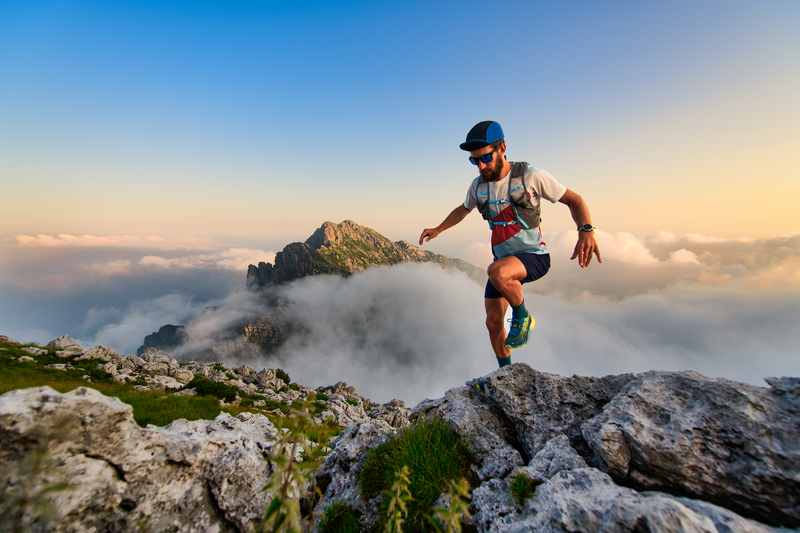
95% of researchers rate our articles as excellent or good
Learn more about the work of our research integrity team to safeguard the quality of each article we publish.
Find out more
ORIGINAL RESEARCH article
Front. Plant Sci. , 25 September 2019
Sec. Plant Metabolism and Chemodiversity
Volume 10 - 2019 | https://doi.org/10.3389/fpls.2019.01033
This article is part of the Research Topic Polyamines in Plant Biotechnology, Food Nutrition and Human Health View all 25 articles
Polyamines (PAs) constituting putrescine (Put), spermidine (Spd), and spermine (Spm) are ubiquitous in all organisms and play essential roles in the growth and developmental processes in living organisms, including plants. Evidences obtained through genetic, biochemical, and transgenic approaches suggest a tight homeostasis for cellular PA levels. Altered cellular PA homeostasis is associated with abnormal phenotypes. However, the mechanisms involved for these abnormalities are not yet fully understood, nor is it known whether cellular ratios of different polyamines play any role(s) in specific plant processes. We expressed a yeast spermidine synthase gene (ySpdSyn) under a constitutive promoter CaMV35S in tomato and studied the different phenotypes that developed. The constitutive expression of ySpdSyn resulted in variable flower phenotypes in independent transgenic lines, some of which lacked fruit and seed set. Quantification of PA levels in the developing flowers showed that the transgenic plants without fruit and seed set had significantly reduced Spd levels as well as low Spd/Put ratio compared to the transgenic lines with normal fruit and seed set. Transcript levels of SlDELLA, GA-20oxidase-1, and GA-3oxidase-2, which impact gibberellin (GA) metabolism and signaling, were significantly reduced in bud tissue of transgenic lines that lacked fruit and seed set. These findings indicate that PAs, particularly Spd, impact floral organ identity and fruit set in tomato involving GA metabolism and signaling. Furthermore, we suggest that a nexus exists between PA ratios and developmental programs in plants.
Polyamines (PAs), putrescine (Put), spermidine (Spd), spermine (Spm), and thermospermine (Tspm) are hormone-like biogenic amines ubiquitously present in all living organisms including plants. They have been implicated in regulating growth and developmental processes in plants (Kaur-Sawhney et al., 1988; Martin-Tanguy, 2001; Alcazar et al., 2005; Mattoo and Handa, 2008; Nambeesan et al., 2008; Igarashi and Kashiwagi, 2010; Nambeesan et al., 2010; Pegg, 2016; Handa et al., 2018; Tiburcio and Alcázar, 2018). PAs impact primary, lateral and adventitious root development (Flores and Galston, 1982), plant architecture (Cui et al., 2010), in vitro plant regeneration by somatic embryogenesis (Bastola and Minocha, 1995) and organogenesis (Ikeuchi et al., 2016), flowering (Malmberg and McIndoo, 1983; Tiburcio et al., 1988), fruit ripening (Mehta et al., 2002), and leaf and flower senescence (Kaur-Sawhney et al., 1980; Nambeesan et al., 2010; Mattoo and Sobieszczuk-Nowicka, 2019). Genetic, biochemical, and transgenic approaches have demonstrated that the metabolism of various PAs is tightly regulated, and imbalance in their homeostasis results in abnormal phenotypes (Kaur-Sawhney et al., 1980; Flores and Galston, 1982; Malmberg and McIndoo, 1983; Kaur-Sawhney et al., 1988; Tiburcio et al., 1989; Bastola and Minocha, 1995; Mehta et al., 2002; Ge et al., 2006; Cui et al., 2010; Nambeesan et al., 2010; Ikeuchi et al., 2016; Murray-Stewart et al., 2018). Furthermore, each PA may have a different effect on metabolism, growth, and development in plants (Handa and Mattoo, 2010; Mattoo et al., 2010; Tiburcio and Alcázar, 2018). Mutations in PA biosynthetic pathway or inhibitors that reduce Put and Spd levels cause plant lethality (Kusano et al., 2008). In addition, it is now apparent that Spm and Tspm play significant role(s) in abiotic responses and xylem differentiation, respectively (Takahashi and Kakehi, 2009; Yoshimoto et al., 2016; Seiff and Shelp, 2019). However, mechanisms that impart PA-associated phenotypes are not yet fully understood.
A close association of PAs with floral development in plants is known (Galston and Sawhney, 1990; Walden et al., 1997). Specifically, PA levels have been correlated with flower development and fruit bearing in olive (Pritsa and Voyiatzis, 2004; Pritsa and Voyiatzis, 2005), apricot (Alburquerque et al., 2006), and plum (DeDios et al., 2006). Early studies also reported higher Put, Spd, and Spm in floral organs of a male-sterile stamenless-2 (sl-2/sl-2) tomato mutant (Rastogi and Sawhney, 1990a; Rastogi and Sawhney, 1990b). In addition, regeneration of tobacco plant from a cell line impaired in PA metabolism produced flowers with a second row of petals in place of anthers (Malmberg, 1980), while a MGBG-[methylglyoxal-bis (guanylhydrazone)]-resistant tobacco cell line with elevated PA levels also exhibited abnormal floral phenotype producing flowers with anthers in place of ovules (Malmberg and McIndoo, 1983). MGBG is a known inhibitor of S-adenosyl methionine decarboxylase (SAMdc), a critical enzyme in PA biosynthesis pathway. Inhibition of SAMdc by MGBG led to impaired flowering, which was reversed by Spd in Spirodela punctata (De Cantu and Kanderler, 1989). Similarly, inhibition of other PA-biosynthesis enzymes using alpha-difluoromethylarginine (DFMA), an arginine decarboxylase inhibitor, and alpha-difluoromethylornithine (DMFO), an ornithine decarboxylase inhibitor, prevented floral bud initiation and subsequent development of floral bud in tobacco cell culture explants (Tiburcio et al., 1988). Tobacco and petunia mutants impaired in PA production also exhibited abnormal flower phenotype (Malmberg and McIndoo, 1983, Gerats et al., 1988; Malmberg and McIndoo, 1988), whereas high levels of PA in a tomato mutant resulted in abnormal stamen development (Rastogi and Sawhney, 1990a). Although these studies provided indirect evidence in favor of a role of PAs in flower development, the differential role(s) of specific PAs in these processes has remained to be discerned.
To determine the role of Spd during plant development including flower initiation, maturation, fruit set, and seed set, we introduced a yeast spermidine synthase gene (ySpdSyn) under the control of either a fruit-ripening-specific promoter (SlE8) or a constitutive CaMV35S promoter in tomato (Nambeesan et al., 2010). The ySpdSyn transgenic line with fruit-specific promoter SlE8 developed normal fruit and seed set, and the fruits had longer shelf life (Nambeesan et al., 2010). However, the expression of ySpdSyn under CaMV35S promoter resulted in two types of transgenic plant populations: one that normally set fruit and seed and the other that did not set fruit/seed and exhibited a range of flower and fruit phenotypes. Thus, these lines with constitutive expression of ySpdSyn were employed in our studies presented here to address the question of PA role in floral organ identity and fruit/seed set in tomato.
Our results demonstrate that flowers of the transgenic plants that set fruit and seeds exhibit significantly higher Spd/Put and Spd/Spm ratios compared to flowers from the transgenic plants that failed to set fruit and seeds. The lack of fruit and seed set was found associated with a reduction in the transcript levels of GA biosynthesis genes and DELLA, a negative regulator of GA signaling. We interpret these results to indicate that PAs influence floral organ identity and fruit set in tomato by modifying the genetic program that controls expression of GA biosynthesis and signaling genes.
Transgenic plants overexpressing yeast spermidine synthase (ySpdSyn) were generated as described previously (Nambeesan et al., 2010). The ySpdSyn gene (ScSpe3, systematic name: YPR069C) was amplified from a yeast genomic library and cloned in the sense orientation between a CaMV35S promoter and the 3’ end of a pea rbcS-E9 gene in pKYLX71 (Nambeesan et al., 2010). This construct was introduced into disarmed Agrobacterium tumefaciens LBA4404, and Agrobacterium strains harboring the chimeric constructs were used for transformation of tomato cv. Ohio 8245 cotyledons (Tieman et al., 1992). Successful plantlets obtained were transplanted into potted soil and grown in the greenhouse.
Fifteen independent transgenic plants expressing CaMV35S-ySpdSyn were generated. Two independent transgenic lines C13, C14 that exhibited floral morphological defects, impaired fruit and seed set, and sterility were propagated as vegetative cuttings and characterized alongside the isogenic wild-type (WT) Ohio 8245 control.
For phenotypic measurements, 27–36 flowers were harvested from WT and transgenic plants, and the number of sepals, petals, and stamens were counted. Any abnormality in the stamen morphology was noted. The flower size was measured diagonally across the flower. The flowers were dissected to observe the morphology of the gynoecium. Pollen morphology from dissected stamens was observed under a light microscope (Olympus, Center Valley, PA).
For pollen germination assay, open flowers were hydrated for 1 h in a Petri dish with wet Kim wipes to maintain a relative humidity close to 100%. The hydrated pollen from the flowers was spread on a glass slide with 30 µl germination medium [20 mM MES, 2% sucrose, 15% PEG 4000, 1 mM KNO3, 3 mM Ca(NO3)2, 0.8 mM MgSO4, and 1.6 mM H3BO3, pH 6.0]. The pollen grains were incubated for 3 h in the dark at 25°C under high relative humidity by placing the glass slide on a moist filter paper in a Petri dish. Germination was terminated by the addition of 5 µl of phenol solution. Pollen germination was scored using a light microscope (Olympus, Center Valley, PA). Pollen tubes that were twice the diameter of the pollen grain were scored as positive for successful pollination. Pollen grains were examined from at least five flowers with 100–200 pollen counted per flower. Digital images were captured using the SPOT software (Draper, Utah).
Mature leaf and tissue from various stages of flower development, including early buds (0.1–0.7 mm in length), late buds (0.8–1.5 cm in length), and fully open flowers were harvested. Three independent biological replicates at each developmental stage were collected and were frozen immediately in liquid nitrogen and stored at −80°C until analyzed. All samples were processed and quantified in triplicates for polyamine measurement as described previously (Nambeesan et al., 2010).
Mature leaf and flowers were collected at two stages of development, ∼135 early buds (0.1–0.7 mm in length) and 75 late buds (0.8–1.5 cm in length) from WT, C13, and C14 lines. Total RNA was extracted, and cDNA synthesis was performed following the manufacture’s recommendation (Promega, Madison, WI). Briefly, total RNA (1 μg) was treated with DNase and ImPromII reverse transcriptase to perform reverse transcription. The cDNA was diluted five times, and 1 μl of diluted cDNA was used for semiquantitative reverse transcription PCR (RT-PCR) in a 10-μl total reaction volume. For each primer pair, cycle number was determined for exponential amplification, and all subsequent reactions were performed for the predetermined number of cycles. Each PCR reaction typically included the following cycles: 95°C (3 min); [95°C (30 s); 55/56°C (30 s); 70°C (1 min)] 25 cycles; 72°C, 10 min. Tomato ACTIN gene was used for normalization. After PCR amplification, the products were separated on a 1.2% agarose gel, stained with 0.5 μg/ml ethidium bromide, and images were captured and processed using Image J software (National Institutes of Health, Bethesda, Maryland). Primers used for PCR amplification are listed in Supplemental Table 1.
Quantitative RT-PCR analysis was performed using real-time PCR (Stratagene Mx3005P, San Diego, CA). All primers (Supplemental Table 1) along with control ACTIN (SlACTIN) were validated for relative gene expression analysis. One microliter of the cDNA was utilized in a 15-μl reaction using the SYBR green-PCR master mix (Applied Biosystems, Foster City, CA). Melting curve analysis was performed after the PCR reaction to determine specificity of the PCR products. At least three biological replicates were analyzed for gene expression analyses. Statistical analyses were performed using one-way analysis of variance to compare across transgenic C13 and C14 lines and WT separately for a given developmental timepoint or treatment, using JMP Pro 12 (SAS Institute, Cary, NC, USA). Means were separated using Tukey’s honestly significant difference (HSD) test (α = 0.05).
Eleven independent events in transgenic tomato lines positive for the expression of CaMV35S‐ySpdSyn were evaluated in greenhouse. Five of them—C5, C11, C12, C13, and C14—were found severely impaired in fruit set. They varied from having no seeds to very few seeds and exhibited partial parthenocarpy. Other four independent transgenic lines—C1, C3, C4, and C15—exhibited normal fruit set, but their seed number/fruit varied being 18, 15, and 30% lower in C1, C3, and C4, respectively, except for C15 in which seed numbers were similar to the WT control (Table 1). Variable phenotypes, including impaired fruit and seed set and seed germination among the independent transgenic plants, may have resulted from the influence of transgene integration site(s) (De Buck et al., 2013).
Expression of ySpdSyn and endogenous SlSpdSyn in tomato leaves of C13 and C14 T0 lines is shown in Figure 1A. Both transgenic lines expressed ySpdSyn and SlSpdSyn, while, as expected, WT leaves expressed only the endogenous SlSpdSyn gene. The SlSpdSyn transcript levels remained unaltered in C13 and C14 lines compared to WT leaves, which indicated lack of endogenous gene silencing (Figure 1A). Quantification of different polyamines in these leaves indicated that Spd levels increased by 1.3- and 1.7-fold in C13 and C14 lines, respectively, as compared to the WT (Figure 1B). However, the transgenic event caused a 2.1- and 1.5-fold decrease in Spm in C13 and C14, respectively, compared to WT plants. Leaves from C14 lines exhibited significant 1.4-fold increase in Put compared to WT leaves, whereas C13 leaves did not show any change in Put levels compared to WT leaves (Figure 1B).
Figure 1 Generation and characterization of transgenic plants overexpressing yeast spermidine synthase (ySpdSyn). (A) The ySpdSyn construct was cloned under the CaMV35S promoter in pKYLX71 vector. Shown are levels of endogenous SlSpdSyn and the control SlACT using semiquantitative RT-PCR analysis indicating the expression of ySpdSyn and endogenous tomato SlSpdSyn in WT, C13, and C14 leaves. The tomato Actin gene was used as an internal control (SlACT). (B) Polyamine levels in WT, C13, and C14 leaves measured using HPLC. Shown are mean values and standard from three independent biological leaf replicates. Means followed by the same letter are not significantly different from each other based on one-way analysis of variance (α = 0.05) for a given polyamine (PA). Put, putrescine, Spd, spermidine; and Spm, spermine.
The floral and fruit morphology in transgenic and WT plants was evaluated to determine the basis of impaired fruit and seed set in C13 and C14 lines. Flower size or floral organ number did not differ significantly among these lines compared to WT plants. All genotypes had five sepals per flower. However, the average petal number was significantly different in line C13 (6.6 petal/flower) as compared to WT and C14 which had 5.8 and 5.3 petals/flower, respectively (Figure 2D). Similarly, the average number of stamens in C13 (7 per flower) was significantly higher than the WT and C14 lines that had 5.8 and 5.4 stamens per flower, respectively (Figure 2D). However, C13 line had severe morphological defects, with ∼17% flowers having 8–10 stamens in comparison to 5.4 in the WT. Most flowers from C14 and C13, however, did not form a normal staminal cone (Figure 2A–C). Dissection of flowers from transgenic lines revealed that carpels from C13 (Figure 2F) and C14 flowers (Figure 2G) were significantly enlarged as compared to the WT flowers (Figure 2E). Occasionally, fusion of multiple gynoecia at the base was observed in C13 flowers. The length of the style was reduced in C13 and C14 flowers (Figures 2E–G) with the tip of the style close to the stigmatic surface curved in transgenic flowers (Figures 2E–G). Other phenotypes in transgenic flowers included delayed abscission of petals during fruit growth (Figure 2K), multiple fruit set in the same flower by fusion of multiple gynoecia at the base (Figures 2L, M), and altered fruit shape with multiple locules (Figure 2N).
Figure 2 Altered floral and fruit characteristics in lines overexpressing ySpdSyn. (A) Wild-type flower. (B) Impaired staminal cone formation in C14. (C) Higher stamen number with impaired staminal cone in C13. (D) Higher stamen number and petal number in C13. (E–G) Alterations in carpel development in C13 (F) and C14 (G) in comparison to the wild type (E). (H–J) Changes in pollen morphology of C13 (I), C14 (J) in comparison to wild type (H). (K) Delayed petal abscission in C13. (L) Multiple fruit formation from a single flower in C13. (M, N). Altered fruit shape and multilocular fruits in C13 in comparison to wild-type fruit.
We next investigated pollen morphology and germination to determine the basis of differences in fruit set in transgenic lines. WT pollen appeared spherical and intact, and 96% of the pollen displayed a normal morphology (Figure 2H). However, ∼68% of pollen from C13 and 37% from C14 flowers exhibited a collapsed morphology (Figures 2I, J). In vitro pollen germination assays indicated reduced pollen germination in C13 and C14 lines compared with WT. Among the intact-appearing pollen, 79% of WT and only 17% of C13 and 40% of C14 pollen grains germinated (data not shown). None of the abnormal looking pollen grains germinated (data not shown). The reciprocal crosses using C13 and C14 pollen on WT stigma did not result in fruit set, but pollination of C13 and C14 using WT pollen resulted in 100 and 80% parthenocarpic fruit set, respectively (data not shown), Among the fruit that set seed in C14, only one seed was present. These results indicated that fruit development occurred without fertilization. Reduced seed set in C14 indicated partial female sterility.
We determined the levels of free polyamines (Put, Spd, and Spm) in fully opened flowers from WT and four CaMV-ySpdSyn lines to evaluate if altered PAs homeostasis was associated with the observed phenotypes (Figure 3). The steady-state Spd level in the flowers of other transgenic lines, viz., C4, C15, and WT plants was similar; these genotypes had normal fruit and seed set (Figure 3). The flowers of C13 and C14 lines exhibited parthenocarpic phenotype and had significantly lower Spd levels than the WT flowers (Figures 2 and 3). Notably, the Spd/Spm ratio in the flowers of C4 and C15 plants was 8.8 and 7.0, respectively, and only 2.9 and 3.1 in C13 and C14 flowers, respectively (Figure 3). Similarly, the Spd/Put ratio was significantly higher in C4 and C15 flowers compared to the WT flowers but significantly lower in C13 to C14 flowers. This pattern was also reflected in the Spd/TPA (Figure 3). Notably, the ratio of Put/TPA was significantly lower in C4 and C15 flowers and significantly higher in C13 and C14 flowers compared to WT flowers, respectively. Consistent patterns for Spm/TPA ratio were not observed for transgenic flowers from the four independent lines examined (Figure 3). Taken together, these results suggest that reduced Spd and Spd/Put ratios are associated with the flower phenotype and may therefore affect fruit and seed set in tomato.
Figure 3 Changes in the free total PAs (TPA), Put, Spd, and Spm (A), ratios of Spd/Put, Spd/Spm, and Spm/Put (B), and ratios of Put, Spd, and Spm to TPA (C) in fully open flowers of WT, C4, C15, C13, and C14 plants. WT, Wild-type Ohio 8245; C4, C15, C13, and C14 transgenic plants harboring CaMV-SpdSyn transgene. C4 and C15 plants displayed fruit and seed set, while the C13 and C14 plants exhibited abnormal flower phenotype and impaired seed set. Shown are the mean values and standard errors from the three independent biological replicates. Means followed by the different letters are significantly different from each other based on one-way analysis of variance (α = 0.05) for a given polyamine (PA).
Expression patterns of ySpdSyn and endogenous SlSpdSyn were compared at various stages of flower development including early and late bud stages and fully open flowers. The ySpdSyn transcripts were present at all the stages of flower development in the C13 and C14 lines and, as expected, not in the WT (Figure 4A). As anticipated, SlSpdSyn transcript was present at all the stages of flower development, indicating the lack of silencing of the endogenous or ySpdSyn transgene in the transgenic lines (Figure 4A).
Figure 4 Expression of ySpdSyn and SlSpdSyn genes (A), changes in the levels of free and total PAs (B), and changes in their ratios (C) during early and late stages of flower bud development in WT, C13, and C14 lines. Tomato ACTIN was used as a reference (SlACTIN). Flower development stages include early buds (eb; 0.1–0.7 cm) and late buds (lb; 0.8–1.5 cm). PA levels were measured in three independent biological replicates. Other details are the same as in Figure 3. Means followed by the different letters are significantly different from each other based on one-way analysis of variance (α = 0.05) for a given polyamine (PA).
We quantified the levels of Put, Spd, and Spm in early and late flower buds to determine if the observed changes in various PA levels described in fully opened WT flower were also present during the flower development in C13 and C14 genotypes that had abnormal fruit and seed set (Figure 4B). The Spd level was not significantly different at early and late bud stages in the C13 line but was significantly lower in the C14 line at both stages of bud development. Furthermore, Spm levels in the C13 and C14 genotypes were significantly lower than the WT at early and late bud stages of flower development, whereas TPA and Put levels were lower at the latter stage.
The Spd/Put ratio was significantly higher at early bud stage in the C13 and C14 lines but not at late bud stage as compared with the WT. Spd/Spm ratio was significantly higher in the early and late bud stage in C13, whereas it decreased at the late bud stage in C14 line (Figure 4C). A significant decrease in Spm/Put ratio in both the C13 and C14 genotypes compared to WT was apparent only at the early bud development stage (Figure 4). Although the ratio of Spd/TPA was higher at the early bud stage, however, in general, Put/TPA and Spm/TPA ratios in both genotypes at the early and late bud stages of development were not different from the WT.
Flower initiation and development are known to be regulated by the hormone gibberellins (GAs) (Davis, 2009). Therefore, we surmised that PAs and GAs may interact to regulate floral programs. To understand such an association between GAs and PAs, we analyzed the expression of tomato DELLA (SlDELLA) and GA-biosynthesis genes (Figure 5). DELLA expression decreased significantly at early stages of bud development in C13 and C14 lines being 1.8- and 1.4-fold lower in C13 and C14, respectively, as compared to WT (Figure 5A).
Figure 5 Transcript levels of SlDELLA and GA-biosynthesis genes during flower development of ySpdSyn and WT tomato lines. Flower development stages, early buds (0.1–0.7 cm) and late buds (0.8–1.5 cm), were harvested from plants grown in a green house. Expression of the tomato homologue of (A) DELLA (SlDELLA); (B) GA-20oxidase-1 (GA20ox1); (C) GA-20oxidase-2 (GA20ox2); and (D) GA-3oxidase-2 (GA3ox2), during flower development in wild type, C13 and C14 flower buds was analyzed using quantitative RT-PCR (Supplemental File 1). The relative gene expression analysis was performed by the ∆∆Ct method using tomato ACTIN as a reference gene. Data shown are mean + standard error based on ting three independent biological replicates of WT, C13, and C14 at early and late bud stage of flower development. Means followed by the same letter are not significantly different from each other based on one-way analysis of variance (α = 0.05) for a given tissue type of a gene.
The expression of GA20-oxidase1 (GA20ox1) decreased by 2.2-fold in the C13 line at the early bud stage, decreasing further by 5.6-fold in the late bud stage (Figure 5B). A similar pattern was obtained for flower bud development in C14 line. Expression of GA20-oxidase2 (GA20ox2) was not significantly different at the early bud stage of both the transgenic lines; however, at the late bud stage, GA20ox2 expression was elevated ∼2- and ∼2.5-fold in the C13 and C14 lines, respectively (Figure 5C). In contrast, GA3ox2 expression was significantly downregulated ∼4-fold in both C13 and C14 lines at the early bud stage with no significant differences at the late bud stage between the WT and two transgenic lines (Figure 5D).
Put, Spd, and Spm have two, three, and four positive charges, respectively, and thus can compete differentially to bind various biomolecules (Igarashi and Kashiwagi, 2010). Changes in the levels of any specific PAs (Put, Spd, or Spm) may affect their binding to biomolecules resulting in the perturbation of various biological processes, including transcription and metabolome (Mattoo et al., 2006; Srivastava et al., 2007). We demonstrate here that the expression of CaMV35S-ySpdSyn plants results in significant changes in the ratios of Spd/Put, Put/TPA, and Spd/TPA in transgenic flowers. Transgenic plants expressing ySpdSyn gene under the control of CaMV35S promoter gave rise to two distinct populations of transgenic plants. About one-half of the independent transgenic plants exhibited normal flower development with fruit and seed set, while the other half of the transgenic population showed floral abnormalities and parthenocarpic fruit set (Figure 2). The Spd level in the transgenic flower with normal fruit and seed set phenotype was similar to WT flowers but lower by threefold in transgenic flowers with abnormal parthenocarpic fruit than WT flowers. We propose that such alterations of individual PAs and their ratios affect the phenotypes observed in transgenic flowers. Altered PA homeostasis with increased PA ratios such as Spd to Spm ratio has been recently reported in Snyder–Robinson syndrome (Murray-Stewart et al., 2018). These findings provide further support and add to the role(s) of PAs in flower development and parthenocarpy (Rastogi and Sawhney, 1990b; Antognoni et al., 2002; Fos et al., 2003; Sinha and Rajam, 2013; Chen et al., 2014)
Furthermore, these data corroborate earlier envisaged reports that each polyamine Put, Spd, and Spm differentially influence plant phenotypes, metabolism, and gene expression (Handa and Mattoo, 2010; Mattoo et al., 2010; Tiburcio and Alcázar, 2018). Thus, Spd and Spm were positively correlated with tomato fruit-quality attributes and specific metabolites in poplar cell culture and tomato pericarp in contrast to Put, which exhibited a negative correlation with these same traits (Handa and Mattoo, 2010; Mattoo et al., 2010). The importance of PA ratios in regulating various biological systems including plants and mammalian systems has been previously suggested, but their physiological significance has remained elusive (Huang et al., 2004; Murray-Stewart et al., 2018). Changes in PA ratios were also found in Gy mice and human patients with Snyder–Robinson syndrome, a genetic condition, resulting from the lack of SpmSyn activity. Both these phenotypes were found associated with increased Spd/Spm ratio due to a decrease in Spm (Pegg, 2016). Since high levels of Spd restored the normal growth of Gy mice lacking SpdSyn in cultured fibroblast, it has also been suggested that the Spm/Spd ratio plays an important role in myocyte differentiation (Luchessi et al., 2009). Interestingly, the Spd/Spm ratio has also been implicated in altering activity of Kir channels affecting the resting membrane potential, cardiac and neuronal electrical activity, and electrolyte balance (Stanfield and Sutcliffe, 2003). In addition, our findings are in sync with the contention that cellular levels of individual PAs, their homeostasis, particularly ratio among different PAs, regulate various physiological responses (Murray-Stewart et al., 2018).
Molecular basis of reduced levels of Put, Spm, and total PAs in transgenic flowers from all the transgenic lines tested here and reduction in Spd levels in only about half the independent transgenic lines tested here is not clear. Variable expression of the transgenes under the CaMV35S promoter has been previously observed in several investigations (Tieman et al., 1992; Somssich, 2019). Nonetheless, that the ratio of Spd/Spm and Spd/Put in transgenic flower with normal fruit and seed set were higher compared to transgenic abnormal transgenic flowers (Figure 3) may indicate that it is Spd ratio with Put and Spm that is crucial for the observed flower phenotypes. This warrants further investigation.
PA and GA metabolism and signaling were linked to floral and early fruit development in tomato, but the nature of these interactions was not clear (Anwar et al., 2015). High GA levels in pat-2 ovaries were associated with increased PA biosynthesis and higher Spm levels (Fos et al., 2003). In Arabidopsis, GA promotes flower development by suppressing expression of DELLA group of proteins and, in turn, promotes the expression of floral homeotic genes (Okamuro et al., 1997; Cheng et al., 2004; Tyler et al., 2004; Yu et al., 2004; Cao et al., 2006). The tomato procera mutant in which SlDELLA gene is mutated has a constitutively induced GA phenotype with facultative parthenocarpy and meristematic alterations (Carrera et al., 2012). In addition, overexpression of GA20oxidase resulted in some flowers having protruding stigma and partial parthenocarpy in tomato (García-Hurtado et al., 2012). In the present study, the expression of SlDELLA and GA-biosynthesis-related genes, GA20ox1 and GA3ox2, was significantly downregulated in those transgenic lines that had abnormal flower phenotype. Furthermore, DELLA-silenced lines are downregulated in GA20ox and GA3ox during early fruit development, suggesting that silencing of DELLA may result in higher GA levels which in turn reduce the expression of GA-biosynthetic genes through a feedback regulation (Martí et al., 2007). For instance, GA-deficient Arabidopsis mutants have a higher expression of GA biosynthesis genes, AtGA20ox1 and AtGA3ox1, which could be reduced by GA application (Yamaguchi et al., 1998; Xu et al., 1999; Matsushita et al., 2007). In light of these observations, our data on decreased expression of GA20ox1 and GA3ox2 in the C13 and C14 transgenic lines may be a result of a similar feedback regulation and suggest that PAs play an important role in flower development and fruit set likely by interacting with GA signal transduction and biosynthetic pathways in tomato. The contention that Spd levels may have an important role in flower development has been previously proposed (Malmberg, 1980; Malmberg and McIndoo, 1983; Gerats et al., 1988; Malmberg and McIndoo, 1988; Tiburcio et al., 1988; Galston and Sawhney, 1990; Rastogi and Sawhney, 1990a, Rastogi and Sawhney, 1990b; Pritsa and Voyiatzis, 2004, Pritsa and Voyiatzis, 2005; Alburquerque et al., 2006; DeDios et al., 2006). However, much more definitive evidence is required to conclude that altered PA ratios regulate floral developmental process(es). In conclusion, our studies present a compelling suggestion for a nexus between PA ratios and regulation of developmental programs in plants.
All datasets for this study are included in the manuscript/Supplementary files.
All authors had equal roles in conceptualization, data analyses, and manuscript preparation.
Studies were partly supported by a US–Israel Binational Agricultural Research and Development Fund to AH and AM (grant no. IS-3441-03) and USDA-IFAFS program grant (award no. 741740) and USDA/NIFA Hatch IND011872 to AH. AM is supported through USDA-ARS intramural Project No. 8042-21000-143-00D.
The authors declare that the research was conducted in the absence of any commercial or financial relationships that could be construed as a potential conflict of interest.
We thank Purdue University Library and University of Georgia for providing the publication cost of this manuscript.
The Supplementary Material for this article can be found online at: https://www.frontiersin.org/articles/10.3389/fpls.2019.01033/full#supplementary-material
Alburquerque, N., Egea, J., Burgos, L., Martinez-Romero, D., Valero, D., Serrano, M. (2006). The influence of polyamines on apricot ovary development and fruit set. Ann. Appl. Biol. 149, 27–33. doi: 10.1111/j.1744-7348.2006.00067.x
Alcazar, R., Garcia-Martinez, J. L., Cuevas, J. C., Tiburcio, A. F., Altabella, T. (2005). Overexpression of ADC2 in Arabidopsis induces dwarfism and late-flowering through GA deficiency. Plant J. 43, 425–436. doi: 10.1111/j.1365-313X.2005.02465.x
Anwar, R., Mattoo, A. K., Handa, A. K. (2015). “Polyamine interactions with plant hormones: crosstalk at several levels,” in Polyamines universal molecular nexus for growth, survival and specialized metabolism. Eds. Kusano, T., Suzuki, H. (Japan: Springer), 267–302. doi: 10.1007/978-4-431-55212-3_22
Antognoni, F., Ghetti, F., Mazzucato, A., Franceschetti, M., Bagni, N. (2002). Polyamine pattern during flower development in the parthenocarpicfruit (pat) mutant of tomato. Physiol. Plantarum 116, 539–547. doi: 10.1034/j.1399-3054.2002.1160413.x
Bastola, D. R., Minocha, S. C. (1995). Increased putrescine biosynthesis through transfer of mouse ornithine decarboxylase cDNA in carrot promotes somatic embryogenesis. Plant Physiol. 109, 63–71. doi: 10.1104/pp.109.1.63
Cao, D., Cheng, H., Wu, W., Soo, H. M., Peng, J. (2006). Gibberellin mobilizes distinct DELLA-dependent transcriptomes to regulate seed germination and floral development in Arabidopsis. Plant Physiol. 142, 509–525. doi: 10.1104/pp.106.082289
Carrera, E., Ruiz-Rivero, O., Peres, L. E., Atares, A., Garcia-Martinez, J. L. (2012). Characterization of the procera tomato mutant shows novel functions of the SlDELLA protein in the control of flower morphology, cell division and expansion, and the auxin-signaling pathway during fruit-set and development. Plant Physiol. 160, 1581–1596. doi: 10.1104/pp.112.204552
Chen, M., Chen, J., Fang, J., Guo, Z., Lu, S. (2014). Down-regulation of S-adenosylmethionine decarboxylase genes results in reduced plant length, pollen viability, and abiotic stress tolerance. Plant Cell Tissue Organ Cult. 116, 311–322. doi: 10.1007/s11240-013-0405-0
Cheng, H., Qin, L. J., Lee, S. C., Fu, X. D., Richards, D. E., Cao, D. N., et al. (2004). Gibberellin regulates Arabidopsis floral development via suppression of DELLA protein function. Development 131, 1055–1064. doi: 10.1242/dev.00992
Cui, X., Ge, C., Wang, R., Wang, H., Chen, W., Fu, Z., et al. (2010). The BUD2 mutation affects plant architecture through altering cytokinin and auxin responses in Arabidopsis. Cell Res. 20, 576–586. doi: 10.1038/cr.2010.51
Davis, S. J. (2009). Integrating hormones into the floral-transition pathway of Arabidopsis thaliana. Plant Cell Environ. 32, 1201–1210. doi: 10.1111/j.1365-3040.2009.01968.x
De Buck, S., De Paepe, A., Depicker, A. (2013). “Transgene expression in plants, control of,” in Sustainable food production. Eds. Christou, P., Savin, R., Costa-Pierce, B. A., Misztal, I., Whitelaw, C. B. A. (New York, NY: Springer), 1970–1593.
De Cantu, L. B., Kanderler, R. (1989). Significance of polyamines for flowering in Spirodela punctate.Plant Cell Physiol. 30, 455–458. doi: 10.1093/oxfordjournals.pcp.a077762
DeDios, P., de Matilla, A. J., Gallardo, M. (2006). Flower fertilization and fruit development prompt changes in free polyamines and ethylene in damson plum (Prunus insititia L.). J. Plant Physiol. 163, 86–97. doi: 10.1016/j.jplph.2005.03.007
Flores, H. E., Galston, A. W. (1982). Polyamines and plant stress: activation of putrescine biosynthesis by osmotic shock. Science 217, 1259–1261. doi: 10.1126/science.217.4566.1259
Fos, M., Proaño, K., Alabadí, D., Nuez, F., Carbonell, J., García-Martínez, J. L. (2003). Polyamine metabolism is altered in unpollinated parthenocarpic pat-2 tomato ovaries. Plant Physiol. 131, 359–366. doi: 10.1104/pp.013037
Galston, A. W., Sawhney, R. K. (1990). Polyamines in plant physiology. Plant Physiol. 94, 406–410. doi: 10.1104/pp.94.2.406
García-Hurtado, N., Carrera, E., Ruiz-Rivero, O., López-Gresa, M. P., Hedden, P., Gong, F., et al. (2012). The characterization of transgenic tomato overexpressing gibberellin (20-oxidase reveals induction of parthenocarpic fruit growth, higher yield, and alteration of the gibberellin biosynthetic pathway. J. Exp. Bot. 63, 5803–5813. doi: 10.1093/jxb/ers229
Ge, C., Cui, X., Wang, Y., Hu, Y., Fu, Z., Zhang, D., et al. (2006). BUD2, encoding an Sadenosylmethionine decarboxylase, is required for Arabidopsis growth and development. Cell Res. 16, 446–456. doi: 10.1038/sj.cr.7310056
Gerats, A. G., Kaye, C., Collins, C., Malmberg, R. L. (1988). Polyamine levels in petunia genotypes with normal and abnormal floral morphologies. Plant Physiol. 86, 390–393. doi: 10.1104/pp.86.2.390
Handa, A. K., Mattoo, A. K. (2010). Differential and functional interactions emphasize the multiple roles of polyamines in plants. Plant Physiol. Biochem. 48, 540–546. doi: 10.1016/j.plaphy.2010.02.009
Handa, A. K., Fatima, T., Mattoo, A. K. (2018). Polyamines: bio-molecules with diverse functions in plant and human health and disease. Front. Chem. 6, 10. doi: 10.3389/fchem.2018.00010
Huang, C. K., Chang, B. S., Wang, K. C., Her, S. J., Chen, T. W., Chen, Y. A., et al. (2004). Changes in polyamine pattern are involved in floral initiation and development in Polianthes tuberosa. J. Plant Physiol. 161, 709–713. doi: 10.1078/0176-1617-01256
Igarashi, K., Kashiwagi, K. (2010). Modulation of cellular function by polyamines. Int. J. Biochem. Cell Biol. 42, 39–51. doi: 10.1016/j.biocel.2009.07.009
Ikeuchi, M., Ogawa, Y., Iwase, A., Sugimoto, K. (2016). Plant regeneration: cellular origins and molecular mechanisms. Development 143, 1442–1451. doi: 10.1242/dev.134668
Kaur-Sawhney, R., Flores, H. E., Galston, A. W. (1980). Polyamine-induced DNA synthesis and mitosis in oat leaf protoplasts. Plant Physiol. 65, 368–371. doi: 10.1104/pp.65.2.368
Kaur-Sawhney, R., Tiburcio, A. F., Galston, A. W. (1988). Spermidine and flower-bud differentiation in thin-layer explants of tobacco. Planta 173, 282–284. doi: 10.1007/BF00403022
Kusano, T., Berberich, T., Tateda, C., Takahashi, Y. (2008). Polyamines: essential factors for growth and survival. Planta 228, 367–381. doi: 10.1007/s00425-008-0772-7
Luchessi, A. D., Cambiaghi, T. D., Hirabara, S. M., Lambertucci, R. H., Silveira, L. R., Baptista, I. L., et al. (2009). Involvement of eukaryotic translation initiation factor 5A (eIF5A) in skeletal muscle stem cell differentiation. J. Cell. Physiol. 218, 480–489.
Malmberg, R. L. (1980). Biochemical, cellular and developmental characterization of a temperature-sensitive mutant of Nicotiana tabacum and its second site revertant. Cell 22, 603–609. doi: 10.1016/0092-8674(80)90370-0
Malmberg, R. L., McIndoo, J. (1983). Abnormal floral development of a tobacco mutant with elevated polyamine levels. Nature 305, 623–625. doi: 10.1038/305623a0
Malmberg, R. L., McIndoo, J. (1988). Nicotiana plants with altered polyamine levels and floral organs. Patent US4751348.
Martí, C., Orzáez, D., Ellul, P., Moreno, V., Carbonell, J., Granell, A. (2007). Silencing of DELLA induces facultative parthenocarpy in tomato fruits. Plant J. 52, 865–876. doi: 10.1111/j.1365-313X.2007.03282.x
Martin-Tanguy, J. (2001). Metabolism and function of polyamines in plants: recent development (new approaches). Plant Growth Reg. 34, 135–148. doi: 10.1023/A:1013343106574
Matsushita, A., Furumoto, T., Ishida, S., Takahashi, Y. (2007). AGF1, an AT-hook protein, is necessary for the negative feedback of AtGA3ox1 encoding GA 3-oxidase. Plant Physiol. 143, 1152–1162. doi: 10.1104/pp.106.093542
Mattoo, A. K., Handa, A. K. (2008). Higher polyamines restore and enhance metabolic memory in ripening fruit. Plant Sci. 174, 386–393. doi: 10.1016/j.plantsci.2008.01.011
Mattoo, A. K., Minocha, S. C., Minocha, R., Handa, A. K. (2010). Polyamines and cellular metabolism in plants: transgenic approaches reveal different responses to diamine putrescine versus higher polyamines spermidine and spermine. Amino Acids 38, 405–413. doi: 10.1007/s00726-009-0399-4
Mattoo, A. K., Sobieszczuk-Nowicka, E. (2019). “Polyamine as signaling molecules and leaf senescence,” in Senescence signalling and control in plants. Eds. Sarwat, M., Tuteja, N.Elsevier: Academic Press 125–138. doi: 10.1016/B978-0-12-813187-9.00008-1
Mattoo, A. K., Sobolev, A., Goyal, R., Neelam, A., Handa, A. K., Segre, A. L. (2006). Metabolite profiles of transgenic tomato fruit engineered to accumulate polyamines spermidine and spermine using NMR spectroscopy reveal enhanced nitrogen-carbon interactions. Plant Physiol. 142, 1759–1770. doi: 10.1104/pp.106.084400
Mehta, R. A., Cassol, T., Li, N., Ali, N., Handa, A. K., Mattoo, A. K. (2002). Engineered polyamine accumulation in tomato enhances phytonutrient content, juice quality, and vine life. Nature Biotech. 20, 613–618. doi: 10.1038/nbt0602-613
Murray-Stewart, T., Dunworth, M., Foley, J. R., Schwartz, C. E., Casero, R. A., Jr (2018). Polyamine homeostasis in Snyder–Robinson syndrome. Med. Sci. (Basel). 6 (4), 112. doi: 10.3390/medsci6040112
Nambeesan, S., Datsenka, T., Ferruzzi, M. G., Malladi, A., Mattoo, A. K., Handa, A. K. (2010). Overexpression of yeast spermidine synthase impacts ripening, senescence and decay symptoms in tomato. Plant J. 63, 836–847. doi: 10.1111/j.1365-313X.2010.04286.x
Nambeesan, S., Handa, A. K., Mattoo, A. K. (2008). “Polyamines and regulation of ripening and senescence,” in Postharvest biology and technology of fruits, vegetables and flowers. Eds. Paliyath, G., Murr, D. P., Handa, A. K., Lurie, S. (Ames, IA: Blackwell Publishers), 319–340.
Okamuro, J. K., Szeto, W., Lotys-Prass, C., Jofuku, K. D. (1997). Photo and hormonal control of meristem identity in the Arabidopsis flower mutants apetala2 and apetala1. Plant Cell 9, 37–47. doi: 10.1105/tpc.9.1.37
Pegg, E. A. (2016). Functions of polyamines in mammals. J. Biol. Chem. 291, 14904–14912. doi: 10.1074/jbc.R116.731661
Pritsa, T. S., Voyiatzis, D. G. (2004). Seasonal changes in polyamine content of vegetative and reproductive olive organs in relation to floral initiation, anthesis and fruit development. Aust. J. Agri. Res. 55, 1039–1046. doi: 10.1071/AR04056
Pritsa, T. S., Voyiatzis, D. G. (2005). Correlation of ovary and leaf spermidine and spermine content with the alternate bearing habit of olive. J. Plant Physiol. 162, 1284–1291. doi: 10.1016/j.jplph.2005.01.017
Rastogi, R., Sawhney, V. K. (1990a). Polyamines and flower development in the male sterile stamenless-2 mutant of tomato (Lycopersicon esculentum Mill.). Plant Physiol. 93, 439–445. doi: 10.1104/pp.93.2.439
Rastogi, R., Sawhney, V. K. (1990b). Polyamines and flower development in the male sterile stamenless-2 mutant of tomato (Lycopersicon esculentum Mill.). II. Effects of polyamines and their biosynthetic inhibitors on the development of normal and mutant floral buds cultured in vitro.Plant Physiol. 93, 446–452. doi: 10.1104/pp.93.2.446
Seiff, H. S., Shelp, B. J. (2019). Spermine differentially refines plant defense response against biotic and abiotic stresses. Front. Plant Sci. 10, 117. doi: 10.3389/fpls.2019.00117
Sinha, R., Rajam, M. V. (2013). RNAi silencing of three homologues of S-adenosylmethionine decarboxylase gene in tapetal tissue of tomato results in male sterility. Plant Mol. Biol. 82, 169–180. doi: 10.1007/s11103-013-0051-2
Somssich, M. (2019). A short history of the CaMV 35S promoter. Peer J. Preprints 7, e27096v3. doi: 10.7287/peerj.preprints.27096
Srivastava, A., Chung, S. H., Fatima, T., Datsenka, T., Handa, A. K., Mattoo, A. K. (2007). Polyamines as anabolic growth regulators revealed by transcriptome analysis and metabolite profiles of tomato fruits engineered to accumulate spermidine and spermine. Plant Biotech. 24, 57–70. doi: 10.5511/plantbiotechnology.24.57
Stanfield, P. R., Sutcliffe, M. J. (2003). Spermine is fit to block inward rectifier (kir) channels. J. Gen. Physiol. 122, 481–484. doi: 10.1085/jgp.200308957
Stewart, T. M., Dunston, T. T., Woster, P. M., Casero, R. A., Jr. (2018). Polyamine catabolism and oxidative damage. J. Biol. Chem. 293, 18736–18745. doi: 10.1074/jbc.TM118.003337
Takahashi, T., Kakehi, J. (2009). Polyamines: ubiquitous polycations with unique roles in growth and stress responses. Ann. Bot. 105, 1–6. doi: 10.1093/aob/mcp259
Tiburcio, A. F., Alcázar, R. (2018). Potential applications of polyamines in agriculture and plant biotechnology. Methods Mol. Biol. 1694, 489–508. doi: 10.1007/978-1-4939-7398-9_40
Tiburcio, A. F., Amin Gendy, C., Tran Than Van, K. (1989). Morphogenesis in tobacco subepidermal cells: putrescine as a marker of root differentiation. Plant Cell Tissue Organ Cult. 19, 43–54. doi: 10.1007/BF00037775
Tiburcio, A. F., Kaur-Sawhney, R., Galston, A. W. (1988). Polyamine biosynthesis during vegetative- and floral-bud differentiation in thin-layer tobacco tissues cultures. Plant Cell Physiol. 29, 1241–1249. doi: 10.1093/oxfordjournals.pcp.a077629
Tieman, D. M., Harriman, R. W., Ramamohan, G., Handa, A. K. (1992). An antisense pectin methylesterase gene alters pectin chemistry and soluble solids in tomato fruit. Plant Cell 4, 667–679. doi: 10.2307/3869525
Tyler, L. S., Thomas, S. G., Hu, J. H., Dill, A., Alonso, J. M., Ecker, J. R., et al. (2004). DELLA proteins and gibberellin-regulated seed germination and floral development in Arabidopsis. Plant Physiol. 135, 1008–1019. doi: 10.1104/pp.104.039578
Walden, R., Cordeiro, A., Tiburcio, A. F. (1997). Polyamines: small molecules triggering pathways in plant growth and development. Plant Physiol. 113, 1009–1013. doi: 10.1104/pp.113.4.1009
Xu, Y. L., Li, L., Gage, D. A., Zeevaart, J. A. (1999). Feedback regulation of GA5 expression and metabolic engineering of gibberellin levels in Arabidopsis. Plant Cell 11, 927–936. doi: 10.1105/tpc.11.5.927
Yamaguchi, S., Smith, M. W., Brown, R. G., Kamiya, Y., Sun, T. (1998). Phytochrome regulation and differential expression of gibberellin 3β-hydroxylase genes in germinating Arabidopsis seeds. Plant Cell 10, 2115–2126. doi: 10.1105/tpc.10.12.2115
Yoshimoto, K., Takamura, H., Kadota, I., Motose, H., Takahashi, T. (2016). Chemical control of xylem differentiation by thermospermine, xylemin, and auxin. Sci. Rep. 6, 21487. doi: 10.1038/srep21487
Keywords: polyamines, seed set, parthenocarpy, gibberellins, transgenic tomatoes
Citation: Nambeesan SU, Mattoo AK and Handa AK (2019) Nexus Between Spermidine and Floral Organ Identity and Fruit/Seed Set in Tomato. Front. Plant Sci. 10:1033. doi: 10.3389/fpls.2019.01033
Received: 14 March 2019; Accepted: 24 July 2019;
Published: 25 September 2019.
Edited by:
Ana Margarida Fortes, University of Lisbon, PortugalReviewed by:
Pedro Carrasco, University of Valencia, SpainCopyright © 2019 Nambeesan, Mattoo and Handa. This is an open-access article distributed under the terms of the Creative Commons Attribution License (CC BY). The use, distribution or reproduction in other forums is permitted, provided the original author(s) and the copyright owner(s) are credited and that the original publication in this journal is cited, in accordance with accepted academic practice. No use, distribution or reproduction is permitted which does not comply with these terms.
*Correspondence: Avtar K. Handa, YWhhbmRhQHB1cmR1ZS5lZHU=
Disclaimer: All claims expressed in this article are solely those of the authors and do not necessarily represent those of their affiliated organizations, or those of the publisher, the editors and the reviewers. Any product that may be evaluated in this article or claim that may be made by its manufacturer is not guaranteed or endorsed by the publisher.
Research integrity at Frontiers
Learn more about the work of our research integrity team to safeguard the quality of each article we publish.