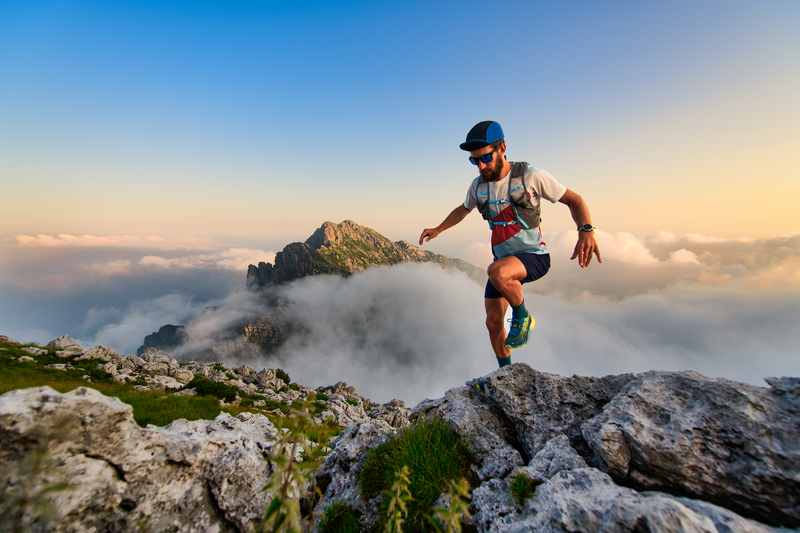
95% of researchers rate our articles as excellent or good
Learn more about the work of our research integrity team to safeguard the quality of each article we publish.
Find out more
REVIEW article
Front. Plant Sci. , 21 April 2020
Sec. Plant Physiology
Volume 11 - 2020 | https://doi.org/10.3389/fpls.2020.00455
This article is part of the Research Topic Lipids, Lipid-Related Biomolecules and Lipid-Protein Interactions Involvement in Photosynthesis View all 10 articles
The xanthophyll cycles of higher plants and algae represent an important photoprotection mechanism. Two main xanthophyll cycles are known, the violaxanthin cycle of higher plants, green and brown algae and the diadinoxanthin cycle of Bacillariophyceae, Xanthophyceae, Haptophyceae, and Dinophyceae. The forward reaction of the xanthophyll cycles consists of the enzymatic de-epoxidation of violaxanthin to antheraxanthin and zeaxanthin or diadinoxanthin to diatoxanthin during periods of high light illumination. It is catalyzed by the enzymes violaxanthin or diadinoxanthin de-epoxidase. During low light or darkness the back reaction of the cycle, which is catalyzed by the enzymes zeaxanthin or diatoxanthin epoxidase, restores the epoxidized xanthophylls by a re-introduction of the epoxy groups. The de-epoxidation reaction takes place in the lipid phase of the thylakoid membrane and thus, depends on the nature, three dimensional structure and function of the thylakoid lipids. As the xanthophyll cycle pigments are usually associated with the photosynthetic light-harvesting proteins, structural re-arrangements of the proteins and changes in the protein-lipid interactions play an additional role for the operation of the xanthophyll cycles. In the present review we give an introduction to the lipid and fatty acid composition of thylakoid membranes of higher plants and algae. We introduce the readers to the reaction sequences, enzymes and function of the different xanthophyll cycles. The main focus of the review lies on the lipid dependence of xanthophyll cycling. We summarize the current knowledge about the role of lipids in the solubilization of xanthophyll cycle pigments. We address the importance of the three-dimensional lipid structures for the enzymatic xanthophyll conversion, with a special focus on non-bilayer lipid phases which are formed by the main thylakoid membrane lipid monogalactosyldiacylglycerol. We additionally describe how lipids and light-harvesting complexes interact in the thylakoid membrane and how these interactions can affect the structure of the thylakoids. In a dedicated chapter we offer a short overview of current membrane models, including the concept of membrane domains. We then use these concepts to present a model of the operative xanthophyll cycle as a transient thylakoid membrane domain which is formed during high light illumination of plants or algal cells.
Xanthophyll cycles, which consist of the de-epoxidation of epoxy-xanthophylls during high light and the epoxidation of epoxy-free xanthophylls during low light or darkness, are found in all eukaryotic photoautotrophs (for reviews see Goss and Jakob, 2010; Niyogi and Truong, 2013; Goss and Lepetit, 2015). The xanthophyll cycles act as an important protection mechanism against damage of the photosynthetic apparatus by supersaturating light conditions (for reviews see Horton, 2014; Ruban, 2016). The main part of photoprotection provided by the xanthophyll cycles operates on the time-scale of minutes and thus allows the plants to react to short-term changes of the light intensities in their natural environment (Demmig-Adams and Adams, 2006; Demmig-Adams et al., 2014). For higher plants fast fluctuations of the light intensity can be induced by clouds or by rapid changes of the leaf coverage in shaded environments such as the tropical rainforests. For algae even moderate water mixing can result in a rapid change of the light intensity from full sunlight to almost complete darkness. In addition, tidal changes affect the light exposure of those species inhabiting the coastal regions. Besides the fast photoprotection the xanthophyll cycles provide long-term protection which lasts for days, weeks or even months (Demmig-Adams and Adams, 2006; Demmig-Adams et al., 2014). These long-lasting photoprotection components can be observed in evergreen plant species which are exposed to prolonged environmental stress like the combination of cold temperatures and high light intensities during the winter months.
The observation that changes in the light intensities lead to inter-conversions of specific leaf xanthophylls dates back to the late 1950s/early 1960s (Hager, 1957; Saphozhnikov et al., 1957; Yamamoto et al., 1962). These first experiments revealed that the xanthophyll violaxanthin (Vx) is converted to the intermediate xanthophyll antheraxanthin (Ax) and then finally to zeaxanthin (Zx) during high light illumination. Later, it was demonstrated that darkness or low light lead to the reversal of the light-driven xanthophyll inter-conversions (Hager, 1967a) and the term Vx cycle was introduced (section Types of Xanthophyll Cycles). In addition, the existence of a second xanthophyll cycle, namely the diadinoxanthin (Ddx) cycle, in several groups of algae was reported (Hager and Stransky, 1970; Stransky and Hager, 1970). Newer measurements have shown that, besides the dominant Vx- and Ddx cycles, further less common light-driven cyclic inter-conversions of xanthophylls exist (Rabinowitch et al., 1975; Goss et al., 1998; Bungard et al., 1999). The following investigations were concerned with the characteristics of the enzymes which carry out the de-epoxidation and epoxidation reactions such as pH-optimum and co-substrate requirements (Hager, 1967a, b; section Reaction Sequences and Xanthophyll Cycle Enzymes). With regard to the function of the xanthophyll cycles it took until the late 1980s that a connection between the conversion of Vx to Zx and the quenching of chlorophyll a fluorescence, which indicates a thermal dissipation of excitation energy, could be obtained and described (Demmig et al., 1987, 1988, section Function of Xanthophyll Cycles). Since then numerous studies have dealt with this process which was called NPQ (reviewed in Horton, 2014; Ruban, 2016) and even today work on the molecular mechanism of NPQ in higher plants and algae represents an important research topic. With respect to the localization of the xanthophyll cycle pigments it was clear from the beginning that the pigments are located within the chloroplast. Later studies have presented evidence that within the plastidic thylakoid membranes the xanthophyll cycle pigments are associated with the light-harvesting complexes of the photosystems (Bassi et al., 1993; Ruban et al., 1994, section Localization of Xanthophyll Cycle Pigments in the Thylakoid Membrane). Regarding the main topic of the present review, i.e., the influence of lipids on the operation of the xanthophyll cycles, first evidence that the main thylakoid membrane monogalactosyldiacylglycerol (MGDG) plays an important role in the conversion of Vx to Ax and Zx dates back to the 1970s (Yamamoto and Higashi, 1978, sections Lipid Classes and Lipids as Solvents for Xanthophyll Cycle Pigments). Later measurements demonstrated that the thylakoid membrane lipids, and especially MGDG, act as solvents of the xanthophyll cycle pigments (Latowski et al., 2004; Goss et al., 2005, section Lipids as Solvents for Xanthophyll Cycle Pigments) and that special three-dimensional lipid structures or phases, i.e., non-bilayer lipid phases, are needed for the efficient operation of the xanthophyll cycles of higher plants and algae (Latowski et al., 2002, 2004; Goss et al., 2005, 2007, sections Three Dimensional Structures of Lipids and Role of Non-bilayer Lipid Phases for Xanthophyll Cycling). Additional investigations could show that the xanthophyll cycle pigments are not only associated with the light-harvesting proteins via special protein binding sites but also exist in lipid shields surrounding the complexes (Lepetit et al., 2010; Schaller et al., 2010; section Localization of Non-bilayer Lipid Phases in the Thylakoid Membrane). Recent research on the lipid dependence of xanthophyll cycling has focused on the localization of the non-bilayer lipid phases within the thylakoid membrane (Garab et al., 2017, section Localization of Non-bilayer Phases Involved in Xanthophyll Cycling) and how these structures are formed during high light illumination (Goss et al., 2007; Jahns et al., 2009, section Formation of Non-bilayer Lipid Phases by Structural Changes of Light-Harvesting Proteins). Models describing the operation of the xanthophyll cycle within the thylakoid membrane have also included information from the newest concepts on the structure and function of biological membranes (Goni, 2014; Nicholson, 2014, section The Xanthophyll Cycle Membrane Domain in the Light of Recent Membrane Models).
One of the most important biological functions of lipids is the formation of membranes within the cell and the cell organelles. In plant cells the largest membrane system is the thylakoid membrane within the chloroplast. Thylakoid membranes, like the inner membranes of mitochondria, are characterized by a high protein per lipid ratio which already indicates that the thylakoid lipids play an important role not only as membrane building blocks, but also as regulators of the structure and function of both integral and peripheral membrane proteins, including the photosynthetic complexes or the xanthophyll cycle enzymes (Krauss, 2001; Kirchhoff et al., 2002; Kobayashi et al., 2016, see also section Xanthophyll Cycles of Higher Plants and Algae). In the thylakoid membranes representatives of the following three lipid classes are present: glycosylglycerol lipids (GGLs), glycerophospholipids (GPs), and prenol lipids (PRs). Both GGL and GP molecules are characterized by the presence of two fatty acyl residues and one trihydric alcohol, namely glycerol. The main difference between these two lipid groups is the presence of a phosphate residue attached to glycerol in GPs, whereas in GGL molecules one or more sugar residues are directly associated with glycerol through glycosidic bonds (Li-Beisson et al., 2016). PRs also represent an important group of thylakoid lipids. They include, among others, quinones such as plastoquinone, phylloquinone (vitamin K1) or tocopherols, as well as isoprenoids such as the carotenoids or the phytol chains of the chlorophyll molecules (Li-Beisson et al., 2016). PRs, and especially carotenoids, play an important role for the variability of the thylakoid lipid composition and can be used for the classification of various groups of algae.
While the thylakoid membranes of different photoautotrophs may differ in the nature of their prenol lipids, the other lipid classes appear to be comparable for different types of thylakoids. Differences are not observed in the general structure of the different lipid classes but rather in their fatty acid (FA) composition and their contribution to the total thylakoid membrane lipid. The most common lipids of thylakoid membranes are three types of GGLs and one representative of the GPs (Figure 1). Among the lipids belonging to the GGLs two lipids, namely digalactosyldiacylglycerol (DGDG) and monogalactosyldiacylglycerol (MGDG), contain a galactopyranosyl residue, whereas the sulfolipid sulfoquinovosyldiacylglycerol (SQDG) carries a glucopyranosyl residue with a sulfonic group at the C6 position of the glucose residue. The first galactose residues of MGDG and DGDG are β-anomeric forms, while the second galactose of DGDG, as well as the glucose residue of SQDG are α-sugars (Hölzl and Dörmann, 2019). In addition to MGDG, DGDG, and SQDG phosphatidylglycerol (PG), a lipid belonging to the class of GPs, is present in the thylakoid membrane (Harwood and Guschina, 2009; Figure 1). While DGDG and MGDG are neutral lipids, SQDG and PG are negatively charged due to the presence of the sulfate and phosphate residues, respectively (Boudiere et al., 2014). The presence of three other representatives of the GPs, namely phosphatidylinositol (PI), phosphatidylcholine (PC), and in the case of the green alga Chlamydomonas reinhardii phosphatidylethanolamine (PE), in thylakoid membranes has been proposed. However, it is still under discussion if these lipids really represent specific components of the inner thylakoid membrane. While in all thylakoids isolated from both plants and algae PI was commonly detected with concentrations up to 5% of the total membrane lipid, it is not clear whether PC and PE simply represent contaminations of thylakoid preparations with lipids from membranes rich in GPs, such as cellular or mitochondrial membranes. In addition, nothing is known so far about the putative role of these three GPs in photosynthesis (Boudiere et al., 2014).
Figure 1. Schematic structures of the four main thylakoid membrane lipids, i.e., the three glycosylglycerol lipids (GGLs) monogalactosyldiacylglycerol (MGDG), digalactosyldiacylglycerol (DGDG) and sulfoquinovosyldiacylglycerol (SQDG), and the one glycerophospholipid (GP) phosphatidylglycerol (PG).
Whereas the presence of PI, and especially that of PC or PE, in thylakoid membranes still needs verification, MGDG, DGDG, SQDG, and PG unequivocally represent the typical lipids of all oxygenic photosynthetic membranes, ranging from cyanobacteria to thylakoids of algae and plants (Boudiere et al., 2014; Kobayashi et al., 2016). Among these four thylakoid lipids, MGDG contributes up to 50% to the total higher plant membrane lipid, DGDG contributes approximately 25%, whereas the content of PG reaches up to 10% and SQDG oscillates in the range from about 2 to 20%. Despite the fact that SQDG is widely considered as the least concentrated of the four main thylakoid lipids, many results have shown that especially the SQDG levels are highly variable in response to different environmental conditions. Thylakoid membranes of higher plants or marine cyanobacteria under phosphate depletion can show maximum levels of SQDG of up to 50 or even 70%, respectively. Additionally, under conditions of phosphate limitation, PG seems to be the only representative of the GP lipids with a content of solely 2% of the total membrane lipid of marine cyanobacteria (van Mooy et al., 2006; Shimojima, 2011; Boudiere et al., 2014). A replacement of PG by SQDG is commonly observed and is considered to be a ubiquitous phenomenon in photosynthetic organisms. Keeping constant the concentrations of the negatively charged lipids most likely ensures the stability of an anionic lipid environment within the photosynthetic membranes under phosphate limitation (Boudiere et al., 2014). With respect to function, SQDG appears to be important for the protection of PSII in halophytes. In general, the level of SQDG was found to be considerably higher in many salt treated halophytes, e.g., in Aster tripolium, Sesuvium portulacastrum, or Crithmum maritimum, although no changes in the sulfolipid contents were observed in glycophytes which were treated with different salt concentration (Ben Hamed et al., 2005; Ramani et al., 2006). In the light of these results it is possible that the high levels of SQDQ reported for various marine photoautotrophs represent an adaptation to the high salt concentration in their natural environment. In 2013 a small amount of a new negatively charged glycolipid was detected in several plant species under phosphorus depletion. The lipid was identified as glucuronosyldiacylglycerol (GlcADG), which contains a glucuronic acid instead of the glucose residue (Okazaki et al., 2013). GlcADG is synthesized by sulfoquinovosyltransferase SQD2, the same glycosyltransferase which is located in the inner envelope membrane of the chloroplast and is responsible for SQDG synthesis in plants and eukaryotic algae (Yu et al., 2002).
In general, the level of SQDG is significantly higher in cyanobacteria and algae than in plants. Within the algae diatoms, brown and red algae seem to be characterized by higher SQDG contents compared with the green algae. High concentrations of SQDG have been reported for the pennate diatom Phaeodactylum tricornutum and the centric diatoms Cyclotella meneghiniana and Skeletonema sp. (Goss et al., 2009; Yan et al., 2011; Lepetit et al., 2012). The high SQDG contents were especially obvious in thylakoid membranes isolated from cultures of P. tricornutum or C. meneghiniana which were grown under high light intensities (Lepetit et al., 2012). In these membranes SQDG represented the most abundant lipid and SQDG and PG contributed to more than 50% to the total membrane lipid. The ratio of neutral to negatively charged lipids was in the range between 1 and 2 whereas in higher plants and green algae values between 3 and 4 are typically observed. The data suggest a significantly higher negative charge of diatom thylakoid membranes compared to the thylakoids of higher plants or green algae. The significance of these extremely charged membranes for the diatom physiology is, however, still unknown (Vieler et al., 2007; Goss and Wilhelm, 2009).
Whereas SQDG and MGDG are almost undetectable in extraplastidic membranes of algae and plant cells, DGDG was shown to be able to substitute phospholipids in non-photosynthetic membranes, like the tonoplast (Andersson et al., 2005), the mitochondrial (Jouhet et al., 2004) or other plasma membranes (Andersson et al., 2003). Replacement of phospholipids by DGDG usually takes place under phosphate-deficient conditions (Härtel and Benning, 2000; Li et al., 2006). Under these limitations DGDG can amount to high concentrations and DGDG concentrations of up to 25% of the total membrane lipid were found in the plasma membranes and tonoplasts of oat root cells (Andersson et al., 2005). DGDG together with MGDG, seems to play a key role in the stabilization of chloroplast membranes under various kinds of environmental stresses such as drought, exposure to ozone, cold or heat stress, which generally result in an increase of the DGDG to MGDG ratio in the photosynthetic membrane (van Besouw and Wintermans, 1978; Heinz and Roughan, 1983; Heemskerk et al., 1986; Chen et al., 2006; Moellering et al., 2010; Moellering and Benning, 2011). This is not surprising, considering that MGDG is a substrate for DGDG synthases which belong to the glycosyltransferases and are upregulated by environmental stress like e.g., phosphate limitation. With respect to the role of MGDG, reduction of the MGDG concentrations of the thylakoid membrane by a down-regulation of MGDG synthases led to an impairment of the photosynthetic performance and photoautotrophic growth (Kobayashi et al., 2007). Moreover, in one of two MGDG-deficient mutants of Arabidopsis thaliana, mgd1-1, with about 40% less MGDG compared to the wild-type plant, a limitation of the Vx de-epoxidation to Ax and Zx was observed (see section Xanthophyll Cycles of Higher Plants and Algae). The reason for this limitation is an almost 40% reduction of the proton conductivity of the thylakoid membrane of the mgd1-1 mutant under light stress (more than 1000 μmol photons m–2 s–1, Aronsson et al., 2008). The lower MGDG concentrations of the mgd1-1 mutant thylakoid membrane led to an increased permeability of the membrane for protons and thus a decreased lumen acidification, resulting in a decreased pH-dependent activation of the xanthophyll cycle enzyme Vx de-epoxidase (VDE, see section Xanthophyll Cycles of Higher Plants and Algae).
Besides the lipids described above, two further lipids were identified in the thylakoid membranes of the green alga C. reinhardtii. One of them, acylsulfoquinovosyldiacylglycerol belongs to the GGLs whereas the other lipid, diacylgyceryl-N-trimethylhomoserine (DGTS), is a representative of the betaine lipids. DGTS was also detected in several other species of green algae as well as in ferns and mosses, but it is unknown if this lipid really represents a genuine thylakoid membrane lipid (Goss and Wilhelm, 2009). Similarly, other exotic lipids, which are often characteristic for only one algal species, such as the betaine lipids DGTA (diacylglycerylhydroxymethyl-N,N,N-trimethyl-β-alanine) and DGCC (diacylglycerylcarboxyhydroxymethylcholine) are found in brown algae or diatoms (Guschina and Harwood, 2006; Goss and Wilhelm, 2009).
However, in the case of C. reinhardtii it was not only demonstrated that DGTS represents a thylakoid lipid, but also that thylakoid DGTS is richer in trienes and C20 FAs than DGTS of other cellular membranes, which contains equal amounts of saturated and tetraene FAs (Janero and Barrnett, 1982). Interestingly, not only the composition of C. reinhardtii thylakoid membranes involves untypical lipids, but also the VDE of this algae seems to be unique (see also section Xanthophyll Cycles of Higher Plants and Algae). The enzyme is located at the stromal side of the thylakoid membrane and is related to a lycopene cyclase of photosynthetic bacteria but not to the typical VDE of plants or other algae (Li et al., 2016). Interestingly, the unique lipid and VDE composition of C. reinhardtii may be seen as one of the indicators of a strong relationship between the xanthophyll de-epoxidases and the lipid composition of the thylakoid membrane.
Both the cross-species acclimation and the species-specific adaptation of biological membranes to various environmental conditions are based not only on changes of the stoichiometry of the individual lipid classes but also on changes of the respective FA residues of the lipids.
In plant cells, plastids, including chloroplasts, play the most important role in these adaptation processes since they represent the organelles where about 95% of the total plant FAs are produced (Ohlrogge et al., 1979). The fundamental importance of FAs was shown by null mutations in many single locus genes of FA synthesis which resulted in plant death during gamete or embryo development. Mutations in genes responsible for the later steps of the GP lipid synthesis seem to be not as deleterious for the establishment of photosynthetically active chloroplasts like mutations in the genes for FA synthesis (Hölzl and Dörmann, 2019).
The first products of phosphatidic acid (PA) acylation in chloroplasts are mainly PAs with residues of oleic acid (18:1) at the sn-1 and palmitic acid (16:0) at the sn-2 position, because the plastidic acyltransferases are specific for 16:0 and 18:1 acyl groups (Frentzen et al., 1983). Subsequently, after dephosphorylation of PA to diacylglycerol (DAG), FA specific desaturases (FADs) form double bonds in the acyl groups. This results in the transformation of 18:1 into linoleic (18:2) and α-linolenic (18:3) acid and the conversion of 16:0 into hexadecenoic (16:1) and hexadecatrienoic (16:3) FA residues. These FAs are commonly found in thylakoid PG (especially 16:1) and MGDG (especially 16:3), as a result of the so-called prokaryotic pathway of FA incorporation into glycerolipids (Higashi and Saito, 2019). On this pathway FAs are directly integrated into the glycerol backbones within the thylakoid membranes. Another way to combine FAs with PA, the so-called eukaryotic pathway, takes place in the membranes of the endoplasmic reticulum (ER). DAGs synthesized within the ER are subsequently transported to the chloroplast to be converted into GGLs. Recently it was determined that under physiological conditions in A. thaliana approximately one half of the plastidic GGLs is formed via the prokaryotic pathway and the other half is synthesized within the eukaryotic pathway. However, the main representative of the thylakoid GPs, namely PG, is predominantly derived from the prokaryotic pathway. Moreover, A. thaliana, like about 12% of the Angiosperm species, belongs to the so-called 16:3 plants, i.e., plants, which under physiological growth conditions, contain more than 10% of the total MGDG pool with 16:3 and 18:3 FA residues (Higashi and Saito, 2019). Plant mutants, with decreased levels of 16:3 or 16:3 and 18:3 FAs, appeared to be more sensitive to low temperatures and expressed growth inhibition and leaf chlorosis at 6 but not at 22°C (Hölzl and Dörmann, 2019). Numerous plant species, including wheat and turf grasses, in which the prokaryotic pathway is not used for the synthesis of GGLs, are termed 18:3 plants. In the thylakoids of these plants GGLs are synthesized via the eukaryotic pathway and thus contain 18:3 at the sn-2 position, while GGLs with 16:3 FAs can only be found in trace amounts (Roughan and Slack, 1984; Mongrand et al., 1998; Higashi and Saito, 2019). Additionally, nowadays 468 plant species are known, whose leaf FA profile suggests a loss of the prokaryotic pathway during evolution (Mongrand et al., 1998). Moreover, in plants possessing both active pathways of PA acylation, the temperature was shown to play an important role in the balance between the prokaryotic and eukaryotic pathways. Decreases of the expression of important genes of the prokaryotic pathway during heat stress lead to reduced levels of 16:3 FAs in MGDG and DGDG at the sn-2 position (Higashi et al., 2015; Li et al., 2015). In addition, FAD8, which introduces double bonds at the ω-3 position of the saturated acyl chains of the MGDG molecule, is degraded under high temperatures (Matsuda et al., 2005). Heat-stress also leads to a hydrolization of the 18:3 FAs of MGDG by a chloroplast heat-inducible lipase (HIL1), which in this way initiates and contributes to the MGDG degradation under high temperatures. The concerted action of these mechanisms explains the decreased levels of MGDG and DGDG-bound polyunsaturated FAs (PUFAs) during heat stress (Higashi et al., 2018). Interestingly, in chloroplasts, the decrease of 18:3 FAs is accompanied by an increased concentration 18:2 acyl chains in the GGLs (Higashi and Saito, 2019).
Temperature is not the sole environmental factor resulting in modification of the FA composition of the thylakoid lipids. Thylakoid membrane lipids and the FAs bound to the lipids are involved in the protection against a great number of biotic and abiotic stresses generated by various environmental factors (Wang et al., 2014). Among the thylakoid lipids, MGDG plays a central role in these protection mechanisms. MGDG is highly enriched in 18:3 and 16:3 PUFAs whereas the other membrane lipids DGDG, SQDG, and PG also contain saturated FAs in the form of 16:0. MGDG seems to be involved in the protection against reactive oxygen species (ROS) and it has been suggested that MGDG molecules surrounding photosystem (PS) I and II act as efficient scavengers of 1O2 as well as hydroxyl radicals which are mainly created within PSII (Schmid-Siegert et al., 2016). MGDG is furthermore engaged in a cyclic mechanism with antioxidative function. In this process lipid peroxidation products such as malondialdehyde (MDA) are formed within the GGL molecules, which, after self-regeneration, can again be fragmented into MDA. This way, the PUFAs bound to the thylakoid membrane GGLs act as a sink for various types of ROS (Mène-Saffrané et al., 2009; Schmid-Siegert et al., 2016). Moreover, 18:3 FAs are postulated to act as direct non-enzymatic scavengers of ROS (Mène-Saffrané et al., 2009).
Besides the formation of MDA, oxidative stresses, including heat and high light stress, lead to a conversion of the lipid-bound PUFAs to lipid peroxides (LOOHs). This lipid peroxidation generates different types of harmful chemical components such as the secondary products of lipid peroxidation and ROS. Lipid peroxidation products subsequently cause protein cleavage, protein oxidation or crosslinking between proteins, lipids and proteins with lipids. The numerous molecules which provide protection against or are formed by the action of ROS and lipid peroxidation products within the thylakoid membrane include (i) large complexes, like the central PSII dimers which dissociate from the surrounding LHCs, (ii) smaller molecules, like the photodamaged D1 protein together with degraded zexanthin expoxidase (ZEP, see section Xanthophyll Cycles of Higher Plants and Algae), and finally (iii) small molecules, like the de-epoxidized xanthophylls of the xanthophyll cycle (see section Xanthophyll Cycles of Higher Plants and Algae). All of these molecules and processes require a free movement in the thylakoid membrane to exert their protective function (Jahns et al., 2009; Kirchhoff, 2014; Yamamoto, 2016). Free movement in the thylakoid membrane depends on the fluidity of the membrane which itself is determined by the structure and composition of the FAs bound to the thylakoid membrane lipids. Highly unsaturated FAs lead to a membrane in a more fluid state whereas a high concentration of saturated FAs increases the rigidity of the thylakoids. Thus, changes of the FA composition of thylakoid membrane lipids in response to various environmental stresses seem to play an important role in the remodeling and functional stabilization of the membranes and the integrated protection mechanisms. With regard to the FA composition it has become clear that photoautotrophs inhabiting different ecosystems show pronounced differences in the FA profiles of both MGDG and the other thylakoid lipids. While in general the FA composition of the thylakoid lipids of the majority of freshwater green algae is comparable to that of 16:3 plants, the algal lipids seem to be enriched in 16C PUFAs while the C18 PUFAs exhibit lower concentrations. On the other hand, marine green algae are rich in C18 PUFAs, whereas red algae show high contents of C20 PUFAs, such as the 20:5 eicosapentaenoic acid (EPA) or the 20:4 arachidonic acid (AA). High levels of both C18 and C20 PUFAs are also typical for brown algae (Goss and Wilhelm, 2009; Kumari et al., 2013).
In diatoms MGDG contains the main long-chain FA of diatoms, i.e., eicosapentaenoic acid (EPA, 20:5, Yongmanitchai and Ward, 1993; Yan et al., 2011; Dodson et al., 2013; Dodson et al., 2014). EPA is preferentially bound to the sn-1 position of the glycerol backbone whereas the sn-2 position is usually occupied by C16 FAs with varying degrees of unsaturation (16:1, 16:2, 16:3, 16:4). MGDG with C20:5 and C16:3 seems to represent the most abundant form of the diatom GGL. Besides the C20/C16 forms of MGDG, MGDG molecules with C16 FAs at both the sn-1 and sn-2 position can be observed. DGDG exhibits a comparable FA composition to MGDG and is also enriched in EPA (Yongmanitchai and Ward, 1993; Yan et al., 2011; Dodson et al., 2013, 2014). Like in the MGDG molecule EPA usually occupies the sn-1 position of DGDG while at the sn-2 position C16 FAs are observed. As it has been demonstrated for MGDG, DGDG forms with C16 FAs at both the sn-1 and sn-2 positions can be found and DGDG with C20:5 and C16:3 seems to represent the most abundant diatom DGDG molecule. Earlier studies have reported that a difference exists in the FA composition of centric and pennate diatoms and that in the pennate diatoms EPA is replaced by C18 FAs in the MGDG and DGDG molecules (Dodson et al., 2013). However, more recent studies have indicated that the differences in the FA composition of MGDG and DGDG of centric and pennate diatoms may be related to differences in their reaction to environmental temperatures and that EPA is present at lower temperatures and may be replaced by C18 FAs at higher temperatures (Dodson et al., 2014). The anionic membrane lipid SQDG of diatoms seems to be enriched in FAs with a shorter chain length and C14 and C16 FAs are usually observed in both the sn-1 and sn-2 positions (Yongmanitchai and Ward, 1993; Yan et al., 2011). The second anionic lipid PG, on the other hand, seems to contain C18:1 FAs as the main molecular species (Yan et al., 2011).
The FA composition of MGDG also influences the three-dimensional structure of the MGDG molecule (see section Three Dimensional Structures of Lipids). Among the thylakoid lipids, MGDG represents the sole non-bilayer lipid and forms the so-called inverted hexagonal phases (HII-phases) in aqueous solutions. The ability of MGDG to form HII-phases as well as the properties of the HII-phase, such as the flexibility, strongly depend both on the proportion of MGDG in a lipid mixture and the FA composition of the MGDG molecule. High concentrations of MGDG-bound PUFAs seem to facilitate the HII formation (Kobayashi et al., 2016).
All lipids can be divided into two groups depending on the type of lipid phases they create in aqueous systems. These groups are the bilayer-forming and the non-bilayer-forming lipids. Lipids of the first group aggregate to bilayers, which form lamellar phases in one of the two main states: (i) “fluid” lamellar liquid crystalline (Lα) phases, also referred to as liquid-disordered (Ld, Ll/d) phases, or (ii) “solid” lamellar gel (Lβ) phases, which are also designated as ordered solid (So) phases. The second group of lipids includes non-bilayer-forming lipids which can aggregate to normal or inverted (reversed) phases which are denoted with subscripts I and II, respectively. Normal phases, which are commonly observed in neutral lipid/water systems, are micellas (L1), normal discontinuous cubic phases (II), normal hexagonal phases (HI) and the normal bicontinuous cubic phases (QI). The reversed phases include inverted micellas (L2), reversed bicontinuous cubic phases (QII), the reversed discontinuous cubic phases (QIII), and the inverted hexagonal phases (HII) (Huang and Gui, 2018). Types of aggregates, as well as types of phases created by the lipids, are determined both by geometry of the lipid molecule and several physicochemical parameters of its surroundings. The chemical geometry of the lipid molecule is described by the critical packing parameter (CPP) which denotes the ratio of the maximum volume, which can be occupied by the FA residues (V), and the product of the length of these residues (l) and the cross-sectional area of the hydrophilic lipid headgroups (a) (Table 1; Yamashita, 2018). The relatively small polar headgroup of the MGDG molecule, accompanied by the large area occupied by the expanded PUFA tails, results in a cone-like geometry of the molecule. This structure enables MGDG to spontaneously form HII-phases in both aqueous systems and model or natural lipid membranes (Shipley et al., 1973). HII-phases consist of a great number of tightly packed cylindrical micelles with a diameter between 1–2 nm and contain 30–60 weight percent of water. DGDG, SQDG and PG possess a cylindrical shape due to the large headgroup areas and the lower content of longer-chain PUFAs and thus belong to the bilayer-forming lipids (Jouhet, 2013). Besides the influence of the inherent chemical structures of the lipid molecules, the formation of lipid phases is strongly affected by the neighboring molecules like proteins, pigments, other lipids or even ions. Divalent cations, for example, facilitate the formation of HII-phases. With respect to the lipid phases formed by MGDG it has been demonstrated that the proteins involved in the phototransformation of protochlorophylls support the formation of a cubic phase (Selstam, 1998). For the function of the thylakoid membrane it is of high importance that the LHCII strongly interacts with MGDG and forces the MGDG molecules into a membrane bilayer structure (Simidjiev et al., 2000). In thylakoid membranes, MGDG seems to play a key role in providing the membrane fluidity which is essential for the efficient diffusion of the xanthophll cycle pigments (see section Xanthophyll Cycles of Higher Plants and Algae), LHC proteins or proteins involved in the turnover and repair of PSII and ZEP. Thus, MGDG and the HII-phases created by MGDG are not only important for chlorophyll biosynthesis during chloroplast development (Jarvis et al., 2000), but also play significant role in the proper functioning of the photosynthetic machinery in differentiated chloroplasts (Zhou et al., 2009; Schaller et al., 2011). On a molecular level MGDG molecules have been shown to be integral parts of the PSI and PSII core complexes (van Eerden et al., 2017) where they promote the PSII dimerization or form a cavity for the docking of plastoquinone QB (Guskov et al., 2009; Kansy et al., 2014). However, the importance of the three-dimensional structures formed by MGDG for the function of the MGDG molecules located within the PSI and PSII core complexes remains to be clarified.
Table 1. Dependence of the lipid self-assembly structures on the value of the critical packing parameter (CPP).
Recently, the results of an in silico study using the PSII x-ray structure of the thermophilic cyanobacterium Thermosynechococcus vulcanus showed that lipid domains surrounding the PSII core complex may be also enriched in SQDG. Like it was discussed above for MGDG, it is not clear if and how the three-dimensional phases formed by SQDG influence the PSII structure and function (van Eerden et al., 2017).
With respect to the HII-phases formed by MGDG it was demonstrated that physical factors, like a high temperature or dehydration, can also induce the formation of non-lamellar phases. Low pH-values, as they are typical for the thylakoid lumen during high light illumination have also been shown to promote the formation of HII-phases by MGDG (Garab et al., 2017, see also section Localization of Non-bilayer Lipid Phases in the Thylakoid Membrane).
The main xanthophyll cycles that are known today are the violaxanthin (Vx) cycle of higher plants, green and brown algae and the diadinoxanthin (Ddx) cycle of diatoms, haptophytes and dinophytes (Figure 2, for a review see Goss and Jakob, 2010). Algae containing the Ddx cycle also contain the pigments of the Vx cycle because Vx is a precursor in the biosynthesis pathway of the Ddx cycle pigments (Lohr and Wilhelm, 1998, 2001). The presence of Vx cycle pigments in Ddx cycle containing algae is especially visible during longer periods of high light exposure when a pronounced de novo synthesis of Ddx cycle pigments is taking place. In addition to these two xanthophyll cycles, the existence of a lutein epoxide (Lx) cycle has been shown which is, however, restricted to some families of higher plants (Esteban and Garcia-Plazaola, 2014). Some members of the Prasinophyceae, which represent a class of unicellular green algae, or some species within the genus Gracilaria belonging to the Rodophyta are characterized by a modified Vx cycle (Goss et al., 1998; Bertrand and Schoefs, 1999; Cardol et al., 2008).
Figure 2. Reaction sequences and enzymes of the violaxanthin (A) and diadinoxanthin (B) cycle. The violaxanthin cycle is present in higher plants and green and brown algae, the diadinoxanthin cycle is found in diatoms, haptophytes and dinophytes. Figure 2 also shows the cofactor requirements of the enzymes catalyzing the de-epoxidation reaction (violaxanthin de-epoxidase, VDE and diadinoxanthin de-epoxidase, DDE) and the epoxidation reaction (zeaxanthin epoxidase, ZEP and diatoxanthin epoxidase, DEP), respectively. Representation of the cofactors in bold or normal type indicates whether high or low concentrations of the respective cofactors are needed for high enzyme activity. The establishment of a proton gradient inhibits diatoxanthin epoxidation (high ΔpH control) and is thus presented in bold type whereas zeaxanthin epoxidation is unaffected by the presence of the transmembrane ΔpH (ΔpH control depicted in normal type). The pH value of the thylakoid lumen which leads to VDE and DDE activation (possibly by VDE or DDE dimerization) and membrane binding is also indicated for the two xanthophyll cycles.
The xanthophyll cycles consist of forward reactions which take place during illumination of plants or algae with high light illumination and back reactions which revert the forward reaction during periods of low light exposure or darkness. In the Vx cycle the forward reaction consists of a two-step de-epoxidation of the di-epoxy xanthophyll Vx to the mono-epoxide Ax and finally to the epoxy-free Zx (Yamamoto et al., 1962; Hager, 1967a). The back reaction re-introduces the two epoxy groups into the Zx molecule and regenerates Vx via the intermediate reaction product Ax (Hager, 1967a). The Vx cycle of the Prasinophyceae and of some species of the genus Gracilaria is incomplete and during high light illumination an accumulation of the intermediate de-epoxidation product Ax is observed in Prasinophycean cells (Goss et al., 1998; Cardol et al., 2008). In the red algae Graciliaria gracilis or G. multipartite an absence of Vx was detected and Ax seems to be the only substrate which can be converted to Zx (Bertrand and Schoefs, 1999). Despite the ongoing uncertainties about the presence of a xanthophyll cycle in Rodophyta, a recent study has presented clear evidence that at least a Zx epoxidase, which is able to convert Zx to Ax, is present in the red algae (Dautermann and Lohr, 2017).
Thus, these two modifications of the Vx cycle, like the Ddx and the Lx cycles, consist of only one de-epoxidation and one epoxidation step. In the Vx cycle of the Prasinophyceae the xanthophyll di-epoxide Vx is converted to the mono-epoxide Ax whereas in all other one-step xanthophyll cycles mono-epoxides such as Ax, Ddx and Lx are converted to the epoxy-free xanthophylls such as Zx, diatoxanthin (Dtx), and lutein (L), respectively (Hager and Stransky, 1970; Stransky and Hager, 1970; Rabinowitch et al., 1975; Bungard et al., 1999; Bertrand and Schoefs, 1999). In the back reaction one epoxy-group is re-introduced into the epoxy-free xanthophylls Dtx or L and Ddx or Lx are regenerated. The forward reaction of the Vx cycle is catalyzed by the enzyme Vx de-epoxidase (VDE), the back reaction by the enzyme Zx epoxidase (ZEP). Both the VDE and the ZEP belong to a family of diverse proteins, the so-called lipocalins (Hieber et al., 2000; Grzyb et al., 2006). Lipocalins usually bind hydrophobic molecules and act as carrier proteins for their substrates. VDE is localized in the thylakoid lumen and has a pH-optimum of around pH 5.2 (Hager, 1969; Pfündel et al., 1994). During high light illumination the light-driven proton gradient leads to a decrease of the pH-value of the thylakoid lumen, thereby activating the VDE. Activation of VDE is probably driven by a dimerization of the water-soluble monomeric VDE, followed by the binding of the active, dimeric VDE to the lumen side of the thylakoid membrane (Hager and Holocher, 1994; Arnoux et al., 2009; Saga et al., 2010). With regard to the dimerization it has been proposed that the C-terminus of the VDE plays a role in the interaction of the VDE monomers (Hallin et al., 2016) and that four specific amino acid residues are important for the pH-dependent activation (Fufezan et al., 2012). In addition, it has been suggested that the conserved cysteine residues and the disulfide bridges formed by the cysteines are sensitive to redox changes of the thylakoid membrane induced by the photosynthetic electron transport (Hallin et al., 2015; Simionato et al., 2015). Changes of the thylakoid redox potential may play a role in the regulation and activation of the VDE via dithiol/disulfide exchange reactions. Reduced ascorbate has been identified as the co-substrate of the de-epoxidation reaction and is important for the reduction of the epoxy group and the subsequent abstraction as H2O (Hager, 1969). Interestingly, a recent study has reported that the atypical VDE of the green algae Chlamydomonas reinhardtii (Li et al., 2016) does not require the presence of ascorbate (Vidal-Meireles et al., 2020). In contrast to the VDE the ZEP seems to be permanently associated with the stromal side of the thylakoid membrane as a peripheral membrane protein (Schaller et al., 2012b). ZEP uses O2 and NADPH + H+ as co-substrates to re-introduce the epoxy group into the Zx and Ax molecule, respectively (Hager, 1967a). The VDE of higher plants and green algae is characterized by a higher substrate affinity for Ax compared with Vx which results in faster kinetics of the second de-epoxidation step from Ax to Zx compared with the first de-epoxidation step from Vx to Ax (Frommolt et al., 2001; Goss, 2003). However, the VDE of the Prasinophyceae differs from the VDE of higher plants and other green algae and exhibits a higher substrate affinity for Vx compared with Ax (Goss, 2003). This results in a very slow second de-epoxidation step from Ax to Zx and together with the simultaneous epoxidation reaction results in the accumulation of Ax during high light illumination in these green algae (Frommolt et al., 2001). Interestingly, the decreased substrate affinity of the VDE of the Prasinophyceae is not restricted to the mono-epoxide Ax but the enzyme is characterized by a generally low substrate affinity for xanthophyll mono-epoxides whereas xanthophyll di-epoxides like Vx are converted with high efficiency. For the Lx cycle which occurs in some families of higher plants it has been suggested that the VDE and ZEP also carry out the additional de-epoxidation of Lx to L and from L back to Lx (Esteban et al., 2009). The enzymes of the Ddx cycle in diatoms show some differences to the respective enzymes in higher plants and green algae. VDEs in diatoms and some other groups of algae, like dinophytes, haptophytes or phaeophytes, are also denoted as diadinoxanthin de-epoxidases (DDEs). Before 2007 the presence of different VDE genes and thus the presence of different VDEs/DDEs in organisms containing the Ddx cycle was not known and the measurements dealing with the properties of the DDE were ascribed to one single enzyme. It was shown that the DDE is characterized by a pH-optimum which is shifted toward higher pH-values (Jakob et al., 2001). In addition, it has been reported that DDE activity and thus the de-epoxidation of Ddx to Dtx can take place at neutral pH-values. In addition, DDE is able to operate efficiently with lower concentrations of the co-substrate ascorbate compared with the VDE of higher plants (Grouneva et al., 2006).
It might be possible that a relationship exists between the higher SQDG and lower MGDG concentrations of the diatom thylakoid membrane (see section Lipid Classes) and the broad pH-optimum of the diatom DDE. Taking into account that the thylakoid membranes of the A. thaliana mgd1-1 mutant, which are strongly reduced in their MGDG content, are impaired in their ability to build-up a strong proton gradient during illumination (Aronsson et al., 2008, see also section Lipid Classes), a comparable situation might occur in the diatom thylakoids with their low MGDG concentration. The possible inability of the diatom thylakoid membranes to generate a very strong ΔpH would then require the onset of DDE activity at a weaker pH-gradient across the membrane and thus at higher luminal pH-values. Such a behavior, i.e., DDE activity at almost neutral pH-values, has been observed in in vitro experiments where the pH-activity and pH-optimum of the DDE were determined (Jakob et al., 2001).
Today, it is clear that the diatom genome codes for more than one de-epoxidase. Two DDE-encoding genes were shown to be present in the centric diatom Thalassiosira pseudonana (Montsant et al., 2007). One of these genes (labeled as TpVDE) is similar to the genes encoding the typical plant VDEs while the other (designated as violaxanthin de-epoxidase-like; TpVDL) is more distantly related. Later, it was demonstrated that the genome of the pennate diatom Phaeodactylum tricornutum contains one gene similar to the genes of “typical VDEs” (termed PtVDE) and two VDE-like genes, designated as PtVDL1 and PtVDL2 (Coesel et al., 2008). Important differences can also be observed for the epoxidation reaction of the Ddx cycle. Here it has been demonstrated that the Dtx epoxidase (DEP) shows significantly higher Dtx epoxidation rates than the ZEP of higher plants and green algae (Mewes and Richter, 2002; Goss et al., 2006). The kinetics of the conversion of Dtx to Ddx are almost comparable to the very fast de-epoxidation of Ddx to Dtx by the DDE. To avoid a futile cycle and to enable a fast accumulation of Dtx during illumination with high light intensities the DEP underlies a strict light-dependent control (Mewes and Richter, 2002; Goss et al., 2006). DEP activity is completely suppressed during high light illumination by the build-up of the light-driven proton gradient. During low light illumination or during periods of darkness when no or only a weak trans-membrane proton gradient is present, DEP is fully activated and Dtx is rapidly converted back to Ddx.
With respect to the localization of the xanthophyll cycle pigments in the thylakoid membrane two main pools can be differentiated. The first pool consists of xanthophyll cycle pigments which are bound to the light-harvesting proteins. In higher plants and green algae the majority of the protein-bound Vx cycle pigments is associated with the light-harvesting complex of photosystem II (LHCII), which represents the main thylakoid membrane protein (Ruban et al., 1999), although proteins of the light-harvesting complex of photosystem I (LHCI) also bind Vx, as well as L and β-carotene (Croce et al., 2002, 2007). Interestingly, recent data have shown that the binding of the xanthophyll cycle pigments can have an impact on the supramolecular structure of LHCII (Zhou et al., 2020). LHCII contains a special binding site for Vx which has been termed V1. V1 is located at the periphery of each LHCII apoprotein and contains a loosely bound Vx molecule (Morosinotto et al., 2003). In the minor PSII antenna proteins CP29, CP26, and CP24, which contain higher concentrations of Vx cycle pigments compared to the LHCII, Vx seems to occupy the L2 binding site (Morosinotto et al., 2003). This binding site is usually responsible for the association of one of the two lutein molecules in the LHCII. In contrast to the V1 site the L2 site is not located at the periphery but represents an internal pigment binding site. Experiments with recombinant LHCII and minor PSII antenna proteins have shown that only Vx, which is associated with the peripheral V1 binding site, can be efficiently converted to Ax and Zx (Jahns et al., 2001; Wehner et al., 2006). Vx bound to the internal L2 binding site is not easily accessible by the VDE and no or only a very limited de-epoxidation can be observed. The loose association of Vx with the LHCII apoprotein at the peripheral binding site is thought to enable the efficient detachment of Vx from the protein during high light illumination followed by the diffusion into the lipid phase of the thylakoid membrane where the actual enzymatic conversion to Ax and Zx by the enzyme VDE is taking place (Latowski et al., 2002; Goss et al., 2007). Recently, it has been shown that the binding of neoxanthin (Nx) to the LHCII affects the binding affinity of Vx (Wang et al., 2017). In the presence of Nx Vx is only weakly bound to the LHCII, easily dissociates into the lipid phase of the membrane, thereby enhancing the first step of the de-epoxidation reaction. In contrast to the general assumption that Zx rebinds to the Vx binding sites at the LHCII and the minor PSII antenna proteins after the de-epoxidation of Vx, Xu et al. (2015) provided evidence that Zx does not necessarily exchange for Vx in the internal binding sites. It may be located in the periphery of the complexes and exert its quenching capacity in a position between the LHCs.
In diatoms the protein bound Ddx cycle pigments are located in the different fucoxanthin chlorophyll protein (FCP) complexes. The recent elucidation of the molecular structure of an FCP complex composed of Lhcf3 and Lhcf4 by x-ray crystallography (Wang et al., 2019) showed that, like Vx in the LHCII, Ddx is located at the periphery of the apoprotein and thus most likely also loosely bound. Like the easy detachment of Vx from the LHCII followed by the rebinding of Zx, the peripheral binding of Ddx is thought to facilitate the exchange with Dtx during the operation of the Ddx cycle. Interestingly, the binding site of the Ddx molecule seems to be located at the opposite side of the apoprotein compared with the Vx binding site in the LHCII. Besides the Lhcf proteins which build-up the peripheral antenna system of diatoms, but may also be more closely associated with the PSII core complex, the Lhcr proteins which form the PSI-specific antenna of diatoms, bind Ddx cycle pigments. According to Lepetit et al. (2008) the concentration of Ddx and Dtx seems to be even slightly higher in the PSI antenna compared to the peripheral FCP complex. Like higher plants and green algae diatoms show an increase of the xanthophyll cycle pigment pool upon cultivation with higher light intensities (Lavaud et al., 2003; Schumann et al., 2007; Gundermann and Büchel, 2008; Lepetit et al., 2010; Gundermann et al., 2019). The increase of the Ddx cycle pigment pool size is significantly more pronounced compared with the increase of the Vx cycle pigment concentrations. With respect to the additional Ddx and Dtx synthesized during exposure to high light intensities it has been proposed that a part of these additional Ddx cycle pigments is bound by the photoprotective Lhcx proteins which also show a stronger expression under high light conditions (Lepetit et al., 2013). In the centric diatoms which are characterized by a more complicated antenna system than the pennate diatoms, it could be shown that both the FCPa and FCPb complexes bind Ddx cycle pigments (Gundermann and Büchel, 2008). In the FCPa complex increased Ddx binding during high light cultivation was accompanied by an increased content of the Fcp6 and Fcp7 proteins. Interestingly, Dtx-induced decreases of the FCP fluorescence emission could only be observed in the FCPa.
It is noteworthy that the differences in the main thylakoid membrane proteins, i.e., the light-harvesting complexes (LHCs), go together with differences in the lipid and FA composition of the thylakoids (see sections Lipid Classes and Fatty Acids). In higher plants and green algae, which contain the LHCII and LHCI, the thylakoid lipids are rich in C16 and C18 FAs. Diatoms and brown algae, which are characterized by the presence of Fx, contain MGDG and DGDG molecules with a high concentration of C18 and C20 FAs. It may be possible that the differences in the FA composition of MGDG and DGDG between the green lineage and diatoms/brown algae are related to differences in the structures of LHCs and FCPs. Furthermore, these differences may represent an optimal harmonization of the thylakoid membranes to allow the best possible LHC/FCP structure and function, i.e., light-harvesting or non-photochemical quenching of Chl a fluorescence (NPQ, see section Function of Xanthophyll Cycles). With respect to the different xanthophyll cycles in higher plants/green algae and diatoms the differences in the lipid and FA composition may even influence the interplay between the LHCs/FCPs and the xanthophyll cycle enzymes. In this regard, it is interesting that the Vx cycle enzymes of brown algae, which contain FCP complexes and are enriched in C18 and C20 FAs, show some of the typical features of the Ddx cycle enzymes of diatoms, like e.g., a fast epoxidation reaction (Garcia-Mendoza and Colombo-Pallotta, 2007).
The second main pool of xanthophyll cycle pigments consists of Vx or Ddx cycle pigments which are not protein bound but localized as free pigments in the lipid phase of the thylakoid membrane. First evidence for the existence of free Zx molecules was obtained from studies on the fluidity of thylakoid membranes which showed that the conversion of Vx to Zx leads to a rigidification of the membrane (Gruszecki and Strzalka, 1991, 2005; Tardy and Havaux, 1997, see also section Function of Xanthophyll Cycles). Isolation of LHCII with different preparation methods led to the purification of LHCII with different concentrations of native lipids and Vx cycle pigments (Schaller et al., 2010). Further analysis of the LHCII preparations demonstrated that the concentration of LHCII-associated Vx was correlated with the amount of MGDG which was isolated with the complexes. Decreases of the MGDG content led to a decrease of the Vx concentration, indicating that a part of the Vx cycle pigment pool was protein-bound whereas another part was localized within an MGDG-shield surrounding the LHCII. Comparable results were obtained for the Prasinophyceae Mantoniella squamata where LHC could be isolated which contained high concentrations of MGDG and Vx (Schaller et al., 2012a). In diatoms which are characterized by a strong increase of the Ddx cycle pigment pool during high light exposure a comparable separation between a protein bound and lipid dissolved Ddx cycle pigments could be observed. The first indication for a pool of Ddx cycle pigments, which are not bound to FCP complexes, was obtained from measurements of NPQ of high light cultivated diatoms (Schumann et al., 2007). These measurements indicated that additional Dtx synthesized during high light treatment is not able to enhance NPQ and thus cannot be bound to the respective binding sites of the FCP complexes. Additional experiments with isolated FCP complexes from low and high light cultivated diatom cells demonstrated that the additional Ddx cycle pigments show the same absorption spectrum as purified Ddx which is dissolved in MGDG (Lepetit et al., 2010). The enrichment of MGDG in the isolated FCP complexes led to the conclusion that, like in higher plants, the diatom antenna complexes are surrounded by an MGDG shield which incorporates a part of the Ddx cycle pigments. Also like in higher plants the free Ddx cycle pigments have been shown to play a role in the modulation of the thylakoid membrane fluidity (Bojko et al., 2019, see section Function of Xanthophyll Cycles).
The xanthophyll cycles of plants and algae are important protection mechanisms against damage to the photosynthetic apparatus induced by high light intensities. The function of the xanthophyll cycles as photoprotective mechanisms is trifold: (i) they play an important role in the dissipation of excessive excitation energy as heat in the process of NPQ (Goss and Lepetit, 2015; Ruban, 2016), (ii) they are able to directly scavenge ROS within the thylakoid membrane (Havaux, 1998; Triantaphylidès and Havaux, 2009) and (iii) they serve as stabilizers of the lipid phase of the thylakoid membrane (Gruszecki and Strzalka, 2005; Bojko et al., 2019).
With respect to the process of NPQ the de-epoxidized xanthophylls Zx and Dtx have been shown to induce a structural change of the light-harvesting complexes of higher plants (Ruban et al., 1992, 1997; Holzwarth et al., 2009) and diatoms (Gundermann and Büchel, 2008; Miloslavina et al., 2009; Schaller-Laudel et al., 2015). This structural change leads to the transformation of excessive excitation energy into heat followed by the harmless dissipation of thermal energy. In higher plants protonation of the LHCII and the presence of the PsbS protein (Li et al., 2000) are further essential factors which regulate NPQ and thus the structural change of the PSII antenna. In this respect, Welc et al. (2016) have shown that Vx cycle pigments which are not bound to the LHCII can nonetheless modulate the structure of the LHCII. Vx seems to promote the formation of LHCII supramolecular structures whereas free Zx induced an LHCII structure suitable for the dissipation of excessive excitation energy. Recently, an interaction between Zx and the PsbS protein has been described which leads to a preferential association of PsbS with the minor PSII antenna proteins (Sacharz et al., 2017). PsbS itself seems to form clusters and may act as initiator for LHCII aggregation. LHCII aggregation can also be induced in vitro by the addition of Mg2+ ions (Schaller et al., 2014) which in the chloroplast act as counter-ions to the light-induced proton influx from the stroma to the thylakoid lumen during the build-up of the transmembrane proton gradient. In green algae comparable LHCII aggregation has been observed during the induction of NPQ, the role of the PsbS protein in the establishment of NPQ has, however, been replaced by the LHCSR proteins (Peers et al., 2009; Bonente et al., 2011; Gerotto and Morosinotto, 2013). Like PsbS the LHCSR3 protein has been proposed to sense the decrease of the pH in the thylakoid lumen during illumination (Ballottari et al., 2016). Nawrocki et al. (2019) have shown that LHCSR proteins are responsible for NPQ in Chlamydomonas reinhardtii but that PsbS proteins also play a role in photoprotection. LHCII aggregation is characterized by a shift of the chlorophyll a fluorescence emission to longer wavelengths (Holzwarth et al., 2009) which, interestingly, can also be observed upon the aggregation of FCP complexes in diatoms (Miloslavina et al., 2009; Lavaud and Lepetit, 2013). In diatoms the Lhcx proteins have been demonstrated to adopt the role of the PsbS protein (Bailleul et al., 2010; Zhu and Green, 2010; Taddei et al., 2016, 2018). Recently, it was demonstrated that NPQ depends on the concerted action of the Ddx cycle and the Lhcx proteins and that Lhcx proteins provide photoprotection via the thermal dissipation of excitation energy (Buck et al., 2019). For both higher plants (Holzwarth et al., 2009; Jahns and Holzwarth, 2012) and diatoms (Goss and Lepetit, 2015; Taddei et al., 2018) models for the localization and function of NPQ have been established. These models predict the formation of two quenching sites where the transformation of excitation energy into heat is taking place. Quenching site Q1 is composed of detached LHCII and FCP complexes, respectively, which after their dissociation from the PSII core complex undergo a structural change and form aggregates. NPQ at quenching site Q1 seems to be independent of the de-epoxidation of Vx to Zx. For the formation of quenching site Q2, however, the presence of Zx is important. Quenching site Q2 is located in the vicinity of the PSII core complex and most likely involves the minor PSII antenna proteins in higher plants and green algae and special FCP proteins which are more closely associated with the PSII core in diatoms. Newer data indicates that NPQ in trimeric LHCIIs does depend on Zx but not on lutein whereas NPQ in the monomeric LHC proteins requires Zx and L and involves the formation of a radical pair (Dall’Osto et al., 2017). Recently, it has been demonstrated that PsbS-dependent NPQ occurs mainly in the LHCII whereas another quenching site operates within the PSII core complex (Nicol et al., 2019). For plants containing the Lx cycle and green algae exhibiting the shortened Vx/Ax cycle it was demonstrated that L or Ax can play a similar role in NPQ induction and enhancement as it is normally observed for Zx (Goss et al., 1998; Garcia-Plazaola et al., 2003; Leonelli et al., 2017).
While the xanthophyll cycle-dependent induction of NPQ is linked to Zx or Dtx bound to the antenna proteins of higher plants and algae, the anti-oxidative function of Zx or Dtx is related to those molecules which are localized as free pigment in the lipid phase of the membrane. For both Zx and Dtx it has been shown that these xanthophylls are able to detoxify ROS which are generated by alternative electron pathways or via the triplet excited state of Chl a under supersaturating light conditions (Havaux and Niyogi, 1999; Havaux et al., 2007; Lepetit et al., 2010). Deactivation of ROS by Zx or Dtx minimizes the damaging effects of ROS on membrane lipids, the embedded photosynthetic membrane proteins and the photosynthetic pigments. The antioxidant function of Zx may be located at the interface between LHCII and the membrane lipids because it was shown that Zx associated with oligomeric LHCII is active in the detoxification of ROS (Johnson et al., 2007).
Recently, a further function of de-epoxidized xanthophyll cycle pigments has been proposed. From former studies it was known that the conversion of Vx to Zx increases the membrane rigidity of thylakoids (Gruszecki and Strzalka, 1991, 2005). The membrane stabilizing effect of Zx was attributed to the fact that Zx spans the complete thylakoid membrane and that the polar head groups of the xanthophyll molecule are anchored within the hydrophilic part of the membrane where the lipid head groups are located (Gruszecki and Strzalka, 2005, see also Grudzinski et al., 2017). Furthermore, it was proposed that the xanthophyll molecules play a comparable role in the modulation of the physical membrane properties as cholesterol in animal or human membranes. The recent analysis of the effect of Dtx on membrane properties provided additional information on the action of the de-epoxidized xanthophylls (Bojko et al., 2019). Based on EPR measurements with the 5-SASL and 16-SASL spin probes it was shown that during the conversion of Ddx to Dtx a dynamic effect takes place whereas the high Dtx concentrations after de-epoxidation exert a stable effect on the properties of the diatom thylakoid membrane. The combined action of both effects results in a temporary increase of the rigidity of both peripheral and central parts of the membrane bilayer whereas the stable effect leads to a more permanent increase of the rigidity of the hydrophobic core of the membrane. Both effects are supposed to play a role in the short-term adaptation of diatom thylakoid membranes to changing temperatures.
First evidence for the role of lipids in the process of Vx de-epoxidation was obtained by Yamamoto et al. (1974) and Yamamoto and Higashi (1978) who isolated VDE which contained MGDG as single lipid component and VDE without attached MGDG. In in vitro enzyme assays with the isolated VDEs it became clear that MGDG is needed for the de-epoxidation of Vx to Ax and Zx and that a ratio of MGDG to Vx of about 30 is ideal for the efficient conversion. The authors concluded that one of the functions of MGDG is the solubilization of the hydrophobic pigment, thereby presenting the substrate in a form that meets the requirements of the active site of the VDE. More recent experiments with single lipids have shown that membrane lipids which form inverted hexagonal structures, i.e., MGDG and phosphatidylethanolamine (PE), have a high capacity to solubilize the xanthophyll cycle pigments Vx and Ddx (Latowski et al., 2004; Goss et al., 2005). Membrane lipids like DGDG, SQDG or PC, which form bilayers in aqueous solutions, are also able to solubilize Vx or Ddx but significantly higher concentrations are needed to achieve complete solubilization. Solubilization converts aggregates of the hydrophobic pigments Vx or Ddx into lipid dissolved single molecules which can then be de-epoxidized by the enzyme VDE. The higher capacity of the non-bilayer lipids MGDG and PE to solubilize the xanthophyll cycle pigments can also be seen in artificial membranes which are composed of a non-bilayer and bilayer lipid (Goss et al., 2007). Liposomes constructed with equal amounts of PC/PE, PC/MGDG or DGDG/MGDG show a strong enrichment of Vx or Ddx in non-bilayer lipids. The preferential localization of the xanthophyll cycle pigments in the liposome non-bilayer phase is in line with the presence of Vx or Ddx in the MGDG shield surrounding the higher plant or diatom antenna complexes (Lepetit et al., 2010; Schaller et al., 2010). Taken together this indicates that in the native membrane the xanthophyll cycle pigments are enriched in non-bilayer lipid phases. With respect to the comparison of the two main xanthophyll cycle pigments Vx and Ddx the experiments demonstrated that higher concentrations of inverted hexagonal phase forming lipids are needed for the complete solubilization of Vx compared with Ddx (Goss et al., 2005). The increased solubility of Ddx in MGDG is in line with the decreased concentration of MGDG and the increased amount of Ddx cycle pigments in the diatom thylakoid membrane compared with the thylakoids of higher plants and green algae (Lavaud et al., 2003; Gundermann and Büchel, 2008; Lepetit et al., 2010, 2012). Complete solubilization of Ddx and Dtx under these conditions can only be achieved if a low lipid per pigment ratio is sufficient for solubilization. While in the studies detailed above no or only a very slow conversion of Vx or Ddx to Zx or Dtx was observed upon the complete solubilization of the substrates in bilayer lipids, comparable experiments performed by Yamamoto (2006) yielded slightly different results. These results indicated that MGDG or DGDG support Vx de-epoxidation in different ways. While the presence of MGDG leads to a fast and complete conversion of Vx to Zx, the de-epoxidation is slow but nevertheless complete when DGDG is added to the enzyme assay. Based on the results it was concluded that the solubilization of aggregated Vx by DGDG proceeds during the time course of the de-epoxidation reaction thereby circumventing the negative effects of the decreased solubilization capacity of the bilayer forming lipid.
The first results on the role of inverted hexagonal phases for xanthophyll de-epoxidation were reported by Latowski et al. (2000, 2002, 2004). The authors used unilamellar liposomes composed of PC, which were supplemented with different concentrations of the non-bilayer lipid MGDG, to study the conversion of Vx to Ax and Zx. In the liposome systems an increase in the de-epoxidation efficiency was observed with increasing ratios of MGDG to PC. In addition, through the use of 31P-NMR spectroscopy, the presence of inverted hexagonal phases formed by MGDG was detected. Based on the results it was concluded that Vx diffuses into the inverted hexagonal phase where the actual conversion to Ax and Zx by the enzyme VDE is taking place. The importance of inverted hexagonal phases for Vx de-epoxidation was demonstrated in a subsequent study where different non-bilayer and bilayer lipids where tested for their ability to enhance the de-epoxidation reaction (Latowski et al., 2004). The non-bilayer lipids MGDG and PE induced a fast and efficient conversion of Vx whereas in the presence of the bilayer lipids DGDG and PC no or only a very slow Vx de-epoxidation could be observed. The use of either GGLs or GPs demonstrated that not the nature of the lipid but the three-dimensional structures formed by the lipids are responsible for the stimulation of Vx de-epoxidation. Later it could be shown that the bilayer lipids DGDG or PC are able to completely solubilize either Vx or Ddx (Goss et al., 2005). However, no or only a very slow conversion of Vx to Ax and Zx or Ddx to Dtx was observed in the single bilayer lipid systems. In liposome systems composed of only bilayer lipids, i.e., DGDG or PC, a complete solubilization of Vx or Ddx could be achieved as well, but Vx or Ddx de-epoxidation could only be detected when the liposomes where supplemented with a certain concentration of the inverted hexagonal phase forming lipids MGDG or PE (Goss et al., 2007). Later it was demonstrated that the main lipid of diatom thylakoid membranes, the negatively charged SQDG has a pronounced inhibitory effect on Ddx de-epoxidation (Goss et al., 2009). A comparable inhibition of the DDE could be demonstrated for the anionic GP PG. The results from the solubilization and de-epoxidation experiments in single lipid systems and liposomes composed of different lipids implied that the solubilization of the lipids represents an important factor for the de-epoxidation of Vx or Ddx but that, despite efficient solubilization, the three-dimensional structures of the lipids are mandatory for an efficient conversion of the xanthophyll cycle pigments. Based on these results it was suggested that the inverted hexagonal structure and not the bilayer structure enables the access of the enzymes VDE or DDE to their respective solubilized substrates. The penetration of the VDE or DDE into the hydrophobic interior of the lipid phase has to be deep enough to allow the interaction of the catalytic site of the enzymes with the substrates Vx or Ddx. Penetration of the β-barrel structure, which forms the catalytic site of the lipocalins to which both VDE and DDE belong (Hieber et al., 2000), may be facilitated by a decreased surface tension of the inverted hexagonal phase compared with the bilayer phase (van den Brink-van der Laan et al., 2004). The decreased surface tension is most probably caused by the conical shape of the non-bilayer lipids like MGDG molecules which are characterized by a small headgroup and long unsaturated FAs which cover a significantly larger area than the mono-galactose headgroup (Garab et al., 2000, see section Three Dimensional Structures of Lipids). Bilayer lipids like DGDG, on the other hand, have a cylindrical shape because the larger headgroups and FA chains cover a comparable area. Lipid phases composed of bilayer lipids are thus rather tightly sealed, show a high surface tension and do not enable the penetration of VDE or DDE into the hydrophobic membrane interior. Yamamoto (2006) proposed a different concept of Vx de-epoxidation in the thylakoid membrane. Based on the observation that Vx could be completely converted to Zx in the presence of the bilayer lipid DGDG, albeit with a significantly lower Vx de-epoxidation rate compared to MGDG, it was proposed that Vx de-epoxidation is not restricted to MGDG reverse micelles and that the VDE is able to operate throughout the lipid phase of the single bilayer thylakoid membrane.
The existence of non-bilayer lipid phases in thylakoid membranes has been studied by freeze-fracture electron microscopy. It was demonstrated that exposure of higher plant thylakoid membranes to increasing temperatures in the range from 35 to 45°C leads to a destacking of grana membranes (Gounaris et al., 1984). Further increases of the temperature up to 45–55°C induces a pronounced lipid phase separation and the formation of large areas of inverted hexagonal lipid phases. Lipid phase separation and irreversible induction of inverted hexagonal phases can also be triggered by exposure of thylakoid membranes to pH-values lower than pH 4.5 or treatment with phospholipase A2 (Thomas et al., 1985). In addition, incubation of isolated thylakoids in reaction buffers complemented with high concentrations of compatible solutes, such as sucrose, trehalose, sorbitol or betaine, induces the phase separation of non-bilayer forming lipids, followed by the establishment of large areas of inverted hexagonal lipid phases (Williams et al., 1992). Later, first measurements of isolated wheat thylakoid membranes with 31P-NMR spectroscopy supported the formation of inverted hexagonal lipid phases when the thylakoids were exposed to high temperatures between 55 and 60°C (Haranczyk et al., 1995). Krumova et al. (2008) used PG as an intrinsic bulk label lipid for 31P-NMR studies to analyze the lipid phases of the thylakoid membrane. The data showed that besides the lamellar phase a non-bilayer isotropic phase exists which becomes predominant at higher temperatures. In addition, it was demonstrated that the phospholipid was not restricted to the lamellar phase of the membrane. Using molecular dynamics simulations for the characterization of the thylakoid membranes of higher plants and cyanobacteria differences between these membranes could be detected (van Eerden et al., 2015). The simulations revealed that the thylakoid membrane is in a state close to the formation of the inverted hexagonal phase. Furthermore, the molecular dynamic simulations showed that the higher plant thylakoid membrane more readily undergoes the transition to the inverted hexagonal phase compared with the cyanobacterial membrane which is most likely caused by the higher degree of unsaturation of the FA moieties of the plant lipids. It is interesting to note that the simulations of van Eerden et al. (2015) did not present evidence for an enrichment of MGDG molecules in the inverted hexagonal phase. Instead, a well-mixed system of lipids could be observed in both the lamellar and the inverted hexagonal phase. Recent studies employing 31P-NMR measurements and time-resolved fluorescence spectroscopy of the fluorescent, lipophilic dye MC540 revealed the presence of not only one but three inverted hexagonal phases which coexist with one bilayer phase in the thylakoid membrane of higher plants (Garab et al., 2017). According to the data the three inverted hexagonal phases are located at the luminal and stromal sides of the thykakoid membrane, accompanied by a lipid phases in the junction region of the grana and stroma membranes. Garab et al. (2017) showed that the non-bilayer lipid phases were sensitive to the osmolarity and the ionic strength of the medium which was later confirmed by the results of Kotakis et al. (2018) who used high concentrations of the compatible solute sucrose to induce the formation of the inverted hexagonal phases. Besides the presence of osmolytes low pH-values led to a pronounced increase of the non-bilayer thylakoid lipid phases which could additionally be modulated by the degree of unsaturation of the lipid FAs.
It has been proposed that the non-bilayer lipid phases involved in xanthophyll cycling are localized in the vicinity of the LHCII in higher plants and green algae or the FCP complexes in diatoms. This proposal is based on the purification of LHCII and FCP complexes which, under mild solubilization conditions, leads to the isolation of LHCII and FCP complexes with a shield of MGDG molecules incorporating the Vx or Ddx cycle pigments (see section Localization of Xanthophyll Cycle Pigments in the Thylakoid Membrane and references therein). In addition, a fast and efficient rebinding of the de-epoxidized xanthophyll cycle pigments Zx or Dtx to the light-harvesting complexes has to take place in order to establish rapid xanthophyll-dependent photoprotection via NPQ (section Function of Xanthophyll Cycles and references). With respect to the binding of the pigment molecules to the LHC apoproteins it is interesting to note that the de-epoxidized Ddx cycle pigment Dtx shows a decreased solubility in MGDG compared with the epoxidized Ddx which possibly accelerates the rebinding of Dtx to the FCP complex (Goss et al., 2007). Since in higher plants and green algae the majority of the Vx cycle pigments are bound to the LHCII (section Localization of Xanthophyll Cycle Pigments in the Thylakoid Membrane and references) it is reasonable to believe that most of the non-bilayer lipid phases are located in the vicinity of LHCII and thus in the grana regions of the thylakoid membrane. On the other hand, Vx de-epoxidation is not restricted to the LHCII but also occurs in the LHCI (Lee and Thornber, 1995; Arvidsson et al., 1997; Zhu et al., 1997) which would require the presence of inverted hexagonal phases in the stroma thylakoids in the vicinity of LHCI. However, based on the observation that Vx de-epoxidation in the LHCI is less efficient than that in LHCII (Wehner et al., 2004) the non-bilayer lipid phases in the stroma thylakoid membranes may be less well-defined as the non-bilayer lipid phases in the grana membranes. The idea of a tight association of the non-bilayer lipid phase and the light-harvesting complexes is supported by the analysis of the molecular structure of the LHCII at 3.2 Å resolution which revealed the presence of a putative binding site for the enzyme VDE (Liu et al., 2004). It is thus possible that the local non-bilayer lipid phase enables the direct interaction between the VDE and the LHCII. A close association between the LHCII and the VDE is furthermore corroborated by the recent direct isolation of a functional Vx cycle membrane domain from thylakoid membranes of higher plants (Goss et al., 2017). With the use of the very mild detergent α-dodecylmaltoside and a preparation at pH5, which ensured the binding of VDE to the luminal side of the thylakoid membrane, the authors were able to isolate a membrane domain consisting of LHCII, VDE and MGDG. The domain was in functional state as evidenced by the de-epoxidation of Vx to Ax and Zx by the domain-associated VDE. However, the studies of Arvidsson et al. (1997) and Macko et al. (2002) are in favor of a greater distance between the LHCII and the lipid phase of the membrane where the actual Vx de-epoxidation is taking place. In both studies the activity of isolated, exogenous VDE was analyzed. VDE was added to the stromal side of thylakoid membranes where the activity of the endogenous VDE at the luminal site of the membrane was inhibited by DTT (Arvidsson et al., 1997) or to the stromal side of thylakoids isolated from Arabidopsis thaliana mutants that did not contain VDE (Macko et al., 2002). Both studies show that the de-epoxidation efficiency of VDE located at the stromal side of the membrane is comparable to the activity of native VDE at the luminal side of the thylakoid membrane. Since in these experiments the VDE located at the stromal membrane site had no accession to the grana stacks it was concluded that the lipid phase where de-epoxidation is taking place is located at a certain distance to the central part of the grana membranes and located at the surface of the grana stacks. This notion was supported by the lower VDE activity at reduced temperatures which the authors interpreted in such a way that Vx cycle pigments, which have detached from the LHCII, have to diffuse a certain distance in the membrane until they reach the lipid phase for de-epoxidation.
With respect to the possible localization of the non-bilayer lipid phase in diatom thylakoids it has to be taken into account that the diatom thylakoid membranes show a different architecture compared with the thylakoids of higher plants. The diatom thylakoids are not separated into grana and stroma thylakoids but are characterized by regular stacks of three membranes (Gibbs, 1962, 1970). Based on the observations that MGDG forms a lipid shield around the FCP complexes which incorporates the lipid-dissolved Ddx cycle pigments (Lepetit et al., 2010) and that the negatively charged lipid SQDG, which is present in high concentration in the diatom membranes, inhibits the de-epoxidation of Ddx (Goss et al., 2009) a model for the lipid and protein arrangement in the diatom thylakoid membranes was established (Lepetit et al., 2012). This model predicts that PSII with its tightly associated FCP complexes and its peripheral antenna complexes is located in the inner membranes of the stacks of three membranes. In addition, the inner core membranes are enriched in the PSII-associated MGDG. The outer membranes of the regular stacks contain the PSI complexes with their PSI-specific FCP complexes and the ATP synthase. According to the model, the lipid composition of the outer membranes is dominated by the anionic SQDG. Recently, the model of Lepetit et al. (2012) was supported by new data which show that the thylakoid membranes of the pennate diatom P. tricornutum contain large areas which are exclusively occupied by PSI supercomplexes, consisting of PSI core complexes with their specific antenna complexes (Bina et al., 2016). Flori et al. (2017) also obtained evidence for a compartmentalization of the two photosystems. Like the model of Lepetit et al. (2012) they propose that PSII is located in the core membranes whereas PSI is enriched in the peripheral membranes which are exposed to the chloroplast stroma. Since the FCP complexes associated with PSII are surrounded by an MGDG phase, MGDG is most probably also enriched in the inner membranes. The enrichment of MGDG in the core membranes may lead to the formation of non-bilayer structures which then represent attraction sites for the diatom DDE and support the efficient conversion of Ddx to Dtx. The confinement of SQDG to the outer, peripheral membranes is most likely needed in order to prevent the interaction with the DDE in the non-bilayer phases in the core membranes which would result in a pronounced inhibition of Ddx de-epoxidation.
The high concentration of MGDG in thylakoid membranes of higher plants and green algae poses the risk of the formation of inverted hexagonal phases and the segregation of MGDG out of the membrane bilayer in an aqueous environment. In the native thylakoids the formation of extensive non-bilayer lipid phases is restricted by the high protein content of the membranes and extensive lipid phase separation can only be observed when thylakoids are exposed to various abiotic stresses. For the thylakoids of higher plants it has been demonstrated that the interaction of the main membrane protein LHCII and MGDG plays a crucial role in establishing and maintaining the lipid bilayer structure (Simidjiev et al., 1998, 2000). In vitro studies with isolated LHCII and MGDG showed that in the presence of MGDG large, ordered lamellar structures of protein and lipid are formed. In addition, the presence of LHCII disturbs the inverted hexagonal phase of MGDG and forces the MGDG molecules into a bilayer structure. Based on these results it was proposed that in thylakoid membranes the spatial limitation caused by the high concentration of membrane proteins inhibits the formation of non-bilayer lipid phases. Additional studies demonstrated that MGDG stabilizes the oligomeric states of LHCII (Schaller et al., 2011), FCP complexes and the LHC of the Prasinophyceae M. squamata (Schaller-Laudel et al., 2017). Furthermore, MGDG increases the mechanical stability of the LHCII (Seiwert et al., 2017), thereby preventing the unfolding of trimeric LHCII. Stabilization of LHCII by MGDG was attributed to a steric matching of the conically shaped MGDG and the hourglass shape of trimeric LHCII (Seiwert et al., 2017). Several studies have shown that LHCII in its lipid environment is structurally flexible and can undergo molecular rearrangements (see section Function of Xanthophyll Cycles and references therein). During high light illumination of plants or algae, which triggers the process of NPQ, aggregation of LHCII is taking place. With respect to the formation of non-bilayer lipid phases in the thylakoid membrane the aggregation of LHCII or FCP complexes is thought to play an important role. Protein aggregation, in general, leads to a segregation of lipid molecules which are otherwise associated with the non-aggregated protein complexes. It is thought that in the case of the LHCII and FCP complexes aggregation leads to the liberation of a significant part of the MGDG molecules associated with the light-harvesting proteins followed by a separation from the lipid bilayer phase of the thylakoid membrane and finally the formation of inverted hexagonal phases. Although it is still a matter of debate if the non-bilayer phases are formed within the lipid bilayer (Jahns et al., 2009) or are associated with the outside of the membrane (Garab et al., 2016 see Figure 3), recent results have implicated that non-bilayer lipid phases are located at the luminal and stromal sides of the thylakoid membrane (Garab et al., 2017).
Figure 3. Model for the violaxanthin cycle domain in thylakoid membranes of higher plants. High light illumination leads to a disconnection of the main light-harvesting complex of photosystem II, LHCII from the photosystem II core complex followed by protein aggregation. MGDG molecules, which during low light illumination surround the LHCII, dissociate, segregate and form a non-bilayer lipid phase. This non-bilayer lipid phase may be located outside of the membrane bilayer, i.e., within the thylakoid lumen (A), or within the plane of the thylakoid membrane (B). During high light illumination violaxanthin disconnects from its binding site at the LHCII apoproteins and diffuses into the non-bilayer lipid phase. The non-bilayer lipid phase represents an attraction site for the enzyme violaxanthin de-epoxidase (VDE), which, after its pH-dependent activation and dimerization, binds to the non-bilayer lipid phase. The non-bilayer lipid phase is characterized by a reduced surface tension and thus allows the penetration of the enzyme’s hydrophobic catalytic site into the hydrophobic core of the non-bilayer lipid phase where it gains access to the hydrophobic pigment violaxanthin. After the conversion of violaxanthin to zeaxanthin, zeaxanthin rebinds to the LHCII and participates in photoprotection via NPQ. Since the violaxanthin cycle domain, consisting of LHCII, MGDG, VDE and xanthophyll cycle pigments, is only established during high light illumination of plants, the domain can be described as a transient membrane domain.
More than 40 years ago the fluid mosaic model of biomembranes was introduced by Singer and Nicholson (1972). The first main feature of this model is the amphiphilic nature of the membrane lipids which means that the lipids contain a hydrophilic head group and a hydrophobic area covered by the FA moieties (see section Three Dimensional Structures of Lipids). The amphiphilic character of the lipids leads to the formation of a lipid bilayer in aqueous surroundings. A second important feature concerns the proteins which can be associated with the hydrophilic head groups of the lipids as peripheral proteins or with the hydrophobic core of the membrane as trans-membrane proteins. A third important aspect lies in the fluidity of the membrane which means that both lipids and proteins are in constant motion. Protein and lipid motion can occur in lateral direction, i.e., in the plane of the membrane. In addition, rotation of proteins and lipids along their long axis is possible. Since the first presentation of the fluid mosaic model a wealth of information about the structure of biomembranes has been gathered (for recent reviews see Bagotelli and Mouritsen, 2013; Goni, 2014; Nicholson, 2014). Although these data do not question the general assumptions of the fluid mosaic model they have refined the model in several important ways. The first important aspect concerns the high concentration of membrane proteins. While in the original model only few proteins span the membrane bilayer it is known today that the membrane is densely packed with membrane and peripheral proteins (for a review see Engelmann, 2005). Especially in membranes like the thylakoid membrane or the inner mitochondrial membrane the protein density is so high that bilayer phases composed solely of lipids are hardly observed (for a recent review on the thylakoid architecture see Kirchhoff, 2018). A second new finding, which is also critical for the understanding of the xanthophyll cycle function, is the observation that proteins exist which only transiently bind to biomembranes (reviewed by Goni, 2002). This class of proteins can exist in water-soluble form in an aqueous environment but can also bind to the membrane after certain requirements have been met. The transient proteins supplement the proteins which are permanently associated with the membrane and the water-soluble proteins which never come into contact with the lipid bilayer. A further important extension of the original fluid mosaic model, which is also of fundamental importance for the operation of the xanthophyll cycle, is the finding that non-bilayer phases can co-exist with the lipid bilayer (for the presence of inverted hexagonal phases in thylakoid membranes see section Localization of Non-bilayer Lipid Phases in the Thylakoid Membrane and references therein). Another feature which separates the new membrane models from the original fluid mosaic model concerns the observation that biomembranes are characterized by a pronounced lateral heterogeneity. It has become clear that the membrane bilayer consists of heterogeneous domains (for reviews on domains and lipid rafts in plant membranes see Mongrand et al., 2010; Simon-Plas et al., 2011). These membrane domains contain a specific lipid and protein composition and perform a special function. Other important aspects of the new generation of membrane models describe the membrane not as a flat surface but as a curved structure. In this regard the observation of a hydrophobic mismatch is interesting (for a review see Killian, 1998). Hydrophobic mismatch describes the situation when the hydrophobic thickness of the transmembrane region of a membrane protein does not match the hydrophobic thickness of the membrane in which it is localized. Here it has been shown that both membrane proteins, by re-arrangements of the protein structure, and membrane lipids, by stretching or tilting of the FA chains, can adapt to this situation. According to the results outlined in the preceding chapters (see references therein) the part of the thylakoid membrane where the Vx cycle, and most likely the Ddx cycle, too, takes place can be described as a special membrane domain (Figure 3). The Vx cycle domain contains a specific protein composition, namely the LHCII, a membrane protein which is permanently associated with the membrane, and the VDE, a water-soluble protein which, after its pH-dependent activation, transiently binds to the membrane. The Vx cycle domain is characterized by a specific lipid composition because it is enriched in the non-bilayer lipid MGDG which likely forms an inverted hexagonal structure within the domain. The enrichment of MGDG is driven by the structural re-arrangement, i.e., the aggregation, of the LHCII which leads to a local enrichment of free MGDG molecules. The Vx cycle domain carries out a special function, i.e., the conversion of the di-epoxy xanthophyll Vx to Ax and Zx. The Vx cycle domain can be characterized as a transient domain which is only established during high light illumination when Vx de-epoxidation is needed for photoprotection via NPQ. A transition from high light illumination back to low light conditions or darkness reverses the domain formation by a detachment of the VDE from the membrane and a disaggregation of LHCII. Disaggregation of the LHCII re-enables the tight interaction of the membrane protein with MGDG, thereby re-enforcing the membrane bilayer structure.
Although recent measurements have begun to shed light on the existence and localization of inverted hexagonal phases in the thylakoid membrane of higher plants (Garab et al., 2017), and the isolation of a putative, functional Vx cycle membrane domain has been reported (Goss et al., 2017), there is still a wealth of open questions with respect to the lipid dependence of xanthophyll cycling. Future measurements have to show where the inverted hexagonal phase responsible for Vx de-epoxidation is located, i.e., whether it is located within the plane of the thylakoid membrane or whether it is attached to the luminal side of the membrane. With respect to the localization of the non-bilayer lipid phase it has to be clarified if the lipid phase is located in the direct vicinity of the LHCII or at a greater distance, possibly in the grana margins. A greater distance between the xanthophyll cycle pigment binding proteins and the lipid phase, where the actual enzymatic reaction is taking place, would require significantly longer diffusion times of Vx and Zx. Future measurements have to provide a way to determine the size of the inverted hexagonal phases to answer the question if fewer but larger non-bilayer lipid phases exist or if the xanthophyll cycle pigments are converted in a larger number of smaller sized inverted hexagonal phases. In this respect it has to be demonstrated if the VDE, which seems to be present in only very low concentrations in the thylakoid lumen (Arvidsson et al., 1996), stays attached to a larger non-bilayer phase or if it detaches and rebinds to a higher number of smaller phases. With regard to the conversion of Vx to Ax and Zx in the LHCI (Croce et al., 2002, 2007) it has to be elucidated if non-bilayer phases or even xanthophyll cycle domains exist in the vicinity of PSI and thus the stroma membranes. According to our knowledge it is also unclear if the back reaction of the xanthophyll cycle, which is carried out by the peripheral membrane protein ZEP (Schaller et al., 2012b), requires the presence of special membrane lipid structures, like e.g., the inverted hexagonal phase, and where this hypothetical phase or domain is located. Finally, scientists working with algae may concentrate on the question if similar xanthophyll cycle domains exist in the different algal classes and, taking into account the different thylakoid structures (Lepetit et al., 2012; Flori et al., 2017), where these domains are localized.
RG and DL wrote and corrected the text and constructed the figures.
The authors declare that the research was conducted in the absence of any commercial or financial relationships that could be construed as a potential conflict of interest.
We thank our collaborators, co-workers, and students who have contributed with their work to our research in the field of lipids and xanthophyll cycles.
Andersson, M. X., Larsson, K. E., Tjellström, H., Liljenberg, C., and Sandelius, A. S. (2005). Phosphate limited oat. The plasma membrane and the tonoplast as major targets for phospholipid-to-glycolipid replacement and stimulation of phospholipases in the plasma membrane. J. Biol. Chem. 280, 27578–27586. doi: 10.1074/jbc.M503273200
Andersson, M. X., Stridh, M. H., and Larsson, K. E. (2003). Phosphate deficient oat replaces a major portion of the plasma membrane phospholipids with the galactolipid digalactosyldiacylglycerol. FEBS Lett. 537, 128–132. doi: 10.1016/S0014-5793(03)00109-1
Arnoux, P., Morosinotto, T., Saga, G., Bassi, R., and Pignol, D. (2009). A structural basis for the pH-dependent xanthophyll cycle in Arabidopsis thaliana. Plant Cell 21, 2036–2044. doi: 10.1105/tpc.109.068007
Aronsson, H., Schüttler, M. A., Kelly, A. A., Sundqvist, C., Dörmann, P., Karim, S., et al. (2008). Monogalactosyldiacylglycerol deficiency in Arabidopsis thaliana affects pigment composition in the prolamellar body and impairs thylakoid membrane energization and photoprotection in leaves. Plant Physiol. 148, 580–592. doi: 10.1104/pp.108.123372
Arvidsson, P.-O., Bratt, C. E., Carlsson, M., and Akerlund, H.-E. (1996). Purification and identification of the violaxanthin deepoxidase as a 43 kDa protein. Photosynth. Res. 49, 119–129. doi: 10.1007/bf00117662
Arvidsson, P.-O., Carlsson, M., Stefansson, H., Albertsson, P. A., and Akerlund, H.-E. (1997). Violaxanthin accessibility and temperature dependency for de-epoxidation in spinach thylakoid membranes. Photosynth. Res. 52, 39–48.
Bagotelli, L. A., and Mouritsen, O. G. (2013). Is the fluid mosaic (and the accompanying raft hypothesis) a suitable model to describe fundamental features of biological membranes? What may be missing? Front. Plant Sci. 4:457. doi: 10.3389/fpls.2013.00457
Bailleul, B., Rogato, A., de Martino, A., Coesel, S., Cardol, P., Bowler, C., et al. (2010). An atypical member of the light-harvesting complex stress-related protein family modulates diatom responses to light. Proc. Natl. Acad. Sci. U.S.A. 107, 18214–18219. doi: 10.1073/pnas.1007703107
Ballottari, M., Truong, T. B., De Re, E., Erickson, E., Stella, G. R., Fleming, G. R., et al. (2016). Identification of pH-sensing sites in the light-harvesting complex stress-related 3 protein essential for triggering non-photochemical quenching in Chlamydomonas reinhardtii. J. Biol. Chem. 291, 7334–7346. doi: 10.1074/jbc.m115.704601
Bassi, R., Pineau, B., Dainese, P., and Marquardt, J. (1993). Carotenoid-binding proteins of photosystem II. Eur. J. Biochem. 212, 297–303. doi: 10.1111/j.1432-1033.1993.tb17662.x
Ben Hamed, K., Ben Youssef, N., Ranieri, A., Zarrouk, M., and Abdelly, C. (2005). Changes in content and fatty acid profiles of total lipids and sulfolipids in the halophyte Crithmum maritimum under salt stress. J. Plant Physiol. 162, 599–602. doi: 10.1016/j.jplph.2004.11.010
Bertrand, M., and Schoefs, B. (1999). “Photosynthetic pigment metabolism in plants during stress,” in Handbook of Plant And Crop Stress, 2nd Edn, ed. M. Pessarakli (New York, NY: Marcel Dekker), 527–541.
Bina, D., Herbstova, M., Gardian, Z., Vacha, F., and Litvin, R. (2016). Novel structural aspect of the diatom thylakoid membrane: lateral segregation of photosystem I under red-enhanced illumination. Sci. Rep. 6:25583.
Bojko, M., Olchawa-Pajor, M., Goss, R., Schaller-Laudel, S., Strzalka, K., and Latowski, D. (2019). Diadinoxanthin de-epoxidation as important factor in the short-term stabilization of diatom photosynthetic membranes exposed to different temperatures. Plant Cell Environ. 42, 1270–1286. doi: 10.1111/pce.13469
Bonente, G., Ballotari, M., Truong, T. B., Morosinotto, T., Ahn, T. K., Fleming, G. R., et al. (2011). Analysis of LhcSR3, a protein essential for feedback de-excitation in the green alga Chlamydomonas reinhardtii. PLoS Biol. 9:e1000577. doi: 10.1371/journal.pbio.1000577
Boudiere, L., Michaud, M., Petroutsos, D., Rébeillé, F., Falconet, D., Bastien, O., et al. (2014). Glycerolipids in photosynthesis: composition, synthesis and trafficking. Biochim. Biophys. Acta 1837, 470–480. doi: 10.1016/j.bbabio.2013.09.007
Buck, J. M., Sherman, J., Bartulos, C. R., Serif, M., Halder, M., Henkel, J., et al. (2019). Lhcx proteins provide photoprotection via thermal dissipation of absorbed light in the diatom Phaeodactylum tricornutum. Nat. Commun. 10:4167.
Bungard, R. A., Ruban, A. V., Hibberd, J. M., Press, M. C., Horton, P., and Scholes, J. D. (1999). Unusual carotenoid composition and a new type of xanthophyll cycle in plants. Proc. Natl. Acad. Sci. U.S.A. 96, 1135–1139. doi: 10.1073/pnas.96.3.1135
Cardol, P., Bailleul, B., Rappaport, F., Derelle, E., Béal, D., Breyton, C., et al. (2008). An original adaptation of photosynthesis in the marine green alga Ostreococcus. Proc. Natl. Acad. Sci. U.S.A. 105, 7881–7886. doi: 10.1073/pnas.0802762105
Chen, J., Burke, J. J., Xin, Z., Xu, C., and Velten, J. (2006). Characterization of the Arabidopsis thermosensitive mutant atts02 reveals an important role for galactolipids in thermotolerance. Plant Cell Environm. 29, 1437–1448. doi: 10.1111/j.1365-3040.2006.01527.x
Coesel, S., Obornik, M., Varela, J., Falciatore, A., and Bowler, C. (2008). Evolutionary origins and functions of the carotenoid biosynthetic pathway in marine diatoms. PLoS One 3:e2896. doi: 10.1371/journal.pone.0002896
Croce, R., Morosinotto, T., Castelletti, S., Breton, J., and Bassi, R. (2002). The Lhca antenna complexes of higher plants photosystem I. Biochim. Biophys. Acta 1556, 29–40. doi: 10.1016/s0005-2728(02)00304-3
Croce, R., Mozzo, M., Morosinotto, T., Romeo, A., Hienerwadel, R., and Bassi, R. (2007). Singlet and triplet state transitions of carotenoids in the antenna complexes of higher-plant Photosystem I. Biochemistry 46, 3846–3855. doi: 10.1021/bi602531k
Dall’Osto, L., Cazzaniga, S., Bressan, M., Palecek, D., Zidek, K., Niyogi, K. K., et al. (2017). Two mechanisms for dissipation of excess light in monomeric and trimeric light-harvesting complexes. Nat. Plants 3:17033.
Dautermann, O., and Lohr, M. (2017). A functional zeaxanthin epoxidase from red algae shedding light on the evolution of light-harvesting carotenoids and the xanthophyll cycle in photosynthetic eukaryotes. Plant J. 92, 879–892.
Demmig, B., Winter, K., Krüger, A., and Czygan, F.-C. (1987). Photoinhibition and zeaxanthin formation in intact leaves. A possible role for the xanthophyll cycle in the dissipation of excess light. Plant Physiol. 84, 218–224. doi: 10.1104/pp.84.2.218
Demmig, B., Winter, K., Krüger, A., and Czygan, F.-C. (1988). Zeaxanthin and the heat dissipation of excess light energy in Nerium oleander exposed to a combination of high light and water stress. Plant Physiol. 87, 17–24. doi: 10.1104/pp.87.1.17
Demmig-Adams, B., and Adams, W. W. III (2006). Photoprotection in an ecological context: the remarkable complexity of thermal energy dissipation. New Phytol. 172, 11–21. doi: 10.1111/j.1469-8137.2006.01835.x
Demmig-Adams, B., Koh, S.-C., Cohu, C. M., Muller, O., Stewart, J. J., and Adams, W. W. III (2014). “Non-photochemical fluorescence quenching in contrasting plant species and environments,” in Non-Photochemical Quenching And Energy Dissipation In Plants, Algae And Cyanobacteria, eds B. Demmig-Adams, G. Garab, W. W. Adams, and M. Govindjee (Dordrecht: Springer), 531–552. doi: 10.1007/978-94-017-9032-1_24
Dodson, V. J., Dahmen, J. L., Mouget, J.-L., and Leblond, J. D. (2013). Mono- and digalactosyldiacylglycerol composition of the marennine-producing diatom, Haslea ostrearia: comparison to a selection of pennate and centric diatoms. Phycol. Res. 61, 199–207. doi: 10.1111/pre.12015
Dodson, V. J., Mouget, J.-L., Dahmen, J. L., and Leblond, J. D. (2014). The long and short of it: temperature-dependent modifications of fatty acid chain length and unsaturation in the galactolipid profiles of the diatoms Haslea ostrearia and Phaeodactylum tricornutum. Hydrobiologia 727, 95–107. doi: 10.1007/s10750-013-1790-4
Engelmann, D. M. (2005). Membranes are more mosaic than fluid. Nature 438, 578–580. doi: 10.1038/nature04394
Esteban, R., Becerril, J. M., and Garcia-Plazaola, J. I. (2009). Lutein epoxide cycle-more than a just a forest tale. Plant Signal. Behav. 4, 342–344. doi: 10.4161/psb.4.4.8197
Esteban, R., and Garcia-Plazaola, J. I. (2014). “Involvement of a second xanthophyll cycle in non-photochemical quenching of chlorophyll fluorescence: the lutein epoxide story,” in Non-Photochemical Quenching And Energy Dissipation In Plants, Algae And Cyanobacteria, eds B. Demmig-Adams, G. Garab, and W. W. Adams (Dordrecht: Springer), 277–295. doi: 10.1007/978-94-017-9032-1_12
Flori, S., Jouneau, P., Bailleul, B., Gallet, B., Estrozi, L. F., Moriscot, C., et al. (2017). Plastid thylakoid architecture optimizes photosynthesis in diatoms. Nat. Commun. 18:15885.
Frentzen, M., Heinz, E., McKeon, T. A., and Stumpf, P. K. (1983). Specificities and selectivities of glycerol-3- phosphate acyltransferase and monoacylglycerol-3-phosphate acyltransferase from pea and spinach chloroplasts. Eur. J. Biochem. 129, 629–636. doi: 10.1111/j.1432-1033.1983.tb07096.x
Frommolt, R., Goss, R., and Wilhelm, C. (2001). The de-epoxidase and epoxidase reactions of Mantoniella squamata (Prasinophyceae) exhibit different substrate-specific reaction kinetics compared to spinach. Planta 213, 446–456. doi: 10.1007/s004250100589
Fufezan, C., Simionato, D., and Morosinotto, T. (2012). Identification of key residues for pH dependent activation of violaxanthin de-epoxidase from Arabidopsis thaliana. PLoS One 7:e35669. doi: 10.1371/journal.pone.0035669
Garab, G., Lohner, K., Laggner, P., and Farkas, T. (2000). Self-regulation of the lipid content of membranes by non-bilayer lipids: a hypothesis. Trends Plant Sci. 5, 489–494. doi: 10.1016/s1360-1385(00)01767-2
Garab, G., Ughy, B., de Waard, P., Akhtar, P., Javornik, U., Kotakis, C., et al. (2017). Lipid polymorphism in chloroplast thylakoid membranes - as revealed by 31P-NMR and time resolved merocyanine fluorescence spectroscopy. Sci. Rep. 7:13343.
Garab, G., Ughy, B., and Goss, R. (2016). “Role of MGDG and non-bilayer lipid phases in the structure and dynamics of chloroplast thylakoid membranes,” in Lipids in Plant and Algae Development, eds Y. Nakamura and Y. Li-Beisson (Dordrecht: Springer), 127–157.
Garcia-Mendoza, E., and Colombo-Pallotta, M. F. (2007). The giant kelp Macrocystis pyrifera presents a different nonphotochemical quenching control than higher plants. New Phytol. 173, 526–536. doi: 10.1111/j.1469-8137.2006.01951.x
Garcia-Plazaola, J. I., Hernandez, A., Olano, J. M., and Becerril, J. M. (2003). The operation of the lutein epoxide cycle correlates with energy dissipation. Funct. Plant Biol. 30, 319–324.
Gerotto, C., and Morosinotto, T. (2013). Evolution of photoprotection mechanisms upon land colonization: evidence of PSBS-dependent NPQ in late Streptophyte algae. Physiol. Plant. 149, 583–598. doi: 10.1111/ppl.12070
Gibbs, S. P. (1962). The ultrastructure of the chloroplasts of algae. J. Ultrastruct. Res. 7, 418–435. doi: 10.1016/s0022-5320(62)90038-2
Gibbs, S. P. (1970). The comparative ultrastructure of the algal chloroplast. Ann. N. Y. Acad. Sci. 175, 454–473. doi: 10.1111/j.1749-6632.1970.tb45167.x
Goni, F. M. (2002). Non-permanent proteins in membranes: when proteins come as visitors. Mol. Membr. Biol. 19, 237–245. doi: 10.1080/0968768021000035078
Goni, F. M. (2014). The basic structure and dynamics of cell membranes: an update of the singer-nicholson model. Biochim. Biophys. Acta 1838, 1467–1476. doi: 10.1016/j.bbamem.2014.01.006
Goss, R. (2003). Substrate specificity of the violaxanthin de-epoxidase of the primitive green alga Mantoniella squamata (Prasinophyceae). Planta 217, 801–812. doi: 10.1007/s00425-003-1044-1
Goss, R., Böhme, K., and Wilhelm, C. (1998). The xanthophyll cycle of Mantoniella squamata converts violaxanthin into antheraxanthin but not to zeaxanthin: consequences for the mechanism of enhanced non-photochemical energy dissipation. Planta 205, 613–621. doi: 10.1007/s004250050364
Goss, R., Greifenhagen, A., Bergner, J., Volke, D., Hoffmann, R., Wilhelm, C., et al. (2017). Direct isolation of a functional violaxanthin cycle domain from thylakoid membranes of higher plants. Planta 245, 793–806. doi: 10.1007/s00425-016-2645-9
Goss, R., and Jakob, T. (2010). Regulation and function of xanthophyll cycle-dependent photoprotection in algae. Photosynth. Res. 106, 103–122. doi: 10.1007/s11120-010-9536-x
Goss, R., Latowski, D., Grzyb, J., Vieler, A., Lohr, M., Wilhelm, C., et al. (2007). Lipid dependence of diadinoxanthin solubilization and de-epoxidation in artificial membrane systems resembling the lipid composition of the natural thylakoid membrane. Biochim. Biophys. Acta 1768, 67–75. doi: 10.1016/j.bbamem.2006.06.006
Goss, R., and Lepetit, B. (2015). Biodiversity of NPQ. J. Plant Physiol. 172, 13–32. doi: 10.1016/j.jplph.2014.03.004
Goss, R., Lohr, M., Latowski, D., Grzyb, J., Vieler, A., Wilhelm, C., et al. (2005). Role of hexagonal structure-forming lipids in diadinoxanthin and violaxanthin solubilization and de-epoxidation. Biochemistry 44, 4028–4036. doi: 10.1021/bi047464k
Goss, R., Nerlich, J., Lepetit, B., Schaller, S., Vieler, A., and Wilhelm, C. (2009). The lipid dependence of diadinoxanthin de-epoxidation presents new evidence for a macrodomain organization of the diatom thylakoid membrane. J. Plant Physiol. 166, 1839–1854. doi: 10.1016/j.jplph.2009.05.017
Goss, R., Pinto, E. A., Wilhelm, C., and Richter, M. (2006). The importance of a highly active and ΔpH-regulated diatoxanthin epoxidase for the regulation of the PS II antenna function in diadinoxanthin cycle containing algae. J. Plant Physiol. 163, 1008–1021. doi: 10.1016/j.jplph.2005.09.008
Goss, R., and Wilhelm, C. (2009). “Lipids in algae, lichens and mosses,” in Lipids in Photosynthesis: Essential and Regulatory Functions, eds H. Wada, N. Murata, and M. Govindjee (Berlin: Springer), 119–120.
Gounaris, K., Brain, A. P. R., Quinn, P. J., and Williams, W. P. (1984). Structural reorganization of chloroplast thylakoid membranes in response to heat stress. Biochim. Biophys. Acta 766, 198–208. doi: 10.1016/0005-2728(84)90232-9
Grouneva, I., Jakob, T., Wilhelm, C., and Goss, R. (2006). Influence of ascorbate and pH on the activity of the diatom xanthophyll cycle-enzyme diadinoxanthin de-epoxidase. Physiol. Plant. 126, 205–211. doi: 10.1111/j.1399-3054.2006.00613.x
Grudzinski, W., Nierzwicki, L., Welc, R., Reszczynska, E., Luchowski, R., Czub, J., et al. (2017). Localization and orientation of xanthophylls in a lipid bilayer. Sci. Rep. 7:9619.
Gruszecki, W. I., and Strzalka, K. (1991). Does the xanthophyll cycle take part in the regulation of fluidity of the thylakoid membrane? Biochim. Biophys. Acta 1060, 310–314. doi: 10.1016/s0005-2728(05)80322-6
Gruszecki, W. I., and Strzalka, K. (2005). Carotenoids as modulators of lipid membrane physical properties. Biochim. Biophys. Acta 1740, 108–115. doi: 10.1016/j.bbadis.2004.11.015
Grzyb, J., Latowski, D., and Strzalka, K. (2006). Lipocalins - a family portrait. J. Plant Physiol. 163, 895–915. doi: 10.1016/j.jplph.2005.12.007
Gundermann, K., and Büchel, C. (2008). The fluorescence yield of the trimeric fucoxanthin-chlorophyll-protein FCPa in the diatom Cyclotella meneghiniana is dependent on the amount of bound diatoxanthin. Photosynth. Res. 95, 229–235. doi: 10.1007/s11120-007-9262-1
Gundermann, K., Wagner, V., Mittag, M., and Büchel, C. (2019). Fucoxanthin-chlorophyll protein complexes of the centric diatom Cyclotella meneghiniana differ in Lhcx1 and Lhcx6_1 content. Plant Physiol. 179, 1779–1795. doi: 10.1104/pp.18.01363
Guschina, I. A., and Harwood, J. L. (2006). Lipids and lipid metabolism in eukaryotic algae. Prog. Lipid Res. 45, 160–186. doi: 10.1016/j.plipres.2006.01.001
Guskov, A., Kern, J., and Saenger, W. (2009). Cyanobacterial photosystem II at 2.9-A resolution and the role of quinones, lipids, channels and chloride. Nat. Struct. Mol. Biol. 16, 334–342. doi: 10.1038/nsmb.1559
Hager, A. (1957). Über den Einfluss klimatischer Faktoren auf den Blattfarbstoffgehalt höherer Pflanzen. Planta 49, 524–560. doi: 10.1007/bf01917726
Hager, A. (1967a). Untersuchungen über die lichtinduzierten, reversiblen Xanthophyllumwandlungen an Chlorella und Spinacia oleracea. Planta 74, 148–172. doi: 10.1007/bf00388326
Hager, A. (1967b). Untersuchungen üben die Rückreaktionen im Xanthophyll-Cyclus bei Chlorella, Spinacia und Taxus. Planta 76, 138–148. doi: 10.1007/BF00385460
Hager, A. (1969). Lichtbedingte pH-Erniedrigung in einem Chloroplasten-Kompartiment als Ursache der enzymatischen Violaxanthin-Zeaxanthin-Umwandlung: Beziehungen zur Photophosphorylierung. Planta 89, 224–243. doi: 10.1007/bf00385028
Hager, A., and Holocher, K. (1994). Localization of the xanthophyll-cycle enzyme violaxanthin de-epoxidase within the thylakoid lumen and abolition of its mobility by a (light-dependent) pH decrease. Planta 192, 581–589.
Hager, A., and Stransky, H. (1970). Das Carotinoidmuster und die Verbreitung des lichtinduzierten Xanthophyllcyclus in verschiedenen Algenklassen. Arch. Mikrobiol. 73, 77–89. doi: 10.1007/bf00409954
Hallin, E. I., Guo, K., and Akerlund, H. E. (2015). Violaxanthin de-epoxidase disulphides and their role in activity and thermal stability. Photosynth Res. 124, 191–198. doi: 10.1007/s11120-015-0118-119
Hallin, E. I., Hasan, M., Guo, K., and Akerlund, H.-E. (2016). Molecular studies on structural changes and oligomerisation of violaxanthin deepoxidase associated with the pH-dependent activation. Photosynth. Res. 129, 29–41. doi: 10.1007/s11120-016-0261-y
Haranczyk, H., Strzalka, K., Dietrich, W., and Blicharski, J. S. (1995). 13P-NMR observation of the temperature and glycerol induced non-lamellar phase formation in wheat thylakoid membranes. J. Biol. Phys. 21, 125–139. doi: 10.1007/bf00705595
Härtel, H., and Benning, C. (2000). Can digalactosyldiacylglycerol substitute for phosphatidylcholine upon phosphate deprivation in leaves and roots of Arabidopsis? Biochem. Soc. Trans. 28, 729–732. doi: 10.1042/bst0280729
Harwood, J. L., and Guschina, I. A. (2009). The versatility of algae and their lipid metabolism. Biochimie 91, 679–684. doi: 10.1016/j.biochi.2008.11.004
Havaux, M. (1998). Carotenoids as membrane stabilizers in chloroplasts. Trends Plant Sci. 3, 147–151. doi: 10.1016/s1360-1385(98)01200-x
Havaux, M., Dall’Osto, L., and Bassi, R. (2007). Zeaxanthin has enhanced antioxidant capacity with respect to all other xanthophylls in Arabidopsis leaves and functions independent of binding to PSII antennae. Plant Physiol. 145, 1506–1520. doi: 10.1104/pp.107.108480
Havaux, M., and Niyogi, K. K. (1999). The violaxanthin cycle protects plants from photooxidative damage by more than one mechanism. Proc. Natl. Acad. Sci. U.S.A. 96, 8762–8767. doi: 10.1073/pnas.96.15.8762
Heemskerk, J. W. M., Wintermans, J. F. G. M., Joyard, J., Block, M. A., Dorne, A. J., and Douce, R. (1986). Localization of galactolipid–galactolipid galactosyltransferase and acyltransferase in outer envelope membrane of spinach chloroplasts. Biochim. Biophys. Acta 877, 281–289. doi: 10.1016/0005-2760(86)90305-x
Heinz, E., and Roughan, P. G. (1983). Similarities and differences in lipid metabolism of chloroplasts isolated from 18:3 and 16:3 plants. Plant Physiol. 72, 273–279. doi: 10.1104/pp.72.2.273
Hieber, A. D., Bugos, R. C., and Yamamoto, H. Y. (2000). Plant lipocalins: violaxanthin de-epoxidase and zeaxanthin epoxidase. Biochim. Biophys. Acta 1482, 84–91. doi: 10.1016/s0167-4838(00)00141-2
Higashi, Y., Okazaki, Y., Myouga, F., Shinozaki, K., and Saito, K. (2015). Landscape of the lipidome and transcriptome under heat stress in Arabidopsis thaliana. Sci. Rep. 5:10533.
Higashi, Y., Okazaki, Y., Takano, K., Myouga, F., Shinozaki, K., Knoch, E., et al. (2018). A lipase gene, HEAT INDUCIBLE LIPASE1, is involved in remodeling chloroplastic monogalactosyldiacylglycerol by liberatingα-linolenic acid in Arabidopsis leaves under heat stress. Plant Cell 30, 1887–1905. doi: 10.1105/tpc.18.00347
Higashi, Y., and Saito, K. (2019). Lipidomic studies of membrane glycerolipids in plant leaves under heat stress. Prog. Lipid Res. 75:100990. doi: 10.1016/j.plipres.2019.100990
Hölzl, G., and Dörmann, P. (2019). Chloroplast lipids and their biosynthesis. Annu. Rev. Plant Biol. 70, 51–81. doi: 10.1146/annurev-arplant-050718-100202
Holzwarth, A. R., Miloslavina, Y., Nilkens, M., and Jahns, P. (2009). Identification of two quenching sites active in the regulation of photosynthetic light-harvesting studied by time-resolved fluorescence. Chem. Phys. Lett. 483, 262–267. doi: 10.1016/j.cplett.2009.10.085
Horton, P. (2014). “Developments in research on non-photochemical fluorescence quenching: emergence of key ideas, theories and experimental approaches,” in Non-Photochemical Quenching And Energy Dissipation In Plants, Algae And Cyanobacteria, eds B. Demmig-Adams, G. Garab, and W. W. Adams (Dordrecht: Springer), 73–95. doi: 10.1007/978-94-017-9032-1_3
Huang, Y., and Gui, S. (2018). Factors affecting the structure of lyotropic liquid crystals and the correlation between structure and drug diffusion. RSC Adv. 8, 6978–6987. doi: 10.1039/c7ra12008g
Jahns, P., and Holzwarth, A. R. (2012). The role of the xanthophyll cycle and of lutein in photoprotection of photosystem II. Biochim. Biophys. Acta 1817, 182–193. doi: 10.1016/j.bbabio.2011.04.012
Jahns, P., Latowski, D., and Strzalka, K. (2009). Mechanism and regulation of the violaxanthin cycle: the role of antenna proteins and membrane lipids. Biochim. Biophys. Acta 1787, 3–14. doi: 10.1016/j.bbabio.2008.09.013
Jahns, P., Wehner, A., Paulsen, H., and Hobe, S. (2001). De-epoxidation of violaxanthin after reconstitution into different carotenoid binding sites of light-harvesting complex II. J. Biol. Chem. 276, 22154–22159. doi: 10.1074/jbc.m102147200
Jakob, T., Goss, R., and Wilhelm, C. (2001). Unusual pH-dependence of diadinoxanthin de-epoxidase activation causes chlororespiratory induced accumulation of diatoxanthin in the diatom Phaeodactylum tricornutum. J. Plant Physiol. 158, 383–390. doi: 10.1078/0176-1617-00288
Janero, D. R., and Barrnett, R. (1982). Isolation and characterization of an ether-linked homoserine lipid from the thylakoid membrane of Chlamydomonas reinhardtii 137+. J. Lipid Res. 23, 307–316.
Jarvis, P., Dörmann, P., and Chory, J. (2000). Galactolipid deficiency and abnormal chloroplast development in the Arabidopsis MGD synthase 1 mutant. Proc. Natl. Acad. Sci. U.S.A. 97, 8175–8179. doi: 10.1073/pnas.100132197
Johnson, M. P., Havaux, M., Triantaphylides, C., Ksas, B., Pascal, A. A., Robert, B., et al. (2007). Elevated zeaxanthin bound to oligomeric LHCII enhances the resistance of Arabidopsis to photooxidative stress by a lipid-protective, antioxidant mechanism. J. Biol. Chem. 282, 22605–22618. doi: 10.1074/jbc.m702831200
Jouhet, J. (2013). Importance of the hexagonal lipid phase in biological membrane organization. Front. Plant Sci. 4:494. doi: 10.3389/fpls.2013.00494
Jouhet, J., Maréchal, E., Baldan, B., Bligny, R., Joyard, J., and Block, M. A. (2004). Phosphate deprivation induces transfer of DGDG galactolipid from chloroplast to mitochondria. J. Cell. Biol. 167, 863–874. doi: 10.1083/jcb.200407022
Kansy, M., Wilhelm, C., and Goss, R. (2014). Influence of thylakoid membrane lipids on the structure and function of the plant photosystem II core complex. Planta 240, 781–796. doi: 10.1007/s00425-014-2130-2
Killian, J. A. (1998). Hydrophobic mismatch between proteins and lipids in membranes. Biochim. Biophys. Acta 1376, 401–416. doi: 10.1016/s0304-4157(98)00017-3
Kirchhoff, H. (2014). Diffusion of molecules and macromolecules in thylakoid membranes. Biochim. Biophys. Acta 1837, 495–502. doi: 10.1016/j.bbabio.2013.11.003
Kirchhoff, H. (2018). Structure-function relationships in photosynthetic membranes: challenges and emerging fields. Plant Sci. 266, 76–82. doi: 10.1016/j.plantsci.2017.09.021
Kirchhoff, H., Mukherjee, U., and Galla, H.-J. (2002). Molecular architecture of the thylakoid membrane: lipid diffusion space for plastoquinone. Biochemistry 41, 4872–4882. doi: 10.1021/bi011650y
Kobayashi, K., Endo, K., and Wada, H. (2016). “Roles of lipids in photosynthesis,” in Lipids in Plant and Algae Development, eds Y. Nakamura and Y. Li-Beisson (Berlin: Springer), 21–49. doi: 10.1007/978-3-319-25979-6_2
Kobayashi, K., Kondo, M., Fukuda, H., Nishimura, M., and Ohta, H. (2007). Galactolipid synthesis in chloroplast inner envelope is essential for proper thylakoid biogenesis, photosynthesis, and embryogenesis. Proc. Natl. Acad. Sci. U.S.A. 104, 17216–17221. doi: 10.1073/pnas.0704680104
Kotakis, C., Akhtar, P., Zsiros, O., Garab, G., and Lambrev, P. H. (2018). Increased thermal stability of photosystem II and the macro-organization of thylakoid membranes, induced by co-solutes, associated with changes in the lipid-phase behaviour of thylakoid membranes. Photosynthetica 56, 254–264. doi: 10.1007/s11099-018-0782-z
Krauss, S. (2001). “Mitochondria: structure and role in respiration,” in Encyclopedia of Life Sciences, (New York, NY: Nature Publishing Group), 1–6.
Krumova, S. B., Dijkema, C., de Waard, P., van As, H., Garab, G., and van Amerongen, H. (2008). Phase behavior of phosphatidylglycerol in spinach thylakoid membranes as revealed by 31P-NMR. Biochim. Biophys. Acta 1778, 997–1003. doi: 10.1016/j.bbamem.2008.01.004
Kumari, P., Kumar, M., Reddy, C. R. K., and Jha, B. (2013). “Algal lipids, fatty acids and sterols,” in Functional Ingredients From Algae For Foods And Nutraceuticals, ed. H. Dominguez (Sawston: Woodhead Publishing Ltd), 87–134. doi: 10.1533/9780857098689.1.87
Latowski, D., Akerlund, H.-E., and Strzalka, K. (2004). Violaxanthin de-epoxidase, the xanthophyll cycle enzyme, requires lipid inverted hexagonal structures for its activity. Biochemistry 43, 4417–4420. doi: 10.1021/bi049652g
Latowski, D., Kostecka, A., and Strzalka, K. (2000). Effect of monogalactosyldiacylglycerol and other thylakoid lipids on violaxanthin de-epoxidation in liposomes. Biochem. Soc. Trans. 28, 810–812. doi: 10.1042/bst0280810
Latowski, D., Kruk, J., Burda, K., Skrzynecka-Jaskier, M., Kostecka-Gugala, A., and Strzalka, K. (2002). Kinetics of violaxanthin de-epoxidation by violaxanthin de-epoxidase, a xanthophyll cycle enzyme, is regulated by membrane fluidity in model lipid bilayers. Eur. J. Biochem. 269, 4656–4665. doi: 10.1046/j.1432-1033.2002.03166.x
Lavaud, J., and Lepetit, B. (2013). An explanation for the inter-species variability of the photo-protective non-photochemical chlorophyll fluorescence quenching in diatoms. Biochim. Biophys. Acta 1827, 294–302. doi: 10.1016/j.bbabio.2012.11.012
Lavaud, J., Rousseau, B., and Etienne, A. (2003). Enrichment of the light-harvesting complex in diadinoxanthin and implications for the nonphotochemical fluorescence quenching in diatoms. Biochemistry 42, 5802–5808. doi: 10.1021/bi027112i
Lee, A. L.-C., and Thornber, J. P. (1995). Analysis of the pigment stoichiometry of pigment-protein complexes from barley (Hordeum vulgare). Plant Physiol. 107, 565–574. doi: 10.1104/pp.107.2.565
Leonelli, L., Brooks, M. D., and Niyogi, K. K. (2017). Engineering the lutein epoxide cycle into Arabidopsis thaliana. Proc. Natl. Acad. Sci. U.S.A. 114, E7002–E7008. doi: 10.1073/pnas.1704373114
Lepetit, B., Goss, R., Jakob, T., and Wilhelm, C. (2012). Molecular dynamics of the diatom thylakoid membrane under different light conditions. Photosynth. Res. 111, 245–257. doi: 10.1007/s11120-011-9633-5
Lepetit, B., Sturm, S., Rogato, A., Gruber, A., Sachse, M., Falciatore, A., et al. (2013). High light acclimation in the secondary plastids containing diatom Phaeodactylum tricornutum is triggered by the redox state of the plastoquinone pool. Plant Physiol. 161, 853–865. doi: 10.1104/pp.112.207811
Lepetit, B., Volke, D., Gilbert, M., Wilhelm, C., and Goss, R. (2010). Evidence for the existence of one antenna-associated, lipid-dissolved, and two protein-bound pools of diadinoxanthin cycle pigments in diatoms. Plant Physiol. 154, 1905–1920. doi: 10.1104/pp.110.166454
Lepetit, B., Volke, D., Szabo, M., Hoffmann, R., Garab, G., Wilhelm, C., et al. (2008). “The oligomeric antenna of the diatom P. tricornutum - localization of diadinoxanthin cycle pigments,” in Photosynthesis. Energy from the sun, eds J. F. Allen, E. Gantt, J. H. Golbeck, and B. Osmond (Berlin: Springer), 277–280.
Li, M., Welti, R., and Wang, X. (2006). Quantitative profiling of Arabidopsis polar glycerolipids in response to phosphorus starvation. Roles of phospholipases D zeta1 and D zeta 2 in phosphatidylcholine hydrolysis and digalactosyldiacylglycerol accumulation in phosphorus-starved plants. Plant Physiol. 142, 750–761. doi: 10.1104/pp.106.085647
Li, Q., Zheng, Q., Shen, W., Cram, D., Fowler, D. B., Wei, Y., et al. (2015). Understanding the biochemical basis of temperature induced lipid pathway adjustments in plants. Plant Cell 27, 86–103. doi: 10.1105/tpc.114.134338
Li, X., Björkman, O., Shih, C., Grossman, A. R., Rosenquist, M., Jansson, S., et al. (2000). A pigment-binding protein essential for regulation of photosynthetic light harvesting. Nature 403, 391–395. doi: 10.1038/35000131
Li, Z., Peers, G., Dent, R. M., Bai, Y., Yang, S. Y., Apel, W., et al. (2016). Evolution of an atypical de-epoxidase for photoprotection in the green lineage. Nat. Plants 2:16140.
Li-Beisson, Y., Nakamura, Y., and Harwood, J. (2016). Lipids: from chemical structures, biosynthesis, and analyses to industrial applications. Subcell. Biochem. 86, 1–21.
Liu, Z., Yan, H., Wang, K., Kuang, T., Zhang, J., Gui, L., et al. (2004) crystal structure of spinach major light-harvesting complex at 2.72 A resolution. Nature 428, 287–292. doi: 10.1038/nature02373
Lohr, M., and Wilhelm, C. (1998). Algae displaying the diadinoxanthin cycle also possess the violaxanthin cycle. Proc. Natl. Acad. Sci. U.S.A. 96, 8784–8789. doi: 10.1073/pnas.96.15.8784
Lohr, M., and Wilhelm, C. (2001). Xanthophyll synthesis in diatoms: quantification of putative intermediates and comparison of pigment conversion kinetics with rate constants derived from a model. Planta 212, 382–391. doi: 10.1007/s004250000403
Macko, S., Wehner, A., and Jahns, P. (2002). Comparison of violaxanthin de-epoxidation from the stroma and lumen sides of isolated thylakoid membranes from Arabidopsis: Implications for the mechanism of de-epoxidation. Planta 216, 309–314. doi: 10.1007/s00425-002-0848-8
Matsuda, O., Sakamoto, H., Hashimoto, T., and Iba, K. (2005). A temperature-sensitive mechanism that regulates post-translational stability of a plastidial omega-3 fatty acid desaturase (FAD8) in Arabidopsis leaf tissues. J. Biol. Chem. 280, 3597–3604. doi: 10.1074/jbc.m407226200
Mewes, H., and Richter, M. (2002). Supplementary Ultraviolet-B radiation induces a rapid reversal of the diadinoxanthin cycle in the strong light-exposed diatom Phaeodactylum tricornutum. Plant Physiol. 130, 1527–1535. doi: 10.1104/pp.006775
Miloslavina, Y., Grouneva, I., Lambrev, P. H., Lepetit, B., Goss, R., Wilhelm, C., et al. (2009). Ultra-fast fluorescence study on the location and mechanism of non-photochemical quenching in diatoms. Biochim. Biophys. Acta 1787, 1189–1197. doi: 10.1016/j.bbabio.2009.05.012
Moellering, E. R., and Benning, C. (2011). Galactoglycerolipid metabolism under stress: a time for remodeling. Trends Plant Sci. 16, 98–107. doi: 10.1016/j.tplants.2010.11.004
Moellering, E. R., Muthan, B., and Benning, C. (2010). Freezing tolerance in plants requires lipid remodeling at the outer chloroplast membrane. Science 330, 226–228. doi: 10.1126/science.1191803
Mongrand, S., Bessoule, J.-J., Cabantous, F., and Cassagne, C. (1998). The C16:3/C18:3 fatty acid balance in photosynthetic tissues from 468 plant species. Phytochemistry 49, 1049–1064. doi: 10.1016/s0031-9422(98)00243-x
Mongrand, S., Stanislas, T., Bayer, E. M. F., Lherminier, J., and Simon-Plas, F. (2010). Membrane rafts in plant cells. Trends Plant Sci. 15, 656–663. doi: 10.1016/j.tplants.2010.09.003
Montsant, A., Allen, A. E., Coesel, S., De Martino, A., Falciatore, A., Mangogna, M., et al. (2007). Identification and comparative genomic analysis of signaling and regulatory components in the diatom Thalassiosira pseudonana. J. Phycol. 43, 585–604.
Morosinotto, T., Caffarri, S., Dall’Osto, L., and Bassi, R. (2003). Mechanistic aspects of the xanthophyll dynamics in higher plant thylakoids. Physiol. Plant. 119, 347–354. doi: 10.1034/j.1399-3054.2003.00213.x
Mène-Saffrané, L., Dubugnon, L., Chételat, A., Stolz, S., Gouhier-Darimont, C., and Farmer, E. (2009). Nonenzymatic oxidation of trienoic fatty acids contributes to reactive oxygen species management in Arabidopsis. J. Biol. Chem. 284, 1702–1708. doi: 10.1074/jbc.m807114200
Nawrocki, W. J., Liu, X., and Croce, R. (2019). Chlamydomonas reinhardtii exhibits de facto constitutive NPQ capacity in physiologically relevant conditions. Plant Physiol. 182, 472–479. doi: 10.1104/pp.19.00658
Nicholson, G. L. (2014). The fluid-mosaic model of membrane structure: Still relevant to understanding the structure, function and dynamics of biological membranes after more than 40 years. Biochim. Biophys. Acta 1838, 1451–1466. doi: 10.1016/j.bbamem.2013.10.019
Nicol, L., Nawrocki, W. J., and Croce, R. (2019). Disentangling the sites of non-photochemical quenching in vascular plants. Nature Plants 5, 1177–1183. doi: 10.1038/s41477-019-0526-5
Niyogi, K. K., and Truong, T. B. (2013). Evolution of flexible non-photochemical quenching mechanisms that regulate light harvesting in oxygenic photosynthesis. Curr. Opin. Plant Biol. 16, 307–314. doi: 10.1016/j.pbi.2013.03.011
Ohlrogge, J. B., Kuhn, D. N., and Stumpf, P. K. (1979). Subcellular localization of acyl carrier protein in leaf protoplasts of Spinacia oleracea. Proc. Natl. Acad. Sci. U.S.A. 76, 1194–1198. doi: 10.1073/pnas.76.3.1194
Okazaki, Y., Otsuki, H., Narisawa, T., Kobayashi, M., Sawai, S., Kamide, Y., et al. (2013). A new class of plant lipid is essential for protection against phosphorus depletion. Nat. Commun. 4:1510.
Peers, G., Truong, T. B., Ostendorf, E., Busch, A., Elrad, D., Grossman, A. R., et al. (2009). An ancient light-harvesting protein is critical for the regulation of algal photosynthesis. Nature 462, 518–521. doi: 10.1038/nature08587
Pfündel, E. E., Renganathan, M., Gilmore, A. M., Yamamoto, H. Y., and Dilley, R. A. (1994). Intrathylakoid pH in isolated Pea chloroplasts as probed by violaxanthin deepoxidation. Plant Physiol. 106, 1647–1658. doi: 10.1104/pp.106.4.1647
Rabinowitch, H. D., Budowski, P., and Kedar, N. (1975). Carotenoids and epoxide cycles in mature-green tomatoes. Planta 122, 91–97. doi: 10.1007/bf00385408
Ramani, B., Reeck, T., Debez, A., Stelzer, R., Huchzermeyer, B., Schmidt, A., et al. (2006). Aster tripolium L. and Sesuvium portulacastrum L.: two halophytes, two strategies to survive in saline habitats. Plant Physiol. Biochem. 44, 395–408. doi: 10.1016/j.plaphy.2006.06.007
Roughan, G., and Slack, R. (1984). Glycerolipid synthesis in leaves. Trends Biochem. Sci. 9, 383–386. doi: 10.1016/0968-0004(84)90220-2
Ruban, A. V. (2016). Nonphotochemical fluorescence quenching: mechanism and effectiveness in protecting plants from photodamage. Plant Physiol. 170, 1903–1916. doi: 10.1104/pp.15.01935
Ruban, A. V., Lee, P. J., Wentworth, M., Young, A. J., and Horton, P. (1999). Determination of the stoichiometry and strength of binding of xanthophylls to the photosystem II light harvesting complexes. J. Biol. Chem. 274, 10458–10465. doi: 10.1074/jbc.274.15.10458
Ruban, A. V., Phillip, D., Young, A. J., and Horton, P. (1997). Carotenoid-dependent oligomerization of the major chlorophyll a/b light harvesting complex of photosystem II of plants. Biochemistry 36, 7855–7859. doi: 10.1021/bi9630725
Ruban, A. V., Rees, D., Pascal, A. A., and Horton, P. (1992). Mechanism of ΔpH dependent dissipation of absorbed excitation energy by photosynthetic membranes. II. The relation-ship between LHCII aggregation in vitro and qE in isolated thylakoids. Biochim. Biophys. Acta 1102, 39–44. doi: 10.1016/0005-2728(92)90062-7
Ruban, A. V., Young, A. J., Pascal, A. A., and Horton, P. (1994). The effects of illumination on the xanthophyll composition of the photosystem II light-harvesting complexes of spinach thylakoid membranes. Plant Physiol. 104, 227–234. doi: 10.1104/pp.104.1.227
Sacharz, J., Giovagnetti, V., Ungerer, P., Mastroianni, G., and Ruban, A. V. (2017). The xanthophyll cycle affects reversible interactions between PsbS and light-harvesting complex II to control non-photochemical quenching. Nature Plants 3:16225.
Saga, G., Giorgetti, A., Fufezan, C., Giacometti, G. M., Bassi, R., and Morosinotto, T. (2010). Mutation analysis of violaxanthin de-epoxidase identifies substrate-binding sites and residues involved in catalysis. J. Biol. Chem. 285, 23763–23770. doi: 10.1074/jbc.m110.115097
Saphozhnikov, D. J., Krasovskaya, T. A., and Mayevskaya, A. N. (1957). Changes observed in the relation between the main carotenoids in the plastids of green leaves exposed to light. Dokl. Akad. Nauk. SSSR 1 13, 465–467.
Schaller, S., Latowski, D., Jemiola-Rzeminska, M., Quaas, T., Wilhelm, C., Strzalka, K., et al. (2012a). The investigation of violaxanthin de-epoxidation in the primitive green alga Mantoniella squamata (Prasinophyceae) indicates mechanistic differences in xanthophyll conversion to higher plants. Phycologia 51, 359–370. doi: 10.2216/11-127.1
Schaller, S., Wilhelm, C., Strzalka, K., and Goss, R. (2012b). Investigating the interaction between the violaxanthin cycle enzyme zeaxanthin epoxidase and the thylakoid membrane. J. Photochem. Photobiol. B: Biol. 2012, 119–125. doi: 10.1016/j.jphotobiol.2012.05.019
Schaller, S., Latowski, D., Jemiola-Rzeminska, M., Wilhelm, C., Strzalka, K., and Goss, R. (2010). The main thylakoid membrane lipid monogalactosyldiacylglycerol (MGDG) promotes the de-epoxidation of violaxanthin associated with the light-harvesting complex of photosystem II (LHCII). Biochim. Biophys. Acta 2, 414–424. doi: 10.1016/j.bbabio.2009.12.011
Schaller, S., Latowski, D., Jemioła-Rzemioska, M., Dawood, A., Wilhelm, C., Strzałka, K., et al. (2011). Regulation of LHCII aggregation by different thylakoid membrane lipids. Biochim. Biophys. Acta 1807, 326–335. doi: 10.1016/j.bbabio.2010.12.017
Schaller, S., Richter, K., Wilhelm, C., and Goss, R. (2014). Influence of pH, Mg2 +, and lipid composition on the aggregation state of the diatom FCP in comparison to the LHCII of vascular plants. Photosynth. Res. 119, 305–317. doi: 10.1007/s11120-013-9951-x
Schaller-Laudel, S., Latowski, D., Jemioła-Rzeminska, M., Strzałka, K., Daum, S., Bacia, K., et al. (2017). Influence of thylakoid membrane lipids on the structure of aggregated light-harvesting complexes of the diatom Thalassiosira pseudonana and the green alga Mantoniella squamata. Physiol. Plant. 160, 339–358. doi: 10.1111/ppl.12565
Schaller-Laudel, S., Volke, D., Redlich, M., Kansy, M., Hoffmann, R., Wilhelm, C., et al. (2015). The diadinoxanthin diatoxanthin cycle induces structural rearrangements of the isolated FCP antenna complexes of the pennate diatom Phaeodactylum tricornutum. Plant Physiol. Biochem. 96, 364–376. doi: 10.1016/j.plaphy.2015.09.002
Schmid-Siegert, E., Stepushenko, O., Glauser, G., and Farmer, E. (2016). Membranes as structural antioxidants - recycling of malondialdehyde to its source in oxidation-sensitive chloroplast fatty acids. J. Biol. Chem. 291, 13005–13013. doi: 10.1074/jbc.m116.729921
Schumann, A., Goss, R., Jakob, T., and Wilhelm, C. (2007). Investigation of the quenching efficiency of diatoxanthin in cells of Phaeodactylum tricornutum (Bacillariophyceae) with different pool sizes of xanthophyll cycle pigments. Phycologia 46, 113–117. doi: 10.2216/06-30.1
Seiwert, D., Witt, H., Janshoff, A., and Paulsen, H. (2017). The non-bilayer lipid MGDG stabilizes the major light-harvesting complex (LHCII) against unfolding. Sci. Rep. 7:5158.
Selstam, E. (1998). “Development of thylakoid membranes with respect to lipids,” in Lipids in Photosynthesis: Structure, Function And Genetics, eds P. A. Siegenthaler and N. Murata (Dordrecht: Kluwer Academic Publishers), 209–224. doi: 10.1007/0-306-48087-5_11
Shimojima, M. (2011). Biosynthesis and functions of the plant sulfolipid. Progr. Lipid Res. 50, 234–239. doi: 10.1016/j.plipres.2011.02.003
Shipley, G. G., Green, J. P., and Nichols, B. W. (1973). The phase behaviour of monogalactosyl, digalactosyl, and sulphoquinovosyl diglycerides. Biochim. Biophys. Acta 311, 531–544. doi: 10.1016/0005-2736(73)90128-4
Simidjiev, I., Barzda, V., Mustardy, L., and Garab, G. (1998). Role of thylakoid lipids in the structural flexibility of lamellar aggregates of the isolated light-harvesting chlorophyll a/b complex of photosystem II. Biochemistry 37, 4169–4173. doi: 10.1021/bi972726m
Simidjiev, I., Stoylova, S., Amenitsch, H., Javorfi, T., Mustardy, L., Laggner, P., et al. (2000). Self-assembly of large, ordered lamellae from non-bilayer lipids and integral membrane proteins in vitro. Proc. Natl. Acad. Sci. U.S.A. 97, 1473–1476. doi: 10.1073/pnas.97.4.1473
Simionato, D., Basso, S., Zaffagnini, M., Lana, T., Marzotto, F., Trost, P., et al. (2015). Protein redox regulation in the thylakoid lumen: the importance of disulfide bonds for violaxanthin deepoxidase. FEBS Lett. 589, 919–923. doi: 10.1016/j.febslet.2015.02.033
Simon-Plas, F., Perraki, A., Bayer, E., Gerbot-Pissot, P., and Mongrand, S. (2011). An update on plant membrane rafts. Curr. Opin. Plant Biol. 14, 642–649. doi: 10.1016/j.pbi.2011.08.003
Singer, S. J., and Nicholson, G. L. (1972). The fluid mosaic model of the structure of cell membranes. Science 175, 720–731. doi: 10.1126/science.175.4023.720
Stransky, H., and Hager, A. (1970). Das Carotinoidmuster und die Verbreitung des lichtinduzierten Xanthophyllzyklus in verschiedenen Algenklassen. II: Xanthophyceae. Arch. Mikrobiol. 71, 164–190. doi: 10.1007/bf00417740
Taddei, L., Chukhutsina, V. U., Lepetit, B., Stella, G. R., Bassi, R., van Amerongen, H., et al. (2018). Dynamic changes between two LHCX-related energy quenching sites control diatom photoacclimation. Plant Physiol. 177, 953–965. doi: 10.1104/pp.18.00448
Taddei, L., Stella, G. R., Rogato, A., Bailleul, B., Fortunato, A. E., Annunziata, R., et al. (2016). Multisignal control of expression of the LHCX protein family in the marine diatom Phaeodactylum tricornutum. J. Exp. Bot. 67, 3939–3951. doi: 10.1093/jxb/erw198
Tardy, F., and Havaux, M. (1997). Thylakoid membrane fluidity and thermostability during the operation of the xanthophyll cycle in higher plant chloroplasts. Biochim. Biophys. Acta 1330, 179–193. doi: 10.1016/s0005-2736(97)00168-5
Thomas, P. G., Brain, A. P. R., Quinn, P. J., and Williams, W. P. (1985). Low pH and phospholipase A2 treatment induce the phase separation of non-bilayer lipids within pea chloroplast membranes. FEBS Letts. 183, 161–166. doi: 10.1016/0014-5793(85)80976-5
Triantaphylidès, C., and Havaux, M. (2009). Singlet oxygen in plants: production, detoxification and signaling. Trends Plant Sci. 14, 219–228. doi: 10.1016/j.tplants.2009.01.008
van Besouw, A., and Wintermans, J. F. (1978). Galactolipid formation in chloroplast envelopes. I. Evidence for two mechanisms in galactosylation. Biochim. Biophys. Acta 529, 44–53. doi: 10.1016/0005-2760(78)90102-9
van den Brink-van der Laan, E., Killian, J. A., and de Kruijff, B. (2004). Non-bilayer lipids affect peripheral and integral membrane proteins via changes in the lateral pressure profile. Biochim. Biophys. Acta 1666, 275–288. doi: 10.1016/j.bbamem.2004.06.010
van Eerden, F. J., de Jong, D. H., de Vries, A. H., Wassenaar, T. A., and Marrink, S. J. (2015). Characterization of thylakoid lipid membranes from cyanobacteria and higher plants by molecular dynamics simulations. Biochim. Biophys. Acta 1848, 1319–1330. doi: 10.1016/j.bbamem.2015.02.025
van Eerden, F. J., Melo, M. N., Frederix, P. W. J. M., and Marrink, S. J. (2017). Prediction of thylakoid lipid binding sites on Photosystem II. Biophys. J. 113, 2669–2681. doi: 10.1016/j.bpj.2017.09.039
van Mooy, B. A. S., Rocap, G., Fredricks, H. F., Evans, C. T., and Devol, A. H. (2006). Sulfolipids dramatically decrease phosphorus demand by picocyanobacteria in oligotrophic marine environments. Proc. Natl. Acad. Sci. U.S.A. 103, 8607–8612. doi: 10.1073/pnas.0600540103
Vidal-Meireles, A., Toth, D., Kovacz, L., Neupert, J., and Toth, S. Z. (2020). Ascorbate deficiency does not limit non-photochemical quenching in Chlamydomonas reinhardtii. Plant Physiol. 182:E00916.2019. doi: 10.1104/pp.19.00916
Vieler, A., Wilhelm, C., Goss, R., Süss, R., and Schiller, J. (2007). The lipid composition of the unicellular green alga Chlamydomonas reinhardtii and the diatom Cyclotella menghiniana investigated by MALDI-TOF MS and TLC. Chem. Phys. Lipids 150, 143–155. doi: 10.1016/j.chemphyslip.2007.06.224
Wang, K., Tu, W., Liu, C., Rao, Y., Gao, Z., and Yang, C. (2017). 9-cis-neoxanthin in light harvesting complexes of photosystem II regulates the binding of violaxanthin and the xanthophyll cycle. Plant Physiol. 174, 86–96. doi: 10.1104/pp.17.00029
Wang, S., Uddin, M. I., Tanaka, K., Yin, L., Shi, Z., Qi, Y., et al. (2014). Maintenance of chloroplast structure and function by overexpression of the OsMGD gene leads to enhanced salt tolerance in tobacco. Plant Physiol. 165, 1144–1155. doi: 10.1104/pp.114.238899
Wang, W., Yu, L.-J., Xu, C., Tomizaki, T., Zhao, S., Umena, Y., et al. (2019). Structrual basis for blue-green light harvesting and energy dissipation in diatoms. Science 363:eaav0365. doi: 10.1126/science.aav0365
Wehner, A., Grasses, T., and Jahns, P. (2006). De-epoxidation of violaxanthin in the minor antenna proteins of photosystem II, LHCB4, LHCB5, and LHCB6. J. Biol. Chem. 281, 21924–21933. doi: 10.1074/jbc.m602915200
Wehner, A., Storf, S., Jahns, P., and Schmid, V. H. (2004). De-epoxidation of violaxanthin in light-harvesting complex I proteins. J. Biol. Chem. 279, 26823–26829. doi: 10.1074/jbc.m402399200
Welc, R., Luchowski, R., Grudzinski, W., Puzio, M., Sowinski, K., and Gruszecki, W. I. (2016). A key role of xanthophylls that are not embedded in proteins in regulation of the photosynthetic antenna function in plants, revealed by monomolecular layer studies. J. Phys. Chem. B 120, 13056–13064. doi: 10.1021/acs.jpcb.6b10393
Williams, W. P., Brain, A. P. R., and Dominy, P. J. (1992). Induction of non-bilayer lipid phase separations in chloroplast thylakoid membranes by compatible co-solutes and its relation to the thermal stability of Photosystem II. Biochim. Biophys. Acta 1099, 137–144. doi: 10.1016/0304-4173(92)90019-b
Xu, P., Tian, L., Kloz, M., and Croce, R. (2015). Molecular insights into zeaxanthin-dependent quenching in higher plants. Sci. Rep. 5:13679.
Yamamoto, H., Nakayama, T., and Chichester, C. (1962). Studies on the light and dark interconversions of leaf xanthophylls. Arch. Biochem. Biophys. 97, 168–173. doi: 10.1016/0003-9861(62)90060-7
Yamamoto, H. Y. (2006). Functional roles of the major chloroplast lipids in the violaxanthin cycle. Planta 224, 719–724. doi: 10.1007/s00425-006-0257-5
Yamamoto, H. Y., Chenchin, E. E., and Yamada, D. K. (1974). “Effect of chloroplast lipids on violaxanthin de-epoxidase activity,” in Proceedings of the Third International Congress on Photosynthesis, ed. M. Avron (Amsterdam: Elsevier), 1999–2006.
Yamamoto, H. Y., and Higashi, R. M. (1978). Violaxanthin de-epoxidase. Lipid composition and substrate specificity. Arch. Biochem. Biophys. 190, 514–522.
Yamamoto, Y. (2016). Quality control of Photosystem II: The mechanisms for avoidance and tolerance of light and heat stresses are closely linked to membrane fluidity of the thylakoids. Front. Plant Sci. 7:1136. doi: 10.3389/fpls.2016.01136
Yamashita, Y. (2018). “Recent dispersion technology using liquid crystal,” in Liquid Crystals-Recent Advancements in Fundamental and Device Technologies, ed. P. K. Choudhury (London: IntechOpen), doi: 10.5772/intechopen.74156
Yan, X., Chen, D., Xu, J., and Zhou, C. (2011). Profiles of photosynthetic glycerolipids in three strains of Skeletonema determined by UPLC-Q-TOF-MS. J. Appl. Phycol. 23, 271–282. doi: 10.1007/s10811-010-9553-3
Yongmanitchai, W., and Ward, O. P. (1993). Positional distribution of fatty acids, and molecular species of polar lipids, in the diatom Phaeodactylum tricornutum. J. Gen. Microbiol. 139, 465–472. doi: 10.1099/00221287-139-3-465
Yu, B., Xu, C., and Benning, C. (2002). Arabidopsis disrupted in SQD2 encoding sulfolipid synthase is impaired in phosphate-limited growth. Proc. Natl. Acad. Sci. U.S.A. 99, 5732–5737. doi: 10.1073/pnas.082696499
Zhou, F., Liu, S., and Yang, C. (2009). Effect of monogalactosyldiacylglycerol on the interaction between photosystem II core complex and its antenna complexes in liposomes of thylakoid lipids. Photosynth. Res. 99, 185–193. doi: 10.1007/s11120-008-9388-9
Zhou, J., Sekatskii, S., Welc, R., Dietler, G., and Gruszecki, W. I. (2020). The role of xanthophylls in the supramolecular organization of the photosynthetic complex LHCII in lipid membranes studied by high-resolution imaging and nanospectroscopy. Biochim. Biophys. Acta 1861, 148117. doi: 10.1016/j.bbabio.2019.148117
Zhu, J., Gomez, S. M., Mawson, B. T., Jin, X., and Zeiger, E. (1997). The coleoptile chloroplast: distinct distribution of xanthophyll cycle pigments, and enrichment in photosystem II. Photosynth. Res. 51, 137–147.
Keywords: fatty acid, lipid, MGDG, thylakoid membrane domain, violaxanthin de-epoxidase, xanthophyll cycle
Citation: Goss R and Latowski D (2020) Lipid Dependence of Xanthophyll Cycling in Higher Plants and Algae. Front. Plant Sci. 11:455. doi: 10.3389/fpls.2020.00455
Received: 25 February 2020; Accepted: 27 March 2020;
Published: 21 April 2020.
Edited by:
Yoshitaka Nishiyama, Saitama University, JapanReviewed by:
Koichi Kobayashi, Osaka Prefecture University, JapanCopyright © 2020 Goss and Latowski. This is an open-access article distributed under the terms of the Creative Commons Attribution License (CC BY). The use, distribution or reproduction in other forums is permitted, provided the original author(s) and the copyright owner(s) are credited and that the original publication in this journal is cited, in accordance with accepted academic practice. No use, distribution or reproduction is permitted which does not comply with these terms.
*Correspondence: Reimund Goss, cmdvc3NAcnoudW5pLWxlaXB6aWcuZGU=
Disclaimer: All claims expressed in this article are solely those of the authors and do not necessarily represent those of their affiliated organizations, or those of the publisher, the editors and the reviewers. Any product that may be evaluated in this article or claim that may be made by its manufacturer is not guaranteed or endorsed by the publisher.
Research integrity at Frontiers
Learn more about the work of our research integrity team to safeguard the quality of each article we publish.