- 1Departament of Biology, Universitat de les Illes Balears, Palma, Spain
- 2Plant Physiology Laboratory, Bioscience Faculty, Universitat Autònoma de Barcelona, Barcelona, Spain
Pests and diseases pose a threat to food security, which is nowadays aggravated by climate change and globalization. In this context, agricultural policies demand innovative approaches to more effectively manage resources and overcome the ecological issues raised by intensive farming. Optimization of plant mineral nutrition is a sustainable approach to ameliorate crop health and yield. Zinc is a micronutrient essential for all living organisms with a key role in growth, development, and defense. Competition for Zn affects the outcome of the host–attacker interaction in both plant and animal systems. In this review, we provide a clear framework of the different strategies involving low and high Zn concentrations launched by plants to fight their enemies. After briefly introducing the most relevant macro- and micronutrients for plant defense, the functions of Zn in plant protection are summarized with special emphasis on superoxide dismutases (SODs) and zinc finger proteins. Following, we cover recent meaningful studies identifying Zn-related passive and active mechanisms for plant protection. Finally, Zn-based strategies evolved by pathogens and pests to counteract plant defenses are discussed.
Mineral Nutrients in Plant Defense
Mineral nutrients are directly involved in plant protection as structural components and metabolic regulators (Huber, 1980). As a first line of defense, the nutritional status can determine plants’ susceptibility to pests and pathogens (Walters and Bingham, 2007; Marschner and Marschner, 2012). Essential and beneficial elements affect plant health both directly, by activating enzymes that produce defense metabolites (callose, glucosinolates, lignin, phenols, and phytoalexins), and indirectly, by altering root exudates, rhizosphere pH, and microbial activity (Datnoff et al., 2007). In addition to chemical and/or biochemical factors, plant protection strategies include physical (shape, surface properties, hairs, color, etc.) and mechanical (fibers, silicon) properties (Marschner, 1995); all these features are also influenced by mineral nutrients.
The nutritional status of the host plant affects the colonization success by pests and pathogens. However, it is not possible, either globally or individually, to generalize the effects of nutrients for all plant–pest/pathogen systems (Huber, 1980).
Many studies have focused on the relationship between macronutrients and plant pests and diseases, and several sound reviews link both topics. Most attention has centered on the effects of N, P, and K due to their reduced availability in many soils and their elevated plant demand (Huber, 1980; Walters and Bingham, 2007; Amtmann et al., 2008). However, inconsistent results of macronutrient fertilization and damage by insect herbivory have been reported (Aqueel and Leather, 2011; Chesnais et al., 2016). Furthermore, silicon has deserved special attention, primarily on account of its structural role in the reinforcement of cell walls, and more recently as an activator of defense genes (Fauteux et al., 2005; Cabot et al., 2013; Van Bockhaven et al., 2013) and pest protection (He et al., 2015).
Micronutrient roles in plant defense are predominantly documented for Mn, Cu, Fe, and Zn (Graham and Webb, 1991; Dordas, 2008; Fones and Preston, 2013). Manganese participates in the production of phenolic compounds and other plant defense mechanisms (Fernando et al., 2009), while Ni is involved in the plant antioxidant system and in the plant response to stress (Fabiano et al., 2015). Best studied are the pathogenesis-related mechanisms based on the Fe redistribution at the cellular level (Greenshields et al., 2007; Liu et al., 2007). Zinc seems to be a major player in both animal (Hojyo and Fukada, 2016) and plant immune responses (Shirasu et al., 1999; Gupta et al., 2012a). However, so far, no general model has been proposed for Zn, despite the fact that several processes involving this element have been analyzed in different pathosystems. Furthermore, most of these studies have focused on metal hyperaccumulating species that absorb and accumulate a wide range of elements, including micronutrients like Mn, Fe, Ni, and Zn, or other trace elements like Cd, Se, or As. The high tissue concentrations of these elements may protect plants against pests and diseases (Poschenrieder et al., 2006) (see below).
This review complies the still scant available information characterizing the Zn-related strategies employed by plants to fight their attackers. The generated overview will hopefully permit the reader to see the state of the art of this topic, as well as the existing gaps, where current knowledge established in plant systems is derived either from Fe research or taken from animal systems.
Defense-Related Functions of Zinc in Plants
Zinc is a catalytic and structural protein cofactor in hundreds of enzymes (Hambidge et al., 2000) and has key structural functions in the protein domains that interact with other molecules. The “Zn finger” proteins mediate DNA binding of transcription factors and protein–protein interactions (Sinclair and Kramer, 2012). Zn-binding sites can now be predicted from sequenced metal-binding motifs using bioinformatic approaches. Based on these findings, the Zn proteome may represent about 9% of the entire proteome in eukaryotes and from 5% to 6% in prokaryotes (Andreini et al., 2009).
Both Zn excess and Zn deficiency cause Zn to become prooxidant (Kinraide et al., 2011). Therefore, in all organisms, the cellular concentration and compartmentation of mobile Zn is tightly controlled by different sets of proteins responsible for sensing, transporting, buffering, storing, and releasing Zn. An outstanding reason for the requirement of a strict control of Zn homeostasis comes from studies conducted in animal systems demonstrating that Zn is an intracellular second messenger in several signaling pathways (Yamasaki et al., 2007); therefore, this element plays key roles in the organism’s metallo-neurochemistry. Zinc acts as a neuromodulator in the central nervous system (Goldberg and Lippard, 2018), and Zn transporter ZnT3 is responsible for Zn concentration in synaptic vesicles of certain glutamatergic neurons (McAllister and Dyck, 2017). While glutamate has a recognized function in plant signaling (Forde and Lea, 2007), a connection to Zn in this process has not yet been established in plants.
Zinc plays a pivotal function in the plant response to pests and diseases. Nonetheless, Zn defense-related mechanisms in plants greatly vary. The outcomes of plant–pest/pathogen interactions differ, depending on the effectivity of the Zn-related responses in limiting the invader’s attack as well as on the enemy’s ability to circumvent the plant defenses, in addition to other environmental conditions that can favor either host or invader. Several studies have shown that, in most cases, Zn fertilization decreased plant symptoms (Grewal et al., 1996; Li et al., 2016; Machado et al., 2018). However, a protective Zn concentration against certain pathogens can also induce a higher susceptibility to another pathogen on the same plant (Helfenstein et al., 2015) (see below).
Zn proteins play a dual role in plant defense, with potential to simultaneously aid and abet the plant and its invaders. Table 1 provides evidence of some of the described connections of Zn proteins with the plant defense and/or the invader virulence. The most commonly assigned functions of Zn proteins are listed in this table, along with examples relating a specific protein function with their respective defense mechanisms. The table denotes that certain similar Zn protein-based mechanisms have been described for both plant defense factors and the pathogenicity factors of their invaders. In the reported cases, the described mechanism is either exclusively mediated through Zn or Zn is mentioned to be involved. The results of these studies summarized in Table 1 globally address an effective plant defense/invader attack with increased gene expression or enhanced activity of the referred protein function. It should be underscored that the described strategies of defense are not exclusively assigned to Zn proteins but also combined with other responses. Among those, two broad-spectrum responses involved in plant–pest/pathogen interactions are tightly related to Zn: oxidative stress and regulation of Zn finger proteins, both of which are more extensively discussed in the following subsections.
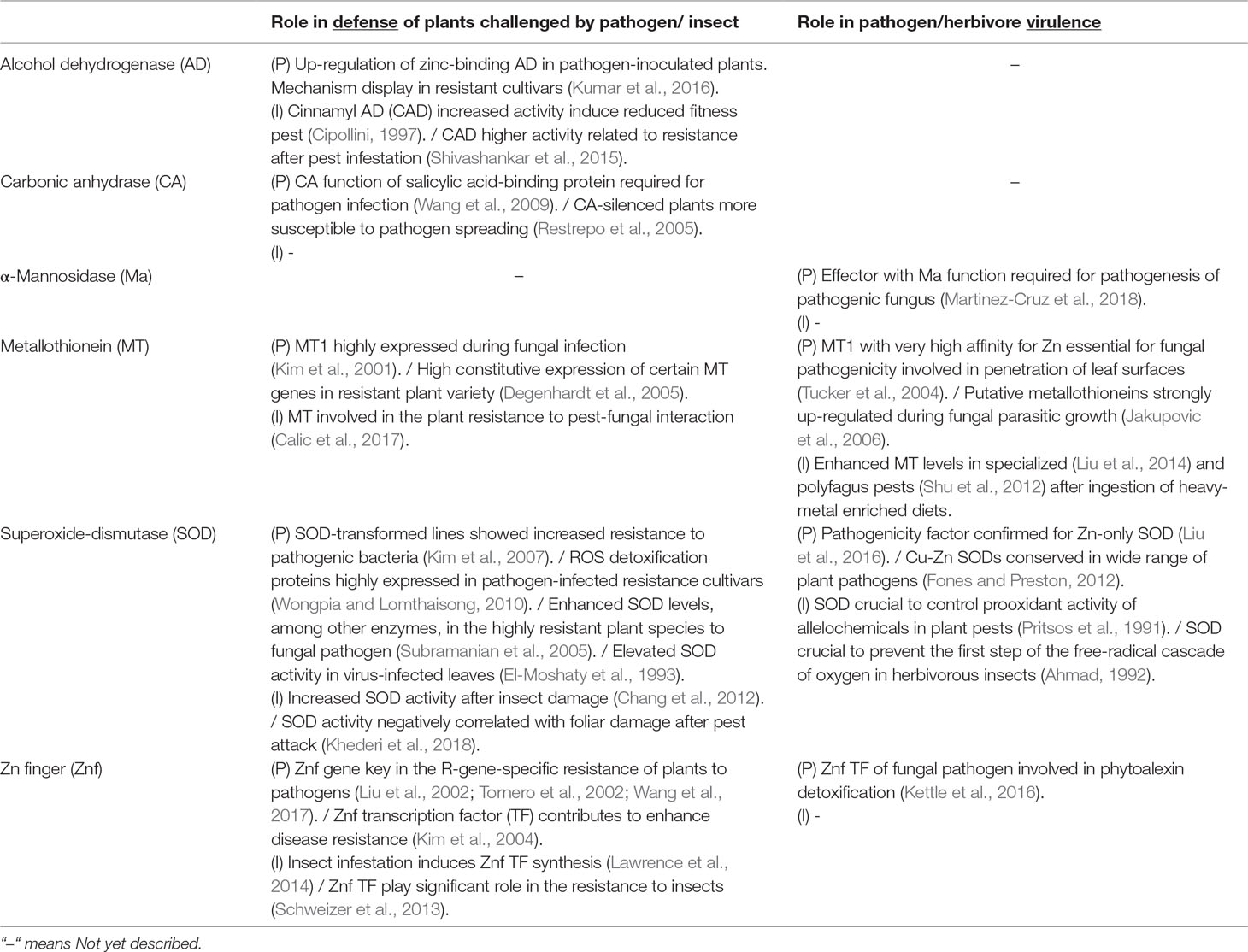
Table 1 Most common assigned functions of Zn proteins with examples relating a specific protein function with defense mechanisms. Similar Zn protein-based mechanisms have been described for the plant defense factors against pathogens (P) or phytophagous insects (I).
Superoxide Dismutases (Sods)
Zinc affects plant–pathogen interactions via its key role in the activation/stabilization of metalloenzymes (Fones and Preston, 2012). A common component in the plant responses to stress conditions caused by insufficient Zn availability and/or pathogen attack is the plant’s capacity to overcome oxidative stress. Under Zn-deficient conditions, reactive oxygen species (ROS) are considered to be the primary factor responsible for plant growth inhibition (Cakmak, 2000). Additionally, changes in the antioxidant capacity and increased ROS formation have also been reported in plants in response to excess Zn (Jain et al., 2010; Feigl et al., 2015). On the other hand, the immune system uses ROS to fight pathogens directly by causing oxidative damage or indirectly by triggering different non-oxidative mechanisms, i.e., pattern recognition and receptor signaling. After plant recognition of pathogen attack, a rapid oxidative burst of oxygen radicals is needed in many cases to trigger plant defense mechanisms, i.e., the hypersensitive response and systemic acquired resistance (SAR) (Sutherland, 1991; Lehman et al., 2015). Oxygen radicals are controlled by the activity of antioxidant enzymes, and among them, SODs catalyze the conversion of superoxide radicals to hydrogen peroxide, which is involved in abiotic and biotic stress signaling (Miller et al., 2008). Cu/Zn-SOD activity is commonly increased in herbivore/pathogen-challenged plants (Fodor et al., 1997; Montalbini and Buonaurio, 1986; Zacheo and Bleve-Zacheo, 1988; Deepak et al., 2006), yet its activity decreased in Zn-deficient plants (see below).
Zinc Finger Proteins
In addition to their role in plant growth and development, zinc finger proteins regulate plant responses to biotic stress conditions (Noman et al., 2019b). With a broad spectrum of structures and functions, these proteins are defined as those with a small, freely folded functional domain that requires one or more zinc ions to stabilize its structure (Laity et al., 2001). Zinc finger binding domains are present in the well-known plant resistance proteins NBS-LRRs (nucleotide binding sites-leucine rich) that are involved in the effector-triggered immune response (Gupta et al., 2012a). The authors of this study analyzed 70 plant disease-resistance proteins from different crops. Of these proteins, 37% contain zinc finger domains, which suggests a major role for this protein class in the host’s resistance to pathogens. One of the R-genes with a zinc finger domain studied was Pi54, which confers durable resistance against the fungus Magnaporthe oryzae. In a transgenic line of rice containing the Pi54 gene, the up-regulation of defense response genes (callose, laccase, PAL, and peroxidase) and genes related to transcription factors (NAC6, Dof zinc finger, MAD box, bZIP, and WRKY) was observed (Gupta et al., 2012b). Additionally, RAR1, a zinc binding protein of wheat, confers resistance against the stripe rust pathogen through salicylic acid (SA)-mediated oxidative burst and hypersensitive response (Wang et al., 2017). Insect infestation has been related to the up-regulation of two zinc finger transcription factors in potato (Lawrence et al., 2014). Some members of the family of transcription regulators cysteine2/histidine2-type zinc finger proteins (ZAT) play key roles in ROS signaling in the response of plants to biotic and abiotic stresses (Miller et al., 2008). A member of this family, AtZAT6, participates in plant development and positively modulates the Arabidopsis response to pathogen infection by activating the expression of SA-related genes such as PATHOGENESIS-RELATED GENE1 [PR1], PR2, and PR5.
In plant and animal systems, direct roles of Zn to control pests and pathogens include mechanisms to produce either low- or high-Zn scenarios (Figure 1). Host-imposed Zn starvation by sequestering Zn from the infection site in a biologically inactive form is a common strategy deployed by the immune system in animals to fight pathogens (Vignesh et al., 2013). Zinc removal strategies from the media to prevent microbial growth have been less documented in plants, yet most of the research on this topic has focused on Fe (Fones and Preston, 2013). On the other hand, hosts may actively deploy high Zn concentrations to control pathogen proliferation. Such active mechanisms have mostly been reported in animal systems (McDevitt et al., 2011), while few studies have been conducted in plants so far (Stolpe et al., 2017). Most of the research in plants concerning the potential protective role of high Zn concentrations has focused on Zn hyperaccumulating species. High Zn concentrations could protect plants from invaders by direct Zn toxicity and by enhancing Zn-triggered organic defenses (Poschenrieder et al., 2006; Fones and Preston, 2013).
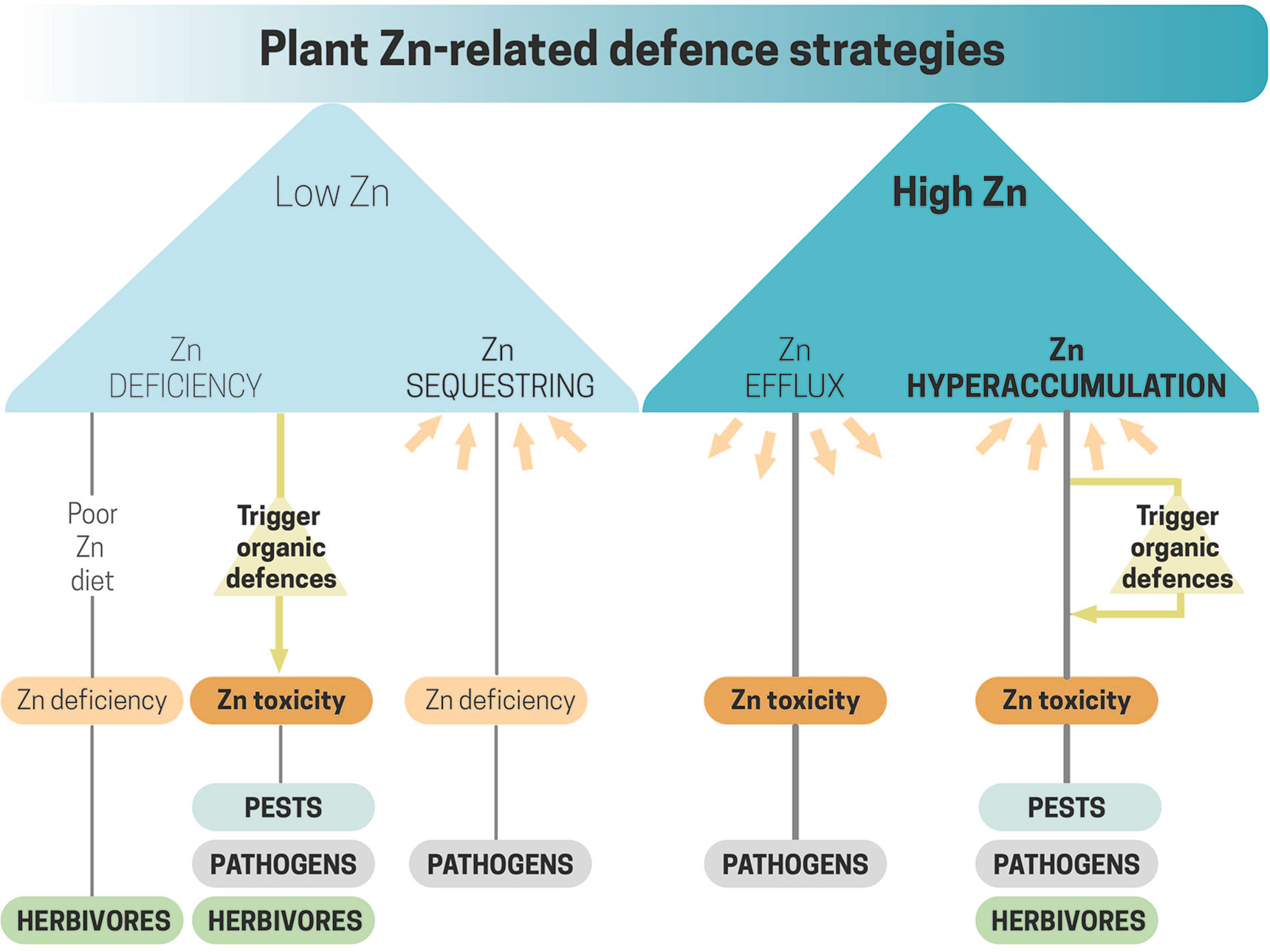
Figure 1 Low- and high-Zn conditions created by plants to confront pests, pathogens, and herbivores. Low-Zn conditions when resulted from a poor Zn diet can trigger the synthesis of organic defenses against a wide variety of plant enemies. Active Zn-sequestering/efflux from/to the extracellular media reduces/increases Zn availability, causing deficiency/toxicity to pathogens. The presence of high Zn concentrations in the above-ground parts of Zn hyperaccumulating species causes Zn toxicity to plant attackers.
In the following sections, plant strategies to fight pests and diseases related to low and high Zn concentrations as well as mechanisms evolved by pathogens and pests to counteract Zn-related plant defenses will be discussed.
Defense Mechanisms Against Biotic Stress Under Low-Zinc Conditions
Role of Zn Deficiency in Plant Defense
In general, Zn-deficient plants are more susceptible to diseases (Marschner, 1995; Grewal et al., 1996; Streeter et al., 2001; Helfenstein et al., 2015). Most studies looking for a potential relationship between plant Zn status and disease severity have reported an ameliorated response to diseases caused by fungi in plants supplemented with Zn (Grewal, 2001; Simoglou and Dordas, 2006; Huber and Haneklaus, 2007; Khoshgoftarmanesh et al., 2010). For example, a study conducted on the effect of different pathogens in soybeans with different Zn treatments showed that plants grown with either normal or high Zn fertilization had fewer positive counts for bacterial pustules caused by Xanthomonas axonopodis pv. glycines and less lesion area affected by the necrotrophic fungus Sclerotinia sclerotiorum than plants grown with low Zn (Helfenstein et al., 2015).
Along this line, Zn-efficient genotypes have been found to be more disease resistant, showing a positive relationship between increased plant growth and reduced pathogen susceptibility (Graham and Webb, 1991). Although the response of plants to Zn deficiency has been extensively studied, the different components and mechanisms involved in the efficient use of Zn remain unclear (Hacisalihoglu and Kochian, 2003). The oxidative damage due to ROS production is reduced in Zn efficient genotypes (Rose et al., 2012). Moreover, supplemental Zn has been found to increase SOD activity in plants under abiotic stress (Noman et al., 2018).
Recently, important advances on microRNA (miRNA) functions have been achieved, which would contribute to clarifying the mechanisms involved in the response of plants to pests and pathogens under Zn scarcity. In addition to plant growth and development, miRNAs modulate plant responses to stress conditions (Noman et al., 2017b). The ROS-quenching metalloenzyme Cu/Zn-SOD is post-transcriptionally regulated by a miRNA; specifically, the miR398 targets isoforms CSD1 and CSD2, which are located at the cytosol and chloroplast, respectively (Jones-Rhoades et al., 2006). The down-regulation of miR398 induces the CSD1 gene expression, a mechanism triggered by bacterial infection (Pseudomonas syringae) in Arabidopsis (Sunkar et al., 2006). However, miR398 was up-regulated under low Cu2+ concentrations. Although the effect of Zn starvation on miR398 has not been reported, the up-regulation response found for Cu could also be associated to Zn, as the protein is cofactored by both elements. Nonetheless, further studies are needed to clearly establish the relationship between Zn deficiency and pathogen susceptibility.
Reduced protein synthesis is one effect of Zn deficiency that leads to a higher accumulation of amino acids. This increase has been related to higher incidences of sucking insects present on the plant (Fageria et al., 2002). Similar results were obtained for Zn-deficient soybean plants that showed increased aphid colonization compared to plants grown at physiologically sufficient Zn concentrations (Helfenstein et al., 2015). Contrastingly, in the same study, the biotrophic obligate pathogen that causes soybean rust, Phakopsora pachyrhizi, had a lesser rate of spread in deficient plants. The authors concluded that one single element can differentially affect the response of a specific host to aphids and pathogens. These results revealed the lack of a Zn-specific requirement to fight pests and pathogens, which is a limiting factor for the use of micronutrient fertilization to manage diseases.
Some of the mechanisms involved in the relationship between low Zn and disease susceptibility are starting to emerge. Plant innate immune mechanisms against biotrophic pathogens are associated with the buildup of SA concentrations, the ROS burst, and the trigger of SA-dependent SAR in non-infected tissues (Cameron et al., 1994; Breitenbach et al., 2014). Different compounds participate in the long-distance transmission of SAR, among them, the C9 dicarboxylic acid, azelaic acid (AzA) (Jung et al., 2009). A recent report revealed an evolutionary conserved Zn sensing-mechanism, which connects root growth to the plant’s pathogen responses (Bouain et al., 2018). The authors found that AZELAIC ACID INDUCED1 [AZI1], a member of the lipid transfer protein family (LTPs) of pathogenesis related (PR) proteins, is triggered by azelaic acid (AzA) during SAR. AZI1 regulated growth and immunity responses depending on Zn availability. The results demonstrated that signaling triggered by low Zn and AzA interact. The low Zn status negatively affected the expression of defense-related genes, among others PR1, a SA-induced marker gene that modulated the response to AzA. During early development, when Zn availability is low, Arabidopsis can prioritize root growth over defense responses, leading to an increase in soil volume mined for available Zn. The authors highlighted the need for more extensive studies on the plant interacting signaling networks between defense and growth. Along this line, it would be very interesting to test these signaling pathways in genotypes with contrasting Zn efficiency. A close connection between biotic stress defense and growth signaling networks becomes especially evident in the view of the fact that AzA is listed among the agricultural chemicals for plant resistance priming (Aranega-Bou et al., 2014), but was found to severely inhibit root growth in Arabidopsis plants supplied with sufficient Zn concentrations (Bouain et al., 2018).
Zn-Sequestering Defense Strategies
The requirement of Zn and other micronutrients by pathogens has impelled the animal immune system to evolve mechanisms to sequestrate them in order to restrict pathogen access to these essential nutrients, a process known as nutritional immunity (Hood and Skaar, 2012). There is still little evidence on metal-withholding mechanisms in plants to fight microbes, and most studies focused on Fe. In the case of pathogenic bacteria, the withholding of Fe within a plant storage compartment is considered one of the first mechanisms of defense (Fones and Preston, 2013). A research by Mila et al. (1998) suggested that polyphenols in plants may play a similar role to that of ion-binding proteins in animals by withholding iron away from pathogens. The sequestration of Fe might induce the expression of genes associated with Fe homeostasis and activation of immune responses against biotrophic pathogens (Dellagi et al., 2005). Recently, the expression levels of an apoplastic iron chelating protein has been found to correlate with tolerance to the necrotrophic bacterium Pectobacterium carotovorum subsp. carotovorum (Pcc) (Hsiao et al., 2017).
In the view of the essential role of Zn in key metabolic processes in both plants and pathogens, nutritional immunity mechanisms based on competition for Zn are highly plausible. Metal-chelating compounds could restrict the availability of metals to pathogens in plants. A recent study on the ZINC-INDUCED FACILITATOR [ZIF1], a nicotianamine (NA) transporter located at the tonoplast in A. thaliana, showed that the perturbation of the subcellular distribution of the Zn-chelator NA has a great effect in the subcellular and inter-organ partitioning of Zn (Haydon et al., 2012). As suggested by the authors, such studies, in addition to helping to advance the biofortification of crops, also contribute in providing clues concerning Zn-related mechanisms to ameliorate the plant’s resistance to pathogens. Along this line, a ZIF-1 orthologue in Zea mays, Zm-mfs1, was previously reported to be induced by fungal infection in resistant as well as in susceptible interactions (Simmons et al., 2003).
Zn Deficiency-Triggered Defense Mechanisms
Nutrient deficiencies usually render plants more susceptible to pests and pathogens. Nonetheless, some signaling pathways triggered in response to nutrient scarcity boost the plant immune system.
Such a defense activation implying altered gene-expression profiles and further metabolite production has specifically been reported for Fe- and Mn-deficient conditions (Rodríguez-Celma et al., 2013; Fourcroy et al., 2014; Schmid et al., 2014; Rodríguez-Celma et al., 2016). Information regarding Zn deficiency is still scarce, but some studies indicate that pathways signaling Zn-deficient conditions can trigger plant defense responses.
Defensins (DFN) are known for their involvement in the innate immune defense system (Stotz et al., 2009). Recently, an increased expression of three DFN-like proteins in A. thaliana grown under Zn-deficient conditions has been found (Inaba et al., 2015). These proteins were under the direct control of the basic region leucine-zipper transcription factor gene bZIP19. This transcription factor plays a role in the Zn-depletion responses by regulating ZIP transporter gene expression (Assunçao et al., 2010). The bZIPs regulate key processes in both plants and animals. In plants, bZIPs participate among other processes, in response to abiotic and biotic stress (Noman et al., 2019a). The bZIP TFs are key players in plant innate immunity regulating genes associated with PAMP-triggered immunity, ET immunity, and hormonal signaling networks (Noman et al., 2017a). Although plant DFN are also implicated in plant growth and development (Stoltz et al., 2009), exploration and functional characterization of novel bZIP TFs in planta could be helpful tools to improve crop resistance against pathogens and environmental stresses (Inaba et al., 2015).
Defense Mechanisms Against Biotic Stress Under High-Zinc Conditions
Many studies have reported enhanced plant resistance to diseases and pests after supplemental Zn concentrations, or when a mix of micronutrients including Zn was applied (Graham and Webb, 1991; Dordas, 2008; Chavez-Dulanto et al., 2018). In fact, several phytosanitary formulae are based on Zn-organic complexes. In this situation, microorganisms and herbivores may be directly affected by the toxic effect of Zn rather than through plant metabolic responses (Graham and Webb, 1991).
Zn Efflux Defense Strategies
High Zn concentrations are toxic to pests and pathogens. In animals, excess Zn can displace other essential metals from their catalytic center within the protein. Accordingly, the deployment of high Zn concentrations in mucosal surfaces infected with Streptococcus pneumoniae has been found to produce a displacement of Mn from the pathogen’s Mn transporters, inducing Mn starvation and enhancing bacterial sensitivity to oxidative stress, among other effects (McDevitt et al., 2011). Few similar Zn efflux mechanisms have been reported in plants, although Stolpe et al. (2017) found that aphid infestation in Arabidopsis halleri increased phloem Zn concentration as a first line of defense. Phloem Zn content varied and was higher in the phloem exudates of highly valuable tissues needing protection; i.e., young leaves were better defended than older ones, which agrees with the optimal defense theory (Strauss et al., 2004).
Zinc-Concentration-Dependent Plant Defense Mechanisms
High Zn concentrations are potentially toxic to all organisms. Zn phytotoxicity usually becomes visible at leaf concentration higher than 300 mg Zn kg−1 (Broadley et al., 2007). Zinc toxicity-associated symptoms in plants include reduced yield and stunted growth, reduced export of photoassimilates from leaves to roots (Ruano et al., 1988), and Fe-deficiency-induced chlorosis through reductions in chlorophyll synthesis and chloroplast degradation (Chaney, 1993). The use of high Zn in plant defense depends on the relative host and pest/pathogen Zn-tolerance. In this context, the potential use of Zn as a toxic compound for plant defense has mostly been studied in metal hyperaccumulating species, which tolerate extremely high Zn tissue concentrations. Metal hyperaccumulation can provide defense against herbivores and pathogens in plants, an adaptive advantage that might have driven the evolution of this trait (Boyd and Martens, 1992). Multiple studies have related metal hyperaccumulation to plant defense against biotic stress. Different mechanisms for such metal-based protection have been proposed. According to the original “Metal Defense Hypothesis,” the metal concentrations reached in shoot tissues of hyperaccumulating species would be high enough to intoxicate the attacking pest or pathogen (Boyd and Martens, 1992; Poschenrieder et al., 2006). Afterwards, it was found that high metal concentration and organic compounds may cooperate in the plant’s defense (Boyd, 2012). This led to the “Joint-Effects Hypothesis” proposing that high metal concentrations in non-hyperaccumulating species in cooperation with organic substances can be effective in plant defense. In fact, high Zn concentrations found in non-hyperaccumulating species can enhance plant defense (Cheruiyot et al., 2013; Martos et al., 2016).
Direct Zn Toxicity
Direct elemental defense by Zn implies that the high Zn accumulation in the plant tissues is more toxic to the pest/pathogen than the plant. A trade-off between metal-based and organic defenses may further benefit the plant as a potential energy-saving mechanism under biotic stress (Strauss et al., 2002). However, no hard data are available comparing the energy costs for production of organic defenses and metal accumulation, chelation, and compartmentation to demonstrate such a trade-off. Few studies have shown a direct Zn toxicity mechanism in plants against pathogens. In the hyperaccumulating species Noccaea caerulescens, high tissue Zn concentrations restricted the infection of the biotrophic pathogen P. syringae pv. maculicola. ROS-dependent defense mechanisms commonly associated with plant defense against biotrophs, such as callose deposition and pathogenesis-related (PR) gene expression, were not triggered despite the fact of increased salicylic acid (SA) levels in the infected plants. This suggests that in this model, a direct toxic effect of Zn would be the main factor limiting bacterial colonization (Fones et al., 2010; Fones and Preston, 2013).
A recent study monitoring the effect of high Zn supply in the response of N. caerulescens to Alternaria brassicicola (Gallego et al., 2017) showed that the average Noccaea leaf Zn concentration, after 5 weeks of growth in 25% modified Hoagland solution with 102 µM ZnSO4, was 5,000 µg/g dry weight. This is equivalent to 10 mM Zn in the cell sap, a concentration 20 times higher than the EC50 for A. brassicicola in vitro (Figure 2A). Because Alternaria is a necrotrophic fungus, a direct effect of Zn would be feasible due to the release of toxic Zn concentrations into the extracellular space as a consequence of cell compartmentation destruction during the colonization process (Figure 2B). However, joint effects may also operate, as in this pathosystem, high Zn also triggered organic defenses.
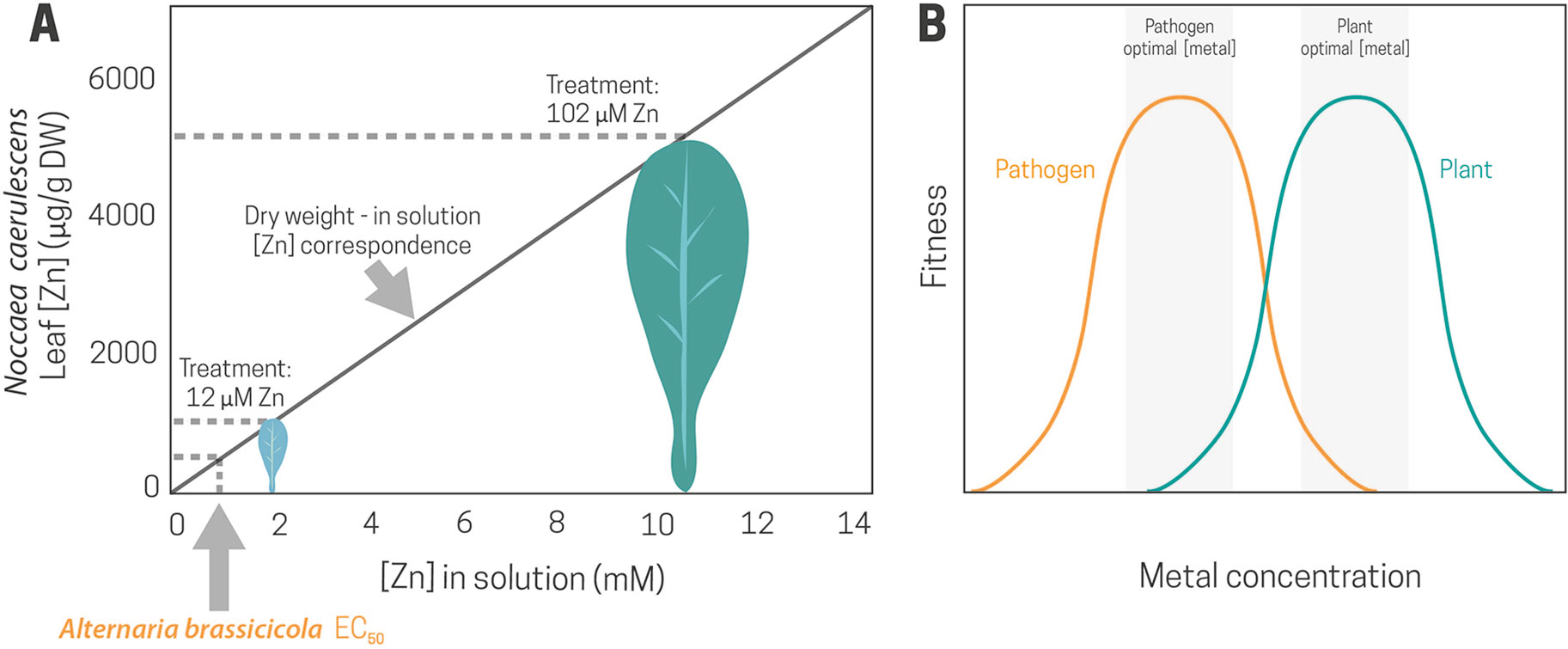
Figure 2 Zinc concentration that caused a 50% inhibition of Alternaria brassicicola growth in vitro (Alternaria brassicicola EC50) and theoretical correspondence between Zn leaf concentration and Zn concentration in solution in Noccaea caerulescens plants grown at 12 and 102 µM Zn (A). Plant and pathogen response curves to metal concentration. A higher optimal metal concentration for the plant fitness than for the pathogen can lead to protection against diseases by elemental defense (B).
Nonetheless, most supporting evidence for direct Zn toxicity as a plant defense mechanism in Zn hyperaccumulating species has come from studies with insect herbivores (Boyd, 2007; Vesk and Reichman, 2009). High Zn exposure induced metal accumulation in insect bodies and eggs, causing lower fecundity (Shu et al., 2009, Shu et al., 2012), reduced growth, and higher mortality rates (Nursita et al., 2005; Jhee et al., 2006; Noret et al., 2007; Lagisz, 2008; Kazemi-Dinan et al., 2014). The high Zn concentration in Zn hyperaccumulating species can affect insect herbivores with a chewing feeding mode. High Zn concentrations in the diet resulted in a clear deterrent effect (Behmer et al., 2005; Kazemi-Dinan et al., 2014; Kazemi-Dinan et al., 2015; Stolpe et al., 2017). Fewer studies have focused on insects with a sucking–feeding mode. A Zn-rich diet increased the growth of the generalist aphid Myzus persicae, while enrichment with Cd had no effect (Stolpe and Müller, 2016). However, the combination of both metals produced a greater negative effect on the phloem-sucking insects with respect to individual metals. The higher phloem concentrations of Cd and Zn found in the metal hyperaccumulator A. halleri growing on metal-rich soils deterred aphid infestation (Stolpe et al., 2017). Furthermore, Zn can additively enhance the toxicity to insects of other metals (Cd, Ni, or Pb). This has been observed both in studies using artificial diets (Jhee et al., 2006) and by adding leaves of hyperaccumulator plants to the insects’ diet (Kazemi-Dinan et al., 2014). A global overview concerning the influence of Zn hyperaccumulation on the plant–predator interaction is given in Table 2.
Direct metal toxicity caused by the ingestion of hyperaccumulator plants is difficult to test, as any other potential organic defense may contribute and mask the results. Most studies have been conducted using species belonging to the Brassicaceae family. In these species, glucosinolates (GS) are constitutive or stress-induced compounds that, upon damage, generate hydrolysis products active against insects and pathogens (Hopkins et al., 2009; Kissen et al., 2009).
In order to evaluate the role of Zn in plant defense, the presence of organic defense compounds such GS in Brassicaceae must be considered. For example, Stolpe et al. (2017) conducted a study on the effect of Cd and Zn exposure and aphid infestation on the phloem exudate composition of A. halleri and found higher amounts of GS in addition to Zn for Zn-supplemented plants.
Several studies on the feeding preferences of snails with Thaspi caerulescens and A. halleri and high Zn showed that Zn-hyperaccumulation did not play a key role in leaf palatability, with GS proving more efficient than Zn in deterring these generalist herbivores (Huitson and Macnair, 2003; Noret et al., 2005, Noret et al., 2007). Recently, it has been observed that the snail Cantareus aspersus preferentially feeds on leaves of the Zn-Cd-hyperaccumulator Noccaea praecox with low metal concentrations (Llugany et al., 2019) and, better yet, low Zn-Cd leaves with the lowest GS concentrations. However, more prevailing for preferred consumption than low metal and GS was a high leaf sugar concentration (Llugany et al., 2019).
High Zn Triggers Organic Defenses
Besides its potential direct toxic role in defense, high Zn leaf concentrations may contribute to defense by the priming of defense signaling pathways and enhanced structural defenses (Poschenrieder et al., 2006). High metal concentrations and pathogens trigger signaling pathways that share common elements (Mithöfer et al., 2004). Salicylic acid and jasmonates (JA) play pivotal roles in the systemic defense of plants against biotic stress (Thomma et al., 1998). Nguyen et al. (2014) reported that a JA-induced enhancement of plant DFN increased Zn tolerance in the non-hyperaccumulating species A. thaliana. Moreover, the Zn-tolerant species, A. halleri, constitutively expressed high DFN and showed an increased tolerance to the necrotrophic fungi Botrytis cinerea.
It is noteworthy that in plants with normal Zn supply, the xylem-colonizing bacteria Xylella fastidiosa requires the expression of Zn detoxification mechanisms for successful host colonization (Navarrete and De La Fuente, 2015). A recent study by Martos et al. (2016) using different non-hyperaccumulating Arabidopsis genotypes infected with the necrotrophic fungi A. brassicicola highlighted the key importance of Zn in plant defense and supported the joint effect hypothesis (Boyd, 2012), i.e., the cooperation between metal and organic defenses in plants. Camalexin is a phytoalexin that is essential for the A. thaliana resistance against A. brassicicola (Thomma et al., 1999). Experimental infection with A. brassicicola of the camalexin-deficient mutant of A. thaliana, pad3, revealed that high leaf Zn concentrations could not completely substitute the role of camalexin. However, in the wild type, Zn enhanced the JA-ethylene (ET)-dependent defense signaling pathway and the expression of PAD3, an enzyme that catalyzes the last step in camalexin synthesis (Figure 3).
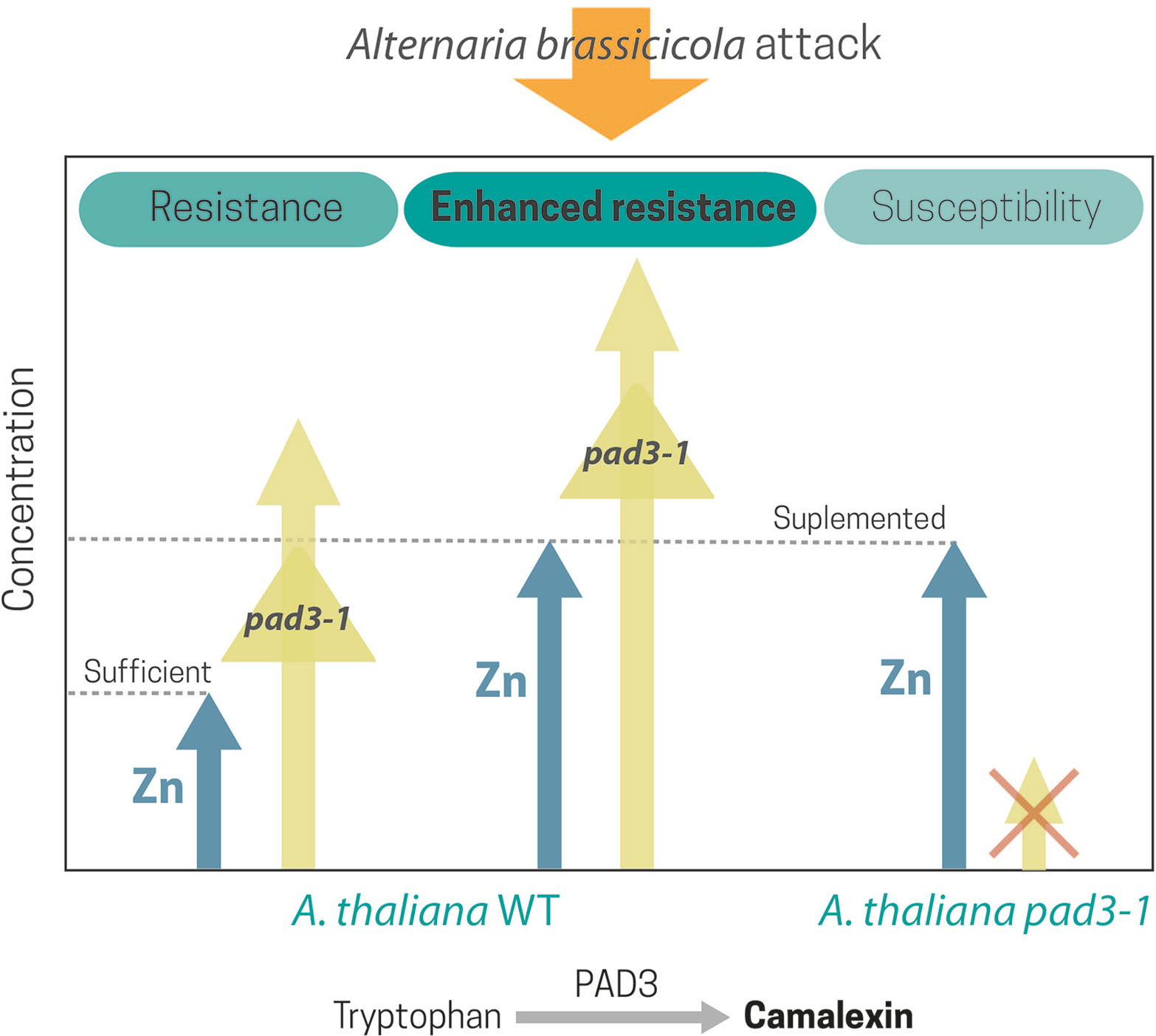
Figure 3 Zn and camalexin, a phytoalexin essential for A. thaliana resistance against Alternaria brassicicola, showed a joint effect in the Arabidopsis response to Alternaria. High leaf Zn concentration could not substitute the role of camalexin in the Arabidopsis camalexin-deficient mutant, pad3, infected with A. brassicicola. Nonetheless, in the wild type, Zn supplementation greatly enhanced the JA-ET-dependent defense signaling pathway and the expression of PAD3, an enzyme that catalyzes the last step in camalexin synthesis.
Moreover, Zn hyperaccumulation is essential for N. caerulescens resistance to A. brassicicola infection. Regardless of the Zn concentration, Alternaria triggers JA-ET- and SA-dependent defense pathways. However, only Zn hyperaccumulating plants showed incompatible interactions with the pathogen. Nonetheless, a joint effect of Zn and organic defenses could not be discarded, as higher GS concentrations were also found in Alternaria-inoculated Zn hyperaccumulating plants (Gallego et al., 2017).
Nitric oxide (NO) is reported to play a role in the plant response to toxic Zn concentrations (Xu et al., 2010). Recently, NO has been found in Solanum nigrum to participate in the expression of many Zn-mediated miRNA, with the predicted target genes indicating that excess Zn modulates pathogen tolerance and the transcriptional process by miRNA pathways (Xie et al., 2017).
Pathogens and Pests Evolve Mechanisms to Counteract Zinc-Related Plant Defense
To counteract the host-imposed Zn scarcity or toxicity, pathogens and pests have evolved efficient Zn management mechanisms and the capability to use Zn to increase virulence.
In pathogens, high Zn use efficiency implies mechanisms that lead to higher Zn import, export, or/and Zn-buffering capacity. In bacteria, the Zn uptake regulator (Zur), a Zn-responsive transcription factor, member of the Fur family of proteins, is the most common transcription factor that regulates cellular Zn uptake and efflux as well as Zn intracellular trafficking (Choi et al., 2017). Several studies have shown that Zur regulates target genes in a graded manner (Mikhaylina et al., 2018). In the model actinobacteria, Streptomyces coelicolor, Zur activates the high-affinity ABC transporter znuABC, and its homologue, znuB2C2 under Zn scarcity. At sufficient Zn levels, the expression of the Zn exporter zitB is low, and genes for Zn uptake are repressed. At high Zn, zitB induction is also elevated (Choi et al., 2017). This fine regulation of Zn activity allows pathogenic bacteria to maintain virulence over a wide range of Zn concentrations in the extracellular media. In addition, under Zn scarcity, pathogenic bacteria and fungi can use host metalloproteins as sources of metals (Neumann et al., 2017). These mechanisms have been predominately studied in human systems that use Zn-sequestering proteins for defense. These include the neutrophil protein calprotectin (CP) to restrict Zn availability at the infection sites (Striz and Trebichavsky, 2004). Host Zn chelation is a key strategy to cope with infectious process (Ma et al., 2015). However, different pathogenic bacteria have evolved strategies to use host Zn-chelating proteins, including CP, as a source of Zn (Stork et al., 2013; Jean et al., 2016; Neumann et al., 2017).
Zinc detoxification has been reported to be an important virulence factor in bacteria infecting Zn hyperaccumulator species (Fones et al., 2010), as well as non-hyperaccumulating species (Nikraftar et al., 2013). In X. fastidiosa-infected plants, the modification of the host leaf ionome correlates with bacterial virulence (Oliver et al., 2014). This xylem-colonizing bacterium has to detoxify Zn for successful host colonization. The knockout of Zur genes increases the sensitivity of X. fastidiosa to Zn and decreased its virulence (Navarrete and De La Fuente, 2015). Both pathogenic bacteria and fungi modify Zn bioavailability to enhance their virulence. High Zn concentrations induce the enhanced production of exopolysaccharides (EPS), a pathogenic factor in X. fastidiosa (Killiny et al., 2013). Enhanced EPS production also indirectly potentiates virulence, as Zn chelation by the negative charges of EPS decreases Zn bioavailability to the bacterial cell (Navarrete and De La Fuente, 2014). Similarly, high Zn concentrations in a lung secretion-mimicking medium enhanced the biofilm formation and virulence of Pseudomonas aeruginosa (Marguerettaz et al., 2014).
Fungal Zn homeostasis is regulated by a major transcription factor, the Zn responsive activator protein 1 (Zap1) (Zhao and Eide, 1997). Sufficient intracellular Zn levels are sensed by a direct interaction of the metal and the protein, causing the inhibition of the expression of Zap1 target genes (Frey and Eide, 2011). Under low Zn conditions, pathogenic fungi develop complex metal detection and signaling networks distinct to those deployed by bacteria for Zn uptake (Gerwien et al., 2018). Nonetheless, in response to Zn scarcity, fungi increase the expression of high-affinity membrane transporters to enhance Zn uptake as well as tonoplast Zn transporters that allow Zn storage into the vacuole, the zincosome (Crawford et al., 2018).
Zinc metalloproteins also play a role in the virulence of pathogenic bacteria and fungi. The oxidative burst is an early event in the plant response to diseases (Govrin and Levine, 2000). In some cases, pathogens also use ROS to enlarge their colonization process; therefore, the potential to detoxify ROS is an enhancing trait for the pathogen’s virulence. Accordingly, the reduced virulence of a B. cinerea strain defective in the expression of Cu-Zn-SOD in tomato and Arabidopsis plants was due to an increase in O2− and decreased H2O2, which was associated with an increase in callose (López-Cruz et al., 2017). On the other hand, pathogenic bacteria have evolved SOD-related mechanisms to evade the host immune system. For example, in the host cells, the withholding of Zn by CP could be expected to decrease the activity of SOD5 in Candida albicans. However, to circumvent the decrease in Zn availability, C. albicans SOD5 has lost Zn-binding activity and evolved a Cu-only cofactor requirement (Gleason et al., 2014).
Metalloproteases that use Zn as a cofactor are further potential virulence factors during bacterial and fungal infection. Metalloproteases have been reported to suppress the host immune responses in animal systems (Staats et al., 2013; Sun et al., 2016). Zn-metalloproteases, in addition to breaking down the host defensive barriers, could interfere in the host-defense mechanisms. However, their specific role in fighting the plant immune system remains elusive (Figaj et al., 2019).
Herbivores have also elaborated protective mechanisms against the presence of toxic Zn concentrations in plant tissues. The ingestion of toxic metal concentrations induces ROS causing oxidative stress in herbivores (Ihechiluru et al., 2015). In order to survive, herbivores activate an effective antioxidant defense system (enzymes and non-enzyme compounds) that inhibits the metal-induced oxidative damage (Barata et al., 2005). Variations of SOD and catalase (CAT) activities have been observed in several herbivores under metal toxicity stress (Zhang et al., 2011; Wu and Yi, 2015). Apart from antioxidant enzymes, acid phosphatase (ACP) and alkaline phosphatase (AKP) have been associated to the detoxification. An example is the observation of enhanced activity of these metalloenzymes in the lepidopter Lymantria dispar when feeding on Zn-treated Populus (Jiang and Yan, 2018).
Additionally, herbivores might deal with the presence of high levels of plant secondary metabolites in leaves as a plant defense response to metal accumulation (Huitson and Macnair, 2003; Noret et al., 2005, Noret et al., 2007). Plant secondary metabolites may induce oxidative stress similarly to that caused by heavy metals. Enhancement of glutathione (GSH) production by herbivores has been proposed as a key mechanism to cope with this stress situation (Schlaeppi et al., 2008; Barbehenn and Kochmanski, 2013). A GSH-mediated detoxification process in response to high Zn has recently been reported (Mohr et al., 2018) in a study identifying relevant novel genes that confer Zn sensitivity or tolerance in Drosophila melanogaster. The authors found that a gene encoding an ABCC-type transporter family protein, related to yeast Cd factor (YCF1) implicated in the GSH-mediated detoxification of Cd, was up-regulated by excess Zn and conferred tolerance to toxic Zn concentrations in Drosophila.
Conclusions and Future Studies
The multifunctionality of Zn in all living organisms provides this element key roles in basal metabolism, defense, and virulence, turning Zn into a highly valuable tool to understand the mechanisms underlying the plant–pest/pathogen interactions.
The presence of highly efficient Zn sensing probes and Zn detoxification systems in herbivores and pathogens indicates the relevance of Zn-related defense mechanisms in plants, which include different stratagems not restricted to Zn-hyperaccumulating species. Nonetheless, some pests and pathogens have evolved mechanisms to circumvent the plant’s Zn-poisoning strategies. Therefore, future studies should tackle this issue in a more integrative manner by contributing to the understanding of the zinc homeostatic mechanisms in plants and pests and pathogens, as well as to their interaction. Keystones for future research advances should further clarify the double role of defensins as Zn-ligand and biotic stress signaling molecules, the ligand exchange processes, and the cellular and subcellular compartmentation mechanisms for both Zn and its ligands. The outcomes of these studies would strongly contribute to visualize potential strategies that might benefit the host immune system and to design specific Zn-related tools to trick plant attackers or/and to ameliorate plant defense.
Author Contributions
All coauthors contributed to search of information and structuring of the review. CC and SM wrote the manuscript with inputs from RT, ML, and CP. BG drew the figures and CP acted as the coordinator and the supervisor.
Funding
This study was funded by the Spanish Government (Ministerio de Ciencia, Innovación y Universidades; projects BFU2013-42839-R and BFU2016-75176-R).
Conflict of Interest
The authors declare that the research was conducted in the absence of any commercial or financial relationships that could be construed as a potential conflict of interest.
References
Ahmad, S. (1992). Biochemical defence of pro-oxidant plant allelochemicals by herbivorous insects. Biochem. Syst. Ecol. 20, 269–296. doi: 10.1016/0305-1978(92)90040-K
Amtmann, A., Troufflard, S., Armengaud, P. (2008). The effect of potassium nutrition on pest and disease resistance in plants. Physiol. Plant. 133, 682–691. doi: 10.1111/j.1399-3054.2008.01075.x
Andreini, C., Bertini, I., Rosato, A. (2009). Metalloproteomes: a bioinformatic approach. Acc. Chem. Res. 42, 1471–1479. doi: 10.1021/ar900015x
Aqueel, M. A., Leather, S. R. (2011). Effect of nitrogen fertilizer on the growth and survival of Rhopalosiphum padi (L.) and Sitobion avenae (F.) (Homoptera: Aphididae) on different wheat cultivars. Crop Protect. 30, 216–221. doi: 10.1016/j.cropro.2010.09.013
Aranega-Bou, P., de la O’Leyva, M., Finiti, I., García-Agustín, P., González-Bosch, C. (2014). Priming of plant resistance by natural compounds. Hexanoic acid as a model. Front. Plant Sci. 5, 488. doi: 10.3389/fpls.2014.00488
Assunçao, A. G., Herrero, E., Lin, Y. F., Huettel, B., Talukdar, S., Smaczniak, C., et al. (2010). Arabidopsis thaliana transcription factors bZIP19 and bZIP23 regulate the adaptation to zinc deficiency. Proc. Natl. Acad. Sci. U.S.A. 107, 10296–10301. doi: 10.1073/pnas.1004788107
Barata, C., Lekumberri, I., Vila-Escalé, M., Prat, N., Porte, C. (2005). Trace metal concentration, antioxidant enzyme activities and susceptibility to oxidative stress in the tricoptera larvae Hydropsyche exocellata from the Llobregat river basin (NE Spain). Aquat. Toxicol. 74, 3–19. doi: 10.1016/j.aquatox.2005.04.002
Barbehenn, R. V., Kochmanski, J. (2013). Searching for synergism: effects of combinations of phenolic compounds and other toxins on oxidative stress in Lymantria dispar caterpillars. Chemoecol. 23, 219–231. doi: 10.1007/s00049-013-0136-z
Behmer, S. T., Lloyd, C. M., Raubenheimer, D., Stewart-Clark, J., Knight, J., Leighton, R. S., Harper, F. A., Smith, J. A. C. (2005). Metal hyperaccumulation in plants: mechanisms of defence against insect herbivores. Funct. Ecol. 19, 55–66. doi: 10.1111/j.0269-8463.2005.00943.x
Bouain, N., Satbhai, S. B., Korte, A., Saenchai, C., Desbrosses, G., Berthomieu, P., et al. (2018). Natural allelic variation of the AZI1gene controls root growth under zinc-limiting condition. PLoS Gen. doi: 10.1371/journal.pgen.1007304
Boyd, R. S., Martens, S. (1992). The Vegetation of Ultramafic (Serpentine) Soils. (Andover, Hampshire: Intercept Limited), 279–289.
Boyd, R. S. (2007). The defense hypothesis of elemental hyperaccumulation: status, challenges and new directions. Plant Soil 293, 153–176. doi: 10.1007/s11104-007-9240-6
Boyd, R. S. (2012). Plant defense using toxic inorganic ions: conceptual models of the defensive enhancement and joint effects hypotheses. Plant Sci. 195, 88–95. doi: 10.1016/j.plantsci.2012.06.012
Breitenbach, H. H., Wenig, M., Wittek, F., Jordá, L., Maldonado-Alconada, A. M., Sarioglu, H., et al. (2014). Contrasting roles of the apoplastic aspartyl protease APOPLASTIC, ENHANCED DISEASE SUSCEPTIBILITY1-DEPENDENT1 and LEGUME LECTIN-LIKE PROTEIN1 in Arabidopsis systemic acquired resistance. Plant Physiol. 165, 791–809. doi: 10.1104/pp.114.239665
Broadley, M. R., White, P. J., Hammond, J. P., Zelko, I., Lux, A. (2007). Zinc in plants. New Phytol. 173, 677–702. doi: 10.1111/j.1469-8137.2007.01996.x
Cabot, C., Gallego, B., Martos, S., Barcelo, J., Poschenrieder, C. (2013). Signal cross talk in Arabidopsis exposed to cadmium, silicon, and Botrytis cinerea. Planta 237, 337–349. doi: 10.1007/s00425-012-1779-7
Cakmak, I. M. (2000). Possible roles of zinc in protecting plant cells from damage by reactive oxygen species. New Phytol. 146, 185–205. doi: 10.1046/j.1469-8137.2000.00630.x
Calic, I., Koch, J., Carey, D., Addo-Quaye, C., Carlson, J. E., Neale, D. B. (2017). Genome-wide association study identifies a major gene for beech bark disease resistance in American beech (Fagus grandifolia Ehrh.). BMC Genomics 18, 547. doi: 10.1186/s12864-017-3931-z
Cameron, R. K., Dixon, R. A., Lamb, C. J. (1994). Biologically induced systemic acquired resistance in Arabidopsis thaliana. Plant J. 5, 715–725. doi: 10.1111/j.1365-313X.1994.00715.x
Chang, R. L., Arnold, R. J., Zhou, X. D. (2012). Association between enzyme activity levels in Eucalyptus clones and their susceptibility to the gall wasp, Leptocybe invasa, in South China. J. Trop. For. Sci. 24, 256–264.
Chaney, R. L. 1993. “Zinc phytotoxicity,” in: Zinc in soil and plants, ed. Robson, A. D.. (Dordrecht, the Netherlands: Kluwer Academic Publishers), 135–150. doi: 10.1007/978-94-011-0878-2_10
Chavez-Dulanto, P. N., Rey, B., Ubillus, C., Razuri, V., Bazan, R., Sarmiento, J. (2018). Foliar application of macro- and micronutrients for pest-mites control in citrus crops. Food Ener. Sec. 7, UNSP e00132. doi: 10.1002/fes3.132
Cheruiyot, D. J., Boyd, R. S., Moar, W. J. (2013). Exploring lower limits of plant elemental defense by cobalt, copper, nickel, and zinc. J. Chem. Ecol. 39, 666–674. doi: 10.1007/s10886-013-0279-y
Chesnais, Q., Couty, A., Catterou, M., Ameline, A. (2016). Cascading effects of N input on tritrophic (plant–aphid–parasitoid) interactions. Ecol. Evol. 6, 7882–7891. doi: 10.1002/ece3.2404
Choi, S. H., Lee, K. L., Shin, J. H., Cho, Y. B., Cha, S. S., Roe, J. H. (2017). Zinc-dependent regulation of zinc import and export genes by Zur. Nat. Comm. 8, 15812. doi: 10.1038/ncomms15812
Cipollini, D. F. (1997). Wind-induced mechanical stimulation increases pest resistance in common bean. Oecologia 111, 84–90. doi: 10.1007/s004420050211
Coleman, C. M., Boyd, R. S., Eubanks, M. D. (2005). Extending the elemental defense hypothesis: Dietary metal concentrations below hyperaccumulator levels could harm herbivores. J. Chem. Ecol. 31, 1669–1681.
Crawford, A. C., Lehtovirta-Morley, L. E., Alamir, O, Niemiec, M. J., Alawfi, B., Alsarraf, M., et al. (2018). Biphasic zinc compartmentalisation in a human fungal pathogen. PLoS Path. 14, e1007013. doi: 10.1371/journal.ppat.1007013
Datnoff, L. E., Elmer, W., Huber, D. M. (2007). Mineral nutrition and plant disease. (St Paul, Minn: APS Press). PLoS Genet. 14(4), e1007304
Deepak, S. A., Ishii, H., Park, P. (2006). Acibenzolar-S-methyl primes cell wall strengthening genes and reactive oxygen species forming/scavenging enzymes in cucumber after fungal pathogen attack. Physiol. Mol. Plant Pathol. 69, 52–61. doi: 10.1016/j.pmpp.2006.12.006
Degenhardt, J., Al-Masri, A. N., Kurkcuoglu, S., Szankowski, I., Gau, A. E. (2005). Characterization by suppression subtractive hybridization of transcripts that are differentially expressed in leaves of apple scab-resistant and susceptible cultivars of Malus domestica. Mol. Genet. Genomics 273, 326–335. doi: 10.1007/s00438-005-1136-7
Dellagi, A., Rigault, M., Segond, D., Roux, C., Kraepiel, Y., Cellier, F., et al. (2005). Siderophore-mediated upregulation of Arabidopsis ferritin expression in response to Erwinia chrysanthemi infection. Plant J. 43, 262–272. doi: 10.1111/j.1365-313X.2005.02451.x
Dordas, C. (2008). Role of nutrients in controlling plant diseases in sustainable agriculture. A review. Agron. Sust. Develop. 28, 33–46. doi: 10.1051/agro:2007051
El-Moshaty, F. I. B., Pike, S. M., Novacky, A. J., Sehgal, O. P. (1993). Lipid peroxidation and superoxide production in cowpea (Vigna unguiculata) leaves infected with tobacco ringspot virus or southern bean mosaic virus. Physiol. Mol. Plant Pathol. 43, 109–119. doi: 10.1006/pmpp.1993.1044
Fabiano, C. C., Tezotto, T., Favarin, J. L., Polacco, J. C., Mazzafera, P. (2015). Essentiality of nickel in plants: a role in plant stresses. Front. Plant Sci. 6754, 15. doi: 10.3389/fpls.2015.00754
Fageria, N. K., Baligar, V. C. and Clark R. B. (2002). Micronutrients in crops. Ad. Agron. 77, 185–268. doi: 10.1016/S0065-2113(02)77015-6
Fauteux, F., Remus-Borel, W., Menzies, J. G., Bélanger, R. R. (2005). Silicon and plant disease resistance against pathogenic fungi. FEMS Microbiol. Lett. 249, 1–6. doi: 10.1016/j.femsle.2005.06.034
Feigl, G., Lehotai, N., Molnár, Á., Ördög, A., Rodríguez-Ruiz, M., Palma, J. M., et al. (2015). Zinc induces distinct changes in the metabolism of reactive oxygen and nitrogen species (ROS and RNS) in the roots of two Brassica species with different sensitivity to zinc stress. Ann. Bot. 116, 613–625. doi: 10.1093/aob/mcu246
Fernando, D. R., Baker, A. J. M., Woodrow, I. E. (2009) Physiological responses in Macadamia integrifolia on exposure to Mn treatment. Austral. J. Bot. 57, 406–413. doi: 10.1071/BT09077
Figaj, D., Ambroziak, P., Przepiora, T., Skorko-Glonek, J. (2019). The role of proteases in the virulence of plant pathogenic bacteria. Int. J. Mol. Sci. 20, 672. doi: 10.3390/ijms20030672
Fodor, J., Gullner, G., Adam, A. L., Barna, B., Komives, T., Kiraly, Z. (1997). Local and systemic responses of antioxidants to tobacco mosaic virus infection and to salicylic acid in tobacco (role in systemic acquired resistance). Plant Physiol. 114, 1443–1451. doi: 10.1104/pp.114.4.1443
Fones, H., Davis, C., Fang, F., Rico, A., Smith, J. A. C., Preston, G. M. (2010). Metal hyperaccumulation armors plants against disease. PLoS Path. 6, e1001093. doi: 10.1371/journal.ppat.1001093
Fones, H. N., Preston, G. M. (2012). Reactive oxygen and oxidative stress tolerance in plant pathogenic Pseudomonas. FEMS Microbiol. Lett. 327, 1–8. doi: 10.1111/j.1574-6968.2011.02449.x
Fones, H. N., Preston, G. M. (2013). The impact of transition metals on bacterial plant disease. FEMS Microbiol. Rev. 37, 495–519. doi: 10.1111/1574-6976.12004
Forde, B. G., Lea, P. J. (2007). Glutamate in plants: metabolism, regulation, and signalling. J. Exp. Bot. 58, 2339–2358. doi: 10.1093/jxb/erm121
Fourcroy, P., Susó-Terraza, P., Sudre, D., Savirón, M., Reyt, G., Gaymard, F., et al. (2014). Involvement of the ABCG37 transporter in secretion of scopoletin and derivatives by Arabidopsis roots in response to iron deficiency. New Phytol. 201, 155–167. doi: 10.1111/nph.12471
Frey, A. G., Eide, D. J. (2011). Roles of two activation domains in Zap1 in the response to zinc deficiency in Saccharomyces cerevisiae. J. Biol. Chem. 286, 6844–6854. doi: 10.1074/jbc.M110.203927
Gallego, B., Martos, S., Cabot, C., Barcelo, J., Poschenrieder, C. (2017). Zinc hyperaccumulation substitutes for defense failures beyond salicylate and jasmonate signaling pathways of Alternaria brassicicola attack in Noccaea caerulescens. Physiol. Plant. 159, 401–415. doi: 10.1111/ppl.12518
Gerwien, F., Skranina, V., Kasper, L., Hube, B., Brunke, S. (2018). Metals in fungal virulence. FEMS Microbiol. Rev. 42, 1–12. doi: 10.1093/femsre/fux050
Gleason, J. E., Galaleldeen, A., Peterson, R. L., Taylor, A. B., Holloway, S. P., Waninger-Saroni, J., et al. (2014). Candida albicans SOD5 represents the prototype of an unprecedented class of Cu-only superoxide dismutases required for pathogen defense. PNAS 111, 5866–5871. doi: 10.1073/pnas.1400137111
Goldberg, J. M., Lippard, S. J. (2018). New tools uncover new functions for mobile zinc in the brain. Biochem. 57, 3991–3992. doi: 10.1021/acs.biochem.8b00108
Govrin, E. M., Levine, A. (2000). The hypersensitive response facilitates plant infection by the necrotrophic pathogen Botrytis cinerea. Curr. Biol. 10, 751–757. doi: 10.1016/S0960-9822(00)00560-1
Graham, D. R., Webb, M. J. (1991). “Micronutrients and disease resistance and tolerance in plants,” in Micronutrients in agriculture, 2nd Edn, eds Mortvedt, J. J., Cox, F. R., Shuman, L. M., Welch, R. M.. (Madison, Wisconsin, USA, Soil Science Society of America Inc.), 329–370.
Greenshields, D. L., Liu, G., Wei, Y. (2007). Roles of iron in plant defence and fungal virulence. Plant Sig. Beh. 2, 300–302. doi: 10.4161/psb.2.4.4042
Grewal, H. S., Graham, R. D., Rengel, Z. (1996). Genotypic variation in zinc efficiency and resistance to crown rot disease (Fusarium graminearum Schw Group 1) in wheat. Plant Soil 186, 219–226. doi: 10.1007/BF02415517
Grewal, H. S. (2001). Zinc influences nodulation, disease severity, leaf drop and herbage yield of alfalfa cultivars. Plant Soil 234, 47–59. doi: 10.1023/A:1010544601267
Gupta, S. K., Rai, A. K., Kanwar, S. S., Sharma, T. R. (2012a). Comparative analysis of zinc finger proteins involved in plant disease resistance. PLoS One 7, e42578. doi: 10.1371/journal.pone.0042578
Gupta, S. K., Rai, A. K., Kanwar, S. S., Chand, D., Singh, N. K., Sharma, T. R. (2012b). The single functional blast resistance gene Pi54 activates a complex defence mechanism in rice. J. Exp. Bot. 63, 757–772. doi: 10.1093/jxb/err297
Hacisalihoglu, G., Kochian, L. V. (2003). How do some plants tolerate low levels of soil zinc? Mechanisms of zinc efficiency in crop plants. New Phytol. 159, 341–350. doi: 10.1046/j.1469-8137.2003.00826.x
Hambidge, M., Cousins, R. J., Costello, R. B. (2000). Zinc and health: current status and future directions. J. Nutr. 130, 1437S–1446S. doi: 10.1093/jn/130.5.1341S
Haydon, M. J., Kawachi, M., Wirtz, M., Hillmer, S., Hell, R., Kramer, U. (2012). Vacuolar nicotianamine has critical and distinct roles under iron deficiency and for zinc sequestration in Arabidopsis. Plant Cell 24, 724–737. doi: 10.1105/tpc.111.095042
He, W. Q., Yang, M., Li, Z. H., Qiu, J. L., Liu, F., Qu, X. S., et al. (2015). High levels of silicon provided as a nutrient in hydroponic culture enhances rice plant resistance to brown planthopper. Crop Protec. 67, 20–25. doi: 10.1016/j.cropro.2014.09.013
Helfenstein, J., Pawlowski, M. L., Hill, C. B., Stewart, J., Lagos-Kutz, D., Bowen, C. R., et al. (2015). Zinc deficiency alters soybean susceptibility to pathogens and pests. J. Plant Nutr. Soil Sci. 178, 896–903. doi: 10.1002/jpln.201500146
Hojyo, S., Fukada, T. (2016). Roles of zinc signalling in the immune system. J. Immunol. Res. (2016). 21. doi: 10.1155/2016/6762343
Hood, M. I., Skaar, E. P. (2012). Nutritional immunity: transition metals at the pathogen–host interface. Nat. Rev. Microbiol. 10, 525–537. doi: 10.1038/nrmicro2836
Hopkins, R. J., van Dam, N. M., van Loon, J. J. A. (2009). Role of glucosinolates in insect–plant relationships and multitrophic interactions. Annu. Rev. Entomol. 54, 57–83. doi: 10.1146/annurev.ento.54.110807.090623
Hsiao, P. Y., Cheng, C. P., Koh, K. W., Chan, M. T. (2017). The Arabidopsis defensin gene, AtPDF1.1, mediates defence against Pectobacterium carotovorum subsp. Carotovorum via an iron-withholding defence system. Scient. Rep. 7, 9175. doi: 10.1038/s41598-017-08497-7
Huber, D. M. (1980). “The role of mineral nutrition in defense,” in Plant disease: An advanced treatise, Vol. 5, How plants defend themselves, eds Horsfall, J. G., Cowling, E. B. (New York, Academic Press), 381–406. doi: 10.1016/B978-0-12-356405-4.50028-9
Huber, D. M., Haneklaus, S. (2007). Managing nutrition to control plant disease. Landbauforsch. Volk. 4, 313–322.
Huitson, S. B., Macnair, M. R. (2003). Does zinc protect the zinc hyperaccumulator Arabidopsis halleri from herbivory by snails? New Phytol. 159, 453–459. doi: 10.1046/j.1469-8137.2003.00783.x
Ihechiluru, N. B., Henry, A. N., Taiwo, I. E. (2015). Heavy metal bioaccumulation and oxidative stress in Austroaeschna inermis (dragon fly) of the lagos urban ecosystem. J. Environ. Chem. Ecotoxicol. 7, 11–19. doi: 10.5897/JECE2014.0336
Inaba, S., Kurata, R., Kobayashi, M., Yamagishi, Y., Mori, I., Ogata, Y., et al. (2015). Identification of putative target genes of bZIP19, a transcription factor essential for Arabidopsis adaptation to Zn deficiency in roots. Plant J. 84, 323–334. doi: 10.1111/tpj.12996
Jain, R., Srivastava, S., Solomon, S., Shrivastava, A. K., Chandra, A. (2010). Impact of excess zinc on growth parameters, cell division, nutrient accumulation, photosynthetic pigments and oxidative stress of sugarcane (Saccharum spp.). Acta Physiol. Plantar. 32, 979–986. doi: 10.1007/s11738-010-0487-9
Jakupovic, M., Heintz, M., Reichmann, P., Mendgen, K., Hahn, M. (2006). Microarray analysis of expressed sequence tags from haustoria of the rust fungus Uromyces fabae. Fungal Genet. Biol. 43, 8–19. doi: 10.1016/j.fgb.2005.09.001
Jean, S., Juneau, R. A., Driss, A. K., Cornelissen, C. N. (2016). Neisseria gonorrhoeae evades calprotectin-mediated nutritional immunity and survives neutrophil extracellular traps by production of TdfH. Infect. Immun. 84, 2982–2994. doi: 10.1128/IAI.00319-16
Jhee, E. M., Dandridge, K. L., Christy, A. M., Jr., Pollard, A. J. (1999). Selective herbivory on low-zinc phenotypes of the hyperaccumulator Thlaspi caerulescens (Brassicaceae). Chemoecol. 9, 93–95.
Jhee, E. M., Boyd, R. S., Eubanks, M. D. (2006). Effectiveness of metal–metal and metal–organic compound combinations against Plutella xylostella: implications for plant elemental defense. J. Chem. Ecol. 32, 239–259. doi: 10.1007/s10886-005-9000-0
Jiang, D., Yan, S. (2018). Effects of Cd, Zn, or Pb stress in Populus alba berolinensis on the antioxidant, detoxifying, and digestive enzymes of Lymantria dispar. Environ. Entomol. 47, 1323–1328. doi: 10.1093/ee/nvy084
Jones-Rhoades, M. W., Bartel, D. P., Bartel, B. (2006). MicroRNAs and their regulatory roles in plants. Ann. Rev. Plant Biol. 57, 19–53. doi: 10.1146/annurev.arplant.57.032905.105218
Jung, H. W., Tschaplinski, T. J., Wang, L., Glazebrook, J., Greenberg, J. T. (2009). Priming in systemic plant immunity. Science 324, 89–91. doi: 10.1126/science.1170025
Kazemi-Dinan, A., Barwinski, A., Stein, R. J., Kramer, U., Muller, C. (2015). Metal hyperaccumulation in Brassicaceae mediates defense against herbivores in the field and improves growth. Entomol. Exp. Appl. 157, 3–10. doi: 10.1111/eea.12333
Kazemi-Dinan, A., Thomaschky, S., Stein, R. J., Kramer, U., Muller, C. (2014). Zinc and cadmium hyperaccumulation act as deterrents towards specialist herbivores and impede the performance of a generalist herbivore. New Phytol. 202, 628–639. doi: 10.1111/nph.12663
Kettle, A. J., Carere, J., Batley, J., Manners, J. M., Kazan, K., Gardiner, D. M. (2016). The Fdb3 transcription factor of the Fusarium detoxification of benzoxazolinone gene cluster is required for MBOA but not BOA degradation in Fusarium pseudograminearum. Fungal Genet. Biol. 88, 44–53. doi: 10.1016/j.fgb.2016.01.015
Khederi, S. J., Khanjani, M., Gholami, M., Panzarino, O., de Lillo, E. (2018). Influence of the erineum strain of Colomerus vitis (Acari: Eriophyidae) on grape (Vitis vinifera) defense mechanisms. Exp. Appl. Acarol. 75, 1–24. doi: 10.1007/s10493-018-0252-0
Khoshgoftarmanesh, A. H., Kabiri, S., Shariatmadari, H., Sharifnabi, B., Schulin, R. (2010). Zinc nutrition effect on the tolerance of wheat genotypes to Fusarium root-rot disease in a solution culture experiment. Soil Sci. Plant Nutr. 56, 234–243. doi: 10.1111/j.1747-0765.2009.00441.x
Killiny, N., Martinez, R. H., Dumenyo, C. K., Cooksey, D. A., Almeida, R. P. (2013). The exopolysaccharide of Xylella fastidiosa is essential for biofilm formation, plant virulence, and vector transmission. Mol. Plant Mic. Interact. 26, 1044–1053. doi: 10.1094/MPMI-09-12-0211-R
Kim, S., Ahn, I. P., Lee, Y. H. (2001). Analysis of genes expressed during rice–Magnaporthe grisea interactions. Mol. Plant Microbe Interact. 14, 1340–1346. doi: 10.1094/MPMI.2001.14.11.1340
Kim, M. S., Kim, H. S., Kim, Y. S., Baek, K. H., Moon, J. S., Choi, D., et al. (2007). Expression of lily chloroplastic Cu, Zn superoxide dismutase enhances resistance to Erwinia carotovora in potatoes. Plant Pathol. J. 23, 300–307. doi: 10.5423/PPJ.2007.23.4.300
Kim, S. H., Hong, J. K., Lee, S. C., Sohn, K. H., Jung, H. W., Hwang, B. K. (2004). CAZFP1, CYS2/HiS(2)-type zinc-finger transcription factor gene functions as a pathogen-induced early-defense gene in Capsicum annuum. Plant Mol. Biol. 55, 883–904. doi: 10.1007/s11103-004-2151-5
Kinraide, T. B., Poschenrieder, C., Kopittke, P. M. (2011). The standard electrode potential (Eq) predicts the prooxidant activity and the acute toxicity of metal ions. J. Inorg. Biochem. 105, 1438–1445. doi: 10.1016/j.jinorgbio.2011.08.024
Kissen, R., Rossiter, J. T., Bones, A. M. (2009). The ‘mustard oil bomb’: Not so easy to assemble? Localization, expression and distribution of the components of the myrosinase enzyme system. Phytochem. Rev. 8, 69–86. doi: 10.1007/s11101-008-9109-1
Kumar, D., Rampuria, S., Singh, N. K., Kirti, P. B. (2016). A novel zinc-binding alcohol dehydrogenase 2 from Arachis diogoi, expressed in resistance responses against late leaf spot pathogen, induces cell death when transexpressed in tobacco. FEBS Open Bio. 6, 200–210. doi: 10.1002/2211-5463.12040
Lagisz, M. (2008). Changes in morphology of the ground beetle Pterostichus oblongopunctatus F. (Coleoptera; Carabidae) from vicinities of a zinc-and-lead smelter. Environ. Toxicol. Chem. 27, 1744–1747. doi: 10.1897/07-661.1
Laity, J. H., Lee, B. M., Wright, P. E. (2001). Zinc finger proteins: New insights into structural and functional diversity. Curr. Op. Str. Biol. 11, 39–46. doi: 10.1016/S0959-440X(00)00167-6
Lawrence, S. D., Novak, N. G., Jones, R. W., Farrar, R. R., Blackburn, M. B. (2014). Herbivory responsive C2H2 zinc finger transcription factor protein StZFP2 from potato. Plant Physiol. Biochem. 80, 226–233. doi: 10.1016/j.plaphy.2014.04.010
Lehman, M. C., Pahls, D. R., Meredith, J. M., Sommer, R. D., Heinekey, D. M., Cundari, T. R., et al. (2015). Oxyfunctionalization with Cp*Ir-III(NHC)(Me)(CI) with O-2: Identification of a rare bimetallic Ir-IV mu-Oxo intermediate. J. Am. Chem. Soc. 137, 3574–3584. doi: 10.1021/ja512905t
Li, Z., Fan, Y. C., Gao, L., Cao, X, Ye, J. L., Li, G. H. (2016). The dual roles of zinc sulfate in mitigating peach gummosis. Plant Dis. 100, 345–351. doi: 10.1094/PDIS-01-15-0131-RE
Liu, G., Greenshields, D. L., Sammynaiken, R., Hirji, R. N., Selvaraj, G., Wei, Y. (2007). Targeted alterations in iron homeostasis underlie plant defense responses. J. Cell Sci. 120, 596–605. doi: 10.1242/jcs.001362
Liu, J., Guan, T., Zheng, P., Chen, L., Yang, Y., Huai, B., et al. (2016). An extracellular Zn-only superoxide dismutase from Puccinia striiformis confers enhanced resistance to host-derived oxidative stress. Environ. Microbiol. 18, 4118–4135. doi: 10.1111/1462-2920.13451
Liu, Y., Wu, H., Kou, L., Liu, X., Zhang, J., Guo, Y., et al. (2014). Two metallothionein genes in Oxya chinensis: molecular characteristics, expression patterns and roles in heavy metal stress. PLoS One 9, e112759. doi: 10.1371/journal.pone.0112759
Liu, Y. L., Schiff, M., Marathe, R., Dinesh-Kumar, S. P. (2002). Tobacco Rar1, EDS1 and NPR1/NIM1 like genes are required for N-mediated resistance to tobacco mosaic virus. Plant J. 30, 415–429. doi: 10.1046/j.1365-313X.2002.01297.x
Llugany, M., Tolrà, R., Barceló, J., Poschenrieder, C. (2019). Snails prefer it sweet: A multifactorial test of the metal defence hypothesis. Physiol. Plant. 165, 209–218. doi: 10.1111/ppl.12821
López-Cruz, J., Oscar, C. S., Emma, F. C., Pilar, G. A., Carmen, G. B. (2017). Absence of Cu-Zn superoxide dismutase BCSOD1 reduces Botrytis cinerea virulence in Arabidopsis and tomato plants, revealing interplay among reactive oxygen species, callose and signalling pathways. Mol. Plant Pathol. 18, 16–31. doi: 10.1111/mpp.12370
Ma, L., Terwilliger, A., Maresso, A. W. (2015). Iron and zinc exploitation during bacterial pathogenesis. Metallomics 7, 1541–1554. doi: 10.1039/C5MT00170F
Machado, P. P., Steiner, F., Zuffo, A. M., Machado, R. A. (2018). Could the supply of boron and zinc improve resistance of potato to early blight? Potato Res. 61, 169–182. doi: 10.1007/s11540-018-9365-4
Marguerettaz, M., Dieppois, G., Que, Y. A., Ducret, V., Zuchuat, S., Perron, K. (2014). Sputum containing zinc enhances carbapenem resistance, biofilm formation and virulence of Pseudomonas aeruginosa. Microb. Pathog. 77, 36–41. doi: 10.1016/j.micpath.2014.10.011
Marschner, H., Marschner, P. (2012). Marschner’s mineral nutrition of higher plants, 3rd ed. (San Diego, Elsevier Academic Press), 1–651.
Martinez-Cruz, J., Romero, D., de la Torre, F. N., Fernandez-Ortuno, D., Tores, J. A., de Vicente, A., et al. (2018). The functional characterization of Podosphaera xanthii candidate effector genes reveals novel target functions for fungal pathogenicity. Mol. Plant Microbe Interact. 31, 914–931. doi: 10.1094/MPMI-12-17-0318-R
Martos, S., Gallego, B., Cabot, C., Llugany, M., Barceló, J., Poschenrieder, C. (2016). Zinc triggers signaling mechanisms and defense responses promoting resistance to Alternaria brassicicola in Arabidopsis thaliana. Plant Sci. 249, 13–24. doi: 10.1016/j.plantsci.2016.05.001
McAllister, B. B., Dyck, R. H. (2017). Zinc transporter 3 (ZnT3) and vesicular zinc in central nervous system function. Neurosci. Biobehav. Rev. 80, 329–350. doi: 10.1016/j.neubiorev.2017.06.006
McDevitt, C. A., Ogunniyi, A. D., Valkov, E., Lawrence, M. C., Kobe, B., McEwan, A. G., et al. (2011). A molecular mechanism for bacterial susceptibility to zinc. PLoS Path. 7, e1002357. doi: 10.1371/journal.ppat.1002357
Mikhaylina, A., Ksibe, A. Z., Scanlan, D. J., Blindauer, C. A. (2018). Bacterial zinc uptake regulator proteins and their regulons. Biochem. Soc. Transac. 46, 98–101. doi: 10.1042/BST20170228
Mila, I., Scalbert, A., Expert, D. (1998). Iron withholding by plant polyphenols and resistance to pathogens and rots. Phytochem. 42, 1551–1555. doi: 10.1016/0031-9422(96)00174-4
Miller, G., Shulaev, V., Mittler, R. (2008). Reactive oxygen signaling and abiotic stress. Physiol. Plant 133, 481–489. doi: 10.1111/j.1399-3054.2008.01090.x
Mithöfer, A., Schulze, B., Boland, W. (2004). Biotic and heavy metal stress response in plants: Evidence for common signals. FEBS Lett. 566, 1–5. doi: 10.1016/j.febslet.2004.04.011
Mohr, S. E., Rudd, K., Hu, Y., Song, W. R., Gilly, Q., Buckner, M., et al. (2018). Zinc detoxification: a functional genomics and transcriptomics analysis in Drosophila melanogaster cultured cells. G3 Genes Genom. Genet. 8, 631–641. doi: 10.1534/g3.117.300447
Montalbini, P., Buonaurio, R. (1986). Effect of tobacco mosaic virus infection on levels of soluble superoxide dismutase (SOD) in Nicotiana tabacum and Nicotiana glutinosa leaves. Plant Sci. 47, 135–143. doi: 10.1016/0168-9452(86)90060-9
Navarrete, F., De La Fuente, L. (2014). Response of Xylella fastidiosa to zinc: decreased culturability, increased exopolysaccharide production, and formation of resilient biofilms under flow conditions. Appl. Environ. Microbiol. 80, 1097–1107. doi: 10.1128/AEM.02998-13
Navarrete, F., De La Fuente, L. (2015). Zinc detoxification is required for full virulence and modification of the host leaf ionome by Xylella fastidiosa. Mol. Plant Mic. Inter. 28, 497–507. doi: 10.1094/MPMI-07-14-0221-R
Neumann, W., Hadley, R. C., Nolan, E. M. (2017). Transition metals at the host–pathogen interface: how Neisseria exploit human metalloproteins for acquiring iron and zinc. Essays Biochem. 61, 211–222. doi: 10.1042/EBC20160084
Nguyen, N. N. T., Ranwez, V., Vile, D., Soulie, M. C., Dellagi, A., Expert, D., et al. (2014). Evolutionary tinkering of the expression of PDFls suggests their joint effect on zinc tolerance and the response to pathogen attack. Front. Plant Sci. 5, 70. doi: 10.3389/fpls.2014.00070
Nikraftar, F., Taheri, P., Rastegar, M. F., Tarighi, S. (2013). Tomato partial resistance to Rhizoctonia solani involves antioxidative defense mechanisms. Physiol. Mol. Plant Pathol. 81, 74–83. doi: 10.1016/j.pmpp.2012.11.004
Noman, A., Liu, Z., Aqeel, M., Zainab, M., Khan, M. I. K., Hussain, A. (2017a). Basic leucine zipper domain transcription factors: the vanguards in plant immunity. Bioch. Let. 39, 1779–1791. doi: 10.1007/s10529-017-2431-1
Noman, A., Fahad, S., Aqeel, M., Ali, U., Anwar, A. S., Baloch, S. K., Zainab, M. (2017b). miRNAs: major modulators for crop growth and development under abiotic stresses. Biotechnol. Lett. 39, 685–700. doi: 10.1007/s10529-017-2302-9
Noman, A., Ali, Q., Maqsood, J., Iqbal, N., Javed, M. T., Rasool, N., Nasem, J. (2018). Deciphering physio-biochemical, yield, and nutritional quality attributes of water-stressed radish (Raphanus sativus L.) plants grown from Zn-Lys primed seeds. Chemosphere 195, 175–189. doi: 10.1016/j.chemosphere.2017.12.059
Noman, A., Hussain, A., Ashraf, M. F., Khan, M. I., Liu, Z., He, S. (2019a). CabZIP53 is targeted by CaWRKY40 and act as positive regulator in pepper defense against Ralstonia solanacearum and thermotolerance. Environ. Exp. Bot. 159, 138–148. doi: 10.1016/j.envexpbot.2018.12.017
Noman, A.; Aqeel, M., Khalid, N., Islam, W., Sanaullah, T., Anwar, M., Khan, S., Ye, W., Lou, Y. (2019b). Zinc finger protein transcription factors: integrated line of action for plant antimicrobial activity. Microb. Pathog. 132, 141–149. doi: 10.1016/j.micpath.2019.04.042
Noret, N., Josens, G., Escarre, J., Lefebvre, C., Panichelli, S., Meerts, P. (2007). Development of Issoria lathonia (Lepidoptera: Nymphalidae) on zinc-accumulating and nonaccumulating Viola species (Violaceae). Environ. Toxicol. Chem. 26, 565–571. doi: 10.1897/06-413R.1
Noret, N., Meerts, P., Tolrà, R., Poschenrieder, C., Barceló, J., Escarré, J. (2005). Palatability of Thlaspi caerulescens for snails: influence of zinc and glucosinolates. New Phytol. 165, 763–772. doi: 10.1111/j.1469-8137.2004.01286.x
Nursita, A. I., Balwant, S., Lees, E. (2005). The effect of cadmium, copper, lead, and zinc on the growth and reproduction of Priosotoma minuta Tullberg (Collembola). Ecotoxicol. Environ. Saf. 60, 306–314. doi: 10.1016/j.ecoenv.2004.05.001
Oliver, J. E., Sefick, S. A., Parker, J. K., Arnold, T., Cobine, P. A., De La Fuente, L. (2014). Ionome changes in Xylella fastidiosa-infected Nicotiana tabacum correlate with virulence and discriminate between subspecies of bacterial isolates. Mol. Plant Mic. Interact. 27, 1048–1058. doi: 10.1094/MPMI-05-14-0151-R
Pollard, A. J., Baker, A. J. M. (1997). Deterrence of herbivory by zinc hyperaccumulation in Thlaspi caerulescens (Brassicaceae). New Phytol. 135, 655–658.
Poschenrieder, C., Tolrà, R., Barceló, J. (2006). Can metals defend plants against biotic stress? Trends Plant Sci. 11, 288–295. doi: 10.1016/j.tplants.2006.04.007
Pritsos, C. A., Pastorf, J., Pardini, R. S. (1991). Role of superoxide dismutase in the protection and tolerance to the prooxidant allelochemical quercetin in Papilio polyxenes, Spodoptera eridania, and Trichloplusia ni. Arch. Insect Biochem. Physiol. 16, 273–282. doi: 10.1002/arch.940160406
Restrepo, S., Myers, K. L., del Pozo, O. Martin, G. B., Hart, A. L., Buell, C. R., et al. (2005). Gene profiling of a compatible interaction between Phytophthora infestans and Solanum tuberosum suggests a role for carbonic anhydrase. Mol. Plant Microbe Interact. 18, 913–922. doi: 10.1094/MPMI-18-0913
Rodríguez-Celma, J., Lin, W. D., Fu, G. M., Abadía, J., López-Millán, A. F., Schmidt, W. (2013). Mutually exclusive alterations in secondary metabolism are critical for the uptake of insoluble iron compounds by Arabidopsis and Medicago truncatula. Plant Physiol. 162, 1473–1485. doi: 10.1104/pp.113.220426
Rodríguez-Celma, J., Tsai, Y. H., Wen, T. N., Wu, Y. C., Curie, C., Schmidt, W. (2016). Systems-wide analysis of manganese deficiency-induced changes in gene activity of Arabidopsis roots. Scien. Rep. 6, 35846. doi: 10.1038/srep35846
Rose, M. T., Rose, T. J., Pariasca-Tanaka, J., Yoshihashi, T., Neuweger, H., Goesmann, A., et al. (2012). Root metabolic response of rice (Oryza sativa L.) genotypes with contrasting tolerance to zinc deficiency and bicarbonate excess. Planta 236, 959–973. doi: 10.1007/s00425-012-1648-4
Ruano, A., Poschenrieder, C., Barceló, J. (1988). Growth and biomass partitioning in zinc-toxic bush beans. J. Plant Nutr. 11, 577–588. doi: 10.1080/01904168809363824
Schlaeppi, K., Bodenhausen, N., Buchala, A., Mauch, F., Reymond, P. (2008). The glutathione-deficient mutant pad2-1 accumulates lower amounts of glucosinolates and is more susceptible to the insect herbivore Spodoptera littoralis. Plant J. 55, 774–786. doi: 10.1111/j.1365-313X.2008.03545.x
Schmid, N. B., Giehl, R. F., Döll, S., Mock, H. P., Strehmel, N., Scheel, D., et al. (2014). Feruloyl-CoA 6′-Hydroxylase1-dependent coumarins mediate iron acquisition from alkaline substrates in Arabidopsis. Plant Physiol. 164, 160–172. doi: 10.1104/pp.113.228544
Schweizer, F., Bodenhausen, N., Lassueur, S., Masclaux, F. G., Reymond, P. (2013). Differential contribution of transcription factors to Arabidopsis thaliana defense against Spodoptera littoralis. Front. Plant Sci. 4, 13. doi: 10.3389/fpls.2013.00013
Shirasu, K., Nielsen, K., Piffanelli, P., Oliver, R., Schulze-Lefert, P. (1999). Cell-autonomous complementation of mlo resistance using a biolistic transient expression system. Plant J. 17, 293–299. doi: 10.1046/j.1365-313X.1999.00376.x
Shivashankar, S., Sumathi, M., Krishnakuma, R. N. K., Ra, V. K. (2015). Role of phenolic acids and enzymes of phenylpropanoid pathway in resistance of chayote fruit (Sechium edule) against infestation by melon fly, Bactrocera cucurbitae. Ann. Appl. Biol. 166, 420–433. doi: 10.1111/aab.12194
Shu, Y., Gao, Y., Sun, H., Zou, Z., Zhou, Q., Zhang, G. (2009). Effects of zinc exposure on the reproduction of Spodoptera litura Fabricius (Lepidoptera: Noctuidae). Ecotoxicol. Environ. Saf. 72, 2130–2136. doi: 10.1016/j.ecoenv.2009.06.004
Shu, Y. H., Zhang, G. R., Wang, J. W. (2012). Response of the common cutworm Spodoptera litura to zinc stress: Zn accumulation, metallothionein and cell ultrastructure of the midgut. Sci. Total Environ. 438, 210–217. doi: 10.1016/j.scitotenv.2012.06.065
Simmons, C. R., Fridlender, M., Navarro, P. A., Yalpani, N. (2003). A maize defense-inducible gene is a major facilitator superfamily member related to bacterial multidrug resistance efflux antiporters. Plant Mol. Biol. 52, 433–446. doi: 10.1023/A:1023982704901
Simoglou, K. B., Dordas, C. (2006). Effect of foliar applied boron, manganese and zinc on tan spot in winter durum wheat. Crop Prot. 25, 656–663. doi: 10.1016/j.cropro.2005.09.007
Sinclair, S. A., Kramer, U. (2012). The zinc homeostasis network of land plants. Biochim. Biophys. Acta 1823, 1553–1567. doi: 10.1016/j.bbamcr.2012.05.016
Staats, C. C., Kmetzsch, L., Schrank, A., Vainstein, M. H. (2013). Fungal zinc metabolism and its connections to virulence. Front. Cell. Infect. Microbiol. 3, 65. doi: 10.3389/fcimb.2013.00065
Stolpe, C., Müller, C. (2016). Effects of single and combined heavy metals and their chelators on aphid performance and preferences. Environ. Toxicol. Chem. 35, 3023–3030. doi: 10.1002/etc.3489
Stolpe, C., Giehren, F., Krämer, U., Müller, C. (2017). Both heavy metal-amendment of soil and aphid-infestation increase Cd and Zn concentrations in phloem exudates of a metal-hyperaccumulating plant. Phytochem. 139, 109–117. doi: 10.1016/j.phytochem.2017.04.010
Stork, M., Grijpstra, J., Bos, M. P., Mañas-Torres, C., Devos, N., Poolman, J. T., et al. (2013). Zinc piracy as a mechanism of Neisseria meningitidis for evasion of nutritional immunity. PLoS Pathog. 9, e1003733. doi: 10.1371/journal.ppat.1003733
Stotz, H. U., Thomson, J. G., Wang, Y. (2009). Plant defensins. Defense, development and application. Plant Sig. Behav. 4, 1010–1012. doi:10.4161/psb.4.11.9755
Strauss, S. Y., Rudgers, J. A., Lau, J. A., Irwin, R. E. (2002). Direct and ecological costs of resistance to herbivory. Trends Ecol. Evol. 17, 278–285. doi: 10.1016/S0169-5347(02)02483-7
Strauss, S. Y., Irwin, R. E., Lambrix, V. M. (2004). Optimal defence theory and flower petal colour predict variation in the secondary chemistry of wild radis. J. Ecol. 92, 132–141. doi: 10.1111/j.1365-2745.2004.00843.x
Streeter, T. C., Rengel, Z., Neate, S. M., Graham, R. D. (2001). Zinc fertilisation increases tolerance to Rhizoctonia solani (AG 8) in Medicago truncatula. Plant Soil 228, 233–242. doi: 10.1023/A:1004874027331
Striz, I., Trebichavsky, I. (2004). Calprotectin—A pleiotropic molecule in acute and chronic inflammation. Physiol. Res. 53, 245–253.
Subramanian, B., Bansal, V. K., Kav, N. N. V. (2005). Proteome-level investigation of Brassica carinata-derived resistance to Leptosphaeria maculans. J. Agric. Food Chem. 53, 313–324. doi: 10.1021/jf048922z
Sun, H., Kamanova, J., Lara-Tejero, M., Galan, J. E. (2016) A family of Salmonella Type III secretion effector proteins selectively targets the NF-kB signaling pathway to preserve host homeostasis. PLoS Pathog. 12, e1005484. doi: 10.1371/journal.ppat.1005484
Sunkar, R., Kapoor, A., Zhu, J. K. (2006). Posttranscriptional induction of two Cu/Zn superoxide dismutase genes in Arabidopsis is mediated by downregulation of miR398 and important for oxidative stress tolerance. Plant Cell 18, 2051–2065. doi: 10.1105/tpc.106.041673
Sutherland, M. W. (1991). The generation of oxygen radicals during host plant responses to infection. Physiol. Mol. Plant Pathol. 39, 79–93. doi: 10.1016/0885-5765(91)90020-I
Thomma, B. P. H. J., Eggermont, K., Penninckk, I. A. M. A., MauchMani, B., Vogelsang, R., Cammue, B. P. A., Broekaert, W. F. (1998). Separate jasmonate-dependent and salicylate-dependent defense-response pathways in Arabidopsis are essential for resistance to distinct microbial pathogens. Proc. Natl. Acad. Sci. U.S.A. 95, 15107–15111. doi: 10.1073/pnas.95.25.15107
Thomma, B. P. H. J., Nelissen, I., Eggermont, K., Broekaert, W. F. (1999). Deficiency in phytoalexin production causes enhanced susceptibility of Arabidopsis thaliana to the fungus Alternaria brassicicola. Plant J. 19, 163–171. doi: 10.1046/j.1365-313X.1999.00513.x
Tornero, P., Merritt, P., Sadanandom, A., Shirasu, K., Innes, R. W., Dangl, J. L. (2002). RAR1 and NDR1 contribute quantitatively to disease resistance in Arabidopsis, and their relative contributions are dependent on the R gene assayed. Plant Cell 14, 1005–1015. doi: 10.1105/tpc.001032
Tucker, S. L., Thornton, C. R. Tasker, K., Jacob, C., Giles, G., Egan, M., et al. (2004). A fungal metallothionein is required for pathogenicity of Magnaporthe grisea. Plant Cell 16, 1575–1588. doi: 10.1105/tpc.021279
Van Bockhaven, J., De Vleesschauwer, D., Hofte, M. (2013). Towards establishing broad-spectrum disease resistance in plants: Silicon leads the way. J. Exp. Bot. 64, 1281–1293. doi: 10.1093/jxb/ers329
Vesk, P., Reichman, S. (2009). Hyperaccumulators and herbivores: a Bayesian meta-analysis of feeding choice trials. J. Chem. Ecol. 35, 289–296. doi: 10.1007/s10886-009-9607-7
Vignesh, K. S., Figueroa, J. A. L., Porollo, A., Caruso, J. A., Deepe, G. S. (2013). Granulocyte macrophage-colony stimulating factor induced Zn sequestration enhances macrophage superoxide and limits intracellular pathogen survival. Immunity 39, 697–710. doi: 10.1016/j.immuni.2013.09.006
Walters, D. R., Bingham, I. J. (2007). Influence of nutrition on disease development caused by fungal pathogens: implications for plant disease control. Ann. App. Biol. 151, 307–324. doi: 10.1111/j.1744-7348.2007.00176.x
Wang, X., Wang, Y., Liu, P., Ding, Y., Mu, X., Liu, X., et al. (2017). TaRar1 is involved in wheat defense against stripe rust pathogen mediated by YrSu. Front. Plant Sci. 8, 156. doi: 10.3389/fpls.2017.00156
Wang, Y. Q., Feechan, A., Yun, B. W., Shafiei, R., Hofmann, A., Taylor, P., et al. (2009). S-nitrosylation of AtSABP3 antagonizes the expression of plant immunity. J. Biol. Chem. 284, 2131–2137. doi: 10.1074/jbc.M806782200
Wongpia, A., Lomthaisong, K. (2010). Changes in the 2DE protein profiles of chilli pepper (Capsicum annuum) leaves in response to Fusarium oxysporum infection. Scienceasia 36, 259–270. doi: 10.2306/scienceasia1513-1874.2010.36.259
Wu, G., Yi, Y. (2015). Effects of dietary heavy metals on the immune and antioxidant systems of Galleria mellonella larvae. Comp. Biochem. Physiol. C. Toxicol. Pharmacol. 167, 131–139 doi: 10.1016/j.cbpc.2014.10.004
Xie, Z., Zhang, P., Zhao, J., Wang, R., Gao, J., Xu, J. (2017). Identification of microRNAs from Zn-treated Solanum nigrum roots by small RNA sequencing. Acta Physiol. Plant. 39, 32. doi: 10.1007/s11738-016-2337-x
Xu, J., Yin, H., Li, Y., Liu, X. (2010). Nitric oxide is associated with long-term zinc tolerance in Solanum nigrum. Plant Physiol. 154, 1319–1334. doi: 10.1104/pp.110.162982
Yamasaki, S., Sakata-Sogawa, K., Hasegawa, A., Suzuki, T., Kabu, K., Sato, E., et al. (2007). Zinc is a novel intracellular second messenger. J. Cell Biol. 177, 637–645. doi: 10.1083/jcb.200702081
Zhao, H., Eide, D. J. (1997). Zap1p, a metalloregulatory protein involved in zinc-responsive transcriptional regulation in Saccharomyces cerevisiae. Mol. Cell Biol. 17, 5044–5052. doi: 10.1128/MCB.17.9.5044
Zacheo, G., Bleve-Zacheo, T. 1988. Involvement of superoxide dismutases and superoxide radicals in the susceptibility and resistance of tomato plants to Meloidogyne incognita attack. Physiol. Mol. Plant Pathol. 32, 313–322. doi: 10.1016/S0885-5765(88)80026-2
Keywords: zinc deprivation, zinc toxicity, zinc hyperaccumulation, Zn-triggered organic defenses, plant enemies
Citation: Cabot C, Martos S, Llugany M, Gallego B, Tolrà R and Poschenrieder C (2019) A Role for Zinc in Plant Defense Against Pathogens and Herbivores. Front. Plant Sci. 10:1171. doi: 10.3389/fpls.2019.01171
Received: 15 January 2019; Accepted: 27 August 2019;
Published: 04 October 2019.
Edited by:
Angélique Besson-Bard, Université de Bourgogne, FranceReviewed by:
Gustavo C. MacIntosh, Iowa State University, United StatesYonggen Lou, Zhejiang University, China
Copyright © 2019 Cabot, Martos, Llugany, Gallego, Tolrà and Poschenrieder. This is an open-access article distributed under the terms of the Creative Commons Attribution License (CC BY). The use, distribution or reproduction in other forums is permitted, provided the original author(s) and the copyright owner(s) are credited and that the original publication in this journal is cited, in accordance with accepted academic practice. No use, distribution or reproduction is permitted which does not comply with these terms.
*Correspondence: Catalina Cabot, Y2NhYm90QHVpYi5lcw==