- 1Laboratory of Plant Physiology, Institute Biology, University of Neuchâtel, Neuchâtel, Switzerland
- 2Aix Marseille University, Centre National de la Recherche Scientifique (CNRS), Commissariat à l’Énergie Atomique et aux Énergies Alternatives (CEA), UMR 7265, Biosciences et Biotechnologies Institute of Aix-Marseille, Saint-Paul-lez-Durance, France
- 3Université Grenoble Alpes, Centre National de la Recherche Scientifique (CNRS), Commissariat à l’Énergie Atomique et aux Énergies Alternatives (CEA), Institut National de la Recherche Agromique (INRA), Interdisciplinary Research Institute of Grenoble - Cell and Plant Physiology Laboratory (IRIG-LPCV), Grenoble, France
Photosynthesis is an essential pathway providing the chemical energy and reducing equivalents that sustain higher plant metabolism. It relies on sunlight, which is an inconstant source of energy that fluctuates in both intensity and spectrum. The fine and rapid tuning of the photosynthetic apparatus is essential to cope with changing light conditions and increase plant fitness. Recently PROTON GRADIENT REGULATION 6 (PGR6-ABC1K1), an atypical plastoglobule-associated kinase, was shown to regulate a new mechanism of light response by controlling the homeostasis of photoactive plastoquinone (PQ). PQ is a crucial electron carrier existing as a free neutral lipid in the photosynthetic thylakoid membrane. Perturbed homeostasis of PQ impairs photosynthesis and plant acclimation to high light. Here we show that a homologous kinase, ABC1K3, which like PGR6-ABC1K1 is associated with plastoglobules, also contributes to the homeostasis of the photoactive PQ pool. Contrary to PGR6-ABC1K1, ABC1K3 disfavors PQ availability for photosynthetic electron transport. In fact, in the abc1k1/abc1k3 double mutant the pgr6(abc1k1) the photosynthetic defect seen in the abc1k1 mutant is mitigated. However, the PQ concentration in the photoactive pool of the double mutant is comparable to that of abc1k1 mutant. An increase of the PQ mobility, inferred from the kinetics of its oxidation in dark, contributes to the mitigation of the pgr6(abc1k1) photosynthetic defect. Our results also demonstrate that ABC1K3 contributes to the regulation of other mechanisms involved in the adaptation of the photosynthetic apparatus to changes in light quality and intensity such as the induction of thermal dissipation and state transitions. Overall, we suggests that, besides the absolute concentration of PQ, its mobility and exchange between storage and active pools are critical for light acclimation in plants.
Introduction
The photosynthetic conversion of light energy into chemical energy occurs via a series of redox reactions resulting in electron transport along the thylakoid membrane. The linear electron transport begins with water splitting at the level of photosystem II (PSII) and ends at photosystem I (PSI) with the reduction of NADP+ by ferredoxin. Both PSII and PSI utilize photonic energy to fuel the redox reactions. Electrons are transferred from PSII to PSI via the cytochrome b6f complex (cyt b6f). At the QB site of PSII, a molecule of plastoquinone (PQ) is reduced twice and protonated to form plastoquinol (PQH2). The PQH2 can then diffuse within the thylakoid membrane to reach the QO site of cyt b6f. Oxidation of PQH2 at cyt b6f occurs through the Q-cycle that releases protons into the thylakoid lumen contributing to the formation of the trans-thylakoid proton gradient. In addition, two electrons are released, one of which returns to PQ pool, while the other is transferred via the plastocyanin to PSI (Tikhonov, 2014). The proportion of PQ that participates in electron transport in the thylakoid membrane is considered as the photoactive PQ pool; whereas the remaining proportion, which is approximately 60–70% of the total PQ, constitutes the non-photoactive pool and is largely stored inside thylakoid-associated lipid droplets known as plastoglobules (PG) (Kruk and Karpinski, 2006; Block et al., 2013; Ksas et al., 2018).
To shuttle electrons, PQ has to rapidly navigate in the thylakoid lipid bilayer (Blackwell et al., 1994). However, the thylakoid membrane is crowded with integral proteins, covering up to 70% of the surface in the grana stacks, which drastically restrict PQ diffusion, especially at the long-range (Kirchhoff et al., 2000). Thylakoid membranes are close to the percolation threshold, therefore it has been suggested that the organization of the protein supercomplexes creates lipid microdomains that facilitate PQ mobility and thus electron shuttling between PSII and cyt b6f (Lavergne and Joliot, 1991; Kirchhoff et al., 2000; Kirchhoff, 2014). On the other hand, long-range mobility of the PQ is also important, for instance to mobilize the non-photoactive pool when damaged PQ molecules have to be replaced as it was proposed to occur during high light stress (Ksas et al., 2018). Therefore, the mobility of plastoquinone/ol molecules within the thylakoid lipid bilayer is a critical parameter to ensure electron transport and maintain the photosynthetic electron transport chain (ETC).
Apart from the role of PQ as electron carrier, its redox state is an important signal in the regulation of many physiological processes within the chloroplast such as state transitions (as described below), gene expression, carotenoid biosynthesis, and antioxidant activity (Karpinski et al., 1999; Li et al., 2009; Suzuki et al., 2012). A rapid readout of the PQ redox state allows photosynthetic adaptation to changes in environmental conditions and therefore increases plant fitness in the natural environment. The adaptation to such varying light conditions is essential for plants to maintain the highest photosynthetic efficiency while avoiding photo-induced damage. To alleviate the negative effects of an imbalance between the activity of the two photosystems, plants have developed a short-term adaptive mechanism: the state transitions. This process allows the re-equilibration of the light energy input between the two photosystems on a time scale of a few minutes by re-allocating part of the mobile light-harvesting complexes II (LHCII) (Allen et al., 1981; Rochaix, 2007, 2013). Phosphorylation of LHCII by the STN7 kinase, the activity of which is dependent on the PQ redox state, allows its movement from PSII to PSI (state 2) (Bellafiore et al., 2005; Shapiguzov et al., 2016; Dumas et al., 2017). The process is reverted by the dephosphorylation of LHCII, operated by the PPH1/TAP38 phosphatase (state 1) (Pesaresi et al., 2010, 2011; Shapiguzov et al., 2010; Trotta et al., 2016). To prevent harmful effects of excess light, plants can dissipate the energy excess as heat by a set of regulated mechanisms summarily referred to as non-photochemical quenching (NPQ). These mechanisms include the rapid rearrangement to a “quenched” state of the LHCII antenna dependent on the PSBS protein and the conversion of the xanthophylls associated to LHCII from violaxanthin to zeaxanthin. The sum of all components creates a system with differential kinetics activated within a few seconds to hours (Dall’Osto et al., 2005; Joliot and Finazzi, 2010; Nilkens et al., 2010; Sylak-Glassman et al., 2014; Ruban, 2016, 2018). Nonetheless, upon continuous light stress, ROS can be produced at the PSII reaction center leading to loss of the PSII activity by damaging the core protein D1 (PsbA). Loss of PSII activity, known as photoinhibition (qI), contributes to the dissipation of excess light (Gong and Ohad, 1991; Miyao, 1994; Vasilikiotis and Melis, 1994). To restore PSII activity after inhibition there is an efficient repair cycle (Aro et al., 1993; Rintamaki et al., 1996, 1997; Theis and Schroda, 2016). The replacement of damaged D1 (PsbA) is facilitated by the phosphorylation of the core proteins of the PSII reaction center by STN8 kinase and requires PSII to migrate from the grana to the stroma lamellae (Aro et al., 1993; Bonardi et al., 2005; Tikkanen et al., 2008; Theis and Schroda, 2016; Li et al., 2018). Photo oxidative stress also triggers other responses allowing the chloroplast to alleviate the damage. These responses involve regulation of gene expression (Pfannschmidt et al., 1999; Pfannschmidt, 2003), structural changes of the thylakoids (Moejes et al., 2017) and synthesis of antioxidant molecules (Munné-Bosch and Alegre, 2002; Kanwischer et al., 2005; Gruszka et al., 2008; Nowicka and Kruk, 2012; Block et al., 2013; Martinis et al., 2014; Ksas et al., 2015, 2018; Spicher et al., 2016; Ferretti et al., 2018).
An important player in the plant stress response is the plastoglobule (PG). PGs are small lipid droplets, attached to the outer lipid leaflet of the thylakoid membrane, delimited by a membrane lipid monolayer consisting mostly of galactolipids and coated with proteins (Austin et al., 2006). Several neutral lipids including prenylquinones, carotenoids, triacylglycerols, phytolesters fill the plastoglobule (Lichtenthaler and Peveling, 1966; Steinmuller and Tevini, 1985; Gaude et al., 2007; Zbierzak et al., 2010; Lippold et al., 2012; Lundquist et al., 2013; Rottet et al., 2016; van Wijk and Kessler, 2017). In response to various stresses PGs increase in size and number (Taylor and Craig, 1971; Hall et al., 1972; Gaude et al., 2007; Martinis et al., 2014; Zechmann, 2019). Physical connections between PG and the thylakoid membranes suggest bidirectional lipid trafficking between these two compartments (Austin et al., 2006). Besides being a lipid storage site, the PG proteome revealed the presence of specific proteins, several of which are involved in prenylquinone metabolism (Vidi et al., 2006; Ytterberg et al., 2006; Lundquist et al., 2012).
After fibrillins (Lundquist et al., 2012), the second most abundant protein family in PG is composed of homologs of the ABC1 (Activity of BC1 complex) atypical kinases (Lundquist et al., 2012). The ABC1 domain has been conserved through evolution, suggesting that it has a crucial role (Lohscheider and Rio Bartulos, 2016). In microorganisms as well as in human cells, ABC1 proteins were shown to be essential in ubiquinone synthesis and in mitochondrial electron transport (Bousquet et al., 1991; Brasseur et al., 1997; Poon et al., 2000; Mollet et al., 2008). Six members of the ABC1-like kinase family are found in the PG proteome. A member of this family, ABC1K1 (At4g31390), was identified as PGR6 in a genetic screen to identify mutants affected in proton gradient formation (PGR) (Shikanai et al., 1999). PGR mutants are characterized by high chlorophyll fluorescence and reduced NPQ under different light conditions (from 50 to 500 μmol of photons m–2 s–1) (Shikanai et al., 1999; Martinis et al., 2014; Yamori and Shikanai, 2016). In the abc1k1 mutant, the electron transport rate as well as NPQ are constitutively limited in a light fluency dependent manner when compared to the wild type. Under prolonged exposure to high light, abc1k1 adult plants exhibited almost complete photoinhibition during the early days of treatment and after several days PSII maximum efficiency recovered despite the plants still being still exposed to high light (Martinis et al., 2014). Nonetheless, the metabolic profile of abc1k1 was profoundly altered. In particular, plants displayed a decrease in tocopherol accumulation and a shift from starch production to soluble sugars (Martinis et al., 2014).
ABC1K1 was identified as BDR1 (Bleached dwarf under red light) (Huang et al., 2015; Yang et al., 2016). During early seedling development under continuous red light, the mutant is severely stunted and has white cotyledons. Since the bleaching phenotype was accompanied by a specific diminishment of the photosystem D1 (PsbA) protein but not that of other photosynthetic proteins tested [D2 (PsbD); PsbC; PsbB] the pale phenotype was attributed to photobleaching (Huang et al., 2015; Yang et al., 2016). A repressor of the bdr1 mutation, RBD1 (repressor of bdr1) was also identified as the ABC1K1 homolog ABC1K3. As the abc1k3 mutation repressed the bdr1(abc1k1) phenotype, it led to the hypothesis that the two homologs have opposing functions (Huang et al., 2015). Furthermore, abc1k3 adult plants are not severely affected by prolonged high light and they showed a decreased plastochromanol accumulation (Martinis et al., 2013). However, previous investigations on adult plants reported that the double abc1k1/abc1k3 mutation results in an additive, senescence-like phenotype characterized by conditional degreening, including the loss of chlorophyll and photosystem proteins, and recruitment of the jasmonate pathway to PG under prolonged high light treatment (Lundquist et al., 2013). Furthermore, ABC1K1 and ABC1K3 may interact and form a complex that may be involved in the stabilization of plastoglobule proteins (Lundquist et al., 2013; Martinis et al., 2013).
Recently, a molecular mechanism explaining the pgr6(abc1k1) defect was proposed: ABC1K1 would be required for the homeostasis of photoactive PQ. Indeed, upon high light, the photoactive PQ pool in abc1k1 mutant becomes limiting and this can explain the diminished linear electron transport and NPQ, the dephosphorylation of the LHCII antenna and perturbation of the state transitions; all these leading to an overall decrease in the photosynthetic efficiency (Pralon et al., 2019).
In this study, we investigated the impact of the abc1k3 mutation in the pgr6(abc1k1) mutant background. In particular we focused on the capacity of a double mutant, lacking both ABC1K1 and ABC1K3, to acclimate to a short high light treatment (3 h, 500 μmol⋅m–2⋅s–1). We found that the abc1k3 mutant has no photosynthetic defect compared to the wild type under the tested conditions. However, by stacking this mutation with abc1k1 we observe a partial alleviation of all the photosynthetic defects previously reported in abc1k1 (Shikanai et al., 1999; Martinis et al., 2014; Pralon et al., 2019). Surprisingly, the phenotype complementation does not originate from an effect on the size of the photoactive PQ pool but rather from an effect on PQ mobility in the thylakoid membrane. This evidence suggests that there is a push-pull relationship of ABC1K1 and ABC1K3 with regard to the mobility of PQ, and that this regulated process is fundamental to ensure the photosynthetic efficiency under high light.
Materials and Methods
Plants Material and Treatments
The wild type Arabidopsis thaliana refers to var. Columbia-0 (Col-0). abc1k1.1 (Salk_068628), abc1k1.2 (Salk_130499C) abc1k3.1 (Salk_128696), or with abc1k3.2 (Sail_918_E10) T-DNA insertion lines were purchased at Nottingham Arabidopsis Stock Centre (NASC)1. The double mutant lines abc1k1/akc1k3.1 and abc1k1/akc1k3.2 were produced by crossing abc1k1.1 mutant with abc1k3.1 and abc1k3.2, respectively. TDNA insertion was verified by PCR, and primers were listed in Supplementary Table 1. The mutant lines stn7/stn8 (Fristedt et al., 2009) and sps2 (Block et al., 2013) were kindly provided by the respective research groups. The ptox mutant was obtained from NASC, and corresponds to the previously characterized immutans variegation mutant (Wetzel et al., 1994).
Plants were grown in pots on soil pre-treated with solbac (Andermatt) with standard light conditions (120 μmol m–2 s–1, 8 h light/16 h dark) in a controlled environment room maintaining a daily temperature of 22 ± 1°C. For high light treatment, 4–5 weeks old plants were exposed for 3 h to 500 μmol m–2 s–1 of white light (FutureLED), always at 22 ± 1°C.
Leaf samples were collected directly under the light in the growth chamber, and immediately snap frozen in liquid nitrogen, and stored at −20°C.
Photosynthetic Parameters
Before measuring the photosynthetic performance, the plants were kept in the dark for at least 10 min. The measurements were performed using a Fluorcam (Photon System Instrument, Czech Republic)2 with a modified light curve protocol. Briefly, after measuring the minimal fluorescence (F0) and the maximal fluorescence during a saturating pulse (FM) in dark, the plants were exposed to increasing blue light (470 nm) intensities for 1 min following this scheme: 2.5–95–347–610–876–1145 μmol m–2 s–1. At the end of every light period, a saturating flash was used to measure the maximal fluorescence in light (FM’). The fluorescence recorded before the peak were used as FS (steady-state chlorophyll fluorescence in the light). Three parameters were calculated from the above values: Maximum quantum yield of photosystem II ΦMAX = (FV/FM); quantum yield of photosystem II ΦPSII = (FM’ – FS)/FM’ and Non-Photochemical Quenching NPQ = (FM – FM’)/FM’. To assess the extent of the “state transitions” the plants were exposed to 10 min red light (50 μmol m–2 s–1 660 nm peak measured as PPFD) supplemented with far-red (17 μmol m–2 s–1 calculated from the 733 nm peak area considering values between 500 and 800 nm) followed by 10 min of the red light only. The maximal fluorescence in the light was measured at the end of both 10 min periods to obtain FMST1 when far-red was supplemented and “state I” promoted and FMST2 after 10 min of pure red, which promotes the transition toward “state II.” The extent of the quenching related to state transition (qT) was calculated as qT = (FMST1 – FMST2)/FM.
P700 Oxidation
The photooxidation of the photosystem I reaction center (P700) was assessed by the increase in absorption at 810 nm after deconvolution of the plastocyanin absorption as previously described (Joliot and Joliot, 2006). The measurement was performed with a JTS-10 LED spectrometer (BioLogic Science Instruments) in absorbance mode equipped with a Far-red (FAR) LED peaking at 735 nm filtered through three Wratten filters 55 to block the wavelengths shorter than 700 nm, a red LED, peaking at 640 nm, was used for the actinic light. To estimate maximum extent of P700+ a saturating white light flash was superimposed on the top of the FAR. The measurement of the maximum number of electrons contained in the electron transport chain (ETC) per PSI was performed as follows. The plants were pre-incubated 2 min under a strong white light (500 μmol m–2 s–1) to activate CO2 assimilation in the leaves and thus reduce the contribution of the cyclic electron flow (Joliot and Joliot, 2006), which can only be reactivated after prolonged dark incubation (Joliot and Joliot, 2006; Trouillard et al., 2012). This allows only electrons from the linear ETC, including the photoactive PQ pool, to be available for PSI reduction. Detached leaves were put inside the holder and subjected to 2 min of FAR to oxidize the ETC followed by 2 s of dark allowing the reduction of P700. The kinetic of the subsequent reoxidation induced by FAR were followed with a saturating flash of actinic light (1000 μmol m–2 s–1 for 100 μs) to fully reduce the ETC or without so that the ETC was fully oxidized. The ratio between the lag times between the FAR onset and the beginning of the P700 oxidation in these two conditions is used as a proxy for the number of available electrons per PSI (Pralon et al., 2019).
Chlorophyll a Fluorescence Curve Kinetics (OJIP, JIP-Test)
Plants were dark-adapted for at least 10 min before measurements and then a single leaf detached in dark and put in a clip holder for the Plant Efficiency Analyzer (M-PEA 2; Hansatech Ltd.). The chlorophyll a fluorescence induction curve was recorded and the JIP-parameters recovered with M-PEA software Hansatech Ltd. The measurement was automatically repeated after increasing intervals of dark (4, 8, 12, 16, 20, 24 s) and far-red light (0.05, 0.1, 0.2, 0.4, 0.8, 1.6 s). The parameters extracted from the first pulse after the dark incubation were used to assess the steady state of the ETC after moderate light or 3 h of high light (Stirbet et al., 2018). The yield of the electron transport from QA to QB (ΦET2o) and the yield of the transport to final PSI acceptors (ΦRE1o) were calculated according to the JIP-Model described in Strasser et al. (2010) and Kalaji et al. (2014a, b). The variable fluorescence at 3 ms (VJ = FJ/FM) value was used as a proxy of the redox state of the photoactive plastoquinone (Tóth et al., 2007). The data points of VJ over time were interpolated with a logarithmic function with RStudio (RStudio, Inc.).
Plastoquinone Analysis
The analysis of the photoactive PQ pool, and of the total pool were performed as previously described (Pralon et al., 2019). Briefly, leaf disks of 0.8 cm of diameter were collected from 5 weeks old plants. The disks were exposed either to 15 s of saturating light (2000 μmol m–2 s–1) or to 2 min of far-red light (735 nm, 5.5 μmol m–2 s–1). The samples were flash frozen and used for total lipid analysis as described in Kruk and Karpinski (2006) and Ksas et al. (2015, 2018). The photoactive PQ pool was determined from the difference between the reduced PQ after high light (maximal reduction of the photoactive PQ pool) and the amount measured after far-red light (maximal oxidation of the photoactive PQ pool).
Immunoblot Analysis
Fully expanded leaves of adult plants (at least 4 weeks old) were collected under the relevant light condition and flash frozen in liquid nitrogen. The leaves were ground to a fine powder in a 1.5 mL microtube with a micro-pestle. Four hundred mircroliter of lysis buffer [100 mM Tris-HCl pH 8.5, 2% SDS, 10 mM NaF, 0.05% of protease inhibitor cocktail for plant (Sigma)] was add to the powder and the material incubated at 37°C for 30 min, debris were removed by centrifugation (5 min at 16,000 g) at room temperature. The chlorophyll concentration of the sample was determined according to Arnon (1949). Proteins were precipitated in chloroform-methanol and the pellet solubilized directly in the gel loading buffer (50 mM Tris-HCl pH 6.8, 100 mM Dithiothreitol, 2% SDS, 0.1% Bromophenol Blue, 10% Glycerol) to a concentration of 0.5 μg chlorophyll/μL. Following denaturation at 65°C for 10 min, 4 μL of each sample were loaded into a 12% Acrylamide SDS gel. After separation by electrophoresis the proteins were transferred to a nitrocellulose membrane. The membrane was decorated using the following antibodies: anti-Actin (Sigma, A 0480) at 1/3000 dilution in 5% fat free milk/PBS, anti-Lhcb2 (Agrisera, AS01 003), anti-D1 (PsbA) (Agrisera, AS05 084), anti-PetC (Agrisera, AS08 330); anti-PsaD (Agrisera, AS09 461), anti-PsaC (Agrisera, AS04 042P), anti-AtpC (Agrisera, AS08 312); at 1/5000 dilution in 5% fat free milk/TBS, and anti-Phosphothreonine (Cell Signaling Technology, #9381) at 1/10,000 in 3% BSA/TBS Tween20 0.1%; anti-PTOX (Agrisera, AS16 3692) at 1/2000 in 6% BSA/TBS Tween20 0.05%. Secondary antibodies (anti-rabbit (Merck, AP132P) or anti-mouse (Sigma, A5278) at 1/3000) conjugated with HRP allow the detection of proteins of interest with 1 mL of enhanced chemiluminescence and 3.3 μL of H2O2 3% using an imager for chemiluminescence (Amersham Imager 600, Amersham Biosciences, Inc.).
Results
Isolation and Selection of abc1k1/abc1k3 Double Mutants
ABC1K1 and ABC1K3 are two homologous, atypical kinases located at plastoglobules and share 31.6% identity at the level of the global amino acid sequence (Supplementary Figure 1). To analyze potential interactions between ABC1K1 and ABC1K3 in the regulation of photosynthesis, two double mutant abc1k1/abc1k3 lines were produced by crossing abc1k1.1 (Salk_068628) with abc1k3.1 (Salk_128696) or with abc1k3.2 (Sail_918_E10). Double abc1k1/abc1k3 mutant lines were selected and verified by PCR. The genotyping confirmed the presence of TDNA insertions in both genes (Figure 1).
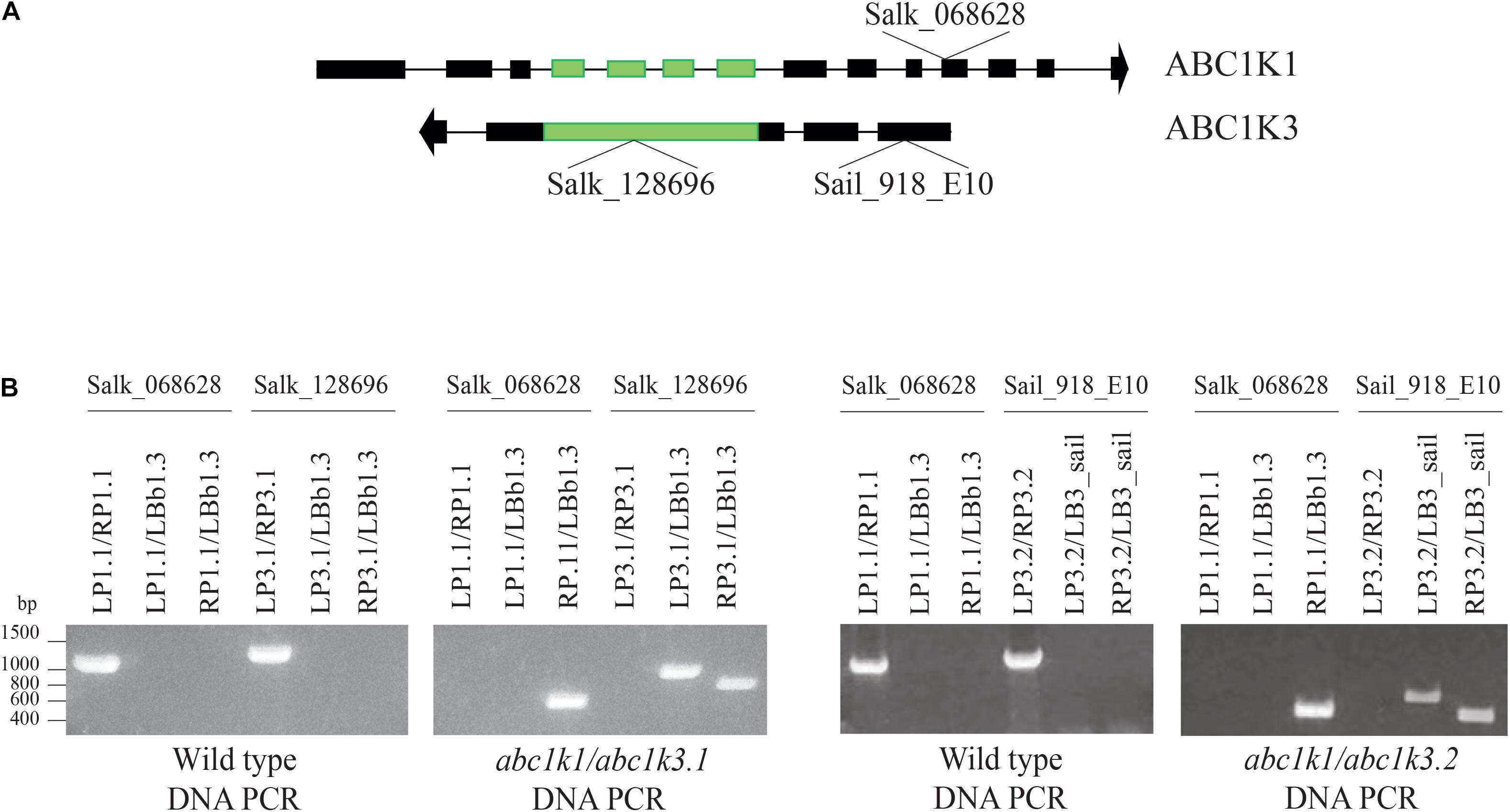
Figure 1. Selection and isolation of abc1k1/abc1k3 double mutant lines. (A) Schematic representation of the position of the T-DNA insertions in ABC1K1 (At4g31390) and ABC1K3 (At1g79600) gene sequences. (B) Electrophoresis of the amplification products obtained from genomic DNA extracted from Wild type, abc1k1/abc1k3.1 and abc1k1/abc1k3.2 confirm the homozygous mutation of both loci. The double mutant abc1k1/abc1k3.1 was obtained from the crossing between abc1k1.1 (SALK_068628) and abc1k3.1 (SALK_128696), while abc1k1/abc1k3.2 is the result of the crossing between abc1k1.1 (SALK_068628) and abc1k3.2 (SAIL_918_E10). The primer used for the amplification are listed in Supplementary Table 1.
Thermal Dissipation and Electron Transport Capacities Are Partially Recovered in abc1k1/abc1k3
Independent studies indicate that abc1k1 is impaired in NPQ as well as electron transport (Shikanai et al., 1999; Martinis et al., 2014; Pralon et al., 2019), while the abc1k3 mutant did not show defects in those parameters even after prolonged high light exposure (Martinis et al., 2013). To further characterize the abc1k1/abc1k3 double mutant, we measured photosynthetic parameters such as PSII maximum efficiency [φMAX (= FV/FM)], NPQ as well as electron capacity of the ETC in 4–5 weeks old plants grown under moderate light (120 μmol m–2 s–1) (ML) and after 3 h of high light (500 μmol m–2 s–1).
The maximum quantum yield of the PSII (φMAX) in wild type (WT), abc1k1, abc1k3, and abc1k1/abc1k3 slightly decreased after 3 h of high light but without any significant difference between the lines (Figure 2A). Furthermore, after 3 h of high light, there was no major impact on the PSII yield, and this allowed the measurement of the efficiency of the ETC avoiding potential bias caused by PSII photodamage.
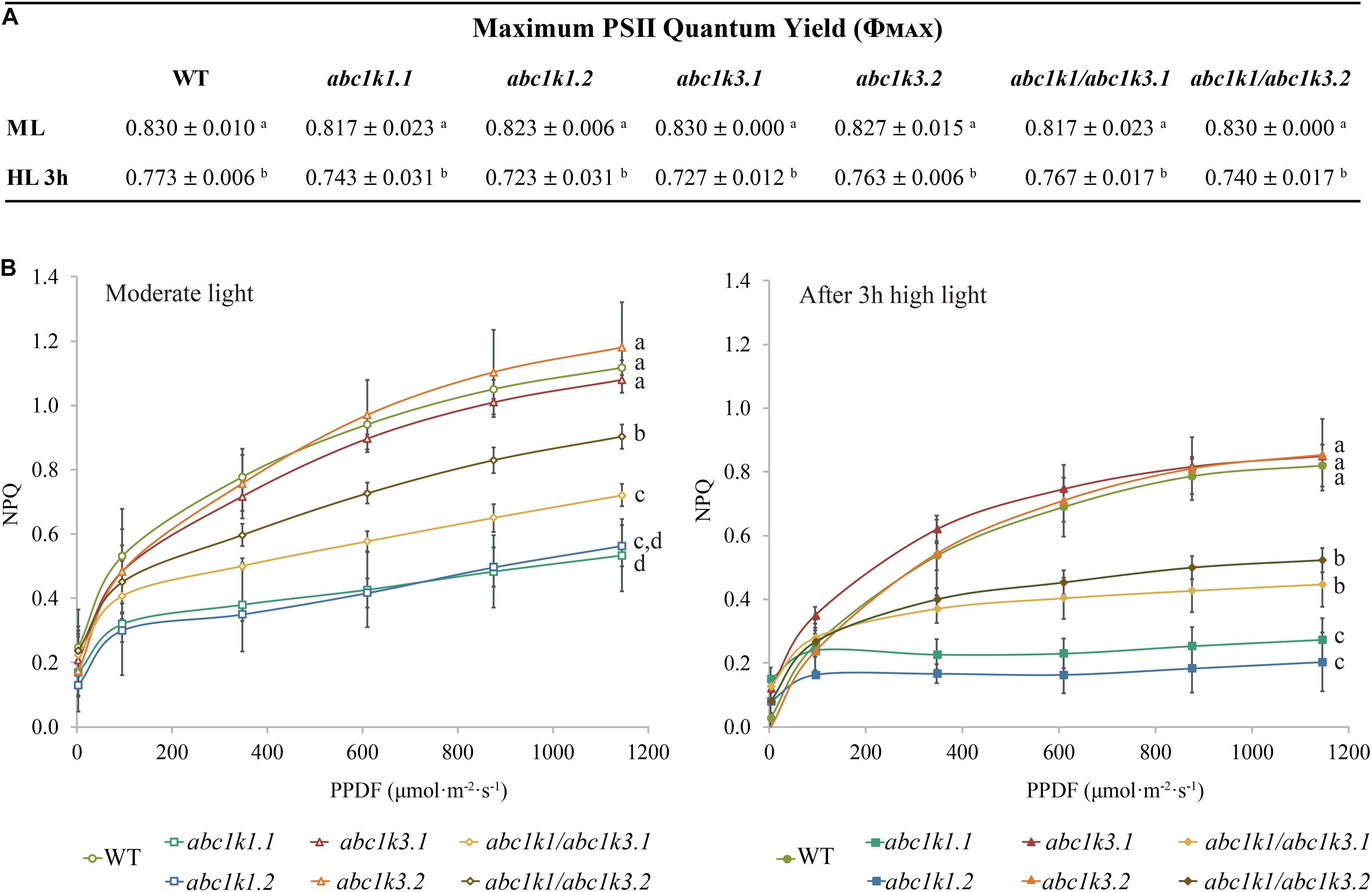
Figure 2. Non-photochemical quenching is partially recovered in abc1k1/abc1k3 lines compared to abc1k1. (A) PSII maximum quantum efficiency (ΦPSII = (FM – FO)/FM) and (B) non-photochemical quenching (NPQ) of 4–5 weeks old plants of wild type (WT), abc1k1.1, -2, abc1k3.1, -2 and abc1k1/abc1k3.1, -2 under moderate light and after 3 h of high light. Plants were dark-adapted 10 min before measurement. Non-photochemical quenching (NPQ = (FM – FM’)/FM’) was calculated from the maximal fluorescence at room temperature recorded after 1 min of exposure at increasing blue light intensities (470 nm). These measures were performed with a Fluorcam (MF800 – PSI). Each value represents the average of a pot containing 2–3 plants. Superscript letters are used to indicate statistically different groups (p < 0.05) by paired Student’s t-test. Error bars indicate ± SD between different pots (n = 3).
Short-term high light treatment affected NPQ induction, which decreased in all the tested genotypes compared to moderate light conditions. Nonetheless, the NPQ in WT and in abc1k3 was always higher than the one measured in abc1k1 lines. Intriguingly, both under moderate light and after high light, the double mutants showed greater NPQ compared to abc1k1 lines but still less than the WT level (Figure 2B), suggesting a partial rescue of the pgr6(abc1k1) phenotype in the double mutant.
Starting from P700 oxidation kinetics (Joliot and Joliot, 2006) and the JIP-test (Strasser et al., 2010; Kalaji et al., 2014a, b), we evaluated the ETC capacity at the PSI and PSII sides under moderate light and after exposure to high light to determine whether the rescued NPQ in abc1k1/abc1k3 correlated with an increase in the electron capacity of the ETC.
P700 oxidation kinetics were analyzed after full reduction of the ETC by a saturating flash and its full oxidation by far-red illumination (Figure 3A; Joliot and Joliot, 2006; Pralon et al., 2019). The maximum number of electrons (e–) present in the electron transport chain per PSI was assessed from P700 oxidation kinetic curves: by dividing the lag time after a strong light pulse (time required to oxidize P700 when ETC is fully reduced) by the lag time after far-red exposure (oxidation time of a single electron present in P700 reaction center) (Joliot and Joliot, 2006). When sampled under moderate light, abc1k3 and WT ETC had the same electron capacity per PSI (20 ± 3 and 20 ± 4 electrons respectively), whereas the abc1k1 ETC carried fewer electrons per PSI (8 ± 1 electrons) (Figure 3B). In the abc1k1/abc1k3 mutant grown under moderate light the number of electrons carried by the ETC per PSI (16 ± 5) was intermediate between abc1k1 and the WT. The measure was repeated on plants kept 3 h under high light. After high light treatment, in the WT the number of electrons carried by the ETC per PSI was 13 ± 3, thus it decreased in comparison to the same genotype sampled under moderate light. Similarly, in abc1k1 as well as abc1k1/abc1k3 the number of electrons per PSI dropped after high light treatment to 4 ± 2 and 8 ± 1 respectively. The measured electron transport capacity in abc1k1/abc1k3 was double than that in abc1k1, indicating partial rescue of the ETC capacity. The abc1k3 mutant displayed only a very slight decrease in the number of the ETC carriers per PSI (18 ± 4) upon shifting from moderate light to high light for 3 h, thus more ETC carriers than WT in this condition (Figure 3B).
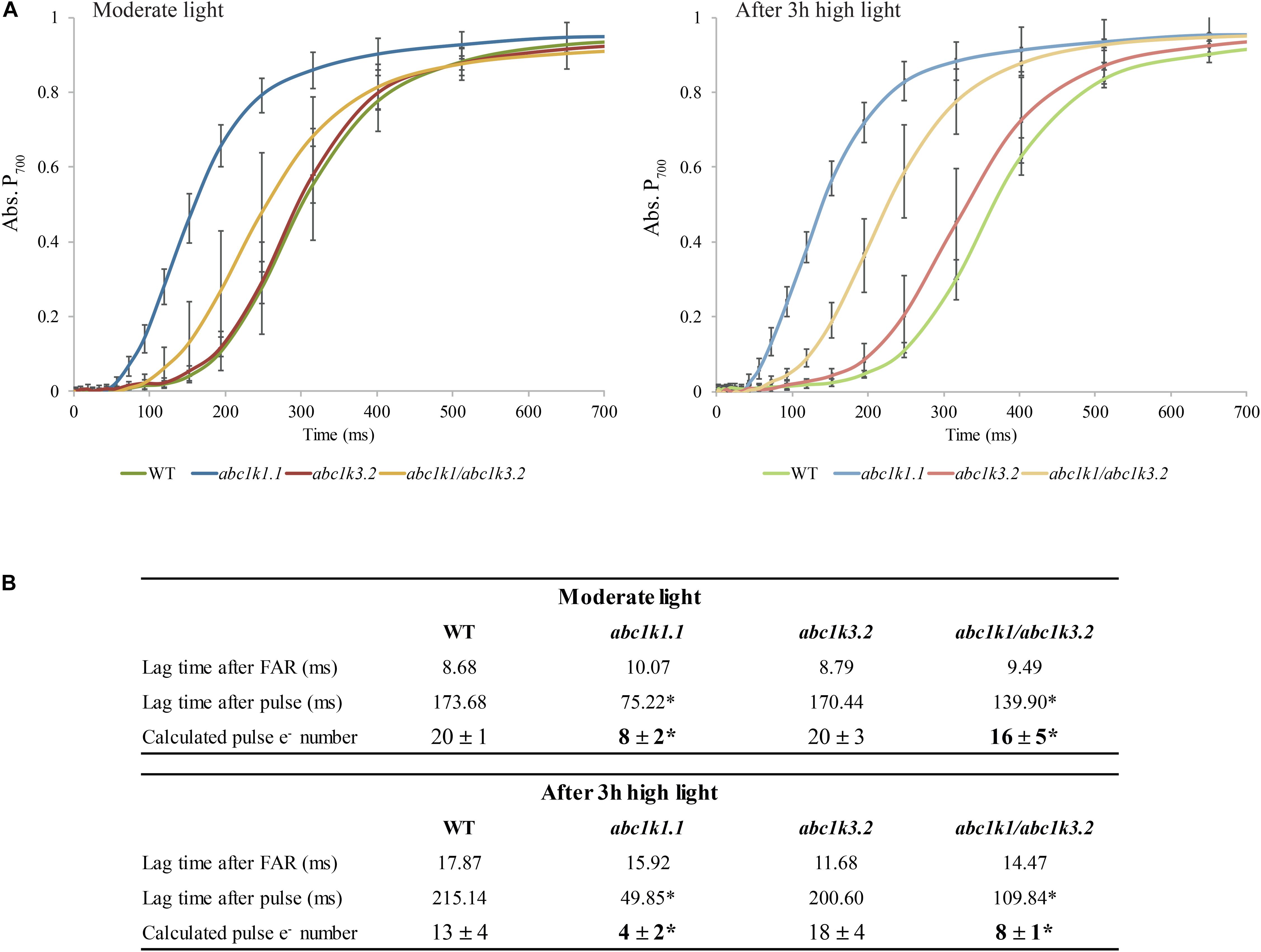
Figure 3. The shortage of electron carriers per photosystem I of abc1k1 is partially recovered in abc1k1/abc1k3. (A) Kinetics of P700 re-oxidation induced by far-red light were recorded after a saturating light pulse (Time 0 = far-red ON) in wild type, abc1k1.1, abc1k3.2, and abc1k1/abc1k3.2 kept under moderate light and after 3 h of high light. The P700 oxidation status was measured by the increase in absorption at 810 nm on fully expanded leaves of each genotype. (B) Electron (e–) carried by the electron transport chain per photosystem I calculated from the lag time of P700 oxidation after a saturating pulse divided by the lag time of the oxidation after dark. Asterisk are used to indicate statistically different groups by Student’s t-test (p < 0.05) (n = 4 biologically independent samples).
The ETC capacity, before and after the high light treatment, was also estimated using fast chlorophyll a fluorescence (JIP-test) by normalizing the area above curve over variable fluorescence [Area/(FM-FO)] (Strasser et al., 2010; Kalaji et al., 2014a, b). This area positively correlates with the number of turnovers of the QA site of PSII before being fully closed. Since each turnover corresponds to a single electron injected in the ETC, the area offers a proxy of the number of available electron acceptors per PSII. These acceptors are internal to PSII, pheophytin and QA, or external, PQ molecules of the photoactive plastoquinone pool, the cyt b6f complex and plastocyanin. Under moderate light, the electron capacity estimated by the normalized area, was bigger in abc1k3 than in WT (17 ± 2), while it was smaller in both abc1k1 lines (15 ± 1; 14 ± 1). In the two abc1k1/abc1k3 lines the estimated electron capacity (18 ± 2; 20 ± 3) was comparable to WT (Figure 4A). A second measurement was performed on leaves sampled after 3 h of high light, in this sample, compared to the moderate light condition, the availability of the electron acceptors per PSII remained essentially unchanged in WT (19 ± 2) and in both abc1k1/abc1k3 lines (19 ± 2; 18 ± 3). In the two abc1k1 lines, after high light treatment, the electron transport capacity was diminished (13 ± 1; 11 ± 2) compared to the moderate light conditions. In contrast, both abc1k3 lines showed a tendency toward an increase in electron carriers (22 ± 2; 24 ± 3) when comparing leaves sampled after 3 h of high light with those harvested in moderate light condition (Figure 4A).
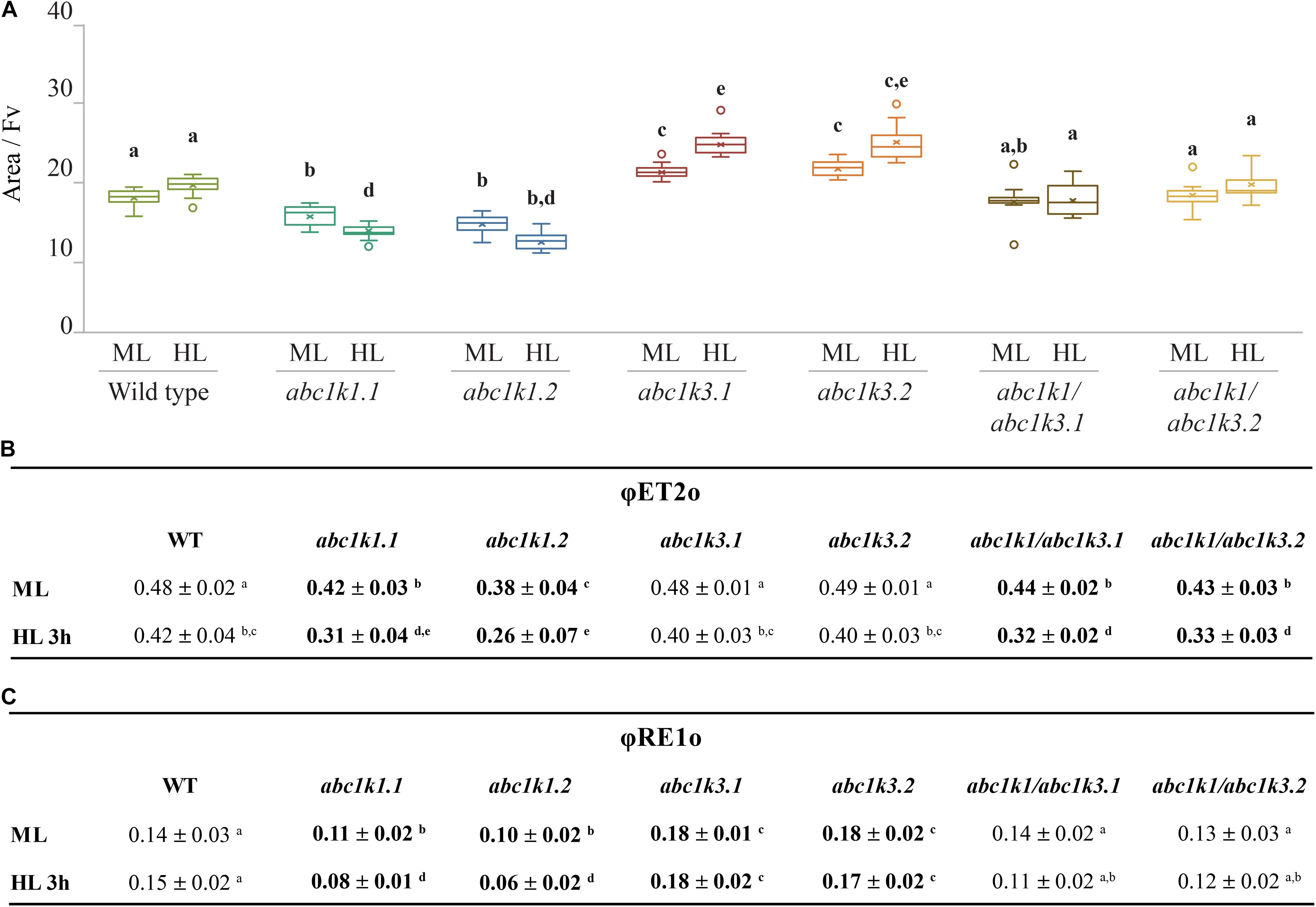
Figure 4. High light as a moderate impact on photosynthetic electron transport fluxes in abc1k1/abc1k3. Fully expanded leaves from Wild type (WT), abc1k1.1, -2, abc1k3.1, -2, and abc1k1/abc1k3.1, -2 plants were collected under moderate light conditions (120 μmol of photons m–2 s–1) (ML) and after 3 h of high light (500 μmol of photons m–2 s–1) (HL). (A) Normalized area (Area/FV) above the chlorophyll fluorescence induction curve measured after 15 min of dark incubation. This value estimates the number of available electron carriers per PSII reaction center. (B) Quantum electron transport yield from QA to PQ pool (ΦET2o), and (C) until the PSI electron acceptors (ΦRE1o). These measures were performed with Handy-PEA (Hansatech Instruments). Whiskers and box plot shows the minimum, first quartile, median, average, third quartile, and maximum of each data set (n = 12 biologically independent samples). Superscript letters are used to indicate statistically different groups by paired Student’s t-test (p < 0.05).
To obtain an indication of the impact of the mutations on different components of the electron transport chain, we calculated the quantum yields of the electron transport flux from QA– to PQ (ΦET2o) and to the PSI electron acceptors (ΦRE1o) by analyzing chlorophyll fluorescence inductions of JIP-curves at 3 ms (FJ) and 30 ms (FI).
In leaves collected under moderate light, ΦET2o was lower in abc1k1/abc1k3 and abc1k1 compared to WT and abc1k3. After challenging the plants with 3 h of high light, the measurement revealed that ΦET2o dropped in all lines, with the most severe decrease in abc1k1 lines followed by abc1k1/abc1k3 (Figure 4B).
The quantum yield of electron transport to PSI final acceptors (ΦRE1o), in plants maintained under moderate light, was similar in WT and abc1k1/abc1k3, lower in abc1k1 and higher in abc1k3. After 3 h of high light, ΦRE1o in abc1k1diminished even further, while it remained essentially unchanged in abc1k1/abc1k3 when compared to moderate light. Therefore, abc1k1/abc1k3 quantum yield was comparable to the WT in both light condition. For this parameter, the double mutation appears to partially attenuate the photosynthetic defects due to the abc1k1 mutation. Finally, after high light, ΦRE1o in abc1k3 did not change compared to moderate light, thus being always higher than in the WT (Figure 4C).
To verify whether the recovery of photosynthetic parameters in abc1k1/abc1k3 as well as the higher photosynthetic capacities measured in abc1k3, were due to an increased cytochrome b6f activity, the turnover rate was measured (Finazzi et al., 2002). The measurement showed that there was no negative impact on the level of activity among all the tested lines (Supplementary Figure 2).
Together, these results indicate that the mutation of abc1k3 has no negative impact the electron transport between the photosynthetic complexes, since the transport efficiency in this mutant line was elevated also after exposure to high light. Conversely, the electron transport in abc1k1 was impaired in the moderate light condition and worsened upon exposure of the plants to 3 h of high light. The double mutation of abc1k1 and abc1k3 resulted in a partial recovery of the electron transport and NPQ to WT levels when compared to abc1k1.
Mutation of abc1k3 Has No Effect on the Photoactive PQ Pool Size
The level of NPQ and the electron acceptor capacity of the ETC may be related to the size of the photoactive PQ pool (Block et al., 2013; Pralon et al., 2019). Therefore, we examined whether the partial rescue of the electron transport and NPQ capacities in abc1k1/abc1k3 compared to abc1k1 can be attributed to the size of the photoactive plastoquinone pool (i.e., the number of PQ molecules readily available per PSII). For this, we measured the total PQ (nmol cm–2) and the relative photoactive PQ pool (in% of total PQ pool) in 4–5 weeks old plants under moderate light and after 3 h of high light exposure. The photoactive PQ pool is defined as the fraction of total PQ that is rapidly reduced during a saturating light pulse and oxidized when the sample is exposed to far-red light. To measure this fraction, PQH2 and PQ amounts were analyzed by HPLC-MS on leaves illuminated either with a strong light flash in order to completely reduce the photoactive PQ pool, or with far-red to obtain its complete oxidation (Kruk and Karpinski, 2006; Block et al., 2013; Ksas et al., 2018; Pralon et al., 2019). The difference between the amount of reduced PQ after the saturating flash and that measured after far-red illumination determines the photoactive PQ pool.
Total plastoquinone levels (photoactive PQ pool + non-photoactive PQ pool) in abc1k1 and in abc1k1/abc1k3 lines were similar when compared to WT and decreased only slightly after 3 h of high light exposure. Conversely, the total PQ level was lower in abc1k3 compared to WT (Figure 5A). The photoactive PQ pool (photoactive PQ/total PQ) measured in abc1k3 was larger under moderate light condition and identical to the WT after 3 h of high light (Figure 5B). Whereas, in abc1k1 after 3 h of high light, the photoactive PQ pool was strongly diminished compared to WT. Although the double mutant was not severely impaired in either ETC capacity or NPQ (Figures 2, 4), the relative photoactive PQ pool, measured in plants grown under moderate light, in abc1k1/abc1k3 was unexpectedly small and similar to the one of abc1k1. High light had no negative effect on the size of the photoactive PQ pool in the abc1k1/abc1k3 double mutant. However, after 3 h of high light, its photoactive PQ pool size was smaller than that of the WT or abc1k3, and comparable to abc1k1 (Figure 5B).
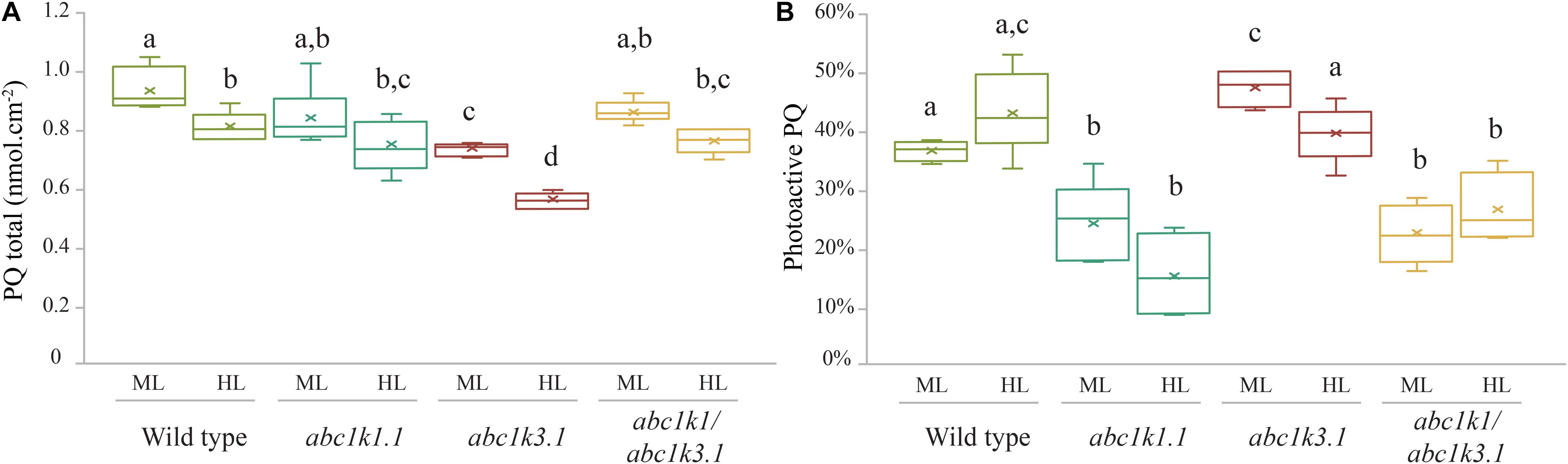
Figure 5. Photoactive PQ pool is smaller in all the lines containing the abc1k1 mutation. (A) Total plastoquinone content (nmol cm–2) and (B) photoactive plastoquinone pool (as% of total PQ) were analyzed from leaves of Wild type, abc1k1.1, abc1k3.2, and abc1k1/abc1k3.2 under moderate light and after 3 h high light. The photoactive plastoquinone pool was determined by the difference of the plastoquinol (PQH2) amount measured when the PQ pool is maximally reduced by strong white light pulse (15 s at 2,000 m–2 s–1), and the amount of PQH2 measured when the PQ pool is fully oxidized after far-red illumination (2 min at 5.5 μmol of photons m–2 s–1). Whiskers and box plot shows the minimum, first quartile, median, average, third quartile, and maximum of each data set (n = 4 biologically independent samples). Superscript letters are used to indicate statistically different groups by paired Student’s t-test (p < 0.05).
The analysis of the photoactive PQ pool suggests that the complementation of the pgr6(abc1k1) photosynthetic phenotype, induced by the abc1k3 mutation, does not simply arise from a change of the amount of PQ readily available at PSII.
Mutation of ABC1K1 and ABC1K3 Impacts the Kinetics of PQ Re-oxidation in the Dark
The redox state of PQ in the light is dependent on the activity of the PSII, which will reduce the photoactive pool, and on that of the cytochrome b6f, which oxidizes the PQ pool transferring electrons along the ETC toward PSI. However, the photosynthetic complexes are inactive in the dark and therefore the redox state of PQ is mostly dependent on light-independent electron routes alternative to the cytochrome b6f. In the transition from light to dark the photoactive PQ pool will tend to start in a reduced form and be re-oxidized. The principal actor of this re-oxidation is PTOX. This enzyme, in Arabidopsis, is mostly located in the stroma lamellae fraction of the thylakoid membrane (Joet et al., 2002; Lennon et al., 2003; Houyoux et al., 2011). PSII being more abundant in the grana stacks, and considering the timescale of the mobility of PQ (Kirchhoff, 2014), we may assume that a large portion of the photoactive PQ pool is located within the grana stacks. Therefore, in order to be oxidized by PTOX the photoactive PQ has to migrate from the grana stacks to the stroma lamellae and then return to the grana stacks. Considering this, the kinetics of the oxidation of the photoactive PQ pool represents a proxy of the mobility of the PQ across the different portions of the thylakoid membrane. To estimate the redox state of the photoactive PQ pool we used the rapid chlorophyll a fluorescence induction, we based the analysis on the relative fluorescence at 3 ms (VJ). It has been shown that the fluorescence recorded at this time interval correlates with the redox state of the photoactive PQ pool (Strasser et al., 2010; Kalaji et al., 2014a). A high VJ value is recorded in samples where the photoactive PQ pool is mostly reduced, and it decreases with its oxidation (Tóth et al., 2007). The chlorophyll a fluorescence induction was measured at increasing time intervals of dark incubation after a saturating light pulse. The results show that the measured oxidation, which appears to be almost completely PTOX dependent, is faster in the abc1k3 mutants, while severely impaired in abc1k1 (Figure 6A).
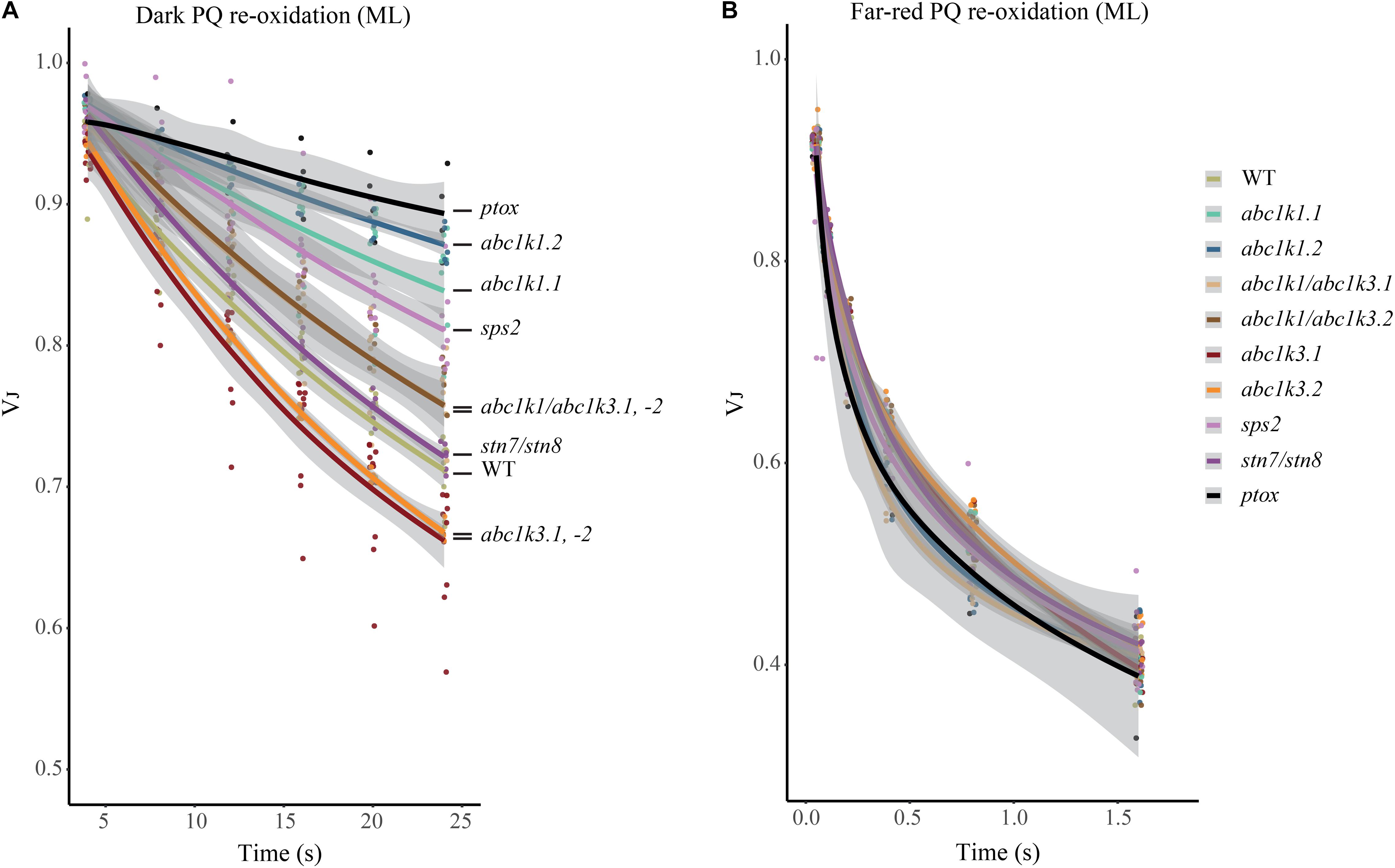
Figure 6. Mutations of ABC1K1 and ABC1K3 influence the dark re-oxidation kinetic of the photoactive plastoquinone. The relative fluorescence after 3 ms (VJ) is plotted over the time from the previous saturating pulse. The sample was incubated in dark (A) or with far-red light (B). Kinetics of the re-oxidation are shown by interpolation of the data points with a logarithmic curve with the deviation of the model in gray (R-Studio) for wild type (WT) abc1k1.1, -2, abc1k3.1, -2, and abc1k1/abc1k3.1, -2, sps2, stn7/stn8, ptox plants grown under moderate light (ML). These measures were performed with Handy-PEA (Hansatech Instruments) on detached leaves incubated for 10 min in dark.
A limitation of total PQ, as in the sps2 mutant (Block et al., 2013; Pralon et al., 2019), resulted in a slower oxidation in the dark as well. The PQ oxidation in the abc1k1/abc1k3 double mutant progressed more rapidly than in the abc1k1 single mutant (Figure 6A). The dark re-oxidation of the photoactive PQ pool is independent on the photosynthetic ETC. In fact, all the tested mutant lines displayed the same kinetics when the PQ oxidation was performed by the cytochrome b6f, as observed when PSI was excited with far-red light (Figure 6B). The kinetics of PQ oxidation in the dark may be influenced by the level of accumulation of the PTOX protein (Ivanov et al., 2012). We therefore used immunodetection to test the PTOX accumulation in total protein samples from the different mutants. The result showed no differences at the protein level, which appeared to be uniform among the lines (Supplementary Figure 3). After 3 h under high light the dark re-oxidation of the photoactive PQ in the abc1k1 mutant is almost completely blocked, and it is slower overall in all lines analyzed. Once again, the defect was milder in the abc1k1/abc1k3 double mutant (Supplementary Figure 4). It is worth noting that after 3 h of high light the oxidation kinetics under far-red light were also affected in abc1k1, suggesting that 3 h of high light exposure induce a perturbation of the ETC, consistently with the previous report (Pralon et al., 2019).
This experiment shows that abc1k1 is impaired in the regulation of the photoactive PQ redox state independently of the activity of the ETC. Considering the specific localization and identical protein levels of PTOX, this supports a model of limited mobility of PQ. Interestingly, said defect is partially complemented by abc1k3 mutation.
Major Thylakoid Membrane Protein Phosphorylation and State Transitions Are Partially Restored in abc1k1/abc1k3
A smaller photoactive PQ pool (Figure 5B) should be prone to over-reduction or at least to “mimic” a condition of over-reduction as fewer PQ molecules are available (Figure 4B). This would be expected to perturb state transitions (Bellafiore et al., 2005; Shapiguzov et al., 2010, 2016; Tikkanen et al., 2012; Trotta et al., 2016; Pralon et al., 2019). Cytochrome b6f activity is dependent on the redox state of PQ and regulates the activation of STN7, the principal kinase involved in LHCII phosphorylation. Therefore, we evaluated the phosphorylation status of the major thylakoid membrane proteins by immunodetection. Moreover, we measured the re-allocation of the mobile light harvesting complex II (LHCII) between the two photosystems by room temperature chlorophyll fluorescence in abc1k1/abc1k3 during state 1 to state 2 transition induced by changes in the light spectrum. Both approaches were used as proxies to assess the redox state of the PQ pool in vivo.
The phosphorylation status of the thylakoid protein was assessed by anti-phosphothreonine immunoblotting on total protein extracts from leaves collected under moderate light and after 3 h high light exposure. Under moderate light, the thylakoid phosphoprotein pattern was similar among all the lines tested (Figure 7A). Compared to the moderate light, after 3 h of high light exposure PSII core proteins D1 (PsbA) and D2 (PsbD) were only slightly more phosphorylated in abc1k1, while their phosphorylation increased markedly in WT, abc1k3 as well as in abc1k1/abc1k3. Similarly, after the high light stress, the LHCII proteins were highly phosphorylated in abc1k3, abc1k1/abc1k3, and WT, while the LHCII phosphorylation was clearly lower in abc1k1 (Figure 7A). This suggests that, despite the shortage of photoactive PQ in abc1k1/abc1k3 (Figure 5B), the STN7 kinase maintains its activity toward LHCII even after exposure to high light. Furthermore, neither the change in total PQ nor in the photoactive fraction had an impact on the abundance of selected photosynthetic proteins after 3 h of high light (Figure 7B).
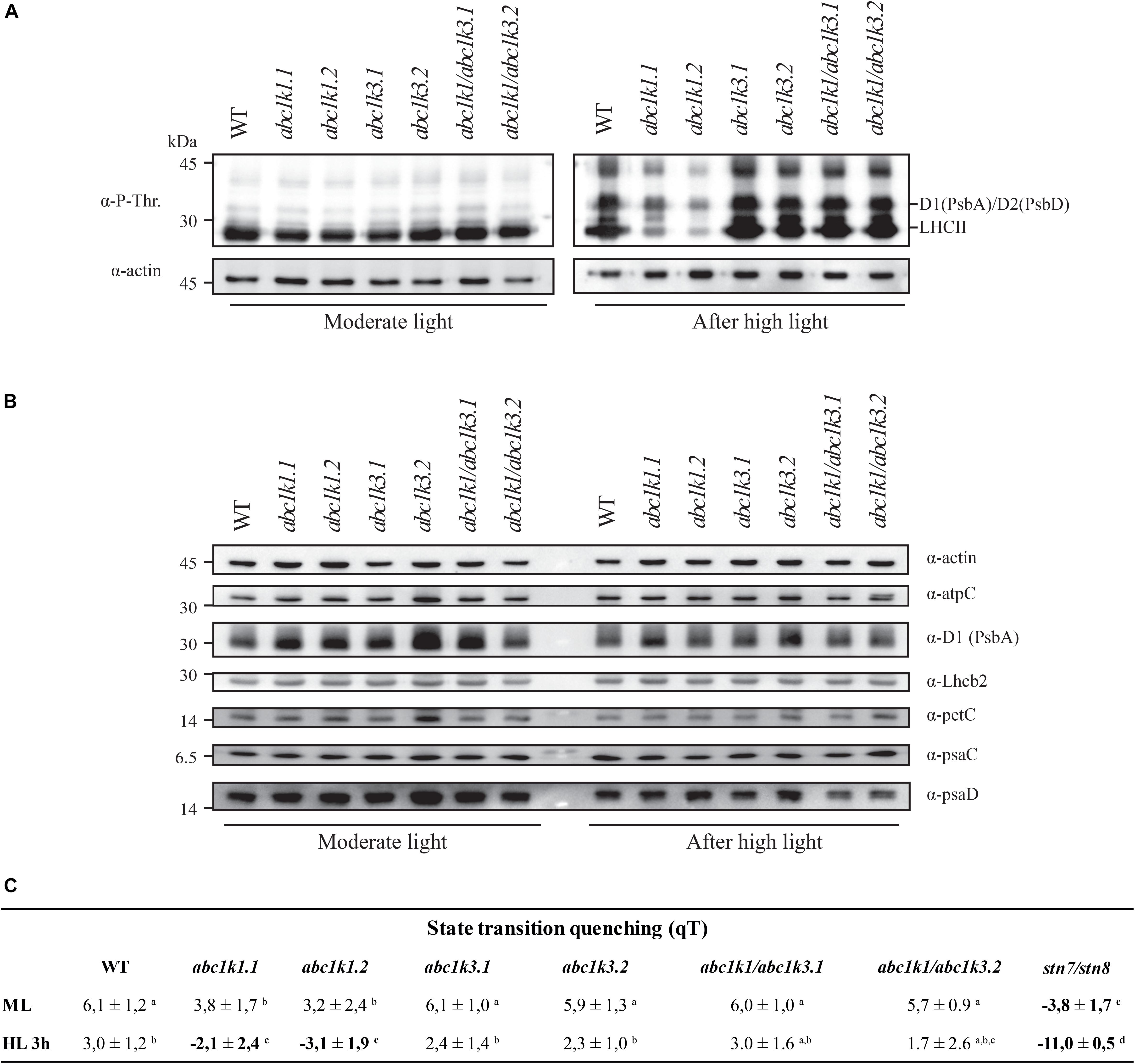
Figure 7. Double mutant maintains thylakoid protein phosphorylation and state transitions after high light. (A) Total protein extracts of wild type (WT), abc1k1.1, -2, abc1k3.1, -2, and abc1k1/abc1k3.1, -2 light-exposed leaves were separated by SDS PAGE, transferred on nitrocellulose membrane and decorated with anti-phosphothreonine antibody. The main thylakoid phospho-proteins are indicated on the right according to their size. Core photosystem II proteins D1 (PsbA) and D2 (PsbD) are indicated together due their poor resolution. (B) The accumulation of the principal photosynthetic complexes was assessed using antibodies against specific subunits of each complex: anti-Lhcb2 for the major LHCII, anti-D1 (PsbA) for PSII, anti-PetC for cytochrome b6f, anti-PsaD and anti-PsaC for PSI, and anti-AtpC for ATP synthase. Actin signal is shown as a loading control. (C) Fluorescence quenching related to the state transitions (qT) of wild type (WT), abc1k1.1, -2, abc1k3.1, -2, and abc1k1/abc1k3.1, -2 under moderate light (120 μmol of photons m–2 s–1) (ML) and after 3 h of high light (500 μmol of photons m–2 s–1) (HL). qT was calculated from the maximal chlorophyll fluorescence measured after 10 min exposure to red light (660 nm) supplemented with far-red illumination (720 nm) “State 1” (FMST1) or to pure red light “State 2” (FMST2). Quenching related to state transition was calculated as qT = (FMST1 – FMST2)/FM. Each value represents the average of a pot containing 2–3 plants. Superscript letters are used to indicate statistically different groups (p < 0.05) by paired Student’s t-test.
To determine whether thylakoid protein phosphorylation observed in abc1k1/abc1k3 correlated with the ability to perform state transitions we followed and measured room temperature chlorophyll a fluorescence kinetics on dark-adapted plants by switching red light supplemented with far-red light (State 1) to red light only (State 2). The quenching (fluorescence decline) caused by state transitions (qT), was calculated as the difference between the maximal fluorescence (FM) after “State 1” illumination (FM_ST1) and the one after “State 2” light (FM_ST2) normalized on maximal fluorescence (FM) (qT = (FM_ST1 – FM_ST2)/FM), which reflects the dissociation of antenna from PSII and its association with PSI.
Under moderate light, the qT in all genotypes was comparable to the WT, indicating their ability to perform transition from State 1 to State 2 and only abc1k1 lines appeared to be slightly impaired (Figure 7C). stn7/stn8, which is completely unable to perform state transitions, was used as a negative control. After 3 h of high light exposure, abc1k3 and WT maintained their capacity to perform state transitions, while abc1k1 lines were defective in state transitions. After 3 h of high light, state transitions in abc1k1/abc1k3 lines exhibited a level of quenching comparable to WT and abc1k3 (Figure 7C). This shows that LHCII phosphorylation, and thus the activity of the STN7 kinase, which is maintained in abc1k1/abc1k3 mutants, allows the state transitions after high light exposure.
Discussion
The photosynthetic apparatus has to adapt to photo-oxidative stress induced by excess light in order to prevent thylakoid membrane damage and maintain photosynthetic efficiency. Photo-protective strategies comprise adjustment of electron transport capacity (ETC) (Rochaix, 2011), equilibration of energy between photosystems (state transitions) (Rochaix, 2007) and induction of NPQ (Müller et al., 2001; Ruban, 2016). All three mechanisms are directly or indirectly related to the activity of the plastoquinone as an electron carrier. Recently, it has been demonstrated that ABC1K1 is implicated in photosynthesis regulation by homeostasis of photoactive PQ under high light (Pralon et al., 2019). In this study, we describe the involvement of ABC1K3 (Lundquist et al., 2013; Martinis et al., 2013; Huang et al., 2015), a close homolog of ABC1K1, in the same process.
We tested two abc1k3 mutant lines for their ability to induce NPQ under increasing light intensities. Contrary to abc1k1, this mutation did not cause any perturbation in NPQ induction when compared with the WT (Figure 2). A previous report shows that, during several days of exposure to high light (500 μmol of photons m–2 s–1), abc1k3 had a tendency to induce slightly less NPQ compared to WT, however this difference was not statistically significant nor constant over the time course (Martinis et al., 2013). This suggests that the NPQ parameter alone is not sufficient to discriminate the photosynthetic adaptation of abc1k3 from WT. In fact, the differences between WT and abc1k3 are significant only when the photosynthetic ETC capacity is analyzed in detail (Figures 3, 4). To further address the role of abc1k3 in the photosynthetic regulation, we crossed this mutant with abc1k1 to obtain abc1k1/abc1k3 double mutant plants (Figure 1). The abc1k3 mutation was capable to partially alleviate the NPQ defect observed in abc1k1; while the NPQ level in abc1k1/abc1k3 was higher than in the single abc1k1 mutant, it remained lower than in the WT (Figure 2).
In order to confirm that the NPQ perturbation is due to a lack of transport through the ETC, we measured the number of electrons transported per PSI after a saturating light pulse by analyzing the lag time of PSI oxidation. As expected from previous reports (Shikanai et al., 1999; Martinis et al., 2014; Pralon et al., 2019), abc1k1 transfers less electrons to PSI, and the difference compared to the WT increases after exposure to 3 h high light. On the contrary, abc1k3 was not impaired and appeared capable of maintaining a high level of electron transfer after 3 h of high light (Figure 3). In abc1k1/abc1k3, the electron transport capacity was still lower than the WT, however, more electrons were transferred per PSI compared to abc1k1 and the decrease after 3 h of high light was comparable to the one observed in the WT (Figure 3). This finding suggests that the defect in the photosynthetic electron carriers of abc1k1 was partially rescued in abc1k1/abc1k3, presumably by increasing the efficiency of the electron transfer in the ETC. In a previous report, the limitation of the electron transport capacity observed in abc1k1 was linked to a depletion of the photoactive PQ pool (Pralon et al., 2019). However, the decrease in the number of electrons transported to PSI appeared to be more severe than the measured decrease in the size of the photoactive PQ pool. Therefore, it was hypothesized that the PQ mobility in the ETC plays an additional role in limiting the electron transport capacity. To investigate this hypothesis the energy fluxes along the ETC were analyzed by rapid fluorescence induction curves. The estimation of the number of electrons present in the ETC before saturation, expressed as the Area/FV (Figure 4A), was consistent with the P700 oxidation analysis (Figure 3). In fact, already under moderate light the normalized area was smaller in abc1k1 mutant and larger in abc1k3 in comparison to WT, consistent with abc1k1 having limited electron transport and abc1k3 having more carriers than the WT. In this condition, the abc1k1/abc1k3 double mutant had an Area/FV value in between those of the two single mutants, suggesting once again, a partial recovery of the photosynthetic electron transport. Upon exposure to high light only the abc1k1 mutant showed a decrease in the number of available carriers; while all the other lines had a tendency to increase the Area/Fv value, compared to moderate light, indicating a better, or at least unchanged, electron transport capacity (Figure 4A). By analyzing the induction curve’s principal steps, we can obtain hints regarding the different components that may be affected in the ETC. The first step, at 3 ms from the start of the saturating flash, was reported to be dependent of the QB redox state at the PSII and therefore linked to the redox state of the photoactive PQ pool (Tóth et al., 2007), which is also affected by the size of the photoactive PQ pool (Pralon et al., 2019). From the fluorescence value at 3 ms (FJ) it is possible to calculate the ΦET2o, the efficiency of the electron transport between QA and QB (which depends on the status of the photoactive PQ pool) (Strasser et al., 2010; Kalaji et al., 2014a, b). We observed that the ΦET2o is lower both in abc1k1 and in abc1k1/abc1k3, suggesting a similar defect in the photoactive PQ pool. However, the defect appears to be somewhat milder in the double mutant compared to abc1k1 (Figure 4B). The second step in the fluorescence rise occurs at 30 ms (FI), and the level of the fluorescence recorded at this step is linked to the electron transport to the PSI final acceptors in the OJIP model (Strasser et al., 2010; Kalaji et al., 2014a, b). From the FI value is also possible to calculate the electron transport efficiency, defined as ΦRE1o. Comparison of this efficiency revealed that abc1k1 has a lower efficiency compared to the WT but that was not the case for abc1k1/abc1k3. In the latter, the ΦRE1o was the same as in the WT in moderate light, and was less affected upon the exposure to 3 h high light compared to abc1k1 (Figure 4C). The abc1k3 single mutation did not create any measurable defect in electron transport. On the contrary, the efficiency of the transport to PSI acceptors appeared to be even higher than in the WT, both under moderate light and after exposure to high light (Figure 4C). This suggests that the mutation of the ABC1K3 gene leads to an increased efficiency in the electron mobility between PSII and PSI.
To support the fluorescence induction results we biochemically measured the size of the photoactive pool in the mutants exposed to moderate light and to high light. Consistent with the biophysical observations, the photoactive PQ pool was smaller in both abc1k1 and abc1k1/abc1k3 compared to the WT in moderate light condition (Figure 5B). Exposure to 3 h of high light had a limited effect on the size of the photoactive PQ pool in the four tested lines (Figure 5B). However, while the WT and the double abc1k1/abc1k3 mutant display a tendency toward the increase of the photoactive PQ after 3 h of high light, we detected a slight decrease of the photoactive PQ pool in abc1k1 as previously reported (Pralon et al., 2019). The depletion observed in this report was not significant as it was in the previous report, this may suggest that the photoactive PQ pool homeostasis relies on multiple factors (e.g., thylakoid organization, lipid distribution) and that ABC1K1, despite its prominent role, is not the only factor regulating the photoactive PQ pool size. A similar depletion of the photoactive PQ pool after 3 h of high light, was observed in abc1k3 even though the relative photoactive PQ pool size was still larger than in abc1k1, and comparable to the WT (Figure 5B). On the contrary, the abc1k1/abc1k3 double mutant constitutively displayed a small photoactive PQ pool that was not depleted by the exposure to high light (Figure 5B). This leads to the conclusion that the photoactive PQ pool size per se has a limited influence on photosynthetic efficiency, and that the photosynthetic defect becomes symptomatic only when PQ limitation is associated with an additional impairment in its mobility. Impaired PQ mobility may be the cause affecting the reoxidation of the photoactive PQ by PTOX, since it may require an exchange between grana stacks and stroma lamellae (Figure 6A; Stepien and Johnson, 2018). Furthermore, it would affect the exchange between the photoactive pool and the reservoir stored in the PGs necessary to maintain the photoactive PQ pool size (Pralon et al., 2019). We cannot exclude that the defect in the mobility and exchange between the different PQ pools stems from a defect in the thylakoid membrane composition. In fact, it has been previously reported that the mutation of abc1k1 results in a lower amount of several prenyl lipids (Martinis et al., 2014) compared to the WT under control growth conditions (150 μmol of photons m–2 s–1) as well as after several days of growth in high light (500 μmol of photons m–2 s–1). However, the abc1k3 mutant has a similar defect in the level of accumulation of these lipophilic compounds (Martinis et al., 2013). Therefore, no obvious correlation between the photosynthetic defect and the amount of the principal chloroplast lipids can be drawn at this stage.
A lower amount of total PQ, which decreased after 3 h of high light, was observed in abc1k3 (Figure 5A). This decrease may be an indirect effect of the increased photosynthetic electron transport observed in abc1k3 compared to WT. A possible explanation is that the amount or the redox state of PQ in the photoactive pool act as a signal to regulate PQ biosynthesis. This is supported also by previous reports showing a correlation between PQ biosynthesis and increase in light intensity (Zbierzak et al., 2010; Ksas et al., 2015). Consistent with this hypothesis, the increased relative PQ accumulation in the photoactive pool, observed in the abc1k3 mutant under moderate light (Figure 5B), would alter this signal and therefore limit the biosynthesis resulting in a lower amount of total PQ (Figures 5A, 8).
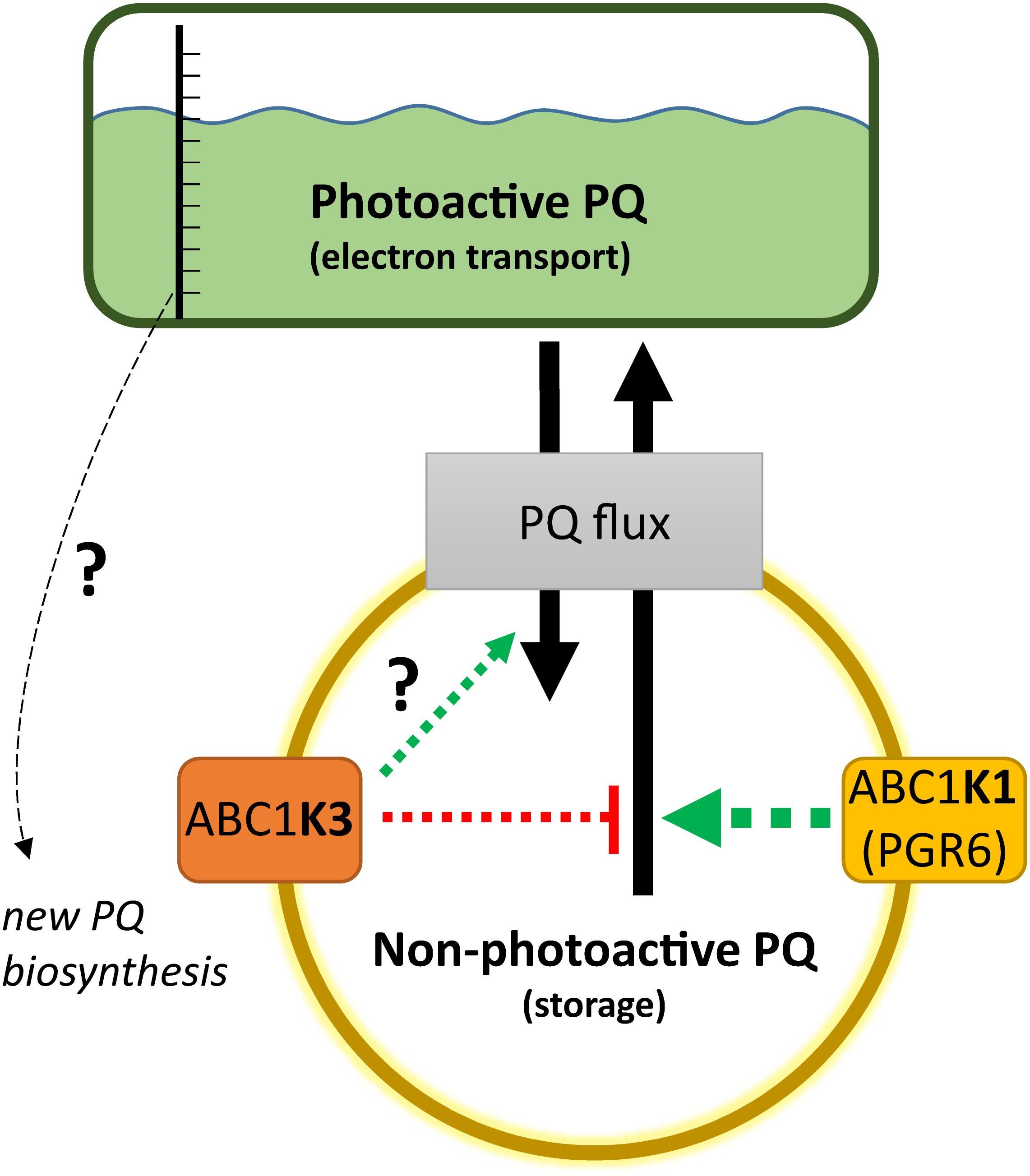
Figure 8. Working model on the activity of ABC1K1 and ABC1K3 on the plastoquinone (PQ) redistribution. The two atypical kinases are enriched in the plastoglobule proteome, therefore presumably in the proximity of the non-photoactive PQ pool. The activity of the ABC1K1 promotes the mobilization of PQ toward the photoactive pool, while ABC1K3 counteracts this mobilization thus favoring the accumulation of PQ in the non-photoactive pool. In the absence of both kinases the PQ tends to accumulate in the non-photoactive pool, therefore a positive regulation of ABC1K3 on the flux from the photoactive to the non-photoactive pool is not essential, as highlighted by the question mark, but cannot be excluded in this model. The observation that in absence of both kinases PQ tends to accumulate in the non-photoactive pool suggests a prominent role of ABC1K1 in the PQ flux regulation (larger arrow). A not yet identified sensor of the size and/or the redox state of the photoactive PQ pool may serve to regulate the level of PQ biosynthesis. When the relative size of the photoactive pool is larger, and the electron transport more efficient as in the abc1k3 mutant, the signal promoting PQ biosynthesis may be dimmed resulting in a lower amount of total PQ. The dashed arrows represent that the regulation of the PQ fluxes, mediated by the two atypical kinases, is likely to be indirect. Although the effect on PQ flux is opposite, the presented working model cannot exclude that the two atypical kinases act via separate mechanisms and that the impact observed on PQ mobility is a consequence of the alteration of their primary targets.
A crosstalk between photoactive PQ pool and biosynthesis would ensure the coupling of the photosynthetic electron transport efficiency with the production of a PQ reserve in the non-photoactive pool allowing fast adaptation to changing environmental conditions. It is necessary to point out that the decrease of total PQ after 3 h of high light was likely to be transitory since, under permissive light intensity, abc1k3 was capable of restoring the total PQ amount to WT levels as previously shown (Martinis et al., 2013). We cannot exclude that ABC1K1 and ABC1K3 play an active role in this signaling pathway, through still unidentified targets, therefore the mutation of these two kinases would result in a combined effect on the source of the signal, the photoactive PQ pool, as well as on the transmission of said signal.
Another feature linked to the abc1k1 mutation was the loss of thylakoid protein phosphorylation. These phosphorylation events are mostly dependent on STN7 and STN8 kinases, activities of which depend on the redox status of the PQ pool (Aro and Ohad, 2003; Pesaresi et al., 2010; Puthiyaveetil, 2011; Puthiyaveetil et al., 2012). The mutation of abc1k3 in the abc1k1 background was sufficient to re-establish the phosphorylation of the thylakoid proteins to WT level after 3 h of high light. This observation has an important implication to understand the factors regulating the redox state of the photoactive PQ pool. In fact, the PQ pool redox state appears to be dependent also on the exchanges between the photoactive pool and storage sites and not only on the size of the photoactive pool and the activity of the ETC. We cannot exclude that protein phosphorylation has a positive feedback loop effect; in fact, the phosphorylation of the thylakoid proteins may favor their mobility in the membrane and by doing so increase also the mobility of the PQ between different portions of the thylakoid membrane (Kirchhoff et al., 2000; Fristedt et al., 2009; Kirchhoff, 2014). We also cannot exclude the involvement of the phosphorylation of other, less evident, targets such as CURT1b, the phosphorylation of which is also dependent on STN8 kinase (Trotta et al., 2019). By their phosphorylation level, the proteins imbedded in the thylakoid membrane may change the overall conformation of the membrane system and by this also the mobility and exchange of the PQ, and other lipids, between the photoactive pool and the reservoir (Armbruster et al., 2013). Moreover, ABC1K1 and ABC1K3 are also predicted to function as kinases; therefore, they may phosphorylate yet unknown target proteins leading to the regulation of the photosynthetic activity and potentially influencing membrane fluidity and/or thylakoid protein organization (Yokoyama et al., 2016).
The results lead us to propose the following model for the action of ABC1K1 and ABC1K3, being two kinases located at the plastoglobule (Vidi et al., 2006; Lundquist et al., 2012; Martinis et al., 2013, 2014). The role of ABC1K1 would be to promote the release, or the exchange of the PQ between the storage and the photoactive pool, while ABC1K3 would act in limiting such diffusion blocking this PQ flux (Figure 8). This model would explain the slightly bigger size of the photoactive pool in abc1k3 and also the difference between abc1k1 and abc1k1/abc1k3, the latter having a better photosynthetic performance since it is missing the ABC1K3 protein that would otherwise act as a “brake” to the PQ supply to the photoactive pool (Figure 8). To fully explain the observed results, we also have to assume that, without the activity of ABC1K1, the PQ tends to over-accumulate in the non-photoactive pool thus leading to a depletion of the photoactive pool. In this regard, the role of ABC1K1 is crucial to maintaining the photosynthetic efficiency by promoting the movement and accumulation of the PQ against its “passive” distribution. In contrast ABC1K3, acting as a brake to the mobilization of the non-photoactive pool, may have a more important role in other processes and phases of plant development when lipids need to be efficiently accumulated in the reserve compartments (e.g., senescence, fruit maturation). Previous reports describe a direct interaction between ABC1K1 and ABC1K3 (Lundquist et al., 2013). It is possible to hypothesize that this interaction allows a reciprocal regulation of the two kinases. For instance, a negative regulation of ABC1K3, mediated by ABC1K1, could partially explain the results of this report. In this scenario, the abc1k1 mutation would cause a deregulation, or over activation, of the ABC1K3 kinase and this would then cause the photosynthetic defect. Following this model, the removal of ABC1K3, as in the double abc1k1/abc1k3 mutant, would partially rescue the defect caused by the first mutation. It has to be underlined that the physiological state of the double mutant abc1k1/abc1k3 is still not optimal and that the absence of ABC1K3 only partially rescues the photosynthetic phenotype. This observation is thus consistent with previous reports showing that the double mutant, lacking both kinases, displays a stunted phenotype under prolonged stress conditions to which is unable to adapt (Lundquist et al., 2013).
Conclusion
In conclusion, we show that the abc1k3 mutation allows a partial recovery of the pgr6/abc1k1 phenotype. Consequently, ABC1K1 and ABC1K3 act in an opposite manner in order to cope with short-term high light. Therefore, we suggest that the system of the atypical kinases ABC1K1 and ABC1K3 allows a dynamic regulation of the PQ pool mobility and availability, which is fundamental for the plant to cope with variations in environmental conditions. In the working model ABC1K3 would act by limiting the distribution of PQ to the photoactive pool, while ABC1K1 acts in the opposite way. The role of ABC1K1 would be prominent considering that, without any regulation, the relative amount of PQ in the photoactive pool is lower, as is the case for the double abc1k1/abc1k3 mutant. The opposing activities of these two atypical kinases would allow to equilibrate the amount of PQ, and potentially other lipids, between the storage compartments and the thylakoid membrane. Finally, a hypothetical signaling mechanism from the photoactive PQ pool to the PQ biosynthesis could explain the decreased amount of total PQ observed in abc1k3 (Figure 8). Overall, the ABC1K1/ABC1K3 mechanism, by regulating lipid partitioning, could be important to allow plastid plasticity that is essential for the progression into different developmental stages of the plant life.
Data Availability Statement
The raw data supporting the conclusions of this article will be made available by the authors, without undue reservation, to any qualified researcher.
Author Contributions
TP, VS, PL, and FK designed the experiments. TP, JC, RP, BK, VS, PL, MH, and GF performed all the experiments. TP, VS, PL, MH, GF, and FK contributed to the analysis and the interpretation of the results. TP, PL, MH, GF, and FK wrote the manuscript.
Funding
This work was supported by the Swiss National Science Foundation (SNSF) grants 31003A_156998, 31003A_179417, and 31003A_176191. GF acknowledges support from the LabEX GRAL, ANR-10-LABX-49-01 financed within the University Grenoble Alpes graduate school (Ecoles Universitaires de Recherche) CBH-EUR-GS (ANR-17-EURE-0003).
Conflict of Interest
The authors declare that the research was conducted in the absence of any commercial or financial relationships that could be construed as a potential conflict of interest.
Acknowledgments
The Laboratory of Plant Physiology thanks Véronique Douet for her technical support during the project.
Supplementary Material
The Supplementary Material for this article can be found online at: https://www.frontiersin.org/articles/10.3389/fpls.2020.00337/full#supplementary-material
Footnotes
References
Allen, J. F., Bennett, J., Steinback, K. E., and Arntzen, C. J. (1981). Chloroplast protein phosphorylation couples plastoquinone redox state to distribution of excitation energy between photosystems. Nature 291, 25–29. doi: 10.1038/291025a0
Armbruster, U., Labs, M., Pribil, M., Viola, S., Xu, W., Scharfenberg, M., et al. (2013). Arabidopsis CURVATURE THYLAKOID1 proteins modify thylakoid architecture by inducing membrane curvature. Plant Cell 25, 2661–2678. doi: 10.1105/tpc.113.113118
Aro, E. M., and Ohad, I. (2003). Redox regulation of thylakoid protein phosphorylation. Antioxid. Redox Signal. 5, 55–67. doi: 10.1089/152308603321223540
Aro, E. M., Virgin, I., and Andersson, B. (1993). Photoinhibition of photosystem II. Inactivation, protein damage and turnover. Biochim. Biophys. Acta 1143, 113–134. doi: 10.1016/0005-2728(93)90134-2
Austin, J. R. II, Frost, E., Vidi, P. A., Kessler, F., and Staehelin, L. A. (2006). Plastoglobules are lipoprotein subcompartments of the chloroplast that are permanently coupled to thylakoid membranes and contain biosynthetic enzymes. Plant Cell 18, 1693–1703. doi: 10.1105/tpc.105.039859
Bellafiore, S., Barneche, F., Peltier, G., and Rochaix, J. D. (2005). State transitions and light adaptation require chloroplast thylakoid protein kinase STN7. Nature 433, 892–895. doi: 10.1038/nature03286
Blackwell, M., Gibas, C., Gygax, S., Roman, D., and Wagner, B. (1994). The plastoquinone diffusion coefficient in chloroplasts and its mechanistic implications. Biochim. Biophys. Acta Bioenerg. 1183, 533–543. doi: 10.1016/0005-2728(94)90081-7
Block, A., Fristedt, R., Rogers, S., Kumar, J., Barnes, B., Barnes, J., et al. (2013). Functional modeling identifies paralogous solanesyl-diphosphate synthases that assemble the side chain of plastoquinone-9 in plastids. J. Biol. Chem. 288, 27594–27606. doi: 10.1074/jbc.M113.492769
Bonardi, V., Pesaresi, P., Becker, T., Schleiff, E., Wagner, R., Pfannschmidt, T., et al. (2005). Photosystem II core phosphorylation and photosynthetic acclimation require two different protein kinases. Nature 437, 1179–1182. doi: 10.1038/nature04016
Bousquet, I., Dujardin, G., and Slonimski, P. P. (1991). ABC1, a novel yeast nuclear gene has a dual function in mitochondria: it suppresses a cytochrome b mRNA translation defect and is essential for the electron transfer in the bc 1 complex. EMBO J. 10, 2023–2031. doi: 10.1002/j.1460-2075.1991.tb07732.x
Brasseur, G., Tron, P., Dujardin, G., Slonimski, P. P., and Brivet-Chevillotte, P. (1997). The nuclear ABC1 gene is essential for the correct conformation and functioning of the cytochrome bc1 complex and the neighbouring complexes II and IV in the mitochondrial respiratory chain. Eur. J. Biochem. 246, 103–111. doi: 10.1111/j.1432-1033.1997.t01-1-00103.x
Dall’Osto, L., Caffarri, S., and Bassi, R. (2005). A mechanism of nonphotochemical energy dissipation, independent from PsbS, revealed by a conformational change in the antenna protein CP26. Plant Cell 17, 1217–1232. doi: 10.1105/tpc.104.030601
Dumas, L., Zito, F., Blangy, S., Auroy, P., Johnson, X., Peltier, G., et al. (2017). A stromal region of cytochrome b6f subunit IV is involved in the activation of the Stt7 kinase in Chlamydomonas. Proc. Natl. Acad. Sci. U.S.A. 114, 12063–12068. doi: 10.1073/pnas.1713343114
Ferretti, U., Ciura, J., Ksas, B., Rac, M., Sedlarova, M., Kruk, J., et al. (2018). Chemical quenching of singlet oxygen by plastoquinols and their oxidation products in Arabidopsis. Plant J. 95, 848–861. doi: 10.1111/tpj.13993
Finazzi, G., Rappaport, F., Furia, A., Fleischmann, M., Rochaix, J. D., Zito, F., et al. (2002). Involvement of state transitions in the switch between linear and cyclic electron flow in Chlamydomonas reinhardtii. EMBO Rep. 3, 280–285. doi: 10.1093/embo-reports/kvf047
Fristedt, R., Willig, A., Granath, P., Crèvecoeur, M., Rochaix, J. D., and Vener, A. V. (2009). Phosphorylation of photosystem II controls functional macroscopic folding of photosynthetic membranes in Arabidopsis. Plant Cell 21, 3950–3964. doi: 10.1105/tpc.109.069435
Gaude, N., Bréhélin, C., Tischendorf, G., Kessler, F., and Dormann, P. (2007). Nitrogen deficiency in Arabidopsis affects galactolipid composition and gene expression and results in accumulation of fatty acid phytyl esters. Plant J. 49, 729–739. doi: 10.1111/j.1365-313x.2006.02992.x
Gong, H. S., and Ohad, I. (1991). The PQ/PQH2 ratio and occupancy of photosystem II-QB site by plastoquinone control the degradation of D1 protein during photoinhibition in vivo. J. Biol. Chem. 266, 21293–21299.
Gruszka, J., Pawlak, A., and Kruk, J. (2008). Tocochromanols, plastoquinol, and other biological prenyllipids as singlet oxygen quenchers-determination of singlet oxygen quenching rate constants and oxidation products. Free Radic. Biol. Med. 45, 920–928. doi: 10.1016/j.freeradbiomed.2008.06.025
Hall, J. D., Barr, R., Al-Abbas, A. H., and Crane, F. L. (1972). The Ultrastructure of chloroplasts in mineral-deficient maize leaves. Plant Physiol. 50, 404–409. doi: 10.1104/pp.50.3.404
Houyoux, P.-A., Ghysels, B., Lecler, R., and Franck, F. (2011). Interplay between non-photochemical plastoquinone reduction and re-oxidation in pre-illuminated Chlamydomonas reinhardtii: a chlorophyll fluorescence study. Photosynth. Res. 110:13. doi: 10.1007/s11120-011-9686-5
Huang, H., Yang, M., Su, Y., Qu, L., and Deng, X. W. (2015). Arabidopsis atypical kinases ABC1K1 and ABC1K3 act oppositely to cope with photodamage under red light. Mol Plant 8, 1122–1124. doi: 10.1016/j.molp.2015.04.003
Ivanov, A. G., Rosso, D., Savitch, L. V., Stachula, P., Rosembert, M., Oquist, G., et al. (2012). Implications of alternative electron sinks in increased resistance of PSII and PSI photochemistry to high light stress in cold-acclimated Arabidopsis thaliana. Photosynth. Res. 113, 191–206. doi: 10.1007/s11120-012-9769-y
Joet, T., Genty, B., Josse, E. M., Kuntz, M., Cournac, L., and Peltier, G. (2002). Involvement of a plastid terminal oxidase in plastoquinone oxidation as evidenced by expression of the Arabidopsis thaliana enzyme in tobacco. J. Biol. Chem. 277, 31623–31630. doi: 10.1074/jbc.m203538200
Joliot, P., and Finazzi, G. (2010). Proton equilibration in the chloroplast modulates multiphasic kinetics of nonphotochemical quenching of fluorescence in plants. Proc. Natl. Acad. Sci. U.S.A. 107, 12728–12733. doi: 10.1073/pnas.1006399107
Joliot, P., and Joliot, A. (2006). Cyclic electron flow in C3 plants. Biochim. Biophys. Acta 1757, 362–368. doi: 10.1016/j.bbabio.2006.02.018
Kalaji, H. M., Oukarroum, A., Alexandrov, V., Kouzmanova, M., Brestic, M., Zivcak, M., et al. (2014a). Identification of nutrient deficiency in maize and tomato plants by in vivo chlorophyll a fluorescence measurements. Plant Physiol. Biochem. 81, 16–25. doi: 10.1016/j.plaphy.2014.03.029
Kalaji, H. M., Schansker, G., Ladle, R. J., Goltsev, V., Bosa, K., Allakhverdiev, S. I., et al. (2014b). Frequently asked questions about in vivo chlorophyll fluorescence: practical issues. Photosynth. Res. 122, 121–158. doi: 10.1007/s11120-014-0024-6
Kanwischer, M., Porfirova, S., Bergmüller, E., and Dörmann, P. (2005). Alterations in tocopherol cyclase activity in transgenic and mutant plants of Arabidopsis affect tocopherol content, tocopherol composition, and oxidative stress. Plant Physiol. 137, 713–723. doi: 10.1104/pp.104.054908
Karpinski, S., Reynolds, H., Karpinska, B., Wingsle, G., Creissen, G., and Mullineaux, P. (1999). Systemic signaling and acclimation in response to excess excitation energy in Arabidopsis. Science 284, 654–657. doi: 10.1126/science.284.5414.654
Kirchhoff, H. (2014). Diffusion of molecules and macromolecules in thylakoid membranes. Biochim. Biophys. Acta Bioenerg. 1837, 495–502. doi: 10.1016/j.bbabio.2013.11.003
Kirchhoff, H., Horstmann, S., and Weis, E. (2000). Control of the photosynthetic electron transport by PQ diffusion microdomains in thylakoids of higher plants. Biochim. Biophys. Acta Bioenerg. 1459, 148–168. doi: 10.1016/s0005-2728(00)00143-2
Kruk, J., and Karpinski, S. (2006). An HPLC-based method of estimation of the total redox state of plastoquinone in chloroplasts, the size of the photochemically active plastoquinone-pool and its redox state in thylakoids of Arabidopsis. Biochim. Biophys. Acta 1757, 1669–1675. doi: 10.1016/j.bbabio.2006.08.004
Ksas, B., Becuwe, N., Chevalier, A., and Havaux, M. (2015). Plant tolerance to excess light energy and photooxidative damage relies on plastoquinone biosynthesis. Sci. Rep. 5:10919. doi: 10.1038/srep10919
Ksas, B., Legeret, B., Ferretti, U., Chevalier, A., Pospisil, P., Alric, J., et al. (2018). The plastoquinone pool outside the thylakoid membrane serves in plant photoprotection as a reservoir of singlet oxygen scavengers. Plant Cell Environ. 41, 2277–2287. doi: 10.1111/pce.13202
Lavergne, J., and Joliot, P. (1991). Restricted diffusion in photosynthetic membranes. Trends Biochem. Sci. 16, 129–134. doi: 10.1016/0968-0004(91)90054-y
Lennon, A. M., Prommeenate, P., and Nixon, P. J. (2003). Location, expression and orientation of the putative chlororespiratory enzymes, Ndh and IMMUTANS, in higher-plant plastids. Planta 218, 254–260. doi: 10.1007/s00425-003-1111-7
Li, L., Aro, E. M., and Millar, A. H. (2018). Mechanisms of photodamage and protein turnover in photoinhibition. Trends Plant Sci. 23, 667–676. doi: 10.1016/j.tplants.2018.05.004
Li, Z., Wakao, S., Fischer, B. B., and Niyogi, K. K. (2009). Sensing and responding to excess light. Annu. Rev. Plant Biol. 60, 239–260. doi: 10.1146/annurev.arplant.58.032806.103844
Lichtenthaler, H. K., and Peveling, E. (1966). Plastoglobuli in different types of plastids from Allium cepa L. Planta 72, 1–13. doi: 10.1007/BF00388140
Lippold, F., vom Dorp, K., Abraham, M., Holzl, G., Wewer, V., Yilmaz, J. L., et al. (2012). Fatty acid phytyl ester synthesis in chloroplasts of Arabidopsis. Plant Cell 24, 2001–2014. doi: 10.1105/tpc.112.095588
Lohscheider, J. N., and Rio Bartulos, C. (2016). Plastoglobules in algae: a comprehensive comparative study of the presence of major structural and functional components in complex plastids. Mar. Genomics 28, 127–136. doi: 10.1016/j.margen.2016.06.005
Lundquist, P. K., Poliakov, A., Bhuiyan, N. H., Zybailov, B., Sun, Q., and van Wijk, K. J. (2012). The functional network of the Arabidopsis plastoglobule proteome based on quantitative proteomics and genome-wide coexpression analysis. Plant Physiol. 158, 1172–1192. doi: 10.1104/pp.111.193144
Lundquist, P. K., Poliakov, A., Giacomelli, L., Friso, G., Appel, M., McQuinn, R. P., et al. (2013). Loss of plastoglobule kinases ABC1K1 and ABC1K3 causes conditional degreening, modified prenyl-lipids, and recruitment of the jasmonic acid pathway. Plant Cell 25, 1818–1839. doi: 10.1105/tpc.113.111120
Martinis, J., Glauser, G., Valimareanu, S., and Kessler, F. (2013). A chloroplast ABC1-like kinase regulates vitamin E metabolism in Arabidopsis. Plant Physiol. 162, 652–662. doi: 10.1104/pp.113.218644
Martinis, J., Glauser, G., Valimareanu, S., Stettler, M., Zeeman, S. C., Yamamoto, H., et al. (2014). ABC1K1/PGR6 kinase: a regulatory link between photosynthetic activity and chloroplast metabolism. Plant J. 77, 269–283. doi: 10.1111/tpj.12385
Miyao, M. (1994). Involvement of active oxygen species in degradation of the D1 protein under strong illumination in isolated subcomplexes of photosystem II. Biochemistry 33, 9722–9730. doi: 10.1021/bi00198a043
Moejes, F. W., Matuszynska, A., Adhikari, K., Bassi, R., Cariti, F., Cogne, G., et al. (2017). A systems-wide understanding of photosynthetic acclimation in algae and higher plants. J. Exp. Bot. 68, 2667–2681. doi: 10.1093/jxb/erx137
Mollet, J., Delahodde, A., Serre, V., Chretien, D., Schlemmer, D., Lombes, A., et al. (2008). CABC1 gene mutations cause ubiquinone deficiency with cerebellar ataxia and seizures. Am. J. Hum. Genet. 82, 623–630. doi: 10.1016/j.ajhg.2007.12.022
Müller, P., Li, X. P., and Niyogi, K. K. (2001). Non-photochemical quenching. A response to excess light energy. Plant Physiol. 125, 1558–1566. doi: 10.1104/pp.125.4.1558
Munné-Bosch, S., and Alegre, L. (2002). Plant aging increases oxidative stress in chloroplasts. Planta 214, 608–615. doi: 10.1007/s004250100646
Nilkens, M., Kress, E., Lambrev, P., Miloslavina, Y., Müller, M., Holzwarth, A. R., et al. (2010). Identification of a slowly inducible zeaxanthin-dependent component of non-photochemical quenching of chlorophyll fluorescence generated under steady-state conditions in Arabidopsis. Biochim. Biophys. Acta Bioenerg. 1797, 466–475. doi: 10.1016/j.bbabio.2010.01.001
Nowicka, B., and Kruk, J. (2012). Plastoquinol is more active than alpha-tocopherol in singlet oxygen scavenging during high light stress of Chlamydomonas reinhardtii. Biochim. Biophys. Acta 1817, 389–394. doi: 10.1016/j.bbabio.2011.12.002
Pesaresi, P., Hertle, A., Pribil, M., Schneider, A., Kleine, T., and Leister, D. (2010). Optimizing photosynthesis under fluctuating light. Plant Signal. Behav. 5, 21–25. doi: 10.4161/psb.5.1.10198
Pesaresi, P., Pribil, M., Wunder, T., and Leister, D. (2011). Dynamics of reversible protein phosphorylation in thylakoids of flowering plants: the roles of STN7, STN8 and TAP38. Biochim. Biophys. Acta 1807, 887–896. doi: 10.1016/j.bbabio.2010.08.002
Pfannschmidt, T. (2003). Chloroplast redox signals: how photosynthesis controls its own genes. Trends Plant Sci. 8, 33–41. doi: 10.1016/s1360-1385(02)00005-5
Pfannschmidt, T., Nilsson, A., and Allen, J. F. (1999). Photosynthetic control of chloroplast gene expression. Nature 397:625. doi: 10.1038/17624
Poon, W. W., Davis, D. E., Ha, H. T., Jonassen, T., Rather, P. N., and Clarke, C. F. (2000). Identification of Escherichia coli ubiB, a gene required for the first monooxygenase step in ubiquinone biosynthesis. J. Bacteriol. 182, 5139–5146. doi: 10.1128/jb.182.18.5139-5146.2000
Pralon, T., Shanmugabalaji, V., Longoni, P., Glauser, G., Ksas, B., Collombat, J., et al. (2019). Plastoquinone homoeostasis by Arabidopsis proton gradient regulation 6 is essential for photosynthetic efficiency. Commun. Biol. 2:220. doi: 10.1038/s42003-019-0477-4
Puthiyaveetil, S. (2011). A mechanism for regulation of chloroplast LHC II kinase by plastoquinol and thioredoxin. FEBS Lett. 585, 1717–1721. doi: 10.1016/j.febslet.2011.04.076
Puthiyaveetil, S., Ibrahim, I. M., and Allen, J. F. (2012). Oxidation-reduction signalling components in regulatory pathways of state transitions and photosystem stoichiometry adjustment in chloroplasts. Plant Cell Environ. 35, 347–359. doi: 10.1111/j.1365-3040.2011.02349.x
Rintamaki, E., Kettunen, R., and Aro, E. M. (1996). Differential D1 dephosphorylation in functional and photodamaged photosystem II centers. Dephosphorylation is a prerequisite for degradation of damaged D1. J. Biol. Chem. 271, 14870–14875. doi: 10.1074/jbc.271.25.14870
Rintamaki, E., Salonen, M., Suoranta, U. M., Carlberg, I., Andersson, B., and Aro, E. M. (1997). Phosphorylation of light-harvesting complex II and photosystem II core proteins shows different irradiance-dependent regulation in vivo. Application of phosphothreonine antibodies to analysis of thylakoid phosphoproteins. J. Biol. Chem. 272, 30476–30482. doi: 10.1074/jbc.272.48.30476
Rochaix, J. D. (2007). Role of thylakoid protein kinases in photosynthetic acclimation. FEBS Lett. 581, 2768–2775. doi: 10.1016/j.febslet.2007.04.038
Rochaix, J. D. (2011). Regulation of photosynthetic electron transport. Biochim. Biophys. Acta 1807, 375–383. doi: 10.1016/j.bbabio.2010.11.010
Rochaix, J. D. (2013). Redox regulation of thylakoid protein kinases and photosynthetic gene expression. Antioxid. Redox Signal. 18, 2184–2201. doi: 10.1089/ars.2012.5110
Rottet, S., Devillers, J., Glauser, G., Douet, V., Besagni, C., and Kessler, F. (2016). Identification of plastoglobules as a site of carotenoid cleavage. Front. Plant Sci. 7:1855. doi: 10.3389/fpls.2016.01855
Ruban, A. V. (2016). Nonphotochemical chlorophyll fluorescence quenching: mechanism and effectiveness in protecting plants from photodamage. Plant Physiol. 170, 1903–1916. doi: 10.1104/pp.15.01935
Ruban, A. V. (2018). Light harvesting control in plants. FEBS Lett. 592, 3030–3039. doi: 10.1002/1873-3468.13111
Shapiguzov, A., Chai, X., Fucile, G., Longoni, P., Zhang, L., and Rochaix, J. D. (2016). Activation of the Stt7/STN7 Kinase through dynamic interactions with the cytochrome b6f complex. Plant Physiol. 171, 82–92. doi: 10.1104/pp.15.01893
Shapiguzov, A., Ingelsson, B., Samol, I., Andres, C., Kessler, F., Rochaix, J. D., et al. (2010). The PPH1 phosphatase is specifically involved in LHCII dephosphorylation and state transitions in Arabidopsis. Proc. Natl. Acad. Sci. U.S.A. 107, 4782–4787. doi: 10.1073/pnas.0913810107
Shikanai, T., Munekage, Y., Shimizu, K., Endo, T., and Hashimoto, T. (1999). Identification and characterization of Arabidopsis mutants with reduced quenching of chlorophyll fluorescence. Plant Cell Physiol. 40, 1134–1142. doi: 10.1093/oxfordjournals.pcp.a029498
Spicher, L., Glauser, G., and Kessler, F. (2016). Lipid antioxidant and galactolipid remodeling under temperature stress in tomato plants. Front. Plant Sci. 7:167. doi: 10.3389/fpls.2016.00167
Steinmuller, D., and Tevini, M. (1985). Composition and function of plastoglobuli : 1. Isolation and purification from chloroplasts and chromoplasts. Planta 163, 201–207. doi: 10.1007/BF00393507
Stepien, P., and Johnson, G. N. (2018). Plastid terminal oxidase requires translocation to the grana stacks to act as a sink for electron transport. Proc. Natl. Acad. Sci. U.S.A. 115, 9634–9639. doi: 10.1073/pnas.1719070115
Stirbet, A., Lazár, D., Kromdijk, J., and Govindjee (2018). Chlorophyll a fluorescence induction: can just a one-second measurement be used to quantify abiotic stress responses? Photosynthetica 56, 86–104. doi: 10.1007/s11099-018-0770-3
Strasser, R. J., Tsimilli-Michael, M., Qiang, S., and Goltsev, V. (2010). Simultaneous in vivo recording of prompt and delayed fluorescence and 820-nm reflection changes during drying and after rehydration of the resurrection plant Haberlea rhodopensis. Biochim. Biophys. Acta 1797, 1313–1326. doi: 10.1016/j.bbabio.2010.03.008
Suzuki, N., Koussevitzky, S., Mittler, R., and Miller, G. (2012). ROS and redox signalling in the response of plants to abiotic stress. Plant Cell Environ. 35, 259–270. doi: 10.1111/j.1365-3040.2011.02336.x
Sylak-Glassman, E. J., Malnoe, A., De Re, E., Brooks, M. D., Fischer, A. L., Niyogi, K. K., et al. (2014). Distinct roles of the photosystem II protein PsbS and zeaxanthin in the regulation of light harvesting in plants revealed by fluorescence lifetime snapshots. Proc. Natl. Acad. Sci. U.S.A. 111, 17498–17503. doi: 10.1073/pnas.1418317111
Taylor, A. O., and Craig, A. S. (1971). Plants under climatic stress: II. Low temperature, high light effects on chloroplast ultrastructure. Plant Physiol. 47, 719–725. doi: 10.1104/pp.47.5.719
Theis, J., and Schroda, M. (2016). Revisiting the photosystem II repair cycle. Plant Signal. Behav. 11:e1218587. doi: 10.1080/15592324.2016.1218587
Tikhonov, A. N. (2014). The cytochrome b6f complex at the crossroad of photosynthetic electron transport pathways. Plant Physiol. Biochem. 81, 163–183. doi: 10.1016/j.plaphy.2013.12.011
Tikkanen, M., Gollan, P. J., Suorsa, M., Kangasjarvi, S., and Aro, E. M. (2012). STN7 operates in retrograde signaling through controlling redox balance in the electron transfer chain. Front. Plant Sci. 3:277. doi: 10.3389/fpls.2012.00277
Tikkanen, M., Nurmi, M., Kangasjarvi, S., and Aro, E. M. (2008). Core protein phosphorylation facilitates the repair of photodamaged photosystem II at high light. Biochim. Biophys. Acta 1777, 1432–1437. doi: 10.1016/j.bbabio.2008.08.004
Tóth, S. Z., Schansker, G., and Strasser, R. J. (2007). A non-invasive assay of the plastoquinone pool redox state based on the OJIP-transient. Photosynth. Res. 93, 193. doi: 10.1007/s11120-007-9179-8
Trotta, A., Bajwa, A. A., Mancini, I., Paakkarinen, V., Pribil, M., and Aro, E. M. (2019). The role of phosphorylation dynamics of CURVATURE THYLAKOID 1B in plant thylakoid membranes. Plant Physiol. 181, 1615–1631. doi: 10.1104/pp.19.00942
Trotta, A., Suorsa, M., Rantala, M., Lundin, B., and Aro, E. M. (2016). Serine and threonine residues of plant STN7 kinase are differentially phosphorylated upon changing light conditions and specifically influence the activity and stability of the kinase. Plant J. 87, 484–494. doi: 10.1111/tpj.13213
Trouillard, M., Shahbazi, M., Moyet, L., Rappaport, F., Joliot, P., Kuntz, M., et al. (2012). Kinetic properties and physiological role of the plastoquinone terminal oxidase (PTOX) in a vascular plant. Biochim. Biophys. Acta 1817, 2140–2148. doi: 10.1016/j.bbabio.2012.08.006
van Wijk, K. J., and Kessler, F. (2017). Plastoglobuli: plastid microcompartments with integrated functions in metabolism, plastid developmental transitions, and environmental adaptation. Annu. Rev. Plant Biol. 68, 253–289. doi: 10.1146/annurev-arplant-043015-111737
Vasilikiotis, C., and Melis, A. (1994). Photosystem II reaction center damage and repair cycle: chloroplast acclimation strategy to irradiance stress. Proc. Natl. Acad. Sci. U.S.A. 91, 7222–7226. doi: 10.1073/pnas.91.15.7222
Vidi, P. A., Kanwischer, M., Baginsky, S., Austin, J. R., Csucs, G., Dormann, P., et al. (2006). Tocopherol cyclase (VTE1) localization and vitamin E accumulation in chloroplast plastoglobule lipoprotein particles. J. Biol. Chem. 281, 11225–11234. doi: 10.1074/jbc.m511939200
Wetzel, C. M., Jiang, C. Z., Meehan, L. J., Voytas, D. F., and Rodermel, S. R. (1994). Nuclear-organelle interactions: the immutans variegation mutant of Arabidopsis is plastid autonomous and impaired in carotenoid biosynthesis. Plant J. 6, 161–175. doi: 10.1046/j.1365-313x.1994.6020161.x
Yamori, W., and Shikanai, T. (2016). Physiological functions of cyclic electron transport around photosystem I in sustaining photosynthesis and plant growth. Annu. Rev. Plant Biol. 67, 81–106. doi: 10.1146/annurev-arplant-043015-112002
Yang, M., Huang, H., Zhang, C., Wang, Z., Su, Y., Zhu, P., et al. (2016). Arabidopsis atypical kinase ABC1K1 is involved in red light-mediated development. Plant Cell Rep. 35, 1213–1220. doi: 10.1007/s00299-016-1953-7
Yokoyama, R., Yamamoto, H., Kondo, M., Takeda, S., Ifuku, K., Fukao, Y., et al. (2016). Grana-localized proteins, RIQ1 and RIQ2, affect the organization of light-harvesting complex II and grana stacking in Arabidopsis. Plant Cell 28, 2261–2275. doi: 10.1105/tpc.16.00296
Ytterberg, A. J., Peltier, J. B., and van Wijk, K. J. (2006). Protein profiling of plastoglobules in chloroplasts and chromoplasts. A surprising site for differential accumulation of metabolic enzymes. Plant Physiol. 140, 984–997. doi: 10.1104/pp.105.076083
Zbierzak, A. M., Kanwischer, M., Wille, C., Vidi, P. A., Giavalisco, P., Lohmann, A., et al. (2010). Intersection of the tocopherol and plastoquinol metabolic pathways at the plastoglobule. Biochem. J. 425, 389–399. doi: 10.1042/BJ20090704
Keywords: photosynthetic electron transport, plastoquinone pool, plastoglobule, high light acclimation, NPQ
Citation: Pralon T, Collombat J, Pipitone R, Ksas B, Shanmugabalaji V, Havaux M, Finazzi G, Longoni P and Kessler F (2020) Mutation of the Atypical Kinase ABC1K3 Partially Rescues the PROTON GRADIENT REGULATION 6 Phenotype in Arabidopsis thaliana. Front. Plant Sci. 11:337. doi: 10.3389/fpls.2020.00337
Received: 27 November 2019; Accepted: 06 March 2020;
Published: 25 March 2020.
Edited by:
Przemysław Malec, Jagiellonian University, PolandReviewed by:
Claire Remacle, University of Liège, BelgiumPeter J. Gollan, University of Turku, Finland
Copyright © 2020 Pralon, Collombat, Pipitone, Ksas, Shanmugabalaji, Havaux, Finazzi, Longoni and Kessler. This is an open-access article distributed under the terms of the Creative Commons Attribution License (CC BY). The use, distribution or reproduction in other forums is permitted, provided the original author(s) and the copyright owner(s) are credited and that the original publication in this journal is cited, in accordance with accepted academic practice. No use, distribution or reproduction is permitted which does not comply with these terms.
*Correspondence: Paolo Longoni, cGFvbG8ubG9uZ29uaUB1bmluZS5jaA==; Felix Kessler, ZmVsaXgua2Vzc2xlckB1bmluZS5jaA==