- Institute of Plant Science and Resources (IPSR), Okayama University, Okayama, Japan
Protein homeostasis in the thylakoid membranes is dependent on protein quality control mechanisms, which are necessary to remove photodamaged and misfolded proteins. An ATP-dependent zinc metalloprotease, FtsH, is the major thylakoid membrane protease. FtsH proteases in the thylakoid membranes of Arabidopsis thaliana form a hetero-hexameric complex consisting of four FtsH subunits, which are divided into two types: type A (FtsH1 and FtsH5) and type B (FtsH2 and FtsH8). An increasing number of studies have identified the critical roles of FtsH in the biogenesis of thylakoid membranes and quality control in the photosystem II repair cycle. Furthermore, the involvement of FtsH proteolysis in a singlet oxygen- and EXECUTER1-dependent retrograde signaling mechanism has been suggested recently. FtsH is also involved in the degradation and assembly of several protein complexes in the photosynthetic electron-transport pathways. In this minireview, we provide an update on the functions of FtsH in thylakoid biogenesis and describe our current understanding of the D1 degradation processes in the photosystem II repair cycle. We also discuss the regulation mechanisms of FtsH protease activity, which suggest the flexible oligomerization capability of FtsH in the chloroplasts of seed plants.
Introduction
Chloroplasts are the essential organelles of seed plants in which photosynthesis takes place. The biogenesis and functions of chloroplasts are dependent on protein homeostasis; therefore, protein quality control, which is orchestrated by the protein synthesis and degradation machinery, is an important process. Chloroplasts originated from a cyanobacterium through endosymbiosis. Thus, the prokaryotic machineries derived from the ancestral cyanobacterium appear to dominate most of the proteolysis mechanisms in chloroplasts. More than 18 kinds of chloroplast proteases have been identified (reviewed by van Wijk, 2015; Nishimura et al., 2016, 2017). Of these, two ATP-dependent proteases, Clp and FtsH, are considered to have major roles in chloroplast protein homeostasis on the basis of their physiological functions. Clp functions in the stroma as housekeeping machinery (reviewed by Clarke, 2012; Nishimura and van Wijk, 2015). In contrast, FtsH protease plays critical roles in the biogenesis of thylakoid membranes and the quality control of thylakoid membrane proteins (Figure 1). In this mini review, we describe the functions of FtsH in the quality control of thylakoid membrane proteins and recent findings pertaining to the functions and regulation of FtsH during photooxidative stress.
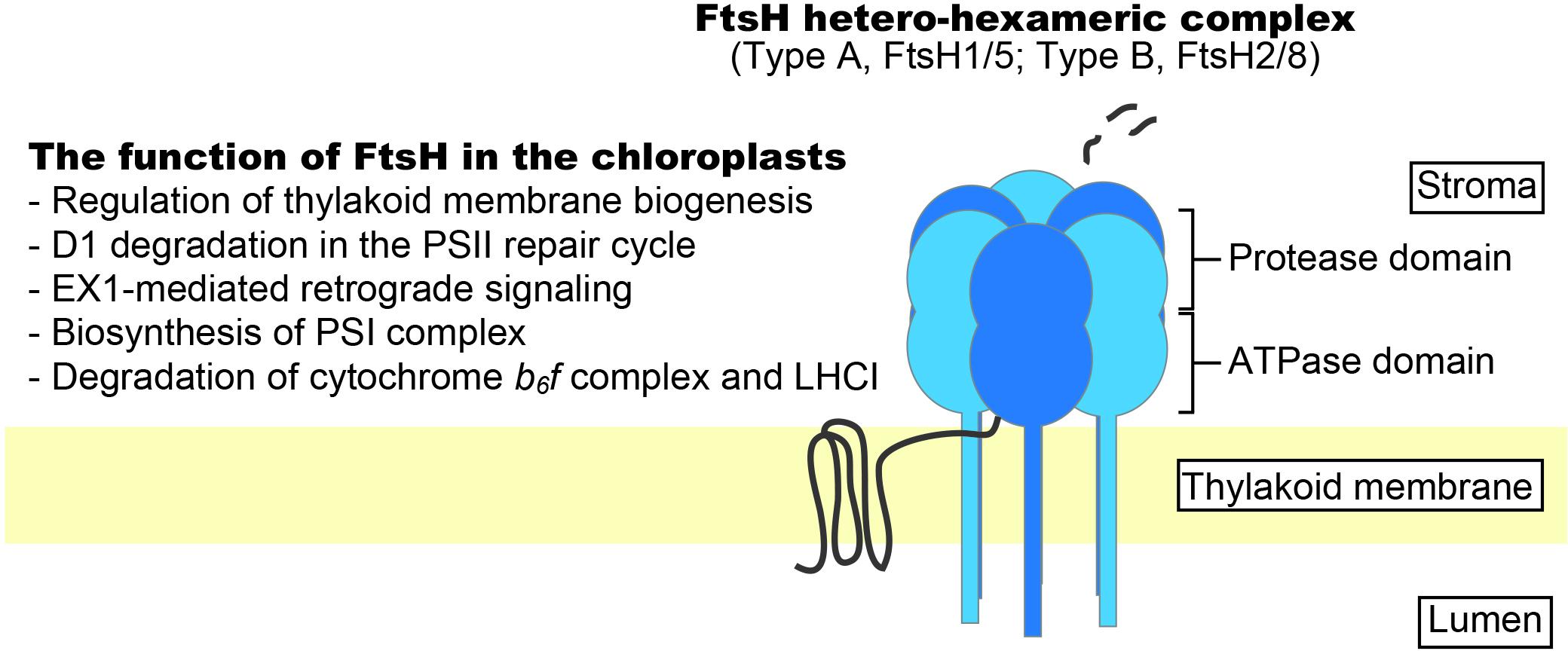
FIGURE 1. Schematic representation of FtsH protease complex in chloroplasts. Thylakoid FtsH forms a hetero-hexameric structure integrated into the thylakoid membrane. Increasing evidence shows the importance of FtsH in protein quality control in the thylakoid membrane.
Overview of FtsH Proteases in Chloroplasts
FtsH is an ATP-dependent zinc metalloprotease complex that belongs to the AAA (ATPase associated with diverse cellular activities) protease subfamily and is unique because it is membrane anchored (Ito and Akiyama, 2005). The ATPase domain of FtsH functions as an unfoldase and translocates substrates into the degradation chamber through a narrow pore (Krzywda et al., 2002; Niwa et al., 2002). Through this mode of action, FtsH pulls integral membrane proteins out of the membrane and degrades them. According to studies in Escherichia coli, more than 20 amino acid residues from either the N- or C-terminal end are required for substrate recognition (Chiba et al., 2000, 2002). Arabidopsis thaliana has 12 FtsH-encoding genes. Nine of the corresponding proteins are targeted to chloroplasts and three are localized to mitochondria (Sakamoto et al., 2003). Thylakoid-localized FtsHs possess an N-terminal transmembrane domain and a C-terminal region that extends into the stroma and contains the ATPase and protease domain (Lindahl et al., 1996). FtsHs in the thylakoid membrane form a hetero-hexameric complex consisting of two types of FtsH subunits (type A, FtsH1 and FtsH5; type B, FtsH2 and FtsH8); FtsH2 is the most abundant, followed by FtsH5, FtsH8, and FtsH1 (Sakamoto et al., 2003; Yu et al., 2004, 2005). Mutants lacking the more abundant subunits (i.e., FtsH2 and FtsH5) tend to show stronger phenotypes (e.g., leaf variegation and photosensitivity; see below). Although there is high sequence similarity between the type A and type B subunits, they are integrated into the thylakoid membranes via different pathways; the Sec (secretion) pathway integrates type A subunit FtsH5, whereas the Tat (twin-Arg translocation) pathway integrates type B subunit FtsH2 (Rodrigues et al., 2011). Mutants lacking FtsH2 are known as yellow variegated2 (var2) and show severe leaf variegation (Chen et al., 2000; Takechi et al., 2000). Mutants lacking FtsH5 (var1) show weak leaf variegation (Sakamoto et al., 2002), but leaf-variegation phenotypes are not observed in ftsh8 and ftsh1 mutants. Meanwhile, the double mutants ftsh1 ftsh5 and ftsh2 ftsh8 show an albino-like phenotype, suggesting that both types of subunits are required for active complex formation (Yu et al., 2004, 2005; Zaltsman et al., 2005b). For the Arabidopsis FtsH complex, the stoichiometry of type A:type B subunits was estimated to be 1:2 using a mass spectrometry-based approach (Moldavski et al., 2012). The stoichiometry of subunit types in the FtsH complex in cyanobacteria was shown to be 1:1 (Boehm et al., 2012).
Functions of FtsH in Chloroplast Biogenesis
Characterization of var2 mutants has shown that their variegated leaves are composed of green sectors with normal-looking chloroplasts and white sectors with abnormal plastids (Chen et al., 1999; Kato et al., 2007). Accumulation of abnormal plastids in the white sectors is due to arrest in the early stage of plastid differentiation. Notably, normal-looking chloroplasts in the green sectors developed more slowly (Sakamoto et al., 2009). These observations suggest that FtsH is involved in the formation of thylakoid membranes during early chloroplast development. In addition, RNA interference of chloroplast FtsHs in Nicotiana tabacum showed a collapse of thylakoid membranes during the late stages of leaf development, suggesting that FtsH also plays a crucial role in the maintenance of thylakoid membranes (Kato et al., 2012a). Based on the correlation between the level of the FtsH complex and the degree of leaf variegation, a threshold model in which total FtsH levels define the fate of plastids during leaf development has been proposed (Chen et al., 2000; Yu et al., 2004; Zaltsman et al., 2005a; Miura et al., 2007). Furthermore, complementation of var2 by site-directed mutagenesis of FtsH2 demonstrated that not all catalytic sites are required for the adequate development of thylakoid membranes (Zhang et al., 2010). Although the exact role of FtsH in thylakoid development remains to be elucidated, it has also been suggested that FtsH acts as a scaffold protein during thylakoid development. On the other hand, multiple lines of evidence demonstrate that reduction in protein biosynthesis in plastids suppresses leaf variegation (Miura et al., 2007; Yu et al., 2008; see details. Putarjunan et al., 2013 for a review). The balance between protein biosynthesis and FtsH function during leaf development seems to be crucial for chloroplast differentiation.
Protein Quality Control in the Photosystem II Repair Cycle
The main function of FtsH in chloroplasts is protein quality control during photosynthesis. Light energy is required for the initial step of photosynthesis but it simultaneously causes unavoidable damage to the photosystem II (PSII) protein complex, in particular to the reaction center protein D1 (Murata et al., 2007). Photodamaged D1 needs to be removed specifically by proteolysis and replaced by newly synthesized D1 to maintain photosynthetic activity. This sophisticated repair system that photosynthetic organisms have developed is called the “PSII repair cycle,” and it consists of a sequential process of (i) light-induced damage to the reaction center protein D1, (ii) partial disassembly of the PSII complex to expose damaged D1, (iii) proteolysis of damaged D1, and (iv) de novo synthesis of D1 and reassembly of functional PSII (Figure 2). FtsH is involved in the proteolysis of damaged D1 in the PSII repair cycle (Nixon et al., 2010). This fundamental process is conserved in various photosynthetic organisms and is crucial for avoiding photoinhibition caused by the accumulation of photodamaged PSII. Indeed, Arabidopsis mutants lacking FtsH show increased sensitivity to high-light stress, which is indicative of accelerated photoinhibition (Bailey et al., 2002; Sakamoto et al., 2002, 2004).
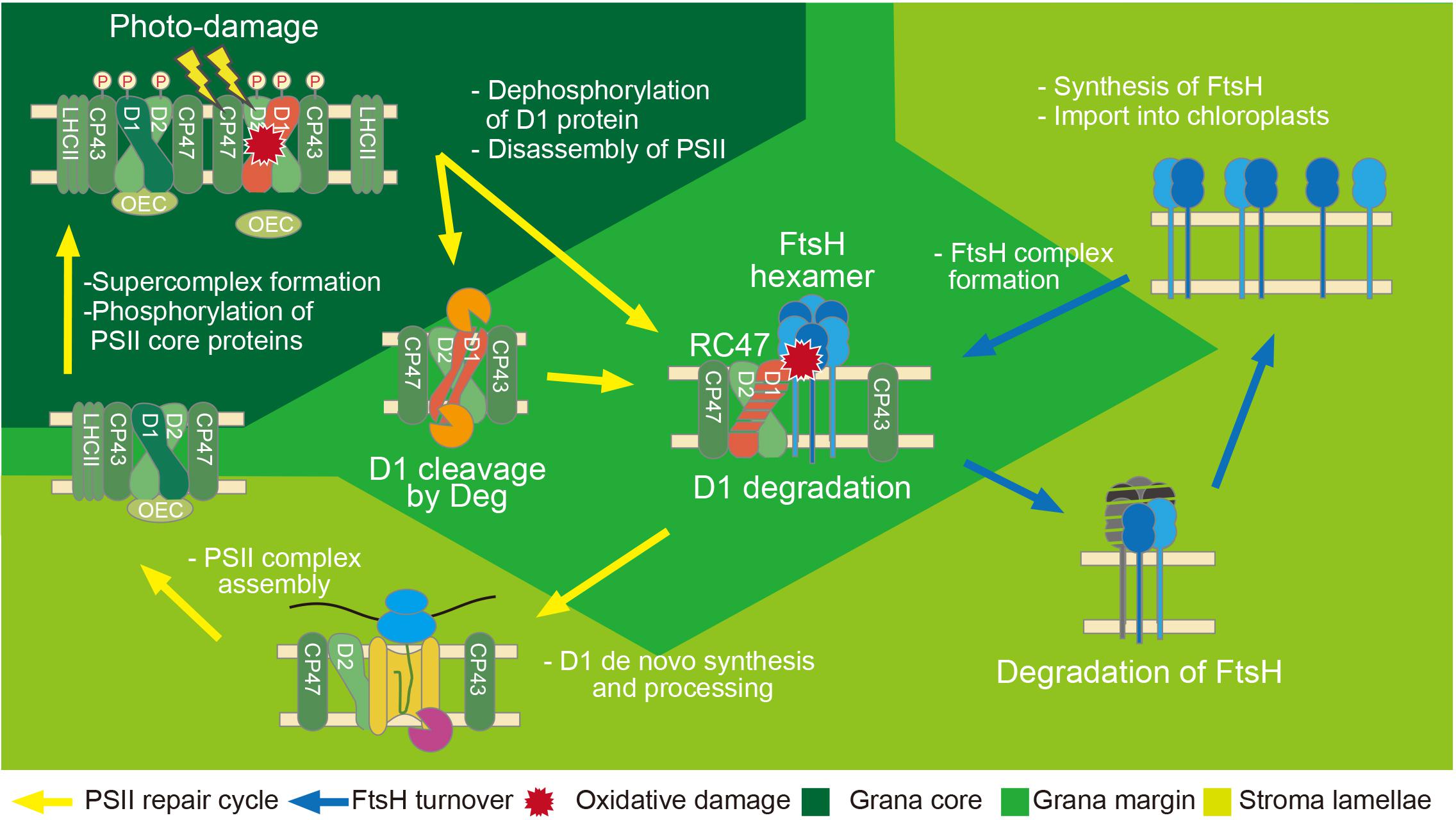
FIGURE 2. Model of PSII repair and regulation of FtsH activity in chloroplasts. In chloroplasts, photodamaged D1 migrates from the grana stacks to the grana margins, which is the location of D1 degradation in the PSII repair cycle. Dephosphorylation of PSII core proteins by chloroplast PP2C phosphatase and partial disassembly of the PSII complex occur prior to the degradation process. D1 degradation is mainly conducted by FtsH (fundamental degradation), and endopeptidic cleavages by Deg proteases facilitate the effective degradation by FtsH in photoinhibitory conditions. Newly synthesized D1 is processed at its C-terminus by CtpA peptidase. Repaired PSII migrates to the grana core to form functional PSII. Phosphorylation of PSII core proteins is carried out by STN8 kinase. On the other hand, the PSII repair cycle might require proper FtsH turnover in chloroplasts. When FtsH has access to damaged PSII, FtsH concomitantly suffers from oxidative damage induced by ROS. The damaged FtsH should be repaired by proper turnover. Newly synthesized FtsH proteins are imported into chloroplasts and subsequently integrated into the thylakoid membrane. FtsH forms functional complexes in the grana margin. The post-translational modification in FtsH might regulate protease activity and/or complex formation.
Thus far, two proteolytic pathways involving FtsH for removing damaged D1 have been described. The first pathway is processive D1 degradation, which is carried out predominantly by FtsH (Lindahl et al., 2000; Bailey et al., 2002; Kato et al., 2009). D1 has five transmembrane domains and a stroma-exposed N terminus, at which FtsH might initiate processive proteolysis. Studies using cyanobacteria mutants and transgenic tobacco plants with truncations at the N-terminus of D1 showed that the N-terminus is required for D1 degradation (Komenda et al., 2007; Michoux et al., 2016). In addition, post-translational modifications (PTMs) at the N-terminus seem to regulate D1 degradation by FtsH. Earlier studies reported that an N-terminal threonine residue of D1 is reversibly phosphorylated in a light-dependent manner (Aro et al., 1992), and its dephosphorylation is a prerequisite for its degradation (Koivuniemi et al., 1995; Rintamaki et al., 1996). The fact that phosphorylation of D1 increased more in mutants lacking FtsH in a light-dependent manner suggests an interaction between D1 phosphorylation and processive degradation by FtsH (Kato and Sakamoto, 2014). While more phosphorylation prevents FtsH from degrading D1 efficiently, it appears to accelerate the alternative degradation pathway mediated by Deg proteases concomitantly (see below). This coordinated D1 degradation was shown to be compromised in the mutant lacking STN8 kinase that phosphorylates D1, when combined with var2. The N-terminus of D1 is also processed by methionine aminopeptidases, and enzymatic removal of the N-terminal-initiating methionine seems to be required for recognition of the N-terminus by FtsH (Adam et al., 2011).
The second D1 degradation pathway consists of the cooperative action of FtsH and Deg proteases in photoinhibitory conditions. Deg family members are ATP-independent serine proteases and are localized to both the stromal and luminal sides of thylakoid membranes (Schuhmann and Adamska, 2012). Endopeptidic cleavage of D1 by Deg proteases seems to facilitate D1 degradation by creating additional recognition sites for FtsH (Haußühl et al., 2001; Kapri-Pardes et al., 2007; Sun et al., 2007, 2010; Knopf and Adam, 2018). Evidence for this cooperative D1 degradation pathway comes from the increased accumulation of D1 cleavage fragments in Arabidopsis mutants lacking FtsH (Kato et al., 2012b). Accumulation of D1 cleavage fragments has also been observed in Chlamydomonas reinhardtii ftsh mutants (Malnoë et al., 2014). However, the degradation rate of D1 protein in a deg triple mutant in the cyanobacteria Synechocystis sp. PCC 6803 is similar to that in wild-type controls, suggesting that the contribution of Deg proteases is less important in cyanobacteria (Barker et al., 2006). A detailed analysis of D1 cleavage fragments in Arabidopsis suggests that fragmentation is initiated by Deg proteases that cleave the luminal loop connecting the C and D transmembrane helices of D1. This hypothesis might be reinforced by the fact that D1 fragmentation is enhanced by primary damage to the Mn-cluster, which might induce dissociation of the PSII oxygen-evolving complex and accelerate the access of Deg proteases to damaged D1 (Kato et al., 2015). Accelerated D1 degradation through D1 fragmentation appears to cause a simultaneous increase in reactive oxygen species (ROS) levels. This relationship could be explained by cytotoxicity of D1 cleavage fragments, which may retain chlorophyll that can still absorb light energy. Although this energy could not be used for photosynthesis, it could be transferred to oxygen, resulting in the generation of ROS. Cooperative D1 degradation is considered to be an escape pathway that occurs in photoinhibitory conditions (Kato and Sakamoto, 2014).
Oxidative Stress and Retrograde Signaling
Mutants lacking FtsH generate high levels of ROS as a result of the accumulation of damaged PSII in chloroplasts (Kato et al., 2009). Because ROS have been implicated as second messengers that transduce retrograde signals from chloroplasts to the nucleus (Laloi et al., 2006), we were interested in examining gene expression in mutants lacking FtsH. However, our attempt to do this via microarray analysis showed that there was no obvious up-regulation of ROS-related genes in cells suffering from photooxidative stress (Miura et al., 2010). This unexpected result suggests that ROS from photodamaged PSII do not act as second messengers or that the signaling might be inhibited by the loss of FtsH. Notably, a recent study by Wang et al. (2016) reported the involvement of FtsH in an EXECUTER1 (EX1)-mediated retrograde signaling pathway activated by singlet oxygen (1 O2). EX1 was originally identified through a suppressor screen of fluorescent (flu) mutants, which generate 1 O2 in chloroplasts upon a dark-to-light shift (Lee et al., 2007; Przybyla et al., 2008). In the flu mutant, an 1 O2 burst resulting from the abnormal accumulation of a chlorophyll precursor causes the degradation of EX1 and eventually leads to programmed cell death. In this process, FtsH degrades EX1, which might be oxidized by 1 O2 (Wang et al., 2016). Because the inactivation of FtsH in flu mutants prevents 1 O2 signaling and related gene expression (Wang et al., 2016; Dogra et al., 2017), the degradation of EX1 by FtsH is necessary for the retrograde signaling pathway. The mechanisms by which EX1 degradation products act as retrograde signaling molecules remains to be investigated.
Other Biological Functions of FtsH
FtsH is involved in the degradation and assembly of several thylakoid proteins other than D1. An early in vitro study suggested that FtsH participates in the degradation of unassembled Rieske Fe-S protein, a component of the thylakoid-bound cytochrome b6f complex that may undergo a conformational change upon absorption of light energy (Ostersetzer and Adam, 1997). A later study in Chlamydomonas showed that the cytochrome b6f complex is degraded by FtsH in conditions of sulfur or nitrogen starvation, indicating that FtsH plays a role in protein quality control during nutrient deficiency (Malnoë et al., 2014; Wei et al., 2014). On the other hand, Järvi and coworkers showed that FtsH functions in the biosynthesis of PSI (Järvi et al., 2016). Furthermore, the low levels of FtsH in Arabidopsis ftsh mutants seem to affect the entire photosynthetic electron transfer chain. Analysis of chlorophyll fluorescence implies that mutants lacking FtsH exhibit high rates of cyclic electron transfer around photosystem I (PSI), which results in a higher non-photochemical quenching value (Järvi et al., 2016). Additionally, Chlamydomonas FtsH plays a role in degrading the light-harvesting chlorophyll a/b-binding proteins associated with PSI (Bujaldon et al., 2017). How FtsH is involved in the early assembly of PSI complexes remains unknown; however, these results expand our understanding of the physiological function of FtsH in thylakoid membranes.
Regulation of FtsH Protease Activity
Several important questions remain to be answered, as to how the protease activity of FtsH in chloroplasts is regulated. E. coli FtsH hexamers interact with other membrane protein complexes composed of the prohibitin-like proteins HflK and HflC (Kihara et al., 1996; Saikawa et al., 2004). Increasing evidence suggests that prohibitin-like proteins act as a negative regulator in the selection of FtsH substrates (Kihara et al., 1996, 1998). Prohibitin homologs (PHBs) have also been implicated in regulating FtsH activity in other bacteria, yeast, and plant mitochondria (Steglich et al., 1999; Piechota et al., 2010). In photosynthetic organisms, however, supercomplexes consisting of thylakoid FtsH and PHBs have been reported only in cyanobacteria (Boehm et al., 2012). Although FtsH-containing supercomplexes have been observed in Chlamydomonas chloroplasts (Wang et al., 2017), PHBs have not been found in the chloroplasts; therefore, the composition of these supercomplexes remains unknown. In Arabidopsis, blue native polyacrylamide gel electrophoresis analysis of thylakoid membranes showed that most FtsH proteins migrated in small complexes, likely in the form of dimers, whereas a small amount was present as a larger complex, at the position where a functional complex would be expected (Takabayashi et al., 2017). Yoshioka et al. (2010) suggested that smaller FtsH complexes are mainly present in the stroma-exposed thylakoid, whereas the large functional complex is detected in PSII-enriched thylakoid membranes, including grana margins where D1 degradation occurs during the PSII repair cycle. These results suggested the flexible oligomerization capability of FtsH proteins and the existence of different mechanisms for the regulation of FtsH activity in the chloroplasts of seed plants. Instead of forming supercomplexes with PHBs, an interesting hypothesis is that FtsHs in seed plants might transiently form a functional hexameric complex to fulfill its degradation function. A recent proteomics analysis demonstrated that thylakoid FtsH proteins themselves show a higher turnover rate than other chloroplast proteases (Li et al., 2017). Given that damaged PSII complexes are a potential site of ROS generation, FtsH might suffer from oxidative damage as well and require its own quality control by means of its fast turnover (Figure 2). Supporting this possibility, degradation of FtsH was suggested to be accelerated during high-light stress (Zaltsman et al., 2005a). Sinvany-Villalobo et al. (2004) reported that upon high-light exposure, some FtsH genes are dramatically upregulated at the mRNA level (e.g., FtsH8), whereas FtsH protein levels showed only modest increase. Overall, these data implicate a fast turnover rate of FtsH, which should be further investigated in the future works.
In addition to the possible turnover of FtsH during light stress, PTMs seem to contribute to its regulation. Thus far, two PTMs are thought to regulate FtsH function. In Arabidopsis, phosphorylation of FtsH in the chloroplasts was suggested by a proteome study on calcium-dependent protein phosphorylation (Reiland et al., 2009; Stael et al., 2012; Roitinger et al., 2015). Whether phosphorylation controls FtsH activity remains unclear, and we are currently testing this possibility by means of site-directed mutagenesis of predicted phosphorylation sites. On the other hand, a recent study in Chlamydomonas showed that the formation of intermolecular disulfide bridges promoted the oligomerization of FtsH (Wang et al., 2017). Of note, these results suggested that the proteolytic activity of FtsH could be regulated by the thylakoid redox state, because the formation of disulfide bridges in chloroplasts is controlled mainly by the thioredoxin system in the stroma and thylakoid membrane.
Perspectives and Remaining Questions
A recent study in cyanobacteria suggests that Thylakoid formation 1 (THF1) protein, which seems to positively affect the accumulation of FtsH, physically interacts with FtsH (Beèková et al., 2017). Together, increasing evidence indicates that the stability and complex formation of FtsH are key to understanding the details of FtsH function. Another remaining question is the issue of substrate recognition. Focusing on the PSII repair cycle, selective D1 recognition by FtsH after disassembly of CP43, the core antenna protein of PSII, from the damaged PSII complex might be important for enhancing photosynthesis in plants by improving the PSII repair. Krynická et al. (2015) showed that the accessibility of FtsH to the D1 protein is crucial for its selection of substrates in the PSII complex. For example, oxidative modification of the PSII reaction center may be associated with D1 degradation. Recent progress in mass spectrometry has enabled the identification of specific photooxidative protein modifications in PSII core proteins (Dreaden et al., 2011). Future research in this area will uncover the relationship between photooxidative protein modifications and proteolysis by FtsH.
Author Contributions
All authors listed have made a substantial, direct and intellectual contribution to the work, and approved it for publication.
Funding
The work from the authors’ laboratory presented in this article is partially supported by KAKENHI grants (16H06554 and 17H03699 to WS) and by the Ohara Foundation (to WS and YK).
Conflict of Interest Statement
The authors declare that the research was conducted in the absence of any commercial or financial relationships that could be construed as a potential conflict of interest.
References
Adam, Z., Frottin, F., Espagne, C., Meinnel, T., and Giglione, C. (2011). Interplay between N-terminal methionine excision and FtsH protease is essential for normal chloroplast development and function in Arabidopsis. Plant Cell 23, 3745–3760. doi: 10.1105/tpc.111.087239
Aro, E. M., Kettunen, R., and Tyystjärvi, E. (1992). ATP and light regulate D1 protein modification and degradation Role of D1∗ in photoinhibition. FEBS Lett. 297, 29–33. doi: 10.1016/0014-5793(92)80320-G
Bailey, S., Thompson, E., Nixon, P. J., Horton, P., Mullineaux, C. W., Robinson, C., et al. (2002). A critical role for the Var2 FtsH homologue of Arabidopsis thaliana in the photosystem II repair cycle in vivo. J. Biol. Chem. 277, 2006–2011. doi: 10.1074/jbc.M105878200
Barker, M., De Vries, R., Nield, J., Komenda, J., and Nixon, P. J. (2006). The Deg proteases protect Synechocystis sp. PCC 6803 during heat and light stresses but are not essential for removal of damaged D1 protein during the photosystem two repair cycle. J. Biol. Chem. 281, 30347–30355. doi: 10.1074/jbc.M601064200
Bečková, M., Yu, J., Krynická, V., Kozlo, A., Shao, S., Koník, P., et al. (2017). Structure of Psb29/Thf1 and its association with the FtsH protease complex involved in photosystem II repair in cyanobacteria. Philos. Trans. R. Soc. B Biol. Sci. 372:20160394. doi: 10.1098/rstb.2016.0394
Boehm, M., Yu, J., Krynicka, V., Barker, M., Tichy, M., Komenda, J., et al. (2012). Subunit organization of a Synechocystis hetero-oligomeric thylakoid FtsH complex involved in photosystem II repair. Plant Cell 24, 3669–3683. doi: 10.1105/tpc.112.100891
Bujaldon, S., Kodama, N., Rappaport, F., Subramanyam, R., de Vitry, C., Takahashi, Y., et al. (2017). Functional accumulation of antenna proteins in chlorophyll b-less mutants of Chlamydomonas reinhardtii. Mol. Plant 10, 115–130. doi: 10.1016/j.molp.2016.10.001
Chen, M., Choi, Y., Voytas, D. F., and Rodermel, S. (2000). Mutations in the Arabidopsis VAR2 locus cause leaf variegation due to the loss of a chloroplast FtsH protease. Plant J. 22, 303–313. doi: 10.1046/j.1365-313X.2000.00738.x
Chen, M., Jensen, M., and Rodermel, S. (1999). The yellow variegated mutant of Arabidopsis is plastid autonomous and delayed in chloroplast biogenesis. J. Hered. 90, 207–214. doi: 10.1093/jhered/90.1.207
Chiba, S., Akiyama, Y., and Ito, K. (2002). Membrane protein degradation by FtsH can be initiated from either end. J. Bacteriol. 184, 4775–4782. doi: 10.1128/JB.184.17.4775
Chiba, S., Akiyama, Y., Mori, H., Matsuo, E., and Ito, K. (2000). Length recognition at the N-terminal tail for the initiation of FtsH-mediated proteolysis. EMBO Rep. 1, 47–52. doi: 10.1093/embo-reports/kvd005
Clarke, A. K. (2012). The chloroplast ATP-dependent Clp protease in vascular plants - new dimensions and future challenges. Physiol. Plant. 145, 235–244. doi: 10.1111/j.1399-3054.2011.01541.x
Dogra, V., Duan, J., Lee, K. P., Lv, S., Liu, R., and Kim, C. (2017). FtsH2-dependent proteolysis of EXECUTER1 is essential in mediating singlet oxygen-triggered retrograde signaling in Arabidopsis thaliana. Front. Plant Sci. 8:1145. doi: 10.3389/fpls.2017.01145
Dreaden, T. M., Chen, J., Rexroth, S., and Barry, B. A. (2011). N-formylkynurenine as a marker of high light stress in photosynthesis. J. Biol. Chem. 286, 22632–22641. doi: 10.1074/jbc.M110.212928
Haußühl, K., Andersson, B., and Adamska, I. (2001). A chloroplast DegP2 protease performs the primary cleavage of the photodamaged D1 protein in plant photosystem II. EMBO J. 20, 713–722. doi: 10.1093/emboj/20.4.713
Ito, K., and Akiyama, Y. (2005). Cellular functions, mechanism of action, and regulation of FtsH protease. Annu. Rev. Microbiol. 59, 211–231. doi: 10.1146/annurev.micro.59.030804.121316
Järvi, S., Suorsa, M., Tadini, L., Ivanauskaite, A., Rantala, S., Allahverdiyeva, Y., et al. (2016). Thylakoid-bound FtsH proteins facilitate proper biosynthesis of photosystem I. Plant Physiol. 171, 1333–1343. doi: 10.1104/pp.16.00200
Kapri-Pardes, E., Naveh, L., and Adam, Z. (2007). The thylakoid lumen protease Deg1 is involved in the repair of photosystem II from photoinhibition in Arabidopsis. Plant Cell 19, 1039–1047. doi: 10.1105/tpc.106.046573
Kato, Y., Kouso, T., and Sakamoto, W. (2012a). Variegated tobacco leaves generated by chloroplast FtsH suppression: Implication of Ftsh function in the maintenance of thylakoid membranes. Plant Cell Physiol. 53, 391–404. doi: 10.1093/pcp/pcr189
Kato, Y., Miura, E., Ido, K., Ifuku, K., and Sakamoto, W. (2009). The variegated mutants lacking chloroplastic FtsHs are defective in D1 degradation and accumulate reactive oxygen species. Plant Physiol. 151, 1790–1801. doi: 10.1104/pp.109.146589
Kato, Y., Miura, E., Matsushima, R., and Sakamoto, W. (2007). White leaf sectors in yellow variegated2 are formed by viable cells with undifferentiated plastids. Plant Physiol. 144, 952–960. doi: 10.1104/pp.107.099002
Kato, Y., Ozawa, S. I., Takahashi, Y., and Sakamoto, W. (2015). D1 fragmentation in photosystem II repair caused by photo-damage of a two-step model. Photosynth. Res. 126, 409–416. doi: 10.1007/s11120-015-0144-7
Kato, Y., and Sakamoto, W. (2014). Phosphorylation of photosystem II core proteins prevents undesirable cleavage of D1 and contributes to the fine-tuned repair of photosystem II. Plant J. 79, 312–321. doi: 10.1111/tpj.12562
Kato, Y., Sun, X., Zhang, L., and Sakamoto, W. (2012b). Cooperative D1 degradation in the photosystem II repair mediated by chloroplastic proteases in Arabidopsis. Plant Physiol. 159, 1428–1439. doi: 10.1104/pp.112.199042
Kihara, A., Akiyama, Y., and Ito, K. (1996). A protease complex in the Escherichia coli plasma membrane: HflKC (HflA) forms a complex with FtsH (HflB), regulating its proteolytic activity against SecY. EMBO J. 15, 6122–6131.
Kihara, A., Akiyama, Y., and Ito, K. (1998). Different pathways for protein degradation by the FtsH/HflKC membrane-embedded protease complex: an implication from the interference by a mutant form of a new substrate protein, YccA. J. Mol. Biol. 279, 175–188. doi: 10.1006/jmbi.1998.1781
Knopf, R. R., and Adam, Z. (2018). Lumenal exposed regions of the D1 protein of PSII are long enough to be degraded by the chloroplast Deg1 protease. Sci. Rep. 8:5230. doi: 10.1038/s41598-018-23578-x
Koivuniemi, A., Aro, E. M., and Andersson, B. (1995). Degradation of the D1- and D2-proteins of photosystem II in higher plants is regulated by reversible phosphorylation. Biochemistry 34, 16022–16029. doi: 10.1021/bi00049a016
Komenda, J., Tichy, M., Prasil, O., Knoppova, J., Kuvikova, S., de Vries, R., et al. (2007). The exposed N-terminal tail of the D1 subunit is required for rapid D1 degradation during photosystem II repair in Synechocystis sp PCC 6803. Plant Cell 19, 2839–2854. doi: 10.1105/tpc.107.053868
Krynická, V., Shao, S., Nixon, P. J., and Komenda, J. (2015). Accessibility controls selective degradation of photosystem II subunits by FtsH protease. Nat. Plants 1:15168. doi: 10.1038/nplants.2015.168
Krzywda, S., Brzozowski, A. M., Karata, K., Ogura, T., and Wilkinson, A. J. (2002). Crystallization of the AAA domain of the ATP-dependent protease FtsH of Escherichia coli. Acta Crystallogr. Sect. D Biol. Crystallogr. 58, 1066–1067. doi: 10.1107/S0907444902006972
Laloi, C., Przybyla, D., and Apel, K. (2006). A genetic approach towards elucidating the biological activity of different reactive oxygen species in Arabidopsis thaliana. J. Exp. Bot. 57, 1719–1724. doi: 10.1093/jxb/erj183
Lee, K. P., Kim, C., Landgraf, F., and Apel, K. (2007). EXECUTER1- and EXECUTER2-dependent transfer of stress-related signals from the plastid to the nucleus of Arabidopsis thaliana. Proc. Natl. Acad. Sci. U.S.A. 104, 10270–10275. doi: 10.1073/pnas.0702061104
Li, L., Nelson, C. J., Trösch, J., Castleden, I., Huang, S., and Millar, A. H. (2017). Protein degradation rate in Arabidopsis thaliana leaf growth and development. Plant Cell 29, 207–228. doi: 10.1105/tpc.16.00768
Lindahl, M., Spetea, C., Hundal, T., Oppenheim, A. B., Adam, Z., and Andersson, B. (2000). The thylakoid FtsH protease plays a role in the light-induced turnover of the photosystem II D1 protein. Plant Cell 12, 419–432. doi: 10.1105/tpc.12.3.419
Lindahl, M., Tabak, S., Cseke, L., Pichersky, E., Andersson, B., and Adam, Z. (1996). Identification, characterization, and molecular cloning of a homologue of the bacterial FtsH protease in chloroplast of higher plants. J. Biol. Chem. 271, 229334–293329. doi: 10.1074/jbc.271.46.29329
Malnoë, A., Wang, F., Girard-Bascou, J., Wollman, F.-A., and de Vitry, C. (2014). Thylakoid FtsH protease contributes to photosystem II and cytochrome b6f remodeling in Chlamydomonas reinhardtii under stress conditions. Plant Cell 26, 373–390. doi: 10.1105/tpc.113.120113
Michoux, F., Ahmad, N., Wei, Z.-Y., Belgio, E., Ruban, A. V., and Nixon, P. J. (2016). Testing the role of the N-terminal tail of D1 in the maintenance of photosystem II in tobacco chloroplasts. Front. Plant Sci. 7:844. doi: 10.3389/fpls.2016.00844
Miura, E., Kato, Y., Matsushima, R., Albrecht, V., Laalami, S., and Sakamoto, W. (2007). The balance between protein synthesis and degradation in chloroplasts determines leaf variegation in Arabidopsis yellow variegated mutants. Plant Cell 19, 1313–1328. doi: 10.1105/tpc.106.049270
Miura, E., Kato, Y., and Sakamoto, W. (2010). Comparative transcriptome analysis of green/white variegated sectors in Arabidopsis yellow variegated2: Responses to oxidative and other stresses in white sectors. J. Exp. Bot. 61, 2433–2445. doi: 10.1093/jxb/erq075
Moldavski, O., Levin-Kravets, O., Ziv, T., Adam, Z., and Prag, G. (2012). The hetero-hexameric nature of a chloroplast AAA+ FtsH protease contributes to its thermodynamic stability. PLoS One 7:e36008. doi: 10.1371/journal.pone.0036008
Murata, N., Takahashi, S., Nishiyama, Y., and Allakhverdiev, S. I. (2007). Photoinhibition of photosystem II under environmental stress. Biochim. Biophys. Acta Bioenerg. 1767, 414–421. doi: 10.1016/j.bbabio.2006.11.019
Nishimura, K., Kato, Y., and Sakamoto, W. (2016). Chloroplast proteases: Updates on proteolysis within and across suborganellar compartments. Plant Physiol. 171, 2280–2293. doi: 10.1104/pp.16.00330
Nishimura, K., Kato, Y., and Sakamoto, W. (2017). Essentials of proteolytic machineries in chloroplasts. Mol. Plant 10, 4–19. doi: 10.1016/j.molp.2016.08.005
Nishimura, K., and van Wijk, K. J. (2015). Organization, function and substrates of the essential Clp protease system in plastids. Biochim. Biophys. Acta 1847, 915–930. doi: 10.1016/j.bbabio.2014.11.012
Niwa, H., Tsuchiya, D., Makyio, H., Yoshida, M., and Morikawa, K. (2002). Hexameric ring structure of the ATPase domain of the membrane-integrated metalloprotease FtsH from Thermus thermophilus HB8. Structure 10, 1415–1423. doi: 10.1016/S0969-2126(02)00855-9
Nixon, P. J., Michoux, F., Yu, J., Boehm, M., and Komenda, J. (2010). Recent advances in understanding the assembly and repair of photosystem II. Ann. Bot. 106, 1–16. doi: 10.1093/aob/mcq059
Ostersetzer, O., and Adam, Z. (1997). Light-stimulated degradation of an unassembled Rieske FeS protein by a thylakoid-bound protease: the possible role of the FtsH protease. Plant Cell 9, 957–965. doi: 10.1105/tpc.9.6.957
Piechota, J., Kolodziejczak, M., Juszczak, I., Sakamoto, W., and Janska, H. (2010). Identification and characterization of high molecular weight complexes formed by matrix AAA proteases and prohibitins in mitochondria of Arabidopsis thaliana. J. Biol. Chem. 285, 12512–12521. doi: 10.1074/jbc.M109.063644
Przybyla, D., Göbel, C., Imboden, A., Hamberg, M., Feussner, I., and Apel, K. (2008). Enzymatic, but not non-enzymatic, 1O2-mediated peroxidation of polyunsaturated fatty acids forms part of the EXECUTER1-dependent stress response program in the flu mutant of Arabidopsis thaliana. Plant J. 54, 236–248. doi: 10.1111/j.1365-313X.2008.03409.x
Putarjunan, A., Liu, X., Nolan, T., Yu, F., and Rodermel, S. (2013). Understanding chloroplast biogenesis using second-site suppressors of immutans and var2. Photosynth. Res. 116, 437–453. doi: 10.1007/s11120-013-9855-9
Reiland, S., Messerli, G., Baerenfaller, K., Gerrits, B., Endler, A., Grossmann, J., et al. (2009). Large-scale Arabidopsis phosphoproteome profiling reveals novel chloroplast kinase substrates and phosphorylation networks. Plant Physiol. 150, 889–903. doi: 10.1104/pp.109.138677
Rintamaki, E., Kettunen, R., and Aro, E. (1996). Differential D1 dephosphorylation in functional and photodamaged photosystem II centers. J. Biol. Chem. 271, 14870–14875. doi: 10.1074/jbc.271.25.14870
Rodrigues, R. A. O., Silva-Filho, M. C., and Cline, K. (2011). FtsH2 and FtsH5: two homologous subunits use different integration mechanisms leading to the same thylakoid multimeric complex. Plant J. 65, 600–609. doi: 10.1111/j.1365-313X.2010.04448.x
Roitinger, E., Hofer, M., Köcher, T., Pichler, P., Novatchkova, M., Yang, J., et al. (2015). Quantitative phosphoproteomics of the ataxia telangiectasia-mutated (ATM) and ataxia telangiectasia-mutated and Rad3-related (ATR) dependent DNA damage response in Arabidopsis thaliana. Mol. Cell. Proteomics 14, 556–571. doi: 10.1074/mcp.M114.040352
Saikawa, N., Akiyama, Y., and Ito, K. (2004). FtsH exists as an exceptionally large complex containing HflKC in the plasma membrane of Escherichia coli. J. Struct. Biol. 146, 123–129. doi: 10.1016/j.jsb.2003.09.020
Sakamoto, W., Miura, E., Kaji, Y., Okuno, T., Nishizono, M., and Ogura, T. (2004). Allelic characterization of the leaf-variegated mutation var2 identifies the conserved amino acid residues of FtsH that are important for ATP hydrolysis and proteolysis. Plant Mol. Biol. 56, 705–716. doi: 10.1007/s11103-004-4561-9
Sakamoto, W., Tamura, T., Hanba-Tomita, Y., Sodmergen, and Murata, M. (2002). The VAR1 locus of Arabidopsis encodes a choloroplastic FtsH and is responsible for leaf variegation in the mutant alleles. Genes Cells 7, 769–780. doi: 10.1046/j.1365-2443.2002.00558.x
Sakamoto, W., Uno, Y., Zhang, Q., Miura, E., Kato, Y., and Sodmergen . (2009). Arrested differentiation of proplastids into chloroplasts in variegated leaves characterized by plastid ultrastructure and nucleoid morphology. Plant Cell Physiol. 50, 2069–2083. doi: 10.1093/pcp/pcp127
Sakamoto, W., Zaltsman, A., Adam, Z., and Takahashi, Y. (2003). Coordinated regulation and complex formation of YELLOW VARIEGATED1 and YELLOW VARIEGATED2, chloroplastic FtsH metalloproteases involved in the repair cycle of photosystem II in Arabidopsis thylakoid membranes. Plant Cell 15, 2843–2855. doi: 10.1105/tpc.017319
Schuhmann, H., and Adamska, I. (2012). Deg proteases and their role in protein quality control and processing in different subcellular compartments of the plant cell. Physiol. Plant. 145, 224–234. doi: 10.1111/j.1399-3054.2011.01533.x
Sinvany-Villalobo, G., Davydov, O., Ben-Ari, G., Zaltsman, A., Raskind, A., and Adam, Z. (2004). Expression in multigene families. Analysis of chloroplast and mitochondrial proteases. Plant Physiol. 135, 1336–1345. doi: 10.1104/pp.104.043299
Stael, S., Rocha, A. G., Wimberger, T., Anrather, D., Vothknecht, U. C., and Teige, M. (2012). Cross-talk between calcium signalling and protein phosphorylation at the thylakoid. J. Exp. Bot. 63, 1725–1733. doi: 10.1093/jxb/err403
Steglich, G., Neupert, W., and Langer, T. (1999). Prohibitins regulate membrane protein degradation by the m-AAA protease in mitochondria. Mol. Cell. Biol. 19, 3435–3442. doi: 10.1128/MCB.19.5.3435
Sun, X., Fu, T., Chen, N., Guo, J., Ma, J., Zou, M., et al. (2010). The stromal chloroplast Deg7 protease participates in the repair of photosystem II after photoinhibition in Arabidopsis. Plant Physiol. 152, 1263–1273. doi: 10.1104/pp.109.150722
Sun, X., Peng, L., Guo, J., Chi, W., Ma, J., Lu, C., et al. (2007). Formation of DEG5 and DEG8 complexes and their involvement in the degradation of photodamaged photosystem II reaction center D1 protein in Arabidopsis. Plant Cell 19, 1347–1361. doi: 10.1105/tpc.106.049510
Takabayashi, A., Takabayashi, S., Takahashi, K., Watanabe, M., Uchida, H., Murakami, A., et al. (2017). PCoM-DB update: A protein co-migration database for photosynthetic organisms. Plant Cell Physiol. 58, e10. doi: 10.1093/pcp/pcw219
Takechi, K., Sodmergen, Murata, M., Motoyoshi, F., and Sakamoto, W. (2000). The YELLOW VARIEGATED (VAR2) locus encodes a homologue of FtsH, an ATP-dependent protease in Arabidopsis. Plant Cell Physiol. 41, 1334–1346. doi: 10.1093/pcp/pcd067
van Wijk, K. J. (2015). Protein maturation and proteolysis in plant plastids, mitochondria, and peroxisomes. Annu. Rev. Plant Biol. 66, 75–111. doi: 10.1146/annurev-arplant-043014-115547
Wang, F., Qi, Y., Malnoë, A., Choquet, Y., Wollman, F. A., and de Vitry, C. (2017). The high light response and redox control of thylakoid FtsH protease in Chlamydomonas reinhardtii. Mol. Plant 10, 99–114. doi: 10.1016/j.molp.2016.09.012
Wang, L., Kim, C., Xu, X., Piskurewicz, U., Dogra, V., Singh, S., et al. (2016). Singlet oxygen- and EXECUTER1-mediated signaling is initiated in grana margins and depends on the protease FtsH2. Proc. Natl. Acad. Sci. U.S.A. 113, E3792–E3800. doi: 10.1073/pnas.1603562113
Wei, L., Derrien, B., Gautier, A., Houille-Vernes, L., Boulouis, A., Saint-Marcoux, D., et al. (2014). Nitric oxide-triggered remodeling of chloroplast bioenergetics and thylakoid proteins upon nitrogen starvation in Chlamydomonas reinhardtii. Plant Cell 26, 353–372. doi: 10.1105/tpc.113.120121
Yoshioka, M., Nakayama, Y., Yoshida, M., Ohashi, K., Morita, N., Kobayashi, H., et al. (2010). Quality control of photosystem II: FtsH hexamers are localized near photosystem II at grana for the swift repair of damage. J. Biol. Chem. 285, 41972–41981. doi: 10.1074/jbc.M110.117432
Yu, F., Liu, X., Alsheikh, M., Park, S., and Rodermel, S. (2008). Mutations in SUPPRESSOR OF VARIEGATION1, a factor required for normal chloroplast translation, suppress var2-mediated leaf variegation in Arabidopsis. Plant Cell 20, 1786–1804. doi: 10.1105/tpc.107.054965
Yu, F., Park, S., and Rodermel, S. R. (2004). The Arabidopsis FtsH metalloprotease gene family: Interchangeability of subunits in chloroplast oligomeric complexes. Plant J. 37, 864–876. doi: 10.1111/j.1365-313X.2003.02014.x
Yu, F., Park, S., and Rodermel, S. R. (2005). Functional redundancy of AtFtsH metalloproteases in thylakoid membrane complexes. Plant Physiol. 138, 1957–1966. doi: 10.1104/pp.105.061234
Zaltsman, A., Feder, A., and Adam, Z. (2005a). Developmental and light effects on the accumulation of FtsH protease in Arabidopsis chloroplasts - implications for thylakoid formation and photosystem II maintenance. Plant J. 42, 609–617. doi: 10.1111/j.1365-313X.2005.02401.x
Zaltsman, A., Ori, N., and Adam, Z. (2005b). Two types of FtsH protease subunits are required for chloroplast biogenesis and photosystem II repair in Arabidopsis. Plant Cell 17, 2782–2790. doi: 10.1105/tpc.105.035071
Keywords: chloroplast development, FtsH protease, photosystem II repair, photosynthesis, post-translational modification (PTM), reactive oxygen species (ROS)
Citation: Kato Y and Sakamoto W (2018) FtsH Protease in the Thylakoid Membrane: Physiological Functions and the Regulation of Protease Activity. Front. Plant Sci. 9:855. doi: 10.3389/fpls.2018.00855
Received: 26 April 2018; Accepted: 01 June 2018;
Published: 20 June 2018.
Edited by:
Peter Jahns, Heinrich-Heine-Universität Düsseldorf, GermanyReviewed by:
Jean-David Rochaix, Université de Genève, SwitzerlandFranz Narberhaus, Ruhr-Universität Bochum, Germany
Copyright © 2018 Kato and Sakamoto. This is an open-access article distributed under the terms of the Creative Commons Attribution License (CC BY). The use, distribution or reproduction in other forums is permitted, provided the original author(s) and the copyright owner are credited and that the original publication in this journal is cited, in accordance with accepted academic practice. No use, distribution or reproduction is permitted which does not comply with these terms.
*Correspondence: Wataru Sakamoto, c2FrYUBva2F5YW1hLXUuYWMuanA=; c2FrYUByaWIub2theWFtYS11LmFjLmpw