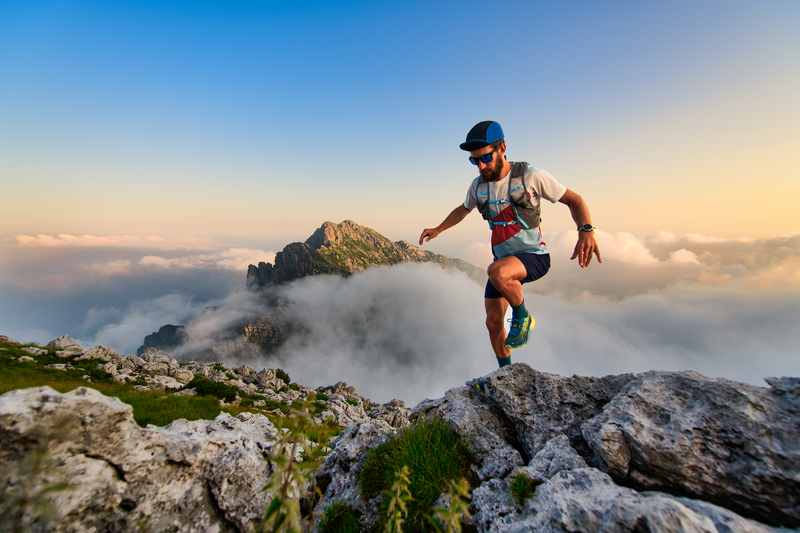
94% of researchers rate our articles as excellent or good
Learn more about the work of our research integrity team to safeguard the quality of each article we publish.
Find out more
ORIGINAL RESEARCH article
Front. Plant Sci. , 03 June 2020
Sec. Plant Metabolism and Chemodiversity
Volume 11 - 2020 | https://doi.org/10.3389/fpls.2020.00682
This article is part of the Research Topic Subcellular Compartmentalization of Plant Antioxidants and ROS Generating Systems View all 9 articles
The current study was conducted to demonstrate the possible roles of exogenously applied flavonoid naringenin (Nar) on the efficiency of PSII photochemistry and the responses of chloroplastic antioxidant of salt and osmotic-stressed Phaseolus vulgaris (cv. Yunus90). For this aim, plants were grown in a hydroponic culture and were treated with Nar (0.1 mM and 0.4 mM) alone or in a combination with salt (100 mM NaCl) and/or osmotic (10% Polyethylene glycol, −0.54 MPa). Both caused a reduction in water content (RWC), osmotic potential (ΨΠ), chlorophyll fluorescence (Fv/Fm), and potential photochemical efficiency (Fv/Fo). Nar reversed the changes on these parameters. The phenomenological fluxes (TRo/CS and ETo/CS) altered by stress were induced by Nar and Nar led to a notable increase in the performance index (PIABS) and the capacity of light reaction [ΦPo/(1-ΦPo)]. Besides, Nar-applied plants exhibited higher specific fluxes values [ABS/RC, ETo/RC, and ΨEo/(1-ΨEo)] and decreasing controlled dissipation of energy (DIo/CSo and DIo/RC). The transcripts levels of psbA and psbD were lowered in stress-treated bean but upregulated in Nar-treated plants after stress exposure. Nar also alleviated the changes on gas exchange parameters [carbon assimilation rate (A), stomatal conductance (gs), intercellular CO2 concentrations (Ci), transpiration rate (E), and stomatal limitation (Ls)]. By regulating the antioxidant metabolism of the isolated chloroplasts, Nar was able to control the toxic levels of hydrogen peroxide (H2O2) and TBARS (lipid peroxidation) produced by stresses. Chloroplastic superoxide dismutase (SOD) activity reduced by stresses was increased by Nar. In response to NaCl, Nar increased the activities of ascorbate peroxidase (APX), glutathione reductase (GR), monodehydroascorbate reductase (MDHAR), and dehydroascorbate reductase (DHAR), as well as peroxidase (POX). Nar protected the bean chloroplasts by minimizing disturbances caused by NaCl exposure via the ascorbate (AsA) and glutathione (GSH) redox-based systems. Under Nar plus PEG, Nar maintained the AsA regeneration by the induction of MDHAR and DHAR, but not GSH recycling by virtue of no induction in GR activity and the reduction in GSH/GSSG and GSH redox state. Based on these advances, Nar protected in bean chloroplasts by minimizing disturbances caused by NaCl or PEG exposure via the AsA or GSH redox-based systems and POX activity.
Plants are simultaneously subjected to the combination of salinity and osmotic stresses rather than the effects of an individual stress. Plants have common defense responses against these stresses. Photosynthesis is inhibited by decreasing intercellular CO2 concentrations depending on stomatal closure under both salt and osmotic stresses (Cheeseman, 2013). Chloroplasts, one of the cell compartments, have a central role in cell pathways such as biosynthesis of aromatic amino acids, fatty acids and carotenoids, and sulfate and nitrogen assimilation, as well as photosynthesis and are sensitive to stress conditions. Some of symptoms triggered by stress in plants is associated with the process occurred in chloroplasts. Due to the highly energetic reactions of photosynthesis, the reduction of molecular oxygen generates toxic reactive oxygen species (ROS), which interacts with essential and important molecules such as DNA, photosynthetic pigments, and proteins (Miller et al., 2010; Flowers et al., 2015). The accumulation of ROS causes the photoinhibition of photosystem I (PSI), the disruption in the structure of photosynthetic pigments, the inactivation of the elongation factor of D1 protein, the inhibition in the repair of photosystem II (PSII) and the decline of rubisco activity (Acosta-Motos et al., 2017; Bose et al., 2017). Stress treatments easily occur the damage in the reaction centers (RCs) of PSII (Strauss et al., 2006). The data on the photosynthetic performance is provided with the phenomenological and biophysical parameters in plants (Gururani et al., 2015). The responses of photosynthetic apparatus with these parameters are evaluated through OJIP fluorescence transients (called a JIP test). The OJIP test was developed by Strasser et al. (1995), and the parameters are calculated using the generated chlorophyll fluorescence induction curve according to the JIP-test method (Zeliou et al., 2009). The JIP test shows the measurement of several phenological and biophysical expressions of PSII including the fluxes of absorption, trapping, and electron transport (Strasser et al., 2000).
Cell detoxification mechanisms against ROS accumulation include the induced gene expression of enzymatic antioxidants including superoxide dismutase (SOD), peroxidase (POX), and ascorbate peroxidase (APX), glutathione reductase (GR), monodehydroascorbate reductase (MDHAR), dehydroascorbate reductase (DHAR) that are related to AsA-GSH cycle (Asada-Halliwell pathway) (Dai et al., 2012; Uzilday et al., 2012; Carvalho et al., 2015). APX, along with the oxidation of AsA, generates monodehydroascorbate (MDHA). MDHA is spontaneously turned into the oxidized state (dehydroascorbate, DHA). MDHA and DHA are reduced to AsA by the activities of NADPH-dependent MDHAR and GSH-dependent DHAR, respectively. As well as AsA, GSH has important roles to cope with the damage caused by ROS (Batth et al., 2017). To maintain of GSH pool, oxidized glutathione (GSSG) is reduced by GR via the consumption of NADPH (de Sousa et al., 2016).
Flavonoids are small molecular secondary metabolites synthesized by plants. The phenylpropanoid pathway is responsible for the synthesis of flavonoids (D’Amelia et al., 2018). After stress exposure to plants, the biosynthesis of flavonoids is induced. The flavonoids diminished the negative effects of stressful conditions by inhibition of ROS-generating enzymes such as lipoxygenase and xanthine oxidase, the chelation of transition metal ions, and the scavenging activity as an antioxidant agent (Baskar et al., 2018). Their eliminating feature against ROS comes from their catechol group in the B-ring of the flavonoid skeleton. They accumulate in mesophyll cells, vacuole and chloroplasts and stabilize the chloroplast membrane against stress (Martinez et al., 2016). Depending on the location of the flavonoids, some reports suggested them as an important component in photoprotection under stress (Agati and Tattini, 2010). For example, chloroplast-localized flavonoids reduced the ROS generation in excess light irradiance-treated Phyllirea latifolia (Agati et al., 2002). Also, flavonoids within chloroplasts protect the function of the membrane during cellular dehydration (Inoue, 2011). Many data revealed that there is an interaction between the levels of induced flavonoids and antioxidant action under different stress treatments such as ozone, heat and salinity (Gondor et al., 2016; Martinez et al., 2016; Pheomphun et al., 2019). Mahajan and Yadav (2013) found that exogenous flavonoid quercetin and epicatechin regulates the antioxidant enzymes at transcriptional levels in tobacco seedlings. As well as abiotic factors, upon exposure to biotic stress conditions, bacterial or fungal-mediated infection is inhibited by flavanols (such as myricetin) and anthocyanins (such as delphinidin) (Karageorgou and Manetas, 2006).
Naringenin (Nar) is a flavonoid belonging to flavanones subclass. It is widely distributed in several citrus fruits, bergamot, tomatoes, and other fruits, being found in its glycosides form (mainly naringin) as well. The chemical name of Nar is 2,3-dihydro-5,7-dihydroxy-2-(4-hydroxyphenyl)-4H-1-benzopyran-4-one (Salehi et al., 2019). Nar is derived from the hydrolysis of glycone forms of this flavanone, such as naringin or narirutin. The effects of Nar have been one of the immense study topics in animal systems (Salehi et al., 2019). However, the number of researches on its possible roles on plant growth, metabolism, and stress responses in plants is rather scanty. In several of those, Nar has been reported to suppress the growth of annual plant species, acting as an allelochemical. Similarly, Nar has been reported to cause a decrease in the growth of Arabidopsis thaliana (Hernandez and Munne-Bosch, 2012). This inhibitory effect of Nar was attributed at least to some extent, through impaired auxin transport. Deng et al. (2004) suggested that it exert its inhibitory effect by inhibiting the activity of the key enzyme of the phenylpropanoid pathway, 4-coumarate: CoA ligase, whereas Bido et al. (2010) assumed that the action site of naringenin may be related to other enzymes working at later steps of the phenylpropanoid pathway, such as cell wall–bound POX or, perhaps, cinnamyl alcohol dehydrogenase. Hence, the mode of action of naringenin still remains an open question in plant systems. However, the information about the interaction flavonoids including Nar with the activity of antioxidants localized in chloroplasts under individual or combined-treated stress treatments is unclear. Besides, hardly any data is available about the influence of exogenous applied flavonoid on quantum efficiencies and phenomenological energy fluxes indicating the vitality of PSII in photosynthetic machinery after stress exposure. In this regard, after chloroplast isolation of sampling groups in bean (Phaseolus vulgaris L.) leaves, we have focused on explanation the effects of exogenously applied Nar in five steps: (i) the effects of Nar on water content and osmotic potential under salt and/or osmotic stresses; (ii) the effects of Nar on gas exchange parameters such as carbon assimilation rate, transpiration rate, stomatal conductance and stomatal limitation; (iii) the determination the effects of Nar on the photochemical reactions and fluorescence transients and, on the expression levels of genes encoding the major extrinsic proteins of PSII such as psbA and psbD; (iv) the effects of Nar on the responses of antioxidant in chloroplasts of stress-treated plants; and (v) the effects of Nar on ROS content and lipid peroxidation in chloroplasts.
Common bean seeds (P. vulgaris cv. Yunus90) were obtained from the Bahri Dagdas International Agricultural Research Institute, Turkey. The procedures of germination and growth were cited from Yildiztugay et al. (2014).
For naringenin (Nar; 0.1 mM and 0.4 mM) and salt (NaCl, 100 mM)/osmotic Polyethylene glycol, 10% PEG6000, −0.54 MPa (Hellal et al., 2018) stress treatments, it was prepared by being dissolved in Hoagland solution and was added to the growth medium at the stage of 21 days old. An experiment was designed as twelve groups and was listed in Supplementary Table S1. The toxic levels of NaCl and PEG were chosen base on the study of Kurniasih et al. (2016) and Shatpathy et al. (2018), respectively. For determination of Nar application, the doses of Nar were selected as 0.1 mM and 0.4 mM according to Liu et al. (2012). Plants were harvested after 72 hours (h) of treatment.
After harvest, six leaves were obtained and their fresh weight (FW) was determined. The leaves were floated on de-ionized water for 6 h and the turgid tissue was blotted dry prior to determining turgid weight (TW). Dry weight (DW) was determined after oven drying at 70°C. The leaf relative water content (RWC) was calculated by the following formula (Smart and Bingham, 1974):
Leaves were extracted by crushing the material with a glass rod. Leaf osmotic potential (ΨPi) was measured by Vapro Vapor pressure Osmometer 5600. ΨPi was converted to MPa according to Santa-Cruz et al. (2002) by multiplying by a coefficient of 2.408 × 10–3.
A portable fluorometer (Handy PEA, Hansatech Instruments Ltd., Norfolk, United Kingdom) was used to determine the maximal quantum yield of PSII photochemistry (Fv/Fm), physiological state of the photosynthetic apparatus (Fo/Fm) and potential photochemical efficiency (Fv/Fo). Many parameters showing the structure and function of photosynthetic apparatus were detected by Handy PEA (Plant Efficiency Analyzer, Hansatech Instruments Ltd). The measurement was defined in Table 1 and the radar plot included the average values of the photosynthetic parameters of treatments groups in bean plants.
RNA isolation was completed given by Ozgur et al. (2015). The nucleic acid concentrations of total RNA and cDNA were measured by a Multiskan Go (Thermo Fisher Scientific, Waltham, MA, United States). To detect relative gene expression for each group, the threshold cycle value was normalized the actin and each sample was evaluated with three replications. The primer sequences and qRT-PCR conditions are given in Supplementary Table S2.
Carbon assimilation rate (A), stomatal conductance (gs), intercellular CO2 concentration (Ci) and transpiration rate (E) were detected with a portable gas exchange system (LCpro+; ADC, Hoddesdon, United Kingdom). The stomatal limitation value (Ls) was calculated as 1 – Ci/Ca (Ma et al., 2011).
The leaves were homogenized using a blender in isolation buffer containing 0.1 M Tris–HCl (pH 7⋅8), 0.3 M sorbitol, 5 mM MgCl2, 10 mM NaCl, 0.1% bovine serum albumin (BSA). The homogenate was filtered through four layers of cheesecloth, and the filtrate was centrifuged at 1000 × g for 6 min at 4°C. The supernatant was discarded, and the pellet was resuspended in the isolation buffer. Resuspended chloroplasts were overlaid on a 40% Percoll solution and centrifuged at 1700 × g for 7 min at 4°C. Intact chloroplasts were obtained after this centrifugation as a pellet which was resuspended again in the isolation buffer without BSA. Intactness of the chloroplasts was determined by using a ferricyanide reduction test (Lilley et al., 1975). For analysis of enzyme activity and for the native activity gels, chloroplasts were lysed with a lysis solution (10 mM HEPES-KOH (pH 7.2), 0.1 mM EDTA, 1 mM MgCl2, 0.1% Triton-X100) for 1 h at 4°C. Where APX was estimated, 5 mM ascorbate was added into the isolation buffer.
The total soluble protein content was analyzed (Bradford, 1976). The electrophoretic separation was detected on non-denaturing polyacrylamide miniature slab gels (8 cm × 10 cm) using the Mini PROTEAN Tetra Cell electrophoresis (Bio-Rad). Due to the high number of treatment groups and the low number of wells in the electrophoresis apparatus, the groups were divided into two as (i) C, Nar1, Nar2, S, S + Nar1, S + Nar2; and (ii) D, S, D + Nar1, D + Nar2, SD, SD + Nar1, SD + Nar2 and the gels were loaded to two different running modules at the same time. Samples were exposed to non-denaturing polyacrylamide gel electrophoresis (PAGE) as shown by Laemmli (1970). Chloroplastic SOD (EC 1.15.1.1) activity assay was based on the method of Beauchamp and Fridovich (1971). SOD isozyme activity was detected by staining with riboflavin and nitroblue tetrazolium. SOD isozyme patterns were determined by incubating the gels with 5 mM H2O2 to inhibit both Cu/Zn-SOD and Fe-SOD, or with 5 mM KCN to inhibit only Cu/Zn-SOD. Before staining of SOD activity, the control group was preincubated with 50 mM potassium phosphate buffer (pH 7.8) alone, buffer plus H2O2, or buffer plus KCN. POX isozymes were detected according to Seevers et al. (1971). The POX (EC 1.11.1.7) activity was done according to the procedure given by Herzog and Fahimi (1973). Electrophoretic APX separation was performed according to Mittler and Zilinskas (1993). Glutathione S-transferase (GST, EC: 2.5.1.18) activity was calculated following the method of Hossain et al. (2006). For GST isozyme activity, the method of Ricci et al. (1984) was used. NADPH oxidase (NOX) isozymes were identified by NBT reduction method as described by Sagi and Fluhr (2001). The samples containing 40 mg protein was loaded per lane. Total NOX (EC 1.6.3.1) activity was measured according to Jiang and Zhang (2002). The assay medium contained 50 mM Tris–HCl buffer, 0.5 mM XTT, 100 mM NADPH.Na4 and 20 mg of protein sample. After addition of NADPH, XTT reduction was followed at 470 nm. Activity was calculated using the extinction coefficient, 2.16 × 104 M–1 cm–1. One unit of NOX was defined as 1 nmol ml–1 XTT oxidized min–1.
Chloroplastic activities of APX (EC 1.11.1.11), GR (EC 1.6.4.2), monodehydroascorbate reductase (MDHAR; EC 1.6.5.4), dehydroascorbate reductase (DHAR; EC 1.8.5.1) were detected according to Foyer and Halliwell (1976); Nakano and Asada (1981), Dalton et al. (1986), and Miyake and Asada (1992), respectively. Total and reduced contents of ascorbate (AsA) were determined according to the method of Dutilleul et al. (2003). The oxidized form of ascorbate (DHA, dehydroascorbate) was calculated using the formula DHA = Total AsA - Reduced AsA. Glutathione (GSH) was assayed according to Paradiso et al. (2008). Oxidized glutathione (GSSG) was determined after removal of GSH by 2-vinylpyridine derivatization.
Gels stained with chloroplastic SOD, POX, APX, NOX, and GST activities were photographed with the Gel Doc XR+ System and then analyzed with Image Lab software v4.0.1 (Bio-Rad, Hercules, CA, United States). Activities of isoenzymes (0.5 units of SOD and 0.2 units of POX) were measured according to the known standard amounts.
H2O2 was determined according to Cheeseman (2006) using eFOX reagent. This modified ferrous ammonium sulphate/xylenol orange (FOX) assay was used due to its sensitivity, stability, and adaptability to a large number of samples. In this assay, 1% ethanol is added to the reagent, which increases its sensitivity to H2O2 by 50% (i.e., eFOX). Extraction was carried out using ice-cold acetone containing 25 mM H2SO4 for intact chloroplasts. Samples were then centrifuged for 5 min at 3000 × g at 4°C. eFOX reagent [950 μL of 250 μM ferrous ammonium sulfate, 100 μM xylenol orange, 100 μM sorbitol, 1% ethanol (v/v)] was used for 50 μL of supernatant. Reaction mixtures were incubated at room temperature for 30 min and then absorbance at 550 and 800 nm was measured. H2O2 concentrations were calculated using a standard curve prepared with known concentrations of H2O2.
Lipid peroxidation (thiobarbituric acid reactive substances (TBARS) content) was determined according to Rao and Sresty (2000). TBARS concentration was calculated from the absorbance at 532 nm, and measurements were corrected for non-specific turbidity by subtracting the absorbance at 600 nm. The concentration of TBARS was calculated using an extinction coefficient of 155 mM–1 cm–1.
The experiments were repeated thrice independently, and each data point was the mean of six replicates. All data obtained were subjected to a one-way analysis of variance (ANOVA). Statistical analysis of the values was performed by using SPSS 20.0. Tukey’s post-test was used to compare the treatment groups. Comparisons with p < 0.05 were considered significantly different. In all figures, the error bars represent standard errors of the means.
Figure 1A shows that there was a significant decrement in RWC under the alone or the combined treatments of stresses. There was a maximum reduction in the combination form of stresses (NaCl plus PEG) by 1.4-fold. The presence of Nar provided the high levels of RWC under stress-treated plants. On the other hand, Nar alone did not affect RWC compared to the control group.
Figure 1. The effects of exogenously applied Nar (Nar1, 0.1 mM and Nar2, 0.4 mM) on water status in bean leaves under alone or combination of salt (S, 100 mM NaCl) and PEG-induced osmotic (D, 10% PEG6000) stress. (A) the relative water content (RWC), (B) the osmotic potential (ΨΠ). For each group, vertical bars indicate ± SE and the different lowercase letters are significantly different (p < 0.05) values according to the Tukey test.
Salinity and osmotic stresses led to a considerable decrease in ΨΠ of bean (Figure 1B). The decreased values of ΨΠ were reversed by exogenously applied Nar in response to stress. Similar to the results of RWC, no effect was created on ΨΠ through Nar alone under control conditions.
Figures 2A–C revealed that both stress treatments significantly inhibited Fv/Fm and Fv/Fo of bean leaves. The lowest levels of Fv/Fm and Fv/Fo were at the combination form of stresses (by 18.5 and 54.6% declines, respectively). However, stress caused an induction in Fo/Fm and this effect induced by stress was more noticeable at NaCl + PEG group by 88.2% increase (Figure 2B). When bean plants were treated with Nar applications under stress, remarkable responses were created on Fv/Fm, Fo/Fm, and Fv/Fo levels. The results for these parameters were close to control group or higher than that of one. However, no effect on Fv/Fm, Fo/Fm, and Fv/Fo was detected after the solo applications of Nar (except for Nar2 on Fv/Fo).
Figure 2. The effects of exogenously applied Nar (Nar1, 0.1 mM and Nar2, 0.4 mM) on the photosynthetic machinery and the phenomenological energy fluxes in bean leaves under alone or combination of salt (S, 100 mM NaCl) and PEG-induced osmotic (D, 10% PEG6000) stress. (A) the maximal quantum yield of PSII photochemistry (Fv/Fm), (B) the physiological state of the photosynthetic apparatus (Fo/Fm), (C) the potential photochemical efficiency (Fv/Fo), (D) the electron transport flux per cross section (ETo/CSo), (E) the energy flux trapped per cross section (CS) (TRo/CSo), (F) The dissipated energy flux per cross section (DIo/CSo). For each group, vertical bars indicate ± SE and the different lowercase letters are significantly different (p < 0.05) values according to the Tukey test.
The phenomenological energy fluxes are presented in Figures 2D–F. Stress treatments resulted in a decline in ETo/CSo (Figure 2D), and TRo/CSo (Figure 2E), but the increment in DIo/CSo was observed at NaCl and/or PEG stress (Figure 2F). On the other hand, there was the opposite changes in all these parameters of Nar-treated bean plants, as compared to the stress alone. Similar to stress + Nar groups, Nar applications under control conditions promoted the levels of ETo/CSo and TRo/CSo.
Figure 3 showed the values of photosynthetic parameters in all the treatment groups as a radar plot. NaCl or PEG and their combined form caused the similar responses in photosynthetic machinery. While, the specific energy fluxes in thylakoid membranes per reaction centers of sample (ABS/RC, ETo/RC, and TRo/RC) decreased in chloroplasts of bean with stress, this effect was reversed by Nar applications. These parameters were similar to the control group or increased in Nar-treated plants.
Figure 3. The effects of exogenously applied Nar (Nar1, 0.1 mM and Nar2, 0.4 mM) on the radar plot with a series parameter derived from JIP-test analyses of the experimental fluorescence OJIP transients in bean leaves under alone or combination of salt (S, 100 mM NaCl) and PEG-induced osmotic (D, 10% PEG6000) stress. ABS/RC, average absorption per active reaction center; ETo/RC, electron transport flux per active reaction centers; TRo/RC, flux or exciton trapped per active reaction center; ΦPo/(1-ΦPo), QA-reducing RCs per PSII antenna chlorophyll; ΨEo/(1-ΨEo), the efficiency with which a trapped exciton transfers an electron to the photosynthetic electron transfer chain; γRC/(1-γRC), QA- reducing reaction centers per PSII antenna chlorophyll; DIo/RC, ratio of total dissipation to the amount of active reaction center; PIABS, performance index based on the absorption of light energy; PItotal, performance index (potential) for energy conservation from exciton to the reduction of PSI and acceptors.
NaCl and/or PEG caused a decrease in the efficiency of light reaction [ΦPo/(1-ΦPo)] and the rate of biochemical reaction [ΨEo/(1-ΨEo)] and γRC/(1-γRC), but Nar applications resulted in an increase in these parameters. Interestingly, stress resulted in an increment in energy dissipation (DIo/RC) of bean chloroplasts. However, these values were reversed by the Nar applications. Depending on the changes of these parameters [ΦPo/(1-ΦPo), ΨEo/(1-ΨEo) and γRC/(1-γRC)], stress lowered the performance index detected on energy absorption of chloroplasts (PIABS and PItotal) and the reduction rate was lower in PEG-treated plants than that of the NaCl ones. Also, the lowest reduction was under the combined stress treatments. Both Nar applications alone were provided the high index levels.
Stress had considerable effect on the relative transcription of the psbA gene in bean leaves, which was a maximum reduction in NaCl-treated plants (Figure 4A). Both Nar alone and Nar together with stress assuaged the stress-triggered reduction in psbA transcription compared to that of the control and stress alone group, respectively. The transcription levels of this gene were noticeably increased by a 0.4 mM Nar application under stress or non-stress.
Figure 4. The effects of exogenously applied Nar (Nar1, 0.1 mM and Nar2, 0.4 mM) on the relative expression of genes encoding reaction center core proteins in photosystem of bean chloroplasts under alone or combination of salt (S, 100 mM NaCl) and PEG-induced osmotic (D, 10% PEG6000) stress. (A) the relative expression of psbA, (B) the relative expression of psbD. For each group, vertical bars indicate ± SE and the different lowercase letters are significantly different (p < 0.05) values according to the Tukey test.
While NaCl-induced salinity suppressed the gene expression of psbD to its lowest levels (by 37.5% decrease), there was no significant difference among the control plants, PEG- and NaCl + PEG-treated plants (Figure 4B). Exogenously applied Nar to stressed plants upregulated the psbD gene to a level above that observed in the stress-treated alone. The psbD gene detected the highest upregulation after S + D + Nar1 by 4.1-fold. In terms of the transcript levels of psbD gene, similar response was observed at Nar alone as compared to the control group.
As well as the combined treatment, salt and osmotic stresses reduced A values, which reached the minimum levels at NaCl + PEG by 64.05% (Figure 5A). Similar trend was observed in gs (Figure 5B), E (Figure 5C), and Ci (Figure 5D) values of stress-treated bean plants. The reduction rate of A, gs, E, and Ci was higher in the combination form of stresses. Also, these reductions in A, gs, E, and Ci were prevented by Nar treatments under stress conditions. On the other hand, after exposure to NaCl or PEG, the stomatal limitations resulted in 58.6 or 28.9% increase, respectively (Figure 5E). Both Nar applications caused a decline in Ls in response to stress or control conditions.
Figure 5. The effects of exogenously applied Nar (Nar1, 0.1 mM and Nar2, 0.4 mM) on the gas exchange parameters in bean leaves under alone or combination of salt (S, 100 mM NaCl) and PEG-induced osmotic (D, 10% PEG6000) stress. (A) Carbon assimilation rate (A), (B) stomatal conductance (gs), (C) transpiration rate (E), (D) intercellular CO2 concentration (Ci), (E) stomatal limitation value (Ls). For each group, vertical bars indicate ± SE and the different lowercase letters are significantly different (p < 0.05) values according to the Tukey test.
As illustrated by Figure 6A, three SOD isozymes (Fe-SODs, Fe-SOD1-3) were detected by native page analysis in bean chloroplasts. However, Cu/Zn-SOD could not be identified. As compared to the control group, the chloroplastic SOD activity was reduced by PEG and NaCl + PEG (Figure 6B), depending on especially the intensities of Fe-SOD2 (Figure 6A). However, the plants treated with NaCl exhibited any effect in SOD activity. There was an increment in SOD activity of Nar-treated plants under stress. The induction in SOD (24.8%) was only observed the high Nar application under non-stress conditions.
Figure 6. The effects of exogenously applied Nar (Nar1, 0.1 mM and Nar2, 0.4 mM) on some antioxidant enzyme activities in bean leaves under alone or combination of salt (S, 100 mM NaCl) and PEG-induced osmotic (D, 10% PEG6000) stress. (A) The relative band intensity of chloroplastic superoxide dismutase isoenzymes (SOD), (B) chloroplastic SOD activity, (C) the relative band intensity of different types of chloroplastic peroxidase isoenzymes (POX), (D) chloroplastic POX activity. Due to the high number of treatment groups and the low number of wells in the electrophoresis apparatus, the groups were divided into two as (i) C, Nar1, Nar2, S, S + Nar1, S + Nar2) and (ii) D, S, D + Nar1, D + Nar2, SD, SD + Nar1, SD + Nar2) and the gels were loaded to two different running modules at the same time. For the determination of SOD isozymes, before staining of SOD activity, the control group was preincubated with 50 mM potassium phosphate buffer (pH 7.8) alone, buffer plus 5 mM H2O2, or buffer plus 4 mM KCN. For each group, vertical bars indicate ± SE and the different lowercase letters are significantly different (p < 0.05) values according to the Tukey test.
Gel analysis revealed that four chloroplastic POX isozymes (POX1-4) were viewed in the evaluation of POX isozyme profiles (Figure 6C). The isozyme activity of POX of bean was higher by NaCl or PEG, providing the intensities of POX1-2. Interestingly, when applied a combination of both, no response was observed in POX activity (Figure 6D). In Nar plus stress-treated plants, the intensities of POX isoforms were stronger than stress treatments alone. This effect was related to the induced intensities of POX2-4 and the new defined isozyme, POX3. Exogenously applied Nar could maintain this induction produced by stress in POX under non-stress conditions as well.
Quantification of the GST band intensities detected that three GST isozymes (GST1-3) revealed during experimental period (Figure 7A). The chloroplastic enzyme activity increased under all the stress treatments, as compared to the control group (Figure 7B). This induction of GST activity was related to the intensities of GST1-2 and GST1-2-3 in NaCl or PEG and NaCl + PEG-treated bean plants, respectively (Figure 7A). After NaCl treatment, Nar-treated plants had in induction in GST activity and it reached to the maximum level at S + Nar1, which was observed an enhancement by 2.03-fold. Similar trend was detected in the bean exposed to Nar together with PEG. On the other hand, chloroplastic GST activity of bean was lowered by Nar plus NaCl + PEG. Also, there was an enhancement in GST activity of Nar-treated plants under non-stress, as demonstrated by the stronger intensities of all GST isoforms.
Figure 7. The effects of exogenously applied Nar (Nar1, 0.1 mM and Nar2, 0.4 mM) on some antioxidant enzyme activities in bean leaves under alone or combination of salt (S, 100 mM NaCl) and PEG-induced osmotic (D, 10% PEG6000) stress. (A) The relative band intensity of different types of chloroplastic glutathione S-transferase (GST), (B) chloroplastic GST activity, (C) the relative band intensity of different types of chloroplastic NADPH oxidase isoenzymes (NOX), (D) chloroplastic NOX activity. Due to the high number of treatment groups and the low number of wells in the electrophoresis apparatus, the groups were divided into two as (i) C, Nar1, Nar2, S, S + Nar1, S + Nar2) and (ii) D, S, D + Nar1, D + Nar2, SD, SD + Nar1, SD + Nar2) and the gels were loaded to two different running modules at the same time. For each group, vertical bars indicate ± SE and the different lowercase letters are significantly different (p < 0.05) values according to the Tukey test.
As shown in Figure 7C, a total of four NOX isoenzymes was detected as NOX1-4 by native PAGE analysis. Chloroplastic NOX activity in bean was either unaffected or lower by the alone and the combined form of stress treatments (Figure 7D). However, bean leaves exposed to Nar and stress treatments exhibited the high NOX activity. This induction in chloroplastic NOX activity reached the maximum levels in Nar1 + NaCl-treated plants (6.2-fold). Although NOX3 isoform decreased in this treatment group (Nar1 + NaCl), the induction of the intensities of NOX1-2 was responsible for the response in activity. While 0.1 mM Nar alone created no remarkable effect on NOX activity, 0.4 mM Nar caused an increase in this enzyme compared to the control group (1.22-fold enhancement).
Examination of chloroplastic APX isoenzymes in bean identified five isoforms (APX1-5) (Figure 8A). The bean leaves treated with NaCl or PEG had no change in the activity of APX enzyme. However, at both NaCl and PEG, a remarkable increase in APX was measured (66.2%) and especially APX1 and APX4 isozymes were responsible for this change. After Nar applications to the stress-applied bean, the activity levels of APX isozymes were induced throughout the experimental period. Also, this induction in APX activity was observed in only the 0.1 mM Nar-treated bean leaves under the combination form of stresses (Figure 8B), as provided the isozyme-staining pattern (Figure 8A). On the other hand, 0.1 and 0.4 mM Nar alone enhanced chloroplastic APX activity by 52.3% and 60.1% increment.
Figure 8. The effects of exogenously applied Nar (Nar1, 0.1 mM and Nar2, 0.4 mM) on some antioxidant enzyme activities in bean leaves under alone or combination of salt (S, 100 mM NaCl) and PEG-induced osmotic (D, 10% PEG6000) stress. (A) The relative band intensity of different types of chloroplastic ascorbate peroxidase isoenzymes (APX), (B) chloroplastic APX activity, (C) chloroplastic glutathione reductase (GR). Due to the high number of treatment groups and the low number of wells in the electrophoresis apparatus, the groups were divided into two as (i) C, Nar1, Nar2, S, S + Nar1, S + Nar2) and (ii) D, S, D + Nar1, D + Nar2, SD, SD + Nar1, SD + Nar2) and the gels were loaded to two different running modules at the same time. For each group, vertical bars indicate ± SE and the different lowercase letters are significantly different (p < 0.05) values according to the Tukey test.
Except for PEG-treated plants, NaCl alone or together with PEG caused a reduction in chloroplastic GR activity (Figure 8C). Nar prevented this reduction in GR only in plants with NaCl. GR activity of the bean was either lower or unaffected by Nar + PEG and Nar+ the combined stress treatments. When compared to the control group, exogenously applied Nar created no remarkable effect on chloroplastic GR activity.
There was a similar response between chloroplastic MDHAR (Figure 9A) and DHAR (Figure 9B) in stress-treated bean plants. Single stress treatments (NaCl or PEG) were reduced or did not change the activities of MDHAR and DHAR. However, in response to the combined stress treatment, chloroplastic MDHAR activity was induced by 82.1% (Figure 9A). After Nar application was added to NaCl or PEG stress-treated plants, the activities of MDHAR and DHAR were elevated in comparison with those of the stress-treated plants alone. On the other hand, the increased activity of these enzymes was not maintained with the applications of Nar under the combined stress treatments. While, there was no effect on MDHAR activity in chloroplasts of bean exposed to 0.1 mM Nar, the elevated activity of MDHAR was detected by 0.4 mM Nar (1.2-fold), as compared to the control group. When Nar was applied alone, a reduction in DHAR of bean chloroplasts was observed (Figure 9B).
Figure 9. The effects of exogenously applied Nar (Nar1, 0.1 mM and Nar2, 0.4 mM) on some antioxidant enzyme activities related to AsA-GSH cycle in bean leaves under alone or combination of salt (S, 100 mM NaCl) and PEG-induced osmotic (D, 10% PEG6000) stress. (A) Chloroplastic monodehydroascorbate reductase activity (MDHAR), (B) chloroplastic dehydroascorbate reductase activity (DHAR), (C) chloroplastic ascorbate content (tAsA), (D) chloroplastic dehydroascorbate content (DHA), (E) chloroplastic glutathione content (GSH), (F) chloroplastic oxidized glutathione (GSSG). For each group, vertical bars indicate ± SE and the different lowercase letters are significantly different (p < 0.05) values according to the Tukey test.
Compared with control group, when the bean plants were exposed to stress, chloroplastic AsA content decreased or was similar to levels of control (Figure 9C). The combined stress-treated plants had an increase in AsA content. A remarkable increase in AsA was detected only in bean plants with Nar plus stress (NaCl or PEG). However, the induction in AsA was not maintained by Nar applications along with NaCl + PEG. In contrast to the results of AsA, single stress treatments caused an increment in DHA content which not observe in the combination form of stresses (Figure 9D). NaCl or PEG-induced increment in DHA content was not maintained by exogenously applied Nar. Besides, the chloroplasts of bean with S + D + Nar2 exhibited the increment in DHA content. Under non-stress conditions, Nar alone decreased DHA content as compared to the control group.
The contents of GSH (Figure 9E) or GSSG (Figure 9F) in chloroplasts of the bean decreased or increased for the experimental period when under stress, compared to the content level of the control group, respectively. While, only the bean leaves with Nar plus NaCl had high GSH content (Figure 9E), there was a reduction in NaCl or PEG (exception for PEG + NaCl) in GSSG content of Nar-treated bean chloroplasts (Figure 9F). Besides, when comparison to the control group, the maximum induction in GSH and GSSG was at Nar2 and Nar1 by 1.6-fold and 1.2-fold, respectively. Additionally, Nar applications minimized the risk of reducing GSH redox state [the ratio of GSH content to total glutathione (GSH + GSSG)] in response to NaCl stress.
As detected in Figure 10A, H2O2 content gradually increased under stress treatments and reached the maximum levels (by 2.3-fold) in plants with NaCl plus PEG stress. While, Nar alone did not affect H2O2 content, after Nar treatments to the stress-treated bean plants, a decline in H2O2 content was detected.
Figure 10. The effects of exogenously applied Nar (Nar1, 0.1 mM and Nar2, 0.4 mM) on the ROS content and lipid peroxidation in bean leaves under alone or combination of salt (S, 100 mM NaCl) and PEG-induced osmotic (D, 10% PEG6000) stress. (A) hydrogen peroxide (H2O2), (B) lipid peroxidation (TBARS content). For each group, vertical bars indicate ± SE and the different lowercase letters are significantly different (p < 0.05) values according to the Tukey test.
Figure 10B reveals that stress treatments resulted in an induction in the levels of lipid peroxidation. The maximum rate of this increment in TBARS content was at NaCl + PEG by 74.1%. However, Nar alleviated the increased in TBARS content of both stress-treated plants. In contrast to H2O2 content, as compared to the control group, Nar applications had no increase in TBARS content.
Many reports revealed that stress reduces the osmotic potential (ΨPi) in plants (Sheldon et al., 2017). In the current study, when ΨPi reached to more negative levels in bean under stress, the uptake of water or the efficiency of water use were reduced and then the water content (RWC) decreased. There was previous data where flavonoid levels can promote water content of rice seedlings under stress, as detected also in our study (Chutipaijit et al., 2009). In bean treated with Nar plus stress, ΨPi reduced by NaCl and PEG was alleviated. This indicated that the induced levels of ΨPi might help to conserve of RWC and to improve in hydration status, which was related to the higher levels of water content.
To investigate for determination of the tolerance range of plants against to stress, the photochemical performance of PSII plays essential roles as an informative tool. As mentioned earlier, the responses of PSII are important in determining the differences on the photosynthetic machinery between salinity and osmotic stresses (Kalaji et al., 2018). The induction kinetics in fluorescence show the reduction of electron acceptors in electron transport system (ETS) (Gururani et al., 2015). A decline in Fv/Fm and Fv/Fo levels is reported in stress-treated plants (Kalaji et al., 2011). This reduction is evidence of the damage of reaction centers in PSII, the decrease in electron transfer rates at oxidizing site of PSII and the declined number of quanta absorbed per unit time (Mehta et al., 2010). In our study, NaCl and PEG stresses caused a decrease in electron flow to reaction center of PSII, by providing a decline in Fm (acceptor side of PSII) and the area (reduced plastoquinone pool size, data not shown). However, Nar had positive effects on Fv/Fm and Fv/Fo by promotion of this value reduced by stress. By increased levels in Fv/Fm, Nar was able to induce the capacity of the energy absorbed to the reaction center by PSII under dark-adapted conditions, as suggested by Shu et al. (2016). The possible reason behind Nar-triggered increase of Fv/Fm and Fv/Fo in bean might be repairment of the degraded photosynthetic pigments in the thylakoid membrane of chloroplasts or regulation of the reduction/re-oxidation levels of quinones. Phenomenological fluxes include energy flux in the excited cross section (CS) of the sample (ETo/CS and TRo/CS). After NaCl treatments, a decrease in ETo/CSo caused the inactivation of reaction center. However, in stress-treated bean, Nar might regulate re-oxidation of reduced quinone through electron transfer (ET) over a CS of active and inactive reaction center (RC). In the current study, the lowered the electron transport per CS (TRo/CS) in stress-treated plants was alleviated by Nar application. NaCl and PEG-treated bean plants had a lower energy absorption by antenna pigments and energy trapping by RC. This is why there is a decrease in ETo/CS and TRo/CS under stress. However, the Nar-applied bean had the ability to protection of photosynthetic pigments such as chlorophyll-a. Similar phenomenon had been observed by Dolatabadian et al. (2012) that flavonoid genistein promoted photosynthesis rate by enhancing chlorophyll content in soybean exposed to salt stress.
NaCl and/or PEG caused an induction in DIo/CS, which was related to the levels of dissipated energy per leaf cross section. When DIo/CS was reduced by Nar application, the energy trapping efficiency in RC of PSII was higher than that of the stressed plants, which was also connected with the higher values of Fv/Fm. ABS/RC, ETo/RC and TRo/RC are the specific energy flux in membranes measured per the RC of sample. ABS/RC is the ratio of a number of absorbed energies by chlorophylls to the number of active RCs (Gururani et al., 2015). In the present study, this ratio was reduced under stress conditions (NaCl and/or PEG). This reduction was related to the decline in the amount of energy flow reaching to the RC of PSII and the antenna size of RCs. The stress-induced damage in PSII in this study was reversible after Nar application and the values reached to the higher levels than that of stress alone. ETo/RC and TRo/RC denote electron transfer and trap per active RC of PSII, respectively (Varghese et al., 2019). Trapping of an excitation by the RC results in the reduction of QA to QA– (Gururani et al., 2015). Under stress conditions, the overproduction of reduced QA– causes a damage in reaction center (Zhao et al., 2017). In the present study, stress decreased these parameters indicating a disruption in the transport and capture of electrons by photosynthetic systems, but this situation was successfully reversed by both of two different Nar application levels. Nar also protected bean leaves from photoinhibition by maintaining the reduced quinone pool. Exogenously applied Nar resulted in the positive effects on the forward electron transport rates by increasing ΨEo/(1-ΨEo) values. This recovery on ΨEo/(1-ΨEo) was related to the increased fluxes of absorption, trapping and electron transport in photosynthetic machinery (Rapacz et al., 2019). As well as the rate of biochemical reaction, both Nar treatments promoted the capacity of light reaction by the alleviated levels of ΦPo/(1-ΦPo). Besides, Nar promoted the reduction by stress on γRC/(1-γRC), which shows contribution of ET beyond reduced form of quinone. As contradistinction to the other parameters, DIo/RC was induced under stress treatments, as also suggested by Varghese et al. (2019). This situation has been regarded as an adaptive response for the dissipation of excess energy in a heat form based on the inactivation of PSII in photooxidative damage (Kalaji et al., 2014), in which electrons cannot be captured by RC of PSII. However, when Nar applied to the bean plants, this value did not need to increase, even it decreased in Nar alone and Nar + stress groups. The reason for this is that the excess energy caused stress-related damage did not occur in chloroplasts due to the exogenous application of Nar.
The other parameter of the OJIP test, the performance index (PI), indicates the vitality of sample under stress conditions and three critic steps in photosynthesis: absorption, trapping and electron transfer (Mehta et al., 2010; Varghese et al., 2019). In the present study, the reduced PI triggered by stress showed the decrement in density of the RC of PSII and this influence was greater under the combined stress treatments. Depending on efficiency of ΨEo/(1-ΨEo) and ΦPo/(1-ΦPo), Nar, in response to stress, encouraged the photosynthetic performance (PIABS and PItotal) in bean chloroplasts. Our data are in contention with previous reports where measurement of diminished fluorescence in different stressed plants (Mehta et al., 2010; Lazar et al., 2013; Varghese et al., 2019). When all the structural and functional parameters mentioned above were evaluated, stress conditions (NaCl and/or PEG) reversibly resulted an inactivation in photosynthetic machinery in bean chloroplasts. However, Nar applications recovered the damage at the acceptor/donor side of PSII, the decrease in the pool size of reduced quinones or maintained the stability of PSII by enhancing the turnover of D1 protein (as detected in the increased transcription levels of psbA gene).
Because of containing several components which are necessary for the photochemical reactions, the D1 protein is a functional protein in PSII complex (Mukherjee, 2020). The regulation of gene expression related to the photosynthesis shows the tolerance against the stress conditions (Wang et al., 2017). One of them is psbA gene, which encodes the D1 protein and is responsible for the reproduction of impaired D1 protein by stress. The other gene is psbD which protects the stability of PSII complex and regulates D2 protein expression (Chen et al., 2016). In the present study, our data showed that NaCl and PEG disrupted the relative expression of psbA and psbD by triggering damage in PSII system, as shown by the results of Fv/Fm and Fv/Fo. Similar conclusions were observed by Wang et al. (2018). However, both Nar applications protected transcription levels of psbA and psbD from the negative effects produced by NaCl or PEG, accelerating in the functional repair of PSII and assisting the synthesis of D2. This alleviation induced by Nar might be connected with newly synthesizing or repairing the impaired D1 protein.
One of the factors associated with reduction of the photosynthetic rate in stress-treated plants is disruption on gas exchange parameters and water management. The water status in the plants is directly correlated with the changes in stomatal conductance (Wada et al., 2019). In the present study, the decline in gs observed in salt and/or PEG-treated bean, signed to stomatal closure, which was accordance with significant declines in A, Ci, and E. This data is consistent with report of Engineer et al. (2016). In the present study, the decline of A/Ci under NaCl and/or PEG showed that depending on decrease in A and Ci, the assimilation values of CO2 in chloroplasts of NaCl or the PEG-treated bean might be influenced with the stomatal limitation, as evident by the increase in Ls (1- Ci/Ca). A similar observation was noted by Wang et al. (2018) in rice with stress. Nar applications could repair the restriction on photosynthetic regulation, as provided by a promotion of gs, A, Ci, E, and a reduction in Ls. Exogenously applied Nar in response to stress triggered stomatal opening by increasing gs levels. This situation promoted transport of internal CO2 to chloroplasts (as proved increased Ci) and carboxylation efficiency was alleviated. Under stress conditions, Nar applications could provide an increase in CO2 from the mesophyll (non-stomatal), as well as the increased entrance of CO2 through the stoma opening. Ci/gs is an important tool for identification of mesophyll efficiency and there is an opposite relationship between Ci/gs and mesophyll efficiency (Ramanjulu et al., 1998). In the current study, after individual treatments of NaCl or PEG to bean plants, both Nar applications caused a decline in Ci/gs, which verified by the increased mesophyll efficiency, but that was not the case under the combined form of these stresses. Also, Nar applications in bean chloroplasts subjected to NaCl and/or PEG provided the recovery on the restriction in mesophyll and stomata, as evident from the results of A, E, gs, E, Ls, and Fv/Fm.
Because of the disruption in redox regulation in photosynthesis, the electrons with a high energy state of chlorophyll reduce molecular oxygen by forming singlet oxygen (1O2). Also, the thylakoidal electron transport components on the PSI side such as the Fe-S centers result in the reduction of oxygen by a reaction called the Mehler reaction (Cruz de Carvalho, 2008) thus forming superoxide and H2O2. SOD, converting superoxide anion radical to oxygen and H2O2, is the front-line enzyme against toxic accumulation of ROS (Zou et al., 2018). In the present study, despite no observation in response of chloroplastic SOD activity under NaCl and/or PEG, H2O2 content was induced. This result contradict data presented by Guan et al. (2017) who reported an increase in chloroplastic Cu/Zn-SOD of rice under salinity. Other than photosynthesis, photorespiration also produces ROS. During carbon assimilation, ribulose-1,5-bisphosphate is also oxidized with oxygen by the activity of enzyme Rubisco. The yielding glycolate is transported from chloroplasts to peroxisomes and H2O2 is generated (Hodges et al., 2016). Hence, a possible source of H2O2 is also the activation of some enzymes such as glycolate oxidases, glucose oxidases, amino-acid oxidases, sulfite oxidases and NOX (Wu et al., 2018). In the chloroplasts of bean, there was no contribution of NOX activity in H2O2 accumulation under stress. The produced H2O2 is eliminated by POX or APX in the so-called water-water cycle (Asada, 1999). In present study, POX activity increased only after individual application of NaCl or PEG, but, not under combined application of NaCl and PEG. Whereas, APX activity was induced only under NaCl plus PEG stress. Our observations are in line with that of Zhang et al. (2017) who reported increased activity of APX under NaCl + PEG in Glycyrrhiza uralensis, but not in NaCl alone-treated plants. The bean leaves showed more sensitivity to NaCl or PEG due to the reduction in AsA/DHA and GSH/GSSG ratio. In response to stress, the inadequate enzyme activity might also be related to the increased accumulation of H2O2. This was accordance with the higher TBARS content in bean chloroplasts under NaCl and/or PEG, as also reported by Wang et al. (2019).
Naringenin applications to NaCl and/or PEG-applied bean resulted in an increase of SOD activity, resulting in an increase in H2O2 content. Also, the induction of NOX activity also contributed to this increase. After Nar applications to bean with NaCl or PEG treatments, the differences were observed in the responses for scavenging of H2O2. When Nar was applied under NaCl, H2O2 produced by SOD or NOX was eliminated by the activities of POX and, APX, GR, MDHAR and DHAR which activated the AsA-GSH cycle. In response to NaCl stress, Nar increased chloroplastic DHA levels, which are converted from MDHA, depending on APX activity. Nar might trigger the transformation to AsA from MDHA via the activation of MDHAR. The induced DHA was regenerated to AsA by the catalysis of DHAR. The GSH regeneration required for the activity of DHAR was provided by GR which regenerates GSH and so, maintains the GSH pool (Shan et al., 2020). Under salt stress, the applications of Nar had direct capability to protect a high level of AsA/DHA. Therefore, Nar could maintain the pool of AsA and GSH under salt stress in bean chloroplasts by the regulation the AsA-GSH cycle. Also, the overexpressed enzymes presented in regeneration and biosynthesis of AsA and GSH display the enhanced the tolerance to stress (Li et al., 2012). The high ratio in GSH/GSSG and the elevated GSH content/redox state, which was detected in Nar-treated bean chloroplasts in response to salinity, showed that it could have a role in recycling of AsA and GSH by providing a redox state and reducing oxidative stress, as mentioned by Wang et al. (2013) and Garg and Singla (2015). Another enzyme, GST detoxifies toxic lipid peroxides and reduces dehydroascorbate with GSH-dependent reductase activity (DHAR) and so maintains the reductant pools such as AsA (Souri et al., 2020). In the current study, induced GST activity was continued by Nar applications with NaCl or PEG. However, under the combination of stresses, the increase in GST was not maintained via Nar applications. This result was inconsistent with the changes in AsA and GSH which interacts with ROS and abscisic acid in signaling pathway (Szalai et al., 2009). Hence, Nar might have promoted the signaling pathway via the increased GSH activity under salinity.
Exogenous addition of Nar together with PEG-induced osmotic stress to bean plants removed H2O2 accumulation through the activities of POX, APX, MDHAR, and DHAR. Like the responses to NaCl stress, Nar could be role in recycling of AsA through the induction of chloroplastic AsA content. Although DHAR converts to GSSG from GSH, it was not possible to regenerate GSH from GSSG due to the inactivity of GR. Therefore, Nar had no effect on GSH regeneration in bean chloroplasts treated with PEG. These results were consistent with the high ratio of AsA/DHA and the reduced GSH/GSSG and GSH redox state.
Interestingly, the same variation in this cycle was not observed in the combination with Nar and NaCl + PEG stress. No increases in the activities of GR, MDHAR, DHAR not only was observed in bean chloroplasts but also exhibited a reduction in the contents of AsA, DHA and GSH. So, the regeneration of both AsA and GSH contents was reduced by Nar applications under the combined form of these stresses. However, POX activity induced by Nar was successfully stimulated to remove of the toxic levels of H2O2 produced under NaCl + PEG. Our findings are in accordance with Agati et al. (2013) that the accumulation of H2O2 are eliminated by flavonoid-peroxidase reaction.
As a biomarker to measure the degree of damage, TBARS is important tool in plants treated with stress (Parvin et al., 2020) and TBARS is induced with salinity or osmotic stress in bean chloroplasts. When all this data was interpreted, Nar applications to stress-treated bean chloroplasts showed lower oxidative damage as indicated by lower H2O2 and TBARS levels. Similar observation was noted by Garg and Singla (2015), where Nar applications decreased both H2O2 and TBARS contents in Cicer arietinum under salt stress. Because of the decreasing amount of H2O2, Nar might trigger a signal role of H2O2 rather than its toxic effects.
Our study confirms that Nar applications prevented inhibition on RWC, osmotic potential and photosynthetic efficiency (Fv/Fm, Fv/Fo, and Fo/Fm) effected by NaCl and/or PEG in bean chloroplasts. Nar could reverse the restriction on stomatal regulation, as evident by alleviation of gs, A, E and Ls. Nar recovered the photosynthetic machinery by altering the stability of PSII [ABS/RC, ETo/RC and ΨEo/(1-ΨEo)] and regulation of dissipated energy levels absorbed energy by chlorophyll of all reaction center in PSII (DIo/CSo and DIo/RC) and protection the damage at the acceptor/donor side of PSII. These regulations triggered by Nar led to a notable increase in the performance index (PIABS) and the capacity of light reaction [ΦPo/(1-ΦPo)]. Both Nar applications (0.1 and 0.4 mM) protected from the negative effects produced by NaCl or PEG on transcription levels of psbA and psbD, accelerating in the functional repair of PSII and assisting the synthesis of D2. By regulating the antioxidant metabolism in chloroplasts of bean plants, Nar was able to control the toxic levels of ROS and TBARS produced by stress. Chloroplastic SOD activity reduced by stress in bean exposed to stress was increased by Nar. Since Nar exposure increased the activities of APX, GR, MDHAR, and DHAR as well as POX, Nar could maintain both AsA and GSH redox state in response to NaCl, as evident by enhancement in AsA/DHA and GSH/GSSG. Nar protected the bean chloroplasts by minimizing disturbances caused by salinity via the ascorbate and glutathione redox-based systems. Despite of the induction of MDHAR and DHAR under Nar plus PEG, Nar maintained the AsA regeneration, but not GSH recycling because of no induction in GR activity and the reduction in GSH/GSSG and GSH redox state. Consequently, Nar protected bean chloroplasts by minimizing disturbances caused by NaCl or PEG exposure via the AsA or GSH redox-based systems and POX activity.
All datasets generated for this study are included in the article/Supplementary Material.
EY, CO-K, MK, and IT conceived and designed the research. CO-K and EY conducted the experiments. CO-K, EY, and IT analyzed the data. CO-K, EY, and IT wrote the manuscript. All authors read and approved the manuscript.
Financial support for this work was provided by the Selcuk University Scientific Research Projects Coordinating Office (Project number: 20401044).
The authors declare that the research was conducted in the absence of any commercial or financial relationships that could be construed as a potential conflict of interest.
We are thankful to Dr. Ramazan Keles from Bahri Dagdas International Agricultural Research Institute for providing the seeds of wheat.
The Supplementary Material for this article can be found online at: https://www.frontiersin.org/articles/10.3389/fpls.2020.00682/full#supplementary-material
Acosta-Motos, J., Ortuno, M. F., Bernal-Vicente, A., Diaz-Vivancos, P., Sanchez-Blanco, M., and Hernandez, J. A. (2017). Plant responses to salt stress: adaptive mechanisms. Agronomy 7:18. doi: 10.3390/agronomy7010018
Agati, G., Brunetti, C., Di Ferdinando, M., Ferrini, F., Pollastri, S., and Tattini, M. (2013). Functional roles of flavonoids in photoprotection: new evidence, lessons from the past. Plant Physiol. Biochem. 72, 35–45. doi: 10.1016/j.plaphy.2013.03.014
Agati, G., Galardi, C., Gravano, E., Romani, A., and Tattini, M. (2002). Flavonoid distribution in tissues of Phillyrea latifolia L. leaves as estimated by microspectrofluorometry and multispectral fluorescence microimaging. Photochem. Photobiol. 76, 350–360. doi: 10.1562/0031-8655(2002)0760350fditop2.0.co2
Agati, G., and Tattini, M. (2010). Multiple functional roles of flavonoids in photoprotection. New Phytol. 186, 786–793. doi: 10.1111/j.1469-8137.2010.03269.x
Asada, K. (1999). The water–water cycle in chloroplasts: scavenging of active oxygen and dissipation of excess photons. Annu. Rev. Plant Physiol. Plant Mol. Biol. 50, 601–639. doi: 10.1146/annurev.arplant.50.1.601
Baskar, V., Venkatesh, R., and Ramalingam, S. (2018). Flavonoids (Antioxidants Systems) in Higher Plants and their Response to Stresses, in Antioxidants and Antioxidant Enzymes in Higher Plants. Cham: Springer, 253–268.
Batth, R., Singh, K., Kumari, S., and Mustafiz, A. (2017). Transcript profiling reveals the presence of abiotic stress and developmental stage specific ascorbate oxidase genes in plants. Front. Plant Sci. 8:2. doi: 10.3389/fpls.2017.00198
Beauchamp, C., and Fridovich, I. (1971). Superoxide dismutase: improved assays and an assay applicable to acrylamide gels. Anal. Biochem. 44, 276–287. doi: 10.1016/0003-2697(71)90370-8
Bido, G. S., Ferrarese, M. L. L., Marchiosi, R., and Ferrarese-Filho, O. (2010). Naringenin inhibits the growth and stimulates the lignification of soybean root. Braz. Arch. Biol. Technol. 53, 533–542. doi: 10.1590/s1516-89132010000300005
Bose, J., Munns, R., Shabala, S., Gilliham, M., Pogson, B., and Tyerman, S. D. (2017). Chloroplast function and ion regulation in plants growing on saline soils: lessons from halophytes. J. Exp. Bot. 68, 3129–3143. doi: 10.1093/jxb/erx142
Bradford, M. M. (1976). A rapid and sensitive method for the quantitation of microgram quantities of protein utilizing the principle of protein-dye binding. Anal. Biochem. 72, 248–254. doi: 10.1016/0003-2697(76)90527-3
Carvalho, L. C., Vidigal, P., and Amâncio, S. (2015). Oxidative stress homeostasis in grapevine (Vitis vinifera L.). Front. Environ. Sci. 3:20. doi: 10.3389/fenvs.2015.00020
Cheeseman, J. M. (2006). Hydrogen peroxide concentrations in leaves under natural conditions. J. Exp. Bot. 57, 2435–2444. doi: 10.1093/jxb/erl004
Cheeseman, J. M. (2013). The integration of activity in saline environments: problems and perspectives. Funct. Plant Biol. 40, 759–774.
Chen, Y. E., Yuan, S., and Schroder, W. P. (2016). Comparison of methods for extracting thylakoid membranes of Arabidopsis plants. Physiol. Plant. 156, 3–12. doi: 10.1111/ppl.12384
Chutipaijit, S., Cha-Um, S., and Sompornpailin, K. (2009). Differential accumulations of proline and flavonoids in indica rice varieties against salinity. Pakistan J. Bot. 41, 2497–2506.
Cruz de Carvalho, M. H. (2008). Drought stress and reactive oxygen species: production, scavenging and signaling. Plant Signal. Behav. 3, 156–165. doi: 10.4161/psb.3.3.5536
Dai, A. H., Nie, Y. X., Yu, B., Li, Q., Lu, L. Y., and Bai, J. G. (2012). Cinnamic acid pretreatment enhances heat tolerance of cucumber leaves through modulating antioxidant enzyme activity. Environ. Exp. Bot. 79, 1–10. doi: 10.1016/j.envexpbot.2012.01.003
Dalton, D. A., Russell, S. A., Hanus, F. J., Pascoe, G. A., and Evans, H. J. (1986). Enzymatic-reactions of ascorbate and glutathione that prevent peroxide damage in soybean root-nodules. Proc. Natl. Acad. Sci. U.S.A. 83, 3811–3815. doi: 10.1073/pnas.83.11.3811
D’Amelia, V., Aversano, R., Ruggiero, A., Batelli, G., Appelhagen, I., Dinacci, C., et al. (2018). Subfunctionalization of duplicate MYB genes in Solanum commersonii generated the cold-induced ScAN2 and the anthocyanin regulator ScAN1. Plant Cell Environ. 41, 1038–1051. doi: 10.1111/pce.12966
de Sousa, A., Abdelgawad, H., Han, A., Teixeira, J., Matos, M., and Fidalgo, F. (2016). Oxidative metabolism of rye (Secale cereale L.) after short term exposure to aluminum: uncovering the glutathione–ascorbate redox network. Front. Plant Sci. 7:685. doi: 10.3389/fpls.2016.00685
Deng, F., Aoki, M., and Yogo, Y. (2004). Effect of naringenin on the growth and lignin biosynthesis of gramineous plants. Weed Biol. Manag. 4, 49–55. doi: 10.1111/j.1445-6664.2003.00119.x
Dolatabadian, A., Sanavy, S. A. M. M., Ghanati, F., and Gresshoff, P. M. (2012). Morphological and physiological response of soybean treated with the microsymbiont Bradyrhizobium japonicum pre-incubated with genistein. South Afr. J. Bot. 79, 9–18. doi: 10.1016/j.sajb.2011.11.001
Dutilleul, C., Driscoll, S., Cornic, G., De Paepe, R., Foyer, C. H., and Noctor, G. (2003). Functional mitochondrial complex I is required by tobacco leaves for optimal photosynthetic performance in photorespiratory conditions and during transients. Plant Physiol. 131, 264–275. doi: 10.1104/pp.011155
Engineer, C. B., Hashimoto-Sugimoto, M., Negi, J., Israelsson-Nordström, M., Azoulay-Shemer, T., Rappel, W.-J., et al. (2016). CO2 sensing and CO2 regulation of stomatal conductance: advances and open questions. Trends Plant Sci. 21, 16–30. doi: 10.1016/j.tplants.2015.08.014
Flowers, T. J., Munns, R., and Colmer, T. D. (2015). Sodium chloride toxicity and the cellular basis of salt tolerance in halophytes. Ann. Bot. 115, 419–431. doi: 10.1093/aob/mcu217
Foyer, C. H., and Halliwell, B. (1976). The presence of glutathione and glutathione reductase in chloroplasts: a proposed role in ascorbic acid metabolism. Planta 133, 21–25. doi: 10.1007/bf00386001
Garg, N., and Singla, P. (2015). Naringenin- and Funneliformis mosseae-mediated alterations in redox state synchronize antioxidant network to alleviate oxidative stress in Cicer arietinum L. genotypes under salt stress. J. Plant Growth Regul. 34, 595–610. doi: 10.1007/s00344-015-9494-9
Gondor, O. K., Szalai, G., Kovacs, V., Janda, T., and Pal, M. (2016). Relationship between polyamines and other cold-induced response mechanisms in different cereal species. J. Agron. Crop Sci. 202, 217–230. doi: 10.1111/jac.12144
Guan, Q., Liao, X., He, M., Li, X., Wang, Z., Ma, H., et al. (2017). Tolerance analysis of chloroplast OsCu/Zn-SOD overexpressing rice under NaCl and NaHCO3 stress. PLoS One 12:e0186052. doi: 10.1371/journal.pone.0186052
Gururani, M. A., Venkatesh, J., Ganesan, M., Strasser, R. J., Han, Y., Kim, J. I., et al. (2015). In vivo assessment of cold tolerance through chlorophyll-a fluorescence in transgenic zoysiagrass expressing mutant phytochrome a. PLoS One 10:5. doi: 10.1371/journal.pone.0127200
Hellal, F., El-Shabrawi, H., El-Hady, M. A., Khatab, I., El-Sayed, S., and Abdelly, C. (2018). Influence of PEG induced drought stress on molecular and biochemical constituents and seedling growth of Egyptian barley cultivars. J. Genet. Eng. Biotechnol. 16, 203–212. doi: 10.1016/j.jgeb.2017.10.009
Hernandez, I., and Munne-Bosch, S. (2012). Naringenin inhibits seed germination and seedling root growth through a salicylic acid-independent mechanism in Arabidopsis thaliana. Plant Physiol. Biochem. 61, 24–28. doi: 10.1016/j.plaphy.2012.09.003
Herzog, V., and Fahimi, H. (1973). Determination of the activity of peroxidase. Anal. Biochem. 55:e62.
Hodges, M., Dellero, Y., Keech, O., Betti, M., Raghavendra, A. S., Sage, R., et al. (2016). Perspectives for a better understanding of the metabolic integration of photorespiration within a complex plant primary metabolism network. J. Exp. Bot. 67, 3015–3026. doi: 10.1093/jxb/erw145
Hossain, M. Z., Hossain, M. D., and Fujita, M. (2006). Induction of pumpkin glutathione S-transferases by different stresses and its possible mechanisms. Biol. Plant. 50, 210–218. doi: 10.1007/s10535-006-0009-1
Inoue, K. (2011). Emerging roles of the chloroplast outer envelope membrane. Trends Plant Sci. 16, 550–557. doi: 10.1016/j.tplants.2011.06.005
Jiang, M., and Zhang, J. (2002). Involvement of plasma-membrane NADPH oxidase in abscisic acid- and water stress-induced antioxidant defense in leaves of maize seedlings. Planta 215, 1022–1030. doi: 10.1007/s00425-002-0829-y
Kalaji, H. M., Govindjee, G., Bosa, K., Koscielniak, J., and Zuk-Golaszewska, K. (2011). Effects of salt stress on photosystem II efficiency and CO2 assimilation of two Syrian barley landraces. Environ. Exp. Bot. 73, 64–72. doi: 10.1016/j.envexpbot.2010.10.009
Kalaji, H. M., Rackova, L., Paganova, V., Swoczyna, T., Rusinowski, S., and Sitko, K. (2018). Can chlorophyll-a fluorescence parameters be used as bio-indicators to distinguish between drought and salinity stress in Tilia cordata Mill? Environ. Exp. Bot. 152, 149–157. doi: 10.1016/j.envexpbot.2017.11.001
Kalaji, H. M., Schansker, G., Ladle, R. J., Goltsev, V., Bosa, K., Allakhverdiev, S. I., et al. (2014). Frequently asked questions about in vivo chlorophyll fluorescence: practical issues. Photosynth. Res. 122, 121–158.
Karageorgou, P., and Manetas, Y. (2006). The importance of being redwhen young: anthocyanins and the protection of young leaves of Quercus coccifera from insect herbivory and excess light. Tree Physiol. 26, 613–621. doi: 10.1093/treephys/26.5.613
Kurniasih, B., Greenway, H., and Colmer, T. D. (2016). Energetics of acclimation to NaCl by submerged, anoxic rice seedlings. Ann. Bot. 119, 129–142. doi: 10.1093/aob/mcw189
Laemmli, U. K. (1970). Cleavage of structural proteins during the assembly of the head of bacteriophage T4. Nature 227, 680–685. doi: 10.1038/227680a0
Lazar, D., Murch, S. J., Beilby, M. J., and Al Khazaaly, S. (2013). Exogenous melatonin affects photosynthesis in characeae Chara australis. Plant Signal. Behav. 8:e23279. doi: 10.4161/psb.23279
Li, Y. H., Liu, Y. J., Xu, X. L., Jin, M., An, L. Z., and Zhang, H. (2012). Effect of 24-epibrassinolide on drought stress-induced changes in Chorispora bungeana. Biol. Plant. 56, 192–196. doi: 10.1007/s10535-012-0041-2
Lilley, R. M., Fitzgerald, M., Rienits, K., and Walker, D. (1975). Criteria of intactness and the photosynthetic activity of spinach chloroplast preparations. New Phytol. 75, 1–10. doi: 10.1111/j.1469-8137.1975.tb01365.x
Liu, M. H., Zou, W., Yang, C. P., Peng, W., and Su, W. W. (2012). Metabolism and excretion studies of oral administered naringin, a putative antitussive, in rats and dogs. Biopharm. Drug Disposition 33, 123–134. doi: 10.1002/bdd.1775
Ma, Y., An, Y., Shui, J., and Sun, Z. (2011). Adaptability evaluation of switchgrass (Panicum virgatum L.) cultivars on the Loess Plateau of China. Plant Science 181, 638–643. doi: 10.1016/j.plantsci.2011.03.003
Mahajan, M., and Yadav, S. K. (2013). Effect of quercetin and epicatechin on the transcript expression and activity of antioxidant enzymes in tobacco seedlings. Am. J. Biochem. Mol. Biol. Fertil. Soils 3, 81–90. doi: 10.3923/ajbmb.2013.81.90
Martinez, V., Mestre, T. C., Rubio, F., Girones-Vilaplana, A., Moreno, D. A., Mittler, R., et al. (2016). Accumulation of flavonols over hydroxycinnamic acids favors oxidative damage protection under abiotic stress. Front. Plant Sci. 7:3. doi: 10.3389/fpls.2016.00838
Mehta, P., Jajoo, A., Mathur, S., and Bharti, S. (2010). Chlorophyll a fluorescence study revealing effects of high salt stress on Photosystem II in wheat leaves. Plant Physiol. Biochem. 48, 16–20. doi: 10.1016/j.plaphy.2009.10.006
Miller, G., Suzuki, N., Ciftci-Yilmaz, S., and Mittler, R. (2010). Reactive oxygen species homeostasis and signaling during drought and salinity stresses. Plant Cell Environ. 33, 453–467. doi: 10.1111/j.1365-3040.2009.02041.x
Mittler, R., and Zilinskas, B. A. (1993). Detection of ascorbate peroxidase-activity in native gels by inhibition of the ascorbate-dependent reduction of nitroblue tetrazolium. Anal. Biochem. 212, 540–546. doi: 10.1006/abio.1993.1366
Miyake, C., and Asada, K. (1992). Thylakoid-bound ascorbate peroxidase in spinach-chloroplasts and photoreduction of its primary oxidation-product monodehydroascorbate radicals in thylakoids. Plant Cell Physiol. 33, 541–553.
Mukherjee, A. (2020). Role of DnaK-DnaJ proteins in PSII repair. Plant Physiol. 182, 1804–1805. doi: 10.1104/pp.20.00265
Nakano, Y., and Asada, K. (1981). Hydrogen peroxide is scavenged by ascorbate-specific peroxidase in spinach chloroplasts. Plant Cell Physiol. 22, 867–880.
Ozgur, R., Uzilday, B., Sekmen, A. H., and Turkan, I. (2015). The effects of induced production of reactive oxygen species in organelles on endoplasmic reticulum stress and on the unfolded protein response in Arabidopsis. Ann. Bot. 116, 541–553. doi: 10.1093/aob/mcv072
Paradiso, A., Berardino, R., De Pinto, M. C., Sanita Di Toppi, L., Storelli, M. M., Tommasi, F., et al. (2008). Increase in ascorbate-glutathione metabolism as local and precocious systemic responses induced by cadmium in durum wheat plants. Plant Cell Physiol. 49, 362–374. doi: 10.1093/pcp/pcn013
Parvin, K., Nahaar, K., Hasaanuzzaman, M., Bhuyan, B., Mohsin, S. M., and Fujita, M. (2020). Exogenous vanillic acid enhances salt tolerance of tomato: insight into plant antioxidant defense and glyoxalase systems. Plant Physiol. Biochem. 150, 109–120. doi: 10.1016/j.plaphy.2020.02.030
Pheomphun, P., Treesubsuntorn, C., Jitareerat, P., and Thiravetyan, P. (2019). Contribution of Bacillus cereus ERBP in ozone detoxification by Zamioculcas zamiifolia plants: effect of ascorbate peroxidase, catalase and total flavonoid contents for ozone detoxification. Ecotoxicol. Environ. Saf. 171, 805–812. doi: 10.1016/j.ecoenv.2019.01.028
Ramanjulu, S., Sreenivasulu, N., and Sudhakar, C. (1998). Effect of water stress on photosynthesis in two mulberry genotypes with different drought tolerance. Photosynthetica 35, 279–283.
Rao, K. M., and Sresty, T. (2000). Antioxidative parameters in the seedlings of pigeonpea (Cajanus cajan (L.) Millspaugh) in response to Zn and Ni stresses. Plant Science 157, 113–128. doi: 10.1016/s0168-9452(00)00273-9
Rapacz, M., Wójcik-Jagła, M., Fiust, A., Kalaji, H. M., and Koscielniak, J. (2019). Genome-wide associations of chlorophyll fluorescence OJIP transient parameters connected with soil drought response in barley. Front. Plant Sci. 10:78. doi: 10.3389/fpls.2019.00078
Ricci, G., Bello, M. L., Caccuri, A. M., Galiazzo, F., and Federici, G. (1984). Detection of glutathione S-transferase activity on polyacrylamide gels. Anal. Biochem. 143, 226–230. doi: 10.1016/0003-2697(84)90657-2
Sagi, M., and Fluhr, R. (2001). Superoxide production by plant homologues of the gp91phox NADPH oxidase. Modulation of activity by calcium and by tobacco mosaic virus infection. Plant Physiol. 126, 1281–1290. doi: 10.1104/pp.126.3.1281
Salehi, B., Fokou, P. V., Sharifi-Rad, M., Zucca, P., Pezzani, R., Martins, N., et al. (2019). The Therapeutic potential of naringenin: a review of clinical trials. Pharmaceuticals 12:11. doi: 10.3390/ph12010011
Santa-Cruz, A., Martinez-Rodriguez, M. M., Perez-Alfocea, F., Romero-Aranda, R., and Bolarin, M. C. (2002). The rootstock effect on the tomato salinity response depends on the shoot genotype. Plant Sci. 162, 825–831. doi: 10.1016/s0168-9452(02)00030-4
Seevers, P., Daly, J., and Catedral, F. (1971). The role of peroxidase isozymes in resistance to wheat stem rust disease. Plant Physiol. 48, 353–360. doi: 10.1104/pp.48.3.353
Shan, C. J., Jin, Y. Y., Zhou, Y., and Li, H. (2020). Nitric oxide participates in the regulation of ascorbate-glutathione cycle and water physiological characteristics of Arabidopsis thaliana by NaHS. Photosynthetica 58, 80–86. doi: 10.32615/ps.2019.158
Shatpathy, P., Kar, M., Dwibedi, S. K., and Dash, A. (2018). Seed priming with salicylic acid improves germination and seedling growth of rice (Oryza sativa L.) under PEG-6000 induced water stress. Int. J. Curr. Microbiol. Appl. Sci. 7, 907–924. doi: 10.20546/ijcmas.2018.710.101
Sheldon, A. R., Dalal, R. C., Kirchhof, G., Kopittke, P. M., and Menzies, N. W. (2017). The effect of salinity on plant-available water. Plant Soil 418, 477–491. doi: 10.1007/s11104-017-3309-7
Shu, S., Tang, Y. Y., Yuan, Y. H., Sun, J., Zhong, M., and Guo, S. R. (2016). The role of 24-epibrassinolide in the regulation of photosynthetic characteristics and nitrogen metabolism of tomato seedlings under a combined low temperature and weak light stress. Plant Physiol. Biochem. 107, 344–353. doi: 10.1016/j.plaphy.2016.06.021
Smart, R. E., and Bingham, G. E. (1974). Rapid estimates of relative water content. Plant Physiol. 53, 258–260. doi: 10.1104/pp.53.2.258
Souri, Z., Karimi, N., Farooq, M., and Sandalio, L. (2020). Nitric oxide improves tolerance to arsenic stress in Isatis cappadocica desv. shoots by enhancing antioxidant defenses. Chemosphere 239:124523. doi: 10.1016/j.chemosphere.2019.124523
Strasser, R. J., Srivastava, A., and Govindjee, G. (1995). Polyphasic chlorophyll a fluorescence transient in plants and cyanobacteria. Photochem. Photobiol. 61, 32–42. doi: 10.1111/j.1751-1097.1995.tb09240.x
Strasser, R. J., Tsimilli- Michael, M., and Srivastava, A. (2000). “The fluorescence transient as a tool to characterize and screen photosynthetic samples,” in Probing Photosynthesis: Mechanisms, Regulation and Adaptation, eds M. Yunus, U. Pather, and P. Mohanly (Boca Raton, FL: CRC Press), 445–483.
Strauss, A. J., Kruger, G. H. J., Strasser, R. J., and Van Heerden, P. D. R. (2006). Ranking of dark chilling tolerance in soybean genotypes probed by the chlorophyll a fluorescence transient O-J-I-P. Environ. Exp. Bot. 56, 147–157. doi: 10.1016/j.envexpbot.2005.01.011
Szalai, G., Kellõs, T., Galiba, G., and Kocsy, G. (2009). Glutathione as an antioxidant and regulatory molecule in plants under abiotic stress conditions. J. Plant Growth Regul. 28, 66–80. doi: 10.1007/s00344-008-9075-2
Uzilday, B., Turkan, I., Sekmen, A. H., Ozgur, R., and Karakaya, H. (2012). Comparison of ROS formation and antioxidant enzymes in Cleome gynandra (C4) and Cleome spinosa (C3) under drought stress. Plant Sci. 182, 59–70. doi: 10.1016/j.plantsci.2011.03.015
Varghese, N., Alyammahi, O., Nasreddine, S., Alhassani, A., and Gururani, M. A. (2019). Melatonin positively influences the photosynthetic machinery and antioxidant system of Avena sativa during salinity stress. Plants 8:610. doi: 10.3390/plants8120610
Wada, S., Takagi, D., Miyake, C., Makino, A., and Suzuki, Y. (2019). Responses of the photosynthetic electron transport reactions stimulate the oxidation of the reaction center chlorophyll of Photosystem I, P700, under drought and high temperatures in rice. Int. J. Mol. Sci. 20:2068. doi: 10.3390/ijms20092068
Wang, C., Lu, G., Hao, Y., Guo, H., Guo, Y., Zhao, J., et al. (2017). ABP9, a maize bZIP transcription factor, enhances tolerance to salt and drought in transgenic cotton. Planta 246, 453–469. doi: 10.1007/s00425-017-2704-x
Wang, G. F., Li, W. Q., Li, W. Y., Wu, G. L., Zhou, C. Y., and Chen, K. M. (2013). Characterization of rice NADPH oxidase genes and their expression under various environmental conditions. Int. J. Mol. Sci. 14, 9440–9458. doi: 10.3390/ijms14059440
Wang, R., Zhao, J., Jia, M., Xu, N., Liang, S., Shao, J., et al. (2018). Balance between cytosolic and chloroplast translation affects leaf variegation. Plant Physiol. 176, 804–818. doi: 10.1104/pp.17.00673
Wang, Y., Zhang, X., Hu, Y., Teng, Z., Zhang, S., Chi, Q., et al. (2019). Phenotypic response of tobacco leaves to simulated acid rain and its impact on photosynthesis. Int. J. Agric. Biol. 21, 391–398.
Wu, D., Liang, M. L., Dang, H. X., Fang, F., Xu, F., and Liu, C. J. (2018). Hydrogen protects against hyperoxia-induced apoptosis in type II alveolar epithelial cells via activation of PI3K/Akt/Foxo3a signaling pathway. Biochem. Biophys. Res. Commun. 495, 1620–1627. doi: 10.1016/j.bbrc.2017.11.193
Yildiztugay, E., Ozfidan-Konakci, C., and Kucukoduk, M. (2014). Exogenous nitric oxide (as sodium nitroprusside) ameliorates polyethylene glycol-induced osmotic stress in hydroponically grown maize roots. J. Plant Growth Regul. 33, 683–696. doi: 10.1007/s00344-014-9417-1
Zeliou, K., Manetas, Y., and Petropoulou, Y. (2009). Transient winter leaf reddening in Cistus creticus characterizes weak (stress-sensitive) individuals, yet anthocyanins cannot alleviate the adverse effects on photosynthesis. J. Exp. Bot. 60, 3031–3042. doi: 10.1093/jxb/erp131
Zhang, W., Xie, Z., Wang, L., Li, M., Lang, D., and Zhang, X. (2017). Silicon alleviates salt and drought stress of Glycyrrhiza uralensis seedling by altering antioxidant metabolism and osmotic adjustment. J. Plant Res. 130, 611–624. doi: 10.1007/s10265-017-0927-3
Zhao, X., Chen, T. T., Feng, B. H., Zhang, C. X., Peng, S. B., Zhang, X. F., et al. (2017). Non-photochemical quenching plays a key role in light acclimation of rice plants differing in leaf color. Front. Plant Sci. 7:4. doi: 10.3389/fpls.2016.01968
Keywords: antioxidant enzymes, chloroplast isolation, naringenin, photosynthetic efficiency, stress
Citation: Yildiztugay E, Ozfidan-Konakci C, Kucukoduk M and Turkan I (2020) Flavonoid Naringenin Alleviates Short-Term Osmotic and Salinity Stresses Through Regulating Photosynthetic Machinery and Chloroplastic Antioxidant Metabolism in Phaseolus vulgaris. Front. Plant Sci. 11:682. doi: 10.3389/fpls.2020.00682
Received: 19 February 2020; Accepted: 30 April 2020;
Published: 03 June 2020.
Edited by:
Francisco J. Corpas, Estación Experimental del Zaidín (EEZ), SpainReviewed by:
José A. Hernández, Consejo Superior de Investigaciones Científicas (CSIC), SpainCopyright © 2020 Yildiztugay, Ozfidan-Konakci, Kucukoduk and Turkan. This is an open-access article distributed under the terms of the Creative Commons Attribution License (CC BY). The use, distribution or reproduction in other forums is permitted, provided the original author(s) and the copyright owner(s) are credited and that the original publication in this journal is cited, in accordance with accepted academic practice. No use, distribution or reproduction is permitted which does not comply with these terms.
*Correspondence: Ismail Turkan, aXNtYWlsLnR1cmthbkBlZ2UuZWR1LnRy
Disclaimer: All claims expressed in this article are solely those of the authors and do not necessarily represent those of their affiliated organizations, or those of the publisher, the editors and the reviewers. Any product that may be evaluated in this article or claim that may be made by its manufacturer is not guaranteed or endorsed by the publisher.
Research integrity at Frontiers
Learn more about the work of our research integrity team to safeguard the quality of each article we publish.