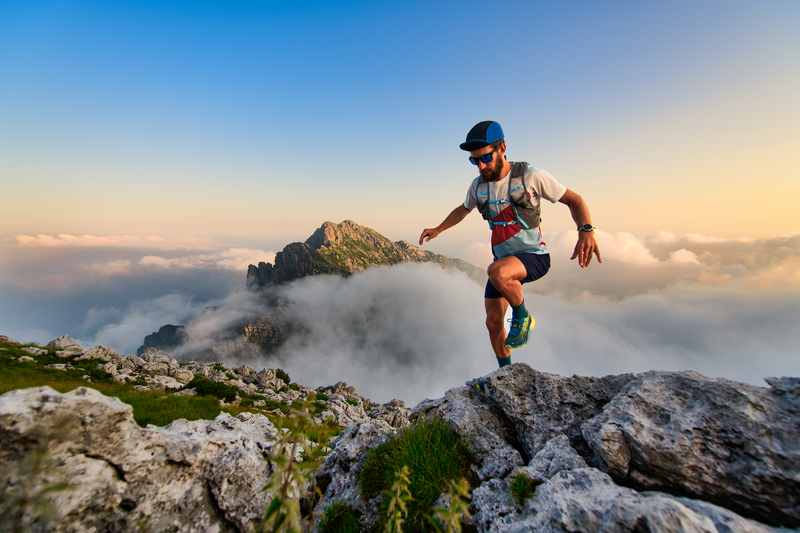
95% of researchers rate our articles as excellent or good
Learn more about the work of our research integrity team to safeguard the quality of each article we publish.
Find out more
ORIGINAL RESEARCH article
Front. Plant Sci. , 12 March 2019
Sec. Plant Physiology
Volume 10 - 2019 | https://doi.org/10.3389/fpls.2019.00242
This article is part of the Research Topic Proceedings of RBMP 2018 - Plant Molecular Biology View all 19 articles
Zea mays Brittle1-1 (ZmBT1-1) is an essential component of the starch biosynthetic machinery in maize endosperms, enabling ADPglucose transport from cytosol to amyloplast in exchange for AMP or ADP. Although ZmBT1-1 has been long considered to be an amyloplast-specific marker, evidence has been provided that ZmBT1-1 is dually localized to plastids and mitochondria (Bahaji et al., 2011b). The mitochondrial localization of ZmBT1-1 suggested that this protein may have as-yet unidentified function(s). To understand the mitochondrial ZmBT1-1 function(s), we produced and characterized transgenic Zmbt1-1 plants expressing ZmBT1-1 delivered specifically to mitochondria. Metabolic and differential proteomic analyses showed down-regulation of sucrose synthase (SuSy)-mediated channeling of sucrose into starch metabolism, and up-regulation of the conversion of sucrose breakdown products generated by cell wall invertase (CWI) into ethanol and alanine, in Zmbt1-1 endosperms compared to wild-type. Electron microscopic analyses of Zmbt1-1 endosperm cells showed gross alterations in the mitochondrial ultrastructure. Notably, the protein expression pattern, metabolic profile, and aberrant mitochondrial ultrastructure of Zmbt1-1 endosperms were rescued by delivering ZmBT1-1 specifically to mitochondria. Results presented here provide evidence that the reduced starch content in Zmbt1-1 endosperms is at least partly due to (i) mitochondrial dysfunction, (ii) enhanced CWI-mediated channeling of sucrose into ethanol and alanine metabolism, and (iii) reduced SuSy-mediated channeling of sucrose into starch metabolism due to the lack of mitochondrial ZmBT1-1. Our results also strongly indicate that (a) mitochondrial ZmBT1-1 is an important determinant of the metabolic fate of sucrose entering the endosperm cells, and (b) plastidic ZmBT1-1 is not the sole ADPglucose transporter in maize endosperm amyloplasts. The possible involvement of mitochondrial ZmBT1-1 in exchange between intramitochondrial AMP and cytosolic ADP is discussed.
Mitochondria are the main sites of cellular respiration and ATP supply. They also play important roles in diverse processes such as redox homeostasis and provision of molecules that act as metabolic intermediates in essential biosynthetic pathways or as specific signals that modulate nuclear-encoded protein expression (Chandel, 2014). To connect internal metabolism with that of the surrounding cell, mitochondria possess solute carrier proteins in the inner membrane which, due to their common basic structure, are classed as members of the mitochondrial carrier family (MCF) (Haferkamp and Schmitz-Esser, 2012; Taylor, 2017). In plants, MCF proteins are involved in the transport of nucleotides, phosphate, di- and tri-carboxylates, amino acids, and cofactors across the mitochondrial membrane. They are all presumed to be targeted to the mitochondrial inner membrane, although some of them have been shown to occur in peroxisomes, glyoxysomes, plasma membrane, and plastids (Sullivan and Kaneko, 1995; Fukao et al., 2001; Bedhomme et al., 2005; Palmieri et al., 2006; Bahaji et al., 2011a,b).
Brittle1 (BT1) proteins are members of the MCF that occur only in plants. At the transcriptional level, maize plants express two BT1 homologs: ZmBT1-1 and ZmBT1-2 (Kirchberger et al., 2007). Whereas ZmBT1-2 shows a ubiquitous expression pattern in heterotrophic and autotrophic tissues, ZmBT1-1 expression is developmentally regulated, being high in maize endosperms 12–25 days after pollination (DAP) and undetectable in non-endosperm tissues and suspension cultures (Sullivan et al., 1991; Cao et al., 1995; Sullivan and Kaneko, 1995; Cao and Shannon, 1996; Kirchberger et al., 2007). ZmBT1-1 encodes a protein with a predicted molecular mass of ca. 47 kDa (Sullivan et al., 1991). In maize endosperms, ZmBT1-1 is present as three 39, 40, and 44 kDa proteins (Cao et al., 1995; Sullivan and Kaneko, 1995), the former two being processing products generated within the plastidial compartment (Li et al., 1992).
The bt1-1 locus of maize was identified in 1926 by mutations that severely decreased the amount of starch deposition in the endosperm and resulted in seeds with a collapsed angular appearance at maturity that germinated slowly and produced plants of low vigor (Mangelsdorf, 1926). Zmbt1-1 endosperms accumulate high levels of the starch precursor molecule, ADPglucose (Shannon et al., 1996). Import studies using amyloplasts isolated from maize endosperms showed that amyloplasts can transport ADPglucose in counter-exchange with AMP and ADP (Shannon et al., 1998). These studies also showed that the incorporation of externally applied ADPglucose into starch in Zmbt1-1 amyloplasts was reduced to about 25% compared with wild type (WT) amyloplasts (Shannon et al., 1998). Overall, the data indicated that ZmBT1-1 is an essential component of the starch biosynthetic machinery in maize endosperms, enabling the transport into the amyloplast of cytosolic ADPglucose produced by ADPglucose pyrophosphorylase (AGP) and sucrose synthase (SuSy) (Bahaji et al., 2014; Boehlein et al., 2018) in exchange with ADP produced by starch synthase as schematically illustrated in Supplemental Figure 1 (Kleczkowski, 1996) (Shannon et al., 1996, 1998).
Although ZmBT1-1 has been long considered to be an amyloplast-specific marker (Sullivan and Kaneko, 1995; Shannon et al., 1998; Kirchberger et al., 2007), confocal fluorescence microscopy studies using plants stably expressing GFP fusions of ZmBT1-1, and electron microscopic immunocytochemical analyses of maize endosperms, have provided evidence that ZmBT1-1 is dually localized to plastids and mitochondria (Bahaji et al., 2011b). These studies also showed that ZmBT1-1 N-terminal extensions comprise targeting sequences recognized exclusively by the plastidial compartment, whereas sequences targeting to mitochondria are localized within the mature part of ZmBT1-1. The mitochondrial localization of ZmBT1-1 suggested that this protein may have as-yet unidentified function(s). To get insights into the role(s) of mitochondrial ZmBT1-1, in this work we conducted proteomic, metabolic, and microscopic characterization of developing endosperms of Zmbt1-1 plants and transgenic Zmbt1-1 plants expressing ZmBT1-1 delivered specifically to mitochondria. Our findings show that mitochondrial ZmBT1-1 is a decisive factor in primary metabolism and mitochondrial function in developing maize endosperms and raise important questions regarding the role of BT1 in cereal endosperms.
The work was performed using WT plants (the hybrid W23/M14/W64A), a Zmbt1-1 mutant in a W23/M14/W64A background provided by the Maize Genetics COOP Stock Center (bt1-m1::dSpm, ref. 514N), which contains a ca. 3.3 kbp defective Suppressor-mutator (dSpm) in the third exon of ZmBT1-1 (Sullivan et al., 1991) (Supplemental Figure 2A) and a Sh2 mutant in a OH43 background provided by the Maize Genetics COOP Stock Center (sh2/OH43, ref. 333D). The indentity of the Zmbt1-1 mutant was confirmed by PCR using the O1 (5′-CGAGACGCTGAAGCGGCTCTAC-3′) and O2 (5′-CACGATCCGGAAACACCACATC-3′) ZmBT1-1 specific primers (the latter hybridizes with a genomic sequence occurring downstream of the ZmBT1-1 stop codon, Supplemental Figures 2A,B) as well as the dSpm-specific O3 primer (5′-GGACTTGAACTTGTATGAATATTG-3′) (Supplemental Figures 2A,B). We also used Zmbt1-1 plants transformed with UBI-ZmBT1-1, UBI-ΔTP-ZmBT1-1, and UBI-MitTPr-ΔTP-ZmBT1-1 (designated Zmbt1-1::ZmBT1-1, Zmbt1-1::ΔTP-ZmBT1-1, Zmbt1-1::MitTPr-ΔTP-ZmBT1-1, respectively) that were generated in two steps:
Step one: Production of HiII plants (Armstrong et al., 1991) transformed with UBI-ZmBT1-1, UBI-ΔTP-ZmBT1-1 and UBI-MitTPr-ΔTP-ZmBT1-1. To achieve this, HiII immature embryo-derived callus cultures were transformed using the biolistic gun-mediated method (Wang and Frame, 2009) and the pAHC25-ZmBT1-1, pAHC25-ΔTP-ZmBT1-1 and pAHC25-MiTPr-ΔTP-ZmBT1-1 plasmids (Supplemental Figure 3). These plasmids were produced from the pAHC25 plasmid (Christensen and Quail, 1996) by Gateway technology (Invitrogen), and their identities confirmed by sequencing. They contain, respectively, the ZmBT1-1, ΔTP-ZmBT1-1, and MitTPr-ΔTP-ZmBT1-1 genes, each under the transcriptional control of the Ubi-1 promoter, and a selectable (bar) gene (Supplemental Figure 3). Plantlets regenerated in medium containing the herbicide glufosinate were transplanted into pots, and further selected by spraying with the herbicide glufosinate (0.1%). Herbicide selection and PCR analyses (see below) were conducted for every generation, and the herbicide-resistant and PCR-positive plants were self-pollinated until homozygous transgenic HiII lines were generated. PCR-confirmation of the integration of UBI-ZmBT1-1, UBI-ΔTP-ZmBT1-1, and UBI-MitTPr-ΔTP-ZmBT1-1 into the plant genome was conducted using the Ubi-1 promoter-specific O4 primer (5′-GCATATGCAGCAGCTATATGTG-3′) and the ZmBT1-1-specific O5 primer (5′-GGTGCGGGTTGGCGATCTTG-3′) (Supplemental Figures 2C,D). Transformation with UBI-ΔTP-ZmBT1-1 was further confirmed by PCR using O4 and the O6 primer (5′-GGGACCTGCAATGACGACCA-3′) specific for the ZmBT1-1 TP encoding sequence (Supplemental Figures 2C,E). Transformation with UBI-MitTPr-ΔTP-ZmBT1-1 was further PCR-confirmed using O5 and the O7 primer (5′-ATGGCTATGGCTGTTTTCCGC-3′) specific for the MitTPr encoding sequence (Supplemental Figures 2C,F). All the transformations were further confirmed by sequencing of the amplicons obtained by PCR.
Step two: Crossing Zmbt1-1 plants with the transgenic HiII plants generated as above. The seeds obtained were germinated and plants selected by spraying with glufosinate. Herbicide selection and PCR analyses were conducted, and herbicide-resistant and PCR-positive plants were self-pollinated, backcrossed to Zmbt1-1 for a total of three generations, and self-pollinated until homozygous Zmbt1-1::ZmBT1-1, Zmbt1-1::ΔTP-ZmBT1-1, and Zmbt1-1::MitTPr-ΔTP-ZmBT1-1 plants were generated.
For subcellular localization of GFP-tagged proteins, we produced HiII plants transformed with UBI-ΔTP-ZmBT1-1-GFP, UBI-MiTPr-GFP, and UBI-MitTPr-ΔTP-ZmBT1-1-GFP (designated as ΔTP-ZmBT1-1-GFP, MiTPr-GFP, and MitTPr-ΔTP-ZmBT1-1-GFP, respectively) using the pAHC25-ΔTP-ZmBT1-1-GFP, pAHC25-MiTPr-GFP, and pAHC25MiTPr-ΔTP-ZmBT1-1-GFP plasmids (Supplemental Figure 3).
Ten plants per line were grown in 35 L pots in greenhouse conditions. For biochemical and proteomic analyses, seeds were collected at the indicated developmental stage, and endosperms immediately extracted, freeze-clamped, and ground to a fine powder in liquid nitrogen with a pestle and mortar.
For measurement of glucose-6-phosphate (G6P), fructose-6-phosphate, glucose-1-phosphate, UDPglucose and ADPglucose, a 0.5 g aliquot of the frozen powdered endosperm (see above) was resuspended in 1 ml of 1 M HClO4, left at 4°C for 2 h and centrifuged at 10,000 × g for 5 min. The supernatant was neutralized with K2CO3 and centrifuged at 10,000 × g. G6P, fructose-6-phosphate, and glucose-1-phosphate in supernatants were determined by HPLC with pulsed amperometric detection on a DX-500 Dionex system with a CarboPac 10 column according to the gradient separation application method suggested by the supplier (100 mM NaOH/100 mM sodium acetate to 100 mM NaOH/500 mM sodium acetate over 40 min). UDPglucose and ADPglucose were measured as described in Li et al. (2013), by HPLC on a system obtained from Waters Associates fitted with a Partisil-10-SAX column. Alanine and GABA contents were measured as described by Loiret et al. (2009). Ethanol was measured as described by Licausi et al. (2010). Recovery experiments were carried out by adding known amounts of metabolite standards to the frozen tissue slurry immediately after the addition of extraction solutions. The difference between the measurements from samples with and without added standards was used as an estimate of the percentage of recovery. All data were corrected for losses during extraction. Starch from 24 DAP endosperms and dry seeds was measured with an amyloglucosidase–based test kit (Boehringer Mannheim, Germany).
One g of the frozen powder (see above) was resuspended at 4°C in 5 ml of 100 mM HEPES (pH 7.5), 2 mM EDTA, and 5 mM dithiothreitol (extraction buffer). The suspension was desalted, resuspended in 5 ml extraction buffer, and assayed for enzymatic activities. We verified that this procedure did not result in loss of enzymatic activity by comparing activity in extracts prepared from the frozen powder with extracts prepared by homogenizing fresh tissue in extraction medium. Total invertase and SuSy activities were measured as described by Baroja-Fernández et al. (2009). One unit (U) is defined as the amount of enzyme that catalyzes the production of 1 μmol of product per min.
For immunoblot analyses, 100 mg of the fine frozen endosperm powder was resuspended in 300 μl of 50 mM Hepes pH 7.0, 2 mM EDTA, and 10 mM dithiothreitol, incubated 30 min at 4°C, and centrifuged at 10,000 × g for 15 min. Proteins occurring in the supernatant were then separated on 15% SDS-PAGE, transferred to PVDF filters, and immunodetected using the antisera raised against recombinant ZmBT1-1 (Bahaji et al., 2011a), and a goat anti-rabbit IgG alkaline phosphatase conjugate as secondary antibody (Sigma).
Thirty DAP seeds were stained in iodine solution [KI 2% (w/v), I2 1% (w/v)] for 30 min, rinsed briefly in deionized water and photographed.
These analyses were conducted essentially as decribed in Sánchez-López et al. (2016) for Arabidopsis leaves but with the following modifications. For protein sample preparation samples were prepared by grinding 200 mg of endosperm material from 24 DAP developing seeds into a fine powder under liquid nitrogen using a pre-cooled mortar and pestle. For the data analyses, MS/MS spectra were exported to MGF format using Peak View v1.2.0.3 (Sciex, Redwood City, CA) and searched using Mascot Server 2.5.1, OMSSA 2.1.9, X!TANDEM 2013.02.01.1 and MyriMatch 2.2.140 against a composite target/decoy database for maize built from the 85,535 sequences from UniProt Knowledgebase, together with commonly occurring contaminants. The cut-off for differentially regulated proteins was set at a FDR ≤ 5%. Functional characterization of the differentially expessed proteins was performed using the MapMan tool (https://mapman.gabipd.org/) (Thimm et al., 2004).
Subcellular localization of GFP in ΔTP-ZmBT1-1-GFP, MiTPr-GFP, and MitTPr-ΔTP-ZmBT1-1-GFP plants was performed using D-Eclipse C1 confocal microscope (Nikon, Japan) equipped with standard Ar 488 laser excitation, BA515/30 filter for green emission, and a BA650LP filter for red emission.
We characterized samples from leaves and 24 DAP endosperms of WT, Zmbt1-1, Sh2, and Zmbt1-1::MitTPr-ΔTP-ZmBT1-1 plants. Once excised, samples were immediately transferred to aluminum sample holders, cryoprotected with 150 mM sucrose, frozen in a Leica electron microscopic HPM-100 high-pressure freezer (Leica Microsystems, Vienna), transferred to liquid nitrogen, and processed according to Seguí-Simarro (2015). In brief, samples were freeze substituted with anhydrous acetone +2% OsO4 at −80°C for 4 days, followed by slow warming to room temperature over a period of 24 h. After rinsing several times in acetone, they were removed from the holders and embedded with increasing concentrations of Spurr resin in acetone according to the following schedule: 3 h in 2% resin, 3 h in 5% resin, 15 h in 10% resin, 8 h in 25% resin, 15 h in 50% resin, 8 h in 75% resin, and 40 h in 100% resin. Resin polymerization was performed at 70°C for 30 h. Ultrathin (~80 nm) sections were then obtained using a Leica UC6 ultramicrotome, mounted on formvar-coated, 150 mesh copper grids, stained with 2% uranyl acetate for 6 min and with lead citrate prepared as described in Reynolds (1963) for 4 min, and observed in a Jeol JEM 1010 transmission electron microscope.
The data are presented as the means of four independent experiments, with 3–5 replicates for each experiment (means ± SE). The significance of differences between WT, Zmbt1-1::ZmBT1-1, Zmbt1-1::ΔTP-ZmBT1-1, and Zmbt1-1::MitTPr-ΔTP-ZmBT1-1 endosperms was statistically evaluated by Student's t-test using the SPSS software. Differences were considered significant at a probability level of P < 0.05.
We generated transgenic homozygous Zmbt1-1 maize plants transformed with either UBI-ZmBT1-1 or UBI-ΔTP-ZmBT1-1 as described in Methods. These plants express, respectively, ZmBT1-1 and ΔTP-ZmBT1-1 under the control of the Ubi-1 promoter; the latter construct encodes a ca. 44 kDa truncated form of ZmBT1-1 (designated as ΔTP-ZmBT1-1) that lacks 24 amino acids from the N-terminal extension which potentially acts as a plastidial transit peptide (TP). We also generated Zmbt1-1 plants transformed with UBI-MitTPr-ΔTP-ZmBT1-1, which express a mitochondria-targeting pre-sequence [the N-terminus of the F1-ATPase γ-subunit (Niwa et al., 1999)] fused to ΔTP-ZmBT1-1. The identities of the Zmbt1-1 mutant, and the homozygous Zmbt1-1 maize plants transformed with UBI-ZmBT1-1, UBI-ΔTP-ZmBT1-1, or UBI-MitTPr-ΔTP-ZmBT1-1 (designated as Zmbt1-1::ZmBT1-1, Zmbt1-1::ΔTP-ZmBT1-1, and Zmbt1-1::MitTPr-ΔTP-ZmBT1-1, respectively) were confirmed by PCR and sequencing of the amplicons obtained (Supplemental Figure 2).
Western blot analyses of ZmBT1-1 revealed bands of ca. 39, 40, and 44 kDa in WT endosperms (Figure 1), which is consistent with previous reports (Cao et al., 1995; Sullivan and Kaneko, 1995). As expected, no polypeptides cross-reacting with the ZmBT1-1 antisera could be detected in Zmbt1-1 endosperms (Figure 1). Similar to WT endosperms, Zmbt1-1::ZmBT1-1 endosperms expressed three proteins of ca. 39, 40, and 44 kDa that cross-reacted with ZmBT1-1 antisera (Figure 1). In contrast, Zmbt1-1::ΔTP-ZmBT1-1 and Zmbt1-1::MitTPr-ΔTP-ZmBT1-1 endosperms accumulated a single ca. 44 kDa protein that cross-reacted with the ZmBT1-1 antisera (Figure 1). No plastidial ZmBT1-1 processing product (e.g., 39 and 40 kDa proteins; Li et al., 1992) could be detected in Zmbt1-1::ΔTP-ZmBT1-1 and Zmbt1-1::MitTPr-ΔTP-ZmBT1-1 endosperms (Figure 1).
Figure 1. Western blot analysis of ZmBT1-1 in proteins extracted from 24 DAP WT (W23/M14/W64A), Zmbt1-1::ZmBT1-1, Zmbt1-1::ΔTP-ZmBT1-1, Zmbt1-1::MitTPr-ΔTP-ZmBT1-1, and Zmbt1-1 endosperms. The gel was loaded with 30 μg protein per lane.
TargetP (http://www.cbs.dtu.dk/services/TargetP/) (Emanuelsson et al., 2000) predicts mitochondrial localization for both ΔTP-ZmBT1-1 and MitTPr-ΔTP-ZmBT1-1. To confirm that ΔTP-ZmBT1-1 was specifically targeted to mitochondria in Zmbt1-1::ΔTP-ZmBT1-1 and Zmbt1-1::MitTPr-ΔTP-ZmBT1-1 endosperms we carried out confocal fluorescence microscopy analyses of WT maize plants expressing ΔTP-ZmBT1-1-GFP and MitTPr-ΔTP-ZmBT1-1-GFP. We also analyzed fluorescence distribution in plants expressing the mitochondrial-targeting pre-sequence MitTPr fused with GFP (MitTPr-GFP). These analyses revealed that the GFP fluorescence distribution and motility patterns in ΔTP-ZmBT1-1-GFP- and MitTPr-ΔTP-ZmBT1-1-GFP- expressing plants were identical to those of plants expressing the mitochondrial marker MitTPr-GFP (see movies in Supplemental Videos 1–3), which is consistent with previous studies using ΔTP-ZmBT1-1-GFP- and MitTPr-ΔTP-ZmBT1-1-GFP- expressing Arabidopsis plants (cf. Figure 4, Bahaji et al., 2011b). These findings confirm that ΔTP-ZmBT1-1-GFP has a mitochondrial localization in ΔTP-ZmBT1-1-GFP- and MitTPr-ΔTP-ZmBT1-1-GFP- expressing maize plants.
Zmbt1-1 seeds showed a collapsed, angular appearance at maturity (Figure 2A) and were of reduced weight (Figure 2B) and starch content (Figure 2C), a finding which is consistent with previous reports (Mangelsdorf, 1926; Sullivan et al., 1991). As expected, these phenotypes could be reverted to WT by ectopic expression of ZmBT1-1 (Figure 2). Notably, Zmbt1-1::ΔTP-ZmBT1-1 and Zmbt1-1::MitTPr-ΔTP-ZmBT1-1 seeds displayed a normal external appearance (Figure 2A), and their weights and starch contents were like those of WT seeds (Figures 2B,C). Iodine staining for starch localization in excised 30 DAP seeds showed an even distribution of starch in WT, Zmbt1-1::ZmBT1-1, Zmbt1-1::ΔTP-ZmBT1-1, and Zmbt1-1::MitTPr-ΔTP-ZmBT1-1 endosperms, whereas in Zmbt1-1 seeds starch accumulated exclusively in the upper part of the endosperm (Figure 2D).
Figure 2. Delivery of ZmBT1-1 specifically to mitochondria complements the collapsed angular appearance and the low endosperm starch content of Zmbt1-1 seeds. External appearance (A), weight (B), and starch content (C) of WT, Zmbt1-1, Zmbt1-1::ZmBT1-1, Zmbt1-1::ΔTP-ZmBT1-1, and Zmbt1-1::MitTPr-ΔTP-ZmBT1-1 dry seeds. (D) Iodine staining of 30 DAP WT, Zmbt1-1, Zmbt1-1::ZmBT1-1, Zmbt1-1::ΔTP-ZmBT1-1, and Zmbt1-1::MitTPr-ΔTP-ZmBT1-1 seeds. In (B,C), values represent the mean ± SD of determinations on four independent samples from three independent lines each of Zmbt1-1::ZmBT1-1, Zmbt1-1::ΔTP-ZmBT1-1, and Zmbt1-1::MitTPr-ΔTP-ZmBT1-1. Asterisks indicate significant differences based on Student's t-tests. (P < 0.05, Zmbt1-1 mutants vs. WT).
Zmbt1-1 seeds germinated slowly when compared with WT seeds (Supplemental Figure 4A), which is consistent with the findings of Mangelsdorf (1926). Slow germination resulted in delayed growth of Zmbt1-1 plants when compared with WT plants of various genetic backgrounds (Supplemental Figure 4B). As expected, the slow germination and delayed growth phenotype of Zmbt1-1 plants could be complemented by the ectopic expression of ZmBT1-1 (Supplemental Figure 4). Zmbt1-1::ΔTP-ZmBT1-1 and Zmbt1-1::MitTPr-ΔTP-ZmBT1-1 seeds displayed WT germination and growth phenotypes (Supplemental Figure 4).
WT, Zmbt1-1, Zmbt1-1::ZmBT1-1, Zmbt1-1::ΔTP-ZmBT1-1, and Zmbt1-1::MitTPr-ΔTP-ZmBT1-1 developing (24 DAP) endosperms were analyzed for contents of metabolic intermediates of the sucrose-to-starch conversion process. As expected, the starch content of Zmbt1-1 endosperms was lower than that of WT endosperms. Levels of hexose-phosphates (e.g., fructose-6-phosphate, G6P and glucose-1-phosphate) in Zmbt1-1 endosperms were higher than those in WT endosperms. UDPglucose and ADPglucose contents of Zmbt1-1 endosperms were ca. 2- and 12-fold higher, respectively, than those of WT endosperms (Figure 3), an observation which is consistent with Shannon et al. (1996). Notably, the low starch content, and the high hexose-phosphate and nucleotide-sugar contents of developing Zmbt1-1 endosperms could be reverted to WT levels not only by ectopic expression of ZmBT1-1, but also through expression of ΔTP-ZmBT1-1 and MitTPr-ΔTP-ZmBT1-1 (Figure 3).
Figure 3. Delivery of ZmBT1-1 specifically to mitochondria reverts to WT the content of ADPglucose and other sucrose-to-starch biosynthetic intermediates in developing Zmbt1-1 endosperms. The graphics represent the amounts of the metabolites indicated in 24 DAP endosperms from WT, Zmbt1-1, Zmbt1-1::ZmBT1-1(1), Zmbt1-1::ΔTP-ZmBT1-1(1), and Zmbt1-1::MitTPr-ΔTP-ZmBT1-1(1) seeds. Values represent the mean ± SD of determinations on four independent samples. Asterisks indicate significant differences based on Student's t-tests. (P < 0.05, Zmbt1-1 mutants vs. WT).
The results presented above provided evidence that mitochondrial ZmBT1-1 plays an important role in the sucrose-to-starch conversion process in maize endosperms. To obtain insights into the mechanisms influenced by the mitochondrial ZmBT1-1, we carried out high-throughput, isobaric labeling-based differential proteomic analyses of 24 DAP Zmbt1-1 and WT endosperms, and 24 DAP Zmbt1-1 and Zmbt1-1::MitTPr-ΔTP-ZmBT1-1 endosperms.
As shown in Supplemental Tables 1, 2, 414 out of the 2183 proteins identified were differentially expressed in Zmbt1-1 and WT endosperms, 35 of them being annotated as “uncharacterized proteins.” Among the population of 379 differentially expressed proteins (DEPs) with known functions, 191 were up-regulated, and 188 were down-regulated in Zmbt1-1 (Supplemental Table 1). By using the broad classifications outlined by MapMan, the 379 proteins with known functions that were differentially expressed in Zmbt1-1 and WT endosperms were assembled into 25 functional groups (Figure 4). The absence of ZmBT1-1 resulted in the down-regulation of the expression of several soluble starch synthase (SSS) isoforms and the major endosperm SuSy isoform, SH1 (Supplemental Table 1, Figure 4), and up-regulation of the expression of the major endosperm invertase isoform, the cell wall invertase 2 (CWI-2), sorbitol dehydrogenase (SDH), fructokinase (FK), glycolytic enzymes, and enzymes of the ethanolic fermentation pathway and the TCA cycle (Supplemental Table 1, Figure 4).
Figure 4. Functional categorization of the proteins differentially expressed in endosperms of Zmbt1-1 and WT seeds. The number of proteins that are up- and down-regulated in Zmbt1-1 endosperms is indicated by gray and black bars, respectively. Proteins are indicated by their accession numbers. Their descriptions are shown in Supplemental Table 1. Proteins discussed here are boxed.
One hundred and twenty-five out of the 2,152 proteins identified were differentially expressed in Zmbt1-1 and Zmbt1-1::MitTPr-ΔTP-ZmBT1-1 endosperms, 7 of them being annotated as “uncharacterized proteins” (Supplemental Tables 3, 4). Among the population of 118 DEPs with known functions, 60 were up-regulated, and 58 were down-regulated in Zmbt1-1 endosperms. When comparing the DEPs in Zmbt1-1 and Zmbt1-1::MitTPr-ΔTP-ZmBT1-1 endosperms (Supplemental Table 3) with those of Zmbt1-1 and WT endosperms (Supplemental Table 1) we found that 53% of the proteins that were differentially expressed in the Zmbt1-1 and Zmbt1-1::MitTPr-ΔTP-ZmBT1-1 endosperms were also differentially expressed in the Zmbt1-1 and WT endosperms (Supplemental Table 3). This indicates that the absence of mitochondrial ZmBT1-1 largely accounts for the differences in protein expression observed in ZmBT1-1 and WT endosperms.
The 118 DEPs with known functions that were differentially expressed in Zmbt1-1 and Zmbt1-1::MitTPr-ΔTP-ZmBT1-1 endosperms were assembled into 17 functional groups (Figure 5). Notably, mitochondrial delivery of ZmBT1-1 in Zmbt1-1 strongly up-regulated the expression of SH1 and xylose isomerase, and down-regulated the expresions of CWI-2, SDH, FK, glycolytic, and TCA cycle enzymes (Supplemental Table 3, Figure 5). Also, mitochondrial-specific delivery of ZmBT1-1 in Zmbt1-1 up-regulated the expression of numerous starch metabolism enzymes [e.g., granule-bound starch synthase, several SSS isoforms, starch phosphorylase, and the small and large subunits of AGP (BT2a and SH2, respectively)] (Supplemental Table 3, Figure 5).
Figure 5. Functional categorization of the proteins differentially expressed in endosperms of Zmbt1-1::MitTPr-ΔTP-ZmBT1-1 and Zmbt1-1 seeds. The number of proteins that are up- and down-regulated in Zmbt1-1::MitTPr-ΔTP-ZmBT1-1 endosperms is indicated by gray and black bars, respectively. Proteins are indicated by their accession numbers. Their descriptions are shown in Supplemental Table 3. Proteins discussed here are boxed. Proteins that are differentially expressed in WT and Zmbt1-1 seeds are highlighted in red.
In maize, the level of expression of CWI-2 is greatest early during seed development and it then drops from the 12 DAP stage (Cheng et al., 1996; Prioul et al., 2008) whereas SH1 expression increases from the 14 DAP developmental stage (Doehlert et al., 1988; Méchin et al., 2007; Prioul et al., 2008; Li et al., 2013). The results of the differential proteomic analyses described above suggested that a lack of mitochondrial ZmBT1-1 could result in impairment of the transition from CWI-2- to SH1- mediated sucrose breakdown during endosperm development. To test this hypothesis we measured total invertase and SuSy activities during the development of WT, Zmbt1-1 and Zmbt1-1::MitTPr-ΔTP-ZmBT1-1 endosperms. As shown in Figure 6 these analyses revealed that, unlike WT and Zmbt1-1::MitTPr-ΔTP-ZmBT1-1 endosperms, total invertase and SuSy activities did not vary much during development of Zmbt1-1 endosperms.
Figure 6. Lack of mitochondrial ZmBT1-1 impedes down-regulation of CWI-2 expression and up-regulation of SH1 expression during endosperm development. The graphics represent total invertase and SuSy activities in WT, Zmbt1-1, and Zmbt1-1::MitTPr-ΔTP-ZmBT1-1(1) endosperms during seed development. Values represent the mean ± SD of determinations on four independent samples. Asterisks indicate significant differences based on Student's t-tests. (P < 0.05, Zmbt1-1 mutants vs. WT).
The proteomic analyses suggested that a lack of ZmBT1-1 could promote the accumulation of ethanol and alanine from glycolytically produced pyruvate, as expression of glycolytic and ethanolic fermentation enzymes, and TCA cycle enzymes involved in GABA shunt-mediated alanine production, were higher in Zmbt1-1 endosperms than in WT endosperms. This inference was corroborated by the analysis of the ethanol, GABA and alanine contents of 24 DAP WT, Zmbt1-1, and Zmbt1-1::ZmBT1-1 endosperms. As shown in Figure 7, the levels of these compounds were higher in Zmbt1-1 endosperms than in WT and Zmbt1-1::ZmBT1-1 endosperms. To test the possibility that the absence of mitochondrial ZmBT1-1 causes ethanol, GABA, and alanine over-accumulation in Zmbt1-1 endosperms we characterized Zmbt1-1::MitTPr-ΔTP-ZmBT1-1 endosperms. We observed that delivering ZmBT1-1 specifically to mitochondria rescues the WT levels of ethanol, GABA, and alanine in Zmbt1-1 endosperms (Figure 7).
Figure 7. Lack of mitochondrial ZmBT1-1 promotes the accumulation of high levels of ethanol, GABA, and alanine. The graphics represent the contents of the metabolites indicated in 24 DAP endosperms from WT, Zmbt1-1, Zmbt1-1::ZmBT1-1(1), and Zmbt1-1::MitTPr-ΔTP-ZmBT1-1(1) seeds. Values represent the mean ± SD of determinations on four independent samples. Asterisks indicate significant differences based on Student's t-tests. (P < 0.05, Zmbt1-1 mutants vs. WT).
Down-regulation of storage metabolism, up-regulation of glycolytic and ethanolic fermentation, and alanine accumulation promoted by the lack of mitochondrial ZmBT1 resemble the responses of plants to conditions in which mitochondrial functioning is compromised (Miyashita and Good, 2008; Shingaki-Wells et al., 2014). Under such conditions, mitochondria fail to develop normally and exhibit signs of degeneration such as loss of cristae, clarification of the matrix and swelling (Shingaki-Wells et al., 2014). To investigate whether the metabolic disorder observed in Zmbt1-1 endosperms was associated with aberrant mitochondrial development, we analyzed the ultrastructure of mitochondria in 24 DAP WT and Zmbt1-1 endosperms using ultrafast high-pressure freezing fixantion and transmission electron microscopy. As a control, we also analyzed leaf mitochondria.
As shown in Figures 8A,C mitochondria of leaves from the two genotypes exhibited a similar, conventional morphology, being oval or elongated, and having conspicuous cristae distributed throughout the matrix. In striking contrast, whereas mitochondria of WT endosperms were elongated and had cristae (Figure 8B, Supplemental Figure 5A), the vast majority of mitochondria in Zmbt1-1 endosperms were round-oval in shape and had no cristae in the matrix (Figure 8D, Supplemental Figure 5B). In Zmbt1-1 endosperm mitochondria, only small, round cristae-like invaginations were identified at the organelle periphery closely apposed to the inner membrane (Figure 8D). To investigate whether the ultrastructural differences in mitochondria of Zmbt1-1 endosperms could be a consequence of reduced starch content, we conducted high-pressure freezing and transmission electron microscopy analyses of 24 DAP endosperms of Sh2, a starch-deficient mutant lacking the large SH2 subunit of AGP (Bhave et al., 1990).
Figure 8. Ultrastructure of mitochondria (m) in leaves (A,C,E,G) and 24 DAP endosperms (B,D,F,H) of WT (A,B), Zmbt1-1 (C,D), Sh2 (E,F), and Zmbt1-1::MitTPr-ΔTP-ZmBT1-1(1) seeds (G,H). Arrowheads point to mitochondrial cristae. Note their absence in (D), where only round, peripheral invaginations (arrows) are observed. chl, chloroplast; ct, cytoplasm; cw, cell wall; v, vacuole. Bars: 100 nm.
As shown in Figures 8E,F mitochondria of Sh2 leaves and endosperms exhibited conventional morphology, indicating that the aberrant morphology of Zmbt1-1 endosperm mitochondria is not due to reduced starch.
We next addressed the possibility that the aberrant ultrastructure of mitochondria in Zmbt1-1 endosperms might be related to the absence of mitochondrial ZmBT1-1. To this end we analyzed mitochondria from Zmbt1-1::MitTPr-ΔTP-ZmBT1-1 plants. As shown in Figures 8G,H Zmbt1-1::MitTPr-ΔTP-ZmBT1-1 leaf and endosperm mitochondria exhibited a ultrastructure indistinguishable from that of WT leaf and endosperm mitochondria. Overall, the data showed that a lack of mitochondrial ZmBT1-1 expression results in aberrant development and architecture of the mitochondria in maize endosperm.
The channeling of incoming sucrose into sink metabolism in the maize endosperm requires its cleavage by invertase and SuSy. In maize, the major endosperm invertase, CWI-2, is involved in the transport of photoassimilates into the developing kernel, and its expression is highest early in seed development in the lower endosperm (Cheng et al., 1996; Prioul et al., 2008). The main SuSy isoform in maize endosperms, SH1, is involved in cellulose and starch synthesis (Shannon et al., 1996; Chourey et al., 1998; Thévenot et al., 2005). During seed development, SH1 is expressed first in the upper endosperm; expression then extends to the lower endosperm (Doehlert et al., 1988; Prioul et al., 2008). Like that of ZmBT1-1, SH1 expression increases from the 14 DAP developmental stage and parallels starch accumulation (Doehlert et al., 1988; Méchin et al., 2007; Prioul et al., 2008; Li et al., 2013). Increased SuSy activity during endosperm development can therefore be regarded as a marker for the onset of endosperm starch filling (Prioul et al., 2008).
Differential proteomic analysis of developing Zmbt1-1 and WT endosperms indicated that glycolytic metabolism of sucrose breakdown products generated by CWI-2, and their subsequent conversion into ethanol and alanine, is more active in Zmbt1-1 than in WT endosperms, as expression levels of CWI-2, SDH, FK, and enzymes of glycolysis, ethanolic fermentation and the TCA cycle were higher in Zmbt1-1 endosperms than in WT endosperms (Supplemental Table 1, Figure 4). Furthermore, expression level of alanine aminotransferase was lower in Zmbt1-1 endosperms than in WT endosperms (Supplemental Table 1, Figure 4). These analyses also indicated that channeling of sucrose into starch metabolism is less active in Zmbt1-1 than in WT endosperms, as expression levels of SH1 and several SSS isoforms in Zmbt1-1 endosperms were lower than in WT endosperms (Supplemental Table 1, Figure 4). It thus appears that ZmBT1-1 expression is an important determinant of the switch from invertase- to SuSy-mediated metabolism of the incoming sucrose in maize endosperms. This idea, which is schematically illustrated in Figure 9A, is supported by the fact that the contents of ethanol, alanine and its precursor GABA in developing Zmbt1-1 endosperms are higher than those in WT endosperms (Figure 7). The hypothesis is given further weight by the results of analyzing total invertase and SuSy activities during the development of WT and Zmbt1-1 endosperms, which show that, in contrast to WT endosperms, total invertase and SuSy activities do not vary much during the development of Zmbt1-1 endosperms (Figure 6). The fact that starch accumulates only in the upper part of developing Zmbt1-1 endosperms (Figure 2D) would strongly indicate that the reduced starch content in this mutant is partly due to impairments in the developmental activation of SH1 expression in the lower part of the endosperm.
Figure 9. Metabolic schemes illustrating sucrose metabolism pathways in maize endosperms. Panel (A) illustrates differences between Zmbt1-1 and WT endosperms. Enzymatic activities and pathways that are up-regulated in Zmbt1-1 endosperms are highlighted with large letters and thick arrows, respectively, whereas enzymatic activities and pathways that are down-regulated in Zmbt1-1 endosperms are highlighted with small letters and gray arrows, respectively. Panel (B) illustrates differences between Zmbt1-1 and Zmbt1-1::MitTPr-ΔTP-ZmBT1-1 endosperms. Enzymatic activities and pathways that are up-regulated in Zmbt1-1::MitTPr-ΔTP-ZmBT1-1 endosperms are highlighted with large, black arrows, whereas enzymatic activities and pathways that are down-regulated in Zmbt1-1::MitTPr-ΔTP-ZmBT1-1 endosperms are highlighted with small letters and gray arrows, respectively. In (B), note the operation of an as-yet unidentified plastidial transporter (?) that facilitates the incorporation of cytosolic ADPglucose into the amyloplast of Zmbt1-1::MitTPr-ΔTP-ZmBT1-1 endosperms. ADH, alcohol dehydrogenase; ALD, aldolase; G3PDH, glyceraldehyde-3-phosphate dehydrogenase; GBSS, granule-bound starch synthase; HK, hexokinase; PDC, pyruvate decarboxylase; PFP, pyrophosphate–fructose-6-phosphate 1-phosphotransferase; PGI, glucose-6-phosphate isomerase; PGK, phosphoglycerate kinase; PGM, phosphoglucomutase; SP, starch phosphorylase; TPI, triose-phosphate-isomerase; UGP, UDPglucose pyrophosphorylase; XI, xylose isomerase.
The results presented in Figure 2, which show that Zmbt1-1::ΔTP-ZmBT1-1 and Zmbt1-1::MitTPr-ΔTP-ZmBT1-1 endosperms accumulate WT levels of starch, demonstrate that the delivery of ZmBT1-1 specifically to mitochondria is enough to complement the “low starch content” phenotype of Zmbt1-1 endosperms. Differential proteomic analysis of developing Zmbt1-1 and Zmbt1-1::MitTPr-ΔTP-ZmBT1-1 endosperms showed that mitochondrial expression of ZmBT1-1 in Zmbt1-1 endosperms up-regulates the expression of enzymes involved in the sucrose-to-starch conversion process (i.e., SH1, AGP, starch phosphorylase, SSS, and granule-bound starch synthase), and down-regulates the expression of CWI-2, SDH, FK, and glycolytic enzymes (Supplemental Table 3, Figure 5). These data indicate that, as schematically illustrated in Figure 9B, delivery of ZmBT1-1 specifically to mitochondria in Zmbt1 endosperms reduces the glycolytic conversion of sucrose breakdown products generated by CWI-2 into ethanol and alanine, and enhances the SH1-mediated sucrose-to-starch conversion pathway. It therefore appears that mitochondrial ZmBT1-1 plays a key role in the transition from invertase- to SuSy-mediated metabolism of the incoming sucrose during endosperm development and thus acts as a major determinant of the metabolic fate of incoming sucrose. In support of this view, analyses of enzymatic activities during endosperm development revealed that the delivery of ZmBT1-1 specifically to mitochondria restored the WT patterns of total invertase and SuSy activities (Figure 6). Furthermore, metabolic analyses showed that levels of ethanol, GABA, and alanine in 24 DAP Zmbt1-1::MitTPr-ΔTP-ZmBT1-1 endosperms were lower than those in Zmbt1-1 endosperms, and similar to those of WT endosperms (Figure 7). Notably, these analyses also revealed that mitochondrial expression of ZmBT1-1 rescued WT contents of starch, ADPglucose, and other metabolic intermediates of the sucrose-to-starch conversion process in Zmbt1-1 endosperms (Figures 2, 3).
Zmbt1-1 endosperms accumulate high levels of ADPglucose in the cytosol, which can be due to reduced ZmBT1-1-mediated transport of ADPglucose from the cytosol to the amyloplast (Shannon et al., 1996). Plastidial ZmBT1-1-lacking Zmbt1-1::ΔTP-ZmBT1-1 and Zmbt1-1::MitTPr-ΔTP-ZmBT1-1 endosperms accumulate WT levels of ADPG and starch (Figure 3), which indicates that plastidial ZmBT1-1 is not strictly required for normal starch production in maize endosperms. One explanation to this phenomenon could be that specific delivery of ZmBT1-1 to mitochondria promotes the transit of cytosolic G6P into the amyloplast for its subsequent conversion to starch as schematically illustrated in Figure 9B. In such case, however, high ADPG levels would be expected to occur in Zmbt1-1::ΔTP-ZmBT1-1 and Zmbt1-1::MitTPr-ΔTP-ZmBT1-1 endosperm cells. Alternatively, the enhancement of starch content and the reduction of ADPG content to WT levels in Zmbt1-1 endosperms by specific delivery of ZmBT1-1 to mitochondria could be due to the fact that delivery of ZmBT1-1 to the mitochondrial compartment of Zmbt1-1 endosperm cells causes the up-regulation of the expression (or the activation) of an as-yet unidentified plastidial ADPglucose transporter enabling the transport of ADPglucose from the cytosol to the amyloplast. At present it is not possible to draw any firm conclusions as to the class of the translocator responsible for moving ADPglucose across the amyloplast envelope membrane of Zmbt1-1::ΔTP-ZmBT1-1 and Zmbt1-1::MitTPr-ΔTP-ZmBT1-1 endosperms. However, we must emphasize that amyloplasts from plants and organs other than cereal endosperms are capable of transporting ADPglucose (Pozueta-Romero et al., 1991; Naeem et al., 1997). Furthermore, amyloplasts isolated from bt1 maize and rice endosperms still possess 25% of the capacity of WT amyloplasts to transport ADPglucose (Shannon et al., 1998; Cakir et al., 2016). This residual activity appears to be catalyzed by a uniporter system, as ADPglucose incorporation into isolated amyloplasts of bt1 rice endosperms is not stimulated by preincubation with ADP (Cakir et al., 2016). Recent studies have shown that nucleoside transporters are capable of transporting extracellular ADPglucose into bacteria (Almagro et al., 2018). Therefore, it is tempting to speculate that plastidial nucleoside transporters could be involved, at least partly, in the transport of ADPglucose across the envelope membrane of Zmbt1-1::ΔTP-ZmBT1-1 and Zmbt1-1::MitTPr-ΔTP-ZmBT1-1 endosperms.
Mitochondria are sources of specific signaling molecules that relay information on their energetic and metabolic status to the nucleus. Under conditions in which mitochondrial functioning is compromised, the cell is capable of modulating the expression of nuclear-encoded proteins through a retrograde regulation process, so as to partially counteract the energy crisis that ensues (Rhoads and Subbaiah, 2007; Chandel, 2014). For instance, under conditions of oxygen deprivation, or when the expression of proteins that are important for proper mitochondrial function is compromised, plants down-regulate storage metabolism, up-regulate glycolysis to maintain ATP synthesis, enhance ethanolic fermentative metabolism to regenerate the NAD necessary for glycolysis, and accumulate alanine to store carbon and nitrogen, regulate intracellular pH balance or prevent pyruvate and lactate accumulation (Wiseman et al., 1977; Paumard, 2002; Miyashita and Good, 2008; Busi et al., 2011; Shingaki-Wells et al., 2014). Under such conditions, mitochondria fail to develop normally and exhibit signs of degeneration (Shingaki-Wells et al., 2014). Results presented in this work provide strong evidence that mitochondrial ZmBT1-1 plays an important role in mitochondrial function, energy provision and primary metabolism in maize endosperms, and indicate that its absence invokes changes in the expression of nuclear-encoded proteins to compensate for the associated metabolic perturbations. This is supported by facts showing that (i) vast majority of mitochondria in Zmbt1-1 endosperms are aberrant (Figure 8), (ii) Zmbt1-1 endosperms accumulate low levels of starch and high levels of ethanol and alanine in Zmbt1-1 endosperms (Figures 3, 7) due to altered expression of nuclear-encoded starch biosynthetic enzymes and enzymes involved in glycolysis and ethanol and alanine production (Figure 4, Supplemental Table 1); and (iii) delivering ZmBT1-1 specifically to mitochondria reverts the Zmbt1-1 phenotype and protein expression pattern (Figures 2–8, Supplemental Table 3).
Dual targeting of proteins to mitochondria and plastids occurs mainly when proteins have overlapping functions in the two organelles (Smith et al., 1998; Peeters and Small, 2001; Goggin et al., 2003; Christensen et al., 2005; Duchêne et al., 2005; Kmiec et al., 2014), although dually targeted proteins may also fulfill different roles in plastids and mitochondria. In some cases, proteins with dual targeting play important roles in mitochondria, but not in plastids (Tarasenko et al., 2016).
ZmBT1-1 and homologs in other cereal species are counter-exchange transporters that recognize not only ADPglucose, but also ADP and AMP (Shannon et al., 1998; Bowsher et al., 2007; Cakir et al., 2016). Accordingly, as illustrated in Figure 10, we propose that in maize endosperms ZmBT1-1 could play a decisive role in exporting AMP from mitochondria to the cytosol in exchange for ADP. Such transporters are not without precedent, since mitochondrial AMP exporters have been reported in both yeast and mammals (Fiermonte et al., 2004; Todisco et al., 2006). Possible sources of AMP in the mitochondrial matrix include reactions leading to the activation of amino acids for protein synthesis, and formation of various CoA-derivatives (catalyzed by amino-acyl tRNA synthetases and acyl-activating enzymes, respectively) (Shockey et al., 2003; Duchêne et al., 2005; Igamberdiev and Kleczkowski, 2006). Although it has been suggested that de novo purine biosynthesis that leads to AMP production is exclusively located in plastids (Zrenner et al., 2006), immunolocalization and proteomic analyses have provided evidence that enzymes of the de novo purine biosynthesis pathway are also localized to mitochondria in several organs and species (Atkins et al., 1997; Goggin et al., 2003; cf. Supplemental Table 3, Krath and Hove-Jensen, 1999; Huang et al., 2009; cf. Supplemental Table 1, Lee et al., 2013).
Figure 10. Metabolic scheme illustrating the suggested roles of ZmBT1-1 in the mitochondrion and in the amyloplast of maize endosperms. In amyloplasts, ZmBT1-1 could play a role in facilitating the incorporation of cytosolically produced ADPglucose in exchange for newly synthesized AMP or ADP produced by the starch synthase reaction. In mitochondria, ZmBT1-1 could play a decisive role in exporting AMP to the cytosol in exchange for ADP which, once in the matrix, could be converted to ATP by oxidative phosphorylation. ATP generated in the mitochondrion could be exported to the cytosol by means of the ADP/ATP carrier. GBSS, granule-bound starch synthase; PGM, phosphoglucomutase; SP, starch phosphorylase; UGP, UDP glucose pyrophosphorylase.
ADP incorporated into the matrix by mitochondrial ZmBT1-1 could be converted to ATP to be exported to the cytosol by means of the ADP/ATP carrier (Figure 10). Thus, the net balance inherent in the suggested mechanism of ZmBT1-1-mediated adenylate transport in maize endosperms would imply the export to the cytosol of one molecule each of mitochondrially synthesized AMP and ATP in exchange for two molecules of cytosolic ADP (Figure 10). Mitochondrial ZmBT1-1 could therefore play a role in energy provision by mitochondria in maize endosperms. In amyloplasts, ZmBT1-1 could participate in facilitating the incorporation of cytosolic ADPglucose in exchange for newly synthesized AMP or ADP produced by the starch synthase reaction (Figure 10).
AB, FM, JS-S, MO, EB-F, and JP-R designed the experiments and analyzed the data. AB, FM, CC-F, AR-S, VP-V, MO, JL, ÁS-L, GA, and EB-F performed most of experiments. AB, JS-S, and JP-R supervised the experiments. AB, JS-S, and JP-R wrote the article.
This research was partially supported by the grants BIO2010-18239, BI2013-49125-C2-2-P and BIO2016-78747-P from the Comisión Interministerial de Ciencia y Tecnología and Fondo Europeo de Desarrollo Regional (Spain) and by the ERDF project Plants as a tool for sustainable global development (No. CZ.02.1.01/0.0/0.0/16_019/0000827).
The authors declare that the research was conducted in the absence of any commercial or financial relationships that could be construed as a potential conflict of interest.
We acknowledge support of the publication fee by the CSIC Open Access Publication Support Initiative through its Unit of Information Resources for Research (URICI). We thank Francisco Carreto-Cano (Institute of Agrobiotechnology of Navarra) for technical support.
The Supplementary Material for this article can be found online at: https://www.frontiersin.org/articles/10.3389/fpls.2019.00242/full#supplementary-material
AGP, ADPglucose pyrophosphorylase; CWI-2, Cell wall invertase 2; DAP, days after pollination; DEPs, differentially expressed proteins; FK, fructokinase; G6P, glucose-6-phosphate; MCF, Mitochondrial carrier family; SuSy, sucrose synthase; SSS, soluble starch synthase; SDH, sorbitol dehydrogenase; TP, transit peptide; ZmBT1-1, Zea mays Brittle1-1.
Almagro, G., Viale, A. M., Montero, M., Muñoz, F. J., Baroja-Fernández, E., Mori, H., et al. (2018). A cAMP/CRP-controlled mechanism for the incorporation of extracellular ADP-glucose in Escherichia coli involving NupC and NupG nucleoside transporters. Sci. Rep. 8:15509. doi: 10.1038/s41598-018-33647-w
Armstrong, C. L., Green, C. E., and Phillips, R. L. (1991). Development and availability of germplasm with high Type II culture formation response. Maize Genet. Coop. Newsl. 65, 92–93.
Atkins, C. A., Smith, P., and Storer, P. J. (1997). Reexamination of the intracellular localization of de novo purine synthesis in cowpea nodules. Plant Physiol. 113, 127–135. doi: 10.1104/pp.113.1.127
Bahaji, A., Li, J., Sánchez-López, A. M., Baroja-Fernández, E., Muñoz, F. J., Ovecka, M., et al. (2014). Starch biosynthesis, its regulation and biotechnological approaches to improve crop yields. Biotechnol. Adv. 32, 87–106. doi: 10.1016/j.biotechadv.2013.06.006
Bahaji, A., Muñoz, F. J., Ovecka, M., Baroja-Fernández, E., Montero, M., Li, J., et al. (2011a). Specific delivery of AtBT1 to mitochondria complements the aberrant growth and sterility phenotype of homozygous Atbt1 Arabidopsis mutants. Plant J. 68, 1115–1121. doi: 10.1111/j.1365-313X.2011.04767.x
Bahaji, A., Ovecka, M., Bárány, I., Risueño, M. C., Muñoz, F. J., Baroja-Fernández, E., et al. (2011b). Dual targeting to mitochondria and plastids of AtBT1 and ZmBT1, two members of the mitochondrial carrier family. Plant Cell Physiol. 52, 597–609. doi: 10.1093/pcp/pcr019
Baroja-Fernández, E., Muñoz, F. J., Montero, M., Etxeberria, E., Sesma, M. T., Ovecka, M., et al. (2009). Enhancing sucrose synthase activity in transgenic potato (Solanum tuberosum L.) tubers results in increased levels of starch, ADPglucose and UDPglucose and total yield. Plant Cell Physiol. 50, 1651–1662. doi: 10.1093/pcp/pcp108
Bedhomme, M., Hoffmann, M., McCarthy, E. A., Gambonnet, B., Moran, R. G., Rébeillé, F., et al. (2005). Folate metabolism in plants: an Arabidopsis homolog of the mammalian mitochondrial folate transporter mediates folate import into chloroplasts. J. Biol. Chem. 280, 34823–34831. doi: 10.1074/jbc.M506045200
Bhave, M. R., Lawrence, S., Barton, C., and Hannah, L. C. (1990). Identification and molecular characterization of shrunken-2 cDNA clones of maize. Plant Cell 2, 581–558. doi: 10.1105/tpc.2.6.581
Boehlein, S. K., Shaw, J. R., Boehlein, T. J., Boehlein, E. C., and Hannah, L. C. (2018). Fundamental differences in starch synthesis in the maize leaf, embryo, ovary and endosperm. Plant J. 96, 595–606. doi: 10.1111/tpj.14053
Bowsher, C. G., Scrase-Field, E. F., Esposito, S., Emes, M. J., and Tetlow, I. J. (2007). Characterization of ADP-glucose transport across the cereal endosperm amyloplast envelope. J. Exp. Bot. 58, 1321–1332. doi: 10.1093/jxb/erl297
Busi, M. V., Gomez-Lobato, M. E., Rius, S. P., Turowski, V. R., Casati, P., Zabaleta, E. J., et al. (2011). Effect of mitochondrial dysfunction on carbon metabolism and gene expression in flower tissues of Arabidopsis thaliana. Mol. Plant 4, 127–143. doi: 10.1093/mp/ssq065
Cakir, B., Shiraishi, S., Tuncel, A., Matsusaka, H., Satoh, R., Singh, S., et al. (2016). Analysis of the rice ADP-glucose transporter (OsBT1) indicates the presence of regulatory processes in the amyloplast stroma that control ADP-glucose flux into starch. Plant Physiol. 170, 1271–1283. doi: 10.1104/pp.15.01911
Cao, H., and Shannon, J. C. (1996). BT1, a protein critical for in vivo starch accumulation in maize endosperm, is not detected in maize endosperm suspension cultures. Physiol. Plant 97, 665–673. doi: 10.1111/j.1399-3054.1996.tb00530.x
Cao, H., Sullivan, T. D., Boyer, C. D., and Shannon, J. C. (1995). Btl, a structural gene for the major 39–44 kDa amyloplast membrane polypeptides. Physiol. Plant 95, 176–186. doi: 10.1111/j.1399-3054.1995.tb00825.x
Chandel, N. S. (2014). Mitochondria as signaling organelles. BMC Biol. 12:34. doi: 10.1186/1741-7007-12-34
Cheng, W. H., Taliercio, E. W., and Chourey, P. S. (1996). The miniature1 seed locus of maize encodes a cell wall invertase required for normal development of endosperm and maternal cells in the pedicel. Plant Cell 8, 971–983. doi: 10.1105/tpc.8.6.971
Chourey, P. S., Taliercio, E. W., Carlson, S. J., and Ruan, Y. L. (1998). Genetic evidence that the two isozymes of sucrose synthase present in developing maize endosperm are critical, one for cell wall integrity and the other for starch biosynthesis. Mol. Gen. Genet. 259, 88–96. doi: 10.1007/s004380050792
Christensen, A. C., Lyznik, A., Mohammed, S., Elowsky, C. G., Elo, A., Yule, R., et al. (2005). Dual-domain, dual-targeting organellar protein presequences in Arabidopsis can use non-AUG start codons. Plant Cell 17, 2805–2816. doi: 10.1105/tpc.105.035287
Christensen, A. H., and Quail, P. H. (1996). Ubiquitin promoter-based vectors for high-level expression of selectable and/or screenable marker genes in monocotyledonous plants. Transgenic Res. 5, 213–218. doi: 10.1007/BF01969712
Doehlert, D. C., Kuo, T. M., and Felker, F. C. (1988). Enzymes of sucrose and hexose metabolism in developing kernels of two inbreds of maize. Plant Physiol. 86, 1013–1019. doi: 10.1104/pp.86.4.1013
Duchêne, A. M., Giritch, A., Hoffmann, B., Cognat, V., Lancelin, D., Peeters, N. M., et al. (2005). Dual targeting is the rule for organellar aminoacyl-tRNA synthetases in Arabidopsis thaliana. Proc. Natl. Acad. Sci. U.S.A. 102, 16484–16489. doi: 10.1073/pnas.0504682102
Emanuelsson, O., Nielsen, H., Brunak, S., and von Heijne, G. (2000). Predicting subcellular localization of proteins based on their N-terminal amino acid sequence. J. Mol. Biol. 300, 1005–1016. doi: 10.1006/jmbi.2000.3903
Fiermonte, G., De Leonardis, F., Todisco, S., Palmieri, L., Lasorsa, F. M., and Palmieri, F. (2004). Identification of the mitochondrial ATP-Mg/Pi transporter: bacterial expression, reconstitution, functional characterization, and tissue distribution. J. Biol. Chem. 279, 30722–30730. doi: 10.1074/jbc.M400445200
Fukao, Y., Hayashi, Y., Mano, S., Hayashi, M., and Nishimura, M. (2001). Developmental analysis of a putative ATP/ADP carrier protein localized on glyoxysomal membranes during the peroxisome transition in pumpkin cotyledons. Plant Cell Physiol. 42, 835–841. doi: 10.1093/pcp/pce108
Goggin, D. E., Lipscombe, R., Federova, E., Millar, A. H., Mann, A., Atkins, C. A., et al. (2003). Dual intracellular localization and targeting of aminoimidazole ribonucleotide synthetase in cowpea. Plant Physiol. 131, 1033–1041. doi: 10.1104/pp.102.015081
Haferkamp, I., and Schmitz-Esser, S. (2012). The plant mitochondrial carrier family: functional and evolutionary aspects. Front. Plant Sci. 3:2. doi: 10.3389/fpls.2012.00002
Huang, S., Taylor, N. L., Narsai, R., Eubel, H., Whelan, J., and Millar, A. H. (2009). Experimental analysis of the rice mitochondrial proteome, its biogenesis, and heterogeneity. Plant Physiol. 149, 719–734. doi: 10.1104/pp.108.131300
Igamberdiev, A. U., and Kleczkowski, L. A. (2006). Equilibration of adenylates in the mitochondrial intermembrane space maintains respiration and regulates cytosolic metabolism. J. Exp. Bot. 57, 2133–2141. doi: 10.1093/jxb/erl006
Kirchberger, S., Leroch, M., Huynen, M. A., Wahl, M., Neuhaus, H. E., and Tjaden, J. (2007). Molecular and biochemical analysis of the plastidic ADP-glucose transporter (ZmBT1) from Zea mays. J. Biol. Chem. 282, 22481–22491. doi: 10.1074/jbc.M702484200
Kleczkowski, L. A. (1996). Back to the drawing board: redefining starch synthesis in cereals. Trends Plant Sci. 1, 363–364. doi: 10.1016/1360-1385(96)83884-2
Kmiec, B., Teixeira, P. F., and Glaser, E. (2014). Shredding the signal: targeting peptide degradation in mitochondria and chloroplasts. Trends Plant Sci. 19, 771–778. doi: 10.1016/j.tplants.2014.09.004
Krath, B. N., and Hove-Jensen, B. (1999). Organellar and cytosolic localization of four phosphoribosyl diphosphate synthase isozymes in spinach. Plant Physiol. 119, 497–506. doi: 10.1104/pp.119.2.497
Lee, C. P., Taylor, N. L., and Millar, A. H. (2013). Recent advances in the composition and heterogeneity of the Arabidopsis mitochondrial proteome. Front. Plant Sci. 4:4. doi: 10.3389/fpls.2013.00004
Li, H. M., Sullivan, T. D., and Keegstra, K. (1992). Information for targeting to the chloroplastic inner envelope membrane is contained in the mature region of the maize Bt1-encoded protein. J. Biol. Chem. 267, 18999–19004.
Li, J., Baroja-Fernández, E., Bahaji, A., Muñoz, F. J., Ovecka, M., Montero, M., et al. (2013). Enhancing sucrose synthase activity results in increased levels of starch and ADP-glucose in maize (Zea mays L.) seed endosperms. Plant Cell Physiol. 54, 282–294. doi: 10.1093/pcp/pcs180
Licausi, F., van Dongen, J. T., Giuntoli, B., Novi, G., Santaniello, A., Geigenberger, P., et al. (2010). HRE1 and HRE2, two hypoxia-inducible ethylene response factors, affect anaerobic responses in Arabidopsis thaliana. Plant J. 62, 302–315. doi: 10.1111/j.1365-313X.2010.04149.x
Loiret, F. G., Grimm, B., Hajirezaei, M. R., Kleiner, D., and Ortega, E. (2009). Inoculation of sugarcane with Pantoea sp. increases amino acid contents in shoot tissues; serine, alanine, glutamine and asparagine permit concomitantly ammonium excretion and nitrogenase activity of the bacterium. J. Plant Physiol. 166, 1152–1161. doi: 10.1016/j.jplph.2009.01.002
Mangelsdorf, P. C. (1926). The genetics and morphology of some endosperm characters in maize. Conn. Agric. Exp. Stn. Bull. 279, 509–614.
Méchin, V., Thévenot, C., Le Guilloux, M., Prioul, J.-L., and Damerval, C. (2007). Developmental analysis of maize endosperm proteome suggests a pivotal role for pyruvate orthophosphate dikinase. Plant Physiol. 143, 1203–1219. doi: 10.1104/pp.106.092148
Miyashita, Y., and Good, A. G. (2008). Contribution of the GABA shunt to hypoxia-induced alanine accumulation in roots of Arabidopsis thaliana. Plant Cell Physiol. 49, 92–102. doi: 10.1093/pcp/pcm171
Naeem, M., Tetlow, I. J., and Emes, M. J. (1997). Starch synthesis in amyloplasts purified from developing potato tubers. Plant J. 11, 1095–1103. doi: 10.1046/j.1365-313X.1997.11051095.x
Niwa, Y., Hirano, T., Yoshimoto, K., Shimizu, M., and Kobayashi, H. (1999). Non-invasive quantitative detection and applications of non-toxic, S65T-type green fluorescent protein in living plants. Plant J. 18, 455–463. doi: 10.1046/j.1365-313X.1999.00464.x
Palmieri, L., Arrigoni, R., Blanco, E., Carrari, F., Zanor, M. I., Studart-Guimaraes, C., et al. (2006). Molecular identification of an Arabidopsis S-adenosylmethionine transporter. Analysis of organ distribution, bacterial expression, reconstitution into liposomes, and functional characterization. Plant Physiol. 142, 855–865. doi: 10.1104/pp.106.086975
Paumard, P. (2002). The ATP synthase is involved in generating mitochondrial cristae morphology. EMBO J. 21, 221–230. doi: 10.1093/emboj/21.3.221
Peeters, N., and Small, I. (2001). Dual targeting to mitochondria and chloroplasts. Biochim. Biophys. Acta Mol. Cell Res. 54–63. doi: 10.1016/S0167-4889(01)00146-X
Pozueta-Romero, J., Ardila, F., and Akazawa, T. (1991). ADP-glucose transport by the chloroplast adenylate translocator is linked to starch biosynthesis. Plant Physiol. 97, 1565–1572. doi: 10.1104/pp.97.4.1565
Prioul, J. L., Méchin, V., Lessard, P., Thévenot, C., Grimmer, M., Chateau-Joubert, S., et al. (2008). A joint transcriptomic, proteomic and metabolic analysis of maize endosperm development and starch filling. Plant Biotechnol. J. 6, 855–869. doi: 10.1111/j.1467-7652.2008.00368.x
Reynolds, E. S. (1963). The use of lead citrate at high pH as an electron-opaque stain in electron microscopy. J. Cell Biol. 17, 208–212. doi: 10.1083/jcb.17.1.208
Rhoads, D. M., and Subbaiah, C. C. (2007). Mitochondrial retrograde regulation in plants. Mitochondrion 7, 177–194. doi: 10.1016/j.mito.2007.01.002
Sánchez-López, Á. M., Bahaji, A., De Diego, N., Baslam, M., Li, J., Muñoz, F. J., et al. (2016). Arabidopsis responds to Alternaria alternata volatiles by triggering plastid phosphoglucose isomerase-independent mechanisms. Plant Physiol. 172, 1989–2001. doi: 10.1104/pp.16.00945
Seguí-Simarro, J. M. (2015). “High-pressure freezing and freeze substitution of in vivo and in vitro cultured plant samples,” in Plant Microtechniques and Protocols, eds E. C. T. Yeung, C. Stasolla, M. J. Sumner, and B.Q. Huang (Cham: Springer International Publishing), 117–134.
Shannon, J. C., Pien, F., and Liu, K. (1996). Nucleotides and nucleotide sugars in developing maize endosperms (Synthesis of ADP-glucose in brittle-1). Plant Physiol. 110, 835–843. doi: 10.1104/pp.110.3.835
Shannon, J. C., Pien, F. M M., Cao, H., and Liu, K. C. (1998). Brittle-1, an adenylate translocator, facilitates transfer of extraplastidial synthesized ADP–glucose into amyloplasts of maize endosperms. Plant Physiol. 117, 1235–1252. doi: 10.1104/pp.117.4.1235
Shingaki-Wells, R., Millar, A. H., Whelan, J., and Narsai, R. (2014). What happens to plant mitochondria under low oxygen? An omics review of the responses to low oxygen and reoxygenation. Plant Cell Environ. 37, 2260–2277. doi: 10.1111/pce.12312
Shockey, J. M., Fulda, M. S., and Browse, J. (2003). Arabidopsis contains a large superfamily of acyl-activating enzymes. Phylogenetic and biochemical analysis reveals a new class of acyl-coenzyme A synthetases. Plant Physiol. 132, 1065–1076. doi: 10.1104/pp.103.020552
Smith, P. M. C., Mann, A. J., Goggin, D. E., and Atkins, C. A. (1998). Air synthetase in cowpea nodules: a single gene product targeted to two organelles? Plant Mol. Biol. 36, 811–820. doi: 10.1023/A:1005969830314
Sullivan, T. D., and Kaneko, Y. (1995). The maize brittle1 gene encodes amyloplast membrane polypeptides. Planta An Int. J. Plant Biol. 196, 477–484. doi: 10.1007/BF00203647
Sullivan, T. D., Strelow, L. I., Illingworth, C. A., Phillips, R. L., and Nelson, O. E. (1991). Analysis of maize brittle-1 alleles and a defective suppressor-mutator-induced mutable allele. Plant Cell 3, 1337–1348. doi: 10.1105/tpc.3.12.1337
Tarasenko, V. I., Katyshev, A. I., Yakovleva, T. V., Garnik, E. Y., Chernikova, V. V., Konstantinov, Y. M., et al. (2016). RPOTmp, an Arabidopsis RNA polymerase with dual targeting, plays an important role in mitochondria, but not in chloroplasts. J. Exp. Bot. 67, 5657–5669. doi: 10.1093/jxb/erw327
Taylor, E. B. (2017). Functional properties of the mitochondrial carrier system. Trends Cell Biol. 27, 663–644. doi: 10.1016/j.tcb.2017.04.004
Thévenot, C., Simond-Côte, E., Reyss, A., Manicacci, D., Trouverie, J., Le Guilloux, M., et al. (2005). QTLs for enzyme activities and soluble carbohydrates involved in starch accumulation during grain filling in maize. J. Exp. Bot. 56, 945–958. doi: 10.1093/jxb/eri087
Thimm, O., Bläsing, O., Gibon, Y., Nagel, A., Meyer, S., Krüger, P., et al. (2004). MAPMAN: a user-driven tool to display genomics data sets onto diagrams of metabolic pathways and other biological processes. Plant J. 37, 914–939. doi: 10.1111/j.1365-313X.2004.02016.x
Todisco, S., Agrimi, G., Castegna, A., and Palmieri, F. (2006). Identification of the mitochondrial NAD+ transporter in Saccharomyces cerevisiae. J. Biol. Chem. 281, 1524–1531. doi: 10.1074/jbc.M510425200
Wang, K., and Frame, B. (2009). Biolistic gun-mediated maize genetic transformation. Methods Mol. Biol. 526, 29–45. doi: 10.1007/978-1-59745-494-0_3
Wiseman, A., Gillham, N. W., and Boynton, J. E. (1977). Nuclear mutations affecting mitochondrial structure and function in Chlamydomonas. J. Cell Biol. 73, 56–77. doi: 10.1083/jcb.73.1.56
Keywords: ADPglucose, dual targeting, mitochondrial carrier family, mitochondrial retrograde signaling, starch, sucrose synthase, Zea mays
Citation: Bahaji A, Muñoz FJ, Seguí-Simarro JM, Camacho-Fernández C, Rivas-Sendra A, Parra-Vega V, Ovecka M, Li J, Sánchez-López ÁM, Almagro G, Baroja-Fernández E and Pozueta-Romero J (2019) Mitochondrial Zea mays Brittle1-1 Is a Major Determinant of the Metabolic Fate of Incoming Sucrose and Mitochondrial Function in Developing Maize Endosperms. Front. Plant Sci. 10:242. doi: 10.3389/fpls.2019.00242
Received: 05 December 2018; Accepted: 13 February 2019;
Published: 12 March 2019.
Edited by:
Andrea Chini, Centro Nacional de Biotecnología (CNB), SpainReviewed by:
Leszek A. Kleczkowski, Umeå University, SwedenCopyright © 2019 Bahaji, Muñoz, Seguí-Simarro, Camacho-Fernández, Rivas-Sendra, Parra-Vega, Ovecka, Li, Sánchez-López, Almagro, Baroja-Fernández and Pozueta-Romero. This is an open-access article distributed under the terms of the Creative Commons Attribution License (CC BY). The use, distribution or reproduction in other forums is permitted, provided the original author(s) and the copyright owner(s) are credited and that the original publication in this journal is cited, in accordance with accepted academic practice. No use, distribution or reproduction is permitted which does not comply with these terms.
*Correspondence: Javier Pozueta-Romero, amF2aWVyLnBvenVldGFAY3NpYy5lcw==
Disclaimer: All claims expressed in this article are solely those of the authors and do not necessarily represent those of their affiliated organizations, or those of the publisher, the editors and the reviewers. Any product that may be evaluated in this article or claim that may be made by its manufacturer is not guaranteed or endorsed by the publisher.
Research integrity at Frontiers
Learn more about the work of our research integrity team to safeguard the quality of each article we publish.