently placed using plastome (Franzke et al., 2011; Hohmann et al., 2015; Guo et al., 2017; Figure 3A), but not nuclear genome data (Nikolov et al., 2019; Figure 3B), and lineage III/E. Comparative cytogenomic analyses of lineage III/E and Arabideae genera have revealed extensive chromosome reshuffling in these potentially earlier diverging branches of Brassicaceae (Willing et al., 2015; Mandáková et al., 2017a). Our analysis of GBs in Ae. arabicum shows that four block associations are shared between Aethionema and Arabis, indicating that these associations may have been the ancestral state. The breakpoints of these blocks and sub-blocks coincided with assembly borders in the genome sequence of Euclidium in one case, and thus we had to rely on evidence from cytogenetic data to infer the status of synteny at this region in lineage III/E. One shared association was observed in Aethionema, Arabis and Euclidium. This boundary notably does not involve any within-block borders, but the outer borders of blocks O and V (from AK 6 and AK7, respectively). Two further associations were shared between Aethionema and Arabis on LG5 of the Aethionema genome. The three sub-blocks (O1-V2-J1) were detected in the same order in Aethionema and Arabis, but the genome assembly of Euclidium indicated two gaps and an inversion at this location. Interestingly, here the V2 sub-block was not located at the edge of GB V from ACK, thus only internal breakpoints were involved.
The presence of identical characters in different lineages can, in short, be explained by two different processes: Either they are derived and originated independently in the respective lineages, or they are ancestral and were lost sometime in the past in the lineages that do not contain them. Two possible explanations and evolutionary scenarios may thus be invoked for the interpretation of our results. The first scenario follows previous interpretations of the ACK as the ancestral genome of Brassicaceae. In this case, the rearranged genomes of lineage III/E, A. alpina and Ae arabicum are derived from an ancestral Brassicaceae genome similar to the ACK. Their apparent similarity could be the result of frequent reuse of breakpoints. In the second scenario, the blocks from the ACK are the derived state and originated from an ancestral Brassicaceae genome somewhat resembling the genomes of Aethionema, Arabis and lineage III/E. Having a lower number of required changes, this seems to be the slightly more parsimonious scenario given our current data, and synteny with continuous At-α derived blocks additionally supports our claim. Altogether, our results suggest that the Arabis clade diverged first within the Brassicaceae crown group, followed by lineage III/E and finally the most species-rich groups of lineages I/A and II/B (see Figure 1C).
To further advance our understanding of genome evolution in Brassicaceae, genome reconstruction of the family’s most recent common ancestor, the post At-α genome, before divergence of Aethionema, is needed. This would allow for a redefinition of GBs relative to this presumed ancestral genome and for analysis of genome evolution in all lineages of the family. Whereas multiple high-quality genomes from lineages I/A and II/B are available, comparable genome sequences are not available yet for other crucifer clades. Chromosome-level assemblies from lineage III/E would allow us to test hypotheses regarding the backbone phylogeny and placement of lineage III/E as well as tribe Arabideae in more detail. In particular, the similarity of lineage III/E genomes with the ACK should be studied further. Additionally, the genome sequences of other Aethionema species, preferably some that diverged from Ae. arabicum early in the evolution of the tribe, would allow us to determine an ancestral karyotype of tribe Aethionemeae and to conclude whether the eleven Aethionema linkage groups represent the relic At-α genome frozen in time or a reshuffled paleotetraploid genome. This would also give us the opportunity to study the genome evolution of this species-poor sister clade, and could shed some light on why Aethionemeae did not diversify like the rest of Brassicaceae.
We identified syntenic blocks in the updated reference genome of Ae. arabicum v.3 (Nguyen et al., 2019) relative to the 22 GBs in ACK (Schranz et al., 2006; Lysak et al., 2016). Note that throughout the manuscript, we refer to bioinformatically or cytogenomically detected syntenic blocks in extant species as “syntenic blocks” or simply “blocks” and to those of the ACK as “genomic blocks” or “GBs.” The CoGe platform2 (Lyons et al., 2008) was used to detect syntenic regions between Ae. arabicum and A. thaliana, as GBs in the ACK are defined using the A. thaliana gene IDs as start and end coordinates. Orthologous genes were identified using the BlastZ algorithm, and synteny was identified using DAGchainer (Haas et al., 2004). To obtain larger syntenic regions, we set the maximum distance between two matches in DAGchainer to 25, and only retained blocks with a minimum number of 20 retained pairs. For reasons of consistency, an exception was made for block G, which could only be identified with default settings, as it only contained six genes. We merged syntenic regions using QuotaAlign (Tang et al., 2011) with a maximum distance of 50 genes. In order to differentiate between syntenic blocks representing the GBs and those retained from At-α, we calculated the synonymous substitution rate (Ks) using CodeML (Yang, 2007) as implemented in CoGe. For values <2, Ks is relatively linear with time (Vanneste et al., 2013) and can be used to distinguish between orthologs between species, At-α derived genes or blocks with a Ks value around 0.8 (Schranz et al., 2012), recently duplicated genes, and duplicated genes with an even older origin, for example from the At-β WGD. Blocks with median Ks values corresponding to a WGD were discarded. Note that due to the additive effect of lineage-specific substitutions, Ks values for pairwise comparisons are higher with longer divergence time. In the Aethionema-Arabidopsis comparison, orthologs had a mean Ks of 0.77, while it was 0.42 in the Arabis-Arabidopsis comparison; mean Ks values of At-α derived paralogs were 1.37 and 1.01, respectively. Additionally, we checked each syntenic block for redundancy, i.e., if a block spanning the same part of the ACK was present more than once; the block with lower Ks was retained. The Aethionema blocks were generated from the remaining syntenic blocks; in the few cases where neighboring blocks from the same GB were not merged by QuotaAlign because of their distance, we merged them manually. Note that while gene names from A. thaliana define the borders, direction of blocks is given relative to the ACK. For visualization, we generated a syntenic dotplot and Ks plot using Ae. arabicum and Arabidopsis lyrata, a species with high similarity to the ACK. In this case, default parameters were set for DAGchainer and syntenic regions were not merged. Minimum length of chromosomes was set to 5,000,000 bp to retain only chromosomes from the genomes.
We compared the GBs from Aethionema with those from other species by running similar CoGe analyses with the following three species pairs: Ae. arabicum – A. lyrata (representative of lineage I/A and close to ACK), Ae. arabicum – A. alpina (unclear phylogenetic position), Ae. arabicum – E. syriacum (representative of lineage III/E) and A. alpina – A. thaliana. Blocks were only reconstructed for A. alpina (using the same parameters as above) and used to identify boundaries between (sub-)blocks shared between Aethionema and Arabis. Syntenic dotplots of these regions were finally compared between all species. Minimum length of chromosomes was again set to 5,000,000 bp to retain only chromosomes from the genomes, except for Euclidium, where shorter chromosomal length of 500,000 bp was allowed. The genome sequence of Euclidium is not quite assembled on a chromosomal-level, and block boundaries sometimes coincided with assembly borders. As we could not determine whether this was an artefact of assembly or the syntenic block boundary was located at the chromosome (arm) edge, we also used cytogenetic evidence from the literature for interpretation of our results.
Publicly available datasets were analyzed in this study and download links are given in Supplementary Table 1.
MS conceived the study. NW and T-PN analyzed the data. NW wrote the manuscript with input from TM, ML, and MS.
The authors declare that the research was conducted in the absence of any commercial or financial relationships that could be construed as a potential conflict of interest.
The reviewer ZL declared past co-authorship with one of the authors, MS, to the handling editor.
NW was supported by the German Research Foundation (DFG) with grant no. HO 6443/1. ML and TM were funded by a research grant from the Czech Science Foundation (Grant No. 15-18545S) to ML and by the CEITEC 2020 project (Grant No. LQ1601). T-PN and MS were funded by a grant from the Netherlands Organization for Scientific Research (NWO 0849.13.004) as part of the ERA-CAPS “SeedAdapt” consortium project (www.seedadapt.eu).
We thank the members of the SeedAdapt Consortium and Dr. Laurie Grandont for fruitful discussions about the work.
The Supplementary Material for this article can be found online at: https://www.frontiersin.org/articles/10.3389/fpls.2020.00719/full#supplementary-material
Beilstein, M. A., Nagalingum, N. S., Clements, M. D., Manchester, S. R., and Mathews, S. (2010). Dated molecular phylogenies indicate a miocene origin for Arabidopsis thaliana. Proc. Natl. Acad. Sci. U.S.A. 107, 18724–18728. doi: 10.1073/pnas.0909766107
Cheng, F., Mandáková, T., Wu, J., Xie, Q., Lysak, M. A., and Wang, X. (2013). Deciphering the diploid ancestral genome of the mesohexaploid Brassica rapa. Plant Cell 25, 1541–1554. doi: 10.1105/tpc.113.110486
Drillon, G., Champeimont, R., Oteri, F., Fischer, G., and Carbone, A. (2020). Phylogenetic reconstruction based on synteny block and gene adjacencies. Molecular Biol. Evol. msaa114. doi: 10.1093/molbev/msaa114
Edger, P. P., Hall, J. C., Harkess, A., Tang, M., Coombs, J., Mohammadin, S., et al. (2018). Brassicales phylogeny inferred from 72 plastid genes: a reanalysis of the phylogenetic localization of two paleopolyploid events and origin of novel chemical defenses. Am. J. Bot. 105, 463–469. doi: 10.1002/ajb2.1040
Edger, P. P., Heidel-Fischer, H. M., Bekaert, M., Rota, J., Glöckner, G., Platts, A. E., et al. (2015). The butterfly plant arms-race escalated by gene and genome duplications. Proc. Natl. Acad. Sci. U.S.A. 112, 8362–8366. doi: 10.1073/pnas.1503926112
Franzke, A., Lysak, M. A., Al-Shehbaz, I. A., Koch, M. A., and Mummenhoff, K. (2011). Cabbage family affairs: the evolutionary history of Brassicaceae. Trends Plant Sci. 16, 108–116. doi: 10.1016/j.tplants.2010.11.005
Guo, X., Liu, J., Hao, G., Zhang, L., Mao, K., Wang, X., et al. (2017). Plastome phylogeny and early diversification of Brassicaceae. BMC Genomics 18:e176. doi: 10.1186/s12864-017-3555-3553
Haas, B. J., Delcher, A. L., Wortman, J. R., and Salzberg, S. L. (2004). DAGchainer: a tool for mining segmental genome duplications and synteny. Bioinformatics 20, 3643–3646. doi: 10.1093/bioinformatics/bth397
Hofberger, J. A., Lyons, E., Edger, P. P., Chris Pires, J., and Eric Schranz, M. (2013). Whole genome and tandem duplicate retention facilitated glucosinolate pathway diversification in the mustard family. Genome Biol. Evol. 5, 2155–2173. doi: 10.1093/gbe/evt162
Hohmann, N., Wolf, E. M., Lysak, M. A., and Koch, M. A. (2015). A time-calibrated road map of Brassicaceae species radiation and evolutionary history. Plant Cell 27, 2770–2784. doi: 10.1105/tpc.15.00482
Huang, C.-H., Sun, R., Hu, Y., Zeng, L., Zhang, N., Cai, L., et al. (2016). Resolution of Brassicaceae phylogeny using nuclear genes uncovers nested radiations and supports convergent morphological evolution. Mol. Biol. Evol. 33, 394–412. doi: 10.1093/molbev/msv226
Kiefer, C., Willing, E.-M., Jiao, W.-B., Sun, H., Piednoël, M., Hümann, U., et al. (2019). Interspecies association mapping links reduced CG to TG substitution rates to the loss of gene-body methylation. Nat. Plants 5, 846–855. doi: 10.1038/s41477-019-0486-489
Koch, M. A., and Al-Shehbaz, I. A. (2009). “Molecular systematics and evolution of ‘wild’ crucifers (Brassicaceae or Cruciferae),” in Biology and Breeding of Crucifers, ed. S. K. Gupta (Boca Raton, FL: CRC Press), 1–19.
Koch, M. A., German, D. A., Kiefer, M., and Franzke, A. (2018). Database taxonomics as key to modern plant biology. Trends Plant Sci. 23, 4–6. doi: 10.1016/j.tplants.2017.10.005
Lenser, T., Graeber, K., Cevik, O. S., Adiguzel, N., Donmez, A. A., Grosche, C., et al. (2016). Developmental control and plasticity of fruit and seed dimorphism in Aethionema arabicum. Plant Physiol. 172, 1691–1707. doi: 10.1104/pp.16.00838
Lenser, T., Tarkowská, D., Novák, O., Wilhelmsson, P. K. I., Bennett, T., Rensing, S. A., et al. (2018). When the BRANCHED network bears fruit: how carpic dominance causes fruit dimorphism in Aethionema. Plant J. 94, 352–371. doi: 10.1111/tpj.13861
Lyons, E., Pedersen, B., Kane, J., Alam, M., Ming, R., Tang, H., et al. (2008). Finding and comparing syntenic regions among Arabidopsis and the outgroups papaya, poplar, and grape: CoGe with Rosids. Plant Physiol. 148, 1772–1781. doi: 10.1104/pp.108.124867
Lysak, M. A., Mandáková, T., and Schranz, M. E. (2016). Comparative paleogenomics of crucifers: ancestral genomic blocks revisited. Curr. Opin. Plant Biol. 30, 108–115. doi: 10.1016/j.pbi.2016.02.001
Mabry, M. E., Brose, J. M., Blischak, P. D., Sutherland, B., Dismukes, W. T., Bottoms, C. A., et al. (2019). Phylogeny and multiple independent whole-genome duplication events in the Brassicales. BioRxiv [Preprint]. doi: 10.1101/789040
Mandáková, T., Hloušková, P., German, D. A., and Lysak, M. A. (2017a). Monophyletic origin and evolution of the largest crucifer genomes. Plant Physiol. 174, 2062–2071. doi: 10.1104/pp.17.00457
Mandáková, T., Li, Z., Barker, M. S., and Lysak, M. A. (2017b). Diverse genome organization following 13 independent mesopolyploid events in Brassicaceae contrasts with convergent patterns of gene retention. Plant J. 91, 3–21. doi: 10.1111/tpj.13553
Mandáková, T., Hloušková, P., Koch, M. A., and Lysak, M. A. (2020). Genome evolution in Arabideae was marked by frequent centromere repositioning. Plant Cell 32, 650–665. doi: 10.1105/tpc.19.00557
Mandáková, T., and Lysak, M. A. (2008). Chromosomal phylogeny and karyotype evolution in x = 7 crucifer species (Brassicaceae). Plant Cell 20, 2559–2570. doi: 10.1105/tpc.108.062166
Mandáková, T., Pouch, M., Brock, J. R., Al-Shehbaz, I. A., and Lysak, M. A. (2019). Origin and evolution of diploid and allopolyploid Camelina genomes were accompanied by chromosome shattering. Plant Cell 31:2596. doi: 10.1105/tpc.19.00366
Mérai, Z., Graeber, K., Wilhelmsson, P., Ullrich, K. K., Arshad, W., Grosche, C., et al. (2019). Aethionema arabicum: a novel model plant to study the light control of seed germination. J. Exp. Bot. 70, 3313–3328. doi: 10.1093/jxb/erz146
Mohammadin, S., Peterse, K., Kerke, S. J., van de Chatrou, L. W., Dönmez, A. A., Mummenhoff, K., et al. (2017). Anatolian origins and diversification of Aethionema, the sister lineage of the core Brassicaceae. Am. J. Bot. 104, 1042–1054. doi: 10.3732/ajb.1700091
Nguyen, T.-P., Mühlich, C., Mohammadin, S., van den Bergh, E., Platts, A. E., Haas, F. B., et al. (2019). Genome improvement and genetic map construction for Aethionema arabicum, the first divergent branch in the Brassicaceae family. G3 9:3521. doi: 10.1534/g3.119.400657
Nikolov, L. A., Shushkov, P., Nevado, B., Gan, X., Al-Shehbaz, I. A., Filatov, D., et al. (2019). Resolving the backbone of the Brassicaceae phylogeny for investigating trait diversity. New Phytol. 222, 1638–1651. doi: 10.1111/nph.15732
One Thousand Plant Transcriptomes Initiative (2019). One thousand plant transcriptomes and the phylogenomics of green plants. Nature 574, 679–685. doi: 10.1038/s41586-019-1693-2
Schranz, M., Lysak, M., and Mitchellolds, T. (2006). The ABC’s of comparative genomics in the Brassicaceae: building blocks of crucifer genomes. Trends Plant Sci. 11, 535–542. doi: 10.1016/j.tplants.2006.09.002
Schranz, M. E., and Mitchell-Olds, T. (2006). Independent ancient polyploidy events in the sister families Brassicaceae and Cleomaceae. Plant Cell 18, 1152–1165. doi: 10.1105/tpc.106.041111
Schranz, M. E., Mohammadin, S., and Edger, P. P. (2012). Ancient whole genome duplications, novelty and diversification: the WGD radiation lag-time model. Curr. Opin. Plant Biol. 15, 147–153. doi: 10.1016/j.pbi.2012.03.011
Tang, H., and Lyons, E. (2012). Unleashing the genome of brassica rapa. Front. Plant Sci. 3:172. doi: 10.3389/fpls.2012.00172
Tang, H., Lyons, E., Pedersen, B., Schnable, J. C., Paterson, A. H., and Freeling, M. (2011). Screening synteny blocks in pairwise genome comparisons through integer programming. BMC Bioinform. 12:102. doi: 10.1186/1471-2105-12-102
Tank, D. C., Eastman, J. M., Pennell, M. W., Soltis, P. S., Soltis, D. E., Hinchliff, C. E., et al. (2015). Nested radiations and the pulse of angiosperm diversification: increased diversification rates often follow whole genome duplications. New Phytol. 207, 454–467. doi: 10.1111/nph.13491
Vanneste, K., Van de Peer, Y., and Maere, S. (2013). Inference of genome duplications from age distributions revisited. Mol. Biol. Evol. 30, 177–190. doi: 10.1093/molbev/mss214
Wilhelmsson, P. K. I., Chandler, J. O., Fernandez-Pozo, N., Graeber, K., Ullrich, K. K., Arshad, W., et al. (2019). Usability of reference-free transcriptome assemblies for detection of differential expression: a case study on Aethionema arabicum dimorphic seeds. BMC Genom. 20:95. doi: 10.1186/s12864-019-5452-5454
Willing, E.-M., Rawat, V., Mandáková, T., Maumus, F., James, G. V., Nordström, K. J. V., et al. (2015). Genome expansion of Arabis alpina linked with retrotransposition and reduced symmetric DNA methylation. Nat. Plants 1:14023. doi: 10.1038/nplants.2014.23
Yang, Z. (2007). PAML 4: phylogenetic analysis by maximum likelihood. Mol. Biol. Evol. 24, 1586–1591. doi: 10.1093/molbev/msm088
Keywords: Aethionema, Brassicaceae, comparative genomics, genomic blocks, synteny, Arabideae
Citation: Walden N, Nguyen T-P, Mandáková T, Lysak MA and Schranz ME (2020) Genomic Blocks in Aethionema arabicum Support Arabideae as Next Diverging Clade in Brassicaceae. Front. Plant Sci. 11:719. doi: 10.3389/fpls.2020.00719
Received: 03 February 2020; Accepted: 06 May 2020;
Published: 03 June 2020.
Edited by:
Steven Dodsworth, University of Bedfordshire, United KingdomReviewed by:
Patrick P. Edger, Michigan State University, United StatesCopyright © 2020 Walden, Nguyen, Mandáková, Lysak and Schranz. This is an open-access article distributed under the terms of the Creative Commons Attribution License (CC BY). The use, distribution or reproduction in other forums is permitted, provided the original author(s) and the copyright owner(s) are credited and that the original publication in this journal is cited, in accordance with accepted academic practice. No use, distribution or reproduction is permitted which does not comply with these terms.
*Correspondence: Michael Eric Schranz, ZXJpYy5zY2hyYW56QHd1ci5ubA==
Disclaimer: All claims expressed in this article are solely those of the authors and do not necessarily represent those of their affiliated organizations, or those of the publisher, the editors and the reviewers. Any product that may be evaluated in this article or claim that may be made by its manufacturer is not guaranteed or endorsed by the publisher.
Research integrity at Frontiers
Learn more about the work of our research integrity team to safeguard the quality of each article we publish.