- 1MSU-DOE Plant Research Laboratory, Michigan State University, East Lansing, MI, United States
- 2Department of Biochemistry and Molecular Biology, Michigan State University, East Lansing, MI, United States
- 3Department of Plant Biology, Michigan State University, East Lansing, MI, United States
- 4Plant Resilience Institute, Michigan State University, East Lansing, MI, United States
The exchange of reduced carbon across the inner chloroplast envelope has a large impact on photosynthesis and growth. Under steady-state conditions it is thought that glucose 6-phosphate (G6P) does not cross the chloroplast membrane. However, growth at high CO2, or disruption of starch metabolism can result in the GPT2 gene for a G6P/Pi translocator to be expressed presumably allowing G6P exchange across the chloroplast envelope. We found that after an increase in light, the transcript for GPT2 transiently increases several 100-fold within 2 h in both the Col-0 and WS ecotypes of Arabidopsis thaliana. The increase in transcript for GPT2 is preceded by an increase in transcript for many transcription factors including Redox Responsive Transcription Factor 1 (RRTF1). The increase in GPT2 transcript after exposure to high light is suppressed in a mutant lacking the RRTF1 transcription factor. The GPT2 response was also suppressed in a mutant with a T-DNA insert in the gene for the triose-phosphate/Pi translocator (TPT). However, plants lacking TPT still had a robust rise in RRTF1 transcript in response to high light. From this, we conclude that both RRTF1 (and possibly other transcription factors) and high amounts of cytosolic triose phosphate are required for induction of the expression of GPT2. We hypothesize that transient GPT2 expression and subsequent translation is adaptive, allowing G6P to move into the chloroplast from the cytosol. The imported G6P can be used for starch synthesis or may flow directly into the Calvin-Benson cycle via an alternative pathway (the G6P shunt), which could be important for regulating and stabilizing photosynthetic electron transport and carbon metabolism.
Introduction
Plants live in a stochastic environment with drivers of photosynthesis such as CO2 availability and light changing rapidly as abiotic factors such as cloud cover or humidity change over the course of minutes and hours. Hexose phosphate transport into plastids has been shown to allow plants achieve higher rates of photosynthesis over the course of several days when irradiance is increased (Dyson et al., 2015). This process of acclimation involves the differential expression (DE) and changes in abundance of hundreds of proteins in the chloroplast (Miller et al., 2017). The glucose-6-phosphate/phosphate translocator 2 (GPT2) in Arabidopsis is of particular interest as the expression for this protein increases rapidly, hours as opposed to days, when irradiance is increased (Athanasiou et al., 2010). We wanted to better understand the more rapid changes in gene expression, as opposed to acclimation, that occur when the photosynthetic rate of a plant increases. To this end, we focused on the role of GPT2 in carbon exchange across the chloroplast envelope.
Hexose phosphate transport into plastids was first documented 30 years ago. It was determined that starch synthesis in heterotrophic amyloplasts used imported hexose phosphates rather than triose phosphates (Keeling et al., 1988; Tyson and ap Rees, 1988). In 1995 it was discovered that autotrophic chloroplasts could also transport glucose 6-phosphate (G6P) if they were made heterotrophic by feeding sugars in vitro or when sugars accumulate in the cytosol in vivo (Quick et al., 1995; Lloyd and Zakhleniuk, 2004). It is now known that the hexose phosphate/phosphate (Pi) translocators are part of a family of sugar phosphate and phosphoenolpyruvate translocators that include triose phosphate/Pi translocators, pentose phosphate/Pi translocators, and phosphoenolpyruvate/Pi translocators (Fischer et al., 1997; Kammerer et al., 1998; Hejazi et al., 2014). The hexose phosphate/Pi translocators (GPTs) work like the triose-phosphate/Pi translocators (TPTs). One molecule of hexose monophosphate is exchanged for one molecule of Pi or another sugar phosphate (Lee et al., 2017). The GPTs are promiscuous and will transport triose phosphates as well as, or better than, G6P, but GPTs will not transport G1P or F6P (Kammerer et al., 1998; Eicks et al., 2002).
The concentration of G6P in the chloplast and cytosol has been measured in the light five times in four species, spinach,potato, bean, and Arabidopsis and one species, bean, in the dark. In all cases the G6P concentration was found to be greater in the cytosol than the stroma both day and night (Gerhardt et al., 1987; Sharkey and Vassey, 1989; Schleucher et al., 1999; Weise et al., 2006; Szecowka et al., 2013). This makes it unlikely that expression of a glucose-6-phosphate/Pi translocator results in net G6P export from the chloroplast but rather G6P would be transported from the cytosol to the stroma. Two GPTs have been identified in Arabidopsis, GPT1 At5g54800, and GPT2 At1g61800 (Niewiadomski et al., 2005). GPT1 has a low constitutive level of expression in almost all tissue and is necessary for embryo sac development and pollen maturation; gpt1 mutants are embryo lethal (Niewiadomski et al., 2005). GPT2 has low, almost undetectable, expression in all tissues but high expression amounts have been seen in leaves when plants are fed or accumulate sugars (Lloyd and Zakhleniuk, 2004; Dyson et al., 2014). Plants in which starch metabolism is compromised have higher expression of GPT2 and chloroplast membranes are able to transport G6P (Kunz et al., 2010). GPT2 transcript also increases in leaves in response to an increase in light and plays a role in the photosynthetic acclimation to high light (Athanasiou et al., 2010; Dyson et al., 2015).
Our goal was to better understand the regulation and metabolic role of GPT2 in day time metabolism. We measured transcript amounts using both qPCR and RNA-Seq of GPT2 and other related genes in both the Columbia-0 and Wassilewskija ecotypes of Arabidopsis and a number of mutants under a variety of photosynthetic conditions. From this work, we found that GPT2 is transcriptionally regulated and requires both triose phosphate export from the chloroplast and the Redox Responsive Transcription Factor 1 (RRTF1) for expression. We hypothesize that GPT2 plays an important role in facilitating starch synthesis in stochastic high light conditions and may be necessary to maintain the redox poise of the cytosol.
Materials and Methods
Plant Material and Growing Conditions
Wild-type Arabidopsis thaliana ecotype Columbia-0 (Col-0) and Wassilewskija (WS) and the mutants listed in Table 1 were used. All mutants used have been previously characterized with the exception of tpt-3 SALK_028503. We found that the T-DNA insert was in the first exon based on the primer sites used to confirm the line was homozygous for the insert. Transcript of the TPT gene was checked by qPCR and was below our detection limit (results not shown).
Plants were grown in a fluorescently lit growth chamber under a 12 h photoperiod with a photon flux density (PFD) of 120 μmol m–2 s–1. The daytime temperature was 23°C and the nighttime temperature was 20°C. Humidity was held at a minimum of 60% relative humidity. All plants used in this study were grown hydroponically to prevent drought stress and other stresses that might affect expression of genes being studied. The hydroponic setup used also minimized water vapor and CO2 loss from soil which can confound gas exchange measurements. Seeds were germinated on rockwool plugs which were placed in 1.5 ml microfuge tubes with the lower 1.5 cm tip cut off and watered with half-strength Hoagland’s solution (Hoagland and Arnon, 1938). Once roots started to grow through the bottom of the plug, the plants were transferred to a water culture hydroponic system in which plants were suspended over a vigorously aerated half-strength Hoagland’s solution contained in a dark tub. For all experiments, except RNA-Seq, data was collected from five biological replicates.
Changing Light and CO2 Environments
Plants were placed so that their roots were suspended in a 50 ml disposable conical centrifuge tube containing half-strength Hoagland’s solution. The tube and plant were then placed in a LI-COR 6800 or 6400 portable gas exchange system (LI-COR Biosciences, Lincoln, NE, United States). Both systems used the small plant chamber lit with a LI-COR 6800-03 or LI-COR 6400-18A light source set to white light. Plants from each ecotype and mutant line were used in both systems to control for any system effect on gene expression. White light was maintained at a PFD of 120 μmol m–2 s–1 or 500 μmol m–2 s–1. Air temperature was held constant at 23°C and humidity was controlled at a dew point of 17 to 18.5°C. The CO2 concentration in the sample air, Ca, was held at 20, 400, or 1000 ppm. A single leaf was taken from each plant after 0, 15, 30, 60, 120, and 240 min.
Photosynthesis Measurements
For photosynthetic and fluorescence measurements a single leaf was placed in a LI-COR 6800-01A multiphase flash fluorometer gas exchange chamber. For these measurements, leaf temperature was held constant at 23°C and humidity was controlled at a dew point of 17 to 18.5°C. The lighting was 50% red and 50% blue actinic light which was provided by the LEDs in the fluorometer head. All fluorometer settings were left at the factory default. The relative electron transport rate was calculated as ΦPSII × I (light intensity) × α (leaf absorptivity assumed to be 0.85) × β (fraction of quanta absorbed by PSII, assumed to be 0.5). The numbers for α and β are the default parameters provided by LI-COR. Because these were not measured in our leaves the ETR is taken as a relative rather than absolute measurement.
qPCR and Transcript Analysis
RNA was extracted using a Qiagen RNeasy Plant Mini Kit according to the manufacturer’s directions. Once RNA was isolated, cDNA was synthesized using 1 μg of total RNA. Invitrogen Super Script II reverse transcriptase (18064, Thermo Fisher Scientific, Waltham, MA, United States) was used according to manufacturer’s directions. An Eppendorf Mastercycler ep Realplex qPCR thermocycler with a 96 position silver block with SYBR green PCR master mix (4309155 Applied Biosystems, Carlsbad, CA United States) was used according to the manufacturer’s directions. The thermal profile was: 95°C for 10 min; 40 cycles of 95°C for 15 s, and 60°C for 1 min during which fluorescence was measured. This was followed by a melting curve of: 95°C for 15 s, 60°C for 15 s, a ramp from 60 to 95°C over a 20 min period during which time fluorescence was monitored, and 95°C for 15 s. Transcript amounts were normalized using the ACTIN2 (ACT2) or ISOPENTENYL DIPHOSPHATE ISOMERASE2 (IDI2) housekeeping genes. Absolute transcript amounts of these genes were checked during the 4-h time course in altered light or CO2 environments. This was done to ensure that the experimental treatment did not result in changes in housekeeping gene transcripts. Sequences for primers used are listed in Supplementary Table S1. Absolute copy number of transcripts was determined by using a slightly larger cDNA fragment of each target sequence as a standard. These cDNA standards were quantitated and dilutions were used to prepare standard curves.
Statistics
Box plots and statistical differences in GPT2 expression between WT and mutants were tested by one-way ANOVA followed by Tukey’s test using Microcal Origin 8.0 (Origin Lab Corporation, Northampton, MA, United States). Three levels of significance were tested and indicated by + α = 0.1; *α = 0.05; ∗∗α = 0.01. Box plots are presented with the box encompassing the middle two quartiles, the mean shown as an open square inside the box, the median as a line inside the box, and the whiskers showing the standard error of the data.
RNA-Seq Sampling and Sequencing
Three Col-0 and WS plants were used for RNA-Seq analysis and treated to a PFD of 500 μmol m–2 s–1 and sampled as above. All samples had an RNA integrity number of at least 7.0 as determined with a 2100 Bioanalyzer (Agilent Technologies, Santa Clara, CA, United States).
Single-end 50-bp mRNA sequencing was performed at the Michigan State University Research Technology Support Facility Genomics Core1. Libraries were prepared using the Illumina TruSeq Stranded mRNA Library Preparation Kit (Illumina, San Diego, CA, United States) following the manufacturer’s recommendations. Completed libraries were assessed for quality and quantified using a combination of Qubit dsDNA High Sensitivity Assay Kit (Qubit, Carlsbad, CA, United States), Caliper LabChipGX System and a DNA High Sensitivity Assay Kit (Caliper Life Sciences, Waltham, MA, United States), and Kapa Illumina Library Quantification qPCR assays (Illumina, San Diego, CA, United States). Each set of libraries were pooled in equimolar quantities and loaded on one lane of an Illumina HiSeq 2500 High Output flow cell (v4) (Illumina, San Diego, CA, United States). Sequencing was carried out in a 1 × 50 bp (SE50) format using HiSeq SBS reagents (v4) (Illumina, San Diego, CA, United States). Base calling was done by Illumina Real-Time Analysis (R-TA) v1.18.64 and output of RTA was demultiplexed and converted to FastQ format with Illumina Bcl2fastq v1.8.4. The average number of sequencing reads was 41.7 ± 11.2 million per sample.
RNA-Seq Data Analysis
RNA-Seq data were subjected to gene DE analysis. Briefly, sequencing adapters and low quality bases were trimmed from sequencing reads using Trimmomatic version 0.32 (Bolger et al., 2014), and cleaned reads were examined using FASTQC software2 for quality evaluation. Reads were aligned to the reference genome, A. thaliana TAIR10 (Berardini et al., 2015), using the STAR (2.3.0e) alignment program (Dobin et al., 2013). The featureCounts function in the Rsubread (version 3) package for R was used to calculate read counts for each gene (Liao et al., 2013), and data were normalized as reads per kilobase of transcript per million mapped reads, (RPKM). We removed data for genes that had no reading higher that 7 RPKM and genes whose expression did not vary by more than two-fold (log2 < 1). Data represented in heat maps is after 15, 30, 60, 120, or 240 min at a PFD of 500 μmol m–2 s–1. For metabolic genes the data is the log2 of the ratio of the average RPKM value before and after light treatment. Data represented in heat maps of transcription factors is the log2 of the difference in the average RPKM value plus one before and after light treatment. Data for heat maps of transcription factors was calculated as a difference rather than a ratio because many transcription factors were not expressed or had expression amounts below our detection limit, RPKM = 0, before the light treatment. Heat maps were generated using the online Morpheus software3. The raw and processed data were deposited in the Gene Expression Omnibus (Edgar et al., 2002) and are accessible through GEO Series accession number GSEXXXXX4. (Raw RNA-Seq data is being submitted to NCBI, and accession numbers will be added during the revision process).
Results
GPT2 Expression Is Related to Daytime Rather Than Nighttime Metabolism
In mutants of Arabidopsis that are unable to synthesize starch due to a mutation in the gene encoding the plastid phosphoglucomutase (pgm1-1), GPT2 transcripts amounts were more than two-fold higher than in the wild type WS (Figure 1A). A similar result is seen in a mutant lacking the glucan water dikinase 1 (sex1-8) (Figure 1B). In both the pgm1-1 and sex1-8 mutants starch synthesis does not occur in fully mature leaves. Although sex1-8 mutant leaves have a lot more starch than wild-type, a threshold is reached at some point in the life cycle of the leaf and further accumulation does not occur (Caspar et al., 1991; Trethewey and ap Rees, 1994; Hejazi et al., 2014). However, when the ability to make G6P from starch degradation in the plastid is blocked by eliminating the plastidic starch phosphorylase (phs1-1), GPT2 transcript amounts were unchanged (Figure 1C). GPT2 transcript amounts were also elevated in a mutant lacking plastidic Calvin-Benson cycle enzyme fructose 1,6-bisphosphatase (hcef1) (Figure 1D). GPT2 transcript increases when starch synthesis or early steps in the Calvin-Benson cycle are blocked. However, the lack of GPT2 transcript when, G6P producing, phosphorolytic starch degradation is blocked, suggests a more significant role for GPT2 in daytime metabolism.
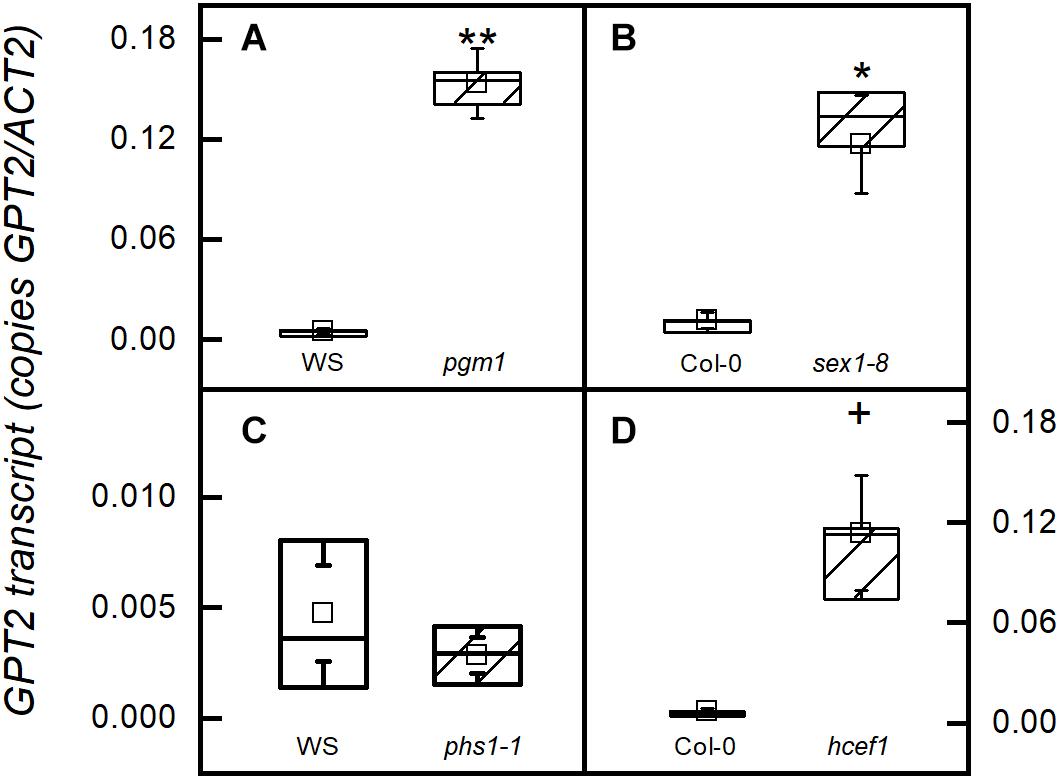
Figure 1. GPT2 expression in starch related mutants. All samples were taken from plants in growth conditions, PFD 120 μmol m–2 s–1 and 400 ppm CO2. Transcripts were measured in (A) a starchless mutant (pgm1-1), (B) a starch excess mutant (sex1-8) that cannot degrade starch, (C) a starch phosphorylase knock out (phs1-1) that cannot carry out phosphorolytic starch degradation, and (D) a chloroplastic FBPase mutant (hcef1). *α = 0.05; ∗∗α = 0.01; + α = 0.1, n = 5.
GPT2 Is Expressed When Photosynthesis Is Increased by Light
A switch from a PFD of 120 to 500 μmol m–2 s–1 increased GPT2 expression in Col-0, but 500 μmol m–2 s–1 of light with photosynthesis restricted by 20 ppm CO2 did not result in increased expression (Figures 2A,B). 1000 ppm CO2 alone did not result in an increase in GPT2 transcript (Figure 2C). Plants were also placed in the gas exchange system at growth conditions of 120 μmol m–2 s–1 of light and 400 ppm CO2 to make sure that moving the plants into the gas exchange system for 4 h did not result in an increase in transcript (Figure 2D). The same patterns were observed in the WS ecotype (Supplementary Figure S1).
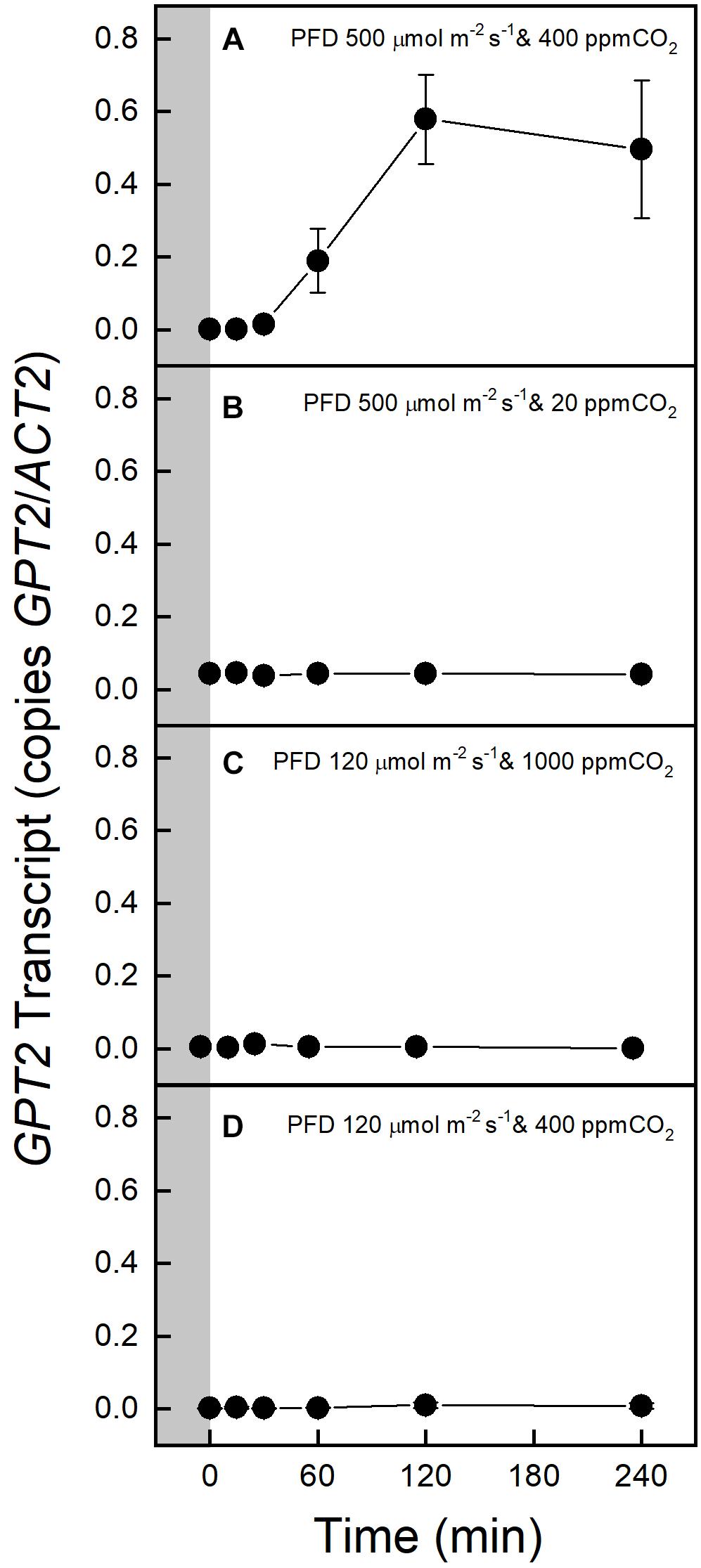
Figure 2. Expression of GPT2 in Col-0 before and after transfer to an altered light or CO2 environment. The gray block indicates sampling in growth conditions, PFD 120 μmol m–2 s–1 and 400 ppm CO2. Panels A–D show responses to various light and CO2 treatments as indicated on the figure. n = 5.
The effect of an increased PFD of 500 μmol m–2 s–1 on photosynthesis was determined by taking A-Ci curves before and after the 4-h light treatment. No change was observed in the Col-0 or WS ecotype, indicating that there were no significant non-photochemical processes or photo damage (Figure 3 and Supplementary Figure S2). To better understand why increased light but not increased CO2 resulted in an increase in GPT2 transcript both photosynthesis and relative electron transport rates (ETR) were measured at a variety of different PFDs and CO2 concentrations. The relative electron transport rate divided by four was taken as an approximate turnover rate of the Calvin-Benson cycle. This is because four electrons are used per two NADPH needed for RuBP regeneration. Increasing the PFD from 120 to 500 μmol m–2 s–1 increased the photosynthetic rate by 86% and increased the Calvin-Benson cycle turnover by 122% (Supplementary Figures S3A,B). Increasing CO2 alone caused a 40% increase in assimilation but only a 1.4% increase in Calvin-Benson cycle turnover (Supplementary Figures 3C,D). The increase in light resulted in a much larger increase in photosynthesis and Calvin-Benson cycle turnover than did an increase in CO2.
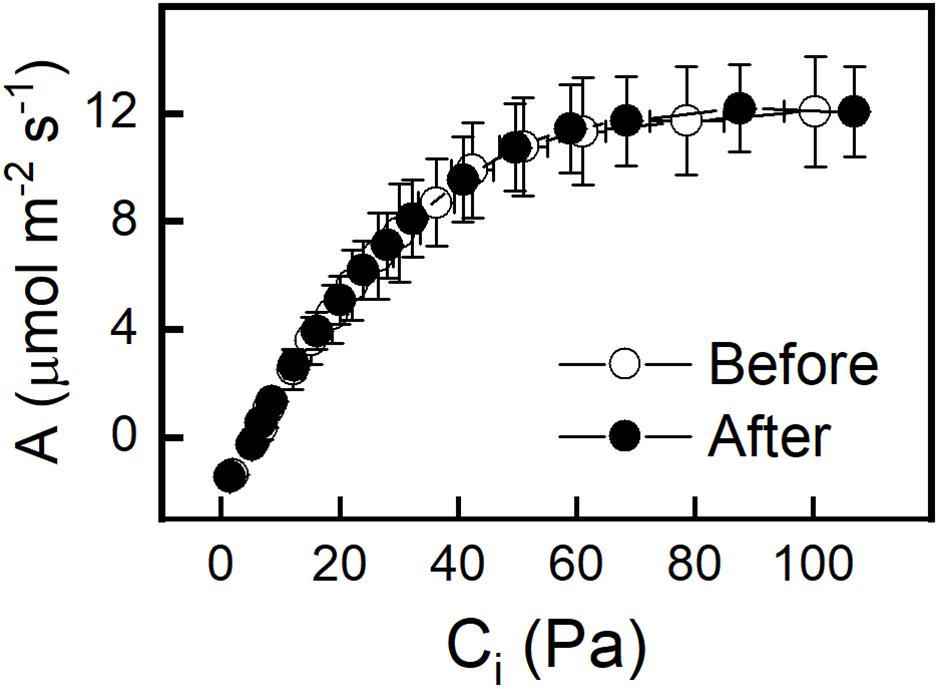
Figure 3. A – Ci curves of Col-0 taken before and after 4 h incubation at a PFD of 500 μmol m–2 s–1 and Ca of 400 ppm. A – Ci curves were done at a PFD of 500 μmol m–2 s–1. n = 5.
GPT2 and XPT Expression Are Not Affected by Each Other
The XPT may transport pentose phosphates into the chloroplast from the cytosolic oxidative pentose phosphate pathway (cytosolic G6P shunt). GPT2 transcript was measured in the xpt-2 mutant. No significant difference was observed between the xpt-2 mutant and wild type in response to an increased PFD of 500 μmol m–2 s–1 (Supplementary Figure S4A). There was also no difference in GPT2 expression when the plastidic starch phosphorylase (phs1-1) was missing (Supplementary Figure S4B). Conversely XPT transcript was measured in response to increased light in the gpt2-1 mutant. There was no transcriptional evidence for XPT compensating for GPT2. Transcript amount of XPT was low and unchanged and was also unchanged in the phs1-1 mutant background (Supplementary Figures S4C,D).
Starch Synthesis Is Upregulated While Other Pathways Are Downregulated
An RNA-Seq analysis was performed to examine changes in the entire transcriptome when plants were taken from low to high light. RNA was taken from leaves after they were exposed to high light at ambient CO2 conditions for 15, 30, 60, 120, and 240 min and the ratio in transcript amount from the zero time point (low light, ambient CO2) was determined. Genes coding for enzymes in the Calvin-Benson cycle, the chloroplast G6P shunt, starch synthesis, sucrose synthesis, and cytosolic glycolysis were identified. A complete list of the gene loci for biochemical pathways of interest was collated (Supplementary Appendix S1). From these lists, only transcripts with an RPKM value greater than 7 and an absolute fold change greater than 2 (log2 value > | 1|) at any one time point were examined. The Arabidopsis gene loci from Supplementary Appendix S1 that were identified as being differentially expressed are listed in Supplementary Table S2.
Transcripts for every enzyme in the Calvin-Benson cycle and of genes coding for electron transport proteins, identified by Dyson et al. (2015), decreased during the 240 min high light treatment. The transcripts meeting the cutoff stated above for enzymes in the Calvin-Benson cycle are shown in Figure 4. There was a large increase in transcript amounts for most enzymes involved in starch synthesis (Figure 4). There was a steep decline in transcripts for the most highly expressed sucrose synthesizing enzymes sucrose-phosphate synthase 4 (SPS4) and sucrose-phosphate phosphatase 2 (SPP2) (Figure 4). GPT2 transcript was much higher in the RNA-Seq experiment as had been seen with qPCR (Figure 2A). The transcript amount for the TPT decreased by 15 min and was down 65% after 120 min. The same pattern of changes was observed in the WS ecotype (Supplementary Figure S5).
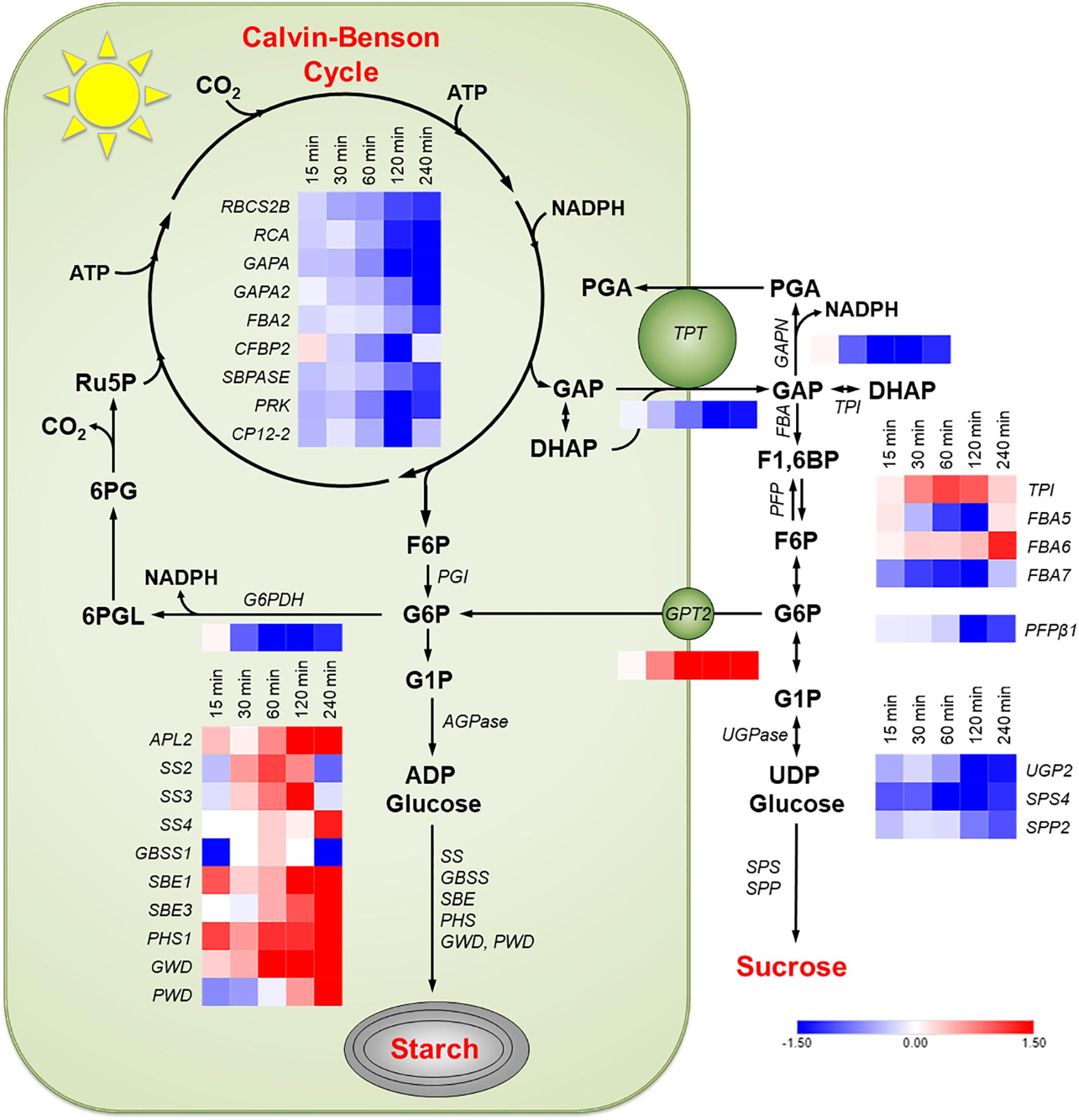
Figure 4. Changes in transcription in Col-0 in response to an increase in PFD from 120 to 500 μmol m–2 s–1. Transcripts of the following pathways or genes are shown: Calvin-Benson cycle, starch synthesis, sucrose synthesis, the G6P shunt, the non-phosphorylating GAPDH (GAPN) in the cytosol, the glucose-6-phosphate transporter (GPT2), and the triose phosphate transporter (TPT). Red boxes represent an increase in transcription and blue a decrease. Expression values shown had a log2 RPKM fold change greater than one and are on an absolute scale of –1.5 to 1.5. n = 3.
Transcript amounts for proteins involved in the cytosolic glycolysis pathway were examined. Most genes in this pathway were not differentially expressed. Two uncharacterized pyruvate kinases, At5g63680, and At3g52990 had increased transcript amounts (data not shown). Arabidopsis has three glyceraldehyde phosphate dehydrogenases in the cytosol. The only one that was differentially expressed in response to high light was the non-phosphorylating glyceraldehyde-3-phosphate dehydrogenase (GAPN). GAPN transcript was down 56% after 120 min (Figure 4). This pattern was also observed in the WS ecotype (Supplementary Figure S5). G6P shunt (Sharkey and Weise, 2016) transcripts were also examined in Col-0 and WS. Transcript for glucose-6-phosphate dehydrogenase 1 (G6PDH) decreased by 87% (Figure 3 and Supplementary Figure S6). This decrease was validated by qPCR. G6PDH2 also decreased and G6PDH3 had a very low and unchanging expression amount (Figure 5 and Supplementary Figure S6).
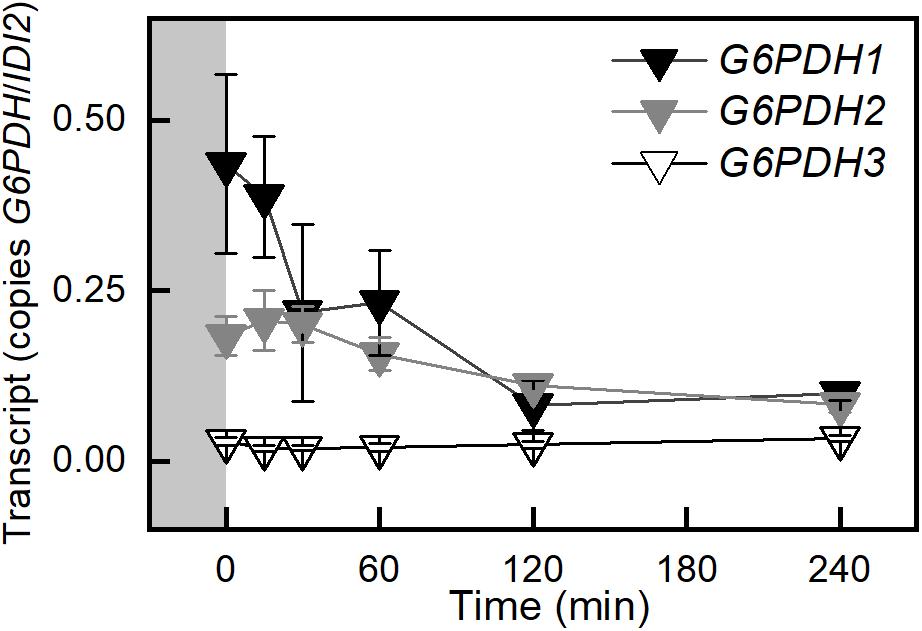
Figure 5. Changes in transcription of the plastid G6PDHs in Col-0 in response to an increase in PFD from 120 to 500 μmol m–2 s–1 Ca 400 ppm. Gray block indicates sampling in growth conditions, PFD 120 μmol m–2 s–1 and 400 ppm CO2. n = 5.
Transcript amounts for proteins involved in protecting photosynthetic electron transport and PSII repair were examined to determine if light stress was a factor in these experiments. Transcripts of eight genes were differentially expressed according to the cutoff criteria stated above. From this group of eight, seven had decreasing transcript amounts and only one PSII assembly factor Slr1768 At5g51570 (Nickelsen and Rengstl, 2013), had an approximate 2.7 fold increase after 4 h (data not shown). Because there was no decrease in photosynthesis after 4 h at a PFD of 500 μmol m–2 s–1 and transcript of only one gene for PSII repair increased, it was concluded that light stress was not a significant factor.
Transcripts for 30% of Transcription Factors Were Differentially Expressed
Transcript amounts were examined from the RNA-Seq data of all genes identified as transcription factors or possible transcription factors by the Arabidopsis Gene Regulatory Information Server (AGRIS5). From this initial pool of 1823 genes 183 were discarded because their sequences were not detected in this experiment. Four hundred and 84 genes, 30%, were selected that had a change in the RPKM value (ΔRPKM) between zero and either 15, 30, 60, 120, or 240 min time points in either Col-0 or WS that was greater than or equal to seven. This cutoff eliminated as many non-responding transcription factors as possible while still containing 15 of the 19 transcription factors that were identified by Vogel et al. (2014) as being quickly upregulated in response to high light and involved in retrograde, chloroplast to nucleus, signaling. From this pool 227 and 209 transcription factors, 13%, in Col-0 and WS, respectively, were selected that showed increased expression in response to light. Increased expression was defined as transcripts with a RPKM value that increased from the initial time zero value by two or more at any of the sampling time points and did not decrease at any time point by more than the initial RPKM value minus two. These pools contained 11 and 10 of the Vogel et al. (2014) transcription factors in Col-0 and WS, respectively.
Waves of increased expression of transcription factors were observed between the 15 and 240 min sampling time points (Figure 6 and Supplementary Figure S5). Transcription factors that had a maximal transcript abundance at the 30 or 60 min sampling time points were of particular interest because they preceded the maximal abundance of GPT2 at 120 min (Figures 2, 4). From this list of 19 transcription factors identified by Vogel et al. (2014) the RRTF1 had the highest transcript abundance at the 30 or 60 min sampling time (Figure 6 and Supplementary Figure S7).
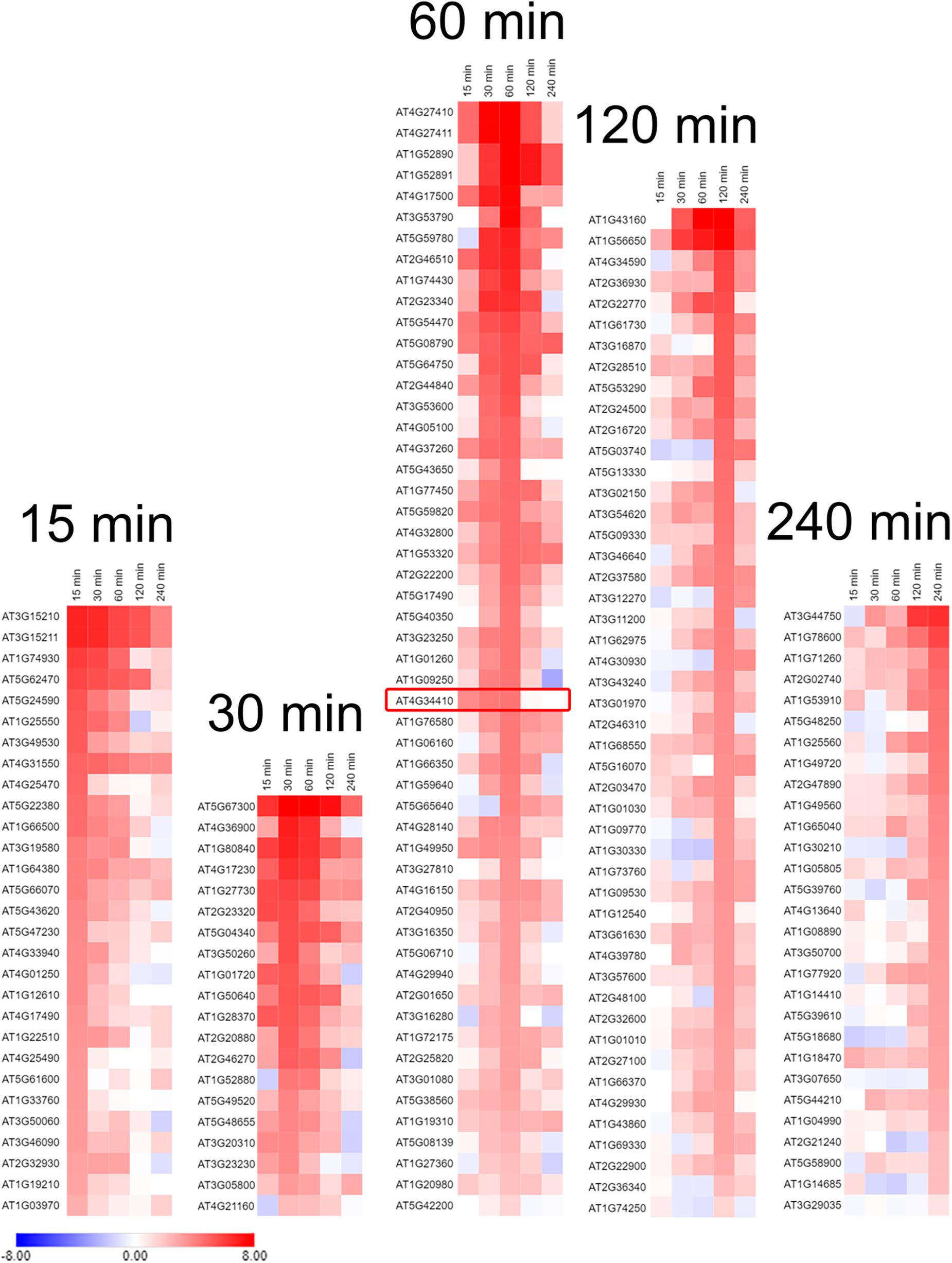
Figure 6. Transcription factors in Col-0 whose expression was increased in response to an increase in PFD from 120 to 500 μmol m–2 s–1. Transcription factors are grouped according to the time they had the highest transcript abundance after transfer to high light. The red box is around the RRTF1 transcription factor. Expression values used for heat map generation are the log2 ΔRPKM + 1 and are on an absolute scale of –8 to 8. n = 3.
GPT2 Expression Is Repressed in the tpt-3 and rrtf1-1 Mutants
Based on the results of transcript analysis by qPCR or RNA-Seq the expression of GPT2 or RRTF1 was examined in various mutant backgrounds. GPT2 transcript was elevated 34 fold in the pgm1-1 mutant at time 0 in a PFD of 120 μmol m–2 s–1 (Figure 7A). This was similar in magnitude to the increased GPT2 expression in the pgm1-1 mutant observed in Figure 1. This 34 fold increase in GPT2 was dwarfed by the 1,321 fold increase observed after 4 h at a PFD of 500 μmol m–2 s–1 and thus cannot be seen in the figure (Figure 7A). In Col-0 or WS after 240 min the expression of GPT2 was attenuated. This attenuation did not occur in pgm1-1 mutant plants with transcript amounts of 120% higher than WT (Figure 7A). In plants missing the TPT, the increase in expression of GPT2 in response to high light was reduced by 74% (Figure 7A). This was also observed in a tpt-1 mutant line in the WS background (Supplementary Figure S8A). GPT2 expression was reduced in rrtf1-1 mutant plants (Figure 7A). To determine if triose phosphates in the cytosol controlled the expression of RRTF1, RRFT1 expression was measured in tpt-3 mutant plants. RRTF1 transcript increased in response to high light in the tpt-3 mutant to the same degree as WT in with the Col-0 and WS backgrounds (Figure 7B and Supplementary Figure S8B). The pattern of RRTF1 expression was the same as was observed in the RNA-Seq data.
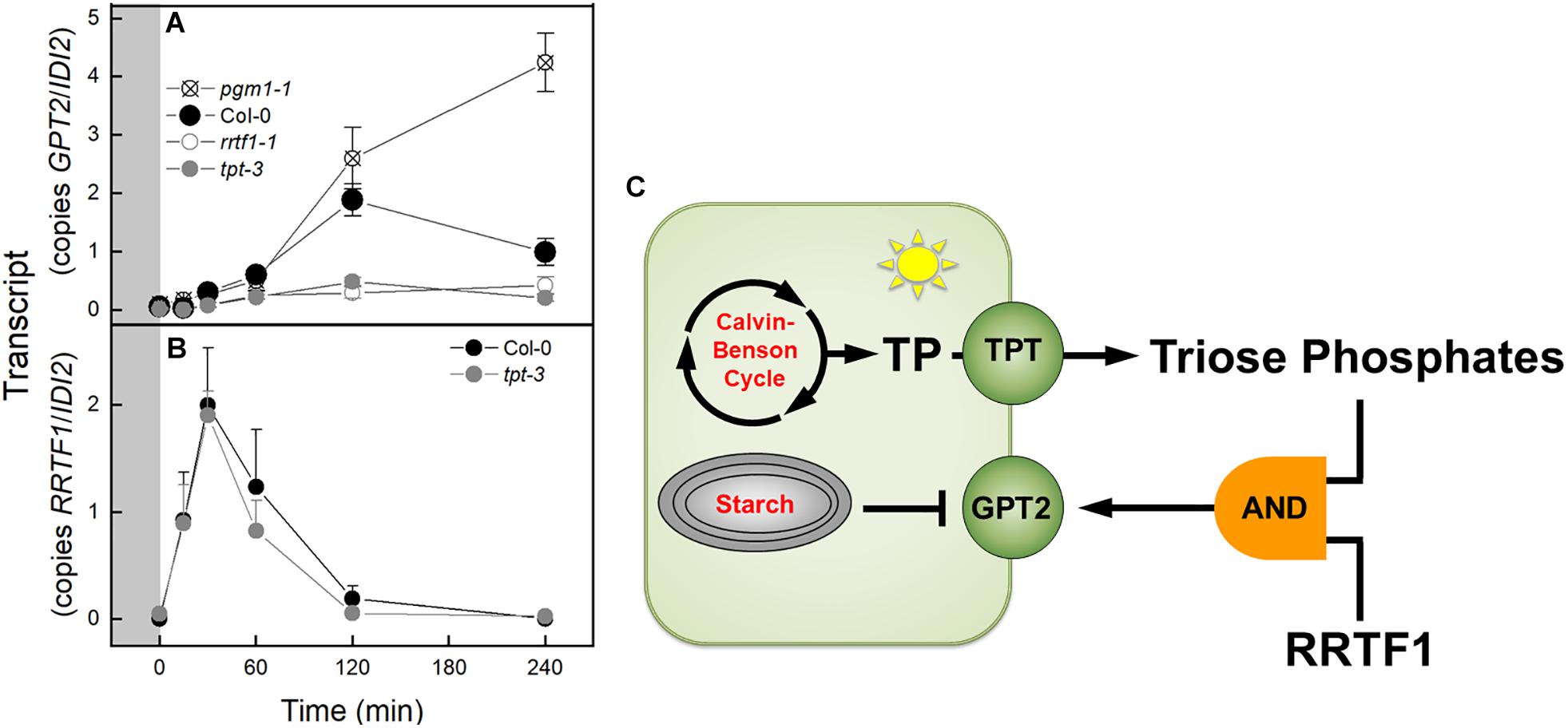
Figure 7. Expression of GPT2 and RRTF1 in response to an increase in PFD from 120 to 500 μmol m–2 s–1 Ca 400 ppm in Col-0 and mutants and model of transcriptional regulation of GPT2. (A) qPCR data of expression of GPT2 in Col-0 and pgm1-1, rrtf1-1, and tpt-3 mutants. n = 5 (B) qPCR data of expression of RRTF1 in Col-0 and tpt-3 mutant. n = 5 (C) Model of transcriptional regulation of GPT2 expression. Triose phosphates in the cytosol and RRTF1 are both required for expression of GPT2 (shown as an “AND” logic gate) while starch synthesis represses GPT2 expression.
Discussion
Expression of GPT2 Requires Triose Phosphate Export and RRTF1
Expression of both TPT and RRTF1 are necessary for the increased expression of GPT2. If either one of these is missing transcript of GPT2 does not increase in response to an increase in light. The lack of GPT2 transcript response to an increase in light in a tpt mutant has been seen before (Kunz et al., 2010; Heinrichs et al., 2012). This is presumably due to low triose phosphate concentration in the cytosol. The triose phosphates themselves could act as a signal (Häusler et al., 2014). Another possibility is that the sucrose generated from the triose phosphates is acting as a signal. In similar light jump experiments to ours, Schmitz et al. (2014) measured sucrose amounts in Col-0 and the adg1-1 starchless mutant of Arabidopsis for 8.3 h after being transferred from a PFD of 30 to 300 μmol m–2 s–1. The pattern of sucrose accumulation in the leaves mirrors the pattern of GPT2 transcript seen in WT and the pgm1-1 mutant. Sucrose would present a more stable signal than rapidly fluctuating triose phosphate amounts and signaling by sucrose via invertases and hexokinase is well documented (Li and Sheen, 2016).
A third possibility is that a change in export of triose phosphates could cause change in the redox status of the cytosol. Glyceraldehyde 3-phosphate (GAP) could be converted to phosphoglycerate (PGA) by the non-phosphorylating glyceraldehyde-3-phosphate dehydrogenase (GAPN) resulting in the production of NADPH in the cytosol (Habenicht, 1997). The PGA could then be reimported to the chloroplast via the TPT or further metabolized and imported as PEP, or pyruvate. This could result in a change in the redox poise of the cytosol that is concomitant with a change in triose phosphate export. This change in redox could be the signal that activates RRTF1 (Vogel et al., 2014). However, based on our results, RRTF1 is induced independently of triose phosphate concentration in the cytosol. The increase in RRTF1 transcript in the tpt-3 mutant (Figure 7B) was not seen by Vogel et al. (2014). After carefully scrutinizing the methods of the Vogel et al. (2014) study the reason for this difference is unclear.
There are likely other transcription factors that influence GPT2 expression. Our RNA-Seq results revealed over 70 transcription factors with a similar transcript profile to RRTF1 (Figure 6). Further the regulation of GPT2 transcript by RRTF1 may be indirect. However, RRTF1 was of particular interest because of its identification by Vogel et al. (2014) as early acting – minutes as opposed to days – in response to increased light and to be involved in retrograde, chloroplast to nucleus, signaling. RRTF1 had the highest expression of the 19 transcription factors identified by Vogel in the 30–60 min window preceding the rise in GPT2 expression. To determine if the interaction between GPT2 and RRTF1 is direct, experiments could be done in which the promoter of GPT2 is tested for interaction with RRTF1 protein using a yeast one-hybrid system or a plant cell GUS-dependent assay (Berger et al., 2007). Based on our results with both tpt-3 and rrtf1-1 mutants we conclude that at least both TPT and RRTF1 are required for expression of GPT2, the two acting as inputs to a logic “AND” gate (Figure 7C).
When starch metabolism is blocked, GPT2 transcript continues to increase in response to increased light but in WT, GPT2 transcript amount declines after 4 h (Figure 7A). This attenuation could be caused by a reduction of triose phosphate entry into the cytosol. It could also be caused by an increase in triose phosphate conversion to hexose phosphate and re-import into the chloroplast and starch synthesis. This possibility is consistent with the increase in TPI and FBP aldolase (FBA6) expression (Figure 4). Transcript amounts for enzymes in starch synthesis increased after 15 min of higher light and an increase in protein and activity would be possible after 2- 4 h (Figure 4 and Supplementary Figure S5), when a drop in GPT2 transcript is observed (Figure 2A and Supplementary Figure S1A).
GPT2 could play an important role in controlling the source-sink balance of triose phosphates in the cytosol. When there is a rapid increase in the photosynthetic rate, caused by light, transcripts of genes related to sugar phosphate anabolism might be expected to increase. However, there is a large decline in transcripts of genes in the Calvin-Benson cycle and of the triose phosphate transporter. This leads to the hypothesis that unregulated triose phosphate production and export are a problem for the plant. GPT2 expression could lead to more GPT2 protein in the chloroplast envelope. This may result in a larger plastid sink for hexose phosphate and in turn a greater proportion of triose phosphates being converted to hexose phosphates and used in starch synthesis. This would decrease the amount of GAP that could be acted upon by GAPN (Figure 4, Pathway) and prevent an over reduction of the cytosol.
Starch and sucrose are the two largest end products of photosynthesis (McClain and Sharkey, 2019). When photosynthesis increases, an increase in transcript amounts for enzymes in both starch and sucrose synthesis could be expected. However, a reduction in expression of three critical genes involved in sucrose synthesis was observed (Figure 4 and Supplementary Figure S5). In the process of acclimation, days as opposed to hours, an increase in enzymes related to sucrose synthesis has been observed in response to increased light (Miller et al., 2017). In the short term, there is generally a stronger increase of transcripts for processes leading from triose phosphates to hexose phosphate with triose phosphate isomerase standing out, having over a twofold increase in transcript amounts 60 min after being transferred to high light. Changes in expression related to G6P metabolism and transport may serve two functions. It may increase starch synthesis by bypassing the plastidic PGI, or it may provide an additional sink for triose phosphates so that GAP is not converted to PGA by GAPN, disturbing the redox poise of the cytosol.
Decreasing Transcript for G6PDH1 May Limit Carbon Entry Into the G6P Shunt
The import of G6P via GPT2 could be problematic because it could also be acted on by glucose-6-phosphate dehydrogenase (G6PDH) in the chloroplast (Preiser et al., 2019). G6PDH is generally considered to be deactivated in the light when the chloroplast stroma is reduced. During the day, when the stroma is reduced, the Km of G6PDH increases, but some residual activity remains (Scheibe et al., 1989; Hauschild and von Schaewen, 2003; Preiser et al., 2019). It has been shown that this inhibition of activity can be reversed in bacteria by low mM concentrations of G6P (Cossar et al., 1984). This would result in the G6P entering the G6P shunt leading to a futile cycle consuming ATP and releasing CO2 (Sharkey and Weise, 2016; Preiser et al., 2019). Under certain circumstances this shunt may be adaptive allowing the generation of pentose phosphates to restart the Calvin-Benson cycle when triose phosphates are depleted. It could also mitigate a high ATP/NADPH ratio under conditions of high cyclic electron transport (Sharkey and Weise, 2016). Arabidopsis has three G6PDH enzymes in the chloroplast, G6PDH1, 2, and 3 (Wakao and Benning, 2005). Transcript for G6PDH1 (Figure 5 and Supplementary Figure S6), and to a lesser extent G6PDH2, rapidly decrease after the light is increased. It has been proposed that these two contribute the majority of activity for the chloroplast G6PDHs (Wakao and Benning, 2005). One interpretation of the data is that expression of GPT2 in response to increased light leads to an increase in G6P concentration in the stroma and the reduction in expression of G6PDH1 and 2 lead to reduced G6PDH activity, limiting the flow of carbon into the G6P shunt.
GPT2 Expression May Reflect Daytime Metabolic Needs Rather Than Nighttime
Mutants of Arabidopsis that are unable to make starch due to a mutation in the plastidic PGM have elevated transcript for GPT2 (Figure 1). The effect of mutations in genes required for starch synthesis on GPT2 expression has been seen before in mutants in PGI1, PGM1, and AGPase (Bläsing et al., 2005; Kunz et al., 2010). A mutant with a T-DNA insert in the gene for the glucan water dikinase enzyme, the sex1-81 mutant, can make starch but cannot use it (Ritte et al., 2002). An increase in GPT2 transcript is also seen in this mutant. The starch phosphorylase, phs1-1, mutant does not have elevated GPT2 transcript (Figure 1). Because of this the increase in GPT2 transcript in mutants unable to make or mobilize starch is probably not caused by a depletion of hexose phosphates at night. Additionally, it has been shown that GPT2 transcript in starchless mutants is repressed in the dark (Kunz et al., 2010).
The increase in GPT2 transcript in the starchless or starch excess mutants could be the result of increased triose phosphates in the cytosol that are not being partitioned into starch. It has been suggested that in starchless mutants, GPT2 may serve to export G6P from the chloroplast (Kunz et al., 2010). In a wild type plant, export of G6P would be unlikely due to the large concentration gradient of G6P measured between the cytosol and chloroplast (Gerhardt et al., 1987; Weise et al., 2006; Szecowka et al., 2013). The concentration difference of G6P between the chloroplast and cytosol in starchless mutant or starch excess mutants is unknown. GPT2 can transport triose phosphates as well as or better than hexose phosphates (Kammerer et al., 1998; Eicks et al., 2002) leaving open the possibility that GPT2 simply serves as an additional export mechanism for triose phosphates in the starchless background.
GPT2 Expression Allows a Bypass of Calvin-Benson Cycle FBPase
When the Calvin-Benson cycle enzyme fructose-1,6-bisphosphatase (FBPase) is missing, GPT2 transcript amounts are increased (Figure 1). The cytosolic FBPase could be used, bypassing the stromal FBPase when GPT2 is present. This comes at a cost of growth and increased cyclic electron transport (Livingston et al., 2010). The chloroplast PGI favors the reaction from F6P to G6P (Preiser et al., 2018). Therefore, GPT2 expression could result in the chloroplastic G6P concentration being higher than normal. This G6P could be acted on by G6PDH and enter the G6P shunt, detailed above. Any increase in the G6P shunt would result in the need for extra ATP which could be provided by cyclic electron transport. A similar scenario has been seen in Arabidopsis plants in which the plastidic triose phosphate isomerase is inhibited by high concentrations of 2-phosphoglycolate. These plants also have elevated expression of GPT2 and have higher cyclic electron transport (Li et al., 2019). Plants lacking the stromal FBP aldolase have higher cyclic electron flow consistent with this hypothesis (Gotoh et al., 2010) although it is not known if they have increased expression of GPT2. In another study using a chloroplast FBPase mutant, elevated GPT2 transcript was seen but only in the roots (Soto-Suárez et al., 2016). The reasons for the spatial difference in GPT2 expression observed between our study and that one are unclear. Another possible explanation for the survival of the hcef1 mutant is a novel redox insensitive plastid FBPase that was found in strawberry but appears to have an ortholog in Arabidopsis (Serrato et al., 2009). This use of an alternative FBPase does not explain the increased GPT2 transcript and high cyclic ETR seen in the hcef1 mutant.
Another possible pathway for carbon exit and reentry into the chloroplast is the oxidative pentose phosphate pathway in the cytosol. It has been shown that the cytosolic G6PDH in potato is sensitive to the sugar status of the cell and is transcriptionally regulated (Hauschild and von Schaewen, 2003). Using this pathway, carbon could reenter the chloroplast though the xylulose-5-phosphate transporter (XPT). If this pathway is used there is no evidence for transcriptional regulation of it. The transcript amount for XPT was unchanged in the wild type, gpt2-1 or phs1-1 mutants (Supplementary Figure S4). Further, no change in transcript was seen in the RNA-Seq data for XPT or any enzyme, including the three G6PDHs in the cytosolic pentose phosphate pathway (data not shown).
Only an Increase in Calvin-Benson Cycle Activity Caused GPT2 Transcript to Increase
Increasing CO2 has been shown to increase the expression of a homologous gene to GPT2 in soybean in a Free Atmosphere CO2 Enrichment (FACE) experiment (Leakey et al., 2009). In our experiment, CO2 was increased under a PFD of 120 μmol m–2 s–1 rather than the higher PFD of the FACE site field conditions. No change in GPT2 expression was observed (Figure 2C and Supplementary Figure S1C). Under these conditions the assimilation rate and electron transport rate increased only modestly (Supplementary Figure S3). This most likely did not result in a large change in cytosolic triose phosphate or sucrose concentration or redox status that appears likely to be the drivers of GPT2 expression.
Conclusion
Glucose-6-phosphate/phosphate translocator 2 is at the confluence of metabolic adjustment to sudden increases in carbon assimilation. While normally not expressed, GPT2 transcript rapidly increases when the rate of photosynthesis increases. The time course of RNA-Seq results after an increase in photosynthesis provides valuable insights into the metabolic tradeoffs plants make. When photosynthesis increases, GPT2 may act as a safety-valve, shunting sugar phosphates away from the NADPH-producing non-phosphorylating GAPDH. At the same time GPT2 can also shunt extra carbon toward starch synthesis, bypassing the regulatory stromal PGI. Connecting the cytosolic and stromal G6P pools comes at a cost. The increase in G6P in the chloroplast may activate the stromal G6PDH leading to loss of ATP and loss of carbon, when 6-phosphogluconate dehydrogenase in the G6P shunt releases CO2. However, the reduction in expression of genes related to sucrose synthesis is puzzling.
Data Availability
The datasets generated for this study can be found in Gene Expression Omnibus. The raw and processed data were deposited in the Gene Expression Omnibus (Edgar et al., 2002) and are accessible through the GEO Series accession number GSE132626 (http://www.ncbi.nlm.nih.gov/geo/query/acc.cgi?acc=GSE132626) (Raw RNA-Seq data is being submitted to the NCBI, and accession numbers will be added during the revision process).
Author Contributions
SW and TS designed the experiments. SW carried out the bulk of the research. KC and TL carried out the RNA-Seq and the initial analysis of RNA-Seq data. AP, HK, and CP-P worked out some of the qPCRs and analyzed the results. SW wrote the manuscript. TS edited the manuscript.
Funding
This work was supported by the United States Department of Energy Grant DE-FG02-91ER2002 and partially supported by Michigan AgBioResearch to TS. AP was partially supported by a fellowship from Michigan State University under the Training Program in Plant Biotechnology for Health and Sustainability (T32-GM110523).
Conflict of Interest Statement
The authors declare that the research was conducted in the absence of any commercial or financial relationships that could be construed as a potential conflict of interest.
Acknowledgments
We thank Dr. Ching Man (Jennifer) Wai for many thoughtful discussions about hexose phosphate transport and RNA-Seq data, Dr. David Kramer for many thoughtful insights and discussion, and Dr. Mio Sato-Cruz for help with hcef1, and the two reviewers for pointing out the potential for sucrose signaling in GPT2 expression.
Supplementary Material
The Supplementary Material for this article can be found online at: https://www.frontiersin.org/articles/10.3389/fpls.2019.00827/full#supplementary-material
Footnotes
- ^ https://rtsf.natsci.msu.edu/genomics/
- ^ https://www.bioinformatics.babraham.ac.uk/projects/fastqc/
- ^ https://software.broadinstitute.org/morpheus
- ^ http://www.ncbi.nlm.nih.gov/geo/query/acc.cgi?acc=GSEXXXXX
- ^ https://agris-knowledgebase.org/
References
Athanasiou, K., Dyson, B. C., Webster, R. E., and Johnson, G. N. (2010). Dynamic acclimation of photosynthesis increases plant fitness in changing environments. Plant Physiol. 152, 366–373. doi: 10.1104/pp.109.149351
Berardini, T. Z., Reiser, L., Li, D. H., Mezheritsky, Y., Muller, R., Strait, E., et al. (2015). The Arabidopsis information resource: making and mining the “gold standard” annotated reference plant genome. Genesis 53, 474–485. doi: 10.1002/dvg.22877
Berger, B., Stracke, R., Yatusevich, R., Weisshaar, B., Flügge, U.-I., and Gigolashvili, T. (2007). A simplified method for the analysis of transcription factor–promoter interactions that allows high-throughput data generation. Plant J. 50, 911–916. doi: 10.1111/j.1365-313X.2007.03088.x
Bläsing, O. E., Gibon, Y., Günther, M., Höhne, M., Morcuende, R., Osuna, D., et al. (2005). Sugars and circadian regulation make major contributions to the global regulation of diurnal gene expression in Arabidopsis. Plant Cell 17, 3257–3281. doi: 10.1105/tpc.105.035261
Bolger, A. M., Lohse, M., and Usadel, B. (2014). Trimmomatic: a flexible trimmer for Illumina sequence data. Bioinformatics. 30, 2114–2120. doi: 10.1093/bioinformatics/btu170
Caspar, T., Lin, T. P., Kakefuda, G., Benbow, L., Preiss, J., and Somerville, C. (1991). Mutants of Arabidopsis with altered regulation of starch degradation. Plant Physiol. 95, 1181–1188. doi: 10.1104/pp.95.4.1181
Cossar, J. D., Rowell, P., and Stewart, W. D. P. (1984). Thioredoxin as a modulator of glucose-6-phosphate dehydrogenase in a N2-fixing cyanobacterium. Microbiology. 130, 991–998. doi: 10.1099/00221287-130-4-991
Dobin, A., Davis, C. A., Schlesinger, F., Drenkow, J., Zaleski, C., Jha, S., et al. (2013). STAR: ultrafast universal RNA-seq aligner. Bioinformatics. 29, 15–21. doi: 10.1093/bioinformatics/bts635
Dyson, B. C., Allwood, J. W., Feil, R., Xu, Y., Miller, M., Bowsher, C. G., et al. (2015). Acclimation of metabolism to light in Arabidopsis thaliana: the glucose 6-phosphate/phosphate translocator GPT2 directs metabolic acclimation. Plant Cell Environ. 38, 1404–1417. doi: 10.1111/pce.12495
Dyson, B. C., Webster, R. E., and Johnson, G. N. (2014). GPT2: a glucose 6-phosphate/phosphate translocator with a novel role in the regulation of sugar signalling during seedling development. Ann. Bot. 113, 643–652. doi: 10.1093/aob/mct298
Edgar, R., Domrachev, M., and Lash, A. E. (2002). Gene Expression Omnibus: NCBI gene expression and hybridization array data repository. Nucleic Acids Res. 30, 207–210. doi: 10.1093/nar/30.1.207
Egli, B., Kolling, K., Kohler, C., Zeeman, S. C., and Streb, S. (2010). Loss of cytosolic phosphoglucomutase compromises gametophyte development in Arabidopsis. Plant Physiol. 154, 1659–1671. doi: 10.1104/pp.110.165027
Eicks, M., Maurino, V., Knappe, S., Flügge, U. I., and Fischer, K. (2002). The plastidic pentose phosphate translocator represents a link between the cytosolic and the plastidic pentose phosphate pathways in plants. Plant Physiol. 128, 512–522. doi: 10.1104/pp.128.2.512
Fischer, K., Kammerer, B., Gutensohn, M., Arbinger, B., Weber, A., Häusler, R. E., et al. (1997). A new class of plastidic phosphate translocators: a putative link between primary and secondary metabolism by the phosphoenolpyruvate/phosphate antiporter. Plant Cell 9, 453–462. doi: 10.1105/tpc.9.3.453
Gerhardt, R., Stitt, M., and Heldt, H. W. (1987). Subcellular metabolite levels in spinach leaves. Regulation of sucrose synthesis during diurnal alterations in photosynthetic partitioning. Plant Physiol 83, 399–407. doi: 10.1104/pp.83.2.399
Gotoh, E., Matsumoto, M., Ogawa, K., Kobayashi, Y., and Tsuyama, M. (2010). A qualitative analysis of the regulation of cyclic electron flow around photosystem I from the post-illumination chlorophyll fluorescence transient in Arabidopsis: a new platform for the in vivo investigation of the chloroplast redox state. Photosynthesis Res. 103, 111–123. doi: 10.1007/s11120-009-9525-0
Habenicht, A. (1997). The non-phosphorylating glyceraldehyde-3-phosphate dehydrogenase: biochemistry, structure, occurrence and evolution. Biol. Chem. 378, 1413–1419. doi: 10.1515/bchm.1997.378.12.1413
Hauschild, R., and von Schaewen, A. (2003). Differential regulation of glucose-6-phosphate dehydrogenase isoenzyme activities in potato. Plant Physiol. 133, 47–62. doi: 10.1104/pp.103.025676
Häusler, R. E., Heinrichs, L., Schmitz, J., and Flügge, U. I. (2014). How sugars might coordinate chloroplast and nuclear gene expression during acclimation to high light intensities. Mol. Plant. 7, 1121–1137. doi: 10.1093/mp/ssu064
Heinrichs, L., Schmitz, J., Flügge, U.-I., and Häusler, R. E. (2012). The mysterious rescue of adg1-1/tpt-2 - an Arabidopsis thaliana double mutant impaired in acclimation to high light – by exogenously supplied sugars. Front. Plant Sci. 3:265. doi: 10.3389/fpls.2012.00265
Hejazi, M., Mahlow, S., and Fettke, J. (2014). The glucan phosphorylation mediated by α-glucan, water dikinase (GWD) is also essential in the light phase for a functional transitory starch turn-over. Plant Signal. Behav. 9:e28892. doi: 10.4161/psb.28892
Hilgers, E. J. A., Schöttler, M. A., Mettler-Altmann, T., Krueger, S., Dörmann, P., Eicks, M., et al. (2018). The combined loss of triose phosphate and xylulose 5-phosphate/phosphate translocators leads to severe growth retardation and impaired photosynthesis in Arabidopsis thaliana tpt/xpt double mutants. Front. Plant Sci. 9:1331. doi: 10.3389/fpls.2018.01331
Hoagland, D. R., and Arnon, D. I. (1938). The water culture method for growing plants without soil. UC Agric. Exp. Sta. Circular 347, 1–39.
Kammerer, B., Fischer, K., Hilpert, B., Schubert, S., Gutensohn, M., Weber, A., et al. (1998). Molecular characterization of a carbon transporter in plastids from heterotrophic tissues: the glucose 6-phosphate/phosphate antiporter. Plant Cell 10, 105–117. doi: 10.1105/tpc.10.1.105
Keeling, P. L., Wood, J. R., Tyson, R. H., and Bridges, I. G. (1988). Starch biosynthesis in developing wheat-grain: evidence against the direct involvement of triose phosphates in the metabolic pathway. Plant Physiol. 87, 311–319. doi: 10.1104/pp.87.2.311
Khandelwal, A., Elvitigala, T., Ghosh, B., and Quatrano, R. S. (2008). Arabidopsis transcriptome reveals control circuits regulating redox homeostasis and the role of an AP2 transcription factor. Plant Physiol. 148, 2050–2058. doi: 10.1104/pp.108.128488
Kiss, J. Z., Wright, J. B., and Caspar, T. (1996). Gravitropism in roots of intermediate-starch mutants of Arabidopsis. Physiol. Plant. 97, 237–244. doi: 10.1034/j.1399-3054.1996.970205.x
Kunz, H. H., Häusler, R. E., Fettke, J., Herbst, K., Niewiadomski, P., Gierth, M., et al. (2010). The role of plastidial glucose-6-phosphate/phosphate translocators in vegetative tissues of Arabidopsis thaliana mutants impaired in starch biosynthesis. Plant Biol. 12, 115–128. doi: 10.1111/j.1438-8677.2010.00349.x
Leakey, A. D. B., Xu, F., Gillespie, K. M., McGrath, J. M., Ainsworth, E. A., and Ort, D. R. (2009). Genomic basis for stimulated respiration by plants growing under elevated carbon dioxide. Proc. Natl Acad. Sci. U.S.A. 106, 3597–3602. doi: 10.1073/pnas.0810955106
Lee, Y., Nishizawa, T., Takemoto, M., Kumazaki, K., Yamashita, K., Hirata, K., et al. (2017). Structure of the triose-phosphate/phosphate translocator reveals the basis of substrate specificity. Nature Plants. 3, 825–832. doi: 10.1038/s41477-017-0022-8
Li, J., Weraduwage, S. M., Peiser, A. L., Tietz, S., Weise, S. E., Strand, D. D., et al. (2019). A cytosolic bypass and G6P shunt in plants lacking peroxisomal hydroxypyruvate reductase. Plant Physiol. 180, 783–792. doi: 10.1104/pp.19.00256
Li, L., and Sheen, J. (2016). Dynamic and diverse sugar signaling. Curr. Opin. Plant Biol. 33, 116–125. doi: 10.1016/j.pbi.2016.06.018
Liao, Y., Smyth, G. K., and Shi, W. (2013). The Subread aligner: fast, accurate and scalable read mapping by seed-and-vote. Nucleic Acids Res. 41:e108. doi: 10.1093/nar/gkt214
Livingston, A. K., Cruz, J. A., Kohzuma, K., Dhingra, A., and Kramer, D. M. (2010). An Arabidopsis mutant with high cyclic electron flow around Photosystem I (hcef) involving the NADPH dehydrogenase complex. Plant Cell 22, 221–233. doi: 10.1105/tpc.109.071084
Lloyd, J. C., and Zakhleniuk, O. V. (2004). Responses of primary and secondary metabolism to sugar accumulation revealed by microarray expression analysis of the Arabidopsis mutant, pho3. J. Exp. Bot. 55, 1221–1230. doi: 10.1093/jxb/erh143
McClain, A. M., and Sharkey, T. D. (2019). Triose phosphate utilization and beyond: from photosynthesis to end product synthesis. J. Exp. Bot. 70, 1755–1766. doi: 10.1093/jxb/erz058
Miller, M. A. E., O’Cualain, R., Selley, J., Knight, D., Karim, M. F., Hubbard, S. J., et al. (2017). Dynamic acclimation to high light in Arabidopsis thaliana involves widespread reengineering of the leaf proteome. Front. Plant Sci. 8:1239. doi: 10.3389/fpls.2017.01239
Nickelsen, J., and Rengstl, B. (2013). Photosystem II assembly: from cyanobacteria to plants. Annu. Rev. Plant Biol. 64, 609–635. doi: 10.1146/annurev-arplant-050312-120124
Niewiadomski, P., Knappe, S., Geimer, S., Fischer, K., Schulz, B., Unte, U. S., et al. (2005). The Arabidopsis plastidic glucose 6-phosphate/phosphate translocator GPT1 is essential for pollen maturation and embryo sac development. Plant Cell 17, 760–775. doi: 10.1105/tpc.104.029124
Preiser, A. L., Banerjee, A., Fisher, N., and Sharkey, T. D. (2018). Supply and consumption of glucose 6-phosphate in the chloroplast stroma. bioRxiv 442434. doi: 10.1101/442434
Preiser, A. L., Fisher, N., Banerjee, A., and Sharkey, T. D. (2019). Plastidic glucose-6-phosphate dehydrogenase is regulated to maintain activity in the light. Biochem. J. 476, 1539–1551. doi: 10.1042/bcj20190234
Quick, W. P., Scheibe, R., and Neuhaus, H. E. (1995). Induction of hexose-phosphate translocator activity in spinach chloroplasts. Plant Physiol. 109, 113–121. doi: 10.1104/pp.109.1.113
Ritte, G., Heydenreich, M., Mahlow, S., Haebel, S., Kotting, O., and Steup, M. (2006). Phosphorylation of C6- and C3-positions of glucosyl residues in starch is catalysed by distinct dikinases. FEBS Lett. 580, 4872–4876. doi: 10.1016/j.febslet.2006.07.085
Ritte, G., Lloyd, J. R., Eckermann, N., Rottmann, A., Kossmann, J., and Steup, M. (2002). The starch-related R1 protein is an α-glucan, water dikinase. Proc. Natl Acad. Sci. U.S.A. 99, 7166–7171. doi: 10.1073/pnas.062053099
Scheibe, R., Geissler, A., and Fickenscher, K. (1989). Chloroplast glucose-6-phosphate dehydrogenase: Km shift upon light modulation and reduction. Arch. Biochem. Biophys. 274, 290–297. doi: 10.1016/0003-9861(89)90441-4
Schleucher, J., Vanderveer, P., Markley, J. L., and Sharkey, T. D. (1999). Intramolecular deuterium distributions reveal disequilibrium of chloroplast phosphoglucose isomerase. Plant Cell Environ. 22, 525–533. doi: 10.1046/j.1365-3040.1999.00440.x
Schmitz, J., Heinrichs, L., Scossa, F., Fernie, A. R., Oelze, M.-L., Dietz, K.-J., et al. (2014). The essential role of sugar metabolism in the acclimation response of Arabidopsis thaliana to high light intensities. J. Exp. Bot. 65, 1619–1636. doi: 10.1093/jxb/eru027
Schneider, A., Hausler, R. E., Kolukisaoglu, U., Kunze, R., van der Graaff, E., Schwacke, R., et al. (2002). An Arabidopsis thaliana knock-out mutant of the chloroplast triose phosphate/phosphate translocator is severely compromised only when starch synthesis, but not starch mobilisation is abolished. Plant J. 32, 685–699. doi: 10.1046/j.1365-313X.2002.01460.x
Serrato, A. J., Yubero-Serrano, E. M., Sandalio, L. M., Munoz-Blanco, J., Chueca, A., Caballero, J. L., et al. (2009). cpFBPaseII, a novel redox-independent chloroplastic isoform of fructose-1,6-bisphosphatase. Plant Cell Environ. 32, 811–827. doi: 10.1111/j.1365-3040.2009.01960.x
Sharkey, T. D., and Vassey, T. L. (1989). Low oxygen inhibition of photosynthesis Is caused by inhibition of starch synthesis. Plant Physiol. 90, 385–387. doi: 10.1104/pp.90.2.385
Sharkey, T. D., and Weise, S. E. (2016). The glucose 6-phosphate shunt around the Calvin-Benson cycle. J. Exp. Bot. 67, 4067–4077. doi: 10.1093/jxb/erv484
Soto-Suárez, M., Serrato, A. J., Rojas-González, J. A., Bautista, R., and Sahrawy, M. (2016). Transcriptomic and proteomic approach to identify differentially expressed genes and proteins in Arabidopsis thaliana mutants lacking chloroplastic 1 and cytosolic FBPases reveals several levels of metabolic regulation. BMC Plant Biol. 16:258. doi: 10.1186/s12870-016-0945-7
Szecowka, M., Heise, R., Tohge, T., Nunes-Nesi, A., Vosloh, D., Huege, J., et al. (2013). Metabolic fluxes in an illuminated Arabidopsis rosette. Plant Cell. 25, 694–714. doi: 10.1105/tpc.112.106989
Trethewey, R. N., and ap Rees, T. (1994). The role of the hexose transporter in the chloroplasts of Arabidopsis thaliana L. Planta 195, 168–174. doi: 10.1007/bf00199675
Tyson, R. H., and ap Rees, T. (1988). Starch synthesis by isolated amyloplasts from wheat endosperm. Planta 175, 33–38. doi: 10.1007/bf00402879
Vogel, M. O., Moore, M., Konig, K., Pecher, P., Alsharafa, K., Lee, J., et al. (2014). Fast retrograde signaling in response to high light involves metabolite export, MITOGEN-ACTIVATED PROTEIN KINASE6, and AP2/ERF transcription factors in Arabidopsis. Plant Cell 26, 1151–1165. doi: 10.1105/tpc.113.121061
Wakao, S., and Benning, C. (2005). Genome-wide analysis of glucose-6-phosphate dehydrogenases in Arabidopsis. Plant J. 41, 243–256. doi: 10.1111/j.1365-313X.2004.02293.x
Weise, S. E., Schrader, S. M., Kleinbeck, K. R., and Sharkey, T. D. (2006). Carbon balance and circadian regulation of hydrolytic and phosphorolytic breakdown of transitory starch. Plant Physiol. 141, 879–886. doi: 10.1104/pp.106.081174
Keywords: glucose 6-phosphate, G6P, GPT2, RRTF1, TPT, GAP, PGA, redox regulation
Citation: Weise SE, Liu T, Childs KL, Preiser AL, Katulski HM, Perrin-Porzondek C and Sharkey TD (2019) Transcriptional Regulation of the Glucose-6-Phosphate/Phosphate Translocator 2 Is Related to Carbon Exchange Across the Chloroplast Envelope. Front. Plant Sci. 10:827. doi: 10.3389/fpls.2019.00827
Received: 15 March 2019; Accepted: 07 June 2019;
Published: 27 June 2019.
Edited by:
Jürgen Soll, Ludwig Maximilian University of Munich, GermanyReviewed by:
Hans-Henning Kunz, Washington State University, United StatesRainer Erich Häusler, University of Cologne, Germany
Anja Schneider, Ludwig Maximilian University of Munich, Germany
Copyright © 2019 Weise, Liu, Childs, Preiser, Katulski, Perrin-Porzondek and Sharkey. This is an open-access article distributed under the terms of the Creative Commons Attribution License (CC BY). The use, distribution or reproduction in other forums is permitted, provided the original author(s) and the copyright owner(s) are credited and that the original publication in this journal is cited, in accordance with accepted academic practice. No use, distribution or reproduction is permitted which does not comply with these terms.
*Correspondence: Thomas D. Sharkey, dHNoYXJrZXlAbXN1LmVkdQ==