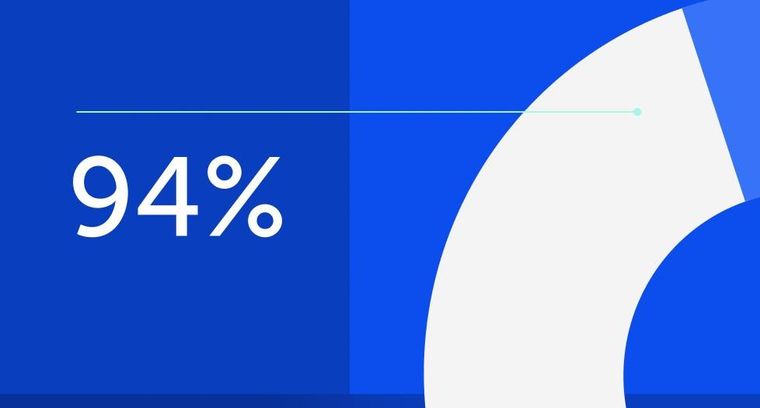
94% of researchers rate our articles as excellent or good
Learn more about the work of our research integrity team to safeguard the quality of each article we publish.
Find out more
REVIEW article
Front. Plant Sci., 30 January 2018
Sec. Plant Physiology
Volume 9 - 2018 | https://doi.org/10.3389/fpls.2018.00055
This article is part of the Research TopicStructure and Function of ChloroplastsView all 25 articles
Measurements of chlorophyll fluorescence provide an elegant and non-invasive means of probing the dynamics of photosynthesis. Advances in video imaging of chlorophyll fluorescence have now made it possible to study photosynthesis at all levels from individual cells to entire crop populations. Since the technology delivers quantitative data, is easily scaled up and can be readily combined with other approaches, it has become a powerful phenotyping tool for the identification of factors relevant to photosynthesis. Here, we review genetic chlorophyll fluorescence-based screens of libraries of Arabidopsis and Chlamydomonas mutants, discuss its application to high-throughput phenotyping in quantitative genetics and highlight potential future developments.
Since the discovery of the rapid fluorescence transient associated with the initial exposure of dark-adapted leaves to light (the Kautsky effect) in 1931, chlorophyll (Chl) fluorescence has emerged as an indispensable probe in photosynthesis research. There are several reasons for this remarkable development: (i) measurements of Chl fluorescence dynamics can be carried out on intact plants or algal cell cultures in an essentially non-invasive manner, (ii) multiple quantitative photosynthetic parameters can be extracted in short measuring times, (iii) Chl fluorescence measurements can be easily combined with other analytical tools, (iv) instrumentation capable of automated quantification and analysis of Chl fluorescence is now commercially available to a broad range of plant scientists and the technique is no longer restricted to a small group of experts, (v) technical advances achieved in recent decades now permit investigations from the single-cell level (Oxborough and Baker, 1997; Küpper et al., 2000; Tseng and Chu, 2017) to crop plants in the field (Virlet et al., 2017), and open up numerous applications, such as the use of Chl fluorescence-derived parameters as indicators of abiotic (Baker, 2008; Rungrat et al., 2016) or biotic stress (reviewed in: Chaerle et al., 2009). A particularly important technological breakthrough in this field was the development of video imaging systems (Omasa et al., 1987; Fenton and Crofts, 1990), which not only paved the way for the examination of the spatial heterogeneity within a sample, but also made it possible to assess large numbers of samples (e.g., individual plants or cell colonies) in a single experimental run. Thus, Chl fluorescence video imaging (CFVI) can be regarded as an ideal phenotyping technology for the identification of mutants affected in photosynthesis.
In the following, we will give an overview of CFVI-based screens which have been carried out on plant and green algal mutant libraries in the past, discuss recent progress and consider how the technology may be further developed in the future. Technical and theoretical aspects of Chl fluorescence imaging have been described in detail in Nedbal and Whitmarsh (2004), as well as in Oxborough (2004). The interested reader is also referred to several excellent review articles for introductions to the biophysical basis and biochemical implications of Chl fluorescence-derived photosynthetic parameters (Maxwell and Johnson, 2000; Roháček, 2002; Baker, 2008; Kalaji et al., 2017).
In brief, a typical state-of-the-art CFVI analysis is based on the application of pulse-amplitude-modulated (PAM), measuring light (ML), which is generated by a powerful array of LEDs placed in a defined working distance to the sample. Those LEDs can also serve for the generation of short saturation pulses (SPs) and for actinic illumination (AL) of the samples to drive photosynthesis. Emitted red Chl fluorescence is detected by a computer-connected charge-coupled device (CCD) video camera which is protected from excitation light or near-infrared radiation by appropriate color glass filters. Custom software allows the conversion of Chl fluorescence signals into false color images, calculation of different photosynthetic parameters and quantitative analyses of the results. In general, plant or algal samples are dark-adapted prior to the measurements to open all PSII reaction centers. Then, samples are exposed to ML for dark fluorescence yield (F0) determination and to a short SP for maximum fluorescence yield (Fm) measurement, respectively (see also Figure 1A). In this state, the PSII quantum yield (Fv/Fm) is maximal and can be calculated according to the equation Fv/Fm = (Fm-F0)/Fm. AL is switched on and application of SPs provides maximum fluorescence yields (Fm′) of illuminated samples. Effective PSII quantum yields (ΦII) are calculated by the equation ΦII = (Fm′-F)/Fm′ (Genty et al., 1989), whereas the fluorescence yield (F) is recorded every time shortly before a SP and represents an average of several current fluorescence yield (Ft) pictures. Electron transport rates through PSII [ETR(II)] at a given photosynthetically active radiation (PAR) can be calculated according to Schreiber et al. (1995), using the equation ETR(II) = ΦII × PAR × 0.84 × 0.5. Maximum ETR(II) measured at saturating light intensity provides an estimate of the maximum photosynthesis rate (Pmax). Fm′ values of illuminated samples are in general lowered compared to Fm by non-photochemical quenching (NPQ), which can be quantified according to the equation NPQ = (Fm-Fm′)/Fm′ (Bilger and Björkman, 1990). NPQ mechanisms can be further examined in dark relaxation experiments. To this end, actinic light is switched off after a period of actinic light exposure and minimum fluorescence (F0′′) and maximum fluorescence yields (Fm′′) are determined by application of SPs in the dark relaxation phase (Figure 1A). The two NPQ components qE (ΔpH-dependent feedback de-excitation, the major component of NPQ), and qI (photo-inhibitory quenching) can be calculated according to the equations qE = Fm/Fm′-Fm/Fm′′ (Thiele et al., 1997) and qI = (Fv-Fv′′)/Fv (Björkman and Demmig, 1987).
The first instance of the successful use of Chl fluorescence imaging to identify photosynthetic mutants was the detection of a ‘high-chlorophyll-fluorescence’ (hcf) phenotype in a population of methyl-methane sulfonate-mutagenized Chlamydomonas reinhardtii cells by Bennoun and Levine (1967) (Table 1). The screen was based on the fact that severe perturbations in photosynthetic electron transport, such as those caused by incubating algal cells in the presence of the photosynthetic electron transport inhibitor 3-(3,4-dichlorophenyl)-1,1-dimethylurea (DCMU), lead to high steady-state levels of Chl fluorescence. Following its application for screening of Chlamydomonas mutant libraries (see for example Harris, 1989) the concept was tested in higher plants (Miles and Daniel, 1973) and employed for the screening of maize (Miles and Daniel, 1974; Barkan et al., 1986; Taylor et al., 1987) and Arabidopsis mutant libraries (Dinkins et al., 1994; Meurer et al., 1996b). Several factors involved in chloroplast biogenesis were identified using the hcf phenotyping method, including the maize proteins HCF106, HCF60, and HCF136 (reviewed in: Belcher et al., 2015) and the Arabidopsis proteins HCF5 (Dinkins et al., 1997), HCF101 (Lezhneva et al., 2004), HCF107 (Felder et al., 2001), HCF109 (Meurer et al., 1996a), HCF145 (Lezhneva and Meurer, 2004; Manavski et al., 2015), HCF152 (Meierhoff et al., 2003) and LPA1 (Peng et al., 2006).
Even though such hcf mutant screens can be performed rapidly and efficiently, and have significantly enhanced our knowledge of the molecular repertoire required for photosynthesis and chloroplast biogenesis, only mutants with severe defects can be unequivocally detected, and these are often lethal under photoautotrophic conditions. However, technological progress in Chl fluorescence analyses during the 1980s and 1990s allowed the technique to be employed for more elaborate modes of screening, and led to the identification of algal or plant mutants with relatively modest alterations in photosynthetic performance. For example, Varotto et al. (2000a,b) identified ‘photosynthesis affected mutants’ (pam) in Arabidopsis on the basis of their lower effective quantum yields (ΦII) (Genty et al., 1989) using a combination of a pulse-amplitude-modulation fluorometer (Schreiber et al., 1986) and an automated screening system. This set-up facilitated the screening of large En transposon or T-DNA mutagenized Arabidopsis populations, and pam mutants disrupted in the nucleus-encoded photosystem I subunits PsaE1 (pam4) (Varotto et al., 2000b) and PsaD1 (pam62) (Ihnatowicz et al., 2004), the metal-ion transporter IRT1 (pam25) (Varotto et al., 2002), and the cytoplasmic N-acetyltransferase AtMAK3 (pam21) (Pesaresi et al., 2003), as well as the PSII assembly factor PAM68 (pam68) (Armbruster et al., 2010), were isolated and functionally characterized in subsequent studies.
Due to their sessile lifestyle, many multicellular photosynthetic organisms have evolved various strategies to cope with light stress (Ort, 2001). When the photosynthetic machinery is exposed to excessively high levels of light, short- and long-term adaptive responses are triggered at the molecular level, which allow for the thermal dissipation of excited energy by NPQ mechanisms to prevent over-reduction of the electron transport chain. At least four processes contribute to NPQ: qE, qZ (zeaxanthin-dependent quenching), qT (state-transition-dependent quenching) and qI (reviewed in: Ruban, 2016). Several CFVI-based screens have been performed on mutagenized Chlamydomonas and Arabidopsis populations with the aim of dissecting the genetics of NPQ (Table 1). Mutant identification was essentially based on the comparison of two video images of Chl fluorescence captured under different illumination conditions. The first picture was taken in the dark-adapted state during a saturating light pulse (Fm), or shortly after the onset of high-light treatment (F). The second image was recorded after several minutes of exposure to high light either during a saturating light pulse (Fm′) or not (F′). The NPQ values derived using the equation (F -F′)/F′ or (Fm-Fm′)/Fm′ were then visualized as false-color images, and several Chlamydomonas and Arabidopsis mutants affected in NPQ of excited Chl states could be identified. Subsequent analyses revealed three distinct groups of mutants with aberrant NPQ (reviewed in: Golan et al., 2004). Mutants in the first group were impaired in the generation of a proton gradient across the thylakoid membrane, which is a prerequisite for the induction of qE (the ΔpH-dependent quenching component of NPQ), and were consequently defined as ‘proton gradient regulation’ mutants (pgr). One such mutant, pgr1, was further characterized, and shown to be defective in the photosynthetic electron transfer C (PETC) gene, which encodes the Rieske subunit of the cytochrome b6f complex (Munekage et al., 2001). Another mutant line impaired in the build-up of the proton gradient is pgr5 (Shikanai et al., 1999; Munekage et al., 2002). It lacks a component of the antimycin A-sensitive cyclic electron flow (CEF) pathway, which is mediated by the ferredoxin-plastoquinone reductase PGRL1/PGR5 (Hertle et al., 2013). The second group with aberrant NPQ comprised the mutants npq1 and npq2, which display defects in the xanthophyll cycle and are disrupted in the violaxanthin de-epoxidase and zeaxanthin epoxidase, respectively (Niyogi et al., 1997). The third type of mutant (npq4) showed normal pigment composition, xanthophyll cycle activity and photosynthetic electron transport, but this mutant was nevertheless specifically affected at the level of qE (Li et al., 2000). It turned out that the npq4 mutant lacks the PSII-associated protein S (PsbS), which is now known to be the luminal pH sensor that triggers NPQ within the PSII antenna in plants (Li et al., 2000). In a subsequent study, which was designed to isolate Arabidopsis lines affected in other slowly reversible NPQ components, CFVI was used to screen mutagenized seedlings for suppressors of the npq4 phenotype (Brooks et al., 2013). This screen yielded the suppressor of quenching 1 (soq1), which has a high NPQ even in the absence of PsbS and lacks a thylakoid membrane protein (SOQ1) that harbors a thioredoxin-like, a β-propeller and a haloacid-dehalogenase domain. SOQ1 maintains light-harvesting efficiency and prevents formation of a slow, reversible NPQ mechanism that is independent of qE, qZ, and qT, but participates in a photoprotective, ‘qI-like’ mechanism termed qH (Malnoë et al., 2017). To identify factors involved in qH, suppressors of soq1 npq4 were screened for by CFVI and two mutants affected either in chlorophyllide a oxygenase (CAO) or the plastid lipocalin (LCNP) showed a reversion to the low NPQ phenotype of npq4. In-depth analyses of both mutants provided evidence that qH operates under high-light and cold stress, and can be localized to the peripheral LHCII antenna of PSII and requires LCNP (Malnoë et al., 2017).
A further step toward an understanding of the molecular basis of NPQ was the identification of so-called ‘state transition’ (stt) mutants with alterations in qT. State transitions involve the reversible association of the mobile pool of light-harvesting-complex II proteins (LHCIIs) with either PSII (state 1) or PSI (state 2) and re-establish a balanced distribution of light energy between the photosystems. Several studies (Fleischmann et al., 1999; Kruse et al., 1999) took advantage of the fact that the green alga Chlamydomonas undergoes large changes in Chl fluorescence during state transitions, which can be attributed to its significantly higher fraction of mobile LHCIIs (about 80%, Delosme et al., 1996) compared to land plants (15–20%) (Allen, 1992). Mutants affected in state transitions were identified by comparing fluorescence images taken under state-1 and state-2 conditions, and this type of differential fluorescence screen enabled Fleischmann et al. (1999) to isolate four stt mutants (stt2, stt3, stt5, and stt7). These mutants were characterized by high Chl fluorescence levels at room temperature even under state-2 conditions, indicating that they were physiologically locked in state 1. Further analyses showed that stt7 lacks the thylakoid serine-threonine protein kinase Stt7, which is required for phosphorylation of LHCII in response to state-2 conditions (Depège et al., 2003).
In addition to the predominant linear electron flow pathway, which results in the production of both NADPH and ATP, two CEF routes around PSI have been described that are important for balancing the ATP/NADPH budget of photosynthesis, as well as for protecting the photosystems from photodamage in plants (reviewed in: Yamori and Shikanai, 2016). One of these pathways is mediated by the NADH-like dehydrogenase (NDH) complex, which is also responsible for chlororespiration in the dark. To identify ‘chlororespiratory reduction’ mutants (crr) which are disrupted in NDH function, Hashimoto et al. (2003) established a CFVI-based screening system, in which the post-illumination rise of Chl fluorescence (PIF) after a low-light treatment was monitored in an Arabidopsis mutant population. Under such conditions, the fluorescence signal is almost proportional to the reduction state of the plastoquinone pool (Krause and Weis, 1991), so that the chlororespiratory activity of the NDH complex can be derived from the degree to which the PIF is depressed in mutants with a dysfunctional NDH complex (Shikanai et al., 1998). Screening of over 50,000 M2 seedlings for aberrant PIFs led to the identification of 17 crr mutants in Arabidopsis. These could be assigned to at least 11 loci, and further analyses revealed the existence of novel NDH subunits and allowed the functional characterization of factors required for efficient NDH complex biogenesis (reviewed in: Peng et al., 2011).
Plants and algae can undergo photosynthetic acclimation processes which take place over periods of hours or days and entail substantial changes in plastid and nuclear gene expression, as well as adjustments of the photosynthetic apparatus. For instance, the long-term response to high light levels has been thoroughly studied and, instead of reducing the demands on light harvesting, it actually enhances the capacity for electron transport and carbon dioxide fixation. To investigate the molecular mechanisms behind the signal cascades that activate the acclimation response to high light, Walters et al. (2003) screened an Arabidopsis mutant population for alterations in ‘acclimation of photosynthesis to the environment’ (ape). Their CFVI screen was based on the observation that in wild-type Arabidopsis plants a 3-day exposure to high light raises effective quantum yields (ΦII), and its goal was to identify mutants that were unable to increase Pmax under these conditions. Among the three ape mutants obtained, which showed distinct acclimation-defective phenotypes, ape2 exhibited a lower Pmax under all light regimes, and was disrupted in the chloroplast envelope triose-phosphate/phosphate translocator (TPT). Subsequent studies using Arabidopsis double and triple mutants altered in the day and night modes of photoassimilate export from the chloroplast provided evidence that carbohydrates act as chloroplast-to-nucleus retrograde signals and modulate the acclimation response to high light (Schmitz et al., 2012, 2014).
Ribulose-1,5-bisphosphate carboxylase/oxygenase (RuBisCO) not only fixes atmospheric CO2 but also oxygen. The phosphoglycolate generated by the latter reaction must be degraded via a complex mechanism which is known as photorespiration, because CO2 is released during the process. The photorespiratory pathway is distributed between four compartments (chloroplasts, cytosol, peroxisomes and mitochondria) and requires the action of several transporters and enzymes. Most of the early mutants affected in photorespiration were identified by their ability to grow normally in a high concentration (1%) of CO2, while becoming chlorotic when shifted to ambient air (Somerville, 1986). Recently, it was shown that mutations in components involved in the photorespiratory pathway also impair photosynthetic light reactions, as revealed by the observation that photorespiratory mutants transferred from high to ambient CO2 concentrations showed a decline in PSII functionality (Takahashi et al., 2007). Thus, Badger et al. (2009) set up a CFVI screen designed to detect mutants with more subtle photorespiratory phenotypes. To this end, levels of PSII function in mutagenized Arabidopsis seedlings grown under high concentrations of CO2, and in its absence, were compared. Two major mutant phenotype classes could be distinguished. One group comprised ‘photorespiration-like’ mutants, which were characterized by Fv/Fm values that were close to those of wild-type plants under high CO2 concentrations, but significantly lower than normal in the absence of CO2. The second group consisted of lines in which Fv/Fm values were depressed even under high concentrations of CO2. Remarkably, some members of the second group were able to partially recover PSII functionality after exposure to zero CO2 concentrations, and were therefore named for this ‘reverse photorespiration’ phenotype (Badger et al., 2009).
As aquatic organisms, many unicellular green algae are characterized by a remarkably flexible metabolism, and can acclimate rapidly to anaerobic conditions (Terashima et al., 2010; Grossman et al., 2011). As part of an extensive response to anaerobiosis, expression and synthesis of oxygen-labile [Fe–Fe] hydrogenases are induced in C. reinhardtii and hydrogen production is linked to photosynthesis by ferredoxin-mediated electron supply. Several factors required for expression, maturation and activity of [Fe–Fe] hydrogenases have been identified, most of them through a H2-sensing, chemochromic screening system that can discriminate Chlamydomonas mutants with aberrant H2 production capacities (reviewed in: Hemschemeier et al., 2009). An alternative, less time-consuming approach has been demonstrated by Godaux et al. (2013), and takes advantage of the observation that mutants with defects in [Fe–Fe] hydrogenase activity exhibit low effective PSII quantum yields shortly after a shift from dark anaerobiosis to saturating light conditions. As a proof of concept, screening of a small Chlamydomonas population of about 3000 strains generated by insertional mutagenesis yielded five mutants with a Chl fluorescence signature similar to that of the [Fe–Fe] hydrogenase-deficient control strain, and one of them turned out to be defective in the previously characterized [Fe–Fe] hydrogenase assembly factor G (HydG) (Posewitz et al., 2004). Moreover, in various mutants affected in anaerobic energy metabolism, the effective quantum yield of PSII was shown to be correlated with the level of [Fe–Fe] hydrogenase activity. Thus, the screening system represents a time-saving, alternative approach to the chemochromic method, and is capable of detecting mutants impaired in [Fe–Fe] hydrogenase biogenesis, regulation or activity.
Although respiration and photosynthesis take place in different organelles in photosynthetic eukaryotes, the energy metabolisms of mitochondria and chloroplasts are intertwined at multiple levels. Not only do these organelles share over 100 dual-targeted proteins (reviewed in: Carrie and Small, 2013), provide both ATP and contribute to photorespiration, chloroplasts can shuttle reducing power to mitochondria via the malate valve (reviewed in: Scheibe, 2004; Kramer and Evans, 2011). Functional cooperation between mitochondria and chloroplasts in balancing the cellular ATP/NADPH ratio becomes even more obvious when compensatory acclimation processes are studied in mutants affected in photosynthesis or respiration. For instance, in Chlamydomonas mutants defective in different complexes of the respiratory electron transport chain, the resulting ATP deficiency is counterbalanced by increased non-photochemical reduction of the plastoquinone pool mediated by the chlororespiratory pathway, LHCII protein association to PSI and cyclic photophosphorylation (Cardol et al., 2003). Furthermore, the Chlamydomonas strain pgrl1 disrupted in the proton regulation 5 like 1 protein (PGRL1), which was identified as a CEF mutant in CFVI-based screen (Tolleter et al., 2011), compensates for ATP deficiency by increasing oxygen photoreduction downstream of PSI and shows higher susceptibility to mitochondrial inhibitors (Dang et al., 2014). These results are consistent with the finding that overall fitness and yields of photosynthesis were only significantly reduced when state transitions and mitochondrial respiration were concomitantly impaired in the Chlamydomonas double mutant stt7-9 dum22 (Cardol et al., 2009). Thus, increased cyclic electron transport rates induced by state 2 transitions can supply extra ATP when respiratory ATP production becomes limiting and, conversely, mitochondrial cooperation is increased when CEF is downregulated in Chlamydomonas. One important conclusion that could be drawn from these studies was that PSII efficiencies were reduced in respiratory mutants and was explained by enhanced rates of non-photochemical reduction of plastoquinone mediated by the chlororespiratory pathway and preferential association of LHCII proteins with PSI (Cardol et al., 2003). Massoz et al. (2015) therefore used Fv/Fm values as an initial criterion to select mutants affected in mitochondrial respiration. Several mutants disrupted in subunits of the respiratory complex I or the isocitrate lyase were isolated from a collection of about 2900 insertional mutants generated in either a wild-type or a state transition-defective strain (stt7-9). A later refinement of the screening procedure used the CEF mutant pgrl1 as the starting strain with a view to isolating mutants impaired in mitochondrial complex I (Massoz et al., 2017). As proof of concept, the double mutant pgrl1Δnd4, which is deficient in both CEF and complex 1, was generated and shown to exhibit a lower PSII efficiency than either of the single mutants. Subsequent screening of about 3000 insertional mutants created in the pgrl1 background resulted in 46 mutants with reduced PSII efficiency, of which three were complex I mutants. Further analyses revealed that one of these was disrupted in NADH dehydrogenase [ubiquinone] 1 alpha subcomplex assembly factor 3 (NDUFAF3), a complex I assembly factor also conserved in humans (Massoz et al., 2017).
Forward genetic approaches still dominated mutant searches in the late 1990s and early 2000s (Lloyd and Meinke, 2012), but thanks to advances in genome sequencing technologies, the establishment of large mutant libraries and the development of new genetic tools such as RNA silencing techniques (Mohr et al., 2014), and more recently genome editing tools (Yin et al., 2017), reverse genetics has since come to the fore. Indeed, in conjunction with the tremendous rise in the availability of myriad ‘omics’ datasets, reverse genetic strategies have become the more practicable choice, since laborious screens of large mutant libraries are circumvented and the underlying genetic defects are already known. Relative to classical forward genetic approaches, reverse genetic screens start with a significantly reduced number of lines or strains, which are generally disrupted in genes with poorly characterized or unknown functions. Depending on the stringency of preselection criteria (e.g., coregulation or phylogenomic studies), ‘the starting material’ can be narrowed down to a reasonable number of candidates which is compatible with the complexity of the required screening procedure. One example for the power of such ‘guilt-by-association’ approaches is the identification of three subunits of the NDH complex – NDF1, NDF2, and NDF4 (Takabayashi et al., 2009) now called photosynthetic NDH subcomplex B subunit PnsB1, PnsB2, and PnsB3 (Ifuku et al., 2011). In that study, genes of unknown function were selected on the basis of their co-expression with nucleus-encoded NDH subunits L, N, and O (NDHL, NDHN, and NDHO). In addition, Arabidopsis genes (of unknown function) were considered together with homologs found in cyanobacteria but not in green algae, since C. reinhardtii lacks a plant-type NDH complex. Insertion lines were identified for 21 of the 36 genes pre-selected by means of the bioinformatics screen, and these were tested for NDH activity. Remarkably, four of them (nearly 20%) failed to exhibit the post-illumination rise in fluorescence. Further studies provided evidence that the respective genes indeed code for the NDH subunits NDF1 (PnsB1), NDF2 (PnsB2), and NDF4 (PnsB3), whereas NDF3 corresponds to the chlororespiratory reduction protein 6 (CRR6), which is involved in NDH subcomplex A assembly (Munshi et al., 2006).
Since Chl fluorescence-based phenotyping is no longer as time-consuming as it once was, and manageable numbers of candidates can be examined in reverse genetics projects, contemporary screening approaches can be extended to more elaborate protocols in which subtle or multiple photosynthetic phenotypes can be detected in a single, albeit longer, experimental run. Commercial Chl video imaging systems now make it possible to set up automated measuring routines composed of several analytical blocks that can last for days. One example of such a combined screening protocol is shown in Figure 1, which we use routinely for initial phenotyping of selected Arabidopsis mutant lines.
FIGURE 1. Example of a combined screening protocol based on Chl fluorescence video imaging which is able to identify hcf, crr, npq, pam and PSII repair mutants. (A) Chl fluorescence was monitored using an Imaging PAM system (Walz®) and the indicated sequence of actinic light conditions was executed in series to determine, in a single experimental run, the various photosynthetic parameters listed at the top. Plants were dark-adapted for 20 min and acclimated to measuring light for 5 min prior to the analysis. For further explanations, see the main text. Fv/Fm, maximum quantum yield of PSII; NPQ, non-photochemical quenching; qL, fraction of open PSII centers; qP, photochemical quenching coefficient; qN non-photochemical quenching coefficient; ΦII, effective quantum yield of PSII; qE, energy-dependent quenching; qI, photo-inhibitory quenching. (B) Example of an hcf mutant phenotype, which can be detected in measurement block 1. F0 and Fv/Fm images of wild-type and psbO1 psbO2 (Steinberger et al., 2015) Arabidopsis plants. (C) Identification of a crr mutant phenotype in block 2. Detail of the post-illumination fluorescence rise (PIF) analysis of pam68L (Armbruster et al., 2013), which is disrupted in NDH complex assembly. (D) Detection of a high NPQ phenotype in block 3. Fm′ values were recorded every minute by applying saturating light pulses after a dark-light transition (100 μE m-2 s-1) and calculated NPQ values of the chloroplast ATP synthase-deficient mutant cgl160 (Rühle et al., 2014) were compared to a wild-type control. (E) Example of a pam mutant phenotype, which can be distinguished at the end of block 3. ΦII values of the PSII assembly mutant pam68 (Armbruster et al., 2010) were compared to a wild-type control. (F) Detection of an npq phenotype in measurement block 5. NPQ analyses were carried out with the CEF mutant pgrl1ab (DalCorso et al., 2008) and compared to a wild-type control. False-color images for F0, Fv/Fm, NPQ/4, and ΦII depicted at the time points highlighted by a black arrow represent values on a rainbow scale from 0 to 1 shown below (B). Note that NPQ parameters in (D,F) are displayed in NPQ/4 to fit the standard color code ranging from 0 to 1. Chl fluorescence signals were normalized to Fm and are shown in gray on a scale from 0 to 1 in (D–F).
In principle, the approach comprises six phases, in which most of the previously described Chl fluorescence signatures of photosynthetic mutants can be identified (Figure 1A). In the first block, the Fv/Fm measurement allows one to assess PSII functionality and pinpoint mutants with an hcf phenotype, such as the Arabidopsis PSII subunit O (PsbO) knockdown mutant psbO1 psbO2 (Figure 1B) (Steinberger et al., 2015). The second analytical block was designed to identify mutant lines with a crr phenotype, and detects NDH activity by means of a PIF measurement (Figure 1C). Block 3 implements a standard slow induction experiment, which is carried out under moderate actinic light intensities. Several informative parameters can be extracted in block 3 which reveal aspects of the transient dynamics of photosynthesis upon a dark-light shift. For instance, pgr mutants can be already identified at this stage by their low transient NPQ phenotype (DalCorso et al., 2008). Conversely, mutants affected in chloroplast F1F0-ATP synthase activity can be identified on the basis of their high NPQ (Figure 1D) (Rühle et al., 2014; Grahl et al., 2016; Zhang et al., 2016). The increased NPQ in such mutants can be attributed to a high operating qE, which is established as a result of proton accumulation in the thylakoid lumen already under moderate light intensities. Parameters determined at the end of block 3 reflect photosynthetic performance in the steady state, and an analysis of effective quantum yields (ΦII) uncovers pam mutants (Figure 1E). Samples in block 4 are shifted back into the dark and NPQ relaxation kinetics provide values of qI and qE, which was recently determined in an initial screening step to identify ‘high cyclic electron flow around PSI’ (hcef) mutants with altered CEF (Livingston et al., 2010). Block 5 also implements a dark-light shift experiment, but using excessive light intensities (1200 μE m-2 s-1) and longer exposure times (20 min), which allow the detection of npq mutants due to their aberrant NPQ induction patterns under high light (Niyogi et al., 1998). After the photodamage-inducing high-light treatment in block 5, the protocol ends with a recovery phase from high light (block 6) under low light intensities (10 μE m-2 s-1) and was designed to pick up mutants that are defective in the PSII repair mechanism (Schroda et al., 1999; Malnoe et al., 2014). Overall, the CFVI protocol outlined above can already uncover a wide range of phenotypes, but can be further expanded to cover a larger collection of photosynthetic parameters, such as the determination of Pmax from light saturation curves (van Rooijen et al., 2015) and measurements of qT in state transitions (Pribil et al., 2010) or effective quantum yields/NPQ parameters under fluctuating light conditions (Cruz et al., 2016).
The photosynthetic lifestyle of plants and algae requires a high degree of flexibility and the ability to adapt to rapidly fluctuating environments. However, for reasons of scalability and reproducibility most of the screening studies referred to here were conducted with small Arabidopsis plants or algae grown in stable, standardized laboratory settings and would have been impossible with fully developed crops under field conditions. Furthermore, phenotyping of mutant collections involved measurements of only one or a few photosynthetic parameters, which were determined at one or more time points, thus providing a rather static picture of the highly dynamic process of photosynthesis. It is therefore obvious that many factors that contribute to the fine tuning of photosynthesis in response to dynamic environmental changes will have not been identified by previous screening procedures (Cruz et al., 2016). This assertion is also supported by the observation that plant lines lacking PsbS (Külheim et al., 2002), the LHCII serine/threonine-protein kinase STN7 (Grieco et al., 2012) or PGR5 (Suorsa et al., 2012) showed higher levels of photodamage and notable reductions in their growth rates (or lethality) only under fluctuating light conditions that were not observed under unchanging conditions. One logical and straightforward way to bypass this limitation would be to carry out phenotyping of mutant collections in the field, and suitable large-scale Chl fluorescence image analyzers are now available for this task (e.g., Field Scanalyzer) (Virlet et al., 2017). However, besides the fact that in several countries the cultivation of genetically modified plants in the field is either prohibited or subject to legal restrictions, such studies are complicated by a multitude of overlapping, unpredictable abiotic and biotic stress factors, and statistical evaluation of the results become particularly challenging. For these reasons, the dynamic environmental photosynthesis imager (DEPI) platform was developed for replication of natural, fluctuating growth conditions in the laboratory (Cruz et al., 2016). Several parameters can be controlled (light intensity, CO2 concentration, humidity and temperature) in the growth chamber, and rapid responses as well as long-term acclimation processes of photosynthesis can be assessed in situ by the integrated CFVI system in more than two hundred plants simultaneously. As a proof of concept, a library of over 300 T-DNA Arabidopsis lines disrupted in nuclear genes coding for chloroplast-targeted proteins (Ajjawi et al., 2010) was exposed to a 5-day regime of fluctuating light levels and screened for alterations in photosynthetic performance. As a result, psb33 plants lacking PSII protein 33 (PSB33) (Fristedt et al., 2015) and several other conditional mutant lines showed transient, spatiotemporal-dependent phenotypes which could not be detected or were not reliably expressed under standard growth conditions. PSB33 is a green-lineage-specific protein (Merchant et al., 2007) predominantly found in non-appressed thylakoids of Arabidopsis chloroplasts and sustains D1 of PSII under fluctuating light conditions (Fristedt et al., 2017). Thus, the DEPI system can reveal new, complex and previously unseen phenotypes, and provides a versatile experimental platform with which to identify factors required for remodeling and regulation of photosynthesis under dynamic environmental conditions.
Forward and reverse genetics are efficient strategies for elucidating the functions of a single gene or of small gene families, but these approaches reach their limits when the genetic architecture of a quantitative trait and its interaction with the environment needs to be determined. Most agronomically important traits (e.g., grain yield, grain size, ripening or flowering time) are controlled by multiple genes which have to be analyzed by quantitative genetic approaches, such as classical linkage mapping or genome-wide association studies (GWAS) (reviewed in: Bazakos et al., 2017). Natural variation also exists for photosynthetic traits and can be roughly divided into morphological and physiological variations, which have been investigated in several studies with different plant species (reviewed in: Flood et al., 2011). For instance, Jung and Niyogi (2009) examined natural NPQ variation in different Arabidopsis accessions and provided evidence that thermal dissipation is a quantitative trait that depends on multiple, nucleus-encoded genetic factors. Two high-NPQ QTLs (HQE1 and HQE2) were identified in a quantitative trait locus (QTL) analysis which was performed with a F2 mapping population generated from a cross between a low-NPQ and a high-NPQ Arabidopsis accession (Jung and Niyogi, 2009). Remarkably, HQE1 and HQE2 were not mapped to previously characterized factors identified in forward genetic approaches, indicating that quantitative genetics can serve as a complementary strategy to dissect the genetic architecture of thermal dissipation.
Even though quantitative genetic approaches have a long history in plant science, their potential for photosynthesis research has not yet been fully explored. This may simply reflect the high complexity of the genetic architecture of photosynthesis, which not only comprises the several hundred genes directly involved in biogenesis processes, regulation or acclimation of photosynthesis, but also involves two quite distinct genetic systems (plastid and nuclear genome) with different inheritance modes. Moreover, successful quantitative genetic approaches in photosynthesis research require reproducible, non-invasive, high-throughput phenotyping pipelines that were not available until recently. However, several platforms have been developed in recent years. Examples include FluorImager (Barbagallo et al., 2003), GROWSCREEN FLUORO (Jansen et al., 2009), PlantScreen (Humplík et al., 2015), Phenovator (Flood et al., 2016), the DEPI system (Cruz et al., 2016) or the crop population growth information detection system (Wang et al., 2017), which now integrate CFVI analyses into their phenotyping facilities. As an example for the combination of a GWAS and a high-throughput Chl fluorescence phenotyping approach, van Rooijen et al. (2015) have explored the natural genetic variation for acclimation of photosynthetic light use efficiency (ΦII) in 344 Arabidopsis accessions. Of 63 newly identified gene candidates, 13 encode chloroplast-localized proteins, most of which are either associated with abiotic stress responses or have unknown functions.
Due to the advances in imaging and data acquisition technologies in the last two decades, Chl fluorescence-based analyses have entered the ‘phenomics’ field and promise to increase our knowledge of photosynthesis substantially. Modern phenotyping systems are highly flexible and will allow the identification of new genotype-phenotype-environment relationships and accelerate gene discovery studies significantly.
The next obvious step will be to determine photosynthetic parameters of large-scale, indexed mutant collections like the Arabidopsis unimutant (O’Malley and Ecker, 2010), the Arabidopsis Chloroplast 2010 Project (Ajjawi et al., 2010) and the GABI-DUPLO double mutant (Bolle et al., 2013) collections, or the recently generated Chlamydomonas mutant collection CLiP (Li et al., 2016). Consequently, with the exception of screening approaches under highly specialized conditions, tedious forward genetic screening procedures, which were carried out by single researchers or research groups in the past, will become obsolete. A major task in the future will lie in the processing, handling, quality control, maintenance, storage, analysis and sharing of the vast amount of data collected by CFVI-based phenotyping studies, which will become even more challenging when such screens are combined with other non-invasive phenotyping technologies (Walter et al., 2015; Tardieu et al., 2017). But computational techniques for assessing the quality of phenotypic data (Xu et al., 2015) and analyzing massive amounts of data in order to reveal dynamic relationships between phenotypes and environment (Yang et al., 2017) have already been developed, and these will eventually replace manual evaluation methods.
Chl fluorescence video imaging also has the potential to be an important technological driver in crop science, since it offers an efficient screening technology for rapid evaluation of plant performance under stress conditions such as drought, salinity, freezing, chilling, high temperature or nutrient deficiency (reviewed in: Baker and Rosenqvist, 2004). CFVI is of particular interest in plant breeding programs, since low-cost and precise high-throughput phenotyping technologies have been regarded as one of the major bottleneck in the postgenomic era of plant breeding (reviewed in: Araus and Cairns, 2014). A further challenge is the difficulty to extrapolate results gained under a strictly controlled environment (such as a growth chamber or greenhouse) to field conditions. It is therefore inevitable to establish high throughput phenotyping technologies under heterogeneous field conditions to analyze quantitative traits and to elucidate their underlying genetic architecture for future breeding efforts. Significant progress in non-invasive sensor and imaging technology has been made (reviewed in: White et al., 2012; Fiorani and Schurr, 2013) and the Field Scanalyzer system installed at Rothamsted Research (United Kingdom) by LemnaTec GmbH (Germany) is one example, which now employs Chl fluorescence based measurements for high-throughput phenotyping in the field (Virlet et al., 2017).
While recent work has mainly focused on scaling up CFVI screening systems for simultaneous evaluation of large sample collections, a future direction might be to explore the potential of screening single cells by exploiting their Chl fluorescence fingerprints. Flow cytometry technologies are well established for unicellular microalgae in environmental and toxicological studies (reviewed in: Hyka et al., 2013) and several flow cytometry studies with plant protoplasts have been reported (Harkins et al., 1990; Galbraith, 2007; Berendzen et al., 2012; You et al., 2015). Flow cytometry is generally coupled to fluorescence-activated cell sorting, which permits the isolation of a desired cell population with specific physiological properties. Recently, this technique has been successfully employed to screen high-lipid Chlamydomonas mutants that were stained with the lipid-sensitive dye Nile Red prior to screening (Xie et al., 2014; Terashima et al., 2015) or to identify protein-protein interactions in plant protoplasts by combining bimolecular fluorescence complementation with flow cytometry (Berendzen et al., 2012). Moreover, Chl autofluorescence has been used in flow cytometry studies as an endogenous probe to sort tobacco mesophyll protoplasts (Harkins et al., 1990) and to discriminate between different phytoplankton species by cytometric approaches (Hildebrand et al., 2016). Although implementation will be challenging, the combination of flow cytometry and Chl fluorescence kinetics-based cell sorting can provide a fast means of screening mutagenized cell populations for specific Chl fluorescence phenotypes.
TR designed and wrote the article. BR and DL wrote the article.
This work was funded by the German Science Foundation (DFG, Research Unit FOR2092, grant RU 1945/2-1).
The authors declare that the research was conducted in the absence of any commercial or financial relationships that could be construed as a potential conflict of interest.
We thank Paul Hardy for critical comments on the manuscript.
Ajjawi, I., Lu, Y., Savage, L. J., Bell, S. M., and Last, R. L. (2010). Large-scale reverse genetics in Arabidopsis: case studies from the Chloroplast 2010 Project. Plant Physiol. 152, 529–540. doi: 10.1104/pp.109.148494
Allen, J. F. (1992). Protein phosphorylation in regulation of photosynthesis. Biochim. Biophys. Acta 1098, 275–335. doi: 10.1016/S0005-2728(09)91014-3
Araus, J. L., and Cairns, J. E. (2014). Field high-throughput phenotyping: the new crop breeding frontier. Trends Plant Sci. 19, 52–61. doi: 10.1016/j.tplants.2013.09.008
Armbruster, U., Rühle, T., Kreller, R., Strotbek, C., Zühlke, J., Tadini, L., et al. (2013). The photosynthesis affected mutant68-like protein evolved from a PSII assembly factor to mediate assembly of the chloroplast NAD(P)H dehydrogenase complex in Arabidopsis. Plant Cell 25, 3926–3943. doi: 10.1105/tpc.113.114785
Armbruster, U., Zühlke, J., Rengstl, B., Kreller, R., Makarenko, E., Rühle, T., et al. (2010). The Arabidopsis thylakoid protein PAM68 is required for efficient D1 biogenesis and photosystem II assembly. Plant Cell 22, 3439–3460. doi: 10.1105/tpc.110.077453
Badger, M. R., Fallahi, H., Kaines, S., and Takahashi, S. (2009). Chlorophyll fluorescence screening of Arabidopsis thaliana for CO2 sensitive photorespiration and photoinhibition mutants. Funct. Plant Biol. 36, 867–873. doi: 10.1071/FP09199
Baker, N. R. (2008). Chlorophyll fluorescence: a probe of photosynthesis in vivo. Annu. Rev. Plant Biol. 59, 89–113. doi: 10.1146/annurev.arplant.59.032607.092759
Baker, N. R., and Rosenqvist, E. (2004). Applications of chlorophyll fluorescence can improve crop production strategies: an examination of future possibilities. J. Exp. Bot. 55, 1607–1621. doi: 10.1093/jxb/erh196
Barbagallo, R. P., Barbagallo, R. P., Oxborough, K., Oxborough, K., Pallett, K. E., Pallett, K. E., et al. (2003). Rapid noninvasive screening for perturbations of metabolism and plant growth using chlorophyll fluorescence imaging. Plant Physiol. 132, 485–493. doi: 10.1104/pp.102.018093
Barkan, A., Miles, D., and Taylor, W. C. (1986). Chloroplast gene expression in nuclear, photosynthetic mutants of maize. EMBO J. 5, 1421–1427. doi: 10.1002/j.1460-2075.1986.tb04378.x
Bazakos, C., Hanemian, M., Trontin, C., Jiménez-Gómez, J. M., and Loudet, O. (2017). New strategies and tools in quantitative genetics: How to go from the phenotype to the genotype. Annu. Rev. Plant Biol. 68, 435–455. doi: 10.1146/annurev-arplant-042916-40820
Belcher, S., Williams-Carrier, R., Stiffler, N., and Barkan, A. (2015). Large-scale genetic analysis of chloroplast biogenesis in maize. Biochim. Biophys. Acta 1847, 1004–1016. doi: 10.1016/j.bbabio.2015.02.014
Bennoun, P., and Levine, R. P. (1967). Detecting mutants that have impaired photosynthesis by their increased level of fluorescence. Plant Physiol. 42, 1284–1287.
Berendzen, K. W., Bohmer, M., Wallmeroth, N., Peter, S., Vesic, M., Zhou, Y., et al. (2012). Screening for in planta protein-protein interactions combining bimolecular fluorescence complementation with flow cytometry. Plant Methods 8:25. doi: 10.1186/1746-4811-8-25
Bilger, W., and Björkman, O. (1990). Role of the xanthophyll cycle in photoprotection elucidated by measurements of light-induced absorbance changes, fluorescence and photosynthesis in leaves of Hedera canariensis. Photosynth. Res. 25, 173–185. doi: 10.1007/BF00033159
Björkman, O., and Demmig, B. (1987). Photon yield of O2 evolution and chlorophyll fluorescence characteristics at 77 K among vascular plants of diverse origins. Planta 170, 489–504. doi: 10.1007/BF00402983
Bolle, C., Huep, G., Kleinbölting, N., Haberer, G., Mayer, K., Leister, D., et al. (2013). GABI-DUPLO: A collection of double mutants to overcome genetic redundancy in Arabidopsis thaliana. Plant J. 75, 157–171. doi: 10.1111/tpj.12197
Brooks, M. D., Sylak-Glassman, E. J., Fleming, G. R., and Niyogi, K. K. (2013). A thioredoxin-like/β-propeller protein maintains the efficiency of light harvesting in Arabidopsis. Proc. Natl. Acad. Sci. U.S.A. 110, E2733–E2740. doi: 10.1073/pnas.1305443110
Cardol, P., Alric, J., Girard-Bascou, J., Franck, F., Wollman, F.-A., and Finazzi, G. (2009). Impaired respiration discloses the physiological significance of state transitions in Chlamydomonas. Proc. Natl. Acad. Sci. U.S.A. 106, 15979–15984. doi: 10.1073/pnas.0908111106
Cardol, P., Gloire, G., Havaux, M., Remacle, C., Matagne, R., and Franck, F. (2003). Photosynthesis and state transitions in mitochondrial mutants of Chlamydomonas reinhardtii affected in respiration. Plant Physiol. 133, 2010–2020. doi: 10.1104/pp.103.028076
Carrie, C., and Small, I. (2013). A reevaluation of dual-targeting of proteins to mitochondria and chloroplasts. Biochim. Biophys. Acta 1833, 253–259. doi: 10.1016/j.bbamcr.2012.05.029
Chaerle, L., Lenk, S., Leinonen, I., Jones, H. G., Van Der Straeten, D., and Buschmann, C. (2009). Multi-sensor plant imaging: towards the development of a stress-catalogue. Biotechnol. J. 4, 1152–1167. doi: 10.1002/biot.200800242
Cruz, J. A., Savage, L. J., Zegarac, R., Hall, C. C., Satoh-Cruz, M., Davis, G. A., et al. (2016). Dynamic environmental photosynthetic imaging reveals emergent phenotypes. Cell Syst. 2, 365–377. doi: 10.1016/j.cels.2016.06.001
DalCorso, G., Pesaresi, P., Masiero, S., Aseeva, E., Schünemann, D., Finazzi, G., et al. (2008). A complex containing PGRL1 and PGR5 is involved in the switch between linear and cyclic electron flow in Arabidopsis. Cell 132, 273–285. doi: 10.1016/j.cell.2007.12.028
Dang, K.-V., Plet, J., Tolleter, D., Jokel, M., Cuiné, S., Carrier, P., et al. (2014). Combined increases in mitochondrial cooperation and oxygen photoreduction compensate for deficiency in cyclic electron flow in Chlamydomonas reinhardtii. Plant Cell 26, 3036–3050. doi: 10.1105/tpc.114.126375
Delosme, R., Olive, J., and Wollman, F. A. (1996). Changes in light energy distribution upon state transitions: an in vivo photoacoustic study of the wild type and photosynthesis mutants from Chlamydomonas reinhardtii. Biochim. Biophys. Acta 1273, 150–158. doi: 10.1016/0005-2728(95)00143-3
Depège, N., Bellafiore, S., and Rochaix, J. D. (2003). Role of chloroplast protein kinase Stt7 in LHCII phosphorylation and state transition in Chlamydomonas. Science 299, 1572–1575. doi: 10.1126/science.1081397
Dinkins, R. D., Bandaranayake, H., Baeza, L., Griffiths, A. J., and Green, B. R. (1997). hcf5, a nuclear photosynthetic electron transport mutant of Arabidopsis thaliana with a pleiotropic effect on chloroplast gene expression. Plant Physiol. 113, 1023–1031. doi: 10.1104/pp.113.4.1023
Dinkins, R. D., Bandaranayake, H., Green, B. R., and Griffiths, A. J. (1994). A nuclear photosynthetic electron transport mutant of Arabidopsis thaliana with altered expression of the chloroplast petA gene. Curr. Genet. 25, 282–288. doi: 10.1007/BF00357174
Felder, S., Meierhoff, K., Sane, A. P., Meurer, J., Driemel, C., Plücken, H., et al. (2001). The nucleus-encoded HCF107 gene of Arabidopsis provides a link between intercistronic RNA processing and the accumulation of translation-competent psbH transcripts in chloroplasts. Plant Cell 13, 2127–2141. doi: 10.1105/TPC.010090
Fenton, J. M., and Crofts, A. R. (1990). Computer aided fluorescence imaging of photosynthetic systems - Application of video imaging to the study of fluorescence induction in green plants and photosynthetic bacteria. Photosynth. Res. 26, 59–66. doi: 10.1007/BF00048977
Fiorani, F., and Schurr, U. (2013). Future scenarios for plant phenotyping. Annu. Rev. Plant Biol. 64, 267–291. doi: 10.1146/annurev-arplant-050312-120137
Fleischmann, M. M., Ravanel, S., Delosme, R., Olive, J., Zito, F., Wollman, F.-A., et al. (1999). Isolation and characterization of photoautotrophic mutants of Chlamydomonas reinhardtii deficient in state transition. J. Biol. Chem. 274, 30987–30994. doi: 10.1074/jbc.274.43.30987
Flood, P. J., Harbinson, J., and Aarts, M. G. M. (2011). Natural genetic variation in plant photosynthesis. Trends Plant Sci. 16, 327–335. doi: 10.1016/j.tplants.2011.02.005
Flood, P. J., Kruijer, W., Schnabel, S. K., van der Schoor, R., Jalink, H., Snel, J. F. H., et al. (2016). Phenomics for photosynthesis, growth and reflectance in Arabidopsis thaliana reveals circadian and long-term fluctuations in heritability. Plant Methods 12:14. doi: 10.1186/s13007-016-0113-y
Fristedt, R., Herdean, A., Blaby-Haas, C. E., Mamedov, F., Merchant, S. S., Last, R. L., et al. (2015). PHOTOSYSTEM II PROTEIN33, a protein conserved in the plastid lineage, is associated with the chloroplast thylakoid membrane and provides stability to photosystem II supercomplexes in Arabidopsis. Plant Physiol. 167, 481–492. doi: 10.1104/pp.114.253336
Fristedt, R., Trotta, A., Suorsa, M., Nilsson, A. K., Croce, R., Aro, E.-M., et al. (2017). PSB33 sustains photosystem II D1 protein under fluctuating light conditions. J. Exp. Bot. 68, 4281–4293. doi: 10.1093/jxb/erx218
Galbraith, D. W. (2007). Protoplast Analysis Using Flow Cytometry and Sorting. Flow Cytometry with Plant Cells. Weinheim: Wiley-VCH, 231–250. doi: 10.1002/9783527610921.ch10
Genty, B., Briantais, J.-M., and Baker, N. R. (1989). The relationship between the quantum yield of photosynthetic electron transport and quenching of chlorophyll fluorescence. Biochim. Biophys. Acta 990, 87–92. doi: 10.1016/S0304-4165(89)80016-9
Godaux, D., Emonds-Alt, B., Berne, N., Ghysels, B., Alric, J., Remacle, C., et al. (2013). A novel screening method for hydrogenase-deficient mutants in Chlamydomonas reinhardtii based on in vivo chlorophyll fluorescence and photosystem II quantum yield. Int. J. Hydrogen Energy 38, 1826–1836. doi: 10.1016/j.ijhydene.2012.11.081
Golan, T., Li, X.-P., Muller-Moule, P., and Niyogi, K. K. (2004). “Using mutants to understand light stress acclimation in plants,”,” in Chlorophyll a Fluorescence: A Signature of Photosynthesis, eds G. C. Papageorgiou and Govindjee (Dordrecht: Springer Netherlands), 525–554.
Grahl, S., Reiter, B., Gügel, I. L., Vamvaka, E., Gandini, C., Jahns, P., et al. (2016). The Arabidopsis protein CGLD11 is required for chloroplast ATP synthase accumulation. Mol. Plant 9, 885–899. doi: 10.1016/j.molp.2016.03.002
Grieco, M., Tikkanen, M., Paakkarinen, V., Kangasjarvi, S., and Aro, E.-M. (2012). Steady-state phosphorylation of light-harvesting complex II proteins preserves photosystem I under fluctuating white light. Plant Physiol. 160, 1896–1910. doi: 10.1104/pp.112.206466
Grossman, A. R., Catalanotti, C., Yang, W., Dubini, A., Magneschi, L., Subramanian, V., et al. (2011). Multiple facets of anoxic metabolism and hydrogen production in the unicellular green alga Chlamydomonas reinhardtii. New Phytol. 190, 279–288. doi: 10.1111/j.1469-8137.2010.03534.x
Harkins, K. R., Jefferson, R. A., Kavanagh, T. A., Bevan, M. W., and Galbraith, D. W. (1990). Expression of photosynthesis-related gene fusions is restricted by cell type in transgenic plants and in transfected protoplasts. Proc. Natl. Acad. Sci. U.S.A. 87, 816–820. doi: 10.1073/pnas.87.2.816
Harris, E. H. (1989). “Nuclear mutations described in C. reinhardtii,” in The Chlamydomonas Sourcebook: A Comprehensive Guide to Biology and Laboratory Use, ed. E. H. Harris (San Diego, CA: Academic Press, Inc.), 461–542.
Hashimoto, M., Endo, T., Peltier, G., Tasaka, M., and Shikanai, T. (2003). A nucleus-encoded factor, CRR2, is essential for the expression of chloroplast ndhB in Arabidopsis. Plant J. 36, 541–549. doi: 10.1046/j.1365-313X.2003.01900.x
Hemschemeier, A., Melis, A., and Happe, T. (2009). Analytical approaches to photobiological hydrogen production in unicellular green algae. Photosynth. Res. 102, 523–540. doi: 10.1007/s11120-009-9415-5
Hertle, A. P., Blunder, T., Wunder, T., Pesaresi, P., Pribil, M., Armbruster, U., et al. (2013). PGRL1 is the elusive ferredoxin-plastoquinone reductase in photosynthetic cyclic electron flow. Mol. Cell 49, 511–523. doi: 10.1016/j.molcel.2012.11.030
Hildebrand, M., Davis, A., Abbriano, R., Pugsley, H. R., Traller, J. C., Smith, S. R., et al. (2016). “Applications of imaging flow cytometry for microalgae,” in Imaging Flow Cytometry, eds N. Barteneva and I. Vorobjev (New York, NY: Humana Press), 47–67.
Houille-Vernes, L., Rappaport, F., Wollman, F.-A., Alric, J., and Johnson, X. (2011). Plastid terminal oxidase 2 (PTOX2) is the major oxidase involved in chlororespiration in Chlamydomonas. Proc. Natl. Acad. Sci. U.S.A. 108, 20820–20825. doi: 10.1073/pnas.1110518109
Humplík, J. F., Lazár, D., Fürst, T., Husičková, A., Hýbl, M., and Spíchal, L. (2015). Automated integrative high-throughput phenotyping of plant shoots: a case study of the cold-tolerance of pea (Pisum sativum L.). Plant Methods 11:20. doi: 10.1186/s13007-015-0063-9
Hyka, P., Lickova, S., Přibyl, P., Melzoch, K., and Kovar, K. (2013). Flow cytometry for the development of biotechnological processes with microalgae. Biotechnol. Adv. 31, 2–16. doi: 10.1016/j.biotechadv.2012.04.007
Ifuku, K., Endo, T., Shikanai, T., and Aro, E.-M. (2011). Structure of the chloroplast NADH dehydrogenase-like complex: nomenclature for nuclear-encoded subunits. Plant Cell Physiol. 52, 1560–1568. doi: 10.1093/pcp/pcr098
Ihnatowicz, A., Pesaresi, P., Varotto, C., Richly, E., Schneider, A., Jahns, P., et al. (2004). Mutants for photosystem I subunit D of Arabidopsis thaliana: effects on photosynthesis, photosystem I stability and expression of nuclear genes for chloroplast functions. Plant J. 37, 839–852. doi: 10.1111/j.1365-313X.2003.02011.x
Jansen, M., Gilmer, F., Biskup, B., Nagel, K. A., Rascher, U., Fischbach, A., et al. (2009). Simultaneous phenotyping of leaf growth and chlorophyll fluorescence via Growscreen Fluoro allows detection of stress tolerance in Arabidopsis thaliana and other rosette plants. Funct. Plant Biol. 36, 902–914. doi: 10.1071/FP09095
Jung, H.-S., and Niyogi, K. K. (2009). Quantitative genetic analysis of thermal dissipation in Arabidopsis. Plant Physiol. 150, 977–986. doi: 10.1104/pp.109.137828
Kalaji, H. M., Schansker, G., Brestic, M., Bussotti, F., Calatayud, A., Ferroni, L., et al. (2017). Frequently asked questions about chlorophyll fluorescence, the sequel. Photosynth. Res. 132, 13–66. doi: 10.1007/s11120-016-0318-y
Kalituho, L., Graßes, T., Graf, M., Rech, J., and Jahns, P. (2006). Characterization of a nonphotochemical quenching-deficient Arabidopsis mutant possessing an intact PsbS protein, xanthophyll cycle and lumen acidification. Planta 223, 532–541. doi: 10.1007/s00425-005-0093-z
Kramer, D. M., and Evans, J. R. (2011). The importance of energy balance in improving photosynthetic productivity. Plant Physiol. 155, 70–78. doi: 10.1104/pp.110.166652
Krause, G. H., and Weis, E. (1991). Chlorophyll fluorescence and photosynthesis: the basics. Annu. Rev. Plant Physiol. Plant Mol. Biol. 42, 313–349. doi: 10.1146/annurev.pp.42.060191.001525
Kruse, O., Nixon, P. J., Schmid, G. H., and Mullineaux, C. W. (1999). Isolation of state transition mutants of Chlamydomonas reinhardtii by fluorescence video imaging. Photosynth. Res. 61, 43–51. doi: 10.1023/A:1006229308606
Külheim, C., Agren, J., and Jansson, S. (2002). Rapid regulation of light harvesting and plant fitness in the field. Science 297, 91–93. doi: 10.1126/science.1072359
Küpper, H., Šetlík, I., Trtílek, M., and Nedbal, L. (2000). A microscope for two-dimensional measurements of in vivo chlorophyll fluorescence kinetics using pulsed measuring radiation, continuous actinic radiation, and saturating flashes. Photosynthetica 38, 553–570. doi: 10.1023/A:1012461407557
Lezhneva, L., Amann, K., and Meurer, J. (2004). The universally conserved HCF101 protein is involved in assembly of [4Fe-4S]-cluster-containing complexes in Arabidopsis thaliana chloroplasts. Plant J. 37, 174–185. doi: 10.1046/j.1365-313X.2003.01952.x
Lezhneva, L., and Meurer, J. (2004). The nuclear factor HCF145 affects chloroplast psaA-psaB-rps14 transcript abundance in Arabidopsis thaliana. Plant J. 38, 740–753. doi: 10.1111/j.1365-313X.2004.02081.x
Li, X., Zhang, R., Patena, W., Gang, S. S., Blum, S. R., Ivanova, N., et al. (2016). An indexed, mapped mutant library enables reverse genetics studies of biological processes in Chlamydomonas reinhardtii. Plant Cell 28, 367–387. doi: 10.1105/tpc.16.00465
Li, X. P., Björkman, O., Shih, C., Grossman, A. R., Rosenquist, M., Jansson, S., et al. (2000). A pigment-binding protein essential for regulation of photosynthetic light harvesting. Nature 403, 391–395. doi: 10.1038/35000131
Livingston, A. K., Cruz, J. A., Kohzuma, K., Dhingra, A., and Kramer, D. M. (2010). An Arabidopsis mutant with high cyclic electron flow around photosystem I (hcef) involving the NADPH dehydrogenase complex. Plant Cell 22, 221–233. doi: 10.1105/tpc.109.071084
Lloyd, J., and Meinke, D. (2012). A comprehensive dataset of genes with a loss-of-function mutant phenotype in Arabidopsis. Plant Physiol. 158, 1115–1129. doi: 10.1104/pp.111.192393
Malnoë, A., Schultink, A., Shahrasbi, S., Rumeau, D., Havaux, M., and Niyogi, K. K. (2017). The plastid lipocalin LCNP is required for sustained photoprotective energy dissipation in Arabidopsis. Plant Cell doi: 10.1105/tpc.17.00536 [Epub ahead of print].
Malnoe, A., Wang, F., Girard-Bascou, J., Wollman, F.-A., and de Vitry, C. (2014). Thylakoid FtsH protease contributes to photosystem II and cytochrome b6f remodeling in Chlamydomonas reinhardtii under stress conditions. Plant Cell 26, 373–390. doi: 10.1105/tpc.113.120113
Manavski, N., Torabi, S., Lezhneva, L., Arif, M. A., Frank, W., and Meurer, J. (2015). HIGH CHLOROPHYLL FLUORESCENCE145 binds to and stabilizes the psaA 5′ UTR via a newly defined repeat motif in Embryophyta. Plant Cell 27, 2600–2615. doi: 10.1105/tpc.15.00234
Massoz, S., Hanikenne, M., Bailleul, B., Coosemans, N., Radoux, M., Miranda-Astudillo, H., et al. (2017). In vivo chlorophyll fluorescence screening allows the isolation of a Chlamydomonas mutant defective for NDUFAF3, an assembly factor involved in mitochondrial complex I assembly. Plant J. 17, 2045–2054. doi: 10.1111/tpj.13677
Massoz, S., Larosa, V., Horrion, B., Matagne, R. F., Remacle, C., and Cardol, P. (2015). Isolation of Chlamydomonas reinhardtii mutants with altered mitochondrial respiration by chlorophyll fluorescence measurement. J. Biotechnol. 215, 27–34. doi: 10.1016/j.jbiotec.2015.05.009
Maxwell, K., and Johnson, G. N. (2000). Chlorophyll fluorescence–a practical guide. J. Exp. Bot. 51, 659–668. doi: 10.1093/jexbot/51.345.659
Meierhoff, K., Felder, S., Nakamura, T., Bechtold, N., and Schuster, G. (2003). HCF152, an Arabidopsis RNA binding pentatricopeptide repeat protein involved in the processing of chloroplast psbB-psbT-psbH-petB-petD RNAs. Plant Cell 15, 1480–1495. doi: 10.1105/tpc.010397
Merchant, S. S., Prochnik, S. E., Vallon, O., Harris, E. H., Karpowicz, S. J., Witman, G. B., et al. (2007). The Chlamydomonas genome reveals the evolution of key animal and plant functions. Science 318, 245–250. doi: 10.1126/science.1143609
Meurer, J., Berger, A., and Westhoff, P. (1996a). A nuclear mutant of Arabidopsis with impaired stability on distinct transcripts of the plastid psbB, psbD/C, ndhH, and ndhC operons. Plant Cell 8, 1193–1207. doi: 10.1105/tpc.8.7.1193
Meurer, J., Meierhoff, K., and Westhoff, P. (1996b). Isolation of high-chlorophyll-fluorescence mutants of Arabidopsis thaliana and their characterisation by spectroscopy, immunoblotting and northern hybridisation. Planta 198, 385–396. doi: 10.1007/BF00620055
Miles, C. D., and Daniel, D. J. (1973). A rapid screening technique for photosynthetic mutants of higher plants. Plant Sci. Lett. 1, 237–240. doi: 10.1016/0304-4211(73)90025-4
Miles, C. D., and Daniel, D. J. (1974). Chloroplast reactions of photosynthetic mutants in Zea mays. Plant Physiol. 53, 589–595. doi: 10.1104/pp.53.4.589
Mohr, S. E., Smith, J. A., Shamu, C. E., Neumüller, R. A., and Perrimon, N. (2014). RNAi screening comes of age: improved techniques and complementary approaches. Nat. Rev. Mol. Cell Biol. 15, 591–600. doi: 10.1038/nrm3860
Munekage, Y., Hojo, M., Meurer, J., Endo, T., Tasaka, M., and Shikanai, T. (2002). PGR5 Is involved in cyclic electron flow around photosystem I and is essential for photoprotection in Arabidopsis. Cell 110, 361–371. doi: 10.1016/S0092-8674(02)00867-X
Munekage, Y., Takeda, S., Endo, T., Jahns, P., Hashimoto, T., and Shikanai, T. (2001). Cytochrome b6f mutation specifically affects thermal dissipation of absorbed light energy in Arabidopsis. Plant J. 28, 351–359. doi: 10.1046/j.1365-313X.2001.01178.x
Munshi, M. K., Kobayashi, Y., and Shikanai, T. (2006). CHLORORESPIRATORY REDUCTION 6 is a novel factor required for accumulation of the chloroplast NAD(P)H dehydrogenase complex in Arabidopsis. Plant Physiol. 141, 737–744. doi: 10.1104/pp.106.080267.1
Nedbal, L., and Whitmarsh, J. (2004). “Chlorophyll fluorescence imaging of leaves and fruits,” in Chlorophyll a Fluorescence: A Signature of Photosynthesis, eds G. C. Papageorgiou and Govindjee (Dordrecht: Springer Netherlands), 389–407. doi: 10.1007/978-1-4020-3218-9
Niyogi, K., Bjorkman, O., and Grossman, A. (1997). Chlamydomonas xanthophyll cycle mutants identified by video imaging of chlorophyll fluorescence quenching. Plant Cell 9, 1369–1380. doi: 10.1105/tpc.9.8.1369
Niyogi, K. K., Grossman, A. R., and Bjorkman, O. (1998). Arabidopsis mutants define a central role for the xanthophyll cycle in the regulation of photosynthetic energy conversion. Plant Cell 10, 1121–1134. doi: 10.1105/tpc.10.7.1121
O’Malley, R. C., and Ecker, J. R. (2010). Linking genotype to phenotype using the Arabidopsis unimutant collection. Plant J. 61, 928–940. doi: 10.1111/j.1365-313X.2010.04119.x
Omasa, K., Shimazaki, K., Aiga, I., Larcher, W., and Onoe, M. (1987). Image analysis of chlorophyll fluorescence transients for diagnosing the photosynthetic system of attached leaves. Plant Physiol. 84, 748–752. doi: 10.1104/pp.84.3.748
Ort, D. R. (2001). When there is too much light. Plant Physiol. 125, 29–32. doi: 10.1104/pp.125.1.29
Oxborough, K. (2004). “Using chlorophyll a fluorescence imaging to monitor photosynthetic performance,” in Chlorophyll a Fluorescence: A Signature of Photosynthesis, eds G. C. Papageorgiou and Govindjee (Dordrecht: Springer Netherlands), 409–428.
Oxborough, K., and Baker, N. R. (1997). An instrument capable of imaging chlorophyll a fluorescence from intact leaves at very low irradiance and at cellular and subcellular levels of organization. Plant Cell Environ. 20, 1473–1483. doi: 10.1046/j.1365-3040.1997.d01-42.x
Peng, L., Ma, J., Chi, W., Guo, J., Zhu, S., Lu, Q., et al. (2006). LOW PSII ACCUMULATION1 is involved in efficient assembly of photosystem II in Arabidopsis thaliana. Plant Cell 18, 955–969. doi: 10.1105/tpc.105.037689
Peng, L., Yamamoto, H., and Shikanai, T. (2011). Structure and biogenesis of the chloroplast NAD(P)H dehydrogenase complex. Biochim. Biophys. Acta 1807, 945–953. doi: 10.1016/j.bbabio.2010.10.015
Pesaresi, P., Gardner, N. A., Masiero, S., Dietzmann, A., Eichacker, L., Wickner, R., et al. (2003). Cytoplasmic N-terminal protein acetylation is required for efficient photosynthesis in Arabidopsis. Plant Cell 15, 1817–1832. doi: 10.1105/tpc.012377.NatA
Posewitz, M. C., King, P. W., Smolinski, S. L., Zhang, L., Seibert, M., and Ghirardi, M. L. (2004). Discovery of two novel radical S-adenosylmethionine proteins required for the assembly of an active [Fe] hydrogenase. J. Biol. Chem. 279, 25711–25720. doi: 10.1074/jbc.M403206200
Pribil, M., Pesaresi, P., Hertle, A., Barbato, R., and Leister, D. (2010). Role of plastid protein phosphatase TAP38 in LHCII dephosphorylation and thylakoid electron flow. PLOS Biol. 8:e1000288. doi: 10.1371/journal.pbio.1000288
Roháček, K. (2002). Chlorophyll fluorescence parameters: the definitions, photosynthetic meaning, and mutual relationships. Photosynthetica 40, 13–29. doi: 10.1023/A:1020125719386
Ruban, A. V. (2016). Nonphotochemical chlorophyll fluorescence quenching: mechanism and effectiveness in protecting plants from photodamage. Plant Physiol. 170, 1903–1916. doi: 10.1104/pp.15.01935
Rühle, T., Razeghi, J. A., Vamvaka, E., Viola, S., Gandini, C., Kleine, T., et al. (2014). The Arabidopsis protein CONSERVED ONLY IN THE GREEN LINEAGE160 promotes the assembly of the membranous part of the chloroplast ATP synthase. Plant Physiol. 165, 207–226. doi: 10.1104/pp.114.237883
Rungrat, T., Awlia, M., Brown, T., Cheng, R., Sirault, X., Fajkus, J., et al. (2016). Using phenomic analysis of photosynthetic function for abiotic stress response gene discovery. Arabidopsis Book 14:e0185. doi: 10.1199/tab.0185
Scheibe, R. (2004). Malate valves to balance cellular energy supply. Physiol. Plant. 120, 21–26. doi: 10.1111/j.0031-9317.2004.0222.x
Schmitz, J., Heinrichs, L., Scossa, F., Fernie, A. R., Oelze, M. L., Dietz, K. J., et al. (2014). The essential role of sugar metabolism in the acclimation response of Arabidopsis thaliana to high light intensities. J. Exp. Bot. 65, 1619–1636. doi: 10.1093/jxb/eru027
Schmitz, J., Schöttler, M., Krueger, S., Geimer, S., Schneider, A., Kleine, T., et al. (2012). Defects in leaf carbohydrate metabolism compromise acclimation to high light and lead to a high chlorophyll fluorescence phenotype in Arabidopsis thaliana. BMC Plant Biol. 12:8. doi: 10.1186/1471-2229-12-8
Schreiber, U., Bilger, W., and Neubauer, C. (1995). “Chlorophyll fluorescence as a nonintrusive indicator for rapid assessment of In Vivo photosynthesis,” in Ecophysiology of Photosynthesis, eds E. D. Schulze and M. Caldwell (Berlin: Springer), 49–70.
Schreiber, U., Schliwa, U., and Bilger, W. (1986). Continuous recording of photochemical and non-photochemical chlorophyll fluorescence quenching with a new type of modulation fluorometer. Photosynth. Res. 10, 51–62.
Schroda, M., Vallon, O., Wollman, F. A., and Beck, C. F. (1999). A chloroplast-targeted heat shock protein 70 (HSP70) contributes to the photoprotection and repair of photosystem II during and after photoinhibition. Plant Cell Online 11, 1165–1178. doi: 10.1105/tpc.11.6.1165
Shikanai, T., Endo, T., Hashimoto, T., Yamada, Y., Asada, K., and Yokota, A. (1998). Directed disruption of the tobacco ndhB gene impairs cyclic electron flow around photosystem I. Proc. Natl. Acad. Sci. U.S.A. 95, 9705–9709.
Shikanai, T., Munekage, Y., Shimizu, K., Endo, T., and Hashimoto, T. (1999). Identification and characterization of Arabidopsis mutants with reduced quenching of chlorophyll fluorescence. Plant Cell Physiol. 40, 1134–1142.
Somerville, C. R. (1986). Analysis of photosynthesis with mutants of higher plants and algae. Annu. Rev. Plant Physiol. 37, 467–506. doi: 10.1146/annurev.pp.37.060186.002343
Steinberger, I., Egidi, F., and Schneider, A. (2015). Chlorophyll fluorescence measurements in Arabidopsis wild-type and photosystem II mutant leaves. Bio-protocol 5:e1532. doi: 10.21769/BioProtoc.1532
Suorsa, M., Jarvi, S., Grieco, M., Nurmi, M., Pietrzykowska, M., Rantala, M., et al. (2012). PROTON GRADIENT REGULATION5 is essential for proper acclimation of Arabidopsis photosystem I to naturally and artificially fluctuating light conditions. Plant Cell 24, 2934–2948. doi: 10.1105/tpc.112.097162
Takabayashi, A., Ishikawa, N., Obayashi, T., Ishida, S., Obokata, J., Endo, T., et al. (2009). Three novel subunits of Arabidopsis chloroplastic NAD(P)H dehydrogenase identified by bioinformatic and reverse genetic approaches. Plant J. 57, 207–219. doi: 10.1111/j.1365-313X.2008.03680.x
Takahashi, S., Bauwe, H., and Badger, M. (2007). Impairment of the photorespiratory pathway accelerates photoinhibition of photosystem II by suppression of repair but not acceleration of damage processes in Arabidopsis. Plant Physiol. 144, 487–494. doi: 10.1104/pp.107.097253
Tardieu, F., Cabrera-Bosquet, L., Pridmore, T., and Bennett, M. (2017). Plant phenomics, from sensors to knowledge. Curr. Biol. 27, R770–R783. doi: 10.1016/j.cub.2017.05.055
Taylor, W. C., Barkan, A., and Martienssen, R. A. (1987). Use of nuclear mutants in the analysis of chloroplast development. Dev. Genet. 8, 305–320. doi: 10.1002/dvg.1020080503
Terashima, M., Freeman, E. S., Jinkerson, R. E., and Jonikas, M. C. (2015). A fluorescence-activated cell sorting-based strategy for rapid isolation of high-lipid Chlamydomonas mutants. Plant J. 81, 147–159. doi: 10.1111/tpj.12682
Terashima, M., Specht, M., Naumann, B., and Hippler, M. (2010). Characterizing the anaerobic response of Chlamydomonas reinhardtii by quantitative proteomics. Mol. Cell. Proteomics 9, 1514–1532. doi: 10.1074/mcp.M900421-MCP200
Thiele, A., Winter, K., and Krause, G. H. (1997). Low inactivation of D1 protein of photosystem II in young canopy leaves of Anacardium excelsum under high-light stress. J. Plant Physiol. 151, 286–292. doi: 10.1016/S0176-1617(97)80254-4
Tolleter, D., Ghysels, B., Alric, J., Petroutsos, D., Tolstygina, I., Krawietz, D., et al. (2011). Control of hydrogen photoproduction by the proton gradient generated by cyclic electron flow in Chlamydomonas reinhardtii. Plant Cell 23, 2619–2630. doi: 10.1105/tpc.111.086876
Tseng, Y.-C., and Chu, S.-W. (2017). High spatio-temporal-resolution detection of chlorophyll fluorescence dynamics from a single chloroplast with confocal imaging fluorometer. Plant Methods 13:43. doi: 10.1186/s13007-017-0194-2
van Rooijen, R., Aarts, M. G. M., and Harbinson, J. (2015). Natural genetic variation for acclimation of photosynthetic light use efficiency to growth irradiance in Arabidopsis. Plant Physiol. 167, 1412–1429. doi: 10.1104/pp.114.252239
Varotto, C., Maiwald, D., Pesaresi, P., Jahns, P., Salamini, F., and Leister, D. (2002). The metal ion transporter IRT1 is necessary for iron homeostasis and efficient photosynthesis in Arabidopsis thaliana. Plant J. 31, 589–599. doi: 10.1046/j.1365-313X.2002.01381.x
Varotto, C., Pesaresi, P., Maiwald, D., Kurth, J., Salamini, F., and Leister, D. (2000a). Identification of photosynthetic mutants of Arabidopsis by automatic screening for altered effective quantum yield of photosystem 2. Photosynthetica 38, 497–504. doi: 10.1023/A:1012445020761
Varotto, C., Pesaresi, P., Meurer, J., Oelmüller, R., Steiner-Lange, S., Salamini, F., et al. (2000b). Disruption of the Arabidopsis photosystem I gene psaE1 affects photosynthesis and impairs growth. Plant J. 22, 115–124. doi: 10.1046/j.1365-313x.2000.00717.x
Virlet, N., Sabermanesh, K., Sadeghi-Tehran, P., and Hawkesford, M. J. (2017). Field Scanalyzer: An automated robotic field phenotyping platform for detailed crop monitoring. Funct. Plant Biol. 44, 143–153. doi: 10.1071/FP16163
Walter, A., Liebisch, F., and Hund, A. (2015). Plant phenotyping: from bean weighing to image analysis. Plant Methods 11:14. doi: 10.1186/s13007-015-0056-8
Walters, R. G., Shephard, F., Rogers, J. J. M., Rolfe, S. A., and Horton, P. (2003). Identification of mutants of Arabidopsis defective in acclimation of photosynthesis to the light environment. Plant Physiol. 131, 472–481. doi: 10.1104/pp.015479
Wang, H., Qian, X., Zhang, L., Xu, S., Li, H., Xia, X., et al. (2017). Detecting crop population growth using chlorophyll fluorescence imaging. Appl. Opt. 56, 9762–9769. doi: 10.1364/AO.56.009762
White, J. W., Andrade-Sanchez, P., Gore, M. A., Bronson, K. F., Coffelt, T. A., Conley, M. M., et al. (2012). Field-based phenomics for plant genetics research. Field Crop Res. 133, 101–112. doi: 10.1016/j.fcr.2012.04.003
Xie, B., Stessman, D., Hart, J. H., Dong, H., Wang, Y., Wright, D. A., et al. (2014). High-throughput fluorescence-activated cell sorting for lipid hyperaccumulating Chlamydomonas reinhardtii mutants. Plant Biotechnol. J. 12, 872–882. doi: 10.1111/pbi.12190
Xu, L., Cruz, J. A., Savage, L. J., Kramer, D. M., and Chen, J. (2015). Plant photosynthesis phenomics data quality control. Bioinformatics 31, 1796–1804. doi: 10.1093/bioinformatics/btu854
Yamori, W., and Shikanai, T. (2016). Physiological functions of cyclic electron transport around photosystem I in sustaining photosynthesis and plant growth. Annu. Rev. Plant Biol. 67, 81–106. doi: 10.1146/annurev-arplant-043015-112002
Yang, Y., Xu, L., Feng, Z., Cruz, J. A., Savage, L. J., Kramer, D. M., et al. (2017). PhenoCurve: capturing dynamic phenotype-environment relationships using phenomics data. Bioinformatics 33, 1370–1378. doi: 10.1093/bioinformatics/btw673
Yin, K., Gao, C., and Qiu, J.-L. (2017). Progress and prospects in plant genome editing. Nat. Plants 3:17107. doi: 10.1038/nplants.2017.107
You, M. K., Lim, S. H., Kim, M. J., Jeong, Y. S., Lee, M. G., and Ha, S. H. (2015). Improvement of the fluorescence intensity during a flow cytometric analysis for rice protoplasts by localization of a green fluorescent protein into chloroplasts. Int. J. Mol. Sci. 16, 788–804. doi: 10.3390/ijms16010788
Keywords: photosynthesis, Arabidopsis, Chlamydomonas, chloroplast, screening, chlorophyll fluorescence, forward genetic screen, reverse genetic screen
Citation: Rühle T, Reiter B and Leister D (2018) Chlorophyll Fluorescence Video Imaging: A Versatile Tool for Identifying Factors Related to Photosynthesis. Front. Plant Sci. 9:55. doi: 10.3389/fpls.2018.00055
Received: 26 October 2017; Accepted: 10 January 2018;
Published: 30 January 2018.
Edited by:
Juliette Jouhet, UMR5168 Laboratoire de Physiologie Cellulaire Vegetale (LPCV), FranceReviewed by:
Dimitris Petroutsos, Univ. Grenoble Alpes, CNRS, CEA, INRA, BIG-LPCV, Grenoble, FranceCopyright © 2018 Rühle, Reiter and Leister. This is an open-access article distributed under the terms of the Creative Commons Attribution License (CC BY). The use, distribution or reproduction in other forums is permitted, provided the original author(s) and the copyright owner are credited and that the original publication in this journal is cited, in accordance with accepted academic practice. No use, distribution or reproduction is permitted which does not comply with these terms.
*Correspondence: Thilo Rühle, dGhpbG8ucnVlaGxlQGJpb2xvZ2llLnVuaS1tdWVuY2hlbi5kZQ==; dGhpbG9ydWVobGVAaG90bWFpbC5jb20=
Disclaimer: All claims expressed in this article are solely those of the authors and do not necessarily represent those of their affiliated organizations, or those of the publisher, the editors and the reviewers. Any product that may be evaluated in this article or claim that may be made by its manufacturer is not guaranteed or endorsed by the publisher.
Research integrity at Frontiers
Learn more about the work of our research integrity team to safeguard the quality of each article we publish.