- College of Life Science, Shandong Normal University, Jinan, China
Abiotic stresses, such as low or high temperature, deficient or excessive water, high salinity, heavy metals, and ultraviolet radiation, are hostile to plant growth and development, leading to great crop yield penalty worldwide. It is getting imperative to equip crops with multistress tolerance to relieve the pressure of environmental changes and to meet the demand of population growth, as different abiotic stresses usually arise together in the field. The feasibility is raised as land plants actually have established more generalized defenses against abiotic stresses, including the cuticle outside plants, together with unsaturated fatty acids, reactive species scavengers, molecular chaperones, and compatible solutes inside cells. In stress response, they are orchestrated by a complex regulatory network involving upstream signaling molecules including stress hormones, reactive oxygen species, gasotransmitters, polyamines, phytochromes, and calcium, as well as downstream gene regulation factors, particularly transcription factors. In this review, we aimed at presenting an overview of these defensive systems and the regulatory network, with an eye to their practical potential via genetic engineering and/or exogenous application.
Introduction
Land plants are living in an inherently harsh environment ever since their emergence. A large variety of physical or chemical factors are hostile to them, including low or high temperature, deficient or excessive water, high salinity, heavy metals, and ultraviolet (UV) radiation, among others. These stresses, collectively referred to as abiotic stresses, are posing a severe threat to agriculture and the ecosystem, accounting for great crop yield loss (Wang et al., 2003; Wania et al., 2016). Salt stress is the most stubborn one magnified by ever-increasing salinization of arable land worldwide (Munns and Tester, 2008; Yuan et al., 2015b). Most plants cannot survive when NaCl concentrations exceed 200 mM (Flowers and Colmer, 2008; Zhou J.C. et al., 2016) because high salinity extensively impinges on their lifecycle comprising, if available, seed germination, seedling establishment, vegetative growth, and flower fertility (Guo et al., 2012, 2015, 2018), as a consequence of ionic toxicity, osmotic pressure, oxidative damage, and nutritional shortage (Zhao et al., 2010; Feng et al., 2014a,b). More seriously, it is interlinked with drought, another global issue, which can be aggravated by extreme temperatures (Ashraf and Foolad, 2007; Slama et al., 2015).
Due to their sessile nature, plants have to confront the stresses and develop potent adaptive tactics to avoid or tolerate their adverse effects so as to survive and to thrive. Plenty of cellular, physiological and morphological defenses have been established. The most apparent one is the cuticle, a universal outmost shield (Shepherd and Wynne Griffiths, 2006; Yeats and Rose, 2013; Fich et al., 2016). It is also impressive that recretohalophytes even evolved a specialized organ to excrete salt, as represented by the epidermal salt gland of Limonium bicolor (Yuan et al., 2013, 2016a). Tremendous progress has been made toward understanding the biochemical and molecular mechanisms underpinning the defenses, owing to forward and reverse genetic approaches as well as genome-wide analyses conducted on various model species like the classical model Arabidopsis thaliana and its extremophyte relative Thellungiella salsuginea that has exceptional multistress resistance (Amtmann, 2009; Wang J.S. et al., 2017).
It is thus emerging that desaturation of membrane lipids, activation of reactive species (RS) scavengers, induction of molecular chaperones, and accumulation of compatible solutes are more generalized and conserved cellular defense responses. This is in line with the fact that membrane injury, RS damage, protein denaturation, and osmotic stress (primarily dehydration) can be provoked by a multitude of abiotic stresses. In stress response, these defenses are orchestrated by a complex regulatory network involving upstream signaling molecules including stress hormones [e.g., abscisic acid (ABA)], reactive oxygen species (ROS), hydrogen sulfide (H2S), nitric oxide (NO), polyamines (PAs), phytochromes, and calcium (Ca2+), as well as downstream gene regulation factors, particularly transcription factors (TFs) (Figure 1).
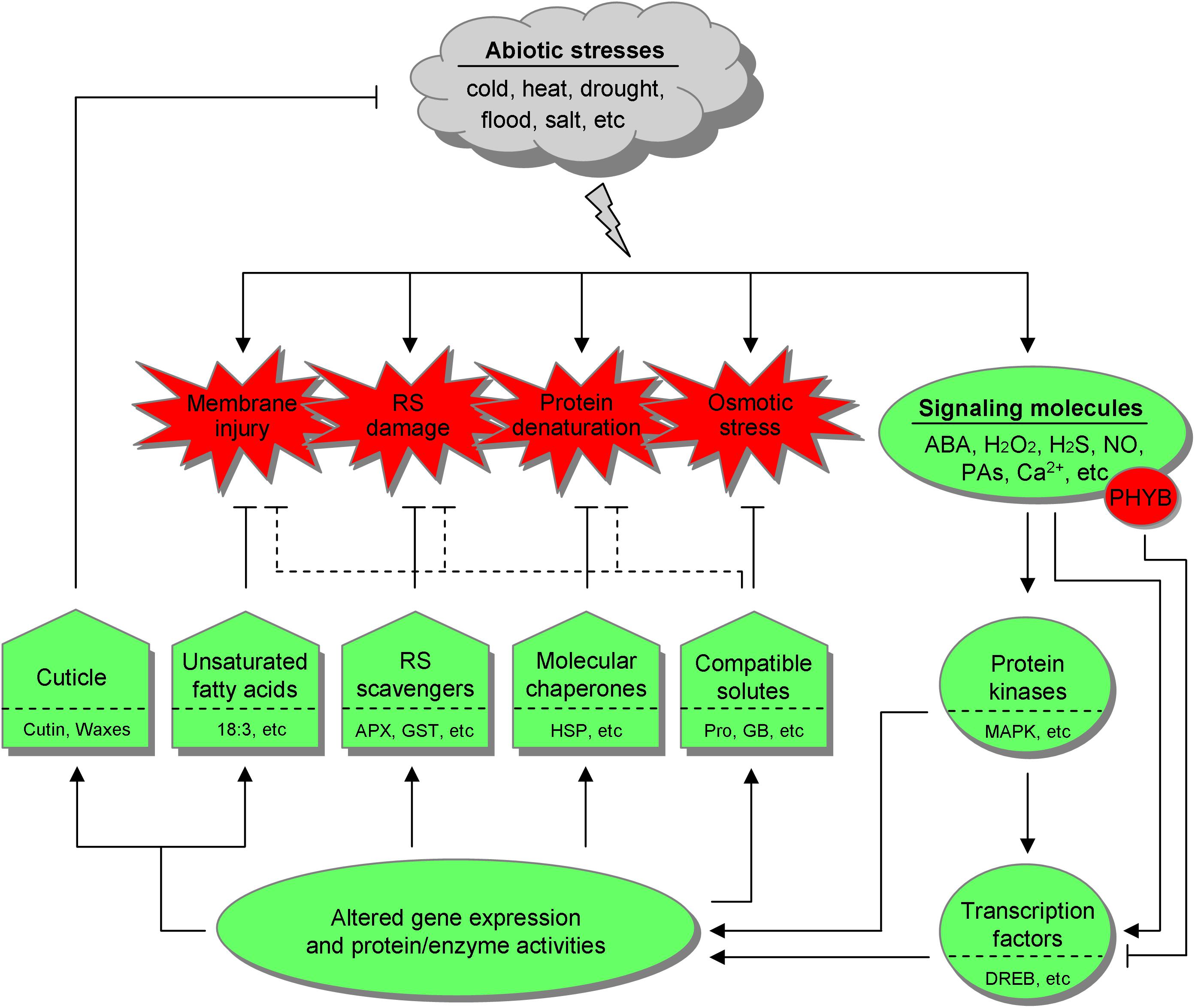
Figure 1. The general defense systems and the underlying regulatory network in botanic responses to abiotic stresses. Different abiotic stresses, such as cold, heat, drought, flood, and salt can provoke common cellular disorder and secondary stresses, including membrane injury, reactive species (RS) damage, protein denaturation, and osmotic stress, which are also interconnected with each other. Accordingly, land plants have resorted to unsaturated fatty acids, RS scavengers, molecular chaperones, and compatible solutes. Some compatible solutes may also be involved in counteracting other adverse effects, as indicated with dotted inhibitory lines. Besides, the cuticle serves as the universal outermost shield. Upon stress stimulation, signaling molecules mobilize the downstream effectors, primarily protein kinases and transcription factors, leading to altered gene expression and protein/enzyme activities, thereby launching the defense systems. Notably, phytochrome B (PHYB) is emerging as a negative regulator in stress tolerance. 18:3, linolenic acid; APX, ascorbate peroxidase; GST, glutathione S-transferase; HSP, heat shock protein; Pro, proline; GB, glycine betaine; ABA, abscisic acid; PAs, polyamines; MAPK, mitogen-activated protein kinase; DREB, dehydration responsive element binding factor.
In the field, plants are routinely exposed to an unpredictable combination of different stresses rather than a single one (Slama et al., 2015; Wania et al., 2016), which is even worse in the context of climate change, soil salinization and environmental pollution. Particularly, on demand of population growth, it is getting imperative to equip crops with multistress tolerance. To this end, in this review, we are attempting to present an overview of the general defense systems and the major nodes of their underlying regulatory network (Figure 1), with an eye to their practical potential via genetic engineering and/or exogenous application.
General Defenses Against Abiotic Stresses
In this section, five general botanic defenses against abiotic stresses will be addressed, comprising cuticle as outermost shield, unsaturated fatty acids (UFAs) as membrane modulator and oxylipin precursor, RS scavengers that govern RS homeostasis, molecular chaperones that stabilize proteins and subcellular structures (e.g., membrane), as well as compatible solutes that act more than osmoprotectants (Figure 1). Chances to acquire multistress tolerance based on them are given in Table 1. Notably, cuticular waxes, UFAs, antioxidant compounds, and compatible solutes are important economic traits as well. For example, waxes are raw materials of manifold products including biofuels, cosmetics, detergents, plastics, and pharmaceuticals (Lee and Suh, 2015). Therefore, increasing their yield actually serves a double purpose in crop improvement.
Cuticle
Land plants have an exterior translucent lipid structure, namely the cuticle, sealing the aerial surfaces of their organs. The thin hydrophobic layer is basically a cutin matrix filled with and coated by cuticular waxes. As the primary interface between plant and environment, the cuticle plays critical roles in restricting liquid and gas fluxes, defending pathogen and insect attacks, and resisting various abiotic stresses. It is an elegant innovation of land plants to deploy an outermost shield derived from simple molecules, which is fundamental to their success in terrestrial colonization (for review, see Shepherd and Wynne Griffiths, 2006; Yeats and Rose, 2013; Fich et al., 2016). By contrast, cell wall, the second barrier that is actively remodeled under abiotic stresses (Shen et al., 2014; Fernandes et al., 2016), is much more complex and less understood thus far (for review, see Le Gall et al., 2015; Tenhaken, 2016).
The cuticle is exclusively created by epidermal cells. Typically, cutin is a macromolecular polyester of C16 or C18 oxygenated fatty acids (FAs), whereas waxes are a complex mixture of C24 to C34 FA derivatives, including alcohols, aldehydes, alkanes, esters, and ketones. Their biosynthetic pathways are nearly resolved and have been well documented (see Pollard et al., 2008; Beisson et al., 2012; Fich et al., 2016 for cutin; see Shepherd and Wynne Griffiths, 2006; Kunst and Samuels, 2009; Bernard and Joubès, 2013 for waxes). Briefly, both of them stem from acetyl-coenzyme A (CoA) via de novo FA synthesis in plastids, with the accession of two carbons in each recurring cycle until the emergence of C16/C18 products, which are then transported to the endoplasmic reticulum (ER) to undergo either oxidation and incorporation to become cutin precursors (monoacylglycerols) or elongation and modifications to become wax components. Notably, two distinct modification pathways are involved in wax generation, the alcohol-forming (or acyl-reduction) pathway for primary alcohols and esters, together with the alkane-forming (or decarbonylation) pathway for aldehydes, alkanes, secondary alcohols, and ketones.
To assemble the apoplastic cuticle, these materials need to be exported from the ER to the plasma membrane (PM), and then across the PM through the cell wall onto the outer surface where cutin monomers polymerize and wax members crystallize. Membrane vesicle trafficking (McFarlane et al., 2014) is one of the ways involved in intracellular cargo delivery to the ATP-binding cassette (ABC) transporters that channel the PM (Pighin et al., 2004; Yeats and Rose, 2013; Fich et al., 2016). The likely extracellular relays for traversing the hydrophilic cell wall are non-specific lipid transfer proteins (nsLTPs), a group of small and basic proteins bearing a hydrophobic pocket for lipid binding. Indeed, two glycosylphosphatidylinositol-anchored LTPs, LTPG1 and LTPG2, as well as a secreted one, TsnsLTP4 from Thellungiella, have been reported to be implicated in wax deposition (Debono et al., 2009; Kim et al., 2012; Sun et al., 2015).
Then comes the last procedure of cutin production, i.e., the esterification of the monomers into a polymeric matrix. The crosslink is formed directly or via a bridging molecule, e.g., glycerol or ferulic acid (Deng et al., 2015; Fich et al., 2016). However, both the polymerization mechanism and the polyester architecture have been longstanding enigmas. The former is now beginning to be unveiled with the identification of CUTIN SYNTHASE 1 (CUS1), a cuticle-localized member of the GDSL lipase/hydrolase superfamily, from tomato (Solanum lycopersicum) (Girard et al., 2012; Yeats et al., 2012). Nevertheless, since the fruit of CUS1 null mutant, cutin deficient 1 (cd1), are not fully deprived of cutin, non-enzymatic mechanisms cannot be ruled out yet (Yeats et al., 2012; Fich et al., 2016).
Drought tolerance is closely associated with wax accumulation in a wide variety of plant species (see reviews Borisjuk et al., 2014; Xue et al., 2017). With respect to multistress tolerance, however, wax composition makes a difference and the alcohol-forming pathway seems to outperform the alkane-forming one. ECERIFERUM 1 (CER1), the aldehyde decarbonylase (AD) responsible for n-alkane synthesis, could be reduced by cold, though induced by NaCl, mannitol (dehydration), and ABA in Arabidopsis (Bourdenx et al., 2011). It is highly possible that in CER1-overexpressing plants, cold tolerance was compromised, in parallel with pathogen defense and leaf growth, although water deficit resistance was improved (Bourdenx et al., 2011). Indeed, increased level of n-alkane coupled with decreased level of primary alcohols led to cold susceptibility and growth retardation. In contrast, higher contents of both resulted in better viability under drought and freezing without disturbing plant growth (Zhang et al., 2007). Besides, fatty acyl-CoA reductases (FARs) that produce primary alcohols could be up-regulated by cold, heat, polyethylene glycol (PEG; dehydration), ABA, methyl jasmonate (MeJA), and fungal infection in wheat (Triticum aestivum) (Chai et al., 2018). The stress-resistant performance of FAR-overexpressing plants is thus intriguing.
Another good candidate for genetic engineering might be CER6, the major 3-ketoacyl-CoA synthase (KCS) that catalyzes the initial and rate-limiting condensation step of FA elongation, as its overexpression could elevate total wax output with little alteration of the composition. Of note, it was not the cauliflower mosaic virus (CaMV) 35S promoter but the native one that could drive CER6 expression high enough to achieve significantly greater wax quantity in transgenic Arabidopsis (Hooker et al., 2002). In addition to the enzymes, transporters can also be taken into consideration. Actually, TsnsLTP4, responsive to cold, heat, NaCl, PEG, and ABA, has been introduced into Arabidopsis and augmented its tolerance to drought and salt (Sun et al., 2015). Notably, more chances reside in manipulating the TFs that control cuticle generation, which will be discussed in the end.
Unsaturated Fatty Acids
C16/C18 FAs are not only the prime stocks for the cuticle, but the key ingredients of the membranes, the fundamental biological barriers. The main building blocks of botanic membranes are phospholipids and glycolipids that both contain a glycerol core linked with two FA-derived “tails”. FAs thereby have a profound impact on membrane properties. Particularly, their unsaturation degree is a major determinant of membrane fluidity in that UFA chain will create a kink at a cis-double bond, which serves as steric hindrance in intermolecular package leading to a more fluid state (Hazel, 1995; Mikami and Murata, 2003).
Membrane fluidity is susceptible to various abiotic stresses, extreme temperatures in particular. Both cold-driven rigidification and heat-driven fluidization can cause biomembrane dysfunction, as exemplified by protein deactivation and ion leakage (Hazel, 1995). Cytoskeleton destabilization is also a direct consequence (Sangwan et al., 2002; Liu et al., 2013). Membrane remodeling is thus of especial importance in plants, which are poikilothermic organisms. Indeed, adjusting the unsaturation degree of the FA tails in bilayer interior is favored by plants in offsetting thermal perturbations to maintain the optimal range of fluidity. Particularly, there is a very close relationship between chilling tolerance and the unsaturation level of chloroplastic phosphatidylglycerol (PG) (for review, see Nishida and Murata, 1996; Iba, 2002).
In thylakoid membranes that are biased toward glycolipids, PG is the only phospholipid species present. Actually, it is an indispensable component of the membrane-bound photosynthetic apparatus including Photosystem II (PSII) (Wada and Murata, 2007). PSII is vulnerable to photoinhibition, in which the D1 protein of the reaction center is bound to continuous photodamage followed by repair via proteolysis and synthesis (Takahashi and Murata, 2008; Liu et al., 2016). Desaturation of PG has been shown to protect PSII against cold-enhanced photoinhibition, which contributes to chilling tolerance (Moon et al., 1995). This is also applicable to other stresses that can intensify photoinhibition (Takahashi and Murata, 2008). Upon NaCl treatment, for instance, alleviated photoinhibition of PSII pertained to increased contents of UFAs in membrane lipids including PG (Sui et al., 2010; Sui and Han, 2014; Liu et al., 2017). Indeed, specifically elevating the unsaturation level of PG accelerated the turnover of the D1 protein (Sun et al., 2010).
It is noteworthy that polyunsaturated UFAs, upon liberation by lipase from glycerolipids, also serve as the raw material of oxylipins, bioactive molecules involved in diverse physiological processes, including stress resistance (see review Savchenko et al., 2014). Particularly, linolenic acid (18:3) gives birth to jasmonic acid and its derivatives, namely jasmonates (JAs), a group of stress hormones with a well-understood role in launching wound response. There is emerging evidence that JA is also implicated in defense against other stresses, such as salt (Ryu and Cho, 2015; Yang et al., 2017) and UV (Mackerness et al., 1999; Conconi et al., 2006). It is of economic interest that applying MeJA to fruits and vegetables can reduce chilling injury, which is conducive to the maintenance of their post-harvest quality (González-Aguilar et al., 2000; Ding et al., 2002).
Unsaturation is administered by position-specific FA desaturases (FADs). To synthesize C18 UFAs that are more active in stress tolerance, C18 product of de novo FA synthesis linked to acyl-carrier protein (ACP), namely 18:0-ACP, is first converted to 18:1(9)-ACP by stearoyl-ACP desaturase (SAD). After being incorporated into glycerolipids, 18:1(9) is processed to 18:2(9, 12) by ω-6 desaturases and then to 18:3(9, 12, 15) by ω-3 desaturases (Murata and Los, 1997). To resist various stresses like cold and wounding, the level of 18:3 is usually elevated. This is much achieved by activation of ω-3 desaturases comprising ER-associated FAD3 and plastid-localized FAD7 and FAD8, as revealed by mounting evidence (Shi Y. et al., 2018; Sui et al., 2018). On the contrary, gene silencing of FAD7 enabled transgenic tobacco (Nicotiana tabacum) to abide high temperatures (Murakami et al., 2000). Hence, inducible overexpression of FADs might be better so that heat tolerance can be covered.
The unsaturation level of chloroplast PG is otherwise determined by the substrate specificity of plastid glycerol-3-phosphate acyltransferase (GPAT), which catalyzes the first reaction to esterify FAs into glycerolipids. A preference for 18:1-ACP matters as the second reaction always utilizes 16:0-ACP (Nishida and Murata, 1996; Iba, 2002). Interestingly, GPATs from Arabidopsis (resistant) and squash (Cucurbita moschata) (sensitive) respectively assimilated the chilling behavior of tobacco (intermediate) (Murata et al., 1992), whereas GPAT from Suaeda salsa (euhalophyte) ameliorated salt tolerance of Arabidopsis (glycophyte) (Sui et al., 2017). Actually, under saline situations, S. salsa also exhibited chilling resistance (Cheng et al., 2014). Moreover, ACPs, as essential cofactors for FA synthase (FAS), SAD and GPAT, are also implicated in the alteration of FA composition. Transformation with AhACP1 from peanut (Arachis hypogaea) into tobacco resulted in significantly higher contents of 18:2 and 18:3 accompanied with more tolerance against cold (Tang et al., 2012).
Reactive Species Scavengers
An inherent paradox in normal metabolism of aerobic organisms is the endless generation of noxious RS, particularly ROS including superoxide anion (O2•-), hydrogen peroxide (H2O2), hydroxyl radical (•OH), and singlet oxygen (1O2), as well as reactive carbonyl species (RCS) like malondialdehyde [MDA; CH2(CHO)2] and methylglyoxal (MG; CH3COCHO). The two types of RS are intertwined with each other. RCS can arise from ROS-induced lipid peroxidation, while ROS can be raised by RCS activities the other way round. Virtually all abiotic stresses can trigger a burst of both ROS and RCS, turning their scavengers into general defenses. Nevertheless, ROS and MG have been identified to play a signaling role at low levels, which are also tactically exploited to facilitate stress perception and retort their elicitors [see review (Hasanuzzaman et al., 2017) for both ROS and MG]. Therefore, it is pivotal to maintain the delicate RS homeostasis, which needs to be taken into account in manipulating RS scavengers for multistress tolerance.
Reactive Oxygen Species
Plant cells carry an even heavier burden of ROS imposed by the electron transport chain of chloroplasts. Once overproduced, these small chemicals can readily attack various biomolecules encompassing carbohydrates, lipids, proteins, and nucleic acids, leading to oxidative catastrophe including enhanced photoinhibition and membrane lesions, which can be measured by the production of MDA from UFA peroxidation (Takahashi and Murata, 2008; Guo et al., 2012; Sui, 2015). Actually, MDA is a latent RCS that can initiate a new round of attack in acidic conditions, forming covalent adducts known as advanced lipoxidation end-products (ALEs), leading to protein dysfunction and consequent ROS proliferation (Farmer and Davoine, 2007; Deng et al., 2010).
Plants have therefore developed a sophisticated ROS scavenging system utilizing both non-enzymatic and enzymatic means. A good many metabolites possess antioxidant properties, such as betalains, carotenoids, flavonoids, and vitamin E (Gechev et al., 2006; Zhao S.Z. et al., 2011). Specialized enzymes comprise superoxide dismutases (SODs), catalases (CATs), and various peroxidases (PODs). SODs convert O2•- into H2O2 for further reduction to water by CATs and PODs. Besides, the ascorbate-glutathione (ASA-GSH) cycle required for ascorbate peroxidase (APX) involves dehydroascorbate reductase (DHAR), monodehydroascorbate reductase (MDHAR) and glutathione reductase (GR). Other enzymes, such as glutathione S-transferase (GST) and ferritins, also partake in detoxification (see reviews Mittler et al., 2004; Gechev et al., 2006; Sharma et al., 2012).
Undoubtedly, multistress tolerance can be acquired via engineering the detoxifying enzymes. APXs, key enzymes ensuring H2O2 removal, helped transgenic plants oppose drought, salt and high light (Pang et al., 2011; Li K. et al., 2012; Cao et al., 2017), while GSTs, which catalyze GSH conjugation and bear glutathione peroxidase (GPX) activity as well, appended cold, heat, paraquat, UV, and heavy metals to the list (Roxas et al., 2000; Qi et al., 2010a; Kumar and Trivedi, 2018). However, exogenous application of cerium oxide nanoparticles (nanoceria) is emerging to be a more facile alternative, owing to their distinct capacities to catalytically clear ROS without substrate restriction and then readily regenerate via switch between the two oxidative states (Ce3+ and Ce4+) (Newkirk et al., 2018). One successful case is that anionic nanoceria with a low Ce3+/Ce4+ ratio applied to Arabidopsis leaves protected the photosynthetic machinery from chilling, heat and high light (Wu et al., 2017). Additionally, it should be cautious in crop cultivation that excessive use of fertilizer nitrogen can depress the ROS scavenging system leading to increased stress susceptibility (Kong et al., 2017).
Reactive Carbonyl Species
Methylglyoxal, a major type of RCS, is drawing increasing attention in stress scenario. In plant cells, glycolysis operates as the principal source of this cytotoxin, due to the non-enzymatic dephosphorylation of two intermediates, glyceraldehyde-3-phosphate and dihydroxyacetone phosphate. Once overaccumulated, MG can also damage various biomolecules, especially with its aldehyde group. In addition to forming advanced glycation end-products (AGEs) in analogy to ALEs, MG can further ROS production by catalyzing the photoreduction of O2 to O2•- in chloroplasts and consuming GSH via spontaneous combination into hemithioacetal, leading to a vicious cycle and ultimate cell death (for review, see Hoque et al., 2016; Mostofa et al., 2018).
To detoxify MG and other 2-oxoaldehydes, plants have been armed with the glyoxalase system consisting of Gly I, Gly II, and Gly III. The former two enzymes work sequentially in a GSH-dependent way. The hemithioacetal adduct from MG and GSH is isomerized by Gly I into S-D-lactoylglutathione, which is then hydrolyzed by Gly II into D-lactate with the regeneration of GSH. By contrast, MG is directly converted to D-lactate by Gly III without the assistance of GSH, rendering a shortcut for its detoxification. Subsequently, D-lactate is processed into pyruvate by D-lactate dehydrogenase. It is ingenious that the toxic byproduct is not just eliminated, but recycled into an essential metabolite (Hoque et al., 2016; Hasanuzzaman et al., 2017; Mostofa et al., 2018). Remarkably, GSH not only serves as a bridge between the antioxidant and glyoxalase systems, but can also trap NO (see below), the primary reactive nitrogen species (RNS) interwoven with ROS, highlighting its significance in RS homeostasis and stress defense.
Genetic manipulation of the glyoxalase system, individually or together, to potentiate tolerance against multiple abiotic stresses has worked in various plant species, as exemplified by tobacco plants transformed with Gly I, which are capable of resisting drought, salt, heavy metals, and oxidative stress (Hoque et al., 2016). There are also some minor routes available for MG neutralization. For instance, MG, as with MDA, can be reduced by aldo-keto reductases (AKRs). Accordingly, ectopic expression of AKR guarded transgenic tobacco exposed to heat and oxidative stress (Hasanuzzaman et al., 2017). Interestingly, γ-aminobutyric acid (GABA), a natural amino acid with versatile roles including anti-stress signaling (see reviews Kinnersley, 2000; Bouché and Fromm, 2004), can trap MDA via direct reaction with the aldehyde groups (Deng et al., 2010), which means that MG can be blocked likewise.
Molecular Chaperones
Heat shock proteins (HSPs) are well-known molecular chaperones, which are induced or constitutively expressed to facilitate protein folding, assembly, transport, and degradation. The anti-stress role of HSPs is not limited to their definition. In fact, this large family is a universal salvation system employed by virtually all living organisms to counteract all detrimental conditions that can induce protein damage, wherein they function to prevent aggregation of denatured proteins, assist in their refolding or present them to lysosomes or proteasomes for proteolysis, thereby restoring cellular homeostasis (for review, see Kregel, 2002; Wang et al., 2004). Besides, some unusually hydrophilic proteins, such as late embryogenesis abundant (LEA) and cold-regulated (COR) members might also function as chaperones to stabilize proteins and membranes against stress injury (see review Thomashow, 1999).
According to the molecular weight, there are five conserved HSP classes, namely HSP100/Clp, HSP90, HSP70/DnaK, HSP60/Chaperonin, and small HSP (smHSP). HSP70 is the most conserved one across different species, which consists of an N-terminal ATPase domain and a C-terminal substrate-binding domain. Binding and release of hydrophobic peptides rely on hydrolysis and recycling of ATP, which require the assistance of its co-chaperones including J-domain proteins (HSP40/DnaJ) that stimulate ATPase activity, and nucleotide exchange factors (NEFs) (e.g., HSPBP-1) that promote release of ADP and binding of fresh ATP. Intriguingly, Arabidopsis Fes1A, an ortholog of HSPBP-1, did not show NEF activity in vitro, but acted as an antagonist of HSP70 degradation (Zhang J.X. et al., 2010; Fu et al., 2015).
Notably, via physical interaction, HSP70 can modulate the activities of signal transducers, TFs, and/or metabolic enzymes, thereby exerting a profound influence on signaling pathways. Despite that it seems to be a negative regulator of HS response, substantial evidence has bonded it to thermoprotection in living organisms (Sung and Guy, 2003; Montero-Barrientos et al., 2010). Interestingly, higher SOD and POD activities even without HS were also brought to tobacco by HSP70 from Brassica campestris (Wang X. et al., 2016), implying cross-tolerance to other stresses. However, it should be noted that in Arabidopsis its constitutive overproduction had pleiotropic consequences, dwarfism for instance (Sung and Guy, 2003).
By contrast, smHSP is the most diverse one in higher plants, with many subclasses distinct in protein sequence, cellular location and induction pattern, highlighting their special importance. smHSPs are clustered by the conserved C-terminal domain shared with vertebrate α-crystallin found in the eye lens, which is involved in oligomer formation and ATP-independent chaperone activity. Under stress challenges, drastically accumulated smHSPs are likely to seize non-native proteins to avoid their aggregation and then transfer them to ATP-dependent chaperones such as the HSP70 system for renaturation (see reviews Sun et al., 2002; Wang et al., 2004). It was newly reported that Arabidopsis transformed with HSP16.4 from pepper (Capsicum annuum) were less vulnerable to drought, heat and their combination, with the ROS scavenging enzymes being more active under stressful conditions (Huang et al., 2018).
Compatible Solutes
Compatible solutes are small organic compounds with electrical neutrality, high solubility and low toxicity that can even mount up to fairly high concentrations inside cells with few perturbations. Basically, qualified molecules are sugars, amino acids and their derivatives such as raffinose, trehalose, inositol, mannitol, proline (Pro), and glycine betaine (GB). In general, under stressful circumstances, these metabolites may accrue to act as osmoprotectants against dehydration, scavengers of RS, and/or stabilizers of proteins and membranes. Pro, a widely present one, is also able to buffer cellular redox potential and induce gene expression (for review, see Yancey, 2005; Ashraf and Foolad, 2007; Slama et al., 2015).
There have been successful cases of improving stress tolerance via genetic manipulating the metabolic enzymes of some compatible solutes. For example, raffinose is derived from sucrose via addition of galactose, which is donated by galactinol created from UDP-galactose and myo-inositol by galactinol synthase (GOLS). Transgenic plants expressing this key enzyme could withstand cold, drought and salt (Sun et al., 2013; Zhuo et al., 2013). Remarkably, antisense suppression of proline dehydrogenase (ProDH), which implements the first degradation step of Pro, even allowed Arabidopsis to survive -7°C and 600 mM NaCl (Nanjo et al., 1999). Alternatively, in spite that further knowledge is required to optimize the efficiency and minimize side effects, exogenous application of these solutes is emerging to be a more feasible and effective way, especially for GB, as the productivity of its biosynthetic enzymes was limited by substrate availability in engineered plants (Ashraf and Foolad, 2007).
Regulatory Network Underlying Defense Systems
In combat against abiotic stresses, the five general defenses are orchestrated by an intricate regulatory network composed of numerous signaling molecules and gene regulation factors. Here, we will just deal with some better characterized ones. Once triggered, stress hormones (ABA), ROS (H2O2), H2S, NO, PAs, phytochrome B (PHYB), and Ca2+, extensively interplay with others at various levels, synergistically or antagonistically (Figure 2), to establish a precise directive for downstream effectors, TFs in particular, to alter gene expression and protein/enzyme activities in a specific pattern, thereby launching a proper response (Figure 1). The regulatory factors render considerable opportunities to generate multistress tolerance, with examples related to the focused ones being listed in Table 2.
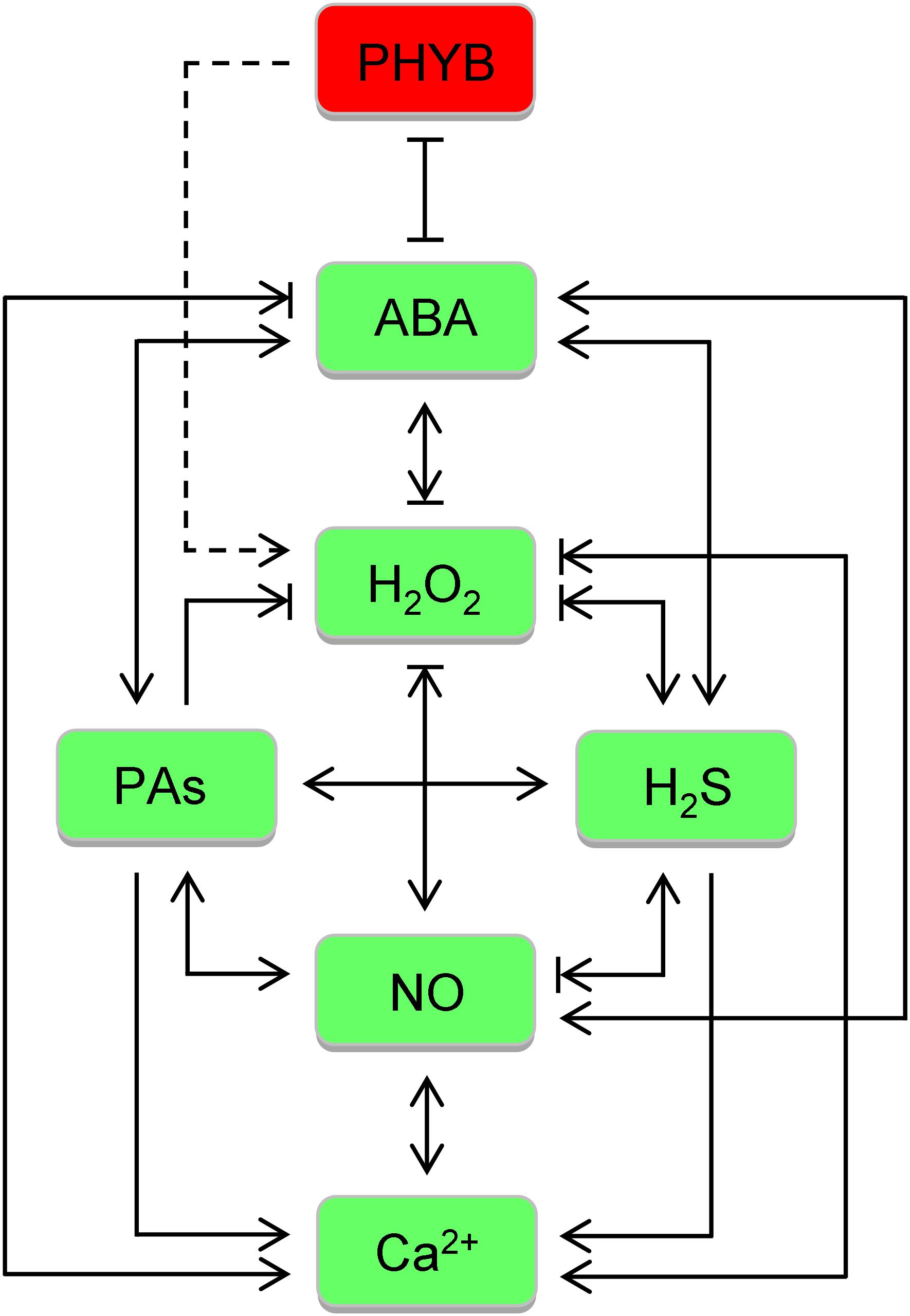
Figure 2. Crosstalk between signaling molecules focused in the review in botanic responses to abiotic stresses. Once triggered, abscisic acid (ABA), H2O2, H2S, NO, polyamines (PAs), phytochrome B (PHYB), and Ca2+, extensively interplay with others at various levels, synergistically or antagonistically. For simplification, the two effects are shown in combination. Dashed line is used between PHYB and H2O2 as PHYB is emerging to play a negative role in its scavenging. Of note, H2O2, H2S, NO, and PAs can actually block each other via chemical reaction, though not indicated.
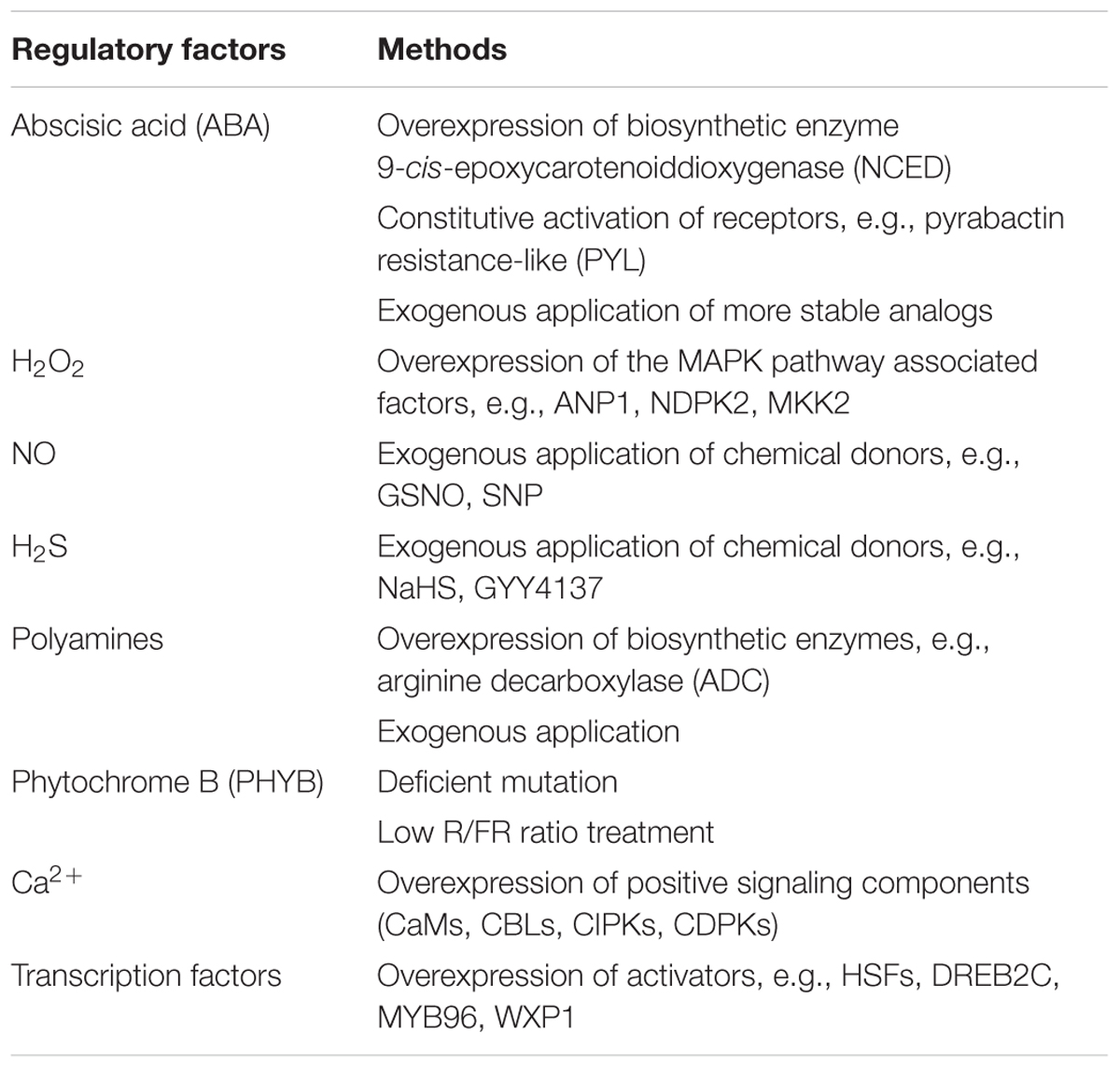
Table 2. Chances to generate multistress tolerance based on the regulatory network underlying the general defenses.
Stress Hormones
Phytohormones such as ABA, ethylene (ET), JA, and salicylic acid (SA) are important organizers of systemic stress defense, which coordinate in the elaborate hormonal signalsome (for review, see Wania et al., 2016; Tiwari et al., 2017). Notably, melatonin, a universal multi-regulatory molecule across all lifeforms, is increasingly recognized as a potent biostimulator against stress in plant. One notable aspect of this yet to be licensed phytohormone is that it operates as if a commander of other phytohormones (see reviews Arnao and Hernández-Ruiz, 2018; Kanwar et al., 2018). Nevertheless, ABA is the most prominent stress hormone, which not only extensively interplays with other phytohormones (see Table 3 for some updates), but with all following signaling molecules (Figure 2). Particularly, components of all biochemical defenses remarked above can be mobilized by ABA, including cuticular waxes (Lee and Suh, 2015), 18:3 (Yin et al., 2018), HSPs (Huang et al., 2016), Pro (Ashraf and Foolad, 2007), antioxidants (Liu F. et al., 2018), and RS detoxifying enzymes (Chen et al., 2013; Hoque et al., 2016).
Stress stimuli can rapidly trigger de novo synthesis of ABA from oxidative cleavage of β-carotene, with 9-cis-epoxycarotenoiddioxygenase (NCED) being the rate-limiting enzyme. For sequestration of this hormone, two ways are available. One is hydroxylation involving cytochrome P450 type enzyme (CYP707A), the other is conjugation to glucose by glycosyltransferase. The latter actually creates a bin for ABA recycling carried out by glycosidase, offering a shortcut for stress induction. To establish systemic response, ABA can be actively transported across the PM by ABC transporters and then recognized by pyrabactin resistance (PYR), PYR-like (PYL) or regulatory component of ABA receptors (RCAR) proteins. Coupled with ABA, PYR/PYL/RCAR binds to and inhibits protein phosphatase 2Cs (PP2Cs), allowing activation of the sucrose non-fermenting 1-related protein kinase 2 (SnRK2) family members. Upon phosphorylation, downstream targets, such as ion channels, metabolic enzymes and TFs, effectuate robust stress response. Besides, the mitogen-activated protein kinase (MAPK) pathway is also involved in ABA signaling (for review, see Sreenivasulu et al., 2012; Ng et al., 2014; Sah et al., 2016).
Significantly, ABA can also induce organic changes to cope with unfavorable situations. The well-known one is the closure of stomata, minute pores formed by paired guard cells to permit gas exchange, which can reduce water loss from transpiration and thus mitigate dehydration. This movement is achieved by modulating the activities of ion channels and aquaporins. As a result, outflow of K+ and anions drags water out by osmosis, leading to guard cell shrinkage, which is ensured by corresponding rearrangement of actin filaments (Zhao Y. et al., 2011; Zhao et al., 2016). Another special one is the dormancy of seed, which can avoid the existing stresses and await conditions suitable for germination. The viability of stressed seed is largely dependent on their coat properties (Xu et al., 2016; Song et al., 2017b) and cotyledon chlorophyll content (Zhang S.R. et al., 2010; Li X. et al., 2012).
Both endogenous elevation and exogenous addition of ABA are efficient in supporting plants confronting with various stresses. The development of ABA analogs with higher stability is promising in field application. However, overloaded ABA signaling, as in the case of overexpressed NCED or constitutively active PYLs, may also lead to vegetative growth retardation and grain yield reduction (Sreenivasulu et al., 2012; Ng et al., 2014), while foliar spraying of ABA can induce leaf senescence, as observed in rice (Oryza sativa) and maize (Zea mays) (Sah et al., 2016). Therefore, it is important to get better understandings of ABA homeostasis, its extensive biological effects and crosstalk with other pathways for designing strategies that can impart crop stress tolerance at little expense of the economic traits.
Reactive Oxygen Species
In planta, ROS are continuously generated as byproducts of aerobic metabolism in distinct intracellular compartments involving chloroplasts, mitochondria, and peroxisomes. However, as already mentioned, they are not just toxins that need to be removed, but signaling molecules indispensable for diverse physiological processes including stress resistance. Of note, an ROS signal is shaped by multiple factors such as dose, duration, origin, and type (Gechev et al., 2006). Herein, the focus is on H2O2, the relatively stable and less reactive ROS that takes the core node of stress signaling. The extensive crosstalk between H2O2 and other signaling molecules, including ABA, ET, JA, SA, NO, and Ca2+, has been reviewed in (Saxena et al., 2016). Particularly, Ca2+ influx is a notable event in H2O2 signaling, which, in turn, modulates the level of H2O2 via activating the producing (e.g., RBOHs below) or scavenging enzymes. Notably, H2O2 is intrinsically tied to PAs as a product of their degradation (Liu et al., 2007). The more complicated interactions between H2O2 and two gasotransmitters (H2S and NO) will be discussed later on.
In the apoplast, ROS can be initiatively produced upon stress stimuli by various enzymes. The major ones are respiratory burst oxidase homologs (RBOHs), the PM-localized NADPH oxidases that are activated by binding of Ca2+ to the EF-hand motifs in the N-terminal cytosolic region, with the synergy of phosphorylation by, for instance, receptor-like cytoplasmic protein kinases (RLCKs). In Arabidopsis, two RBOH isoforms, RBOHD and RBOHF, are employed to yield ROS against both abiotic and biotic stresses. Particularly, they are essential in ROS-dependent ABA signaling such as stomatal closure and seed dormancy. Their product is O2•-, which then gives rise to H2O2 via both spontaneous and SOD-catalyzed dismutation. The low abundance of apoplastic ASA and GSH, the two major redox buffers, allows propagation of H2O2 to be perceived extracellularly by yet unclear sensors and/or imported via aquaporins (though freely diffusible) into cells to turn on intracellular signaling, thereby launching rapid local or systemic response (see reviews Jalmi and Sinha, 2015; Kimura et al., 2017).
In intracellular signaling of H2O2 originating from metabolic disturbance and/or apoplastic release, the MAPK signalsome plays a central role. Actually, it is an important converging node of stress signaling. Each phosphorylation cascade is constituted by three kinases, MAPK kinase kinase (MAPKKK), MAPK kinase (MAPKK), and MAPK. To date, many MAPKs and different cascades have been identified to differentially decode H2O2 signal, albeit it remains elusive how the specificity is determined. Inversely, the MAPK pathway is also functioning upstream of ROS via modulating, positively or negatively, the activities of RBOHs. In Arabidopsis, two MAPKs, MPK3 and MPK6, are positioned in defensive response, which can ultimately elevate the levels of defensive factors like GSTs and HSPs. H2O2 can prompt them not only via ANP1, a MAPKKK, but via other kinases, including oxidative signal-inducible 1 (OXI1) that is necessary for full activation of MPK3/6, and NUCLEOTIDE DIPHOSPHATE KINASE 2 (NDPK2) that can interact with, and potentiate the activities of, MPK3/6. As expected, NPK1 (tobacco ANP1 ortholog) and AtNDPK2 both granted transgenic plants tolerance to multiple stresses, including salt and extreme temperatures, so did MKK2, a MAPKK in another anti-stress cascade, namely MEKK1-MKK2-MPK4/6 (see reviews Jalmi and Sinha, 2015; Liu and He, 2017).
Besides, H2O2 may also ensure MAPK activation by inhibiting their repressors like protein tyrosine phosphatases (PTPs) via oxidizing the thiol (-SH) group of the cysteine residue (Jalmi and Sinha, 2015). Actually, reversible thiol oxidation may be a direct and important way in conveying H2O2 signal, rendering a large pool of substrates as potential sensors (see reviews Choudhury et al., 2017; Kimura et al., 2017). With the consequently altered functions of diverse effectors, including kinases, phosphatases, TFs, metabolic enzymes, and ion channels, cellular processes are extensively rearranged. Interestingly, thiol modification may also offer a node for signal crosstalk and modulation via raising a competition between H2O2 and other factors, including the two gasotransmitters (see below).
Hydrogen Sulfide and Nitric Oxide
As with H2O2, two toxic gaseous molecules, H2S and NO, at low concentrations, also display impressive powers in safeguarding plants against a broad spectrum of stresses. The two share in common many anti-stress mechanisms. For example, both can alleviate salt toxicity via activating SALT OVERLY SENSITIVE 1 (SOS1), a PM Na+/H+ antiporter, to increase Na+ exclusion (Deng et al., 2016; Kong et al., 2016). The most conspicuous role should be their ability to squash oxidative stress, wherein both of them not only act as antioxidants in their own right but can repress ROS production and activate ROS elimination. Particularly, as a source of sulfur, H2S can be assimilated into GSH, leading to a boost in this essential RS scavenger (see reviews Siddiqui et al., 2011; Hancock and Whiteman, 2014). Not surprisingly, carbon monoxide (CO), the first recognized gasotransmitter, is also an elicitor of stress response, though CO research in this theme is still in its infancy (see review Wang and Liao, 2016).
The three RS, H2O2, H2S, and NO, are usually present together during various stresses and exhibit intricate interactions depending on the context. For example, in stomata regulation, H2O2 and NO synergistically mediate ABA-induced closure, whereas H2S acts as a Janus. With the antagonist face, it can ablate NO accumulation and abet stomata opening (see review Lisjak et al., 2013). However, NO is a mediator of H2S in promoting adventitious root formation (Zhang et al., 2009), which can increase the uptake of O2 and thus attenuate hypoxia stress from waterlogging (Song et al., 2011; Chen et al., 2016). On the contrary, H2S is a mediator of NO in heat tolerance of maize (Li Z.G. et al., 2013) and in cadmium resistance of bermudagrass (Cynodon dactylon) (Shi et al., 2014). Noteworthily, this reverse demonstrates that H2S is not a referee that functions through monitoring ROS and NO, as once proposed (Hancock and Whiteman, 2014), but indeed an active player, albeit the three are not always in the same team. Moreover, with the emergence of another RS player, namely MG, the situation will be further complicated.
Their chemical reactions add one more layer of complexity, which can block each other yet bring about new compounds with potential physiological effects, such as peroxynitrite (ONOO-) formed by NO and O2•-, as well as nitrosothiols formed by NO and H2S. Interestingly, as already mentioned, there is even a competition among them, with the participance of MG and GSH, since all of them can directly modulate protein function via thiol modification, namely, oxidation by H2O2, sulfhydration by H2S, nitrosylation by NO, glycation by MG, and glutathionylation by GSH (Lisjak et al., 2013; Mostofa et al., 2018). Actually, GSH per se offers an additional way for their crosstalk, as it is a derivative of H2S, but a quencher of the other three. Besides, the MAPK pathway is likely to be a convergent point of the four signaling RS.
Being notorious as air pollutants, the two gasotransmitters are actually natural products of botanic metabolism from diverse origins. Enzymatic examples include cysteine desulfhydrases (DES) and sulfite reductase (SIR) for H2S, as well as nitrate reductase (NR) and the nitric oxide synthase (NOS)-like way for NO, though a real NOS has as yet not been identified in planta. With respect to their removal, O-acetylserine(thiol)lyase (OAS-TL) can consume H2S in cysteine production, while being trapped by GSH in the conjugate S-nitrosoglutathione (GSNO) provides a way for NO storage, transport and degradation, which will be deaminated by GSNO reductase (GSNOR) into glutathione disulfide (GSSG) and NH3 (see reviews Calderwood and Kopriva, 2014; Farnese et al., 2016).
For practical use, however, both of them are readily supplied by chemical donors, such as GSNO and sodium nitroprusside (SNP) for NO, as well as sodium hydrosulfide (NaHS) and GYY4137 for H2S. Notably, GYY4137 is a phosphorodithioate derivative that can release H2S slowly and steadily under physiological conditions. A pile of literature has substantially proved that foliar spray of these donors is a highly effective approach to aid plants in combating manifold stresses (see reviews Guo H. et al., 2016; Fancy et al., 2017).
Polyamines
Polyamines are a group of organic compounds with aliphatic nitrogen structure. The diamine putrescine (Put), triamine spermidine (Spd), and tetraamine spermine (Spm) are natural PAs shared by almost all living organisms, which are short-chain polycations derived from arginine/ornithine via clear pathways (see Liu et al., 2007; Gill and Tuteja, 2010). The protective role of PAs in plant response to a wide range of stresses has long been recognized (see reviews Liu et al., 2007; Alcázar et al., 2010; Gill and Tuteja, 2010; Minocha et al., 2014). Indeed, overexpression of every PA biosynthetic enzyme, such as arginine decarboxylase (ADC), spermidine synthase (SPDS) and S-adenosylmethionine synthetase (SAMS), advanced stress tolerance in various plant species, so did exogenous application of PAs (Alcázar et al., 2010; Qi et al., 2010b; Minocha et al., 2014).
A complexity arises in dissecting the mechanisms underlying the anti-stress effects of PAs. It is plausible that these multi-faceted substances contribute to stress defense in diverse ways, owing to their polycationic nature, RS-scavenging property, and signaling function. For example, at the physiological PH, protonated PAs not only participate in ion homeostasis per se, but can bind to negatively charged molecules including membrane lipids and integral proteins, which help mitigate stress-induced membrane damage. In stress signaling, PAs not only communicate with ABA, but can induce rapid production of NO. Peculiarly, PAs are affiliated with other metabolites involved in stress response, including Pro and ET interconnected with PA anabolism, as well as H2O2 and GABA generated from PA catabolism (see Alcázar et al., 2010; Minocha et al., 2014). Of note, due to the special link with H2O2, PAs also fall into the Janus category (see review Gupta et al., 2016), which need to be taken into consideration in their practical application.
Phytochromes
PHYB is emerging as a negative regulator in stress tolerance, which belongs to a small family of chromophore-containing proteins that serve as photoreceptors to perceive red (R) and far-red (FR) light. The signaling activity of PHYB is subjected to reversible photoconversion composed of R activation and FR deactivation based on conformational change. Nascent PHYB is in the inactive Pr (R-absorbing) form. Once converted to the bioactive Pfr (FR-absorbing) form, dimeric PHYB will translocate into the nucleus, where it can interact with, and trigger the proteasomal degradation of, phytochrome interacting factors (PIFs), a subfamily of basic helix-loop-helix (bHLH) TFs, so as to remodel the expression profile of thousands of light-responsive genes, thereby guiding photomorphogenesis (Franklin and Quail, 2010; Zhou et al., 2014).
More recently, PHYB was identified to be a thermosensor as well (Jung et al., 2016; Legris et al., 2016). Warm ambient temperatures can effectively induce elongation growth, which phenocopies shade avoidance controlled by the PHY-PIF cascade. Indeed, Pfr can also revert to Pr in a spontaneous way called thermal (or dark) reversion, which is independent of light but sensitive to temperature. Therefore, warm temperatures, particularly during night, can relieve the repression of PIF4 via quickly deactivating PHYB, together with enhancing the transcription of PIF4, thereby driving thermomorphogenesis. As active PHYB was found to interact with PIF-binding sites (G-boxes) at PIF4-targeted promoters, it was proposed to have an additional inhibitory role as a co-repressor or competitor of PIF4 in gene regulation (see review Delker et al., 2017). Another cascade downstream of PHYB in light- and temperature-induced growth involves the RING E3 ligase CONSTITUTIVE PHOTOMORPHOGENIC 1 (COP1) and the TF ELONGATED HYPOCOTYL 5 (HY5), with COP1 ubiquitinating HY5 for degradation to derepress the growth genes. Notably, COP1 can indirectly potentiate the activity of PIF4, thereby connecting the two branches (see review Legris et al., 2017).
Loss-of-function of PHYB upon mutation or low R/FR ratio treatment can ameliorate cold tolerance in that active PHYB indirectly represses the expression of TFs belonging to the C-REPEAT BINDING FACTOR/DEHYDRATION RESPONSIVE ELEMENT BINDING FACTOR 1 (CBF/DREB1) family, which play a central role in cold acclimation via activating downstream targets such as the COR genes (Franklin and Whitelam, 2007; He et al., 2016; Wang F. et al., 2016). The direct preys of PHYB are activators of the CBF/DREB1 regulon, such as the ABA-dependent JA signaling in tomato (Wang F. et al., 2016) and the PIF-like protein OsPIL16 in rice (He et al., 2016). Notably, in rice phyB mutant, UFA content is much higher than in wild type (WT), leading to better chloroplast structure and less photoinhibition under chilling stress (Yang et al., 2013). Interestingly, stronger heat tolerance has been observed in Arabidopsis phyB mutant, with the HS damper of lateral root development being relieved (Song et al., 2017a). This might be related to the reduction of HY5, which is a negative regulator of the unfolded protein response (UPR) that can be triggered by HS (Nawkar et al., 2017).
Rice phyB mutant also exhibits better drought tolerance owing to decreased transpiration rate involving two morphological changes. One is reduced total leaf area per plant, which is probably due to inhibited leaf cell proliferation. The other is reduced stomatal density, which results from enhanced epidermal cell expansion by activation of putative ERECTA family and EXPANSIN family genes (Liu et al., 2012). However, in contrast to the observations in Arabidopsis (Boccalandro et al., 2009; Casson et al., 2009), stomatal development is not affected in rice by phyB deficiency, albeit reduced stomatal density is the common outcome. Besides, PHYB is the mediator of R light-induced stomatal opening (Wang et al., 2010), which might be related to its repression effect on PIFs as well, as maize ZmPIF1 was found to contribute in ABA-induced stomatal closure (Gao et al., 2018). Interestingly, it was later noted in rice phyB mutant that the expression levels and enzymatic activities of APX and CAT were significantly higher than those in WT when deprived of water, reflecting a negative role of PHYB in ROS scavenging (Yoo et al., 2017).
Similarly, antioxidant enzymes were more active in tobacco phyB mutant, which was newly reported to be more tolerant to salt (Yang et al., 2018). NtPHYB could suppress the biosynthesis of ABA and JA via targeting the enzyme genes, as has been suggested for aforementioned tomato SlPHYB (Wang F. et al., 2016). Inversely, ABA cooperated with JA to inhibit the expression of NtPHYB, in consistence with another new finding that ABA significantly reduced the transcription of OsPHYB for drought escape (Du et al., 2018). It seems that such mutual regulation is common to various stress responses. Moreover, PHYA, the only light-labile family member, antagonized PHYB in regulating chilling signaling of tomato (Wang F. et al., 2016); however, it synergized, and even surpassed, PHYB in attenuating salinity response of tobacco (Yang et al., 2018).
Calcium Ion
Ca2+, a versatile secondary messenger, is involved in plant responses to virtually all abiotic stresses, directly or indirectly via other signaling molecules, serving as a key integration node of the regulatory network. Upon stimulation, Ca2+ is mobilized from the reservoirs, apoplast and vacuole in particular. The signaling is ignited by sharp influx through Ca2+ channels, such as the PM cyclic nucleotide-gated channels (CNGCs) and the tonoplast TWO PORE CHANNEL 1 (TPC1) (Lu et al., 2016; Zheng et al., 2017), and soon quenched by efflux via Ca2+-ATPases and Ca2+/H+ exchangers (Han et al., 2011, 2012). Notably, the latter, as well as other secondary transporters, are energized by virtue of the proton gradient established by PM H+-ATPase, vacuolar H+-ATPase (V-ATPase) and vacuolar H+-translocating inorganic pyrophosphatase (V-PPase). These H+ pumps are thus pivotal in ionic and osmotic homeostasis, thereby contributing to salt tolerance in particular (Chen M. et al., 2010; Yang et al., 2010; Yuan et al., 2016b).
Influent Ca2+ is perceived by various Ca2+ sensors, including calmodulins (CaMs), calcineurin B-like proteins (CBLs), and Ca2+-dependent protein kinases (CDPKs) that contain EF-hand Ca2+-binding motifs. Unlike CDPKs that are immediate effectors, CaMs and CBLs usually need to evoke downstream effectors. CaMs can interact with a large variety of targets, such as kinases, phosphatases and TFs, whereas CBLs primarily bind to CBL-interacting protein kinases (CIPKs). With gene expression and protein/enzyme activities being altered by different effectors, positively or negatively, Ca2+ signatures are deciphered into specific cellular responses. Besides ABA biosynthetic enzymes, a good example is glutamate decarboxylase (GAD), which is rapidly activated by binding of Ca2+-CaM to convert L-glutamate into GABA, leading to accumulation of this multifunctional anti-stress molecule (for review, see Luan et al., 2002; Shi S. et al., 2018). NO production can also be promoted by CaMs, but via inhibiting the degradation enzyme GSNOR under saline conditions (Zhou S. et al., 2016).
Moreover, as a cation antagonistic to Na+, Ca2+ is intrinsically implicated in salt detoxification with modulating ion transporters being an important means. To maintain a properly high K+/Na+ ratio in the cytosol that is critical in salt tolerance (Chen et al., 2005; Feng et al., 2015), Na+ scarcity is accomplished by activating the Na+/H+ antiporter SOS1 via the SOS3-SOS2 (CBL4-CIPK24) cascade to increase Na+ efflux, together with blocking non-selective cation channels (NSCCs) to decrease Na+ influx (Luan et al., 2002; Ding et al., 2010). Meanwhile, K+ equilibrium is monitored by CBLs through adjusting, directly or indirectly, the activity of the inward rectifier ARABIDOPSIS K+ TRANSPORTER 1 (AKT1) (Ren et al., 2013). Furthermore, Ca2+ itself is necessary for stabilizing cell wall and membranes (Shi S. et al., 2018).
It is thus not surprising that overexpression of CaMs (Zhou S. et al., 2016), CBLs (Cheong et al., 2003; Cheong et al., 2010), CIPKs (Pan et al., 2018), or CDPKs (Xu et al., 2010; Asano et al., 2012), even exogenous supplement of CaCl2 (Ahmad et al., 2018), all enabled plants to bear salt toxicity. Remarkably, transgenic rice harboring CIPKs from wild barley (Hordeum spontaneum) displayed enhanced tolerance to drought and heavy metals as well. Besides, some HsCIPKs were also subject to induction by cold, heat and ABA (Pan et al., 2018). However, the behavior of Arabidopsis cbl1 mutant upon cold exposure was really inconsequent, with higher (Cheong et al., 2003), similar (Albrecht et al., 2003), and lower (Huang et al., 2011) tolerance all being reported. Taking into account that plants were correspondingly cultured under light, dark, and 16 h light/8 h dark during the treatment, as well as the opposite roles of PHYA and PHYB in cold response, one possibility is that CBL1 might be involved in signaling of both phytochromes, with different CIPKs being evoked.
Gene Regulation Factors
Once the transduced stress cue is received, gene regulation factors active at different levels, including histone acetyltransferases (HATs) (Stockinger et al., 2001), TFs, alternative splicing factors (Laloum et al., 2018), microRNAs (Zhang et al., 2013), and ubiquitination enzymes (Lee and Suh, 2015) are engaged in fine-tuning the defense systems. Transcriptional level is still the key regulatory node. Scores of TFs have been identified to be stress-responsive, such as ABA-responsive element (ABRE) binding proteins/factors (AREBs/ABFs) (Sah et al., 2016), DELLAs (An et al., 2015), NACs (Tang et al., 2017), WRKYs (Bai et al., 2018), zinc finger proteins (Han et al., 2014; Wang K. et al., 2018), and the APETALA2/ETHYLENE RESPONSE FACTOR (AP2/ERF) superfamily, to which the aforementioned CBF/DREB1 family belongs (Mizoi et al., 2012).
Notably, heat shock factors (HSFs) not only serve as the master regulator of HSPs, but can monitor their own members and many other defense factors, including APX and GST that eliminate ROS, as well as GOLS essential for raffinose synthesis, via binding upon oligomerization to the heat shock elements (HSEs) located in their promoter regions. Therefore, HSFs are actually capable of launching three general defensive systems. Strikingly, the number of HSFs in plants is large and highly variable, 16 in peanut (A. duranensis) vs. 52 in soybean (Glycine max) for example (Scharf et al., 2012; Wang P.F. et al., 2017), not to mention their functional diversification. Such multiplicity perplexes their study and application. Besides, negative effects have been observed in ectopic expression of HSFs. A case is that tomato SlHSFA3 increased seed germination sensitivity to salt in Arabidopsis (Li Z. et al., 2013). Nonetheless, genetic manipulation of HSFs is still a promising avenue to confer plants multiplex tolerance (for review, see Scharf et al., 2012; Guo M. et al., 2016). Moreover, it is worth mentioning that HSFA6b and HSFA3 enable ABA to be a bona fide participant of HS response. The former is directly activated by AREB1, while the latter is downstream of DREB2A, a target shared by AREB1 and HSFA6b (Huang et al., 2016).
DREB2C, another class 2 DREB member, is an activator of HSFA3 as well (Chen H. et al., 2010; Hwang et al., 2012). It can also target potential chaperones like COR15A and DESICCATION-RESPONSIVE PROTEIN 29A (RD29A) in mitigating salt toxicity (Song et al., 2014). Interestingly, DREB2C from Ammopiptanthus mongolicus, a evergreen broadleaf shrub living in desert, was newly reported to up-regulate not only the three factors, but Δ1-pyrroline-5-carboxylate synthetase (P5CS) that initiates Pro biosynthesis, as well as FADs that catalyze 18:3 production, thereby promoting Arabidopsis endurance to drought, freezing and heat (Yin et al., 2018). A safe conclusion can then be drawn that this single TF governs all of the four cellular general defenses. Besides, computational analysis conducted on Arabidopsis DREB2C promoter has identified diverse types of cis-acting elements, which are responsive to ABA (ABRE), MeJA, SA (TCA), heat (HSE), low temperature (LTR), and stress (TC rich), respectively (Sazegari et al., 2015), suggesting that this TF is a core converging point in stress signaling. Indeed, it is an ABA-inducible TF and can exert a positive feedback on ABA biosynthesis via trans-activating NCED9 to delay seed germination (Je et al., 2014).
To finalize, light is shed back on transcriptional regulation of cuticle biosynthesis. In Arabidopsis, the enzyme genes are controlled primarily by TFs of two families, including SHINE 1 (SHN1), -2, -3, and DEWAX of the AP2/ERF superfamily, as well as MYB16, MYB30 and MYB106 of the R2R3-MYB family. Interestingly, MYB96 is an activator of wax production, whereas MYB41 is a repressor of cutin synthesis (see reviews Borisjuk et al., 2014; Lee and Suh, 2015). Notably, MYB96 is a prominent implementer of ABA signaling, with the whole wax metabolism being put under control. Not only elongation and modification enzymes, but ABC transporters and nsLTPs have at least one isoform gene targeted, directly or indirectly (Seo et al., 2011). MYB96 transgenesis upgraded drought and freezing tolerance of Arabidopsis; however, significant dwarfism was a concomitant (Seo et al., 2009; Guo et al., 2013). By contrast, wax production 1 (WXP1), an ERF member from Medicago truncatula, might be a better candidate, which was the one accounting for the previously mentioned observation that higher contents of both n-alkane and primary alcohols resulted in better viability under drought and freezing without disturbing the growth of transgenic Arabidopsis (Zhang et al., 2007).
Conclusion and Perspectives
As one of the successful habitants thriving on the earth, there is no great surprise that plants have found smart ways to deal with abiotic stresses. The existence of general defense systems raises the feasibility to endow crops and other plants with multistress tolerance in a simplified way, albeit there are still many gaps need to be filled in before their field application. To avoid the pleiotropic effects from over-activation, as observed for ABA and HSFs, synthetic stress-inducible promoters (Hou et al., 2012) could be a method of choice. It is worth trying to find an optimal cocktail of the defensive molecules that can balance each other to minimize undesired effects. Of note, NO may be able to antagonize ABA in leaf senescence (Kong et al., 2016). Screening for mutants, generated from gamma irradiation (Yuan et al., 2015a) for instance, that are stress-resilient but overcome side effects is pretty sound. Moreover, defense genes from stress-resistant species that might have acquired adaptive function, as in the case of GPAT, are good candidates for transgenesis.
With the help of high-throughput techniques and bioinformatic platforms, we will be able to learn more from the natural existing extremophiles like Thellungiella, and acquire more comprehensive and in-depth understandings of the stress responses of different crops. It would be more informative to challenge them with a combination of stresses that mimics to some extent the field conditions. Interestingly, the earth has actually been offering silicon (Si) as “compensation”. This abundant element in the crust plays significant roles in the easing of both abiotic and biotic stresses, though the mechanisms are under debating (see reviews Debona et al., 2017; Coskun et al., 2018). With a better knowledge of Si utilization, benefits from the addition of Si in fertilizers can be envisaged. Furthermore, plant species that have the capacity to deprive soils of salt and heavy metals, such as S. salsa (Song and Wang, 2015), are highly instrumental in the restoration of arable land for sustainable agriculture.
Author Contributions
All authors listed have made a substantial, direct and intellectual contribution to the work, and approved it for publication.
Funding
This work was supported by grants from the National Natural Science Foundation of China (31771659).
Conflict of Interest Statement
The authors declare that the research was conducted in the absence of any commercial or financial relationships that could be construed as a potential conflict of interest.
References
Ahmad, P., Abd Allah, E. F., Alyemeni, M. N., Wijaya, L., Alam, P., Bhardwaj, R., et al. (2018). Exogenous application of calcium to 24-epibrassinosteroid pre-treated tomato seedlings mitigates NaCl toxicity by modifying ascorbate-glutathione cycle and secondary metabolites. Sci. Rep. 8:13515. doi: 10.1038/s41598-018-31917-1
Albrecht, V., Weinl, S., Blazevic, D., D’Angelo, C., Batistic, O., Kolukisaoglu, U., et al. (2003). The calcium sensor CBL1 integrates plant responses to abiotic stresses. Plant J. 36, 457–470. doi: 10.1046/j.1365-313X.2003.01892.x
Alcázar, R., Altabella, T., Marco, F., Bortolotti, C., Reymond, M., Koncz, C., et al. (2010). Polyamines: molecules with regulatory functions in plant abiotic stress tolerance. Planta 231, 1237–1249. doi: 10.1007/s00425-010-1130-0
Amtmann, A. (2009). Learning from evolution: Thellungiella generates new knowledge on essential and critical components of abiotic stress tolerance in plants. Mol. Plant 2, 3–12. doi: 10.1093/mp/ssn094
An, J., Hou, L., Li, C., Wang, C. X., Xia, H., Zhao, C. Z., et al. (2015). Cloning and expression analysis of four DELLA genes in peanut. Russ. J. Plant Physiol. 62, 116–126. doi: 10.1134/s1021443715010021
Arnao, M. B., and Hernández-Ruiz, J. (2018). Melatonin and its relationship to plant hormones. Ann. Bot. 121, 195–207. doi: 10.1093/aob/mcx114
Asano, T., Hayashi, N., Kobayashi, M., Aoki, N., Miyao, A., Mitsuhara, I., et al. (2012). A rice calcium-dependent protein kinase OsCPK12 oppositely modulates salt-stress tolerance and blast disease resistance. Plant J. 69, 26–36. doi: 10.1111/j.1365-313X.2011.04766.x
Ashraf, M., and Foolad, M. R. (2007). Roles of glycine betaine and proline in improving plant abiotic stress resistance. Environ. Exp. Bot. 59, 206–216. doi: 10.1016/j.envexpbot.2005.12.006
Ashraf, M. F., Yang, S., Ruijie, W., Yuzhu, W., Hussain, A., Noman, A., et al. (2018). Capsicum annuum HsfB2a positively regulates the response to Ralstonia solanacearum infection or high temperature and high humidity forming transcriptional cascade with CaWRKY6 and CaWRKY40. Plant Cell Physiol. doi: 10.1093/pcp/pcy181 [Epub ahead of print].
Bai, Y., Sunarti, S., Kissoudis, C., Visser, R. G. F., and van der Linden, C. G. (2018). The role of tomato WRKY genes in plant responses to combined abiotic and biotic stresses. Front. Plant Sci. 9:801. doi: 10.3389/fpls.2018.00801
Beisson, F., Li-Beisson, Y., and Pollard, M. (2012). Solving the puzzles of cutin and suberin polymer biosynthesis. Curr. Opin. Plant Biol. 15, 329–337. doi: 10.1016/j.pbi.2012.03.003
Bernard, A., and Joubès, J. (2013). Arabidopsis cuticular waxes: advances in synthesis, export and regulation. Prog. Lipid Res. 52, 110–129. doi: 10.1016/j.plipres.2012.10.002
Boccalandro, H. E., Rugnone, M. L., Moreno, J. E., Ploschuk, E. L., Serna, L., Yanovsky, M. J., et al. (2009). Phytochrome B enhances photosynthesis at the expense of water-use efficiency in Arabidopsis. Plant Physiol. 150, 1083–1092. doi: 10.1104/pp.109.135509
Borisjuk, N., Hrmova, M., and Lopato, S. (2014). Transcriptional regulation of cuticle biosynthesis. Biotechnol. Adv. 32, 526–540. doi: 10.1016/j.biotechadv.2014.01.005
Bouché, N., and Fromm, H. (2004). GABA in plants: just a metabolite? Trends Plant Sci. 9, 110–115. doi: 10.1016/j.tplants.2004.01.006
Bourdenx, B., Bernard, A., Domergue, F., Pascal, S., Léger, A., Roby, D., et al. (2011). Overexpression of Arabidopsis ECERIFERUM1 promotes wax very-long-chain alkane biosynthesis and influences plant response to biotic and abiotic stresses. Plant Physiol. 156, 29–45. doi: 10.1104/pp.111.172320
Calderwood, A., and Kopriva, S. (2014). Hydrogen sulfide in plants: from dissipation of excess sulfur to signaling molecule. Nitric Oxide 41, 72–78. doi: 10.1016/j.niox.2014.02.005
Cao, S., Du, X. H., Li, L. H., Liu, Y. D., Zhang, L., Pan, X., et al. (2017). Overexpression of Populus tomentosa cytosolic ascorbate peroxidase enhances abiotic stress tolerance in tobacco plants. Russ. J. Plant Physiol. 64, 224–234. doi: 10.1134/s1021443717020029
Casson, S. A., Franklin, K. A., Gray, J. E., Grierson, C. S., Whitelam, G. C., and Hetherington, A. M. (2009). Phytochrome B and PIF4 regulate stomatal development in response to light quantity. Curr. Biol. 19, 229–234. doi: 10.1016/j.cub.2008.12.046
Chai, G., Li, C., Xu, F., Li, Y., Shi, X., Wang, Y., et al. (2018). Three endoplasmic reticulum-associated fatty acyl-coenzyme a reductases were involved in the production of primary alcohols in hexaploid wheat (Triticum aestivum L.). BMC Plant Biol. 18:41. doi: 10.1186/s12870-018-1256-y
Chen, H., Hwang, J. E., Lim, C. J., Kim, D. Y., Lee, S. Y., and Lim, C. O. (2010). Arabidopsis DREB2C functions as a transcriptional activator of HsfA3 during the heat stress response. Biochem. Biophys. Res. Commun. 401, 238–244. doi: 10.1016/j.bbrc.2010.09.038
Chen, M., Song, J., and Wang, B. S. (2010). NaCl increases the activity of the plasma membrane H+-ATPase in C3 halophyte Suaeda salsa callus. Acta Physiol. Plant. 32, 27–36. doi: 10.1007/s11738-009-0371-7
Chen, M., Zhang, W. H., Lv, Z. W., Zhang, S. L., Hidema, J., Shi, F. M., et al. (2013). Abscisic acid is involved in the response of Arabidopsis mutant sad2-1 to ultraviolet-B radiation by enhancing antioxidant enzymes. S. Afr. J. Bot. 85, 79–86. doi: 10.1016/j.sajb.2012.11.006
Chen, T. S., Yuan, F., Song, J., and Wang, B. S. (2016). Nitric oxide participates in waterlogging tolerance through enhanced adventitious root formation in the euhalophyte Suaeda salsa. Funct. Plant Biol. 43, 244–253. doi: 10.1071/fp15120
Chen, Y., Chi, Y., Meng, Q., Wang, X., and Yu, D. (2018). GmSK1, an SKP1 homologue in soybean, is involved in the tolerance to salt and drought. Plant Physiol. Biochem. 127, 25–31. doi: 10.1016/j.plaphy.2018.03.007
Chen, Z., Newman, I., Zhou, M., Mendham, N., Zhang, G., and Shabala, S. (2005). Screening plants for salt tolerance by measuring K+ flux: a case study for barley. Plant Cell Environ. 28, 1230–1246. doi: 10.1111/j.1365-3040.2005.01364.x
Cheng, S., Yang, Z., Wang, M. J., Song, J., Sui, N., and Fan, H. (2014). Salinity improves chilling resistance in Suaeda salsa. Acta Physiol. Plant. 36, 1823–1830. doi: 10.1007/s11738-014-1555-3
Cheong, Y. H., Kim, K. N., Pandey, G. K., Gupta, R., Grant, J. J., and Luan, S. (2003). CBL1, a calcium sensor that differentially regulates salt, drought, and cold responses in Arabidopsis. Plant Cell 15, 1833–1845. doi: 10.1105/tpc.012393
Cheong, Y. H., Sung, S. J., Kim, B. G., Pandey, G. K., Cho, J. S., Kim, K. N., et al. (2010). Constitutive overexpression of the calcium sensor CBL5 confers osmotic or drought stress tolerance in Arabidopsis. Mol. Cells 29, 159–165. doi: 10.1007/s10059-010-0025-z
Choudhury, F. K., Rivero, R. M., Blumwald, E., and Mittler, R. (2017). Reactive oxygen species, abiotic stress and stress combination. Plant J. 90, 856–867. doi: 10.1111/tpj.13299
Conconi, A., Smerdon, M. J., Howe, G. A., and Ryan, C. A. (2006). The octadecanoid signalling pathway in plants mediates a response to ultraviolet radiation. Nature 383, 826–829. doi: 10.1038/383826a0
Coskun, D., Deshmukh, R., Sonah, H., Menzies, J. G., Reynolds, O., Ma, J. F., et al. (2018). The controversies of silicon’s role in plant biology. New Phytol. doi: 10.1111/nph.15343 [Epub ahead of print].
Debona, D., Rodrigues, F. A., and Datnoff, L. E. (2017). Silicon’s role in abiotic and biotic plant stresses. Annu. Rev. Phytopathol. 55, 85–107. doi: 10.1146/annurev-phyto-080516-035312
Debono, A., Yeats, T. H., Rose, J. K., Bird, D., Jetter, R., Kunst, L., et al. (2009). Arabidopsis LTPG is a glycosylphosphatidylinositol-anchored lipid transfer protein required for export of lipids to the plant surface. Plant Cell 21, 1230–1238. doi: 10.1105/tpc.108.064451
Delker, C., van Zanten, M., and Quint, M. (2017). Thermosensing enlightened. Trends Plant Sci. 22, 185–187. doi: 10.1016/j.tplants.2017.01.007
Deng, Y., Xu, L., Zeng, X., Li, Z., Qin, B., and He, N. (2010). New perspective of GABA as an inhibitor of formation of advanced lipoxidation end-products: it’s interaction with malondiadehyde. J. Biomed. Nanotechnol. 6, 318–324. doi: 10.1166/jbn.2010.1130
Deng, Y. Q., Bao, J., Yuan, F., Liang, X., Feng, Z. T., and Wang, B. S. (2016). Exogenous hydrogen sulfide alleviates salt stress in wheat seedlings by decreasing Na+ content. Plant Growth Regul. 79, 391–399. doi: 10.1007/s10725-015-0143-x
Deng, Y. Q., Feng, Z. T., Yuan, F., Guo, J. R., Suo, S. S., and Wang, B. S. (2015). Identification and functional analysis of the autofluorescent substance in Limonium bicolor salt glands. Plant Physiol. Biochem. 97, 20–27. doi: 10.1016/j.plaphy.2015.09.007
Ding, C. K., Wang, C. Y., Gross, K. C., and Smith, D. L. (2002). Jasmonate and salicylate induce the expression of pathogenesis-related-protein genes and increase resistance to chilling injury in tomato fruit. Planta 214, 895–901. doi: 10.1007/s00425-001-0698-9
Ding, F., Chen, M., Sui, N., and Wang, B. S. (2010). Ca2+ significantly enhanced development and salt-secretion rate of salt glands of Limonium bicolor under NaCl treatment. S. Afr. J. Bot. 76, 95–101. doi: 10.1016/j.sajb.2009.09.001
Du, H., Huang, F., Wu, N., Li, X., Hu, H., and Xiong, L. (2018). Integrative regulation of drought escape through ABA-dependent and -independent pathways in rice. Mol. Plant 11, 584–597. doi: 10.1016/j.molp.2018.01.004
Fancy, N. N., Bahlmann, A. K., and Loake, G. J. (2017). Nitric oxide function in plant abiotic stress. Plant Cell Environ. 40, 462–472. doi: 10.1111/pce.12707
Farmer, E. E., and Davoine, C. (2007). Reactive electrophile species. Curr. Opin. Plant Biol. 10, 380–386. doi: 10.1016/j.pbi.2007.04.019
Farnese, F. S., Menezes-Silva, P. E., Gusman, G. S., and Oliveira, J. A. (2016). When bad guys become good ones: the key role of reactive oxygen species and nitric oxide in the plant responses to abiotic stress. Front. Plant Sci. 7:471. doi: 10.3389/fpls.2016.00471
Feng, Z. T., Deng, Y. Q., Fan, H., Sun, Q. J., Sui, N., and Wang, B. S. (2014a). Effects of NaCl stress on the growth and photosynthetic characteristics of Ulmus pumila L. seedlings in sand culture. Photosynthetica 52, 313–320. doi: 10.1007/s11099-014-0032-y
Feng, Z. T., Sun, Q. J., Deng, Y. Q., Sun, S. F., Zhang, J. G., and Wang, B. S. (2014b). Study on pathway and characteristics of ion secretion of salt glands of Limonium bicolor. Acta Physiol. Plant. 36, 2729–2741. doi: 10.1007/s11738-014-1644-3
Feng, Z. T., Deng, Y. Q., Zhang, S. C., Liang, X., Yuan, F., Hao, J. L., et al. (2015). K+ accumulation in the cytoplasm and nucleus of the salt gland cells of Limonium bicolor accompanies increased rates of salt secretion under NaCl treatment using NanoSIMS. Plant Sci. 238, 286–296. doi: 10.1016/j.plantsci.2015.06.021
Fernandes, J. C., Goulao, L. F., and Amâncio, S. (2016). Regulation of cell wall remodeling in grapevine (Vitis vinifera L.) callus under individual mineral stress deficiency. J. Plant Physiol. 190, 95–105. doi: 10.1016/j.jplph.2015.10.007
Fich, E. A., Segerson, N. A., and Rose, J. K. (2016). The plant polyester cutin: biosynthesis, structure, and biological roles. Annu. Rev. Plant Biol. 67, 207–233. doi: 10.1146/annurev-arplant-043015-111929
Flowers, T. J., and Colmer, T. D. (2008). Salinity tolerance in halophytes. New Phytol. 179, 945–963. doi: 10.1111/j.1469-8137.2008.02531.x
Franklin, K. A., and Quail, P. H. (2010). Phytochrome functions in Arabidopsis development. J. Exp. Bot. 61, 11–24. doi: 10.1093/jxb/erp304
Franklin, K. A., and Whitelam, G. C. (2007). Light-quality regulation of freezing tolerance in Arabidopsis thaliana. Nat. Genet. 39, 1410–1413. doi: 10.1038/ng.2007.3
Fu, C., Zhang, J. X., Liu, X. X., Yang, W. W., Yu, H. B., and Liu, J. (2015). AtFes1A is essential for highly efficient molecular chaperone function in Arabidopsis. J. Plant Biol. 58, 366–373. doi: 10.1007/s12374-015-0181-y
Gao, Y., Wu, M., Zhang, M., Jiang, W., Ren, X., Liang, E., et al. (2018). A maize phytochrome-interacting factors protein ZmPIF1 enhances drought tolerance by inducing stomatal closure and improves grain yield in Oryza sativa. Plant Biotechnol. J. 16, 1375–1387. doi: 10.1111/pbi.12878
Gechev, T. S., Van Breusegem, F., Stone, J. M., Denev, I., and Laloi, C. (2006). Reactive oxygen species as signals that modulate plant stress responses and programmed cell death. BioEssays 28, 1091–1101. doi: 10.1002/bies.20493
Gill, S. S., and Tuteja, N. (2010). Polyamines and abiotic stress tolerance in plants. Plant Signal. Behav. 5, 26–33. doi: 10.4161/psb.5.1.10291
Girard, A. L., Mounet, F., Lemaire-Chamley, M., Gaillard, C., Elmorjani, K., Vivancos, J., et al. (2012). Tomato GDSL1 is required for cutin deposition in the fruit cuticle. Plant Cell 24, 3119–3134. doi: 10.1105/tpc.112.101055
González-Aguilar, G. A., Fortiz, J., Cruz, R., Baez, R., and Wang, C. Y. (2000). Methyl jasmonate reduces chilling injury and maintains postharvest quality of mango fruit. J. Agric. Food Chem. 48, 515–519. doi: 10.1021/jf9902806
Guo, H., Xiao, T., Zhou, H., Xie, Y., and Shen, W. (2016). Hydrogen sulfide: a versatile regulator of environmental stress in plants. Acta Physiol. Plant 38:16. doi: 10.1007/s11738-015-2038-x
Guo, M., Liu, J. H., Ma, X., Luo, D. X., Gong, Z. H., and Lu, M. H. (2016). The plant heat stress transcription factors (HSFs): structure, regulation, and function in response to abiotic stresses. Front. Plant Sci. 7:114. doi: 10.3389/fpls.2016.00114
Guo, J. R., Li, Y. D., Han, G. L., Song, J., and Wang, B. S. (2018). NaCl markedly improved the reproductive capacity of the euhalophyte Suaeda salsa. Funct. Plant Biol. 45, 350–361. doi: 10.1071/fp17181
Guo, J. R., Suo, S. S., and Wang, B. S. (2015). Sodium chloride improves seed vigour of the euhalophyte Suaeda salsa. Seed Sci. Res. 25, 335–344. doi: 10.1017/s0960258515000239
Guo, L., Yang, H., Zhang, X., and Yang, S. (2013). Lipid transfer protein 3 as a target of MYB96 mediates freezing and drought stress in Arabidopsis. J. Exp. Bot. 64, 1755–1767. doi: 10.1093/jxb/ert040
Guo, Y. H., Jia, W. J., Song, J., Wang, D. A., Chen, M., and Wang, B. S. (2012). Thellungilla halophila is more adaptive to salinity than Arabidopsis thaliana at stages of seed germination and seedling establishment. Acta Physiol. Plant. 34, 1287–1294. doi: 10.1007/s11738-012-0925-y
Gupta, K., Sengupta, A., Chakraborty, M., and Gupta, B. (2016). Hydrogen peroxide and polyamines act as double edged swords in plant abiotic stress responses. Front. Plant Sci. 7:1343. doi: 10.3389/fpls.2016.01343
Han, G. L., Wang, M. J., Yuan, F., Sui, N., Song, J., and Wang, B. S. (2014). The CCCH zinc finger protein gene AtZFP1 improves salt resistance in Arabidopsis thaliana. Plant Mol. Biol. 86, 237–253. doi: 10.1007/s11103-014-0226-5
Han, N., Lan, W. J., He, X., Shao, Q., Wang, B. S., and Zhao, X. J. (2012). Expression of a Suaeda salsa vacuolar H+/Ca2+ transporter gene in Arabidopsis contributes to physiological changes in salinity. Plant Mol. Biol. Rep. 30, 470–477. doi: 10.1007/s11105-011-0353-y
Han, N., Shao, Q., Bao, H. Y., and Wang, B. S. (2011). Cloning and characterization of a Ca2+/H+ antiporter from halophyte Suaeda salsa L. Plant Mol. Biol. Rep. 29, 449–457. doi: 10.1007/s11105-010-0244-7
Hancock, J. T., and Whiteman, M. (2014). Hydrogen sulfide and cell signaling: team player or referee? Plant Physiol. Biochem. 78, 37–42. doi: 10.1016/j.plaphy.2014.02.012
Hasanuzzaman, M., Nahar, K., Hossain, M. S., Mahmud, J. A., Rahman, A., Inafuku, M., et al. (2017). Coordinated actions of glyoxalase and antioxidant defense systems in conferring abiotic stress tolerance in plants. Int. J. Mol. Sci. 18:200. doi: 10.3390/ijms18010200
Hazel, J. R. (1995). Thermal adaptation in biological membranes: is homeoviscous adaptation the explanation? Annu. Rev. Physiol. 57, 19–42. doi: 10.1146/annurev.ph.57.030195.000315
He, Y. A., Li, Y. P., Cui, L. X., Xie, L. X., Zheng, C. K., Zhou, G. H., et al. (2016). Phytochrome B negatively affects cold tolerance by regulating OsDREB1 gene expression through phytochrome interacting factor-like protein OsPIL16 in rice. Front. Plant Sci. 7:1963. doi: 10.3389/fpls.2016.01963
Hooker, T. S., Millar, A. A., and Kunst, L. (2002). Significance of the expression of the CER6 condensing enzyme for cuticular wax production in Arabidopsis. Plant Physiol. 129, 1568–1580. doi: 10.1104/pp.003707
Hoque, T. S., Hossain, M. A., Mostofa, M. G., Burritt, D. J., Fujita, M., and Tran, L. S. (2016). Methylglyoxal: an emerging signaling molecule in plant abiotic stress responses and tolerance. Front. Plant Sci. 7:1341. doi: 10.3389/fpls.2016.01341
Hou, L., Chen, L. J., Wang, J. Y., Xu, D. F., Dai, L. X., Zhang, H., et al. (2012). Construction of stress responsive synthetic promoters and analysis of their activity in transgenic Arabidopsis thaliana. Plant Mol. Biol. Rep. 30, 1496–1506. doi: 10.1007/s11105-012-0464-0
Huang, C., Ding, S., Zhang, H., Du, H., and An, L. (2011). CIPK7 is involved in cold response by interacting with CBL1 in Arabidopsis thaliana. Plant Sci. 181, 57–64. doi: 10.1016/j.plantsci.2011.03.011
Huang, L. J., Cheng, G. X., Khan, A., Wei, A. M., Yu, Q. H., Yang, S. B., et al. (2018). CaHSP16.4, a small heat shock protein gene in pepper, is involved in heat and drought tolerance. Protoplasma doi: 10.1007/s00709-018-1280-7 [Epub ahead of print].
Huang, X., Zhang, X., Gong, Z., Yang, S., and Shi, Y. (2017). ABI4 represses the expression of type-A ARRs to inhibit seed germination in Arabidopsis. Plant J. 89, 354–365. doi: 10.1111/tpj.13389
Huang, Y. C., Niu, C. Y., Yang, C. R., and Jinn, T. L. (2016). The heat stress factor HSFA6b connects ABA signaling and ABA-mediated heat responses. Plant Physiol. 172, 1182–1199. doi: 10.1104/pp.16.00860
Hwang, J. E., Lim, C. J., Chen, H., Je, J., Song, C., and Lim, C. O. (2012). Overexpression of Arabidopsis dehydration-responsive element-binding protein 2C confers tolerance to oxidative stress. Mol. Cells 33, 135–140. doi: 10.1007/s10059-012-2188-2
Iba, K. (2002). Acclimative response to temperature stress in higher plants: approaches of gene engineering for temperature tolerance. Annu. Rev. Plant Biol. 53, 225–245. doi: 10.1146/annurev.arplant.53.100201.160729
Jalmi, S. K., and Sinha, A. K. (2015). ROS mediated MAPK signaling in abiotic and biotic stress- striking similarities and differences. Front. Plant Sci. 6:769. doi: 10.3389/fpls.2015.00769
Je, J., Chen, H., Song, C., and Lim, C. O. (2014). Arabidopsis DREB2C modulates ABA biosynthesis during germination. Biochem. Biophys. Res. Commun. 452, 91–98. doi: 10.1016/j.bbrc.2014.08.052
Jung, J. H., Domijan, M., Klose, C., Biswas, S., Ezer, D., Gao, M., et al. (2016). Phytochromes function as thermosensors in Arabidopsis. Science 354, 886–889. doi: 10.1126/science.aaf6005
Kang, C., He, S., Zhai, H., Li, R., Zhao, N., and Liu, Q. (2018). A sweetpotato auxin response factor gene (IbARF5) is involved in carotenoid biosynthesis and salt and drought tolerance in transgenic Arabidopsis. Front. Plant Sci. 9:1307. doi: 10.3389/fpls.2018.01307
Kanwar, M. K., Yu, J., and Zhou, J. (2018). Phytomelatonin: recent advances and future prospects. J. Pineal Res. 65:e12526. doi: 10.1111/jpi.12526
Kim, H., Lee, S. B., Kim, H. J., Min, M. K., Hwang, I., and Suh, M. C. (2012). Characterization of glycosylphosphatidylinositol-anchored lipid transfer protein 2 (LTPG2) and overlapping function between LTPG/LTPG1 and LTPG2 in cuticular wax export or accumulation in Arabidopsis thaliana. Plant Cell Physiol. 53, 1391–1403. doi: 10.1093/pcp/pcs083
Kimura, S., Waszczak, C., Hunter, K., and Wrzaczek, M. (2017). Bound by fate: the role of reactive oxygen species in receptor-like kinase signaling. Plant Cell 29, 638–654. doi: 10.1105/tpc.16.00947
Kinnersley, A. M. (2000). Gamma aminobutyric acid (GABA) and plant responses to stress. Crit. Rev. Plant Sci. 19, 479–509. doi: 10.1080/07352680091139277
Kong, L. A., Xie, Y., Hu, L., Si, J. S., and Wang, Z. S. (2017). Excessive nitrogen application dampens antioxidant capacity and grain filling in wheat as revealed by metabolic and physiological analyses. Sci. Rep. 7:43363. doi: 10.1038/srep43363
Kong, X. Q., Wang, T., Li, W. J., Tang, W., Zhang, D. M., and Dong, H. Z. (2016). Exogenous nitric oxide delays salt-induced leaf senescence in cotton (Gossypium hirsutum L.). Acta Physiol. Plant. 38:61. doi: 10.1007/s11738-016-2079-9
Kregel, K. C. (2002). Heat shock proteins: modifying factors in physiological stress responses and acquired thermotolerance. J. Appl. Physiol. (1985) 92, 2177–2186. doi: 10.1152/japplphysiol.01267.2001
Kumar, S., and Trivedi, P. K. (2018). Glutathione S-transferases: role in combating abiotic stresses including arsenic detoxification in plants. Front. Plant Sci. 9:751. doi: 10.3389/fpls.2018.00751
Kunst, L., and Samuels, L. (2009). Plant cuticles shine: advances in wax biosynthesis and export. Curr. Opin. Plant Biol. 12, 721–727. doi: 10.1016/j.pbi.2009.09.009
Laloum, T., Martín, G., and Duque, P. (2018). Alternative splicing control of abiotic stress responses. Trends Plant Sci. 23, 140–150. doi: 10.1016/j.tplants.2017.09.019
Le Gall, H., Philippe, F., Domon, J. M., Gillet, F., Pelloux, J., and Rayon, C. (2015). Cell wall metabolism in response to abiotic stress. Plants 4, 112–166. doi: 10.3390/plants4010112
Lee, S. B., and Suh, M. C. (2015). Advances in the understanding of cuticular waxes in Arabidopsis thaliana and crop species. Plant Cell Rep. 34, 557–572. doi: 10.1007/s00299-015-1772-2
Legris, M., Klose, C., Burgie, E. S., Rojas, C. C., Neme, M., Hiltbrunner, A., et al. (2016). Phytochrome B integrates light and temperature signals in Arabidopsis. Science 354, 897–900. doi: 10.1126/science.aaf5656
Legris, M., Nieto, C., Sellaro, R., Prat, S., and Casal, J. J. (2017). Perception and signalling of light and temperature cues in plants. Plant J. 90, 683–697. doi: 10.1111/tpj.13467
Li, K., Pang, C. H., Ding, F., Sui, N., Feng, Z. T., and Wang, B. S. (2012). Overexpression of Suaeda salsa stroma ascorbate peroxidase in Arabidopsis chloroplasts enhances salt tolerance of plants. S. Afr. J. Bot. 78, 235–245. doi: 10.1016/j.sajb.2011.09.006
Li, X., Liu, Y., Chen, M., Song, Y. P., Song, J., Wang, B. S., et al. (2012). Relationships between ion and chlorophyll accumulation in seeds and adaptation to saline environments in Suaeda salsa populations. Plant Biosyst. 146, 142–149. doi: 10.1080/11263504.2012.727880
Li, Z., Zhang, L., Wang, A., Xu, X., and Li, J. (2013). Ectopic overexpression of SlHsfA3, a heat stress transcription factor from tomato, confers increased thermotolerance and salt hypersensitivity in germination in transgenic Arabidopsis. PLoS One 8:e54880. doi: 10.1371/journal.pone.0054880
Li, Z. G., Yang, S. Z., Long, W. B., Yang, G. X., and Shen, Z. Z. (2013). Hydrogen sulphide may be a novel downstream signal molecule in nitric oxide-induced heat tolerance of maize (Zea mays L.) seedlings. Plant Cell Environ. 36, 1564–1572. doi: 10.1111/pce.12092
Lian, C., Yao, K., Duan, H., Li, Q., Liu, C., Yin, W., et al. (2018). Exploration of ABA responsive miRNAs reveals a new hormone signaling crosstalk pathway regulating root growth of Populus euphratica. Int. J. Mol. Sci. 19:1481. doi: 10.3390/ijms19051481
Lisjak, M., Teklic, T., Wilson, I. D., Whiteman, M., and Hancock, J. T. (2013). Hydrogen sulfide: environmental factor or signalling molecule? Plant Cell Environ. 36, 1607–1616. doi: 10.1111/pce.12073
Liu, F., Yang, Y. J., Gao, J. W., Ma, C. L., and Bi, Y. P. (2018). A comparative transcriptome analysis of a wild purple potato and its red mutant provides insight into the mechanism of anthocyanin transformation. PLoS One 13:e0191406. doi: 10.1371/journal.pone.0191406
Liu, S., Lv, Z., Liu, Y., Li, L., and Zhang, L. (2018). Network analysis of ABA-dependent and ABA-independent drought responsive genes in Arabidopsis thaliana. Genet. Mol. Biol. 41, 624–637. doi: 10.1590/1678-4685-GMB-2017-2229
Liu, J., Zhang, F., Zhou, J. J., Chen, F., Wang, B. S., and Xie, X. Z. (2012). Phytochrome B control of total leaf area and stomatal density affects drought tolerance in rice. Plant Mol. Biol. 78, 289–300. doi: 10.1007/s11103-011-98603
Liu, J. H., Kitashiba, H., Wang, J., Ban, Y., and Moriguchi, T. (2007). Polyamines and their ability to provide environmental stress tolerance to plants. Plant Biotechnol. 24, 117–126. doi: 10.5511/plantbiotechnology.24.117
Liu, S. S., Wang, W. Q., Li, M., Wan, S. B., and Sui, N. (2017). Antioxidants and unsaturated fatty acids are involved in salt tolerance in peanut. Acta Physiol. Plant. 39:207. doi: 10.1007/s11738-017-2501-y
Liu, W., Ji, S. X., Fang, X. L., Wang, Q. G., Li, Z., Yao, F. Y., et al. (2013). Protein kinase LTRPK1 influences cold adaptation and microtubule stability in rice. J. Plant Growth Regul. 32, 483–490. doi: 10.1007/s00344-012-9314-4
Liu, X. X., Fu, C., Yang, W. W., Zhang, Q., Fan, H., and Liu, J. (2016). The involvement of TsFtsH8 in Thellungiella salsuginea tolerance to cold and high light stresses. Acta Physiol. Plant. 38:62. doi: 10.1007/s11738-016-2080-3
Liu, Y., and He, C. (2017). A review of redox signaling and the control of MAP kinase pathway in plants. Redox Biol. 11, 192–204. doi: 10.1016/j.redox.2016.12.009
Lu, M., Zhang, Y. Y., Tang, S. K., Pan, J. B., Yu, Y. K., Han, J., et al. (2016). AtCNGC2 is involved in jasmonic acid-induced calcium mobilization. J. Exp. Bot. 67, 809–819. doi: 10.1093/jxb/erv500
Luan, S., Kudla, J., Rodriguez-Concepcion, M., Yalovsky, S., and Gruissem, W. (2002). Calmodulins and calcineurin B-like proteins: calcium sensors for specific signal response coupling in plants. Plant Cell 14, S389–S400. doi: 10.1105/tpc.001115
Mackerness, S. A. H., Surplus, S. L., Blake, P., John, C. F., Buchanan-Wollaston, V., Jordan, B. R., et al. (1999). Ultraviolet-B-induced stress and changes in gene expression in Arabidopsis thaliana: role of signalling pathways controlled by jasmonic acid, ethylene and reactive oxygen species. Plant Cell Environ. 22, 1413–1423. doi: 10.1046/j.1365-3040.1999.00499.x
McFarlane, H. E., Watanabe, Y., Yang, W., Huang, Y., Ohlrogge, J., and Samuels, A. L. (2014). Golgi- and TGN-mediated vesicle trafficking is required for wax secretion from epidermal cells. Plant Physiol. 164, 1250–1260. doi: 10.1104/pp.113.234583
Mikami, K., and Murata, N. (2003). Membrane fluidity and the perception of environmental signals in cyanobacteria and plants. Prog. Lipid Res. 42, 527–543. doi: 10.1016/S0163-7827(03)00036-5
Minocha, R., Majumdar, R., and Minocha, S. C. (2014). Polyamines and abiotic stress in plants: a complex relationship. Front. Plant Sci. 5:175. doi: 10.3389/fpls.2014.00175
Mittler, R., Vanderauwera, S., Gollery, M., and Van Breusegem, F. (2004). Reactive oxygen gene network of plants. Trends Plant Sci. 9, 490–498. doi: 10.1016/j.tplants.2004.08.009
Mizoi, J., Shinozaki, K., and Yamaguchi-Shinozaki, K. (2012). AP2/ERF family transcription factors in plant abiotic stress responses. Biochim. Biophys. Acta 1819, 86–96. doi: 10.1016/j.bbagrm.2011.08.004
Montero-Barrientos, M., Hermosa, R., Cardoza, R. E., Gutiérrez, S., Nicolás, C., and Monte, E. (2010). Transgenic expression of the Trichoderma harzianum hsp70 gene increases Arabidopsis resistance to heat and other abiotic stresses. J. Plant Physiol. 167, 659–665. doi: 10.1016/j.jplph.2009.11.012
Moon, B. Y., Higashi, S., Gombos, Z., and Murata, N. (1995). Unsaturation of the membrane lipids of chloroplasts stabilizes the photosynthetic machinery against low-temperature photoinhibition in transgenic tobacco plants. Proc. Natl. Acad. Sci. U.S.A. 92, 6219–6223. doi: 10.1073/pnas.92.14.6219
Mostofa, M. G., Ghosh, A., Li, Z. G., Siddiqui, M. N., Fujita, M., and Tran, L. P. (2018). Methylglyoxal – A signaling molecule in plant abiotic stress responses. Free Radic. Biol. Med. 122, 96–109. doi: 10.1016/j.freeradbiomed.2018.03.009
Munns, R., and Tester, M. (2008). Mechanisms of salinity tolerance. Annu. Rev. Plant Biol. 59, 651–681. doi: 10.1146/annurev.arplant.59.032607.092911
Murakami, Y., Tsuyama, M., Kobayashi, Y., Kodama, H., and Iba, K. (2000). Trienoic fatty acids and plant tolerance of high temperature. Science 287, 476–479. doi: 10.1126/science.287.5452.476
Murata, N., and Los, D. A. (1997). Membrane fluidity and temperature perception. Plant Physiol. 115, 875–879. doi: 10.1104/pp.115.3.875
Murata, N., shizaki-Nishizawa, O., Higashi, S., Hayash, H., Tasaka, Y., and Nishida, I. (1992). Genetically engineered alteration in the chilling sensitivity of plants. Nature 356, 710–713. doi: 10.1038/356710a0
Nanjo, T., Kobayashia, M., Yoshibab, Y., Kakubaric, Y., Yamaguchi-Shinozaki, K., and Shinozaki, K. (1999). Antisense suppression of proline degradation improves tolerance to freezing and salinity in Arabidopsis thaliana. FEBS Lett. 461, 205–210. doi: 10.1016/S0014-5793(99)01451-9
Nawkar, G. M., Kang, C. H., Maibam, P., Park, J. H., Jung, Y. J., Chae, H. B., et al. (2017). HY5, a positive regulator of light signaling, negatively controls the unfolded protein response in Arabidopsis. Proc. Natl. Acad. Sci. U.S.A. 114, 2084–2089. doi: 10.1073/pnas.1609844114
Newkirk, G. M., Wu, H., Santana, I., and Giraldo, J. P. (2018). Catalytic scavenging of plant reactive oxygen species in vivo by anionic cerium oxide nanoparticles. J. Vis. Exp. 138:e58373. doi: 10.3791/58373
Ng, L. M., Melcher, K., Teh, B. T., and Xu, H. E. (2014). Abscisic acid perception and signaling: structural mechanisms and applications. Acta Pharmacol. Sin. 35, 567–584. doi: 10.1038/aps.2014.5
Nishida, I., and Murata, N. (1996). Chilling sensitivity in plants and cyanobacteria: the crucial contribution of membrane lipids. Annu. Rev. Plant Physiol. Plant Mol. Biol. 47, 541–568. doi: 10.1146/annurev.arplant.47.1.541
Pan, W., Shen, J., Zheng, Z., Yan, X., Shou, J., Wang, W., et al. (2018). Overexpression of the Tibetan Plateau annual wild barley (Hordeum spontaneum) HsCIPKs enhances rice tolerance to heavy metal toxicities and other abiotic stresses. Rice (NY) 11:51. doi: 10.1186/s12284-018-0242-1
Pang, C. H., Li, K., and Wang, B. S. (2011). Overexpression of SsCHLAPXs confers protection against oxidative stress induced by high light in transgenic Arabidopsis thaliana. Physiol. Plant. 143, 355–366. doi: 10.1111/j.1399-3054.2011.01515.x
Pighin, J. A., Zheng, H., Balakshin, L. J., Goodman, I. P., Western, T. L., Jetter, R., et al. (2004). Plant cuticular lipid export requires an ABC transporter. Science 306, 702–704. doi: 10.1126/science.1102331
Pollard, M., Beisson, F., Li, Y., and Ohlrogge, J. B. (2008). Building lipid barriers: biosynthesis of cutin and suberin. Trends Plant Sci. 13, 236–246. doi: 10.1016/j.tplants.2008.03.003
Qi, Y. C., Liu, W. Q., Qiu, L. Y., Zhang, S. M., Ma, L., and Zhang, H. (2010a). Overexpression of glutathione S-transferase gene increases salt tolerance of Arabidopsis. Russ. J. Plant Physiol. 57, 233–240. doi: 10.1134/s102144371002010x
Qi, Y. C., Wang, F. F., Zhang, H., and Liu, W. Q. (2010b). Overexpression of suadea salsa S-adenosylmethionine synthetase gene promotes salt tolerance in transgenic tobacco. Acta Physiol. Plant. 32, 263–269. doi: 10.1007/s11738-009-0403-3
Ren, C. G., Kong, C. C., and Xie, Z. H. (2018). Role of abscisic acid in strigolactone-induced salt stress tolerance in arbuscular mycorrhizal Sesbania cannabina seedlings. BMC Plant Biol. 18:74. doi: 10.1186/s12870-018-1292-7
Ren, X. L., Qi, G. N., Feng, H. Q., Zhao, S., Zhao, S. S., Wang, Y., et al. (2013). Calcineurin B-like protein CBL10 directly interacts with AKT1 and modulates K+ homeostasis in Arabidopsis. Plant J. 74, 258–266. doi: 10.1111/tpj.12123
Roxas, V. P., Lodhi, S. A., Garrett, D. K., Mahan, J. R., and Allen, R. D. (2000). Stress tolerance in transgenic tobacco seedlings that overexpress glutathione S-transferase/glutathione peroxidase. Plant Cell Physiol. 41, 1229–1234. doi: 10.1093/pcp/pcd051
Ryu, H., and Cho, Y. G. (2015). Plant hormones in salt stress tolerance. J. Plant Biol. 58, 147–155. doi: 10.1007/s12374-015-0103-z
Sah, S. K., Reddy, K. R., and Li, J. (2016). Abscisic acid and abiotic stress tolerance in crop plants. Front. Plant Sci. 7:571. doi: 10.3389/fpls.2016.00571
Sangwan, V., Örvar, B. L., Beyerly, J., Hirt, H., and Dhindsa, R. S. (2002). Opposite changes in membrane fluidity mimic cold and heat stress activation of distinct plant MAP kinase pathways. Plant J. 31, 629–638. doi: 10.1046/j.1365-313X.2002.01384.x
Savchenko, T. V., Zastrijnaja, O. M., and Klimov, V. V. (2014). Oxylipins and plant abiotic stress resistance. Biochemistry (Moscow) 79, 362–375. doi: 10.1134/S0006297914040051
Saxena, I., Srikanth, S., and Chen, Z. (2016). Cross talk between H2O2 and interacting signal molecules under plant stress response. Front. Plant Sci. 7:570. doi: 10.3389/fpls.2016.00570
Sazegari, S., Niazi, A., and Ahmadi, F. S. (2015). A study on the regulatory network with promoter analysis for Arabidopsis DREB-genes. Bioinformation 11, 101–106. doi: 10.6026/97320630011101
Scharf, K. D., Berberich, T., Ebersberger, I., and Nover, L. (2012). The plant heat stress transcription factor (Hsf) family: structure, function and evolution. Biochim. Biophys. Acta 1819, 104–119. doi: 10.1016/j.bbagrm.2011.10.002
Seo, P. J., Lee, S. B., Suh, M. C., Park, M. J., Go, Y. S., and Park, C. M. (2011). The MYB96 transcription factor regulates cuticular wax biosynthesis under drought conditions in Arabidopsis. Plant Cell 23, 1138–1152. doi: 10.1105/tpc.111.083485
Seo, P. J., Xiang, F., Qiao, M., Park, J. Y., Lee, Y. N., Kim, S. G., et al. (2009). The MYB96 transcription factor mediates abscisic acid signaling during drought stress response in Arabidopsis. Plant Physiol. 151, 275–289. doi: 10.1104/pp.109.144220
Sharma, P., Jha, A. B., Dubey, R. S., and Pessarakli, M. (2012). Reactive oxygen species, oxidative damage, and antioxidative defense mechanism in plants under stressful conditions. J. Bot. 2012:217037. doi: 10.1155/2012/217037
Shen, X. Y., Wang, Z. L., Song, X. F., Xu, J. J., Jiang, C. Y., Zhao, Y. X., et al. (2014). Transcriptomic profiling revealed an important role of cell wall remodeling and ethylene signaling pathway during salt acclimation in Arabidopsis. Plant Mol. Biol. 86, 303–317. doi: 10.1007/s11103-014-0230-9
Shepherd, T., and Wynne Griffiths, D. (2006). The effects of stress on plant cuticular waxes. New Phytol. 171, 469–499. doi: 10.1111/j.1469-8137.2006.01826.x
Shi, H., Ye, T., and Chan, Z. (2014). Nitric oxide-activated hydrogen sulfide is essential for cadmium stress response in bermudagrass (Cynodon dactylon (L). Pers.). Plant Physiol. Bioch. 74, 99–107. doi: 10.1016/j.plaphy.2013.11.001
Shi, S., Li, S., Asim, M., Mao, J., Xu, D., Ullah, Z., et al. (2018). The Arabidopsis calcium-dependent protein kinases (CDPKs) and their roles in plant growth regulation and abiotic stress responses. Int. J. Mol. Sci. 19:1900. doi: 10.3390/ijms19071900
Shi, Y., Yue, X., and An, L. (2018). Integrated regulation triggered by a cryophyte ω-3 desaturase gene confers multiple-stress tolerance in tobacco. J. Exp. Bot. 69, 2131–2148. doi: 10.1093/jxb/ery050
Siddiqui, M. H., Al-Whaibi, M. H., and Basalah, M. O. (2011). Role of nitric oxide in tolerance of plants to abiotic stress. Protoplasma 248, 447–455. doi: 10.1007/s00709-010-0206-9
Slama, I., Abdelly, C., Bouchereau, A., Flowers, T., and Savoure, A. (2015). Diversity, distribution and roles of osmoprotective compounds accumulated in halophytes under abiotic stress. Ann. Bot. 115, 433–447. doi: 10.1093/aob/mcu239
Song, C., Je, J., Hong, J. K., and Lim, C. O. (2014). Ectopic expression of an Arabidopsis dehydration-responsive element-binding factor DREB2C improves salt stress tolerance in crucifers. Plant Cell Rep. 33, 1239–1254. doi: 10.1007/s00299-014-1612-9
Song, J., Liu, Q., Hu, B., and Wu, W. (2017a). Photoreceptor PhyB involved in Arabidopsis temperature perception and heat-tolerance formation. Int. J. Mol. Sci. 18:1194. doi: 10.3390/ijms18061194
Song, J., Shi, W. W., Liu, R. R., Xu, Y. G., Sui, N., Zhou, J. C., et al. (2017b). The role of the seed coat in adaptation of dimorphic seeds of the euhalophyte Suaeda salsa to salinity. Plant Spec. Biol. 32, 107–114. doi: 10.1111/1442-1984.12132
Song, J., Shi, G. W., Gao, B., Fan, H., and Wang, B. S. (2011). Waterlogging and salinity effects on two Suaeda salsa populations. Physiol. Plant 141, 343–351. doi: 10.1111/j.1399-3054.2011.01445.x
Song, J., and Wang, B. S. (2015). Using euhalophytes to understand salt tolerance and to develop saline agriculture: Suaeda salsa as a promising model. Ann. Bot. 115, 541–553. doi: 10.1093/aob/mcu194
Sreenivasulu, N., Harshavardhan, V. T., Govind, G., Seiler, C., and Kohli, A. (2012). Contrapuntal role of ABA: does it mediate stress tolerance or plant growth retardation under long-term drought stress? Gene 506, 265–273. doi: 10.1016/j.gene.2012.06.076
Stockinger, E. J., Mao, Y., Regier, M. K., Triezenberg, S. J., and Thomashow, M. F. (2001). Transcriptional adaptor and histone acetyltransferase proteins in Arabidopsis and their interactions with CBF1, a transcriptional activator involved in cold-regulated gene expression. Nucleic Acids Res. 29, 1524–1533. doi: 10.1093/nar/29.7.1524
Sui, N. (2015). Photoinhibition of Suaeda salsa to chilling stress is related to energy dissipation and water-water cycle. Photosynthetica 53, 207–212. doi: 10.1007/s11099-015-0080-y
Sui, N., and Han, G. L. (2014). Salt-induced photoinhibition of PSII is alleviated in halophyte Thellungiella halophila by increases of unsaturated fatty acids in membrane lipids. Acta Physiol. Plant. 36, 983–992. doi: 10.1007/s11738-013-1477-5
Sui, N., Li, M., Li, K., Song, J., and Wang, B. S. (2010). Increase in unsaturated fatty acids in membrane lipids of Suaeda salsa L. enhances protection of photosystem II under high salinity. Photosynthetica 48, 623–629. doi: 10.1007/s11099-010-0080-x
Sui, N., Tian, S. S., Wang, W. Q., Wang, M. J., and Fan, H. (2017). Overexpression of glycerol-3-phosphate acyltransferase from Suaeda salsa improves salt tolerance in Arabidopsis. Front. Plant Sci. 8:1337. doi: 10.3389/fpls.2017.01337
Sui, N., Wang, Y., Liu, S. S., Yang, Z., Wang, F., and Wan, S. B. (2018). Transcriptomic and physiological evidence for the relationship between unsaturated fatty acid and salt stress in peanut. Front. Plant Sci. 9:7. doi: 10.3389/fpls.2018.00007
Sun, W., Li, Y., Zhao, Y. X., and Zhang, H. (2015). The TsnsLTP4, a nonspecific lipid transfer protein involved in wax deposition and stress tolerance. Plant Mol. Biol. Rep. 33, 962–974. doi: 10.1007/s11105-014-0798-x
Sun, W., Van Montagu, M., and Verbruggen, N. (2002). Small heat shock proteins and stress tolerance in plants. Biochim. Biophys. Acta 1577, 1–9. doi: 10.1016/S0167-4781(02)00417-7
Sun, Y. L., Li, F., Su, N., Sun, X. L., Zhao, S. J., and Meng, Q. W. (2010). The increase in unsaturation of fatty acids of phosphatidylglycerol in thylakoid membrane enhanced salt tolerance in tomato. Photosynthetica 48, 400–408. doi: 10.1007/s11099-010-0052-1
Sun, Z. B., Qi, X. Y., Wang, Z. L., Li, P. H., Wu, C. X., Zhang, H., et al. (2013). Overexpression of TsGOLS2, a galactinol synthase, in Arabidopsis thaliana enhances tolerance to high salinity and osmotic stresses. Plant Physiol. Biochem. 69, 82–89. doi: 10.1016/j.plaphy.2013.04.009
Sung, D. Y., and Guy, C. L. (2003). Physiological and molecular assessment of altered expression of Hsc70-1 in Arabidopsis. Evidence for pleiotropic consequences. Plant Physiol. 132, 979–987. doi: 10.1104/pp.102.019398
Takahashi, S., and Murata, N. (2008). How do environmental stresses accelerate photoinhibition? Trends Plant Sci. 13, 178–182. doi: 10.1016/j.tplants.2008.01.005
Tang, G. Y., Shao, F. X., Xu, P. L., Shan, L., and Liu, Z. J. (2017). Overexpression of a peanut NAC gene, AhNAC4, confers enhanced drought tolerance in tobacco. Russ. J. Plant Physiol. 64, 525–535. doi: 10.1134/s1021443717040161
Tang, G. Y., Wei, L. Q., Liu, Z. J., Bi, Y. P., and Shan, L. (2012). Ectopic expression of peanut acyl carrier protein in tobacco alters fatty acid composition in the leaf and resistance to cold stress. Biol. Plant. 56, 493–501. doi: 10.1007/s10535-012-0057-7
Tenhaken, R. (2016). Cell wall remodeling under abiotic stress. Front. Plant Sci. 5:771. doi: 10.3389/fpls.2014.00771
Thomashow, M. F. (1999). Plant cold acclimation: freezing tolerance genes and regulatory mechanisms. Annu. Rev. Plant Physiol. Plant Mol. Biol. 50, 571–599. doi: 10.1146/annurev.arplant.50.1.571
Tiwari, S., Lata, C., Chauhan, P. S., Prasad, V., and Prasad, M. (2017). A functional genomic perspective on drought signalling and its crosstalk with phytohormone-mediated signalling pathways in plants. Curr. Genomics 18, 469–482. doi: 10.2174/1389202918666170605083319
Wada, H., and Murata, N. (2007). The essential role of phosphatidylglycerol in photosynthesis. Photosynth. Res. 92, 205–215. doi: 10.1007/s11120-007-9203-z
Wang, F., Guo, Z., Li, H., Wang, M., Onac, E., Zhou, J., et al. (2016). Phytochrome A and B function antagonistically to regulate cold tolerance via abscisic acid-dependent jasmonate signaling. Plant Physiol. 170, 459–471. doi: 10.1104/pp.15.01171
Wang, X., Yan, B., Shi, M., Zhou, W., Zekria, D., Wang, H., et al. (2016). Overexpression of a Brassica campestris HSP70 in tobacco confers enhanced tolerance to heat stress. Protoplasma 253, 637–645. doi: 10.1007/s00709-015-0867-5
Wang, F. F., Lian, H. L., Kang, C. Y., and Yang, H. Q. (2010). Phytochrome B is involved in mediating red light-induced stomatal opening in Arabidopsis thaliana. Mol. Plant 3, 246–259. doi: 10.1093/mp/ssp097
Wang, H., Tang, J., Liu, J., Hu, J., Liu, J., Chen, Y., et al. (2018). Abscisic acid signaling inhibits brassinosteroid signaling through dampening the dephosphorylation of BIN2 by ABI1 and ABI2. Mol. Plant 11, 315–325. doi: 10.1016/j.molp.2017.12.013
Wang, W., Wang, X., Huang, M., Cai, J., Zhou, Q., Dai, T., et al. (2018). Hydrogen peroxide and abscisic acid mediate salicylic acid-induced freezing tolerance in wheat. Front. Plant Sci. 9:1137. doi: 10.3389/fpls.2018.01137
Wang, X., Wang, Y., Wang, L., Liu, H., Zhang, B., Cao, Q., et al. (2018). Arabidopsis PCaP2 functions as a linker between ABA and SA signals in plant water deficit tolerance. Front. Plant Sci. 9:578. doi: 10.3389/fpls.2018.00578
Wang, J. S., Zhang, Q., Cui, F., Hou, L., Zhao, S. Z., Xia, H., et al. (2017). Genome-wide analysis of gene expression provides new insights into cold responses in Thellungiella salsuginea. Front. Plant Sci. 8:713. doi: 10.3389/fpls.2017.00713
Wang, P. F., Song, H., Li, C. S., Li, P. C., Li, A. Q., Guan, H. S., et al. (2017). Genome-wide dissection of the heat shock transcription factor family genes in Arachis. Front. Plant Sci. 8:106. doi: 10.3389/fpls.2017.00106
Wang, K., Ding, Y., Cai, C., Chen, Z., and Zhu, C. (2018). The role of C2H2 zinc finger proteins in plant responses to abiotic stresses. Physiol. Plant. doi: 10.1111/ppl.12728 [Epub ahead of print].
Wang, M., and Liao, W. (2016). Carbon monoxide as a signaling molecule in plants. Front. Plant Sci. 7:572. doi: 10.3389/fpls.2016.00572
Wang, W., Vinocur, B., and Altman, A. (2003). Plant responses to drought, salinity and extreme temperatures: towards genetic engineering for stress tolerance. Planta 28, 1–14. doi: 10.1007/s00425-003-1105-5
Wang, W., Vinocur, B., Shoseyov, O., and Altman, A. (2004). Role of plant heat-shock proteins and molecular chaperones in the abiotic stress response. Trends Plant Sci. 9, 244–252. doi: 10.1016/j.tplants.2004.03.006
Wania, S. H., Kumar, V., Shriram, V., and Sah, S. K. (2016). Phytohormones and their metabolic engineering for abiotic stress tolerance in crop plants. Crop J. 4, 162–176. doi: 10.1016/j.cj.2016.01.010
Wei, W., Cui, M. Y., Hu, Y., Gao, K., Xie, Y. G., Jiang, Y., et al. (2018). Ectopic expression of FvWRKY42, a WRKY transcription factor from the diploid woodland strawberry (Fragaria vesca), enhances resistance to powdery mildew, improves osmotic stress resistance, and increases abscisic acid sensitivity in Arabidopsis. Plant Sci. 275, 60–74. doi: 10.1016/j.plantsci.2018.07.010
Wu, H., Tito, N., and Giraldo, J. P. (2017). Anionic cerium oxide nanoparticles protect plant photosynthesis from abiotic stress by scavenging reactive oxygen species. ACS Nano 11, 11283–11297. doi: 10.1021/acsnano.7b05723
Xu, J., Tian, Y. S., Peng, R. H., Xiong, A. S., Zhu, B., Jin, X. F., et al. (2010). AtCPK6, a functionally redundant and positive regulator involved in salt/drought stress tolerance in Arabidopsis. Planta 231, 1251–1260. doi: 10.1007/s00425-010-1122-0
Xu, Y. G., Liu, R. R., Sui, N., Shi, W. W., Wang, L., Tian, C. Y., et al. (2016). Changes in endogenous hormones and seed-coat phenolics during seed storage of two Suaeda salsa populations. Aust. J. Bot. 64, 325–332. doi: 10.1071/bt16014
Xue, D., Zhang, X., Lu, X., Chen, G., and Chen, Z. H. (2017). Molecular and evolutionary mechanisms of cuticular wax for plant drought tolerance. Front. Plant Sci. 8:621. doi: 10.3389/fpls.2017.00621
Yancey, P. H. (2005). Organic osmolytes as compatible, metabolic and counteracting cytoprotectants in high osmolarity and other stresses. J. Exp. Biol. 208, 2819–2830. doi: 10.1242/jeb.01730
Yang, J. C., Li, M., Xie, X. Z., Han, G. L., Sui, N., and Wang, B. S. (2013). Deficiency of phytochrome B alleviates chilling-induced photoinhibition in rice. Am. J. Bot. 100, 1860–1870. doi: 10.3732/ajb.1200574
Yang, M. F., Song, J., and Wang, B. S. (2010). Organ-specific responses of vacuolar H+-ATPase in the shoots and roots of C3 halophyte Suaeda salsa to NaCl. J. Integr. Plant Biol. 52, 308–314. doi: 10.1111/j.1744-7909.2010.00895.x
Yang, T., Lv, R., Li, J., Lin, H., and Xi, D. (2018). Phytochrome A and B negatively regulate salt stress tolerance of Nicotiana tobacum via abscisic acid-jasmonic acid synergistic cross talk. Plant Cell Physiol. 59, 2381–2393. doi: 10.1093/pcp/pcy164
Yang, Z., Wang, Y., Wei, X. C., Zhao, X., Wang, B. S., and Sui, N. (2017). Transcription profiles of genes related to hormonal regulations under salt stress in sweet sorghum. Plant Mol. Biol. Rep. 35, 586–599. doi: 10.1007/s11105-017-1047-x
Yeats, T. H., Martin, L. B., Viart, H. M., Isaacson, T., He, Y, Zhao, L., et al. (2012). The identification of cutin synthase: formation of the plant polyester cutin. Nat. Chem. Biol. 8, 609–611. doi: 10.1038/nchembio.960
Yeats, T. H., and Rose, J. K. (2013). The formation and function of plant cuticles. Plant Physiol. 163, 5–20. doi: 10.1104/pp.113.222737
Yin, Y., Jiang, X., Ren, M., Xue, M., Nan, D., Wang, Z., et al. (2018). AmDREB2C, from Ammopiptanthus mongolicus, enhances abiotic stress tolerance and regulates fatty acid composition in transgenic Arabidopsis. Plant Physiol. Biochem. 130, 517–528. doi: 10.1016/j.plaphy.2018.08.002
Yoo, Y. H., Nalini Chandran, A. K., Park, J. C., Gho, Y. S., Lee, S. W., An, G., et al. (2017). OsPhyB-mediating novel regulatory pathway for drought tolerance in rice root identified by a global RNA-seq transcriptome analysis of rice genes in response to water deficiencies. Front. Plant Sci. 8:580. doi: 10.3389/fpls.2017.00580
Yuan, F., Chen, M., Leng, B. Y., and Wang, B. S. (2013). An efficient autofluorescence method for screening Limonium bicolor mutants for abnormal salt gland density and salt secretion. S. Afr. J. Bot. 88, 110–117. doi: 10.1016/j.sajb.2013.06.007
Yuan, F., Chen, M., Yang, J. C., Song, J., and Wang, B. S. (2015a). The optimal dosage of 60Co gamma irradiation for obtaining salt gland mutants of exo-recretohalophyte Limonium bicolor (bunge) O. kuntze. Pak. J. Bot. 47, 71–76.
Yuan, F., Leng, B. Y., and Wang, B. S. (2016a). Progress in studying salt secretion from the salt glands in recretohalophytes: how do plants secrete salt? Front. Plant Sci. 7:977. doi: 10.3389/fpls.2016.00977
Yuan, F., Lyu, M.-J. A., Leng, B.-Y., Zheng, G.-Y., Feng, Z.-T., Li, P.-H., et al. (2015b). Comparative transcriptome analysis of developmental stages of the Limonium bicolor leaf generates insights into salt gland differentiation. Plant Cell Environ. 38, 1637–1657. doi: 10.1111/pce.12514
Yuan, F., Lyu, M. J. A., Leng, B. Y., Zhu, X. G., and Wang, B. S. (2016b). The transcriptome of NaCl-treated Limonium bicolor leaves reveals the genes controlling salt secretion of salt gland. Plant Mol. Biol. 91, 241–256. doi: 10.1007/s11103-016-0460-0
Zhang, H., Tang, J., Liu, X. P., Wang, Y., and Yu, W. (2009). Hydrogen sulfide promotes root organogenesis in Ipomoea batatas, Salix matsudana and Glycine max. J. Integr. Plant Biol. 51, 1086–1094. doi: 10.1111/j.1744-7909.2009.00885.x
Zhang, J. X., Wang, C., Yang, C. Y., Wang, J. Y., Chen, L., Bao, X. M., et al. (2010). The role of Arabidopsis AtFes1A in cytosolic Hsp70 stability and abiotic stress tolerance. Plant J. 62, 539–548. doi: 10.1111/j.1365-313X.2010.04173.x
Zhang, S. R., Song, J., Wang, H., and Feng, G. (2010). Effect of salinity on seed germination, ion content and photosynthesis of cotyledons in halophytes or xerophyte growing in Central Asia. J. Plant Ecol. 3, 259–267. doi: 10.1093/jpe/rtq005
Zhang, J. Y., Broeckling, C. D., Sumner, L. W., and Wang, Z. Y. (2007). Heterologous expression of two Medicago truncatula putative ERF transcription factor genes, WXP1 and WXP2, in Arabidopsis led to increased leaf wax accumulation and improved drought tolerance, but differential response in freezing tolerance. Plant Mol. Biol. 64, 265–278. doi: 10.1007/s11103-007-9150-2
Zhang, Q., Zhao, C. Z., Li, M., Sun, W., Liu, Y., Xia, H., et al. (2013). Genome-wide identification of Thellungiella salsuginea microRNAs with putative roles in the salt stress response. BMC Plant Biol. 13:180. doi: 10.1186/1471-2229-13-180
Zhao, K.-F., Song, J., Fan, H., Zhou, S., and Zhao, M. (2010). Growth response to ionic and osmotic stress of NaCl in salt-tolerant and salt-sensitive maize. J. Integr. Plant Biol. 52, 468–475. doi: 10.1111/j.1744-7909.2010.00947.x
Zhao, S. S., Jiang, Y. X., Zhao, Y., Huang, S. J., Yuan, M., Zhao, Y. X., et al. (2016). CASEIN KINASE1-LIKE PROTEIN2 regulates actin filament stability and stomatal closure via phosphorylation of actin depolymerizing factor. Plant Cell 28, 1422–1439. doi: 10.1105/tpc.16.00078
Zhao, S. Z., Sun, H. Z., Gao, Y., Sui, N., and Wang, B. S. (2011). Growth regulator-induced betacyanin accumulation and dopa-4,5-dioxygenase (DODA) gene expression in euhalophyte Suaeda salsa calli. In Vitro Cell. Dev. Biol. Plant 47, 391–398. doi: 10.1007/s11627-011-9339-6
Zhao, Y., Zhao, S. S., Mao, T. L., Qu, X. L., Cao, W. H., Zhang, L., et al. (2011). The plant-specific actin binding protein SCAB1 stabilizes actin filaments and regulates stomatal movement in Arabidopsis. Plant Cell 23, 2314–2330. doi: 10.1105/tpc.111.086546
Zheng, Y., Liao, C. C., Zhao, S. S., Wang, C. W., and Guo, Y. (2017). The glycosyltransferase QUA1 regulates chloroplast-associated calcium signaling during salt and drought stress in Arabidopsis. Plant Cell Physiol. 58, 329–341. doi: 10.1093/pcp/pcw192
Zhou, J. C., Fu, T. T., Sui, N., Guo, J. R., Feng, G., Fan, J. L., et al. (2016). The role of salinity in seed maturation of the euhalophyte Suaeda salsa. Plant Biosyst. 150, 83–90. doi: 10.1080/11263504.2014.976294
Zhou, S., Jia, L., Chu, H., Wu, D., Peng, X., Liu, X., et al. (2016). Arabidopsis CaM1 and CaM4 promote nitric oxide production and salt resistance by inhibiting S-nitrosoglutathione reductase via direct binding. PLoS Genet. 12:e1006255. doi: 10.1371/journal.pgen.1006255
Zhou, J. J., Liu, Q. Q., Zhang, F., Wang, Y. Y., Zhang, S. Y., Cheng, H. M., et al. (2014). Overexpression of OsPIL15, a phytochrome-interacting factor-like protein gene, represses etiolated seedling growth in rice. J. Integr. Plant Biol. 56, 373–387. doi: 10.1111/jipb.12137
Keywords: abiotic stresses, land plants, general defenses, regulatory network, multistress tolerance
Citation: He M, He C-Q and Ding N-Z (2018) Abiotic Stresses: General Defenses of Land Plants and Chances for Engineering Multistress Tolerance. Front. Plant Sci. 9:1771. doi: 10.3389/fpls.2018.01771
Received: 16 August 2018; Accepted: 14 November 2018;
Published: 07 December 2018.
Edited by:
Rosa M. Rivero, Centro de Edafología y Biología Aplicada del Segura (CEBAS), SpainReviewed by:
Nobuhiro Suzuki, Sophia University, JapanParvathi Madathil Sreekumar, Kerala Agricultural University, India
Copyright © 2018 He, He and Ding. This is an open-access article distributed under the terms of the Creative Commons Attribution License (CC BY). The use, distribution or reproduction in other forums is permitted, provided the original author(s) and the copyright owner(s) are credited and that the original publication in this journal is cited, in accordance with accepted academic practice. No use, distribution or reproduction is permitted which does not comply with these terms.
*Correspondence: Nai-Zheng Ding, bnpkaW5nQHNkbnUuZWR1LmNu