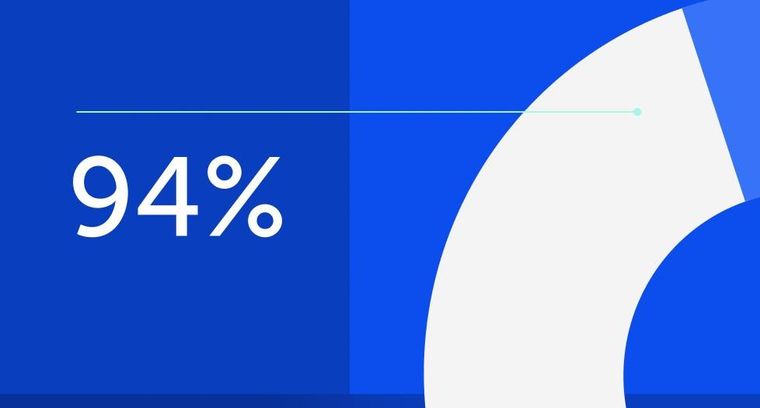
94% of researchers rate our articles as excellent or good
Learn more about the work of our research integrity team to safeguard the quality of each article we publish.
Find out more
ORIGINAL RESEARCH article
Front. Plant Sci., 28 November 2019
Sec. Plant Development and EvoDevo
Volume 10 - 2019 | https://doi.org/10.3389/fpls.2019.01565
Introduction: The Cape Floristic Region (CFR) is a biodiversity hotspot, recognized globally for its unusually high levels of endemism. The origins of this biodiversity are a long-standing topic of research. The largest “Cape clade,” Erica, radiated dramatically in the CFR, its ca. 690 species arising within 10–15 Ma. Notable between- and within-species flower color variation in Erica may have contributed to the origins of species diversity through its impact on pollinator efficiency and specificity.
Methods: We investigate the expression and function of the genes of the anthocyanin biosynthesis pathway that controls floral color in 12 Erica species groups using RT-qPCR and UPLC-MS/MS.
Results: Shifts from ancestral pink- or red- to white- and/or yellow flowers were associated with independent losses of single pathway gene expression, abrogation of the entire pathway due to loss of the expression of a transcription factor or loss of function mutations in pathway genes.
Discussion: Striking floral color shifts are prevalent amongst the numerous species of Cape Erica. These results show independent origins of a palette of mutations leading to such shifts, revealing the diverse genetic basis for potentially rapid evolution of a speciation-relevant trait.
The Cape Floristic Region (CFR) at the southern tip of Africa is a globally recognized biodiversity hotspot (Mittermeier et al., 2004). It has levels of species richness not normally seen outside of the tropics, with ca.10,000 plant species of which ca. 7,000 are endemic to the region (Linder, 2003). Which factors have shaped and driven this spectacular radiation has been a long-standing question in evolutionary biology. The largest clade in the CFR is that of Cape Erica (Linder, 2003; Pirie et al., 2018). The ca. 690 species represent an endemic radiation (Pirie et al., 2016), with species of Erica found throughout the CFR, making it an ideal model system to study these forces.
The genus Erica (Ericaceae) is a group of acidophilic, woody shrubs. It originated in Europe (Mcguire and Kron, 2005; Mugrabi De Kuppler et al., 2015) and migrated southwards through Africa, colonizing the mountains of Central Africa, the Indian Ocean islands, the Drakensberg mountains of Southern Africa and the CFR at the southern tip of South Africa where colonization appears to have been followed by rapid radiation (Mcguire and Kron, 2005; Mugrabi De Kuppler et al., 2015; Pirie et al., 2016, Pirie et al., 2018). Most Erica species are found in the CFR, where ca. 690 of the ca. 865 species in the genus occur (Oliver, 1989; Oliver, 2000; Linder, 2003). Diversification rates, as well as ancestral areas and patterns of character evolution of Erica have been inferred based on phylogenies of nuclear and plastid DNA sequence markers representing up to around 60% of known species (Pirie et al., 2011; Mugrabi De Kuppler et al., 2015; Pirie et al., 2016). Speciation within the Cape clade was particularly rapid (Pirie et al., 2016), and this may be linked to the complex patterns of morphological variation and regional endemism displayed by Erica species and populations distributed across the mountainous landscape of the CFR. During the radiation of the Cape Erica clade, floral morphology and floral color shifted numerous times (Pirie et al., 2011; Van der Niet et al., 2014; Le Maitre, 2017). Shifts between distinct pollination syndromes (suites of characteristics that promote specific vectors such as different species of insects and birds), could have resulted in gene flow restrictions between populations ultimately driving speciation (Pirie et al., 2011). Floral color shifted from the plesiomorphic (ancestral) pink most common in the older, European, lineages (Mugrabi De Kuppler et al., 2015), that are primarily insect pollinated, to white, green, red, and yellow, (Le Maitre, 2017), notably in the Cape where these colors are associated with a greater diversity of pollinators, including sunbirds (Rebelo et al., 1985).
In angiosperms, floral color plays a major role in pollinator specificity and changes in color may result in pollinator shifts and pollinator mediated speciation (Waser, 1998; Rausher, 2008; Carlson and Holsinger, 2013; Van Der Niet et al., 2014). Flower colors are determined by three major classes of pigments: anthocyanin pigments formed by the anthocyanin biosynthesis pathway; (Mol et al., 1998; Grotewold, 2006), carotenoids; and betalains (reviewed in Tanaka et al., 2008). Crowden and Jarman (1976) analysed flower pigments in a number of Erica species using paper chromatography. They did not report carotenoids or betalains, but documented several different anthocyanins: pelargonidin-hexose, cyanidin-hexose, peonidin-hexose, delphinidin-hexose, petunidin-hexose and malvidin-hexose with cyanidin-hexose the most prevalent (Crowden and Jarman, 1976).
Six enzymes form the primary anthocyanin biosynthesis pathway (Figure 1), beginning with chalcone synthase (CHS), followed by chalcone isomerase (CHI), flavanone hydroxylase (F3H), dihydroflavanol-4-reductase (DFR), anthocyanin synthase (ANS) and lastly, UDP 3-O-gylcosyltransferase (UDP-GST) to synthesize pelargonidin 3-hexose. Two additional enzymes, flavonoid 3′ hydroxylase (F3′H) and flavonoid 3′, 5′ hydroxylase (F3′,5′H), can also further hydroxylate the product of F3H, resulting in the production of cyanidin 3-hexose and delphinidin 3-hexose respectively. Methylation of cyanidin–hexose produces peonidin–hexose, whilst methylation of delphinidin–hexose produces petunidin–hexose and/or malvidin–hexose. Intermediates in the pathway may be converted by flavanol synthase (FLS) or leucoanthocyanidin reductase (LAR) into flavanols or leucoanthocyanidins respectively (Wessinger and Rausher, 2012). The pathway is regulated by a complex of three transcription factors, WDR, MYB and bHLH in Ipomoea purpurea, and is conserved across angiosperms (Zhu et al., 2015). The complex of these three transcription factors binds to two highly conserved sites upstream of the 5′ UTR of each of the genes in the pathway, with MYB binding to the MYB recognition element (MRE) and bHLH binding to the bHLH recognition element (BRE), providing for unified control of the pathway (Zhu et al., 2015). Changes in floral color are due to loss of function mutations in enzyme(s) in the pathway; or loss of transcription factors by either loss of function mutations or loss of expression; or loss of transcription factor binding sites upstream of a gene (or genes) in the pathway through mutations (Wessinger and Rausher, 2012).
Figure 1 The anthocyanin biosynthesis pathway and side branches. Chalcone synthase (CHS), chalcone isomerase (CHI), flavanone hydroxylase (F3H), flavonoid 3′ hydroxylase (F3′H), flavonoid 3′, 5′ hydroxylase (F3′5′H) dihydroflavanol-4-reductase (DFR), anthocyanin synthase (ANS), UDP 3-O-gylcosyltransferase (UDP-GST), flavanol synthase (FLS) and leucoanthocyanidin reductase (LAR).
In this paper we describe genotypic changes potentially underlying the phenotypic changes in flower color in species broadly representative of the Cape Erica clade. Le Maitre et al. (2019) identified and sequenced the genes of the anthocyanin biosynthesis pathway and their trans-acting transcription factors in Erica plukenetii and developed a RT-qPCR assay for determining their expression levels. We use these techniques and UPLC MS/MS (Willemse et al., 2013; Willemse et al., 2014; Willemse et al., 2015; Stander et al., 2017) to test the hypothesis that changes in floral color are due to loss of function mutations in enzyme(s) in the pathway; or loss of transcription factors by either loss of function mutations or loss of expression; or loss of transcription factor binding sites upstream of a gene (or genes) in the pathway through mutations (Wessinger and Rausher, 2012). By analysing multiple examples of independent shifts in color in different clades in the genus we aim to link genotype to phenotype (floral anthocyanins and color) and identify general processes that may have played a role in driving the rapid diversification of Erica in the CFR.
Based on a phylogeny of Erica (Pirie et al., 2011; Pirie et al., 2016, Pirie ms. in prep) (Figure 2), we selected examples of color shifts from pink- to red-, white- or yellow flowered within the Cape Erica clade. These examples are distributed across the Cape Erica clade such that each would be expected to represent one or more independent color shifts. We analysed a total of 12 sets of closely related color variable species. We did not attempt to sample all members of monophyletic groups, nor strict sister-species pairs; instead for each example we selected a putatively plesiomorphic pink-flowered taxon and (a) closely related red-, and/or white- and/or yellow-flowered one(s) for investigation:
A. Erica cameronii (red, bird) and Erica tetrathecoides (pink, insect)
B. Erica cerinthoides (red, bird) and Erica sparmannii (white, insect)
C. Erica discolor ssp. speciosa (red, bird), Erica unicolor ssp. unicolor (red, bird), and Erica prolata (pink, insect)
D. E. plukenetii spp. plukenetii (red/pink/white, bird) and E. plukenetii ssp. breviflora (white, insect)
E. Erica monadelphia (red, bird) and Erica coccinea ssp. coccinea (yellow, bird)
F. Erica mammosa (red, bird), Erica sessiliflora (white, bird), and Erica filipendula ssp. filipendula (white, insect)
G. Erica massonii (red, bird) and Erica squarrosa (pink, insect)
H. Erica regia ssp. regia (red, bird), Erica abietina ssp. abietina (red, bird), Erica viscaria spp. longifolia (white, insect), and Erica vestita (red, bird)
I. Erica haematocodon (red, insect) and Erica hirtiflora (pink, insect)
J. Erica stagnalis ssp. stagnalis (yellow, insect), Erica leucotrachela (red, bird), Erica pillansii (red, bird), Erica parviflora (pink, insect), Erica verticillata (pink, bird), and E. annectans (red, bird)
Figure 2 Phylogenetic tree of the genus Erica, with the major clades indicated with numbers, and the pink/red/white/yellow flowered species groups we investigated in the Cape clade indicated with letters (Le Maitre, 2017; Pirie et al., 2011, 2016). Outgroup species (black), 1. European clade (yellow), 2. African clade (light green), 3. Indian Ocean islands clade (purple), 4. Drakensberg clade (pink), E. trimera sister group to Cape Clade (orange) and Cape Clade (dark green). Red flowered species (red). (A) E. cameronii and E. tetrathecoides (B) E. cerinthoides and E. sparrmannii (C) E. discolor ssp. speciosa, E. unicolor ssp. unicolor and E. prolata (D) Erica plukenetii spp. plukenetii and E. plukenetii ssp. breviflora, (E) E. monadelphia and E. coccinea ssp. coccinea (F) E. mammosa, E. sessiliflora and E. filipendula ssp. filipendula (G) E. massonii and E. squarrosa (H) E. regia ssp. regia, E. abietina ssp. abietina, E. viscaria spp. longifolia and E. vestita (I) E. haematocodon and E. hirtiflora (J) E. stagnais ssp. stagnalis, E. leucotrachela, E. pillansii, E. parviflora, E. verticillata and E. annectans. Images of species are in the same order, clockwise from top left. Photo credits: South African National Biodiversity Institute, casabio.org (E. tetrathecoides and E. massonii © G Kirsten, E. filipendula ssp. filipendula © Cameron McMaster) and iNaturalist (E. squarrosa © Hayley-May Wittridge, E. pillansii and E. stagnalis ssp. stagnalis © Linkie).
All specimens were collected in the CFR. Identifications were confirmed by Dr E.G.H. Oliver, the longstanding taxonomic expert on the genus. Pollinator syndromes from Rebelo et al. (1985).
The analyses were restricted to the anthocyanin biosynthesis pathway. Other pigments, notably carotenoids (Tanaka et al., 2008) can also influence flower color, but have not yet been found in species of Erica (Crowden and Jarman, 1976; Ojeda et al., 2019), and we did not further test for their presence. Primer pairs were designed to bind to the conserved exonic regions of the genes of the anthocyanin biosynthesis pathway (Le Maitre, 2017). Using the methodology of Le Maitre et al. (2019), expression of the genes of the anthocyanin biosynthesis pathway and its trans-acting transcription factors at three different growth points of the corolla in each species was evaluated. The Qiagen Total RNA Qiagen RNeasy Power Plant Kit with the Qiagen RNase-Free DNase Set was used to extract total RNA from 50 mg of corolla tissue. Gel electrophoresis was used to check RNA quality. To confirm that these samples contained high quality RNA their 260/280 absorbance ratios were determined using a Nanodrop ND-1000 spectrophotometer. For the RT-qPCRs, the RT-qPCR mix was prepared using the Kapa SYBR Fast Universal Kit as follows: “10 µl PCR Buffer; 0.4 µl 10 mM Forward and 0.4 µl 10 mM Reverse Primer (Table 1); 0.4 µl 50X Reverse Transcriptase; 2 µl extracted RNA and 6.8 µl milliQ H2O to make up a total of 20 µl. RT-qPCR was performed in a Roche LightCycler 96, with two preincubation steps of 61°C for 5 min, then 95°C for 30 s, followed by 50 cycles of 95°C for 3 s, 58°C for 10 s and 72°C for 2 s. A melting step followed with 95°C for 10 s, 65°C for 1 min and 95°C for 1 s, verifying the presence of a single amplicon per reaction” (Le Maitre et al., 2019).
Table 1 The primers used for RT-qPCR of the genes of the anthocyanin biosynthesis pathway and its transcription factors (Le Maitre et al., 2019).
For each taxon (species or subspecies) assessed, expression of each gene of the anthocyanin biosynthesis pathway was analysed with three biological replicates (flowers of very similar age from the same plant) of juvenile, intermediate and adult flowers, with two technical replicates of each. These groups of reactions per gene (and technical duplicates) included both no-reverse transcriptase and no-template controls. Numerous different primer pair combinations for each gene were tested to identify those that gave consistent amplification across all species. For this subset of consistently performing primers pairs, optimal primer concentrations and annealing temperatures were compared in order to select a final subset of primer pairs and identify a universal set of conditions that would deliver similar quantification cycle values (Cq), amplification efficiencies, and slopes when analysed in a single 96 well plate. Presence of a single amplicon was verified using melting curve analysis (Le Maitre et al., 2019). The reference gene, Ep-PBT1, was used to normalise the expression of the genes of the anthocyanin biosynthesis pathway, using the Roche LightCycler 96 software.
UPLC-MS/MS analysis was performed to evaluate differences in anthocyanin pigment production in each species. Corolla tube tissue (200 mg) was immersed in a 500 µl methanol, 1% formic acid, solution for 1 h in a sonicator and then centrifuged briefly in a low speed benchtop Eppendorf tube centrifuge. Supernatants were loaded on a Waters Synapt G2 instrument for UPLC analysis. The compounds were run in ESI negative on a Waters T3, HH, 2.1×150 mm column using a linear 0.1% formic acid (A) to acetonitrile gradient (B) at a flow rate of 0.250 ml/min. Initial conditions were 0% B and the conditions were kept constant for 1 min. The gradient was increased to 28% B at 22 min, with a subsequent increase to 40% B at 22.5 min. After a further increase to 100% B at 23 min, conditions were kept isocratic for 1.5 min. Conditions were changed back to the initial conditions and were kept constant for 4.5 min to wash the column. The 29 min run was monitored with a tandem mass spectrometer. Two biological replicates were used for each species analysed. The tandem mass spectrometer data were acquired using identical methodology and parameters as described by Willemse et al. (2014) and collected using MassLynx v4.1 software (Waters).
Where the RT-qPCR expression analysis implicated loss of expression of genes and/or UPLC-MS/MS analysis implicated loss of function mutations, in floral color shifts, those genes and/or their upstream regulatory regions were amplified by PCR using primers derived from the Next Generation Sequencing data acquired during the partial sequencing of the E. plukenetii genome (Le Maitre et al., 2019) and sequenced using the BigDye Terminator v3.1 cycle sequencing kit (Thermo Fischer Scientific) and the STeP sequencing protocol (Platt et al., 2007) in an Applied Biosystems Veriti thermal cycler. Sequencing electrophoresis was performed at the Central Analytical Facility, Stellenbosch University.
The RT-qPCR methodology from Le Maitre et al. (2019) originally developed for E. plukenetii was successfully used in multiple Erica species (Figure 3, Table 2 and Supplementary Figure 1). The anthocyanins and the intermediates in the anthocyanin biosynthesis pathway were successfully detected using UPLC-MS/MS in each of the species tested (Figure 4 and Supplementary Figure 2). Using these results, we followed a logical path for determining the lack of production of an anthocyanin precursor or product in a species or subspecies. Firstly, we assessed expression levels of the anthocyanin synthesis genes or transcription factors. Loss of expression of ANS, F3′H and DFR due to mutations in the upstream promotor binding region in E. plukenetii ssp. plukenetii, E. coccinea and E. sessiliflora respectively and mutations in the upstream promotor binding region of UDP-GST in both E. plukenetii ssp. breviflora and E. sparmannii, and a complete knockdown of all anthocyanin biosynthesis pathway expression due to loss of bHLH transcription factor expression in E. viscaria were detected using RT-qPCR (Table 2). In these instances, abrogation of transcription factor binding was the reason for the loss of anthocyanin intermediates or anthocyanins per se. Secondly, where gene expression analyses could not explain the floral color shifts, the non-functional enzymes, DFR and F3′H, were identified by the presence of their precursors and absence of their products in the UPLC-MS/MS analysis in E. filipendula and E. stagnalis respectively. This was confirmed by sequencing of their respective genes, which led to the identification of the loss of much of exon 2 of DFR in E. filipendula and a frameshift mutation in exon 1 of F3′H in E. stagnalis. Normal production of anthocyanin pigments was shifted to proanthocyanidins in E. filipendula and pelargonidin-hexoses in E. stagnalis (Table 2), thereby confirming the non-functionality of the respective enzymes that would lead to their formation. In E. sessiliflora, which possessed a defective transcription factor binding motif of the DFR gene, and in E. filipendula, which possessed a mutation in the DFR gene itself, the metabolites were channelled into kaempherol and quercetin, in both cases. Shifts between pink- and red flowers could not be explained by differences in gene expression profiles (Figure 3). Comparing the UPLC-MS/MS data from pink- and red flowers did not show any qualitative differences in the anthocyanins produced (Figure 4). There were some differences in non-anthocyanin related compounds but these are unlikely to determine to the final floral color.
Figure 3 RT-qPCR analysis of the expression of the genes of the anthocyanin biosynthesis at three different growth points of the corolla in each species was evaluated, with three biological replicates of each growth point.
Table 2 The results of the RT-qPCR and UPLC-MS/MS testing on the Erica species that were collected (Le Maitre et al., 2019). MYB recognition element (MRE).
Figure 4 UPLC-MS/MS results from white-, red- and pink flowered species. Anthocyanin biosynthesis pathway products and intermediates are indicated (1-9). Dihydroflavanol-4-reductase (DFR) was knocked out in the white flowered species.
Shifts from red- or pink- to yellow flowered such as in E. stagnalis and E. coccinea were associated with flux shifts through the pathway, producing pelargonidin-hexose rather than cyanidin-hexose. In cases where enzymes in the pathway were either not present or non-functional such as E. plukenetii ssp. plukenetii, E. plukenetii ssp. breviflora, E. sparrmanii and E. sessiliflora, flux was diverted to other end products such as the leucoanthocyanidins, flavanols or procyanidins. Where the entire pathway was downregulated due to the loss of a transcription factor such as in E. viscaria, some central metabolites, such as quercetin were still found, probably produced by catabolism of other compounds.
Our approach, using RT-qPCR and UPLC-MS/MS, was successful in determining the probable causes of the floral color shifts between red- and/or pink- and white- or yellow flowered species. RT-qPCR and UPLC-MS/MS could not distinguish between pink- and red flowered species, likely due a combination of factors: it may well be that the shift between pink- and red flowers is due to a minor shift in anthocyanin intensity (Grotewold, 2006), a modification of the anthocyanin pigment with covalent metal ions (Akbari et al., 2012; Akbari et al., 2013), changes in vacuolar pH (Akbari et al., 2012; Akbari et al., 2013) or even cell shape (Kay et al., 1981; Noda et al., 1994; Bailes and Glover, 2018), or even the influence of other pigments, such as carotenoids (Tanaka et al., 2008), that might yet be detected in species of Erica.
Numerous mutations were identified as probable causes of floral color shifts in the Erica species studied. These covered the full spectrum of potential causes (Wessinger and Rausher, 2012) from loss of single gene expression due to transcription factor binding site mutations, loss of transcription factor expression, or functional mutations in a gene resulting in flux shifts between legs of the pathway. The wide variety of mutations associated with the color shifts supports the independent origins of the floral color shifts from the plesiomorphic pink form that had been identified using phylogenetic inference (Pirie et al., 2011; Pirie et al., 2016).
A single study had previously used paper chromatography to identify anthocyanins as the pigments that color flowers in Erica species (Crowden and Jarman, 1976). Ours is the first study to make use of UPLC-MS/MS. Six of the 30 species assessed by Crowden and Jarman (1976) were also analysed here: E. discolor, E. plukenetii, E. coccinea, E. mammosa, E. regia and E. vestita. However, Crowden and Jarman (1976) did not report the colors or the subspecies for the flowers they used, which makes comparison of the results problematic. Insofar as the results can be directly compared, i.e. with regard presence of particular anthocyanins in E. mammosa and E. vestita, they are consistent. However, our results go beyond those of Crowden and Jarman (1976), showing that the production of anthocyanins, by the anthocyanin biosynthesis pathway in Erica, appears to be identical to that of other angiosperms (Wessinger and Rausher, 2012; Zhu et al., 2015). Ojeda et al. (2019) studied E. coccinea ssp. coccinea using HPLC and their results correspond with ours, with red flowers due to anthocyanin production and its loss associated with floral color shifts.
Floral color shifts are associated with pollinator shifts and consequent reproductive isolation and diversification/speciation (Rausher, 2008; Wessinger and Rausher, 2012). Pollinator shifts are also associated with changes in a suite of characteristics relevant to pollinator selection. These include, but are not limited to, floral color, shape, size, scent and position, branch strength, growth form and habitat (Fenster et al., 2004; Van der Niet et al., 2014). Pollinator shifts are therefore the result of an accumulation of changes that may gradually change the optimal pollinator type from one to another. The color shifts that we have investigated are an important part of these shifts between different biotic pollinators and from biotic- to wind-pollination (Wolowski and Freitas, 2015).
The shifts from pink- to red flowers in Cape Erica likely represent adaptation to bird pollinators that are not found in the genus’ ancestral European range. These shifts are associated with other changes in a suite of characteristics that make the plants more suited to bird pollination, such as sturdier branches more suitable for perching; longer, more curved floral tubes that better mimic the beaks of local nectarivores such as Anthobaphes violacea (Orange Breasted sunbird), Nectarinia famosa (Malachite sunbird), Cinnyris chalybeus (Southern double-collared sunbird) and Cinnyris afer (Greater double-collared sunbird) (Rebelo et al., 1985; Rebelo and Siegfried, 1985; Van der Niet et al., 2014). In E. plukenetii ssp. breviflora, which has reverted to insect pollination, loss of anthocyanin production and the consequent shifts from red- or pink- to white flowered are associated with branches becoming less sturdy, reduction of floral tube length and flowers producing a distinct scent, promoting pollination by short-tongued noctuid moths (Van der Niet et al., 2014), rather than sunbirds. In other species of Erica, distinct combinations of multiple morphological traits (“syndromes“) are linked to pollination by different guilds of insects, including both generalist pollinators such as bees and specialists such as long proboscid flies; wind pollination; or even pollination by mammals (Rebelo et al., 1985; Rebelo and Siegfried, 1985; Turner et al., 2011; Lombardi et al., 2013; Bouman et al., 2017). Both the color shifts that we have investigated, and the associated changes in other characteristics, may have contributed to a niche occupation and specialization process, potentially in sympatry, and have therefore played a role in driving the diversification of Erica within the CFR.
Previous studies of anthocyanin synthesis and the genes regulating its production have focussed on color variants in a single species (Rausher and Fry, 1993; Coberly and Rausher, 2003; Jin et al., 2016) or a number of variants in closely related species (Forest et al., 2014; Ng and Smith, 2016) and thus general information across all angiosperms could be accumulated. The high species richness of Erica and the independent shifts in flower color apparent given the phylogeny, have enabled us to investigate the different mechanisms controlling anthocyanin synthesis within a single clade of closely related species. In accordance with our hypotheses, we have found evidence that mutations in transcription factor binding sites and functional genes as well as abrogation of the expression of a transcription factor were implicated in color shifts. An analysis of the frequency of these mutational changes shows that five can be attributed to transcription factor binding site mutations, two can be attributed to the mutation of functional genes and only one can be attributed to loss of transcription factor expression i.e. bHLH. This gives support to the evo devo hypothesis of Carrol et al. (2005), which has been extensively debated by Hoekstra and Coyne (2007), that: cis-regulatory changes are more likely to occur as they are thought to be relatively free of negative pleiotropic effects on fitness; mutational changes to functional genes are less likely because they are will have greater effects; and mutational changes to transcription factors genes are the least likely due to potentially more wide-reaching effects. In no instances were identical mechanisms found across species. This is to be expected, given independent origins and the stochastic nature of the evolutionary process, but is nevertheless striking when considered in the context of the multiple changes in flower color between and within the numerous closely related species in Erica. It points to a highly dynamic system allowing rapid adaptation to specific pollinators.
The gene expression data generated can be found in the SUNScholarData repository under https://scholardata.sun.ac.za/s/8eb50fa0cf88c0000ed0.
NL and DB collected samples and designed the study. MP provided phylogenetic data. NL performed the experiments and conducted the data analyses. NL , DB, and MP wrote the manuscript.
This work was supported by the South African National Research Foundation (grant number 98867). MP was supported by the Heisenberg Program of the Deutsche Forschungsgemeinschaft (PI 1169/3-1).
The authors declare that the research was conducted in the absence of any commercial or financial relationships that could be construed as a potential conflict of interest.
We thank Dr EGH Oliver for his invaluable assistance in confirming species identifications.
The Supplementary Material for this article can be found online at: https://www.frontiersin.org/articles/10.3389/fpls.2019.01565/full#supplementary-material
Supplementary Figure 1 | The expression of the genes of the anthocyanin biosynthesis pathway as determined by RT-qPCR for all of the species analysed in the study.
Supplementary Figure 2 | The UPLC-MS/MS for all of the species analysed in the study.
Akbari, R., Hatamzadeh, A., Sariri, R., Bakhshi, D. (2012). Relationship of flower color parameters and metal ions of petal tissue in fully opened flowers of gerbera. J. Plant Stud. 2, 89–96. doi: 10.5539/jps.v2n1p89
Akbari, R., Hatamzadeh, A., Sariri, R., Bakhshi, D. (2013). Analysis of petal pH and metal ions to investigate the mechanism of colour development in Gerbera hybrid. AJCS 7, 941–947.
Bailes, E. J., Glover, B. J. (2018). Intraspecific variation in the petal epidermal cell morphology of Vicia faba L. (Fabaceae). Flora 244–245, 29–36. doi: 10.1016/J.FLORA.2018.06.005
Bouman, R., Steenhuisen, S.-L., van der Niet, T. (2017). The role of the pollination niche in community assembly of Erica species in a biodiversity hotspot. J. Plant Ecol. 10, 634–648. doi: 10.1093/jpe/rtw068
Carlson, J. E., Holsinger, K. E. (2013). Direct and indirect selection on floral pigmentation by pollinators and seed predators in a color polymorphic South African shrub. Oecologia 171, 905–919. doi: 10.1007/s00442-012-2453-2
Carroll, S. B. (2005). Evolution at two levels: on genes and form. PLoS Biol. 3 (7), e245. doi: 10.1371/journal.pbio.0030245
Coberly, L. C., Rausher, M. D. (2003). Analysis of a chalcone synthase mutant in Ipomoea purpurea reveals a novel function for flavonoids: Amelioration of heat stress. Mol. Ecol. 12, 1113–1124. doi: 10.1046/j.1365-294X.2003.01786.x
Crowden, R. K., Jarman, S. J. (1976). Anthocyanins in the genus Erica. Phytochemistry 15, 1796–1797. doi: 10.1016/S0031-9422(00)97497-1
Fenster, C. B., Armbruster, W. S., Wilson, P., Dudash, M. R., Thomson, J. D. (2004). Pollination syndromes and floral specialization. Annu. Rev. Ecol. Evol. Syst. 35, 375–403. doi: 10.1146/annurev.ecolsys.34.011802.132347
Forest, F., Goldblatt, P., Manning, J. C., Baker, D., Colville, J. F., Devey, D. S., et al. (2014). Pollinator shifts as triggers of speciation in painted petal irises (Lapeirousia: Iridaceae). Ann. Bot. 113, 357–371. doi: 10.1093/aob/mct248
Grotewold, E. (2006). The genetics and biochemistry of floral pigments. Annu. Rev. Plant Biol. 57, 761–780. doi: 10.1111/j.1558-5646.2007.00105.x
Hoekstra, H. E., Coyne, J. A. (2007). The locus of evolution: evo devo and the genetics of adaptation. Evolution 61, 995–1016. doi: 10.1111/j.1558-5646.2007.00105.x
Jin, X., Huang, H., Wang, L., Sun, Y., Dai, S. (2016). Transcriptomics and metabolite analysis reveals the molecular mechanism of anthocyanin biosynthesis branch pathway in different Senecio cruentus cultivars. Front. Plant Sci. 7, 1307. doi: 10.3389/fpls.2016.01307
Kay, Q. O. N., Daoud, H. S., Stirton, C. H. (1981). Pigment distribution, light reflection and cell structure in petals. Bot. J. Linn. Soc 83, 57–83. doi: 10.1111/j.1095-8339.1981.tb00129.x
Le Maitre, N. C., Pirie, M. D., Bellstedt, D. U. (2019). An approach to determining anthocyanin synthesis enzyme gene expression in an evolutionary context: an example from Erica plukenetii. Ann. Bot. 124, 121–130. doi: 10.1093/aob/mcz046
Le Maitre, N. C. (2017). Phylogenetics of the genus Erica and anthocyanin synthesis gene expression in Erica plukenetii (Stellenbosch University: PhD Thesis). http://scholar.sun.ac.za/handle/10019.1/101060.
Linder, H. P. (2003). The radiation of the Cape flora, southern Africa. Biol. Rev. 78, 597–638. doi: 10.1017/S1464793103006171
Lombardi, G., Peter, C., Midgley, J. J., Turner, R. (2013). Evidence for rodent-pollination in Erica langinosa (Ericaceae). South Afr. J. Bot. 86, 175–176. doi: 10.1016/j.sajb.2013.02.138
Mcguire, A. F., Kron, K. A. (2005). Phylogenetic relationships of European and African Ericas. Int. J. Pl. Sci. 166, 311–318. doi: 10.1086/427478
Mittermeier, R. A., Gil, P., Hoffman, M., Pilgrim, J., Brooks, T., Mittermeier, C. G., et al. (2004). “Hotspots revisited,” in Earth’s biologically richest and most endangered terrestrial ecoregions (Cemex). (Chicago, USA: University of Chicago Press). Available at: https://press.uchicago.edu/ucp/books/book/distributed/H/bo3707156.html.
Mol, J., Grotewold, E., Koes, R. (1998). How genes paint flowers and seeds. Trends Plant Sci. 3, 212–217. doi: 10.1016/S1360-1385(98)01242-4
Mugrabi De Kuppler, A. L., Fagúndez, J., Bellstedt, D. U., Oliver, E. G. H., Léon, J., Pirie, M. D. (2015). Testing reticulate versus coalescent origins of Erica lusitanica using a species phylogeny of the northern heathers (Ericeae, Ericaceae). Mol. Phylogenet. Evol. 88, 121–131. doi: 10.1016/j.ympev.2015.04.005
Ng, J., Smith, S. D. (2016). Widespread flower color convergence in Solanaceae via alternate biochemical pathways. New Phytol. 209, 407–417. doi: 10.1111/nph.13576
Noda, K., Glover, B. J., Linstead, P., Martin, C. (1994). Flower colour intensity depends on specialized cell shape controlled by a Myb-related transcription factor. Nature 369, 661–664. doi: 10.1038/369661a0
Ojeda, F., Midgley, J., Pauw, A., Lavola, A., Casimiro-Soriguer, R., Hattas, D., et al. (2019). Flower colour divergence is associated with post-fire regeneration dimorphism in the fynbos heath Erica coccinea subsp. coccinea (Ericaceae). Evol. Ecol. . doi: 10.1007/s10682-019-09985-0
Oliver, E. G. H. (1989). The Ericoideae and the southern African heathers. Bot. J. Linn. Soc 101, 319–327. doi: 10.1111/j.1095-8339.1989.tb00167.x
Oliver, E. G. H. (2000). Systematics of Ericeae (Ericaceae-Ericoideae): species with indehiscent and partially dehiscent fruits (Cape Town: Bolus Herbarium, University of Cape Town).
Pirie, M. D., Oliver, E. G. H., Bellstedt, D. U. (2011). A densely sampled ITS phylogeny of the Cape flagship genus Erica L. suggests numerous shifts in floral macro-morphology. Mol. Phylogenet. Evol. 61, 593–601. doi: 10.1016/j.ympev.2011.06.007
Pirie, M. D., Oliver, E. G. H., Mugrabi de Kuppler, A., Gehrke, B., Le Maitre, N. C., Kandziora, M., et al. (2016). The biodiversity hotspot as evolutionary hot-bed: spectacular radiation of Erica in the Cape Floristic Region. BMC Evol. Biol. 16, 190. doi: 10.1186/s12862-016-0764-3
Pirie, M. D., Kandziora, M., Nürk, N. M., Le Maitre, N. C., Mugrabi de Kuppler, A., Gehrke, B., et al. (2018). Leaps and bounds: geographical and ecological distance constrained the colonisation of the Afrotemperate by Erica. bioRxiv. doi: 10.1101/290791
Platt, A. R., Woodhall, R. W., George, A. L. (2007). Improved DNA sequencing quality and efficiency using an optimized fast cycle sequencing protocol. Biotechniques 43, 58–62. doi: 10.2144/000112499
Rausher, M. D., Fry, J. D. (1993). Effects of a locus affecting floral pigmentation in Ipomoea purpurea on female fitness components. Genetics 134, 1237–1247.
Rausher, M. D. (2008). Evolutionary transitions in floral color. Int. J. Plant Sci. 169, 7–21. doi: 10.1086/523358
Rebelo, A. G., Siegfried, W. R. (1985). Colour and size of flowers in relation to pollination of Erica species. Oecologia 4, 584–590. doi: 10.1007/BF00379677
Rebelo, A. G., Siegfried, W. R., Oliver, E. G. H. (1985). Pollination syndromes of Erica species in the south-western Cape. S. Afr. J. Bot. 51, 270–280. doi: 10.1016/S0254-6299(16)31657-X
Stander, M. A., Van Wyk, B. E., Taylor, M. J. C., Long, H. S. (2017). Analysis of Phenolic Compounds in Rooibos Tea (Aspalathus linearis) with a Comparison of Flavonoid-Based Compounds in Natural Populations of Plants from Different Regions. J. Agric. Food Chem. 65, 10270–10281. doi: 10.1021/acs.jafc.7b03942
Tanaka, Y., Sasaki, N., Ohmiya, A. (2008). Biosynthesis of plant pigments: anthocyanins, betalains and carotenoids. Plant J. 54, 733–749. doi:10.1111/j.1365-313X.2008.03447.x
Turner, R. C., Midgley, J. J., Johnson, S. D. (2011). Evidence for rodent pollination in Erica hanekomii (Ericaceae). Bot. J. Linn. Soc 166, 163–170. doi: 10.1111/j.1095-8339.2011.01139.x
Van der Niet, T., Pirie, M. D., Shuttleworth, A., Johnson, S. D., Midgley, J. J. (2014). Do pollinator distributions underlie the evolution of pollination ecotypes in the Cape shrub Erica plukenetii? Ann. Bot. 113, 301–315. doi: 10.1093/aob/mct193
Van Der Niet, T., Peakall, R., Johnson, S. D. (2014). Pollinator-driven ecological speciation in plants: new evidence and future perspectives. Ann. Bot. 113, 199–211. doi: 10.1093/aob/mct290
Waser, N. M. (1998). Polliantion, angiosperm speciation, and the nature of species boundaries. Oikos 82, 198–201. doi: 10.2307/3546930
Wessinger, C. A., Rausher, M. D. (2012). Lessons from flower colour evolution on targets of selection. J. Exp. Bot. 63, 5741–5749. doi: 10.1093/jxb/ers267
Willemse, C. M., Stander, M. A., de Villiers, A. (2013). Hydrophilic interaction chromatographic analysis of anthocyanins. J. Chromatogr. A 1319, 127–140. doi: 10.1016/j.chroma.2013.10.045
Willemse, C. M., Stander, M. A., Tredoux, A. G. J., de Villiers, A. (2014). Comprehensive two-dimensional liquid chromatographic analysis of anthocyanins. J. Chromatogr. A 1359, 189–201. doi: 10.1016/j.chroma.2014.07.044
Willemse, C. M., Stander, M. A., Vestner, J., Tredoux, A. G. J., de Villiers, A. (2015). Comprehensive two-dimensional hydrophilic interaction chromatography (HILIC) × Reversed-Phase Liquid Chromatography Coupled to High-Resolution Mass Spectrometry (RP-LC-UV-MS) Analysis of Anthocyanins and Derived Pigments in Red Wine. Anal. Chem. 87, 12006–12015. doi: 10.1021/acs.analchem.5b03615
Wolowski, M., Freitas, L. (2015). An overview on pollination of the Neotropical Poales Rodriguésia. 66, 329–336. doi: 10.1590/2175-7860201566204
Keywords: floral color, anthocyanin, gene expression, Erica, RT-qPCR, UPLC-MS/MS
Citation: Le Maitre NC, Pirie MD and Bellstedt DU (2019) Floral Color, Anthocyanin Synthesis Gene Expression and Control in Cape Erica Species. Front. Plant Sci. 10:1565. doi: 10.3389/fpls.2019.01565
Received: 30 August 2019; Accepted: 07 November 2019;
Published: 28 November 2019.
Edited by:
Jill Christine Preston, University of Vermont, United StatesReviewed by:
Carolyn Wessinger, University of Kansas, United StatesCopyright © 2019 Le Maitre, Pirie and Bellstedt. This is an open-access article distributed under the terms of the Creative Commons Attribution License (CC BY). The use, distribution or reproduction in other forums is permitted, provided the original author(s) and the copyright owner(s) are credited and that the original publication in this journal is cited, in accordance with accepted academic practice. No use, distribution or reproduction is permitted which does not comply with these terms.
*Correspondence: Dirk U. Bellstedt, ZHViQHN1bi5hYy56YQ==
†Present address: N C Le Maitre, Steenhuisen Laboratory, Department of Plant Science, University of the Free State, Bloemfontein, South Africa
Disclaimer: All claims expressed in this article are solely those of the authors and do not necessarily represent those of their affiliated organizations, or those of the publisher, the editors and the reviewers. Any product that may be evaluated in this article or claim that may be made by its manufacturer is not guaranteed or endorsed by the publisher.
Research integrity at Frontiers
Learn more about the work of our research integrity team to safeguard the quality of each article we publish.