- 1Wageningen UR Plant Breeding, Wageningen University and Research (WUR), Wageningen, Netherlands
- 2Biometris, Wageningen University and Research (WUR), Wageningen, Netherlands
- 3Fédération Nationale des Producteurs de Chanvre (FNPC), Le Mans, France
- 4VanDinter Semo BV, Scheemda (VDS), Netherlands
- 5Centro di ricerca cerealicoltura e colture industriale (CRA), Rovigo, Italy
- 6Department of Post Harvest Technology, Leibniz-Institute for Agricultural Engineering and Bioeconomy (ATB), Potsdam-Bornim, Germany
- 7Department of Sustainable Crop Production, Università Cattolica del Sacro Cuore (UCSC), Piacenza, Italy
- 8The Biological Materials Group, Biomimetics, City University of Applied Sciences Bremen (HSB), Bremen, Germany
Hemp (Cannabis sativa L.) is a bast-fiber crop well-known for the great potential to produce sustainable fibers. Nevertheless, hemp fiber quality is a complex trait, and little is known about the phenotypic variability and heritability of fiber quality traits in hemp. The aim of this study is to gain insights into the variability in fiber quality within the hemp germplasm and to estimate the genetic components, environmental components, and genotype-by-environment (G×E) interactions on fiber quality traits in hemp. To investigate these parameters, a panel of 123 hemp accessions was phenotyped for 28 traits relevant to fiber quality at three locations in Europe, corresponding to climates of northern, central, and southern Europe. In general, hemp cultivated in northern latitudes showed a larger plant vigor while earlier flowering was characteristic of plants cultivated in southern latitudes. Extensive variability between accessions was observed for all traits. Most cell wall components (contents of monosaccharides derived from cellulose and hemicellulose; and lignin content), bast fiber content, and flowering traits revealed large genetic components with low G×E interactions and high broad-sense heritability values, making these traits suitable to maximize the genetic gains of fiber quality. In contrast, contents of pectin-related monosaccharides, most agronomic traits, and several fiber traits (fineness and decortication efficiency) showed low genetic components with large G×E interactions affecting the rankings across locations. These results suggest that pectin, agronomic traits, and fiber traits are unsuitable targets in breeding programs of hemp, as their large G×E interactions might lead to unexpected phenotypes in untested locations. Furthermore, all environmental effects on the 28 traits were statistically significant, suggesting a strong adaptive behavior of fiber quality in hemp to specific environments. The high variability in fiber quality observed in the hemp panel, the broad range in heritability, and adaptability among all traits prescribe positive prospects for the development of new hemp cultivars of excellent fiber quality.
Introduction
Hemp (Cannabis sativa L.) is a well-known bast-fiber crop with evident phenotypic diversity in plant morphology between genotypes. For instance, de Meijer et al. described large diversity in plant height, stem diameter, and stem yield among 206 genotypes. They described accessions up to 4 m high while other plants had a dwarf phenotype with less than 1 m of height (Meijer et al., 1992; Meijer, 1994; Meijer and Keizer, 1996). Extensive diversity has also been described in cannabinoid content, in particular for the major cannabinoids: Δ9-tetrathydrocannabinol (THC) and cannabidiol (CBD) (Meijer et al., 1992; Meijer and Keizer, 1996).
The out-crossing behavior of hemp (Sawler et al., 2015) and its dioecious nature contribute to the variability. Male, female, and monoecious plants are characterized by large sexual dimorphism affecting plant morphology, flowering time, and fiber quality. Male plants have a slender stature, few leaves, flower early, and die after flowering. Moreover, male plants have less lignified cell walls, fine fibers, and large proportion of primary compared to secondary bast fibers. In contrast, female plants are more leafy, flower later, remain alive until seed maturation, accumulate larger content of lignin in the cell walls, and develop larger amount of secondary bast fibers (Amaducci and Gusovius, 2010; Faux et al., 2013). Monoecious plants resemble female plants and are more uniform (Mandolino and Carboni, 2004; Faux et al., 2016). Furthermore, sex determination in cannabis is a quantitative trait. A range of flowers of the opposite sex determined by the genetics can occur in dioecious hemp, and the ratio of female-to-male flowers in monoecious plants is highly variable (Faux et al., 2013; Faux et al., 2014; Faux et al., 2016). A genetic expression analysis between male and female dioecious plants identified nine mRNAs overexpressed in female plants putatively involved in auxin-related gene expression. The study suggested that the repression of female characteristics in male plants implies the downregulation of the genes involved in pathways more strictly related to the differentiation of the female sex (Moliterni et al., 2004). In addition, a range of studies revealed that sex determination of hemp is strongly sensitive to external factors, such as accumulation of Cu++, Zn++, and Pb++ ions or hormonal treatment (Chailakhyan and Khryanin, 1978; Freeman et al., 1980; Soldatova and Khryanin, 2010; Galoch, 2015; Faux et al., 2016). Such studies suggested that in hemp, non-genetic mechanisms, such as epigenetics, might probably affect the control of sex determination (Heikrujam et al., 2014). Consequently, the sexual variation in hemp is expected to be influenced by genetic and environmental components.
Morphological measurements, fiber quality, and flowering traits of hemp respond strongly to environmental factors, particularly to photoperiod and temperature but also to soil composition and crop management (Faux et al., 2013; Amaducci et al., 2015; Sawler et al., 2015). Hemp is a short day plant, and its flowering time is influenced by changes in the photoperiod regime (Amaducci et al., 2012). In locations where the shift from long-day toward short-day photoperiod regimes occurs early, hemp plants flower early, whereas in locations where the shift occurs later, the critical photoperiod for flowering is reached later (reviewed in Salentijn et al., 2019). This behavior affects plant development because plants accumulate biomass during the vegetative growing period, but nutrients are shifted from the production of stem, leaves, and roots toward the production of flowers and seeds around the onset of flowering. In addition, lignification of cell walls intensifies after flowering, along with secondary bast fiber formation (Van Der Werf and Turunen, 2008; Liu et al., 2015). Crop management features such as plant density, irrigation, and harvesting time are also reported to generate differences in phenological traits, such as plant height and stem diameter (Amaducci et al., 2015). Therefore, hemp accessions cultivated under specific environmental conditions are expected to have specific fiber composition and properties.
Hemp is a sustainable fiber crop with great potential for the production of a plethora of bio-based products. Yet, hemp cultivars with improved fiber properties are needed to promote hemp in the emerging bio-based economy. The first step in a breeding program is to characterize the genetic variability for the traits of interest, and that can be done by characterizing them in a wide range of accessions. Understanding the contribution of the genetic (G), environment (E), and genotype-by-environment (G×E) interaction components in fiber quality traits is essential to study the stability of fiber quality across different environments and thus improve the success of breeding programs.
To date, little research has been conducted on the variability of hemp traits relevant to fiber quality, such as cell wall composition, stem decortication, bast fiber content after decortication, or fineness of extracted fiber bundles. The objectives of this study are to evaluate the genetic variability, G×E interactions, and heritability of 28 traits relevant for fiber quality of hemp and identify which traits are worth to be further investigated with mapping studies. The relationships between the 28 traits will also be investigated.
Materials and Methods
Plant Material
A test panel of 123 hemp accessions was used in this study to investigate the phenotypic variability of fiber quality in hemp. This panel included mainly fiber accessions, one oil accession, cultivar Finola, one ornamental, and few accessions with other uses (Table 1).
Field Experimental Design
The effects of the environment and the G×E interactions on the phenotypic variation of fiber quality were assessed on the basis of three locations across Europe at respectively high, mid, and low latitude. The environments mostly differed in photoperiod and temperature regimes and water availability, as shown in Table 2. The 123 hemp accessions were grown in: Rovigo, at CRA (Centro di ricerca cerealicoltura e colture industriale) in Italy (45°N 11°E); Chèvrenolles, Neuville-sur-Sarthe, at FNPC (Fédération Nationale des Producteurs de Chanvre) in France (48°N 0.2°E); and Westerlee, at VDS (VanDinter Semo BV) in Netherlands (53°N 6°E). Field trials were performed between April and September 2013. Each field trial had a randomized complete block design with three biological replicates (plots) per accession and location. The experimental units were plots of 1 m2 in Italy and Netherlands and of 1.5 m2 in France. In all three locations, the same sowing density was used to aim a density of 100 plants/m2. Plants in the three middle rows were used for phenotyping. In dioecious accessions, phenotyping was performed only in female plants given the difference in fiber quality due to sex dimorphism in hemp. Field trials were harvested at temperature degree days (∑°C, the cumulated Celsius degree day over a period at a base temperature of 1°C) of 1,740.25°C, 1,421.1°C, and 1,843.3°C in CRA, FNPC, and VDS, respectively, corresponding to full flowering for most accessions in each location (Table 2).
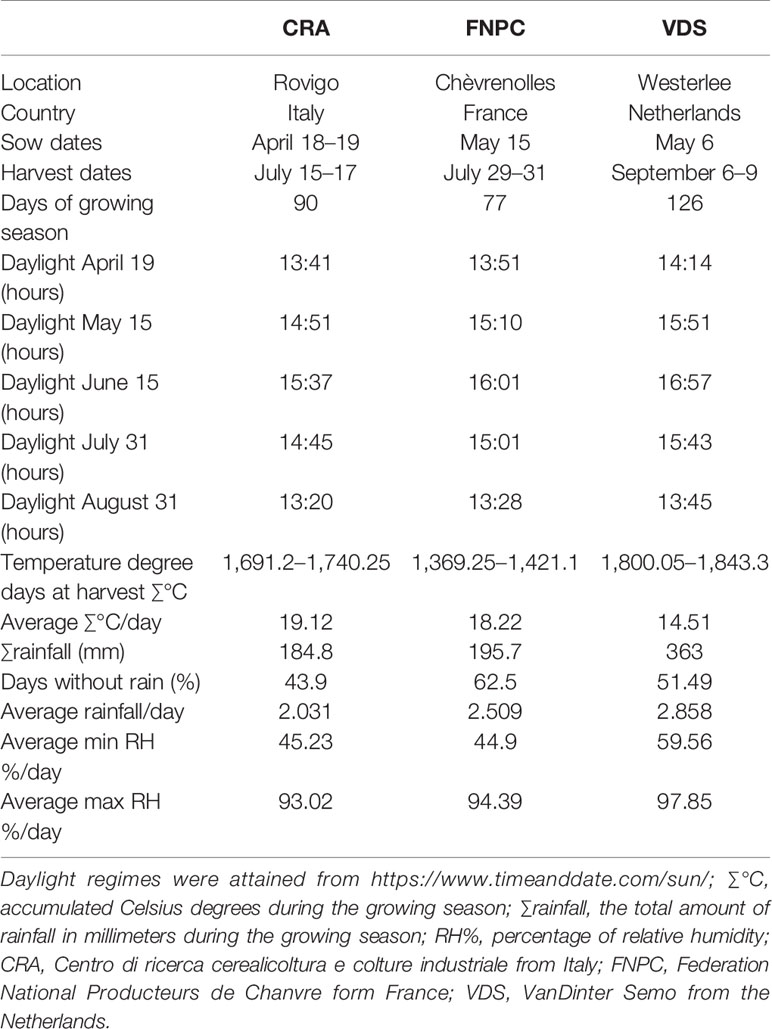
Table 2 Environmental characteristics of the three field trial locations (CRA, FNPC, and VDS) during the growing season of the MultiHemp project in 2013.
Phenotypic Data Analysis
In total, 28 parameters were phenotyped, including five agronomic traits, four different flowering traits including sex determination, nine fiber measurements (morphological and processing-related properties), and 10 parameters of cell wall composition (Table 3).
Agronomic Measurements
At harvesting time, plants were cut at the base of the stem, and agronomic traits were measured. Stem height (H in centimeters) was measured after harvest. Stem diameter (D in millimeters) was measured at 10 cm above the ground. H and D were phenotyped at three plants per plot, and data were provided as the mean per plot.
Fresh weight was measured in five entire plants per plot including stem and leaves. Thereafter, leaves and stems were separated and dried at 60°C for 48 h. Different dry weights were calculated: dry weight of five stems (DW_5S in grams), dry weight as a fraction of the fresh weight of five stems (DW_S% in percentage), and dry weight as a fraction of the fresh weight of five plants (DW% in percentage).
Flowering Parameters
Emergence of the plants was scored as the accumulated Celsius degree days or temperature sum (∑°C) at the day of first emergence. Emergence was scored in one row per plot at day = N, N + 2, N + 4, and N + 7, where N is the day of sowing. Flowering time traits were also measured in ∑°C at 10 plants per plot. Beginning of flowering (FL_Begin in ∑°C) and full flowering (FL_Full in ∑°C) were calculated relative to the emergence as:
where ∑°CBeginning flowering, ∑°CFull flowering, and ∑°CEmergence are the accumulated Celsius degree days, respectively, at the beginning of flowering, full flowering, and at the day of first emergence. The length of vegetative growth period (VEG in days) is the growing period of the plants in days, as measured from the day of first emergence until FL_Begin. Sex determination was phenotyped assessing “1” for predominantly dioecious plants, “2” for the mix of dioecious and monoecious plants, and “3” for predominantly monoecious.
Fiber Traits (Morphological and Processing-Related Properties)
The measurements of the processing-related properties were performed on stem segments of at least 100 cm, discarding 20 scm from the base of the plant and removing 30 cm from the top. Stem portions were naturally dried, in open air under a roof, until the water content was less than 18% of the mass. Thereafter, stems were warm water retted for 3 days at an average temperature of 23°C according to (Van Den Oever et al., 2003). After water retting, stems were naturally dried again and stored at 20°C ± 3°C and relative humidity of 60% ± 5%. All stem weight measurements were calculated as an average in grams of 10 stems. The first measurement of stem weight, M0, was performed straight before the decortication. Each specimen was decorticated individually with a lab-scale roller-breaker decortication system according to Wang et al. (2018). Stem portions passed through all decortication steps six times. The weight of each decorticated specimen was measured and recorded after each passage through the decorticator, Mi, in grams, where “i” is the passage number from 1 to 6. After the sixth passage, the remaining shives [also known as woody hemp core (WHC)] were removed manually from the bast, and the shives-free bast was weighed (M7). The fiber bundles of the shive-free bast were separated using a Worthmann coarse separator unit (Worthmann Maschinenbau GmbH, Barßel-Harkebrügge, Germany). The weight of the separated fiber bundles were measured and recorded before (MF0 in grams) and after (MF1 in grams) the separation.
The stem weight (M0) as well as the remaining weights after respective decortication steps (M2, M6, and M7) were used to calculate the bast content and the decortication efficiency parameters, according to Wang et al. (2018). Bast content after decortication (BCD in percentage) was calculated as the fraction between the mass of shives-free bast (M7) and the mass of the initial non-decorticated stems (M0):
The initial decortication efficiency (ηDec_1 in percentage) describes the efficiency of the initial stage of the decortication process. It was calculated by using the following formula:
The ultimate decortication efficiency (ηDec_2 in percentage) estimates the efficiency of the overall decortication process known as decorticability. The decorticability indicates the difference between the weight of the bast fiber after the final removal of the remaining shives after the decortication (M7) and the weight of the bast after the last round of the decortication process (M6):
Shives content after decortication (χ in percentage) describes the ratio of the shives that remained stuck to the bast after the decortication:
Fineness of extracted fiber bundles was indirectly characterized by measuring the permeability of air flow injected in the bast fiber bundles with a defined mass (Müssig and Amaducci, 2018). The permeability of air is an indicator of the fiber bundle surface. Fineness was measured using a Shirley IIC Fineness and Maturity Tester (Shirley FMT) according to Müssig (2001) and Müssig et al. (2010). Twelve technical replicates of 4 ± 0.005 g of separated bast fiber bundles were weighed for the analysis after 24 h of acclimatization at 20°C and 65% relative humidity of air for sample standardization. Two different air compressions were injected in each sample: low compression of air at a flow rate of 4 L of air per minute and high compression at a flow rate of 1 L of air per minute (Montalvo and Faught, 1999). Two different Shirley values were obtained: PL and PH. PL (in millimeter water) is the pressure of the air injected at a low compression and PH (in millimeter water) is the pressure of the air injected at a high compression of air. Both measurements were calculated as the mean of the twelve specimens per sample.
Biochemical Analysis of Hemp Cell Walls
Hemp cell walls are mostly composed of polysaccharides and lignin, and this was therefore the main target of the biochemical analysis. Polysaccharide composition was measured based on the content of the monosaccharides that are specific for each polysaccharide. In total, 10 cell wall parameters were measured: the monosaccharide glucose (Glc%dm) that is mostly composing cellulose; mannose (Man%dm) composing mannan; xylose (Xyl%dm) and glucuronic acid (GlcA%dm) composing xylan; arabinose (Ara%dm), galactose (Gal%dm), galacturonic acid (GalA%dm), and rhamnose (Rha%dm) composing pectin, and furthermore two measurements for lignin, Klasson lignin (KL%dm) and acid detergent lignin (ADL%dm). All parameters were calculated as percentage of the dry matter. All cell wall traits were measured with multivariate prediction models based on near-infrared spectroscopy (NIRS), after a calibration curve for the 10 different cell wall traits in hemp was developed. In detail, five stems of each plot were harvested, after which the un-retted stem were dried, pooled and grinded according to Petit et al. (2019) and scanned using a Foss DS2500 near-infrared spectrometer (Foss, Hillerød, Denmark) to obtain the NIR spectra of stem samples [details in (Van Der Weijde et al., 2016)]. A subset of 114 samples was selected based on the variation of the NIR spectra and biochemically analyzed (Petit et al., 2019) to develop the prediction models. Details of the quality of the models can be found in Supplementary Table 1 and 2.
Statistical Analyses
In order to study the variability of fiber quality in hemp, an ANOVA model was used to determine the significant differences of each variance component in the 28 traits: genotype (G), environment (E), blocks within environment (B), G×E interactions, and residual variance (ϵ). The analysis was performed following the model:
where y is the trait, µ is the grand mean, E is the effect due to the environment, B is the effect of block within environment, G is the genotypic effect, G×E is the genotype-by-location interactions, and ϵ is the residual error. In addition, a random effects model was used to determine the estimates of variance components of the phenotypic variation following the model:
where σy2, σE2, σB2, σG2, σG×E2, σϵ2 are the variances for y, E, B, G, G×E, and ϵ, respectively. The variance components were reported as the percentage of each component to the total phenotypic variation. Both ANOVA and random effects models were performed using a restricted maximum likelihood (REML) algorithm. For each trait, the stability of the accessions across locations was determined with the size of the variation due to G×E interactions relative to the main genotypic component, as in (Gitonga et al., 2014):
The broad-sense heritability values (H2) were calculated across the three environments, as the fraction of the genetic component () to the total genotypic effect (, , and ) including the G×E interactions and the residual variance corrected by the number of blocks and environments, as in Renaud et al. (2014):
Where n·E is the number of environments, and n·B is the number of blocks. REML and broad-sense heritability (H2) analyses were performed using Genstat 19th edition software (VSN International, Hemel Hempstead, UK).
Summary statistics of the 28 traits and the accessions was performed in Genstat 19th edition software. Correlation analysis between the 28 traits was performed in R (http://www.r-project.org/) version 3.4.3 statistical software using corrplot function. The adjusted mean of the phenotypic values across the three locations was used for each trait to study the main correlations independently of the effect of the environment.
Results
Fiber Quality Variability of the Hemp Accession Panel
Significant differences between the averaged performance across the three environments for all accessions were found for all traits (p < 0.001). Most traits showed extensive variation among the accessions of the hemp panel, as revealed by the wide range and the large coefficients of variation for each trait presented in Table 3. Traits with wide variation between accessions included Glc%dm, Man%dm, Xyl%dm, ADL%dm, KL%dm, BCD%, PH, PL, and four flowering traits (Table 3). Tables 4–6 show the averaged phenotypic values and the coefficients of variation across all environments of these 12 traits for the accessions that displayed the most contrasting phenotypic values.
Accessions IWNRZ-902 (Beniko) and WU-111 (Kompolti Sargászáru) showed the largest contents of Glc%dm, Man%dm, and BCD% while LARC-501 (Katlakalna) and UOY-801 (Finola) showed the opposite phenotypic characteristics. In contrast, the opposite patterns were found for Xyl%dm, ADL%dm, and KL%dm where IWNRZ-902 and WU-111 showed the lowest phenotypic values and LARC-501 and UOY-801 showed the largest values (Tables 4 and 5). CAAS-601 showed the finest fiber bundles while IWNRZ-902 showed the coarsest ones, as presented in Table 5. Chinese accessions (CAAS) were the latest to flower and showed the longest vegetative growth period (VEG). In addition, some Chinese accessions (CAAS-601, CAAS-602, CAAS-603, and CAAS-605) did not reach full flowering, before the end of the field trials, in Netherlands and France but they did in Italy (data not shown). In contrast, LARC-501, UOY-801, and WU-122 were the earliest accession to flower and to reach full flowering, and they showed the shortest VEG (Table 6). Finally, contrasting accessions for sex determination can be found in Table 6. Sex determination highlighted large range of variation between predominantly dioecious and predominantly monoecious. For instance, Chinese accessions and UOY-801 showed only dioecious plants (score = 1), and IWNRZ-901, IWNRZ-902, and IWNRZ-903 showed only monoecious plants (score = 3) while other accessions showed dioecious plants mixed with monoecious plants in different proportions. LARC-501 showed larger number of dioecious than monoecious plants (score = 1.22), WU-119 showed approximately equal amount of dioecious and monoecious plants, while CRA-415 showed more monoecious than dioecious plants (score = 2.44).
Elucidating the Key Components of Fiber Quality Variability in the Hemp Panel
The ANOVA model highlighted significant differences (p < 0.001) for all variance components in all traits. In addition, as shown in Table 7 and Supplementary Figure 1, the random effects model revealed traits with phenotypic variations strongly influenced by the genetic component and traits mostly influenced by the environment component.
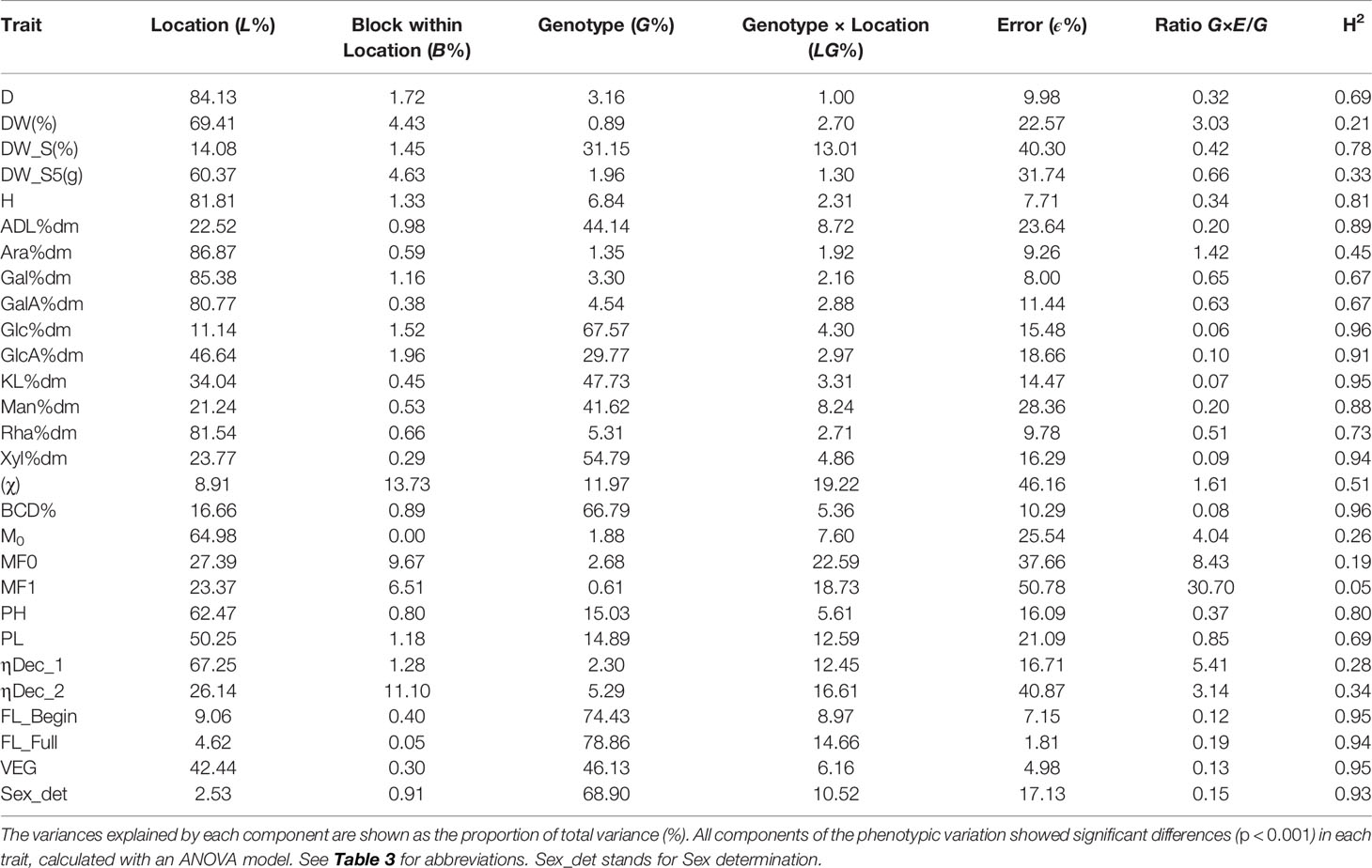
Table 7 Variance components and broad-sense heritability (H2) of 28 traits calculated with a random effects model.
Traits with extensive influence of the genetic component (>40%) comprised flowering traits, cell wall traits including contents of monosaccharides derived from cellulose and hemicelluloses, lignin content, and the fiber trait BCD% (Table 7 and Supplementary Figure 1). The variation in flowering traits: FL_Begin, FL_Full, VEG, and Sex_det showed genetic components of respectively 74%, 79%, 46%, and 69%; the content of the monosaccharide from cellulose, Glc%dm, was 68%, and the contents of monosaccharides from hemicelluloses were calculated respectively 55% (for Xyl%dm) and 42% (for Man%dm). The two measurements of lignin displayed genetic components of 44% (for ADL%dm) and 48% (for KL%dm), respectively. BCD% showed a genetic component of 67%. All these 10 traits showed larger genetic component than G×E interaction. Ratios G×E/G close to zero were detected for all these traits indicating large stability of the accession ordering across environments. Consequently, all these traits displayed high H2, ranging from 0.88 to 0.96, as detailed in Table 7.
Traits with large influence of the environment component (>30%) comprised several agronomic traits, cell wall traits such as the content of monosaccharides composing pectin and GlcA%dm, and most fiber traits (Table 7 and Supplementary Figure 1). Agronomic traits such as D, H, DW(%), and DW_S5(g) showed environment components larger than 60%. The composition of pectin, reflected by the contents of Ara%dm, Gal%dm, GalA%dm, and Rha%dm, was extensively influenced by the environment component (>80%). Glucuronic acid, a component of xylan (hemicellulose), expressed as GlcA%dm, showed a strong influence of the environment (47%) but also highlighted a substantial genetic component (30%). In addition, fiber traits such as ηDec_1, PH, PL, and M0 showed environment components of respectively 67%, 62%, 50%, and 65%. The ratios G×E/G showed different performances in these agronomic measurements, fiber traits, and pectin-related monosaccharides. DW(%), Ara%dm, M0, and ηDec_1 showed large G×E/G ratios ranging from 1.42 to 5.41. These traits showed large differences between accessions in environmental sensitivity, indicating alteration of the accession ordering across environments. These differences in sensitivity were reflected by low H2, ranging from 0.21 to 0.45. In contrast, D, DW_S5(g), H, Gal%dm, GalA%dm, Rha%dm, PH, and PL showed interaction ratios ranging from 0.32 to 0.85. These results may indicate that, despite the significant genetic component of the phenotypic variation, the small effects of the genetic component in some traits [particularly D, DW_S5(g), H, Gal%dm, GalA%dm, and Rha%dm] hampered the assessment of the G×E% interactions, and thus the ratios G×E/G are small. As a consequence, considering the definition of H2 (Renaud et al., 2014), the ratios G×E/G below 1 can explain unexpected H2 (Gitonga et al., 2014), ranging from 0.33 to 0.8, from traits with mostly environment component.
Large Adaptive Behavior of Hemp Fiber Quality Under Specific Environments
The significant effect of the environment component of all traits suggested strong adaptability of hemp fiber quality to different environmental conditions. Figure 1 shows environmental specific responses or adaptations of these traits in different locations. Plants grown in Netherlands were quite different from plants grown in the other two locations. They produced larger biomass [DW(%), DW_S5(g), lignin content, and GlcA%dm], thicker stems, taller plants, and plants flowered later and over a shorter period than in the other locations. In addition, the decortication parameters [ηDec_1, ηDec_2, and (χ)] showed larger efficiencies in stems from plants grown in this location. In contrast, monosaccharides composing pectin showed the largest contents in plants grown in France while the lowest contents were found in plants grown in Netherlands. Fineness properties followed the same pattern as pectin-related monosaccharides. Finally, plants grown in Italy flowered earlier and over a longer period of time.
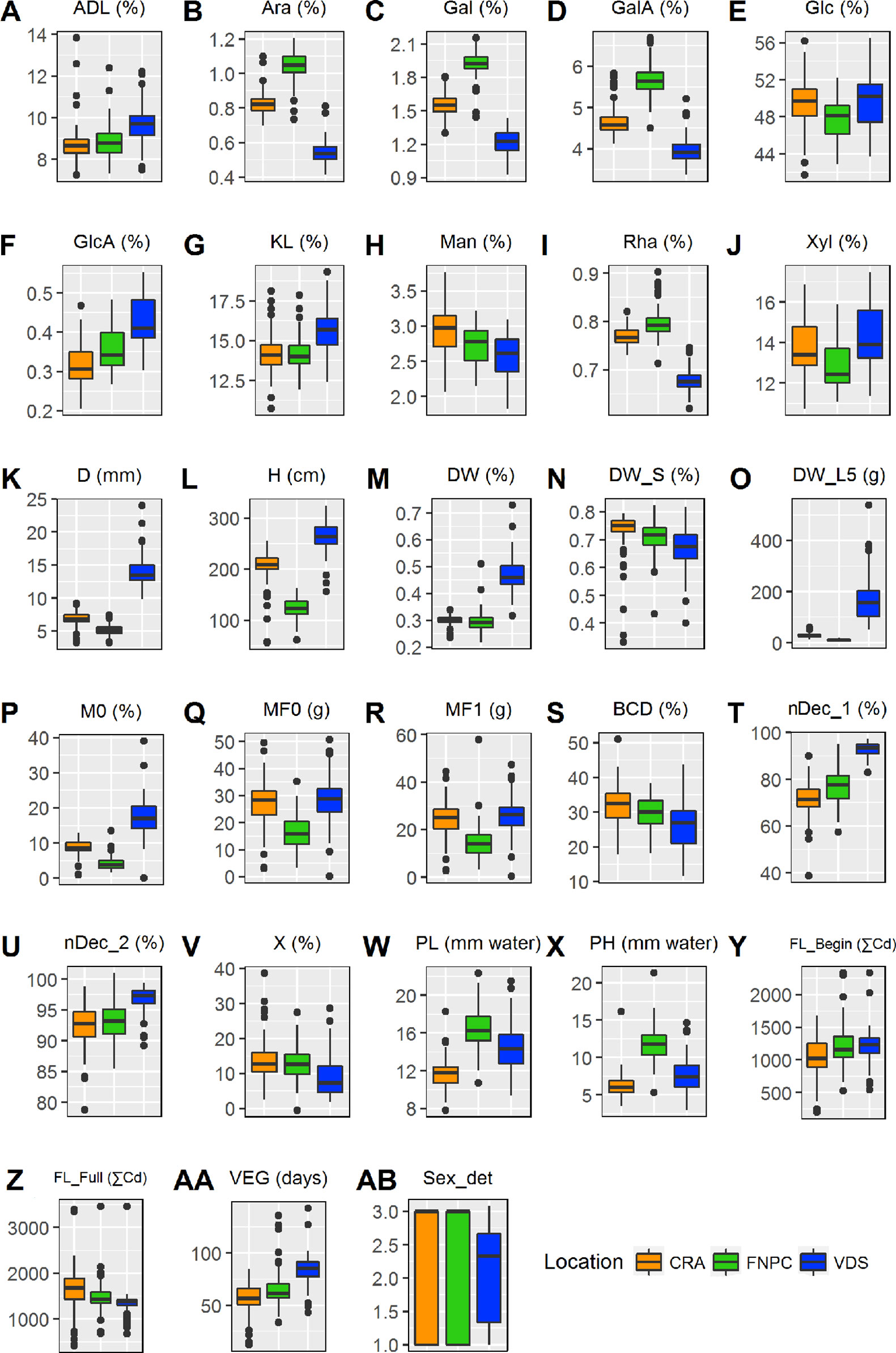
Figure 1 Box plots summarizing the variation of a hemp panel for 28 diverse traits in three locations with contrasting environments. For every box plot, the horizontal line represents the median of the trait, the box represent the interquartile range, the bars outside the box represent the extremes and the crosses indicate the outliers. In every panel, the x-axis indicates the location, as specified in the legend. Range of statistical differences across locations are available in Supplementary Table 3. See Table 3 for abbreviations. Sex_det stands for Sex determination.
Elucidating the Relationships Between Traits Relevant to Hemp Fiber Quality
The fiber trait BCD% showed strong correlations with cell wall components, as detailed in Figure 2 and Supplementary Figure 2. BCD% was positively correlated with Glc%dm and Man%dm (respectively r2 = 0.94 and r2 = 0.82) and negatively correlated with Xyl%dm and GlcA%dm (respectively r2 = -0.91 and r2 = -0.87). In addition, the decortication trait (χ) showed a small but significant positive correlation with GalA%dm (r2 = 0.33). The contents of lignin (KL%dm) and Glc%dm highlighted the largest negative correlation (r2 = -0.93). Lignin measurement ADL%dm was negatively correlated to the fraction of the total dry weight derived from stems [DW_S(%)] and to flowering time traits. Flowering time traits were positively correlated to D and DW_S5(g). Finally, sex determination was positively correlated to the BCD%, Glc%dm, and Man%dm while it was negatively correlated to the contents of lignin (KL%dm), Xyl%dm, GlcA%dm D and flowering time traits. The positive correlations with sex determination were associated to monoecious accessions or accessions with a larger fraction of monoecious plants while the negative correlations were associated to dioecious accessions or accessions with a larger fraction of dioecious plants.
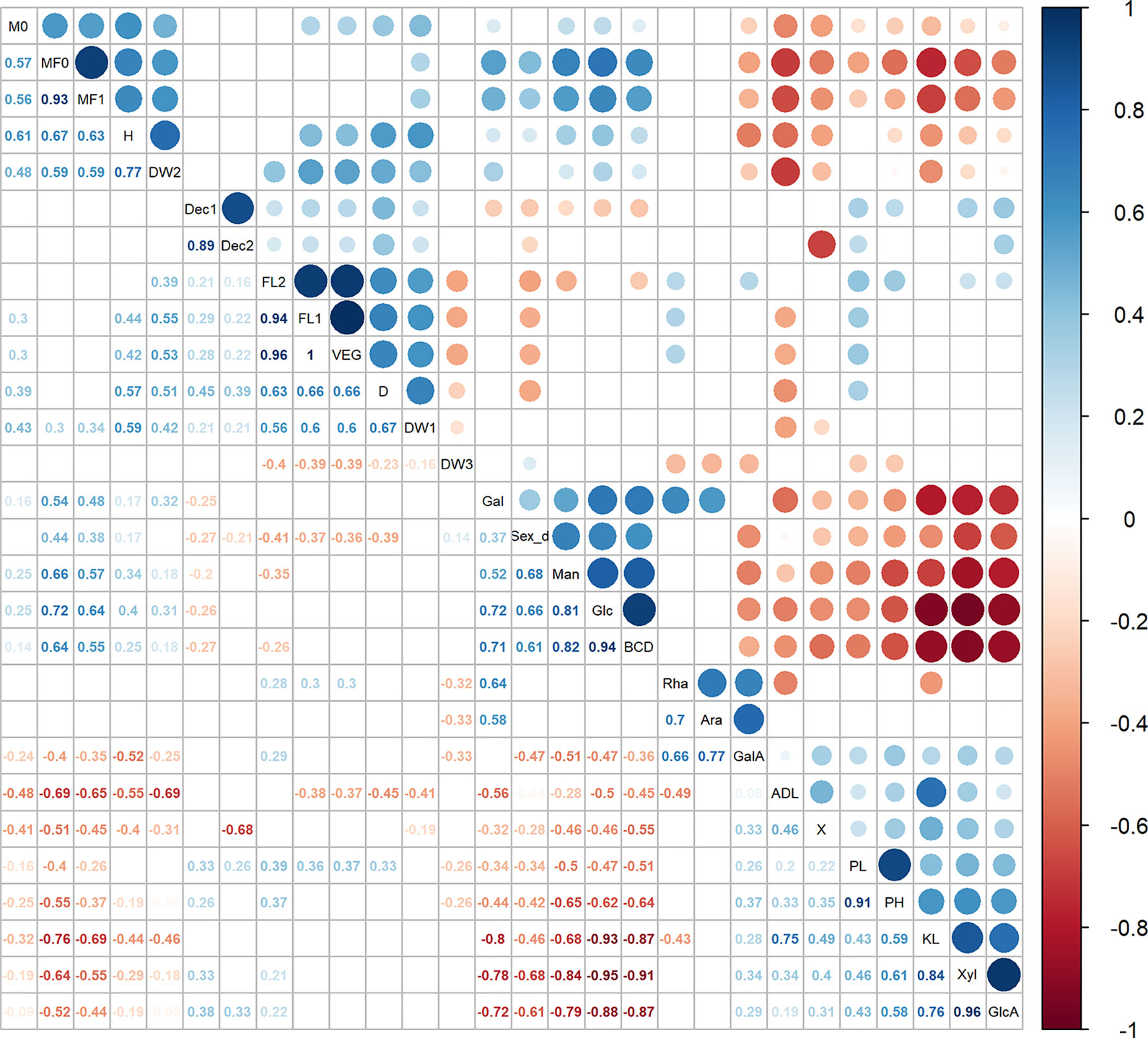
Figure 2 Correlation analysis between 28 agronomic measurements, flowering stages, fiber traits, and cell wall components. Significant correlations were set at a confidence level of 0.95, and blank cells represent no significant correlations. Rha, Rha%dm; Ara, Ara%dm; Gal, Gal%dm; GalA, GalA%dm; Glc, Glc%dm; Man, Man%dm; Xyl, Xyl%dm; GlcA, GlcA%dm; KL, KL%dm; ADL, ADL%dm; FL1, FL_Begin; FL2, FL_Full; Sex_d, Sex determination; DW1, DW_S5(g); DW2, DW_S(%); DW3, DW(%); BCD, BCD%; X, (χ); Dec1, ηDec_1; Dec2, ηDec_2. See Table 3 for abbreviations.
Discussion
Fiber Quality Traits Are Extensively Diverse and Heritable but Also Adaptable to Specific Environments
In the present study, a panel of 123 hemp accessions was used to study the variability in hemp fiber quality and to enlighten key components of this variability. The analysis of the hemp panel revealed extensive variation in 28 fiber quality-related traits among the hemp accessions. In addition, some accessions displayed characteristics that are highly appreciated by the hemp industry. Such traits included large contents of bast fiber and cellulose, low contents of lignin and pectin, fine fiber bundles (high PL and PH values), and late flowering time (Ranalli, 2004; Salentijn et al., 2015). Accessions IWRNZ-902 and WU-111 exhibited several of these phenotypes and thus have a considerably higher quality fiber compared to many other accessions. These accessions indicate valuable germplasm to include in breeding programs, aiming to improve hemp fiber quality.
We observed that important fiber quality traits have a large fraction of heritable phenotypic variation, as indicated by the large G% and H2. As in the present study, extensive genetic studies have shown large heritability values for cell wall components (cellulose, hemicellulose, and lignin) in other fiber crops, such as poplar and eucalyptus (Raymond and Schimleck, 2002; Klasnja et al., 2003; Schimleck et al., 2004; Poke et al., 2006; Davis, 2008), miscanthus (Slavov et al., 2014; Van Der Weijde et al., 2017), switchgrass (Mclaughlin et al., 2006; Boe and Lee, 2007), and maize (Torres et al., 2015). Furthermore, similar heritability values for flowering time were reported in several plant species such as almond [reviewed in Sánchez-Pérez et al. (2014)], apricot (Campoy et al., 2011), arabidopsis (Sasaki et al., 2015), cotton (Kushanov et al., 2017), flax (Soto-Cerda et al., 2014; You et al., 2017), and rice (Takahashi et al., 2001; Huang et al., 2010). It seems plausible that a large fraction of the phenotypic variation of biomass and flowering traits might be controlled by highly “robust genetic systems,” although they are highly complex and polygenic traits, since respectively ~4,000 (Wang et al., 2012) and ~300 genes are estimated to be involved in cell wall synthesis and flowering in arabidopsis (Wang et al., 2012; Bouché et al., 2016). The robust genetic systems might work to control the performance of these traits so that they are less sensitive to environmental differences. This guarantees important functions such as fiber production and reproduction regardless of the environment.
The crucial functions of these traits might support such high heritability values controlled by robust genetic mechanisms. From an evolution point of view, the cell wall performs a structural function in shaping the cells and consequently to plant bodies (Sarkar et al., 2009). Particularly, cellulose and lignin can withstand mechanical pressure exerted by the gravitational pull and the load of the plant body, providing mechanical strength to the plant (Volkmann and Baluška, 2006). In addition, lignin provides protection functions against UV radiation and against pathogens, such as microbes, fungi, and animals, that allowed plants to conquer terrestrial habitats (Iiyama et al., 1994; Popper et al., 2011). Hemicellulosic polysaccharides also provide structural rigidity to the cell walls (Pauly et al., 2013). Hemicellulose and lignin create a matrix around microfibrils of cellulose affecting the recalcitrance of the cell walls (Torres et al., 2015). Furthermore, flowering is an essential biological process for many plants as the survival of the species depends on it (Mouradov et al., 2002). Consequently, these biomass and flowering traits seem to perform essential roles that cannot be widely modified, as the consequences might be lethal for the plant.
Furthermore, we observed that hemp fiber quality-related traits are not strongly stable across environments, as indicated by the significance of G×E interaction components. The results showed that some accessions were more sensitive in some environments than in others. It seems likely that the phenotypic variation associated to the G×E interactions might be controlled by “plastic genetic systems” where certain genes are expressed when combined with specific environmental conditions. This is the first study describing significant G×E interactions in fiber and flowering traits in hemp. Studying these interactions is important owing to their implications for the setup of selection experiments, as the ranking of accessions is dependent on the environment (Van Der Weijde et al., 2017). Selection for traits with large G×E interactions in breeding programs might lead to biased selection decisions, owing to the unknown effects in untested environments. Nonetheless, the G×E interactions were small in most important cell wall components (contents of glucose, mannose, xylose, ADL, and KL), fiber content, and flowering traits in hemp. Similar small G×E interactions have been shown for biomass traits, especially contents of cellulose, hemicellulose, and lignin, in several fiber crops, such as alfalfa (Sheaffer et al., 1998), maize (Dolstra et al., 1992; Cox et al., 1994; Argillier et al., 1997; Barrière et al., 2008; Torres et al., 2015), miscanthus (Van Der Weijde et al., 2017), and switchgrass (Hopkins et al., 1995). Therefore, the extent of the G×E interaction effects on these fiber quality-related traits might not strongly affect the ordering of hemp accessions across environments and might not interfere in selection decisions.
Interesting examples of large G×E interactions in hemp are the contents of some pectin-related monosaccharides. The evolution and the functions of pectin in plants might explain these results. In the stem of plants, pectins are mostly present in the middle lamella between cells and are involved in the intracellular adhesion, providing integrity and rigidity to plant tissues and to the stem. They also play important roles in the defence mechanisms against pathogens. In addition, they are involved in the regulation of the ion transport and in the water holding capacity (Voragen et al., 2009). Yet, pectic polysaccharides are highly dynamic structures, and their content dramatically changes across tissues and plant species (Willats et al., 2001). Pectin has almost disappeared in the stems from several modern plants, such as grasses (Carpita and Gibeaut, 1993; Carpita, 1996; Sarkar et al., 2009; Voragen et al., 2009), suggesting that their important functions might be evolutionary replaced by other cell wall components. Lignin is the newest cell wall component to appear in plants and has some parallel functions with pectin, such as structural support and defence functions (Sarkar et al., 2009). As a consequence, dramatic changes of pectin content might not be lethal to plants, owing to a putative partial compensation from other cell wall components, which might allow larger plasticity in sensitivity of certain accessions in different environments.
Fiber quality-related traits were strongly influenced by the differences of the environments across the trial locations. Previous studies have reported large sensitivity of hemp to the environment, particularly to the photoperiod and temperature regimes, affecting the vegetative growth and flowering of the plants (Faux et al., 2013; Amaducci et al., 2015; Sawler et al., 2015). This large sensitivity can be understood as a strong general response of hemp accessions to adapt to the environment, independently of the heritable genetic control of the traits described in previous paragraphs. This behavior might be the result of the optimization of the plant fitness under specific environmental conditions. Consequently, the environment of the growing locations should be taken into account when selecting the cultivation purpose of hemp, and subsequent breeding should be done for use in a specific environment.
An example of the adaptive behavior of hemp is the difference in biomass production and flowering time across environments. Plants of the same accession grown in the Netherlands produced larger biomass and flowered later than plants grown in the other two locations. These variations can be explained by differences in photoperiod regimes across locations. Hemp is a short-day plant, and the length of the vegetative growth period depends on the shift from long- to short-day photoperiod regimes (Amaducci et al., 2012). The vegetative growth period is characterized by biomass production, after which this behavior shifts toward fiber maturation (secondary fiber formation and lignification) and plant reproduction during flowering development (Van Der Werf and Turunen, 2008; Liu et al., 2015). At northern latitudes, the shift from long-day toward short-day photoperiod regimes occurs later and therefore the critical photoperiod for flowering (~14–16 h) is reached later in the growing season of hemp (Struik et al., 2000; Hall et al., 2012). As a result, the cultivation of hemp focused on the production of fibers may be better in northern latitudes, while the cultivation of hemp for seeds or dual purpose seed/fiber in southern latitudes may be more adapted to the environment (Amaducci et al., 2015). The selection of the cultivation purposes based on the environment might increase the profitability of hemp cultivation, complementing the high fiber quality achieved by the breeding programs using the heritable phenotypic variation.
Another example of the adaptive behavior of hemp's biomass is the difference in production of monosaccharides composing pectin from plants across environments. Plants grown in France showed the largest content of monosaccharides composing pectin while plants of the same accessions in the Netherlands showed the lowest contents. It has been previously reported that pectin plays a role in modulating cell wall architecture in response to low availability of water [reviewed in Le Gall et al. (2015)], owing to its water holding capacity function (Voragen et al., 2009). As shown in Table 2, France had lower rainfall and larger days without rain than Netherlands. Based on the environmental differences between the locations and the functions of pectin, it seems plausible to hypothesize that hemp plants may increase the content of pectin in the stem partially as a response to the changes in water availability. This relationship may have important implications in the improvement of the fiber quality of hemp, as the contents of monosaccharides composing pectin are poorly heritable traits and pectin plays a key role in the fiber quality. Pectin has been associated to difficulties in decortication which results in increased fiber damage (Ranalli, 2004; Müssig et al., 2008; Salentijn et al., 2015) after fiber decortication. Furthermore, plants grown in Netherlands showed larger decortication efficiencies than in the other two locations, and the content of galacturonic acid, the main component of pectin (Willats et al., 2001), was positively correlated to the shive content after decortication (χ). These results indicate that lower contents of galacturonic acid in the stems results in easier decortication. In addition, it suggests that water availability may play a role in the pectin content, and if that is the case, it could be used as a tool to improve fiber quality associated to poor heritable traits. Crop management, such as irrigation, could contribute to the decrease in the pectin content and thus improve the quality. The present study provides interesting results to further investigate the influence of water availability on pectin content of hemp. The use of a wider range of locations with contrasting and more detailed environmental conditions and the use of controlled experiments may be useful to get insights into the role of specific environmental factors in hemp fiber quality.
Finally, the correlation analysis revealed that monoecious and dioecious plants have a different relationship with fiber quality. The results of the hemp panel analysis confirm that monoecious accessions have larger fiber qualities than dioecious. These differences may be explained by the larger uniformity in fiber production common in monoecious accessions compared to dioecious accessions (Mandolino and Carboni, 2004; Amaducci and Gusovius, 2010; Faux et al., 2013; Faux et al., 2014; Salentijn et al., 2015; Faux et al., 2016). Finally, the sex determination of hemp is another key element that should be taken into account when selecting the germplasm for breeding programs as it has important implications in fiber quality (Amaducci and Gusovius, 2010; Amaducci et al., 2015).
Implications of the Fiber Quality Variability in the Development of New Hemp Cultivars With Improved Fiber Properties
In the present study, the extent of fiber quality variation among accessions reveals a good hemp panel to further study the genetic architecture of fiber quality, flowering, and sex characteristics of hemp. As Davis (2008) described in a previous study, in order to genetically improve some traits, they must be heritable. The contents of most cell wall components [glucose, mannose, xylose, glucuronic acid, and lignin (ADL and KL)], content of bast fiber, flowering time measurements, and sex determination of hemp have high heritability values, meaning that they are good candidates for genetic association studies. The selection of plants harboring favorable alleles for these traits would maximize the genetic gains expected from the breeding programs aiming to increase the quality of the bast fiber. However, traits with low genetic components and relatively large G×E interactions, such as monosaccharides composing pectin, are not appropriate candidates for mapping studies, as the statistical tools currently available have low power to discriminate between phenotypic variations owing to genetic or environmental effects, when the genetic components are small. As a result, the statistical power of the association for these traits would drop, leading to high false-positives and/or false-negative rates (Huang and Han, 2014; Bernardo, 2016). Yet, the large adaptive behavior of these traits suggests that crop management practices may be a good alternative to breeding for traits poorly heritable. Therefore, the combination of breeding programs to target traits with large genetic components and crop management for traits with small genetic component may be a good strategy to improve the potential of hemp as a high-yielding, sustainable crop of excellent fibers.
Conclusions
The results of this study prescribe positive prospects for the development of new hemp cultivars with improved fiber quality properties. In particular, the hemp accession panel reveals to be a good dataset for mapping studies owing to the extensive phenotypic variability of 28 fiber quality-related traits. The content of most cell wall components (cellulose, hemicellulose, and lignin), bast fiber content, flowering time traits, and sex determination showed large heritable variation, controlled by robust genetic mechanisms that can be used in breeding programs. In addition, all traits showed statistically significant G×E interaction components in different percentages depending on the traits. These results suggest that the phenotypic variation in fiber quality of hemp has a fraction of heritable variation sensitive to the environment, controlled by plastic genetic mechanisms.
In addition, fiber quality traits were strongly affected by the environment, such as photoperiod and temperature regimes and probably water availability. These sensitivities can be understood as adaptations to the environment, independently of the heritable genetic variation. The adaptive behavior of poorly heritable traits, such as pectin, might be used to develop strategies, such as crop management practices, to increase fiber quality alternatively to breeding programs. Finally, the correlation analysis revealed that monoecious plants have larger fiber quality than dioecious hemp owing to probably uniformity in fiber production common in monoecious accessions. Altogether, we advocate for novel hemp breeding programs that breed for highly heritable traits, taking into account the sex determination of the germplasm in the breeding schemes and considering the environmental sensitivity of fiber quality.
Data Availability Statement
All datasets generated for this study are included in the article/Supplementary Material.
Author Contributions
JP designed and performed the experiments, analyzed the data, and wrote the manuscript. ES helped designing and performing the experiments, helped analyzing the data, and revised the manuscript. M-JP helped analyzing the data and revised the manuscript. CT, BD, and GM performed field trials and revised the manuscript. H-JG, KT, SA, SW, BU, and JM performed experiments and revised the manuscript. LT coordinated and supervised this study, defined the experimental strategy, discussed the outcomes, and revised the manuscript.
Funding
This work was conducted as part of the MultiHemp project (Multipurpose hemp for industrial bioproducts and biomass) funded by the European Union's Seventh Framework Programme for research, technological developments, and demonstration under grant agreement number 311849.
Conflict of Interest
The authors declare that the research was conducted in the absence of any commercial or financial relationships that could be construed as a potential conflict of interest.
Acknowledgments
The authors are grateful to Annemarie Dechesne, Wen Fang, Dominik Ewald, Wei Xiong, and Li Gu from Wageningen University and Research for their technical assistance during laboratory analysis. The authors also gratefully acknowledge the help of Salvatore Musio from Università Cattolica del Sacro Cuore, Carsten Lühr from Leibniz-Institut für Agrartechnik Potsdam-Bornim e.V., and Felix Beck, Christopher Graf, Kevin Janke, Niklas Lehmensieck, Anne-Kristin Lenz, Sebastian Mulot, Fabian Plum, Simon Riechel, Tobias Riphaus, Fabian Uhrlaub, and Timo Wunsch from City University of Applied Sciences Bremen for their help during harvest of the field trials and decortication of stems.
Supplementary Material
The Supplementary Material for this article can be found online at: https://www.frontiersin.org/articles/10.3389/fpls.2020.00102/full#supplementary-material
References
Amaducci, S., Gusovius, H. J. (2010). “Hemp-Cultivation, Extraction and Processing,” in Industrial Applications of Natural Fibres: Structure, Properties and Technical Applications. Ed. Müssig, J. (Chichester, UK: John Wiley & Sons). 109–134. doi: 10.1002/9780470660324.ch5
Amaducci, S., Colauzzi, M., Bellocchi, G., Cosentino, S. L., Pahkala, K., Stomph, T. J., et al. (2012). Evaluation of a phenological model for strategic decisions for hemp (Cannabis Sativa L.) biomass production across European sites. Ind. Crop Prod. 37, 100–110. doi: 10.1016/j.indcrop.2011.11.012
Amaducci, S., Scordia, D., Liu, F. H., Zhang, Q., Guo, H., Testa, G., et al. (2015). Key cultivation techniques for hemp in Europe and China. Ind. Crop Prod. (Chichester, UK: John Wiley & Sons) 68, 2–16. doi: 10.1016/j.indcrop.2014.06.041
Argillier, O., Barrière, Y., Traineau, R., Emile, J. C., Hebert, Y. (1997). Genotype × environment interactions for digestibility traits in silage maize estimated from in vivo measurements with standard sheep. Plant Breed. 116, 423–427. doi: 10.1111/j.1439-0523.1997.tb01025.x
Barrière, Y., Thomas, J., Denoue, D. (2008). QTL mapping for lignin content, lignin monomeric composition, p-hydroxycinnamate content, and cell wall digestibility in the maize recombinant inbred line progeny F838×F286. Plant Sci. 175, 585–595. doi: 10.1016/j.plantsci.2008.06.009
Bernardo, R. (2016). Bandwagons I, too, have known. Theor. Appl. Genet. 129, 2323–2332. doi: 10.1007/s00122-016-2772-5
Boe, A., Lee, D. K. (2007). Genetic Variation for Biomass Production in Prairie Cordgrass and Switchgrass. Crop Sci. 47, 959–934. doi: 10.2135/cropsci2006.05.0323
Bouché, F., Lobet, G., Tocquin, P., Perilleux, C. (2016). FLOR-ID: an interactive database of flowering-time gene networks in Arabidopsis thaliana. Nucleic Acids Res. 44, D1167–D1171. doi: 10.1093/nar/gkv1054
Campoy, J. A., Ruiz, D., Egea, J., Rees, D. J. G., Celton, J. M., Martínez-Gómez, P. (2011). Inheritance of flowering time in apricot (Prunus armeniaca L.) and analysis of linked quantitative trait Loci (QTLs) using simple sequence repeat (SSR) markers. Plant Mol. Biol. Rep. 29, 404–410. doi: 10.1007/s11105-010-0242-9
Carpita, N. C., Gibeaut, D. M. (1993). Structural models of primary cell walls in flowering plants: consistency of molecular structure with the physical properties of the walls during growth. Plant J. 3, 1–30. doi: 10.1111/j.1365-313X.1993.tb00007.x
Carpita, N. C. (1996). Structure and biogenesis of the cell walls of grasses. Annu. Rev. Plant Physiol. Plant Mol. Biol. 47, 445–476. doi: 10.1146/annurev.arplant.47.1.445
Chailakhyan, M. K., Khryanin, V. N. (1978). The influence of growth regulators absorbed by the root on sex expression in hemp plants. Planta 138, 181–184. doi: 10.1007/BF00391176
Cox, W. J., Cherney, J. H., Pardee, W. D., Cherney, D. J. R. (1994). Forage quality and harvest index of corn hybrids under different growing conditions. Agron. J. 86, 277–282. doi: 10.2134/agronj1994.00021962008600020013x
Davis, J. M. (2008). “Genetic Improvement of Poplar (Populus spp.) as a bioenergy crop,” in Genetic Improvement of Bioenergy Crops (New York: Springer). Ed. Vermerris, W., 1–449.
Dolstra, O., Medema, J. H., De Jong, A. W. (1992). Genetic improvement of cell-wall digestibility in forage maize (Zea mays L.). I. Performance of inbred lines and related hybrids. Euphytica 65, 187–194. doi: 10.1007/BF00023082
Faux, A. M., Draye, X., Lambert, R., D'andrimont, R., Raulier, P., Bertin, P. (2013). The relationship of stem and seed yields to flowering phenology and sex expression in monoecious hemp (Cannabis sativa L.). Eur. J. Agron. 47, 11–22. doi: 10.1016/j.eja.2013.01.006
Faux, A. M., Berhin, A., Dauguet, N., Bertin, P. (2014). Sex chromosomes and quantitative sex expression in monoecious hemp (Cannabis sativa L.). Euphytica 196, 183–197. doi: 10.1007/s10681-013-1023-y
Faux, A. M., Draye, X., Flamand, M. C., Occre, A., Bertin, P. (2016). Identification of QTLs for sex expression in dioecious and monoecious hemp (Cannabis sativa L.). Euphytica 209, 357–376. doi: 10.1007/s10681-016-1641-2
Freeman, D. C., Harper, K. T., Charnov, E. L. (1980). Sex change in plants: old and new observations and new hypotheses. Oecologia 47, 222–232. doi: 10.1007/BF00346825
Galoch, E. (2015). The hormonal control of sex differentiation in dioecious plants of hemp (Cannabis sativa L.). The influence of plant growth regulators on sex expression in male and female plants. Acta Soc Bot. Pol. 47, 153–162. doi: 10.5586/asbp.1978.013
Gitonga, V. W., Koning-Boucoiran, C. F., Verlinden, K., Dolstra, O., Visser, R. G., Maliepaard, C., et al. (2014). Genetic variation, heritability and genotype by environment interaction of morphological traits in a tetraploid rose population. BMC Genet. 15, 146. doi: 10.1186/s12863-014-0146-z
Hall, J., Bhattarai, S. P., Midmore, D. J. (2012). Review of flowering control in industrial Hemp. J. Nat. Fibers 9, 23–36. doi: 10.1080/15440478.2012.651848
Heikrujam, M., Sharma, K., Prasad, M., Agrawal, V. (2014). Review on different mechanisms of sex determination and sex- linked molecular markers in dioecious crops- A current update. Euphytica 201, 161–194. doi: 10.1007/s10681-014-1293-z
Hopkins, A. A., Vogel, K. P., Moore, K. J., Johnson, K. D., Carlson, I. T. (1995). Genotypic variability and genotype × environment interactions among switchgrass accessions from the midwestern USA. Crop Sci. 35, 565–571. doi: 10.2135/cropsci1995.0011183X003500020047x
Huang, X., Han, B. (2014). Natural variations and genome-wide association studies in crop plants. Ann. Rev. Plant Biol. 65, 531–551. doi: 10.1146/annurev-arplant-050213-035715
Huang, X., Wei, X., Sang, T., Zhao, Q., Feng, Q., Zhao, Y., et al. (2010). Genome-wide association studies of 14 agronomic traits in rice landraces. Nat. Genet. 42, 961–967. doi: 10.1038/ng.695
Iiyama, K., Lam, T. B. T., Stone, B. A. (1994). Covalent cross-links in the cell wall. Plant Physiol. 104, 315–320. doi: 10.1104/pp.104.2.315
Klasnja, B., Kopitovic, S., Orlovic, S. (2003). Variability of some wood properties of eastern cottonwood (Populus deltoides Bartr.) clones. Wood Sci. Technol. 37, 331–337. doi: 10.1007/s00226-003-0179-3
Kushanov, F. N., Buriev, Z. T., Shermatov, S. E., Turaev, O. S., Norov, T. M., Pepper, A. E., et al. (2017). QTL mapping for flowering-time and photoperiod insensitivity of cotton Gossypium darwinii Watt. PLoS One 12, e0186240–e0186240. doi: 10.1371/journal.pone.0186240
Le Gall, H., Philippe, F., Domon, J. M., Gillet, F., Pelloux, J., Rayon, C. (2015). Cell Wall Metabolism in Response to Abiotic Stress. Plants-Basel 4, 112–166. doi: 10.3390/plants4010112
Liu, M., Fernando, D., Daniel, G., Madsen, B., Meyer, A. S., Ale, M. T., et al. (2015). Effect of harvest time and field retting duration on the chemical composition, morphology and mechanical properties of hemp fibers. Ind. Crop Prod. 69, 29–39. doi: 10.1016/j.indcrop.2015.02.010
Mandolino, G., Carboni, A. (2004). Potential of marker-assisted selection in hemp genetic improvement. Euphytica 140, 107–120. doi: 10.1007/s10681-004-4759-6
Mclaughlin, S. B., Kiniry, J. R., Taliaferro, C. M., De La Torre Ugarte, D. (2006). “Projecting Yield and Utilization Potential of Switchgrass as an Energy Crop,” in Advances in Agronomy (Academic Press), 267–297. doi: 10.1016/S0065-2113(06)90007-8
Meijer, E. P. M. D., Keizer, L. C. P. (1996). Patterns of diversity in Cannabis. Genet. Resour. Crop Evol. 43, 41–52. doi: 10.1007/BF00126939
Meijer, E. P. M. D., Van Der Kamp, H. J., Van Eeuwijk, F. A. (1992). Characterisation of Cannabis accessions with regard to cannabinoid content in relation to other plant characters. Euphytica 62, 187–200. doi: 10.1007/BF00041753
Moliterni, V. M. C., Cattivelli, L., Ranalli, P., Mandolino, G. (2004). The sexual differentiation of Cannabis sativa L.: a morphological and molecular study. Euphytica 140, 95–106. doi: 10.1007/s10681-004-4758-7
Montalvo, J. G., Faught, S. E. (1999). Headspace resistance standards for the Shirley developments Ltd. micromat tester. Tex. Res. J. 69, 269–277. doi: 10.1177/004051759906900406
Mouradov, A., Cremer, F., Coupland, G. (2002). Control of flowering time: interacting pathways as a basis for diversity. Plant Cell 14 Suppl, S111–S130. doi: 10.1105/tpc.001362
Müssig, J., Amaducci, S. (2018). Scanner based image analysis to characterise the influence of agronomic factors on hemp (Cannabis sativa L.) fibre width. Ind. Crop Prod. 113, 28–37. doi: 10.1016/j.indcrop.2017.12.059
Müssig, J., Zatta, A., Venturi, G. (2008). “An innovative production system for hemp fibre for textile destinations: from laboratory results to industrial validation,” in International Conference on Flax and Other Bast Plants(Saskatoon, Canada: International Conference on Flax and Other Bast Plants ).
Müssig, J., Fischer, H., Graupner, N., Drieling, A. (2010). “Testing methods for measuring physical and mechanical fibre properties (Plant and Animal Fibres,” in Industrial Applications of Natural Fibres. (Chichester, UK: John Wiley & Sons) Ed. Müssig, J.. doi: 10.1002/9780470660324.ch13
Müssig, J. (2001). Untersuchung der Eignung heimischer Pflanzenfasern für die Herstellung von naturfaserverstärkten Duroplasten - vom Anbau zum Verbundwerkstoff (Düsseldorf, DE: VDI Verlag GmbH). (ISBN 3-18-363005-2).
Pauly, M., Gille, S., Liu, L., Mansoori, N., De Souza, A., Schultink, A., et al. (2013). Hemicellulose biosynthesis. Planta 238, 627–642. doi: 10.1007/s00425-013-1921-1
Petit, J., Gulisano, A., Dechesne, A., Trindade, L. M. (2019). Phenotypic variation of cell wall composition and stem morphology in Hemp (Cannabis sativa L.): optimization of methods. Front. Plant Sci. 10. doi: 10.3389/fpls.2019.00959
Poke, F. S., Potts, B. M., Vaillancourt, R. E., Raymond, C. A. (2006). Genetic parameters for lignin, extractives and decay in Eucalyptus globulus. Ann. For. Sci. 63, 813–821. doi: 10.1051/forest:2006080
Popper, Z. A., Michel, G., Herve, C., Domozych, D. S., Willats, W. G., Tuohy, M. G., et al. (2011). Evolution and diversity of plant cell walls: from algae to flowering plants. Annu. Rev. Plant Biol. 62, 567–590. doi: 10.1146/annurev-arplant-042110-103809
Ranalli, P. (2004). Current status and future scenarios of hemp breeding. Euphytica 140, 121–131. doi: 10.1007/s10681-004-4760-0
Raymond, C. A., Schimleck, L. R. (2002). Development of near infrared reflectance analysis calibrations for estimating genetic parameters for cellulose content in Eucalyptus globulus. Can. J. For. Res. 32, 170–176. doi: 10.1139/x01-174
Renaud, E. N., Lammerts Van Bueren, E. T., Myers, J. R., Paulo, M. J., Van Eeuwijk, F. A., Zhu, N., et al. (2014). Variation in broccoli cultivar phytochemical content under organic and conventional management systems: implications in breeding for nutrition. PLoS One 9, e95683. doi: 10.1371/journal.pone.0095683
Sánchez-Pérez, R., Del Cueto, J., Dicenta, F., Martínez-Gómez, P. (2014). Recent advancements to study flowering time in almond and other Prunus species. Front. Plant Sci. 5, 334–334. doi: 10.3389/fpls.2014.00334
Salentijn, E. M. J., Zhang, Q., Amaducci, S., Yang, M., Trindade, L. M. (2015). New developments in fiber hemp (Cannabis sativa L.) breeding. Ind. Crop Prod. 68, 32–41. doi: 10.1016/j.indcrop.2014.08.011
Salentijn, E. M. J., Petit, J., Trindade, L. M. (2019). The complex interactions between flowering behavior and fiber quality in Hemp. Front. Plant Sci. 10. doi: 10.3389/fpls.2019.00614
Sarkar, P., Bosneaga, E., Auer, M. (2009). Plant cell walls throughout evolution: towards a molecular understanding of their design principles. J. Exp. Bot. 60, 3615–3635. doi: 10.1093/jxb/erp245
Sasaki, E., Zhang, P., Atwell, S., Meng, D., Nordborg, M. (2015). “Missing” G x E variation controls flowering time in Arabidopsis thaliana. PLoS Genet. 11, e1005597–e1005597. doi: 10.1371/journal.pgen.1005597
Sawler, J., Stout, J. M., Gardner, K. M., Hudson, D., Vidmar, J., Butler, L., et al. (2015). The genetic structure of marijuana and Hemp. PLoS One 10, e0133292. doi: 10.1371/journal.pone.0133292
Schimleck, L. R., Kube, P. D., Raymond, C. A. (2004). Genetic improvement of kraft pulp yield in Eucalyptus nitens using cellulose content determined by near infrared spectroscopy. Can. J. For. Res. 34, 2363–2370. doi: 10.1139/x04-119
Sheaffer, C. C., Cash, D., Ehlke, N. J., Henning, J. C., Jewett, J. G., Johnson, K. D., et al. (1998). Entry ✕ Environment Interactions for Alfalfa Forage Quality. Agron. J. 90, 774–780. doi: 10.2134/agronj1998.00021962009000060010x
Slavov, G. T., Nipper, R., Robson, P., Farrar, K., Allison, G. G., Bosch, M., et al. (2014). Genome-wide association studies and prediction of 17 traits related to phenology, biomass and cell wall composition in the energy grass Miscanthus sinensis. New Phytol. 201, 1227–1239. doi: 10.1111/nph.12621
Soldatova, N. A., Khryanin, V. N. (2010). The effects of heavy metal salts on the phytohormonal status and sex expression in marijuana. Russ. J. Plant Physl. 57, 96–100. doi: 10.1134/S1021443710010139
Soto-Cerda, B. J., Duguid, S., Booker, H., Rowland, G., Diederichsen, A., Cloutier, S. (2014). Genomic regions underlying agronomic traits in linseed (Linum usitatissimum L.) as revealed by association mapping. J. Integr. Plant Biol. 56, 75–87. doi: 10.1111/jipb.12118
Struik, P. C., Amaducci, S., Bullard, M. J., Stutterheim, N. C., Venturi, G., Cromack, H. T. H. (2000). Agronomy of fibre hemp (Cannabis sativa L.) in Europe. Ind. Crop Prod. 11, 107–118. doi: 10.1016/S0926-6690(99)00048-5
Takahashi, Y., Shomura, A., Sasaki, T., Yano, M. (2001). Hd6, a rice quantitative trait locus involved in photoperiod sensitivity, encodes the α subunit of protein kinase CK2. Proc. Natl. Acad. Sci. 98, 7922–7927. doi: 10.1073/pnas.111136798
Torres, A. F., Noordam-Boot, C. M. M., Dolstra, O., Van Der Weijde, T., Combes, E., Dufour, P., et al. (2015). Cell wall diversity in forage maize: genetic complexity and bioenergy potential. Bioenergy Res. 8, 187–202. doi: 10.1007/s12155-014-9507-8
Van Den Oever, M. J. A., Bas, N., Van Soest, L. J. M., Melis, C., Van Dam, J. E. G. (2003). Improved method for fibre content and quality analysis and their application to flax genetic diversity investigations. Ind. Crop Prod. 18, 231–243. doi: 10.1016/S0926-6690(03)00063-3
Van Der Weijde, T., Torres, A. F., Dolstra, O., Dechesne, A., Visser, R. G. F., Trindade, L. M. (2016). Impact of different lignin fractions on Saccharification efficiency in diverse species of the bioenergy crop Miscanthus. Bioenerg. Res. 9, 146–156. doi: 10.1007/s12155-015-9669-z
Van Der Weijde, T., Dolstra, O., Visser, R. G. F., Trindade, L. M. (2017). Stability of cell wall composition and saccharification efficiency in miscanthus across diverse environments. Front. Plant Sci. 7. doi: 10.3389/fpls.2016.02004
Van Der Werf, H. M. G., Turunen, L. (2008). The environmental impacts of the production of hemp and flax textile yarn. Ind. Crop Prod. 27, 1–10. doi: 10.1016/j.indcrop.2007.05.003
Volkmann, D., Baluška, F. (2006). Gravity: one of the driving forces for evolution. Protoplasma 229, 143–148. doi: 10.1007/s00709-006-0200-4
Voragen, A. G. J., Coenen, G.-J., Verhoef, R. P., Schols, H. A. (2009). Pectin, a versatile polysaccharide present in plant cell walls. Struct. Chem. 20, 263. doi: 10.1007/s11224-009-9442-z
Wang, S., Yin, Y., Ma, Q., Tang, X., Hao, D., Xu, Y. (2012). Genome-scale identification of cell-wall related genes in Arabidopsis based on co-expression network analysis. BMC Plant Biol. 12, 138–138. doi: 10.1186/1471-2229-12-138
Wang, S., Gusovius, H.-J., Lühr, C., Musio, S., Uhrlaub, B., Amaducci, S., et al. (2018). Assessment system to characterise and compare different hemp varieties based on a developed lab-scaled decortication system. Ind. Crop Prod. 117, 159–168. doi: 10.1016/j.indcrop.2018.02.083
Willats, W. G. T., Mccartney, L., Mackie, W., Knox, J. P. (2001). Pectin: cell biology and prospects for functional analysis. Plant Mol. Biol. 47, 9–27. doi: 10.1023/A:1010662911148
Keywords: genetic variability, genotype-by-environment (G×E) interactions, hemp, Cannabis sativa, fiber quality, cell wall composition, flowering time, sex determination
Citation: Petit J, Salentijn EMJ, Paulo M-J, Thouminot C, van Dinter BJ, Magagnini G, Gusovius H-J, Tang K, Amaducci S, Wang S, Uhrlaub B, Müssig J and Trindade LM (2020) Genetic Variability of Morphological, Flowering, and Biomass Quality Traits in Hemp (Cannabis sativa L.). Front. Plant Sci. 11:102. doi: 10.3389/fpls.2020.00102
Received: 11 September 2019; Accepted: 23 January 2020;
Published: 20 February 2020.
Edited by:
Soren K. Rasmussen, University of Copenhagen, DenmarkReviewed by:
Axel Diederichsen, Agriculture and Agri-Food Canada (AAFC), CanadaShivali Sharma, International Crops Research Institute for the Semi-Arid Tropics (ICRISAT), India
Copyright © 2020 Petit, Salentijn, Paulo, Thouminot, van Dinter, Magagnini, Gusovius, Tang, Amaducci, Wang, Uhrlaub, Müssig and Trindade. This is an open-access article distributed under the terms of the Creative Commons Attribution License (CC BY). The use, distribution or reproduction in other forums is permitted, provided the original author(s) and the copyright owner(s) are credited and that the original publication in this journal is cited, in accordance with accepted academic practice. No use, distribution or reproduction is permitted which does not comply with these terms.
*Correspondence: Luisa M. Trindade, bHVpc2EudHJpbmRhZGVAd3VyLm5s