- 1AMAP, IRD, CIRAD, CNRS, INRA, Université de Montpellier, Montpellier, France
- 2AMAP, IRD, Herbier de Nouméa, Nouméa, New Caledonia
- 3Endemia, Plant Red List Authority, Nouméa, New Caledonia
- 4Laboratoire Chrono-Environnement UMR 6249 CNRS, Université Bourgogne Franche-Comté, Besançon, France
- 5Jardin Botanique de la Ville de Besançon et de l'Université de Franche-Comté, Besançon, France
- 6CIRAD, UMR AMAP, Montpellier, France
The diversification of ecological roles and related adaptations in closely related species within a lineage is one of the most important processes linking plant evolution and ecology. Plant architecture offers a robust framework to study these processes as it can highlight how plant structure influences plant diversification and ecological strategies. We investigated a case of gradual evolution of branching architecture in Atractocarpus spp. (Rubiaceae), forming a monophyletic group in New Caledonia that has diversified rapidly, predominantly in rainforest understory habitats. We used a transdisciplinary approach to depict architectural variations and revealed multiple evolutionary transitions from a branched (Stone's architectural model) to a monocaulous habit (Corner's architectural model), which involved the functional reduction of branches into inflorescences. We propose an integrative functional index that assesses branching incidence on functional traits influencing both assimilation and exploration functions. We showed that architectural transitions correlate with ecologically important functional traits. Variation in ecologically important traits among closely relatives, as supported by the architectural analysis, is suggestive of intense competition that favored divergence among locally coexisting species. We propose that Pleistocene climatic fluctuations causing expansion and contraction of rainforest could also have offered ecological opportunities for colonizers in addition to the process of divergent evolution.
Introduction
Convergent and divergent evolution are widely recognized as important drivers of plant diversification at large scale (Givnish and Sytsma, 1997; Gianoli, 2004; Drummond et al., 2012; Couvreur et al., 2015; Givnish, 2016). Well known examples of large scale convergence in plants (i.e., the appearance of phenotypic similarities among distantly related taxa) include the evolution of a cushion growth habit in alpine environments (Boucher et al., 2012, 2016; Aubert et al., 2014), rosette-shrubs in islands (Carlquist, 1974; Givnish, 2010; Lens et al., 2013), climbing mechanisms in lianas (Sousa-Baena et al., 2014, 2018), and succulence in arid environments (Ogburn and Edwards, 2010; Arakaki et al., 2011). These and others examples demonstrate that similar habitats or micro-habitats can produce selective pressures that favor some morphological and physiological traits. Another evolutionary process, sometimes linked with convergence, is the diversification of ecological roles among closely related species, i.e., divergent evolution, which results in the exploitation of different ecological resources (e.g., Bramwell, 1975; Givnish et al., 2009, 2014). Convergent and divergent evolution have been attributed to morpho-physiological traits, whose gradual evolution or rapid innovation can lead to adaptive radiation, sometimes involving increased diversification rates (Givnish and Sytsma, 1997; Givnish et al., 2014; Couvreur et al., 2015). A striking feature of convergent and divergent evolution is that it often involves variation in growth habit, which represents the ultimate form of a plant expressed in its physiognomy (Warming, 1909). Growth habit results from the integration of a set of traits, e.g., branching pattern and structure, body size and shape, position of inflorescences, and anatomy, among others, which have generally been studied independently (e.g., Carlquist, 1984; Rowe and Speck, 2005; Givnish et al., 2009; Isnard et al., 2012; Wagner et al., 2014). Few work has, however, used the integrative approach provided by plant architecture to study the evolution of plant growth habit.
Plant architecture characterizes the spatial arrangement and specialization of structures (morphological origin, branching pattern, axis categorization) and their evolution during ontogeny (Hallé et al., 1978; Barthélémy and Caraglio, 2007). As such, it can highlight how plant structure impacts plant diversification and can help identify evolutionary processes underlying plant evolution (Bateman, 1994, 1999; Sussex and Kerk, 2001; Meyer-Berthaud et al., 2010). Architectural studies have shown that plants are modular organisms comprising elements that can differ in their organization and functions (Hallé et al., 1978; Barthélémy and Caraglio, 2007). For instance, in many tree and treelet species, the trunk functions primarily for exploration and to provide support, while branches are involved in assimilation and reproduction. Plant architecture thus influences spatial and temporal exploitation of resources (Smith et al., 2014). Some architectural traits have been shown to impact plant fitness, either directly (Küppers, 1989; Millet et al., 1999; Charles-Dominique et al., 2010, 2012, 2017; Millan, 2016) or in interaction with other functional traits (Pérez-Harguindeguy et al., 2013; Trueba et al., 2016). Among the best known examples are Corner's rules, which stipulate that “the greater the ramification, the smaller become the branches and their appendages” (leaves, flowers, and fruits, Corner, 1949). This statement points toward an effect of plant architecture on leaf size and linked architecture and plant ecological strategies (White, 1983a; Ackerly, 1996). To date, approaches linking plant architecture and function are scarce although some architectural indexes can assess interesting strategies such as defense against herbivores (“Index of caginess,” Charles-Dominique et al., 2017) or leaf-to-stem relation that trigger flowering (“Index of axialisation,” Lauri, 1988; Lauri and Kelner, 2001).
Despite the functional importance of plant architectural traits, little is known about their evolution (Kurmann and Hemsley, 1999; León Enriquez et al., 2008). In a recent review, Chomicki et al. (2017) investigated the evolution of plant architecture for several groups. Their results showed preferential transitions between models, suggesting pre-requisite morphological ability for the evolution of a given plant architecture. In contrast, some model transitions seem never to occur, suggesting that genetic constraints might prevent them. Chomicki et al. (2017) did not, however, investigate processes involved in plant architectural evolution. Bateman (Bateman and DiMichele, 1994; Bateman, 1999) argued that the changes involved in the transition from one architecture to another are too important and deep for such transitions to take place gradually. Rather, such evolution is suggested to occur preferentially by saltation, which involves the direct shift from one competitive architecture to another without passing through intermediate states associated with lower fitness. Other authors have proposed the notion of an “architectural continuum” (Oldeman, 1974; Barthélémy et al., 1989), suggesting a continuous transition from one model to another in some groups (Hallé et al., 1978). In the context of this diversity of possible models to explain evolution in plant architecture, detailed studies within individual clades that combine results from molecular phylogenetic work with detailed architectural analysis may be particularly informative.
Atractocarpus Schltr. & K. Krause (Gardenieae, Rubiaceae), a Pacific genus of about 40 species, of which 32 are endemics to New Caledonia (Mouly et al., under review), is ideally suited to study the evolution of plant architecture and to test hypotheses regarding the role of architecture in plant ecological diversification. Especially since a recently published phylogeny based on comprehensive sampling is available for the genus (Mouly et al., under review). Most members of this genus are treelets occurring in the understory of tropical and subtropical rainforests, and they exhibit substantial variation in branching pattern (Tirvengadum and Sastre, 1979; Robbrecht and Puff, 1986), ranging from monocaulous (i.e., unbranched) to well-branched species, including various intermediate states (Figure 1). The New Caledonia members of Atractocarpus, which originated from a single colonization event that occurred ca. 2.4 Myr ago (Mouly et al., under review), has the highest diversification rate of any genus studied to date (1.17 species species−1 Myr−1), approaching that of the well-known Hawaiian lobelioid genus Cyanea on the island of Maui (1.36 species species−1 Myr−1, Givnish et al., 2009).
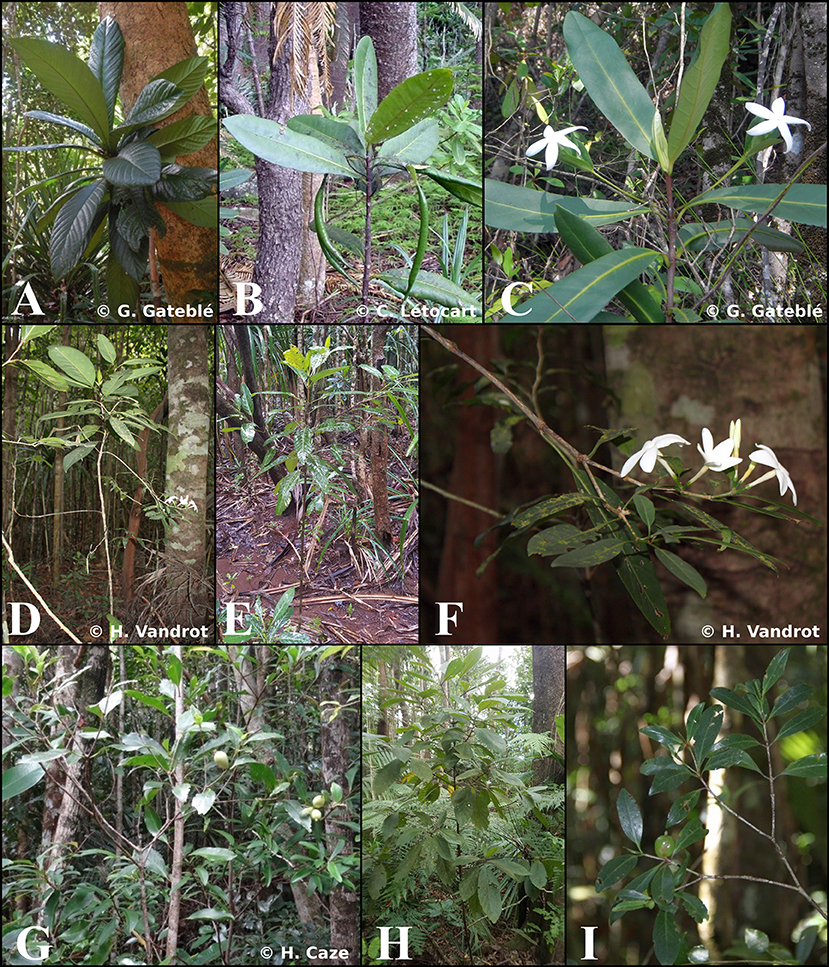
Figure 1. Photographs of different Atractocarpus species in their environment showing variability in growth habit and lateral axis. Monocaulous species: (A) A. confertus, (B) A. bracteatus, (C) A. bracteatus. Intermediate species: (D) A. ngoyensis, (E) A. ngoyensis, (F) A. ngoyensis. Branched species: (G) A. pseudoterminalis, (H) A. sp. nov. 10, (I) A. pseudoterminalis.
The presence of a high diversification rate combined with architectural diversity is thought to provide an indication of ecological diversification, a situation that is often associated with island colonization (Silvertown, 2004; Silvertown et al., 2005). In groups adapted to growing in the forest understory, light is a variable and limiting resource, and in response, plants have deployed various growth strategies related to leaf photosynthesis and the economization of carbon. To quantify the architectural gradient from monocaulous (unbranched) to well-branched species, we developed a new “functional branching index,” which assesses branching incidence on functional traits that influence assimilation and exploration functions. Considering each understory species of Atractocarpus in New Caledonia, we analyzed correlations between architecture and traits associated with key ecologically significant functions (viz., photosynthesis, hydraulic, mechanics, and dispersal). We investigated the evolution of plant architecture (branched vs. unbranched) in forest understory species and its impact on the exceptional diversification rate of the genus. Finally, we explored how ecological opportunities might stimulate the diversification of architecture through spatial differentiation in resource use (niche partitioning) in closely related species following the colonization of New Caledonian rainforests.
Materials and Methods
Sampling
We sampled all the 27 known rainforest species of Atractocarpus occurring in New Caledonia (list of taxa in Appendix 1 in Supplementary Material). For Ancestral Character Estimation (ACE), three Australian Atractocarpus species and one species in each of the most closely related genera for which DNA sequences were available (Mouly et al., 2014, under review) were included as outgroups.
Individuals were sampled in 20 rainforest sites occurring on Grande Terre, New Caledonia's main island (Appendix 1 in Supplementary Material).
Branching Index and Plant Architectural Traits
Based on the observation that an observed architectural gradient might result from differential allocation in branch length and supported leaf area (Figure 1), we used two functional branching indexes based on the differentiation of function from branches to trunk (Corner, 1958). Given that photosynthetic function can be approximated by leaf area (Pérez-Harguindeguy et al., 2013) and exploration function by stem length (Barthélémy and Caraglio, 2007; Smith et al., 2014), branching indexes were calculated as follows:
(i) Photosynthetic branching index:
(ii) Exploration branching index:
A value of zero indicates that photosynthesis and exploration are assumed only by the trunk and that branches (lateral exploration) are lacking, which corresponds to the monocaulous habit, physiognomically defined as “trees with a single trunk or visible stem of the plant” (Hallé et al., 1978). The higher the value of the index, the greater the functional role played by branches. We built a single integrative branching index that expresses the architectural gradient by combining these two variables using Principal Component Analysis (correlation between photosynthetic and exploration branching indexes: rho = 0.86) employed in the ade4 package for R (Dray and Dufour, 2007).
Species were segregated into three architectural classes (Monocaulous, Intermediate, and Branched) using Wilcoxon signed-rank tests that enable detection of breaks in the distribution of the integrative branching index.
Using morphological criteria reviewed by Barthélémy and Caraglio (2007), we then described main traits commonly used in architectural analyses (growth process, branching pattern, position of reproductive structures, etc.) for the 27 New Caledonian species of Atractocarpus. The number of individuals studied varied from more than a hundred in some species (e.g., A. pseudoterminalis, A. ngoyensis and A. bracteatus) to 12 in A. sezitat, a rare species whose habitat is highly disturbed by introduced herbivores. All species were studied at different ontogenetic stages (from very young plants to senescent adults) except A. sezitat and A. sp. nov. 12, for which no young plants could be found.
Plant Functional Traits
We tested the correlation of 14 traits with branching index. The traits were selected to reflect important features of plant ecological strategies (Table 1). They were measured on five individuals per species and, when possible (e.g., for leaf and internode traits), five times per individual. The selection of individuals was standardized for environment (rainforest understory) and for ontogeny following three criteria: (i) recently mature individuals, (ii) non reiterated individuals (see Oldeman, 1974; Barthélémy and Caraglio, 2007), and (iii) non-traumatized trunks. It was not possible to locate individuals meeting all three conditions for A. sezitat and A. sp. nov. 12 due to small population sizes and habitat degradation, so these taxa were removed from this analysis. We concentrated our sampling in the apical part of the trunk because (i) this enabled standardization of physiological age and (ii) unbranched and branched species can be compared only on the basis of the main stem. Moreover, variation in resource allocation and anatomy between unbranched and branched species might be more prevalent toward the apex, where large leaves are deployed (Carlquist, 1974). Stem and leaf measurements were standardized as follows. Leaf traits (SLA, leaf area) were measured on the five youngest, fully expended leaves of the main axis (trunk). Wood was collected below the terminal leaf tuft for the measurement of anatomical traits (proportion of tissues, specific wood density, and specific stem density). Internode length and diameter were measured on the five youngest, well-developed trunk-internodes (before secondary growth). Branch traits (cumulated length, leaf number, leaf area) were measured on two representative branches per individual and the total number of branches was counted.
Most of the individuals studied in the field were infertile, so fruit traits were measured on herbarium specimens [NOU and P; acronyms follow Thiers (continuously updated)]. We selected undamaged and unflattened fruits from which length and diameter were measured. As Atractocarpus fruits are often ellipsoid to tubular (Puttock, 1999), these two dimensions are used to approximate fruit volume (Table 1). A total of 592 fruits were measured, and only the five largest fruits per taxon (four for A. confertus and A. sessilifolius) were used to ensure that values from only mature fruits were included in our analyses. One of the species, A. sp. nov. 6, is a very rare species whose fruits have never been observed; it was consequently excluded from the fruit volume dataset.
Data Analysis
Phylogenetic Tree
The molecular phylogeny of Mouly et al. (under review) was used for analysis. This ultrametric phylogenetic tree was constructed under Bayesian Inference using one nuclear (ITS) and two chloroplastic (trnTL, rpl32) loci. Since the current study focused on rainforest species, we pruned the five dry forest and maquis species using the R software (Ver. 3.4.3).
Phylogenetic Signal
We assessed phylogenetic independence of measured traits (Felsenstein, 1985) using two complementary statistics calculated under a Brownian Motion (BM) model of evolution. Pagel's Lambda (Pagel, 1999; Freckleton et al., 2002) is widely used for low rates of type I error and robustness, even for poorly or moderately informative phylogenies (Freckleton et al., 2002; Münkemüller et al., 2012). Because this statistic loses statistical power when used on small phylogenies (<30 terminals, Freckleton et al., 2002), we also used Blomberg's Kappa (Blomberg et al., 2003; Kembel, 2009) that is less robust but more appropriate for small taxonomic sampling (until 20 taxa, Kamilar and Cooper, 2013). Both statistics were calculated considering intraspecific variation using the phytools package for R (Revell, 2012).
Trait Correlations and Functional Characterization
We used phylogenetic regression based on the method proposed by Ives et al. (2007) and implemented in the phytools package for R (Revell, 2012) to test whether architectural variation was correlated with functional specialization. Using maximum likelihood, this method fits bivariate models taking into account both phylogenetic framework and intraspecific variation. The response variable was the integrative branching index, and the explanatory variables were all other functional traits (Table 1). Significance of relations was tested using a likelihood ratio test between the model and a model constrained with a slope of zero.
To characterize the functional space of each architectural classes, we performed a Principal Component Analysis. We used the species arithmetical mean of each functional traits (branching index excluded), and functional differences between architectural classes (Monocaulous, Intermediate, Branched) were tested with a permanova (Vegan package for R, Oksanen et al., 2018).
Ancestral Character Estimation
To determine the putative ancestral architectural class of Atractocarpus and infer the evolution of architecture in the genus, Ancestral Character Estimation (ACE) was performed. We assigned an architectural class to each of the 11 outgroup species based on published descriptions (Fosberg, 1987; Smith and Darwin, 1988; Fosberg et al., 1993; Puttock, 1999; Wong, 2004; Zahid and Wong, 2004, 2010; Tong et al., 2013), herbaria specimens (P, K, BM, E) and available photos. The ACE were performed using a maximum likelihood method under the ape package for R (Paradis et al., 2004). Three possible models of evolution fitted the data characteristics: (i) equal transition rates between classes (ER), (ii) different transition rates between classes but with equal rates for reversions (SYM), and (iii) different rates for every transition (ARD). The best model was selected using the corrected Akaike Information Criterion (AICc).
Trait Based Diversification
To test whether one of the architectural classes has contributed more than the others to the diversification of New Caledonian Atractocarpus (by increasing speciation rates and/or decreasing extinction rates), we used the Multiple State Speciation Extinction (MuSSE) framework (Fitzjohn et al., 2009) as implemented in the diversitree package for R (Fitzjohn, 2012). For this analysis, outgroups were dropped from the phylogeny to consider only the 27 rainforest Atractocarpus species. Fifteen models of diversification were used, each differing in whether or not of speciation, extinction and transition rates were equal between classes (Table 2). Model selection was done according to AICc.
Results
Branching Index
Branching indexes confirmed the existence of a morpho-functional gradient from branched to monocaulous species of Atractocarpus, for both exploration and photosynthetic functions (Figures 2A,B). For both indexes, interspecific variation was too gradual to allow the partitioning in distinct classes, but when combined (i.e., using the branching index), three distinct architectural groups could be differentiated (Wilcoxon tests: P < 0.1, Figure 2C), viz. the branched, intermediate and monocaulous architectural classes. On average, branched species had 82% of photosynthesis and 76% of exploration provided by branches, vs. 36 and 45%, respectively, for intermediate species, and 3 and 16% for monocaulous species.
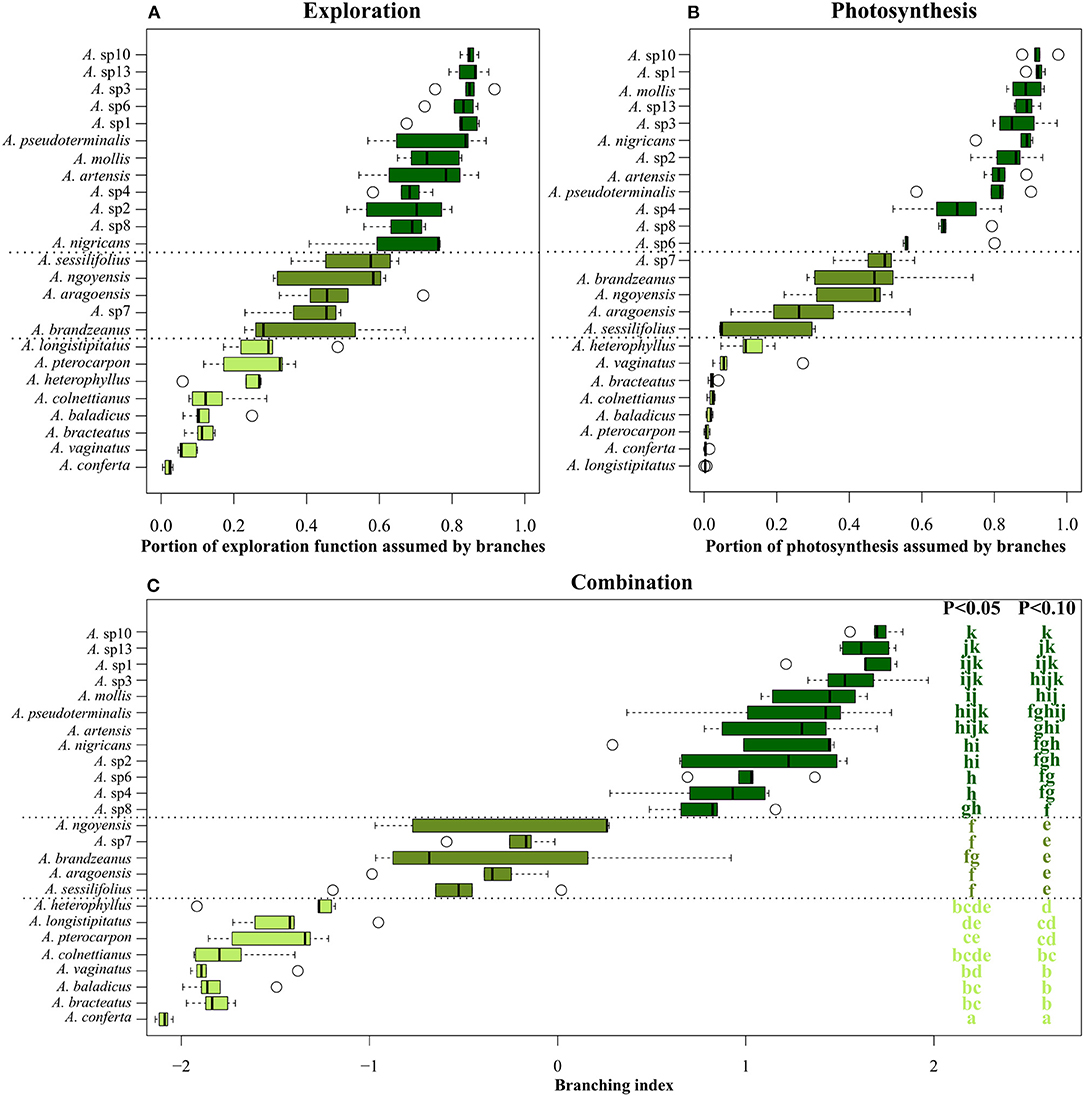
Figure 2. Branching indexes calculated on the base of (A) exploration function, (B) photosynthetic function and (C) the combination of both, for 25 Atractocarpus species. Letters in (C) correspond to the result of the Wilcoxon test; species with shared letters are not significantly different for a given risk of error.
Architectural Characterization
The architecture of New Caledonian Atractocarpus species involves two axis categories: (i) a trunk (C1), i.e., an orthotropic monopodium with continuous indeterminate growth, and (ii) “branches” (C2), i.e., orthotropic sympodia with terminal sexuality conferring rhythmic growth (Table 3, Figure 3). The sympodial branching of C2 always originates in a hypotonic (i.e., on the lower surface) or amphitonic position, resulting in upward flower exposure (Figure 1). The only qualitative architectural variation observed between species concerned the branching position of C1, which was continuous for some monocaulous species while diffuse for others. Two main quantitative architectural traits varied greatly among species, the number of modules per branch and the length of modules, which decreased in monocaulous species (Table 3, Figures 3, 4). For example, A. longistipitatus is a monocaulous species whose C2 comprise many very short modules (apart from the first one). By contrast, A. bracteatus is also monocaulous but its C2 comprise a single long module (Figure 4). The number of internodes per module vary little in the genus but tend to be reduced in monocaulous species, with an extreme case of one node per module in A. confertus (Table 3). All species showed the ability to develop delayed reiterate on damaged or aged individuals.
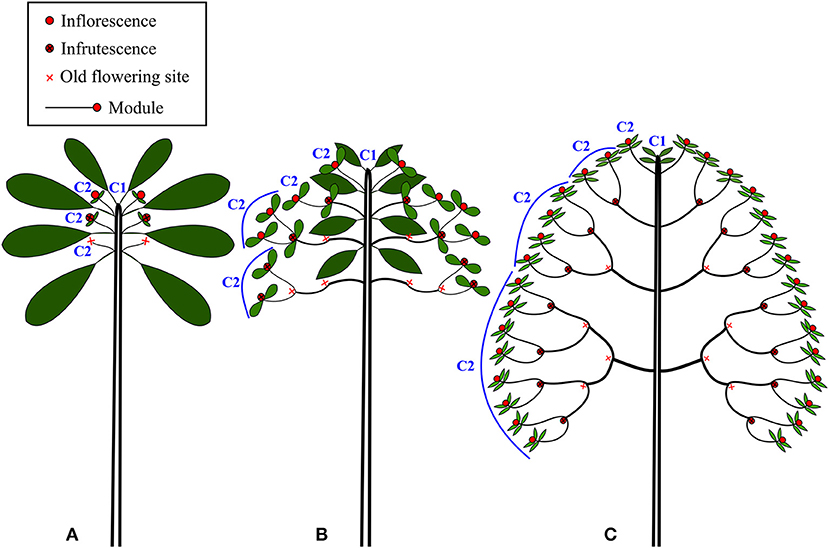
Figure 3. Schematic representation of the three main architectural classes found in the rainforest understory species of Atractocarpus: (A) Monocaulous, (B) Intermediate (C) Branched.
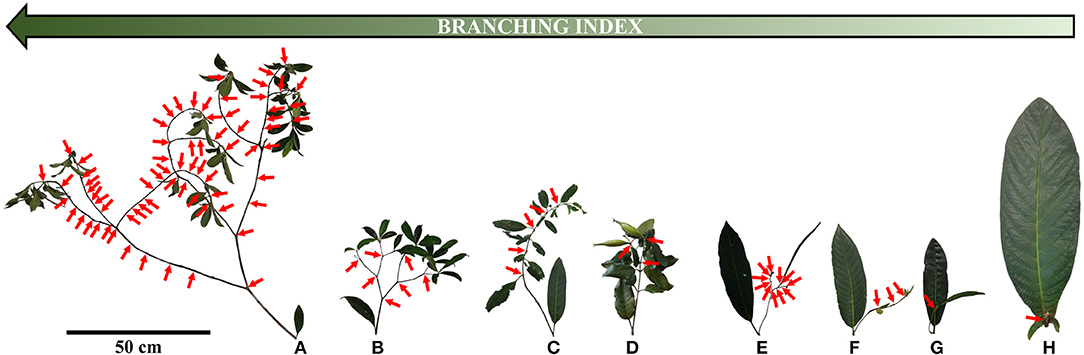
Figure 4. Photographs of branches with their axillary leaves (i.e., from trunk) for height Atractocarpus species with different branching degrees. Arrows indicate apical death, i.e., flowering sites (for A. longistipitatus (E), only half of apical death has been represented). Branched species: (A) A. pseudoterminalis, (B) A. sp4. Intermediate species: (C) A. ngoyensis, (D) A. brandzeanus. Monocaulous species: (E) A. longistipitatus, (F) A. pterocarpon, (G) A. bracteatus, (H) A. confertus.
Functional Characterization
Phylogenetic signal varied substantially depending on which statistic was used (Lambda or Kappa, Table 4). This was probably due to the small size of our phylogeny (25 terminals), since the statistics differ in sensitivity to the number of terminals (Freckleton et al., 2002; Kamilar and Cooper, 2013). Blomberg's Kappa captures significant phylogenetic signal for stem specific density, proportion of pith, Huber value, and mean leaf area, whereas Pagel's Lambda captures significant signal for internode diameter (Table 4). Among all measured functional traits, only the branching index had a highly significant phylogenetic signal calculated with both Pagel's Lambda and Blomberg's Kappa.
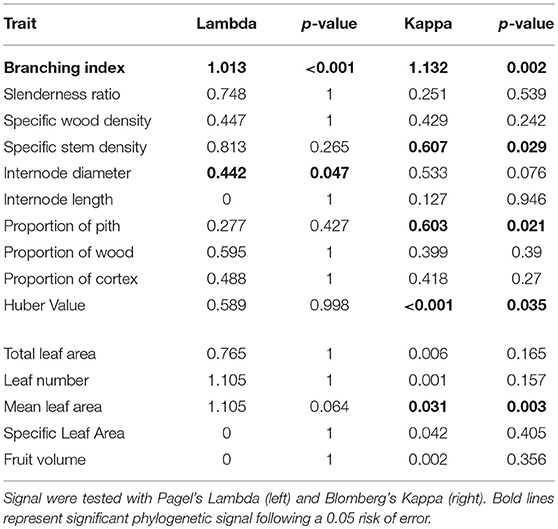
Table 4. Phylogenetic signals of measured traits across the phylogeny of 25 Atractocarpus species (24 for fruit volume).
Several functional traits were significantly related to the branching index (Table 5), including biomechanics (e.g., slenderness ratio, internode diameter) as well as photosynthesis (e.g., leaf area, SLA), hydraulics (e.g., Huber value), and even dispersal (fruit volume). These correlations between architecture and functions were confirmed by PCA and Permanova (P = 0.016), which showed significant differences between the functional space occupied by the three architectural classes (Figure 5). Intermediate species were confounded between the two other architectural classes, but monocaulous and branched species appeared to be functionally very different. In comparison to monocaulous species, branched taxa tend to have numerous small leaves, higher SLA, smaller fruits and, smaller internode diameters with a lower proportion of wood, but a higher wood area-leaf area ratio (Huber value). The monocaulous A. confertus (the lowest point on Figure 5) was distinct from the other Atractocarpus species examined in having numerous very large leaves, leading to a disproportionately higher total leaf area (up to 2.4 m2 vs. a mean of 0.5 m2 for the other species).
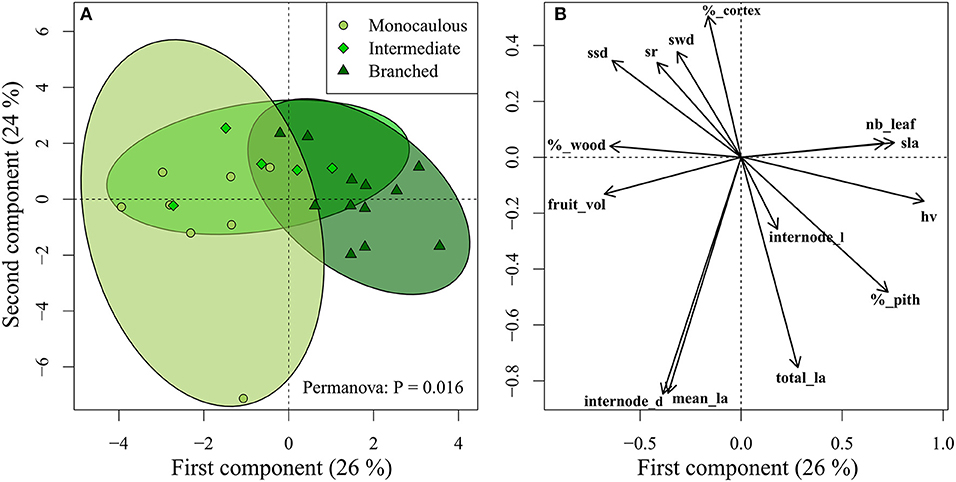
Figure 5. Projection of (A) species and (B) traits on the two first axis of Principal Component Analysis (see Table 1 for trait abbreviations). Ellipses represent the 95% confidence interval for each architectural classes. Functional differences between architectural classes were tested with Permanova.
Ancestral Character Estimation
All considered outgroup species belonged to the branched architectural class, except for the Fijian Sukunia pentagonoides (Seem.) A.C.Sm, which is monocaulous. Among the three possible models of evolution that fitted the data, the best supported one was that of equal transition rates between classes (ER), with an AICc of 84.4 vs. 96.1 and 104.2 for SYM and ARD, respectively. According to this model, the ancestral architecture of Atractocarpus was most likely branched (probability = 0.98, Figure 6), and the monocaulous habit evolved two or three times in the two main New Caledonian clades (referred to as “monocaulous clade A” and “monocaulous clade B” in Figure 6), ca. 1.4 and 0.9–0.6 Myr ago respectively. In each clade, species with an intermediate architecture are closely related to monocaulous species, the only exception being A. brandzeanus, whose closest relatives are branched. Conversely, pairs of sister or closely related species never showed branched and monocaulous habit.
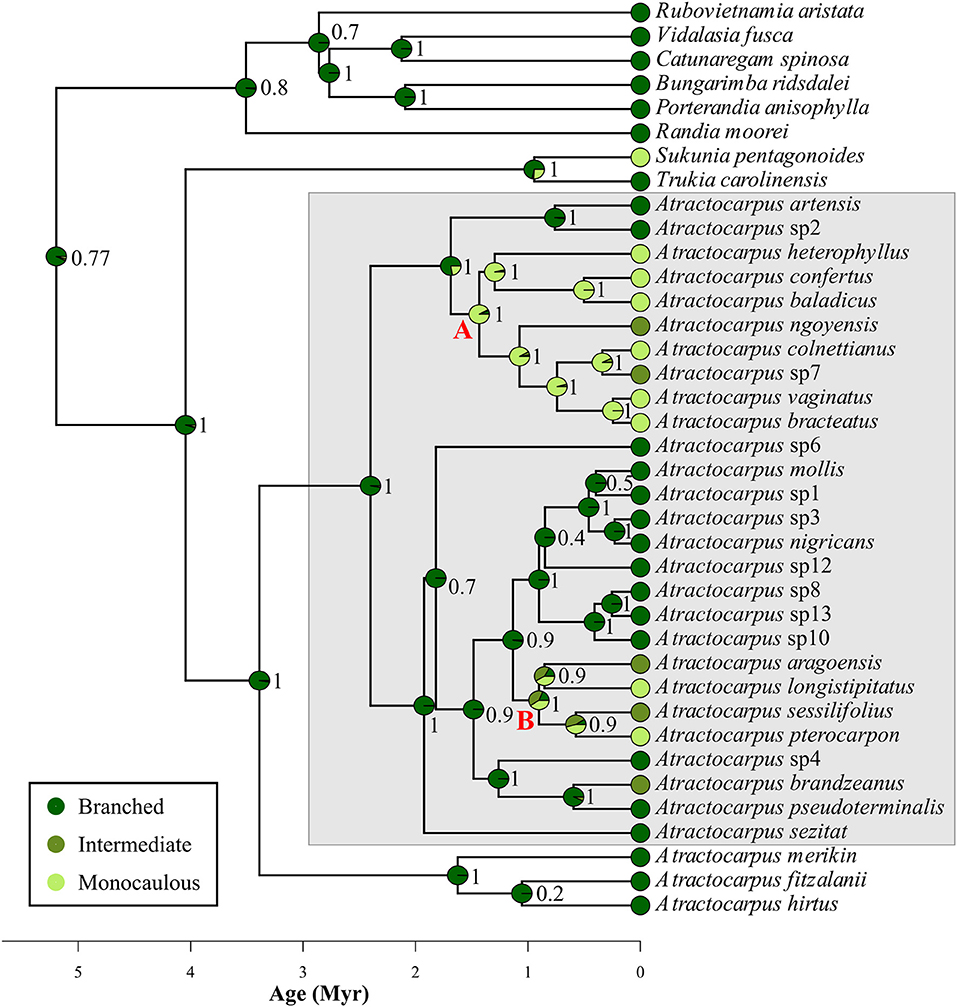
Figure 6. Estimation of ancestral architectural class in the genus Atractocarpus. Numbers correspond to the Bayesian probability for each reconstituted node. Gray box highlights New Caledonian clade. Letters (A, B) highlight major clades were shifts from branched to monocaulous or intermediate architecture operate.
Trait Based Diversification
Following the IACc, models 2 and 10 are the best fitted, in which speciation and transition rates are equal for each state and extinction rates are equal or null (Table 2). This means that diversification was not greater among the members of any of the architectural classes during the evolution of Atractocarpus in New Caledonia.
Discussion
Evolution of Plant Architecture
Despite the diversity of growth habits in the genus Atractocarpus, ranging from well branched to monocaulous species, the architectural construction of members of the group is fairly homogenous. Differences between species mainly result from quantitative variations in which the number of modules per branches and module length tend to increase with increasing branching index.
The measurement of functionally explicit variables (branch length and leaf area) that link plant architecture and function (Lauri, 1988; Lauri and Kelner, 2001; Charles-Dominique et al., 2017) showed that variation of growth habit in Atractocarpus is correlated with a gradual variation from branched species, for which photosynthesis and exploration are largely assumed by branches, toward monocaulous species, for which these functions are assumed by the trunk. The reduced C2 in monocaulous species supports a limited number of small leaves (on average 4 leaves of 9 cm2) and are dedicated almost exclusively to reproduction. These “axes” are functionally closer to inflorescences than branches (Van Steenis, 1963) and are often considered as such by taxonomists (e.g., Fosberg et al., 1993; Puttock, 1999).
The branched architectural class appears to be ancestral in Atractocarpus and our results indicated at least two independent origins of monocauly in New Caledonia. Other examples of derived monocauly have recently been revealed in flowering plants (Chomicki et al., 2017; Barrabé et al., 2018). The intermediate architectural class is evolutionary closer to the monocaulous habit since intermediate and monocaulous species are always sister taxa or very close relatives. There is a high probability that the putative ancestor of “monocaulous clade B,” had an intermediate architecture. This insight, as well as the architectural gradient observed among extant species, suggests a gradual evolutionary transition from the branched architecture toward monocauly through an intermediate architectural class. Reversion from a monocaulous architecture toward an intermediate one appears to have been possible (e.g., Atractocarpus sp. nov. 7) but full reversion to a branched architecture was not observed.
The similarity of developmental origin and the gradual quantitative architectural variation of lateral axes (C2) across closely related taxa suggest a homologous origin of these axes in the Atractocarpus species studied here (see Nozeran, 1955; Rossignol and Rossignol, 1985; Haicour et al., 1989). A homologous origin between vegetative and inflorescence axes has been suspected in several groups such as Ranunculaceae and Papaveraceae (Stebbins, 1973), as well as several families of Alismatales (Posluszny and Charlton, 1993, 1999) and Pandanales (Rudall and Bateman, 2006), though none of these studies combined architectural analysis with molecular phylogenetic results.
Only a few studies to date have explored evolutionary links between architectural models (see Chomicki et al., 2017). In New Caledonia Atractocarpus, we found recurrent transitions from Stone's model for branched species (with C2 functioning as branches) to Corner's model for monocaulous species (with C2 functioning as inflorescences), although the limit between them remains fuzzy, as illustrated by species whose architecture is intermediate. The variation observed in branching degree across species illustrates an “architectural continuum” (Oldeman, 1974; Barthélémy et al., 1989) rather than saltational evolution involving rapid and profound change in architecture (Bateman and DiMichele, 1994; Bateman, 1999). Nothing suggests that the intermediate architectural class confers lower fitness (i.e., represents a fitness valley) compared to monocaulous or branched architecture, particularly in situations where the environment (rainforest understory) varies gradually. Saltation from one model to another might take place when gradual changes are impossible, for example in the colonization of contrasted habitats (Bateman and DiMichele, 1994). Gradual variation between and within models has already been noted (Hallé et al., 1978), suggesting that preferential transition between models might occur.
The two independent origins of monocauly inferred in Atractocarpus illustrate two different evolutionary pathways involved in the transition from branches into inflorescences. In “monocaulous clade A,” reduction in the branching index occurred through a reduction in the number of modules, while in “monocaulous clade B,” module length is involved. Architectural analysis actually showed that the two species with intermediate architecture and the two monocaulous species belonging to the clade B are those with the higher number of modules (up to 46 for the intermediate A. sessilifolius and 26 the for monocaulous A. longistipitatus, i.e., as much as certain branched species) and with shorter module length (excluding the monocaulous A. confertus). This type of morphological reduction observed at the module level in Atractocarpus could affect any elementary level of organization in other plant groups (e.g., annual shoots, growth units, metamers, etc.).
The iterative gradual transition of axillary structure (C2) from branches to inflorescences occurs as a functional reduction of vegetative growth and a change in the timing of flowering. Such evolutionary changes, often result from growth heterochrony (Smith, 2001). In the case of New Caledonian Atractocarpus, the axillary structure (C2) of descendants is either a truncated part (in “monocaulous clade A”) or a miniaturized copy (in “monocaulous clade B”) of that of their ancestors. This suggests heterochronic evolution through “neoteny” in the first case and through “proportional dwarfism” in the second (Gould, 1977; Alberch et al., 1979; Smith, 2001), but more details of the ontogenetic sequences are required to test these hypotheses in Atractocarpus.
In this context, our original approach showed that a careful study of low organization levels is needed to depict the evolution of plant architecture. This involves a deep characterization of architectural units (Edelin, 1977, 1984), combining qualitative and quantitative traits.
Branching Index, Corner's Rules, and Ecological Strategies
In the original Durian theory, Corner (1949, 1953-1954) proposed two fundamental rules governing plant morphology: (i) Axial conformity, stipulating that “the stouter, or more massive, the axis in a given species, the larger and more complicated its appendages”; and (ii) Diminution on ramification, stipulating that “the greater the ramification, the smaller become the branches and their appendages”. Axial conformity is by far the most studied since the leaf size—twig size and fruit size—leaf size spectra are among the best-documented (White, 1983b; Bond and Midgley, 1988; Brouat et al., 1998; Cornelissen, 1999; Brouat and McKey, 2001; Westoby et al., 2002; Preston and Ackerly, 2003; Westoby and Wright, 2003; Pickup et al., 2005; Sun et al., 2006; Normand et al., 2008). Large leaves and fruit carried by a large single stem, as seen in monocaulous species, illustrate one extremity of this spectrum. The second rule (diminution of ramification), has received much less consideration (but see White, 1983a; Ackerly and Donoghue, 1998). Our results agree with this statement, as we have shown that internode diameter, as well as fruit and leaf size, are negatively correlated with branching index, even with phylogenetic corrections. Beyond Corner's rules, we found a strong correlation between branching index and several functional traits related to various ecological strategies in areas with a similar habitat (i.e., rainforest).
We found that branching index tends to be correlated with ecologically important dimensions (Mooney, 1972; Grime, 1974; Grime et al., 1988; Westoby et al., 2002). The large leaves of monocaulous species increase light interception in shady understory (Poorter, 1999; Rozendaal et al., 2006), their low SLA and Huber value are likely associated with low photosynthetic capacity (Field and Mooney, 1986; Shipley et al., 2005; Poorter and Bongers, 2006; Rozendaal et al., 2006; Pérez-Harguindeguy et al., 2013) and their small internode length can result from a slow growth rate (Mooney, 1972; Chuah, 1977; Grime et al., 1988; Westoby et al., 2002). Monocaulous species are likely skewed toward a high resource conservation strategy (Grime et al., 1997; Díaz et al., 2004, 2016) suited to the shady understory of rainforest.
In sparsely branched to unbranched species, a distal part of the stem with thicker diameter and higher wood proportion was found to be suited to supporting large and numerous energetically costly leaves (higher leaf area and SLA). However, higher wood area does not fully compensate for high total leaf area of the main stem since the Huber value (the ratio of wood area to leaf area) was positively correlated with branching index. Detailed anatomical studies, particularly on vessel size and density, are needed to understand the hydraulic and mechanical trade-off involved in the pervasive link between leaf area, stem thickness and branching degree (Lehnebach et al., 2018).
Divergence and Ecological Opportunities in New Caledonian Rainforests
New Caledonian Atractocarpus appear to be the result of a single colonization event (Mouly et al., under review) and the archipelago is the center of diversity for the genus. Island colonization is a two steps process involving dispersal and successful establishment (Silvertown, 2004). The large, fleshy fruits of Atractocarpus suggest a rather limited ability for long-distance dispersal. Such niche preemption, claimed to act as a major driver of monophyly and diversification in island floras (Silvertown, 2004), could have prevailed in the diversification of the New Caledonian clade of Atractocarpus. Its young age (estimated at 2.4 My) coincides with colonization during late Pleistocene, a period of intense climatic Fluctuation (Bowler et al., 1976; Hope and Pask, 1998; Stevenson and Hope, 2005). Increasing evidence supports the persistence of taxa in rainforest refugia during the Pleistocene in New Caledonia (Pintaud et al., 2001; Pillon, 2012; Nattier et al., 2013; Poncet et al., 2013; Pouteau et al., 2015; Tournebize et al., 2017). Climatic fluctuations causing expansion and contraction of rainforests could also have offered ecological opportunities for new colonizers. Other diversified rainforest clades in New Caledonia have been shown to result from recent colonization (e.g., palms, Pintaud et al., 2001; Pillon, 2012). Similarities in form and physiology among close relatives, as suggested by our character state reconstruction and architectural analysis, are suggested to involve intense competition that favors divergence among locally coexisting species (Givnish, 2016). In support of this, we found notable variation in module length and number between sisters species, even when they belong to the same architectural class. In Atractocarpus, variation in growth habit is correlated with a gradual switch in assimilation function from branches to trunk, and is associated with a vertical differentiation of major functions. Leaf arrangement in monocaulous species results in important overlap that impacts photosynthesis, while stem slenderness suggests a rather small structural investment in vertical support. Branched species tend to increase light harvesting and reduce leaf overlap (via increase branch length), a strategy that require more investment in stem tissue (Givnish, 1995), as allocation to non-leaf structures might increase with ramification. Atractocarpus species thus exhibit a gradient of foraging for light in which leaf size and disposition vary with the degree of ramification.
New Caledonian rainforests are characterized by a low canopy (ca. 20 m) and trees with small crowns (Blanchard et al., 2016), two structural features that increase the number and intensity of sunflecks (Chazdon and Pearcy, 1991). A low canopy and its corollary, reduced forest stratification, could result in stronger competition within a given stratum. These forest features (high sunfleck variability, reduced stratification) favor niche partitioning through a gradient in architecture and related functional traits. Moreover, limited dispersal of the large fruits of Atractocarpus might interact with niche partitioning to promote divergent evolution. Finally, divergent selection may be especially favored in permissive environments where competition prevails over the external environment or in the colonization of islands where resources are underutilized (Givnish, 2016). New Caledonia exhibits both components of divergent selection: (i) climatically permissive rainforests and (ii) ecological opportunities offered by recent climatic fluctuations. Divergent selection, caused by competition among closely related taxa, leads to adaptive radiation, i.e., the rise of a diversity of ecological roles and related adaptations in different species among a lineage (Givnish, 2016). The theory of niche pre-emption holds that adaptive radiation creates a barrier that inhibits the establishment of closely related taxa, thus reducing the likelihood of repeated colonization.
Conclusion
Despite the fundamental importance of plant architecture, little work has been done to integrate this aspect into key domains of plant science such as evolution and functional ecology. However, increasing availability of information on ecologically important traits and molecular phylogenies provides a basis for testing and developing new concepts. Our study clearly highlights evolutionary processes behind architectural transitions and their link to plant ecological strategy and perhaps also to diversification. We have shown that gradual transition from one architecture to a very different one is possible through morpho-functional reduction of morphological units. Quantifying plant architecture through functional indexes appears to offer a promising avenue toward further understand the implications of architectural variation on plant fitness under different environmental conditions. Based on such an index, our study provides a functional definition of monocauly that is ecologically and evolutionary more explicit than one based solely on physiognomy (cf. Hallé et al., 1978; Chomicki et al., 2017): we define monocaulous species as woody plants whose cardinal functions (e.g., assimilation and exploration) rely on a single apparent stem.
Although our work focused on a small clade, it opens new perspectives and proposes a general framework for further understanding evolution of plant architecture and its functional implications in other plant groups and other geographical areas, and at larger scales. We believe that Pleistocene climatic fluctuations have played a major role in the evolution of monocauly and more widely in shaping the current diversity of the New Caledonian flora. This hypothesis now needs to be tested at a larger phylogenetic scale in New Caledonia, as the monocaulous habit, which occurs repetitively in many different groups (Veillon, 1976; Schmid, 1979, 1990), has much to offer to understand the evolution, biogeography and ecology of this “very old Darwinian island” (Grandcolas et al., 2008).
Data Availability Statement
All datasets generated for this study are included in the manuscript and the supplementary files. Data concerning the phylogenetic tree was obtained from Mouly et al. (under review) and genetic sequences are available in the European Nucleotide Archive (accession numbers on Appendix 2 in Supplementary Material). Information about study sites (habitat characteristics…) is available through the voucher specimens of which duplicates have been deposited in various herbaria.
Author Contributions
SI and DBr contributed to conception and design of the study. DBr, TH and LB collected field samples. TH and DBr measured functional traits. TH, DBr, and DBa performed architectural study. LB and AM produced phylogenetical tree. DBr, TH, and LB performed the statistical analysis. DBr and SI wrote the paper. All authors contributed to manuscript revision, read and approved the submitted version.
Conflict of Interest Statement
The authors declare that the research was conducted in the absence of any commercial or financial relationships that could be construed as a potential conflict of interest.
Acknowledgments
We are grateful to all those who helped with field data collection, particularly D. Fleurot and the staff of the Parc Naturel des Grandes Fougères. We also thank S. Lavergne for advice about data analysis and Y. Caraglio for sharing thoughts about our work. Thanks to P. Lowry for the thorough revision of the manuscript. Thanks also to H. Caze, C. Létocart, G. Gateblé, and H. Vandrot for allowing us to use their excellent photographs, and to the NOU and P herbaria for permitting fruit measurements on specimens.
Supplementary Material
The Supplementary Material for this article can be found online at: https://www.frontiersin.org/articles/10.3389/fpls.2018.01775/full#supplementary-material
References
Ackerly, D. D. (1996). “Canopy structure and dynamics: integration of growth processes in tropical pioneer trees,” in Tropical Forest Plant Ecophysiology, eds S. S. Mulkey, R. L. Chazdon and A. P. Smith (New York, NY: Chapman & Hall), 619–658.
Ackerly, D. D. (2004). Adaptation, niche conservatism, and convergence: comparative studies of leaf evolution in the california chaparral. Am. Nat. 163, 654–671. doi: 10.1086/383062
Ackerly, D. D., and Donoghue, M. J. (1998). Leaf size, sapling allometry, and corner's rules: phylogeny and correlated evolution in maples (Acer). Am. Nat. 152, 767–791.
Alberch, P., Gould, S. J., Oster, G. F., and Wake, D. B. (1979). Size and shape in ontogeny and phylogeny. Paleobiology 5, 296–317. doi: 10.1017/S0094837300006588
Almeras, T., Costes, E., and Salles, J.-C. (2004). Identification of biomechanical factors involved in stem shape variability between apricot tree varieties. Ann. Bot. 93, 455–468. doi: 10.1093/aob/mch054
Arakaki, M., Christin, P. A., Nyffeler, R., Lendel, A., Eggli, U., Ogburn, R. M., et al. (2011). Contemporaneous and recent radiations of the world's major succulent plant lineages. Proc. Natl. Acad. Sci. U.S.A. 108, 8379–8384. doi: 10.1073/pnas.1100628108
Aubert, S., Boucher, F., Lavergne, S., Renaud, J., and Choler, P. (2014). 1914–2014: A revised worldwide catalogue of cushion plants 100 years after Hauri and Schröter. Alpine Bot. 124, 59–70. doi: 10.1007/s00035-014-0127-x
Barrabé, L., Lavergne, S., Karnadi-Abdelkader, G., Drew, B. T., Birnbaum, P., and Gâteblé, G. (2018). Changing ecological opportunities facilitated the explosive diversification of New Caledonian Oxera (Lamiaceae). Syst. Biol. doi: 10.1093/sysbio/syy070. [Epub ahead of print].
Barthélémy, D., and Caraglio, Y. (2007). Plant architecture: a dynamic, multilevel and comprehensive approach to plant form, structure and ontogeny. Ann. Bot. 99, 375–407. doi: 10.1093/aob/mcl260
Barthélémy, D., Edelin, C., and Hall,é, F. (1989). “Architectural concepts for tropical trees,” in Tropical Forests: Botanical Dynamics, Speciation and Diversity, eds L. B. Holm-Nielsen and H. Balslev (London: Academic Press), 89–100.
Bateman, R. M. (1994). Evolutionary-developmental change in the growth architecture of fossil rhizomorphic lycopsids: scenarios constructed on cladistic foundations. Biol. Rev. 69, 527–597. doi: 10.1111/j.1469-185X.1994.tb01249.x
Bateman, R. M. (1999). “Architectural radiations cannot be optimally interpreted without morphological and molecular phylogenies,” in The Evolution of Plant Architecture, eds M. H. Kurmann and A. R. Hemsley (Kew: Royal Botanic Gardens), 221–250.
Bateman, R. M., and DiMichele, W. A. (1994). “Saltational evolution of form in vascular plants: a neoGoldschmidtian synthesis,” in Shape and Form in Plants and Fungi, eds D. S. Ingram and A. Hudson (London: Academic Press), 63–102.
Blanchard, E., Birnbaum, P., Ibanez, T., Boutreux, T., Antin, C., Ploton, P., et al. (2016). Contrasted allometries between stem diameter, crown area, and tree height in five tropical biogeographic areas. Trees 30, 1953–1968. doi: 10.1007/s00468-016-1424-3
Blomberg, S. P., Garland, T., and Ives, A. R. (2003). Testing for phylogenetic signal in comparative data: behavioral traits are more labile. Evolution 57, 717–745. doi: 10.1111/j.0014-3820.2003.tb00285.x
Bond, W. J., and Midgley, J. J. (1988). Allometry and sexual differences in leaf size. Am. Nat. 131, 901–910. doi: 10.1086/284830
Boucher, F. C., Lavergne, S., Basile, M., Choler, P., and Aubert, S. (2016). Evolution and biogeography of the cushion life form in angiosperms. Perspect. Plant Ecol. Evol. Syst. 20, 22–31. doi: 10.1016/j.ppees.2016.03.002
Boucher, F. C., Thuiller, W., Roquet, C., Douzet, R., Aubert, S., Alvarez, N., et al. (2012). Reconstructing the origins of high-alpine niches and cushion life form in the genus Androsace S.L. (Primulaceae). Evolution 66, 1255–1268. doi: 10.1111/j.1558-5646.2011.01483.x
Bowler, J. M., Hope, G. S., Jennings, J. N., Singh, G., and Walker, D. (1976). Late quaternary climates of Australia and New Guinea. Quat. Res. 6, 359–394. doi: 10.1016/0033-5894(67)90003-8
Bramwell, D. (1975). Some morphological aspects of the adaptive radiation of Canary Islands Echium species. Anal. Inst. Bot. Cavanilles 32, 241–254.
Brouat, C., Gibernau, M., Amsellem, L., and McKey, D. (1998). Corner's rules revisited: ontogenetic and interspecific patterns in leaf-stem allometry. New Phytol. 139, 459–470. doi: 10.1046/j.1469-8137.1998.00209.x
Brouat, C., and McKey, D. (2001). Leaf-stem allometry, hollow stems, and the evolution of caulinary domatia in myrmecophytes. New Phytol. 151, 391–406. doi: 10.1046/j.0028-646x.2001.00197.x
Carlquist, S. (1974). Island Biology. New York, NY: Columbia University Press. doi: 10.5962/bhl.title.63768
Carlquist, S. (1984). Wood and stem anatomy of Lardizabalaceae with comments on the vining habit, ecology and systematics. Bot. J. Linn. Soc. 88, 257–277. doi: 10.1111/j.1095-8339.1984.tb01575.x
Charles-Dominique, T., Barczi, J.-F., Le Roux, E., and Chamaillé-Jammes, S. (2017). The architectural design of trees protects them against large herbivores. Funct. Ecol. 31, 1710–1717. doi: 10.1111/1365-2435.12876
Charles-Dominique, T., Edelin, C., and Bouchard, A. (2010). Architectural strategies of Cornus sericea, a native but invasive shrub of Southern Quebec, Canada, under an open or a closed canopy. Ann. Bot. 105, 205–220. doi: 10.1093/aob/mcp273
Charles-Dominique, T., Edelin, C., Brisson, J., and bouchard, A. (2012). Architectural strategies of Rhamnus cathartica, in relation to canopy openness. Botany 90, 976–989. doi: 10.1139/b2012-069
Chazdon, R. L., and Pearcy, R. W. (1991). The importance of sunflecks for forest understory plants: photosynthetic machinery appears adapted to brief, unpredictable periods of radiation. Bioscience 41, 760–766. doi: 10.2307/1311725
Chomicki, G., Coiro, M., and Renner, S. S. (2017). Evolution and ecology of plant architecture: integrating insights from the fossil record, extant morphology, developmental genetics and phylogenies. Ann. Bot. 120, 855–891. doi: 10.1093/aob/mcx113
Chuah, M. S. (1977). Exemples de Corrélations Quantitatives entre Quelques Paramètres Déterminant la forme végétale. Ph.D. thesis, Université de Montpellier, Montpellier.
Cornelissen, J. H. (1999). A triangular relationship between leaf size and seed size among woody species: allometry, ontogeny, ecology and taxonomy. Oecologia 118, 248–255. doi: 10.1007/s004420050725
Cornelissen, J. H., Lavorel, S., Garnier, E., Díaz, S., Buchmann, N., Gurvich, D. E., et al. (2003). A handbook of protocols for standardised and easy measurement of plant functional traits worldwide. Aust. J. Bot. 51, 335–380. doi: 10.1071/BT02124
Corner, E. J. H. (1949). The Durian theory or the origin of the modern tree. Ann. Bot. 13, 367–414. doi: 10.1093/oxfordjournals.aob.a083225
Couvreur, T. L., Kissling, W. D., Condamine, F. L., Svenning, J. C., Rowe, N. P., and Baker, W. J. (2015). Global diversification of a tropical plant growth form: environmental correlates and historical contingencies in climbing palms. Front. Genet. 5:452. doi: 10.3389/fgene.2014.00452
Díaz, S., Hodgson, J. G., Thompson, K., Cabido, M., Cornelissen, J. H. C., Jalili, A., et al. (2004). The plant traits that drive ecosystems: evidence from three continents. J. Veg. Sci. 15, 295–304. doi: 10.1111/j.1654-1103.2004.tb02266.x
Díaz, S., Kattge, J., Cornelissen, J. H., Wright, I. J., Lavorel, S., Dray, S., et al. (2016). The global spectrum of plant form and function. Nature 529, 167–171. doi: 10.1038/nature16489
Dong, B.-C., Yu, G.-L., Guo, W., Zhang, M.-X., Dong, M., and Yu, F.-H. (2010). How internode length, position and presence of leaves affect survival and growth of Alternanthera philoxeroides after fragmentation? Evol. Ecol. 24, 1447–1461. doi: 10.1007/s10682-010-9390-5
Dray, S., and Dufour, A.-B. (2007). The ade4 package: implementing the duality diagram for ecologists. J. Stat. Softw. 22, 1–20. doi: 10.18637/jss.v022.i04
Drummond, C. S., Eastwood, R. J., Miotto, S. T., and Hughes, C. E. (2012). Multiple continental radiations and correlates of diversification in lupinus (leguminosae): testing for key innovation with incomplete taxon sampling. Syst. Biol. 61, 443–460. doi: 10.1093/sysbio/syr126
Duncan, W. G., and Hesketh, J. D. (1968). Net photosynthetic rates, relative leaf growth rates, and leaf numbers of 22 races of maize grown at eight temperatures. Crop Sci. 8, 670–674. doi: 10.2135/cropsci1968.0011183X000800060009x
Edelin, C. (1977). Image sur l'architecture des Conifères. Ph.D. thesis. Université de Montpellier, Montpellier.
Edelin, C. (1984). L'architecture Monopodiale: l'exemple de quelques Arbres d'Asie tropicale. Ph.D. thesis, Université de Montpellier, Montpellier.
Felsenstein, J. (1985). Phylogenies and the comparative method. Am. Nat. 125, 1–15. doi: 10.1086/284325
Field, C. B., and Mooney, H. A. (1986). “The photosynthesis–nitrogen relationship in wild plants,” in On the Economy of Plant Form and Function, ed T. J. Givnish (Cambridge: Cambridge University Press), 25–55.
Fitzjohn, R. G. (2012). Diversitree: comparative phylogenetic analyses of diversification in R. Methods Ecol. Evol. 3, 1084–1092. doi: 10.1111/j.2041-210X.2012.00234.x
Fitzjohn, R. G., Maddison, W. P., and Otto, S. P. (2009). Estimating trait-dependent speciation and extinction rates from incompletely resolved phylogenies. Syst. Biol. 58, 595–611. doi: 10.1093/sysbio/syp067
Fosberg, F. R., Sachet, M.-H., and Olivier, R. L. (1993). “Bignoniaceae-Rubiaceae,” in Flora of Micronesia. (Washington, DC: Smithsonian Contributions to Botany), 1–135. doi: 10.5479/si.0081024X.81
Freckleton, R. P., Harvey, P. H., and Pagel, M. (2002). Phylogenetic analysis and comparative data: a test and review of evidence. Am. Nat. 160, 712–726. doi: 10.1086/343873
Gianoli, E. (2004). Evolution of a climbing habit promotes diversification in flowering plants. Proc. R. Soc. Biol. Sci. 271, 2011–2015. doi: 10.1098/rspb.2004.2827
Givnish, T. J. (1995). “Plant stems: biomechanical adaptation for energy capture and influence on species distributions,” in Plant Stems, ed B. L. Gartner. (San Diego, CA: Academic Press), 3–49.
Givnish, T. J. (2010). Giant lobelias exemplify convergent evolution. BMC Biol. 8:3. doi: 10.1186/1741-7007-8-3
Givnish, T. J. (2016). “convergent evolution, adaptive radiation, and species diversification in plants,” in Encyclopedia of Evolutionary Biology, ed R. M. Kliman (Oxford: Academic Press), 362–373.
Givnish, T. J., Barfuss, M. H., Van Ee, B., Riina, R., Schulte, K., Horres, R., et al. (2014). Adaptive radiation, correlated and contingent evolution, and net species diversification in Bromeliaceae. Mol. Phylogenet. Evol. 71, 55–78. doi: 10.1016/j.ympev.2013.10.010
Givnish, T. J., Millam, K. C., Mast, A. R., Paterson, T. B., Theim, T. J., Hipp, A. L., et al. (2009). Origin, adaptive radiation and diversification of the Hawaiian lobeliads (Asterales: Campanulaceae). Proc. R. Soc. Biol. Sci. 276, 407–416. doi: 10.1098/rspb.2008.1204
Givnish, T. J., and Sytsma, K. J. (1997). Molecular Evolution and Adaptive Radiation. Cambridge: Cambridge University Press.
Gleason, S. M., Butler, D. W., Zieminska, K., Waryszak, P., and Westoby, M. (2012). Stem xylem conductivity is key to plant water balance across Australian angiosperm species. Funct. Ecol. 26, 343–352. doi: 10.1111/j.1365-2435.2012.01962.x
Grandcolas, P., Murienne, J., Robillard, T., Desutter-Grandcolas, L., Jourdan, H., Guilbert, E., et al. (2008). New Caledonia: a very old Darwinian island? Philos. Trans. R. Soc. Lond. B Biol. Sci. 363, 3309–3317. doi: 10.1098/rstb.2008.0122
Grime, J. P. (1974). Vegetation classification by reference to strategies. Nature 250, 26–31. doi: 10.1038/250026a0
Grime, J. P., Hodgson, J. G., and Hunt, R. (1988). Comparative Plant Ecology: a Functional Approach to Common British Species. London: Springer.
Grime, J. P., Thompson, K., Hunt, R., Hodgson, J. G., Cornelissen, J. H. C., Rorison, I. H., et al. (1997). Integrated screening validates primary axes of specialisation in plants. Oikos 79, 259–281. doi: 10.2307/3546011
Haicour, R., Nozeran, R., Rossignol, L., and Rossignol, M. (1989). Les Phyllanthus: un excellent matériel pour l'étude du polymorphisme. Bulletin de la Société Botanique de France. Actualités Botaniques 136, 139–150. doi: 10.1080/01811789.1989.10826968
Hallé, F., Oldeman, R. A. A., and Tomlinson, P. B. (1978). Tropical Trees and Forests an Architectural Analysis. New York, NY: Springer-Verlag.
Homeier, J., Breckle, S.-W., Günter, S., Rollenbeck, R. T., and Leuschner, C. (2010). Tree diversity, forest structure and productivity along altitudinal and topographical gradients in a species-rich Ecuadorian montane rain forest. Biotropica 42, 140–148. doi: 10.1111/j.1744-7429.2009.00547.x
Hope, G., and Pask, J. (1998). Tropical vegetational change in the late Pleistocene of New Caledonia. Palaeogeogr. Palaeoclimatol. Palaeoecol. 142, 1–21. doi: 10.1016/S0031-0182(97)00140-5
Hummel, I., Vile, D., Violle, C., Devaux, J., Ricci, B., Blanchard, A., et al. (2007). Relating root structure and anatomy to whole-plant functioning in 14 herbaceous Mediterranean species. New Phytol. 173, 313–321. doi: 10.1111/j.1469-8137.2006.01912.x
Isnard, S., Prosperi, J., Wanke, S., Wagner, S. T., Samain, M. S., Trueba, S., et al. (2012). Growth form evolution in Piperales and its relevance for understanding the angiosperm diversification – An integrative approach combining plant architecture, anatomy and biomechanics. Int. J. Plant Sci. 173, 610–639. doi: 10.1086/665821
Ives, A. R., Midford, P. E., and Garland, T. (2007). Within-species measurement error in phylogenetic comparative methods. Syst. Biol. 56, 252–270. doi: 10.1080/10635150701313830
Kamilar, J. M., and Cooper, N. (2013). Phylogenetic signal in primate behaviour, ecology and life history. Phil. Trans. R. Soc. Biol. Sci. 368, 1–10. doi: 10.1098/rstb.2012.0341
Kembel, S. W. (2009). Disentangling niche and neutral influences on community assembly: assessing the performance of community phylogenetic structure tests. Ecol. Lett. 12, 949–960. doi: 10.1111/j.1461-0248.2009.01354.x
Küppers, M. (1989). Ecological significance of above-ground architectural patterns in woody plants: a question of cost-benefit relationships. Trends Ecol. Evol. 4, 375–379. doi: 10.1016/0169-5347(89)90103-1
Kurmann, M. H., and Hemsley, A. R. (eds.). (1999). The Evolution of Plant Architecture. London: Kew Royal Botanic Garden.
Lauri, P.-E. (1988). Le Mouvement Morphogenetique, Approche Morphometrique et Restitution Graphique: L'exemple de Quelques Plantes Tropicales. Ph.D. thesis, Université de Montpellier, Montpellier.
Lauri, P.-E., and Kelner, J.-J. (2001). Shoot type demography and dry matter partitioning: a morphometric approach in apple (Malus × domestica). Can. J. Bot. 79, 1270–1273. doi: 10.1139/b01-113
Lehnebach, R., Beyer, R., Letort, V., and Heuret, P. (2018). The pipe model theory half a century on: a review. Ann. Bot. 121, 773–795. doi: 10.1093/aob/mcx194
Lens, F., Davin, N., Smets, E., and del Arco, M. (2013). Insular woodiness on the Canary Islands: a remarkable case of convergent evolution. Int. J. Plant Sci. 174, 992–1013. doi: 10.1086/670259
León Enriquez, B. L., Vester, H., Franciscus, M., and Hallé, F. (2008). The architecture of Phyllanthus acuminatus Vahl: a prelude to understanding the architectural evolution in the Phyllanthaceae. Adansonia 30, 137–149.
Levionnois, S., Coste, S., Nicolini, E., Stahl, C., Morel, H., and Heuret, P. (2018). Sent to the Corner: xylem vessel anatomy not surface area determines megaphyll hydraulics in Cecropia obtusa Trécul (Urticaceae). bioRxiv. doi: 10.1101/259036
Meyer-Berthaud, B., Soria, A., and Decombeix, A.-L. (2010). The land plant cover in the Devonian: a reassessment of the evolution of the tree habit. Geol. Soc. Lond. Spec. Public. 339, 59–70. doi: 10.1144/SP339.6
Millan, M. (2016). Analyse de la variabilité des traits architecturaux des formes de croissance dans les communautés végétales. Ph.D. thesis, Université de Montpellier, Montpellier.
Millet, J., Bouchard, A., and Edelin, C. (1999). Relationship between architecture and successional status of trees in the temperate deciduous forest. Ecoscience 6, 187–203. doi: 10.1080/11956860.1999.11682520
Mooney, H. A. (1972). The carbon balance of plants. Annu. Rev. Ecol. Syst. 3, 315–346. doi: 10.1146/annurev.es.03.110172.001531
Mouly, A., Kainulainen, K., Persson, C., Davis, A., Meng Wong, K., Razafimandimbison, S., et al. (2014). Phylogenetic structure and clade circumscriptions in the Gardenieae complex (Rubiaceae). Taxon 63, 801–818. doi: 10.12705/634.4
Münkemüller, T., Lavergne, S., Bzeznik, B., Dray, S., Jombart, T., Schiffers, K., et al. (2012). How to measure and test phylogenetic signal. Methods Ecol. Evol. 3, 743–756. doi: 10.1111/j.2041-210X.2012.00196.x
Nattier, R., Grandcolas, P., Pellens, R., Jourdan, H., Couloux, A., Poulain, S., et al. (2013). Climate and soil type together explain the distribution of microendemic species in a biodiversity hotspot. PLoS ONE 8:e80811. doi: 10.1371/journal.pone.0080811
Niklas, K. J. (1992). Plant Biomechanics: An Engineering Approach to Plant Form and Function. Chicago, IL: University of Chicago Press.
Niklas, K. J., Cobb, E. D., and Marler, T. (2006). A comparison between the record height-to-stem diameter allometries of pachycaulis and leptocaulis species. Ann. Bot. 97, 79–83. doi: 10.1093/aob/mcj002
Normand, F., Bissery, C., Damour, G., and Lauri, P. E. (2008). Hydraulic and mechanical stem properties affect leaf-stem allometry in mango cultivars. New Phytol. 178, 590–602. doi: 10.1111/j.1469-8137.2008.02380.x
Nozeran, R. (1955). À propos des phénomènes de transfert des fonctions reproductrices. Rec. Trav. Inst. Bot. Montpellier 7, 75–82.
Ogburn, R. M., and Edwards, E. J. (2010). “The ecological water-use strategies of succulent plants,” in Advances in Botanical Research, eds J.-C. Kader and M. Delseny (Burlington, NJ: Academic Press), 179–225.
Oksanen, J., Blanchet, F. G., Friendly, M., Kindt, R., Legendre, P., McGlinn, D., et al. (2018). vegan: Community Ecology Package. R package version 2.4-6. Available online at: http://CRAN.R-project.org/package=vegan
Olson, M. E., Aguirre-Hernàndez, R., and Rosell, J. A. (2009). Universal foliage-stem scaling across environments and species in dicot trees: plasticity, biomechanics and Corner's Rules. Ecol. Lett. 12, 210–219. doi: 10.1111/j.1461-0248.2008.01275.x
Pagel, M. (1999). Inferring the historical patterns of biological evolution. Nature 401, 877–884. doi: 10.1038/44766
Paradis, E., Claude, J., and Strimmer, K. (2004). APE: analyses of phylogenetics and evolution in R language. Bioinformatics 20, 289–290. doi: 10.1093/bioinformatics/btg412
Penfound, W. T. (1931). Plant anatomy as conditioned by light intensity and soil moisture. Am. J. Bot. 18, 558–572. doi: 10.1002/j.1537-2197.1931.tb09609.x
Pérez-Harguindeguy, N., Díaz, S., Garnier, E., Lavorel, S., Poorter, H., Jaureguiberry, P., et al. (2013). New handbook for standardised measurement of plant functional traits worldwide. Aust. J. Bot. 61, 167–234. doi: 10.1071/BT12225
Pickup, M., Westoby, M., and Basden, A. (2005). Dry mass costs of deploying leaf area in relation to leaf size. Funct. Ecol. 19, 88–97. doi: 10.1111/j.0269-8463.2005.00927.x
Pillon, Y. (2012). Time and tempo of diversification in the flora of New Caledonia. Bot. J. Linn. Soc. 170, 288–298. doi: 10.1111/j.1095-8339.2012.01274.x
Pintaud, J. C., Jaffré, T., and Puig, H. (2001). Chorology of New Caledonian palms and possible evidence of Pleistocene rain forest refugia. C. R. Acad. Sci. III. 324, 453–463. doi: 10.1016/S0764-4469(01)01312-9
Poncet, V., Munoz, F., Munzinger, J., Pillon, Y., Gomez, C., Couderc, M., et al. (2013). Phylogeography and niche modelling of the relict plant Amborella trichopoda (Amborellaceae) reveal multiple Pleistocene refugia in New Caledonia. Mol. Ecol. 22, 6163–6178. doi: 10.1111/mec.12554
Poorter, H., Niinemets, U., Poorter, L., Wright, I. J., and Villar, R. (2009). Causes and consequences of variation in leaf mass per area (LMA): a meta-analysis. New Phytol. 182, 565–588. doi: 10.1111/j.1469-8137.2009.02830.x
Poorter, L. (1999). Growth responses of 15 rain-forest tree species to a light gradient, the relative importance of morphological and physiological traits. Funct. Ecol. 13, 396–410. doi: 10.1046/j.1365-2435.1999.00332.x
Poorter, L., and Bongers, F. (2006). Leaf traits are good predictors of plant performance across 53 rain forest species. Ecology 87, 1733–1743. doi: 10.1890/0012-9658(2006)87[1733:LTAGPO]2.0.CO;2
Posluszny, U., and Charlton, W. A. (1993). Evolution of the helobial flower. Aquat. Bot. 44, 303–324. doi: 10.1016/0304-3770(93)90074-7
Posluszny, U., and Charlton, W. A. (1999). “Multiple redundancy in hydrocharis morsus-ranae,” in The Evolution of Plant Architecture, eds M. H. Kurmann and A. R. Hemsley (London: Royal Botanical Garden, Kew), 135–146.
Pouteau, R., Trueba, S., Feild, T. S., and Isnard, S. (2015). New Caledonia: a Pleistocene refugium for rain forest lineages of relict angiosperms. J. Biogeogr. 42, 2062–2077. doi: 10.1111/jbi.12581
Preston, K. A., and Ackerly, D. D. (2003). Hydraulic architecture and the evolution of shoot allometry in contrasting climates. Am. J. Bot. 90, 1502–1512. doi: 10.3732/ajb.90.10.1502
Puttock, C. F. (1999). Revision of Atractocarpus (Rubiaceae: Gardenieae) in Australia and new combinations for some Extra-Australian Taxa. Aust. Syst. Bot. 12, 271–309. doi: 10.1071/SB97030
Revell, L. (2012). Phytools: an R package for phylogenetic comparative biology (and other things). Methods Ecol. Evol. 3, 217–223. doi: 10.1111/j.2041-210X.2011.00169.x
Robbrecht, E., and Puff, C. (1986). A survey of the Gardenieae and related tribes (Rubiaceae). Botanische Jahrbücher fur Systematik 108, 63–137.
Rossignol, L., and Rossignol, M. (1985). Architecture et tendances évolutives dans le genre Phyllanthus (Euphorbiaceae). Adansonia 3, 67–80.
Rowe, N., and Speck, T. (2005). Plant growth forms: an ecological and evolutionary perspective. New Phytologist 166, 61–72. doi: 10.1111/j.1469-8137.2004.01309.x
Rozendaal, D. M. A., Hurtado, V. H., and Poorter, L. (2006). Plasticity in leaf traits of 38 tropical tree species in response to light; relationships with light demand and adult stature. Funct. Ecol. 20, 207–216. doi: 10.1111/j.1365-2435.2006.01105.x
Rudall, P., and Bateman, R. M. (2006). Morphological phylogenetic analysis of pandanales: testing contrasting hypotheses of floral evolution. Syst. Bot. 31, 223–238. doi: 10.1600/036364406777585766
Schmid, M. (1979). “Les écosystèmes forestiers mélanésiens (Nouvelle Calédonie, Nouvelles-Hébrides, Fidji et îles Salomon),” in Ecosystèmes forestiers tropicaux, ed UNESCO (Paris: UNESCO - Natural Resources Research), 709–740.
Schmid, M. (1990). Conditions d'évolution et caractèristiques du peuplement végétal insulaire en Mélanésie Occidentale: Nouvelle-Calédonie et Vanuatu. Bulletin de la Société Zoologique de France 112, 233–254.
Schuerger, A. C., Brown, C. S., and Stryjewsky, E. (1997). Anatomical features of pepper plants (Capsicum annuum L.) grown under red light-emitting diodes supplemented with blue or far-red light. Ann. Bot. 79, 273–282. doi: 10.1006/anbo.1996.0341
Shipley, B., Vile, D., Garnier, E., Wright, I. J., and Poorter, H. (2005). Functional linkages between leaf traits and net photosynthetic rate: reconciling empirical and mechanistic models. Funct. Ecol. 19, 602–615. doi: 10.1111/j.1365-2435.2005.01008.x
Silvertown, J. (2004). The ghost of competition past in the phylogeny of island endemic plants. J. Ecol. 92, 168–173. doi: 10.1111/j.1365-2745.2004.00853.x
Silvertown, J., Francisco-Ortega, J., and Carine, M. A. (2005). The monophyly of island radiations: an evaluation of niche pre-emption and some alternative explanations. Journal of Ecology 93, 653–657. doi: 10.1111/j.1365-2745.2005.01038.x
Smith, A. C., and Darwin, S. P. (1988). “Rubiaceae,” in Flora Vitiensis Nova: a new flora of Fiji, ed A. C. Smith (Lawai: Pacific Tropical Botanical Garden), 143–376.
Smith, D. D., Sperry, J. S., Enquist, B. J., Savage, V. M., McCulloh, K. A., and Bentley, L. P. (2014). Deviation from symmetrically self-similar branching in trees predicts altered hydraulics, mechanics, light interception and metabolic scaling. New Phytol. 201, 217–229. doi: 10.1111/nph.12487
Smith, K. K. (2001). Heterochrony revisited: the evolution of developmental sequences. Biol. J. Linn. Soc. 73, 169–186. doi: 10.1111/j.1095-8312.2001.tb01355.x
Sousa-Baena, M. S., Sinha, N. R., Hernandes-Lopes, J., and Lohmann, L. G. (2018). Convergent evolution and the diverse ontogenetic origins of tendrils in angiosperms. Front. Plant Sci. 9:403. doi: 10.3389/fpls.2018.00403
Sousa-Baena, M. S., Sinha, N. R., and Lohmann, L. G. (2014). Evolution and Development of Tendrils in Bignonieae (Lamiales, Bignoniaceae). Ann. Missouri Bot. Gard. 99, 323–347. doi: 10.3417/2011018
Stebbins, G. L. (1973). Evolutionary trends in the inflorescence of angiosperms. Flora 162, 501–528. doi: 10.1016/S0367-2530(17)31733-4
Stevenson, J., and Hope, G. (2005). A comparison of late Quaternary forest changes in New Caledonia and northeastern Australia. Quat. Res. 64, 372–383. doi: 10.1016/j.yqres.2005.08.011
Sun, S., Jin, D., and Shi, P. (2006). The leaf size-twig size spectrum of temperate woody species along an altitudinal gradient: an invariant allometric scaling relationship. Ann. Bot. 97, 97–107. doi: 10.1093/aob/mcj004
Sussex, I. M., and Kerk, N. M. (2001). The evolution of plant architecture. Curr. Opin. Plant Biol. 4, 33–37. doi: 10.1016/S1369-5266(00)00132-1
Thiers, B., (continuously updated). Index Herbariorum: A Global Directory of Public Herbaria Associated Staff [Online]. New York Botanical Garden's Virtual Herbarium. Available: http://sweetgum.nybg.org/science/ih/ (Accessed Mar 20, 2018).
Tirvengadum, D. D., and Sastre, C. (1979). La signification taxonomique des modes de ramification de Randia et genres affines. Maur. Instit. Bull. 8, 77–98.
Tong, Y. H., Xu, W. B., Deng, Y. F., Wong, K. M., and Xia, N. H. (2013). Rubovietnamia sericantha (Rubiaceae: Gardenieae), a new combination and notes on the genus in China. Gard. Bull. Singapore 65, 107–114.
Tournebize, R., Manel, S., Vigouroux, Y., Munoz, F., de Kochko, A., and Poncet, V. (2017). Two disjunct Pleistocene populations and anisotropic postglacial expansion shaped the current genetic structure of the relict plant Amborella trichopoda. PLoS ONE 12:e0183412. doi: 10.1371/journal.pone.0183412
Trueba, S., Isnard, S., Barthélémy, D., and Olson, M. E. (2016). Trait coordination, mechanical behaviour and growth form plasticity of Amborella trichopoda under variation in canopy openness. AoB Plants 8, 1–18. doi: 10.1093/aobpla/plw068
Van Steenis, C. G. G. J. (1963). Definition of the concept “inflorescence” with special reference to ligneous plants. Flora Malesiana Bull. 18, 1005–1007.
Veillon, J. M. (1976). Architecture Végétative de Quelques Arbres de l'archipel Néo-caledonien. Ph.D. thesis, Université des sciences et techniques du Languedoc, Montpellier.
Vertessy, R. A., Benyon, R. G., O'Sullivan, S. K., and Gribben, P. R. (1995). Relationships between stem diameter, sapwood area, leaf area and transpiration in a young mountain ash forest. Tree Physiol. 15, 559–567. doi: 10.1093/treephys/15.9.559
Wagner, S. T., Hesse, L., Isnard, S., Samain, M. S., Bolin, J., Maass, E., et al. (2014). Major trends in stem anatomy and growth forms in the perianth-bearing Piperales, with special focus on Aristolochia. Ann. Bot. 113, 1139–1154. doi: 10.1093/aob/mcu044
Warming, E. (1909). Oecology of Plants: An Introduction to the Study of Plant-Communities. Oxford: Clarendon Press.
Weijschedé, J., Berentsen, R., de Kroon, H., and Huber, H. (2007). Variation in petiole and internode length affects plant performance in Trifolium repens under opposing selection regimes. Evol. Ecol. 22, 383–397. doi: 10.1007/s10682-007-9224-2
Westoby, M., Falster, D. S., Moles, A. T., Vesk, P. A., and Wright, I. J. (2002). Plant Ecological strategies: some leading dimensions of variation between species. Annu. Rev. Ecol. Syst. 33, 125–159. doi: 10.1146/annurev.ecolsys.33.010802.150452
Westoby, M., and Wright, I. J. (2003). The leaf size-twig size spectrum and its relationship to other important spectra of variation among species. Oecologia 135, 621–628. doi: 10.1007/s00442-003-1231-6
White, P. S. (1983a). Corner's rules in eastern deciduous trees: allometry and its implications for the adaptive architecture of trees. Bull. Torrey Botan. Club 110, 203–212. doi: 10.2307/2996342
White, P. S. (1983b). Evidence that temperate east noth american evergreen woody plants follow Corner's rules. New Phytol. 95, 139–145. doi: 10.1111/j.1469-8137.1983.tb03477.x
Wong, K. M. (2004). Bungarimba (Rubiaceae), a new genus distinguished from Porterandia and other allies. Sandakania 15, 25–54.
Wright, I. J., Ackerly, D. D., Bongers, F., Harms, K. E., Ibarra-Manriquez, G., Martinez-Ramos, M., et al. (2007). Relationships among ecologically important dimensions of plant trait variation in seven neotropical forests. Ann. Bot. 99, 1003–1015. doi: 10.1093/aob/mcl066
Zahid, M. S., and Wong, K. M. (2004). Tree architecture in Porterandia (Rubiaceae). Sandakania 15, 79–91.
Keywords: branching index, convergence, corner's rules, gardenieae, Island, rainforest, treelet, understory
Citation: Bruy D, Hattermann T, Barrabé L, Mouly A, Barthélémy D and Isnard S (2018) Evolution of Plant Architecture, Functional Diversification and Divergent Evolution in the Genus Atractocarpus (Rubiaceae) for New Caledonia. Front. Plant Sci. 9:1775. doi: 10.3389/fpls.2018.01775
Received: 10 July 2018; Accepted: 15 November 2018;
Published: 04 December 2018.
Edited by:
Jill Christine Preston, University of Vermont, United StatesReviewed by:
Marie-Stéphanie Samain, Instituto de Ecología (INECOL), MexicoJohn R. Paul, University of San Francisco, United States
Copyright © 2018 Bruy, Hattermann, Barrabé, Mouly, Barthélémy and Isnard. This is an open-access article distributed under the terms of the Creative Commons Attribution License (CC BY). The use, distribution or reproduction in other forums is permitted, provided the original author(s) and the copyright owner(s) are credited and that the original publication in this journal is cited, in accordance with accepted academic practice. No use, distribution or reproduction is permitted which does not comply with these terms.
*Correspondence: David Bruy, ZGF2aWQuYnJ1eUBpcmQuZnI=