- Institute of Vegetables and Flowers, Chinese Academy of Agricultural Sciences, Beijing, China
Cucumber (Cucumis sativus L.) is an economically important vegetable crop worldwide, but it is sensitive to low temperatures. Cucumber seedlings exposed to long-term low temperature stress (LT), i.e., below 20°C during the day, and 8°C at night, exhibit leaf yellowing, accelerated senescence, and reduced yield, therefore posing a threat to cucumber production. Studying the underlying mechanisms involved in LT tolerance in cucumber seedlings, and developing germplasm with improved LT-tolerance could provide fundamental solutions to the problem. In this study, an F2 population was generated from two parental lines, “CG104” (LT-tolerant inbred line) and “CG37” (LT-sensitive inbred line), to identify loci that are responsible for LT tolerance in cucumber seedlings. Replicated phenotypic analysis of the F2-derived F3 family using a low-temperature injury index (LTII) suggested that the LT tolerance of cucumber seedlings is controlled by multiple genes. A genetic map of 990.8 cM was constructed, with an average interval between markers of 5.2 cM. One quantitative trait loci (QTL) named qLTT5.1 on chromosome 5, and two QTLs named qLTT6.1 and qLTT6.2 on chromosome 6 were detected. Among them, qLTT6.2 accounted for 26.8 and 24.1% of the phenotypic variation in two different experiments. Single-nucleotide polymorphism (SNP) variations within the region of qLTT6.2 were analyzed using two contrasting in silico bulks generated from the cucumber core germplasm. Result showed that 214 SNPs were distributed within the 42-kb interval, containing three candidate genes. Real-time quantitative reverse transcription PCR and sequence analysis suggested that two genes Csa6G445210, an auxin response factor, and Csa6G445230, an ethylene-responsive transmembrane protein, might be candidate genes responsible for LT tolerance in cucumber seedlings. This study furthers the understanding of the molecular mechanism underlying LT tolerance in cucumber seedlings, and provides new markers for molecular breeding.
Introduction
Cucumber (Cucumis sativus L.) is an economically important vegetable crop. Global production reached 80 million metric tons in 2016, with a steady increase in production since 1998 (Chen et al., 2019). Cucumber originated from tropical regions, and the suitable temperature range for growth is 18–30°C. As an annual vegetable crop which is sensitive to low temperature (LT) (Cabrera et al., 1992), cucumber easily suffers from LT injury in winter and early spring in regions such as Northern China, necessitating greenhouse-cultivation in these regions. Depending on its intensity and duration, LT-stress may be divided into long-term moderate LT (below 20°C during daytime and 8°C at night for days or weeks) and short-term extreme LT (15°C during the day and 4°C at night for hours) (Smeets and Wehner, 1997). Long-term moderate LT stress occurs more often during production, leading to yellowed leaves, accelerated senescence, and decreased yield (Smeets and Wehner, 1997), such that even this moderate stress has become a serious obstacle for the cucumber production industry. Thus, LT tolerance at the seedling stage is a desirable trait for cucumber breeding. With the continuous expansion of the cucumber planting area into early spring and winter in China, it is critical to identify candidate genes responsible for LT tolerance, and to develop resistant cultivars.
LT tolerance of cucumber seedling is a complex trait controlled by multiple alleles at multiple loci (Chung et al., 2003; Kozik and Wehner, 2008; Kozik et al., 2012). Chung et al. (2003) found that LT tolerance at the seedling stage is maternally inherited, and that the gene conferring resistance comes from the chloroplast genome of the female parent “Chipper.” Kozik and Wehner (2008) reported that the LT tolerance of cucumber seedlings was controlled by a single dominant gene Ch. More recently, a study of two genotypes, LT-tolerant “PI390953” and cold-sensitive “Gy14” suggested that LT tolerance of cucumber seedlings was determined by two genes (Kozik et al., 2012).
There are few recent reports on the gene or quantitative trait loci (QTL) mapping of LT tolerance in cucumber seedlings. A few studies were carried out under short-term extreme LT (Li, 2014; Wang, 2014; Zhou, 2015). When a qualitative chilling injury index (CII) was used as an indicator of LT stress, one simple sequence repeat (SSR) marker closely linked to a LT-tolerant gene on chromosome 6 was identified (Li, 2014). Zhou (2015) identified six loci associated with LT tolerance on chromosome 3. Wang (2014) used CII and a recovery index as indicators of LT tolerance, and identified three QTLs on chromosome 3 and one QTL on chromosome 7, respectively. However, there are no reports of LT-tolerant QTLs or gene mapping using long-term moderate LT as a selective criterion, and long-term moderate LT stress occurs more often during cucumber production.
Since the International Cucumber Genome Team announced the whole genome sequence of “Chinese long” line 9930 (Huang et al., 2009), multiple lines have been re-sequenced, revealing all genotypic variability (Qi et al., 2013). A new approach called “in silico BSA (bulked segregant analysis)” was reported (Bello et al., 2014). Its application avoids the construction of near isogenic lines and greatly improves the efficiency of gene mapping. The method is based on the results of gene/QTL mapping and genetically diverse germplasm with known phenotype. Two bulks with contrasting target phenotype are constructed, and the single-nucleotide polymorphism (SNP) variations within the target QTL region between two bulks are inspected. The SNPs that are identical within each bulk but different between two bulks may be highly associated with the target phenotype. With this method, Li et al. (2016) quickly narrowed the Cn locus into a 16-kb region, and Bo et al. (2019) fine-mapped the fruit spine density related major QTL qfsd6.2 to a 50-kb region, and finally identified the candidate gene Csgl3.
So far, studies on LT tolerance in cucumber mainly focused on the LT stress conditions applied, genetic mechanisms and germplasm evaluation criteria (Kozik and Wehner, 2008; Kozik et al., 2012). However, very few QTL analyses of LT tolerance in cucumber have been reported and no genes have been identified. Such information would provide practical tools to enhance breeding strategies for this trait. Therefore, the objective of this study was to identify QTL and candidate genes responsible for LT stress tolerance. Our preliminary study showed that the cucumber inbred line “CG104” exhibits high tolerance to LT, while inbred line “CG37” is sensitive to LT. An F2 population from a cross of these two inbred lines was constructed, and used for QTL mapping. Then, SNP variations within the major QTL region between two contrasting in silico bulks were analyzed. Sequence analysis and quantitative reverse transcription PCR analysis were then performed to identify the potential candidate gene. This study thus helps to further the understanding of the molecular mechanism underlying LT tolerance in cucumber seedlings, and provides new tools for breeding LT-tolerant cucumber germplasm via molecular marker-assisted breeding.
Materials and Methods
Plant Materials
Two cucumber inbred lines, “CG104” (LT-tolerant) and “CG37” (LT-sensitive) were used in this study. “CG37” and “CG104” were crossed to generate an F1 (“CG104” x “CG37”) and F1' (“CG37” x “CG104”) populations. The F1 (“CG104” x “CG37”) was self-pollinated to produce an F2 population of 189 individuals. A total of 189 F2:3 families were derived from F2 populations accordingly. Moreover, 10 core germplasms with high LT-sensitive or LT-resistant phenotype (Table S1) that have been resequenced (Qi et al., 2013) were used for in silico BSA analysis. The F2 population was used to verify mutation sites in candidate genes. All materials were preserved by the Cucumber Research Group, Institute of Vegetables and Flowers (IVF), Chinese Academy of Agricultural Sciences (CAAS).
Investigation of Low Temperature Injury at Seedling Stage
LT treatments were carried out twice in the greenhouse and plastic greenhouse of the IVF, CAAS at Changping, Beijing (40°13'N, 116°05'E) in early spring, respectively (Table S2). Three-week-old seedlings of the two parental lines, F1 and F2-derived F3 families were exposed to 15–17°C for 2 weeks, and the LT injury was classified into six grades, based on the degree of yellowing and dryness of the cotyledons and the first true leaf (Figure 1). The index used was as follows: 0: no symptoms on either the cotyledons or the first true leaf; 1: cotyledons were slightly yellow, while the first true leaf was still green; 3: cotyledons were yellow-green with yellowed edges, while the first true leaf was light green; 5: cotyledons were yellowed in large scale, while the first true leaf was yellow-green; 7: cotyledons were completely yellowed, while the first true leaf was yellow-green; 9: cotyledons and the first true leaf dried.
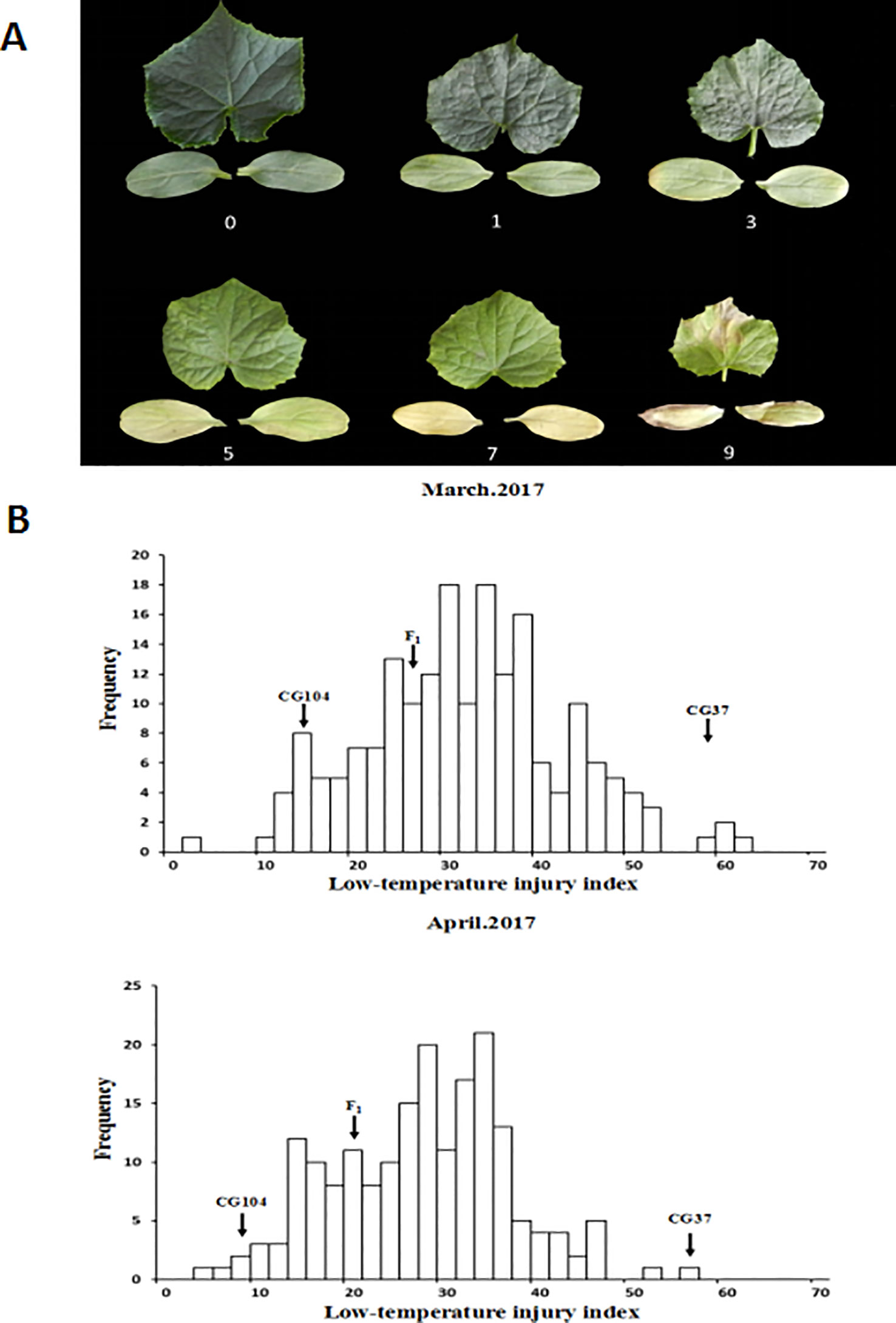
Figure 1 Phenotypic characterization of low temperature tolerance in F2:3 populations. (A) Scales of low-temperature injury index (LTII) based on the degree of yellowing and dryness of the cotyledons and the first true leaf. 0: no symptoms on either the cotyledons or first true leaf; 1: cotyledons were slightly yellow, while the first true leaf was still green; 3: cotyledons were yellow-green with yellowed edges, while the first true leaf was light green; 5: cotyledons were yellowed in large scale, while the first true leaf was yellow-green; 7: cotyledons were completely yellowed, while the first true leaf was yellow-green; 9: cotyledons and the first true leaf dried. (B) Frequency distribution of LTII in F2 populations characterized in March 2017 and April 2017.
A low-temperature injury index (LTII) was used as an indicator to indicate the LT tolerance of each plant. The formula used for the calculation of LTII refers to Wang et al. (2019): LTII = (0×S0+1×S1+3×S3+5×S5+7×S7+9×S9)/N×9. S0–S9 indicates the number of plants corresponding to each grade. N indicates the total number of plants. Three replicates were set for each treatment, and eight plants of each replicate were investigated. The LTII in two experiments was evaluated by two people, respectively.
Deoxyribonucleic Acid Extraction and Simple Sequence Repeats Marker Analysis
A modified CTAB (cetyltrimethylammonium ammonium bromide) procedure (Wang et al., 2006) was applied to extract genomic DNA from the second leaf of each two-leaf stage seedlings. The DNA concentration and quality was examined by electrophoresis on a 1% (w/v) agarose gel and NanoDrop ND-1000 spectrophotometer (NanoDrop Technologies, Wilmington, DE, USA). A total of 1,288 SSR markers designed based on the genome sequence of cucumber (Ren et al., 2009) were used to screen the polymorphism of the two parents. Then, a linkage map was constructed with selected polymorphic markers. PCR amplification and gel electrophoresis were conducted referring to the method of Song et al. (2016).
Linkage Map Construction and Quantitative Trait Locus Mapping
Polymorphic primers were used for genotyping F2 individuals. QTL analysis was performed with the R/QTL software package (http://www.rqtl.org/). Using individual plant data, a whole genome scan was performed to map the QTLs with composite interval mapping (CIM) procedures (Weng et al., 2015). Genome-wide logarithm of the odds (LOD), threshold values (P < 0.05) for declaring the presence of QTLs were determined using 1,000 permutations. For each detected QTL, a 2-LOD support interval was calculated and defined by left and right markers. The QTL naming format is the abbreviation of the character (i.e., low temperature tolerance—LTT), chromosome (Chr.) number and locus number.
Defining the Interval Containing the Major Quantitative Trait Locus Using In Silico Bulked Segregant Analysis
To narrow down the region harboring the major QTL, the association between SNPs and LT tolerance in core germplasm was studied using the in silico BSA strategy. The LT tolerance of 87 sequenced core germplasm (CG) seedlings was previously evaluated in our lab (Wang et al., 2019), two-leaf stage seedlings of CG lines were exposed to 12°C for 11 d and 19.3°C for 14 d, respectively. The LTT of each line was evaluated using the same method as that used to phenotype the F2:3 families described above. Five highly LT-resistant CG lines (“CG29,” “CG56,” “CG61,” “CG90,” “CG104”) and five highly LT-sensitive CG lines (“CG10,” “CG21,” “CG43,” “CG109,” “CG37”) were selected to generate LT-resistant and LT-sensitive bulks (Table S1, Figure S2). The 10 CG lines have different geographical origin, and were re-sequenced (Qi et al., 2013). The sequence data was retrieved from National Center for Biotechnology Information (NCBI) Short Read Archive (SRA; Leionen et al., 2011) under accession SRA056480 (https://www.ncbi.nlm.nih.gov/sra/?term=SRA056480). The detailed accession number of each CG line was listed in Table S1. Then, the SNPs located within the qLTT6.2. region (20,591,185 to 21,186,690 bp) were searched (Table S3) and imported into PilotEdit software. SNPs that were identical within each bulk while different between bulks, were considered to be associated with the LT tolerance.
Real-Time Quantitative Reverse Transcription Polymerase Chain Reaction Analysis
The parental lines at the two-leaf stage were exposed to 14 h light at 18°C and 10 h dark at 10°C, the second true leaf of each seedling was harvested at 0, 10, 24, 48, 96, 144, 216, 288, 384, and 480 h after LT exposure, respectively, and flash frozen in liquid nitrogen. Total RNA was isolated and the first-strand complementary DNA (cDNA) was synthesized. The qPCR primers were designed with Primer Premier 6.0 (http://www.premierbiosoft.com/primerdesign/index.html). The qRT-PCR was performed using SYBR Premix Ex Taq™ (Tli RNaseH Plus) (Takara #RR420Q, Takara Bio, Inc. China) in Roche Diagnostics with Light Cycler 480 System, and PCR amplification was conducted following the instructions. Actin1 (Csa3G806800) was used as the reference gene for the normalization of gene expression values (Wan et al., 2010). Each experiment was conducted with three biological replicates and three technical replicates. The relative expression data of candidate gene was analyzed using 2−ΔΔCt method (Kenneth et al., 2001).
Cloning and Sequence Analysis of Candidate Genes
The second true leaf of seedlings at the two-leaf stage was harvested for DNA extraction. Multiple pairs of primers were designed to amplify the full length candidate gene, and neighboring fragments have at least 100 bp overlap. Primers designed were listed in Table S4. PCR amplification was performed in a 25 µl reaction: 2.5 µl of 10X PCR buffer, 1 µl of 25X Mg2+, 1 µl of 10X nucleoside triphosphate, 1 µl of 10 µM forward and reverse primer, 0.25 µl of KOD FX polymerase (Toyobo, Osaka, Japan) and 250–500 ng of DNA. The PCR conditions were as follows: 94°C for 5 min, 30 cycles of (94°C for 40 s, 55°C for 50 s, 72°C for 90 s), 72°C for 10 min, and a final incubation at 4°C for use. The PCR products were then sequenced by Sangon Biotech (Beijing, China). The sequence of the candidate genes of the parental lines were analyzed using SeqMan, and the amino acid sequences were analyzed using the MEGA 6.0 software (Tamura et al., 2013). The promoter sequences of candidate genes were analyzed using the PlantCARE website (http://bioinformatics.psb.ugent.be/webtools/plantcare/html/), and the protein sequences of candidate genes were predicted by SMART software (Ponting et al., 1999).
Phylogenetic Analysis of Csa6G445210 and Csa6G445230
To further understand the relatedness of the Csa6G445210 and Csa6G445230 sequences, phylogenetic trees were generated. The predicted amino acid sequences encoded by Csa6G445210 and Csa6G445230 in cucumber and orthologues in Cucumis melon, Arabidopsis thaliana, Brassica rapa, Solanum lycopersicum, Oryza sativa cv. Japonica, and Zea mays were obtained using the BLASTP tool (Altschul et al., 1997) in the NCBI database. Multiple sequence alignment was implemented with ClustalW (Thompson et al., 1994) and the phylogenetic tree was structured using the MEGA 6.0 software (Tamura et al., 2013) with a neighbor-joining algorithm.
Allelic Diversity of the Candidate Gene in F2 Individuals
F2 individuals were used to identify the correlation between the phenotype (via F2:3 families) and the SNP mutation sites at the candidate genes. Specific SNP markers at mutation sites were designed (Table S5) to amplify DNA from F2 individuals, and then the amplified products were digested for genotyping. The individuals that produce the same digestion products as “CG104,” “CG37,” and F1 were defined as LT resistant (a), sensitive (b), and hybrid (ab), respectively. Then, the genotype and LTII value of each F2 individual were compared.
Statistical Analysis
All tests for significant differences between “CG104” and “CG37” were done using one-way ANOVA in the R environment (R core Team, 2019).
Results
Inheritance Analysis of Low-Temperature Tolerance in Cucumber Seedlings
To phenotype the LT tolerance of the two parental lines, and individuals in the F1 and F2:3 populations, 3-week-old seedlings were exposed to 15–17°C for 2 weeks in March 2017 and April 2017, respectively. The LTII was used to indicate the LT tolerance of each plant. Results showed that after 2 weeks of LT treatment, cotyledons and the first true leaf of “CG37” showed severe withering and yellowing, and the LTII score of “CG37” in greenhouse and plastic greenhouse were 59.1 and 57.7 respectively. However, “CG104” had no symptoms and the LTII were 14.9 and 8.7 respectively. The LTII of the F1 hybrids were 26.4 and 21.7, respectively, with the symptoms more inclined toward “CG104.” Frequency distribution of the LTII among F2:3 families obeyed a normal distribution (Figure 1 and Figure S1) in two experiments (the coefficient of correlation is 0.87). An additional experiment of phenotyping F1 and F1” showed that there is no maternal effect (Table S6), which all together indicate that LT tolerance of cucumber at the seedling stage is a quantitative trait, controlled by multiple nuclear genes.
Linkage Map Construction and Quantitative Trait Locus Mapping
A total of 1,288 cucumber SSR markers were used to screen the polymorphisms between the parental lines; 509 of them showed polymorphisms, with a polymorphism rate of 39.5%. A total of 190 markers with clear electrophoresis strips were used to generate a linkage map (Figure 2). The markers selected were evenly distributed on seven chromosomes. The total length of the genetic map was 990.8 cM, and the average genetic distance between markers was 5.2 cM (Table S7). Based on the “9930” genome (Huang et al., 2009), the order of all markers on the genetic map was consistent with their physical location, therefore the map was qualified to be used for subsequent QTL mapping.
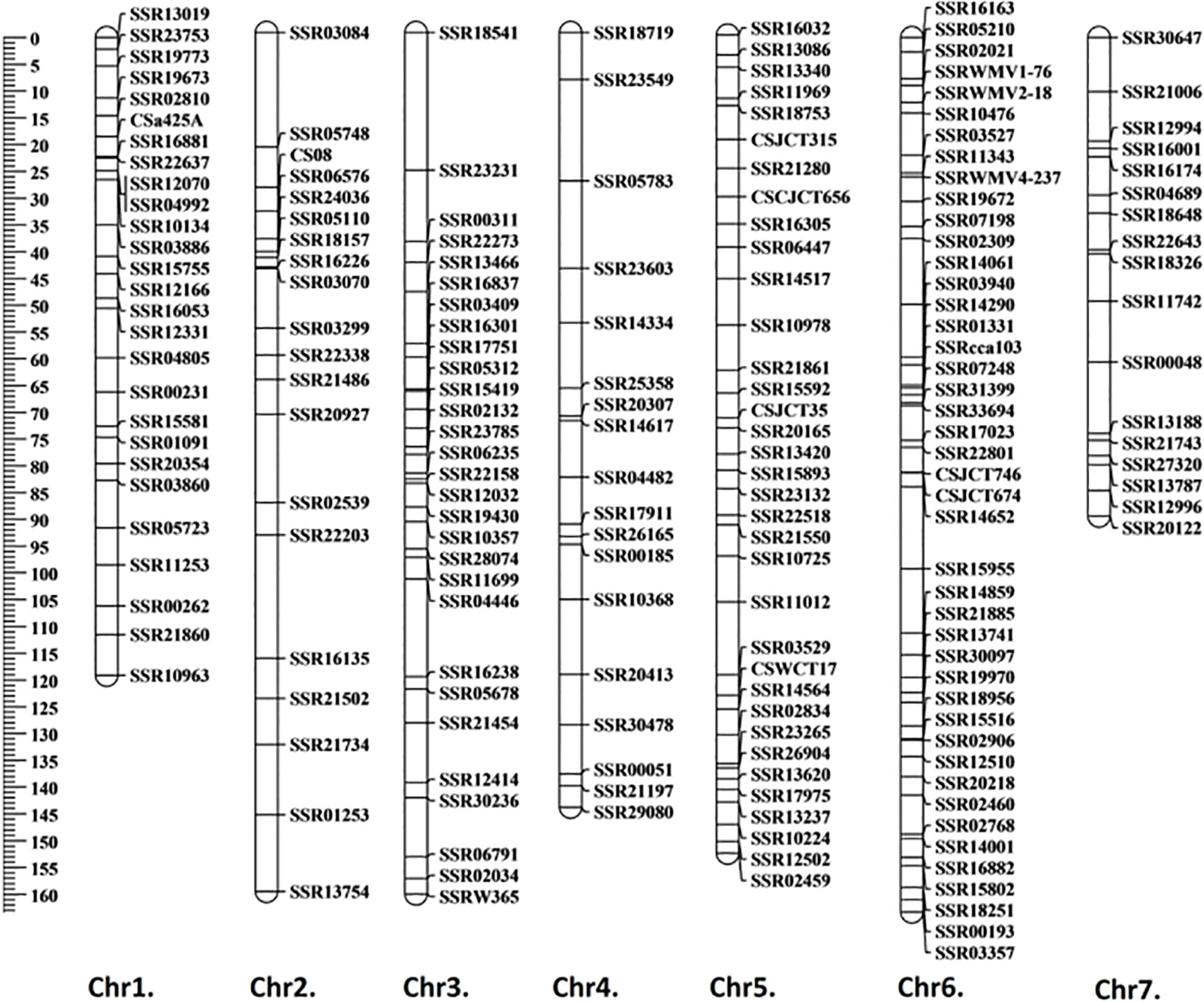
Figure 2 Genetic linkage map generated using F2 population. The generated linkage map contained 190 markers with a total length of 990.8 cM, and the average genetic distance between markers was 5.2 cM. Map distance was given in centimorgans (cM).
To detect QTL responsible for LT tolerance in cucumber seedlings, the phenotypic data for LT tolerance (LTII) from the two experiments and the genetic map constructed were used for QTL mapping. Details of each QTL detected, including map location, peak location, peak logarithm of odds (LOD) support value, confidence intervals, and percentages of total phenotypic variances explained (R2) were shown in Table 1. In total, three QTLs including qLTT5.1 on Chr.5, qLTT6.1 and qLTT6.2 on Chr.6 were repeatedly detected (with a threshold of 3, and the confidence intervals of 2-LOD) in two experiments (Table 1 and Figure 3). Furthermore, LOD scores of qLTT6.2 is 18.2 and 16.8, and accounts for 26.8 and 24.1% of the phenotypic variation. Therefore, it was proposed that qLTT6.2 is a major QTL which is responsible for LT tolerance in cucumber seedlings.

Table 1 Identified quantitative trait loci controlling low temperature tolerance in cucumber seedlings. LTT indicates low temperature tolerance.
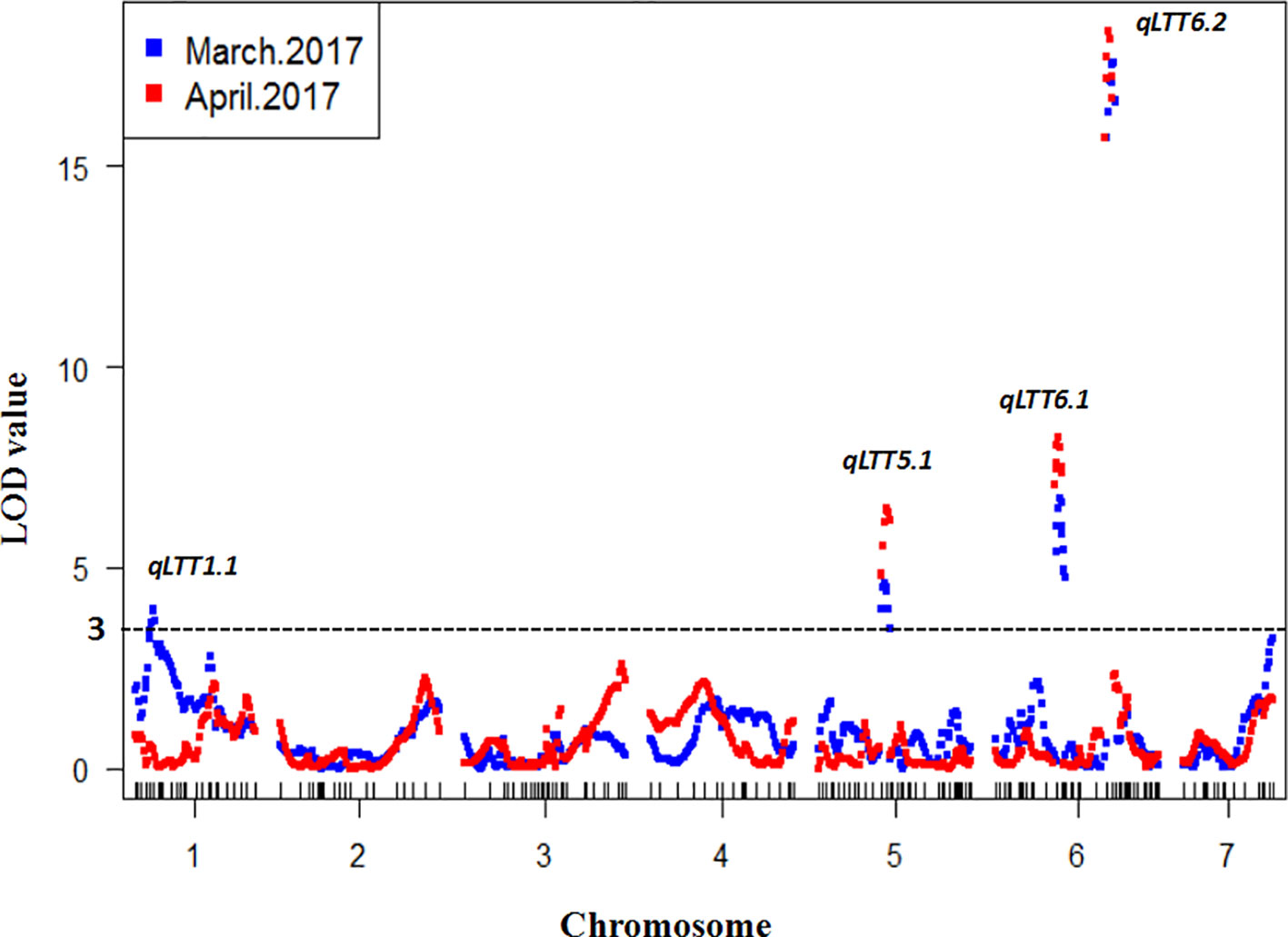
Figure 3 Quantitative trait locus (QTL) analysis of low temperature tolerance in cucumber seedlings. Blue squares indicates the first treatment and red square indicates the second treatment. The x-axis indicates the genetic position of each chromosome, the y-axis indicates the logarithm of the odds value. Four QTLs were detected in two experiments and three QTLs were repeatedly identified.
Reducing the Interval of Locus qLTT6.2 Using In Silico Bulked Segregant Analysis
Because qLTT6.2 showed the highest contribution to LT tolerance in two experiments, we further conducted in silico BSA to narrow the region harboring this locus. Our previous study included the LT tolerance evaluation of 87 CG lines (Figure S2). Among these, five lines: “CG29,” “CG56,” “CG61,” “CG90,” “CG104” with strong LT resistance phenotypes, and another five lines: “CG10,” “CG21,” “CG43,” “CG109,” “CG37,” with high LT sensitive phenotypes were used to generate LT-resistant and -sensitive bulks to identify the SNPs variation. The detailed information of these 10 lines including accession name, accession number in NCBI, geographical origin, and their LT tolerance performance are listed in Table S1. There were 214 non-synonymous SNPs that were identical within each bulk, but different between bulks (Table S3), all of which were distributed within the 42-kb region (20,779,616 to 20,821,620). These data indicated that this 42 kb region was associated with LTII variation among these lines. Annotation of the 42 kb genomic region predicted three genes including one for an auxin response factor, one for a CASP-like protein, and an A. thaliana ethylene insensitive 2 orthologue (Figure 4 and Table 2).
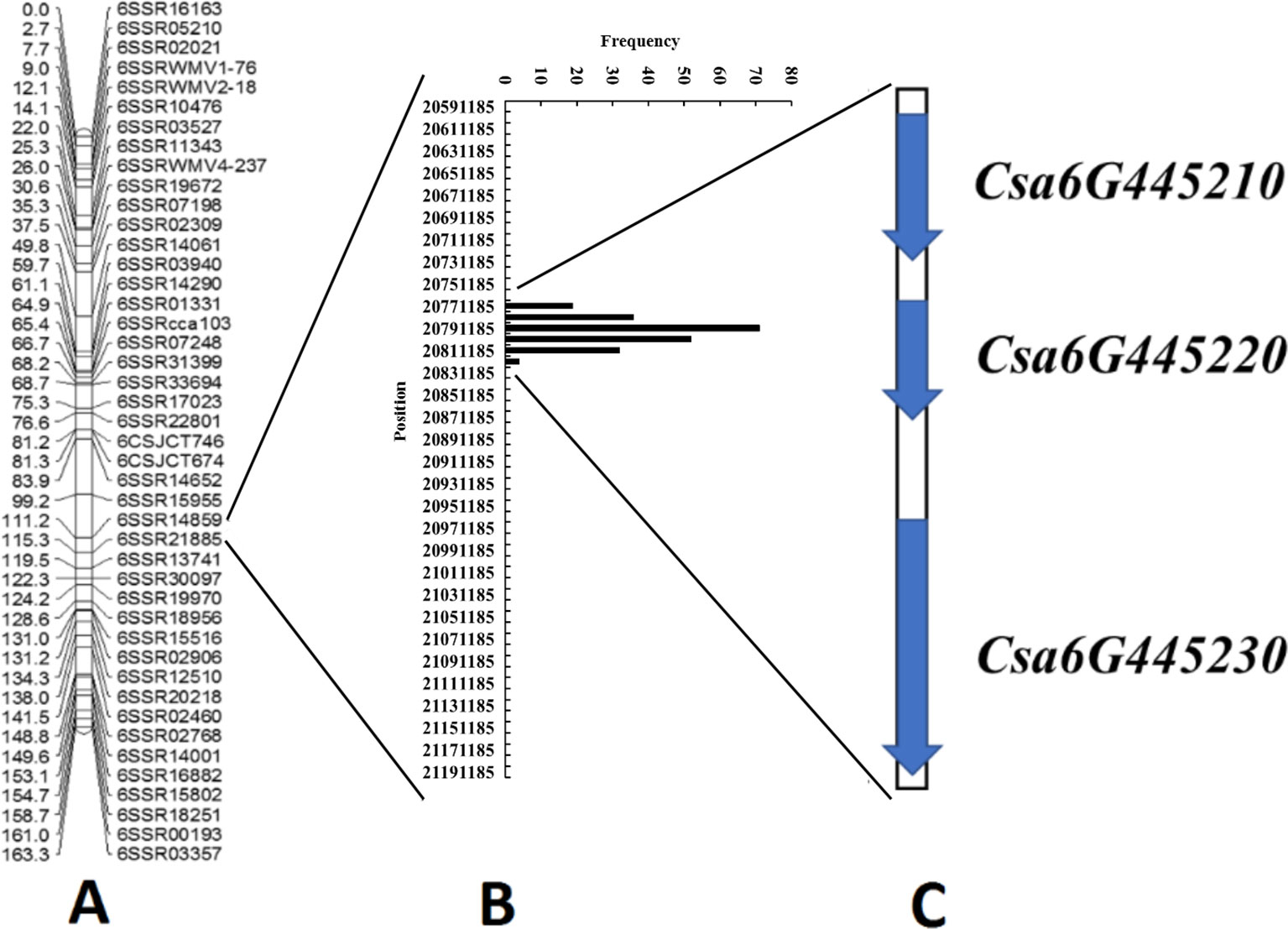
Figure 4 Identification of candidate genes for the qLTT6.2 locus through combined linkage mapping and association analysis. (A) Linkage map for qLTT6.2 locus on chromosome 6 based on 189 F2:3 families from “CG37” × “CG104.” (B) Distribution of 214 single-nucleotide polymorphisms in the “9930” cucumber genome, suggested that a 42-kb region was associated with the variation of low temperature tolerance in cucumber core germplasm materials. (C) Three candidate genes were identified in the 42-kb region.
Gene Expression Pattern Analysis of the Three Candidate Genes
To study the expression pattern of the three candidate genes under LT stress, leaf tissues of the two parental lines exposed to LT stress for 0, 10, 24, 48, 96, 144, 216, 288, 384, and 480 h were harvested, respectively, and then qRT-PCR of each gene was performed (Figure 5). The expression level of Csa6G445210 in “CG104” was significantly higher than that of “CG37” at most time-points, and Csa6G445230 showed a similar expression pattern. However, there was no significant expression difference of Csa6G445220 between the two parental lines. In short, Csa6G445220 did not respond to LT stress, however, both Csa6G445210 and Csa6G445230 were up-regulated by LT stress in “CG104.”
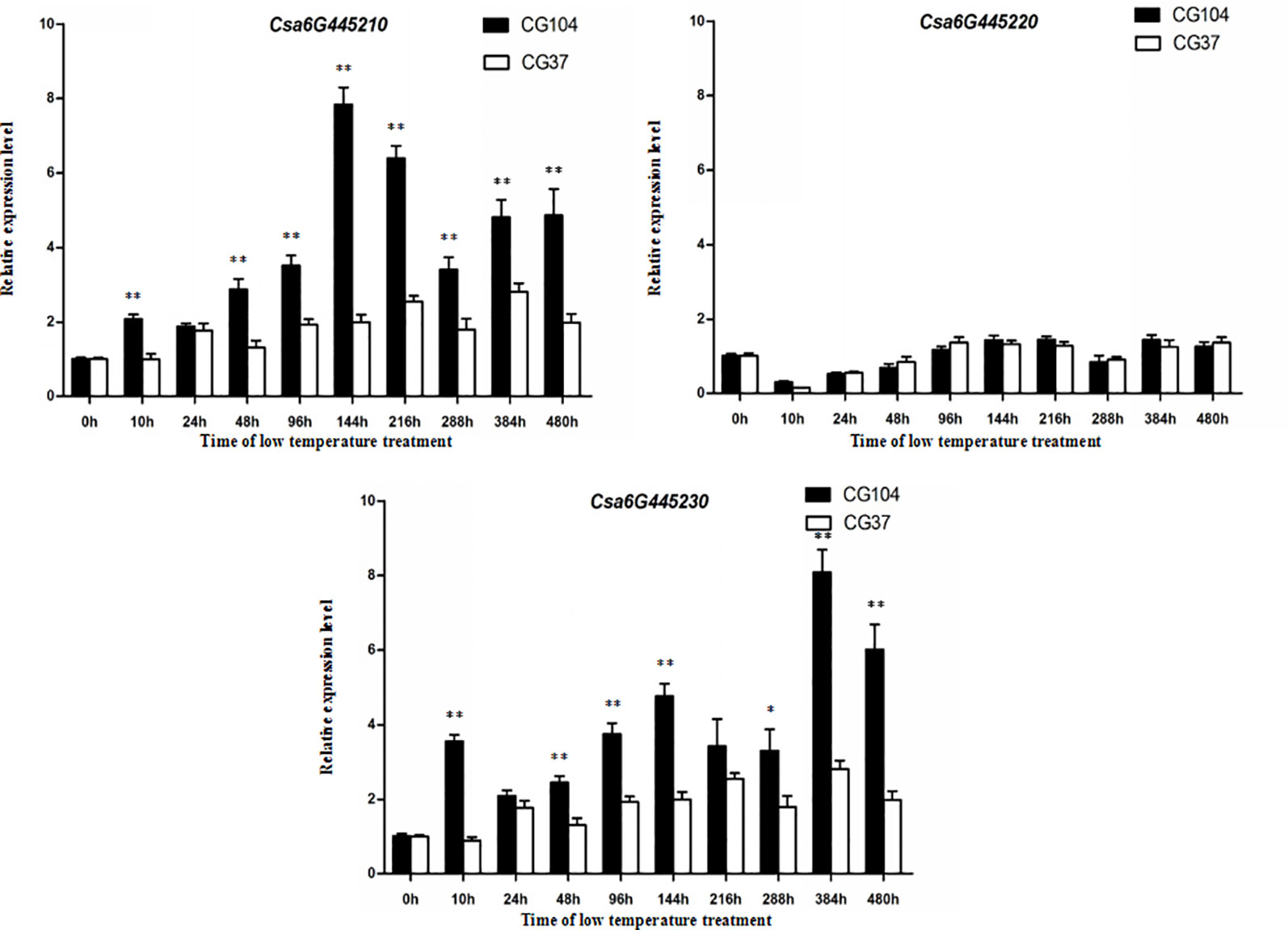
Figure 5 Expression analysis of three candidate genes. The fold-change indicates the relative amount of gene transcripts at different time-points compared to 0 h. The asterisks indicate that there were significant differences in transcript level between “CG104” and “CG37” (“*,” 0.01 < P < 0.05; “**,” 0.001 < P < 0.01; “***,”0 < P < 0.001).
Cloning and Sequence Analysis of Candidate Genes
To further analyze the sequence of Csa6G445210 and Csa6G445230, full-length DNA and cDNA of each gene in the two parental lines were sequenced and compared (Figure 6, Figures S3 and S4). The results showed that two base pair substitutions were detected within the coding sequence region (CDS) in Csa6M445210. The first substitution at position 1733 did not change the amino acid, however a polymorphism at position 1807 resulted in a non-synonymous change (Arg→Cys). Csa6M445230 has seven base pair substitutions within the CDS. Two substitutions were synonymous resulting in no amino acid change, while the rest were non-synonymous (Asp257→Ser, Thr820→Ser, Ser2468→Leu, Leu2678→Pro, Ser3260→Cys). The Asp257 resulted in changes in the transmembrane helix (Table S8).
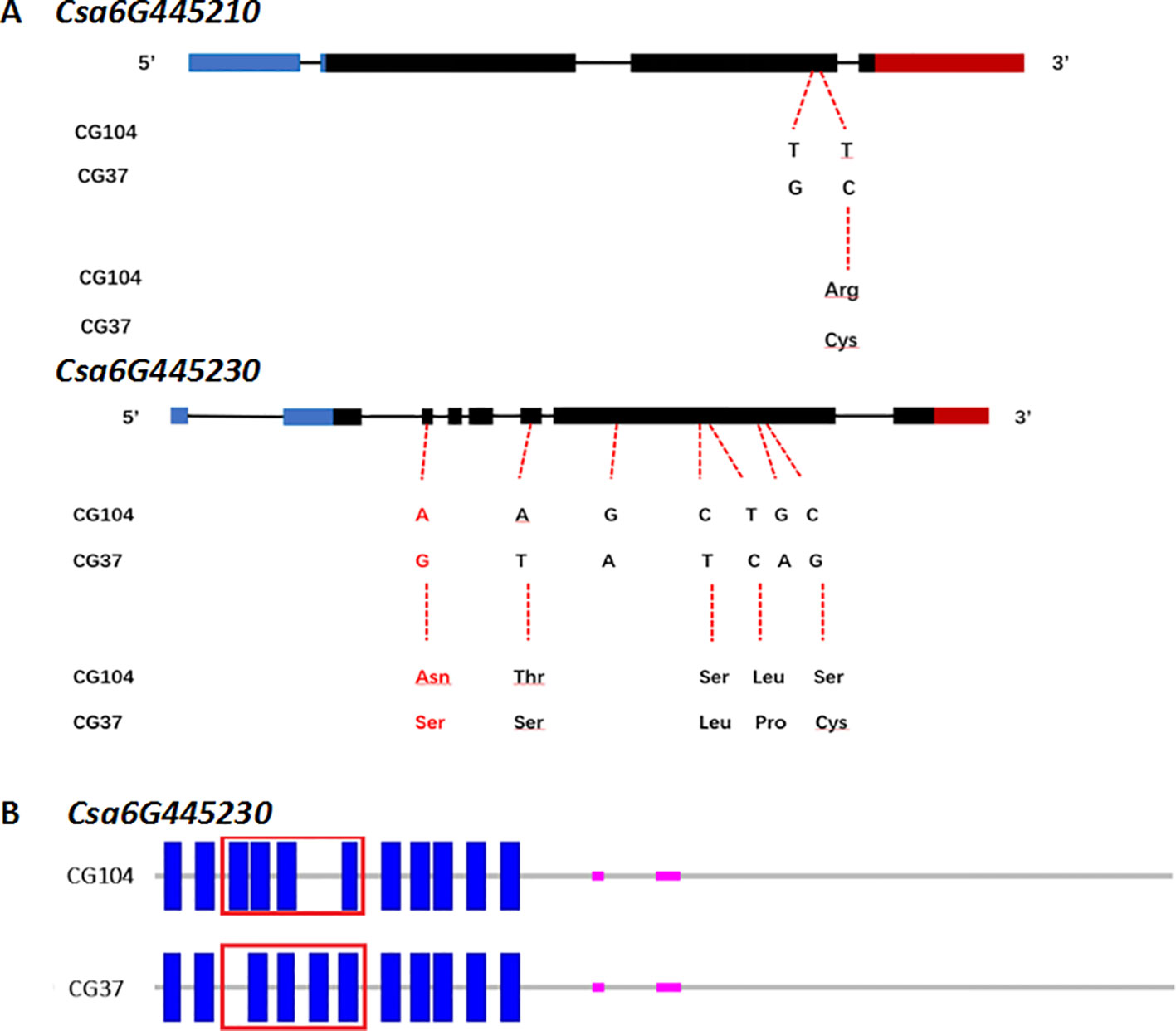
Figure 6 Sequence analysis of Csa6G445210 and Csa6G445230. (A) DNA and predicted amino acid changes between parental lines. Nucleic acid indicates the SNPs on exon of Csa6G445210 and Csa6G445230 between “CG104” and “CG37,” and the consequential amino acid changes. The amino acid in red leads to the changes of protein secondary structure. (B) The secondary structure of protein encoded by Csa6G445230 in “CG104” and “CG37.” The blue solid rectangle indicates the transmembrane helix. The red box indicates the different transmembrane structure between “CG104” and “CG37.”
The consistency between the LT tolerance phenotype, and the genotype of non-synonymous SNP sites within these two genes were examined in F2 population. Primers were designed based on the non-synonymous SNP site of Csa6G445210 and the first base substitution mutation site of Csa6G445230. By comparing the genotype and LTII value of each F2 individual, it was found that both non-synonymous SNP sites within Csa6G445210 and Csa6G445230 were associated with the LT tolerance phenotypes (Table S9).
Phylogenetic Analysis of Csa6G445210 and Csa6G445230
The gene annotation suggests that Csa6M445210 encodes an auxin response factor (ARF), which is a transcription factor that controls the expression of auxin responsive genes. Csa6M445230 encodes a transmembrane protein, ethylene-insensitive 2 (EIN2). To further predict and analyze the function of these two predicted amino acids, the sequences analyzed by BLAST against the NCBI database, and phylogenetic analyses were performed with their orthologues in seven other species (Figure 7). The results showed that the homologs of protein encoded by Csa6M445210 and Csa6G445230 are highly conserved in A. thaliana and other plant species, which suggests that they may share similar functions.
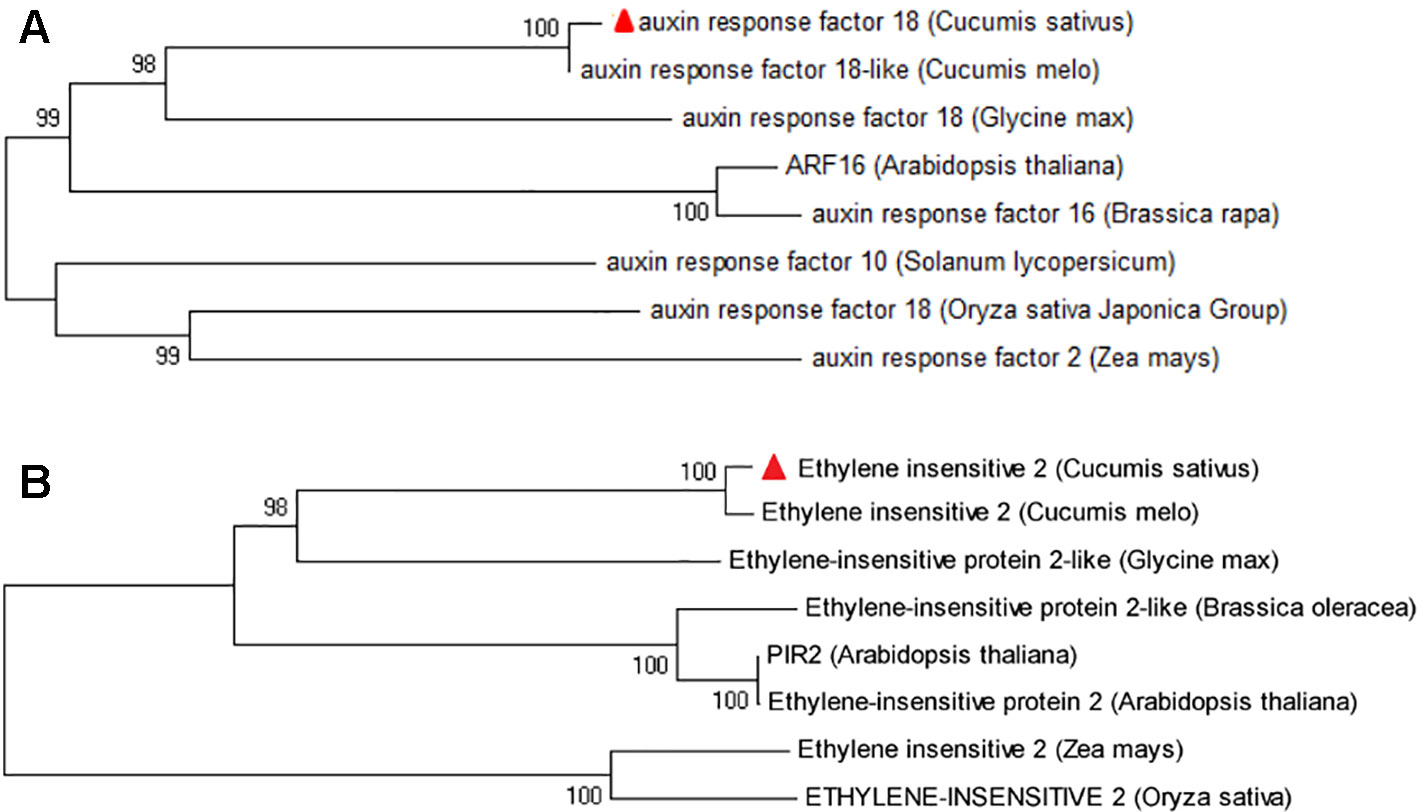
Figure 7 Phylogenetic tree of protein encoded by Csa6G445210 and Csa6G445230. The protein encoded by cucumber Csa6G445210 (A) or Csa6G445230 (B) is shown as a red triangle; the branches refer to the rates of amino acid variation.
Discussions
In our study, the LT stress treatments were carried out under greenhouse conditions in early spring, a natural LT environment in cucumber production. Using the yellowing degree of the first true leaf and the cotyledons as indicators, three QTLs were repeatedly identified on chromosome 5 and 6. The major QTL qLTT6.2 was mapped into a 595-kb interval between markers SSR14859 and SSR21885 with LODs support score of 18.2 and 16.8, and explained 26.8 and 24.1% of the observed phenotypic variations. Several studies on QTL mapping of LT-tolerance in cucumber seedlings have been reported. However, the results differed due to variation in the developmental stage of the plant materials used, differences in treatment conditions including location (environmental controlled facility or field) and temperature (12 or 4°C), and different evaluation standards (degree of yellowing, area of dehydration spot, or area of dryness after recovery). Li (2014) used a CII as indicator, identified a marker SSR07248 that was closely linked to a LT-tolerant locus on Chr.6. Zhou (2015) identified six QTLs associated wtih LT tolerance on chromosome 3, and Wang (2014) identified three QTLs on chromosome 3 and one QTL on chromosome 7 using CII and a recovery index as indicators of LT-stress. However, the flanking markers were not close enough, and the contribution rate of the QTLs was low in above studies. SSR01331, the flanking maker of qLTT6.1 identified in our study, was only 233 kb away from SSR07248 identified in the previous study (Li, 2014). The relationship between these two loci needs to be further investigated.
“In silico BSA” is an effective method to discover or refine markers linked to target genes or QTLs, by inspecting SNP variation among genotyped individuals at specific genomic regions. It has been used successfully in soybean to map virus resistant genes (Bello et al., 2014). With this method in cucumber, Li et al. (2016) quickly narrowed the interval around the gene controlling carpel number from a 1.9 Mb region down to 16 kb. Bo et al. (2019) also used this method to fine-map the fruit spine density related major QTL (qfsd6.2) to a 50 kb region, and finally identified the candidate gene Csgl3 for the trait. In our study, the phenotypic data obtained in two replicated experiments were consistent, and the genotype sequencing depth reached 30 X, therefore this method was employed to narrow the target region. Based on the sequence data and LT-tolerance performance of CG, the genomic region of qLTT6.2 was delimited to a 42-kb region, which only contained three candidate genes. Gene sequence alignments revealed that there were amino acid substitutions in both Csa6G445210 and Csa6G445230. Furthermore, allelic diversity of the candidate gene region in F2 individuals showed that the two markers based on the non-synonymous SNP sites within Csa6G445210 and Csa6G445230 were both associated with the phenotypes of F2 individuals. Therefore, our study demonstrated that the in silico BSA approach can accurately identify SNPs linked to LT tolerance trait in cucumber.
LT is one of the major abiotic stresses that affect plant growth, development, and productivity. The mechanisms plants have evolved to adapt to LT stress have been well-studied over the past two decades (Ding et al., 2019), however, little is known in cucumber. In our study, two candidate genes Csa6G445210 and Csa6G445230 that might be involved in LT-stress response in cucumber seedlings were identified. Csa6G445230 encodes EIN2 which is a key regulator in ethylene signaling pathway, and Csa6G445210 encodes ARF which is responsive to auxin. The role of ethylene in abiotic stress resistance including cold responses was established (Yoo et al., 2009; Shi et al., 2012). In Arabidopsis, EIN2 is a positive regulator of the ethylene signaling pathway, indirectly inhibiting the expression of CBFs, which is achieved by the downstream transcription factor EIN3/EILs (Guo and Ecker, 2003; An et al., 2010; Shi et al., 2012; Li et al., 2015). CBFs could quickly activate the set of downstream cold-responsive genes COR (i.e., cold regulated genes), to improve plant cold tolerance (Gilmour et al., 1998; Medina et al., 1999; Miura and Furumoto, 2013; Barrero-Gil and Salinas, 2017; Shi et al., 2018). However, whether ethylene has a positive or negative effect on cold tolerance might vary among plant species; ethylene may positively regulate a low-temperature response in chilling-sensitive species (Eremina et al., 2016). In A. thaliana, ethylene content decreased when exposed to LT, and the loss-of-function mutant ein2–5 was more resistant to LT stress than the wild-type plants, which indicates that ethylene negatively regulates LT stress response in Arabidopsis (Shi et al., 2012). The same conclusions were drawn in other LT-tolerant plants such as Medicago sativa (Zhao et al., 2014) and Triticum aestivum (Field, 1984). However, in fruit of C. sativus (Wang and Adams, 1982) and S. lycopersicum (Zhao et al., 2009), studies showed that ethylene content increased during rewarming stage after LT treatment. Whether ethylene content increased during LT treatment in leaves is yet unknown. In addition, CsEIN2 was up-regulated in the LT-tolerant line in our study, we thus speculate that CsEIN2 might positively regulate CBF in cucumber seedlings, which deserves further investigation. Although auxin is a master regulator of plant growth and development, little is known regarding its role in cold stress response. Aux/IAA binds to ARF (auxin-responsive factor) and prevents the ARF-mediated expression of downstream genes (Lavy and Estelle, 2016). A recent study showed that several DREB/CBFs genes regulate the Aux/IAA genes directly, which demonstrate that the cold responsive pathway interacts with the auxin gene regulatory network (Shani et al., 2017). However, if and how Csa6G445210 regulates aspects of the cold tolerance signaling pathway should be determined.
Data Availability Statement
The accession numbers of the ten core germplasm lines used in our study can be found in Table S1. The link of the sequence data in NCBI Short Read Archive (SRA) SRA056480 is as follows: (https://www.ncbi.nlm.nih.gov/sra/?term=SRA056480). All SNPs within the 42 kb target region can be found in Table S3.
Author Contributions
WW and SW conducted the experiments and analyzed the data. SD, WW and KB analyzed the data and drafted the manuscript. ZS helped collect the data. HM helped analyze the data. XG and SZ designed the experiments.
Funding
This research was supported by National Key Research and Development Program of China (2018YFD1000800), the Earmarked Fund for Modern Agro-industry Technology Research System (CARS-25), Science and Technology Innovation Program of the Chinese Academy of Agricultural Science (CAAS-ASTIP-IVFCAAS), Key Laboratory of Biology and Genetic Improvement of Horticultural Crops, Ministry of Agriculture, P.R. China, and Central Public-Interest Scientific Institution Basal Research Fund (No.Y2017PT52).
Conflict of Interest
The authors declare that the research was conducted in the absence of any commercial or financial relationships that could be construed as a potential conflict of interest.
Acknowledgments
We thank the reviewers for their insightful comments which improved the manuscript. Dr. Diane M. Beckles and Dr. Karin Albornoz are acknowledged for their helpful input on the manuscript.
Supplementary Material
The Supplementary Material for this article can be found online at: https://www.frontiersin.org/articles/10.3389/fpls.2019.01620/full#supplementary-material
References
Altschul, S. F., Madden, T. L., Schäffer, A. A., Zhang, J., Zhang, Z., Miller, W., et al. (1997). Gapped BLAST and PSI-BLAST: a new generation of protein database search programs. Nucl. Acids Res. 25 (17), 3389–3402. doi: 10.1093/nar/25.173389
An, F., Zhao, Q., Ji, Y., Li, W., Jiang, Z., Yu, X., et al. (2010). Ethylene-induced stabilization of ETHYLENE INSENSITIVE3 and EIN3-LIKE1 is mediated by proteasomal degradation of EIN3 binding F-box 1 and 2 that requires EIN2 in Arabidopsis. Plant Cell 22 (7), 2384–2401. doi: 10.1105/tpc.110.076588
Barrero-Gil, J., Salinas, J. (2017). CBFs at the crossroads of plant hormone signaling in cold stress response. Mol. Plant 10 (4), 542–544. doi: 10.1016/j.molp.2017.03.004
Bello, M. H., Moghaddam, S. M., Massoudi, M., McClean, P. E., Cregan, P. B., Miklas, P. N. (2014). Application of in silico bulked segregant analysis for rapid development of markers linked to bean common mosaic virus resistance in common bean. BMC Genomics 15, 903. doi: 10.1186/1471-2164-15-903
Bo, K., Miao, H., Wang, M., Xie, X., Song, Z., Xie, Q., et al. (2019). Novel loci fsd6. 1 and Csgl3 regulate ultra-high fruit spine density in cucumber. Theor. Appl. Genet. 132 (1), 27–40. doi: 10.1007/s00122-018-3191-6
Cabrera, R. M., Saltveit, M.E., Jr., Owens, K. (1992). Cucumber cultivars differ in their response to chilling temperatures. J. Am. Soc. Hortic. Sci. 117, 802–807. doi: 10.21273/JASHS.117.5.802
Chen, B., Saltveit, M. E., Beckles, D. M. (2019). Chilling-stress modifies DNA methylation level in cucumber (Cucumis sativus L.) seedling radicle to regulate elongate rate. Sci. Hortic. 252, 14–19. doi: 10.1016/j.scienta.2019.03.023
Chung, S. M., Staub, J. E., Fazio, G. (2003). Inheritance of chilling injury: a maternally inherited trait in cucumber. J. Am. Soc. Hort. Sci. 128, 526–530. doi: 10.1111/nph.15696
Ding, Y., Shi, Y., Yang, S. (2019). Advances and challenges in uncovering cold tolerance regulatory mechanisms in plants. New Phytol. 222 (4), 1690–1704. doi: 10.1007/s00018-015-2089-6
Eremina, M., Rozhon, W., Poppenberger, B. (2016). Hormonal control of cold stress responses in plants. Cell Mol. Life Sci. 73 (4), 797–810. doi: 10.1093/oxfordjournals.aob.a086774
Field, R. J. (1984). The role of 1-aminocyclopropane-1-carboxylic acid in the control of low temperature induced ethylene production in leaf tissue of Phaseolus vulgaris L. Ann. Bot. 54, 61–67. doi: 10.1046/j.1365-313x.1998.00310.x
Gilmour, S. J., Zarka, D. G., Stockinger, E. J., Salazar, M. P., Houghton, J. M., et al. (1998). Low temperature regulation of the Arabidopsis CBF family of AP2 transcriptional activators as an early step in cold-induced COR gene expression. Plant J. 16, 433–442. doi: 10.1016/S0092-8674(03)00969-3
Guo, H., Ecker, J. R. (2003). Plant responses to ethylene gas are mediated by SCF(EBF1/EBF2)-dependent proteolysis of EIN3 transcription factor. Cell 115, 667–677. doi: 10.1006/meth.20011262
Huang, S., Li, R., Zhang, Z., Li, L., Gu, X., et al. (2009). The genome of the cucumber, Cucumis sativus L. Nat. Genet. 41, 1275–1281. doi: 10.1038/ng.475
Kenneth, J. L., Thomas, D. S., Livak, K. J., Schmittgen, T. D. (2001). Analysis of relative gene expression data using real-time quantitative PCR and the 2–ΔΔCt method. Methods 25, 402–408. doi: 10.21273/JASHS.133.2.225
Kozik, E. U., Wehner, T. C. (2008). A single dominant gene Ch for chilling resistance in cucumber seedlings. J. Am. Soc. Hort. Sci. 133, 225–227. doi: 10.1093/nar/gkq1019
Kozik, E. U., Klosinska, U., Wehner, T. C. (2012). Inheritance of low temperature seed germination ability in cucumber. In: Proc. of 10th Eucarpia International Meeting on Genetics and Breeding Cucurbitaceae, 575–578. doi: 10.1242/dev.131870.
Lavy, M., Estelle, M. (2016). Mechanisms of auxin signaling. Development 143 (18), 3226–3229. doi: 10.1007/s00122-016-2679-1
Leionen, R., Sugawara, H., Shumway, M. (2011). International nucleotide sequence database collaboration. The sequence read archive. Nucleic Acids Res. 39, D19–D21. doi: 10.1016/j.cell.2015.09.037
Li, W., Ma, M., Feng, Y., Li, H., Wang, Y., Ma, Y., et al. (2015). EIN2-directed translational regulation of ethylene signaling in arabidopsis. Cell 163, 670–683. doi: 10.1104/pp.119.2.463
Li, S., Pan, Y., Wen, C., Li, Y., Liu, X., Zhang, X., et al. (2016). Integrated analysis in bi-parental and natural populations reveals CsCLAVATA3 (CsCLV3) underlying carpel number variations in cucumber. Theor. Appl. Genet. 129, 1007–1022. doi: 10.1007/s00122-016-2679-1
Li, H. (2014). Genetic analysis and linked marker selection of low temperature tolerance in cucumber. [master's thesis]. Shanghai: Shanghai JiaoTong University. doi: 10.3390/ijms14035312.
Medina, J., Bargues, M., Terol, J., Pérez-Alonso, M., Salinas, J. (1999). The Arabidopsis CBF gene family is composed of three genes encoding AP2 domain-containing proteins whose expression is regulated by low temperature but not by abscisic acid or dehydration. Plant Physiol. 119, 463–469. doi: 10.1093/nar/27.1.229
Miura, K., Furumoto, T. (2013). Cold signaling and cold response in plants. Int. J. Mol. Sci. 14, 5312–5337. doi: 10.1038/ng2801
Ponting, C. P., Schultz, J., Milpetz, F., Bork, P. (1999). SMART: identification and annotation of domains from signalling and extracellular protein sequences. Nucleic Acids Res. 27 (1), 229–232. doi: 10.1371/journal.pone.0005795
Qi, J. J., Liu, X., Shen, D., Miao, H., Xie, B., et al. (2013). A genomic variation map provides insights into the genetic basis of cucumber domestication and diversity. Nat. Genet. 45, 1510–1515. doi: 10.1016/j.cub.2016.12.016
R Core Team (2019). R: A language and environment for statistical computing. R Foundation for Statistical Computing. (Vienna, Austria). https://www.R-project.org/.
Ren, Y., Zhang, Z., Liu, J., Staub, J. E., Han, Y., et al. (2009). An integrated genetic and cytogenetic map of the cucumber genome. PloS One 4, e5795. doi: 10.1093/nar/22.224673
Shani, E., Salehin, M., Zhang, Y., Sanchez, S. E., Doherty, C., Wang, R., et al. (2017). Plant stress tolerance requires auxin-sensitive Aux/IAA transcriptional repressors. Curr. Biol. 27 (3), 437–444. doi: 10.1016/j.tplants.2018.04.002
Shi, Y., Tian, S., Hou, L., Huang, X., Zhang, X., Guo, H., et al. (2012). Ethylene signaling negatively regulates freezing tolerance by repressing expression of CBF and Type-A ARR Genes in Arabidopsis. Plant Cell. 24, 2578–2595. doi: 10.1371/journal.pone.0167845
Shi, Y., Ding, Y., Yang, S. (2018). Molecular regulation of CBF signaling in cold acclimation. Trends Plant Sci. 23 (7), 623–637. doi: 10.1023/A:1003084821178
Smeets, L., Wehner, T. C. (1997). Environmental effects on genetic variation of chilling resistance in cucumber. Euphytica 97, 217–225. doi: 10.1093/molbev/mst197
Song, Z. C., Miao, H., Zhang, S., Wang, Y., Zhang, S. P., Gu, X. F. (2016). Genetic analysis and QTL mapping of fruit peduncle length in cucumber (Cucumis sativus L.). PloS One 11, e0167845. doi: 10.1007/BF00227388
Tamura, K., Stecher, G., Peterson, D., Filipski, A., Kumar, S. (2013). MEGA6: molecular evolutionary genetics analysis version 6.0. Mol. Biol. Evol. 30 (12), 2725–2729. doi: 10.1016/j.ab.2009.12.008
Thompson, J. D., Higgins, D. G., Gibson, T. J. (1994). CLUSTAL W: improving the sensitivity of progressive multiple sequence alignment through sequence weighting, position-specific gap penalties and weight matrix choice. Nucleic Acids Res. 22 (22), 4673–4680. doi: 10.1105/tpc.112.098640
Wan, H., Zhao, Z., Qian, C., Sui, Y., Malik, A. A., Chen, J. (2010). Selection of appropriate reference genes for gene expression studies by quantitative real-time polymerase chain reaction in cucumber. Anal. Biochem. 399, 257–261. doi: 10.1016/j.ab.2009.12.008
Wang, C. Y., Adams, D. O. (1982). Chilling-induced ethylene production in cucumbers (Cucumis sativus L.). Plant Physiol. 69, 424–427.
Wang, H. J., Wu, Y., Gu, W., Sun, X. D., Qin, Z. W. (2006). Extraction of DNA from cucumber by improved CTAB method. Heilongjiang Agric. Sci. 5, 124–125.
Wang, W. P., Song , Z.C., B. O. K. L., Dong, S. Y., Wei, S., Miao, H., Li, J. B., et al. (2019). Evaluation and genome-wide association study (GWAS) of low-temperature tolerance at seedling stage in cucumber core germplasm. J. Plant Genet. Resour. 20 (6), 1606–1612. doi: 10.13430/j.cnki.jpgr.20190225001
Wang, H. (2014). Evaluation of low temperature tolerance and QTL mapping in cucumber germplasm. [Doctoral dissertation]. Beijing: Chinese Academy of Agriculture Sciences. doi: 10.1007/s00122-015-2544-7.
Weng, Y., Colle, M., Wang, Y., Yang, L., Rubinstein, M., Sherman, A., et al. (2015). QTL mapping in multiple populations and development stages reveals dynamic QTL for fruit size in cucumbers of different market classes. Theor. Appl. Genet. 128, 1747–1763. doi: 10.1016/j.febslet.2009.09.029
Yoo, S. D., Cho, Y., Sheen, J. (2009). Emerging connections in the ethylene signaling network. Trends Plant Sci. 14, 270–279. doi: 10.1111/ppl.12161
Zhao, D., Shen, L., Fan, B., Yu, M., Zheng, Y., et al. (2009). Ethylene and cold participate in the regulation of LeCBF1 gene expression in postharvest tomato fruits. FEBS Lett. 583, 3329–3334. doi: 10.1016/j.febslet.2009.09.029
Zhao, M., Liu, W., Xia, X., Wang, T., Zhang, W. H. (2014). Cold acclimation-induced freezing tolerance of Medicago truncatula seedlings is negatively regulated by ethylene. Physiol. Plant 152, 115–129. doi: 10.1111/ppl.12161
Keywords: cucumber, low temperature tolerance, quantitative trait locus mapping, in silico bulked segregant analysis, candidate gene analysis
Citation: Dong S, Wang W, Bo K, Miao H, Song Z, Wei S, Zhang S and Gu X (2019) Quantitative Trait Loci Mapping and Candidate Gene Analysis of Low Temperature Tolerance in Cucumber Seedlings. Front. Plant Sci. 10:1620. doi: 10.3389/fpls.2019.01620
Received: 24 April 2019; Accepted: 18 November 2019;
Published: 11 December 2019.
Edited by:
Jordi Garcia-Mas, Institute of Agrifood Research and Technology (IRTA), SpainReviewed by:
Hiroshi Ezura, University of Tsukuba, JapanSanghyeob Lee, Sejong University, South Korea
Copyright © 2019 Dong, Wang, Bo, Miao, Song, Wei, Zhang and Gu. This is an open-access article distributed under the terms of the Creative Commons Attribution License (CC BY). The use, distribution or reproduction in other forums is permitted, provided the original author(s) and the copyright owner(s) are credited and that the original publication in this journal is cited, in accordance with accepted academic practice. No use, distribution or reproduction is permitted which does not comply with these terms.
*Correspondence: Xingfang Gu, Z3V4aW5nZmFuZ0BjYWFzLmNu; Shengping Zhang, emhhbmdzaGVuZ3BpbmdAY2Fhcy5jbg==
†These authors have contributed equally to this work