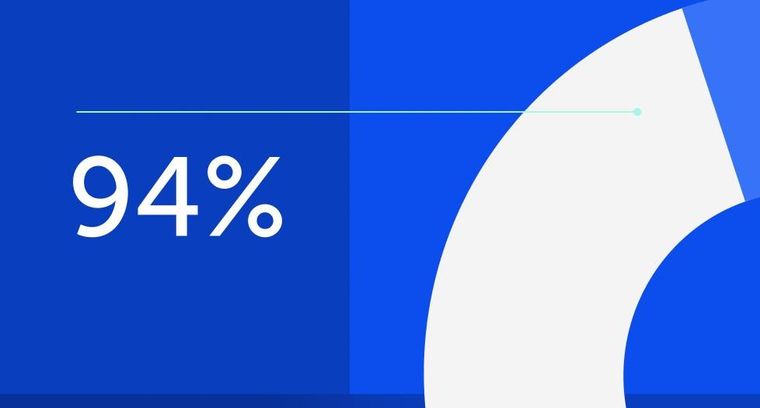
94% of researchers rate our articles as excellent or good
Learn more about the work of our research integrity team to safeguard the quality of each article we publish.
Find out more
ORIGINAL RESEARCH article
Front. Plant Sci., 09 January 2020
Sec. Plant Pathogen Interactions
Volume 10 - 2019 | https://doi.org/10.3389/fpls.2019.01643
This article is part of the Research TopicPlant-Soil Interactions under Changing ClimateView all 14 articles
Examining factors that influence seedling establishment is essential for predicting the impacts of climate change on tree species’ distributions. Seedlings originating from contrasting climates differentially express functional traits related to water and nutrient uptake and drought resistance that reflect their climate of origin and influence their responses to drought. Soil microbes may improve seedling establishment because they can enhance water and nutrient uptake and drought resistance. However, the relative influence of soil microbes on the expression of these functional traits between seedling families or populations from contrasting climates is unknown. To determine if soil microbes may differentially alter functional traits to enhance water and nutrient uptake and drought resistance between dry and wet families, seeds of loblolly pine families from the driest and wettest ends of its geographic range (dry, wet) were planted in sterilized sand (controls) or in sterilized sand inoculated with a soil microbial community (inoculated). Functional traits related to seedling establishment (germination), water and nutrient uptake and C allocation (root:shoot biomass ratio, root exudate concentration, leaf C:N, leaf N isotope composition (δ15N)), and drought resistance (turgor loss point, leaf carbon isotope composition (δ13C)) were measured. Then, plants were exposed to a drought treatment and possible shifts in photosynthetic performance were monitored using chlorophyll fluorescence. Inoculated plants exhibited significantly greater germination than controls regardless of family. The inoculation treatment significantly increased root:shoot biomass ratio in the wet family but not in the dry family, suggesting soil microbes alter functional traits that improve water and nutrient uptake more so in a family originating from a wetter climate than in a family originating from a drier climate. Microbial effects on photosynthetic performance during drought also differed between families, as photosynthetic performance of the dry inoculated group declined fastest. Regardless of treatment, the dry family exhibited a greater root:shoot biomass ratio, root exudate concentration, and leaf δ15N than the wet family. This indicates that the dry family allocated more resources belowground than the wet and the two family may have used different sources of plant available N, which may be related to their contrasting climates of origin and influence their drought resistance. Examination of variation in impacts of soil microbes on seedling physiology improves efforts to enhance seedling establishment and beneficial plant-microbe interactions under climate change.
Increased intensity and frequency of heat waves, drought, and wildfire (IPCC, 2018) have led to widespread forest mortality in recent decades (Allen et al., 2010; Hartmann et al., 2018). To sustain forests for ecosystem services and atmospheric CO2 sequestration, it is essential to understand vegetation responses to changing climate that determine forest species’ geographic distributions under future climate regimes. Successful seedling establishment is a key determinant of future tree species’ distributions (Harper, 1977; Jackson et al., 2009; Zhu et al., 2012; Davis et al., 2016; Simeone et al., 2019). However, the seedling stage is the most vulnerable developmental stage of plants because seedlings are small and delicate with limited access to water and nutrients, which exacerbates their susceptibility to climate change stresses like drought (Johnson et al., 2011; García de la Serrana et al., 2015; Chen et al., 2016; Yao et al., 2018). Therefore, examining factors that influence seedling establishment and mortality (Kursar et al., 2009; Sapes et al., 2019) are crucial for predicting future species’ distributions.
Intraspecific (within-species) variation in the expression of functional traits related to water and nutrient uptake influences seedling establishment and physiological responses to drought (Sultan, 2000; Howe et al., 2003; Kawecki and Ebert, 2004; Isaac-Renton et al., 2018; Ramírez‐Valiente et al., 2018; Roches et al., 2018; Chauvin et al., 2019; Roskilly et al., 2019). Intraspecific adaptations to their climate of origin collectively enable a species to survive in diverse climates and span a large geographic range. As regions shift to more arid conditions under climate change, there is great research interest in identifying populations and families that will thrive under more arid conditions to facilitate adaptation and reforestation efforts (O’Neill et al., 2008; Grady et al., 2015; Moran et al., 2017). Provenance, greenhouse, and common garden studies have been used to examine how the differential expression of functional traits in seedling populations and families from contrasting climates influences physiological responses to drought. Populations and families from drier climates often exhibit functional traits that enable them to enhance water and nutrient uptake and resist drought more effectively than populations and families from wetter climates (Fernández et al., 1999; Cregg and Zhang, 2001; Nguyen-Queyrens and Bouchet-Lannat, 2003; Gratani, 2014; Kerr et al., 2015; Carvalho et al., 2017; Marias et al., 2017; Moran et al., 2017). Drought resistance is defined as the capacity of plants to avoid or tolerate drought, which is achieved through diverse physiological mechanisms (Levitt, 1980; Khanna-Chopra and Singh, 2015; Polle et al., 2019). Compared to seedling populations and families from wetter climates, seedling populations and families from drier climates can exhibit increased resource allocation to root growth which enhances water and nutrient uptake. Seedling populations and families from drier climates also can exhibit greater leaf carbon isotope ratios which indicate greater intrinsic water use efficiency and greater stomatal constraints on gas exchange, and lower leaf turgor loss point which can indicate greater drought tolerance (Grossnickle et al., 1996; Cregg and Zhang, 2001; Nguyen-Queyrens and Bouchet-Lannat, 2003; López et al., 2009; Bartlett et al., 2014; Kerr et al., 2015; Carvalho et al., 2017; Marias et al., 2017).
Soil microbial communities of bacteria and fungi have been suggested as a solution to improve seedling establishment because they can alter functional traits related to water and nutrient uptake and drought resistance (Kim et al., 2012). However, we do not know if and when soil microbial impacts on seedling function are positive or negative. Soil microbial communities and host-specific microbial associates can manipulate plant hormone signaling to stimulate root growth and water uptake (Bent et al., 2001; Vonderwell et al., 2001; Verbon and Liberman, 2016), increase nutrient availability to enhance nutrient uptake (Yang et al., 2009), and alter soil moisture conditions to delay the onset of drought (Gehring et al., 2017; Kannenberg and Phillips, 2017) and promote germination (Ulrich et al., 2019). Therefore, beneficial plant-microbe interactions can improve seedling establishment and adaptation to new conditions (Compant et al., 2010). However, soil microbes can also negatively influence plant function through pathogenesis and disease (Rodriguez et al., 2008; Mendes et al., 2013; Jacoby et al., 2017; Schirawski and Perlin, 2018). Furthermore, sustaining microbial symbionts comes at a significant C cost to plant hosts via the release of root exudates (Bais et al., 2006). The positive and negative impacts of soil microbes on seedlings, plus the overwhelming diversity of microbes in the soil, complicates prediction of soil microbial influences on plant physiological responses to drought (Allison and Martiny, 2008; Mendes et al., 2013; Finkel et al., 2017; Sánchez-Cañizares et al., 2017). Therefore, investigating how soil microbes influence seedling physiological response to drought in diverse systems is greatly needed (Dimkpa et al., 2009; de Zelicourt et al., 2013; Baldrian, 2017).
Given the ability of seedling populations and families from contrasting climates to differentially respond to drought, populations and families from contrasting climates may also differentially interact with soil microbes, which may influence plant water and nutrient acquisition. For example, drought tolerant and drought intolerant Pinus edulis associated with distinct ectomycorrhizal fungal communities in the field (Gehring et al., 2017). This suggests that microbial communities can vary by plant population, family, and genotype (Schweitzer et al., 2008; İnceoğlu et al., 2010; Berendsen et al., 2012; Whitham et al., 2012; Lamit et al., 2016) due to differential gene expression in functional traits such as the quantity and quality of root exudates used to shape the soil and root microbiome (Micallef et al., 2009; Mendes et al., 2013; Patel et al., 2015; Bakker et al., 2018). Variation in root exudate quantity and quality can attract beneficial microbes and repel harmful microbes to increase water and nutrient uptake (e.g., root growth) and drought resistance (Mendes et al., 2013; Coskun et al., 2017; Jacoby et al., 2017). One of the nutrients important to plant drought resistance is nitrogen (N) as numerous proteins underlie plant functional responses to stress. RUBISCO is the main protein that determines photosynthetic capacity, and thus contributes to the ability to maintain gas exchange during stress (Field and Mooney, 1986; Evans, 1989). Drought stress can inhibit RUBISCO activity via reductions in ribulose-1,5-bisphosphate (RuBP) (Gimenez et al., 1992) and reductions in the large subunit of RUBISCO (Majumdar et al., 1991). Different soil microbes are responsible for converting organic N into forms that are accessible to plants: either ammonium (NH4+) or nitrate (NO3-) (Hayatsu et al., 2008; Ohyama, 2010; Jacoby et al., 2017). Therefore, within-species populations and families can use root exudates to recruit and repel different soil microbes (Haney et al., 2015; Wille et al., 2019) that cause the plant populations and families to use different forms of N (i.e., NH4+, NO3-). The form of N used by the plant is reflected in leaf N isotope ratios (δ15N) (Kahmen et al., 2008; Temperton et al., 2012). Therefore, within-species variation in functional traits like leaf δ15N, %N, and root exudates suggests that within-species populations and families may differentially interact with soil microbes, altering nutrient acquisition among populations and families.
Intraspecific variation in plant-microbe interactions suggest that soil microbes may differentially affect the performance of plant populations and families under drought by differentially altering functional traits related to water and nutrient uptake and drought resistance. Indeed, the direction and magnitude of the effects of soil microbes on plant performance can vary among within-species groups (O’Brien et al., 2018). For example, during drought, Ostrya virginiana and Betula nigra seedling populations grew more biomass when grown with soil microbes originating from drier sites than when grown with soil microbes from wetter sites (Allsup and Lankau, 2019). This suggests dry-adapted soil microbes may drive greater improvements in plant productivity in populations and families from wetter climates than drier climates. However, this hypothesis has only been tested on plant biomass and needs to be tested on functional traits more directly related to water and nutrient uptake and drought resistance in diverse systems. Extending this research beyond just plant biomass and focusing on functional traits that elucidate the impact of soil microbes on plant physiology improves efforts to engineer beneficial plant-microbe interactions under climate change. Identifying specific plant populations and families that may gain the greatest physiological benefits from soil microbes facilitates seedling adaptation and reforestation efforts.
Loblolly pine (Pinus taeda L.) is the most widely planted and the most economically valuable species in the southern USA (Schultz, 1997). Its geographic distribution spans a wide range of moisture conditions from its driest edge in eastern Texas to its wettest edge on the mid-Atlantic coast, USA (97.5W to 75.0W). Studies have shown that loblolly pine populations and families can vary in functional traits related to drought resistance including growth (Sierra-Lucero et al., 2002) and physiology, where populations and families from drier locations were often, but not always, more drought resistant (Buijtenen et al., 1976; Wells, 1983; Lambeth et al., 1984; but see (Bongarten and Teskey, 1986; Meier et al., 1992; Yang et al., 2002). Mycorrhizal fungi can improve water and nutrient uptake in loblolly pine (Ford et al., 1985; Constable et al., 2001) and plant growth promoting bacteria can have both positive and negative effects on loblolly pine seedling growth (Enebak et al., 1998).
Here, our objective was to determine if and how soil microbes differentially influence functional traits and photosynthetic performance under drought in loblolly pine families from contrasting climates. We grew seeds of loblolly pine families from the driest and wettest ends of its geographic range (dry, wet) and inoculated seeds of both families with a dry-adapted soil microbial community (inoculated). We measured functional traits related to seedling establishment (germination), water and nutrient uptake, and carbon allocation (root:shoot biomass ratio, root exudate concentration, leaf C:N, leaf N isotope composition), and drought resistance (turgor loss point, leaf C isotope composition) before drought. We imposed a drought by completely withholding water and monitored photosynthetic performance using chlorophyll fluorescence. We hypothesized that soil microbes would differentially alter functional traits between the dry and wet families where soil microbes would alter functional traits to enhance water and nutrient uptake and drought resistance to a greater extent in a family originating from a wetter climate than in a family originating from a drier climate.
To test our hypothesis, we used a controlled greenhouse experiment where loblolly pine seedlings from dry and wet climates of origin were grown from seed in sterilized sand and exposed to an inoculation treatment with a soil microbial community from an arid region. Single family, open-pollinated, geographically distinct (Schultz, 1997) loblolly pine seed originating from Bastrop county in Texas (“dry”) and Orangeburg county in South Carolina (“wet”) were provided by the Western Gulf Forest Tree Improvement Program and International Forest Genetics & Seed Company, respectively. These families originated from climates that represent loblolly pine’s wettest and driest ends of its geographic range (Schultz, 1997). Mean annual precipitation of the wet family’s climate of origin was 33% greater than that of the dry family (Table 1; PRISM, 2018).
Table 1 Location and climate information (1960–2017) for the dry and wet families and the drought-adapted soil microbial community used for inoculation treatment.
Inoculated plants were inoculated with a microbial community from a soil sample collected 0–5 cm deep in north central New Mexico, USA (35.5194, −106.2277), a site that receives 67% less mean annual precipitation than the dry family and 75% less mean annual precipitation than the wet family (Table 1). Because we aimed to focus on how seedling families from contrasting climates may differentially interact with any kind of soil microbial community, we selected a soil microbial community outside the contemporary range of loblolly pine. By choosing a soil microbial community to which neither family has been exposed enabled us to isolate contrasting seedling family responses to a novel microbial community, not confounded by previous exposure to such a microbial community. The microbial community was sequenced as in (Ulrich et al., 2019) to determine its bacterial and fungal composition. Briefly, DNA extractions were performed using the DNeasy PowerSoil DNA extraction kit (Qiagen, Hilden, Germany). DNA samples were quantified with an Invitrogen Quant-iTTM ds DNA Assay Kit on a BioTek Synergy HI Hybrid Reader. PCR templates were prepared by diluting an aliquot of each DNA stock in sterile water to 1 ng/μl. The bacterial (and archaeal) 16 S rRNA gene (V3-V4 region) was amplified using primers 515f-806r (Mueller et al., 2016). The fungal 28 S rRNA gene (D2 hypervariable region) was amplified using the LR22R and the reverse LR3. Amplicons were cleaned using a Mobio UltraClean PCR clean-up kit, quantified using the same procedure as for the extracted DNA, and then pooled at a concentration of 10 ng each. A bioanalyzer was used to assess DNA quality, concentration was verified using qPCR, and paired-end 250 bp reads were obtained using an Illumina MiSeq sequencer at the Los Alamos National Laboratory, NM, USA.
To create the soil inoculum for the microbial inoculation treatment, microbes from the soil were extracted by suspension in sterile DI water to create a 1:20 dilution; this isolates the microbial community and reduces potential biogeochemical effects of the original soil. Before planting, stratified seeds (moistened then stored at 35°C for 45 days) were surface sterilized for 10 min in 10% bleach and rinsed for 15 min in sterile DI water twice. Seeds were then soaked in the soil inoculum (inoculated) or sterile DI water (controls) for 10 min. Seeds were each planted in 2.65 L pots (10.2 cm × 10.2 cm × 34.3 cm) of sterilized sand (1 seed per pot) on 23 August 2017 (day 0). Five ml of soil inoculum (inoculated) or sterile DI water (controls) was applied to each pot once during initial planting and also a second time 13 days (5 September 2017) after planting to ensure effects of soil microbial communities (e.g., Corkidi et al., 2002). Both the soil inoculum and water-only treatments each were applied to 15 pots that formed the inoculated and control groups, respectively (N = 15 in each group and family). Clear plastic was placed over all pots to maintain high humidity to promote germination and then was removed after 13 days when most of the plants had germinated. A fertilizer treatment of 5 ml of ammonium nitrate (1 mg/ml) was applied evenly to each pot 19 days after planting (11 September 2017). All plants were well-watered every 2–3 days to field capacity using reverse osmosis (RO) water filtered with a 0.2-µm filter, until drought treatment during which water was completely withheld. Drought was imposed by completely withholding water beginning on day 395 after planting (22 September 2018).
Plants were grown under a 14-h photoperiod in a temperature-controlled greenhouse at the New Mexico Consortium in Los Alamos, New Mexico, USA. Average daytime temperature in the greenhouse was 22.6°C, average nighttime temperature 20.6°C, average daytime relative humidity 47.5%, and average daily maximum photosynthetic photon flux density (PPFD) was 382.4 umol m-2 s-1.
Germination was determined by counting the total number of individuals that germinated per group (inoculated, control) in each family (dry, wet) 14 days after planting (6 September 2017). Shoot height of all 15 individuals per group in each family was measured 287 days after planting (6 June 2018).
To examine family and treatment effects on gas exchange, photosynthesis (A) and stomatal conductance (gs) were measured on four randomly selected individuals per group in each family using a portable photosynthesis system with an infrared gas analyzer (LI-6400 XT, Licor, Lincoln, NE, USA) on day 307 (26 June 2018) between 08h00 and 11h00. In the cuvette, flow rate was set to 500 µmol s-1, reference [CO2] 400 µmol mol-1, quantum flux 2,000 µmol m-2 s-1 to avoid any light limitation of A, and leaf temperature 20°C. Needles in the cuvette (eventually collected for biomass measurements; see below) were scanned using ImageJ image processing software (Schindelin et al., 2012; Schneider et al., 2012) to determine leaf area and normalize gas exchange values by leaf area.
After gas exchange was measured, root exudate concentration was measured in the same four individuals per group in each family used for gas exchange measurements. Total organic carbon (TOC) released by roots (hereafter referred to as root exudates) was collected using methods adapted from (Phillips et al., 2008; Karst et al., 2017; Preece et al., 2018). Loblolly seedlings were carefully dug up to keep roots intact, and roots were dipped in an antimicrobial solution (10,000 units penicillin, 10 mg streptomycin, and 25 μg amphotericin B per ml) to halt microbial activity. Seedlings were then transplanted into pots of glass beads (500-µm diameter). Pots were wrapped in aluminum foil to exclude light and seedlings were allowed to acclimate for 3 days. For root exudate collection, seedlings were first flushed with 150 ml of sterile DI H2O using a vacuum pump. Another 150 ml of sterile DI H2O was added and seedlings were left to release exudates for 24 h. Root exudates were then collected in vials with a vacuum pump. TOC concentration of the collected root exudates was measured using a microplate reader with a UV-visible absorbance detector (Synergy H1 Hybrid Reader, BioTek, Winooski, VT, USA) at a wavelength of 254 nm. Root exudate concentration was determined by converting absorbance (x) to concentration (y) using the linear relationship: y = 6.3259x + 2.5901 (R2 = 0.6). This relationship was determined using 92 liquid TOC samples that had been measured with both UV-visible absorbance and a wet oxidation TOC analyzer (OI Analytical model 1010, Xylem Inc., Rye Brook, NJ, USA) as in (Jones et al., 2014). This method is advantageous because soil water TOC concentrations can be rapidly and cheaply estimated from spectral properties, yet may be limited because variability in concentration within a soil type was observed (Jones et al., 2014). Root exudate concentration values were normalized by dry root biomass. Roots and shoots from the same four plants per group in each family were then harvested, dried, and weighed for root:shoot biomass measurements. While roots were excavated for root exudate collection, we observed that seedlings were not root bound.
To evaluate intraspecific differences in the effect of inoculation treatment on nutrient uptake and drought resistance, dried leaves from the same four plants per group in each family were then analyzed for C and N content (%) and C and N isotope ratios (δ13C, δ. Approximately 0.8 mg of dried needle powder was packed in tin capsules for combustion for subsequent elemental analysis using a stable isotope ratio mass spectrometer (Finnigan MAT253, Thermo Electron Corporation, Waltham, MA, USA) coupled to an elemental analyzer (Costech Analytical Technologies, Inc., Valencia, CA, USA). The δ and15N were recorded as deviations per thousand (‰) and were calibrated using International Atomic Energy Agency (IAEA) standards C3, C6, C8, N1, and N2. The δ13C of leaf tissue (δ13Cleaf) reflects the δ13C of CO2 in the atmosphere (δ13Cair), the fractionation against the heavier carbon isotope (13C) due to physiological processes and the ratio of the concentration of CO2 inside the leaf (ci) to that in the ambient air (ca):
Before drought treatment, we measured leaf drought tolerance using the water potential at turgor loss or turgor loss point (ΨTLP; Bartlett et al., 2014) on four new individuals randomly selected per group (inoculated, control) in each family (dry, wet; 16 curves total) not used for the aforementioned measurements. ΨTLP was determined from pressure-volume (P-V) curves as in (Meinzer et al., 2014). P-V curves plot leaf water potential in response to changes in water volume as leaves dry and are used to determine bulk leaf parameters related to leaf cellular composition and structural properties such as ΨTLP. Species with lower (more negative) ΨTLP are more tolerant of drought because they are able to maintain turgor and function under more negative soil water potentials (Bucci et al., 2004; Blackman et al., 2010). P-V curves were measured over five days (days 338-343, 27 July–1 August 2018). Seedlings were cut at predawn and were then placed in beakers of water to rehydrate for 2-3 hours. No rehydration-induced plateau was detected (Kubiske and Abrams, 1991). Shoots were allowed to dry out slowly on the laboratory bench. Measurements of shoot mass taken with a balance, and water potential taken with a pressure chamber (PMS Instruments, Albany, OR, USA) were recorded as shoots dried out. Data were plotted with relative water deficit on the x-axis and 1/Ψ on the y-axis. Data were checked during measurement to ensure at least 3–5 points were recorded along the linear portion of the curve. ΨTLP was estimated from the intersection of the linear portion of the curve with a negative exponential function fitted to the nonlinear portion as in (Meinzer et al., 2014).
To compare the effects of drought on inoculated and control groups in both families, drought was imposed by completely withholding water beginning on day 395 after planting (22 September 2018). To detect family and treatment differences in drought effects on seedling physiology related to photosynthetic performance, dark-adapted chlorophyll fluorescence was measured on the remaining seven individuals per group in each family the day before drought began and then weekly until photosynthetic performance declined to zero. Chlorophyll fluorescence measurements were made on mature, fully expanded needles at ambient temperature using a field portable pulse-modulated chlorophyll fluorometer (FMS2, Hansatech, Norfolk, UK) at predawn to ensure plants were dark-adapted. Chlorophyll fluorescence was measured as the ratio of variable to maximum fluorescence (FV/FM) in the convention of Maxwell and Johnson (2000). FV/FM measures the maximum quantum efficiency of PSII photochemistry (Genty et al., 1989) and is calculated as:
A two-way ANOVA was used to evaluate the significance of the main effects of family and treatment on functional traits (i.e. response variables): germination, height, root:shoot biomass ratio, root exudates, turgor loss point, leaf %C, %N and C:N, leaf δ13C and δ15N, photosynthesis (A), and stomatal conductance (gs). Assumptions of equal variance and normality were checked using residual and quantile-quantile plots. Root:shoot biomass was log-transformed to meet the normality assumption. Tukey’s posthoc test was used to identify statistically significant differences in means. To compare differences in inoculation treatment effects between families, we determined the treatment effect size and the 95% confidence interval on the aforementioned functional traits for both the dry and wet families. Effect size was calculated as the mean difference in functional trait between the control and inoculated groups divided by the pooled standard deviation within each family. We corrected the effect size for bias using the standard correction that accounts for small sample averages according to (Hedges et al., 1985; Lakens, 2013; Hedges and Olkin, 2014). Effect size was considered to be significant if the 95% confidence interval did not overlap with an effect size of 0. Effect size was considered to be moderately significant if the 95% confidence interval overlapped an effect size of 0 by less than ± 0.1. A positive effect size indicates an increase in the functional trait.
A linear mixed effects model was used to determine differences in FV/FM between inoculated and control groups through time during the drought treatment. Fixed effects were treatment (inoculated, control), family (dry, wet), and week and the random effect was individual. A linear mixed effects model, as opposed to the two-way ANOVA, enables us to account for repeated measurements through time by fitting models with different correlation structures. The model of best fit was selected based on Akaike information criterion (AIC) values. Assumptions of constant variance and normality were checked using residual and quantile-quantile plots. All interactive and main effects of factors on the response were tested using marginal F-tests (also known as type III tests). Post-hoc comparisons were made using a 95% confidence interval and P ≤ 0.05. All statistical analyses were conducted in R version 3.4.2 (R Core Team, C. T., 2018). The linear mixed effects model was conducted using the nlme and gmodels R packages (Warnes et al., 2018; Pinheiro et al., 2019).
The microbial inoculation treatment affected functional traits of both families similarly for the majority of traits we measured (germination, height, ΨTLP, root exudate concentration, %C, δ13 C, photosynthesis (A), and stomatal conductance (gs)) but the effect size was only significant for one trait: germination (Figure 1, Supplementary Table 1), where inoculated plants exhibited significantly greater germination than controls regardless of family (ANOVA; P < 0.001; Figures 1 and 2A, Supplementary Table 2). In contrast, the inoculation treatment affected the remaining traits (root:shoot biomass ratio, leaf δ15N, %N, C:N) of the dry and wet families in opposite directions but the effect size was only significant or moderately significant for two traits: root:shoot biomass ratio and leaf δ15N (Figure 1, Supplementary Table 1). The inoculation treatment significantly increased the root:shoot biomass ratio in the wet family but not the dry family, as indicated by only the wet family’s significant, positive effect size of 1.7. The inoculation treatment increased leaf δ15N in the dry family but not the wet family, as indicated by only the dry family’s moderately significant, positive effect size of 1.5 (i.e., 95% CI = −0.05 to 3.1, Supplementary Table 1). Despite these significant and moderately significant effect sizes on root:shoot biomass ratio and leaf δ15N, the ANOVA did not result in significant effects of treatment or the interaction on root:shoot biomass ratio and leaf δ15N in either family (ANOVA; P = 0.78, 0.12; P = 0.30, 0.23; Figures 2C, D, Supplementary Table 2), possibly due to sample size (N = 4).
Figure 1 Effect size of inoculation treatment on physiological measurements in dry and wet families (germination, root:shoot biomass ratio, leaf N isotope ratio (δ15N), leaf N content (%N), leaf C:N ratio (C:N), height, turgor loss point (ΨTLP), root exudate concentration, leaf C content (%C), leaf C isotope ratio (δ13C), photosynthesis (A), stomatal conductance (gs)). A positive effect size indicates an increase in the physiological measurement. Bars represent 95% confident intervals. Effect sizes were considered significant if the 95% confidence intervals did not overlap with an effect size of zero. N = 4. Seedlings were ~11 months old for all measurements except germination (14 days old) and height (~9 months old).
Figure 2 Mean number of individuals germinated (A), shoot height (B), root:shoot biomass ratio (root:shoot; C), leaf N isotope ratios (δ15N; D), and root exudate concentration (E) of control and inoculated groups in dry and wet families. Letters indicate statistically significant differences among the four groups at P ≤ 0.05. Error bars represent ± SE. N = 4. Seedlings were ~11 months old for all measurements except germination (14 days old) and height (~9 months old).
Despite the variation in the direction and magnitude of treatment effect size on measured functional traits between plant families, the dry family exhibited functional traits indicative of a greater capacity to withstand drought than the wet family regardless of treatment. Regardless of treatment, the dry family exhibited a significantly greater root:shoot biomass ratio (P = 0.047; Figure 2C), was significantly shorter (P = 0.030, Figure 2B), exhibited significantly greater leaf δ15N (P < 0.001, Figure 2D), and released a greater root exudate concentration compared to the wet family (P = 0.031, Figure 2E).
Leaf δ13C, %C, %N, C:N, A, gs, and ΨTLP were not significantly influenced by treatment, family, or the interaction between treatment and family (P > 0.05; Table 2, Supplementary Table 2). Germination was not affected by family or the interaction between treatment and family (P = 0.27, 0.55, respectively; Figure 2A, Supplementary Table 2). Height was not significantly affected by treatment or the interaction (P = 0.35, 0.51, respectively; Figure 2B, Supplementary Table 2). Root exudate concentration was not affected by treatment or the interaction (P = 0.19, 0.080, respectively; Figure 2E, Supplementary Table 2). In addition to ΨTLP, other parameters derived from pressure-volume curves were not significantly influenced by treatment, family, or the interaction between treatment and family (P > 0.05; Supplementary Table 3). Representative pressure-volume curves for each family and treatment are included in Supplementary Figure 1.
Table 2 Physiological measurements of control and inoculated groups in dry and wet families (leaf C content, N content, C:N ratio, C isotope ratio (δ13C), photosynthesis (A), stomatal conductance (gs), turgor loss point (ΨTLP)).
The effect of the inoculation treatment on the time course of photosynthetic performance during drought as measured with FV/FM differed between dry and wet families. During drought at week 3, FV/FM of the inoculated group declined the fastest and was significantly lower than that of controls in the dry family (P < 0.05), but not the wet family (Figure 3). No statistically significant differences in FV/FM existed between groups before drought or at other weeks (P > 0.05). Model selection parameters for FV/FM are contained in Supplementary Table 4.
Figure 3 FV/FM time courses of control and inoculated groups in dry (A) and wet (B) families measured weekly before and throughout drought. Drought was imposed after week 0 (when seedlings were ~13 months old). Error bars represent ± SE. At week 3, letters indicate statistically significant differences among the four groups at P ≤ 0.05. No significant differences among groups were detected at other time points. N = 7.
The taxonomic profile of the soil microbial community used for the inoculation treatment is presented in Supplementary Tables 1 and 2.
Consistent with our hypothesis, we found evidence that soil microbes can alter functional traits such as root:shoot biomass ratio that improve water and nutrient uptake and drought resistance more so in the wet family than in the dry family. The taxonomic profile of the soil microbial community used for the inoculation treatment contained drought-adapted bacteria (Supplementary Table 5), where the majority (60%) of the top ten most abundant fungal taxa was identified as Pleosporales in Ascomycota, which is dominant in arid soils (Porras-Alfaro et al., 2008; Porras-Alfaro et al., 2011). This supports its potential to benefit the wet family more than the dry family (Allsup and Lankau, 2019). The wet family’s significant and positive treatment effect size on root:shoot biomass ratio and the dry family nonsignificant effect size on root:shoot biomass ratio suggest that the inoculated group in the wet family allocated more resources belowground to increase root growth, which enhances plant water and nutrient acquisition, drought resistance, and the capacity to withstand drought (Brunner et al., 2015; Hagedorn et al., 2016). The inoculation treatment also differentially altered leaf %N, C:N, and δ15N between families where the effect sizes were opposite in sign between families.
Similar to root:shoot biomass ratio, FV/FM was also differentially affected by the inoculation treatment between families. FV/FM of the inoculated group declined faster than controls during the imposed drought only in the dry family and not the wet. One proposed mechanism underlying this observation is that the slightly greater height of the dry inoculated group compared to dry control group (albeit not significant) resulted in increased leaf area which may have contributed to faster desiccation and functional decline during drought. Another possible mechanism underlying the dry inoculated group’s FV/FM decline may be related to the opposite (albeit not significant) effect sizes between families for %N and consequently C:N. In the wet family, we observed a positive treatment effect size on leaf %N and a negative effect size on C:N, while in contrast, the dry family exhibited the opposite: a negative effect size on leaf %N and a positive effect size on C:N. Increased C:N, as we observed in the dry inoculated group, can indicate greater drought stress (Chen et al., 2015; Ma et al., 2016). This may possibly be due to a negative interaction between the soil microbes and the dry family. (Enebak et al., 1998) also observed a negative effect of plant growth-promoting bacteria on loblolly pine seedling growth.
In addition to leaf %N and C:N, the family difference in inoculation treatment effect on FV/FM may also be related to family differences in inoculation treatment effects on N uptake (e.g. NO3-, NH4+), as indicated by the moderately significant, positive treatment effect size on leaf δ15N in the dry family and the nonsignificant effect size in the wet family. Leaf δ15N reflects the 15N abundance of N sources available to plants (Shearer and Kohl, 1986). Our leaf δ15N results suggest that soil microbes may influence N uptake in the dry family differently than the wet family. This may occur because within-species families can interact differently with soil microbes by altering root exudate composition and quantity to recruit and repel different microbes (Haney et al., 2015). Because different soil microbes are responsible for transforming organic N to plant-accessible NH4+ or NO3- (Hayatsu et al., 2008), the form of plant available N and thus leaf δ15N can differ between within-species families. The family differences in inoculation treatment effect sizes on leaf δ15N resulted in the dry inoculated group exhibiting the greatest leaf δ15N, which may indicate higher uptake of NH4+ (Miller and Bowman, 2002; Falkengren-Grerup et al., 2004), the primary form of N for loblolly (Lucash et al., 2008) and coniferous systems (Alexander, 1983). At NH4+ levels of 0.1 to 0.5 mmol/L, NH4+can be toxic to plants but the level at which it becomes toxic varies among species (Britto and Kronzucker, 2002). Therefore, it is possible that this could have contributed to the dry inoculated group’s decline in FV/FM during drought at week 3 if the microbes in the inoculation treatment transformed N into relatively more NH4+ in the dry family compared to in the wet family. However, we present this only as a possibility because we cannot determine the quantity of NH4+ present given only the leaf δ15N data in this study. The low FV/FM values of ~0.6 before drought suggest that seedlings may have been nutrient-deficient because they were grown in pure, fast-draining sand.
The taxonomic profile of the soil microbial community used for the inoculation treatment supports the possibility that the dry inoculated group’s FV/FM decline may have been related to microbial effects on plant N availability due to the presence of fungi involved in plant N acquisition (Supplementary Table 6), which may have interacted with each seedling family differently. The majority of the top most abundant fungi was in Pleosporales, which contains dark septate fungi that can colonize Pinus species and are found in nutrient-stressed environments (Barrow, 2003), such as in our experiment using fast-draining sand. Dark septate fungi can form mutualistic relationships with plants by obtaining C from the plant in return for making nutrients available to the plant (Usuki and Narisawa, 2007; Wagg et al., 2008; Vergara et al., 2018), specifically transforming organic N into plant available forms (Alberton et al., 2010; Newsham, 2011; Bueno de Mesquita et al., 2018). The most abundant fungal genus in the soil microbial community used for the inoculation treatment was Alternaria of Pleosporales, which has been shown to vary with soil N availability (Porras-Alfaro et al., 2011). Given the presence of fungi involved in plant N acquisition, it is possible that the dry family’s significantly greater concentration of root exudates may have differentially affected the microbial community and resulted in different N types available compared to that of the wet family. This may have contributed to the observed variation in treatment effect size on leaf %N, C:N, and δ15N between families. Additionally, contrary to our hypothesis, the soil microbe treatment did not always influence functional traits that increased water and nutrient uptake and drought resistance (e.g. root:shoot biomass ratio) more in the wet family than in the dry family. This suggests that significant effects of soil microbes on plant function can vary depending on the trait of interest (Rincón et al., 2008). Regardless of family, inoculated plants exhibited significantly greater germination than controls, suggesting that soil microbes may improve seedling establishment regardless of family by directly or indirectly enhancing seed germination (Madhaiyan et al., 2005; Balshor et al., 2016). Soil microbes can directly enhance germination via the production of plant growth hormones (Wu et al., 2016), and also indirectly by increasing the soil water holding capacity of soil (Roberson and Firestone, 1992; Kaci et al., 2005; Or et al., 2007; Ulrich et al., 2019) and maintaining high soil moisture required for germination (Schultz, 1997), as well as increasing nutrient acquisition.
The taxonomic profile of the soil microbial community used for the inoculation treatment reflects its arid environment of origin and indicates the presence of fungi involved in plant N acquisition (Supplementary Tables 5 and 6). The majority (60%) of the top ten most abundant bacterial taxa was composed of Rubrobacterales in Actinobacteria, known to be thermophilic, radiation-resistant, and common in arid, desert soils (Holmes et al., 2000).
The dry family’s significantly greater root:shoot biomass ratio and root exudate concentration than that of the wet family suggests that the dry family may be more drought-adapted than the wet family. The dry family’s significantly greater root:shoot biomass ratio and concentration of root exudates supports its greater capacity to resist drought and reflects its drier climate of origin compared to the wet family (regardless of treatment). This greater belowground allocation of resources to root biomass enables the dry family to access deep water sources during limited water availability, a trait observed in families and populations adapted to drier climates (Brunner et al., 2015; Hagedorn et al., 2016). Greater allocation to root growth also enhances nutrient acquisition (López-Bucio et al., 2003; Hodge, 2004; Maire et al., 2009; Chapman et al., 2012), which can vary among loblolly pine families and populations (Li et al., 1991). The dry family’s greater allocation to belowground growth may also underlie why we observed a significant treatment effect size on root:shoot biomass in only the wet family, as the dry family was already allocating more resources belowground independent of treatment. The dry family was also significantly shorter than the wet family, reducing leaf area through which water can be lost, another mechanism of drought resistance.
The dry family’s significantly greater concentration of root exudates compared to the wet family may be used to shape the soil microbial community by attracting or repelling different microbes (Bever et al., 2012; Sasse et al., 2018; Zhalnina et al., 2018), another strategy to increase water and nutrient uptake, enhance drought resistance (Badri and Vivanco, 2009; Lareen et al., 2016; Jacoby et al., 2017), and alter the water holding capacity of the soil (Young, 1995; Angers and Caron, 1998; Walker et al., 2003). Families adapted to drier or wetter climates can differ in their root exudation quantity and quality (Whitham et al., 2003) because root exudate quantity can increase during drought (Liese et al., 2018; Preece et al., 2018) and root exudate composition can also change as a result of dry conditions (Gargallo-Garriga et al., 2018). Furthermore, root exudate rates and composition have been shown to vary across genotypes (Cieslinski et al., 1997; Hoekenga et al., 2003; Badri and Vivanco, 2009) and influence the genotype-specific ability to recruit beneficial soil microbes (Haney et al., 2015).
The dry and wet families may have used different sources of plant available N (e.g. NO3-, NH4+), as suggested by the dry family’s significantly greater leaf δ15N than the wet family regardless of treatment. N use is driven by the quantity of N forms available in the soil and the capacity of plants to take up different N forms (Vasquez et al., 2008). Within-species pine populations and families can differ in their use of NH4+ or NO3- (Miller and Hawkins, 2007; Maire et al., 2009) because plants can switch their N source (Louahlia et al., 2000) depending on precipitation (Houlton et al., 2007) and season (Lucash et al., 2008), suggesting that plasticity exists in the uptake of different N sources between within-species populations and families from contrasting climates. Plasticity in N use can be driven by within-species variation in root morphology, preferential expression of N transporters (NO3- versus NH4+) (Maire et al., 2009), and/or activity patterns of soil enzymes involved in the acquisition of N (Purahong et al., 2016). However, it remains unclear if the relationship between shoot δ15N and N source is universal (Kahmen et al., 2008; Temperton et al., 2012) because the δ15N of NO3- and NH4+ can vary substantially depending on relative mineralization, nitrification, and denitrification (Shearer et al., 1974; Mariotti et al., 1981; Handley et al., 1999).
The family differences in N use may be related to the family differences in root exudates because the dry family both released greater root exudates and exhibited greater leaf δ15N than the wet family. Root exudates can alter the availability of plant available N sources by stimulating microbial growth and activity (Marschner, 2012). An increase in microbial activity can increase microbial N transformations and influence the quantity and type of plant available N (Yin et al., 2013). Root exudates may also affect the N cycling function of the soil microbial community by attracting or repelling specific microbes that may promote the plant availability of NO3- or NH4+ and alter the NO3-:NH4+ ratio (Hayatsu et al., 2008). Root exudates can also alter N availability by influencing different steps of the N cycle such as inhibiting nitrification (Subbarao et al., 2006; Coskun et al., 2017).
Despite significant family differences in root:shoot biomass, root exudates, and δ15N, we did not observe significant family or treatment differences in functional traits related to leaf drought tolerance, intrinsic water use efficiency, and stomatal constraints on gas exchange as indicated by ΨTLP, δ13C, A, and gs, respectively. This suggests that ΨTLP, a metric of drought tolerance (Bartlett et al., 2014), can reflect the conditions under which plant tissues develop and can outweigh ecotypic differences between families and populations. Meier et al. (1992) also did not find significant differences in ΨTLP between P. taeda families and populations from contrasting climates. For leaf δ13C, a metric of intrinsic water use efficiency (Farquhar et al., 1989), some studies of conifer species have observed intraspecific variation in leaf δ13C reflecting climate of origin (Yang et al., 2002; Kerr et al., 2015; Marias et al., 2017) while others have not (Zhang et al., 1993; Zhang et al., 1997). For A, and gs, others also have not observed significant differences in gas exchange parameters among within-species loblolly pine families and populations (Yang et al., 2002; Aspinwall et al., 2011). Given these mixed results, future provenance studies have been urged to focus on growth and survival traits rather than δ13C as a proxy for drought resistance (Moran et al., 2017).
Our results showed that soil microbial inoculation treatment effects varied in direction and magnitude by trait and family. Soil microbes may alter functional traits that improve water and nutrient uptake and drought resistance such as the root:shoot biomass ratio more so in a family originating from a wetter climate than in a family originating from a drier climate. Regardless of treatment, the dry family exhibited a greater root:shoot biomass ratio, root exudate concentration, and leaf δ15N than the wet family. This suggests that the dry family allocated more resources belowground than the wet, and that within-species families may have used different sources of plant available N, which may be related to their climate and soil of origin. Together, this work highlights the need to further investigate in diverse systems how abiotic factors like drought and biotic factors like soil microbes influence diverse functional traits that influence seedling establishment of families and populations from contrasting climates. Given the mix of positive and negative microbial treatment effects on the dry family (e.g. increased germination but reduced FV/FM), more research is needed to inform plant-microbe interactions by identifying potential physiological tradeoffs due to negative interactions with soil microbes. Examination of intraspecific variation in plant physiological impacts of soil microbes informs species’ distributions, improves efforts to engineer beneficial plant-microbe interactions, and facilitates seedling adaptation and reforestation under future climate regimes.
The datasets generated for this study can be found in the Supplementary Information of this article.
DU, SS, and JD conceived and designed the study. DU, MR, and SP set up the experiment and made all measurements. DU conducted data analysis and wrote the first draft of the manuscript. All authors revised and approved of the final version of the manuscript.
This research was funded by the Los Alamos National Laboratory Climate Space and Earth Science Chick Keller Postdoctoral Fellowship, the Los Alamos National Laboratory Directed Research and Development project #20160373ER, and the U.S. Department of Energy Biological System Science Division Science Focus Area Grant (2015SFAF260 and 2019SFAF255).
The authors declare that the research was conducted in the absence of any commercial or financial relationships that could be construed as a potential conflict of interest.
We would like to thank the New Mexico Consortium for greenhouse assistance, the Los Alamos National Laboratory Climate Space and Earth Science Chick Keller Postdoctoral Fellowship, the Los Alamos National Laboratory Directed Research and Development project #20160373ER, the U.S. Department of Energy Biological System Science Division Science Focus Area Grant (2015SFAF260 and 2019SFAF255), and Fred Raley of the Western Gulf Forest Tree Improvement Program and Nick Muir of the International Forest and Genetics Company for donating the loblolly pine seeds.
The Supplementary Material for this article can be found online at: https://www.frontiersin.org/articles/10.3389/fpls.2019.01643/full#supplementary-material
Alberton, O., Kuyper, T. W., Summerbell, R. C. (2010). Dark septate root endophytic fungi increase growth of Scots pine seedlings under elevated CO2 through enhanced nitrogen use efficiency. Plant Soil 328, 459–470. doi: 10.1007/s11104-009-0125-8
Alexander, I. (1983). “The significance of ectomycorrhizas in the nitrogen cycle,” in Nitrogen as an Ecological Factor. Eds. Lee, J. A., McNeill, S., Rorison, I. H., (Oxford: Blackwell Scientific Publications), 69–93.
Allen, C. D., Macalady, A. K., Chenchouni, H., Bachelet, D., McDowell, N., Vennetier, M., et al. (2010). A global overview of drought and heat-induced tree mortality reveals emerging climate change risks for forests. For. Ecol. Manage. 259, 660–684. doi: 10.1016/j.foreco.2009.09.001
Allison, S. D., Martiny, J. B. H. (2008). Resistance, resilience, and redundancy in microbial communities. Proc. Natl. Acad. Sci. 105, 11512–11519. doi: 10.1073/pnas.0801925105
Allsup, C., Lankau, R. (2019). Migration of soil microbes may promote tree seedling tolerance to drying conditions. Ecology 0, e02729. doi: 10.1002/ecy2729
Angers, D. A., Caron, J. (1998). “Plant-induced changes in soil structure: Processes and feedbacks,” in Plant-induced soil changes: Processes and feedbacks Developments in Biogeochemistry (Dordrecht: Springer), 55–72. doi: 10.1007/978-94-017-2691-7_3
Aspinwall, M. J., King, J. S., McKeand, S. E., Domec, J.-C. (2011). Leaf-level gas-exchange uniformity and photosynthetic capacity among loblolly pine (Pinus taeda L.) genotypes of contrasting inherent genetic variation. Tree Physiol. 31, 78–91. doi: 10.1093/treephys/tpq107
Badri, D. V., Vivanco, J. M. (2009). Regulation and function of root exudates. Plant Cell Environ. 32, 666–681. doi: 10.1111/j.1365-3040.2009.01926.x
Bais, H. P., Weir, T. L., Perry, L. G., Gilroy, S., Vivanco, J. M. (2006). The role of root exudates in rhizosphere interactions with plants and other organisms. Annu. Rev. Plant Biol. 57, 233–266. doi: 10.1146/annurev.arplant.57.032905.105159
Bakker, P. A. H. M., Pieterse, C. M. J., de Jonge, R., Berendsen, R. L. (2018). The soil-borne legacy. Cell 172, 1178–1180. doi: 10.1016/j.cell.2018.02.024
Baldrian, P. (2017). Forest microbiome: diversity, complexity and dynamics. FEMS Microbiol. Rev. 41, 109–130. doi: 10.1093/femsre/fuw040
Balshor, B. J., Garrambone, M. S., Austin, P., Balazs, K. R., Weihe, C., Martiny, J. B. H., et al. (2016). The effect of soil inoculants on seed germination of native and invasive species. Botany 95, 469–480. doi: 10.1139/cjb-2016-0248
Barrow, J. R. (2003). Atypical morphology of dark septate fungal root endophytes of Bouteloua in arid southwestern USA rangelands. Mycorrhiza 13, 239–247. doi: 10.1007/s00572-003-0222-0
Bartlett, M. K., Zhang, Y., Kreidler, N., Sun, S., Ardy, R., Cao, K., et al. (2014). Global analysis of plasticity in turgor loss point, a key drought tolerance trait. Ecol. Lett. 17, 1580–1590. doi: 10.1111/ele.12374
Bent, E., Tuzun, S., Chanway, C. P., Enebak, S. (2001). Alterations in plant growth and in root hormone levels of lodgepole pines inoculated with rhizobacteria. Can. J. Microbiol. 47, 793–800. doi: 10.1139/w01-080
Berendsen, R. L., Pieterse, C. M. J., Bakker, P. A. H. M. (2012). The rhizosphere microbiome and plant health. Trends Plant Sci. 17, 478–486. doi: 10.1016/j.tplants.2012.04.001
Bever, J. D., Platt, T. G., Morton, E. R. (2012). Microbial population and community dynamics on plant roots and their feedbacks on plant communities. Annu. Rev. Microbiol. 66, 265–283. doi: 10.1146/annurev-micro-092611-150107
Björkman, O., Demmig, B. (1987). Photon yield of O2 evolution and chlorophyll fluorescence characteristics at 77 K among vascular plants of diverse origins. Planta 170, 489–504. doi: 10.1007/BF00402983
Blackman, C. J., Brodribb, T. J., Jordan, G. J. (2010). Leaf hydraulic vulnerability is related to conduit dimensions and drought resistance across a diverse range of woody angiosperms. New Phytol. 188, 1113–1123. doi: 10.1111/j.1469-8137.2010.03439.x
Bongarten, B. C., Teskey, R. O. (1986). Water relations of loblolly pine seedlings from diverse geographic origins. Tree Physiol. 1, 265–276. doi: 10.1093/treephys/1.3.265
Britto, D. T., Kronzucker, H. J. (2002). NH4+ toxicity in higher plants: a critical review. J. Plant Physiol. 159, 567–584. doi: 10.1078/0176-1617-0774
Brunner, I., Herzog, C., Dawes, M. A., Arend, M., Sperisen, C. (2015). How tree roots respond to drought. Front. Plant Sci. 6, 547. doi: 10.3389/fpls.2015.00547
Bucci, S. J., Goldstein, G., Meinzer, F. C., Scholz, F. G., Franco, A. C., Bustamante, M. (2004). Functional convergence in hydraulic architecture and water relations of tropical savanna trees: from leaf to whole plant. Tree Physiol. 24, 891–899. doi: 10.1093/treephys/24.8.891
Bueno de Mesquita, C. P., Sartwell, S. A., Ordemann, E. V., Porazinska, D. L., Farrer, E. C., King, A. J., et al. (2018). Patterns of root colonization by arbuscular mycorrhizal fungi and dark septate endophytes across a mostly-unvegetated, high-elevation landscape. Fungal Ecol. 36, 63–74. doi: 10.1016/j.funeco.2018.07.009
Buijtenen, J. P., van, Bilan, M. V., Zimmerman, R. H. (1976). Morpho physiological characteristics, related to drought resistance in Pinus taeda. Tree Physiol. Yield Improv. 349–359.
Carvalho, A., Pavia, I., Fernandes, C., Pires, J., Correia, C., Bacelar, E., et al. (2017). Differential physiological and genetic responses of five European Scots pine provenances to induced water stress. J. Plant Physiol. 215, 100–109. doi: 10.1016/j.jplph.2017.05.027
Cernusak, L. A., Winter, K., Turner, B. L. (2009). Plant δ15N correlates with the transpiration efficiency of nitrogen acquisition in tropical trees. Plant Physiol. 151, 1667–1676. doi: 10.1104/pp.109.145870
Chapman, N., Miller, A. J., Lindsey, K., Whalley, W. R. (2012). Roots, water, and nutrient acquisition: let’s get physical. Trends Plant Sci. 17, 701–710. doi: 10.1016/j.tplants.2012.08.001
Chauvin, T., Cochard, H., Segura, V., Rozenberg, P. (2019). Native-source climate determines the Douglas-fir potential of adaptation to drought. For. Ecol. Manage. 444, 9–20. doi: 10.1016/j.foreco.2019.03.054
Chen, D., Wang, S., Xiong, B., Cao, B., Deng, X. (2015). Carbon/nitrogen imbalance associated with drought-induced leaf senescence in sorghum bicolor. PloS One 10, e0137026. doi: 10.1371/journal.pone.0137026
Chen, D., Wang, S., Cao, B., Cao, D., Leng, G., Li, H., et al. (2016). Genotypic variation in growth and physiological response to drought stress and re-watering reveals the critical role of recovery in drought adaptation in maize seedlings. Front. Plant Sci. 6. doi: 10.3389/fpls.2015.01241
Cieslinski, G., Rees, K. C. J. V., Szmigielska, A. M., Huang, P. M. (1997). Low molecular weight organic acids released from roots of durum wheat and flax into sterile nutrient solutions. J. Plant Nutr. 20, 753–764. doi: 10.1080/01904169709365291
Compant, S., van der Heijden, M. G. A., Sessitsch, A. (2010). Climate change effects on beneficial plant-microorganism interactions. FEMS Microbiol. Ecol. 73, 197–214. doi: 10.1111/j.1574-6941.2010.00900.x
Comstock, J. P. (2001). Steady-state isotopic fractionation in branched pathways using plant uptake of NO3- as an example. Planta 214, 220–234. doi: 10.1007/s004250100602
Constable, J. V. H., Bassirirad, H., Lussenhop, J., Zerihun, A. (2001). Influence of elevated CO2 and mycorrhizae on nitrogen acquisition: contrasting responses in Pinus taeda and Liquidambar styraciflua. Tree Physiol. 21, 83–91. doi: 10.1093/treephys/21.2-3.83
Corkidi, L., Rowland, D. L., Johnson, N. C., Allen, E. B. (2002). Nitrogen fertilization alters the functioning of arbuscular mycorrhizas at two semiarid grasslands. Plant Soil 240, 299–310. doi: 10.1023/A:1015792204633
Coskun, D., Britto, D. T., Shi, W., Kronzucker, H. J. (2017). How plant root exudates shape the nitrogen cycle. Trends Plant Sci. 22, 661–673. doi: 10.1016/j.tplants.2017.05.004
Cregg, B. M., Zhang, J. W. (2001). Physiology and morphology of Pinus sylvestris seedlings from diverse sources under cyclic drought stress. For. Ecol. Manage. 1–2, 131–139. doi: 10.1016/S0378-1127(00)00626-5
Davis, F. W., Sweet, L. C., Serra-Diaz, J. M., Franklin, J., McCullough, I., Flint, A., et al. (2016). Shrinking windows of opportunity for oak seedling establishment in southern California mountains. Ecosphere 7, e01573. doi: 10.1002/ecs21573
de Zelicourt, A., Al-Yousif, M., Hirt, H. (2013). Rhizosphere microbes as essential partners for plant stress tolerance. Mol. Plant 6, 242–245. doi: 10.1093/mp/sst028
Dimkpa, C., Weinand, T., Asch, F. (2009). Plant-rhizobacteria interactions alleviate abiotic stress conditions. Plant Cell Environ. 32, 1682–1694. doi: 10.1111/j.1365-3040.2009.02028.x
Enebak, S. A., Wei, G., Kloepper, J. W. (1998). Effects of plant growth-promoting rhizobacteria on loblolly and slash pine seedlings. For. Sci. 44, 139–144. doi: 10.1093/forestscience/44.1.139
Evans, J. R. (1989). Photosynthesis and nitrogen relationships in leaves of C3 plants. Oecologia 78, 9–19. doi: 10.1007/BF00377192
Falkengren-Grerup, U., Michelsen, A., Olsson, M. O., Quarmby, C., Sleep, D. (2004). Plant nitrate use in deciduous woodland: the relationship between leaf N, 15N natural abundance of forbs and soil N mineralisation. Soil Biol. Biochem. 36, 1885–1891. doi: 10.1016/j.soilbio.2004.05.009
Farquhar, G., Richards, R. (1984). Isotopic composition of plant carbon correlates with water-use efficiency of wheat genotypes. Funct. Plant Biol. 11, 539–552. doi: 10.1071/PP9840539
Farquhar, G., O’Leary, M., Berry, J. (1982). On the relationship between carbon isotope discrimination and the intercellular carbon dioxide concentration in leaves. Funct. Plant Biol. 9, 121–137.
Farquhar, G. D., Hubick, K. T., Condon, A. G., Richards, R. A. (1989). “Carbon Isotope Fractionation and Plant Water-Use Efficiency,” in Stable Isotopes in Ecological Research Ecological Studies. Eds. Rundel, P. W., Ehleringer, J. R., Nagy, K. A. (New York: Springer), 21–40. doi: 10.1007/978-1-4612-3498-2_2
Fernández, M., Gil, L., Pardos, J. A. (1999). Response of Pinus pinaster Ait. provenances at early age to water supply. I. water relation parameters. Ann. For. Sci. 56, 179–187. doi: 10.1051/forest:19990209
Field, C., Mooney, H. (1986). “The photosynthesis-nitrogen relationship in wild plants,” in On the economy of form and function. Ed. Givnish, T. J. (Cambridge, UK: Cambridge Univ. Press), 25–55.Photosynth.-Nitrogen Relatsh. Wild Plants P 25–55 TJ Givnish Ed Econ. Form Funct. Camb. Univ Press Camb. UK. .
Finkel, O. M., Castrillo, G., Herrera Paredes, S., Salas González, I., Dangl, J. L. (2017). Understanding and exploiting plant beneficial microbes. Curr. Opin. Plant Biol. 38, 155–163. doi: 10.1016/j.pbi.2017.04.018
Ford, V. L., Torbert, J. L., Burger, J. A., Miller, O. K. (1985). Comparative effects of four mycorrhizal fungi on loblolly pine seedlings growing in a greenhouse in a Piedmont soil. Plant Soil 83, 215–221. doi: 10.1007/BF02184293
García de la Serrana, R., Vilagrosa, A., Alloza, J. A. (2015). Pine mortality in southeast Spain after an extreme dry and warm year: interactions among drought stress, carbohydrates and bark beetle attack. Trees 29, 1791–1804. doi: 10.1007/s00468-015-1261-9
Gargallo-Garriga, A., Preece, C., Sardans, J., Oravec, M., Urban, O., Peñuelas, J. (2018). Root exudate metabolomes change under drought and show limited capacity for recovery. Sci. Rep. 8, 12696. doi: 10.1038/s41598-018-30150-0
Gehring, C. A., Sthultz, C. M., Flores-Rentería, L., Whipple, A. V., Whitham, T. G. (2017). Tree genetics defines fungal partner communities that may confer drought tolerance. Proc. Natl. Acad. Sci. 114, 11169–11174. doi: 10.1073/pnas.1704022114
Genty, B., Briantais, J.-M., Baker, N. R. (1989). The relationship between quantum yield of photosynthetic electron transport and quenching of chlorophyll fluorescence. Biochim. Biophys. Acta 990, 87–92.
Gimenez, C., Mitchell, V. J., Lawlor, D. W. (1992). Regulation of photosynthetic rate of two sunflower hybrids under water stress. Plant Physiol. 98, 516–524. doi: 10.1104/pp.98.2.516
Grady, K. C., Kolb, T. E., Ikeda, D. H., Whitham, T. G. (2015). A bridge too far: cold and pathogen constraints to assisted migration of riparian forests. Restor. Ecol. 23, 811–820. doi: 10.1111/rec.12245
Gratani, L. (2014). Plant phenotypic plasticity in response to environmental factors. Adv. Bot. 2014, 17. doi: 10.1155/2014/208747
Grossnickle, S. C., Sutton, B. C. S., Folk, R. S., Gawley, R. J. (1996). Relationship between nuclear DNA markers and physiological parameters in Sitka × interior spruce populations. Tree Physiol. 16, 547–555. doi: 10.1093/treephys/16.6.547
Hagedorn, F., Joseph, J., Peter, M., Luster, J., Pritsch, K., Geppert, U., et al. (2016). Recovery of trees from drought depends on belowground sink control. Nat. Plants 2, 16111. doi: 10.1038/nplants.2016.111
Handley, L. L., Austin, A. T., Stewart, G. R., Robinson, D., Scrimgeour, C. M., Raven, J. A., et al. (1999). The 15N natural abundance (δ15N) of ecosystem samples reflects measures of water availability. Funct. Plant Biol. 26, 185–199. doi: 10.1071/pp98146
Haney, C. H., Samuel, B. S., Bush, J., Ausubel, F. M. (2015). Associations with rhizosphere bacteria can confer an adaptive advantage to plants. Nat. Plants 1, 15051. doi: 10.1038/nplants.2015.51
Harper, J. L. (1977). Population biology of plants. (Adacemic Press). 900. https://www.cabdirect.org/cabdirect/abstract/19782321379
Hartmann, H., Moura, C. F., Anderegg, W. R. L., Ruehr, N. K., Salmon, Y., Allen, C. D., et al. (2018). Research frontiers for improving our understanding of drought-induced tree and forest mortality. New Phytol. 218, 15–28. doi: 10.1111/nph.15048
Hayatsu, M., Tago, K., Saito, M. (2008). Various players in the nitrogen cycle: diversity and functions of the microorganisms involved in nitrification and denitrification. Soil Sci. Plant Nutr. 54, 33–45. doi: 10.1111/j.1747-0765.2007.00195.x
Hedges, L. V., Olkin, I. (2014). Statistical methods for meta-analysis (Cambridge, Massachusetts, USA: Academic Press).
Hedges, L. V., Olkin, I., Hedges, L. V. (1985). Statistical Methods for Meta-Analysis. Available at: https://www.scholars.northwestern.edu/en/publications/statistical-methods-for-meta-analysis. [Accessed March 8, 2019].
Hodge, A. (2004). The plastic plant: root responses to heterogeneous supplies of nutrients. New Phytol. 162, 9–24. doi: 10.1111/j.1469-8137.2004.01015.x
Hoekenga, O. A., Vision, T. J., Shaff, J. E., Monforte, A. J., Lee, G. P., Howell, S. H., et al. (2003). Identification and Characterization of aluminum tolerance Loci in Arabidopsis (Landsberg erecta × Columbia) by quantitative trait locus mapping. A physiologically simple but genetically complex trait. Plant Physiol. 132, 936–948. doi: 10.1104/pp.103.023085
Holmes, A. J., Bowyer, J., Holley, M. P., O’Donoghue, M., Montgomery, M., Gillings, M. R. (2000). Diverse, yet-to-be-cultured members of the Rubrobacter subdivision of the Actinobacteria are widespread in Australian arid soils. FEMS Microbiol. Ecol. 33, 111–120. doi: 10.1111/j.1574-6941.2000.tb00733.x
Houlton, B. Z., Sigman, D. M., Schuur, E. A. G., Hedin, L. O. (2007). A climate-driven switch in plant nitrogen acquisition within tropical forest communities. Proc. Natl. Acad. Sci. 104, 8902–8906. doi: 10.1073/pnas.0609935104
Howe, G. T., Aitken, S. N., Neale, D. B., Jermstad, K. D., Wheeler, N. C., Chen, T. H. H. (2003). From genotype to phenotype: unraveling the complexities of cold adaptation in forest trees. Can. J. Bot. 81, 1247–1266. doi: 10.1139/b03-141
Ings, J., Mur, L. A. J., Robson, P. R. H., Bosch, M. (2013). Physiological and growth responses to water deficit in the bioenergy crop Miscanthus x giganteus. Front. Plant Sci. 4, 468. doi: 10.3389/fpls.2013.00468
İnceoğlu, Ö., Salles, J. F., van Overbeek, L., van Elsas, J. D. (2010). Effects of plant genotype and growth stage on the betaproteobacterial communities associated with different potato cultivars in two fields. Appl. Env. Microbiol. 76, 3675–3684. doi: 10.1128/AEM.00040-10
IPCC (2018). https://www.ipcc.ch/sr15/. IPCC 2018 Spec. Rep. Glob. Warm. 15C. Available at: .
Isaac-Renton, M., Montwé, D., Hamann, A., Spiecker, H., Cherubini, P., Treydte, K. (2018). Northern forest tree populations are physiologically maladapted to drought. Nat. Commun. 9, 1–9. doi: 10.1038/s41467-018-07701-0
Jackson, S. T., Betancourt, J. L., Booth, R. K., Gray, S. T. (2009). Ecology and the ratchet of events: climate variability, niche dimensions, and species distributions. Proc. Natl. Acad. Sci. 106 (Supplement 2), 19685–19692. doi: 10.1073/pnas.0901644106
Jacoby, R., Peukert, M., Succurro, A., Koprivova, A., Kopriva, S. (2017). The role of soil microorganisms in plant mineral nutrition—current knowledge and future directions. Front. Plant Sci. 8, 1617. doi: 10.3389/fpls.2017.01617
Johnson, D. M., McCulloh, K. A., Reinhardt, K. (2011). “The Earliest Stages of Tree Growth: Development, Physiology and Impacts of Microclimate,” in Size- and Age-Related Changes in Tree Structure and Function. Eds. Meinzer, F. C., Lachenbruch, B., Dawson, T. E. (Dordrecht: Springer Netherlands), 65–87. Available at: [Accessed September 9, 2016].
Jones, D. L., Simfukwe, P., Hill, P. W., Mills, R. T., Emmett, B. A. (2014). Evaluation of dissolved organic carbon as a soil quality indicator in national monitoring schemes. PloS One 9, e90882. doi: 10.1371/journal.pone.0090882
Kaci, Y., Heyraud, A., Barakat, M., Heulin, T. (2005). Isolation and identification of an EPS-producing Rhizobium strain from arid soil (Algeria): characterization of its EPS and the effect of inoculation on wheat rhizosphere soil structure. Res. Microbiol. 156, 522–531. doi: 10.1016/j.resmic.2005.01.012
Kahmen, A., Wanek, W., Buchmann, N. (2008). Foliar δ15N values characterize soil N cycling and reflect nitrate or ammonium preference of plants along a temperate grassland gradient. Oecologia 156, 861–870. doi: 10.1007/s00442-008-1028-8
Kannenberg, S. A., Phillips, R. P. (2017). Soil microbial communities buffer physiological responses to drought stress in three hardwood species. Oecologia 183, 631–641. doi: 10.1007/s00442-016-3783-2
Karst, J., Gaster, J., Wiley, E., Landhäusser, S. M. (2017). Stress differentially causes roots of tree seedlings to exude carbon. Tree Physiol. 37, 154–164. doi: 10.1093/treephys/tpw090
Kawecki, T. J., Ebert, D. (2004). Conceptual issues in local adaptation. Ecol. Lett. 7, 1225–1241. doi: 10.1111/j.1461-0248.2004.00684.x
Kerr, K. L., Meinzer, F. C., McCulloh, K. A., Woodruff, D. R., Marias, D. E. (2015). Expression of functional traits during seedling establishment in two populations of Pinus ponderosa from contrasting climates. Tree Physiol. 35, 535–548. doi: 10.1093/treephys/tpv034
Khanna-Chopra, R., Singh, K. (2015). “Drought Resistance in Crops: Physiological and Genetic Basis of Traits for Crop Productivity,” in Stress Responses in Plants: Mechanisms of Toxicity and Tolerance. Eds. Tripathi, B. N., Müller, M. (Cham: Springer International Publishing), 267–292. doi: 10.1007/978-3-319-13368-3_11
Kim, Y.-C., Glick, B. R., Bashan, Y., Ryu, C.-M. (2012). “Enhancement of Plant Drought Tolerance by Microbes,” in Plant Responses to Drought Stress: From Morphological to Molecular Features. Ed. Aroca, R. (Berlin, Heidelberg: Springer Berlin Heidelberg), 383–413. doi: 10.1007/978-3-642-32653-0_15
Kubiske, M. E., Abrams, M. D. (1991). Seasonal, diurnal and rehydration-induced variation of pressure-volume relationships in Pseudotsuga menziesii. Physiol. Plant 83, 107–116. doi: 10.1111/j.1399-3054.1991.tb01288.x
Kursar, T. A., Engelbrecht, B. M. J., Burke, A., Tyree, M. T., Omari, B. E., Giraldo, J. P. (2009). Tolerance to low leaf water status of tropical tree seedlings is related to drought performance and distribution. Funct. Ecol. 23, 93–102. doi: 10.1111/j.1365-2435.2008.01483.x
Lakens, D. (2013). Calculating and reporting effect sizes to facilitate cumulative science: a practical primer for t-tests and ANOVAs. Front. Psychol. 4, 863. doi: 10.3389/fpsyg.2013.00863
Lambeth, C. C., Dougherty, P. M., Gladstone, W. T., McCullough, R. B., Wells, O. O. (1984). Large-scale planting of north carolina loblolly pine in Arkansas and Oklahoma: a case of gain versus risk. J. For. 82, 736–741. doi: 10.1093/jof/82.12.736
Lamit, L. J., Holeski, L. M., Flores-Rentería, L., Whitham, T. G., Gehring, C. A. (2016). Tree genotype influences ectomycorrhizal fungal community structure: ecological and evolutionary implications. Fungal Ecol. 24, 124–134. doi: 10.1016/j.funeco.2016.05.013
Lareen, A., Burton, F., Schäfer, P. (2016). Plant root-microbe communication in shaping root microbiomes. Plant Mol. Biol. 90, 575–587. doi: 10.1007/s11103-015-0417-8
Levitt, J. (1980). Response of plants to environmental stresses. Water radiat. Salt Stress. 2, 497. doi: 10.1016/s1369-5266(03)00035-9
Li, B., Allen, H. L., McKeand, S. E. (1991). Nitrogen and family effects on biomass allocation of loblolly pine seedlings. For. Sci. 37, 271–283. doi: 10.1093/forestscience/37.1.271
Liese, R., Lübbe, T., Albers, N. W., Meier, I. C. (2018). The mycorrhizal type governs root exudation and nitrogen uptake of temperate tree species. Tree Physiol. 38, 83–95. doi: 10.1093/treephys/tpx131
López, R., Rodríguez-Calcerrada, J., Gil, L. (2009). Physiological and morphological response to water deficit in seedlings of five provenances of Pinus canariensis: potential to detect variation in drought-tolerance. Trees 23, 509–519. doi: 10.1007/s00468-008-0297-5
López-Bucio, J., Cruz-Ramírez, A., Herrera-Estrella, L. (2003). The role of nutrient availability in regulating root architecture. Curr. Opin. Plant Biol. 6, 280–287. doi: 10.1016/s1369-5266(03)00035-9
Louahlia, S., Lainé, P., Thornton, B., Ourry, A., Boucaud, J. (2000). The role of N-remobilisation and the uptake of NH4+ and NO3- by Lolium perenne L. @ in laminae growth following defoliation under field conditions. Plant Soil 220, 175–187. doi: 10.1023/A:1004728327955
Lucash, M., Yanai, R., Joslin, J. (2008). Nutrient uptake by intact and disturbed roots of loblolly pine seedlings. Environ. Sci. Manage. Fac. Publ. Present. 64 (1), 15–20. doi: 10.1016/j.envexpbot.2008.05.013
Ma, F., Na, X., Xu, T. (2016). Drought responses of three closely related Caragana species: implication for their vicarious distribution. Ecol. Evol. 6, 2763–2773. doi: 10.1002/ece32044
Madhaiyan, M., Poonguzhali, S., Lee, H. S., Hari, K., Sundaram, S. P., Sa, T. M. (2005). Pink-pigmented facultative methylotrophic bacteria accelerate germination, growth and yield of sugarcane clone Co86032 (Saccharum officinarum L.). Biol. Fertil. Soils 41, 350–358. doi: 10.1007/s00374-005-0838-7
Maire, V., Gross, N., Pontes, L. D. S., Picon-Cochard, C., Soussana, J.-F. (2009). Trade-off between root nitrogen acquisition and shoot nitrogen utilization across 13 co-occurring pasture grass species. Funct. Ecol. 23, 668–679. doi: 10.1111/j.1365-2435.2009.01557.x
Majumdar, S., Ghosh, S., Glick, B. R., Dumbroff, E. B. (1991). Activities of chlorophyllase, phosphoenolpyruvate carboxylase and ribulose-1,5-bisphosphate carboxylase in the primary leaves of soybean during senescence and drought. Physiol. Plant 81, 473–480. doi: 10.1111/j.1399-3054.1991.tb05087.x
Marias, D. E., Meinzer, F. C., Woodruff, D. R., McCulloh, K. A. (2017). Thermotolerance and heat stress responses of Douglas-fir and ponderosa pine seedling populations from contrasting climates. Tree Physiol. 37, 301–315. doi: 10.1093/treephys/tpw117
Mariotti, A., Germon, J., Hubert, P., Kaiser, P., Letolle, R., Tardieux, A., et al. (1981). Experimental determination of nitrogen kinetic isotope fractionation: some principles; illustration for the denitrification and nitrification processes. Plant Soil 62, 413–430. doi: 10.1007/BF02374138
Marschner, P. (2012). “Chapter 15 - Rhizosphere Biology,” in Marschner’s Mineral Nutrition of Higher Plants (Third Edition). Ed. Marschner, P. (San Diego: Academic Press), 369–388. doi: 10.1016/B978-0-12-384905-2.00015-7
Maxwell, K., Johnson, G. N. (2000). Chlorophyll fluorescence—a practical guide. J. Exp. Bot. 51, 659–668. doi: 10.1093/jexbot/51.345.659
Meier, C. E., Newton, R. J., Puryear, J. D., Sen, S. (1992). Physiological responses of loblolly pine (Pinus taeda L.) seedlings to drought stress: osmotic adjustment and tissue elasticity. J. Plant Physiol. 140, 754–760. doi: 10.1016/S0176-1617(11)81034-5
Meinzer, F. C., Woodruff, D. R., Marias, D. E., Mcculloh, K. A., Sevanto, S. (2014). Dynamics of leaf water relations components in co-occurring iso- and anisohydric conifer species. Plant Cell Environ. 37, 2577–2586. doi: 10.1111/pce.12327
Mendes, R., Garbeva, P., Raaijmakers, J. M. (2013). The rhizosphere microbiome: significance of plant beneficial, plant pathogenic, and human pathogenic microorganisms. FEMS Microbiol. Rev. 37, 634–663. doi: 10.1111/1574-6976.12028
Micallef, S. A., Shiaris, M. P., Colón-Carmona, A. (2009). Influence of Arabidopsis thaliana accessions on rhizobacterial communities and natural variation in root exudates. J. Exp. Bot. 60, 1729–1742. doi: 10.1093/jxb/erp053
Miller, A. E., Bowman, W. D. (2002). Variation in nitrogen-15 natural abundance and nitrogen uptake traits among co-occurring alpine species: do species partition by nitrogen form? Oecologia 130, 609–616. doi: 10.1007/s00442-001-0838-8
Miller, B. D., Hawkins, B. J. (2007). Ammonium and nitrate uptake, nitrogen productivity and biomass allocation in interior spruce families with contrasting growth rates and mineral nutrient preconditioning. Tree Physiol. 27, 901–909. doi: 10.1093/treephys/27.6.901
Moran, E., Lauder, J., Musser, C., Stathos, A., Shu, M. (2017). The genetics of drought tolerance in conifers. New Phytol. 216, 1034–1048. doi: 10.1111/nph.14774
Mueller, R. C., Gallegos-Graves, L. V., Kuske, C. R. (2016). A new fungal large subunit ribosomal RNA primer for high-throughput sequencing surveys. FEMS Microbiol. Ecol. 92, fiv153. doi: 10.1093/femsec/fiv153
Newsham, K. K. (2011). A meta-analysis of plant responses to dark septate root endophytes. New Phytol. 190, 783–793. doi: 10.1111/j.1469-8137.2010.03611.x
Nguyen-Queyrens, A., Bouchet-Lannat, F. (2003). Osmotic adjustment in three-year-old seedlings of five provenances of maritime pine (Pinus pinaster) in response to drought. Tree Physiol. 23, 397–404. doi: 10.1093/treephys/23.6.397
O’Brien, M. J., Pugnaire, F. I., Rodríguez-Echeverría, S., Morillo, J. A., Martín-Usero, F., López-Escoriza, A., et al. (2018). Mimicking a rainfall gradient to test the role of soil microbiota for mediating plant responses to drier conditions. Oikos 127, 1776–1786. doi: 10.1111/oik.05443
O’Neill, G. A., Hamann, A., Wang, T. (2008). Accounting for population variation improves estimates of the impact of climate change on species’ growth and distribution. J. Appl. Ecol. 45, 1040–1049. doi: 10.1111/j.1365-2664.2008.01472.x
Ohyama, T. (2010). “Nitrogen as a major essential element of plants,” in Nitrogen Assim. Kerala, India: Plants Signpost Trivandrum.
Or, D., Smets, B. F., Wraith, J. M., Dechesne, A., Friedman, S. P. (2007). Physical constraints affecting bacterial habitats and activity in unsaturated porous media – a review. Adv. Water Resour. 30, 1505–1527. doi: 10.1016/j.advwatres.2006.05.025
Patel, J. S., Singh, A., Singh, H. B., Sarma, B. K. (2015). Plant genotype, microbial recruitment and nutritional security. Front. Plant Sci. 6, 608. doi: 10.3389/fpls.2015.00608
Phillips, R. P., Erlitz, Y., Bier, R., Bernhardt, E. S. (2008). New approach for capturing soluble root exudates in forest soils. Funct. Ecol. 22, 990–999. doi: 10.1111/j.1365-2435.2008.01495.x
Pinheiro, J., Bates, D., DebRoy, S., Sarkar, D., R Core Team (2019). nlme: Linear and Nonlinear Mixed Effects Models. Rpackage version 3.1-141. Available at: https://CRAN.R-project.org/package=nlme.
Polle, A., Chen, S. L., Eckert, C., Harfouche, A. (2019). Engineering drought resistance in forest trees. Front. Plant Sci. 9, 1875. doi: 10.3389/fpls.2018.01875
Porras-Alfaro, A., Herrera, J., Sinsabaugh, R. L., Odenbach, K. J., Lowrey, T., Natvig, D. O. (2008). Novel root fungal consortium associated with a dominant desert grass. Appl. Environ. Microbiol. 74, 2805–2813. doi: 10.1128/AEM.02769-07
Porras-Alfaro, A., Herrera, J., Natvig, D. O., Lipinski, K., Sinsabaugh, R. L. (2011). Diversity and distribution of soil fungal communities in a semiarid grassland. Mycologia 103, 10–21. doi: 10.3852/09-297
Preece, C., Farré-Armengol, G., Llusià, J., Peñuelas, J. (2018). Thirsty tree roots exude more carbon. Tree Physiol. 38, 690–695. doi: 10.1093/treephys/tpx163
PRISM (2018).PRISM Clim. Group Or.State Univ. Available at: http://prism.oregonstate.edu .
Purahong, W., Durka, W., Fischer, M., Dommert, S., Schöps, R., Buscot, F., et al. (2016). Tree species, tree genotypes and tree genotypic diversity levels affect microbe-mediated soil ecosystem functions in a subtropical forest. Sci. Rep. 6, 36672. doi: 10.1038/srep36672
R Core Team, C. T. (2018). R-project (Vienna, Austria: R Foundation for Statistical Computing;), 2013.
Ramírez-Valiente, J. A., Deacon, N. J., Etterson, J., Center, A., Sparks, J. P., Sparks, K. L., et al. (2018). Natural selection and neutral evolutionary processes contribute to genetic divergence in leaf traits across a precipitation gradient in the tropical oak Quercus oleoides. Mol. Ecol. 27, 2176–2192. doi: 10.1111/mec.14566
Rincón, A., Valladares, F., Gimeno, T. E., Pueyo, J. J. (2008). Water stress responses of two Mediterranean tree species influenced by native soil microorganisms and inoculation with a plant growth promoting rhizobacterium. Tree Physiol. 28, 1693–1701. doi: 10.1093/treephys/28.11.1693
Roberson, E. B., Firestone, M. K. (1992). Relationship between desiccation and exopolysaccharide production in a soil pseudomonas sp. Appl. Environ. Microbiol. 58, 1284–1291.
Roches, S. D., Post, D. M., Turley, N. E., Bailey, J. K., Hendry, A. P., Kinnison, M. T., et al. (2018). The ecological importance of intraspecific variation. Nat. Ecol. Evol. 2, 57. doi: 10.1038/s41559-017-0402-5
Rodriguez, R. J., Henson, J., Van Volkenburgh, E., Hoy, M., Wright, L., Beckwith, F., et al. (2008). Stress tolerance in plants via habitat-adapted symbiosis. ISME J. 2, 404–416. doi: 10.1038/ismej.2007.106
Roskilly, B., Keeling, E., Hood, S., Giuggiola, A., Sala, A. (2019). Conflicting functional effects of xylem pit structure relate to the growth-longevity trade-off in a conifer species. Proc. Natl. Acad. Sci. 201900734, 15282–15287. doi: 10.1073/pnas.1900734116
Sánchez-Cañizares, C., Jorrín, B., Poole, P. S., Tkacz, A. (2017). Understanding the holobiont: the interdependence of plants and their microbiome. Curr. Opin. Microbiol. 38, 188–196. doi: 10.1016/j.mib.2017.07.001
Sapes, G., Roskilly, B., Dobrowski, S., Maneta, M., Anderegg, W. R. L., Martinez-Vilalta, J., et al. (2019). Plant water content integrates hydraulics and carbon depletion to predict drought-induced seedling mortality. Tree Physiol. 39, 1300–1312. doi: 10.1093/treephys/tpz062
Sasse, J., Martinoia, E., Northen, T. (2018). Feed your friends: do plant exudates shape the root microbiome? Trends Plant Sci. 23, 25–41. doi: 10.1016/j.tplants.2017.09.003
Schindelin, J., Arganda-Carreras, I., Frise, E., Kaynig, V., Longair, M., Pietzsch, T., et al. (2012). Fiji: an open-source platform for biological-image analysis. Nat. Methods 9, 676. doi: 10.1038/nmeth.2019
Schirawski, J., Perlin, M. H. (2018). Plant–microbe interaction 2017—the good, the bad and the diverse. Int. J. Mol. Sci. 19, 1374. doi: 10.3390/ijms19051374
Schneider, C. A., Rasband, W. S., Eliceiri, K. W. (2012). NIH Image to ImageJ: 25 years of image analysis. Nat. Methods 9, 671. doi: 10.1038/nmeth.2089
Schultz, R. P. (1997). “Loblolly pine: the ecology and culture of loblolly pine (Pinus taeda L.),” in Agriculture Handbook 713 (Washington DC: US Department of Agriculture Forost Service), 493. Available at: [Accessed December 3, 2018].
Schweitzer, J. A., Bailey, J. K., Fischer, D. G., LeRoy, C. J., Lonsdorf, E. V., Whitham, T. G., et al. (2008). Plant–soil–microorganism interactions: heritable relationship between plant genotype and associated soil microorganisms. Ecology 89, 773–781. doi: 10.1890/07-0337.1
Shearer, G., Kohl, D. H. (1986). N2-Fixation in field settings: estimations based on natural 15N abundance. Funct. Plant Biol. 13, 699–756. doi: 10.1071/pp9860699
Shearer, G., Duffy, J., Kohl, D. H., Commoner, B. (1974). A steady-state model of isotopic fractionation accompanying nitrogen transformations in soil 1. Soil Sci. Soc Am. J. 38, 315–322. doi: 10.2136/sssaj1974.03615995003800020030x
Sierra-Lucero, V., McKeand, S. E., Huber, D. A., Rockwood, D. L., White, T. L. (2002). Performance differences and genetic parameters for four coastal provenances of loblolly pine in the southeastern United States. For. Sci. 48, 732–742. doi: 10.1093/forestscience/48.4.732
Simeone, C., Maneta, M. P., Holden, Z. A., Sapes, G., Sala, A., Dobrowski, S. Z. (2019). Coupled ecohydrology and plant hydraulics modeling predicts ponderosa pine seedling mortality and lower treeline in the US Northern Rocky Mountains. New Phytol. 221, 1814–1830. doi: 10.1111/nph.15499
Subbarao, G. V., Ito, O., Sahrawat, K. L., Berry, W. L., Nakahara, K., Ishikawa, T., et al. (2006). Scope and strategies for regulation of nitrification in agricultural systems—Challenges and Opportunities. Crit. Rev. Plant Sci. 25, 303–335. doi: 10.1080/07352680600794232
Sultan, S. E. (2000). Phenotypic plasticity for plant development, function and life history. Trends Plant Sci. 5, 537–542. doi: 10.1016/S1360-1385(00)01797-0
Temperton, V. M., Märtin, L. L. A., Röder, D., Lücke, A., Kiehl, K. (2012). Effects of four different restoration treatments on the natural abundance of 15N stable isotopes in plants. Front. Plant Sci. 3, 70. doi: 10.3389/fpls.2012.00070
Ulrich, D. E. M., Sevanto, S., Ryan, M., Albright, M. B. N., Johansen, R. B., Dunbar, J. M. (2019). Plant-microbe interactions before drought influence plant physiological responses to subsequent severe drought. Sci. Rep. 9, 249. doi: 10.1038/s41598-018-36971-3
Usuki, F., Narisawa, K. (2007). A mutualistic symbiosis between a dark septate endophytic fungus, Heteroconium chaetospira, and a nonmycorrhizal plant, Chinese cabbage. Mycologia 99, 175–184. doi: 10.1080/15572536.2007.11832577
Vasquez, E., Sheley, R., Svejcar, T. (2008). Creating Invasion Resistant Soils via Nitrogen Management. Invasive Plant Sci. Manage. 1, 304–314. doi: 10.1614/IPSM-07-059.1
Verbon, E. H., Liberman, L. M. (2016). Beneficial microbes affect endogenous mechanisms controlling root development. Trends Plant Sci. 21, 218–229. doi: 10.1016/j.tplants.2016.01.013
Vergara, C., Araujo, K. E. C., Alves, L. S., de Souza, S. R., Santos, L. A., Santa-Catarina, C., et al. (2018). Contribution of dark septate fungi to the nutrient uptake and growth of rice plants. Braz. J. Microbiol. 49, 67–78. doi: 10.1016/j.bjm.2017.04.010
Vonderwell, J. D., Enebak, S. A., Samuelson, L. J. (2001). Influence of two plant growth-promoting Rhizobacteria on loblolly pine root respiration and IAA activity. For. Sci. 47, 197–202. doi: 10.1093/forestscience/47.2.197
Wagg, C., Pautler, M., Massicotte, H. B., Peterson, R. L. (2008). The co-occurrence of ectomycorrhizal, arbuscular mycorrhizal, and dark septate fungi in seedlings of four members of the Pinaceae. Mycorrhiza 18, 103–110. doi: 10.1007/s00572-007-0157-y
Walker, T. S., Bais, H. P., Grotewold, E., Vivanco, J. M. (2003). Root Exudation and Rhizosphere Biology. Plant Physiol. 132, 44–51. doi: 10.1104/pp.102.019661
Warnes, G. R., Bolker, B., Lumley, T., Johnson, R. C. (2018). gmodels: Various R Programming Tools for Model Fitting. R package version 2.18.1. Available at: https://CRAN.R-project.org/package=gmodels. .
Wells, O. O. (1983). Southwide Pine Seed Source Study—Loblolly Pine at 25 Years. South. J. Appl. For. 7, 63–71. doi: 10.1093/sjaf/7.2.63
Whitham, T. G., Young, W. P., Martinsen, G. D., Gehring, C. A., Schweitzer, J. A., Shuster, S. M., et al. (2003). Community and Ecosystem Genetics: A Consequence of the Extended Phenotype. Ecology 84, 559–573. doi: 10.1890/0012-9658(2003)084[0559:CAEGAC]2.0.CO;2
Whitham, T. G., Gehring, C. A., Lamit, L. J., Wojtowicz, T., Evans, L. M., Keith, A. R., et al. (2012). Community specificity: life and afterlife effects of genes. Trends Plant Sci. 17, 271–281. doi: 10.1016/j.tplants.2012.01.005
Wille, L., Messmer, M. M., Studer, B., Hohmann, P. (2019). Insights to plant–microbe interactions provide opportunities to improve resistance breeding against root diseases in grain legumes. Plant Cell Environ. 42, 20–40. doi: 10.1111/pce.13214
Wu, Y.-N., Feng, Y.-L., Paré, P. W., Chen, Y.-L., Xu, R., Wu, S., et al. (2016). Beneficial soil microbe promotes seed germination, plant growth and photosynthesis in herbal crop Codonopsis pilosula. Crop Pasture Sci. 67, 91–99. doi: 10.1071/CP15110
Yang, W. Q., Murthy, R., King, P., Topa, M. A. (2002). Diurnal changes in gas exchange and carbon partitioning in needles of fast- and slow-growing families of loblolly pine (Pinus taeda). Tree Physiol. 22, 489–498. doi: 10.1093/treephys/22.7.489
Yang, J., Kloepper, J. W., Ryu, C.-M. (2009). Rhizosphere bacteria help plants tolerate abiotic stress. Trends Plant Sci. 14, 1–4. doi: 10.1016/j.tplants.2008.10.004
Yao, J., Sun, D., Cen, H., Xu, H., Weng, H., Yuan, F., et al. (2018). Phenotyping of Arabidopsis drought stress response using kinetic chlorophyll fluorescence and multicolor fluorescence imaging. Front. Plant Sci. 9, 9. doi: 10.3389/fpls.2018.00603
Yin, H., Li, Y., Xiao, J., Xu, Z., Cheng, X., Liu, Q. (2013). Enhanced root exudation stimulates soil nitrogen transformations in a subalpine coniferous forest under experimental warming. Glob. Change Biol. 19, 2158–2167. doi: 10.1111/gcb.12161
Young, I. M. (1995). Variation in moisture contents between bulk soil and the rhizosheath of wheat (Triticum aestivum L. cv. Wembley). New Phytol. 130, 135–139. doi: 10.1111/j.1469-8137.1995.tb01823.x
Zhalnina, K., Louie, K. B., Hao, Z., Mansoori, N., da Rocha, U. N., Shi, S., et al. (2018). Dynamic root exudate chemistry and microbial substrate preferences drive patterns in rhizosphere microbial community assembly. Nat. Microbiol. 3 (4), 470. doi: 10.1016/B978-0-12-520920-5.50016-X
Zhang, J., Marshall, J. D., Jaquish, B. C. (1993). Genetic differentiation in carbon isotope discrimination and gas exchange in Pseudotsuga menziesii. Oecologia 93, 80–87. doi: 10.1007/BF00321195
Zhang, J. W., Feng, Z., Cregg, B. M., Schumann, C. M. (1997). Carbon isotopic composition, gas exchange, and growth of three populations of ponderosa pine differing in drought tolerance. Tree Physiol. 17, 461–466. doi: 10.1093/treephys/17.7.461
Keywords: soil microbes, loblolly pine, seedling physiology, genetic variation, drought, turgor loss point, growth, root exudates
Citation: Ulrich DEM, Sevanto S, Peterson S, Ryan M and Dunbar J (2020) Effects of Soil Microbes on Functional Traits of Loblolly Pine (Pinus taeda) Seedling Families From Contrasting Climates. Front. Plant Sci. 10:1643. doi: 10.3389/fpls.2019.01643
Received: 25 June 2019; Accepted: 21 November 2019;
Published: 09 January 2020.
Edited by:
Brigitte Mauch-Mani, Université de Neuchâtel, SwitzerlandReviewed by:
Carla Pinheiro, New University of Lisbon, PortugalCopyright © 2020 Ulrich, Sevanto, Peterson, Ryan and Dunbar. This is an open-access article distributed under the terms of the Creative Commons Attribution License (CC BY). The use, distribution or reproduction in other forums is permitted, provided the original author(s) and the copyright owner(s) are credited and that the original publication in this journal is cited, in accordance with accepted academic practice. No use, distribution or reproduction is permitted which does not comply with these terms.
*Correspondence: Danielle E. M. Ulrich, ZGFuaWVsbGUudWxyaWNoQG1vbnRhbmEuZWR1ZA==
Disclaimer: All claims expressed in this article are solely those of the authors and do not necessarily represent those of their affiliated organizations, or those of the publisher, the editors and the reviewers. Any product that may be evaluated in this article or claim that may be made by its manufacturer is not guaranteed or endorsed by the publisher.
Research integrity at Frontiers
Learn more about the work of our research integrity team to safeguard the quality of each article we publish.