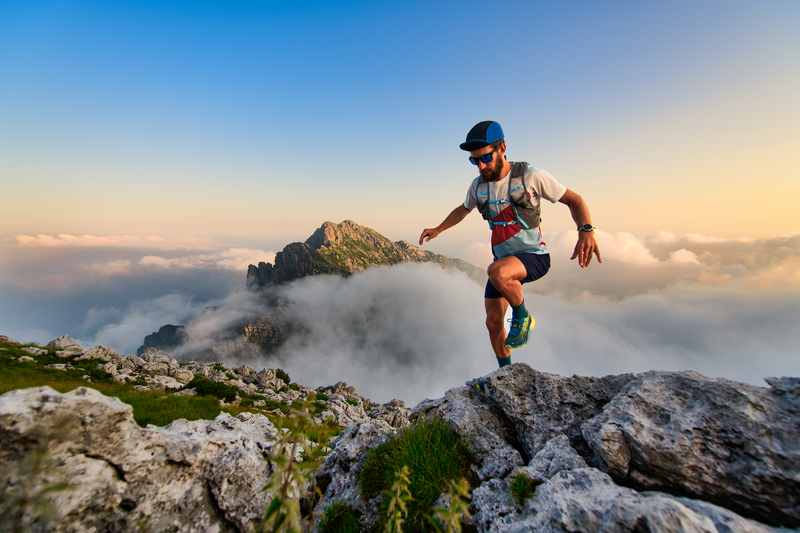
94% of researchers rate our articles as excellent or good
Learn more about the work of our research integrity team to safeguard the quality of each article we publish.
Find out more
REVIEW article
Front. Plant Sci. , 25 February 2020
Sec. Plant Biotechnology
Volume 11 - 2020 | https://doi.org/10.3389/fpls.2020.00003
This article is part of the Research Topic Proceedings of ICPSBBB 2018 - 2nd International Conference on Plant Synthetic Biology, Bioengineering and Biotechnology View all 9 articles
Impacts of climate change like global warming, drought, flooding, and other extreme events are posing severe challenges to global crop production. Contribution of Brassica napus towards the oilseed industry makes it an essential component of international trade and agroeconomics. Consequences from increasing occurrences of multiple abiotic stresses on this crop are leading to agroeconomic losses making it vital to endow B. napus crop with an ability to survive and maintain yield when faced with simultaneous exposure to multiple abiotic stresses. For an improved understanding of the stress sensing machinery, there is a need for analyzing regulatory pathways of multiple stress-responsive genes and other regulatory elements such as non-coding RNAs. However, our understanding of these pathways and their interactions in B. napus is far from complete. This review outlines the current knowledge of stress-responsive genes and their role in imparting multiple stress tolerance in B. napus. Analysis of network cross-talk through omics data mining is now making it possible to unravel the underlying complexity required for stress sensing and signaling in plants. Novel biotechnological approaches such as transgene-free genome editing and utilization of nanoparticles as gene delivery tools are also discussed. These can contribute to providing solutions for developing climate change resilient B. napus varieties with reduced regulatory limitations. The potential ability of synthetic biology to engineer and modify networks through fine-tuning of stress regulatory elements for plant responses to stress adaption is also highlighted.
Plants as sessile organisms frequently face environmental conditions hostile to their growth and development. These disruptive environmental conditions are in the form of various abiotic and biotic stresses. Abiotic stresses have been predicted to limit global crop production by almost 70% (Boyer, 1982). Climate change has increased the frequency of adverse events, with simultaneous occurrence of multiple abiotic stresses leading to exacerbated negative impacts. Abiotic stresses include heat, cold, drought, salinity, waterlogging, heavy metal toxicity, nutrient deficiency, and oxidative stresses. Among these, drought, salt, and temperature stresses affect the geographical distribution and limit crop productivity. Approximately 40% and 7% of the global land area is affected by drought and salinity, making them the major environmental factors affecting crop productivity (Trenberth et al., 2014). The susceptibility of plants to abiotic stresses also varies with factors such as its timing, duration, and intensity of stress. Exposure to abiotic stresses leads to the alteration of numerous physiological, biochemical, and molecular processes in the plant. Under field conditions, plants encounter multiple stresses at once, making it challenging to develop stress-tolerant plants.
The global food market is composed of three main crop groups; cereals, oil crops, and legumes. Oil crops ranks second in world crop production after cereals and are of high economic worth for agriculture and trade worldwide. Among oil crops, Brassica napus (oilseed rape/rapeseed/canola) ranks second worldwide with an annual value of 41 billion U.S. dollars collectively (USDA, 2018).
B. napus is an amphidiploid formed by hybridization of diploid progenitor species, B. rapa and B. oleracea (Wang et al., 2011). Rapeseed/canola is versatile in its uses with the extracted oil being used for cooking, biofuel production, and in the ole-chemical and pharmaceutical industries with the meal after oil extraction used as a high protein animal feed (Friedt and Snowdon, 2009). Other than rapeseed/canola, B. napus also encompasses subspecies which produce tuberous (B. napus subsp. Rapifera, rutabaga) and leafy vegetables (B. napus subsp. Pabularia, leaf rape). Grown in the temperate climates of both northern and southern hemispheres, it is cultivated in different seasons (annuals or biennials) (Shahzadi et al., 2015; Zhu et al., 2016). Just like other temperate field crops, B. napus is also susceptible to multiple abiotic stresses (Elferjani and Soolanayakanahally, 2018). Drought, salinity, extreme temperatures, and cadmium toxicity are the most prevalent abiotic stresses affecting the growth and development of B. napus.
This review summarizes the current knowledge of abiotic stress responses in B. napus with an emphasis on possible biotechnological and synthetic biology routes for the development of climate-resilient varieties. We will first discuss the physiological impact of abiotic stresses followed by the fundamental question of how plants sense and adapt to these disruptive environmental conditions, with a further focus on the stress sensing and signaling cascades in B. napus along with other related crops. Multiple stress-responsive genes and studies focusing on developing abiotic stress-tolerant B. napus will be highlighted. The role of non-coding RNAs in response to stress conditions will also be discussed.
Different abiotic stresses adversely affect major biological processes in plants such as photosynthesis, stomatal conduction, rate of transpiration, protein synthesis, and metabolite accumulation (Zhang X. et al., 2014; Zhu et al., 2016; Elferjani and Soolanayakanahally, 2018). Abiotic stresses and their physiological consequences on plants are both shared and unique (Chinnusamy et al., 2004). For example, both drought and salt stress limit plant growth through somewhat different modes of action. Drought has been shown to lead to a decline in photosynthesis, whereas salt stress limits growth through enhanced ion toxicity (Chinnusamy et al., 2004). Drought or scarcity of water is one of the leading abiotic stresses imposed on plants in the current climatic scenario. In B. napus decrease in plant biomass, reduced chlorophyll content due to loss of pigments and damaged thylakoid membranes, reduced seed oil, and protein content are some of the physiological changes observed under drought (Rizwan et al., 2019). Relative water content (RWC) is a physiological measure of cellular hydration in the plants. Up to 48% of RWC loss in B. napus leaves been reported under drought conditions (Sabagh et al., 2019). However, drought is not the only abiotic stress that leads to cellular dehydration as it is also induced under salt stress, which causes a state of toxicity and osmotic stress (Zhang X. et al., 2014; Rezayian et al., 2018).
Amphidiploid B. napus is more tolerant to salinity in comparison to other diploid Brassica species suggesting an interspecific variation for salt tolerance (Purty et al., 2008). Still, the implications of salt stress on B. napus productivity are manifold due to Reactive Oxygen Species (ROS) mediated cell injury, reduced uptake of essential nutrients such as nitrogen, potassium, and decreased total fatty acid content (Rizwan et al., 2019). Similarly, under cadmium stress, B. napus has been reported to have altered fatty acid content in seeds along with the reduced activity of ROS mitigating antioxidant enzymes (Ding et al., 2018). Cadmium, a non-essential metal ion, when accumulated in high concentration, leads to cytotoxicity (Chmielowska-Bąk et al., 2014). Decreased photosynthesis efficiency due to declined chlorophyll content, reduced root growth, and shoot biomass are some adverse physiological features affected by cadmium (Benáková et al., 2017).
Temperature is a determining factor in plant productivity, which acts as a double-edged sword, extreme fluctuations on either end results in stress conditions. In the case of B. napus, 29.5°C was observed as a threshold and increase in temperature beyond this imposed constraint on the plant reproduction and yield (Morrison and Stewart, 2002). Reproduction is a plant developmental process most vulnerable to heat stress (Angadi et al., 2000; Lohani et al., 2019b). Following exposure of B. napus to heat stress at 35°C, reduction in pollen viability, germinability, fruit abortion, and reduced seed production were observed (Young et al., 2004). High-temperature results in a higher unsaturation ratio (oleic/linoleic acid ratio) of fatty acids leading to decreased oil quality (Gibson and Mullen, 1996; Aksouh-Harradj et al., 2006). The temperature has been reported to modify the C-N metabolism and gaseous exchange favoring protein accumulation at the expense of oil and carbohydrates (Canvin, 1965; Wang and Liu, 2014). In B. napus, exposure to extremely low temperatures has also been shown to reduce photosynthetic efficiency and induce membrane damage leading to electrolyte leakage (Megha et al., 2018a). Elferjani and Soolanayakanahally (2018) reported divergent responses of B. napus to heat, drought, and combination of both stresses in terms of seed yield and oil quality. While drought led to reduction in the carbon assimilation rate due to limitation of stomatal CO2 diffusion, the heat stress largely affected reproductive processes leading to significant reduction in the number of siliques and seed yield.
Figure 1 outlines the physiological impacts of various abiotic stresses on growth, development, and yield in B. napus. The physiological responses of plants to primary stresses such as salt, drought, and temperature are often interconnected, leading to secondary stresses such as osmotic and oxidative stresses (Wang et al., 2003). This interconnectivity requires compartmental cross-talk in plants for stress adaptation when facing multiple stresses simultaneously. For instance, drought-induced changes in cell wall's biophysical properties, temperature stress-induced fluctuations in membrane fluidity, and membrane damage under salt and cadmium stress, cumulatively contribute to the downstream stress signaling cascade. This highlights the intricate and elaborate nature of stress sensing, signaling, and response machinery that evolved in plants to endure abiotic stress conditions.
Figure 1 Physiological impact of multiple abiotic stresses in canola on different developmental stages.
Acclimatization to stress is a complex process that involves a fine-tuned combination of genes, proteins, metabolites, and multiple regulatory pathways. The first step in abiotic stress response is sensing or perception of stress by plants. Sensors can be defined as a molecule or a structure that undergoes structural changes or transient loss of function, initiating a signaling cascade that leads to a response (Ruelland and Zachowski, 2010). These sensors cause reversible physical changes such as the change in membrane fluidity, protein conformational changes, partial separation, or melting of DNA and RNA strands. These changes further set in differential transcription control and regulation of stress-responsive genes, which then eventually constitutes a physiological stress response (Figure 2).
Figure 2 Schematic representation of abiotic stress sensing and signaling in B. napus. Initial incidence of stress sensing through receptors cascades the downstream stress response through secondary messengers such as calcium and ROS. Signal amplification and transduction through CDPK and MAPK signaling cascades leads to differential transcriptional regulation of stress responsive genes. ABA is involved not only in regulation of ABA responsive transcription factors to bring about stress adaption but also interacts with PYL family to coordinate guard cell shrinkage and stomatal closure leading to reduced transpiration. ABI2, ABA insensitive 2; APX, Ascorbate peroxidase; CAT, Catalase; CPKs, Calcium-dependent protein kinases; H2O2, Hydrogen peroxide; •OH, hydroxyl radical; GPCRs, G-protein coupled receptors; GST, Glutathione S-transferases; HKs, Histidine kinases; MAPK, Mitogen activated protein kinases; PYL, Pyrabactin resistance like family; RLK, Receptor like kinases; ROS, Reactive oxygen species; 1O2, Singlet oxygen; O2•⁻, superoxide radical.
Cell surface or the cell membrane is often the first site of stress sensing with external stimuli resulting in fluctuations of cytosolic calcium level. The involvement of calcium (Ca2+) in multiple physiological and developmental processes consolidates its role of being a major player in the stress signaling cross-talk (Knight, 1999; Whalley and Knight, 2013). Variations in the calcium signature can be attributed to the type of stress, site of stress incidence, exposure rate and intensity (Chinnusamy et al., 2004). One of the most common responses of stress perception is increased intracellular accumulation of free Ca2+. Under cold stress, plasma membrane rigidification, and increase in Ca2+ accumulation induce cytosolic calcium signatures leading to altered expression of cold-responsive genes (Miura and Furumoto, 2013). Similarly, within minutes of temperature rise, a conserved transient calcium influx is observed in several model plants such as Arabidopsis, tobacco, and moss Physcomitrella (Saidi et al., 2011). The role of Ca2+ ions in activation of heat shock factors (HSFs) leading to further expression of heat shock proteins (HSPs) is critical in heat stress response (Saidi et al., 2011). As a secondary stress messenger, Ca2+ relays the stress signals from cell surface receptors to effector proteins, and initiate downstream responses. Calcineurin B-like proteins (CBLs), calmodulin (CaMs), calmodulin-like proteins (CMLs), and Calcium Dependent Protein Kinases (CDPKs/CPKs) are sensor relays requiring interaction with other target proteins for their regulation.
CaMs and CMLs are a family of Ca2+ sensors in plants containing helix-loop-helix EF-hand domains and regulate downstream targets based on Ca2+ fluctuations. In Arabidopsis, members of seven CAMs and 50 CMLs have been characterized to be involved in responses to cold, heat, osmotic, and ionic stress (McCormack et al., 2005). These bind to calmodulin-binding transcription factors (CAMTAs), also referred to as signal responsive (SR) proteins. Arabidopsis CAMTA1, CAMTA2, and CAMTA3 contribute to low temperature and freezing tolerance by activation of CBF (C-repeat/DRE binding factor) transcription factors (Doherty et al., 2009). In B. napus, Rahman et al. (2016) identified eighteen CAMTAs, maximum of any other plant species reported so far. Rahman et al. (2016) predicted functional homology between AtCAMTA3, BnCAMTA3A1 and BnCAMTA3C1. AtCAMTA3 has been previously suggested to play a role in enhancing plant tolerance to cold and heat stress (Liu et al., 2005; Virdi et al., 2015). Efforts to identify and characterize CaMs or CMLs in B. napus like other plant species will significantly assist in understanding the specific CAMTA interactions and stress response.
Unlike CaMs, which target a variety of proteins, CBLs explicitly interact with CBL-interacting protein kinases (CIPKs) or SNF1-related protein kinases SnRK3 (Chen et al., 2012). The structural composition of CIPKs involves an N-terminal kinase catalytic domain, a junction domain that connects it to the highly variable C-terminal regulatory domain (Chaves-Sanjuan et al., 2014). The C-terminal regulatory domain consists of the FISL motif with a unique 24 amino acid stretch, which is essential for the CBL-CIPK binding (Albrecht et al., 2001). Ten CBLs and 25 CIPKs have been identified in Arabidopsis (Weinl and Kudla, 2009). Characterization of CBL and CIPK genes in B. napus by Yuan et al. (2014) revealed the presence of 7 CBLs and 23 CIPKs. Interaction studies of BnCBL1-BnCIPK6 protein were confirmed by bimolecular fluorescence complementation (BiFC) and its upregulation during salt stress, osmotic stress, and response to ABA suggested its possible role in salt stress tolerance and ABA signaling in B. napus (Chen et al., 2012).
Overexpression of B. napus CBL gene, BnCBL4, and its interaction with BnCIPK24 was also shown to be responsible in rescuing sos3-1 Arabidopsis mutants and thus resulting in enhanced salt tolerance (Liu et al., 2015). Signal transduction of intracellular accumulation of Ca2+ ion through CBL/CIPK signaling during salt stress includes salt overlay sensitive pathway (SOS). There are three major components of the plant SOS pathway with SOS3 acting as a Ca2+ sensor, SOS2 encoding a serine/threonine kinase, and SOS1 encoding a plasma membrane Na+/H+ antiporter (Chinnusamy et al., 2004). Maintenance of ion homeostasis inside the cell during salt stress is critical for the plant salt stress tolerance. Under salt stress conditions for binding Ca2+, SOS3 encodes a protein with the N-myristoylation motif and three EF-hand domains and activates SOS2. The SOS3-SOS2 complex controls the expression and activity of SOS1 through direct phosphorylation (Gong et al., 2004).
The third components of the Ca2+ sensing machinery in plants are the CDPKs, which are sensor responders with the ability to self-modify the confirmation through enzymatic action (Chen et al., 2012). This makes CPKs unique in their dual functionality in calcium-sensing and then responding through downstream phosphorylation events against the stress condition cues. Immense overlap and cross-talk is observed in CPKs stress response. Against stresses such as drought, cold, salt, and heat, there are multiple CPKs essential for response to specific stress stimuli.
Zhang, H. F., et al (2014) identified 25 CPKs in B. napus and further analyzed their expression levels under various abiotic stresses. Their findings suggested BnCPK4's interaction with Protein phosphatase 2C (PP2C) to regulate ABA-responsive transcription factors such as ABF1 and ABF4/AREB2 for signaling during drought stress. Ca2+ sensing and signal transduction by BnCPK4 in B. napus and activation of bZIP TFs AREB3 and AB15 highlight their involvement in the regulation of ABA and drought stress signaling (Zhang H. F. et al., 2014). Similarly, Wang W., et al (2018) used a mating-based split ubiquitin system (mbSUS) and BiFC to study the BnCPK2 interacting partners. They suggested the role of BnCPK2 in the regulation of ROS and cell death and to have possible interactions with NADPH oxidase-like respiratory burst oxidase homolog D (RbohD). Similar findings have been reported in which the majority of the CPKs are shown to modulate ABA signaling and ROS homeostasis in plant cells (Asano et al., 2012).
GPCRs are a class of stress receptors operating in plants that perceive the stress signal. GPCRs bind to various ligands, which relay information regarding the extracellular stress stimuli. Ligand binding to GPCRs induces conformational changes and facilitates the exchange of GTP for GDP, which then activates heterotrimeric guanine-nucleotide-binding proteins (G proteins). The activated GTP-bound Gα and Gβγ complexes then further bind to downstream cellular effectors. Signal termination requires hydrolysis of GTP to GDP by Gα subunit and reconfiguration into the inactive form (Chakravorty and Assmann, 2018). Coupling of ligand-bound G proteins with GPCRs is responsible for the activation of Ras-related small GTP-binding proteins, which in turn sets in Ca2+ mediated inositol triphosphate (IP3) signaling pathway in response to abiotic stress in canola (Shokri-Gharelo and Noparvar, 2018). In B. napus, heterologous overexpression of an inositol phosphate kinase homolog from Thellungiella halophila (ThIPK2) conferred resistance to salt, dehydration, and oxidative stresses (Zhu et al., 2009). Possible mechanism of action of ThIPK2 in transgenic B. napus plants was a higher accumulation of Na+ ions in roots, higher proline content, and differential expression of stress-responsive genes.
In Arabidopsis GPCR2, the receptor for phytohormone ABA was also reported to be a GPCR. Located in the guard cell, it mediates the stomatal movement and thereby transpiration rate in response to ABA accumulation under stress (Tuteja and Sopory, 2008). GPCRs are also reported to control many cellular processes by regulating phospholipid signaling pathways (Tuteja and Sopory, 2008). In B. napus, expression of Gα, Gβ, and Gγ subunit encoding genes under different stress conditions has been examined. BnGA1, BnGB1, and BnGG2 genes encoding Gα, Gβ, and Gγ subunits respectively showed upregulation under salt and drought stress and downregulation in heat and cold stress (Gao et al., 2010a; Gao et al., 2010b; Gao et al., 2010c). All these genes also exhibited a common ABA regulated induction, indicating a possible role in the hormone signaling pathway. Rate of Gα protein-mediated GTP hydrolysis is fast-tracked by the regulator of G-protein signaling proteins (RGS); hence, they act as negative regulators of G-protein signaling. In B. napus, BnRGS1 was shown to interact with BnGA1. Its upregulation during PEG treatment, which simulates conditions similar to salt and drought stress, indicated a possible mode of action in an ABA-mediated manner during stress conditions (Chen et al., 2014).
RLKs make up the largest gene family in plants with structurally similar proteins having an extracellular ligand-binding domain (ECLB), a single membrane-spanning transmembrane domain (TM), and an intracellular protein kinase catalytic domain (PKC) (Goff et al., 2007; Ye et al., 2017). The intracellular kinase domain plays the central role in signal transduction, which upon binding of a ligand to ECLB, modifies the protein conformation. Various RLK subfamilies such as proline-rich extensin like receptor kinases (PERKs), S-domain containing RLKs, lectin-like RLKs, and wall-associated kinases (WAKs) have been shown to play a role in abiotic stress response (Nongpiur et al., 2019). ABA-mediated abiotic stress response of RLKs has been documented in various crops such as Arabidopsis, rice, and Glycine soja. Many of these have also been characterized for tolerance against specific abiotic stresses such as GsCBLRK in salt stress and AtCRLK1 in cold and salt stress (Virdi et al., 2015). While analyzing the cross-talk and specificity between signaling mechanisms of salt and drought stress in B. napus, Luo et al. (2015) identified two drought stress-responsive RLKs along with glycosylphosphatidylinositol (GPI)-anchored salt receptor protein.
HKs are another class of receptors known to play a role in abiotic stress sensing. Membrane-bound HKs are known for their two-component system (TCS) of functioning. TCS for HK mediated osmosensing is already well established in bacteria and yeast. The system consists of a sensory histidine kinase (HIK) and a response regulator (RR) (Nongpiur et al., 2019). In plants presence of a His-containing phosphotransfer (Hpt) protein connects the initial sensory HIK to the ultimate RR while mediating as a signaling module. This permits for a multiple-step phosphorylation relay with the benefit of regulation through checkpoints for cross-talk and even negative regulation by specific phosphatases. The eventual modification of gene expression for stress response is mediated by the protein-protein and protein-DNA interaction of RR's effector domain. Light perception, cytokinin, and ethylene signaling all involve members of the two-component system (Singh et al., 2015). Previously, characterization of Arabidopsis histidine kinases AHK2, AHK3, and AHK4/CRE1 suggested their role as cytokinin receptors and negative regulators in ABA, drought and high salinity stress signaling (Tran et al., 2010; Kumar and Verslues, 2015). In B. napus, identification and functional characterization of five B. napus histidine kinases (BnCHK1–BnCHK5) revealed BnCHK1-BnCHK4 to be an AHK2 homolog and BnCHK5 as an AHK3 homolog respectively (Kuderova et al., 2015). These findings point towards a probable similar conserved mechanism of histidine kinases in B. napus through cytokinin signaling for developmental and stress response regulation.
Amplification and further transduction of the stress signals downstream of RLKs and HKs are carried out by an intricate cascade of various protein kinases.
MAPK signaling cascade integrates and channels signal transduction for the expression of stress-responsive genes mediated through phosphorylation. Components of MAPK signaling cascade are involved in and act as converging points for multiple abiotic stress tolerance mechanisms (Chinnusamy et al., 2004).
MAPK signaling cascades comprises of MAPKK kinases (MAPKKKs, MAP3K, or MEKK), MAPK kinases (MAPKKs, MAP2Ks, MKKs, or MEKs), and MAPKs (MPKs). MAP kinases through phosphorylation function as on-off signaling switches aiming downstream targets. Successive phosphorylation/dephosphorylation of serine or threonine residues by MAPKKKs dictates activation of MKKs and then threonine and tyrosine residues for activating MPKs (Sun et al., 2014). The activated terminal MAPKs then proceed forward with the signal transduction by phosphorylation mediated control of transcription factors or enzymes. In Arabidopsis, a total of 80 MAPKKK, 10 MKK, and 20 MPK genes have been identified (Liang et al., 2013). A lot more MKK and MPK genes have been functionally characterized compared to MAPKKK genes, even though they constitute the most abundant family out of the three. The Arabidopsis flagellin cascade (AtFLS2-AtMEKK1, AtMKK4/AtMKK5, AtMPK3/AtMPK6, AtWRKY22/AtWRKY29) working in defence response was one of the first complete MAPK signaling cascades to be characterized in plants (Asai et al., 2002). There is a noticeable gap in the comprehensive understanding and characterization of MAPK signaling cascade routes with their target transcription factors and stress-responsive genes in B. napus. Working towards this goal, Liang et al. (2013) identified 7 MKK and 12 MPK members. Combination of Yeast two-hybrid (Y2H) interaction studies and BiFC assay helped in identifying possible interactions in the MAPK signaling cascade and TFs such as BnMKK9-BnMPK5/9/19/20, BnMKK9-BnMPK1/2-BnWRKY53, and BnMKK2/4/5-BnMPK3/6-BnWRKY20/26. Further, Sun et al. (2014) identified 66 MAPKKK genes in B. napus. Expression of BnMAPKKK genes was regulated by hormone-induced stress stimuli and other abiotic stresses, including cold, heat, and oxidative stress. They also reported the role of MAPKKK18 and 19 in eliciting ROS accumulation and hypersensitive response (HR) like cell death upon transient expression in tobacco leaves via a possible interaction with BnMKK9. Its interaction with the previously established MKK9–BnMAPK1/2–BnWRKY53 cascade needs further investigation. BnMAPKKK4 was also reported to cause a similar ROS mediated response upon transient overexpression in tobacco leaves (Li L. et al., 2015). Possible interaction between BnMAPKKK4 and MAPK3 was highlighted in eliciting the ROS response.
Commonly known as the stress hormone, ABA, and its role in plant stress response is highlighted in the ABA-dependent signaling pathway. The ABA signaling pathway, mainly functioning in the plasma membrane, plays a vital role in the plant's response to salt, cold, hypoxia, and drought stress. Ca2+ and protein kinase-mediated sensing of stress leads to activation of SnRK2, which sets in ABA accumulation. This increased ABA accumulation under stress conditions is identified by protein receptors of the pyrabactin resistance like (PYL) family (Vishwakarma et al., 2017). ABA then forms a tri complex with 2C protein phosphatases (PPC2), which almost acts as co-receptors leading to the increased binding affinity of ABA and PYL.
Cis-acting element, ABA-responsive element (ABRE), and transcription factors, ABRE-binding protein/ABRE-binding factors (AREB/ABFs) regulate the ABA-dependent gene expression (Yoshida et al., 2014). Varied targets in stress response mediate the physiological modifications such as stomatal closure, flowering time control, chromatin regulation, and RNA splicing. High levels of endogenous ABA content in drought-tolerant cultivars compared to drought susceptible cultivars have been reported in barley (Zhang X. et al., 2014), indicating an inherent requirement of higher ABA levels for stress-related response and improved tolerance. Exogenous application of ABA also induces a stress response in plants enhancing their adaption ability, thus indicating the importance of its action under stress conditions (Sah et al., 2016).
ABA is also reported to be involved in seed desiccation tolerance and regulating various aspects of seed development and dormancy (Chinnusamy et al., 2004). The role of B. napus BnABI3, a B3 domain-containing protein known for functioning in the ABA signaling pathway was investigated by Xu and Cai (2019) during seed development in Arabidopsis. They reported direct involvement of BnABI3 in seed coat development and desiccation tolerance. Their findings also suggested the possible role of BnABI3 in the coordination of flowering time and response to cold stress as its overexpression led to delayed flowering and rescue of the cold-induced green seed phenotype. Similarly, upregulation of BnABI5, a basic leucine zipper transcription factor responsible for the regulation of multiple LEA genes, was observed under both ABA and PEG induced water stress for enhanced seed dormancy in B. napus (Li et al., 2005).
Shrinking of guard cells in response to drought to prevent loss of water through transpiration is an important stress adaptive response. Proteomic analysis of B. napus guard cell protoplasts after ABA treatment showed upregulation of 66 proteins. The majority of which is involved in photosynthesis and stress responses also showed an overlap with the drought inducible proteins. These observations presented an insight into the physiological changes manifesting out of the ABA signaling pathway, such as reorganization of the cytoskeleton and ROS homeostasis (Zhu et al., 2016). Zhu and Assmann (2017) used exogenous application of ABA hormone for differential metabolomic profiling in B. napus guard cells. They identified 29 primary and 48 secondary ABA-responsive metabolites possibly functioning in this intricate intracellular signaling in guard cell response to drought.
Although among phytohormones, the principal regulator of abiotic stress response is ABA, increasing evidence highlight the involvement of other phytohormones such as salicylic acid, jasmonates, ethylene, brassinosteroids, auxins, cytokinins, and gibberellins as well. The nature of phytohormone mediated regulation is further complex as they can act either directly or orchestrate abiotic stress response via cross talking networks involving other phytohormones, MAP Kinases, ROS, sugar, and other secondary messengers (Smékalová et al., 2014; Ljung et al., 2015). The role of these phytohormones in positively or negatively regulating abiotic stress tolerance has been extensively reviewed (Peleg and Blumwald, 2011; Wani et al., 2016).
Complete understanding of the interaction of phytohormones and stress signaling is lacking, however, in the light of recent investigations, this interaction is becoming very evident. For instance, ethylene signaling may cause repression of CBF pathway in Arabidopsis, thus negatively regulating cold tolerance (Shi et al., 2012). Ethylene signaling also negatively regulates salt and drought tolerance. Similarly, a reduced level of GA has been reported to possibly restrict plant growth in response to several abiotic stresses and increased levels promote growth and assists plant escape during shade or submergence (Bailey-Serres and Voesenek, 2010). Jasmonate biosynthesis and signaling pathway can positively regulate various abiotic stress responses (Dar et al., 2015). Regulation of stress responsive transcriptional machinery by cytokinins and its cross-talk with stress signaling has also been suggested (Zwack and Rashotte, 2015). Possible association of GA signaling with JA signaling via DELLA as well as JA and ethylene signaling further validates the complex cross-talk network (Colebrook et al., 2014; Kazan, 2015).
The exogenous application of phytohormones is well documented to mitigate the negative implications of abiotic stress in canola (Kurepin et al., 2008; Farhoudi and Saeedipour, 2011; Alam et al., 2014; Hasanuzzaman et al., 2014). SA application imparted salt stress tolerance in B. napus by improving the performance of the antioxidant enzymes such as GR, GST, GPX, CAT, thereby reducing the extent of oxidative damage (Hasanuzzaman et al., 2014). Pretreatment of B. napus seedlings with SA alleviated drought induced symptoms by antagonistically interacting with ABA. It also enhanced the expression proline synthesis related and redox reducing genes. Also, SA possibly fosters drought tolerance in B. napus by mediating transcriptional regulation of sugar accumulation under drought stressed conditions. Lee et al. (2019a) reported reduced expression levels of NCED3 (involved in ABA synthesis), PDF1.2 (JA signaling gene) and Lee et al. (2019b) reported reduced expression of ABA-dependent sucrose signaling genes SnRK2.2 and AREB2 strongly indicating an averse relationship between SA and ABA in regulation drought stress response. Similarly, pretreatment of B. napus seedlings with brassinolide enhances thermotolerance by increasing the endogenous levels of ABA. 24-Epibrassinolide treatment also enhances tolerance against heat, cold, and drought (Kagale et al., 2007). Possible mode of action of BRs in abiotic stress is by regulation of stress responsive transcriptional machinery.
Among all the biochemical responses to stress in plants, ROS plays a key role in acclimatization to abiotic stresses. ROS metabolism and the antioxidant defence system involved in abiotic stress response has been extensively reviewed (Gill et al., 2010; Suzuki et al., 2012; Petrov et al., 2015). Produced as a by-product of metabolic reactions in processes such as respiration, fatty acid oxidation, and photosynthesis, ROS helps maintain the ionic balance in various cellular components (Sharma et al., 2012). Contributing to the majority of growth and developmental stages of a plant, the ROS regulatory system implies processes including programmed cell death, autophagy, and response to stress (Mittler, 2017). Production of different forms of ROS, including singlet oxygen (1O2), hydrogen peroxide (H2O2), hydroxyl radical (HO·), and superoxide anion radical (O2·) is enhanced under stress (Sharma et al., 2012). This creates conditions that are highly damaging to biomolecules and sets in a cellular state known as oxidative stress. Increased ROS during stress also triggers transient or permanent protein modifications. These ROS induced post-translational modifications of proteins lead to conformational changes in enzymes, thereby altering transcriptional regulation and plant metabolic processes (Choudhury et al., 2017). Figure 3 provides a schematic representation for functioning of ROS in plant stress response.
Figure 3 Schematic representation for functioning of ROS in plant stress response. During normal functioning of the plant metabolic processes ROS is produced and scavenged in a harmonious balance. Abiotic stresses skew the ROS concentration gradient and lead to an increased accumulation. ROS and its interaction with other signaling mechanisms such as RLKs, phytohormones, G proteins, MAPKs, and Ca2+ play a vital role in determining the subsequent response. Based on the severity of the stress the plant response ranges from adaption, impaired growth, PCD to necrosis. APX, Ascorbate peroxidase; CAT, Catalase; GPX, Glutathione peroxidase; MAPKs, Mitogen activated protein kinases; PCD, Programmed cell death; RLKs, Receptor like kinases; SOD, Sodium Dismutase.
Different ROS signatures arising from abiotic stresses determine the specificity of the acclimation response (Choudhury et al., 2017). In plants NADPH oxidases, homologs to the respiratory burst oxidases (RBOHs) are involved in the ROS production network. They also play a role in the initiation and propagation of cell-to-cell systematic signal through H2O2 accumulation by generating a ROS wave. This acts as an overall signal to systemic tissues about the localized abiotic stress stimuli (Baxter et al., 2014). The Arabidopsis genome encodes 10 RBOH proteins (RBOHA-J) with varying functionality in plants response to different abiotic stresses (Torres and Dangl, 2005; Wang et al., 2013). AtRobhD and AtRobhF genes have been characterized to show ROS dependant ABA mediated signaling in guard cells and stomatal closure (Kwak et al., 2003). AtRBOHD assists AtRBOHF for ROS production to regulate Na+/K+ homeostasis under salt stress (Liu and He, 2016). The nine RBOH genes (OsRbohA-OsRbohI) identified in rice have been shown to have changes in expression patterns in response to various environmental stresses (You and Chan, 2015), with both OsRbohA and OsRbohC having increased abundance in drought stress (Wang et al., 2013). Even with their vital role in the ROS production network, in case of B. napus, there is still an existing gap in identification and characterization of RBOHs, which could potentially lead to better understanding of ROS mediated stress signaling. Production and scavenging of ROS balance dictates the maintenance of cellular homeostasis. This balance of production and scavenging is in harmony during unstressed conditions, but most environmental stresses trigger a disturbance with increased ROS production. As a guarding mechanism against this, plants are equipped with antioxidants of enzymatic and non-enzymatic nature. These include ROS scavenging enzymes such as superoxide dismutase (SOD), glutathione peroxidase (GPX), ascorbate peroxidase (APX), and catalase (CAT) along with compatible solutes such as proline (Pro) and glycinebetaine (GB) working to limit ROS induced injury. Increased activity of APX and glutathione S-transferase (GST) was observed in B. napus seedlings under cadmium stress (Hasanuzzaman et al., 2017). Similarly, Hyola varieties of canola showed a significantly high activity of APX and CAT under salt stress (Heidari, 2010). Comprised of ascorbate, glutathione, ascorbate peroxidase, monodehydroascorbate reductase, dehydroascorbate reductase, and glutathione reductase the ascorbate-glutathione (AsA-GSH) pathway plays a vital role in detoxifying ROS by improving osmoregulation, water use efficiency and nutrient status (Hasanuzzaman et al., 2019).
ROS also communicates with other signaling networks for stress adaption responses. Involvement of H2O2 towards ABA-mediated stomatal closure through inactivation of ABI2, a 2C protein phosphatase, and negative regulator of ABA (Pandhair et al., 2006). The heterologous overexpression of AtABI2 (group A PPC2 gene) in B. napus also resulted in transgenic B. napus lines with decreased drought tolerance, highlighting the negative regulation of ABA. ROS and its role in the maintenance of the cell's redox potential under stress are not only limited to interaction with ABA but also include other plant hormones such as gibberellins (GAs), Auxin, brassinosteroids (BRs), ethylene (Choudhury et al., 2017). ROS induces changes in level of endogenous plant hormones, leading to modification in their impact and activity (Lee et al., 2019a). Recent research in ROS signaling has greatly enhanced the understanding of this cross-talk between these signaling pathways, wherein ROS acts as key a regulator not only coordinating plant development but also response and adaption to stress.
To bring about the required changes for stress adaption and response, the sensing and signaling cascade leads to the activation of molecular networks involved in the expression of specific stress-related genes and metabolites. The availability of the B. napus genome sequence in 2014 (Chalhoub et al., 2014) led to the identification and characterization of several stress-responsive gene families in B. napus (Table 1). Functional characterization of several abiotic stress-responsive B. napus genes has been carried out by ectopic expression in model plants Arabidopsis and tobacco (Table 2). An effective way to enhance crop adaptability or tolerance in multiple stress occurrences is to dissect the multifaceted stress-responsive regulatory networks as well as understand the specificity and cross-talk of those pathways leading to the identification of key genes.
Table 2 Summary of functional characterization of putative stress responsive B. napus genes by overexpression studies in model plants.
In the plant genome, out of the total coding sequences present, 5% to 7% is utilized for the expression of TFs. WRKY, MYB (myeloblastosis), bZIP (basic leucine zipper), AP2/ERF (APETALA2/ethylene-responsive factors), HSF (Heat Shock Factors), and NAC are some of the most prominent families of stress-responsive TFs. Out of these, WRKY, NAC, and AP2/ERFs are unique to plants (Lan Thi Hoang et al., 2017). Genetically manipulating the expression of TFs for imparting or enhancing abiotic stress tolerance is a widespread approach as most of them are involved in early stress response, and they control the expression of stress-responsive genes.
AP2/ERF TFs can integrate responses to various stress stimuli such as Ca2+, ROS, MAPKs, and SnRKs mediated phosphorylation, and partake in stress responsive networks. This TF superfamily is further divided into five subfamilies: DREB (Dehydration Responsive Binding Element), ERF, AP2, Soloist, and RAV (Related to ABI3 and VP1) (Lan Thi Hoang et al., 2017). Owji et al. (2017) identified 321 putative AP2/ERF TFs in B. napus and also suggested the potential role of BnERF245 and BnERF039 in drought and salt tolerance. Functional characterization of the BnERF-2 like gene from B. napus in Arabidopsis highlighted their role in regulating antioxidant machinery for enhancing abiotic stress tolerance (Lv et al., 2016). Members of the DREB subfamily are recognized for their induction during multiple abiotic stresses (Mizoi et al., 2012). Salt stress-induced expression of DREB2C in Arabidopsis and its role in inducing salt stress tolerance by regulation of stress-responsive genes (RD29A, RD29B, and COR15A) upon overexpression was reported by Song et al. (2014). They subsequently overexpressed AtDREB2C in B. napus conferring improved salt tolerance due to higher accumulation of Na+, higher retention of water, better growth, and lower relative water content as compared to wild type.
CBF/DREB1s interact specifically with CRT/DRE cis-elements to direct the transcription of COR (cold regulated) genes. Transgenic B. napus lines overexpressing Arabidopsis CBF genes (CBF1/DREB1b, CBF2/DREB1c, and CBF3/DREB1a) showed enhanced freezing tolerance due to induction of CBF-targeted orthologous B. napus gene Bn115 (Jaglo et al., 2001). Similarly, homologous overexpression of two BnCBF/DREB1-like genes (BnCBF5 and BnCBF17) enhanced freezing tolerance in transgenic B. napus plants (Savitch et al., 2005). BnCBF17 overexpressing plants performed better in comparison to the BnCBF5 overexpressing canola, probably due to higher cor genes expression. Overexpression of these genes also partially regulated the expression of genes involved in chloroplast photosynthetic development, photosynthesis, Calvin cycle, starch, and sucrose biosynthesis, thus enhancing the photosynthetic efficiency in response to cold stress. Other important gene candidates from this superfamily are yet to be investigated in B. napus. However, the role of some of AP2/ERF TFs in imparting stress tolerance in other crops has been reported. For instance, overexpression of ERF1 imparts salt tolerance and freezing tolerance in rice and wheat, respectively (Schmidt et al., 2013; Zhu et al., 2014). Similarly, DREB sourced from various crops such as ZmDREB2A in maize (Qin et al., 2007), OsDREB1s and OsDREB2s in rice (Matsukura et al., 2010) and TaDREB1 in wheat (Shen et al., 2003), when overexpressed in plants enhanced abiotic stress tolerance. Further understanding of abiotic stress responses and the involvement of AP2/ERF TFs in canola is warranted.
HSFs play a crucial role, not only in heat stress response, but other abiotic stresses as well (Guo et al., 2016). HSFs have a conserved DNA-binding domain (DBD) which recognizes heat stress elements (HSE) along with other cis-elements such as STRE (stress-responsive element), DRE/CRT (drought/cold-responsive element), and MYCATRD22 (dehydration, ABA-responsive element) in promoter sequences of the genes they regulate. Zhu et al. (2017) identified 64 Hsf encoding genes in B. napus, making it the largest Hsf gene family in eudicots so far. The role of hybridization and allopolyploidization in shaping the structure of the Hsf gene family has been reported (Lohani et al., 2019a). Most BnHSFs were induced under heat as well as drought stress suggesting their role in multiple abiotic stress acclimatization in canola (Zhu et al., 2017). Lang et al. (2017) functionally characterized BnHSF4a in Arabidopsis and highlighted its role in desiccation tolerance of seeds by upregulation of genes GolS1, GolS2, and raffinose synthase 2 (BnRS2) which are involved in osmoregulation in plant cells. Ectopic overexpression of Hsf genes has been reported to enhance, salt, drought, and thermotolerance in other crops. For instance, overexpression of GmHSFa1 in soybean (Zhu et al., 2006), SlHSFA1, and SlHSFA3 in tomato and TaHSFA6f in wheat enhanced the thermotolerance of transgenic plants (Guo et al., 2016). Similarly, the silencing of Hsf genes resulted in the negative regulation of stress tolerance, e.g., OsHSF4A knockout rice plants showed decreased cadmium tolerance (Shim et al., 2009) and SlHSFA2 knockout tomato lines were reported to have reduced reproductive thermotolerance (Fragkostefanakis et al., 2016).
WRKY TFs are yet another class of TFs playing a crucial role in multiple abiotic stress responses. They are characterized by the presence of the conserved motif WRKYGQK in the sixty amino acid long WRKY domain (Rushton et al., 2010). Phosphorylation induced signal transduction from MAPKKKs to MAPKs activates various substrates, including TFs from the WRKY family. He et al. (2016), through comparative transcriptome analysis, identified 287 WRKY TFs in B. napus and validated the multiple stress responsiveness of BnWRKY147, BnWRKY166, and BnWRKY210 under simultaneous low temperature, salinity, and drought stress. Li, Q., et al (2015) overexpressed BnTTG2 (homolog of Arabidopsis AtWRKY44 TF) in B. napus and observed that BnTTG2 is a transcriptional repressor under salt stress. The transgenic B. napus plants were hypersensitive to salt stress and showing lower expression of genes involved in IAA synthesis such as TRP5 and YUCCAA2. Similarly, GmWRKY13 from soybean conferred increased salt sensitivity in transgenic Arabidopsis plants (Zhou et al., 2008). However, other WRKY factors such as OsWRKY45 and OsWRKY72 when overexpressed conferred enhanced salt and drought tolerance in transgenic Arabidopsis (Song et al., 2010). These studies highlight that the differential stress regulatory nature of different WRKY genes. It will be thus, worthwhile to explore the role of different BnWRKY genes in response to multiple stresses.
MYB TFs function by specifically binding to MYB binding sites and are classified depending on the number of repeats in the MYB domain. Most extensively studied MYB TFs are the R2R3-type MYB proteins. Investigation of the R2R3-MYB gene family in B. napus by Hajiebrahimi et al. (2017) has led to the identification of 249 R2R3-MYB genes. Based on RNA-Seq data, BnMYB21, BnMYB141, and BnMYB148 have been suggested as candidate genes that can be over-expressed to improve salt-tolerance in B. napus. The transgenic studies carried out in other crops highlight the importance of MYB genes in stress tolerance. Transgenic soybean overexpressing AtMYB44 showed enhanced salt and drought tolerance (Seo et al., 2012). Similarly, overexpression of LeAN2 (an anthocyanin associated R2R3-MYB TF) in tomato conferred thermotolerance along with higher anthocyanin accumulation (Meng et al., 2015). Functional characterization of MYB genes from different crops in model plants has also highlighted the positive regulation of abiotic stress response by these TFs.
NACs TFs are plant-specific TFs with a highly conserved N-terminal NAC domain and a variable CT functioning activation domain. The role of NAC TFs in abiotic stress tolerance is well documented (Nakashima et al., 2012). Sixty NAC TFs have been identified in B. napus (Wang B. et al., 2015). A number of BnNAC TFs have already been functionally characterized in model plants. Zhong et al. (2012) identified two B. napus NAC TFs (BnNAC2 and BnNAC5) and reported their role in negative regulation of high salinity and osmotic stress tolerance. BnNAC19 and BnNAC82 have been shown to induce a hypersensitive response and cell death under stress due to ROS accumulation (Wang B. et al., 2015). Independent studies have reported similar observations in tobacco and B. napus protoplasts due to transient expression of BnNAC55, BnNAC56, BnNAC87, and BnNAC103 (Niu et al., 2014; Niu et al., 2016; Chen et al., 2017; Yan et al., 2017).
Contrary to this transgenic rice overexpressing SNAC3 showed enhanced tolerance to high temperature, drought, and oxidative stress caused by methyl viologen (MV) due to lower accumulation of ROS (Fang et al., 2015). These studies highlight the possible regulation of genes involved in ROS machinery by NAC TFs. BnNAC485 exhibits a stress-induced gene expression (Ying et al., 2014). Overexpressing this gene in B. napus and Arabidopsis resulted in salt and osmotic stress tolerant transgenic plants. Under saline, osmotic, and ABA treatments, stress-responsive genes (AtRD29A, AtRD29B, and AtABI5) had higher expression in transgenic Arabidopsis plants compared to the wildtype suggesting possible regulation of BnNAC485 mediates abiotic stress response in an ABA-dependent manner. These studies also elucidate the overlapping nature of stress-responsive pathways.
bZIP TFs are major regulators of the stress response mechanism of plants due to their ability to recognize ABRE (ABA-responsive element), a cis-element commonly present in the promoter region of many stress-responsive genes. Most of the identified stress-responsive bZIPs are known to function in drought-responsive pathways. In B. napus 247 bZIP TFs have been identified, and their differential expression in various tissues and organs has been reported (Zhou et al., 2017). Zhao B. Y., et al (2016) reported the expression of BnABF2 (gene encoding bZIP factor) in response to drought and salt stress. Further, overexpression of BnABF2 in Arabidopsis conferred drought and salt resistance due to regulation of RD29B, RAB18, and KIN2 genes under these stresses in an ABA-dependent manner. These studies suggest cross-talk of stress-responsive pathways and highlight the need of a multigenic approach for engineering stress-tolerant rapeseed plants.
Transporters are a class of transmembrane proteins facilitating the movement of selective molecules across plant membranes. They play a significant role in abiotic stress response as they control the traffic of ions and other biomolecules such as hormones and compatible solutes during stress to sustain vital cellular processes such as ion homeostasis, osmotic adjustment, signal transduction, and detoxification (Vishwakarma et al., 2019). Several transporter gene families (Table 1) have been identified and characterized in B. napus such as aquaporins (Yuan et al., 2017), metal transporter genes [MTGs; Zhang et al. (2018a)], ATP-binding cassette (ABC) transporter (Zhang et al., 2018b), sucrose transporters or sucrose carriers (SUT/SUCs) and Sugars Will Eventually be Exported Transporters [SWEET; Jian et al. (2016a)]. Most of these gene families are involved in response to drought, salt, low/high temperatures, heavy metal, and hormone treatments. The genome-wide identification of other transporter gene families such as NHX, HKT, and TMTs is further required in B. napus to understand their role in response to various abiotic stresses. However, Ford et al. (2012) identified orthologs of AtNHX5 and AtNHX6 using the B. rapa genome as the sequence of B. napus was not available at that time. They reported differential expression of BnNHX6.1 (ortholog of AtNHX5) in response to salt stress. Transgenic B. napus plants overexpressing the AtNHX1 gene have been reported to grow and carry out seed filling in the presence of high concentrations of NaCl (Zhang et al., 2001). Higher Na+ accumulation by the transgenics mediated by the Na+/H+ antiporter helped to mitigate the harmful effects of salt stress. The yield and seed quality of these transgenic plants were comparable to wild type. Similarly, overexpression of the BnNHX1 gene in tobacco plants resulted in transgenic plants displaying enhanced salt tolerance (Wang et al., 2004). This finding highlights the utilization of antiporter genes for developing transgenic B. napus plants which can be grown on saline soils.
In B. napus, the genetic-engineering approach has been applied to modulate the levels of BRs, cytokinins, and ethylene for imparting abiotic stress tolerance, Ectopic expression of AtDWF4 in B. napus was carried out to address the genetic basis by which BR signaling plays a role in abiotic stress response in B. napus (Sahni et al., 2016). The resultant transgenic plants showed enhanced not only plant biomass and seed yield but also significant tolerance to dehydration and heat stress in comparison to wild type. These findings suggest the utilization of genes involved in BR synthesis and signaling pathways for crop improvement. Future investigations are also clearly required to understand abiotic stress-responsive BR signaling pathways. Isopentenyltransferase (IPT) gene isolated from Agrobacterium tumefaciens is involved in cytokinin synthesis. Transgenic B. napus expressing the IPT gene under a developmental stage regulated promoter exhibited higher seed yield under rainfed and irrigated conditions as well as delayed leaf senescence (Kant et al., 2015). Another gene 1-aminocyclopropane-1-carboxylate (ACC) deaminase sourced from a bacterial strain Pseudomonas putida strain UW4 conferred transgenic B. napus plants with enhanced salt tolerance (Sergeeva et al., 2006). This gene decreases the negative implications of ethylene on plant growth and development by lowering the amount of ACC concentration, which is the immediate precursor of ethylene in plants.
Several other B. napus genes or genes sourced from other organisms involved in fatty acid metabolism, 5-Aminolevulinic acid (5-ALA) biosynthesis, phosphatidylinositol-specific signal transduction pathway, and flavonol biosynthesis have also been reported to impart tolerance to various abiotic stresses (Table 3). Utilization of stress-inducible promoters (e.g., pRD29A) can further enhance the stress-response and reduce the negative impacts of gene overexpression, if any. Engineering stress tolerance genes in the tissues more vulnerable to abiotic stress by using tissue specific promoters (Xu et al., 1993) is an efficient approach. The effective application of transgenic technology for imparting stress tolerance in B. napus can be further achieved by introducing multiple stress regulating genes at the same time. This can be attained either by gene pyramiding or utilizing multi-gene transformation vectors. In this direction, Wang, Z., et al (2018) took advantage of Gateway technology and the multiple rounds in vivo site-specific assembly (MISSA) method and introduced five different genes into a multi-gene transformation vector pABA-oriT. NCED3 (Nine-Cis-Epoxycarotenoid Dioxygenase 3), ABAR (ABA Receptor, magnesium-chelatase subunit chlH), CBF3 (C-repeat Binding Factor 3), LOS5 (molybdenum cofactor sulfurase, ABA3), and ICE1 (interactor of little elongation complex ELL subunit 1) were the five genes introduced. The resultant transgenics exhibited enhanced growth when compared to wildtype. Single gene effect or combinatorial effect of multiple genes rendered these transgenic plants tolerant to multiple abiotic stresses including salinity, drought, and heat.
Table 3 Studies with transgenic overexpression of stress responsive genes in B. napus with the aim of imparting abiotic stress tolerance.
Abiotic stresses trigger transcriptional, post-transcriptional, and translational regulation of the expression of stress-responsive genes (Contreras-Cubas et al., 2012; Jeknić et al., 2014). Emerging evidence has shown that non-coding RNAs (ncRNAs) also participate in the modulation of stress-responsive gene expression. However, stress-responsive regulatory networks concerning ncRNAs are poorly understood, and unravelling such mechanisms is further a convoluted yet necessary task.
The ncRNAs are functional RNAs that do not encode or have a lower potential to encode proteins. These are a diverse group of RNA molecules classified mostly based on their location, length, genomic origin, or mode of action. A series of functionally important non-coding RNAs have been identified including the canonical ncRNAs such as transfer RNAs (tRNAs) and ribosomal RNAs (rRNAs); and regulatory RNAs, such as micro- RNAs (miRNAs), long non-coding RNAs (lncRNAs), circular RNAs (circRNAs), and others (Shin and Shin, 2016). In this section, we will review the role of ncRNAs in abiotic stress response and tolerance mechanisms.
miRNAs are short, single-stranded, 20–24 nucleotide long RNA molecules that control the expression and accumulation of target mRNAs. They indirectly modulate various biological processes such as organ development (Chen, 2004; Xie et al., 2015), phase transition (Hong and Jackson, 2015), stress response (Hackenberg et al., 2015; Zhao J. et al., 2016; Megha et al., 2018b), and several other plant regulatory pathways (Curaba et al., 2014). miRNAs originate from primary miRNA (pri-miRNA), which arise from the transcription of nuclear-encoded miRNA (MIR) genes, usually by DNA dependent RNA Polymerase II or RNA polymerase III (Voinnet, 2009; Wang J. et al., 2019). Interestingly, MIR genes are differentially regulated in response to abiotic stress in a species or family-dependent manner. Thus, the differential regulation of a miRNA is also species-specific, and so is the regulation of their target mRNAs. For instance, miR166 is reported to upregulate in wheat, whereas its expression is downregulated in rice under drought conditions (Shriram et al., 2016).
The miRNA dependent regulation of the target mRNA gene expression occurs either at the transcriptional level by site-specific methylation or at the translational level by the degradation of mRNA, inhibition of translation or RNA deadenylation (Wang J. et al., 2019). miRNAs regulate their target genes in a “Universal Reverse Manner”. This means that if a miRNA is upregulated, then its target genes are downregulated and vice versa. The activity of miRNAs in the stress-responsive regulatory networks is not well understood, and this task becomes more challenging as the response of a particular miRNA to the same abiotic stress is species-, developmental stage-, or tissue-specific (Sun X. et al., 2018). In addition, unravelling the exact mechanism of the regulation of stress response at miRNA level becomes difficult due to the regulation of several genes by one miRNA or multiple miRNAs regulating the expression of a single gene (Basso et al., 2019).
Recent investigations have revealed that miRNAs responsive to multiple abiotic stresses (Shriram et al., 2016; Basso et al., 2019). A series of stress-responsive miRNAs have been identified in Arabidopsis and other crop plants in response to drought, salinity, cold, heat, heavy metal toxicity, and nutrient deficiencies. Therefore, we will focus only on the differentially regulated miRNAs in B. napus in response to abiotic stress. In B. napus, the identification and expression analysis of miRNAs has been reported in response to cold temperature (Megha et al., 2018a), high concentrations of cadmium (Huang et al., 2010; Zhou et al., 2012; Jian et al., 2018) and also during early stages of seed germination under salt and drought stress (Jian et al., 2016b). These studies highlight the role of B. napus miRNAs in mediating developmental processes and abiotic stress response.
A total of 129 differentially regulated miRNA were identified in canola under cold stress and out of which only 25 were known (Megha et al., 2018a). Cold Stress (CS) responsive miRNAs such as miR169, miR319, miR396, which were reported in Arabidopsis, sugarcane, and soybean, were not observed in the case of B. napus. Also, cold stress responsive miRNAs did not show any consistent expression patterns with the miRNAs reported in other crops. The target genes of the CS responsive miRNAs were associated with different abiotic stresses such as heat, salt, and drought. Salt- and drought-responsive miRNAs during early-stage seed germination were identified by Jian et al. (2016b). Downregulation of miR156, miR169, miR860, miR399, miR71, and miR395 and upregulation of miR172 in response to drought was reported. MiR393 and miR399 showed an opposite regulation in response to salt stress. The identified miRNAs targeted disease resistance protein (DIRP), drought-responsive family protein (DRRP), early responsive to dehydration stress protein (ERD), stress-responsive alpha-beta barrel domain protein (SRAP), and salt tolerance homolog 2 (STH2), highlighting the regulation of stress-responsive genes by miRNAs.
In response to cadmium stress 44 known miRNAs (belonging to 27 families) and 103 novel miRNAs were identified in B. napus. Genes involved in transcription factor regulation, biotic stress response, ion homeostasis, and secondary metabolism were identified as corresponding target genes (Jian et al., 2018). Several Cd stress-responsive miRNAs have been reported and related regulatory mechanisms have also been discussed by Huang et al. (2010) and Zhou et al. (2012). miR395 and corresponding targets (BnSultr2;1, BnAPS3, and BnAPS4) as key regulators of cadmium stress were reported by Huang et al., 2010. Overexpression of miR395 in B. napus results in enhanced cadmium stress tolerance (Zhang et al., 2013). Transgenics exhibited a lower degree of Cd stress-induced oxidative damage, higher levels of biomass, and lower rates of Cd translocation from roots to leaves. The transgenics had higher expression of genes involved in heavy metal-tolerance such as BnPCS1, BnHO1, and Sultr1;1.
lncRNAs are ncRNA molecules that range from >200 nucleotides to >10 kb in length. In plants, the transcription of lncRNAs is carried out by RNA polymerase II, III, IV/V (Wierzbicki, 2012). The biogenesis of plant lncRNAs is not thoroughly understood. Among the hypotheses, lncRNAs can originate from duplication of existing lncRNAs, the decay of protein sequences, or transposable elements (Hou et al., 2019). lncRNAs have been classified based on their genomic location as (1) natural antisense transcripts (NATs): transcribed from the opposite strand of a gene transcript, (2) sense lncRNAs: transcribed from the same strand of a gene transcript, (3) intronic lncRNAs: transcribed from the introns and (4) intergenic lncRNAs (lincRNAs): transcribed from intergenic regions (Ma et al., 2013).
In B. napus, oil biosynthesis (Shen et al., 2018) and cadmium stress-responsive (Feng et al., 2016) lncRNAs have been identified suggesting their role in fatty acid metabolism and cadmium stress. A number of studies have reported lncRNAs as differentially expressed under various abiotic stresses in other crop plants (Table 4). These lncRNAs are not highly conserved and show a species-specific expression, and thus finding common lncRNAs across species is less probable. For instance, out of 664 drought-responsive lncRNAs identified in maize, only 126 were known, and 538 were novel (Zhang W. et al., 2014). Similarly, 41.9% of the differentially regulated lncRNAs in response to salt and boron stress were species-specific in a hyper-arid maize variety (Huanca-Mamani et al., 2018). lncRNAs also show higher tissue and developmental stage-specific expression than protein-coding genes in response to abiotic stresses. Most lncRNAs were reported to be drought-responsive during the reproductive stage in maize (Pang et al., 2019).
The number of lncRNAs responsive to certain stress also varies across species. In rice, 1434, cadmium stress-responsive lncRNAs are reported as compared to 301 in B. napus (Feng et al., 2016; Chen et al., 2018). This difference can also arise due to differences in methods applied for screening and identifying lncRNAs. 1,832 lncRNAs responsive to drought, cold, salinity, and ABA were reported in Arabidopsis, but the method used only detected lincRNAs (Liu et al., 2012). However, another study in the Medicago truncatula reported 5,634 lncRNAs responsive to drought by employing a method that can identify all types of lncRNAs (Wang T.-Z. et al., 2015).
lncRNAs acting as miRNA precursors have also been reported in response to various abiotic stresses. In B. napus, four lncRNAs act as precursors of miR824, miR167d, miR156d, and 156e in response to cadmium stress (Feng et al., 2016). In Tibetan wild barley out of 535 drought-responsive lncRNAs, 41 are putative miRNA precursors (Qiu et al., 2019). Similarly, in grapevine, 31 cold-responsive lncRNAs were potential precursors for 34 miRNAs where some of the miRNAs had multiple lncRNAs as precursors (Wang P. et al., 2019). Some studies have also reported lncNATs functioning as precursors of siRNAs under abiotic stress. lncNATs then participate in gene silencing of stress-responsive mRNAs through siRNAs. For instance, 34 lncNATS served as putative precursors of siRNAs, potentially targeting 37 cadmium stress-responsive mRNAs in B. napus (Feng et al., 2016). A number of lncNATs out of the 153 cold and/or drought-responsive lncNATs identified in cassava led to the formation of siRNAs (Li S. X. et al., 2017).
Target mimicry is another important mechanism by which lncRNAs regulate gene expression. miRNAs controlling the expression of stress-responsive mRNAs are blocked by binding of decoy lncRNAs. Number of lncRNAs have been reported to act as target mimics under various stresses for, e.g., 16 in cassava under cold and/drought stress (Li S. X. et al., 2017), 186 in wheat under cold stress (Diaz et al., 2019), 40 in Chinese cabbage under heat stress (Wang A. et al., 2019), and 3,560 in Barley under drought stress (Qiu et al., 2019). The number of target mimics and the number of miRNAs is not the same, indicating a complex network of cross-talk between miRNA and lncRNA. The functional validation of three cadmium stress-responsive lncRNAs which were predicted as target mimics identified an association between those lncRNAs and cadmium responsive genes such as natural resistance-associated macrophage protein 1 (Nramp1) type metal transporter and a Cu/Zn superoxide dismutase which was a component of the oxidative response machinery and metal transporters (Feng et al., 2016).
Cross-talk between lncRNA and miRNAs is suggested to impact the expression of various transcription factors with an important role in abiotic stress response. MiRNA-lncRNA co-expression networks in Chinese cabbage identified several transcription factors such as DREB2A, HSFs, ARFs, and HSPs under heat stress (Wang A. et al., 2019). Similarly, under drought and/or cold stress in cassava, 164 lincRNAs targeted NAC TFs, which play a role in drought tolerance, and 169 lincRNAs targeted NF-Y TFs which play a role in abiotic stresses (Li S. X. et al., 2017). These findings suggest an intricate mechanism by which lncRNAs might regulate stress response that needs experimental validation.
Although lncRNAs have been reported to be stress-responsive, their functional characterization is mostly lacking. In Arabidopsis, however, a drought, salt, and ABA-responsive lncRNA have been characterized. The DROUGHT INDUCED lncRNA (DRIR), which when overexpressed in Arabidopsis, imparted salt and drought tolerance and was reported to be more sensitive to ABA treatments. The gene expression in these overexpression lines also elucidated that this lncRNA acts as a positive regulator of abiotic stress response in Arabidopsis (Qin et al., 2017). Similar research is essential for other identified lncRNAs to facilitate the identification of candidates, which can impart multiple abiotic stress tolerance.
circRNAs are a class of newly characterized endogenous ncRNAs which are 100 nt to 4 kb in length. circRNAs were reported for the first time in the year 1976 as single-stranded circular RNA molecules in plant viroids (Sanger et al., 1976). These circular RNA molecules were considered as splicing errors for several decades due to their lower level of expression and difficulties involved in identification (Cocquerelle et al., 1993). However, with the advancement in sequencing technologies, genome-wide investigations have led to the discovery of thousands of circRNAs in bacteria (Danan et al., 2011), fungi (Wang et al., 2014), animals (Memczak et al., 2013), and human cells (Salzman et al., 2012). In plants, the first report of circRNA characterization is in Arabidopsis (Wang et al., 2014). They are highly conserved in eukaryotes and are involved in various biological processes (Ye et al., 2015). The biogenesis of circRNAs is unclear in plants. In animals, circRNAs are suggested to act as miRNA sponges and hence interfere with gene silencing mediated by miRNAs (Memczak et al., 2013). In plants, there is no experimental evidence so far to validate this mode of action; however, there are some studies that predict a potential role of plant circRNAs as miRNA sponges (Conn et al., 2017).
Abiotic stress regulation by circRNAs has not been extensively studied in plants. Few studies have reported stress-responsive expression of circRNAs in crop plants (Zhao et al., 2019). However, such research is missing in B. napus. In Arabidopsis, circRNAs responsive to drought (Zhang P. et al., 2019) and heat (Pan T. et al., 2018) stresses have been identified. Pan T., et al (2018) identified 1,583 heat-responsive circRNAs in Arabidopsis and highlighted that heat stress promotes the expression, abundancy, and exon circularization of circRNAs. Studies in other crops has also uncovered circRNAs responsive to phosphate starvation [rice, Ye et al. (2015)], drought [maize, Zhang, P., et al (2019); wheat, Wang, Y., et al (2017)] and cold [tomato, Zuo et al. (2016); grape, Gao et al. (2019)]. These studies suggest that circRNAs might regulate abiotic stress response in plants by modulating the stress-responsive gene expression. Though, the mechanism is not very clear, but it can be speculated from current research that either circRNAs act as miRNA sponges or they inhibit the biogenesis of sRNAs (Zhang P. et al., 2019) thus protecting the stress responsive transcripts from gene silencing.
Experimental evidence has validated the molecular mechanisms involved in circRNA-mediated stress response in the case of two circRNAs. CircGORK (Guard cell outward-rectifying K+-channel) was overexpressed in Arabidopsis (Zhang P. et al., 2019) for functional characterization. GORK gene is involved in water stress response and regulation of ABA-signaling. CircGORK overexpression lines suggested a positive regulation of drought tolerance by upregulation of several ABA-responsive genes. Similarly, a cold-responsive grape circRNA Vv-circATS1 was overexpressed in Arabidopsis (Gao et al., 2019). The overexpression of this circRNA resulted in enhanced cold tolerance, whereas its linear counterpart failed to show a similar impact. These reports provide functional tools and a framework for further characterization of circRNAs and their role in stress response regulation. circRNAs are also suggested to be utilized as molecular markers for breeding stress-tolerant varieties. Therefore, new research is warranted for the identification of candidate circRNAs that can be explored further for developing multiple stress-tolerant crop varieties.
In recent years, the role of several stress-responsive genes and ncRNAs has been extensively studied in plant's abiotic stress responses. Tremendous work has been carried out in developing databases and bioinformatics tools for stress-responsive gene identification and profiling. A comprehensive and more in-depth understanding of their mode of action, differential regulation patterns, identification of target genes, and ncRNA mediated gene regulatory machinery is necessary for B. napus. Investigations focusing on employing advanced tools of synthetic biology in cooperation with genetic engineering to develop an effective strategy for designing multiple abiotic stress-tolerant varieties in B. napus as well as other economically important crops are required.
Stress tolerance is a polygenic response due to the complex nature of networks involved in abiotic stress sensing, signal transduction, and expression of stress-responsive genes in plants. Gene transformation technologies have been successfully applied for imparting different stress tolerances in plants. However, this genetically complex mechanism of an abiotic stress response makes the task of engineering crops tolerant to multiple abiotic stresses extremely challenging.
Genome editing is an efficient approach for crop improvement either by loss of gene function, the gain of gene function, or a multiplex genome editing approach. Several different strategies have been developed for genome editing in plants due to the advent of engineered or designer nucleases. These nucleases can introduce double-stranded breaks (DSBs) at specific sites on the genome. These DSBs are then repaired either by non-homologous end joining (NHEJ) or homology-directed repair (HDR). NHEJ is error-prone, whereas HDR results in precise insertion, deletion, or substitution events (Sedeek et al., 2019). The nucleases that found application in efficient genome editing in plants are ZFNs (Zinc finger nucleases), TALENs (Transcription activator-like effector nucleases), and CRISPR-Cas9. Each class of nucleases has its advantages and disadvantages. However, CRISPR-Cas9 (Clustered regularly interspaced short palindromic repeats-Cas9) due to its simplicity and precision was quickly established as the preferred gene-editing technique.
The discovery of CRISPR and its subsequent adaptation to CRISPR/Cas9 technology was a revolutionary step in genome editing. Three CRISPR systems have been distinguished (types I–III). However, it is type II, which is successfully modified to the currently renowned CRISPR/Cas9 technology (Hsu et al., 2014). This technology involves the inclusion of Cas9 nuclease, which binds to a constructed single guide RNA (sgRNA) (Doudna and Charpentier, 2014). sgRNA consists of an RNA-duplex and a guide sequence, this sequence determining the target DNA to which the sgRNA will bind before the associated Cas9 induces a double-stranded break (DSB). Although this approach is simple and easy, there is a limitation of offtargets. To reduce off-targeting by 50–1,000 folds, the use of mutant Cas9 known as DNA nickase has been reported (Cong et al., 2013; Ran et al., 2013). Other approaches include the fusion of fok1 nuclease with catalytically inactive cas9 protein (Tsai et al., 2014) and efficient designing of sgRNAs. The advancement in CRISPR/Cas9 system has led to the selection and screening of the transgenic plants in a way that only the plants with the desired mutations and lose of the transgene are screened for (Bao et al., 2019). This provides a possible solution for the development of the non-GM crop, which can bypass the strict biosafety regulations required for genetically modified crops.
In B. napus, a few studies have utilized CRISPR/Cas9 system for editing genes associated with plant/pod development (ALCATRAZ, GA1-3, FRUITFULL, DA1, DA2, CLAVATA, and SPL3), fatty acid synthesis (BnFAD2), and biotic stress response (BnWRKY11and -70) (Sun Q. et al., 2018). The application of CRISPR-Cas9 for climate-resilient transgenic production is yet to be reported in canola (Table 5).
The workflow for generating a genetically engineered plant entails the delivery of DNA to plants, followed by transformants selection and regeneration of the genetically modified progeny. Delivery of DNA into plant cells can be achieved either by direct DNA delivery methods or Agrobacterium-mediated transformation. Direct DNA transfer methods such as PEG-mediated DNA uptake, electroporation, microinjection, and microprojectile bombardment have been explored for B. napus (Bergman and Glimelius, 1993; Jones-Villeneuve et al., 1995; Poulsen, 1996). Isolation of protoplasts and difficulties in regeneration of viable plants due to increased risk of somaclonal variations make the application of PEG mediated DNA uptake and electroporation limited. Microinjection for delivering genes to microspores as well as microprojectile bombardment has been successfully carried out in B. napus (Jones-Villeneuve et al., 1995). However, particle bombardment has low precision and relies on high-pressure delivery of DNA-coated gold particles by physical disruption of the cell wall, which can lead to multiple gene copies, DNA fragmentation, or integration of vector backbone.
The most common method for generating genetically modified B. napus is Agrobacterium-mediated transformation (Zhang et al., 2005; Bhalla and Singh, 2008). This method has also been successfully applied to other Brassica species (Bhalla and Smith, 1998; Smith and Bhalla, 1998; Bhalla and De Weerd, 1999). There are many success stories in the generation of GM canola with insect resistance, herbicide tolerance, or both as well as enhanced production of omega-3 fatty acids. These GM canola varieties are grown successfully, and the agriculture industry has reaped rewards of higher yield, better nutritional quality oil, and other agro-economic benefits by the adoption of GM canola technology. Transgenic research focusing on the development of abiotic stress tolerant B. napus also successfully utilizes Agrobacterium-mediated transformation techniques. However, drawbacks of this approach include the random nature of the gene insertion, possibility of disrupting functional genes, public concerns over genetically modified organisms (GMOs), failure to make use of the native genetic repertoire of the plant and subjections to GMO regulatory regulations. Also, there is a question of recalcitrant commercial canola varieties that require more efficient transformation techniques (Zhang and Bhalla, 2004).
Approaches challenging how GM crops are developed, regulated, and labeled are also required. This can be achieved by controlling biomolecule delivery to plants in an unassisted and accurate manner. In this direction, the application of nanotechnology for DNA delivery provides a potential solution (Landry and Mitter, 2019). Materials having at least one dimension >100 nm are defined as nanoparticles. When compared with the exclusion limit of a typical cell wall, which is 500 nm, these nanoparticles can act as efficient carriers of biomolecules into the plant cells. So far, nanoparticles such as mesoporous silica (few 100 nm) with a gene gun (Torney et al., 2007) and polyethyleneimine-coated Fe3O4 magnetic nanoparticles (MNP) using magnetic force (Zhao et al., 2017) have been investigated as nanocarriers of DNA in plant cells. Use of magnetic nanoparticles for transforming pollen and then pollinating plants with this magnetofected pollen resulted in the successful generation of transgenic cotton. The DNA delivered by this method was stably integrated into the genome and inherited in the successive generations (Zhao et al., 2017). Several other nanoparticles, such as carbon nanotubes (Demirer et al., 2019; Kwak et al., 2019), DNA nanostructures, and DNA origami (Zhang H. et al., 2019) have been successfully investigated for unassisted delivery of exogenous DNA. Recent advances in genome editing tools and pioneering research involving nanotechnology for transformation provides precise, unique, and efficient tools for generating GM crops. The integration of these technologies will shorten the time required for developing GM crop varieties and might assist in bypassing the GM regulatory purview, thus resulting in higher consumer acceptance of GM crops.
Plant synthetic biology, a rapidly emerging field that aims to combine plant biology with engineering principles (Kassaw et al., 2018). Initially, it was applied to bacterial systems, but now it has expanded to eukaryotic systems, including plants. Designing and construction of synthetic regulatory systems exhibiting desirable and predictable behavior using either a top to bottom or bottom-up design approach is a very useful tool which can be applied for developing plant varieties with novel traits.
For developing sentinel plants exhibiting desirable traits, the efficient designing of genetic circuits is a pre-requisite. The designing and assembly of genetic circuits can be carried out using various software such as Bio-CAD for designing (Nielsen et al., 2016) and Cell Modeller (Dupuy et al., 2007) for assembly of genetic parts. The orthogonal genetic components should have the ability to function independently of the endogenous regulatory mechanism of the plant (Morey et al., 2012; Chen et al., 2013). Also, the ability to externally control the activation of the genetic circuits can help us switch off/on the desirable trait as and when required. The incorporation of regulatory components such as terminators and insulators provide better control of the synthetic genetic circuit (Chen et al., 2013). However, plant genetic functions are complex and regulated by various environmental cues such as light, temperature, photoperiod, which in turn impact the synthetic gene circuit control. These factors, along with the gene regulatory information available from different omics studies, should be incorporated while developing sentinel plants.
Plant biosensors have been developed capable of sensing 2,4,6-trinitrotolune (TNT) via a two-component synthetic gene circuit (Antunes et al., 2011). First component acts as the transmembrane signal activator upon exposure to TNT. It then triggers the second component by activating the synthetic promoter. The promoter controls the activity of genes which inhibit chlorophyll synthesis and initiates its degradation. Arabidopsis and lettuce protoplasts were modified to signal for bacterial contamination efficiently via a gene circuit controlled by a positive autoregulatory transcriptional feedback loop (Czarnecka et al., 2012). This emerging technology has the potential to be applied broadly, with the development of sentinel plants that detect specific or multiple abiotic stresses such as heat, salinity, and drought in the field and initiates a gene regulatory network for imparting stress tolerance.
The agronomic importance of B. napus in global agriculture is a vital factor driving the necessity for innovative tools to combat multiple stresses in the current climatic scenario. Will biotechnology and particularly synthetic biology be the essential pieces to this puzzle are still needed to be developed fully, but the early indications do present a promising picture. The availability of the B. napus genome has led to the identification and characterization of various stress-responsive gene families such as HSFs, WRKY TFs, bZIP TFs, metal transporters, and so on. However, its in-depth analysis to untangle the complex stress-responsive networks and their cross-talks require further research. Identification of putative multiple abiotic stress regulatory genes or regulatory sequences and their efficient manipulation can lead to the development of the ultimate climate change resilient B. napus varieties. Several studies have reported the application of conventional genetic engineering for imparting abiotic stress tolerance in B. napus varieties. Overexpression of transcription factors, transporters, and genes involved in phytohormone biosynthesis, miRNAs, and other stress-responsive genes have been reported to be promising approaches for combating multiple abiotic stresses. Multi-gene co-expression for conferring stress tolerance in B. napus, as reported by Wang, Z., et al (2018), highlights the need for similar future research. Application of CRISPR/Cas9 editing technology for developing multiple abiotic stress resistance in B. napus is also required. Further optimization of transformation protocols by multiplex genetic engineering, application of nanoparticles for gene delivery, and synthetic biology for developing gene circuits for rapid signaling and efficient initiation of abiotic stress responses are opening new opportunities in this direction. The integration of innovative tools into the current genetic engineering programs will provide new prospects for the rational design of multiple stress-tolerant traits in B. napus.
PB and MS conceived the concept and the idea and supervised the project. NL and DJ collected the literature and compiled the information and wrote the article. MS and PB extensively edited the article.
The authors declare that the research was conducted in the absence of any commercial or financial relationships that could be construed as a potential conflict of interest.
We gratefully acknowledge financial support from the Australia-India Strategic Research Fund, GCF010009. NL and DJ were supported by The University of Melbourne Research Scholarship during this study.
Abdelmigid, H. M. (2016). Expression analysis of Type 1 and 2 Metallothionein genes in Rape (Brassica napus L.) during short-term stress using sqRT-PCR analysis. Indian J. Exp. Biol. 54, 212–218.
Aksouh-Harradj, N., Campbell, L., Mailer, R. J. (2006). Canola response to high and moderately high temperature stresses during seed maturation. Can. J. Plant Sci. 86, 967–980. doi: 10.4141/P05-130
Alam, M. M., Nahar, K., Hasanuzzaman, M., Fujita, M. (2014). Exogenous jasmonic acid modulates the physiology, antioxidant defense and glyoxalase systems in imparting drought stress tolerance in different Brassica species. Plant Biotechnol. Rep. 8, 279–293. doi: 10.1007/s11816-014-0321-8
Albrecht, V., Ritz, O., Linder, S., Harter, K., Kudla, J. (2001). The NAF domain defines a novel protein–protein interaction module conserved in Ca2+-regulated kinases. EMBO J. 20, 1051–1063. doi: 10.1093/emboj/20.5.1051
Angadi, S., Cutforth, H., Miller, P., Mcconkey, B., Entz, M., Brandt, S., et al. (2000). Response of three Brassica species to high temperature stress during reproductive growth. Can. J. Plant Sci. 80, 693–701. doi: 10.4141/P99-152
Antunes, M. S., Morey, K. J., Smith, J. J., Albrecht, K. D., Bowen, T. A., Zdunek, J. K., et al. (2011). Programmable ligand detection system in plants through a synthetic signal transduction pathway. PloS One 6, e16292. doi: 10.1371/journal.pone.0016292
Asai, T., Tena, G., Plotnikova, J., Willmann, M. R., Chiu, W.-L., Gomez-Gomez, L., et al. (2002). MAP kinase signalling cascade in Arabidopsis innate immunity. Nature 415, 977. doi: 10.1038/415977a
Asano, T., Hayashi, N., Kobayashi, M., Aoki, N., Miyao, A., Mitsuhara, I., et al. (2012). A rice calcium-dependent protein kinase OsCPK12 oppositely modulates salt-stress tolerance and blast disease resistance. Plant J. 69, 26–36. doi: 10.1111/j.1365-313X.2011.04766.x
Babula-Skowrońska, D., Ludwików, A., Cieśla, A., Olejnik, A., Cegielska-Taras, T., Bartkowiak-Broda, I., et al. (2015). Involvement of genes encoding ABI1 protein phosphatases in the response of Brassica napus L. @ to drought stress. Plant Mol. Biol. 88, 445–457. doi: 10.1007/s11103-015-0334-x
Bailey-Serres, J., Voesenek, L. A. (2010). Life in the balance: a signaling network controlling survival of flooding. Curr. Opin. Plant Biol. 13, 489–494. doi: 10.1016/j.pbi.2010.08.002
Bao, A., Burritt, D. J., Chen, H., Zhou, X., Cao, D., Tran, L.-S. P. (2019). The CRISPR/Cas9 system and its applications in crop genome editing. Crit. Rev. In Biotechnol. 39, 321–336. doi: 10.1080/07388551.2018.1554621
Basso, M. F., Ferreira, P. C. G., Kobayashi, A. K., Harmon, F. G., Nepomuceno, A. L., Molinari, H. B. C., et al. (2019). Micro RNA s and new biotechnological tools for its modulation and improving stress tolerance in plants. Plant Biotechnol. J 17 (8), 1482–1500. doi: 10.1111/pbi.13116
Baxter, A., Mittler, R., Suzuki, N. (2014). ROS as key players in plant stress signalling. J. Exp. Bot. 65, 1229–1240. doi: 10.1093/jxb/ert375
Benáková, M., Ahmadi, H., Dučaiová, Z., Tylová, E., Clemens, S., Tůma, J. (2017). Effects of Cd and Zn on physiological and anatomical properties of hydroponically grown Brassica napus plants. Environ. Sci. Pollut. Res. 24, 20705–20716. doi: 10.1007/s11356-017-9697-7
Bergman, P., Glimelius, K. (1993). Electroporation of rapeseed protoplasts–transient and stable transformation. Physiol. Plant. 88, 604–611. doi: 10.1111/j.1399-3054.1993.tb01378.x
Bhalla, P. L., De Weerd, N. (1999). In vitro propagation of cauliflower, Brassica oleracea var. botrytis for hybrid seed production. Plant Cell Tissue Organ Culture 56, 89–95. doi: 10.1023/A:1006221218191
Bhalla, P. L., Singh, M. B. (2008). Agrobacterium-mediated transformation of Brassica napus and Brassica oleracea. Nat. Protoc. 3, 181. doi: 10.1038/nprot.2007.527
Bhalla, P. L., Smith, N. (1998). Agrobacterium tumefaciens-mediated transformation of cauliflower, Brassica oleracea var. botrytis. Mol. Breed. 4, 531–541. doi: 10.1023/A:1009658614579
Boyer, J. S. (1982). Plant productivity and environment. Science 218, 443–448. doi: 10.1126/science.218.4571.443
Canvin, D. T. (1965). The effect of temperature on the oil content and fatty acid composition of the oils from several oil seed crops. Can. J. Bot. 43, 63–69. doi: 10.1139/b65-008
Chakravorty, D., Assmann, S. M. (2018). G protein subunit phosphorylation as a regulatory mechanism in heterotrimeric G protein signaling in mammals, yeast, and plants. Biochem. J. 475, 3331–3357. doi: 10.1042/BCJ20160819
Chalhoub, B., Denoeud, F., Liu, S., Parkin, I. A., Tang, H., Wang, X., et al. (2014). Early allopolyploid evolution in the post-Neolithic Brassica napus oilseed genome. Science 345, 950–953. doi: 10.1126/science.1253435
Chaves-Sanjuan, A., Sanchez-Barrena, M. J., Gonzalez-Rubio, J. M., Moreno, M., Ragel, P., Jimenez, M., et al. (2014). Structural basis of the regulatory mechanism of the plant CIPK family of protein kinases controlling ion homeostasis and abiotic stress. PNAS 111, E4532–E4541. doi: 10.1073/pnas.1407610111
Chen, L., Ren, F., Zhou, L., Wang, Q. Q., Zhong, H., Li, X. B. (2012). The Brassica napus calcineurin B-Like 1/CBL-interacting protein kinase 6 (CBL1/CIPK6) component is involved in the plant response to abiotic stress and ABA signalling. J. Exp. Bot. 63, 6211–6222. doi: 10.1093/jxb/ers273
Chen, Y.-J., Liu, P., Nielsen, A. A., Brophy, J. A., Clancy, K., Peterson, T., et al. (2013). Characterization of 582 natural and synthetic terminators and quantification of their design constraints. Nat. Methods 10, 659. doi: 10.1038/nmeth.2515
Chen, Y., Zhu, X., Zhu, X.-B., Yu, Y.-F., Ge, H.-M., Gao, Y., et al. (2014). Identification of the regulator of G-protein signaling protein responsive to plant hormones and abiotic stresses in Brassica napus. J. Integr. Agric. 13, 2634–2644. doi: 10.1016/S2095-3119(14)60797-8
Chen, Q., Niu, F., Yan, J., Chen, B., Wu, F., Guo, X., et al. (2017). Oilseed rape NAC56 transcription factor modulates reactive oxygen species accumulation and hypersensitive response-like cell death. Physiol. Plant. 160, 209–221. doi: 10.1111/ppl.12545
Chen, L., Shi, S., Jiang, N., Khanzada, H., Wassan, G. M., Zhu, C., et al. (2018). Genome-wide analysis of long non-coding RNAs affecting roots development at an early stage in the rice response to cadmium stress. BMC Genomics 19, 460. doi: 10.1186/s12864-018-4807-6
Chen, X. (2004). A microRNA as a translational repressor of APETALA2 in Arabidopsis flower development. Science 303, 2022–2025. doi: 10.1126/science.1088060
Chinnusamy, V., Schumaker, K., Zhu, J. K. (2004). Molecular genetic perspectives on cross-talk and specificity in abiotic stress signalling in plants. J. Exp. Bot. 55, 225–236. doi: 10.1093/jxb/erh005
Chmielowska-Bąk, J., Gzyl, J., Rucińska-Sobkowiak, R., Arasimowicz-Jelonek, M., Deckert, J. (2014). The new insights into cadmium sensing. Front. Plant Sci. 5, 245. doi: 10.3389/fpls.2014.00245
Choudhury, F. K., Rivero, R. M., Blumwald, E., Mittler, R. (2017). Reactive oxygen species, abiotic stress and stress combination. Plant J. 90, 856–867. doi: 10.1111/tpj.13299
Cocquerelle, C., Mascrez, B., Hetuin, D., Bailleul, B. (1993). Mis-splicing yields circular RNA molecules. FASEB J. 7, 155–160. doi: 10.1096/fasebj.7.1.7678559
Colebrook, E. H., Thomas, S. G., Phillips, A. L., Hedden, P. (2014). The role of gibberellin signalling in plant responses to abiotic stress. J. Exp. Biol. 217, 67–75. doi: 10.1242/jeb.089938
Cong, L., Ran, F. A., Cox, D., Lin, S., Barretto, R., Habib, N., et al. (2013). Multiplex genome engineering using CRISPR/Cas systems. Science 339, 819–823. doi: 10.1126/science.1231143
Conn, V. M., Hugouvieux, V., Nayak, A., Conos, S. A., Capovilla, G., Cildir, G., et al. (2017). A circRNA from SEPALLATA3 regulates splicing of its cognate mRNA through R-loop formation. Nat. Plants 3, 17053. doi: 10.1038/nplants.2017.53
Contreras-Cubas, C., Palomar, M., Arteaga-Vázquez, M., Reyes, J. L., Covarrubias, A. A. (2012). Non-coding RNAs in the plant response to abiotic stress. Planta 236, 943–958. doi: 10.1007/s00425-012-1693-z
Curaba, J., Singh, M. B., Bhalla, P. L. (2014). miRNAs in the crosstalk between phytohormone signalling pathways. J. Exp. Bot. 65, 1425–1438. doi: 10.1093/jxb/eru002
Czarnecka, E., Verner, F. L., Gurley, W. B. (2012). A strategy for building an amplified transcriptional switch to detect bacterial contamination of plants. Plant Mol. Biol. 78, 59–75. doi: 10.1007/s11103-011-9845-2
Dalal, M., Tayal, D., Chinnusamy, V., Bansal, K. C. (2009). Abiotic stress and ABA-inducible Group 4 LEA from Brassica napus plays a key role in salt and drought tolerance. J. Biotechnol. 139, 137–145. doi: 10.1016/j.jbiotec.2008.09.014
Danan, M., Schwartz, S., Edelheit, S., Sorek, R. (2011). Transcriptome-wide discovery of circular RNAs in Archaea. Nucleic Acids Res. 40, 3131–3142. doi: 10.1093/nar/gkr1009
Dar, T. A., Uddin, M., Khan, M. M. A., Hakeem, K., Jaleel, H. (2015). Jasmonates counter plant stress: a review. Environ. Exp. Bot. 115, 49–57. doi: 10.1016/j.envexpbot.2015.02.010
Demirer, G. S., Zhang, H., Matos, J. L., Goh, N. S., Cunningham, F. J., Sung, Y., et al. (2019). High aspect ratio nanomaterials enable delivery of functional genetic material without DNA integration in mature plants. Nat. Nanotechnol. 14, 456. doi: 10.1038/s41565-019-0382-5
Deng, F., Zhang, X., Wang, W., Yuan, R., Shen, F. (2018). Identification of Gossypium hirsutum long non-coding RNAs (lncRNAs) under salt stress. BMC Plant Biol. 18, 23. doi: 10.1186/s12870-018-1238-0
Di, F. F., Jian, H. J., Wang, T. Y., Chen, X. P., Ding, Y. R., Du, H., et al. (2018). Genome-wide analysis of the PYL gene family and identification of PYL genes that respond to abiotic stress in Brassica napus. Genes 9, 156. doi: 10.3390/genes9030156
Diaz, M. L., Soresi, D. S., Basualdo, J., Cuppari, S. J., Carrera, A. (2019). Transcriptomic response of durum wheat to cold stress at reproductive stage. Mol. Biol. Rep. 46, 2427–2445. doi: 10.1007/s11033-019-04704-y
Ding, Y., Jian, H., Wang, T., Di, F., Wang, J., Li, J., et al. (2018). Screening of candidate gene responses to cadmium stress by RNA sequencing in oilseed rape (Brassica napus L.). Environ. Sci. Pollut. Res. Int. 25, 32433–32446. doi: 10.1007/s11356-018-3227-0
Doherty, C. J., Van Buskirk, H. A., Myers, S. J., Thomashow, M. (2009). Roles for Arabidopsis CAMTA transcription factors in cold-regulated gene expression and freezing tolerance. Plant Cell 21, 972–984. doi: 10.1105/tpc.108.063958
Doudna, J. A., Charpentier, E. (2014). The new frontier of genome engineering with CRISPR-Cas9. Science 346, 1258096. doi: 10.1126/science.1258096
Du, H., Yang, C., Ding, G., Shi, L., Xu, F. (2017). Genome-wide identification and characterization of SPX domain-containing members and their responses to phosphate deficiency in Brassica napus. Front. In Plant Sci. 8, 35. doi: 10.3389/fpls.2017.00035
Dupuy, L., Mackenzie, J., Rudge, T., Haseloff, J. (2007). A system for modelling cell–cell interactions during plant morphogenesis. Ann. Bot. 101, 1255–1265. doi: 10.1093/aob/mcm235
Elferjani, R., Soolanayakanahally, R. (2018). Canola responses to drought, heat, and combined stress: shared and specific effects on carbon assimilation, seed yield, and oil composition. Front. Plant Sci. 9, 1224. doi: 10.3389/fpls.2018.01224
Fan, Y. H., Yu, M. N., Liu, M., Zhang, R., Sun, W., Qian, M. C., et al. (2017). Genome-wide identification, evolutionary and expression analyses of the GALACTINOL SYNTHASE gene family in rapeseed and tobacco. Int. J. Mol. Sci. 18, 2768. doi: 10.3390/ijms18122768
Fang, Y., Liao, K., Du, H., Xu, Y., Song, H., Li, X., et al. (2015). A stress-responsive NAC transcription factor SNAC3 confers heat and drought tolerance through modulation of reactive oxygen species in rice. J. Exp. Bot. 66, 6803–6817. doi: 10.1093/jxb/erv386
Farhoudi, R., Saeedipour, S. (2011). Effect of exogenous abscisic acid on antioxidant activity and salt tolerance in rapeseed (Brassica napus) cultivars. Res. Crops 12, 122–130.
Feng, S. J., Zhang, X. D., Liu, X. S., Tan, S. K., Chu, S. S., Meng, J. G., et al. (2016). Characterization of long non-coding RNAs involved in cadmium toxic response in Brassica napus. RSC Adv. 6, 82157–82173. doi: 10.1039/C6RA05459E
Ford, B. A., Ernest, J. R., Gendall, A. R. (2012). Identification and characterization of orthologs of AtNHX5 and AtNHX6 in Brassica napus. Front. Plant Sci. 3, 208. doi: 10.3389/fpls.2012.00208
Fragkostefanakis, S., Mesihovic, A., Simm, S., Paupière, M. J., Hu, Y., Paul, P., et al. (2016). HsfA2 controls the activity of developmentally and stress-regulated heat stress protection mechanisms in tomato male reproductive tissues. Plant Physiol. 170, 2461–2477. doi: 10.1104/pp.15.01913
Friedt, W., Snowdon, R. (2009). “Oilseed rape,” in Oil crops (New York, NY: Springer), 91–126. doi: 10.1007/978-0-387-77594-4_4
Gao, Y., Li, T., Liu, Y., Ren, C., Zhao, Y., Wang, M. (2010a). Isolation and characterization of gene encoding G protein alpha subunit protein responsive to plant hormones and abiotic stresses in Brassica napus. Mol. Biol. Rep. 37, 3957–3965. doi: 10.1007/s11033-010-0054-x
Gao, Y., Li, T., Zhao, Y., Ren, C., Zhang, Y., Wang, M. (2010b). Isolation and characterization of a G protein γ subunit gene responsive to plant hormones and abiotic stresses in Brassica napus L. Acta Physiol. Plant. 33, 391–399. doi: 10.1007/s11738-010-0558-y
Gao, Y., Zhao, Y., Li, T., Ren, C., Liu, Y., Wang, M. (2010c). Cloning and Characterization of a G protein β subunit gene responsive to plant hormones and abiotic stresses in Brassica napus. Plant Mol. Biol. Rep. 28, 450–459. doi: 10.1007/s11105-009-0169-1
Gao, Z., Li, J., Luo, M., Li, H., Chen, Q., Wang, L., et al. (2019). Characterization and cloning of grape circular RNAs identified the cold resistance-related Vv-circATS1. Plant Physiol. 180, 966–985. doi: 10.1104/pp.18.01331
Georges, F., Das, S., Ray, H., Bock, C., Nokhrina, K., Kolla, V. A., et al. (2009). Over-expression of Brassica napus phosphatidylinositol-phospholipase C2 in canola induces significant changes in gene expression and phytohormone distribution patterns, enhances drought tolerance and promotes early flowering and maturation. Plant Cell Environ. 32, 1664–1681. doi: 10.1111/j.1365-3040.2009.02027.x
Gibson, L., Mullen, R. (1996). Soybean seed composition under high day and night growth temperatures. J. Am. Oil Chem. Soc. 73, 733–737. doi: 10.1007/BF02517949
Gill, S. S., Tuteja, N. (2010). Reactive oxygen species and antioxidant machinery in abiotic stress tolerance in crop plants. Plant Physiol. Biochem. 48, 909–930. doi: 10.1016/j.plaphy.2010.08.016
Goff, K. E., Ramonell, K. M. (2007). The role and regulation of receptor-like kinases in plant defense. Gene Regul. Syst. Bio. 1, 117762500700100015. doi: 10.1177/117762500700100015
Gong, D., Guo, Y., Schumaker, K. S., Zhu, J.-K (2004). The SOS3 family of calcium sensors and SOS2 family of protein kinases in Arabidopsis. Plant Physiol. 134, 919–926. doi: 10.1104/pp.103.037440
Guo, M., Liu, J.-H., Ma, X., Luo, D.-X., Gong, Z.-H., Lu, M.-H. (2016). The plant heat stress transcription factors (HSFs): structure, regulation, and function in response to abiotic stresses. Front. Plant Sci. 7, 114. doi: 10.3389/fpls.2016.00114
Hackenberg, M., Gustafson, P., Langridge, P., Shi, B. J. (2015). Differential expression of micro RNA s and other small RNA s in barley between water and drought conditions. Plant Biotechnol. J. 13, 2–13. doi: 10.1111/pbi.12220
Hajiebrahimi, A., Owji, H., Hemmati, S. (2017). Genome-wide identification, functional prediction, and evolutionary analysis of the R2R3-MYB superfamily in Brassica napus. Genome 60, 797–814. doi: 10.1139/gen-2017-0059
Hasanuzzaman, M., Alam, M. M., Nahar, K., Ahamed, K. U., Fujita, M. (2014). Exogenous salicylic acid alleviates salt stress-induced oxidative damage in Brassica napus by enhancing the antioxidant defense and glyoxalase systems. Aust. J. Crop Sci. 8, 631.
Hasanuzzaman, M., Nahar, K., Gill, S. S., Alharby, H. F., Razafindrabe, B. H., Fujita, M. (2017). Hydrogen peroxide pretreatment mitigates cadmium-induced oxidative stress in Brassica napus L.: an intrinsic study on antioxidant defense and glyoxalase systems. Front. Plant Sci 8, 115. doi: 10.3389/fpls.2017.00115
Hasanuzzaman, M., Bhuyan, M., Anee, T. I., Parvin, K., Nahar, K., Mahmud, J. A., et al. (2019). Regulation of ascorbate-glutathione pathway in mitigating oxidative damage in plants under abiotic stress. Antioxidants 8, 384. doi: 10.3390/antiox8090384
He, F., Liu, Q., Zheng, L., Cui, Y., Shen, Z., Zheng, L. (2015). RNA-seq analysis of rice roots reveals the involvement of post-transcriptional regulation in response to cadmium stress. Front. Plant Sci. 6, 1136. doi: 10.3389/fpls.2015.01136
He, Y. J., Mao, S. S., Gao, Y. L., Zhu, L. Y., Wu, D. M., Cui, Y. X., et al. (2016). Genome-wide identification and expression analysis of WRKY transcription factors under multiple stresses in Brassica napus. PloS One 11, e015755. doi: 10.1371/journal.pone.0157558
He, X., Xie, S., Xie, P., Yao, M., Liu, W., Qin, L. W., et al. (2019). Genome-wide identification of stress-associated proteins (SAP) with A20/AN1 zinc finger domains associated with abiotic stresses responses in Brassica napus. Environ. Exp. Bot. 165, 108–119. doi: 10.1016/j.envexpbot.2019.05.007
Heidari, M. (2010). Nucleic acid metabolism, proline concentration and antioxidants enzyme activity in canola (Brassica nupus L.) under salinity stress. Agric. Sci. China 9, 504–511. doi: 10.1016/S1671-2927(09)60123-1
Hong, Y., Jackson, S. (2015). Floral induction and flower formation—the role and potential applications of mi RNA s. Plant Biotechnol. J. 13, 282–292. doi: 10.1111/pbi.12340
Hou, J., Lu, D., Mason, A. S., Li, B., Xiao, M., An, S., et al. (2019). Non-coding RNAs and transposable elements in plant genomes: emergence, regulatory mechanisms and roles in plant development and stress responses. Planta 250 (1), 1–18. doi: 10.1007/s00425-019-03166-7
Hsu, P. D., Lander, E. S., Zhang, F. (2014). Development and applications of CRISPR-Cas9 for genome engineering. Cell 157, 1262–1278. doi: 10.1016/j.cell.2014.05.010
Huanca-Mamani, W., Arias-Carrasco, R., Cardenas-Ninasivincha, S., Rojas-Herrera, M., Sepulveda-Hermosilla, G., Carlos Caris-Maldonado, J., et al. (2018). Long non-coding RNAs responsive to salt and boron stress in the hyper-arid Lluteno maize from atacama desert. Genes 9, 170. doi: 10.3390/genes9030170
Huang, J., Hirji, R., Adam, L., Rozwadowski, K. L., Hammerlindl, J. K., Keller, W. A., et al. (2000). Genetic engineering of glycinebetaine production toward enhancing stress tolerance in plants: metabolic limitations. Plant Physiol. 122, 747–756. doi: 10.1104/pp.122.3.747
Huang, S. Q., Xiang, A. L., Che, L. L., Chen, S., Li, H., Song, J. B., et al. (2010). A set of miRNAs from Brassica napus in response to sulphate deficiency and cadmium stress. Plant Biotechnol. J. 8, 887–899. doi: 10.1111/j.1467-7652.2010.00517.x
Jaglo, K. R., Kleff, S., Amundsen, K. L., Zhang, X., Haake, V., Zhang, J. Z., et al. (2001). Components of the Arabidopsis C-repeat/dehydration-responsive element binding factor cold-response pathway are conserved inbrassica napus and other plant species. Plant Physiol. 127, 910–917. doi: 10.1104/pp.010548
Jeknić, Z., Pillman, K. A., Dhillon, T., Skinner, J. S., Veisz, O., Cuesta-Marcos, A., et al. (2014). Hv-CBF2A overexpression in barley accelerates COR gene transcript accumulation and acquisition of freezing tolerance during cold acclimation. Plant Mol. Biol. 84, 67–82. doi: 10.1007/s11103-013-0119-z
Jian, H., Lu, K., Yang, B., Wang, T., Zhang, L., Zhang, A., et al. (2016a). Genome-wide analysis and expression profiling of the SUC and SWEET gene families of sucrose transporters in oilseed rape (Brassica napus L.). Front. Plant Sci. 7, 1464. doi: 10.3389/fpls.2016.01464
Jian, H., Wang, J., Wang, T., Wei, L., Li, J., Liu, L. (2016b). Identification of rapeseed microRNAs involved in early stage seed germination under salt and drought stresses. Front. Plant Sci. 7, 658. doi: 10.3389/fpls.2016.00658
Jian, H., Yang, B., Zhang, A., Ma, J., Ding, Y., Chen, Z., et al. (2018). Genome-wide identification of MicroRNAs in response to cadmium stress in oilseed rape (Brassica napus L.) using high-throughput sequencing. Int. J. Mol. Sci. 19, 1431. doi: 10.3390/ijms19051431
Jones-Villeneuve, E., Huang, B., Prudhomme, I., Bird, S., Kemble, R., Hattori, J., et al. (1995). Assessment of microinjection for introducing DNA into uninuclear microspores of rapeseed. Plant Cell Tissue Organ Culture 40, 97–100. doi: 10.1007/BF00041124
Kagale, S., Divi, U. K., Krochko, J. E., Keller, W. A., Krishna, P. (2007). Brassinosteroid confers tolerance in Arabidopsis thaliana and Brassica napus to a range of abiotic stresses. Planta 225, 353–364. doi: 10.1007/s00425-006-0361-6
Kant, S., Burch, D., Badenhorst, P., Palanisamy, R., Mason, J., Spangenberg, G. (2015). Regulated expression of a cytokinin biosynthesis gene IPT delays leaf senescence and improves yield under rainfed and irrigated conditions in canola (Brassica napus L.). PloS One 10, e0116349. doi: 10.1371/journal.pone.0116349
Kassaw, T. K., Donayre-Torres, A. J., Antunes, M. S., Morey, K. J., Medford, J. I. (2018). Engineering synthetic regulatory circuits in plants. Plant Sci. 273, 13–22. doi: 10.1016/j.plantsci.2018.04.005
Kazan, K. (2015). Diverse roles of jasmonates and ethylene in abiotic stress tolerance. Trends Plant Sci. 20, 219–229. doi: 10.1016/j.tplants.2015.02.001
Kim, J., Lee, W. J., Vu, T. T., Jeong, C. Y., Hong, S.-W., Lee, H. (2017). High accumulation of anthocyanins via the ectopic expression of AtDFR confers significant salt stress tolerance in Brassica napus L. Plant Cell Rep. 36, 1215–1224. doi: 10.1007/s00299-017-2147-7
Knight, H. (1999). Calcium signaling during abiotic stress in plants in International review of cytology (Academic Press) 195, 269–324. doi: 10.1016/S0074-7696(08)62707-2
Kuderova, A., Gallova, L., Kuricova, K., Nejedla, E., Curdova, A., Micenkova, L., et al. (2015). Identification of AHK2- and AHK3-like cytokinin receptors in Brassica napus reveals two subfamilies of AHK2 orthologues. J. Exp. Bot. 66, 339–353. doi: 10.1093/jxb/eru422
Kumar, M. N., Verslues, P.E. (2015). Stress physiology functions of the Arabidopsis histidine kinase cytokinin receptors. Physiol. Plant. 154, 369–380. doi: 10.1111/ppl.12290
Kurepin, L. V., Qaderi, M. M., Back, T. G., Reid, D. M., Pharis, R. P. (2008). A rapid effect of applied brassinolide on abscisic acid concentrations in Brassica napus leaf tissue subjected to short-term heat stress. Plant Growth Regul. 55, 165–167. doi: 10.1007/s10725-008-9276-5
Kwak, J. M., Mori, I. C., Pei, Z. M., Leonhardt, N., Torres, M. A., Dangl, J. L., et al. (2003). NADPH oxidase AtrbohD and AtrbohF genes function in ROS-dependent ABA signaling in Arabidopsis. EMBO J. 22, 2623–2633. doi: 10.1093/emboj/cdg277
Kwak, S.-Y., Lew, T. T. S., Sweeney, C. J., Koman, V. B., Wong, M. H., Bohmert-Tatarev, K., et al. (2019). Chloroplast-selective gene delivery and expression in planta using chitosan-complexed single-walled carbon nanotube carriers. Nat. Nanotechnol. 14, 447. doi: 10.1038/s41565-019-0375-4
Lan Thi Hoang, X., Du Nhi, N. H., Binh Anh Thu, N., Phuong Thao, N., Phan Tran, L.-S. (2017). Transcription factors and their roles in signal transduction in plants under abiotic stresses. Curr. Genomics 18, 483–497. doi: 10.2174/1389202918666170227150057
Landry, M. P., Mitter, N. (2019). How nanocarriers delivering cargos in plants can change the GMO landscape. Nat. Nanotechnol. 14, 512. doi: 10.1038/s41565-019-0463-5
Lang, S., Liu, X., Xue, H., Li, X., Wang, X. (2017). Functional characterization of BnHSFA4a as a heat shock transcription factor in controlling the re-establishment of desiccation tolerance in seeds. J. Exp. Bot. 68, 2361–2375. doi: 10.1093/jxb/erx097
Lee, B.-R., Islam, M. T., Park, S.-H., Jung, H.-I., Bae, D.-W., Kim, T.-H. (2019a). Characterization of salicylic acid-mediated modulation of the drought stress responses: reactive oxygen species, proline, and redox state in Brassica napus. Environ. Exp. Bot. 157, 1–10. doi: 10.1016/j.envexpbot.2018.09.013
Lee, B.-R., Islam, M. T., Park, S.-H., Lee, H., Bae, D.-W., Kim, T.-H. (2019b). Antagonistic shifting from abscisic acid-to salicylic acid-mediated sucrose accumulation contributes to drought tolerance in Brassica napus. Environ. Exp. Bot. 162, 38–47. doi: 10.1016/j.envexpbot.2019.02.001
Li, F., Wu, X., Tsang, E., Cutler, A. J. (2005). Transcriptional profiling of imbibed Brassica napus seed. Genomics 86, 718–730. doi: 10.1016/j.ygeno.2005.07.006
Li, L., Ye, C. F., Zhao, R., Li, X., Liu, W. Z., Wu, F. F., et al. (2015). Mitogen-activated protein kinase kinase kinase (MAPKKK) 4 from rapeseed (Brassica napus L.) is a novel member inducing ROS accumulation and cell death. Biochem. Biophys. Res. Commun. 467, 792–797. doi: 10.1016/j.bbrc.2015.10.063
Li, Q., Yin, M., Li, Y., Fan, C., Yang, Q., Wu, J., et al. (2015). Expression of Brassica napus TTG2, a regulator of trichome development, increases plant sensitivity to salt stress by suppressing the expression of auxin biosynthesis genes. J. Exp. Bot. 66, 5821–5836. doi: 10.1093/jxb/erv287
Li, H. T., Wang, B., Zhang, Q. H., Wang, J., King, G. J., Liu, K. D. (2017). Genome-wide analysis of the auxin/indoleacetic acid (Aux/IAA) gene family in allotetraploid rapeseed (Brassica napus L.). BMC Plant Biol. 17, 204. doi: 10.1186/s12870-017-1165-5
Li, S. X., Yu, X., Lei, N., Cheng, Z. H., Zhao, P. J., He, Y. K., et al. (2017). Genome-wide identification and functional prediction of cold and/or drought-responsive lncRNAs in cassava (vol 7, 45981, 2017). Sci. Rep. 7, 2. doi: 10.1038/srep46795
Li, N. N., Xiao, H., Sun, J. J., Wang, S. F., Wang, J. C., Chang, P., et al. (2018). Genome-wide analysis and expression profiling of the HMA gene family in Brassica napus under cd stress. Plant Soil 426, 365–381. doi: 10.1007/s11104-018-3637-2
Li, M. D., Wang, R. H., Liu, Z. Y., Wu, X. M., Wang, J. B. (2019). Genome-wide identification and analysis of the WUSCHEL-related homeobox (WOX) gene family in allotetraploid Brassica napus reveals changes in WOX genes during polyploidization. BMC Genomics 20, 317. doi: 10.1186/s12864-019-5684-3
Li, M., Xie, F., Zhang, Y., Tang, H. (2019). Identification and evolution of GRAS transcription factor family in four Brassica species In AIP Conference Proceedings. (AIP Publishing LLC) 2058 (1), 020028. doi: 10.1063/1.5085541
Liang, W., Yang, B., Yu, B.-J., Zhou, Z., Li, C., Jia, M., et al. (2013). Identification and analysis of MKK and MPK gene families in canola (Brassica napus L.). BMC Genomics 14, 392. doi: 10.1186/1471-2164-14-392
Liang, Y., Xiong, Z. Y., Zheng, J. X., Xu, D. Y., Zhu, Z. Y., Xiang, J., et al. (2016). Genome-wide identification, structural analysis and new insights into late embryogenesis abundant (LEA) gene family formation pattern in Brassica napus. Sci. Rep. 6, 24265. doi: 10.1038/srep24265
Liu, Y., He, C. (2016). Regulation of plant reactive oxygen species (ROS) in stress responses: learning from AtRBOHD. Plant Cell Rep. 35, 995–1007. doi: 10.1007/s00299-016-1950-x
Liu, H. T., Sun, D. Y., Zhou, R. G. (2005). Ca2+ and AtCaM3 are involved in the expression of heat shock protein gene in Arabidopsis. Plant Cell Environ. 28, 1276–1284. doi: 10.1111/j.1365-3040.2005.01365.x
Liu, J., Jung, C., Xu, J., Wang, H., Deng, S., Bernad, L., et al. (2012). Genome-wide analysis uncovers regulation of long intergenic noncoding RNAs in Arabidopsis. Plant Cell 24, 4333–4345. doi: 10.1105/tpc.112.102855
Liu, W. Z., Deng, M., Li, L., Yang, B., Li, H., Deng, H., et al. (2015). Rapeseed calcineurin B-like protein CBL4, interacting with CBL-interacting protein kinase CIPK24, modulates salt tolerance in plants. Biochem. Biophys. Res. Commun. 467, 467–471. doi: 10.1016/j.bbrc.2015.10.034
Liu, M., Chang, W., Fan, Y. H., Sun, W., Qu, C. M., Zhang, K., et al. (2018). Genome-wide identification and characterization of NODULE-INCEPTION-like protein (NLP) family genes in Brassica napus. Int. J. Mol. Sci. 19, 2270. doi: 10.3390/ijms19082270
Liu, P., Zhang, C., Ma, J. Q., Zhang, L. Y., Yang, B., Tang, X. Y., et al. (2018). Genome-wide identification and expression profiling of cytokinin oxidase/dehydrogenase (CKX) genes reveal likely roles in pod development and stress responses in oilseed rape (Brassica napus L.). Genes 9, 168. doi: 10.3390/genes9030168
Ljung, K., Nemhauser, J. L., Perata, P. (2015). New mechanistic links between sugar and hormone signalling networks. Curr. Opin. Plant Biol. 25, 130–137. doi: 10.1016/j.pbi.2015.05.022
Lohani, N., Golicz, A. A., Singh, M. B., Bhalla, P. L. (2019a). Genome-wide analysis of the Hsf gene family in Brassica oleracea and a comparative analysis of the Hsf gene family in B. oleracea, B. rapa and B. napus. Funct. Integr. Genomics 19, 515–531. doi: 10.1007/s10142-018-0649-1
Lohani, N., Singh, M. B., Bhalla, P. L.(2019b). High temperature susceptibility of sexual reproduction in crop plants. J. Exp. Bot 71 (2), 555–568. doi: 10.1093/jxb/erz426
Lou, D., Wang, H., Liang, G., Yu, D. (2017). OsSAPK2 confers abscisic acid sensitivity and tolerance to drought stress in rice. Front. Plant Sci. 8, 44. doi: 10.3389/fpls.2017.00993
Lu, X., Chen, X., Mu, M., Wang, J., Wang, X., Wang, D., et al. (2016). Genome-wide analysis of long noncoding RNAs and their responses to drought stress in cotton (Gossypium hirsutum L.). PloS One 11, e0156723. doi: 10.1371/journal.pone.0156723
Luo, J., Tang, S., Peng, X., Yan, X., Zeng, X., Li, J., et al. (2015). Elucidation of cross-talk and specificity of early response mechanisms to salt and PEG-simulated drought stresses in Brassica napus using comparative proteomic analysis. PloS One 10, e0138974. doi: 10.1371/journal.pone.0138974
Luo, J. L., Tang, S. H., Mei, F. L., Peng, X. J., Li, J., Li, X. F., et al. (2017). BnSIP1-1, a trihelix family gene, mediates abiotic stress tolerance and ABA signaling in Brassica napus. Front. Plant Sci. 8, 44. doi: 10.3389/fpls.2017.00044
Lv, Y., Fu, S., Chen, S., Zhang, W., Qi, C. (2016). Ethylene response factor BnERF2-like (ERF2. 4) from Brassica napus L. enhances submergence tolerance and alleviates oxidative damage caused by submergence in Arabidopsis thaliana. Crop J. 4, 199–211. doi: 10.1016/j.cj.2016.01.004
Ma, L., Bajic, V. B., Zhang, Z. (2013). On the classification of long non-coding RNAs. RNA Biol. 10, 924–933. doi: 10.4161/rna.24604
Maryan, K. E., Lahiji, H. S., Farrokhi, N., Komeleh, H. H. (2019). Analysis of Brassica napus dehydrins and their Co-Expression regulatory networks in relation to cold stress. Gene Expression Patterns 31, 7–17. doi: 10.1016/j.gep.2018.10.002
Matsukura, S., Mizoi, J., Yoshida, T., Todaka, D., Ito, Y., Maruyama, K., et al. (2010). Comprehensive analysis of rice DREB2-type genes that encode transcription factors involved in the expression of abiotic stress-responsive genes. Mol. Genet. Genomics 283, 185–196. doi: 10.1007/s00438-009-0506-y
Mccormack, E., Tsai, Y.-C., Braam, J. (2005). Handling calcium signaling: Arabidopsis CaMs and CMLs. Trends Plant Sci. 10, 383–389. doi: 10.1016/j.tplants.2005.07.001
Megha, S., Basu, U., Joshi, R. K., Kav, N. N. V. (2018a). Physiological studies and genome-wide microRNA profiling of cold-stressed Brassica napus. Plant Physiol. Biochem. 132, 1–17. doi: 10.1016/j.plaphy.2018.08.027
Megha, S., Basu, U., Kav, N. N. V. (2018b). Regulation of low temperature stress in plants by microRNAs. Plant Cell Environ. 41, 1–15. doi: 10.1111/pce.12956
Memczak, S., Jens, M., Elefsinioti, A., Torti, F., Krueger, J., Rybak, A., et al. (2013). Circular RNAs are a large class of animal RNAs with regulatory potency. Nature 495, 333. doi: 10.1038/nature11928
Meng, X., Wang, J.-R., Wang, G.-D., Liang, X.-Q., Li, X.-D., Meng, Q.-W. (2015). An R2R3-MYB gene, LeAN2, positively regulated the thermo-tolerance in transgenic tomato. J. Plant Physiol. 175, 1–8. doi: 10.1016/j.jplph.2014.09.018
Miura, K., Furumoto, T. (2013). Cold signaling and cold response in plants. Int. J. Mol. Sci. 14, 5312–5337. doi: 10.3390/ijms14035312
Mizoi, J., Shinozaki, K., Yamaguchi-Shinozaki, K. (2012). AP2/ERF family transcription factors in plant abiotic stress responses. Biochim. Biophys. Acta (BBA)-Gene Regul. Mech. 1819, 86–96. doi: 10.1016/j.bbagrm.2011.08.004
Morey, K. J., Antunes, M. S., Barrow, M. J., Solorzano, F. A., Havens, K. L., Smith, J. J., et al. (2012). Crosstalk between endogenous and synthetic components–synthetic signaling meets endogenous components. Biotechnol. J. 7, 846–855. doi: 10.1002/biot.201100487
Morrison, M. J., Stewart, D. W. (2002). Heat stress during flowering in summer Brassica. Crop Sci. 42, 797–803. doi: 10.2135/cropsci2002.7970
Muthusamy, M., Uma, S., Backiyarani, S., Saraswathi, M. S. (2015). Genome-wide screening for novel, drought stress-responsive long non-coding RNAs in drought-stressed leaf transcriptome of drought-tolerant and -susceptible banana (Musa spp) cultivars using Illumina high-throughput sequencing. Plant Biotechnol. Rep. 9, 279–286. doi: 10.1007/s11816-015-0363-6
Nakashima, K., Takasaki, H., Mizoi, J., Shinozaki, K., Yamaguchi-Shinozaki, K. (2012). NAC transcription factors in plant abiotic stress responses. Biochim. Biophys. Acta (BBA)-Gene Regul. Mech. 1819, 97–103. doi: 10.1016/j.bbagrm.2011.10.005
Nielsen, A. A., Der, B. S., Shin, J., Vaidyanathan, P., Paralanov, V., Strychalski, E. A., et al. (2016). Genetic circuit design automation. Science 352, aac7341. doi: 10.1126/science.aac7341
Niu, F., Wang, B., Wu, F., Yan, J., Li, L., Wang, C., et al. (2014). Canola (Brassica napus L.) NAC103 transcription factor gene is a novel player inducing reactive oxygen species accumulation and cell death in plants. Biochem. Biophys. Res. Commun. 454, 30–35. doi: 10.1016/j.bbrc.2014.10.057
Niu, F., Wang, C., Yan, J., Guo, X., Wu, F., Yang, B., et al. (2016). Functional characterization of NAC55 transcription factor from oilseed rape (Brassica napus L.) as a novel transcriptional activator modulating reactive oxygen species accumulation and cell death. Plant Mol. Biol. 92, 89–104. doi: 10.1007/s11103-016-0502-7
Nongpiur, R. C., Singla-Pareek, S. L., Pareek, A. (2019). The quest for ‘osmosensors' in plants. J. Exp. Bot 71 (2), 595–607. doi: 10.1093/jxb/erz263
Owji, H., Hajiebrahimi, A., Seradj, H., Hemmati, S. (2017). Identification and functional prediction of stress responsive AP2/ERF transcription factors in Brassica napus by genome-wide analysis. Comput. Biol. Chem. 71, 32–56. doi: 10.1016/j.compbiolchem.2017.09.004
Pan, J., Wang, J., Zhou, Z., Yan, Y., Zhang, W., Lu, W., et al. (2009). IrrE, a global regulator of extreme radiation resistance in Deinococcus radiodurans, enhances salt tolerance in Escherichia coli and Brassica napus. PloS One 4, e4422. doi: 10.1371/journal.pone.0004422
Pan, T., Sun, X., Liu, Y., Li, H., Deng, G., Lin, H., et al. (2018). Heat stress alters genome-wide profiles of circular RNAs in Arabidopsis. Plant Mol. Biol. 96, 217–229. doi: 10.1007/s11103-017-0684-7
Pan, Y., Zhu, M. C., Wang, S. X., Ma, G. Q., Huang, X. H., Qiao, C. L., et al. (2018). Genome-wide characterization and analysis of metallothionein family Genes that function in metal stress tolerance in Brassica napus L. Int. J. Mol. Sci. 19, 2181. doi: 10.3390/ijms19082181
Pandhair, V., Sekhon, B. S. (2006). Reactive oxygen species and antioxidants in plants: an overview. J. Plant Biochem. Biotechnol. 15, 71–78. doi: 10.1007/BF03321907
Pang, J., Zhang, X., Ma, X., Zhao, J. (2019). Spatio-temporal transcriptional dynamics of maize long non-coding RNAs responsive to drought stress. Genes 10, 138. doi: 10.3390/genes10020138
Peleg, Z., Blumwald, E. (2011). Hormone balance and abiotic stress tolerance in crop plants. Curr. Opin. Plant Biol. 14, 290–295. doi: 10.1016/j.pbi.2011.02.001
Peng, D., Zhou, B., Jiang, Y., Tan, X., Yuan, D., Zhang, L. (2018). Enhancing freezing tolerance of Brassica napus L. by overexpression of a stearoyl-acyl carrier protein desaturase gene (SAD) from Sapium sebiferum (L.) Roxb. Plant Sci. 272, 32–41. doi: 10.1016/j.plantsci.2018.03.028
Petrov, V., Hille, J., Mueller-Roeber, B., Gechev, T. S. (2015). ROS-mediated abiotic stress-induced programmed cell death in plants. Front. Plant Sci 6, 69. doi: 10.3389/fpls.2015.00069
Ping, X. K., Wang, T. Y., Lin, N., Di, F. F., Li, Y. Y., Jian, H. J., et al. (2019). Genome-wide identification of the LAC gene family and its expression analysis under stress in Brassica napus. Molecules 24, 1985. doi: 10.3390/molecules24101985
Poulsen, G. (1996). Genetic transformation of Brassica. Plant Breed. 115, 209–225. doi: 10.1111/j.1439-0523.1996.tb00907.x
Purty, R. S., Kumar, G., Singla-Pareek, S. L., Pareek, A. (2008). Towards salinity tolerance in Brassica: an overview. Physiol. Mol. Biol. Plants 14, 39–49. doi: 10.1007/s12298-008-0004-4
Qi, X., Xie, S., Liu, Y., Yi, F., Yu, J. (2013). Genome-wide annotation of genes and noncoding RNAs of foxtail millet in response to simulated drought stress by deep sequencing. Plant Mol. Biol. 83, 459–473. doi: 10.1007/s11103-013-0104-6
Qin, F., Kakimoto, M., Sakuma, Y., Maruyama, K., Osakabe, Y., Tran, L. S. P., et al. (2007). Regulation and functional analysis of ZmDREB2A in response to drought and heat stresses in Zea mays L. Plant J. 50, 54–69. doi: 10.1111/j.1365-313X.2007.03034.x
Qin, T., Zhao, H., Cui, P., Albesher, N., Xiong, L. (2017). A nucleus-localized long non-coding RNA enhances drought and salt stress tolerance. Plant Physiol. 175, 1321–1336. doi: 10.1104/pp.17.00574
Qiu, C. W., Zhao, J., Chen, Q., Wu, F. B. (2019). Genome-wide characterization of drought stress responsive long non-coding RNAs in Tibetan wild barley. Environ. Exp. Bot. 164, 124–134. doi: 10.1016/j.envexpbot.2019.05.002
Rahman, H., Xu, Y.-P., Zhang, X.-R., Cai, X.-Z. (2016). Brassica napus genome possesses extraordinary high number of CAMTA genes and CAMTA3 contributes to pamp triggered immunity and resistance to sclerotinia sclerotiorum. Front. Plant Sci 7, 581. doi: 10.3389/fpls.2016.00581
Ran, F. A., Hsu, P. D., Lin, C.-Y., Gootenberg, J. S., Konermann, S., Trevino, A. E., et al. (2013). Double nicking by RNA-guided CRISPR Cas9 for enhanced genome editing specificity. Cell 154, 1380–1389. doi: 10.1016/j.cell.2013.08.021
Rehman, H. M., Nawaz, M. A., Shah, Z. H., Ludwig-Müller, J., Chung, G., Ahmad, M. Q., et al. (2018). Comparative genomic and transcriptomic analyses of Family-1 UDP glycosyltransferase in three Brassica species and Arabidopsis indicates stress-responsive regulation. Sci. Rep. 8, 1875. doi: 10.1038/s41598-018-19535-3
Ren, F., Zhao, C.-Z., Liu, C.-S., Huang, K.-L., Guo, Q.-Q., Chang, L.-L., et al. (2014). A Brassica napus PHT1 phosphate transporter, BnPht1; 4, promotes phosphate uptake and affects roots architecture of transgenic Arabidopsis. Plant Mol. Biol. 86, 595–607. doi: 10.1007/s11103-014-0249-y
Rezayian, M., Niknam, V., Ebrahimzadeh, H. (2018). Effects of drought stress on the seedling growth, development, and metabolic activity in different cultivars of canola. Soil Sci. Plant Nutr. 64, 360–369. doi: 10.1080/00380768.2018.1436407
Rizwan, M., Atta, B., Bilal, M., Noushahi, H. A., Ali, M. Y., Shabbir, M. A., et al. (2019). Effect of abiotic stresses on Brassica Species and role of transgenic breeding for adaptation. Asian J. Res. Crop Sci. 3 (1), 1–10. doi: 10.9734/ajrcs/2019/v3i130037
Ruelland, E., Zachowski, A. (2010). How plants sense temperature. Environ. Exp. Bot. 69, 225–232. doi: 10.1016/j.envexpbot.2010.05.011
Rushton, P. J., Somssich, I. E., Ringler, P., Shen, Q. J. (2010). WRKY transcription factors. Trends Plant Sci. 15, 247–258. doi: 10.1016/j.tplants.2010.02.006
Sabagh, A. E. L., Hossain, A., Barutçular, C., Islam, M. S., Ratnasekera, D., Kumar, N., et al. (2019). Drought and salinity stress management for higher and sustainable canola (Brassica napus L.) production: a critical review. Aust. J. Crop Sci. 13, 88–97. doi: 10.21475/ajcs.19.13.02.p1285
Sah, S. K., Reddy, K. R., Li, J. (2016). Abscisic acid and abiotic stress tolerance in crop plants. Front. Plant Sci 7, 571. doi: 10.3389/fpls.2016.00571
Sahni, S., Prasad, B. D., Liu, Q., Grbic, V., Sharpe, A., Singh, S. P., et al. (2016). Overexpression of the brassinosteroid biosynthetic gene DWF4 in Brassica napus simultaneously increases seed yield and stress tolerance. Sci. Rep. 6, 28298. doi: 10.1038/srep28298
Saidi, Y., Finka, A., Goloubinoff, P. (2011). Heat perception and signalling in plants: a tortuous path to thermotolerance. New Phytol. 190, 556–565. doi: 10.1111/j.1469-8137.2010.03571.x
Sakhno, L., Slyvets, M., Kuchuk, M. (2014). cyp11A1 Canola plants under short time heat stress conditions. Cytol. Genet. 48, 279–284. doi: 10.3103/S0095452714050090
Salzman, J., Gawad, C., Wang, P. L., Lacayo, N., Brown, P. O. (2012). Circular RNAs are the predominant transcript isoform from hundreds of human genes in diverse cell types. PloS One 7, e30733. doi: 10.1371/journal.pone.0030733
Sanger, H. L., Klotz, G., Riesner, D., Gross, H. J., Kleinschmidt, A. K. (1976). Viroids are single-stranded covalently closed circular RNA molecules existing as highly base-paired rod-like structures. Proc. Natl. Acad. Sci. 73, 3852–3856. doi: 10.1073/pnas.73.11.3852
Savitch, L. V., Allard, G., Seki, M., Robert, L. S., Tinker, N. A., Huner, N. P., et al. (2005). The effect of overexpression of two Brassica CBF/DREB1-like transcription factors on photosynthetic capacity and freezing tolerance in Brassica napus. Plant Cell Physiol. 46, 1525–1539. doi: 10.1093/pcp/pci165
Schmidt, R., Mieulet, D., Hubberten, H.-M., Obata, T., Hoefgen, R., Fernie, A. R., et al. (2013). SALT-RESPONSIVE ERF1 regulates reactive oxygen species–dependent signaling during the initial response to salt stress in rice. Plant Cell 25, 2115–2131. doi: 10.1105/tpc.113.113068
Sedeek, K. E., Mahas, A., Mahfouz, M. (2019). Plant genome engineering for targeted improvement of crop traits. Front. Plant Sci. 10, 114. doi: 10.3389/fpls.2019.00114
Seo, J. S., Sohn, H. B., Noh, K., Jung, C., An, J. H., Donovan, C. M., et al. (2012). Expression of the Arabidopsis AtMYB44 gene confers drought/salt-stress tolerance in transgenic soybean. Mol. Breed. 29, 601–608. doi: 10.1007/s11032-011-9576-8
Sergeeva, E., Shah, S., Glick, B. R. (2006). Growth of transgenic canola (Brassica napus cv. Westar) expressing a bacterial 1-aminocyclopropane-1-carboxylate (ACC) deaminase gene on high concentrations of salt. World J. Microbiol. Biotechnol. 22, 277–282. doi: 10.1007/s11274-005-9032-1
Shahzadi, T., Khan, F. A., Zafar, F., Ismail, A., Amin, E., Riaz, S. (2015). An overview of Brassica species for crop improvement. Am. Eurasian J. Agric. Environ. Sci 15, 1573. doi: 10.5829/idosi.aejaes.2015.15.8.12746
Sharma, P., Jha, A. B., Dubey, R. S., Pessarakli, M. (2012). Reactive oxygen species, oxidative damage, and antioxidative defense mechanism in plants under stressful conditions. J. Bot. 2012, 1–26. doi: 10.1155/2012/217037
Shen, Y.-G., Zhang, W.-K., He, S.-J., Zhang, J.-S., Liu, Q., Chen, S.-Y. (2003). An EREBP/AP2-type protein in Triticum aestivum was a DRE-binding transcription factor induced by cold, dehydration and ABA stress. Theor. Appl. Genet. 106, 923–930. doi: 10.1007/s00122-002-1131-x
Shen, E., Zhu, X., Hua, S., Chen, H., Ye, C., Zhou, L., et al. (2018). Genome-wide identification of oil biosynthesis-related long non-coding RNAs in allopolyploid Brassica napus. BMC Genomics 19, 745. doi: 10.1186/s12864-018-5117-8
Shi, Y., Tian, S., Hou, L., Huang, X., Zhang, X., Guo, H., et al. (2012). Ethylene signaling negatively regulates freezing tolerance by repressing expression of CBF and type-A ARR genes in Arabidopsis. Plant Cell 24, 2578–2595. doi: 10.1105/tpc.112.098640
Shi, J., Gao, H., Wang, H., Lafitte, H. R., Archibald, R. L., Yang, M., et al. (2017). ARGOS 8 variants generated by CRISPR-Cas9 improve maize grain yield under field drought stress conditions. Plant Biotechnol. J. 15, 207–216. doi: 10.1111/pbi.12603
Shim, D., Hwang, J.-U., Lee, J., Lee, S., Choi, Y., An, G., et al. (2009). Orthologs of the class A4 heat shock transcription factor HsfA4a confer cadmium tolerance in wheat and rice. Plant Cell 21, 4031–4043. doi: 10.1105/tpc.109.066902
Shin, S.-Y., Shin, C. (2016). Regulatory non-coding RNAs in plants: potential gene resources for the improvement of agricultural traits. Plant Biotechnol. Rep. 10, 35–47. doi: 10.1007/s11816-016-0389-4
Shokri-Gharelo, R., Noparvar, P. M. (2018). Molecular response of canola to salt stress: insights on tolerance mechanisms. PeerJ 6, e4822. doi: 10.7717/peerj.4822
Shriram, V., Kumar, V., Devarumath, R. M., Khare, T. S., Wani, S. H. (2016). MicroRNAs as potential targets for abiotic stress tolerance in plants. Front. Plant Sci. 7, 817. doi: 10.3389/fpls.2016.00817
Singh, A., Kushwaha, H. R., Soni, P., Gupta, H., Singla-Pareek, S. L., Pareek, A. (2015). Tissue specific and abiotic stress regulated transcription of histidine kinases in plants is also influenced by diurnal rhythm. Front. Plant Sci. 6, 711. doi: 10.3389/fpls.2015.00711
Smékalová, V., Doskočilová, A., Komis, G., Šamaj, J. (2014). Crosstalk between secondary messengers, hormones and MAPK modules during abiotic stress signalling in plants. Biotechnol. Adv. 32, 2–11. doi: 10.1016/j.biotechadv.2013.07.009
Smith, N. A., Bhalla, P. L. (1998). Comparison of shoot regeneration potential from seedling explants of Australian cauliflower (Brassica oleracea var. botrytis) varieties. Aust. J. Agric. Res. 49, 1261–1266. doi: 10.1071/A98067
Song, Y., Chen, L., Zhang, L., Yu, D. (2010). Overexpression of OsWRKY72 gene interferes in the abscisic acid signal and auxin transport pathway of Arabidopsis. J. Biosci. 35, 459–471. doi: 10.1007/s12038-010-0051-1
Song, C., Je, J., Hong, J. K., Lim, C. O. (2014). Ectopic expression of an Arabidopsis dehydration-responsive element-binding factor DREB2C improves salt stress tolerance in crucifers. Plant Cell Rep. 33, 1239–1254. doi: 10.1007/s00299-014-1612-9
Song, X., Liu, G., Huang, Z., Duan, W., Tan, H., Li, Y., et al. (2016). Temperature expression patterns of genes and their coexpression with LncRNAs revealed by RNA-Seq in non-heading Chinese cabbage. BMC Genomics 17, 297. doi: 10.1186/s12864-016-2625-2
Sun, Y., Wang, C., Yang, B., Wu, F., Hao, X., Liang, W., et al. (2014). Identification and functional analysis of mitogen-activated protein kinase kinase kinase (MAPKKK) genes in canola (Brassica napus L.). J. Exp. Bot. 65, 2171–2188. doi: 10.1093/jxb/eru092
Sun, X. E., Feng, X. X., Li, C., Zhang, Z. P., Wang, L. J. (2015). Study on salt tolerance with YHem1 transgenic canola (Brassica napus). Physiol. Plant. 154, 223–242. doi: 10.1111/ppl.12282
Sun, Q., Lin, L., Liu, D., Wu, D., Fang, Y., Wu, J., et al. (2018). CRISPR/Cas9-mediated multiplex genome editing of the BnWRKY11 and BnWRKY70 genes in Brassica napus L. Int. J. Mol. Sci. 19, 2716. doi: 10.3390/ijms19092716
Sun, X., Zheng, H., Sui, N. (2018). Regulation mechanism of long non-coding RNA in plant response to stress. Biochem. Biophys. Res. Commun. 503, 402–407. doi: 10.1016/j.bbrc.2018.07.072
Suzuki, N., Koussevitzky, S., Mittler, R., Miller, G. (2012). ROS and redox signalling in the response of plants to abiotic stress. Plant Cell Environ. 35, 259–270. doi: 10.1111/j.1365-3040.2011.02336.x
Torney, F., Trewyn, B. G., Lin, V. S.-Y., Wang, K. (2007). Mesoporous silica nanoparticles deliver DNA and chemicals into plants. Nat. Nanotechnol. 2, 295. doi: 10.1038/nnano.2007.108
Torres, M. A., Dangl, J. L. (2005). Functions of the respiratory burst oxidase in biotic interactions, abiotic stress and development. Curr. Opin. Plant Biol. 8, 397–403. doi: 10.1016/j.pbi.2005.05.014
Tran, L. S., Shinozaki, K., Yamaguchi-Shinozaki, K. (2010). Role of cytokinin responsive two-component system in ABA and osmotic stress signalings. Plant Signal Behav. 5, 148–150. doi: 10.4161/psb.5.2.10411
Trenberth, K. E., Dai, A., Van Der Schrier, G., Jones, P. D., Barichivich, J., Briffa, K. R., et al. (2014). Global warming and changes in drought. Nat. Clim. Change 4, 17. doi: 10.1038/nclimate2067
Tsai, S. Q., Wyvekens, N., Khayter, C., Foden, J. A., Thapar, V., Reyon, D., et al. (2014). Dimeric CRISPR RNA-guided FokI nucleases for highly specific genome editing. Nat. Biotechnol. 32, 569. doi: 10.1038/nbt.2908
Tuteja, N., Sopory, S.K. (2008). Plant signaling in stress: G-protein coupled receptors, heterotrimeric G-proteins and signal coupling via phospholipases . Plant Signal. Behav. 3, 79–86. doi: 10.4161/psb.3.2.5303
USDA FAS. (2018). Oilseeds: World Markets and Trade (Foreign Agricultural Service/USDA). Available from: https://apps.fas.usda.gov/psdonline/circulars/oilseeds.pdf
Virdi, A. S., Singh, S., Singh, P. (2015). Abiotic stress responses in plants: roles of calmodulin-regulated proteins. Front. Plant Sci. 6, 809. doi: 10.3389/fpls.2015.00809
Vishwakarma, K., Upadhyay, N., Kumar, N., Yadav, G., Singh, J., Mishra, R. K., et al. (2017). Abscisic acid signaling and abiotic stress tolerance in plants: a review on current knowledge and future prospects. Front. Plant Sci. 8, 161. doi: 10.3389/fpls.2017.00161
Vishwakarma, K., Mishra, M., Patil, G., Mulkey, S., Ramawat, N., Pratap Singh, V., et al. (2019). Avenues of the membrane transport system in adaptation of plants to abiotic stresses. Crit. Rev. Biotechnol. 39, 861–883. doi: 10.1080/07388551.2019.1616669
Voinnet, O. (2009). Origin, biogenesis, and activity of plant microRNAs. Cell 136, 669–687. doi: 10.1016/j.cell.2009.01.046
Wang, X., Liu, A. (2014). Expression of genes controlling unsaturated fatty acids biosynthesis and oil deposition in developing seeds of Sacha inchi (Plukenetia volubilis L.). Lipids 49, 1019–1031. doi: 10.1007/s11745-014-3938-z
Wang, W., Vinocur, B., Altman, A. (2003). Plant responses to drought, salinity and extreme temperatures: towards genetic engineering for stress tolerance. Planta 218, 1–14. doi: 10.1007/s00425-003-1105-5
Wang, J., Zuo, K., Wu, W., Song, J., Sun, X., Lin, J., et al. (2004). Expression of a novel antiporter gene from Brassica napus resulted in enhanced salt tolerance in transgenic tobacco plants. Biol. Plant. 48, 509–515. doi: 10.1023/B:BIOP.0000047145.18014.a3
Wang, J., Lydiate, D. J., Parkin, I. A., Falentin, C., Delourme, R., Carion, P. W., et al. (2011). Integration of linkage maps for the amphidiploid Brassica napus and comparative mapping with Arabidopsis and Brassica rapa. BMC Genomics 12, 101. doi: 10.1186/1471-2164-12-101
Wang, G.-F., Li, W.-Q., Li, W.-Y., Wu, G.-L., Zhou, C.-Y., Chen, K. -M. (2013). Characterization of rice NADPH oxidase genes and their expression under various environmental conditions. Int. J. Mol. Sci. 14, 9440–9458. doi: 10.3390/ijms14059440
Wang, P. L., Bao, Y., Yee, M.-C., Barrett, S. P., Hogan, G. J., Olsen, M. N., et al. (2014). Circular RNA is expressed across the eukaryotic tree of life. PloS One 9, e90859. doi: 10.1371/journal.pone.0090859
Wang, B., Guo, X., Wang, C., Ma, J., Niu, F., Zhang, H., et al. (2015). Identification and characterization of plant-specific NAC gene family in canola (Brassica napus L.) reveal novel members involved in cell death. Plant Mol. Biol. 87, 395–411. doi: 10.1007/s11103-015-0286-1
Wang, R., Xu, L., Zhu, X., Zhai, L., Wang, Y., Yu, R., et al. (2015). Transcriptome-wide characterization of novel and heat-stress-responsive microRNAs in radish (Raphanus Sativus L.) using next-generation sequencing. Plant Mol. Biol. Rep. 33, 867–880. doi: 10.1007/s11105-014-0786-1
Wang, T.-Z., Liu, M., Zhao, M.-G., Chen, R., Zhang, W.-H. (2015). Identification and characterization of long non-coding RNAs involved in osmotic and salt stress in Medicago truncatula using genome-wide high-throughput sequencing. BMC Plant Biol. 15, 131. doi: 10.1186/s12870-015-0530-5
Wang, L., Chen, L., Li, R., Zhao, R., Yang, M., Sheng, J., et al. (2017). Reduced drought tolerance by CRISPR/Cas9-mediated SlMAPK3 mutagenesis in tomato plants. J. Agric. Food Chem. 65, 8674–8682. doi: 10.1021/acs.jafc.7b02745
Wang, Y., Yang, M., Wei, S., Qin, F., Zhao, H., Suo, B. (2017). Identification of circular RNAs and their targets in leaves of Triticum aestivum L. under dehydration stress. Front. Plant Sci. 7, 2024. doi: 10.3389/fpls.2016.02024
Wang, M. M., Liu, M. M., Ran, F., Guo, P. C., Ke, Y. Z., Wu, Y. W., et al. (2018). Global analysis of WOX transcription factor gene family in Brassica napus reveals their stress- and hormone-responsive patterns. Int. J. Mol. Sci. 19, 3470. doi: 10.3390/ijms19113470
Wang, W., Zhang, H., Wei, X., Yang, L., Yang, B., Zhang, L., et al. (2018). Functional characterization of calcium-dependent protein kinase (CPK) 2 gene from oilseed rape (Brassica napus L.) in regulating reactive oxygen species signaling and cell death control. Gene 651, 49–56. doi: 10.1016/j.gene.2018.02.006
Wang, Y., Gao, L., Zhu, B., Zhu, H., Luo, Y., Wang, Q., et al. (2018). Integrative analysis of long non-coding RNA acting as ceRNAs involved in chilling injury in tomato fruit. Gene 667, 25–33. doi: 10.1016/j.gene.2018.05.030
Wang, Z., Yang, C., Chen, H., Wang, P., Wang, P., Song, C., et al. (2018). Multi-gene co-expression can improve comprehensive resistance to multiple abiotic stresses in Brassica napus L. Plant Sci. 274, 410–419. doi: 10.1016/j.plantsci.2018.06.014
Wang, A., Hue, J., Gao, C., Chen, G., Wang, B., Lin, C., et al. (2019). Genome-wide analysis of long non-coding RNAs unveils the regulatory roles in the heat tolerance of Chinese cabbage (Brassica rapa ssp. chinensis). Sci. Rep. 9, 5002. doi: 10.1038/s41598-019-41428-2
Wang, J., Mei, J., Ren, G. (2019). Plant microRNAs: biogenesis, homeostasis and degradation. Front. Plant Sci. 10, 360. doi: 10.3389/fpls.2019.00360
Wang, P., Dai, L., Ai, J., Wang, Y., Ren, F. (2019). Identification and functional prediction of cold-related long non-coding RNA (lncRNA) in grapevine. Sci. Rep. 9, 6638. doi: 10.1038/s41598-019-43269-5
Wani, S. H., Kumar, V., Shriram, V., Sah, S. K. (2016). Phytohormones and their metabolic engineering for abiotic stress tolerance in crop plants. Crop J. 4, 162–176. doi: 10.1016/j.cj.2016.01.010
Wei, L. J., Zhu, Y., Liu, R. Y., Zhang, A. X., Zhu, M. C., Xu, W., et al. (2019). Genome wide identification and comparative analysis of glutathione transferases (GST) family genes in Brassica napus. Sci. Rep. 9, 9196. doi: 10.1038/s41598-019-45744-5
Weinl, S., Kudla, J. (2009). The CBL–CIPK Ca2+-decoding signaling network: function and perspectives. New Phytol. 184, 517–528. doi: 10.1111/j.1469-8137.2009.02938.x
Wen, J., Guo, P. C., Ke, Y. Z., Liu, M. M., Li, P. F., Wu, Y. W., et al. (2019). The auxin response factor gene family in allopolyploid Brassica napus. PloS One 14, e0214885. doi: 10.1371/journal.pone.0214885
Weng, C.-M., Lu, J.-X., Wan, H.-F., Wang, S.-W., Wang, Z., Lu, K., et al. (2014). Over-Expression of BnMAPK1 in Brassica napus Enhances Tolerance to Drought Stress. J. Integr. Agric. 13, 2407–2415. doi: 10.1016/S2095-3119(13)60696-6
Whalley, H. J., Knight, M. R. (2013). Calcium signatures are decoded by plants to give specific gene responses. New Phytol. 197, 690–693. doi: 10.1111/nph.12087
Wierzbicki, A. T. (2012). The role of long non-coding RNA in transcriptional gene silencing. Curr. Opin. Plant Biol. 15, 517–522. doi: 10.1016/j.pbi.2012.08.008
Wu, Y. W., Ke, Y. Z., Wen, J., Guo, P. C., Ran, F., Wang, M. M., et al. (2018). Evolution and expression analyses of the MADS-box gene family in Brassica napus. PloS One 13, e0200762. doi: 10.1371/journal.pone.0200762
Xiao, L., Shang, X.-H., Cao, S., Xie, X.-Y., Zeng, W.-D., Lu, L.-Y., et al. (2019). Comparative physiology and transcriptome analysis allows for identification of lncRNAs imparting tolerance to drought stress in autotetraploid cassava. BMC Genomics 20, 514. doi: 10.1186/s12864-019-5895-7
Xie, F., Jones, D. C., Wang, Q., Sun, R., Zhang, B. (2015). Small RNA sequencing identifies miRNA roles in ovule and fibre development. Plant Biotechnol. J. 13, 355–369. doi: 10.1111/pbi.12296
Xin, M., Wang, Y., Yao, Y., Song, N., Hu, Z., Qin, D., et al. (2011). Identification and characterization of wheat long non-protein coding RNAs responsive to powdery mildew infection and heat stress by using microarray analysis and SBS sequencing. BMC Plant Biol. 11, 61. doi: 10.1186/1471-2229-11-61
Xu, P., Cai, W. (2017). Functional characterization of the BnNCED3 gene in Brassica napus. Plant Sci. 256, 16–24. doi: 10.1016/j.plantsci.2016.11.012
Xu, P., Cai, W. (2019). Function of Brassica napus BnABI3 in Arabidopsis gs1, an allele of AtABI3, in seed development and stress response. Front. Plant Sci. 10, 67. doi: 10.3389/fpls.2019.00067
Xu, J., Dai, H. (2016). Brassica napus cycling Dof Factor1 (BnCDF1) is involved in flowering time and freezing tolerance. Plant Growth Regul. 80, 315–322. doi: 10.1007/s10725-016-0168-9
Xu, H., Davies, S. P., Kwan, B. Y., O'brien, A. P., Singh, M., Knox, R. B. (1993). Haploid and diploid expression of a Brassica campestris anther-specific gene promoter in Arabidopsis and tobacco. Mol. Gen. Genet. MGG 239, 58–65. doi: 10.1007/BF00281601
Xu, L., Lin, Z. Y., Tao, Q., Liang, M. X., Zhao, G. M., Yin, X. Z., et al. (2014). Multiple NUCLEAR FACTOR Y transcription factors respond to abiotic stress in Brassica napus L. PloS One 30, e111354. doi: 10.1371/journal.pone.0111354
Xu, L., Zeng, W. J., Li, J. J., Liu, H., Yan, G. J., Si, P., et al. (2019). Characteristics of membrane-bound fatty acid desaturase (FAD) genes in Brassica napus L. and their expressions under different cadmium and salinity stresses. Environ. Exp. Bot. 162, 144–156. doi: 10.1016/j.envexpbot.2019.02.016
Yan, J., Tong, T., Li, X., Chen, Q., Dai, M., Niu, F., et al. (2017). A novel NAC-type transcription factor, NAC87, from oilseed rape modulates reactive oxygen species accumulation and cell death. Plant Cell Physiol. 59, 290–303. doi: 10.1093/pcp/pcx184
Ye, C. Y., Chen, L., Liu, C., Zhu, Q. H., Fan, L. (2015). Widespread noncoding circular RNA s in plants. New Phytol. 208, 88–95. doi: 10.1111/nph.13585
Ye, Y., Ding, Y., Jiang, Q., Wang, F., Sun, J., Zhu, C. (2017). The role of receptor-like protein kinases (RLKs) in abiotic stress response in plants. Plant Cell Rep. 36, 235–242. doi: 10.1007/s00299-016-2084-x
Ying, L., Chen, H., Cai, W. (2014). BnNAC485 is involved in abiotic stress responses and flowering time in Brassica napus. Plant Physiol. Biochem. 79, 77–87. doi: 10.1016/j.plaphy.2014.03.004
Yoo, M. J., Ma, T. Y., Zhu, N., Liu, L. H., Harmon, A. C., Wang, Q. M., et al. (2016). Genome-wide identification and homeolog-specific expression analysis of the SnRK2 genes in Brassica napus guard cells. Plant Mol. Biol. 91, 211–227. doi: 10.1007/s11103-016-0456-9
Yoshida, T., Mogami, J., Yamaguchi-Shinozaki, K. (2014). ABA-dependent and ABA-independent signaling in response to osmotic stress in plants. Curr. Opin. Plant Biol. 21, 133–139. doi: 10.1016/j.pbi.2014.07.009
You, J., Chan, Z. (2015). ROS regulation during abiotic stress responses in crop plants. Front. Plant Sci 6, 1092. doi: 10.3389/fpls.2015.01092
Young, L. W., Wilen, R. W., Bonham-Smith, P. C. (2004). High temperature stress of Brassica napus during flowering reduces micro- and megagametophyte fertility, induces fruit abortion, and disrupts seed production. J. Exp. Bot. 55, 485–495. doi: 10.1093/jxb/erh038
Yu, S. W., Zhang, L. D., Chen, C., Li, J. J., Ye, S. F., Liu, G. L., et al. (2014). Isolation and characterization of BnMKK1 responsive to multiple stresses and affecting plant architecture in tobacco. Acta Physiol. Plant. 36, 1313–1324. doi: 10.1007/s11738-014-1510-3
Yuan, F., Yang, H., Xue, Y., Kong, D., Ye, R., Li, C., et al. (2014). OSCA1 mediates osmotic-stress-evoked Ca2+ increases vital for osmosensing in Arabidopsis. Nature 514, 367–371. doi: 10.1038/nature13593
Yuan, D., Li, W., Hua, Y. P., King, G. J., Xu, F. S., Shi, L. (2017). Genome-wide identification and characterization of the aquaporin gene family and transcriptional responses to boron deficiency in Brassica napus. Front. Plant Sci. 8, 1336. doi: 10.3389/fpls.2017.01336
Zhang, Y., Bhalla, P. L. (2004). In vitro shoot regeneration from commercial cultivars of Australian canola (Brassica napus L.). Aust. J. Agric. Res. 55, 753–756. doi: 10.1071/AR03209
Zhang, H.-X., Hodson, J. N., Williams, J. P., Blumwald, E. (2001). Engineering salt-tolerant Brassica plants: characterization of yield and seed oil quality in transgenic plants with increased vacuolar sodium accumulation. Proc. Natl. Acad. Sci. 98, 12832–12836. doi: 10.1073/pnas.231476498
Zhang, Y., Singh, M. B., Swoboda, I., Bhalla, P. L. (2005). Agrobacterium-mediated transformation and generation of male sterile lines of Australian canola. Aust. J. Agric. Res. 56, 353–361. doi: 10.1071/AR04175
Zhang, L. W., Song, J. B., Shu, X. X., Zhang, Y., Yang, Z. M. (2013). miR395 is involved in detoxification of cadmium in Brassica napus. J. Hazard. Mater. 250, 204–211. doi: 10.1016/j.jhazmat.2013.01.053
Zhang, H. F., Liu, W. Z., Zhang, Y. P., Deng, M., Niu, F. F., Yang, B., et al. (2014). Identification, expression and interaction analyses of calcium-dependent protein kinase (CPK) genes in canola (Brassica napus L.). BMC Genomics 15, 211. doi: 10.1186/1471-2164-15-211
Zhang, W., Han, Z., Guo, Q., Liu, Y., Zheng, Y., Wu, F., et al. (2014). Identification of maize long non-coding RNAs responsive to drought stress. PloS One 9, e98958. doi: 10.1371/journal.pone.0098958
Zhang, X., Lu, G., Long, W., Zou, X., Li, F., Nishio, T. (2014). Recent progress in drought and salt tolerance studies in Brassica crops. Breed Sci. 64, 60–73. doi: 10.1270/jsbbs.64.60
Zhang, X. D., Meng, J. G., Zhao, K. X., Chen, X., Yang, Z. M. (2018a). Annotation and characterization of Cd-responsive metal transporter genes in rapeseed (Brassica napus). Biometals 31, 107–121. doi: 10.1007/s10534-017-0072-4
Zhang, X. D., Zhao, K. X., Yang, Z. M. (2018b). Identification of genomic ATP binding cassette (ABC) transporter genes and Cd-responsive ABCs in Brassica napus. Gene 664, 139–151. doi: 10.1016/j.gene.2018.04.060
Zhang, H., Demirer, G. S., Zhang, H., Ye, T., Goh, N. S., Aditham, A. J., et al. (2019). DNA nanostructures coordinate gene silencing in mature plants. Proc. Natl. Acad. Sci. 116, 7543–7548. doi: 10.1073/pnas.1818290116
Zhang, P., Fan, Y., Sun, X., Chen, L., Terzaghi, W., Bucher, E., et al. (2019). A large-scale circular RNA profiling reveals universal molecular mechanisms responsive to drought stress in maize and Arabidopsis. Plant J. 98, 697–713. doi: 10.1111/tpj.14267
Zhao, B.-Y., Hu, Y.-F., Li, J.-J., Yao, X. (2016). BnaABF2, a bZIP transcription factor from rapeseed (Brassica napus L.), enhances drought and salt tolerance in transgenic Arabidopsis. Bot. Stud. 57, 12. doi: 10.1186/s40529-016-0127-9
Zhao, J., He, Q., Chen, G., Wang, L., Jin, B. (2016). Regulation of non-coding RNAs in heat stress responses of plants. Front. Plant Sci. 7, 1213. doi: 10.3389/fpls.2016.01213
Zhao, X., Meng, Z., Wang, Y., Chen, W., Sun, C., Cui, B., et al. (2017). Pollen magnetofection for genetic modification with magnetic nanoparticles as gene carriers. Nat. Plants 3, 956. doi: 10.1038/s41477-017-0063-z
Zhao, W., Chu, S., Jiao, Y. (2019). Present scenario of circular RNAs (circRNAs) in plants. Front. Plant Sci. 10, 379. doi: 10.3389/fpls.2019.00379
Zhong, H., Guo, Q.-Q., Chen, L., Ren, F., Wang, Q.-Q., Zheng, Y., et al. (2012). Two Brassica napus genes encoding NAC transcription factors are involved in response to high-salinity stress. Plant Cell Rep. 31, 1991–2003. doi: 10.1007/s00299-012-1311-3
Zhou, Q. Y., Tian, A. G., Zou, H. F., Xie, Z. M., Lei, G., Huang, J., et al. (2008). Soybean WRKY-type transcription factor genes, GmWRKY13, GmWRKY21, and GmWRKY54, confer differential tolerance to abiotic stresses in transgenic Arabidopsis plants. Plant Biotechnol. J. 6, 486–503. doi: 10.1111/j.1467-7652.2008.00336.x
Zhou, Z. S., Song, J. B., Yang, Z. M. (2012). Genome-wide identification of Brassica napus microRNAs and their targets in response to cadmium. J. Exp. Bot. 63, 4597–4613. doi: 10.1093/jxb/ers136
Zhou, Y., Xu, D. X., Jia, L. D., Huang, X. H., Ma, G. Q., Wang, S. X., et al. (2017). Genome-wide identification and structural analysis of bZIP transcription factor genes in Brassica napus. Genes 8, e288. doi: 10.3390/genes8100288
Zhu, M., Assmann, S. M. (2017). Metabolic signatures in response to abscisic acid (ABA) treatment in Brassica napus guard cells revealed by metabolomics. Sci. Rep. 7, 12875. doi: 10.1038/s41598-017-13166-w
Zhu, B., Ye, C., Lü, H., Chen, X., Chai, G., Chen, J., et al. (2006). Identification and characterization of a novel heat shock transcription factor gene, GmHsfA1, in soybeans (Glycine max). J. Plant Res. 119, 247–256. doi: 10.1007/s10265-006-0267-1
Zhu, J. Q., Zhang, J. T., Tang, R. J., Lv, Q. D., Wang, Q. Q., Yang, L., et al. (2009). Molecular characterization of ThIPK2, an inositol polyphosphate kinase gene homolog from Thellungiella halophila, and its heterologous expression to improve abiotic stress tolerance in Brassica napus. Physiol. Plant. 136, 407–425. doi: 10.1111/j.1399-3054.2009.01235.x
Zhu, X., Qi, L., Liu, X., Cai, S., Xu, H., Huang, R., et al. (2014). The wheat ethylene response factor transcription factor pathogen-induced ERF1 mediates host responses to both the necrotrophic pathogen Rhizoctonia cerealis and freezing stresses. Plant Physiol. 164, 1499–1514. doi: 10.1104/pp.113.229575
Zhu, M., Monroe, J. G., Suhail, Y., Villiers, F., Mullen, J., Pater, D., et al. (2016). Molecular and systems approaches towards drought-tolerant canola crops. New Phytol. 210, 1169–1189. doi: 10.1111/nph.13866
Zhu, X. Y., Huang, C. Q., Zhang, L., Liu, H. F., Yu, J. H., Hu, Z. Y., et al. (2017). Systematic analysis of Hsf family genes in the brassica napus genome reveals novel responses to heat, drought and high CO2 stresses. Front. Plant Sci. 8, 1174. doi: 10.3389/fpls.2017.01174
Zuo, J., Wang, Q., Zhu, B., Luo, Y., Gao, L. (2016). Deciphering the roles of circRNAs on chilling injury in tomato. Biochem. Biophys. Res. Commun. 479, 132–138. doi: 10.1016/j.bbrc.2016.07.032
Keywords: abiotic stress, Brassica napus, canola, multiple stress tolerance, CRISPR-Cas9, biotechnology, synthetic biology
Citation: Lohani N, Jain D, Singh MB and Bhalla PL (2020) Engineering Multiple Abiotic Stress Tolerance in Canola, Brassica napus. Front. Plant Sci. 11:3. doi: 10.3389/fpls.2020.00003
Received: 16 October 2019; Accepted: 03 January 2020;
Published: 25 February 2020.
Edited by:
Henrik Scheller, Lawrence Berkeley National Laboratory, United StatesReviewed by:
Mirza Hasanuzzaman, Sher-e-Bangla Agricultural University, BangladeshCopyright © 2020 Lohani, Jain, Singh and Bhalla. This is an open-access article distributed under the terms of the Creative Commons Attribution License (CC BY). The use, distribution or reproduction in other forums is permitted, provided the original author(s) and the copyright owner(s) are credited and that the original publication in this journal is cited, in accordance with accepted academic practice. No use, distribution or reproduction is permitted which does not comply with these terms.
*Correspondence: Prem L. Bhalla, cHJlbWxiQHVuaW1lbGIuZWR1LmF1
Disclaimer: All claims expressed in this article are solely those of the authors and do not necessarily represent those of their affiliated organizations, or those of the publisher, the editors and the reviewers. Any product that may be evaluated in this article or claim that may be made by its manufacturer is not guaranteed or endorsed by the publisher.
Research integrity at Frontiers
Learn more about the work of our research integrity team to safeguard the quality of each article we publish.