- 1Key Laboratory of Biology and Genetic Improvement of Maize in Southwest Region, Maize Research Institute, Sichuan Agricultural University, Chengdu, China
- 2Institute of Animal Nutrition, Sichuan Agricultural University, Chengdu, China
The regenerative capacity of the embryonic callus, a complex quantitative trait, is one of the main limiting factors for maize transformation. This trait was decomposed into five traits, namely, green callus rate (GCR), callus differentiating rate (CDR), callus plantlet number (CPN), callus rooting rate (CRR), and callus browning rate (CBR). To dissect the genetic foundation of maize transformation, in this study multi-locus genome-wide association studies (GWAS) for the five traits were performed in a population of 144 inbred lines genotyped with 43,427 SNPs. Using the phenotypic values in three environments and best linear unbiased prediction (BLUP) values, as a result, a total of 127, 56, 160, and 130 significant quantitative trait nucleotides (QTNs) were identified by mrMLM, FASTmrEMMA, ISIS EM-BLASSO, and pLARmEB, respectively. Of these QTNs, 63 QTNs were commonly detected, including 15 across multiple environments and 58 across multiple methods. Allele distribution analysis showed that the proportion of superior alleles for 36 QTNs was <50% in 31 elite inbred lines. Meanwhile, these superior alleles had obviously additive effect on the regenerative capacity. This indicates that the regenerative capacity-related traits can be improved by proper integration of the superior alleles using marker-assisted selection. Moreover, a total of 40 candidate genes were found based on these common QTNs. Some annotated genes were previously reported to relate with auxin transport, cell fate, seed germination, or embryo development, especially, GRMZM2G108933 (WOX2) was found to promote maize transgenic embryonic callus regeneration. These identified candidate genes will contribute to a further understanding of the genetic foundation of maize embryonic callus regeneration.
Introduction
As one of the main crops for animals and humans, maize (Zea mays L.) is an important target for genetic manipulation (Zhang et al., 2014; Li et al., 2016). However, during maize transformation, difficulty in embryonic callus induction and regeneration, which occurs in most elite lines, presents a major bottleneck (Shen et al., 2012, 2013; Ge et al., 2016). Previous studies have suggested that both genotypes and exogenous hormones affect embryonic callus induction from maize immature embryos, such as abscisic acid (ABA), indole acetic acid (IAA), and gibberellic acid (GA3) widely considered to play important roles in callus formation (Jiménez and Bangerth, 2001; Ge et al., 2016). Genetic research has suggested that embryonic callus induction is controlled by nuclear genes in maize (Schlappi and Hohn, 1992). Furthermore, eight quantitative trait loci (QTL) and three epistatic interactions were found to control type I callus formation in a maize recombinant inbred line (RIL) population (Krakowsky et al., 2006). In previous studies, some transcription factors and microRNAs in hormone signal transduction pathways were found to regulate the process of embryonic callus induction (Shen et al., 2013; Ge et al., 2016). To date, research exploring callus regenerative capacity has mainly focused on Arabidopsis, rice, wheat, maize, and other plants. In Arabidopsis, PLT genes (PLETHORA) were proved to modulate the regenerative capacity by a two-step mechanism (Kareem et al., 2015). First, PLT3, PLT5, and PLT7 activated the expression of root stem cell regulators PLT1 and PLT2 to establish pluripotency and form shoot progenitors. Then, PLT3, PLT5, and PLT7 up-regulated the expression of shoot-promoting factor Cup-shaped cotyledon1 (CUC1) and Cup-shaped cotyledon2 (CUC2) to complete the shoot regeneration process. Inhibitor of cyclin-dependent kinase (ICK), a cyclin-dependent kinase (CDK) inhibitor, has been shown to enhance the regenerative capacity of Arabidopsis embryonic callus (Cheng et al., 2015). Moreover, WUSCHEL-related homeobox 5 (WOX5) expression in the quiescent center (QC) is considered as a marker of the root stem cell niche in Arabidopsis (Sarkar et al., 2007). In addition, as an AP2/ERF transcription factor, wound induced dedifferentiation1 (WIND1) promoted the Arabidopsis shoot regeneration by up-regulating the expression of enhancer of shoot regeenration1 (ESR1) gene which encoded another AP2/ERF transcription factor (Iwase et al., 2015, 2017). For wheat, genes controlling green shoot re-differentiation were mapped to chromosomal sites 3A, 5B, 2D, and 1B (Szakács et al., 1988). Additionally, QTL mapping showed that two QTLs on chromosomes 1 and 9 control green shoot re-differentiation in rice, with the latter considered to be a major locus (Ping et al., 1998). Nishimura et al. (2005) observed a main QTL encoding ferredoxin-nitrite reductase (NiR) which is responsible for regenerate ability in rice. Recently, WUSCHEL-related homeobox 2 (WOX2) and Baby Boom (BBM) genes were introduced into maize by genetic transformation, which resulted in the increased rate of resistant seedlings from transformed immature embryos (Lowe et al., 2016). So far, the genetic basis of plant regeneration has not been well understood especially for maize, in which few functional genes have been revealed to directly control embryonic callus regeneration. Therefore, more systematic studies are required to reveal the genetic basis of maize embryonic callus regenerative capacity.
Genome-wide association analysis (GWAS) is a useful tool in the dissection of complex traits (Abdel-Ghani et al., 2013; Pace et al., 2015). Using mixed linear model (MLM) and general linear model (GLM), 4 and 263 significant SNPs were found to be associated with root architecture traits at maize seedling stage, respectively. More specifically, GRMZM2G153722, which is located on chromosome 4, was found to contain nine significant SNPs that are likely expressed in the roots and shoots (Pace et al., 2015). When using GWAS, several genes that modulate maize leaf architecture were identified in a nested association mapping (NAM) population (Tian et al., 2011). GWAS also aided in the identification of 74 candidate genes associated with maize oil biosynthesis (Li et al., 2013). Furthermore, another study identified a total of 51 SNPs significantly associated with maize leaf blight by adopting a NAM population, with most of the candidate genes reported in previous studies as relating to plant disease resistance (Kump et al., 2011). To our knowledge, there is no study that has utilized GWAS when detecting the embryonic callus regenerative capacity until now.
In this study, four multi-locus GWAS approaches were used to dissect the genetic foundations for the five regenerative capacity-related traits in a natural population containing rich genetic information across multiple environments. Our objectives were: (i) to understand the significance of genotype, environment, and genotype × environment on traits relating to regenerative capacity; (ii) to identify significant quantitative trait nucleotides (QTNs) and candidate genes that modulate the five traits and resolve the genetic basis of maize embryonic callus regenerative capacity; and (iii) to analyze and compare the detection powers of different methods and identify the optimal multi-locus GWAS approach. To our knowledge, this is the first comprehensive study aimed at understanding the genetic basis of maize embryonic callus regenerative capacity using multi-locus GWAS approaches.
Materials and Methods
Plant Materials and Phenotypic Data Analysis
In a previous study, we examined the embryonic callus induction rate in immature embryos from a natural maize population of 362 inbred lines, with 144 of the lines exhibiting efficient induction (Table S1) and thus they were used to detect regenerative capacity. The details of planting and culturing processes were described by Zhang et al. (2017b). Herein, five regeneration ability-related traits, namely, embryonic green callus rate (GCR), callus differentiating rate (CDR), callus plantlet number (CPN), callus rooting rate (CRR), and callus browning rate (CBR), were examined (the features of the five traits were shown in Figure 1). The data were transformed as previously described with the GCR, CDR, CRR, and CBR values calculated by and the CPN value calculated by , with p being the initial value (Zhang et al., 2017b). The analysis of variance (ANOVA), phenotypic correlation, BLUP values and broad-sense heritability () were all completed in our previous study (Zhang et al., 2017b).

Figure 1. Features of the five traits. The traits include CBR (callus browning rate), CDR (callus differentiating rate), CPN (callus plantlet number), CRR (callus rooting rate), and GCR (green callus rate).
Genotypic Data Analysis
Genomic DNA was extracted from mixed leaf tissues from eight plants per line using the CTAB method (Zhang et al., 2016). All of the accessions were genotyped using the Illumina MaizeSNP50 BeadChip containing 56,110 SNPs (http://support.illumina.com/array/array_kits/maizesnp50_dna_analysis_kit/downloads.html). A total of 43,427 SNPs across 10 chromosomes remained after quality filtering (Figure S1), with SNPs having a missing rate >20%, heterozygosity >20%, and minor allele frequency (MAF) < 0.05 deleted. These 43,427 SNPs were subsequently used for calculating the population structure and kinship and to perform GWAS.
Population Structure, Linkage Disequilibrium, and Multi-Locus Association Studies
STRUCTURE 2.3.4 was used to estimate subgroup numbers within the population structure (Q matrix) (Evanno et al., 2005). Among the 43,427 SNPs, 5,000 high quality SNPs with a rare allele frequency (RAF) >20% were randomly selected for the estimating panel. Based on the subgrouping results, the obtained evaluated data were used for further analysis.
TASSEL 4.0 was utilized to analyze linkage disequilibrium (LD) (Bradbury et al., 2007), with the LD decay calculated by plotting r2 onto the genetic distance in base pairs with a cutoff of r2 = 0.2. The LD decay was calculated using only markers that remained after quality filtering. Additionally, the Loiselle kinship coefficients between inbred lines in a panel (K matrix) were calculated using SpAGeDi software (Hardy and Vekemans, 2002).
In this study, four multi-locus GWAS approaches were used to detect significant QTNs for five embryonic callus regenerative capacity-related traits (mrMLM v2.1, https://cran.r-project.org/web/packages/mrMLM/index.html), including mrMLM (Wang et al., 2016), FASTmrEMMA (Wen et al., 2017), ISIS EM-BLASSO (Tamba et al., 2017), and pLARmEB (Zhang et al., 2017a). Owing to the fact that these multi-locus methods were more powerful and accurate than the single-locus MLM methods in their simulation experiments, thus we adopted these multi-locus methods in this study. Moreover, Q- and K-matrices were applied to correct the population structure and Loiselle kinship coefficients that were calculated between inbred lines. The setting parameters for these methods were as follows: (i) mrMLM, critical P-value of 0.01 in rMLM and critical LOD score of 3.0 in mrMLM (Wang et al., 2016); (ii) FASTmrEMMA, critical P-value of 0.005 in first step of FASTmrEMMA and critical LOD score of 3.0 in the last step of FASTmrEMMA (Wen et al., 2017); (iii) ISIS EM-BLASSO, critical P-value of 0.0002 in ISIS EM-BLASSO (Tamba et al., 2017); and (iv) pLARmEB, critical LOD score of 3.0 in pLARmEB and the number of potentially associated variables for each chromosome: 143 (“144–1”) (Zhang et al., 2017a).
Superior Allele Analysis and Annotation of Candidate Genes
For QTNs (RefGen_v2) that were detected consistently in multiple environments or methods, a superior genotype was determined based on the effect value of each significant QTN. For each QTN, the superior allele percentage in these elite inbred lines was equal to number of lines containing superior alleles divided by the total line number. For each line, the proportion of superior alleles in these QTNs was calculated as superior allele number divided by total QTN number. A heat map visualizing the percentage of superior alleles was obtained in the R (heatmap package) program (Mellbye and Schuster, 2014).
Herein, the QTNs which locate in gene regions were used to identify the candidate genes. Furthermore, the corresponding candidate genes of the consistent QTNs that were stably expressed in multi-environment or multi-method were annotated by performing a GENE search on the NCBI website (RefGen_v2) (https://www.ncbi.nlm.nih.gov/).
Real-Time PCR for Candidate Genes
Four candidate genes GRMZM2G108933 (WOX2), GRMZM2G066749, GRMZM2G163761, and GRMZM2G371033 were randomly selected for identification of expression levels at different regeneration stages (0 d, 3 d, 6 d, and 9 d) by quantitative real-time PCR analysis (qPCR, ABI 7500 real-time PCR System, Torrance, CA, USA). Firstly, RNA samples were extracted using TRIZOL reagent (Invitrogen, Beijing, China) and RNase-free DNase (Takara, Beijing, China). Then, cDNA was obtained by PrimeScript RT Reagent Kit With gDNA Eraser (TaKaRa, Beijing, China). Moreover, the primers were designed using the software Primer Premier 5.0. The detailed PCR amplification programmes were described as Shen et al. (2012), and the 2−ΔΔCt method was used for calculating the expression levels (Schefe et al., 2006). Here, Actin 1 (GRMZM2G126010) was used as the reference gene.
Results
Phenotype for Regenerative Capacity-Related Traits
The phenotypes for CBR, CDR, CPN, CRR, and GCR have been described by Zhang et al. (2017b), readers are encouraged to refer to the original study (Zhang et al., 2017b). The results were briefly described here. The average values for the above five traits across three environments were 37.70, 17.30, 1.28, 11.50, and 43.16 with the standard deviations 26.99, 17.52, 0.51, 14.25 and 24.94, respectively. Additionally, the heritability () of the five traits ranged from 47.09 to 78.91%, suggesting that genetic effects play an important role in the formation of these traits. A significantly positive correlation was observed between CDR and CPN, while a significantly negative correlation was found between CBR and GCR (P = 0.01). The high correlation coefficient between the BLUP value and the phenotypic value in a single environment (>0.9) indicated the reliability of the phenotypic values for most of the traits (Figure S2; Zhang et al., 2017b).
Linkage Disequilibrium Decay in the Population
To obtain the average distance of LD decay, 43,427 SNPs were adopted. As shown in Figure S3, r2 decreased gradually with increased distance. However, the r2-value reached a plateau when it decreased to a certain level. The corresponding distance was considered as the average distance of LD decay in this population. Herein, the average LD decay distance was 220 kb (r2 = 0.2), which is consistent with a previous study (Zhang et al., 2016). Moreover, the distance was greater than the average distance between markers of 48 kb, thus indicating sufficient coverage.
Population Structure
A subset of 5,000 high quality SNPs were randomly chosen to define the subpopulations within the panel of 144 lines. Delta K (ΔK) was calculated using STRUCTURE 2.3.4 (Figure 2A; K = 2–9), with two subpopulations (selected K = 2) presented based on ΔK-values (Figure 2B). These two subgroups contained 109 (75.69%) and 35 (24.31%) lines (Table S1), respectively. The larger subpopulation included tropical, temperate, and mixed germplasms, while the other was composed of mostly temperate lines (Table S1).
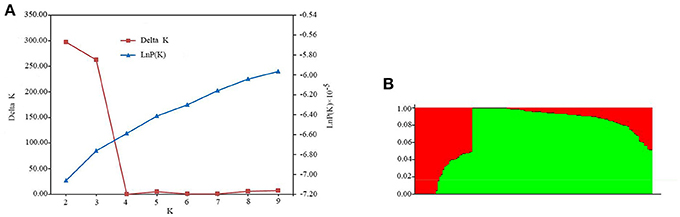
Figure 2. Population structure estimates based on 43,427 SNPs distributed across 10 chromosomes. (A) Plot of lnP(D), with ΔK calculated for K = 2–9. (B) Population structure estimates (K = 2), the areas of the two colors (green and red) illustrate the proportion of each subgroup.
QTNs Detected by Multi-Locus GWAS Methods
Four multi-locus GWAS approaches were utilized in this study. A total of 127, 56, 160, and 130 significant QTNs were identified in mrMLM, FASTmrEMMA, ISIS EM-BLASSO, and pLARmEB, respectively, for five traits across three environments and the BLUP model (Figures 3, 4, Figure S4, Tables S2–S5). Among them, 26, 29, 27, 16, and 29 QTNs were identified for CBR, CDR, CPN, CRR, and GCR, respectively, in multi-location and BLUP model by mrMLM method (Figure 3A; Table S2). When using FASTmrEMMA, the number of QTNs detected for the five traits were 14, 13, 7, 11, and 11, respectively (Figure 3B; Table S3). The ISIS EM-BLASSO method also identified 29, 37, 26, 31, and 37 QTNs for the above five traits (Figure 3C; Table S4). Moreover, 29, 28, 25, 27, and 21 QTNs were identified for the above five traits, respectively, using the pLARmEB approach (Figure 3D; Table S5).
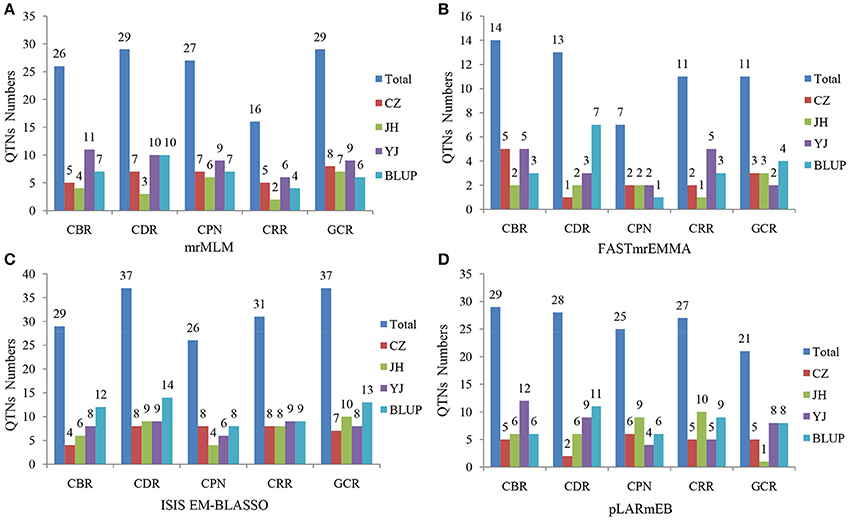
Figure 3. Number of detected QTNs for the five traits across three environments and BLUP model in four methods. The traits include CBR (callus browning rate), CDR (callus differentiating rate), CPN (callus plantlet number), CRR (callus rooting rate), and GCR (green callus rate). CZ, JH, and YJ denote the population planted in Chongzhou (2015), Jinghong (2014), and Yuanjiang (2015), respectively. The approaches utilized included (A) mrMLM, (B) FASTmrEMMA, (C) ISIS EM-BLASSO, and (D) pLARmEB.
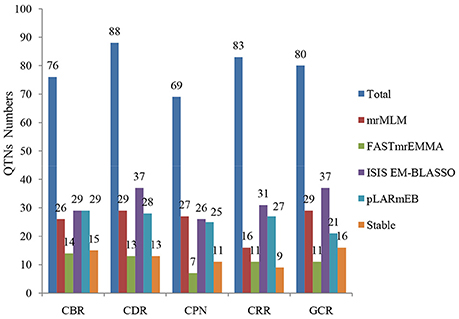
Figure 4. Comparison of the number of detected QTNs from the four methods. The four methods are mrMLM, FASTmrEMMA, ISIS EM-BLASSO, and pLARmEB. The traits include CBR (callus browning rate), CDR (callus differentiating rate), CPN (callus plantlet number), CRR (callus rooting rate), and GCR (green callus rate). Total: denotes the total QTN number for each trait and Stable: denotes the number of stably expressed QTNs across multiple methods for each trait.
We further analyzed the common QTNs that were co-identified in at least two of the environments (or environments and BLUP model) using a certain multi-locus GWAS approach. A total of 15 common QTNs were identified by combination of these four methods (Table 1). Among them, six, two, eight, and three environment-stable QTNs were identified using mrMLM, FASTmrEMMA, ISIS EM-BLASSO, and pLARmEB method, respectively (Table 1). These common QTNs were separately located on chromosomes 1, 2, 3, 4, 5, 7, 8, and 9, with LOD values ranging from 3.06 to 9.40 (Table 1). The proportion of phenotypic variance explained (PVE) by each QTN ranged from 1.83 to 19.26% (Table 1). Furthermore, three, four, two, six, and four common QTNs were found significantly associated with CBR, CDR, CPN, CRR, and GCR, respectively (Table 1).
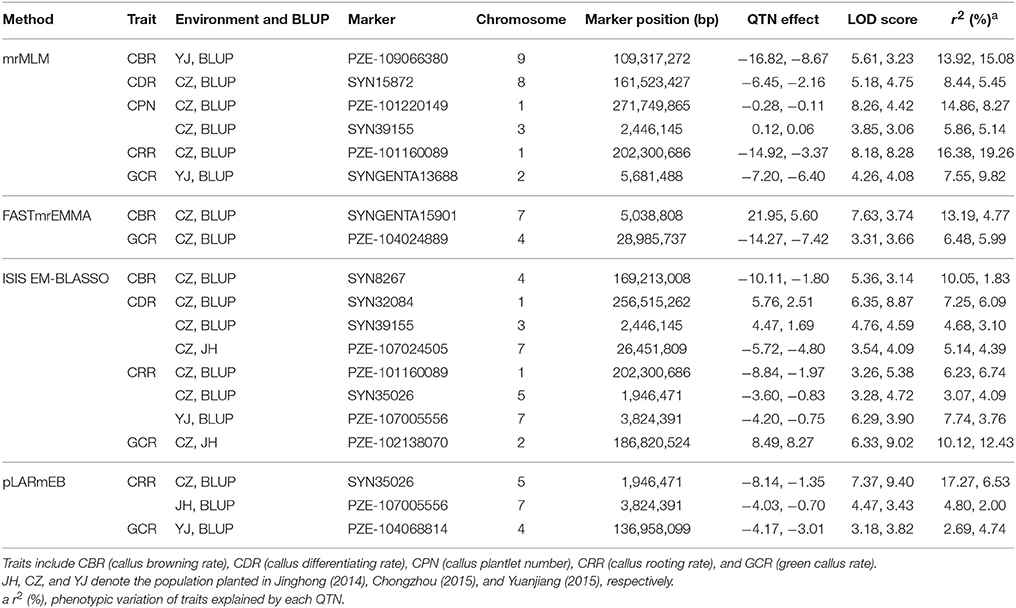
Table 1. Stably expressed QTNs for the five traits in each method across three environments and BLUP model.
When comparing the results across different methods, 58 QTNs were consistently identified by two or more methods (Table 2), and they were associated with the CBR (15), CDR (13), CPN (11), CRR (9), and GCR (16) traits (Figure 4; Table 2). Especially, three QTNs (SYNGENTA15901, SYN39155, and SYN32084) were found to be significantly associated with CBR, CDR, and CDR, respectively, in all the multi-locus methods (Table 2). Meanwhile, the average LOD-values and PVE ranges of the three QTNs for the CBR (5.69; 4.77–13.19%), CDR (4.68; 3.10–6.45%), and CDR (7.61; 2.65–7.25%) traits were also generated (Table 2).
Remarkably, 10 QTNs were co-detected not only in multi-environment (including environment and the BLUP model) but also by different methods (Table 3). Among these QTNs, the three QTNs (SYNGENTA15901, SYN39155, and SYN32084) were detected by all the methods as well as in BLUP model and CZ (Table 3). Furthermore, two other QTNs (SYN8267 and PZE-107005556) that are associated with CBR and CRR were identified by three methods and in two environments. The remaining six QTNs were associated with CPN, CRR, and GCR, and they were identified by two methods and found in two locations (Table 3).
Distribution of Superior Alleles in Elite Inbred Lines
The 63 common QTNs, detected in multiple environments or using multiple methods, were considered as important QTNs associated with regenerative capacity-related traits. Since 31 elite inbred lines were included in the constructed panel, this enabled us to evaluate the utilization of superior alleles during maize breeding. Herein, the allele associated with a higher phenotypic value was defined as the superior allele for each of the traits, except for CBR and CRR, because callus browning and callus rooting are both disadvantageous phenotypes for regeneration. As described in Table 4, the superior allele percentages for the QTNs ranged from 0.00 to 96.67% in the elite lines, with 27 of the QTNs containing ≥50% superior alleles while the remaining 36 QTNs contained <50% (Figure 5; Table 4). Three QTNs (PZE-101213720, PZE-103108199, and PZE-108021239) had superior allele percentages >80%, while eight (PZE-104066682, PZE-103049772, PZE-101220149, PZE-107024505, PZE-102109640, PZE-109067144, PZE-109121058, and PZE-109066380) had percentages <10% (Figure 5; Table 4).
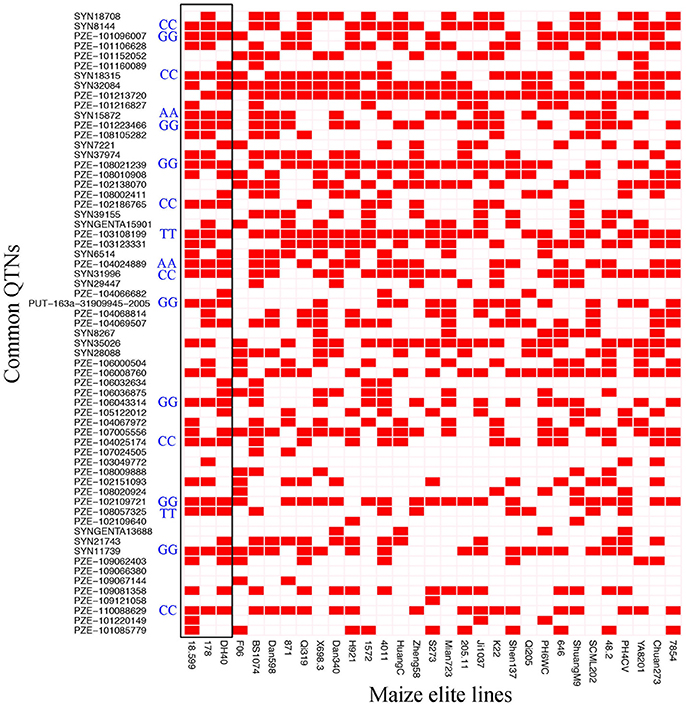
Figure 5. Heat map of the superior alleles distribution for the 63 QTNs in 31 elite inbred lines. Red and White colors represent superior and inferior alleles, respectively. Black box means the superior alleles distribution for the 63 QTNs in high-regeneration lines 18-599, 178, and DH40. AA, TT, GG, and CC represent the genotypes for common superior alleles in 18-599, 178, and DH40.
Moreover, 18 of the elite lines that contained 26–40 superior alleles showed higher phenotypic values, with increased percentages of 109.81% (CDR), 32.91% (CPN), and 75.63% (GCR), relative to the other 13 elite lines that contained 10–25 superior alleles (Table 5 and Table S6). However, for CBR and CRR, the 18 elite lines that contained between 26 and 40 superior alleles had the averaged phenotypic values of 34.17 and 9.68, respectively, which were 26.08 and 28.55% lower than the other 13 elite lines that contained 10–25 superior alleles (Table 5 and Table S6). These findings suggest that the superior alleles have obviously additive effects on regenerative capacity. Therefore, the maize callus regenerative capacity can be improved by increasing the numbers of superior alleles in the lines with low regenerative capacities by marker assisted selection (MAS). Among them, CDR and GCR are the most attractive traits for MAS modification due to them having the most significant enhancement effect. In addition, we found some lines with high regenerative capacity shared common superior alleles, such as lines 178, 18-599, and DH40 which all contained the superior alleles of SYN15872, PZE-104024889, PZE-103108199, PZE108057325, PZE-101096007, PZE-101223466, PZE-108021239, PUT-163a-31909945-2005, PZE-106043314, PZE-102109721, SYN11739, SYN8144, SYN18315, PZE-102186765, SYN31996, PZE-104025174, and PZE-110088629 (Figure 5). This suggested these superior alleles may play an important role in callus regeneration process. All these findings will be more useful in the application of superior alleles in maize breeding.
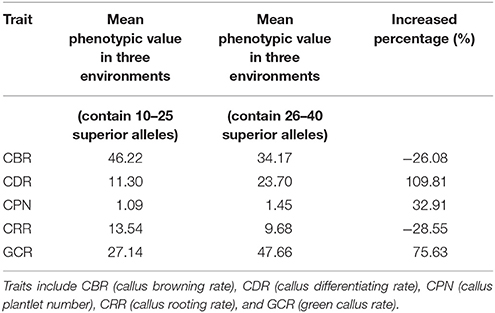
Table 5. Phenotypic values of different numbers of superior alleles for the five traits among the common QTNs within the 31 elite lines.
Candidate Genes Determined Based on Common QTNs
According to the 63 common QTNs, we further focused on the associated candidates. The results showed that a total of 40 candidate genes were obtained based on the B73 genome (RefGen_v2, Table 6). Among them, 8, 11, 7, 8, and 13 candidate genes were associated with CBR, CDR, CPN, CRR, and GCR, respectively (Table 6). Moreover, one QTN correlated with the CDR trait was associated with GRMZM2G589579 and had the largest LOD-value of 16.25 (Tables 2, 6). Based on the functional annotations, these genes were mainly classified as transcription factors and kinases (Table 6). Specifically, seven genes were located on chromosomes 1, 2, 3, and 6, with each associated with two of the regeneration capacity-related traits (Table 6). In detail, gene models GRMZM2G108933, GRMZM2G072264, and GRMZM2G026095 were individually correlated with both CBR and GCR, while GRMZM2G309660 was associated with CBR and CRR (Table 6). Moreover, GRMZM2G163761 was correlated with CDR and GCR, while GRMZM2G097959 and GRMZM5G835276 were associated with CDR and CPN (Table 6).
Expression Patterns of Candidate Genes
To detect the responses of the candidate genes to callus regeneration, two lines 141 (with high regenerative capacity) and ZYDH381-1 (with low regenerative capacity) were submitted to qRT-PCR analysis for four randomly selected genes at three regenerative stages (3 d, 6 d, and 9 d) and CK (0 d). Among these genes, WOX2 was up-regulated at all of the stages compared to 0 d in 141 and ZYDH381-1. However, the expression level of WOX2 in line 141 was higher than that in ZYDH381-1 at each of the stages. Besides, the expression peak occurred at 3 d in 141, which was at 6 d in ZYDH381. These indicate that WOX2 was more susceptive in the response of callus regeneration in 141 (Figure 6A). GRMZM2G066749 was down-regulated at every of regenerative stage when compared with 0 d in 141, whereas it was slightly up-regulated in ZYDH381-1. Interestingly, the expression level of GRMZM2G066749 in 141 was much higher than that in ZYDH381-1 at all of the stages including 0 d (Figure 6B). However, the expression levels of GRMZM2G163761 and GRMZM2G371033 were generally higher in ZYDH381-1 than those in 141 (Figures 6C,D). These findings suggested that the difference of expression patterns in different lines could be an important factor which influenced the regenerative capacity of embryonic callus.
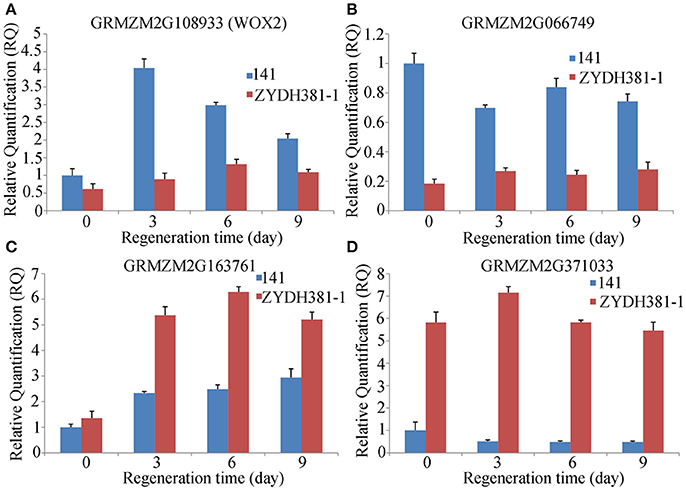
Figure 6. Expression levels of candidate genes at different regeneration stages. Here, 141 is a line with high regeneration and ZYDH381-1 IS the one with low regeneration. (A–D) Represents the relative expression levels of GRMZM2G108933 (WOX2), GRMZM2G066749, GRMZM2G163761 and GRMZM2G371033, respectively.
Discussion
Population Selection
A population of 144 inbred lines was used for the present study, which is slightly smaller than in other maize GWAS studies (Pace et al., 2014, 2015; Zhang et al., 2016). This is due to the specialty of these maize callus regenerative ability-related traits, which are based on the embryonic callus induction. In our previous study, 362 inbred lines were utilized to identify embryonic callus induction, and only 144 lines had a relatively efficient induction. Therefore, the present study is based on a comparatively small maize population. Moreover, population structure analysis showed that this novel population was divided into two subpopulations. The average LD decay distance was 220 kb (r2 = 0.2), which was relatively consistent with the distance obtained for the initial population of 362 inbred lines (Zhang et al., 2016). This finding indicates that the average LD decay distance is almost stable despite the reduced population size. Additionally, some QTNs for the five traits were co-identified in different methods and multi-environment (in Results section). Of particular interest is the candidate gene WOX2 (in Candidate Gene Functions in Callus Regenerative Capacity section), which has been proven to promote the formation of resistant seedlings after callus transformation. These findings confirm the reasonability of the population structure used for this study.
Advantages of the New Multi-Locus GWAS Approaches
Previous studies have dissected some complex traits using a GLM or MLM based on a single-locus GWAS (Zhang et al., 2005; Yu et al., 2006; Pace et al., 2015). However, both of these two models have procedural limitations. GLM has a high false positive rate (FPR) because this model does not correct the population structure (Q) or polygenic background (K; Korte and Farlow, 2013). In the MLM, the correction of Q and K is so stringent that many significant loci are missed, especially small-effect loci (Wang et al., 2016). In recent years, researchers developed some multi-locus methodologies to address these limitations, such as mrMLM, FASTmrEMMA, ISIS EM-BLASSO, and pLARmEB (Wang et al., 2016; Tamba et al., 2017; Wen et al., 2017; Zhang et al., 2017a), and they were used in this study. In these new multi-locus methods, the significance level was set to a LOD score = 3, which was equivalent to P = 0.0002 (Wang et al., 2016). However, in the single-locus MLM GWAS methods, the significance threshold is generally set to P = 0.05/m (m is the number of markers), thus the multi-locus GWAS methods are less stringent. Furthermore, FPRs for these four multi-locus GWAS approaches were smaller than in the single-locus MLM GWAS methods and other multi-locus GWAS methods (Wang et al., 2016; Tamba et al., 2017; Wen et al., 2017; Zhang et al., 2017a). Therefore, these methods were considered effective alternative approaches (Wang et al., 2016; Tamba et al., 2017; Wen et al., 2017; Zhang et al., 2017a). In this study, 127, 56, 160, and 130 significant QTNs were found for the five traits using mrMLM, FASTmrEMMA, ISIS EM-BLASSO, and pLARmEB, respectively (Figure 3; Tables S2–S5). However, only one and six significantly associated SNPs were detected when using MLM (R package GAPIT) and FarmCPU (R package FarmCPU; PCA+K, where PCA and K were calculated by GAPIT and SpAGeDi, respectively) models, respectively (P = 0.05/43427 = 1.15 × 10−6; Table S7). This suggested that these multi-locus methods were more powerful when used for detecting the QTNs for regeneration-related traits of maize. Furthermore, some stably expressed QTNs were commonly detected in multiple environments (or between environment and the BLUP model) (Table 1) and a total of 58 common QTNs were identified by multiple methods (Table 2). These evidence verified the reliability of these new multi-locus methods. Comparison of the four methods illustrates that ISIS EM-BLASSO is slightly more powerful than the other three methods (Figure 3). Additionally, the running time for these four methods when using the data generated herein are as follows: mrMLM > FASTmrEMMA > pLARmEB > ISIS EM-BLASSO (Figure S5). Notably, the validated functional gene WOX2 (mentioned above) was commonly detected in multiple methods for both CBR and GCR. These findings suggest that the most robust approach enabling the identification of the most interesting candidate genes is to use a combination of the methods utilized herein.
Application of Superior Alleles in Maize Breeding
When examining the common QTNs within the 31 elite inbred lines, 36 of the 63 QTNs contained <50% superior alleles (Table 4), suggested that these alleles were not effectively selected during artificial selection. A possible reason is that maize regenerative ability was not previously a main breeding focus. Instead, breeding efforts have focused on yield-related traits, plant type-related traits, resistance-related traits, and high quality-related traits. In the remaining 27 common QTNs, superior alleles proportions ≥50% was observed, with three of these QTNs (PZE-101213720, PZE-103108199, and PZE-108021239) having proportions >80% (Table 4). These findings suggest that in some cases, these superior alleles must be linked with traits of interest for breeders and thus were maintained during artificial selection.
The results presented herein show that the identified superior alleles exhibited additive effects on the regenerative capacity. Furthermore, this study focused on the number of superior alleles in several popular inbred lines (Zheng 58, PH4CV, and PH6WC), whose high yields and high combining abilities were outstanding (Barker, 2005; Ma et al., 2014; Li et al., 2017). The results showed that for each line, the superior allele proportion was <50% in the 63 QTNs (Figure 5, Table S6). Future studies could focus on these lines acquiring more super alleles and an improved regenerative ability that will contribute to the establishment of callus regeneration and a transformation system. These findings also enable the furthering of gene functional research in these lines.
We further investigated the distribution of these superior alleles in those lines that failed to induce the callus. As a result, the proportions of the superior alleles in different lines ranged from 20.64 to 57.14% and the average value was 40.27%, which was very similar to the averaged proportion (40.96%) of superior alleles in the 144 inducible lines. In addition, the averaged proportion of superior alleles for WOX2 (PZE-103123331) was 69.29 and 70.14%, respectively, in the uninduciable and induciable lines (these data were not provided). These suggested that these QTNs associated with callus regeneration were probably not involved in the induction of embryonic callus.
Candidate Genes Involved in Callus Regenerative Capacity
Based on the identified common QTNs, 40 candidate genes were identified, with several previously reported to be associated with transgenic callus regeneration, auxin transport, cell fate, seed germination, or embryo development (Table 6). These gene included GRMZM2G108933, GRMZM2G130442, GRMZM2G315375, GRMZM2G163761, GRMZM2G412611, GRMZM2G066749, and GRMZM2G371033. GRMZM2G108933, which was associated with CBR and CDR, was annotated to WOX2, an embryonic transcription factor (Nardmann et al., 2007) (Table 6). In Arabidopsis, WOX5 is closely associated with the root stem cell niche (Sarkar et al., 2007). In the recent year, WOX2 (a homologous gene to GRMZM2G108933) was introduced into maize by genetic transformation, and it increased the rate of resistant seedlings from transformed immature embryos (Lowe et al., 2016). These findings suggest that GRMZM2G108933 could be an important functional gene controlling maize callus regeneration by inhibiting callus browning and promoting callus differentiation. GRMZM2G130442 (associated with GCR) and GRMZM2G315375 (associated with CRR) are thought to regulate plant embryo development, which is consistent with their assigned associations herein (Table 6). As a member of the HD-Zip (homeo domain-leucine zipper) family, GRMZM2G130442 was annotated to the Zea mays outer cell layer (ZmOCL) family (Table 6), which has been reported to play roles in defining different regions of the epidermis during embryonic development and it controls the maintenance of cell-layer identity in meristematic regions (Ingram et al., 2000). GRMZM2G315375, known as br2, encodes P-glycoproteins (PGPs) (Table 6), which has been implicated in auxin transport. Meanwhile, auxin is widely accepted to be one of the most important hormones for embryo dedifferentiation (Pasternak et al., 2002). Moreover, Cassani et al. (2011) proposed that the interaction between br 2 and br 3 results in an alteration in embryo development. Regeneration is a process involving callus re-differentiation and it is similar to embryo development, but the opposite of embryo dedifferentiation (Yang et al., 2012). Therefore, these findings suggest that GRMZM2G130442 and GRMZM2G315375 could be modulators of callus regeneration.
Gene model GRMZM2G163761 was annotated to KIP1 (knotted interacting protein1) and was associated with CDR and GCR (Table 6). Smith et al. (2002) reported that cell fate in the shoot apical meristem is influenced by the transcriptional regulation from the association of KIP and KN1 (knotted 1), a three amino acid loop extension (TALE) class of homeodomain. Another candidate gene, GRMZM2G412611, which was correlated with CDR was annotated as an alpha-glucan water dikinase 1, chloroplastic-like (Table 6). In wheat, the suppression of glucan water dikinase in the endosperm altered the wheat grain properties, germination, and coleoptile growth (Bowerman et al., 2016). The CDR-associated gene, GRMZM2G066749, was annotated to dek 35 (defective kernel 35) (Table 6). Clark and Sheridan (1988) demonstrated that dek 35 is pleiotropic when affecting endosperm, gametophyte, or embryo development by using two non-allelic defective-kernel mutants of maize. These findings indicate that the above genes probably control the callus regenerative capacity by affecting cell fate determination or development of somatic embryo.
Conclusions
In this study, four new multi-locus GWAS methods were used to identify traits related to regenerative capacity. A total of 127, 56, 160, and 130 significant QTNs, respectively, were identified in mrMLM, FASTmrEMMA, ISIS EM-BLASSO, and pLARmEB for five traits across three environments and the BLUP model. Among these QTNs, 63 were commonly detected in multiple environments or using multiple methods. In total, 40 candidate genes were obtained based on the common QTNs, with several previously reported to correlate with transgenic callus regeneration, auxin transport, or embryo development. For the common QTNs, the percentages of superior alleles ranged from 0.00 to 96.67% within the 31 elite inbred lines. Further analysis revealed that these superior alleles exhibit an additive effect on the regenerative capability of the related traits. These findings suggest that an improvement of the maize callus regenerative ability can be achieved by integrating more superior alleles into maize lines by MAS.
Author Contributions
YS and GP: Designed the experiments; LM, ML, XZ, YY, CQ, YZ, YL, LW, LP, CZ, ZL, YW, and HP: Conducted the experiments and performed the analysis. LM, ML, and YS: Drafted the manuscript. All of the authors provided final approval of this manuscript.
Funding
This work was supported by the Young Scientists Fund of Sichuan Province (2016JQ0008), the National Basic Research Program of China (2014CB138200), and the Key Fund of the Department of Education of Sichuan Province (15ZA0004).
Conflict of Interest Statement
The authors declare that the research was conducted in the absence of any commercial or financial relationships that could be construed as a potential conflict of interest.
Acknowledgments
We would like to thank the Maize Research Institute of Sichuan Agricultural University for providing the platform. We also extend thanks to other graduate students who attended this project.
Supplementary Material
The Supplementary Material for this article can be found online at: https://www.frontiersin.org/articles/10.3389/fpls.2018.00561/full#supplementary-material
Figure S1. Distribution of 43,427 SNPs on 10 chromosomes.
Figure S2. Predictions of the five traits in three environments by BLUP (Zhang et al., 2017b). The traits include CBR (callus browning rate), CDR (callus differentiating rate), CPN (callus plantlet number), CRR (callus rooting rate), and GCR (green callus rate). CZ, JH, and YJ denote the population planted in Chongzhou (2015), Jinghong (2014), and Yuanjiang (2015), respectively. R2 denotes the correlation coefficient between the phenotype value mean and corresponding BLUP-value.
Figure S3. Linkage disequilibrium decay in the mapping population. A cutoff of r2 = 0.2 was utilized.
Figure S4. Manhattan plot of multi-locus GWAS for the five traits. The plots show all of the significant QTNs (LOD score > 3) across three environments and the BLUP model for the four methods (mrMLM, FASTmrEMMA, ISIS EM-BLASSO, and pLARmEB). CZ, JH, and YJ denote the population planted in Chongzhou (2015), Jinghong (2014), and Yuanjiang (2015), respectively. Panels (A–E) denotes the significant QTNs for CBR (callus browning rate), CDR (callus differentiating rate), CPN (callus plantlet number), CRR (callus rooting rate), and GCR (green callus rate), respectively.
Figure S5. Running times for the four methods using 43,427 SNPs.
Table S1. Inbred lines and population Q matrix.
Table S2. Significant QTNs for the five traits across three environments and the BLUP model using the mrMLM method. The traits include CBR (callus browning rate), CDR (callus differentiating rate), CPN (callus plantlet number), CRR (callus rooting rate), and GCR (green callus rate). JH, CZ, and YJ denote the population planted in Jinghong (2014), Chongzhou (2015), and Yuanjiang (2015), respectively. r2 (%), phenotypic variation of traits explained by each QTN.
Table S3. Significant QTNs for the five traits across three environments and the BLUP model using the FASTmrEMMA method. The traits include CBR (callus browning rate), CDR (callus differentiating rate), CPN (callus plantlet number), CRR (callus rooting rate), and GCR (green callus rate). JH, CZ, and YJ denote the population planted in Jinghong (2014), Chongzhou (2015), and Yuanjiang (2015), respectively. r2 (%), phenotypic variation of traits explained by each QTN.
Table S4. Significant QTNs for the five traits across three environments and the BLUP model using the ISIS EM-BLASSO method. The traits include CBR (callus browning rate), CDR (callus differentiating rate), CPN (callus plantlet number), CRR (callus rooting rate), and GCR (green callus rate). JH, CZ, and YJ denote the population planted in Jinghong (2014), Chongzhou (2015), and Yuanjiang (2015), respectively. r2 (%), phenotypic variation of traits explained by each QTN.
Table S5. Significant QTNs for the five traits across three environments and the BLUP model using the pLARmEB method. The traits include CBR (callus browning rate), CDR (callus differentiating rate), CPN (callus plantlet number), CRR (callus rooting rate), and GCR (green callus rate). JH, CZ, and YJ denote the population planted in Jinghong (2014), Chongzhou (2015), and Yuanjiang (2015), respectively. r2 (%), phenotypic variation of traits explained by each QTN.
Table S6. Phenotypic value and superior allele numbers for the 31 elite lines for each trait. The traits include CBR (callus browning rate), CDR (callus differentiating rate), CPN (callus plantlet number), CRR (callus rooting rate), and GCR (green callus rate).
Table S7. SNPs significantly associated with traits detected by GWAS using statistical models MLM and FarmCPU. The traits include CPN (callus plantlet number) and GCR (green callus rate).
References
Abdel-Ghani, A. H., Kumar, B., Reyesmatamoros, J., Gonzalezportilla, P. J., Jansen, C., Jps, M., et al. (2013). Genotypic variation and relationships between seedling and adult plant traits in maize (Zea mays L.) inbred lines grown under contrasting nitrogen levels. Euphytica 189, 123–133. doi: 10.1007/s10681-012-0759-0
Barker, T. C. (2005). Inbred Maize Line PH4CV: U.S. Patent No. 6,897,363. Washington, DC: U.S. patent and Tademark Office.
Bowerman, A. F., Newberry, M., Dielen, A. S., Whan, A., Larroque, O., Pritchard, J., et al. (2016). Suppression of glucan, water dikinase in the endosperm alters wheat grain properties, germination and coleoptile growth. Plant Biotechnol. J. 14, 398–408. doi: 10.1111/pbi.12394
Bradbury, P. J., Zhang, Z., Kroon, D. E., Casstevens, T. M., Ramdoss, Y., and Buckler, E. S. (2007). TASSEL: software for association mapping of complex traits in diverse samples. Bioinformatics 23, 2633–2635. doi: 10.1093/bioinformatics/btm308
Cassani, E., Villa, D., Durante, M., Landoni, M., and Pilu, R. (2011). The brachytic 2 and 3 maize double mutant shows alterations in plant growth and embryo development. Plant Growth Regul. 64, 185–192. doi: 10.1007/s10725-010-9556-8
Cheng, Y., Liu, H., Cao, L., Wang, S., Li, Y., Zhang, Y., et al. (2015). Down-regulation of multiple CDK inhibitor ICK/KRP genes promotes cell proliferation, callus induction and plant regeneration in Arabidopsis. Front. Plant Sci. 6:825. doi: 10.3389/fpls.2015.00825
Clark, J. K., and Sheridan, W. F. (1988). Characterization of the two maize embryo-lethal defective kernel mutants rgh*-1210 and fl*-1253b: effects on embryo and gametophyte development. Genetics 120, 279–290.
Evanno, G., Regnaut, S., and Goudet, J. (2005). Detecting the number of clusters of individuals using the software structure: a simulation study. Mol. Ecol. 14, 2611–2620. doi: 10.1111/j.1365-294X.2005.02553.x
Ge, F., Luo, X., Huang, X., Zhang, Y., He, X., Liu, M., et al. (2016). Genome-wide analysis of transcription factors involved in maize embryonic callus formation. Physiol. Plant. 158, 452–462. doi: 10.1111/ppl.12470
Hardy, O. J., and Vekemans, X. (2002). SPAGeDi: a versatile computer program to analyse spatial genetic structure at the individual or population levels. Mol. Ecol. Resour. 2, 618–620. doi: 10.1046/j.1471-8286.2002.00305.x
Ingram, G. C., Boisnard-Lorig, C., Dumas, C., and Rogowsky, P. M. (2000). Expression patterns of genes encoding HD-ZipIV homeo domain proteins define specific domains in maize embryos and meristems. Plant J. 22, 401–414. doi: 10.1046/j.1365-313X.2000.00755.x
Iwase, A., Harashima, H., Ikeuchi, M., Rymen, B., Ohnuma, M., Komaki, S., et al. (2017). WIND1 promotes shoot regeneration through transcriptional activation of enhancer of shoot regeneration1 in Arabidopsis. Plant Cell 29, 54–69. doi: 10.1105/tpc.16.00623
Iwase, A., Mita, K., Nonaka, S., Ikeuchi, M., Koizuka, C., Ohnuma, M., et al. (2015). WIND1-based acquisition of regeneration competency in Arabidopsis and rapeseed. J. Plant Res. 128, 389–397. doi: 10.1007/s10265-015-0714-y
Jiménez, V. M., and Bangerth, F. (2001). Hormonal status of maize initial explants and of the embryogenic and non-embryogenic callus cultures derived from them as related to morphogenesis in vitro. Plant Sci. 160, 247–257. doi: 10.1016/S0168-9452(00)00382-4
Kareem, A., Durgaprasad, K., Sugimoto, K., Du, Y., Pulianmackal, A. J., Trivedi, Z. B., et al. (2015). PLETHORA genes control regeneration by a two-step mechanism. Curr. Biol. 25, 1017–1030. doi: 10.1016/j.cub.2015.02.022
Korte, A., and Farlow, A. (2013). The advantages and limitations of trait analysis with GWAS: a review. Plant Methods 9:29. doi: 10.1186/1746-4811-9-29
Krakowsky, M., Lee, M., Garay, L., Woodman-Clikeman, W., Long, M., Sharopova, N., et al. (2006). Quantitative trait loci for callus initiation and totipotency in maize (Zea mays L.). Theor. Appl. Genet. 113, 821–830. doi: 10.1007/s00122-006-0334-y
Kump, K. L., Bradbury, P. J., Wisser, R. J., Buckler, E. S., Belcher, A. R., Oropezarosas, M. A., et al. (2011). Genome-wide association study of quantitative resistance to southern leaf blight in the maize nested association mapping population. Nat. Genet. 43, 163–168. doi: 10.1038/ng.747
Li, H., Peng, Z., Yang, X., Wang, W., Fu, J., Wang, J., et al. (2013). Genome-wide association study dissects the genetic architecture of oil biosynthesis in maize kernels. Nat. Genet. 45, 43–50. doi: 10.1038/ng.2484
Li, K., Wang, H., Hu, X., Ma, F., Wu, Y., Wang, Q., et al. (2017). Genetic and quantitative trait locus analysis of cell wall components and forage digestibility in the Zheng 58 × HD568 maize RIL population at anthesis stage. Front. Plant Sci. 8:1472. doi: 10.3389/fpls.2017.01472
Li, X., Zhou, Z., Ding, J., Wu, Y., Zhou, B., Wang, R., et al. (2016). Combined linkage and association mapping reveals QTL and candidate genes for plant and ear height in maize. Front. Plant Sci. 7:833. doi: 10.3389/fpls.2016.00833
Lowe, K., Wu, E., Wang, N., Hoerster, G., Hastings, C., Cho, M.-J., et al. (2016). Morphogenic regulators Baby boom and Wuschel improve monocot transformation. Plant Cell 28, 1998–2015. doi: 10.1105/tpc.16.00124
Ma, D. L., Xie, R. Z., Niu, X. K., Li, S. K., Long, H. L., and Liu, Y. E. (2014). Changes in the morphological traits of maize genotypes in China between the 1950s and 2000s. Eur. J. Agron. 58, 1–10. doi: 10.1016/j.eja.2014.04.001
Mellbye, B., and Schuster, M. (2014). Physiological framework for the regulation of quorum sensing-dependent public goods in Pseudomonas aeruginosa. J. Bacteriol. Mycol. 196, 1155–1164. doi: 10.1128/JB.01223-13
Nardmann, J., Zimmermann, R., Durantini, D., Kranz, E., and Werr, W. (2007). WOX gene phylogeny in Poaceae: a comparative approach addressing leaf and embryo development. Mol. Biol. Evol. 24, 2474–2484. doi: 10.1093/molbev/msm182
Nishimura, A., Ashikari, M., Lin, S., Takashi, T., Angeles, E. R., Yamamoto, T., et al. (2005). Isolation of a rice regeneration quantitative trait loci gene and its application to transformation systems. Proc. Natl. Acad. Sci. U.S.A. 102, 11940–11944. doi: 10.1073/pnas.0504220102
Pace, J., Gardner, C., Romay, C., Ganapathysubramanian, B., and Lübberstedt, T. (2015). Genome-wide association analysis of seedling root development in maize (Zea mays L.). BMC Genomics 16:47. doi: 10.1186/s12864-015-1226-9
Pace, J., Lee, N., Naik, H. S., Ganapathysubramainian, B., and Lübberstedt, T. (2014). Analysis of maize (Zea mays) seedling roots with the high-throughput image analysis tool ARIA (Automatic Root Image Analysis). PLoS ONE 9:e108255. doi: 10.1371/journal.pone.0108255
Pasternak, T. P., Prinsen, E., Ayaydin, F., Miskolczi, P., Potters, G., Asard, H., et al. (2002). The role of auxin, pH, and stress in the activation of embryogenic cell division in leaf protoplast-derived cells of alfalfa. Plant Physiol. 129, 1807–1819. doi: 10.1104/pp.000810
Ping, H., Lishuang, S., Chaofu, L., Ying, C., and Lihuang, Z. (1998). Genetic analysis and mapping the anther calture response genes in rice (Oryza sativa L.). Acta Genet. Sin. 25, 337–344.
Sarkar, A. K., Luijten, M., Miyashima, S., Lenhard, M., Hashimoto, T., Nakajima, K., et al. (2007). Conserved factors regulate signalling in Arabidopsis thaliana shoot and root stem cell organizers. Nature 446, 811–814. doi: 10.1038/nature05703
Schefe, J. H., Lehmann, K. E., Buschmann, I. R., Unger, T., and Funke-Kaiser, H. (2006). Quantitative real-time RT-PCR data analysis: current concepts and the novel “gene expression's CT difference” formula. J. Mol. Med. 84, 901–910. doi: 10.1007/s00109-006-0097-6
Schlappi, M., and Hohn, B. (1992). Competence of immature maize embryos for Agrobacterium-mediated gene transfer. Plant Cell 4, 7–16. doi: 10.1105/tpc.4.1.7
Shen, Y., Jiang, Z., Lu, S., Lin, H., Gao, S., Peng, H., et al. (2013). Combined small RNA and degradome sequencing reveals microRNA regulation during immature maize embryo dedifferentiation. Biochem. Biophys. Res. Commun. 441, 425–430. doi: 10.1016/j.bbrc.2013.10.113
Shen, Y., Jiang, Z., Yao, X., Zhang, Z., Lin, H., Zhao, M., et al. (2012). Genome expression profile analysis of the immature maize embryo during dedifferentiation. PLoS ONE 7:e32237. doi: 10.1371/journal.pone.0032237
Smith, H. M., Boschke, I., and Hake, S. (2002). Selective interaction of plant homeodomain proteins mediates high DNA-binding affinity. Proc. Natl. Acad. Sci. U.S.A. 99, 9579–9584. doi: 10.1073/pnas.092271599
Szakács, E., Kovács, G., Pauk, J., and Barnabás, B. (1988). Substitution analysis of callus induction and plant regeneration from anther culture in wheat (Triticum aestivum L.). Plant Cell Rep. 7, 127–129. doi: 10.1007/BF00270121
Tamba, C. L., Ni, Y. L., and Zhang, Y. M. (2017). Iterative sure independence screening EM-Bayesian LASSO algorithm for multi-locus genome-wide association studies. PLoS Comput. Biol. 13:e1005357. doi: 10.1371/journal.pcbi.1005357
Tian, F., Bradbury, P. J., Brown, P. J., Hung, H., Sun, Q., Flintgarcia, S., et al. (2011). Genome-wide association study of leaf architecture in the maize nested association mapping population. Nat. Genet. 43, 159–162. doi: 10.1038/ng.746
Wang, S. B., Feng, J. Y., Ren, W. L., Huang, B., Zhou, L., Wen, Y. J., et al. (2016). Improving power and accuracy of genome-wide association studies via a multi-locus mixed linear model methodology. Sci. Rep. 6:19444. doi: 10.1038/srep19444
Wen, Y. J., Zhang, H., Ni, Y. L., Huang, B., Zhang, J., Feng, J. Y., et al. (2017). Methodological implementation of mixed linear models in multi-locus genome-wide association studies. Brief. Bioinform. bbw 18:908. doi: 10.1093/bib/bbx028
Yang, X., Zhang, X., Yuan, D., Jin, F., Zhang, Y., and Xu, J. (2012). Transcript profiling reveals complex auxin signalling pathway and transcription regulation involved in dedifferentiation and redifferentiation during somatic embryogenesis in cotton. BMC Plant Biol. 12:110. doi: 10.1186/1471-2229-12-110
Yu, J., Pressoir, G., Briggs, W. H., Bi, I. V., Yamasaki, M., Doebley, J. F., et al. (2006). A unified mixed-model method for association mapping that accounts for multiple levels of relatedness. Nat. Genet. 38, 203–208. doi: 10.1038/ng1702
Zhang, J., Feng, J. Y., Ni, Y. L., Wen, Y. J., Niu, Y., Tamba, C. L., et al. (2017a). pLARmEB: integration of least angle regression with empirical Bayes for multilocus genome-wide association studies. Heredity (Edinb). 118, 517–524. doi: 10.1038/hdy.2017.8
Zhang, Q., Zhang, L., Evers, J., Werf, W. V. D., Zhang, W., and Duan, L. (2014). Maize yield and quality in response to plant density and application of a novel plant growth regulator. Field Crops Res. 164, 82–89. doi: 10.1016/j.fcr.2014.06.006
Zhang, X., Long, Y., Ge, F., Guan, Z., Zhang, X., Wang, Y., et al. (2017b). A genetic study of the regeneration capacity of embryonic callus from the maize immature embryo culture. Hereditas 39, 143–155. doi: 10.16288/j.yczz.16-323
Zhang, X., Zhang, H., Li, L., Lan, H., Ren, Z., Liu, D., et al. (2016). Characterizing the population structure and genetic diversity of maize breeding germplasm in Southwest China using genome-wide SNP markers. BMC Genomics 17:697. doi: 10.1186/s12864-016-3041-3
Keywords: maize, embryonic callus, regenerative capacity, multi-locus GWAS, candidate gene
Citation: Ma L, Liu M, Yan Y, Qing C, Zhang X, Zhang Y, Long Y, Wang L, Pan L, Zou C, Li Z, Wang Y, Peng H, Pan G, Jiang Z and Shen Y (2018) Genetic Dissection of Maize Embryonic Callus Regenerative Capacity Using Multi-Locus Genome-Wide Association Studies. Front. Plant Sci. 9:561. doi: 10.3389/fpls.2018.00561
Received: 24 February 2018; Accepted: 10 April 2018;
Published: 26 April 2018.
Edited by:
Yuan-Ming Zhang, Huazhong Agricultural University, ChinaReviewed by:
Wenqin Wang, Shanghai Jiao Tong University, ChinaZhiyong Zhang, Rutgers University, The State University of New Jersey, United States
Haochuan Li, Henan Agricultural University, China
Copyright © 2018 Ma, Liu, Yan, Qing, Zhang, Zhang, Long, Wang, Pan, Zou, Li, Wang, Peng, Pan, Jiang and Shen. This is an open-access article distributed under the terms of the Creative Commons Attribution License (CC BY). The use, distribution or reproduction in other forums is permitted, provided the original author(s) and the copyright owner are credited and that the original publication in this journal is cited, in accordance with accepted academic practice. No use, distribution or reproduction is permitted which does not comply with these terms.
*Correspondence: Yaou Shen, c2hlbnlhb3VAc2ljYXUuZWR1LmNu
†These authors have contributed equally to this work.