- 1Department of Agricultural Chemistry and Food Science, Autonomous University of Madrid, Madrid, Spain
- 2Kurnakov Institute of General and Inorganic Chemistry, Russian Academy of Sciences, Moscow, Russia
- 3Department of Materials Science, Lomonosov Moscow State University, Moscow, Russia
- 4Department of Chemistry, Lomonosov Moscow State University, Moscow, Russia
- 5Department of Chemistry and Physical Chemistry of Soils, V.V. Dokuchaev Soil Science Institute, Moscow, Russia
- 6National Research Center “Kurchatov Institute”, Moscow, Russia
Iron deficiency is a frequent problem for many crops, particularly in calcareous soils and iron humates are commonly applied in the Mediterranean basin in spite of their lesser efficiency than iron synthetic chelates. Development and application of new fertilizers using nanotechnology are one of the potentially effective options of enhancing the iron humates, according to the sustainable agriculture. Particle size, pH, and kinetics constrain the iron humate efficiency. Thus, it is relevant to understand the iron humate mechanism in the plant–soil system linking their particle size, characterization and iron distribution in plant and soil using 57Fe as a tracer tool. Three hybrid nanomaterials (F, S, and M) were synthesized as iron-humic nanofertilizers (57Fe-NFs) from leonardite potassium humate and 57Fe used in the form of 57Fe(NO3)3 or 57Fe2(SO4)3. They were characterized using Mössbauer spectroscopy, X-ray diffraction (XRD), extended X-ray absorption fine structure spectroscopy (EXAFS), transmission electron microscopy (TEM) and tested for iron availability in a calcareous soil pot experiment carried out under growth chamber conditions. Three doses (35, 75, and 150 μmol pot-1) of each iron-humic material were applied to soybean iron deficient plants and their iron nutrition contributions were compared to 57FeEDDHA and leonardite potassium humate as control treatments. Ferrihydrite was detected as the main structure of all three 57Fe-NFs and the plants tested with iron-humic compounds exhibited continuous long-term statistically reproducible iron uptake and showed high shoot fresh weight. Moreover, the 57Fe from the humic nanofertilizers remained available in soil and was detected in soybean pods. The Fe-NFs offers a natural, low cost and environmental option to the traditional iron fertilization in calcareous soils.
Introduction
Iron (Fe) is an essential micronutrient for humans and plants. Iron deficiency is very common in the human diet and affects an estimated two billion people in the world (Briat et al., 2015). Iron chlorosis is a widespread agricultural problem occurring in about 30–50% of cultivated soils (Cakmak, 2002) and one of the major limiting factor of crop production in calcareous soils. Farmers apply iron synthetic chelates to alleviate iron deficiency in cash crops. Despite the high costs of these fertilizers, they tend to lixiviate and the chelating agents may avoid the precipitation and enhance mobilization of heavy metals (Ylivainio, 2010). Many crops are sensitive to the iron chlorosis, such as citrus and fruit trees but soybean (Glycine max L.) is one of the most studied iron Strategy I plant (Fuentes et al., 2018). Moreover, soybean production reaches levels of about 230 million metric tons per year across the world (Vasconcelos and Grusak, 2014) and this legume is a highly nutritious crop which contains more protein (40%) and oil (20%) than any other ordinary food source (Bolon et al., 2010).
According to the United Nations [UN] (2013), the rapidly growing world population is projected to reach 9.6 billion by the year 2050 and Food and Agriculture Organization of the United Nations [FAO] (2017) has predicted that the global grain production is required to increase by 70% to meet these demands. Therefore, new approaches should be developed for alleviation of iron deficiency in plants and new ecofriendly fertilizers are needed in order to enhance crop environmental quality. Iron fertilizers based on HSs extracted from lignites, such as leonardite, are used in the Mediterranean area (as liquid concentrates) in drip irrigation (Kovács et al., 2013). This kind of iron fertilizers is more ecofriendly than synthetic iron chelates but they are less efficient in correcting iron chlorosis. Moreover, field experiments have demonstrated that the synthetic chelate has a fast effect while the iron humate fertilizers provide increasing iron availability in the root–soil interface resulting in slow uptake of Fe by the plants (Cieschi et al., 2017). Kulikova et al. (2017) have demonstrated that only iron from very small and amorphous nanoparticles of ferric polymers incorporated into humic matrix is readily taken up by plants. Therefore, the synthesis of iron humates should be optimized for developing efficient NFs.
According to Naderi and Danesh-Shahraki (2013), NFs are the most important products of nanotechnology with regard to agriculture. Nanosized active ingredients (from 1 to 100 nm in diameter) have a large specific surface area that can result in significantly enhanced reactivity, and this feature increases absorption of nutritional elements and essential compounds for plant growth and plant metabolism (Janmohammadi et al., 2016). Many attempts has been made to prepare inorganic Fe nanofertilizers. As example Sánchez-Alcalá et al. (2012) synthesized nanosiderite (FeCO3) and demonstrated that it was highly effective in preventing iron chlorosis in chickpea and had a great residual effect. Ghafariyan et al. (2013) reported that low concentrations of superparamagnetic Fe-NPs significantly increased the chlorophyll contents in sub-apical leaves of soybeans in a greenhouse test under hydroponic conditions, suggesting that soybean could use this type of Fe-NPs as source of Fe and reduce chlorotic symptoms of Fe deficiency. However, the research on natural Fe nano-humate complexes is now in progress. Dholakia (2016) developed the preparation of nanoparticulate liquid organic fertilizers employing humic acids. In addition, Kulikova et al. (2017) have synthesized well-defined iron (hydr)oxide NPs of feroxyhyte stabilized by traces of HS (a model of iron-based engineered NPs) and water-soluble Fe-HS complexes of the proven high availability to plants tested their iron materials in wheat plants under hydroponic conditions. These promising results motivated us to follow the research on Fe NFs stabilized with humates.
According to Dimkpa and Bindraban (2017), up to now, the bulk of research in plant nanoscience either consists of experiments conducted in artificial media, such as nutrient solutions, agar, sand, or other non-soil media. Moreover, Liu and Lal (2015) recommend that micronutrient research should focus on enhancing the bioavailability (plant-uptake rate) of NFs to address the field leaching associated with the conventional micronutrient fertilizers and compare the beneficial effects of these micronutrient NFs with commercially available micronutrient counterparts [e.g., FeNPs vs. FeCl3 or Fe(EDTA) as Fe sources] under the field condition. Therefore, it is of particular importance to test the 57Fe-NFs in a soil system in a long-term experiment which would enable for completion of the full growth cycle crop in order to be closer to agronomical conditions. Since the efficacy of an iron fertilizer is related to the iron that the plants can take from the fertilizer, the use of iron isotopes is highly beneficial for monitoring iron uptake by plants (Cesco et al., 2002; Nikolic et al., 2003; Tomasi et al., 2013). The use of stable Fe isotopes instead of radioactive ones gives a high flexibility in the experimental designs and can include field studies, because special safety measurements and trained staff are not required. Moreover, long-term assays can be carried out without taking care of radioactivity decay over time. In addition, the generation of radioactive wastes is avoided (Benedicto et al., 2011). Many studies about 57Fe application in soils experiments (Nadal et al., 2012; Martín-Fernández et al., 2017a,b) were reported, but this work is the first one in preparing 57Fe-NFs and applying them in a calcareous soil.
Here, three 57Fe-labeled humic nanomaterials (F, S, and M) were synthesized using potassium humate as a parent humic material and 57Fe in the form of 57Fe(NO3)3 (product F) and 57Fe2(SO4)3 (products S and M), characterized for iron speciation and phase composition of nanoparticles, and tested for bioavailability to soybean iron deficient plants grown in calcareous soils under growth chamber conditions. This was to establish a link between the Fe-NPs characteristics and their behavior in the soil–plant system using 57Fe as a tracer tool.
Materials and Methods
Reagents
All reagents used were of recognized analytical grade, and solutions were prepared with type-I grade water (ISO 3696:1987, 1987) free of organic contaminants (Millipore, Milford, CT, United States).
Synthesis of 57Fe-NFs
Prior to the synthesis, a known weight of leonardite potassium humate (C 34.9%, H 3.89%, N 0.72%, S 0.06%, Fe 0.45%) (Powhumus, Humintech Ltd., Germany) was dissolved in distilled water and centrifuged at 10,000 min-1 for 10 min to separate and discard any insoluble mineral components. The obtained solution contained 70 g L-1 of leonardite potassium humate (L solution) and was used for the further NF synthesis.
A 57Fe2(SO4)3 solution (0.20 M in 57Fe) was prepared from metallic 57Fe (Isoflex, 96.28% 57Fe isotopic enrichment) by dissolving 0.4008 g in 34 mL 1M H2SO4 and heating till complete dissolution. After that, two products (S and M) were obtained by interaction of potassium humate with 57Fe2(SO4)3 solution. In brief, the product S was synthesized as in Sorkina et al. (2014), 17 mL of 0.2M 57Fe2(SO4)3 solution was added dropwise to 14.3 mL of the L solution and pH was maintained at a value of 10 by adding slowly 1M KOH when needed. For the synthesis of the product M, 17 mL of 57Fe2(SO4)3 solution was slowly added to 40 mL of L solution, maintaining the pH at 9 with 1M KOH. The product M was prepared with the 90% of its maximum complexing capacity (Fe-MCC). Determination of Fe-MCC was conducted as described in Villén et al. (2007) and presented in the Supplementary Figure SM1. According to the obtained titration curve, an amount of 190 mg of Fe (III) per g org C-1 was necessary to obtain 200 mg of complexed Fe (III) per g org C-1 at the MCC.
Similarly, for the preparation of F product, a 57Fe(NO3)3 solution was prepared from the same metallic 57Fe by dissolving 0.2004 g in 5 mL HNO3 (70%, 1,401 g/mL density) and then diluted. The obtained solution was added dropwise to 32 mL of the L solution, maintaining the pH at 9 with 1M KOH.
In all syntheses described above, the final reaction mixtures were frozen “as is” using liquid N2 and freeze-dried using Labconco FreeZone freeze dry system (-50°C, 0.03 mbar pressure).
It should be noted, that the high hydrolysis rate is required to obtain ultradispersed (nanosized) iron (hydr)oxide nanoparticles from iron (III) sulfate or nitrate solutions. For this, we added 57Fe2(SO4)3 and 57Fe(NO3)3 dropwise but rapidly to the strongly alkaline medium of potassium humate solution and prevented the pH drop by simultaneous addition of KOH. The pH values between 9 and 10 were chosen to ensure formation of the disordered and chemically labile iron oxy-hydroxide phases instead of well-crystalline iron oxides like Fe3O4, Fe2O3 or rigid α-FeOOH.
The content of soluble iron in the synthesized fertilizers was determined using ICP AES. It was (in % mass) 2.9, 2.3, and 2.1 in the samples F, S, and M, respectively. The Fe:org C ratios were 0.27, 0.52, and 0.12 (g Fe g C org-1) in the samples F, S, and M, respectively.
Characterization of 57Fe-NFs
The freeze dried preparations of 57Fe-NFs were exhaustively characterized using XRD, TEM with ED, EELS and energy filtered transmission electron microscopy (EFTEM), X-ray absorption spectroscopy (XANES and EXAFS) and Mössbauer spectroscopy. The XRD patterns were collected at CuKα on Rigaku D-MAX 2500 diffractometer in Theta/2Theta geometry. Reference samples, such as ferrihydrite and goethite were synthesized according to the procedure proposed by Schwertmann and Cornell (1992) and described by López-Rayo et al. (2015).
The TEM data were obtained with the use of Zeiss Libra 200MC microscope, equipped with monochromator and Omega-filter. For the TEM measurements, samples were dissolved in distilled water, dropped on the lacey-carbon coated copper grid for the few minutes with the following removal of the solution excess to reduce the concentration of mineral salts in the sample. Details of energy filtered TEM and SAED acquisition and processing are described in the corresponding part and in Supplementary Materials. Image processing was performed with the use of Gwyddion (Nečas and Klapetek, 2012), further data treatment – with the use of scipy and matplotlib (Hunter, 2007). The EELS spectra were acquired using the omega-filter, and integrated with DigitalMicrograph2 (DM2) software, Gatan. The variable slit of 3.5 eV width was placed in the Omega-filter to select the elastic part of scattered electrons. Two EFTEM images were collected with the background signal differing in intensity in the pre-edge energy area, and one image – with the combined signals of iron and background – was collected on the Fe M-line. Final iron distribution map was calculated using the DM2 software.
X-ray absorption spectra were measured at the STM beamline of Kurchatov Synchrotron Source facility, National Research Center “Kurchatov Institute”, Moscow, Russia. The Si (111) channel-cut monochromator was used. Ionization chambers with length of 10 cm filled with argon were used as detectors of incidence and transmitted beams. The samples were used as dry powders mounted onto the kapton tape. Thickness of each sample was adjusted to yield the absorption value of 3. All spectra for the iron-containing NFs under study and the iron (hydr)oxide references were measured by absorption using Fe foil as a reference sample. XAS of the parent humate (L) was measured using fluorescence due to the low iron content in this sample. The Amptek X-123 SDD detector was used for this purpose. Six spectra were acquired for each sample and averaged. All spectra were handled with the use of Athena software. The further modeling and refinements were done using the Artemis software. Refinements were performed by k2-weighted spectra in the range of 3–14 Å-1 in the k-space, and of 1–3.5 Å in the R-space, Hanning window function was used. The value of S02 = 1 was fixed during the refinements.
Mössbauer absorption spectra were obtained on MS1104EM Express Mössbauer spectrometer (Cordon GmbH, Rostov-on-Don). The radiation source with an activity of 6 mCi was 57Co in a metal rhodium matrix (RITVERC GmbH, St. Petersburg, Russia). The spectra were obtained at room temperature (295 ± 3 K) and in a vacuumed cryostat at a liquid nitrogen temperature (77.5 ± 0.5 K). The spectra were collected until the signal to noise ratio was less than 1%. Mathematical processing was carried out for spectra with a high resolution (1,024 points) using SpectrRelax 2.4 (Lomonosov MSU, Russia) software. The isomer shift was determined relative to α Fe.
Soil Pot Experiment
Fertilizers
A stock solution (1,000 μmol Fe L-1) of each 57Fe-humic NF (F, S, and M) at pH 7 was prepared from the freeze dried products (57Fe-NFs) previously obtained, as it was described above A stock solution of 57FeEDDHA (1,000 μmol Fe L-1) was prepared by chelation with Fe3+ from Fe(NO3)3 and o-oEDDHA [ethylenediamine-di (o-o hydroxyphenylacetic acid)] obtained from LGC Standards, Teddington, United Kingdom (93.12%), previously dissolved with three mol of NaOH per mol of chelating agent. The solution was adjusted at pH 7 with 1M KOH.
In order to test the 57Fe-NFs as correctors of iron chlorosis, three doses (35, 75, and 150 μmol 57Fe pot-1) were applied to iron deficient soybean plants and compared to 57FeEDDHA (50 μmol pot-1), as a positive control, and L (providing 8.9 μmol Fe pot-1). The treatments were applied over the soil surface 2 days after the soybean plants were transferred to the pots. Five replicates (five pots) per fertilizer were carried out.
Plant Material
Soybeans (Glycine max AG1835 Asgrow Seed Co.) were germinated in the dark at room temperature on filter paper moistened with distilled water. After germination (7 days), seedlings were transferred to the growth chamber where they grew until the end of the experiment in a Dycometal-type CCK growth chamber provided with fluorescent and sodium vapor lamps with a 16 h, 25°C and 40% humidity day and 8 h, 20°C and 60% humidity night regime. Seedlings were placed on containers filled with 1/5 diluted nutrient solution of the full-strength solution with the following composition: macronutrients (mM) 1.0 Ca(NO3)2, 0.9 KNO3, 0.3 MgSO4, and 0.1 KH2PO4; cationic micronutrients (μM) 2.0 FeHBED, 2.5 MnSO4, 1.0 CuSO4, 10.0 ZnSO4, 1.0 CoSO4, 1.0 NiCl2, and 115.5 EDTANa2; anionic micronutrients (μM) 35.0 NaCl, 10.0 H3BO3, and 0.05 Na2MoO4 and 0.1 mM HEPES. The pH was adjusted to 7.5 with 1.0M KOH. After 8 days, the diluted nutrient solution was replaced by the full-strength solution without Fe. Seedlings were kept in this solution for 2 days in order to induce iron deficiency. In order to simulate calcareous conditions, CaCO3 (0.1 g L-1) was added to each pot. The deficient iron soybean plants (three plants per pot) were transferred into 600 g polystyrene pots filled in with the soil/sand 70/30% (w/w) mixture. The soil was obtained from the top 20 cm of a citrus farm at Picassent, Valencia, Spain (39°21′41.28′′ N, 0°27′42.58′′ W). Physicochemical characteristics of this soil are described in Table 1. Texture, pH, soil E.C., OM, C/N ratio, CaCO3 were measured according to the official methods (MAPA, 1994) and micronutrients availability was determined as described by Soltanpour and Schwab (1977). Normalized calcareous sand (2–4 mm) was used. One day before transferring the seedlings, pots were irrigated till field capacity. Water and iron free nutrient solution were added every day. Two samplings were carried out, at 15 and 48 days after the fertilizers (DAF) were applied.
Analytical Procedures
The sampled roots, stems, and leaves were separated, weighted, and washed with 0.1% HCl and 0.01% non-ionic detergent (Tween 80) solutions, rinsed with distilled water (Álvarez-Fernández et al., 2001) and dried in a forced air oven at 65°C for 3 days. Thereafter, samples were milled and calcined in a muffle furnace (480°C). The ashes were digested using 7M HNO3 Suprapour.
Soil soluble fraction was obtained by washing the soil with distilled water (600 mL) by stirring for 10 min on a rotary shaker at 90 min-1. An aliquot of 40 mL was centrifuged at 9,000 min-1 for 10 min (Sorvall Legend XFR, Thermo Fisher Scientific, United States), the supernatant was first filtered with ashless filters paper (Grade 1238, Filter Lab) and then, through syringe cellulose filters (0.45 μm) (OlimPeak, Teknokroma). Nitric acid (Suprapur, Merck) was added to achieve a 1% acid matrix.
Soil available fraction was obtained from the solid residue in the centrifuge tube by extraction for 20 min with 25 mL using Soltanpour and Schwab (1977) extractant (DTPA + ammonium bicarbonate). After that, the samples were filtered. The extraction was made in triplicate, the extracts joined in a single extract, and volume made up to 100.0 mL. An aliquot of 7.165 mL of 65% HNO3 was added to eliminate the excess of bicarbonate and to allow an acid media for the analytical determinations.
Isotope quantification in the plant organs and soil fractions (soluble and available) were determined by ICP-MS (7500c, Agilent Technologies, Santa Clara, CA, United States) using 57Fe standards and correcting Ca and Ar interferences by means of a collision cell quadrupole ICP-MS instrument.
The specific contribution of each iron fertilizer to the soil and plant nutrition was calculated by isotope pattern deconvolution analysis considering the two iron sources, with a modification of the method proposed by Rodríguez-Castrillón et al. (2008) In brief, the mass balance for the Fe natural isotope can be expressed as shown by matrix notation:
where each ATotal is the isotope abundance of each Fe isotope in the plant sample. AFer is the corresponding isotope abundance in the tracer, and ANat is the natural isotope abundance. Moreover, xFer and xNat denote the molar fractions of Fe in the isotopically altered sample arising from the two different sources of the element (fertilizer or natural). The best values of xNat and xFer are found by least-squares fitting of the error vector e (minimizing the square sum of errors) using the SOLVER tool in Excel®.
To evaluate the influence of Fe on leaf chlorophyll, the SPAD Index was measured every 2 or 3 days, using a Minolta Chlorophyll Meter SPAD-502 (Minolta, Osaka, Japan) after the first application of the Fe fertilizers.
Statistical Analysis
In order to verify the homogeneity of the data, the Levene test was used first, prior to testing the differences between Fe treatments for significance by one-way ANOVA. Means were compared using the Duncan multiple range test (P < 0.05). Results of two-way ANOVA are expressed as ns (not significant), ∗P < 0.05, ∗∗P < 0.01, and ∗∗∗P < 0.001. All the calculations were performed using SPSS v.24.0 software.
Results
Characterization of 57Fe-NFs
The main characterization tools used in this work were57Fe Mössbauer spectroscopy, XRD, EXAFS, and TEM. They were applied to identify the iron phases in the 57Fe-NFs and to estimate the particle size. According to the XRD data, the major crystalline phases in all three samples of 57Fe-NFs are mineral salts of potassium and sodium: nitrates in the product F, and sulfates in the products S and M, the collected data are shown in Supplementary Figure SM2. No iron-containing crystalline phases were observed in the obtained XRD patterns. The XRD data on the synthesized reference samples – ferrihydrite and goethite – are shown in Supplementary Figure SM3.
TEM data demonstrate that all 57Fe-NFs samples contain large low density particles typical for HSs. Inside of these particles the nano-sized contrast variations can be observed, which correspond to the NPs with sizes < 5 nm (Figures 1a,b). Some aggregates of ∼20 nm were observed in the product S (Figure 1c). These NPs can be clearly seen in the zero loss images of the products F and M (Supplementary Figure SM3). Figure 2 shows TEM images, Fe M-line EFTEM, EELS spectra and the scheme of EFTEM measurements for the F sample, which was of particular interest for this study, because its synthesis was specifically run under conditions favoring formation of ferrihydrite phase. The Fe M- and L-lines were clearly observed in the EELS spectrum of this sample (Figure 2). This confirmed the presence of iron in the region of interest. The spatial distribution of iron was investigated using three-point EFTEM, which yielded the pattern of iron-containing NPs (bright inclusions) in the darker matrix (HSs). The obtained results are indicative of the iron-containing composition of the formed NPs. In order to investigate the structures of the observed NPs, the SAED patterns were measured (Supplementary Figure SM6) and compared to the ED of the parent HS (L) and XRD pattern of the synthesized pure two-line ferrihydrite (Ferrihydrite). ED patterns of all iron containing samples were characterized with appearance of two additional reflexes as compared to the parent L sample (Figure 3). The smaller peak was observed at 0.15 nm, and the larger one – at 0.25–0.30 nm. Such a combination of reflexes is similar to the two-line ferrihydrite diffraction pattern. However, for refining this assignment additional information was desirable and obtained with the use of XANES and EXAFS spectroscopy.
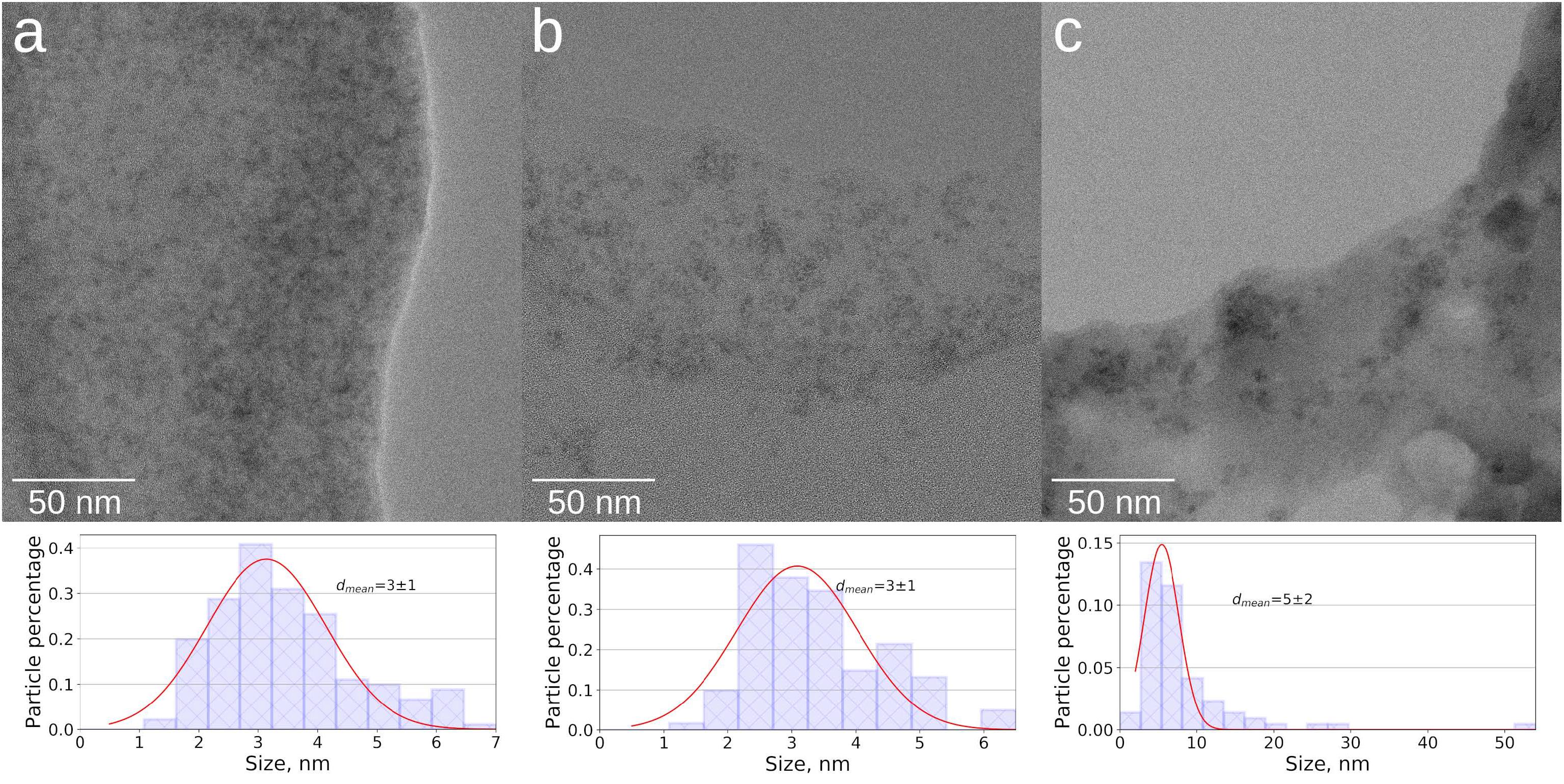
Figure 1. TEM images and size statistics for the three 57Fe-nanofertilizer samples under study: (a) F, (b) M, (c) S.
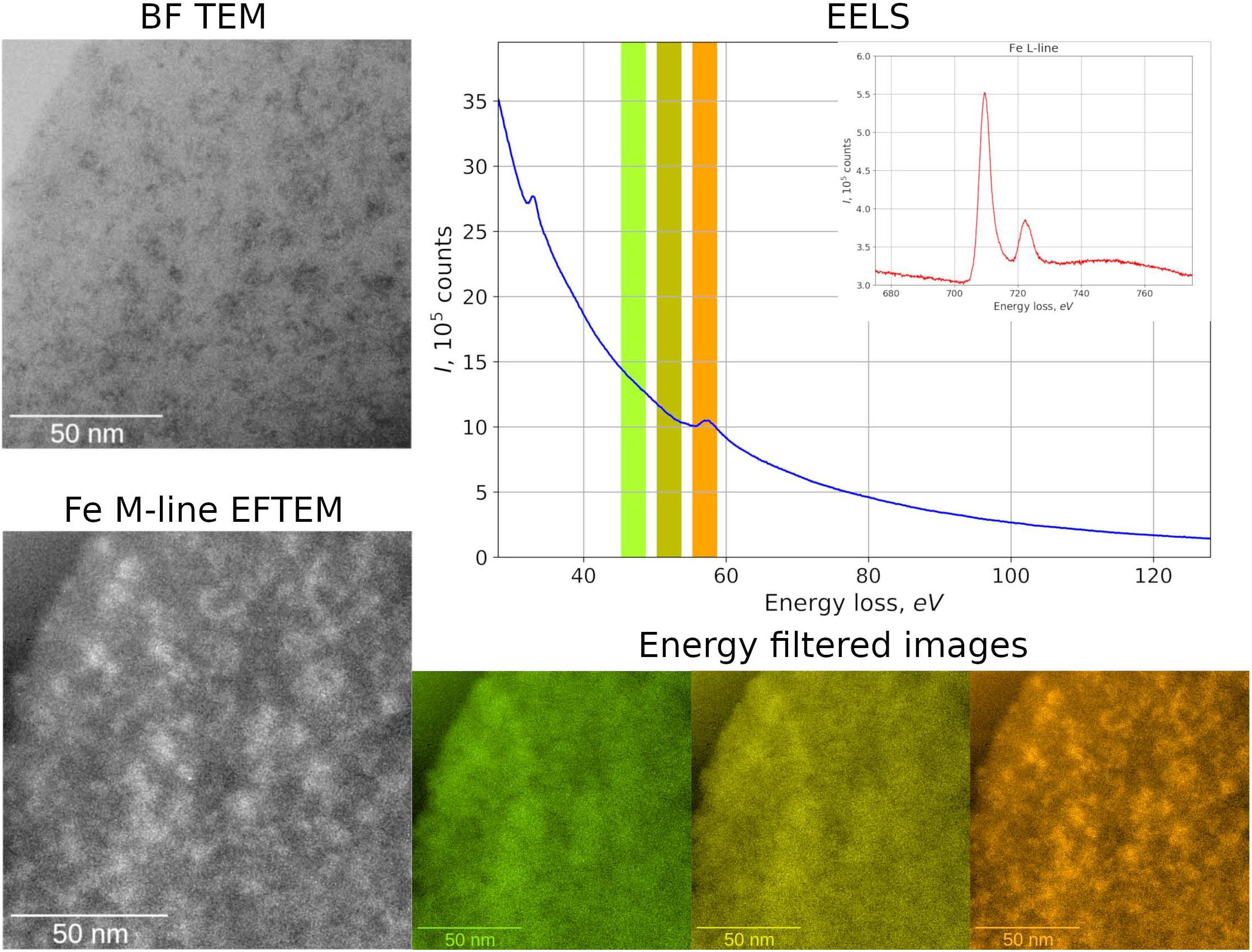
Figure 2. TEM, Fe M-line EFTEM, EELS spectra and the scheme of EFTEM measurements for the product F.
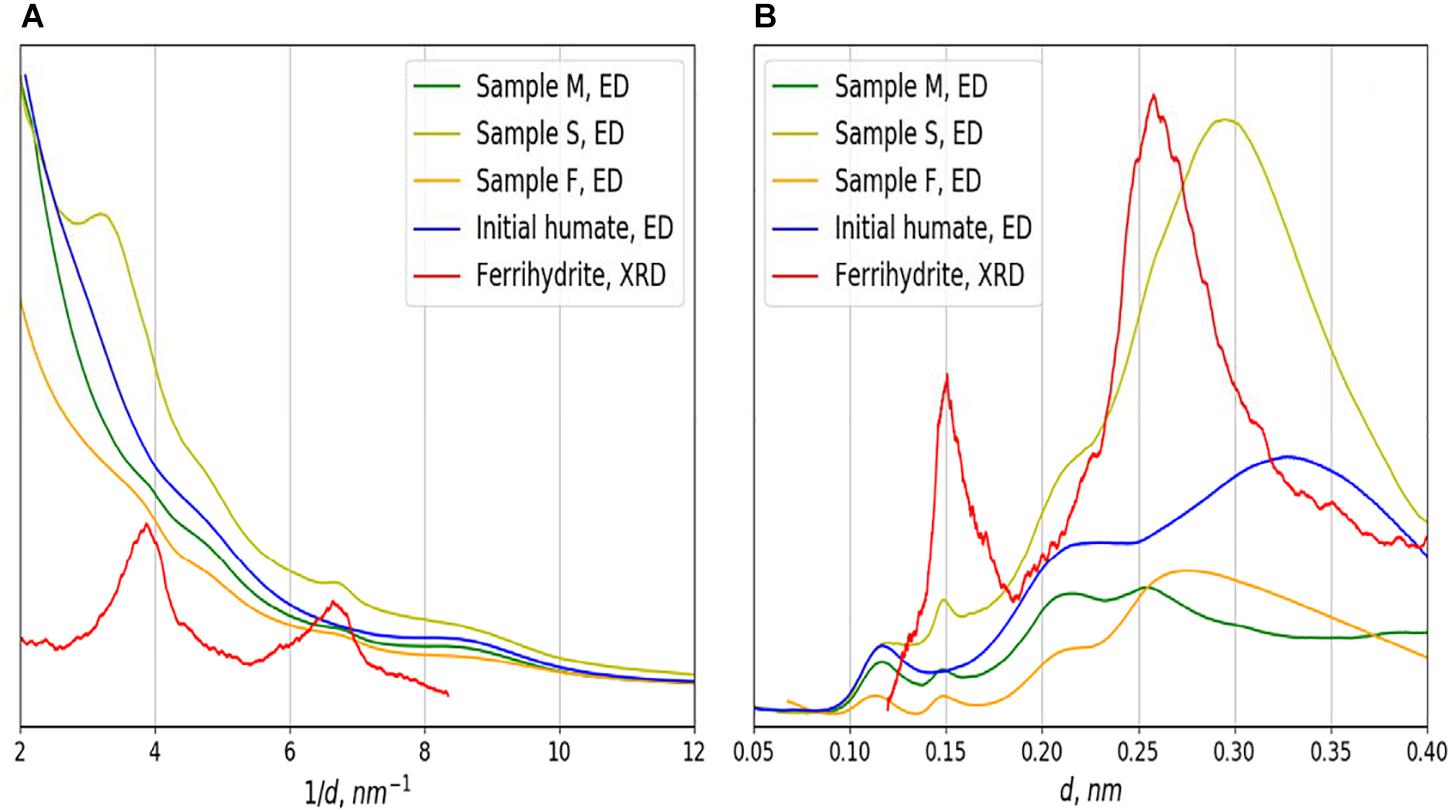
Figure 3. Results of ED integration in reciprocal space (A) and in direct space, after background removal (B) for the products F, M, S. and initial humate (L) in comparison with the XRD data for ferrihydrite.
The acquired XANES spectra of the samples obtained in this study are shown in Supplementary Figure SM7 and their first derivatives are presented in Figure 4A. The spectra obtained for all samples under study were rather similar and demonstrated the presence of ferric ions in octahedral coordination (Manceau and Gates, 1997). EXAFS spectra of the same samples (Figure 4B and Supplementary Figure SM8) were fitted to the two-shell model (Maillot et al., 2011). It assumes that the first shell contains two different oxygen positions (Fe-O), whereas the second one- three iron position (Fe–Fe) with the same value of σ2. The obtained results for ferrihydrite and goethite reference samples (Table 2) are in good agreement with the reported values for Fe–O and Fe–Fe distances (Maillot et al., 2011). For the samples under study, the values of Fe–Fe distances were very close to those of ferrihydrite (Table 2). For example, in the F sample (HS-ferrihydrite), they were 2.98 Å, 3.14 Å, and 3.53 Å, whereas in the reference sample – 2.91 Å, 3.06 Å, and 3.46 Å. These distances belong to face-sharing, edge-sharing, and corner-sharing octahedra in ferrihydrite, respectively (Manceau and Gates, 1997). Coordination number of iron at 2.91 Å and 3.06 Å for all three samples of the NFs are similar and indicative of ferrihydrite (Maillot et al., 2011). The high value of R-factor for the “S” sample results from the poorest quality of its approximation by the above two-shell model. This might be caused by heterogeneity of the S sample which contained different phases of iron (hydr)oxides. The iron in the parent humate resembles closely goethite phase. The differences in Fe–O distances between the reference ferrihydrite (1.82 Å and 1.97 Å) and the samples obtained in the presence of HS (1.94 Å and 2.11 Å) may be connected to very small size of the particles formed (<5 nm) and to respective surface effects. In the ideal ferrihydrite, the relation between tetrahedral Fe (IV Fe) and octahedral Fe (VI Fe) is 1:4. However, according to Michel et al. (2007), the size reduction of ferrihydrite particles leads to a decrease in IV Fe number. Moreover, Manceau and Gates (1997) demonstrated that 1.86Å Fe–O distance observed in the EXAFS spectra could not be directly assigned to the IV Fe due to the presence of short Fe–(O,OH) bonds on the surface. It means that the surface contamination and size reduction in the HS-stabilized ferrihydrite particles may lead to a decrease in a number of short Fe–O bonds as compared to the reference ferrihydrite. The applied EXAFS model involved only two Fe–O distances due to restriction on the amount of independent parameters, the most intense paths for each sample were chosen. The obtained data allow a conclusion that all three samples of the synthesized NFs were very similar with regard to the iron phase and contained iron (hydr)oxide with the polyhedral arrangement motif of the ferrihydrite.
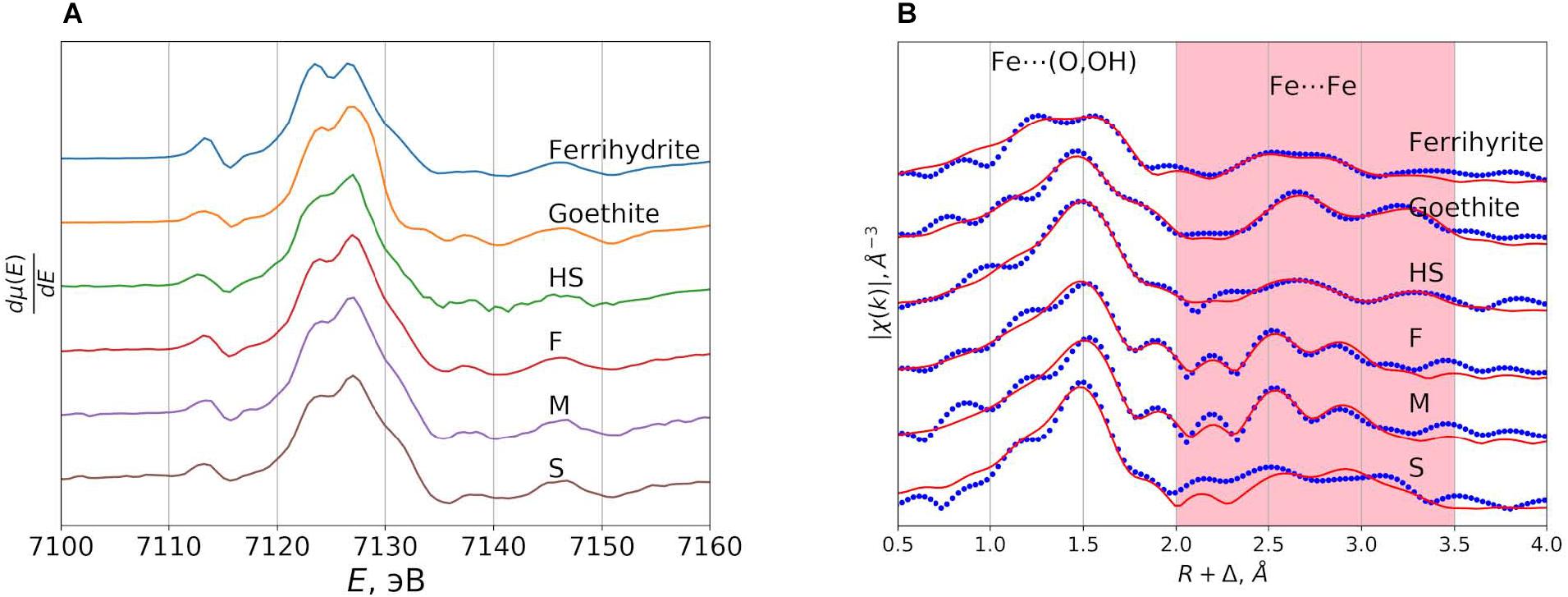
Figure 4. The first derivatives of the XANES spectra shown in Supplementary Figure SM7 (A) and the results of model approximation of the EXAFS spectra in R-space (B) for the 57Fe-labeled nanofertilizer samples under study.
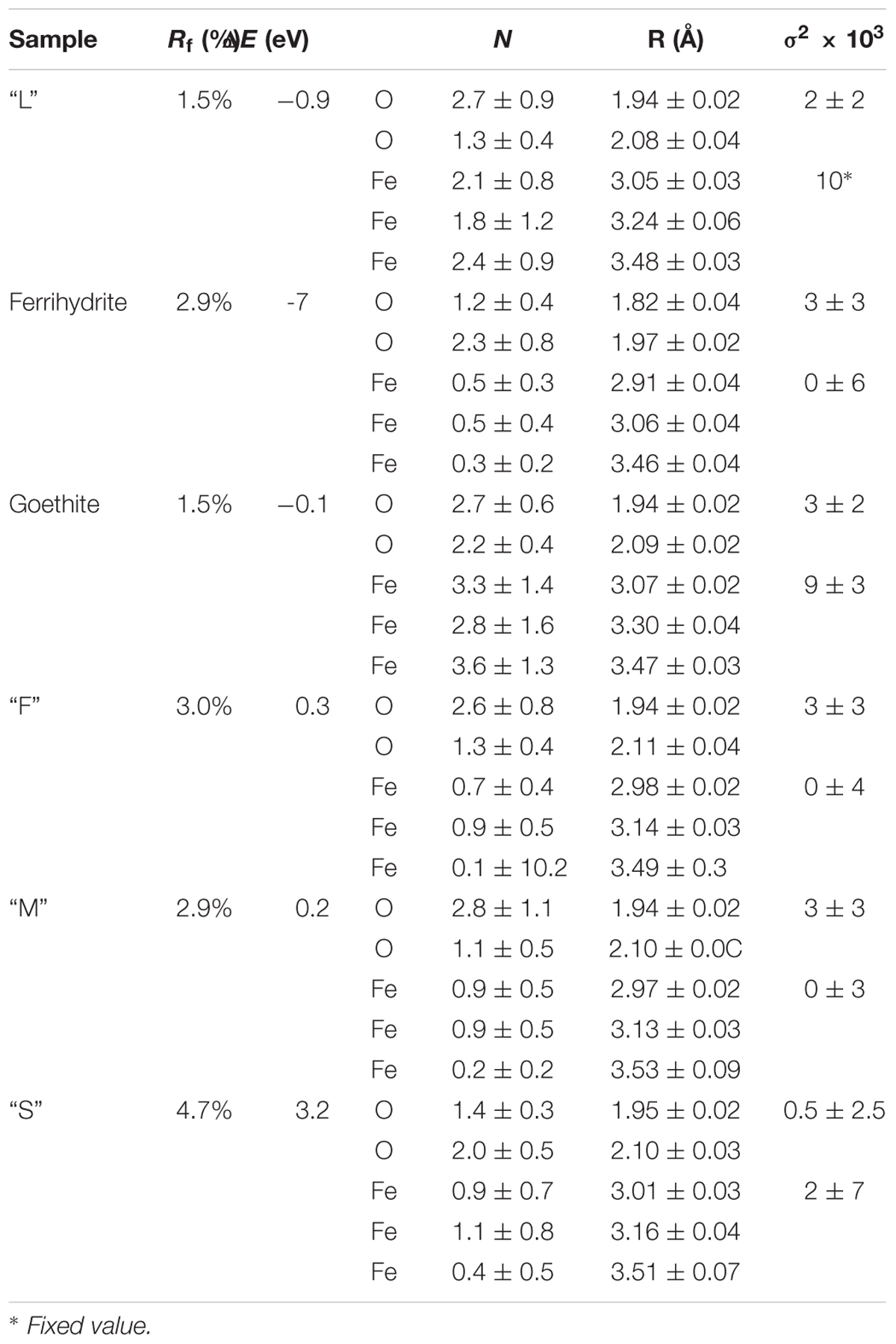
Table 2. The model parameters calculated from the EXAFS spectra for three 57Fe-NFs samples used in this study.
The results of Mössbauer spectroscopy investigation yielded additional support to this conclusion. The Mössbauer spectra of M and F samples recorded at room temperature and at liquid nitrogen showed broadened electric quadrupole doublets as it is demonstrated in Figure 5A on the example of M-sample. The Mössbauer spectra of F-sample is shown in Supplementary Figure SM11. This could be explained by the distribution of gradients of the electric field (Supplementary Figure SM10). For these samples, the distribution of quadrupole splittings has a bimodal character, similar to the distribution for high-temperature spectra of a reference sample of ferrihydrite (Supplementary Figure SM10). The small size of the particles under study and the low blocking temperature might be the reasons for a lack of substantial broadening of the resonance lines as well as the absence of a magnetically ordered fraction in the spectra at low temperature. Even for the reference sample of ferrihydrite with the larger particles as compared to the NPs stabilized by humate, the magnetic ordering (Supplementary Figure SM10) was not observed at the boiling point of liquid nitrogen (Schwertmann et al., 1999).
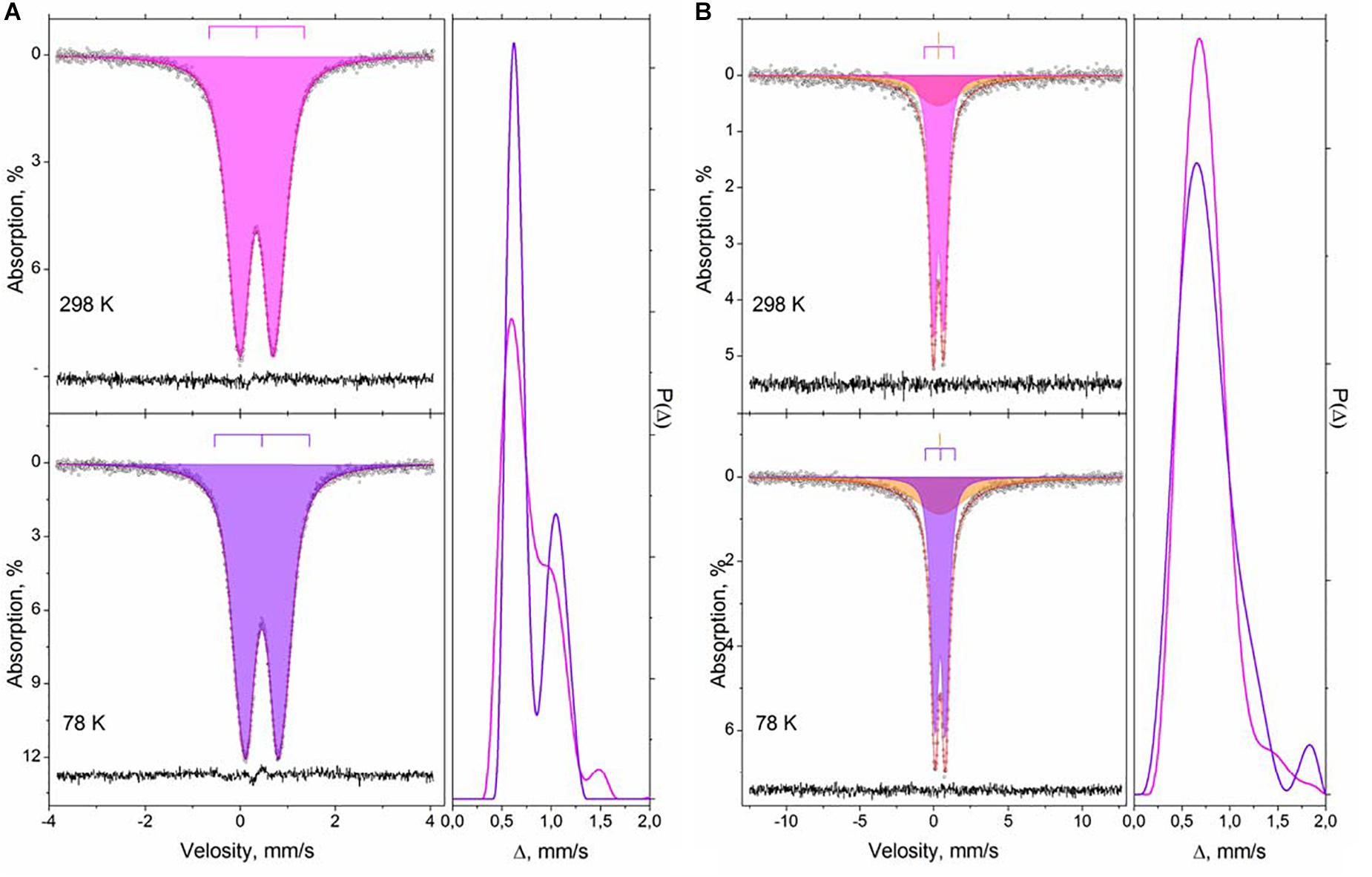
Figure 5. Mössbauer spectra of the M and S samples (A,B, respectively) recorded at 295K and 78K and the quadrupole splitting distributions for these spectra.
The spectra of S sample at these temperatures, in addition to very similar quadrupole doublets, contained extended absorption with low intensity, which can be conditionally described by a singlet line of a large width (Figure 5B). The relative area of extended absorption in the S sample did not change with temperature indicating that it is not related to superparamagnetism. This can be connected with weak spin–spin interactions between iron (III) atoms in strongly inhomogeneous and disordered medium. These interactions could be precisely observed because of the use of 57Fe (Pankratov et al., 2013). Distribution of quadrupole splitting in the S sample is unimodal and it has significantly larger dispersion as compared to those in the samples M and F. This might be indicative of the larger diversity of the local environments of iron atoms in the S sample. The further details on Mössbauer data can be found in the Supplementary Table SM1 and Supplementary text. In general, the data of Mössbauer spectroscopy are in good agreement with the data of TEM and X-ray spectroscopy.
Soil Pot Experiment
In order to evaluate the 57Fe-NFs effect in the plant growth, the SPAD index and the fresh weight of soybean shoots and roots at 48 DAF were measured and presented in Table 3. There was no leaf chlorosis observed and the plants presented SPAD indexes > 25, which is indicative of sufficient iron nutrition (Martín-Fernández et al., 2017b). The shoot biomass of the fertilized plants (in case of F3, S1, S2, S3, and M2) was larger as compared to FeEDDHA. There was no significant differences observed in the root biomass of the plants fertilized with the NFs under study (except for F3, which showed the largest fresh weight of roots). In our former studies, the plants fertilized with the leonardite humates accumulated slightly higher fresh weight than those fertilized with the iron chelate. According to Rose et al. (2014), the HSs generally increase the shoot and root growth by 15–25%. Canellas et al. (2015) reported that the growth response of monocotyledonous plants to exogenously applied HS is more sensitive as compared to for dicotyledonous plants, and the plant physiological responses to HS isolated from brown coal (e.g., lignite, leonardite, and subbituminous coals) are less than those observed in response to the addition of HS isolated from peat, composts or vermicomposts.
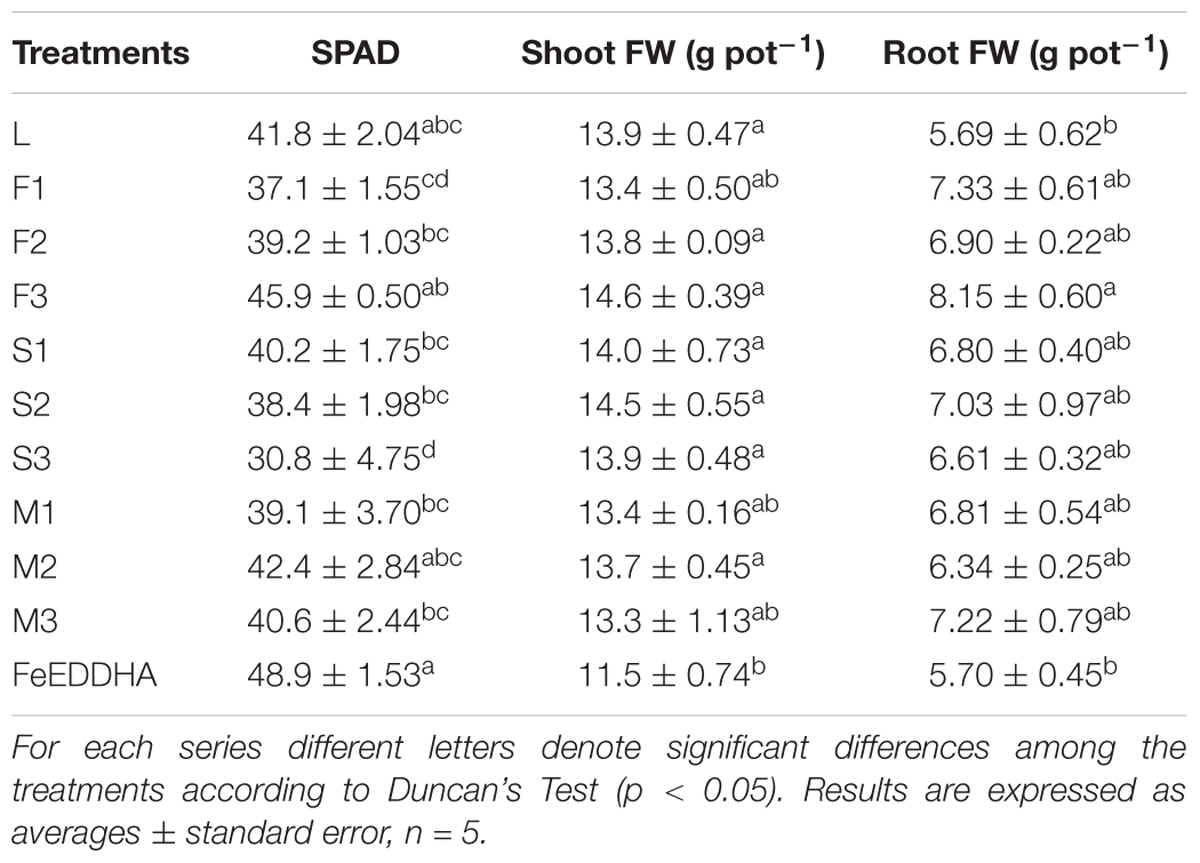
Table 3. SPAD index at the last level of trifoliate well developed soybean leaves and fresh weight (FW) of shoots and roots of soybean control (L) plants and plants fertilized with the 57Fe products F, S, and M in three doses: (1) 35, (2) 75, and (3) 150 μmol 57Fe pot-1 or 50 μmol 57FeEDDHA pot-1 at 48 DAF.
The 57Fe tracer technique allowed monitoring Fe from the fertilizer (FeFer) in the soil experiment and distinguishing it from the native Fe contained in the soil (FeNat). The FeTotal was calculated as the sum of FeFer and FeNat. A combination of 57Fe isotope and mathematical deconvolution was a relevant tool to evaluate the efficacy between different NFs to correct iron deficiency.
The contents of FeFer, FeNat, and FeTotal (μmol pot-1) in soybean shoots were calculated as the sum of the first (15 DAF) and second samplings (48 DAF) and presented in Figure 6. The effect of type and doses of the 57Fe-NFs on the contents of FeFer, FeNat and FeTotal in soybean shoots was studied by ANOVA two-way statistical analysis and presented in Table 4. Significant differences were observed between 57Fe-NFs types and doses. The product M provided the highest FeFer content to the soybean shoots mainly when the third dose was applied (150 μmol 57Fe pot-1). The content of FeTotal expressed as the sum of the FeFer and FeNat showed significant differences only between the doses, and the third dose seemed to be the most adequate. Table 5 presents the FeTotal (mg kg-1) concentration in soybean leaves at 15 and 48 DAF. It ranged from 36.8 mg kg-1 (F1) to 53.6 mg kg-1 (M1) at 15 DAF and from 34.6 mg kg-1 (S1) to 39.9 (S3) mg kg-1 at 48 DAF. According to the iron concentration obtained in a previous work (Rodríguez-Lucena et al., 2010) and the SPAD index detected (Table 4), the soybean plants are sufficiently iron nourished and did not present symptoms of iron chlorosis. Moreover, the iron concentration in leaves decreased for the second sampling because iron was a priority for the pods production, even for plants fertilized with 57FeEDDHA.
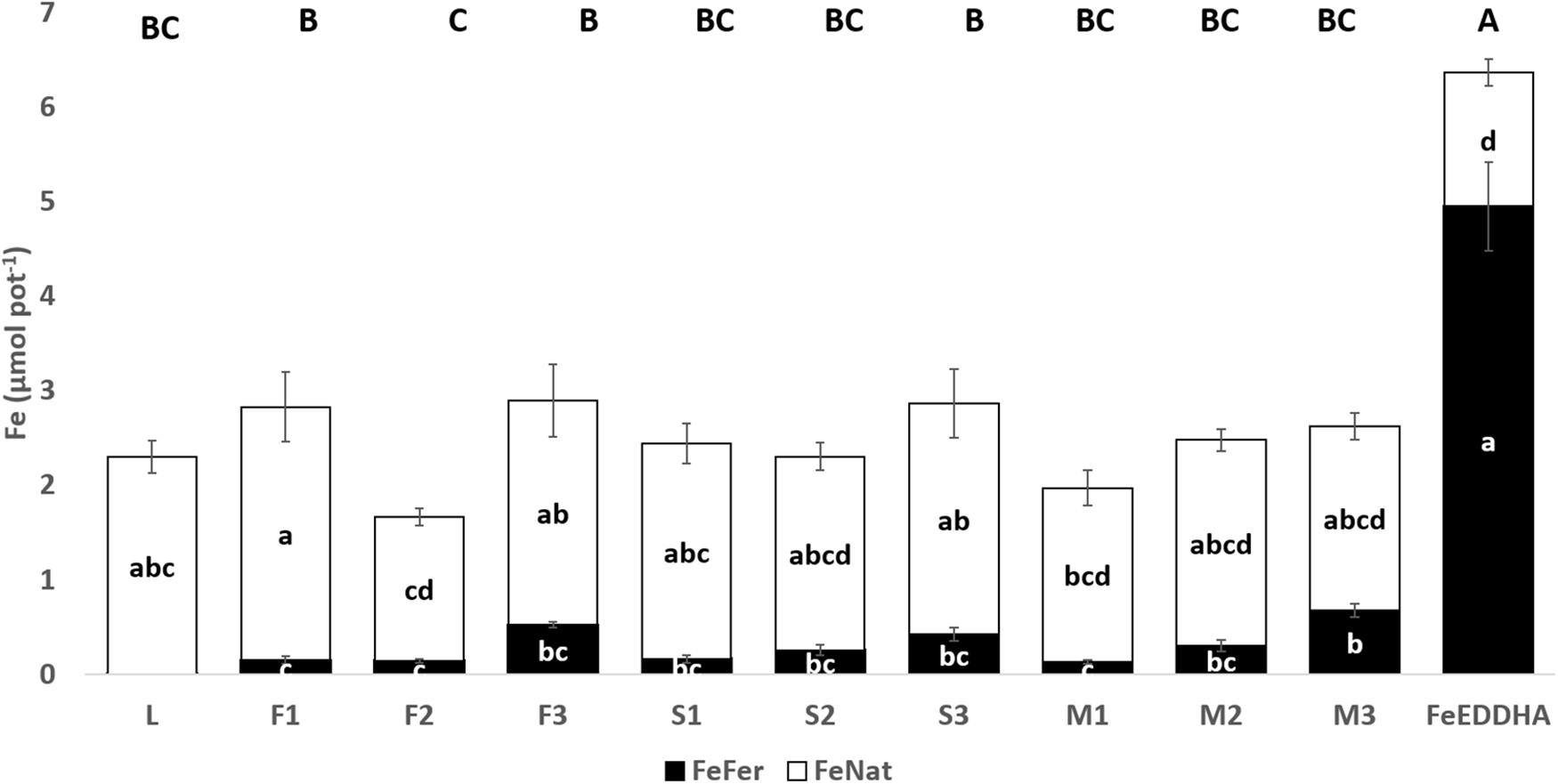
Figure 6. FeFer and FeNat (μmol pot-1) contents in soybean shoots of control plants (L) and plants fertilized with the 57Fe products F, S, and M in three doses: (1) 35, (2) 75, and (3) 150 μmol 57Fe pot-1 or 50 μmol 57FeEDDHA pot-1, calculated as the sum of the first (15 DAF) and second sampling (48 DAF). For each series different letters denote significant differences among the treatments according to Duncan’s Test (p < 0.05). Lowercase letters correspond to FeFer and FeNat and capital letters correspond to FeTotal statistical results. Results are expressed as averages ± standard error, n = 5.
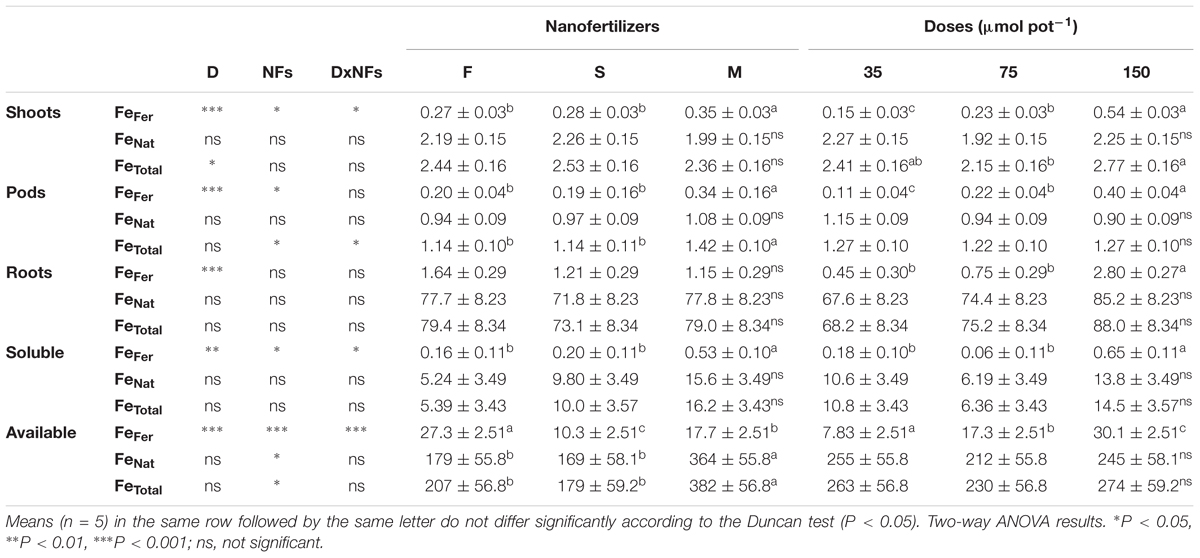
Table 4. Effect of doses (D) and nanofertilizers (NFs) related to the contents of FeFer, FeNat, and FeTotal (μmol pot-1) in soybean shoots, roots, pods, soluble and available soil fraction for soybean plants fertilized with the products F, S, and M with three doses (35, 75, and 150 μmol pot-1) at 48 DAF.
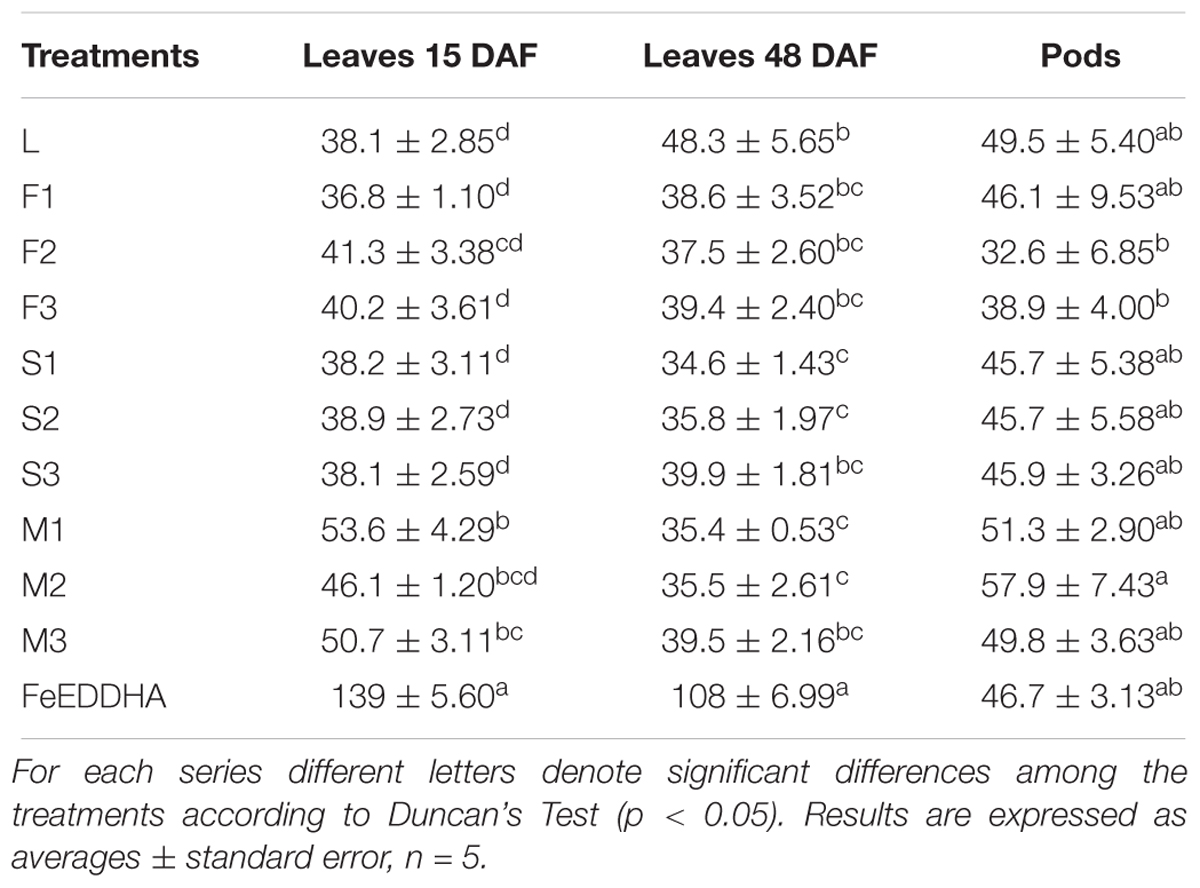
Table 5. FeTotal (mg kg-1) concentration in soybean leaves and pods of plants fertilized with the 57Fe products F, S, and M in three doses: (1) 35, (2) 75, and (3) 150 μmol 57Fe pot-1 or 50 μmol57FeEDDHA pot-1 at 15 and 48 DAF and soybean pods at 48 DAF.
Differences in FeFer uptake (nmol plant-1) in soybean leaves between the first (15 DAF) and the second samplings (48 DAF) were calculated and plotted in Figure 7. The plants fertilized with the 57Fe-NFs, nominally, with S2, M2 and F3, have taken up 93, 88, and 70 nmol FeFer plant-1 in leaves in 33 days, whereas the plants fertilized with FeEDDHA stopped providing FeFer to the leaves after the first sampling. The products S and M, prepared from 57Fe2(SO4)3, at their second dose (75 μmol 57Fe pot-1) and the product F, prepared from 57Fe(NO3)3 at the third dose (150 μmol 57Fe pot-1) showed the highest FeFer increase between sampling times. These results are consistent with our previous data (Cieschi et al., 2017) on fertilization with iron leonardite humate which sustained slow and increasing iron nutrition to citrus growth under conditions of calcareous soil and yielded results similar to FeEDDHA with regard to efficacy of iron deficiency correction during the first year of application.
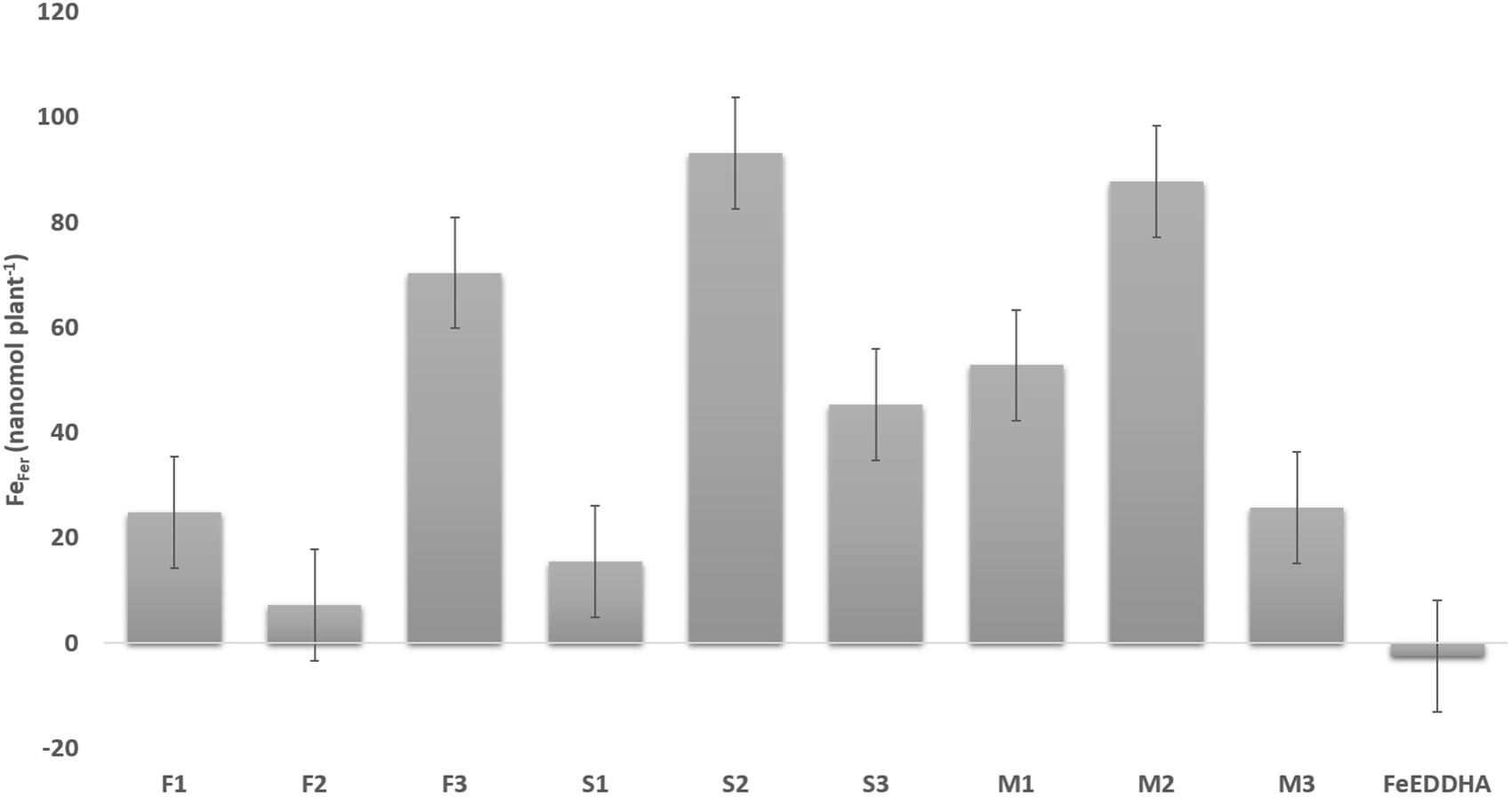
Figure 7. Differences in FeFer uptake (%) in soybean leaves of plants fertilized with the 57Fe products F, S, and M in three doses: (1) 35, (2) 75, and (3) 150 μmol 57Fe pot-1 or 50 μmol 57FeEDDHA pot-1, between the first (15 DAF) and the second sampling (48 DAF). Results are expressed as averages ± standard error, n = 5.
Of particular interest are the contents of FeFer, FeNat and FeTotal (μmol pot-1) in soybean pods at 48 DAF, which are presented in Figure 8. The FeFer content in pods increased along with an increase in the dose of 57Fe-NFs. The significant differences were observed between the doses and the 57Fe-NF type when the obtained results were compared using ANOVA two-way statistical analysis (Table 4). As in case of the shoots, the product M provided the higher content of FeFer as compared to the other two 57Fe-NFs to the soybean pods and the third dose was the most efficient. Moreover, the similar Feotal contents in soybean pods were observed for the plants treated with 57Fe-NFs (except for F2) or FeEDDHA, and the product M was the most efficient in providing FeTotal to the soybean pods regardless of the dose applied. Table 5 shows the FeTotal concentration (mg kg-1) in soybean pods at 48 DAF. It ranged from 32.6 mg kg-1 (F2) to 57.8 mg kg-1 (M2) for the plants fertilized with 57Fe-NFs. According to Römheld and Nikolic (2007), the accumulation of total iron in pods for soybean plants reaches 50 mg kg-1 under conditions of sufficient nourishment. Nadal et al. (2012) and Martín-Fernández et al. (2017a) have observed 57Fe in soybean fruit of plants fertilized with o,oEDDHA/57Fe3+ and HBED/57Fe3+. Still, this study is the first one when 57Fe supplied by iron humates was detected in soybean pods. Shoots of plants fertilized with FeEDDHA showed the highest FeFer, the lowest FeNat and the highest FeTotal contents. The pods showed similar results for FeFer and FeNat contents.
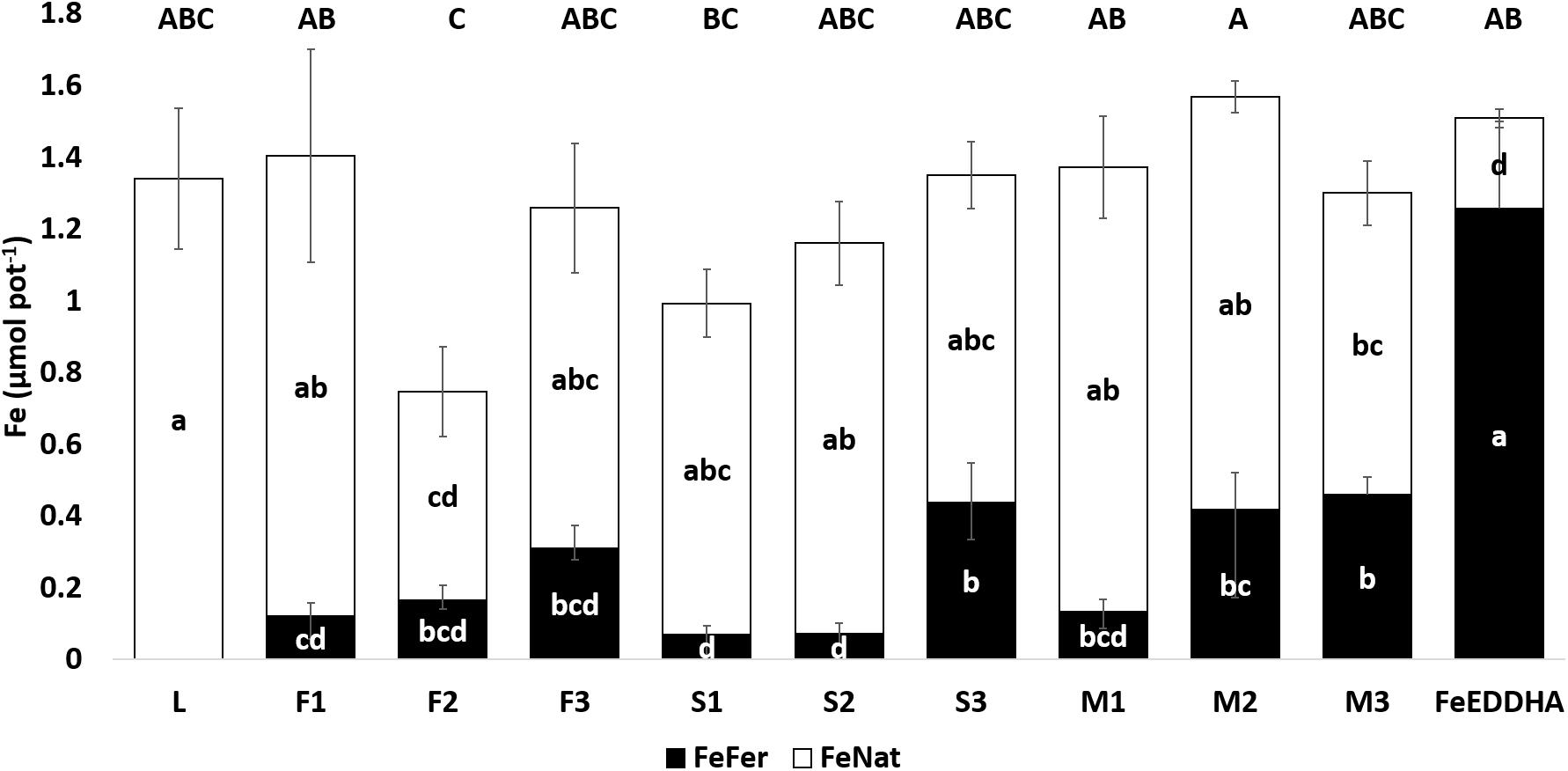
Figure 8. FeFer and FeNat (μmol pot-1) contents in soybean pods of control plants (L) and plants fertilized with the 57Fe products F, S, and M in three doses: (1) 35, (2) 75, and (3) 150 μmol 57Fe pot-1 or 50 μmol 57FeEDDHA pot-1 at 48 DAF. For each series different letters denote significant differences among the treatments according to Duncan’s Test (p < 0.05). Lowercase letters correspond to FeFer and FeNat and capital letters correspond to FeTotal statistical results. Results are expressed as averages ± standard error, n = 5.
The content of FeFer (μmol pot-1) in soybean roots as well as the contents of soluble and available soil fractions at 48 DAF are presented in Figure 9. In general, an increase in the content of FeFer in soybean roots was observed when the plants were treated with the 57Fe-NFs (Figure 9A). The significant differences between the doses were confirmed by the ANOVA two-way statistical analysis (Table 4). The third dose was the most prone to store FeFer in roots, in particular, in case of the F sample.
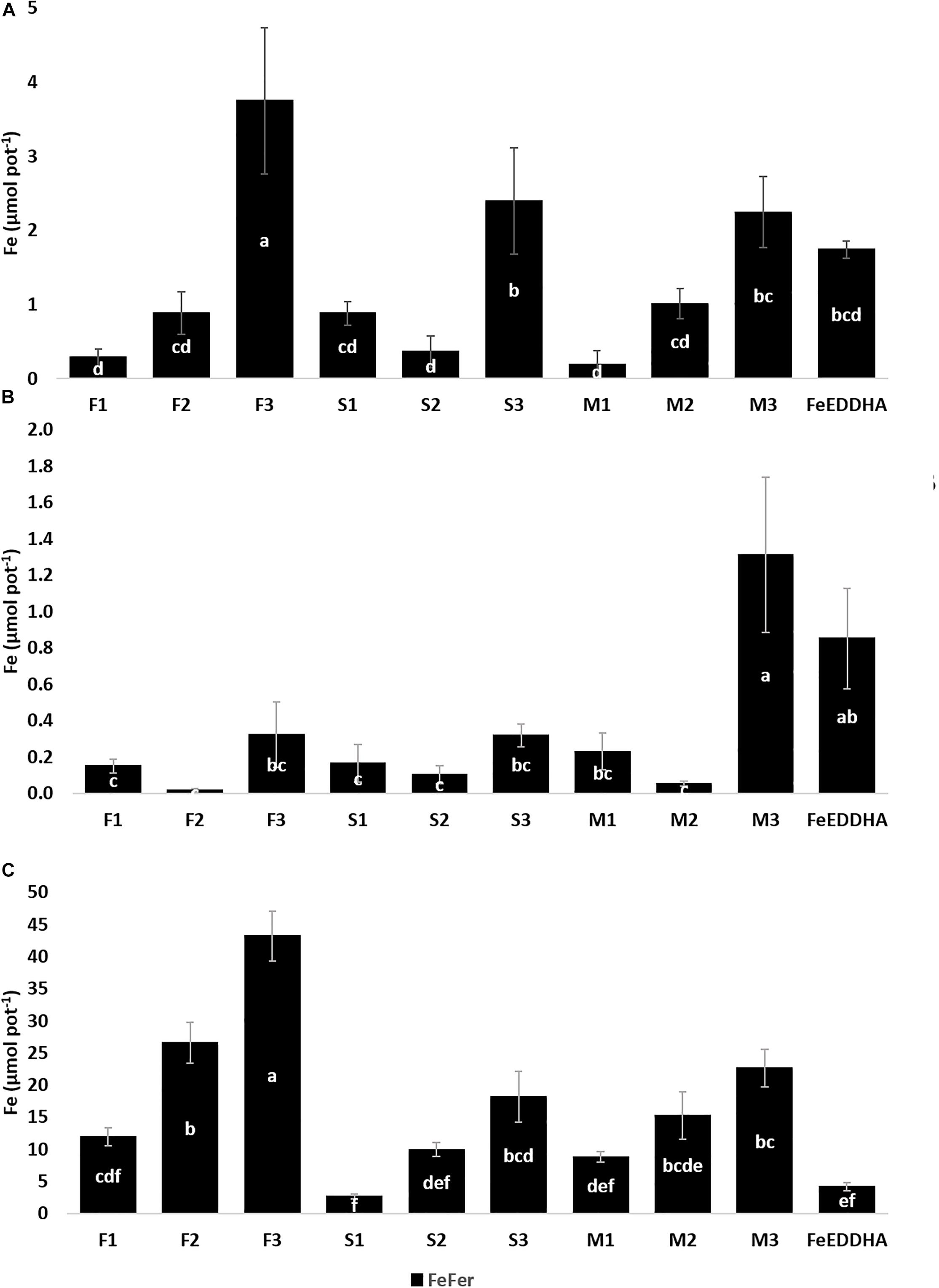
Figure 9. FeFer (μmol pot-1) content in soybean roots of plants fertilized with the 57Fe products F, S, and M in three doses: (1) 35, (2) 75, and (3) 150 μmol 57Fe pot-1 or 50 μmol 57FeEDDHA pot-1 (A), soluble soil fraction (B), and available soil fraction (C) at 48 DAF. For each series different letters denote significant differences among the treatments according to Duncan’s Test (p < 0.05). Results are expressed as averages ± standard error, n = 5.
The FeFer content in the soluble soil fraction was increasing along with the dose of the 57Fe-NFs. The results obtained for F3, S3, M3, and FeEDDHA were similar, though the highest FeFer content was observed in the pots treated with M3 (Figure 9B). The ANOVA two-way statistical analysis has confirmed these results when the effect of the 57Fe-NF type and doses were compared (Table 4). Relating to the FeFer content in the available soil fraction, the pots fertilized with the product F differed substantially from the others, in particular, in case of the third dose (Figure 9C and Table 4). In general, the FeFer from the product F at the highest dose (150 μmol 57Fe pot-1) remained mostly available in soil (Figure 9C) or in the roots (Figure 9A).
Figure 10 shows the 57Fe (%) distribution in soybean plants (shoots, pods and roots) of plants fertilized with the 57Fe products F, S and M in three doses (35, 75 and 150 μmol 57Fe pot-1) and in the soil (soluble and available fraction). In general, the 57Fe-NFs remained in soil and ranged from 80% (S1) to 95% (F1), mainly in the available soil fraction. With respect to the plant, the highest percentage of 57Fe was detected in plants fertilized with S1 (18%), but mainly in roots. The 57Fe content in shoots increased along with the dose for plants fertilized with F and M.
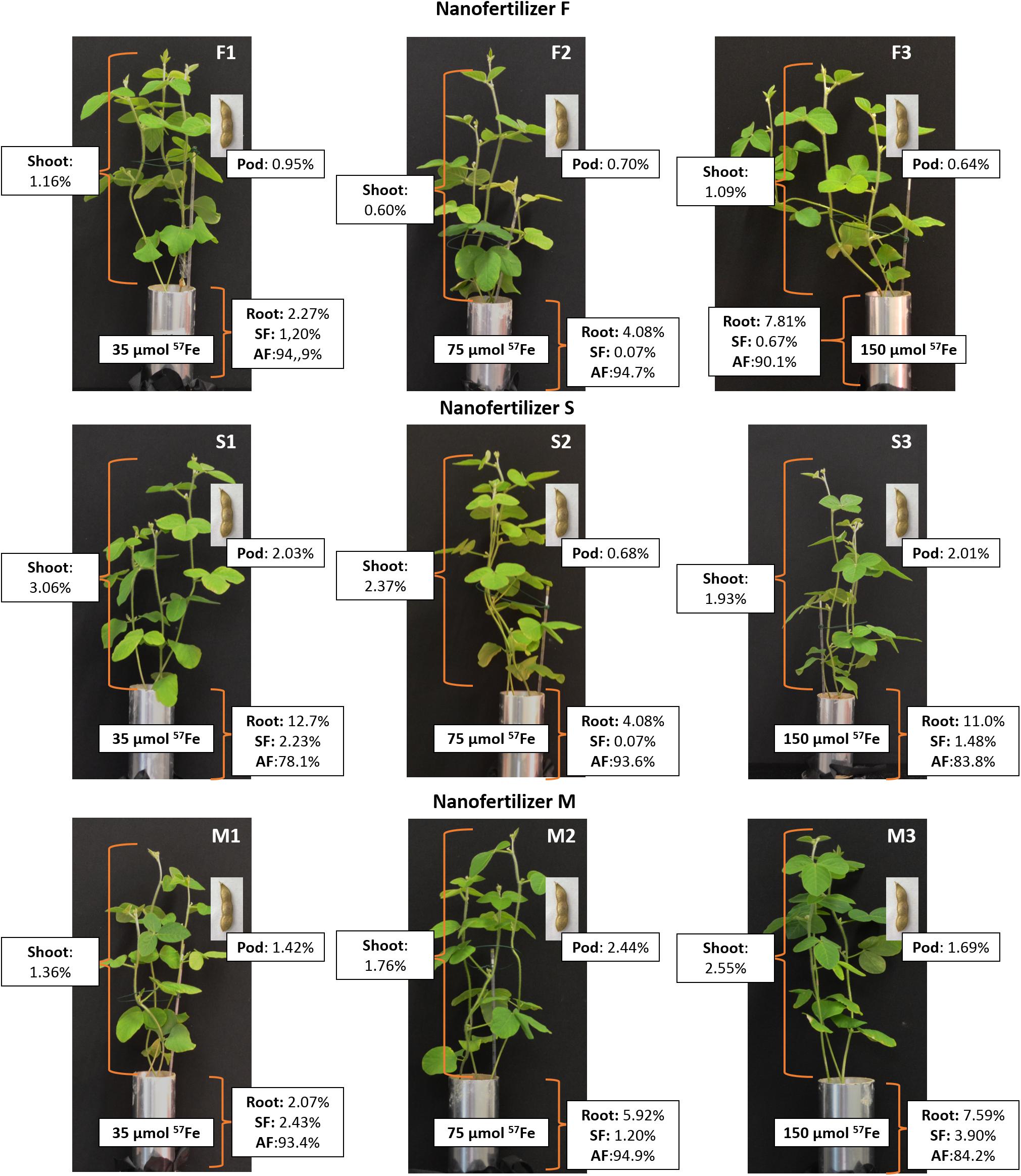
Figure 10. Distribution of 57Fe (%) in soybean shoots, pods and roots of plants fertilized with the 57Fe products F, S, and M in three doses: (1) 35, (2) 75, and (3) 150 μmol 57Fe pot-1 and in the soluble and available fraction soil.
Discussion
In our work, three 57Fe-NFs (F, M, and S) were obtained and exhaustively characterized by XRD, TEM with ED, EELS and EFTEM, X-ray absorption spectroscopy (XANES and EXAFS) and Mössbauer spectroscopy. There were similarities among them with regard to the iron phase and iron (hydr)oxide content with the polyhedral arrangement motif of the ferrihydrite. Most agricultural soils contain natural ferrihydrite NPs, which may contribute to iron nutrition of plants. Many authors (Römheld and Nikolic, 2007; Colombo et al., 2012; Cieschi et al., 2017) reported ferrihydrite formation during the iron humate synthesis, they characterized and studied the relationship between the particle size, pH and stability. Angelico et al. (2014) and Colombo et al. (2015) have shown that the phase of iron (hydr)oxide formed in the presence of HS depends on pH, oxidation rate, and Fe:HS ratio.
Our pot experiments revealed that the 57Fe-NFs were capable of supplying 57Fe to the plants and it was transported from root to shoot and reached the pods (Figures 6, 8, 9A). In particular, we have observed that the plants fertilized with the product M presented the highest contents of 57Fe in shoots, pods and the soil soluble fraction, according to the two-way ANOVA statistical analysis (Table 4). This iron humate was prepared taking into account its maximum complexing capacity in order to avoid the iron flocculation in calcareous conditions. Then, the Fe:HS ratio obtained after the synthesis was the lowest (0.12 g Fe g org. C-1) which suggested that the high content of HS has stabilized the poorly ordered 57Fe structures entrapped into humic matrix and favored the iron uptake by the soybean plants. Similar results were obtained by Kulikova et al. (2017) for their product Fe-HA (4% Fe, 68% HA) which was tested with wheat plants grown under hydroponic conditions.
In soil, the 57Fe-NFs presented an increasing tendency to remain available to the plant requirements for the different growth stages (Figure 9C). In addition, the slow and continuous iron release from these NFs has confirmed their long-term effect in providing iron in calcareous conditions in contrast to the short-term effect of the iron synthetic chelate (Figure 7), reported in the previous studies (Cieschi et al., 2017). Moreover, in a recent hydroponic assay (Cieschi and Lucena, 2018), plants fertilized with FeEDDHA presented the highest Fe contents in roots after 10 days but at longer term exposition (60 days) of the plants treated with iron humates yielded iron uptake similar to the plants fertilized with the iron synthetic chelate. We hypothesized that an increase in iron humate concentration in the rhizosphere might cause a decrease in the transcription level of the genes involved in the iron transport and shoot growth, and so the iron transport from root to shoot decelerated. Several authors (Aguirre et al., 2009; Tomasi et al., 2013; Olaetxea et al., 2015; Zamboni et al., 2016) suggested that the efficiency of the root transcriptional response to Fe supply depends on the nature (physicochemical characteristics) of the ligand and its capability to activate Fe uptake mechanisms and translocations. In particular, Zamboni et al. (2016) demonstrated that Fe complexed to water-extractable HSs from peat did not cause relevant changes in the root transcriptome of tomato plants with respect to Fe-deficient plants. However, Aguirre et al. (2009) observed that high doses of a purified humic acid from leonardite applied to cucumber plants promoted the upregulation of CsFRO1 and CsIRT1 gene expression for 48 and 72 h while these genes were downregulated for 96 h. The authors suggested that it may be associated to root iron accumulation and/or iron translocation. Olaetxea et al. (2018) proposed that it is very likely that the action of HS on plant mineral nutrition involves a coordinated functional crosstalk between indirect and direct HS effects on the soil–plant system. Soil and, in particular, the rhizosphere are extremely complex environments with a large degree of heterogeneity down to the nanoscale where the interactions between soil constituents, plant roots, and microorganisms take place (Mimmo et al., 2014). Thus, the long-term effect would be an expected result.
With respect to the uptake of nanoparticles by plants, Kulikova et al. (2014) have observed that particles of HA were transferred from root to shoot of wheat seedlings through the plant vascular system and Nardi et al. (2002) have previously observed the same mechanism for low molecular weight fraction of HA (<2.5 kDa). Furthermore, Pariona et al. (2016) have recently detected by 3D microscopic techniques, clusters of hematite and ferrihydrite NPs in endodermis, xylem, phloem vessels, and cell walls of the xylem vessels of maize stems of plants grown in hydroponic conditions. The extensive studies of R. Pinton and S. Cesco’s group (Cesco et al., 2000, 2002; Nikolic et al., 2003; Colombo et al., 2012, 2014; Tomasi et al., 2013; Mimmo et al., 2014) modeled variety of interactions in soils of iron humate complexes according to the molecular weight of HS. They have demonstrated that low-molecular weight HS can form soluble complexes of Fe and move toward the root, acting as natural substrates for the membrane Fe (III)-chelate reductase and stimulate the proton release promoting the Fe acquisition for Strategy I plants. Recently, Homonnay et al. (2016) have carried out a preliminary study about iron nanoparticles in plant nutrition and hypothesized that it would be possible to consider for plant nutrition supply in the soil iron-based oxide or oxy-hydroxide nanoparticles since storage of iron in cells usually implies formation of ferritin which has a certain similarity with ferrihydrite/ferric hydrous oxide nanoparticles with variable amounts of phosphate.
Further research is needed to redesign the classical model of the iron uptake by plant with more studies that consider uptake from the Fe-NPs.
Conclusion
According to Lal (2008), in the context of sustainable agriculture, applying innovative nanotechnology in agriculture is regarded as one of the promising approaches to significantly increase crop production. The Fe-NFs can be considered as a part of a novel technology in line with the precision and sustainable agriculture. They are iron-natural complex NPs synthetized from leonardite and they contain ferrihydrite in their structures which was properly and widely characterized. Moreover, the 57Fe-NFs used in this paper are capable of supplying Fe to the plants, transport it from root to shoot and reach the soybean pods. The slow and continuous iron release of these 57Fe-NFs confirms their long-term effect in providing iron in calcareous conditions while in soil, they tends to remain available to the plant requirements for the different growth stages.
Although further research is needed about the contribution of iron nanoparticles in plant nutrition, the Fe-NFs offers a natural, low cost and environmental option to the traditional iron fertilization in calcareous soils.
Author Contributions
MC: synthesis of 57Fe-NFs, carried out of the soil experiment, data processing, and manuscript preparation. AP: synthesis of 57Fe-NFs and manuscript preparation. VL: XRD, TEM, and EXAFS data processing. DV: ICP AES analysis of 57Fe-NFs samples. DP: Mössbauer spectroscopy. AV: EXAFS spectroscopy. IP: design and supervision of the synthesis and characterization of the 57Fe-NFs, data interpretation, and manuscript preparation. JL: design and supervision of the soil experiment, data interpretation, and manuscript preparation.
Funding
The Russian Science Foundation (16-14-00167), the Russian Foundation for Basic Research (18-29-25065), and the Spanish Ministry of Science and Innovation (AGL2013-44474-R) have financially supported this research.
Conflict of Interest Statement
The authors declare that the research was conducted in the absence of any commercial or financial relationships that could be construed as a potential conflict of interest.
Acknowledgments
The authors would like to acknowledge generous help and professional assistance of Dr. Oleg Schlaykhtin with freeze-drying the samples of NFs. MC expresses acknowledgment to Erasmus+ International Credit Mobility Program Alliance 4 Universities (A-4U) KA-107 for an academic stay at the Lomonosov MSU devoted to synthesis of 57Fe-labeled NFs. JL and MC acknowledge the financial support of the Spanish Ministry of Science and Innovation (grant AGL2013-44474-R). JL and MC also acknowledge to the Comunidad de Madrid (Spain) and Structural Funds 2014-2020 (ERDF and ESF) for the financial support (project AGRISOST-CM S2018/BAA-4330). AP, VL, DV, and IP acknowledge financial support of the Russian Science Foundation (grant # 16-14-00167) in part of the ICP-OES, XRD, TEM, and EFTEM measurements. VL and IP acknowledge support of Russian Foundation for Basic Research project 18-29-25065 for EXAFS measurements and interpretations of the obtained samples of 57Fe-labeled NFs. The use of the Carl Zeiss Libra 200MC TEM is supported by Lomonosov Moscow State University Program of Development. XAS were measured at the unique scientific facility Kurchatov Synchrotron Radiation Source supported by the Ministry of Education and Science of the Russian Federation (project code RFMEFI61917X0007).
Supplementary Material
The Supplementary Material for this article can be found online at: https://www.frontiersin.org/articles/10.3389/fpls.2019.00413/full#supplementary-material
Abbreviations
57Fe-NFs, iron-humic nanofertilizers isotopically labeled; ANOVA, analysis of variance; DAF, days after fertilizers applications; DTPA, diethylenetriaminepentaacetic acid; E.C., electrical conductivity; ED, electron diffraction; EELS, electron energy loss spectra; EXAFS, extended X-ray absorption fine structure spectroscopy; Fe-MCC, iron-maximum complexing capacity; Fe-Nps, iron nanoparticles; FeHBED, iron (III) N,N’-bis(o-hydroxybenzyl)-ethylenediamine-N,N’-diacetic acid complex; HEPES, N-(2-hydroxyethyl) piperazine N’-(2-ethanesulfonic acid); HS, humic substances; ICP-OES, inductively coupled plasma – optical emission spectrometry; ICP-MS, inductively coupled plasma mass spectrometry; L, leonardite potassium humate; NFs, nanofertilizers; o-oEDDHA, ethylenediamine-di (o-o hydroxyphenylacetic acid); OM, soil organic matter; SAED, selected area electron diffraction; SPAD, soil–plant analysis development; TEM, transmission electron microscopy; XANES, X-ray absorption near edge structure; XAS, X-ray absorption spectra; XRD, X-ray diffraction.
References
Aguirre, E., Leménager, D., Bacaicoa, E., Fuentes, M., Baigorri, R., Zamarreño, A. M., et al. (2009). The root application of a purified leonardite humic acid modifies the transcriptional regulation of the main physiological root responses to Fe deficiency in Fe-sufficient cucumber plants. Plant Physiol. Biochem. 47, 215–223. doi: 10.1016/j.plaphy.2008.11.013
Álvarez-Fernández, A., Pérez-Sanz, A., and Lucena, J. J. (2001). Evaluation of effect of washing procedures on mineral analysis of orange and peach leaves sprayed with seaweed extracts enriched with iron. Commun. Soil Sci. Plant Anal. 32, 157–170. doi: 10.1081/CSS-100103000
Angelico, R., Ceglie, A., He, J. Z., Liu, Y. R., Palumbo, G., and Colombo, C. (2014). Particle size, charge and colloidal stability of humic acids coprecipitated with ferrihydrite. Chemosphere 99, 239–247. doi: 10.1016/j.chemosphere.2013.10.092
Benedicto, A., Hernandez-Apaolaza, L., Rivas, I., and Lucena, J. J. (2011). Determination of 67Zn distribution in navy bean (Phaseolus vulgaris L.) after foliar application of 67Zn-lignosulfonates using isotope pattern deconvolution. J. Agric. Food. Chem. 59, 8829–8838. doi: 10.1021/jf2002574
Bolon, Y. T., Joseph, B., Cannon, S. B., Graham, M. A., Diers, B. W., Farmer, A. D., et al. (2010). Complementary genetic and genomic approaches help characerize the linkage group I seed protein QTL. BMC Plant Biol. 10:41. doi: 10.1186/1471-2229-10-41
Briat, J. F., Dubos, C., and Gaymard, F. (2015). Iron nutrition, biomass production, and plant product quality. Trends Plant Sci. 20, 33–40. doi: 10.1016/j.tplants.2014.07.005
Cakmak, I. (2002). Plant nutrition research: priorities to meet human needs for food in sustainable ways. Plant Soil 247, 3–24. doi: 10.1007/978-94-017-2789-1_1
Canellas, L. P., Olivares, F. L., Aguiar, N. O., Jones, D. L., Nebbioso, A., Mazzei, P., et al. (2015). Humic and fulvic acids as biostimulants in horticulture. Sci. Hortic. 196, 15–27. doi: 10.1016/j.scienta.2015.09.013
Cesco, S., Nikolic, M., Römheld, V., Varanini, Z., and Pinton, R. (2002). Uptake of 59Fe from soluble 59Fe-humate complexes by cucumber and barley plants. Plant Soil 241, 121–128. doi: 10.1023/A:1016061003397
Cesco, S., Römheld, V., Varanini, Z., and Pinton, R. (2000). Solubilization of iron by water-extractable humic substances. J. Plant Nutr. Soil Sci. 163, 285–290. doi: 10.1002/1522-2624(200006)163:3<285::AID-JPLN285>3.0.CO;2-Z
Cieschi, M. T., Caballero-Molada, M., Menéndez, N., Naranjo, M. A., and Lucena, J. J. (2017). Long-term effect of a leonardite iron humate improving Fe nutrition as revealed in silico, in vivo, and in field experiments. J. Agric. Food Chem. 65, 6554–6563. doi: 10.1021/acs.jafc.7b01804
Cieschi, M. T., and Lucena, J. J. (2018). Iron and humic acid accumulation on soybean roots fertilized with leonardite iron humates under calcarous conditions. J. Agric. Food Chem. 66, 13386–13396. doi: 10.1021/acs.jafc.8b04021
Colombo, C., Palumbo, G., He, J. Z., Pinton, R., and Cesco, S. (2014). Review on iron availability in soil: interaction of Fe minerals, plants, and microbes. J. Soils Sediments 14, 538–548. doi: 10.1007/s11368-013-0814-z
Colombo, C., Palumbo, G., Sellitto, V. M., Cho, H. G., Amalfitano, C., and Adamo, P. (2015). Stability of coprecipitated natural humic acid and ferrous iron under oxidative conditions. J. Geochem. Explor. 151, 50–56. doi: 10.1016/j.gexplo.2015.01.003
Colombo, C., Palumbo, G., Sellitto, V. M., Rizzardo, C., Tomasi, N., Pinton, R., et al. (2012). Characteristics of insoluble, high molecular weight iron-humic substances used as plant iron sources. Soil Sci. Soc. Am. J. 76, 1246–1256. doi: 10.2136/sssaj2011.0393
Dholakia, P. V. (2016). Preparation of nanopartical liquid organic fertilizer and its effect on various crops. Int. J. Sci. Res. Sci. Eng. Tech. 2, 781–785.
Dimkpa, C. O., and Bindraban, P. S. (2017). Nanofertilizers: new products for the industry? J. Agric. Food Chem. 66, 6462–6473. doi: 10.1021/acs.jafc.7b02150
Food and Agriculture Organization of the United Nations [FAO] (2017). The Future of Food and Agriculture. Trends and Challenges. Rome: FAO.
Fuentes, M., Bacaicoa, E., Rivero, M., Zamarreño,ÁM., and García-Mina, J. M. (2018). Complementary evaluation of iron deficiency root responses to assess the effectiveness of different iron foliar applications for chlorosis remediation. Front. Plant Sci. 9:351. doi: 10.3389/fpls.2018.00351
Ghafariyan, M. H., Malakouti, M. J., Dadpour, M. R., Stroeve, P., and Mahmoudi, M. (2013). Effects of magnetite nanoparticles on soybean chlorophyll. Environ. Sci. Technol. 47, 10645–10652. doi: 10.1021/es402249b
Homonnay, Z., Tolnai, G., Fodor, F., Solti,Á, Kovács, K., Kuzmann, E., et al. (2016). Iron oxide nanoparticles for plant nutrition? A preliminary Mössbauer study. Hyperfine Interact. 237, 1–9. doi: 10.1007/s10751-016-1334-1
Hunter, J. D. (2007). Matplotlib: a 2D graphics environment. Comput. Sci. Eng. 9, 99–104. doi: 10.1109/MCSE.2007.55
ISO 3696:1987 (1987). Water for Analytical Laboratory Use–Specification and Test Methods. Available at: https://www.iso.org/standard/9169.html (accessed April, 1987).
Janmohammadi, M., Navid, A., Segherloo, A. E., and Sabaghnia, N. (2016). Impact of nano-chelated micronutrients and biological fertilizers on growth performance and grain yield of maize under deficit irrigation condition. Biologija 62, 134–147. doi: 10.6001/biologija.v62i2.3339
Kovács, K., Czech, V., Fodor, F., Solti, A., Lucena, J. J., Santos-Rosell, S., et al. (2013). Characterization of Fe-leonardite complexes as novel natural iron fertilizers. J. Agric. Food Chem. 61, 12200–12210. doi: 10.1021/jf404455y
Kulikova, N., Badun, G. A., Korobkov, V. I., Chernysheva, M. G., Tsvetkova, E. A., Abroskin, D. P., et al. (2014). Accumulation of coal humic acids by wheat seedlings: direct evidence using tritium autoradiography and occurrence in lipid fraction. J. Plant Nutr. Soil Sci. 177, 875–883. doi: 10.1002/jpln.201300648
Kulikova, N. A., Polyakov, A. Y., Lebedev, V. A., Abroskin, D. P., Volkov, D. S., Pankratov, D. A., et al. (2017). Key roles of size and crystallinity of nanosized iron hydr(oxides) stabilized by humic substances in iron bioavailability to plants. J. Agric. Food Chem. 65, 11157–11169. doi: 10.1021/acs.jafc.7b03955
Lal, R. (2008). Promise and limitations of soils to minimize climate change. J. Soil Water Conserv. 63, 113A–118A. doi: 10.2489/jswc.63.4.113A
Liu, R., and Lal, R. (2015). Potentials of engineered nanoparticles as fertilizers for increasing agronomic productions. Sci. Total Environ. 514, 131–139. doi: 10.1016/j.scitotenv.2015.01.104
López-Rayo, S., Di Foggia, M., Bombai, G., Yunta, F., Rodrigues Moreira, E., Filippini, G., et al. (2015). Blood-derived compounds can efficiently prevent iron deficiency in the grapevine. Aust. J. Grape Wine Res. 21, 135–142. doi: 10.1111/ajgw.12109
Maillot, F., Morin, G., Wang, Y., Bonnin, D., Ildefonse, P., Chaneac, C., et al. (2011). New insight into the structure of nanocrystalline ferrihydrite: EXAFS evidence for tetrahedrally coordinated Iron (III). Geochim. Cosmochim. Acta 75, 2708–2720. doi: 10.1016/j.gca.2011.03.011
Manceau, A., and Gates, W. (1997). Surface structural model for ferrihydrite. Clays Clay Miner. 45, 448–460. doi: 10.1346/CCMN.1997.0450314
MAPA (1994). Métodos Oficiales De Análisis. Servicio De Publicaciones. Spanish Ministry of Agriculture, F. and F. MAPA Official Methods of Analysis, Vol. III. Madrid: MAPA.
Martín-Fernández, C., López-Rayo, S., Hernández-Apaolaza, L., and Lucena, J. J. (2017a). Timing for a sustainable fertilisation of Glycine max by using HBED/Fe3+ and EDDHA/Fe3+ chelates. J. Sci. Food Agric. 97, 2773–2781. doi: 10.1002/jsfa.8105
Martín-Fernández, C., Solti,Á, Czech, V., Kovács, K., Fodor, F., Gárate, A., et al. (2017b). Response of soybean plants to the application of synthetic and biodegradable Fe chelates and Fe complexes. Plant Physiol. Biochem. 118, 579–588. doi: 10.1016/j.plaphy.2017.07.028
Michel, F. M., Ehm, L., Antao, S. M., Lee, P. L., Chupas, P. J., Liu, G., et al. (2007). The structure of ferrihydrite, a nanocrystalline material. Science 316, 1726–1729. doi: 10.1126/science.1142525
Mimmo, T., Del Buono, D., Terzano, R., Tomasi, N., Vigani, G., Crecchio, C., et al. (2014). Rhizospheric organic compounds in the soil-microorganism-plant system: their role in iron availability. Eur. J. Soil Sci. 65, 629–642. doi: 10.1111/ejss.12158
Nadal, P., García-Delgado, C., Hernández, D., López-Rayo, S., and Lucena, J. J. (2012). Evaluation of Fe-N,N’-Bis(2-hydroxybenzyl)ethylenediamine-N,N’-diacetate (HBED/Fe3+) as Fe carrier for soybean (Glycine max) plants grown in calcareous soil. Plant Soil 360, 349–362. doi: 10.1007/s11104-012-1246-z
Naderi, M. R., and Danesh-Shahraki, A. (2013). Nanofertilizers and their roles in sustainable agriculture. Int. J. Agric. Crop Sci. 5, 2229–2232.
Nardi, S., Pizzeghello, D., Muscolo, A., and Vianello, A. (2002). Physiological effects of humic substances on higher plants. Soil Biol. Biochem. 34, 1527–1536. doi: 10.1016/S0038-0717(02)00174-8
Nečas, D., and Klapetek, P. (2012). Gwyddion: an open-source software for SPM data analysis. Cent. Eur. J. Phys. 10, 181–188. doi: 10.2478/s11534-011-0096-2
Nikolic, M., Cesco, S., Römheld, V., Varanini, Z., and Pinton, R. (2003). Uptake of iron (59Fe) Complexed to water-extractable humic substances by sunflower leaves. J. Plant Nutr. 26, 2243–2252. doi: 10.1007/s11104-009-0091-1
Olaetxea, M., De Hitaa, D., Garcia, A., Fuentes, M., Baigorri, R., Mora, V., et al. (2018). Hypothetical framework integrating the main mechanisms involved in the promoting action of rhizospheric humic substances on plant root- and shootgrowth. Appl. Soil Ecol. 123, 521–537. doi: 10.1016/j.apsoil.2017.06.007
Olaetxea, M., Mora, V., Bacaicoa, E., Garnica, M., Fuentes, M., Casanova, E., et al. (2015). Abscisic acid regulation of root hydraulic conductivity and aquaporin gene expression is crucial to the plant shoot growth enhancement caused by rhizosphere humic acids. Plant Physiol. 169, 2587–2596. doi: 10.1104/pp.15.00596
Pankratov, D. A., Dolzhenko, V. D., Stukan, R. A., Al Ansari, Y. F., Savinkina, E. V., and Kiselev, Y. M. (2013). Investigation of Iron (III) complex with crown-porphyrin. Hyperfine Interact. 222, 1–11. doi: 10.1007/s10751-011-0378-5
Pariona, N., Martinez, A. I., Hdz-García, H. M., Cruz, L. A., and Hernandez-Valdes, A. (2016). Effects of hematite and ferrihydrite nanoparticles on germination and growth of maize seedlings. Saudi J. Biol. Sci. 24, 1547–1554. doi: 10.1016/j.sjbs.2016.06.004
Rodríguez-Castrillón, J.Á, Moldovan, M., García Alonso, J. I., Lucena, J. J., García-Tomé, M. L., and Hernández-Apaolaza, L. (2008). Isotope pattern deconvolution as a tool to study iron metabolism in plants. Anal. Bioanal. Chem. 390, 579–590. doi: 10.1007/s00216-007-1716-y
Rodríguez-Lucena, P., Hernández, D., Hernández-Apaolaza, L., and Lucena, J. J. (2010). Revalorization of a two-phase olive mill waste extract into a micronutrient fertilizer. J. Agric. Food Chem. 58, 1085–1092. doi: 10.1021/jf903185z
Römheld, V., and Nikolic, M. (2007). “Iron,” in Handbook of Plant Nutrition, eds A. V. Barker and D. J. Pilbeam (Boca raton, FL: CRC Press).
Rose, M. T., Patti, A. F., Little, K. R., Brown, A. L., Jackson, W. R., and Cavagnaro, T. R. (2014). A meta-analysis and review of plant-growth response to humic substances: practical implications for agriculture. Adv. Agron. 124, 37–89. doi: 10.1016/B978-0-12-800138-7.00002-4
Sánchez-Alcalá, I., del Campillo, M. C., Barrón, V., and Torrent, J. (2012). Pot evaluation of synthetic nanosiderite for the prevention of iron chlorosis. J. SCI. Food Agric. 92, 1964–1973. doi: 10.1002/jsfa.5569
Schwertmann, U., and Cornell, R. (1992). Iron Oxides in the Laboratory: Preparation and Characterization. Hoboken, NJ: John Wiley & Sons.
Schwertmann, U., Friedl, J., and Stanjekl, H. (1999). From Fe (III) ions to ferrihydrite and then to hematite. J. Colloid Interface Sci. 209, 215–223. doi: 10.1006/jcis.1998.5899
Soltanpour, P. N., and Schwab, A. P. (1977). A new soil test for simultaneous extraction of macro- and micro-nutrients in alkaline soils. Commun. Soil Sci. Plant Anal. 8, 195–207. doi: 10.1080/00103627709366714
Sorkina, T. A., Polyakov, A. Y., Kulikova, N. A., Goldt, A. E., Philippova, O. I., Aseeva, A. A., et al. (2014). Nature-inspired soluble iron-rich humic compounds: new look at the structure and properties. J. Soils Sediments 14, 261–268. doi: 10.1007/s11368-013-0688-0
Tomasi, N., De Nobili, M., Gottardi, S., Zanin, L., Mimmo, T., Varanini, Z., et al. (2013). Physiological and molecular characterization of fe acquisition by tomato plants from natural Fe complexes. Biol. Fertil. Soils 49, 187–200. doi: 10.1007/s00374-012-0706-1
United Nations [UN] (2013). World Population Prospects: The 2012 Revision. Available at: https://population.un.org/wpp/Publications/Files/WPP2012_HIGHLIGHTS.pdf (accessed December 1, 2018).
Vasconcelos, M. W., and Grusak, M. A. (2014). Morpho-physiological parameters affecting iron deficiency chlorosis in soybean (Glycine max L.). Plant Soil 374, 161–172. doi: 10.1007/s11104-013-1842-6
Villén, M., Lucena, J. J., Cartagena, M. C., Bravo, R., García-Mina, J., and de la Hinojosa, M. I. M. (2007). Comparison of two analytical methods for the evaluation of the complexed metal in fertilizers and the complexing capacity of complexing agents. J. Agric. Food Chem. 55, 5746–5753. doi: 10.1021/jf070422t
Ylivainio, K. (2010). Effects of iron (III) chelates on the solubility of heavy metals in calcareous soils. Environ. Pollut. 158, 3194–3200. doi: 10.1016/j.envpol.2010.07.004
Keywords: iron nanoparticles, iron nutrition, humic substances, leonardite, 57Fe, soybean, ferrihydrite
Citation: Cieschi MT, Polyakov AY, Lebedev VA, Volkov DS, Pankratov DA, Veligzhanin AA, Perminova IV and Lucena JJ (2019) Eco-Friendly Iron-Humic Nanofertilizers Synthesis for the Prevention of Iron Chlorosis in Soybean (Glycine max) Grown in Calcareous Soil. Front. Plant Sci. 10:413. doi: 10.3389/fpls.2019.00413
Received: 14 January 2019; Accepted: 19 March 2019;
Published: 05 April 2019.
Edited by:
Thomas J. Buckhout, Humboldt-Universität zu Berlin, GermanyCopyright © 2019 Cieschi, Polyakov, Lebedev, Volkov, Pankratov, Veligzhanin, Perminova and Lucena. This is an open-access article distributed under the terms of the Creative Commons Attribution License (CC BY). The use, distribution or reproduction in other forums is permitted, provided the original author(s) and the copyright owner(s) are credited and that the original publication in this journal is cited, in accordance with accepted academic practice. No use, distribution or reproduction is permitted which does not comply with these terms.
*Correspondence: Irina V. Perminova, iperm@org.chem.msu.ru Juan J. Lucena, juanjose.lucena@uam.es