- Department of Agricultural Sciences, Biotechnology and Food Science, Cyprus University of Technology, Limassol, Cyprus
Irrigation with saline water causes significant crop yield loss. However, short-term saline application might cause less negative effects on yield yet at the same time improve quality aspects of edible products. Tagetes (Tagetes patula L.) plants were subjected to salinity (0, 50, and 100 mM NaCl) and harvested flowers were stored up to 14 days in passive modified atmosphere packaging (with or without ethanol application). Salinity of 100 mM NaCl decreased plant biomass and plant size (i.e., height) and had a negative effect on physiological processes such as stomatal closure and chlorophylls content decrease. Salinity increased flower polyphenols, antioxidant activities, and total carotenoids but decreased anthocyanins, and greater impacts were found at salinity of 100 mM NaCl, providing higher antioxidant value of the edible flowers. Short-term saline exposure of tagetes plants activated metabolic processes and as a result there was an accumulation of minerals such as N, P, Na, and Zn on edible flowers. During storage, salinity maintained but ethanol application increased the flower CO2 production. Ethanol application decreased the decay of flowers subjected to 100 mM NaCl. Flower weight losses and marketability accelerated at salinity of 100 mM NaCl after 14 days of storage. Tagetes flowers demonstrated induction in both non-enzymatic (i.e., proline content) and enzymatic mechanisms (catalase) to overcome stress caused by salinity during harvest stage and/or ethanol at storage. Our results have shown that short-term exposure to salinity and/or ethanol is able to achieve higher carotenoids and anthocyanins levels and these compounds can be considered as a new source of nutraceuticals.
Introduction
High consumer preferences in fresh produce with increased popularity of edible flowers is resulting from their important properties for human health because of their abundance in bioactive and nutraceutical components, which offers further marketing opportunities (Mlcek and Rop, 2011). Among others, several plant species are used for disease treatment practices as well as natural additives in foods (Kaisoon et al., 2012; Rachuonyo et al., 2016). The nutritional value of edible flowers is quite similar to the one of leafy vegetables in terms of proteins, fats, polysaccharides, minerals, and vitamins (Upadhyay, 2011) while their antioxidative properties are well appreciated as they are rich in carotenoids and flavonoids (Mato et al., 2000; Friedman et al., 2010).
Intensive cultivation of plants for the production of edible flowers will contribute to the market needs and consumers’ expectations. However, less fertile fields, lack of knowledge about cultivation practices and/or transportation/storage parameters may limit the expansion of edible flowers market. Moreover, salinized land areas are expanding over time along the seaside areas, such as the Mediterranean basin. Salinity is one of the main abiotic factors that decrease crop yields and plant growth by causing hyperionic and hyperosmotic effects on soil solution around rhizosphere (Munns, 2002; Chrysargyris et al., 2018). This results in disturbance of water and minerals uptake by the roots and consequently decrease in yield and quality of the products. Fresh products of lower quality will reflect decrease in storage life of the fresh commodity. As a consequence, producers often have to cope with salinity due to the absence of good quality watering and they have to grow plants in soil or in soilless culture under saline conditions which is of challenge, and occasionally one-way direction. Plants grown in saline environment subjected to physiological and biochemical changes, manage the production of reactive oxygen species (ROS), by activating antioxidative mechanisms. To overcome oxidative stress, plants detoxify ROS by increasing the specific activity of antioxidant enzymes (superoxide dismutase-SOD, catalase-CAT, peroxidase-POX, glutathione peroxidase-GPX, glutathione S-transferases-GST, ascorbate peroxidase-APX, dehydroascorbate reductase-DHAR, glutathione reductase-GR, and monodehydroascorbate reductase-MDHAR) or producing non-enzymatic antioxidant molecules (ascorbate, glutathione, α-tocopherol, etc.) (Chrysargyris et al., 2018). The first line for ROS detoxification initiated by SOD increases in order to convert O2- to H2O2, and thereafter the H2O2 produced is scavenged by catalase and a variety of peroxidases (Tarchoune et al., 2010). Catalase dismutates H2O2 into H2O and O2, whereas POX decomposes H2O2 by oxidation of co-substrates such as phenolic compounds and/or antioxidants (Chrysargyris et al., 2018).
Edible flowers are present in culinary arts by adding flavor, freshness, color, exotic and spicy aroma, improving appearance and are increasingly favored in gourmet cuisine (Kelley et al., 2003). Diverse use of edible flowers is noticed in restaurants and catering services since they are used as garnishes and/or trimming to meals or additives in soups, fresh salads, sweets and savory dishes (Mlcek and Rop, 2011). On top of the fresh use of edible flowers, they can also be consumed dried in drink preparation, in ice cubes in cocktail making and canned in sugar (Mlcek and Rop, 2011).
Like fruit and vegetables, harvested flowers deteriorate quite fast and need to be cooled and stored quickly at chilled temperature (1–5°C) for 2–14 days (Kelley et al., 2003). However, edible flowers could be much more perishable compared to certain fruits. The flowers mainly are highly rotten and their storage duration has a substantial role in determining their retailing value. Fresh produce, including edible flowers, deteriorates with symptoms of browning, chlorophyll bleaching, tissue breakdown, off-flavors and decay. These changes are related to senescence whereas increased rates of respiration, water loss, enzyme activities and/or opportunistic microorganisms’ infection are the key factors for tissue breakdown (Ragaert et al., 2007). The quality and storage duration of edible flowers are firmly associated with the preharvest culturing management used by the producers, including fertigation practices and saline levels. Additionally, postharvest preservation management used in packing houses also influences the quality and storage of fresh commodities, including edible flowers. Among others, ethanol application during postharvest preservation of fresh produce (Han et al., 2006; Tzortzakis and Economakis, 2007; Tzortzakis, 2010) and cut flower (Kaur and Mukherjee, 2013; Begri et al., 2014) has already been examined, while the ethanol application method usually includes dipping rather than vaporization (Begri et al., 2014). The effectiveness of ethanol is related to the increase of the vase life of carnation flowers by preventing the biosynthesis and action of ethylene (Heins and Blakely, 1992).
Tagetes patula, commonly known as tagetes/marigold, has various (orange, yellow, mixed) color flowers and bitterish, clove-like flavor (Mlcek and Rop, 2011). Tagetes species are widely known for their flavonoids and terpenes content (Munhoz et al., 2014). As a result, they possess antimicrobial (Gakuubi et al., 2016), insecticidal (Perich et al., 1995), larvicidal (Giarratana et al., 2017), and antioxidant (Fu and Mao, 2008) properties and are used in various countries as traditional medicines to treat colic, diarrhea, vomit, fever, skin diseases and hepatic disorders (Jain et al., 2012).
Not much information is known about the physiology, biochemistry, and postharvest performance of this species when grown in saline environments. The objectives of the present study were to examine (i) the effects of saline levels on tagetes growth, plant physiology and quality of edible flowers, (ii) the postharvest performance of edible flowers from plants grown in saline environments, (iii) the effects of ethanol-treated flowers during chilled storage, and (iv) the combined effect of salinity (in preharvest) and the ethanol application (in postharvest). As a result, this can achieve a better understanding of the responses of tagetes plants to salinity during growth and storage.
Materials and Methods
Plant and Experimental Conditions
The present study took place at the greenhouse hydroponic infrastructure of Cyprus University of Technology during the autumn of 2017. Air temperature varied from 29 ± 2°C and 21 ± 2°C during day and night, respectively.
Tagetes (T. patula L.) seedlings were grown in nursery (T = 18.5–18.8°C; RH = 72–76%, Light:Dark = 16:8 h) for a 3-week period, fertigated with nutrient solution (20-20-20) of electrical conductivity (EC) 2.1 mS/cm for 1 week in order to get plant growth uniformity at the stage of two-true leaves. Seedlings were transplanted in 400 L capacity tanks (40 plants per tank).
Experimental Set Up
Once tagetes plants were acclimated to the soilless culture environment, they were grown for 42 days to a complete nutrient solution (NS) in deep flow technique (DFT) system. The nutrient solution was refilled with the stock solution every week, to restock nutrients that might have been absorbed. The composition of the stock solution (1:100 v/v) in water was: NO3--N = 13.65, K = 7.05, PO4-P = 1.29, Ca = 7.63, Mg = 2.81, SO4-2-S = 1.12, and Na = 1.92 mmol/L, respectively; and B = 30.00, Fe = 25.00, Mn = 10.23, Cu = 0.75, Zn = 4.00, and Mo = 0.51 μmol/L, respectively. The optimal pH and EC of the NS were 5.9 and 2.0 mS/cm, respectively. The NS pH was recorded every other day and tailored accordingly (because of water alkalinity) using H2SO4 (5% v/v). Nutrient solution was oxygenated twice a day for 0.5 h by means of a pressure pump.
Thereafter, plants were exposed for additional 10 days at three saline (0, 50, and 100 mM NaCl) levels. Each salinity treatment was divided into two tanks and each tank supported four polyethylene trays of 10 plants capacity per tray. The half plants (40 out of 80 plants) were considered further as experimental for the present study. Each saline treatment consisted of four biological replications (10 plants/replication; 40 plants in total for each treatment) which were subjected to further measurements. The regular EC of the nutrient solution was 2.0 mS/cm for the control treatment (0 mM NaCl), 6.0 mS/cm for the salinity of 50 mM NaCl, and 10.0 mS/cm for the salinity of 100 mM NaCl, as it was kept constant during the salinity stress period (Supplementary Figure S1).
Plant Growth Parameters
One hundred and twenty plants of tagetes were used in the present study. After 6 weeks of plant growth under complete NS plus 10 days of saline short-time stress, four biological replications (each replication was a pool of three measurements in individual plants) for each treatment were studied in detail for plant growth measurements. Plant height, fresh and dry plant weight were measured for the aerial part of the plant (i.e., leaves and stems). The dry matter content was acquired by drying samples to constant weight using a thermo-ventilated oven at 65°C.
Physiological Parameters and Photosynthetic Pigment Content
Every 2 days for a period of 10 days of saline stress, maximum Fv/Fm photochemical quantum yields of PSII were determined with an OptiSci OS-30p Chlorophyll Fluorometer (Opti-Sciences) according to Chrysargyris et al. (2017). Chlorophylls (Chl a, Chl b, and total-Chl) content was determined at the end of the experiment based on the method previously described (Chrysargyris et al., 2017). Leaf stomatal conductance was measured on the 4th and 5th sun-exposed leaf from the top of the plant (three measurements per leaf) by using a ΔT-Porometer AP4 (Delta-T Devices-Cambridge, United Kingdom) in accordance with the manufacturer’s instructions.
Plant Mineral Content
At the end of the experiment, leaf and flower minerals were determined as described in Chrysargyris et al. (2018). Sub samples (0.2–0.3 g) were digested using hydrochloric acid (2 N HCl). K and Na were determined by means of flame photometry (JENWAY, PEP-7 Jenway, Dunmow, United Kingdom), P was determined spectrophotometrically (Multiskan GO, Thermo Fischer Scientific, United States), Mg, Ca, Cu, Fe, and Zn, were determined by an atomic absorption spectrophotometer (PG Instruments AA500FG, Leicestershire, United Kingdom) and N with the help of the Kjeldahl method (BUCHI, Digest automat K-439 and Distillation Kjelflex K-360, Switzerland). Data was expressed in g/kg and mg/kg of dry weight for macro- and micronutrient, respectively.
Polyphenols and Antioxidant Activity of Leaves and Flowers
Leaves and flowers (0.5 g) polyphenols were extracted (Chrysargyris et al., 2016) with 10 mL of methanol (50% v/v) and supernatant was analyzed for total phenolics and total antioxidant activity by means of the 2,2-diphenyl-1-picrylhydrazyl (DPPH), ferric reducing antioxidant power (FRAP) and 2,2′-azino-bis(3-ethylbenzothiazoline-6-sulfonic acid) (ABTS) methods. Polyphenols content was determined using the Folin-Ciocalteu method at 755 nm according to Marinou et al. (2013) and the results were expressed as equivalents of gallic acid (Scharlau, Spain) per gram of fresh weight. The antioxidant capacity measurement using the DPPH, FRAP, and ABTS assays was performed as previously described (Wojdylo et al., 2007; Chrysargyris et al., 2017). The results for antioxidant activities were expressed in equivalents of trolox per gram of fresh weight.
Carotenoids and Anthocyanins Content of Flowers
Total carotenoid content was measured at 480 nm as described by Rafiq et al. (2008) with adjustments. Carotenoids were measured using the following equation: Carotenoids (μg) = 4 × A480 × volume (mL) (Pessarakli, 1997) and results were expressed as mg of carotenoids per gram of fresh weight.
Total anthocyanins were measured with the pH-differential method (Loizzo et al., 2015) with slight adjustments. In brief, 1 mL of the extract [500 mg in 15 mL methanol/dH2O/HCl (70:29:1)] was mixed with (a) 3.5 mL of potassium chloride buffer (0.025 M, pH 1) or (b) 3.5 mL of sodium acetate buffer (0.025 M, pH 4.5). The absorbance of each solution was measured at 520 and 700 nm. The absorbance difference was calculated as follows: A = [(A520 -A700)pH1.0 - (A520 - A700)pH4.5] and the anthocyanin content was calculated with the following equation: mg of cyanidin 3-glucoside equivalents/L = (absorbance × MW × dilution factor × 1000)/(𝜀 × 1); using the molar absorptivity (𝜀) and molecular weight (MW) of cyanidin 3-glucoside (𝜀 = 26900; MW = 449.2). Results were expressed in mg of cyanidin 3-glucoside equivalents per gram of fresh weight.
Hydrogen Peroxide Content and Lipid Peroxidation of Flowers
Hydrogen peroxide (H2O2) content of flowers was determined according to the method of Loreto and Velikova (2001). Flower tissue (0.2 g) was ground in ice cold 0.1% trichloroacetic acid (TCA) and centrifuged at 15000 g for 15 min. Aliquot (0.5 mL) of the supernatant was mixed with 0.5 mL of 10 mM potassium-phosphate buffer (pH = 7.0) and 1 mL of 1 M potassium iodide. The absorbance of samples and standards was measured at 390 nm and results were expressed as μmol H2O2/g fresh weight.
Lipid peroxidation was assessed according to Azevedo-Neto et al. (2006) and measured in terms of malondialdehyde content (MDA). Flower tissue (0.2 g) was homogenized in 0.1% TCA and the extract was centrifuged at 15000 g for 10 min. The reaction mixture of 0.5 mL extract and 1.5 mL of 0.5% thiobarbituric acid (TBA) in 20% TCA was incubated at 95°C for 25 min and then cooled on ice bath. The absorbance was determined at 532 nm. Results were expressed as nmol of MDA/g fresh weight.
Proline Content and Antioxidant Enzymes Activities of Flowers
Harvested flowers (four replicates/treatment) were homogenized in a 50 mM potassium-phosphate ice-cold extraction buffer containing 1 mM ethylenediaminetetraacetic acid (EDTA), 1% (w/v) polyvinylpyrrolidone (PVPP), 1 mM phenylmethylsulfonyl fluoride (PMSF) and 0.05% Triton X-100 (pH = 7.0). Protein content was determined using bovine serum albumin as described previously (Chrysargyris et al., 2017).
Proline was determined according to acid-ninhydrin and toluene method at 520 nm (Khedr et al., 2003). Results were expressed in micrograms of proline per gram of fresh weight. Catalase (CAT) and superoxide dismutase (SOD) activity were assayed according to Jiang and Zhang (2002). CAT was assayed in a reaction mixture (1.5 mL) containing 50 mM K-phosphate buffer (pH 7.0), 10 mM H2O2 and an enzyme aliquot. The reduction of H2O2 was measured at 240 nm. The results were expressed in CAT units/mg of protein (1 unit = 1 mM of H2O2 reduction per min). SOD was assayed using a photochemical method; a reaction mixture (1.5 mL) containing 50 mM K-phosphate buffer (pH 7.5), 13 mM methionine, 75 μM nitro blue tetrazolium (NBT), 0.1 mM EDTA, 2 μM riboflavin and an enzyme aliquot. The reaction began by exposing the mixture to a light source of two 15 watt fluorescent lamps for 15 min and was stopped by placing the tubes in the dark. Absorbance was determined at 560 nm and activity was expressed in units/mg of protein. Peroxidase activity (POD) was determined as described by Tarchoune et al. (2012) following the increase in absorbance at 430 nm. Calculations were performed using the coefficient of extinction of 2.47 mM/cm. One POD unit was defined as the amount of enzyme to decompose 1 μmol of H2O2 per minute. Results were expressed in units/mg of protein. The activity of APX was determined according to Zhu et al. (2004) by the decrease in the absorbance of ascorbate at 290 nm. Results were expressed in units APX/mg of protein.
Edible Flower Processing and Shelf-Life Parameters
Postharvest Experimental Set Up for Flowers
Harvested plants with flowers were transferred to the laboratory within 30 min and were chosen on the basis of similar flower quality. In order to examine the impacts of salinity on flowers quality, a batch of flowers (5 flowers; ∼38.2 g total weight) was stored directly in polyethylene terephthalate (PET) plastic trays (1 L capacity) up to 14 days. Another batch of flowers was vaporized once with absolute ethanol (0.1% v/v) during a storage of 14 days. The flowers were placed in PET trays (∼4–5 flowers per tray) with snap-on lids, sealed with parafilm and cooled at 5°C, under passive modified atmospheric packaging (MAP). Moisturized (autoclaved dH2O) filter paper was placed in each container to maintain high relative humidity during the storage period as described previously (Tzortzakis and Economakis, 2007).
The storage life of fresh produce that maintains not only appearance and safety but also nutritional value is reflected in good quality produce and consumer acceptance (Delaquis et al., 1999). For this reason, after 7 and 14 days of storage at 5°C, CO2 concentration inside the plastic trays and quality attributes were recorded on four replications per treatment. Temperature (T°C) and relative humidity (RH %) of the refrigerated trays were monitored during storage period (T 5 ± 0.3°C and RH 87 ± 2%).
Determination of CO2, Weight Loss, and Color of Flowers
Flower respiration was estimated by measuring CO2 concentration of the packages using the Dual gas analyzer (International Control Analyzer Ltd., United Kingdom). Gaseous samples were drawn through septa with a syringe to prevent gas leakage from the packages. Flower weight loss was measured, as the weight of each container was registered before and after storage at 5°C for 7 and 14 days, and results were calculated in weight loss percentage.
The color of the flowers was evaluated with a colorimeter (Chroma meter CR400 Konica Minolta, Japan) where the L∗ (lightness), a∗ (green to red), and b∗ (blue to yellow) values were recorded on day 0, 7, and 14 (three measurements per replicate/four replicates per treatment). The chroma value (C) was calculated with the following equations C = (a∗2+b∗2)1/2 (Bolin and Huxsoll, 1991).
Determination of Phenolics, Antioxidants, Proline, and Lipid Peroxidation of Flowers
Total phenolic content, carotenoids and anthocyanins content, the DPPH, FRAP, and ABTS scavenging activity, proline content, hydrogen peroxide production, lipid peroxidation, and antioxidant enzymes of flowers were determined as described above following 7 and 14 days of storage.
Determination of Decay and Marketability of Flowers
The decay severity was macroscopically evaluated after 14 d of storage with or without ethanol vapor at 5°C/90% RH. The degree of flower decay was rated (at 0.5 intervals) using a scale of 1–4, where 1-clean, no decay, 2-decay less than 25% of the surface, 3-moderate decay (25–50% decay), and 4-severe decay (>50% decay). Plant marketability was also assessed on a 9-point scale (1-low or poor and 9-high or very excellent).
Statistical Methods
Data was statistically analyzed with the use of IBM SPSS v.21 (IBM Corp., Armonk, NY, United States) software, subjected to analysis of variance (ANOVA), and was expressed by means ± SE (n = 4; each replicate consisted of three individual measurements from a pool of plants). Significant differences between mean values were determined using the Duncan Multiple Range Test at P = 0.05.
Results
Growth and Physiological Parameters
Table 1 presents the effects of salinity concentration into the NS on the plant growth and physiology related parameters. Plant height and leaf stomatal conductance significantly (P < 0.05) decreased with salinity of 100 mM NaCl while no differences were found among control and low salinity levels (50 mM NaCl). Both fresh and dry weight of the plants decreased in salinity of 100 mM NaCl. Maximum quantum efficiency of PSII significantly decreased after 3 days of salinity (50 and 100 mM NaCl) stress, whereas it remained unaffected up to the 10th day of saline treatments (Supplementary Figure S2). Chlorophylls (Chl a and total Chls) decreased with the application of 100 mM NaCl (Table 1). No differences were found for leaf total phenolics (averaged in 18.77 mg GAE/g Fw) and antioxidant activity (averaged in 7.48, 4.46, and 2.29 mg trolox/g Fw for FRAP, DPPH, and ABTS, respectively) between control and saline plants (data not shown).
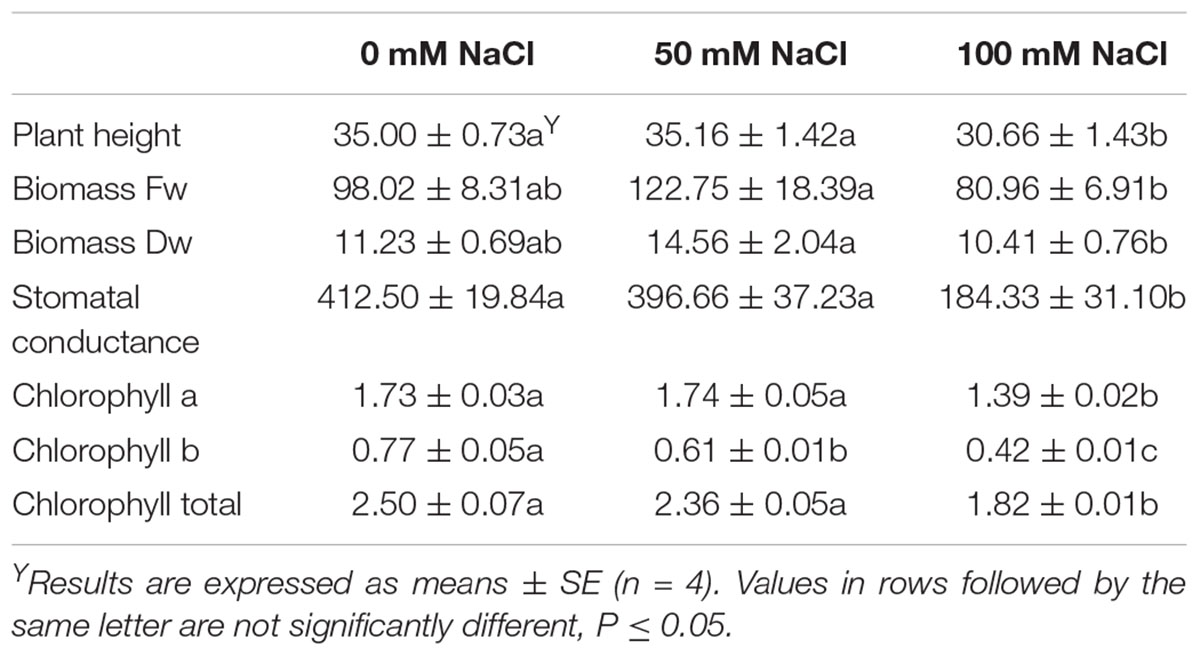
Table 1. Effect of salinity levels (0, 50, and 100 mM NaCl) on tagetes plant height (cm), biomass fresh and dry weight (Fw, Dw; g/plant), leaf stomatal conductance (mmol/m2/s), leaf Chlorophyll a (Chla; mg/g Fw), Chlorophyll b (Chlb; mg/g Fw), total Chlorophylls (total Chl; mg/g Fw) in plants grown hydroponically.
Considering the flowers produced by tagetes plants, salinity treatment increased several flowers physiological parameters tested in this study (Table 2). Total phenolics and carotenoids as well as antioxidant activities (determined by FRAP, DPPH, and ABTS methods) increased with the application of salinity, especially at the high levels of 100 mM NaCl. The opposite was evident concerning the anthocyanins content as salinity decreased the anthocyanins up to 65%, compared with the control treatment (Table 2). No differences were found in flower Chroma, and L, a∗, and b∗ color values (Supplementary Table S1).
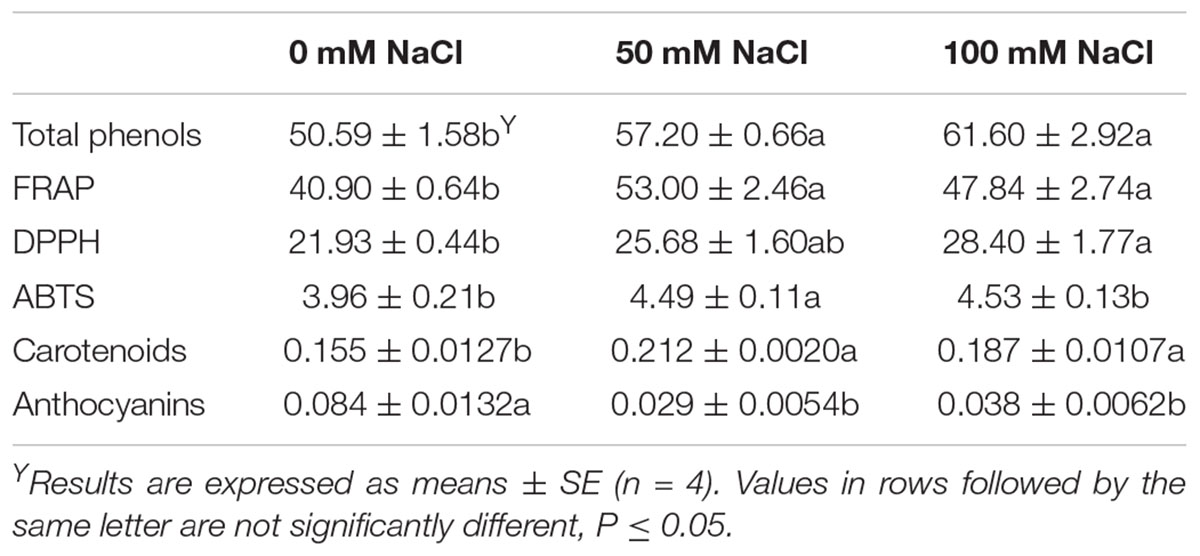
Table 2. Effect of salinity levels (0, 50, and 100 mM NaCl) on tagetes flowers total phenolics (μmol GAE/g Fw), antioxidant activity (FRAP, DPPH, ABTS: mg trolox/g Fw), carotenoids (mg/g Fw), and anthocyanins (mg cyn-3-glu/g Fw) in plants grown hydroponically.
Considering that H2O2 production indicates an induction of salinity stress, the greatest production was found in tagetes flowers derived from 100 mM NaCl-stressed plants, followed by the once subjected to salinity of 50 mM NaCl (Table 3). Salinity increased the production of H2O2 and proline content but decreased the APX activity. Low saline levels of 50 mM NaCl increased the activity of both CAT and POD while high salinity of 100 mM NaCl had similar levels to the control for both enzymes’ activities. No differences were found concerning lipid peroxidation and SOD activities among treatments (Table 3).
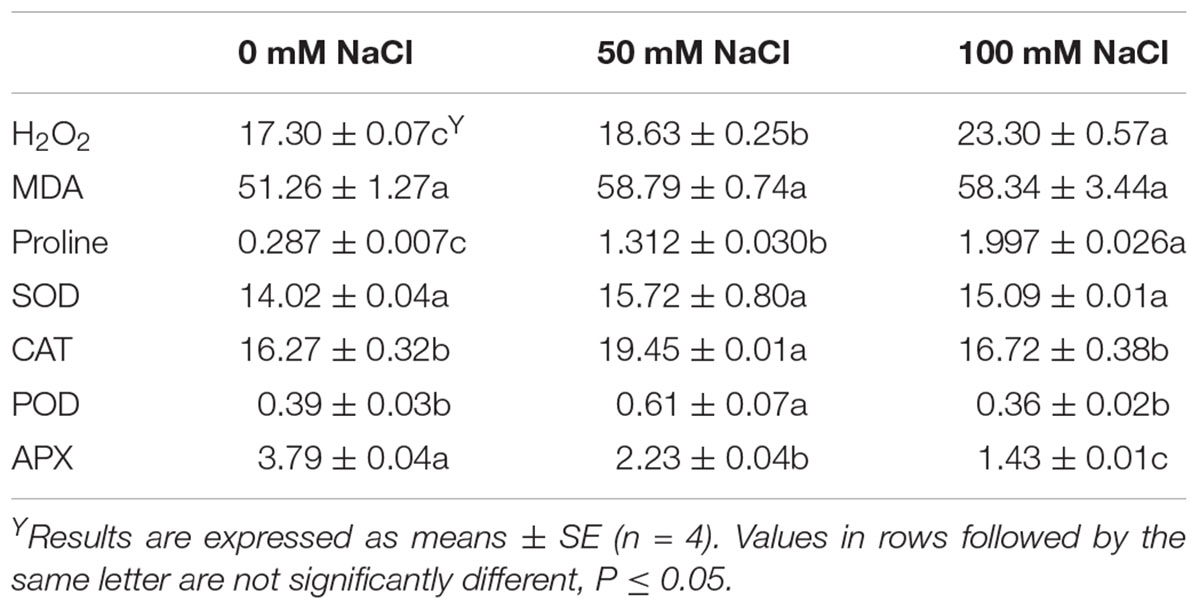
Table 3. Effect of salinity levels (0, 50, and 100 mM NaCl) on tagetes flowers on the lipid peroxidation (MDA; nmol/g Fw), hydrogen peroxide production (H2O2; μmol/g Fw), proline content (μg/g Fw), and antioxidant enzymes activities (SOD, CAT, POD, APX in units/mg protein) in plants grown hydroponically.
Leaf and Flower Mineral Content
Mineral content in leaves and flowers is presented in Table 4, with effects of salinity to be mainly noticed on the flowers rather than the leaves. Therefore, in flowers, increased salinity levels resulted in increased (P < 0.05) Na and N content and decreased Fe content. Flowers accumulated more P and Zn when the tagetes plants were subjected to both salinity levels when compared to the control plants. Salinity of 100 mM NaCl decreased (P < 0.05) the Mg content in flowers. The levels of K and Cu did not vary among the treatments. Interestingly, salinity of 50 mM NaCl accumulated Ca in flowers almost three times compared to control treatment (non-saline treated plants) (Table 4).
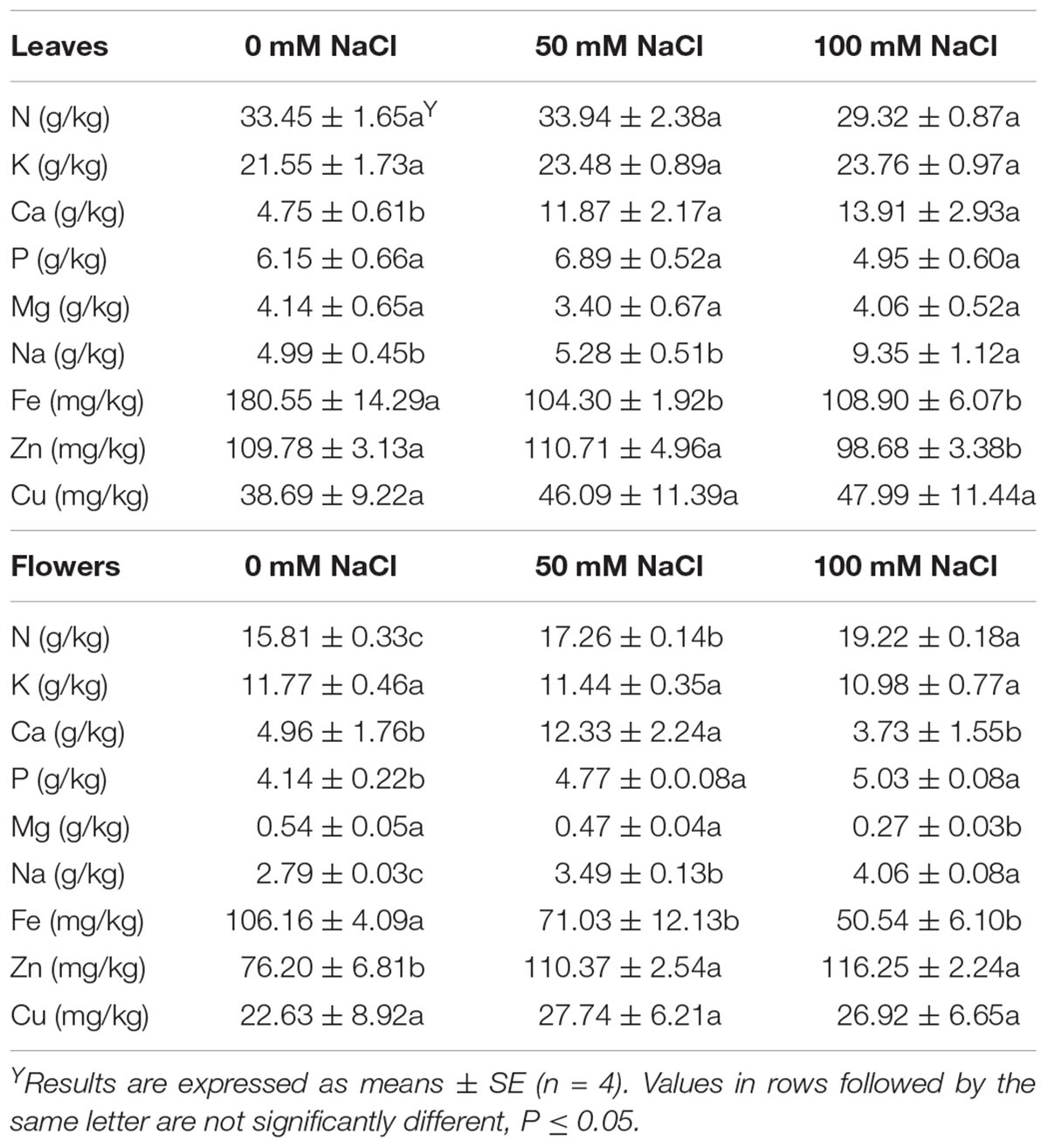
Table 4. Effect of salinity levels (0, 50, and 100 mM NaCl) on tagetes leaves and flowers minerals in plants grown hydroponically.
In the case of tagetes leaves, salinity of 100 mM NaCl accumulated (P < 0.05) more Na but less Zn when compared to low (50 mM NaCl) salinity or control treatment (Table 4). Salinity in both low and high levels accumulated Ca in leaves but had lesser Fe content when compared with the non-saline treated plants. No differences were found for N, K, P, Mg, and Cu content among treatments.
Edible Flower Processing and Shelf-Life Parameters
The levels of CO2 accumulated in the head space of saline and/or ethanol processed tagetes edible flowers, which is depending on the respiration rates, varied according to time of storage as well as the treatments applied (Figure 1). Tagetes flowers obtained by saline-treated plants immediately after harvest had no significant differences in CO2 concentration inside the trays, despite the increase trend of CO2 concentration as the salinity levels were increased. Following storage up to 14 days, salinity did not have any effect on CO2 concentration. Interestingly, the vapor application of ethanol increased CO2 concentration in trays derived by flowers respiration during storage, compared to untreated ethanol flowers. This increase of flowers respiration was greater in plants grown at 100 mM NaCl at 7 days compared to 50 mM NaCl and control treatments.
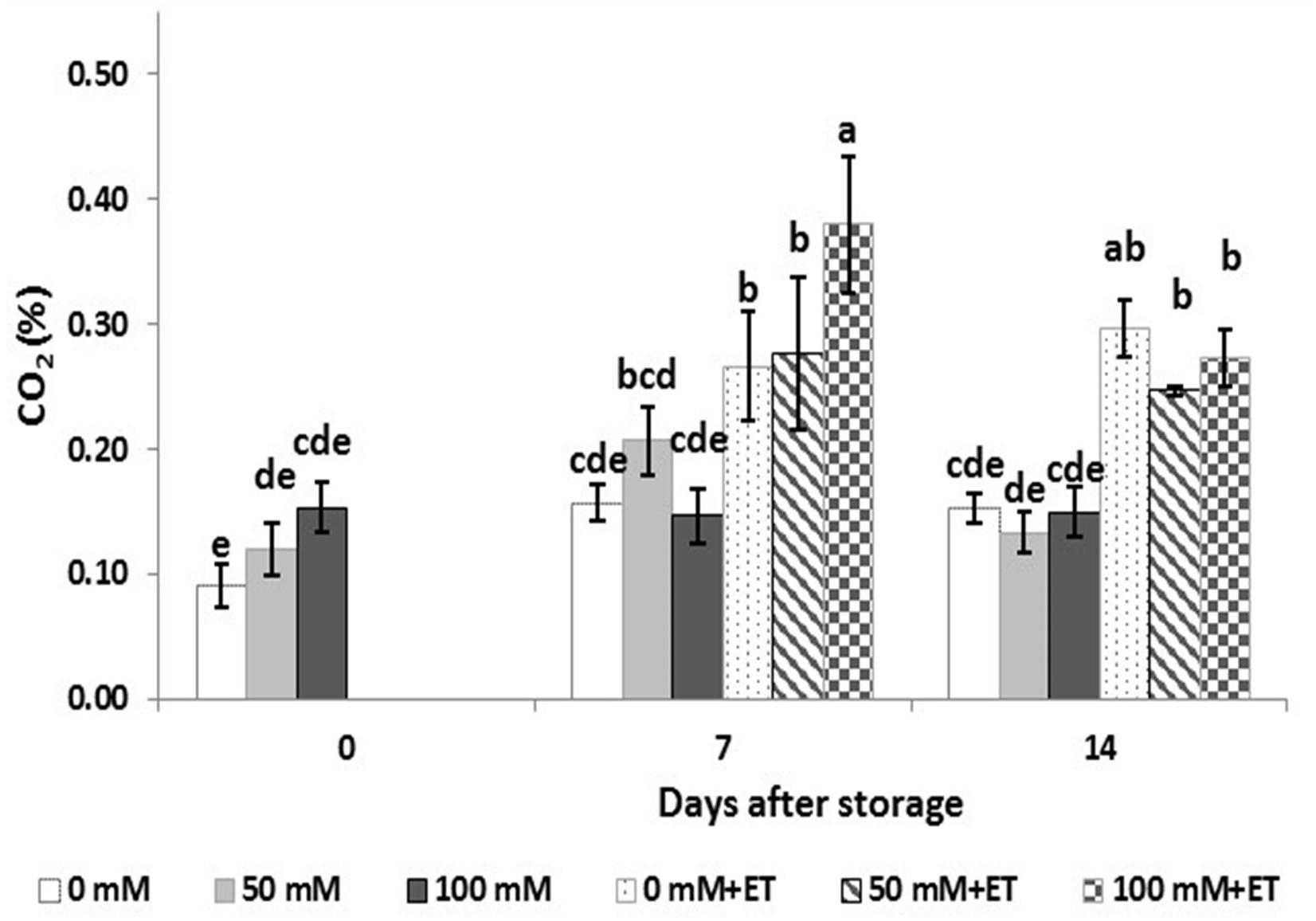
Figure 1. Effect of salinity levels (0-50-100 mM NaCl) on greenhouse-grown tagetes flowers in modified atmosphere packaging (with or without vaporized ethanol-ET) on the respiration of treated flowers during 7 and 14 days of storage at 5°C. Significant differences (P < 0.05) among treatments are indicated by different letters. Error bars show SE (n = 4).
No differences were found on flower color indicated as Chroma (ranged from 83.44 to 94.79), L (ranged from 48.23 to 56.72), a∗ (ranged from 35.99 to 43.13), and b∗ (ranged from 71.86 to 86.11) color values on flowers during postharvest storage (Supplementary Table S2).
Weight loss significantly (P < 0.05) increased at salinity of 100 mM NaCl during storage (Figure 2A), as on days 7 and 14 weight loss of tagetes reached 12.48%, and 19.64%, respectively. Ethanol application increased water loss in both control and 50 mM saline-treated flowers (Figure 2A).
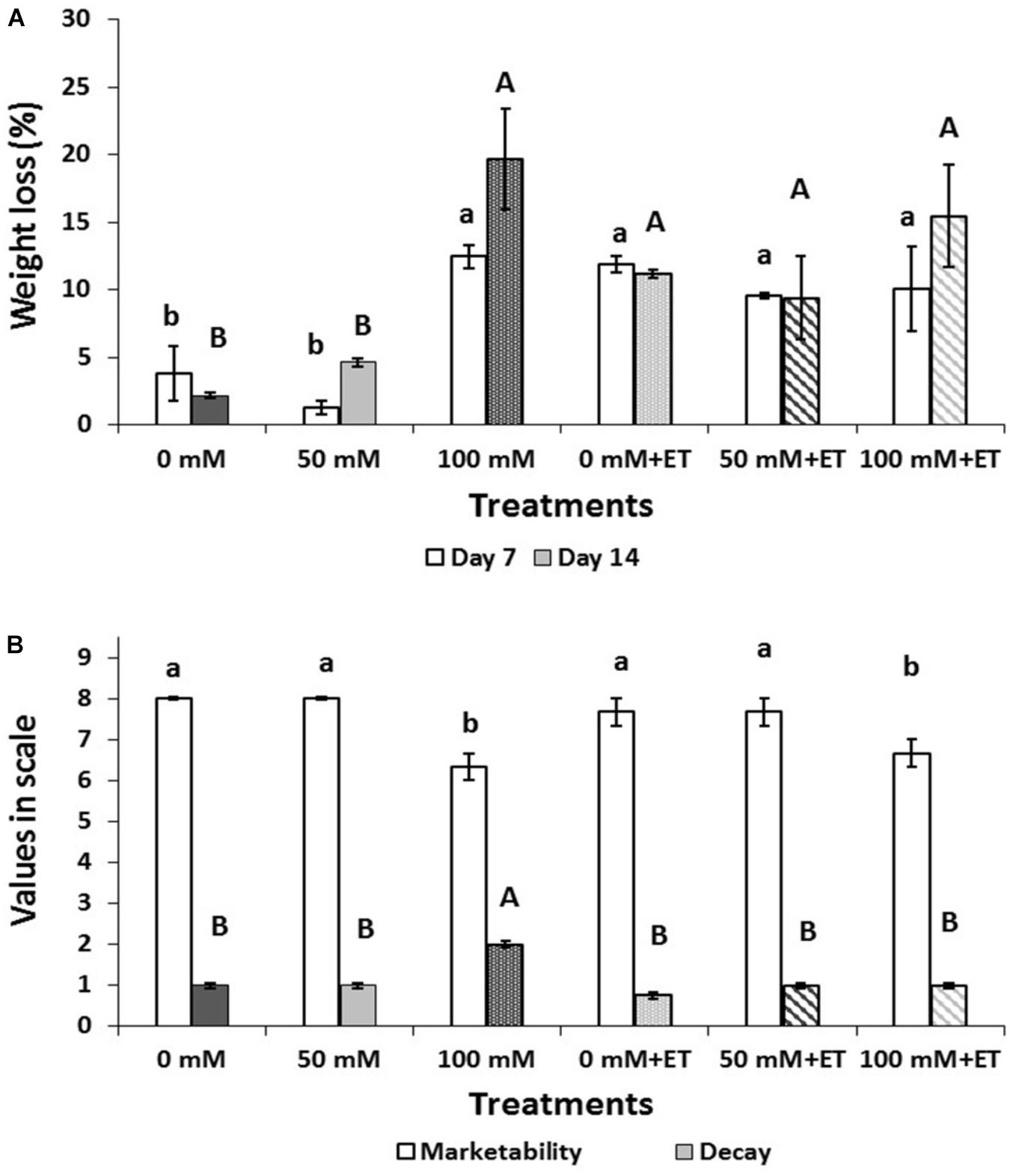
Figure 2. Effect of salinity levels (0-50-100 mM NaCl) on greenhouse-grown tagetes flowers in modified atmosphere packaging (with or without vaporized ethanol-ET) on the (A) weight loss and (B) marketability (scale 1–9) and decay (scale 1–4) of treated flowers during 7 and 14 days of storage at 5°C. Significant differences (P < 0.05) among treatments are indicated by different letters. Error bars show SE (n = 4).
Flower marketability and decay after 14 days of storage are presented in Figure 2B. Therefore, the marketability of flowers decreased (P < 0.05) when plants were grown at high (100 mM NaCl) saline levels, while ET itself did not change the marketability of the edible flowers. Moderate decay up to 50% was found in 100 mM NaCl-treated flowers. Interestingly enough, ET application alleviated the induced decay found in 100 mM NaCl-treated flowers to levels similar to the relevant control treatment (0 mM NaCl+ET).
Total phenolics were reduced in salinity of 100 mM NaCl with ethanol compared to non-saline harvested flowers exposed to ET vapors (0 mM NaCl+ET), following 14 days of storage at 5°C (Figure 3A). Antioxidant activities decreased mainly due to the increased saline levels, storage duration and/or ET vapor as assayed by DPPH, FRAP, and ABTS methods (Figures 3B–D). The content of carotenoids increased due to saline- and ET-application as well as the storage period (7 days versus 14 days of storage) (Figure 3E). Thus, carotenoids increased with the application of low (50 mM NaCl) salinity compared to the control treatment following 7 days of storage. The highest anthocyanins content was found in flowers treated with salinity of 100 mM NaCl and stored for 7 and 14 days (Figure 3F) while ET-vaporized flowers revealed low content of anthocyanins compared to the non-vaporized flowers.
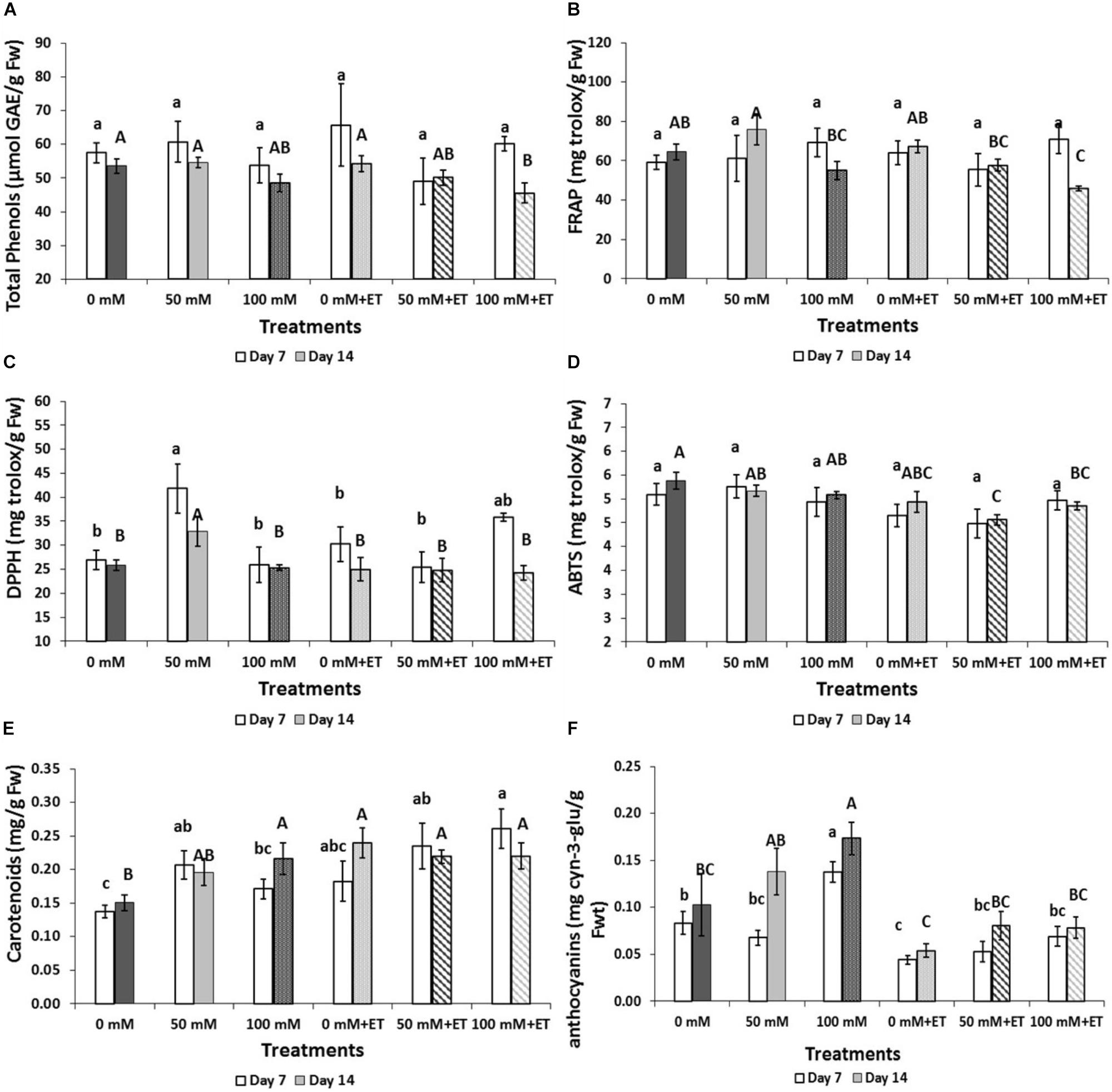
Figure 3. Effect of salinity levels (0-50-100 mM NaCl) on greenhouse-grown tagetes flowers in modified atmosphere packaging (with or without vaporized ethanol-ET) during storage at 5°C on the content of total phenols, carotenoids, anthocyanins, and antioxidant activity. (A) Total phenols, (B) FRAP, (C) DPPH, (D) ABTS (E) carotenoids, and (F) anthocyanins. Significant differences (P < 0.05) among treatments are indicated by different small or capital letters for days 7 and 14, respectively. Error bars show SE (n = 4).
Salinity of 100 mM NaCl increased lipid peroxidation as measured by MDA concentration after 7 days of storage while ET caused less increase in MDA (Figure 4A). After 14 days of storage, MDA decreased in salinity of 100 mM NaCl or in ET non-saline treated flowers. The production of H2O2 increased with the application of ET after 7 days of storage (Figure 4B). Indeed, ET combination with salinity (50 mM NaCl+ET and 100 mM NaCl+ET) revealed H2O2 decreases to similar levels as the 50 mM NaCl.
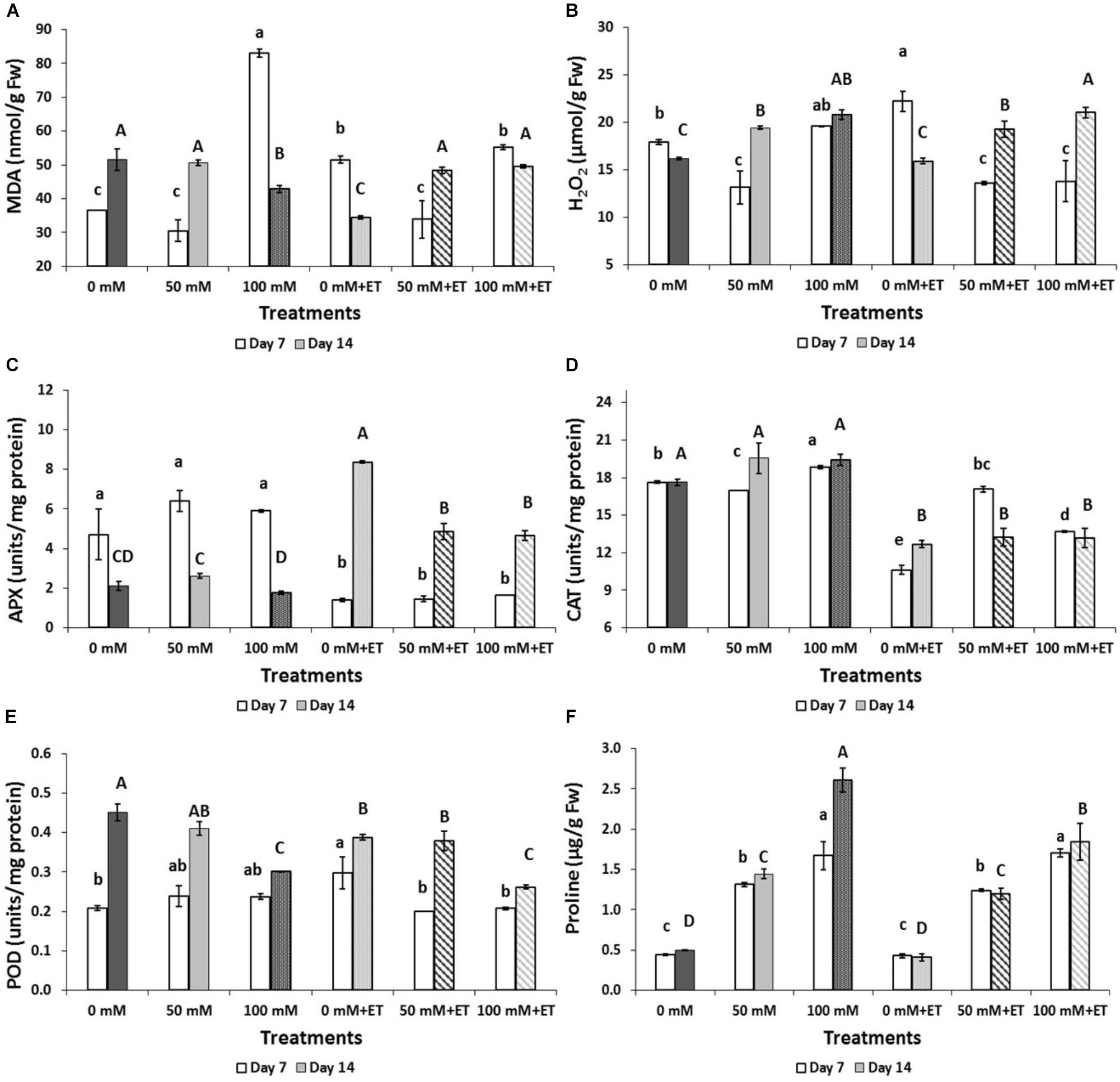
Figure 4. Effect of salinity levels (0-50-100 mM NaCl) on greenhouse-grown tagetes flowers in modified atmosphere packaging (with or without vaporized ethanol-ET) during storage at 5°C on the flower damage index, proline, and antioxidant enzymes activities on greenhouse-grown tagetes. (A) Lipid peroxidation (MDA), (B) H2O2, (C) APX, (D) CAT, (E) POD, (F) proline. Significant differences (P < 0.05) among treatments are indicated by different small or capital letters for Day 7 and 14, respectively. Error bars show SE (n = 4).
Following 7 days of storage, salinity of 100 mM NaCl increased the CAT activity and proline content (Figures 4D,F) while no differences were found in APX and POD activities (Figures 4C,E). At 14 days of storage, salinity of 100 mM NaCl decreased POD activity and increased proline content whereas APX and CAT activities remained at similar levels to the control treatment (non-saline flowers) (Figures 4C–F).
ET application, following 7 days of storage, decreased both APX and CAT but increased proline content (Figures 4C,D,F) and this effect persisted till the 14th day for CAT and proline. The application of ET in saline-treated flowers decreased POD and increased CAT and proline content after 7 days of storage while APX was reduced after 14 days of storage with the salinity+ET combination (Figures 4C–F). No differences were found in SOD activity among treatment and/or days of storage (data not shown).
Discussion
The present study investigated the impacts of saline levels on plant growth and physiology, mineral content and storability of tagetes edible flowers. In addition, ethanol application was tested under passive modified atmospheric packaging as a well-known means of postharvest preservation of fresh produce (Tzortzakis and Economakis, 2007). Cut flowers are the reproductive organs of a plant and they are more sensitive to deterioration and tissue break down than the relevant plant vegetative parts, i.e., leaves and stems (Serek and Reid, 2000). Edible flowers are cut off the stem at the pedicel and, therefore, they are more sensitive and exposed to additional stress. However, edible flowers had previously received less attention than cut flowers and even lesser attention than fresh fruits and vegetables due to their low production and market interest (Kou et al., 2012).
Plants exposed to saline conditions often demonstrate a decrease in the ability to absorb water and minerals causing rapid reduction in growth and yield and inducing several metabolic changes (Hendawy and Khalid, 2005; Chrysargyris et al., 2018). Salt stress is complex and leads to the formation of ROS such as singlet oxygen (O21), superoxide (O2-) and hydrogen peroxide (H2O2) with harmful effects on biomolecules (lipids, proteins, nucleic acids) due to oxidative damage (Gill and Tuteja, 2010; Chrysargyris et al., 2018). In the current study, 100 mM NaCl significantly (P < 0.05) reduced plant biomass and plant size (i.e., height) and affected physiological processes negatively. Therefore, plants closed the leaf stomata to overcome salinity stress and at the same time salinity caused osmotic stress and water deficiency, which was determined by the stomatal conductance reductions. Stomatal conductance reduction is an adaptation plant mechanism to salinity stress and has been reported in Plandago spp. (Izadi-Darbandi and Mehdikhani, 2018) and Calendula officinalis (Khalid and Teixeira da Silva, 2010). The reduction in growth is also related to the decreased content of chlorophylls as observed at 100 mM NaCl application, which can be one of the factors for the photosynthetic rates decrease (Kiarostami et al., 2010). Plant growth and chlorophylls content reductions under saline conditions have been reported for several species (Tarchoune et al., 2010; Chondraki et al., 2012; Klados and Tzortzakis, 2014; Chrysargyris et al., 2018). Chaparzadeh et al. (2004) reported plant biomass reduction in C. officinalis subjected to salinity of 100 mM NaCl on a long term basis while Villarino and Mattson (2011) observed such reductions at the T. patula. Tagetes plants subjected to saline conditions exhibited an Fv/Fm ratio higher than 0.80, indicating absence of severe stress (Bjorkman and Demmig, 1987), as this is related to the short-term exposure to salinity. Indeed, the application of short-term saline stress (10 days) did not change the Chroma and L, a∗, and b∗ color values of the flowers. Therefore, visually flowers maintained their marketability value on chroma related parameters.
Plants cope with salinity induced stress by altering metabolic processes and stimulating the formation of phenolics and antioxidant activity to scavenge free radicals and ions chelators (Balasundram et al., 2006). Examining polyphenols and antioxidant activities in leaves and flowers leads to the conclusion that the effects of salinity could be clearly seen only on the latter (i.e., flowers). Therefore, flowers polyphenols and antioxidant activities (determined by three antioxidant assays of FRAP, DPPH and ABTS) as well as carotenoids increased with the application of salinity, with more pronounced impacts at salinity of 100 mM NaCl. This indicates higher nutraceutical value for the edible flowers when antioxidants and carotenoids increased in flowers subjected to salinity stress. Similar findings were found by Chaparzadeh et al. (2004) at C. officinalis flowers. Considering that plants change metabolic processes due to salinity stress, changes first take place at the vegetative part (i.e., leaves) and then are followed by changes at the reproductive organs (i.e., flowers). Thus, unchanged polyphenols and antioxidants in leaves possibly indicate that any inductions as a reaction of the plant to overcome saline stress took place before the 10th day. The decrease of anthocyanins in flowers subjected to salinity could be correlated with the decrease in plant growth/development as several metabolic processes were slowed down, other pigments accumulation or that anthocyanins have been already involved at the antioxidative mechanisms of the plant and their content had been exhausted. However, further studies requited to that direction, as the effect of salinity on anthocyanins accumulation is varied. Borghesi et al. (2011) for example, showed an opposite behavior in anthocyanins accumulation in two tomato genotypes when subjected to salinity.
Tagetes flowers were richer in potassium (averaged in 11.40 mg/kg dry weight) than in sodium (averaged in 3.45 mg/kg dry weight), which is quite useful for preventing cardiovascular diseases. Short-term saline exposure of tagetes plants activated metabolic processes such as accumulation of minerals (such as N, P, and Zn) on edible flowers which is of great importance to human health. Zinc deficiency causes impaired growth, immune dysfunction, increased morbidity and mortality as well as abnormal neuro-behavioral development (Mayer et al., 2008). Nitrogen and phosphorus have a substantial role in several metabolic processes since phospholipids, as a constitute of nucleic acid, are involved in protein synthesis, DNA, RNA, and ATP (Rouached et al., 2010). Consumption of 12 g of dry T. patula in salads, for example, can provide the 25% of the daily needs of potassium for adults (Rop et al., 2012).
Salinity and/or ET application causes stress which benefits the accumulation of ROS. Plants develop scavenging mechanisms against ROS detoxification by increasing the activity of antioxidant enzymes, such as SOD, APX, CAT, and glutathione reductase (GR) (Foyer and Noctor, 2011). In the present study, tagetes flowers demonstrated activation of both non-enzymatic (i.e., proline content) and enzymatic mechanisms (CAT) to overcome ROS detoxification. High accumulation of proline in plant tissue is an important adaptive mechanism of salt tolerance as proline is regarded as a source of energy, carbon and nitrogen for the recovering tissues, by acting as a compatible solute in osmotic adjustment and reduces membrane oxidative damage (Hossain et al., 2014). Proline acts as an osmolyte and reduces the osmotic potential, thus reducing toxic ion uptake (Chrysargyris et al., 2018). CAT is valuable for the elimination of hydrogen peroxide in peroxisomes by oxidases involved in β-oxidation of fatty acids, photorespiration and purine catabolism (Garg and Manchanda, 2009). The decreased enzymatic activities of APX and POD or the unaffected SOD activity is probably indicating that either enzymes were not involved in H2O2 scavenging or enzymes activities have been spent for H2O2 capture and ROS in general at an earlier stage. SOD is used for plant primary detoxification and is later followed by APX and POD (Chrysargyris et al., 2018).
Very few studies have examined the storage conditions of edible flowers and a gap of knowledge is evidenced on the postharvest preservation of edible flowers (Kou et al., 2012). The storage period of edible flowers is usually limited within a couple of days so high-tech storage and shipping conditions are required to extent their shelf life and simultaneously reduce the deteriorated products waste. Moreover, crop cultivation in controlled manner, as soilless culture, can yield produce of high quality and possible storability. Modified atmospheric packaging is commonly used for fresh produce quality maintenance, prolonging shelf life, and decreasing the microbial load of perishable commodities (Tzortzakis, 2010; Kou et al., 2012). Fresh produce stored on MAP conditions usually demonstrates an increase in carbon dioxide and a decrease in oxygen concentration and this actually retards the produce respiration process. In the present study ethanol enhanced the flower metabolic processes as it increased CO2 production in tagetes flowers stored for 14 days, while salinity and/or storage period had no significant effects on it. Similarly, Kou et al. (2012) reported increased CO2 accumulation in edible carnations and snapdragons following 7 days of storage. Ethanol prevents a rise in respiration rate and autocatalytic ethylene production/action (Asoda et al., 2009).
The increase in CO2 production was followed by weight loss in flowers subjected to ET even from the 7 days of storage. Flower weight loss increased (up to two and eightfold) in 100 mM NaCl-treated plants following 7 and 14 days of storage, respectively. This affected the flower marketability as it was decreased (P < 0.05) when plants were grown at high (100 mM NaCl) saline levels while ET itself did not change the marketability of the flowers. Therefore, tagetes flowers did not benefit on marketability terms with the application of ET, as it was previous reported on fruits and vegetables, whereas ethanol can be used for fresh produce preservation (Pesis, 2005; Asoda et al., 2009). Even though ethanol did not improve flower marketability, ET application alleviated the induced decay found in 100 mM NaCl-treated flowers to levels similar to the relevant control treatment (0 mM NaCl+ET). The antimicrobial properties of ethanol have been reported in several commodities (Karabulut et al., 2004; Tzortzakis, 2010). The increased decay observed at salinity of 100 mM NaCl-treated flowers may be related to the increased respiration metabolism and weight loss of the flowers but also to the high moisture content inside the tagetes packaging, as strong condensation was evidenced.
During storage, flowers maintained their color as several indicators (Chroma, a∗, b∗, L) remained at similar levels. Therefore, neither saline levels nor ET-vapors affected the color of tagetes flowers which established the maintenance of marketability, and the possible utilization of salinity and/or ET for improve/maintain the edible flowers quality.
The content of polyphenols decreased in flowers subjected to stress of salinity of 100 mM NaCl with ethanol after 14 days of storage at chilled temperature of 5°C whereas flower antioxidant activity (assayed by DPPH, FRAP, and ABTS) decreased mainly due to increased saline levels, storage duration and/or ET vapor. Thus, flowers were gradually deprived of their ability to detoxify ROS after 14 days of storage and alternative processes such as carotenoids and flavonoids accumulation might have taken place. The stress caused by saline- and ET-application resulted in increased content of carotenoids whereas higher anthocyanins content was found in flowers treated with salinity of 100 mM NaCl and stored for 7 and 14 days. This is of great importance, as stressed flowers by saline and/or ET were able to achieve higher carotenoids and anthocyanins levels and be a new source of nutraceutical foods. El Kereamy et al. (2002) reported that ethanol triggers gene expression leading to accumulation of anthocyanins during berry ripening, when ethanol (5% v/v) was applied on Cabernet Sauvignon grape fruit at veraison stage. The increased consumer and market demand for plant-based products with high antioxidant status may acknowledge edible flowers subjected to short-term salinity stress as products of added value which could prevent oxidative damage in human health. Fresh produce consumption with antioxidants could prevent chronic diseases such as type-2 diabetes, cancer, cardiovascular, and neurodegenerative disorders (Petrova et al., 2016).
Conclusion
Edible flowers are rich in flavonoids, phenolics, and terpenes, exhibit biocidal activities and possess beneficial properties for human health. Unfortunately, in spite of their agronomic potential, the concept of eating flowers is still viewed with doubt. Considering that salinity maintained the flower quality and storability, despite the reduction in growth and productivity of saline-treated plants, producers can consider the use of saline water for irrigation needs as a short-time stress for tagetes crops. Edible flowers subjected to saline conditions accumulated minerals such as N, P, Na, and Zn. Short-term exposure to saline condition and/or ethanol vapor application trigger flower metabolic process (both non-enzymatic (i.e., proline content) and enzymatic mechanisms (catalase) to overcome stress) and resulted higher carotenoids and anthocyanins levels during storage. It is the activation of several important metabolic processes with higher antioxidant status of the edible flowers that can be considered as a source of nutraceutical foods. Further efforts are needed to improve the postharvest preservation of the flowers when subjected to saline-stress conditions.
Data Availability
All datasets generated for this study are included in the manuscript and the Supplementary Files.
Author Contributions
AC, AT, and NT designed and performed the experiments and physiological measurements. AC, PX, and AT performed the postharvest measurements. AC and NT analyzed the data and critically discussed the data. NT and AC prepared the manuscript. NT coordinated the research.
Conflict of Interest Statement
The authors declare that the research was conducted in the absence of any commercial or financial relationships that could be construed as a potential conflict of interest.
Acknowledgments
Thanks to Mrs. Maria Koutroumani (Ministry of Education, Research and Religious Affairs, Athens, Greece) for language editing.
Supplementary Material
The Supplementary Material for this article can be found online at: https://www.frontiersin.org/articles/10.3389/fpls.2018.01765/full#supplementary-material
FIGURE S1 | Fluctuation of drainage pH and EC (mS/cm) under different salinity levels (0, 50, and 100 mM NaCl) in hydroponically grown tagetes plants.
FIGURE S2 | Effect of salinity levels (0, 50, and 100 mM NaCl) on tagetes maximum quantum efficiency of PSII (Fv/Fm) in plants grown hydroponically.
TABLE S1 | Effect of salinity levels (0, 50, and 100 mM NaCl) on tagetes flowers color values (L, a∗, b∗, Chroma) in plants grown hydroponically. YResults are expressed as means±SE (n = 6). Values in rows followed by the same letter are not significantly different, P ≤ 0.05.
TABLE S2 | Effect of salinity levels (0, 50, and 100 mM NaCl), ethanol application (no ethanol, with ethanol) and storage period (7 and 14 days) on water loss (%), color (L, a∗, b∗ values), total phenolic content (mg GAE/g Fwt), antioxidants (mg trolox/g Fwt), carotenoids (mg/100 g Fw) and anthocyanins (mg cyn-3-glu/100 g Fw) on tagetes flowers during postharvest storage. ns, ∗, ∗∗, and ∗∗∗ indicate non-significant or significant differences at P ≤ 5%, 1%, and 0.1%, respectively, following two-way ANOVA.
References
Asoda, T., Terai, H., Kato, M., and Suzukia, Y. (2009). Effects of postharvest ethanol vapor treatment on ethylene responsiveness in broccoli. Postharvest Biol. Technol. 52, 216–220. doi: 10.1016/j.postharvbio.2008.09.015
Azevedo-Neto, A. D., Prisco, J. T., Enéas-Filho, J., Abreu, C. E. B., and Gomes-Filho, E. (2006). Effect of salt stress on antioxidative enzymes and lipid peroxidation in leaves and roots of salt-tolerant and salt sensitive maize genotypes. Environ. Exp. Bot. 56, 87–94. doi: 10.1016/j.envexpbot.2005.01.008
Balasundram, N., Sundram, K., and Samman, S. (2006). Phenolic compounds in plants and agri-industrial by-products: antioxidant activity, occurrence, and potential uses. Food Chem. 99, 191–203. doi: 10.1016/j.foodchem.2005.07.042
Begri, F., Hadavi, E., and Nabigol, A. (2014). Positive interaction of ethanol with malic acid in postharvest physiology of cut spray carnation ‘White Natila’. J. Hortic. Res. 22, 19–30. doi: 10.2478/johr-2014-0018
Bjorkman, O., and Demmig, B. (1987). Photon yield of O2 evolution and chlorophyll fluorescence characteristics at 77 K among vascular plants of diverse origins. Planta 170, 489–504. doi: 10.1007/BF00402983
Bolin, H. R., and Huxsoll, C. C. (1991). Effect of preparation procedures and storage parameters on quality retention of salad-cut lettuce. J. Food Sci. 56, 60–62. doi: 10.1111/j.1365-2621.1991.tb07975.x
Borghesi, E., González-Miret, M. L., Escudero-Gilete, M. L., Malorgio, F., Heredia, F. J., and Meléndez-Martínez, A. J. (2011). Effects of salinity stress on carotenoids, anthocyanins, and color of diverse tomato genotypes. J. Agric. Food Chem. 59, 11676–11682. doi: 10.1021/jf2021623
Chaparzadeh, N., D’Amico, M. L., Khavari-Nejad, R. A., Izzo, R., and Navari-Izzo, F. (2004). Antioxidative responses of Calendula officinalis under salinity conditions. Plant Physiol. Biochem. 42, 695–701. doi: 10.1016/j.plaphy.2004.07.001
Chondraki, S., Tzerakis, C., and Tzortzakis, N. (2012). Influence of NaCl and calcium foliar spray on hydroponically grown parsley in NFT system. J. Plant Nutr. 35, 1457–1467. doi: 10.1080/01904167.2012.689906
Chrysargyris, A., Michailidi, E., and Tzortzakis, N. (2018). Physiological and biochemical responses of Lavandula angustifolia to salinity under mineral foliar application. Front. Plant Sci. 9:489. doi: 10.3389/fpls.2018.00489
Chrysargyris, A., Panayiotou, C., and Tzortzakis, N. (2016). Nitrogen and phosphorus levels affected plant growth, essential oil composition and antioxidant status of lavender plant (Lavandula angustifolia Mill.). Ind. Crops Prod. 83, 577–586. doi: 10.1016/j.indcrop.2015.12.067
Chrysargyris, A., Xylia, P., Botsaris, G., and Tzortzakis, N. (2017). Antioxidant and antibacterial activities, mineral and essential oil composition of spearmint (Mentha spicata L.) affected by the potassium levels. Ind. Crops Prod. 103, 202–212. doi: 10.1016/j.indcrop.2017.04.010
Delaquis, P. J., Stewart, S., Toivonen, P. M. A., and Moyls, A. L. (1999). Effect of warm, chlorinated water on the microbial flora of shredded iceberg lettuce. Food Res. Int. 32, 7–14. doi: 10.1016/S0963-9969(99)00058-7
El Kereamy, A., Chervin, C., Souquet, J.-M., Moutounet, M., Monje, M.-C., Nepveu, F., et al. (2002). Ethanol triggers grape gene expression leading to anthocyanin accumulation during berry ripening. Plant Sci. 163, 449–454. doi: 10.1016/S0168-9452(02)00142-5
Foyer, C. H., and Noctor, G. (2011). Ascorbate and glutathione: the heart of the redox hub. Plant Physiol. 155, 2–18. doi: 10.1104/pp.110.167569
Friedman, H., Agami, O., Vinokur, Y., Droby, S., Cohen, L., Refaeli, G., et al. (2010). Characterization of yield, sensitivity to Botrytis cinerea and antioxidant content of several rose species suitable for edible flowers. Sci. Hortic. 123, 395–401. doi: 10.1016/j.scienta.2009.09.019
Fu, M. R., and Mao, L. C. (2008). In vitro antioxidant activities of five cultivars of daylily flowers from China. Nat. Prod. Res. 22, 584–591. doi: 10.1080/14786410701592828
Gakuubi, M. M., Wanzala, W., Wagacha, J. M., and Dossaji, S. F. (2016). Bioactive properties of Tagetes minuta L. (Asteraceae) essential oils: a review. Am. J. Essent. Oil Nat. Prod. 4, 27–36.
Garg, N., and Manchanda, G. (2009). ROS generation in plants: boon or bane? Plant Biosys. 143, 81–96. doi: 10.1080/11263500802633626
Giarratana, F., Muscolino, D., Ziino, G., Giuffrida, A., Marotta, S.M., Presti, V. L., et al. (2017). Activity of tagetes minuta linnaeus (asteraceae) essential oil against L3 Anisakis larvae type 1. Asian Pac. J. Trop. Med. 10, 461–465. doi: 10.1016/j.apjtm.2017.05.005
Gill, S. S., and Tuteja, N. (2010). Reactive oxygen species and antioxidant machinery in abiotic stress tolerance in crop plants. Plant Physiol. Biochem. 48, 909–930. doi: 10.1016/j.plaphy.2010.08.016
Han, J. H., Tao, W. Y., Hao, H. K., Zhang, B. L., Jiang, W. B., Niu, T. G., et al. (2006). Physiology and quality responses of fresh-cut broccoli florets pretreated with ethanol vapor. J. Food Sci. 71, S385–S389. doi: 10.1111/j.1750-3841.2006.00042.x
Heins, R. D., and Blakely, N. (1992). Influence of ethanol on ethylene biosynthesis and flower senescence of cut carnation. Sci. Hortic. 13, 361–369. doi: 10.1016/0304-4238(80)90094-1
Hendawy, S. F., and Khalid, K. A. (2005). Response of sage (Salvia officinalis L.) plants to zinc application under different salinity levels. J. Appl. Sci. Res. 1, 147–155.
Hossain, M. A., Hoque, Md. A., Burritt, D. J., and Fujita, M. (2014). “Proline protects plants against abiotic oxidative stress: biochemical and Molecular Mechanisms,” in Oxidative Damage to Plants Antioxidant Networks and Signaling, ed. P. Ahmad (Amsterdam: Elsevier), 620.
Izadi-Darbandi, E., and Mehdikhani, H. (2018). Salinity effect on some of the morphophysiological traits of three plantago species (Plantago spp.). Sci. Hortic. 236, 43–51. doi: 10.1016/j.scienta.2018.01.059
Jain, R., Katare, N., Kumar, V., Amit Kumar, S., Swati, G., and Shrotri, C. K. (2012). In vitro antibacterial potential of different extracts of Tagetes erecta and Tagetes patula. J. Nat. Sci. Res. 2, 84–90.
Jiang, M., and Zhang, J. (2002). Involvement of plasma membrane NADPH oxidase in abscisic acid- and water stress-induced antioxidant defense in leaves of maize seedlings. Planta 215, 1022–1030. doi: 10.1007/s00425-002-0829-y
Kaisoon, O., Konczak, I., and Siriamornpun, S. (2012). Potential health enhancing properties of edible flowers from Thailand. Food Res. Int. 46, 563–571. doi: 10.1016/j.foodres.2011.06.016
Karabulut, O. A., Mlikota-Gabler, F., Mansour, M., and Smilanick, J. L. (2004). Postharvest ethanol and hot water treatments of table grapes to control gray mold. Postharvest Biol. Technol. 34, 169–177. doi: 10.1016/j.postharvbio.2004.05.003
Kaur, P., and Mukherjee, D. (2013). Senescence regulation by alcohols in cut flowers of Calendula officinalis L. Acta Physiol. Plant. 35, 1853–1861. doi: 10.1007/s11738-013-1223-z
Kelley, K. M., Cameron, A. C., Biernbaum, J. A., and Poff, K. L. (2003). Effect of storage temperature on the quality of edible flowers. Postharvest Biol. Technol. 27, 341–344. doi: 10.1016/S0925-5214(02)00096-0
Khalid, K. A., Teixeira, and da Silva, J. A. (2010). Yield, essential oil and pigment content of Calendula officinalis L. flower heads cultivated under salt stress conditions. Sci. Hortic. 126, 297–305. doi: 10.1016/j.scienta.2010.07.023
Khedr, A. A., Abbas, M. A., Wahid, A. A., Quick, W. P., and Abogadallah, G. A. (2003). Proline induces the expression of salt-stress-responsive proteins and may improve the adaptation of Pancratium maritimum L. to salt-stress. J. Exp. Bot. 54, 2553–2562. doi: 10.1093/jxb/erg277
Kiarostami, K. H., Mohseni, R., and Saboora, A. (2010). Biochemical changes of Rosmarinus officinalis under salt stress. J. Stress Physiol. Biochem. 6, 114–122.
Klados,E., and Tzortzakis, N. (2014). Effects of substrate and salinity in hydroponically grown Cichorium spinosum. J. Soil Sci. Plant Nutr. 14, 211–222. doi: 10.4067/S0718-95162014005000017
Kou, L., Turner, E. R., and Luo, Y. (2012). Extending the shelf life of edible flowers with controlled released of 1-methylcyclopropene and modified atmosphere packaging. J. Food Saf. 77, 188–193. doi: 10.1111/j.1750-3841.2012.02683.x
Loizzo, M. R., Pugliese, A., Bonesi, M., Tenuta, M. C., Menichini, F., Xiao, J., et al. (2015). Edible flowers: a rich source of phytochemicals with antioxidant and hypoglycemic properties. J. Agric. Food Chem. 64, 2467–2474. doi: 10.1021/acs.jafc.5b03092
Loreto, F., and Velikova, V. (2001). Isoprene produced by leaves protects the photosynthetic apparatus against ozone damage, quenches ozone products, and reduces lipid peroxidation of cellular membranes. Plant Physiol. 27, 1781–1787. doi: 10.1104/pp.010497
Marinou, E., Chrysargyris, A., and Tzortzakis, N. (2013). Use of sawdust, coco soil and pumice in hydroponically grown strawberry. Plant Soil Environ. 59, 452–459. doi: 10.17221/297/2013-PSE
Mato, M., Onazaki, T., Ozeki, Y., Higeta, D., Itoh, Y., Yoshimoto, Y., et al. (2000). Flavonoid biosynthesis in white flowered sim carnations (Dianthus caryophyllus). Sci. Hortic. 84, 333–347. doi: 10.1016/S0304-4238(99)00140-5
Mayer, J. E., Pfeiffer, W. H., and Beyer, P. (2008). Biofortified crops to alleviate micronutrient malnutrition. Curr. Opin. Plant Biol. 11, 166–170. doi: 10.1016/j.pbi.2008.01.007
Mlcek, J., and Rop, O. (2011). Fresh edible flowers of ornamental plants- A new source of nutraceutical foods. Trends Food Sci. Technol. 22, 651–569. doi: 10.1016/j.tifs.2011.04.006
Munhoz, V. M., Longhini, R., Souza, J. R. P., Zequi, J. A. C., Mello, E. V. S. L., Lopes, G. C., et al. (2014). Extraction of flavonoids from Tagetes patula: process optimization and screening for biological activity. Rev. Bras. Farmacogn. 24, 576–583. doi: 10.1016/j.bjp.2014.10.001
Munns, R. (2002). Comparative physiology of salt and water stress. Plant Cell Environ. 25, 239–250. doi: 10.1046/j.0016-8025.2001.00808.x
Perich, M., Wells, C., Bertsch, W., and Tredway, K. (1995). Isolation of the insecticidal components of Tagetes minuta (Compositae) against mosquito larvae and adults. J. Am. Mosq. Control Assoc. 11, 307–310.
Pesis, E. (2005). The role of the anaerobic metabolites, acetaldehyde and ethanol, in fruit ripening, enhancement of fruit quality and fruit deterioration. Postharvest Biol. Technol. 37, 1–19. doi: 10.1016/j.postharvbio.2005.03.001
Petrova, I., Petkova, N., and Ivanov, I. (2016). Five edible flowers – Valuable source of antioxidants in human nutrition. Int. J. Pharmacogn. Phytochem. Res. 8, 604–610.
Rachuonyo, H., Ogola, P., Arika, W., Wambani, J., and Gatheri, G. (2016). Combined effect of crude leaf extracts of selected medicinal plants against selected enteric bacterial pathogens and Candida albicans. J. Antimicrob Agents 2:110. doi: 10.4172/2472-1212.1000110
Rafiq, S. K., Ganai, B. A., and Bhat, G. A. (2008). Impact of automobile emissions on the productivity of Crocus sativus L. Int. J. Environ. Res. 2, 371–376.
Ragaert, P., Devlieghere, F., and Debevere, J. (2007). Role of microbiological and physiological spoilage mechanisms during storage of minimally processed vegetables. Postharvest Biol. Technol. 44, 185–194. doi: 10.1016/j.postharvbio.2007.01.001
Rop, O., Mlcek, J., Jurikova, T., Neugebauerova, J., and Vabkova, J. (2012). Edible flowers –a new promising source of mineral elements in human nutrition. Molecules 17, 6672–6683. doi: 10.3390/molecules17066672
Rouached, H., Arpat, A. B., and Poirier, Y. (2010). Regulation of phosphate starvation responses in plants: signaling players and cross-talks. Mol. Plant 3, 288–299. doi: 10.1093/mp/ssp120
Serek, M., and Reid, M. (2000). Ethylene and postharvest performance of potted kalanchoe. Postharvest Biol. Technol. 18, 43–48. doi: 10.1016/S0925-5214(99)00055-1
Tarchoune, I., Sgherri, C., Izzo, R., Lachaal, M., Navari-Izzo, F., and Ouerghi, Z. (2012). Changes in the antioxidative systems of Ocimum basilicum L. (cv. Fine) under different sodium salts. Acta Physiol. Plant. 34, 1873–1881. doi: 10.1007/s11738-012-0985-z
Tarchoune, I., Sgherri, C., Izzo, R., Lachaâl, M., Ouerghi, Z., and Navari-Izzo, F. (2010). Antioxidant response of Ocimum basilicum to sodium chloride or sodium sulphate salinization. Plant Physiol. Biochem. 48, 772–777. doi: 10.1016/j.plaphy.2010.05.006
Tzortzakis, N. G. (2010). Ethanol, vinegar and Origanum vulgare oil vapour suppress the development of anthracnose rot in tomato fruit. Int. J. Food Microbiol. 142, 14–18. doi: 10.1016/j.ijfoodmicro.2010.05.005
Tzortzakis, N. G., and Economakis, C. D. (2007). Maintaining postharvest quality of the tomato fruit by employing methyl jasmonate and ethanol vapor treatment. J. Food Qual. 30, 567–580. doi: 10.1111/j.1745-4557.2007.00143.x
Upadhyay, R. K. (2011). Kareel plant: a natural source of medicines and nutrients. Int. J. Green Pharm. 5, 255–265.
Villarino, G. H., and Mattson, N. S. (2011). Assessing tolerance to sodium chloride salinity in fourteen floriculture species. HortTechnology 21, 539–545.
Wojdylo, A., Oszmiański, J., and Czemerys, R. (2007). Antioxidant activity and phenolic compounds in 32 selected herbs. Food Chem. 105, 940–949. doi: 10.1016/j.foodchem.2007.04.038
Keywords: edible flowers, tagetes, Tagetes patula, antioxidant capacity, shelf-life, hydroponics, nutraceutical foods
Citation: Chrysargyris A, Tzionis A, Xylia P and Tzortzakis N (2018) Effects of Salinity on Tagetes Growth, Physiology, and Shelf Life of Edible Flowers Stored in Passive Modified Atmosphere Packaging or Treated With Ethanol. Front. Plant Sci. 9:1765. doi: 10.3389/fpls.2018.01765
Received: 13 October 2018; Accepted: 14 November 2018;
Published: 10 December 2018.
Edited by:
Stefania De Pascale, Università degli Studi di Napoli Federico II, ItalyReviewed by:
María Serrano, Universidad Miguel Hernández de Elche, SpainGiacomo Cocetta, Università degli Studi di Milano, Italy
Copyright © 2018 Chrysargyris, Tzionis, Xylia and Tzortzakis. This is an open-access article distributed under the terms of the Creative Commons Attribution License (CC BY). The use, distribution or reproduction in other forums is permitted, provided the original author(s) and the copyright owner(s) are credited and that the original publication in this journal is cited, in accordance with accepted academic practice. No use, distribution or reproduction is permitted which does not comply with these terms.
*Correspondence: Antonios Chrysargyris, YS5jaHJ5c2FyZ3lyaXNAY3V0LmFjLmN5 Nikos Tzortzakis, bmlrb2xhb3MudHpvcnR6YWtpc0BjdXQuYWMuY3k=