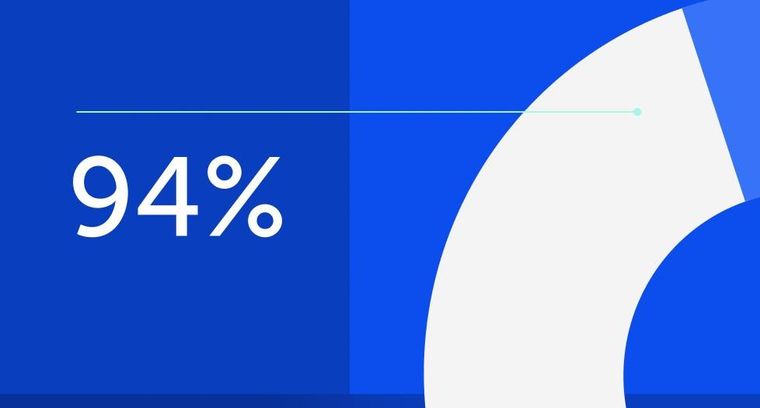
94% of researchers rate our articles as excellent or good
Learn more about the work of our research integrity team to safeguard the quality of each article we publish.
Find out more
ORIGINAL RESEARCH article
Front. Plant Sci., 08 May 2019
Sec. Plant Physiology
Volume 10 - 2019 | https://doi.org/10.3389/fpls.2019.00529
Changes in the environment, specifically rising temperature and increasing atmospheric carbon dioxide concentration [CO2], can alter the growth and physiology of weedy plants. These changes could alter herbicide efficacy, crop-weed interaction, and weed management. The objectives of this research were to quantify the effects of increased atmospheric [CO2] and temperature on absorption, translocation and efficacy of cyhalofop-butyl on multiple-resistant (MR) and susceptible (S) Echinochloa colona genotypes. E. colona, or junglerice, is a troublesome weed in rice and in agronomic and horticultural crops worldwide. Cyhalofop-butyl is a grass herbicide that selectively controls Echinochloa spp. in rice. Maximum 14C-cyhalofop-butyl absorption occurred at 120 h after herbicide treatment (HAT) with >97% of cyhalofop-butyl retained in the treated leaf regardless of [CO2], temperature, or genotype. Neither temperature nor [CO2] affected herbicide absorption into the leaf. The translocation of herbicide was slightly reduced in the MR plants vs. S plants either under elevated [CO2] or high temperature. Although plants grown under high [CO2] or high temperature were taller than those in ambient conditions, neither high [CO2] nor high temperature reduced the herbicide efficacy on susceptible plants. However, herbicide efficacy was reduced on MR plants grown under high [CO2] or high temperature about 50% compared to MR plants at ambient conditions. High [CO2] and high temperature increased the resistance level of MR E. colona to cyhalofop-butyl. To mitigate rapid resistance evolution under a changing climate, weed management practitioners must implement measures to reduce the herbicide selection pressure. These measures include reduction of weed population size through reduction of the soil seedbank, ensuring complete control of current infestations with multiple herbicide modes of action in mixture and in sequence, augmenting herbicides with mechanical control where possible, rotation with weed-competitive crops, use of weed-competitive cultivars, use of weed-suppressive cover crops, and other practices recommended for integrated weed management.
Climatic projections by the Intergovernmental Panel on Climate Change (IPCC) indicate an increase in global mean temperature (2.6–4.8°C) and CO2 atmospheric concentration ([CO2]) (730–1000 μmol⋅mol-1) by the end of the 21st century (Gianessi, 2013; IPCC, 2014; Varanasi et al., 2015). The rapidity of these changes is likely to have consequences on a number of human activities, including agriculture.
Physical environmental changes, such as precipitation and temperature can alter agronomic practices including land preparation, planting, irrigation, and fertilizer application. However, biological interactions, particularly pest population dynamics and severity, may also be affected. Weeds, as an example, can cause 100% crop loss when not controlled. The degree of loss varies by crop, cultivar, weed species, weed infestation level, location, year, and farming practices (Abouziena and Haggag, 2016; Soltani et al., 2016). In corn alone, yield losses due to weeds ranged from 10 to 83% in North America and averaged 51% in Eastern Canada between 2007 and 2013 (Soltani et al., 2016). Rice yield losses from competition with Echinochloa spp. across the globe range from 10 to 79% (Smith, 1974, 1988; Hill et al., 1985; Stauber et al., 1991; Fischer et al., 1997; Chin, 2001). Overall, increasing [CO2] and temperature may alter dominant weed species and increase weed problems (Ziska and Dukes, 2011).
Herbicides are the primary tools used to control weeds and minimize economic losses in crop production. Herbicide use has been increasing globally in traditionally low-herbicide-using countries, including China, India and parts of Africa where hand labor is becoming increasingly scarce and expensive (Gianessi, 2013). However, changes in [CO2] and temperature can potentially alter herbicide efficacy (Varanasi et al., 2015; Korres et al., 2016). For example, stimulation of weed growth by elevated [CO2] could reduce time in the seedling stage when weeds are most sensitive to herbicides. Alternatively, [CO2]-induced changes in stomatal conductance could also reduce herbicide absorption. Increased temperatures, or temperature extremes, could increase herbicide efficacy by accelerating absorption and translocation of foliar herbicides; but could also induce rapid metabolism, which reduces herbicide efficacy in target plants (Johnson and Young, 2002). In addition, increased temperature and [CO2] can change the leaf surface characteristics by increasing leaf thickness, or changing the viscosity of the cuticle wax, with subsequent reductions in herbicide absorption (Ziska and Bunce, 2006).
Among the negative consequences of increasing temperature and [CO2] could be the acceleration of weed resistance evolution to herbicides, specifically, non-target-site resistance. Resistance to herbicides is an example of evolved avoidance mechanism to abiotic stressors. Stress avoidance mechanisms in plants are oftentimes similar, or common, among various environmental stress factors. Non-target-site-based resistance (NTSR) mechanisms, which include increased herbicide metabolism, herbicide sequestration, reduced absorption and translocation increased protection from strong oxidants, and overproduction of herbicide target (Powles and Yu, 2010; Délye, 2013), are strongly influenced by changes in climate (see Ramesh et al., 2017). Non-target site resistance can trigger multiple resistance to herbicides or endow resistance to herbicides not yet used on the weed population. Cases of multiple resistance and NTSR are increasing (Roma-Burgos et al., 2019). For example, we observe increasing cases of NTSR to acetyl-CoA carboxylase (ACCase) inhibitors, in addition to the already widespread target-site resistance to these herbicides (Heap, 2019). Strong NTSR to ACCase inhibitors in grasses has been attributed primarily to herbicide detoxification aided by increased production of monooxygenases (cytochrome P450s), which facilitate phase I detoxification reactions and increased activity of different classes of glutathione-S-transferase (GST) enzymes, which facilitate phase II detoxification (Powles and Yu, 2010; Cummins et al., 2013; Délye, 2013).
Junglerice [Echinochloa colona (L.) Link], together with barnyardgrass [E. crus-galli (L.) Beauv.], is very difficult to control in rice as it mimics rice at the vegetative stage (Peerzada et al., 2016). Cyhalofop-butyl and fenoxaprop-P-ethyl are ACCase inhibitors currently used in lowland rice fields for postemergence control of Echinochloa species and other grass weeds (Scott et al., 2018). These herbicides are absorbed by the leaves and are translocated primarily via the phloem to the meristematic tissues where the herbicide inhibits lipid synthesis. The depletion of lipids stops the growth of cells, organs, and tissues, leading to plant death. The repeated use of grass herbicides (as with most other herbicides) has resulted in the evolution of resistance to ACCase inhibitors among some Echinochloa populations (Huan et al., 2013; Heap, 2014; Rouse et al., 2018a). Resistance to ACCase inhibitors are almost equally driven by mutations at various loci involved in herbicide binding at the target site and by various NTSR mechanisms (Délye et al., 2011; Kaundun, 2014). Selective grass herbicides (i.e., diclofop-methyl, tralkoxydim, pinoxaden) are inactivated in plants through hydroxylation, by cytochrome P450s in phase I detoxification reactions (Wenger et al., 2012) and subsequent conjugation by GTs or GSTs in phase II reactions (Milner et al., 2001; Brazier et al., 2002). Thus, metabolic resistance is a common NTSR mechanism, along with some cases of reduced absorption or translocation (De Prado et al., 2005).
Whether enhanced [CO2] and/or temperature can alter the efficacy of selective grass herbicides, and whether there is differential response between susceptible and resistant grass genotypes to these factors are not known. The objective of the current research was to quantify the effects of increased atmospheric [CO2] and temperature on the absorption, translocation, and efficacy of cyhalofop-butyl on multiple-resistant and susceptible E. colona genotypes under simulated climate change conditions.
Experiments were conducted in 2016 and 2017 at the Altheimer Laboratory in the Department of Crop, Soil and Environmental Sciences, University of Arkansas, Fayetteville, United States.
Five seedlings of E. colona were grown in 0.2 L pots filled with soil (Dardanelle silt loam: silty, mixed, active, thermic Typic Udifluvents) in four replications. The pots were placed in trays and kept in a growth chamber maintained at 23/35°C night/day temperature. The trays were filled with water, allowing irrigation by capillary movement. Three days before herbicide treatment (HAT), nitrogen fertilizer was applied at the recommended field use rate for irrigated rice. To determine the effect of CO2 levels on plant growth and response to herbicide, three factors were tested as a factorial treatment structure. Factor A was CO2 concentration: ambient (a[CO2]) at 400 ± 50 μmol⋅mol-1 and elevated (e[CO2]) at 700 ± 50 μmol⋅mol-1. These values were chosen based on the predicted [CO2] at the end of this century (IPCC, 2014). To control [CO2] levels, a system was designed and built, and installed in two plant growth chambers. This system centralizes information in a single processor, thus facilitating control of [CO2] and recording of data. The processor was programmed to collect sensor readings every 10 s. This interval was determined from previous tests to achieve the best stability of the desired [CO2]. Data from each CO2 sensor were stored in the processor internal memory for subsequent calculation of the amount of CO2 to add into the growth chambers intermittently. The CO2 levels were maintained by automated comparison of the desired concentration programmed into the processor with the CO2 sensor reading. To satisfy the prescribed conditions, the quantity of CO2 to be injected into the chamber was calculated using a mathematical model established in previous experiments (unpublished data).
Factor B was genotype of E. colona, characterized in previous experiment as susceptible (S) and multiple-resistant (MR) to propanil and quinclorac with low level of resistance to cyhalofop (Rouse and Burgos, 2017; Roma-Burgos et al., 2018). This population was collected from East Arkansas, in a field historically planted with rice and occasionally rotated with soybean. Cyhalofop was rarely used in this field. Factor C was cyhalofop-butyl treatment (with, or without). The experimental units (pots) were arranged in a completely randomized design. At the three-leaf stage, the plants were sprayed with commercial formulation of cyhalofop-butyl (Clincher®, Dow AgroSciences, United States) at 1.09 L⋅ha-1 in a laboratory spray chamber equipped with flat-fan nozzles (TeeJet 80015; Spraying Systems Co., Wheaton, IL, United States) at 290 kPa pressure, delivering 183 L⋅ha-1. Crop oil concentrate (Agri-Dex; Helena Chemical Company, Collierville, TN, United States) was added to the herbicide mixture at a final concentration of 1% v/v. Visible injury, plant height, and shoot biomass were evaluated 1 week after HAT. The experiment was repeated.
The same Echinochloa genotypes were planted and cultured as described above. Plants were harvested at 6, 12, 24, 72, and 120 h after HAT. Commercial (or “cold”) cyhalofop-butyl was applied to seedlings as described above. Immediately after the cold HAT, the plants were moved to the radioisotope laboratory.
A stock solution of technical-grade 14C-labeled cyhalofop-butyl (specific activity = 32.8 mCi/mM) was prepared in acetonitrile (500 μL) and water (2:1 by vol.) solution. An aliquot of the non-radiolabeled cyhalofop-butyl (Clincher®, 1.09 L⋅ha-1, Dow AgroSciences, United States) spray solution was spiked with an aliquot of 14C-labeled cyhalofop-butyl stock solution to prepare spotting solutions with a specific activity of 0.25 kBq⋅μL-1. Four 1 μL droplets of this spotting solution were applied on the adaxial surface of the second fully expanded leaf, for a total of 1 kBq⋅μL-1 per plant. Once the droplets had dried, the pots were transferred to growth chambers set for each CO2 concentration.
The treated leaves were harvested at designated times and placed in 20 mL glass vials containing 3 mL methanol. Each vial was shaken gently by hand for 10 s to remove the non-absorbed herbicide. The rinsate was mixed with 10 mL of scintillation cocktail (Ultima GoldTM; PerkinElmer Inc., Waltham, MA, United States) and the radioactivity was quantified using a liquid scintillation spectrometer (LSS) (Packard Tri-Carb 2100TR liquid scintillation spectrometer; Packard Instrument Corp., Downers Grove, IL, United States). Absorption of 14C-cyhalofop-butyl was calculated by subtracting the amount of radioactivity in the leaf rinsate from the total applied. At each harvest time, the plants were fractioned into treated leaf (TL), leaf above TL, leaf below TL, and roots. For statistical analysis, factors A and B were [CO2] and genotype, respectively. Factor C was harvest time and factor D was plant sections. After sectioning, the plant tissues were dried at 60°C for 48 h and then oxidized (OX500TM; R. J. Harvey Instrument Corp., Tappan, NY, United States). The 14CO2 evolved during tissue combustion was trapped in a vial containing 15 mL of scintillation cocktail (Carbon-14 Cocktail; R. J. Harvey Instrument Corp.) and quantified using LSS, as mentioned above. The experiment was repeated.
At each harvest time, one whole plant was used to visualize herbicide translocation using autoradiography. The roots were rinsed with tap water and non-absorbed 14C-labeled cyhalofop-butyl was removed by rinsing the treated leaf with methanol as described above. The plants were pressed immediately with absorptive paper, and leaves were spread carefully to prevent the treated leaf from contacting other plant tissues. The plants were then stored at -55°C for 48 h, exposed to a phosphorscreen for 48 h and then scanned in a Storm 820 PhosphorImagerTM (Molecular Dynamics Inc., Sunnyvale, CA, United States).
The same methodology described in Experiment 1 was used, except that Factor A was temperature: 23/35°C (night/day) and 26/38°C, simulating the current actual (aT) and predicted average temperature (eT) at the end of this century, respectively (Varanasi et al., 2015). Visible injury, plant height, and shoot biomass were evaluated 1 week after HAT. The experiment was repeated.
The treatment effects were the same in both runs of the experiment; therefore, the two runs were combined in the analysis. Data were tested for normality and homogeneity of variance, transformed when needed, and subjected to three-way analysis of variance. The interaction among treatment factors were tested. When significant differences were detected, Tukey’s test (P < 0.05) was performed to separate treatment means. The F-test (P < 0.05) was used to compare two means. Statistical analysis was performed using R Software (Foundation for Statistical Computing, Vienna, Austria). Figures were prepared using Sigmaplot v. 12.5 for Windows (Systat Software, Inc., San Jose, CA, United States).
Three-way interactions among factors wasere not significant in ANOVA, for this reason; therefore, the results were presented separately in two-way factor interactions are presented.
[CO2] differentially affected the extent of E. colona injury between MR and S genotypes from cyhalofop-butyl treatment (Figure 1). At ambient [CO2], the E. colona genotypes responded, similarly, to cyhalofop-butyl; the S genotype was killed and the MR genotype was nearly killed 7 days after treatment. Under elevated [CO2], cyhalofop-butyl did not kill the S plants 100%, but the reduction in herbicide activity was not significant. Conversely, the herbicide efficacy on MR plants declined significantly from almost 100% at a[CO2] to <80% at e[CO2]. This level of weed control would be considered a failure under field conditions by crop producers as 90% control is the minimum level deemed excellent (Scott et al., 2018).
Figure 1. Injury of Echinochloa colona genotypes, 7 days after treatment, grouped by CO2 concentration. MR, multiple-resistant to propanil and quinclorac with low resistance to cyhalofop; S, susceptible; a[CO2] = 400 ± 50 μmol⋅mol-1; e[CO2] = 700 ± 50 μmol⋅mol-1. F-test (P < 0.05); Fayetteville, AR, United States.
The basis for increased resistance of MR-E. colona to cyhalofop-butyl under e[CO2] is not fully understood. Thus far, we know that this MR population is highly resistant to quinclorac and propanil via increased herbicide detoxification, concomitant with constitutive upregulation of activity in carbon fixation, fatty acid synthesis, and trehalose biosynthesis pathways (Rouse and Burgos, 2017; Roma-Burgos et al., 2018; Rouse et al., 2018b). Transcriptome data and subsequent validation of relative expression of candidate genes indicate a joint action of cytochrome P450s, UGT glycosyltransferase, GSTs, and key genes in the trehalose biosynthesis pathway to effect not only herbicide detoxification, but also increased protection from abiotic stress (Roma-Burgos et al., 2018; Rouse et al., 2018b). This population has not been selected intensively with ACCase herbicides in the field; yet the lipid synthesis pathway is constitutively upregulated. Thus, we hypothesize that the low-level resistance to cyhalofop-butyl is a latent effect of mechanisms that endow high levels of resistance to quinclorac and propanil. With the constitutive upregulation of carbon assimilation, lipid biosynthesis, and abiotic-stress-protection genes, the potential exists for elevated tolerance to other herbicides such as ACCase inhibitors. It has been reported recently that resistance to ACCase inhibitors in large crabgrass (Digitaria sanguinalis L.) is due to overexpression of ACCase (Laforest et al., 2017). Hence, we propose that e[CO2] provides more carbon resources to fuel the upregulated carbon assimilation machinery in MR-E. colona, producing more ingredients for fatty acid synthesis, ACCase enzyme, or protection proteins, which could dilute the effect of fatty-acid-synthesis-inhibitors. Late watergrass [E. phyllopogon (Stapf) Koso-Pol.], a close relative and also a major weed problem in rice, has evolved resistance to cyhalofop-butyl via increased metabolism of cyhalofop acid, coupled with reduced herbicide absorption through the cuticle (Ruiz-Santaella et al., 2006a).
Other studies have shown reduced herbicide efficacy under e[CO2] for C3 and C4 weedy species (Ziska and Teasdale, 2000; Ziska et al., 2004; Manea et al., 2011). For example, glyphosate is less effective on Eragrostis curvula (Schrad.) Nees and Paspalum dilatatum Poir under e[CO2] (Manea et al., 2011). These studies suggested that the reduction in glyphosate efficacy could be related to [CO2]-induced increases in plant size (biomass and leaf area), resulting in herbicide dilution. Such growth stimulation, however, contradicts the general idea that C4 plants per se, do not benefit from rising [CO2]. While additional information on this point is desired, there are previous reports that C4 weeds could respond more to elevated [CO2] than C4 crops (Ziska and Bunce, 1997).
Increased [CO2] can induce morphological, physiological, and anatomical changes in plants that could affect herbicide absorption and translocation rate (Manea et al., 2011). Previous research indicated that plants (C3 and C4) grown under elevated [CO2] have thicker cuticle and increased leaf pubescence (Ainsworth and Long, 2005). These traits could reduce herbicide entry into plant leaves. Besides increasing leaf thickness, e[CO2] can also induce partial stomatal closure, which may reduce the absorption and efficacy of herbicides (Jackson et al., 2011). Stomatal closure results in reduced stomatal conductance, which in turn results in reduced transpiration flow, eventually resulting in reduced herbicide absorption, especially those applied on the soil (Bunce and Ziska, 2000; Ziska and McClung, 2008). However, in our experiment, the absorption of 14C-cyhalofop-butyl into leaves of Echinochloa seedlings did not decline under e[CO2]. The interaction effects of [CO2], genotype, and sampling time on absorption of 14C-cyhalofop-butyl were not significant (P > 0.05). Herbicide absorption was almost 45% at 6 HAT and reached a maximum of 65% at 120 HAT (Figure 2), averaged across genotypes and [CO2]. Herbicide absorption did not differ between MR- and S genotypes and was not affected by [CO2] (Supplemental Figure 1). Differential tolerance to cyhalop-butyl in grasses is attributed partly to differential absorption into leaves owing to differences in cuticular traits. Rice, for example, has an even coating of epicuticular waxes, which limits the absorption of cyhalofop-butyl to only 30% in 24 h after treatment vs. 77% in the susceptible E. oryzoides, which has an uneven coating of waxes (Ruiz-Santaella et al., 2006b). Anatomical modification of leaf surfaces toward reduction of herbicide absorption requires long-term evolutionary adaptation; such change cannot be expected in this study.
Figure 2. Percentage of 14C-cyhalofop-butyl absorbed, of the total applied on Echinochloa colona, at 6, 12, 24, 72, and 120 h (95% confidence intervals). Commercial formulation sprayed at V3 stage with 1% crop oil concentrate and spotted with 1 kBq⋅μL-1; Fayetteville, AR, United States.
Aryloxyphenoxypropanoate herbicides, such as cyhalofop-butyl, are formulated as esters to facilitate absorption into leaves, but are converted to their acid (herbicidal) form by esterases such as what happens with cyhalofop-butyl in rice (Oryza sativa L.) and in its major grass weed, Echinochloa spp. (Ruiz-Santaella et al., 2006b). The herbicide is translocated in its acid form in the plant. Therefore, translocation refers to cyhalofop acid. The interaction effect between [CO2] and leaf tissue on detected radioactivity was significant (Table 1). This indicated differential herbicide distribution in the plant between a[CO2] and e[CO2], averaged across genotypes. The translocation of cyhalofop within the plant also differed between genotypes, averaged across [CO2]. Most of the absorbed herbicide (>98%) remained in the treated leaf, regardless of the genotype and [CO2] (Table 1 and Figure 3). Slightly more herbicide moved out of the treated leaf at e[CO2] than a[CO2], but these minute amounts could not be detected by phosphorimaging. The small amount that was translocated was distributed slightly differently under a[CO2] vs. e[CO2]. These small differences in herbicide translocation, though significant in some plant sections, did not matter as the efficacy of cyhalofop declined at e[CO2]. The slight increase in herbicide translocation under e[CO2] may be associated with increased translocation of nutrients and increased metabolic activity because of higher available CO2. Cyhalofop is known to have limited mobility within the plant. In E. phyllopogon, 98% of applied 14C-cyhalofop remained in the treated leaves at 12–24 HAT, regardless of the resistance phenotype (Ruiz-Santaella et al., 2006a).
Table 1. 14C-cyhalofop-butyl translocation to various plant tissues, under two CO2 concentrations ([CO2]) and two Echinochloa colona genotypes, 120 h after treatment.
Figure 3. Autoradiography of 14C-cyhalofop-butyl in MR and S Echinochloa colona genotypes, cultivated in a[CO2] and e[CO2] at 120 HAT. a[CO2] = 400 ± 50 μmol⋅mol-1; e[CO2] = 700 ± 50 μmol⋅mol-1; MR, multiple-resistant to propanil and quinclorac with low resistance to cyhalofop-butyl; S, susceptibl;. Fayetteville, AR, United States.
Averaged across [CO2] levels, the MR genotype retained more 14C-cyhalofop in the treated leaf than the S genotype (Table 1). Again, although significant, these numbers are very close (98.9% vs. 98.6%). This showed slightly more translocation in the S genotype than in the MR genotype. This also indicates that the mechanism lending low-level resistance to cyhalofop partly involves minimal reduction in translocation, at least under a[CO2] condition. This minor difference in cyhalofop translocation between genotypes does not explain the large reduction in cyhalofop efficacy on the MR genotype under e[CO2].
Under ambient conditions, other researchers (Fernández et al., 2016) reported no differences in the translocation of 14C-diclofop, another selective grass herbicide in the same chemical family as cyhalofop, among herbicide-resistant and -susceptible Cynosurus echinatus. The translocation of cyhalofop also did not differ among resistant and susceptible E. phyllopogon (Ruiz-Santaella et al., 2006a). Such cases indicate the involvement of other resistance mechanisms, perhaps increased metabolism of the herbicide or mutation at the ACCase binding site.
As with [CO2], the interaction effect of temperature and E. colona genotypes on cyhalofop-butyl efficacy was significant (Figure 4). Under ambient temperature, the activity of cyhalofop-butyl on S plants was numerically higher (about 90%) than on MR plants (about 80%) at 7 DAT. At elevated temperature, the efficacy of cyhalofop-butyl on the S genotype remained high, but the efficacy on the MR genotype declined significantly to about 50%. Temperature influences several processes related to herbicide efficacy. These include the rate of water absorption and transpiration, the rate of leaf development, cuticle thickness, and stomatal number and aperture (Bailey, 2004; Rodenburg et al., 2011). The ability of plants to tolerate (minimize or sustain damage and recover) the negative effects of abiotic stress, including herbicides, improves with increase in temperature up to the optimum point for growth and development. Thus, we observe, for example, increased injury in rice from bispyribac-sodium herbicide applied during a cold period (Martini et al., 2015). Even evolved resistance to herbicides, such as that of johnsongrass (Sorghum halepense L.) and rigid ryegrass (Lolium rigidum L.) to glyphosate could be overcome under cold temperature (Vila-Aiub et al., 2013). This paradigm does not apply to all weed-herbicide-temperature interactions, of course. Plant response is modified by the herbicide mode of action and other mitigating factors such as the extent to which low temperature impedes herbicide absorption and translocation, or herbicide activation in the plant (as with proherbicides), resulting in reduced efficacy at low temperatures.
Figure 4. Injury in different Echinochloa colona genotypes, 7 d after treatment, grouped by temperature levels. MR, multiple-resistant to propanil and quinclorac with low resistance to cyhalofop; S, susceptible; aT = 23/35°C (night/day); eT = 26/38°C (night/day). F-test (P < 0.05); Fayetteville, AR, United States.
Reduced efficacy at high temperature generally may be due to increased metabolism of, or increased protection from, the herbicide as a consequence of maximal physiological conditions at high temperature. There is support for this hypothesis such as the findings of Godar et al. (2015) where the activity of mesotrione on Palmer amaranth declined when the temperature increased from 25 to 40°C. The same was reported by Matzrafi et al. (2016) on the reduction in activity of pinoxaden (an ACCase inhibitor) on Brachypodium hybridum and other grasses under high temperature. These researchers found significantly higher levels of the glucose-conjugated metabolite of pinoxaden herbicide in B. hybridum under high temperature compared to low temperature. In the same study, the authors also observed that pinoxaden-resistant B. hybridum plants metabolized pinoxaden faster at elevated temperature.
In the case of E. colona in the current study, high temperature increased resistance to cyhalofop-butyl in the MR genotype by a significant margin, whereas herbicide efficacy remained similar in the S genotype. This data is highly informative for achieving sustainable weed management in a warming climate. How is increased resistance possible under high temperature? The MR genotype shows constitutive upregulation of key genes implicated in alleviation of abiotic stress and detoxification of xenobiotics (Roma-Burgos et al., 2018; Rouse et al., 2018b). The MR genotype also shows hypersensitive response to propanil, which allows quick death of affected cells and faster recovery (i.e., regrowth) when coupled with other constitutive coping mechanisms (Roma-Burgos et al., 2018). All together, we propose that these mechanisms are enhanced under high temperature, which is favorable for Echinochloa growth, resulting in increased resistance to cyhalofop-butyl. Follow-up research is needed on the specific effect of rising temperature on these metabolic interactions and the consequences on weed response to herbicides.
Absorption of 14C-cyhalofop-butyl in E. colona under different temperatures (Figure 5) followed the same pattern as obtained with different [CO2] levels. The interaction effects of temperature, genotype, and sampling time on absorption of 14C-cyhalofop-butyl were not significant (P < 0.05). Herbicide absorption approached a plateau at 120 HAT with maximum absorption at 75% averaged across genotypes and temperatures.
Figure 5. 14C-cyhalofop-butyl absorption by Echinochloa colona at different times after application (95% confidence intervals). Commercial formulation was sprayed at V3 stage with 1% crop oil concentrate and the leaf was spotted with 1 kBq⋅μL-1; Fayetteville, AR, United States.
The interaction effect of temperature and genotype on the translocation of 14C-cyhalofop-butyl was not significant (Table 2). A large proportion (at least 97.86%) of absorbed 14C-cyhalofop-butyl remained in the treated leaf of both genotypes (Table 2 and Figure 6). More herbicide remained in the treated leaf at elevated temperature (98.6%) averaged across genotypes, indicating reduced translocation. Although small, this difference was significant. This minimal reduction in translocation might have contributed to the reduced herbicide efficacy at high temperature across genotypes, but does not explain the large reduction in herbicide efficacy on the MR genotype compared to that of the S genotype. Possible reasons for increased resistance to cyhalofop-butyl under high temperature are discussed previously. In related research, Godar et al. (2015) also observed reduced translocation of mesotrione in Palmer amaranth (Amaranthus palmeri S. Watson) grown at 40°C compared to plants grown at 25°C. Optimal temperature is expected to promote optimal translocation of herbicide, resulting in higher herbicide efficacy. However, the effect of temperature on the metabolic activity of the plant overrides increased herbicide translocation, as herbicide detoxification also increases at high temperature (Martini et al., 2015; Matzrafi et al., 2016).
Table 2. 14C-cyhalofop-butyl translocation in two Echinochloa colona genotypes, multiple-resistant (MR) and susceptible (S), averaged over ambient (aT), and elevated (eT) temperature, 120 h after treatment (HAT).
Figure 6. Autoradiography of 14C-cyhalofop-butyl in MR and S Echinochloa colona genotypes, grown in aT and eT, at 120 HAT. MR, multiple-resistant to propanil and quinclorac with low resistance to cyhalofop-butyl; S, susceptible; T = 23/35 °C (night/day); eT = 26/38 °C (night/day); Fayetteville, AR, United States.
The tallest plants were those grown in elevated CO2 concentration or high temperature (Figure 7). The MR and S plants did not differ in height within each of these conditions. Averaged across genotypes, taller plants under climate-change conditions did produce numerically more biomass than the shorter plants under ambient conditions, but could not be separated statistically in this regard (Figure 8). This just reflects the fact that in grasses, culm or leaf elongation does not necessarily equate to more biomass, up to a point. The height difference of the main culm between environmental conditions was only within 20 cm. Unless this was accompanied with significantly more tillering (which it was not), the corresponding increase in biomass would be of lesser magnitude as our data portrayed. Temperature and [CO2] are major determinants of plant growth and development. Concurrent increases in temperature and [CO2] are expected to have significant effects on plants, but some interaction effects are turning out to be contrary to expectations. The herbicide degradation rate or metabolism could be faster in big plants than in small ones, and big plants need higher doses for the herbicide to reach all target sites at effective levels. Thus, it takes more herbicide to kill big plants than small ones as indicated by formulation labels for foliar herbicides specifying small seedlings, or recommending high rates on bigger plants and even more for non-selective desiccation of fully grown vegetation. It is apparent that climate changes will influence (directly or indirectly) how weeds are managed.
Figure 7. Plant height, 21 days after planting, grouped by environmental conditions. Means followed by the same letter do not differ statistically based on Tukey’s test (P < 0.05). a[CO2] = 400 ± 50 μmol⋅mol-1; e[CO2] = 700 ± 50 μmol⋅mol-1; aT = 23/35°C (night/day); eT = 26/38°C (night/day). F-test (P < 0.05); Fayetteville, AR, United States.
Figure 8. Shoot biomass (%), relative to the non-treated check, 21 days after planting, grouped by environmental conditions. Means followed by the same letter do not differ statistically based on Tukey’s test (P < 0.05). a[CO2] = 400 ± 50 μmol⋅mol-1; e[CO2] = 700 ± 50 μmol⋅mol-1; aT = 23/35 °C (night/day); eT = 26/38°C (night/day). F-test (P < 0.05); Fayetteville, AR, United States.
The shoot biomass did not differ between E. colona genotypes nor between environmental conditions (data not shown). The plants were small when harvested (3 weeks after planting), only 7 days after HAT. The observed differences in levels of injury were not reflected on the amounts of biomass.
On a side note, E. colona germinated faster, regardless of genotype, under high temperature (Supplemental Table 1). The germination capacity was the same (90%) at ambient and high temperature. Under ambient temperature maximum germination occurred 6 days after seeding. Seeds attained 50% of maximum germination 1–1.5 days earlier under high temperature than in ambient temperature. This conforms with the germination behavior of E. colona (Chauhan and Johnson, 2009). Optimum germination occurs between 30 and 35°C, but it can germinate up to 40°C. Tropical weeds, such as E. colona, germinate faster at higher temperature. Therefore, under elevated temperature, we can expect E. colona to get established faster and compete with the crop more vigorously early in the season, intensifying the need for effective weed management from crop planting to harvest. E. colona is a C4 plant (Giussani et al., 2001); it responds more to high temperature than the C3 crop, rice.
The data collectively indicate that increasing temperature or CO2 levels alter the nature of plant-herbicide interaction, eventually resulting in reduced efficacy of cyhalofop-butyl, increased resistance of MR E. colona to ACCase inhibitors, and increased potential competitive ability with the crop. Elevated [CO2] and high temperature, two fundamental aspects of global climate change, could act as factors for resistance evolution to herbicides. As mentioned earlier, this MR population harbors NTSR mechanisms to quinclorac (hormone-type action) and propanil (PS II inhibitor). Since the general mechanisms underlying NTSR [herbicide detoxification or sequestration, reduced translocation, protection from damage, overproduction of herbicide target (Délye, 2013)] are the same across weedy species, and the drivers of these mechanisms are affected by abiotic stresses, climate change can accelerate the evolution of NTSR to herbicides of different modes of action in weeds. In nature, atmospheric [CO2] and temperature are going to change concurrently as climate changes. Our follow-up research will focus on modeling the interaction effect of increasing [CO2] and temperature on the response of some key weedy species to herbicides. The expectation is that C3 crops (i.e., rice, soybean) would approach the photosynthetic capacity of C4 weeds (i.e., Echinochloa spp., Amaranthus spp.) under excess supply of CO2. Photosynthetic capacity is also expected to increase further when CO2 and temperature are not limiting. However, weeds generally respond more to resource changes than crops and many major weeds today, like many crops, are C3 (i.e., Abutilon theophrasti L., Ambrosia spp., Avena fatua L., Chenopodium album L., Ipomoea spp.) (Ziska and Bunce, 1997; Ziska and McClung, 2008; Ziska and Dukes, 2011). Many troublesome weeds in rice production, for example, are C3 plants; the most notorious of these is its close relative, weedy rice (Oryza sativa L.) (Delouche et al., 2007). Although both are of the same genus and species, weedy rice responds more to CO2 enrichment (Ziska and McClung, 2008) and nitrogen addition (Burgos et al., 2006). It is also troubling that cases of resistance, or multiple resistance, to herbicides are increasing among key rice weeds, as in other crops (Roma-Burgos et al., 2019). Our data, and those of several other research groups, show that high atmospheric [CO2] or high temperature reduces sensitivity of weed species to various herbicides. More research is needed to understand the complex effects of climate change (combined effect of high temperature and high CO2) on crop-weed-herbicide interactions to help agriculture practitioners attain and sustain food and fiber security in the face of changing climate.
To mitigate rapid resistance evolution under a changing climate, weed management practitioners must implement measures to reduce the herbicide selection pressure. These measures include reduction of weed population size through reduction of the soil seedbank, ensuring complete control of current infestations with multiple herbicide modes of action in mixture and in sequence, augmenting herbicides with mechanical control where possible, rotation with weed-competitive crops, use of weed-competitive cultivars, use of weed-suppressive cover crops, and other practices recommended for integrated weed management (Norsworthy et al., 2012). Implementing the most effective combination of practices to reduce weed infestation is complex, time-consuming, and costly. Currently, weed resistance problems are evolving in developed, developing, or underdeveloped countries wherever herbicides are used intensively (Heap, 2019). Farmers in “resource-sufficient” regions do not hold an advantage over those in “resource-poor” regions. Therefore, it will require a drastic change in mentality, across all groups of people influencing or conducting weed management, to manage weeds with the goal of averting resistance rather than to fix resistance problems.
The absorption of cyhalofop-butyl in herbicide-susceptible and multiple-resistant E. colona does not change under elevated [CO2] or high temperature. The translocation of cyhalofop out of the treated leaf is modified slightly by increased [CO2] or temperature, but the major effect is the significant increase in resistance to cyhalofop-butyl in the multiple-resistant genotype. Therefore, elevated [CO2] or high temperature increases the resistance level of E. colona to the ACCase-inhibitor, cyhalofop-butyl. Differential response between S and MR genotypes under high [CO2] or temperature would, in turn, accelerate the evolution of herbicide resistance in response to global climate change. The fact that the MR genotype has extreme resistance to quinclorac and propanil, which involves NTSR mechanisms as shown in background studies, results of this current study have broad implications on the impact of climate change on the evolution of NTSR to herbicides in weeds.
JR, NR-B, LdA, and EC designed the experiments. JR, CO, and RS-P performed the experiments. JR analyzed the data. JR and NR-B wrote the manuscript. NR-B, JR, CR, LZ, and LdA discussed the results and their implications and revised the manuscript.
Financial support for this research was provided by the BASF Corp. Scholarship for JR, was provided by CNPq Project # 400897/2014-8. LdA was Research Fellow for CNPq Project: 310538/2015-7. This study was financed in part by the Coordenação de Aperfeiçoamento de Pessoal de Nível Superior - Brasil (CAPES) — Finance Code 001 financing JPR Ph.D. Assistantship.
The authors declare that the research was conducted in the absence of any commercial or financial relationships that could be construed as a potential conflict of interest.
Facilities for this research were made available by the Department of Crop, Soil, and Environmental Sciences at the University of Arkansas, Fayetteville, United States. Radiolabeled cyhalofop-butyl was provided by Dow AgroSciences.
The Supplementary Material for this article can be found online at: https://www.frontiersin.org/articles/10.3389/fpls.2019.00529/full#supplementary-material
TABLE 1 |Echinochloa colona germination under ambient (aT) and elevated (eT) temperature, averaged across genotypes, Fayetteville, AR, United States.
FIGURE 1 | Absorption of 14C-cyhalofop-butyl in susceptible- (S) and multiple-resistant (MR) Echinochloa colona under ambient and elevated [CO2] 120 h after application. Lines represent standard errors of the mean. Commercial formulation was sprayed at V3 stage with 1% crop oil concentrate and the leaf was spotted with 1 kBq⋅μL-1; Fayetteville, AR, United States.
Abouziena, H. F., and Haggag, W. M. (2016). Weed control in clean agriculture: a review 1. Planta Daninha 34, 377–392. doi: 10.1590/S0100-83582016340200019
Ainsworth, E. A., and Long, S. P. (2005). What have we learned from 15 years of free- air CO2 enrichment (FACE)? A meta-analytic review of the responses of photosynthesis, canopy properties and plant production to rising CO2. New Phytol. 165, 351–371. doi: 10.1111/j.1469-8137.2004.01224.x
Bailey, S. W. (2004). Climate change and decreasing herbicide persistence. Pest Manag. Sci. 60, 158–162. doi: 10.1002/ps.785
Brazier, M., Cole, D. J., and Edwards, R. (2002). O-Glucosyltransferase activities toward phenolic natural products and xenobiotics in wheat and herbicide-resistant and herbicide-susceptible black-grass (Alopecurus myosuroides). Phytochemistry 59, 149–156. doi: 10.1016/S0031-9422(01)00458-7
Bunce, J. A., and Ziska, L. H. (2000). “Crop ecosystem responses to climatic change: crop/weed interactions,” in Climate Change and Global Crop Productivity, eds K. R. Reddy and H. F. Hodges (New York, NY: CABI), 333–348. doi: 10.1079/9780851994390.0271
Burgos, N. R., Norman, R. J., Gealy, D. R., and Black, H. R. (2006). Competitive N uptake between rice and weedy rice. Field Crops Res. 99, 96–105. doi: 10.1016/j.fcr.2006.03.009
Chauhan, B. S., and Johnson, D. E. (2009). Seed germination ecology of junglerice (Echinochloa colona): a major weed of rice. Weed Sci. 57, 235–240. doi: 10.1614/ws-08-141.1
Chin, V. D. (2001). Biology and management of barnyardgrass, red sprangletop and weedy rice. Weed Biol. Manag. 1, 37–41. doi: 10.1046/j.1445-6664.2001.00009.x
Cummins, I., Wortley, D., Sabbadin, F., He, Z., Coxon, C., Strakera, H., et al. (2013). A key role for a glutathione transferase in multiple herbicide resistance in grass weeds. Proc. Natl. Acad. Sci. U.S.A. 110, 5812–5817. doi: 10.1073/pnas.1221179110
De Prado, J. L., Osuna, M. D., Heredia, A., and De Prado, R. (2005). Lolium rigidum, a pool of resistance mechanisms to ACCase inhibitor herbicides. J. Agric. Food Chem. 53, 2185–2191. doi: 10.1021/jf049481m
Delouche, J. C., Burgos, N. R., Gealy, D. R., Zorilla-San Martin, G., Labrada, R., and Larinde, M. (2007). Weedy Rices: Origin, Biology, Ecology and Control. Rome: Food and Agriculture Organization of the United Nations, 122.
Délye, C. (2013). Unravelling the genetic bases of non-target-site-based resistance (NTSR) to herbicides: a major challenge for weed science in the forthcoming decade. Pest Manag. Sci. 69, 176–187. doi: 10.1002/ps.3318
Délye, C., Gardin, J. A. C., Boucansaud, K., Chauvel, B., and Petit, C. (2011). Non-target-site-based resistance should be the center of attention for herbicide resistance research: Alopecurus myosuroides as an illustration. Weed Res. 51, 433–437. doi: 10.1111/j.1365-3180.2011.00864.x
Fernández, P., Alcántara-de la Cruz, R., Cruz-Hipólito, H., Osuna, M. D., and De Prado, R. (2016). Underlying resistance mechanisms in the Cynosurus echinatus biotype to acetyl CoA carboxylase-inhibiting herbicides. Front. Plant Sci. 7:449. doi: 10.3389/fpls.2016.00449
Fischer, A., Ramirez, H. V., and Lozano, J. (1997). Suppression of junglerice (Echinochloa colona (L.) Link) by irrigated rice cultivars in Latin America. Agron. J. 89, 516–521.
Gianessi, L. P. (2013). The increasing importance of herbicides in worldwide crop production. Pest Manag. Sci. 69, 1099–1105. doi: 10.1002/ps.3598
Giussani, L. M., Cota-Sanchez, J. H., Zuloaga, F. O., and Kellogg, E. A. (2001). A molecular phylogeny of the grass subfamily panicoideae (Poaceae) shows multiple origin of C4 photosynthesis. Am. J. Bot. 88, 1993–2012. doi: 10.2307/3558427
Godar, A. S., Varanasi, V. K., Nakka, S., Prasad, P. V., Thompson, C. R., and Mithila, J. (2015). Physiological and molecular mechanisms of differential sensitivity of palmer amaranth (Amaranthus palmeri) to mesotrione at varying growth temperatures. PLoS One 10:e0126731. doi: 10.1371/journal.pone.0126731
Heap, I. (2014). Global perspective of herbicide-resistant weeds. Pest Manag. Sci. 70, 1306–1315. doi: 10.1002/ps.3696
Heap, I. (2019). The International Survey of Herbicide Resistant Weeds. Online. Internet. Available at: www.weedscience.org (accessed February 21, 2019).
Hill, J. E., Le Strange, M. L., Bayer, D. E., and Williams, J. F. (1985). Integrated weed management in California. Proc. West. Soc. Weed Sci. 38, 100–104.
Huan, Z., Xu, Z., Ly, D., and Wang, J. (2013). Determination of ACCase sensitivity and gene expression in quizalofop–ethyl-resistant and -susceptible barnyardgrass (Echinochloa crus-galli) biotypes. Weed Sci. 61, 537–542. doi: 10.1614/ws-d-13-00010.1
IPCC (2014). Climate Change 2014: Synthesis Report. Contribution of Working Groups I, II and III to the Fifth Assessment Report of the Intergovernmental Panel on Climate Change, eds Core Writing Team, R. K. Pachauri, and L. A. Meyer (Geneva: IPCC), 151.
Jackson, L., Wheeler, S., Hollander, A., O’Geen, A., Orlove, B., Six, J., et al. (2011). Case study on potential agricultural responses to climate change in a California landscape. Clim. Change 109, 407–427. doi: 10.1007/s10584-011-0306-3
Johnson, B. C., and Young, B. G. (2002). Influence of temperature and relative humidity on the foliar activity of mesotrione. Weed Sci. 50, 157–161. doi: 10.1614/0043-1745(2002)050
Kaundun, S. S. (2014). Resistance to acetyl-CoA carboxylase-inhibiting herbicides. Pest Manag. Sci. 70, 1405–1417. doi: 10.1002/ps.3790
Korres, N. E., Norsworthy, J. K., Tehranchian, P., Gitsopoulos, T. K., Loka, D. A., Oosterhuis, D. M., et al. (2016). Cultivars to face climate change effects on crops and weeds: a review. Agron. Sustain. Dev. 36:12.
Laforest, M., Soufiane, B., Simard, M. J., Obeid, K., Page, E., and Nurse, R. E. (2017). Acetyl-CoA carboxylase overexpression in herbicide-resistant large crabgrass (Digitaria sanguinalis). Pest Manag. Sci. 73, 2227–2235. doi: 10.1002/ps.4675
Manea, A., Leishman, M. R., and Downey, P. O. (2011). Exotic C4 grasses have increased tolerance to glyphosate under elevated carbon dioxide. Weed Sci. 59, 28–36. doi: 10.1614/WS-D-10-00080.1
Martini, L. F., Burgos, N. R., Noldin, J. A., Avila, L. A., and Salas, R. A. (2015). Absorption, translocation and metabolism of bispyribac-sodium on rice seedlings under cold stress. Pest Manag. Sci. 71, 1021–1029. doi: 10.1002/ps.3882
Matzrafi, M., Seiwert, B., Reemtsma, T., Rubin, B., and Peleg, Z. (2016). Climate change increases the risk of herbicide-resistant weeds due to enhanced detoxification. Planta 244, 1217–1227. doi: 10.1007/s00425-016-2577-4
Milner, L. J., Reade, J. P. H., and Cobb, A. H. (2001). Developmental changes in glutathione S-transferase activity in herbicide-resistant populations of Alopecurus myosuroides Huds (black-grass) in the field. Pest Manag. Sci. 57, 1100–1106. doi: 10.1002/ps.403
Norsworthy, J. K., Ward, S. M., Shaw, D. R., Llewellyn, R. S., Nichols, R. L., Webster, T. M., et al. (2012). Reducing the risks of herbicide resistance: best management practices and recommendations. Weed Sci. 60, 31–62. doi: 10.1614/ws-d-11-00155.1
Peerzada, A. M., Bajwa, A. A., Ali, H. H., and Chauhan, B. S. (2016). Biology, impact, and management of Echinochloa colona (L.) Link. Crop Prot. 83, 56–66. doi: 10.1016/j.cropro.2016.01.011
Powles, S. B., and Yu, Q. (2010). Evolution in action: plants resistant to herbicides. Annu. Rev. Plant Biol. 61, 317–347. doi: 10.1146/annurev-arplant-042809-112119
Ramesh, K., Matloob, A., Aslam, F., Florentine, S. K., and Chauhan, B. S. (2017). Weeds in a changing climate: vulnerabilities, consequences, and implications for future weed management. Front. Plant Sci. 8:95. doi: 10.3389/fpls.2017.00095
Rodenburg, J., Meinke, H., and Johnson, D. E. (2011). Challenges for weed management in African rice systems in a changing climate. J. Agric. Sci. 149, 427–435. doi: 10.1017/S0021859611000207
Roma-Burgos, N., Heap, I. M., Rouse, C. E., and Lawton-Rauh, A. L. (2019). Evolution of Herbicide-Resistant Weeds, eds N. E. Korres, N. R. Burgos, and S. O. Duke (Boca Raton, FL: CRC Press), 92–132.
Roma-Burgos, N., Rouse, C. E., Saski, C. A., Noorai, R. E., Shankar, V., and Lawton-Rauh, A. L. (2018). “Concerted enrichment of gene networks in multiple-resistant Echinochloa colona,” in Proceedings of the Annual Meeting, (Arlington, VA: Weed Science Society of America), 387.
Rouse, C. E., and Burgos, N. R. (2017). “Physiological mechanisms of resistance to quinclorac in multiple-resistant junglerice (Echinochloa colona),” in Proceedings of the 70th Annual Meeting Southern Weed Science Society,(Birmingham, AL), 282.
Rouse, C. E., Burgos, N. R., Norsworthy, J. K., Tseng, T. M., Starkey, C. E., and Scott, R. C. (2018a). Echinochloa resistance to herbicides continues to increase in Arkansas rice fields. Weed Technol. 32, 34–44. doi: 10.1017/wet.2017.82
Rouse, C. E., Roma-Burgos, N., Saski, C. A., Noorai, R. E., and Shankar, V. (2018b). “Co-evolution of abiotic stress adaptation and quinclorac resistance in Echinochloa colona,” in Proceedings of the Annual Meeting, (Arlington, VA: Weed Science Society of America), 386.
Ruiz-Santaella, J. P., De Prado, R., Wagner, J., Fischer, A. J., and Gerhards, R. (2006a). Resistance mechanisms to cyhalofop-butyl in a biotype of Echinochloa phyllopogon (Stapf) Koss. from California. J. Plant Dis. Prot. 20, 95–100.
Ruiz-Santaella, J. P., Heredia, A., and De Prado, R. (2006b). Basis of selectivity of cyhalofop-butyl in Oryza sativa L. Planta 223, 191–199. doi: 10.1007/s00425-005-0075-1
Scott, R. C., Barber, L. T., Boyd, J. W., Norsworthy, J. K., and Burgos, N. R. (2018). Recommended Chemicals for Weed and Brush Control. Little Rock, AR: University of Arkansas Division of Agriculture, 195.
Smith, R. J. (1988). Weed thresholds in southern U.S. rice, Oryza sativa. Weed Technol. 2, 232–241. doi: 10.1017/s0890037x00030505
Soltani, N., Dille, J. A., Burke, I. C., Everman, W. J., VanGessel, M. J., Davis, V. M., et al. (2016). Potential corn yield losses from weeds in North America. Weed Technol. 30, 979–984. doi: 10.1614/wt-d-16-00046.1
Stauber, L. G., Smith, R. J., and Talbert, R. E. (1991). Density and spatial interference of barnyardgrass (Echinochloa crus-galli) with rice (Oryza sativa). Weed Sci. 39, 163–168.
Varanasi, A., Prasad, P. V. V., and Jugulam, M. (2015). Impact of climate change factors on weeds and herbicide efficacy. Adv. Agron. 135, 107–146. doi: 10.1016/bs.agron.2015.09.002
Vila-Aiub, M. M., Gundel, P. E., Yu, Q., and Powles, S. B. (2013). Glyphosate resistance in Sorghum halepense and Lolium rigidum is reduced at suboptimal growing temperatures. Pest Manag. Sci. 69, 228–232. doi: 10.1002/ps.3464
Wenger, J., Niderman, T., and Mathew, C. (2012). “Acetyl-CoA carboxylase inhibitors,” in Modern Crop Protection Compounds, 2nd Edn, eds W. Krämer, U. Schirmer, P. Jeschke, and W. Matthias (Weinheim: Wiley-VCH), 447–477.
Ziska, L. H., and Bunce, J. A. (1997). Influence of increasing carbon dioxide concentration on the photosynthetic and growth stimulation of selected C4 crops and weeds. Photosynth. Res. 54, 199–208. doi: 10.1023/A:1005947802161
Ziska, L. H., and Bunce, J. A. (2006). “Plant responses to rising carbon dioxide,” in Plant Growth and Climate Change, eds J. I. L. Morison and M. D. Morecroft (Oxford: Blackwell Publishing), 17–47. doi: 10.1002/9780470988695.ch2
Ziska, L. H., and Dukes, J. S. (2011). Weed Biology and Climate Change. Ames, IA: Blackwell Publishing Ltd., 68–205.
Ziska, L. H., Faulkner, S. S., and Lydon, J. (2004). Changes in biomass and root: shoot ratio of field- grown Canada thistle (Cirsium arvense), a noxious, invasive weed, with elevated CO2: implications for control with glyphosate. Weed Sci. 52, 584–588. doi: 10.1614/WS-03-161R
Ziska, L. H., and McClung, A. (2008). Differential response of cultivated and weedy (red) rice to recent and projected increases in atmospheric carbon dioxide. Agron. J. 100, 1259–1263. doi: 10.2134/agronj2007.0324
Keywords: carbon dioxide, climate change, Echinochloa, junglerice, cyhalofop-butyl, multiple-resistance, resistance evolution
Citation: Refatti JP, de Avila LA, Camargo ER, Ziska LH, Oliveira C, Salas-Perez R, Rouse CE and Roma-Burgos N (2019) High [CO2] and Temperature Increase Resistance to Cyhalofop-Butyl in Multiple-Resistant Echinochloa colona. Front. Plant Sci. 10:529. doi: 10.3389/fpls.2019.00529
Received: 07 November 2018; Accepted: 05 April 2019;
Published: 08 May 2019.
Edited by:
Stefano Santabarbara, Italian National Research Council (CNR), ItalyReviewed by:
Francesco Vidotto, University of Turin, ItalyCopyright © 2019 Refatti, de Avila, Camargo, Ziska, Oliveira, Salas-Perez, Rouse and Roma-Burgos. This is an open-access article distributed under the terms of the Creative Commons Attribution License (CC BY). The use, distribution or reproduction in other forums is permitted, provided the original author(s) and the copyright owner(s) are credited and that the original publication in this journal is cited, in accordance with accepted academic practice. No use, distribution or reproduction is permitted which does not comply with these terms.
*Correspondence: Nilda Roma-Burgos, bmJ1cmdvc0B1YXJrLmVkdQ==
†Present address: Christopher Edward Rouse, FMC Corporation, Newark, DE, United States; Reiofeli Salas-Perez Dole Philippines, Inc., South Cotabato, Philippines
Disclaimer: All claims expressed in this article are solely those of the authors and do not necessarily represent those of their affiliated organizations, or those of the publisher, the editors and the reviewers. Any product that may be evaluated in this article or claim that may be made by its manufacturer is not guaranteed or endorsed by the publisher.
Research integrity at Frontiers
Learn more about the work of our research integrity team to safeguard the quality of each article we publish.