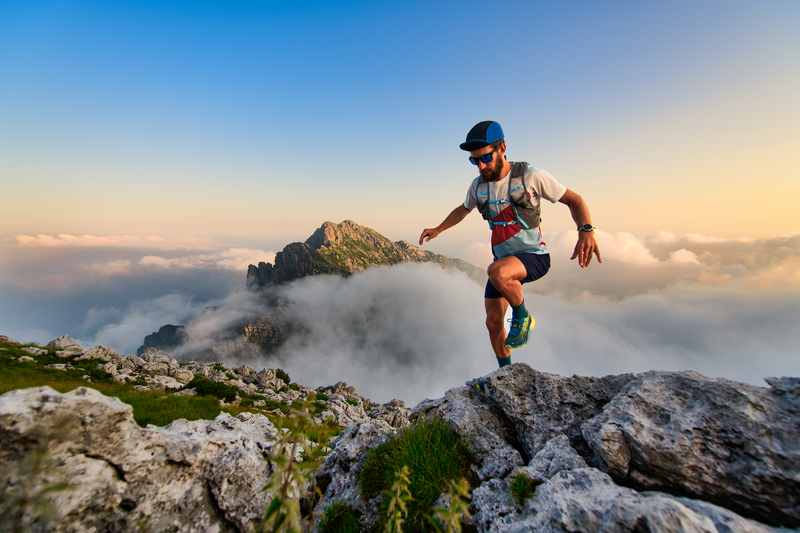
94% of researchers rate our articles as excellent or good
Learn more about the work of our research integrity team to safeguard the quality of each article we publish.
Find out more
ORIGINAL RESEARCH article
Front. Plant Sci. , 30 August 2019
Sec. Plant Breeding
Volume 10 - 2019 | https://doi.org/10.3389/fpls.2019.01043
This article is part of the Research Topic Legumes for Global Food Security View all 37 articles
In alkaline soils in arid and semi-arid areas toxic concentrations of the micronutrient boron (B) are problematic for many cereal and legume crops. Molecular markers have been developed for B toxicity in cereals and Medicago. There is a need for such tools in clovers—Trifolium. To this end, we undertook a genome-wide association study (GWAS) with a diversity panel of subterranean clover (Trifolium subterraneum L.), an established model pasture legume for genetic and genomic analyses for the genus. The panel comprised 124 T. subterraneum genotypes (97 core collection accessions and 27 Australian cultivars). Substantial and useful diversity in B toxicity tolerance was found in T. subterraneum. Such variation was continuously distributed and exhibited a high broad sense heritability H2 = 0.92. Among the subspecies of T. subterraneum, ssp. brachycalycinum was most susceptible to B toxicity (P < 0.05). From the GWAS, the most important discoveries were single-nucleotide polymorphisms (SNPs) located on Chr 1, 2, and 3, which mapped to haplotype blocks providing potential genes for a B toxicity tolerance assay and meriting further investigation. A SNP identified on Chr 1 aligned with Medicago truncatula respiratory burst oxidase-like protein (TSub_ g2235). This protein is known to respond to abiotic and biotic stimuli. The identification of these novel potential genes and their use to design markers for marker-assisted selection offer a pathway in pasture legumes to manage B toxicity by exploiting B tolerance.
Boron (B) is one of the essential micronutrients for healthy plant growth (Tomić et al., 2015) and is available to plants as boric acid (Reid, 2014). Due to its small molecular size and high membrane permeability in comparison to many other nutrients, the uptake and diffusion of B can be difficult for plants to control (Reid, 2014). Boron deficiency and toxicity are known to have adverse effects on agricultural production around the world (Oertli and Kohl, 1961; Nable et al., 1997; Hamilton et al., 2015). Although B deficiency is relatively easy to manage using B-rich fertilizers, B toxicity is more difficult to manage. Soil B concentration can be reduced by leaching, and B availability can be modified by pH adjustment, but this is impractical on a large scale (Yau and Ryan, 2008). Therefore, the use of genetic variation and plant breeding for tolerance is likely the best way to overcome toxicity (Yau and Ryan, 2008).
Boron toxicity mostly occurs in dry areas with an alkaline soil pH, particularly above pH 9, and in areas with low rainfall or in heavier clay soils, where B does not readily leach into deep soil layers below the root zone (Yau and Ryan, 2008). In 1983, a widespread B toxicity problem was reported in South Australia (Cartwright et al., 1984)—recently estimated to affect ∼4.9 million hectares (31%) of the agricultural zone in South Australia (Howie, 2012), and ∼15% in Western Australia (Lacey and Davies, 2009) have been identified as at risk of B toxicity. A concentration of B in the range of only 10 to 54 mg kg-1 in the soil inhibits plant growth (Javid et al., 2015), and soils in the southern Australian cropping region can reach 52 mg kg-1 B (Nuttall et al., 2003). Yield losses due to B toxicity have been reported for cereals (Cartwright et al., 1984; Paull et al., 1988), annual medics (Medicago spp.), field pea (Pisum sativum L.) (Paull et al., 1992), and lentil (Lens culinaris Medik.) (Yau and Erskine, 2000).
Strategies to cope with low and high soil B vary among plant species and genotypes (Reid, 2014). Plants cells are able to adjust the flow of most nutrients by selective membrane transport proteins, but in this regard, B is exceptional as it exists as uncharged boric acid at physiological pH and is therefore highly permeable through the lipid bilayers that form the basis of biological membranes (Reid, 2014). The three known pathways by which B enters and exits cells are: 1) passive, bidirectional diffusion through the lipid bilayer; 2) passive bidirectional diffusion through selective or non-selective channels; and 3) active efflux pumping (Reid, 2014). Hayes and Reid (2004) showed that a B-tolerant barley cultivar (Sahara) was able to maintain an internal B concentration lower than the external medium, presumably with an associated need for energy to preserve the gradient across the plasma membrane.
Boron toxicity symptoms vary with its mobility within the plant (de Abreu Neto et al., 2017). In common crop species, B is largely immobile once inside the cell wall, which leads to an accumulation at the leaf margins where the xylem vessels terminate (Reid, 2014), thereby causing chlorosis or necrosis of leaf tips and margins in older leaves (Brown and Shelp, 1997; Yau and Ryan, 2008). Lentil, barley (Hordeum vulgare L.), alfalfa (Medicago sativa L.), faba bean (Vicia faba L.), chickpea (Cicer arietinum L.), bread wheat (Triticum aestivum L.), durum wheat (T. durum Desf.), vetch (Vicia spp.), and field pea exhibit this type of B toxicity symptom (Yau and Ryan, 2008).
Reid et al. (2004) demonstrated that B inhibited growth by 40 to 60% in a monocot (barley), dicot (Arabidopsis—Arabidopsis thaliana L.) and an alga (Chara) when its soluble concentration reached 10 mM in the growth medium. Additionally, Kohl and Oertli (1961) reported the same concentration of B caused necrotic leaf margins in various plants. There is clearly variation in B tolerance among plant species and even among cultivars of the same species (Nable, 1988), indicating that natural variation in B toxicity tolerance exists within species, which could be used for selection and breeding of tolerant genotypes (Reid et al., 2004), leading to the development of several screening methodologies in crop species (Paull et al., 1992; Yau and Ryan, 2008; Schnurbusch et al., 2010; Javid et al., 2015; Bennett et al., 2017).
Arabidopsis has been used as a model of B tolerance to identify genes involved in B uptake and translocation—AtBOR1 and AtNIP5;1 (Takano et al., 2002; Takano et al., 2006), which conferred tolerance to plants under B deficient conditions. AtBOR4 and OxAtTIP5;1 are over-expression of an AtBOR1 paralog and AtTIP5;1, respectively, in transgenic Arabidopsis and encode transport molecules that prevent or regulate excess intercellular B (Miwa et al., 2007; Pang et al., 2010). Other studies have identified homologous genes related to B toxicity tolerance in other species: HvBot1 in barley (Sutton et al., 2007), MtNIP3 in the model legume Medicago truncatula (Bogacki et al., 2013), along with a single chromosomal region controlling tolerance to B in lentil (Kaur et al., 2014), two additive loci with incomplete dominance that admitted excess B tolerance in peas (Bagheri et al., 1996), and the Bo1 marker allele in durum and bread wheat (Schnurbusch et al., 2007; Schnurbusch et al., 2008). However, there are no reports of the genetic basis of B tolerance in Trifolium.
Subterranean clover (Trifolium subterraneum L.) is the most important sown annual pasture legume species in southern Australia and is grown over an estimated area of 29 million ha in the 250 to 1200 mm annual average rainfall band (Nichols et al., 2013). T. subterraneum is established as a model for Trifolium for genetic and genomic studies (Kaur et al., 2017a) on the basis of its diploidy (2n = 16), self-pollinating habit, and presence of major genomic resources. The species consists of three subspecies: 1) ssp. subterraneum, 2) ssp. yanninicum, and 3) ssp. brachycalycinum, of which subterraneum and yanninicum are adapted to acidic soils, and ssp. brachycalycinum is better adapted to neutral–alkaline soils where B is often problematic (Nichols et al., 2013). Although there is no information available on variation in B toxicity tolerance within the genus Trifolium, T. subterraneum has a wide natural distribution, which includes various soil types, presumably with variable B content, leading to the expectation that there may be a wide range of tolerance to B toxicity in this species.
The objectives of this study, using T. subterraneum as a Trifolium model, were to 1) develop a hydroponic screening system and identify a suitable concentration of B to differentiate B tolerance, 2) investigate variation for B toxicity tolerance in a wide range of germ plasm of T. subterraneum, and 3) investigate the genetic and molecular basis for B toxicity tolerance in T. subterraneum by using the candidate gene approach. We tested the specific hypotheses that: 1) there exists significant level of variation for boron toxicity tolerance within the existing T. subterraneum germ plasm diversity panel, 2) ssp. brachycalycinum is most likely to demonstrate tolerance to excess B among the three subspecies, and 3) a genome-wide association study (GWAS) will indicate potential genomic associations with B tolerance in T. subterraneum. The outcome of this study will help to enhance the efficiency of breeding for B toxicity tolerance in T. subterraneum and other Trifolium species. Increasing the tolerance of T. subterraneum to B toxicity may have a direct productivity benefit in soils with high B levels in the subsoil enabling plants to better access subsoil moisture reserves in dry seasons (Holloway and Alston, 1992).
A diverse panel of 124 T. subterraneum genotypes (Supplementary Table S1) was selected for the study, which included 97 core collection accessions (Nichols et al., 2013) and 27 diverse Australian cultivars (Kaur et al., 2017b). The core collection was developed by K. Ghamkhar, R. Appels and R. Snowball to represent the genetic diversity within the world collection of >10,000 phenotypes (Nichols et al., 2013; Ghamkhar et al., 2015). Selection of the core collection followed the methodology of Ghamkhar et al. (2008) to identify a subset of 760 lines, on the basis of 1) diversity for eco-geographical data from their sites of collection; and 2) agro-morphological data obtained by the Australian Trifolium Genetic Resource Centre (ATGRC) of the Department of Agriculture and Food Western Australia (DAFWA). DNA was then extracted from leaf material of each short-listed line, and 48 single-sequence repeat (SSR) primers, spread across each of the eight T. subterraneum chromosomes, were selected from the results of Ghamkhar et al. (2012) to identify the most diverse lines. Analysis using MSTRAT software (Gouesnard et al., 2001) to optimize maximum diversity within the minimum number of lines, resulted in an optimum core collection of 97 lines, covering 80.1% of the genetic diversity within the whole T. subterraneum collection. For these wild accessions with passport data, a total of 19 bioclimatic variables representing the climate of collection sites were derived from the WorldClim database (Hijmans et al., 2005).
All phenotyping experiments were carried out in the Plant Growth Facility at The University of Western Australia. A preliminary experiment was conducted to develop a hydroponic screening system for B toxicity tolerance in T. subterraneum and also to identify the level of B which showed the maximum discrimination among a selection of genotypes. Ten diverse genotypes of T. subterraneum (Supplementary Table S1) were subjected to four concentrations of B (0, 15, 30, 45 mg B L-1) in a hydroponic system under controlled temperature and photoperiod, an adaptation of the method reported in Bennett et al. (2017). The temperature was set at 24/20°C day/night and a 20-h photoperiod supplied by LED lights (4:3 ratio of model 108D18-V12 tubes from S-Tech Lighting, Australia and AP67L series tubes from Valoya, Helsinki, Finland). Forty seeds of each genotype were scarified to ensure uniform germination, and seeds were placed in plastic Petri dishes on moist filter paper to imbibe. Petri dishes were wrapped in Parafilm to avoid evaporation and aluminum foil to maintain seeds in darkness. The Petri dishes were stored at 15°C for 2 days. Then, for each treatment, 10 seeds of each genotype were sown into moist peat plugs within Styrofoam trays (Garden city plastic, PLT288S). One Styrofoam tray was allocated to each B treatment and placed in separate storage tubs (35L Icon Plastics) in the controlled environment room. The experimental design was based on a strip-plot with full replication among treatments. Genotypes were arranged in rows, and rows were randomized in each B treatment. Seedlings were watered immediately after sowing and again after 24 h with tap water. After a further 24 h (Day 5), 20 L of tap water was added to each storage tub to float the Styrofoam trays. The final number of individuals was greater than 5 for all genotype–treatment combinations. On day 8, the water in the tubs was replaced with a nutrient solution in DI water (adapted from Hoagland and Arnon, 1938) (Supplementary Table S2). The pH was maintained between 6 and 7 with bi-weekly adjustments using KOH to raise pH and H3PO4 to lower pH. On day 14, B (as H3BO3) was added into the hydroponic solution to achieve the desired concentration for treatments. On day 19, individual plants were scored for severity of B toxicity leaf symptoms using a 0.0- to 8.0-rating scale adapted for T. subterraneum from that used by Bagheri et al. (1992) (Supplementary Table S3). Leaf symptom scores were chosen as the B toxicity metric as they are routinely used in phenotyping similar legume species for B toxicity (Yau and Ryan, 2008) Leaf symptom scores for each B treatment were analyzed separately using “LSD.test” (agricolae package) in RStudio (Version 0.99.484, RStudio, Inc. R Core Team 2009-2015). The B treatment that provided the greatest level of discrimination (15 mg B L-1) was selected for further screening of the panel of 125 genotypes.
Screening the panel of 125 genotypes (Supplementary Table S1) was conducted in two sub-experiments, with genotypes allocated randomly to sub-experiments. Each sub-experiment contained four hydroponic culture tubs subjected to identical growth conditions, making a total of eight “blocks” for the 125 genotypes’ screening. To test and correct for block effects, each tub contained five “check” cultivars (Antas, Dalkeith, Gosse, Izmir, and Losa) arranged randomly as partial replicates and 15 “test” entries in rows of up to ten individual plants (Supplementary Figure S1). Test entries were randomly allocated to tubs. Seeds were germinated, planted, and grown for 14 days as previously described. Five days after the plants were transferred to Hoagland’s solution, we observed some mild leaf symptoms on some individuals that could potentially confound B toxicity symptom expression. Hence, all plants were scored for leaf damage prior to the addition of B, and these data were used as a covariate for correction in the final analysis as described below. On day 14, 15 mg B L-1 (as H3BO3) was added to the hydroponic solution. Boron exposure was increased from 5 to 7 days when screening the 125 genotypes to improve the phenotyping result in this wider selection of germ plasm. Plants were scored individually for B toxicity symptoms on day 21 (Supplementary Figures S2, S3A and Supplementary Table S3). The final number of individuals was greater than 3 for all genotype–treatment combinations. After correction for block effects and prior leaf damage, the corrected means of B toxicity score (Supplementary Figure S3B) were used for further analysis.
A covariate was applied to each tub to correct for block effects by averaging the B toxicity scores of all five check lines in each tub. This covariate and a covariate derived from prior leaf damage scores were used to correct the average B toxicity score of each of the 125 genotypes (on an individual plant basis) using “UNIANOVA” in SPSS (IBM Corp., 2013). This corrected mean B toxicity score (B score) was plotted and used in the GWAS, and in further analysis of correlations between B tolerance and data from passport information. The B score was also compared to the climate at the collection site of genotypes using Bioclimatic variables (Hijmans et al., 2005; Nichols et al., 2013) (Supplementary Table S1) as described below.
Differences in the B score among non-continuous variables in the passport data (Soil texture, country of origin, subspecies and category) were analyzed by one-way ANOVA in RStudio with B score as the dependent variable and results were tabulated and plotted (Box plot RStudio default function). Continuous variables (latitude, longitude, altitude, soil pH and 19 BioClim variables) were analyzed by ANOVA in RStudio to produce Rcorr correlation coefficients (Hmisc package) and their significance (R2 and P value).
Phenotypic B tolerance information obtained from the core collection of 97 accessions and the 27 elite Australian cultivars of T. subterraneum were associated with specific regions in the advanced assembly (Tsub_Refv2.0) (Kaur et al., 2017a) using GWAS analyses. Genomic DNA (gDNA) was extracted from a single plant of each of the 124 genotypes of T. subterraneum and sequenced. High-quality whole-genome resequencing (WGRS) data were generated for all 124 of these accessions and cultivars as described by Kaur et al. (2017b). SNP identification was conducted using samtools and bcftools (Li et al., 2009; Li, 2011), then SNPs with at least one heterozygous allele, those with an Minor Allele Frequency (MAF) ≤ 5%, and those that were not present in at least one individual were removed to keep only homozygous SNPs and remove errors of mis-mapping heterozygotes. This lead to removal of clustered SNPs, which is further confounded by the relatively low population. QQ plot was conducted to evaluate the effect of low population size (Supplementary Figure S4). Consecutive SNPs were merged using PLINK v1.9 (Purcell et al., 2007; Chang et al., 2015) into haplotype blocks if their r2 values were above 0.8. Linkage disequilibrium was visualized using Haploview v4.2 (Barrett et al., 2005).
The population structure for GWAS was of two sub-populations: the first sub-population comprised 27 cultivars released in Southern Australia for grazing; while the second sub-population of 97 accessions was a core germ plasm collection—a stratified sample of the world collection of T. subterraneum (Kaur et al., 2017b). Despite these two sub-populations, a principal component analysis reported in Kaur et al. (2017b) revealed the diversity between the sub-species: ssp. subterraneum, ssp. yanninicum and ssp. brachycalycinum. Four principal components were used to correct for population stratification. Individuals are split up in three subpopulations corresponding to the three sub-species (Supplementary Figure S5). GWAS was conducted via a logistic regression using the four principal components as covariates to correct for population stratification using PLINK v1.90b3.42 (Chang et al., 2015) (Supplementary Figure S5). BLASTP v2.2.30+ was used to link the identified genes with known genes within the annotations of M. truncatula Mt4.0v2 (Tang et al., 2014) and Arabidopsis thaliana (TAIR10) (Haas et al., 2005; Lamesch et al., 2012).
Each significant marker-trait association (MTA) resulting from the GWAS was checked for any overlaps with haplotype blocks with r2 values above 0.8. In which case, sequences 25-bp upstream and downstream from the SNP were extracted from the reference and were used to design PCR-ready markers for MAS for this B toxicity tolerance trait in primer3 v2.3.7 (Koressaar and Remm, 2007; Untergasser et al., 2012) (settings: primer product size, 250–500; primer optimum size, 300; primer minimum temperature, 55°C; optimal temperature, 57°C; maximum temperature, 60°C).
Putative candidate genes were proposed for each significant MTA by extracting the genes upstream, downstream, or overlapping with GWAS candidate SNPs.
To establish a suitable screening system and to determine the best concentration of B for phenotypic traits in a hydroponic system, ten genotypes of T. subterraneum were tested under four different concentrations of B (preliminary experiment). Tip chlorosis and necrosis were apparent in leaves after 5 days of B treatment, and plants were scored (Supplementary Figure S2). ANOVA indicated that both treatment and line had a significant effect (P < 0.05) on the score, with a significant interaction between these factors. Overall, the severity of symptoms increased with increasing B concentration (P < 2.2e-16). An LSD test in RStudio for leaf symptom score revealed that 15 mg B L-1 provided the greatest level of discrimination among genotypes, and so this concentration was selected to screen the panel of 125 genotypes (Table 1).
Table 1 Effect of four different concentrations of boron for 10 genotypes of T. subterraneum in preliminary experiment.
In the subsequent screening experiment, 125 genotypes of T. subterraneum were subject to 15 mg B L-1. Among the 125 accessions, there was a continuous distribution of tolerance to B toxicity (Figure 1). The genotype most tolerant to excess B concentration was L44 (ssp. subterraneum) with an average B score of 0.3 (se 0.27). The most tolerant among the cultivars of T. subterraneum tested was Dwalganup, which is also a subterraneum ssp., with a B score of 0.8 (se 0.20) (Figure 1 and Supplementary Table S1).
Figure 1 Boron toxicity symptom score for diverse germ plasm of T. subterraneum after correction for tub effects and the confounding prior leaf damage (on an individual basis). Cultivars are named on the x axis, and the remaining genotypes are referred to as a line name (L01 to L97) that can be cross referenced to the GRC identity using Supplementary Table S1.
In terms of susceptibility, L74 (ssp. subterraneum) showed the most severe B toxicity symptom with an average B score of 4.1 (se 0.27). This genotype was collected from an area with clayey soil texture and pH = 7.3 (Figure 1 and Supplementary Table S1). Among the cultivars, Nungarin (ssp. subterraneum) was the most susceptible with a B score of 3.9 (se 0.21) (Figure 1 and Supplementary Table S1).
Associations between continuous variables of origin (latitude, longitude, altitude, soil pH, and 19 BioClim variables) were tested by Pearson’s correlation (Supplementary Figure S6 and Supplementary Table S4). We anticipated a significant correlation between B score and soil pH. However, the strongest correlation with B score was longitude (P value <0.1, R2 = 0.029). The analysis of B scores compared among discontinuous variables (soil texture, country of origin, subspecies, and category) (Supplementary Figures S7A-D) indicated a significant difference (P value <0.05) existed between B toxicity symptoms of different subspecies (Supplementary Figure S7C). The post hoc LSD test showed subspecies brachycalycinum was significantly more susceptible than ssp. subterraneum or yanninicum (P-value <0.05) (Supplementary Table S5). Soil pH at collections sites ranged from 6 to 9 (mean value = 7.3) at ssp. brachycalycinum sites and from 5 to 9 (mean value = 6.3) in ssp. subterraneum sites (Supplementary Figure S8 and Supplementary Table S1). Soil pH data were not available for ssp. yanninicum accessions (Supplementary Figure S8). Comparing the 28 cultivars with the 97 wild accessions, the means for B toxicity tolerance of the groups were similar as were the ranges (Supplementary Figure S7B).
Potential genes were proposed for each significant MTA by extracting the genes upstream, downstream or overlapping with GWAS candidate SNPs. BLASTP was used to search for homologues of proteins encoded by the candidate genes within M. truncatula Mt4.0v2 and Arabidopsis thaliana (TAIR10) database.
The GWAS identified eight markers which reached suggestive P value below 1e-5 on chromosomes 1, 2, 3, 5, 6, and 7 associated with the B trait (Figure 2andTable 2). QQ plot suggested a relatively weak effect due to the low population size (Supplementary Figure S4). The SNPs located on Chr 1, 2, and 3 were mapped in haplotype blocks containing 21, 13, and 5 other SNPs with a total length of 366.62, 240.97, and 13.43 kbp, respectively (Figure 3 and Table 3). The significant SNP identified on chromosome 1 was located on the region of candidate gene TSub_ g2235 positioned between 32,860,391 and 32,866,822 with a total exon length of 2548 (Table 2) (Kaur et al., 2017a). Two significant SNPs were identified on Chr 2. For the first of these, an upstream endonuclease/exonuclease/phosphatase family protein (Tsub_g4776) and a downstream subtilisin-like serine protease (Tsub_g4777) were identified at a distance of 28878 and 20045 bp, respectively, from the suggestive SNP. For the second, an upstream calcium-binding EF-hand protein (Tsub_g7559) and a downstream pinoid-binding protein 1 (Tsub_g7560) were identified at a distance of 23628 and 5415 bp, respectively, from the suggestive SNP (Table 2).
Figure 2 Significant marker-trait associations (MTAs) for boron toxicity tolerance detected through genome-wide association analysis using the diverse germ plasm panel of Trifolium subterraneum. The x-axis indicates the SNP location along the eight chromosomes of T. subterraneum, y-axis in each graph represents −log 10P for the P value of the MTA. The gray line marks the threshold for genome-wide significance (P value = −log, 10P > 4.0) can be considered as significantly associated.
Table 2 Significant genomic associations identified using the phenotyping data for the boron toxicity tolerance in T. subterraneum.
Figure 3 Scheme of the genomic region with the haplotype block on Chr 1 in Trifolium subterraneum (advanced Tsub_Rv2.0) showing (A) the physical position of the boron toxicity tolerance candidate gene and (B) haplotype blocks based on LD.
Table 3 PCR ready markers designed using primer3 for the boron toxicity tolerance significant SNP identified in the haplotype blocks.
An upstream leucine-rich repeat receptor-like protein kinase (Tsub_g9589) and a downstream ribosomal protein L16p/L10e family (Tsub_g9590) was identified for the significant SNP on Chr 3 at a genetic distance of 2943/289 bp, respectively (Table 2). On Chr 5, an upstream transcription factor-like protein (Tsub_g16463) and a downstream cytochrome P450 family protein (Tsub_g16464) were detected at the distance of 1804 and 43166 bp, respectively, from the suggestive SNP. Two significant SNPs were found on Chr 6, both being in the region of potential gene (TSub_19611) which aligned with signal recognition particle 54-kDa protein in the M. truncatula database (Table 2). On Chr 7, we identified an upstream rare lipoprotein A-like double-psi beta-barrel protein (TSub_g22842), and no significant hits for a downstream (Tsub_g22843) at the distance of 3094 and 57 bp, respectively, from the suggestive SNP (Table 2).
The haplotype block containing the MTA SNPs on Chr 1, 2, and 3 with total length of 366,616, 109,074, and 32,973 bp, and 13,434 bp, respectively, was used to design PCR-ready markers for MAS for this B toxicity tolerance trait (Table 3).
The present study was designed to estimate variation in B stress tolerance and to identify potential B stress-responsive genes in Trifolium using T. subterraneum as a model. The study is the first report to demonstrate that substantial useful variation in B toxicity tolerance exists in T. subterraneum. Furthermore, the high broad-sense heritability H2 = 0.92 indicates that the trait is little influenced by environmental conditions. Variation in B toxicity tolerance has previously been reported for cereals—barley and wheat, and legumes—medics, peas and lentils (Nable, 1988; Paull et al., 1988; Paull et al., 1992; Bagheri et al., 1994; Yau and Erskine, 2000; Schnurbusch et al., 2008). Among T. subterraneum cultivars tested, Dwalganup and Nungarin were the most tolerant and susceptible to B toxicity, respectively. Clearly response to selection for B stress tolerance can be anticipated.
Significant MTAs for B toxicity tolerance were detected through GWAS analysis with the most significant discovery being the SNPs located on chromosome 1, 2, 3, which mapped into haplotype blocks. The potential gene on Chr 1 (TSub_g2235) aligned with M. truncatula respiratory burst oxidase-like protein and respiratory burst oxidase homolog (RBOH) protein B in A. thaliana. Respiratory burst NADPH oxidase is found in plant proteins, such as respiratory burst NADPH oxidase protein, which produces reactive oxygen species (ROS) as a defence mechanism (Suzuki et al., 2011). Respiratory burst oxidase homologues in plants are plasma membrane enzymes which produce ROS. They participate in a variety of mechanisms, such as cell elongation and abiotic stress signaling pathways, hormonal signaling, and pathogen response (Montiel et al., 2016; Arthikala et al., 2017). Recent studies have revealed that RBOHs participate in legume–rhizobia interaction (Montiel et al., 2016). Ozhuner et al. (2013) described B toxicity symptoms as cell wall biosynthesis degradation, inhibition of cell division, and elongation and metabolic decline by binding to the ATP, NADH, and NADPH component of the ribose. Our results suggest that RBOHs may also be involved in B toxicity tolerance in T. subterraneum.
BLAST search revealed that some of the other SNPs identified in MTAs have high-sequence similarities with potential genes known for plant stress responses (Table 2). Based on the results of the current study, these derived proteins may also be expressed in B toxicity conditions in T. subterraneum. Being in haplotype blocks, these genes identified on chr 1, 2, and 3 are the most stable and potential for designing molecular markers to track haplotype variation for this trait (Table 3). We plan to now functionally validate these genes found associated with B toxicity in subterranean clover using the CRISPR-Cas system in a follow-up study.
Although the corresponding proteins for the B transporter/channel genes AtBOR1 and AtNIP5;1 in Arabidopsis are responsible for B uptake in B deficient conditions (Takano et al., 2006), similar proteins in barley and M. truncatula have been found to be linked to B toxicity tolerance (Reid, 2007; Sutton et al., 2007; Bogacki et al., 2013). However, in the present study, no linkage was found between B toxicity tolerance and AtBOR1, AtNIP5;1 in T. subterraneum.
Molecular markers have been identified for selection of B toxicity tolerance in other plant species. Tolerance to excess of B is controlled by a single gene in the model legume M. truncatula (Bogacki et al., 2013), barley (Sutton et al., 2007), and lentil (Kaur et al., 2014). Based on our phenotypic and genotypic results, this trait could be controlled by more than one gene in T. subterraneum.
The hydroponic system developed herein provides an efficient, rapid (21 days) method to screen nutrient toxicity and deficiency in breeding studies. This is the first report of hydroponic screening for B toxicity tolerance in T. subterraneum. Hydroponics have previously been used as a rapid method for B toxicity tolerance screening in barley, Brassica rapa L., wheat, rice (Oryza sativa L.), and field pea (Jefferies et al., 1999; Kaur et al., 2006; Schnurbusch et al., 2007; Pallotta et al., 2014; de Abreu Neto et al., 2017; Bennett et al., 2017), B toxicity and salinity tolerance screening in field pea (Javid et al., 2015), and aluminum tolerance screening in barley and wheat (Baier et al., 1995; Ma et al., 1997). Screening for abiotic stress tolerance in the field is difficult due to environmental heterogeneity and variation of mineral content in soil (Stoddard et al., 2006). This research has provided a robust, high-throughput hydroponic protocol for screening B toxicity which could be readily applicable to screen other plant species and/or for other abiotic stresses.
In previous hydroponic B toxicity screening studies, the B concentrations used ranged from 162 mg L-1 in wheat (Schnurbusch et al., 2007) to 8 mg L-1 in rice (de Abreu Neto et al., 2017). In the latter study, 8 mg B L-1 induced severe toxicity in many varieties of rice (de Abreu Neto et al., 2017). Wheat appears to more tolerant, and rice more susceptible to B concentration compared with T. subterraneum.
Boron toxicity is problematic in soils with high pH (Yau and Ryan, 2008). Our expectation was that ssp. brachycalycinum, which is commonly found on alkaline soils, was more likely to demonstrate B tolerance than the other two subspecies of T. subterraneum. However, B toxicity tolerance was not significantly correlated with any passport data or BIOCLIM variables, including soil pH (Supplementary Table S4) and, among the three subspecies, brachycalycinum was the most susceptible species for B toxicity. Therefore, our expectation was not met. A possible explanation for these results is that the brachycalycinum genotypes tested here did not come from highly alkaline soils. B has relatively high availability in soils of pH 5 to 6.5, with availability then dropping as pH increases to 8.5. In soils above pH 8.5, B once again becomes highly available (Dwivedi et al., 1992). This reduced availability of B in neutral to moderately alkaline soils is particularly prevalent in soils with high calcium content as B has the tendency to bind with Ca in the soil (Dwivedi et al., 1992). Some studies have demonstrated that adding lime to acidic soil increased soil pH to a more moderate pH and, consequently, could result in lower concentrations of B in pea and barley plants tissue (Gupta and Macleod, 1981; Dwivedi et al., 1992). The brachycalycinum genotypes tested here were collected from soils with pH ranging from 6 to 9, with most (48%) collected from soil pH 6.5 to 7.5, where B would have poor availability. In contrast, the ssp. subterraneum genotypes tested in the current study were mostly (70%) collected in soils with pH less than 6.5. As previously highlighted, B is readily available in soil pH 5 to 6.5, consistent with our results indicating that the most tolerant genotypes were found in ssp. subterraneum.
In conclusion, this study demonstrated substantial variation in tolerance to B toxicity in T. subterraneum germ plasm, which was genetically dissected by GWAS. Potential genes were identified through GWAS associated with B toxicity tolerance that merit further investigation. The high throughput hydroponic system developed here could be applicable to other plants for screening for abiotic stress. Furthermore, tolerant cultivars, such as Dwalganup and Napier, would be priorities for use in soil types with potential for B toxicity. The results from this study provide valuable, new information for both plant breeding and gene validation studies using CRISPR technology in T. subterraneum.
HT performed the boron phenotyping research under the guidance of PK, WE, RB, PB, and MP-N and wrote the article with contributions from all. PK designed and performed the sequencing experiments and conducted the bioinformatics analysis with PB. All authors read the article and approved the content.
This study was conducted by the Centre for Plant Genetics and Breeding (PGB) at The University of Western Australia (UWA). Funding for this work was also provided by an Australian Research Council Linkage Grant (LP100200085), UWA RMF Grant, Meat and Livestock Australia (MLA grant B.PBE.037) and the Department of Agriculture and Food Western Australia (DAFWA).
The authors declare that the research was conducted in the absence of any commercial or financial relationships that could be construed as a potential conflict of interest.
The authors gratefully acknowledge the Department of Primary Industry and Regional Development (DPIRD) for the provision of seeds for this study. This work was also supported by resources provided by the Pawsey Supercomputing Centre with funding from the Australian Government and the Government of Western Australia. The support to HT from the Australian Wool Education Trust (AWET) and to PB from the Forrest Research Foundation is gratefully acknowledged.
The Supplementary Material for this article can be found online at: https://www.frontiersin.org/articles/10.3389/fpls.2019.01043/full#supplementary-material.
Arthikala, M.-K., Montiel, J., Sánchez-López, R., Nava, N., Cárdenas, L., Quinto, C. (2017). Respiratory burst oxidase homolog gene A is crucial for rhizobium infection and nodule maturation and function in common bean. Front. Plant Sci. 8 (2003), 1–5. doi: 10.3389/fpls.2017.02003
Bagheri, A., Paull, J. G., Rathjen, A. J. (1994). The response of Pisum sativum L. germplasm to high concentrations of soil boron. Euphytica 75 (1), 9–17. doi: 10.1007/BF00024526
Bagheri, A., Paull, J. G., Rathjen, A. J. (1996). Genetics of tolerance to high concentrations of soil boron in peas (Pisum sativum L.). Euphytica 87 (1), 69–75. doi: 10.1007/BF00022967
Bagheri, A., Paull, J. G., Rathjen, A. J., Ali, S. M., Moody, D. B. (1992). Genetic variation in the response of pea (Pisum sativum L.) to high soil concentrations of boron. Plant Soil 146 (1), 261–269. doi: 10.1007/BF00012020
Baier, A. C., Somers, D. J., Gusiafson, J. P. (1995). Aluminium tolerance in wheat: correlating hydroponic evaluations with field and soil performances. Plant Breed. 114 (4), 291–296. doi: 10.1111/j.1439-0523.1995.tb01236.x
Barrett, J. C., Fry, B., Maller, J., Daly, M. J. (2005). Haploview: analysis and visualization of LD and haplotype maps. Bioinformatics 21 (2), 263–265. doi: 10.1093/bioinformatics/bth457
Bennett, R. G., Ribalta, F. M., Pazos-Navarro, M., Leonforte, A., Croser, J. S. (2017). Discrimination of boron tolerance in Pisum sativum L. genotypes using a rapid, high-throughput hydroponic screen and precociously germinated seed grown under far-red enriched light. Plant Methods 13 (1), 70. doi: 10.1186/s13007-017-0221-3
Bogacki, P., Peck, D. M., Nair, R. M., Howie, J., Oldach, K. H. (2013). Genetic analysis of tolerance to Boron toxicity in the legume Medicago truncatula. BMC Plant Biol. 13 (1), 54. doi: 10.1186/1471-2229-13-54
Brown, P. H., Shelp, B. J. (1997). Boron mobility in plants. Plant Soil 193 (1), 85–101. doi: 10.1023/A:1004211925160
Cartwright, B., Zarcinas, B., Mayfield, A. (1984). Toxic concentrations of boron in a red-brown earth at Gladstone, South Australia. Soil Res. 22 (3), 261–272. doi: 10.1071/SR9840261
Chang, C. C., Chow, C. C., Tellier, L. C., Vattikuti, S., Purcell, S. M., Lee, J. J. (2015). Second-generation PLINK: rising to the challenge of larger and richer datasets. GigaScience 4 (1), 7. doi: 10.1186/s13742-015-0047-8
de Abreu Neto, J. B., Hurtado-Perez, M. C., Wimmer, M. A., Frei, M. (2017). Genetic factors underlying boron toxicity tolerance in rice: genome-wide association study and transcriptomic analysis. J. Exp. Bot. 68 (3), 687–700. doi: 10.1093/jxb/erw423
Dwivedi, B. S., Ram, M., Singh, B. P., Das, M., Prasad, R. N. (1992). Effect of liming on boron nutrition of pea (Pisum sativum L.) and corn (Zea mays L.) grown in sequence in an acid Alfisol. Fertilizer Res. 31 (3), 257–262. doi: 10.1007/BF01051276
Ghamkhar, K., Isobe, S., Nichols, P. G. H., Faithfull, T., Ryan, M. H., Snowball, R., et al. (2012). The first genetic maps for subterranean clover (Trifolium subterraneum L.) and comparative genomics with T. pratense L. and Medicago truncatula Gaertn. to identify new molecular markers for breeding. Mol. Breed. 30 (1), 213–226. doi: 10.1007/s11032-011-9612-8
Ghamkhar, K., Nichols, P. G. H., Erskine, W., Snowball, R., Murillo, M., Appels, R., et al. (2015). Hotspots and gaps in the world collection of subterranean clover (Trifolium subterraneum L.). J. Agric. Sci. 153 (6), 1069–1083. doi: 10.1017/S0021859614000793
Ghamkhar, K., Snowball, R., Wintle, B. J., Brown, A. H. D. (2008). Strategies for developing a core collection of bladder clover (Trifolium spumosum L.) using ecological and agro-morphological data. Aust. J. Agric. Res. 59 (12), 1103–1112. doi: 10.1071/AR08209
Gouesnard, B., Bataillon, T. M., Decoux, G., Rozale, C., Schoen, D. J., David, J. L. (2001). MSTRAT: an algorithm for building germ plasm core collections by maximizing allelic or phenotypic richness. J. Heredity 92 (1), 93–94. doi: 10.1093/jhered/92.1.93
Gupta, U. C., Macleod, J. A. (1981). Plant and soil boron as influenced by soil pH and calcium sources on pondzol soils. Soil Sci. 131 (1), 20. doi: 10.1097/00010694-198101000-00003
Haas, B. J., Wortman, J. R., Ronning, C. M., Hannick, L. I., Smith, R. K., Maiti, R., et al. (2005). Complete reannotation of the Arabidopsis genome: methods, tools, protocols and the final release. BMC Biol. 3 (1), 7. doi: 10.1186/1741-7007-3-7
Hamilton, L. J., Reed, K. F. M., Leach, E. M. A., Brockwell, J. (2015). Boron deficiency in pasture based on subterranean clover (Trifolium subterraneum L.) is linked to symbiotic malfunction. Crop. Pasture Sci. 66 (11), 1197–1212. doi: 10.1071/CP14300
Hayes, J. E., Reid, R. J. (2004). Boron tolerance in barley is mediated by efflux of boron from the roots. Plant Physiol. 136 (2), 3376–3382. doi: 10.1104/pp.103.037028
Hijmans, R. J., Cameron, S. E., Parra, J. L., Jones, P. G., Jarvis, A. (2005). Very high resolution interpolated climate surfaces for global land areas. Int. J. Climatol. 25 (15), 1965–1978. doi: 10.1002/joc.1276
Hoagland, D. R., Arnon, D. I. (1938). The water-culture method for growing plants without soil. Berkeley, Calif: University of California, College of Agriculture, Agricultural Experiment Station.
Holloway, R., Alston, A. (1992). The effects of salt and boron on growth of wheat. Aust. J. Agric. Res. 43 (5), 987–1001. doi: 10.1071/AR9920987
Howie, J. H. (2012). Boron tolerance in annual medics (Medicago spp.). Crop. Pasture Sci. 63 (8-9), 886–892. doi: 10.1071/CP12143
Javid, M., Rosewarne, G. M., Sudheesh, S., Kant, P., Leonforte, A., Lombardi, M., et al. (2015). Validation of molecular markers associated with boron tolerance, powdery mildew resistance and salinity tolerance in field peas. Front. Plant Sci. 6 (917), 1–9. doi: 10.3389/fpls.2015.00917
Jefferies, S. P., Barr, A. R., Karakousis, A., Kretschmer, J. M., Manning, S., Chalmers, K. J., et al. (1999). Mapping of chromosome regions conferring boron toxicity tolerance in barley (Hordeum vulgare L.). Theor. Appl. Genet. 98 (8), 1293–1303. doi: 10.1007/s001220051195
Kaur, P., Bayer, P. E., Milec, Z., Vrána, J., Yuan, Y., Appels, R., et al. (2017a). An advanced reference genome of Trifolium subterraneum L. reveals genes related to agronomic performance. Plant Biotech. J. 15 (8), 1034–1046. doi: 10.1111/pbi.12697
Kaur, P., Appels, R., Bayer, P. E., Keeble-Gagnere, G., Wang, J., Hirakawa, H., et al. (2017b). Climate clever clovers: new paradigm to reduce the environmental footprint of ruminants by breeding low methanogenic forages utilizing haplotype variation. Front. Plant Sci. 8 (1463), 1–10. doi: 10.3389/fpls.2017.01463
Kaur, S., Cogan, N. O. I., Stephens, A., Noy, D., Butsch, M., Forster, J. W., et al. (2014). EST-SNP discovery and dense genetic mapping in lentil (Lens culinaris Medik.) enable candidate gene selection for boron tolerance. Theor. Appl. Genet. 127 (3), 703–713. doi: 10.1007/s00122-013-2252-0
Kaur, S., Nicolas, M. E., Ford, R., Norton, R. M., Taylor, P. W. J. (2006). Selection of Brassica rapa genotypes for tolerance to boron toxicity. Plant Soil 285 (1), 115–123. doi: 10.1007/s11104-006-0063-7
Kohl, H. C., Oertli, J. J. (1961). Distribution of Boron in leaves. Plant Physiol. 36, 420–424. doi: 10.1104/pp.36.4.420
Koressaar, T., Remm, M. (2007). Enhancements and modifications of primer design program Primer3. Bioinformatics 23 (10), 1289–1291. doi: 10.1093/bioinformatics/btm091
Lacey, A., Davies, S. (2009). Boron toxicity in WA soils. WA Department of Agriculture and Food, Farmnote 388/2009. 092495-09/09-ID10350 www.agric.wa.gov.au.
Lamesch, P., Berardini, T. Z., Li, D., Swarbreck, D., Wilks, C., Sasidharan, R., et al. (2012). The Arabidopsis Information Resource (TAIR): improved gene annotation and new tools. Nucleic Acids Res. 40 (D1), D1202–D1210. doi: 10.1093/nar/gkr1090
Li, H. (2011). A statistical framework for SNP calling, mutation discovery, association mapping and population genetical parameter estimation from sequencing data. Bioinformatics 27 (21), 2987–2993. doi: 10.1093/bioinformatics/btr509
Li, H., Handsaker, B., Wysoker, A., Fennell, T., Ruan, J., Homer, N., et al. (2009). The sequence alignment/map format and SAMtools. Bioinformatics 25 (16), 2078–2079. doi: 10.1093/bioinformatics/btp352
Ma, J. F., Zheng, S. J., Li, X. F., Takeda, K., Matsumoto, H. (1997). A rapid hydroponic screening for aluminium tolerance in barley. Plant Soil 191 (1), 133–137. doi: 10.1023/A:1004257711952
Miwa, K., Takano, J., Omori, H., Seki, M., Shinozaki, K., Fujiwara, T. (2007). Plants tolerant of high boron levels. Science 318 (5855), 1417–1417. doi: 10.1126/science.1146634
Montiel, J., Arthikala, M.-K., Cárdenas, L., Quinto, C. (2016). Legume NADPH oxidases have crucial roles at different stages of nodulation. Int. J. Mol. Sci. 17 (5), 680. doi: 10.3390/ijms17050680
Nable, R. O. (1988). Resistance to boron toxicity amongst several barley and wheat cultivars: a preliminary examination of the resistance mechanism. Plant Soil 112 (1), 45–52. doi: 10.1007/BF02181751
Nable, R. O., Bañuelos, G. S., Paull, J. G. (1997). Boron toxicity. Plant Soil 193 (1), 181–198. doi: 10.1023/A:1004272227886
Nichols, P. G. H., Foster, K. J., Piano, E., Pecetti, L., Kaur, P., Ghamkhar, K., et al. (2013). Genetic improvement of subterranean clover (Trifolium subterraneum L.). 1. Germplasm, traits and future prospects. Crop. Pasture Sci. 64 (4), 312–346. doi: 10.1071/CP13118
Nuttall, J., Armstrong, R., Connor, D. (2003). Evaluating physicochemical constraints of Calcarosols on wheat yield in the Victorian southern Mallee. Crop. Pasture Sci. 54, 487–497. doi: 10.1071/AR02168
Oertli, J. J., Kohl, H. C. (1961). Some considerations about the tolerance of various plant species to excessive supplies of boron. Soil Sci. 92 (4), 43–247. doi: 10.1097/00010694-196110000-00004
Ozhuner, E., Eldem, V., Ipek, A., Okay, S., Sakcali, S., Zhang, B., et al. (2013). Boron stress responsive microRNAs and their targets in barley. PLos One 8 (3), e59543–e59543. doi: 10.1371/journal.pone.0059543
Pallotta, M., Schnurbusch, T., Hayes, J., Hay, A., Baumann, U., Paull, J., et al. (2014). Molecular basis of adaptation to high soil boron in wheat landraces and elite cultivars. Nature 514, 88–91. doi: 10.1038/nature13538
Pang, Y., Li, L., Ren, F., Lu, P., Wei, P., Cai, J., et al. (2010). Overexpression of the tonoplast aquaporin AtTIP5;1 conferred tolerance to boron toxicity in Arabidopsis. J. Genet. Genom. 37 (6), 389–397. doi: 10.1016/S1673-8527(09)60057-6
Paull, J. G., Cartwright, B., Rathjen, A. J. (1988). Responses of wheat and barley genotypes to toxic concentrations of soil boron. Euphytica 39 (2), 137–144. doi: 10.1007/BF00039866
Paull, J. G., Nable, R. O., Lake, A. W. H., Materne, M. A., Rathjen, A. J. (1992). Response of annual medics (Medicago spp.) and field peas (Pisum sativum) to high concentration of boron, genetic variation and the mechanism of tolerance. Aust. J. Agric. Res. 43, 203–213. doi: 10.1071/AR9920203
Purcell, S., Neale, B., Todd-Brown, K., Thomas, L., Ferreira, M. A. R., Bender, D., et al. (2007). PLINK: a tool set for whole-genome association and population-based linkage analyses. Am. J. Hum. Genet. 81 (3), 559–575. doi: 10.1086/519795
Reid, R. (2007). Identification of boron transporter genes likely to be responsible for tolerance to boron toxicity in wheat and barley. Plant Cell Physiol. 48 (12), 1673–1678. doi: 10.1093/pcp/pcm159
Reid, R. (2014). Understanding the boron transport network in plants. Plant Soil 385 (1), 1–13. doi: 10.1007/s11104-014-2149-y
Reid, R. J., Hayes, J. E., Post, A., Stangoulis, J. C. R., Graham, R. D. (2004). A critical analysis of the causes of boron toxicity in plants. Plant Cell Environ. 27 (11), 1405–1414. doi: 10.1111/j.1365-3040.2004.01243.x
Schnurbusch, T., Collins, N. C., Eastwood, R. F., Sutton, T., Jefferies, S. P., Langridge, P. (2007). Fine mapping and targeted SNP survey using rice-wheat gene colinearity in the region of the Bo1 boron toxicity tolerance locus of bread wheat. Theor. Appl. Genet. 115 (4), 451–461. doi: 10.1007/s00122-007-0579-0
Schnurbusch, T., Langridge, P., Sutton, T. (2008). The Bo1-specific PCR marker AWW5L7 is predictive of boron tolerance status in a range of exotic durum and bread wheats. Genome 51 (12), 963–971. doi: 10.1139/G08-084
Schnurbusch, T., Hayes, J., Sutton, T. (2010). Boron toxicity tolerance in wheat and barley: Australian perspectives. Breed Sci. 60 (4), 297–304. doi: 10.1270/jsbbs.60.297
Stoddard, F. L., Balko, C., Erskine, W., Khan, H. R., Link, W., Sarker, A. (2006). Screening techniques and sources of resistance to abiotic stresses in cool-season food legumes. Euphytica 147 (1), 167–186. doi: 10.1007/s10681-006-4723-8
Sutton, T., Baumann, U., Hayes, J., Collins, N. C., Shi, B.-J., Schnurbusch, T., et al. (2007). Boron-toxicity tolerance in barley arising from efflux transporter amplification. Science 318 (5855), 1446–1449. doi: 10.1126/science.1146853
Suzuki, N., Miller, G., Morales, J., Shulaev, V., Torres, M. A., Mittler, R. (2011). Respiratory burst oxidases: the engines of ROS signaling. Curr. Opin. Plant Biol. 14 (6), 691–699. doi: 10.1016/j.pbi.2011.07.014
Takano, J., Noguchi, K., Yasumori, M., Kobayashi, M., Gajdos, Z., Miwa, K., et al. (2002). Arabidopsis boron transporter for xylem loading. Nature 420, 337–340. doi: 10.1038/nature01139
Takano, J., Wada, M., Ludewig, U., Schaaf, G., von Wirén, N., Fujiwara, T. (2006). The Arabidopsis major intrinsic protein NIP5;1 is essential for efficient boron uptake and plant development under boron limitation. Plant Cell 18, 1498–1509. doi: 10.1105/tpc.106.041640
Tang, H., Krishnakumar, V., Bidwell, S., Rosen, B., Chan, A., Zhou, S., et al. (2014). An improved genome release (version Mt4.0) for the model legume Medicago truncatula. BMC genomics 15, 312–312. doi: 10.1186/1471-2164-15-312
Tomić, D., Stevović, V., Ðurović, D., Madić, M., Bokan, N., Stanisavljević, R. (2015). Boron application in red clover (Trifolium pratense L.) seed production. Irish J. Agric. Food Res. 54, 59–63. doi: 10.1515/ijafr-2015-0006
Untergasser, A., Cutcutache, I., Koressaar, T., Ye, J., Faircloth, B. C., Remm, M., et al. (2012). Primer3—new capabilities and interfaces. Nucleic Acids Res. 40 (15), e115–e115. doi: 10.1093/nar/gks596
Yau, S.-K., Erskine, W. (2000). Diversity of boron-toxicity tolerance in lentil growth and yield. Genet. Resour. Crop Evol. 47 (1), 55–62. doi: 10.1023/A:1008733106108
Keywords: abiotic stress, boron toxicity, genome-wide association study, haplotype analyses, hydroponic, forage legumes, subterranean clover
Citation: Tahghighi H, Erskine W, Bennett RG, Bayer PE, Pazos-Navarro M and Kaur P (2019) Genetic Diversity Linked to Haplotype Variation in the World Core Collection of Trifolium subterraneum for Boron Toxicity Tolerance Provides Valuable Markers for Pasture Breeding. Front. Plant Sci. 10:1043. doi: 10.3389/fpls.2019.01043
Received: 11 February 2019; Accepted: 26 July 2019;
Published: 30 August 2019.
Edited by:
Jose C. Jimenez-Lopez, Consejo Superior de Investigaciones Científicas (CSIC) Granada, SpainReviewed by:
Hamid Khazaei, University of Saskatchewan, CanadaCopyright © 2019 Tahghighi, Erskine, Bennett, Bayer, Pazos-Navarro and Kaur. This is an open-access article distributed under the terms of the Creative Commons Attribution License (CC BY). The use, distribution or reproduction in other forums is permitted, provided the original author(s) and the copyright owner(s) are credited and that the original publication in this journal is cited, in accordance with accepted academic practice. No use, distribution or reproduction is permitted which does not comply with these terms.
*Correspondence: Parwinder Kaur, cGFyd2luZGVyLmthdXJAdXdhLmVkdS5hdQ==
†ORCID: Parwinder Kaur, orcid.org/0000-0003-0201-0766
Disclaimer: All claims expressed in this article are solely those of the authors and do not necessarily represent those of their affiliated organizations, or those of the publisher, the editors and the reviewers. Any product that may be evaluated in this article or claim that may be made by its manufacturer is not guaranteed or endorsed by the publisher.
Research integrity at Frontiers
Learn more about the work of our research integrity team to safeguard the quality of each article we publish.