- Centro de Biotecnología y Genómica de Plantas, Universidad Politécnica de Madrid (UPM), Instituto Nacional de Investigación y Tecnología Agraria y Alimentaria (INIA), Madrid, Spain
One of the main hurdles to engineer nitrogenase in a non-diazotrophic host is achieving NifB activity. NifB is an extremely unstable and oxygen sensitive protein that catalyzes a low-potential SAM-radical dependent reaction. The product of NifB activity is called NifB-co, a complex [8Fe-9S-C] cluster that serves as obligate intermediate in the biosyntheses of the active-site cofactors of all known nitrogenases. Here we study the diversity and phylogeny of naturally occurring NifB proteins, their protein architecture and the functions of the distinct NifB domains in order to understand what defines a catalytically active NifB. Focus is on NifB from the thermophile Chlorobium tepidum (two-domain architecture), the hyperthermophile Methanocaldococcus infernus (single-domain architecture) and the mesophile Klebsiella oxytoca (two-domain architecture), showing in silico characterization of their nitrogen fixation (nif) gene clusters, conserved NifB motifs, and functionality. C. tepidum and M. infernus NifB were able to complement an Azotobacter vinelandii (ΔnifB) mutant restoring the Nif+ phenotype and thus demonstrating their functionality in vivo. In addition, purified C. tepidum NifB exhibited activity in the in vitro NifB-dependent nitrogenase reconstitution assay. Intriguingly, changing the two-domain K. oxytoca NifB to single-domain by removal of the C-terminal NifX-like extension resulted in higher in vivo nitrogenase activity, demonstrating that this domain is not required for nitrogen fixation in mesophiles.
Introduction
Although nitrogen is abundant on Earth, most of it is in the form of dinitrogen (N≡N or N2). Due to the strength of its triple bound, N2 shows very little reactivity and is therefore not easily available to living organisms (Hoffman et al., 2014). N2 fixing organisms (diazotrophs) capable of converting N2 into NH3, an accessible form of nitrogen, probably appeared in the primordial Earth when the levels of combined nitrogen gradually depleted (Raymond et al., 2004; Canfield et al., 2010). Although evolution and fine-tuning of biological nitrogen fixation (BNF) had an immense impact on the Earth’s nitrogen cycle and allowed life to prosper, only a few bacteria and archaea are actually capable of performing it (Boyd and Peters, 2013). The enzymes that catalyze N2 fixation are called nitrogenases (Burris and Roberts, 1993). Nitrogenases are two-component protein complexes, with a catalytic Component I and a Component II acting as obligate electron donor (Bulen and Lecomte, 1966). Three genetically and biochemically distinct classes of nitrogenases have been described to date: the molybdenum nitrogenase, the vanadium nitrogenase, and the iron-only nitrogenase (Bishop and Joerger, 1990). All diazotrophs carry the Mo-nitrogenase and may or may not carry the V or Fe-only ones, referred to as alternative nitrogenases (Dos Santos et al., 2012; Mcglynn et al., 2013). In the case of the Mo-nitrogenase, the Component I is called MoFe protein and is a heterotetramer of the nifD and nifK gene products, whereas the Component II is called Fe protein and is a homodimer of the nifH gene product. A functional nitrogenase complex requires three metal cofactors embedded in the polypeptide chains to reduce N2 to NH3 (Peters et al., 2011). The NifH homodimer carries a [4Fe–4S] cluster located between the two NifH subunits (Georgiadis et al., 1992), while NifDK harbors an [8Fe–7S] P-cluster at the interface of each NifD (α) and NifK (β) subunits, and an iron-molybdenum cofactor (FeMo-co; [7Fe-9S-C-Mo-homocitrate]) embedded 10 Å beneath the surface of each NifD subunit (Einsle et al., 2002; Spatzal et al., 2011). Alternative nitrogenases contain a third type of subunit in Component I, encoded by vnfG (V-nitrogenase) or anfG (Fe-only nitrogenase), and either FeV or FeFe cofactors at the active site. These cofactors are proposed to be identical to FeMo-co except for containing V or Fe in place of Mo (Eady, 1996).
NifB stands out as the only protein essential for the activity all nitrogenases (in addition to homocitrate synthase) (Joerger and Bishop, 1988; Dos Santos et al., 2012). NifB is an S-adenosyl methionine (SAM)-radical protein that converts [4Fe-4S] clusters into NifB-co, an [8Fe-9S-C] cluster that serves as precursor to FeMo-co, FeV-co and FeFe-co, thus catalyzing the first committed step in nitrogenase active-site cofactor biosynthesis (Shah et al., 1994; Allen et al., 1995; Curatti et al., 2006; George et al., 2008; Wiig et al., 2012) (Supplementary Figure 1). In contrast to FeMo-co, NifB-co is a diamagnetic cluster containing two spectroscopically distinct Fe sites (Guo et al., 2016).
NifB proteins were first purified from the model bacteria Azotobacter vinelandii (Curatti et al., 2006) and Klebsiella oxytoca (Zhao et al., 2007). The NifBAv and the NifBKo proteins contain a C-terminal NifX-like extension that appears to result from gene fusions during evolution of nifB (Boyd et al., 2011). The NifX protein is known to bind and transfer NifB-co to the NifEN scaffold protein for further processing into FeMo-co (Hernandez et al., 2007), but the role of the NifX-domain of NifB is not known. NifB from the archaea Methanocaldococcus infernus, expressed and purified from recombinant Escherichia coli cells, was stable and enabled biochemical characterization (Echavarri-Erasun et al., 2014). Electron paramagnetic resonance (EPR) studies identified three [4Fe–4S] clusters in NifBMi: the SAM-binding [4Fe–4S] cluster and two auxiliary [4Fe–4S] clusters thought to act as substrates for NifB-co synthesis (Wilcoxen et al., 2016). Amino acid residues involved in the coordination of two of these metal clusters were identified by site-directed mutagenesis. NifBMi was found capable of FeMo-co synthesis in vitro, and exhibited both SAM radical chemistry and SAM demethylation reactions. Additionally, NifB proteins from the archaea Methanosarcina acetivorans and Methanobacterium thermoautotrophicum purified from recombinant E. coli cells were found to catalyze carbide insertion into the FeMo-co precursor (Fay et al., 2015). Importantly, none of the studied archaeal NifB proteins contained the NifX-like extension, showing its dispensability in the in vitro FeMo-co synthesis assays for this particular NifB subfamily.
In this work, we have compared the diversity, phylogeny, and domain architecture of 390 putative NifB proteins to understand the minimal requirements for NifB activity. We further used genetic complementation to investigate the in vivo functionality of NifB from a hyperthermophilic anaerobic Euryarchaea, a thermophilic anaerobic green sulfur bacterium, and a mesophilic γ-proteobacterium, representing the three existing NifB protein architectures. Finally, NifB from Chlorobium tepidum was purified from a recombinant A. vinelandii strain and characterized in vitro.
Results
Generation of a Representative NifB Database
The 390 putative NifB sequences found in the Structure and Function Linkage Database (SFLD) (Akiva et al., 2014) are shown in the Supplementary Table 1. Since SFLD “relates specific sequence-structure features to specific chemical capabilities,” and is therefore not immune to faulty annotations, we identified specific NifB fingerprint motifs and applied them as filter to curate the database. By aligning experimentally proven NifB proteins from A. vinelandii (NifBAv) (Curatti et al., 2006), K. oxytoca (NifBKo) (Zhao et al., 2007), Clostridium acetobutylicum (NifBCa) (Chen et al., 2001; Wiig et al., 2011), M. infernus (NifBMi) (Wilcoxen et al., 2016), Methanosarcina acetivorans (NifBMa) (Fay et al., 2015), Methanobacterium thermoautotrophicum (NifBMt) (Fay et al., 2015), and C. tepidum (NifBCt, this work), a number of conserved motifs were identified in the SAM-radical domain including an HPC motif, the AdoMet motif (Cx3Cx2C) common to all SAM-radical proteins, an ExRP motif, an AGPG motif, a TxTxN motif and a Cx2CRxDAxG motif. Putative NifB proteins that did not present all these motifs were eliminated from the dataset, which was then reduced by 28% down to 289 sequences (Figure 1 and Supplementary Table 1).
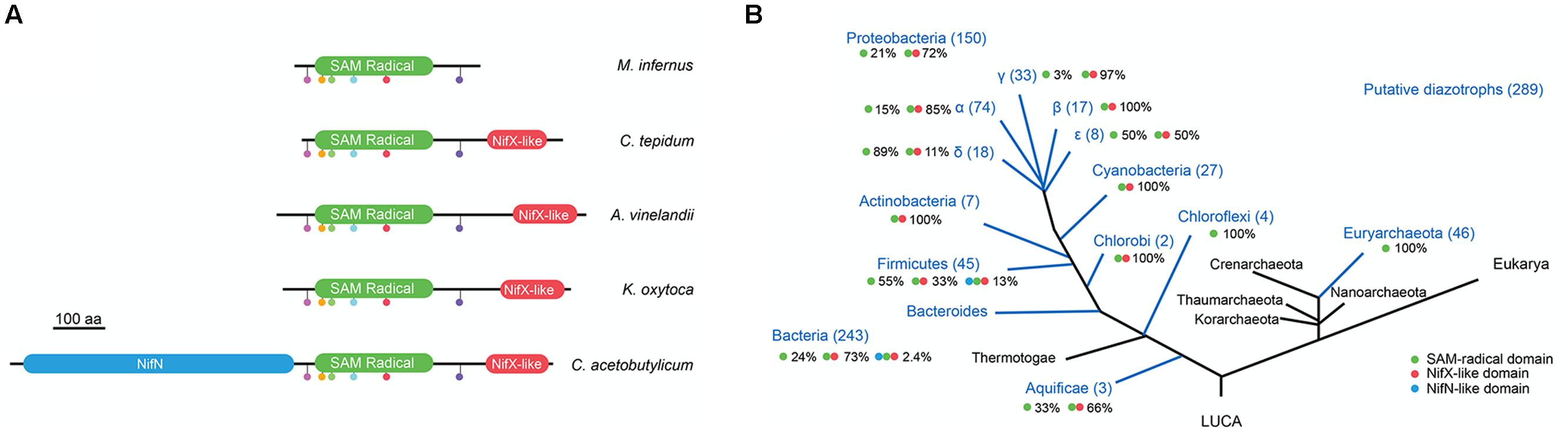
FIGURE 1. Occurrence NifB architectures in diazotrophs. (A) Scheme of the three different NifB architectures and representative species known to possess them. The SAM-radical is shown in green, the NifX-like domain in red, and the NifN-like domain in blue. Color dots represent motifs strictly conserved in the SAM-radical domain. The scale bar represents 100 amino acids. (B) Overall distribution and relative frequency of NifB architectures in putative diazotrophs. Green dot represents the SAM-radical domain; red dot represents the NifX-like domain; blue dot represents the NifN-like domain. This panel was generated by overlapping data from Supplementary Table 1 with a 3-domain taxonomic tree of life modified from Boyd and Peters (2013)..
Phylogenetic Distribution of Three Distinct NifB Domain Architectures
The most widely occurring NifB domain architecture consists of an N-terminal SAM-radical domain linked to a C-terminal NifX-like domain (Figure 1A). This protein configuration accounted for 73% of NifB sequences in the Bacteria domain of the curated database (Figure 1B and Supplementary Table 2). This configuration has been proposed to emerge after an ancestral gene fusion event (Curatti et al., 2006; Boyd et al., 2011). The functionality of this NifB subfamily has been demonstrated in vivo in many bacteria, and in vitro for NifBAv and NifBKo (Curatti et al., 2006; Zhao et al., 2007). A second NifB subfamily that included an additional NifN-like domain was found in 6 NifB sequences in the Bacteria domain (corresponding to 2.4% of the curated database). This NifB subfamily was first described in Clostridia (Chen et al., 2001) and then proven functional in vitro using purified preparations of an A. vinelandii engineered NifN-B fusion that mimicked the Clostridium protein (Wiig et al., 2011). However, in vivo complementation of an A. vinelandii ΔnifB mutant was not shown. Finally, a stand-alone SAM-radical domain was found in 104 NifB sequences, accounting for 100% of the Euryarchaeota and 24% of the Bacteria NifB proteins (Figure 1B). The functionality of this NifB subfamily has been demonstrated exclusively in vitro for M. infernus (NifBMi) (Wilcoxen et al., 2016), M. acetivorans (NifBMa) and M. thermoautotrophicum (NifBMt) (Fay et al., 2015).
Importantly, the Clostridium genus of the Firmicutes phylum is unique in that it contains all three NifB architectures. The curated NifB database contains 45 Firmicutes species likely to be diazotrophic organisms. Among these, 55% carry the stand-alone SAM-radical domain, 33% carry the two-domain architecture, and 13% carry the three-domain architecture (Figure 1B).
NifB Phylogeny Provides Information about the Evolution of Diazotrophs
Using the curated NifB database, 28 organisms representing the diversity of phylogenetic groups having diazotrophic members (Boyd and Peters, 2013) were selected to construct a circular phylogenetic tree (Figure 2A and Supplementary Table 2) and used as a reference to further overlap NifB phylogenetic trees. In this phylogenetic tree Archaea clade together, as out-group to Bacteria, forming two different subclades: the Methanococci (M. infernus and M. villosus) and the Methanobacteria (Methanobrevibacter smithii and Methanothermobacter thermautotrophicus). Bacteria diazotrophic species were distributed as follows: Aquaficae (Thermocrinis albus and Hydrogenobaculum sp.); Bacteroidetes (Dysgonomonas gadei and Paludibacter propionigenes), which clade with Chorobi (Chlorobium ferrooxidans, Chlorobium parvum, and Chlorobaculum tepidum); Actinobacteria (Frankia alni); Chloroflexi (Dehalococcoides mccartyi and Roseiflexus castenholzii); Cyanobacteria (Anabaena sp. and Cyanothece sp.); and Firmicutes (Clostridium kluyveri, C. acetobutylicum, and C. pasteurianum), all found in the same clade. Finally, α-proteobacteria (Rhodopseudomonas palustris, Bradyrhizobium japonicum, Rhodospirillum rubrum, and Rhodobacter capsulatus), β-proteobacteria (Azoarcus sp.), γ-proteobacteria (A. vinelandii, K. oxytoca and Pseudomonas stutzeri), and δ-proteobacteria (Arcobacter nitrofigilis) were all in the same clade.
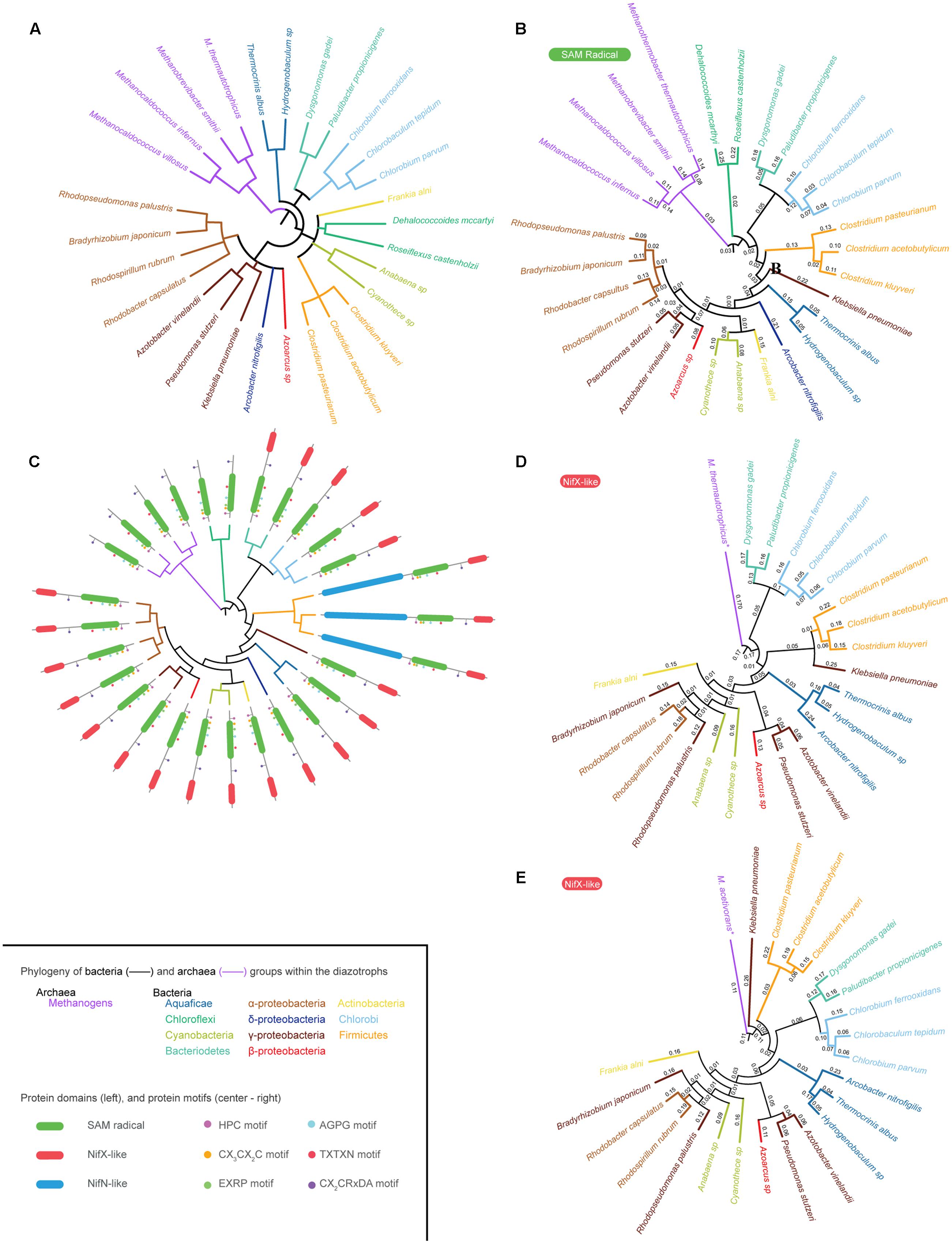
FIGURE 2. NifB phylogeny. (A) Phylogenetic tree of twenty-eight selected species representing all Bacteria and Archaea groups reported to carry nif genes. (B) Phylogenetic tree of twenty-eight NifB proteins based on their SAM-radical domain. (C) Overlap of the phylogenetic tree shown in (B) and existing NifB architectures. (D) Phylogenetic tree of twenty-two NifB proteins based on their NifX-like domain generated using M. thermautotrophicus NifX as root. (E) Phylogenetic tree of twenty-two NifB proteins based on their NifX-like domain generated using M. acetivorans NifX as root. The inset provides color code for the different Archaea and Bacteria groups with diazotroph members shown in the phylogenetic trees. It also details NifB protein domains and the strictly conserved motifs within the SAM-radical domain (C).
Because of the existence of three different NifB architectures, poorly aligned segments could potentially distort the phylogenetic tree analyses. Therefore, these regions were removed with Gblocks software (Talavera and Castresana, 2007) leaving a 315 contiguous amino acid sequence that was used to generate the SAM-radical domain tree (Figures 2B,C) and a 64 contiguous amino acid sequence used to generate two different NifX-like domain trees (Figures 2D,E).
The SAM-radical domain tree was rooted in M. infernus and is shown in Figure 2B. A derivative tree illustrating the distribution of NifB domain architecture is presented in Figure 2C. The Aquaficae, Actinobacteria, and Cyanobacteria did not clade with Firmicutes, as expected according to Figure 2A, but with Proteobacteria classes, leaving the Firmicutes as out-group to all of them. Interestingly, the γ-proteobacteria NifBKo was found as out-group to all proteobacteria in agreement with previous analysis (Boyd et al., 2011). The Chlorobi and Bacteroidetes NifB claded as expected. However, Chloroflexi NifB rooted deeper in the Bacteria, being the closest relative to Archaea NifB. Previous studies proposed that the entire nif operon might have been laterally transferred to Chloroflexi from an ancestral methanogen co-existing in a common ecological niche (Eisen et al., 2002). Our data support this hypothesis.
The phylogenetic signal of the NifX-like domain of NifB was also analyzed (Figures 2D,E). No Archaea NifB with a NifX-like domain has to our knowledge been found. Chloroflexi also lack this domain, suggesting acquisition from Archaea by a lateral gene transfer event (LGT) as previously suggested (Eisen et al., 2002; Boyd et al., 2011). Distinct NifX proteins encoded in the genomes of some methanogens were then used to root the trees. Two substantially different phylogenetic trees were obtained depending on the NifX protein used as root. Since NifX was not found in any Methanococci (i.e., M. infernus), Figure 2D uses NifX from Methanobacteriales (M. thermautotrophicus) and Figure 2E uses NifX from Methanosarcinales (M. acetivorans). The pattern of the first tree is similar to that of the SAM-radical domain tree, suggesting Chloroflexi as the bacterial ancestor from which the lineage emerged. The second tree, however, points to Firmicutes as the bacterial ancestor from which nif genes proliferated in Bacteria. This remains an interesting possibility given that Firmicutes present the three different NifB architectures known to date.
Organization of nif Genes in the Genomes of C. tepidum and M. infernus
In order to define essential and not essential domains for NifB function in vivo in an aerobic mesophilic host, we focused on NifB from the thermophile C. tepidum (two-domain architecture), the hyperthermophile M. infernus (single-domain architecture), and the mesophile K. oxytoca (two-domain architecture). NifBKo and NifBMi have previously been purified and characterized in vitro (Zhao et al., 2007; Wilcoxen et al., 2016) but not NifBCt, which is reported in this study.
Chlorobium tepidum is a well-described diazotroph (Wahlund and Madigan, 1993) with annotated genome (Eisen et al., 2002). Most of its nif genes are located in a single 20-kb cluster containing the Mo-nitrogenase structural genes (nifH, nifD, and nifK), FeMo-co biosynthetic genes (nifB, nifE, nifN, nifV, and fdxN), and regulatory genes (nifA, nifI1, and nifI2) (Supplementary Figure 2). Genome blast with individual nif genes from the model diazotroph K. oxytoca did not reveal anomalies, supporting the current C. tepidum annotation.
While NifBMi expressed in E. coli was shown to support FeMo-co synthesis in vitro (Wilcoxen et al., 2016), M. infernus has not yet been proven to be diazotrophic. The nif genes in the M. infernus genome consist of nifH, nifD, and nifK structural genes, nifB and nifE cofactor biosynthetic genes, and nifI1 and nifI2 regulatory genes. Intriguingly, a second nifH gene is located 17-kb apart from the nif cluster and nifB was found 470-kb apart with no apparent nif genes in close proximity.
NifBCt and NifBMi are Functional in Vivo When Expressed in the Aerobic Mesophilic Host A. vinelandii
Genetic complementation analyses were performed by expressing synthetic codon-optimized nifBCt and nifBMi genes in the A. vinelandii UW140 (ΔnifB) strain under the control of the nifH promoter (Figure 3A). A. vinelandii is a strict aerobe with optimum growth temperature of 30°C and is used here to provide an initial screen of NifB functionality that will be useful for further screening and implementation in Eukaryotic hosts. Strains UW418 (ΔnifB, PnifH::nifBMi) and UW422 (ΔnifB, PnifH::nifBCt) exhibited diazotrophic growth both in solid and liquid culture media (Figures 3B,D), in contrast to the Nif- phenotype of the parental strain UW140 (ΔnifB). Calculated diazotrophic growth rates (ln2/td) were: 0.23 for the wild type, <0.001 for UW140, 0.015 for UW418, and 0.13 for UW422. This data shows that, although both NifBCt and NifBMi originate from strict anaerobic and thermophilic microbes, the proteins were functional and could complement the A. vinelandii ΔnifB mutant phenotype. However, whereas NifBCt supported similar growth rate at 30°C as the A. vinelandii wild type strain, the recombinant NifBMi did not, possibly explained by the almost 40°C difference in optimal growth temperature between C. tepidum (48°C, Wahlund and Madigan, 1993) and M. infernus (85°C, Jeanthon et al., 1998). No difference in growth rate could be observed when using NH4+ as nitrogen source: 0.31 for the wild type, 0.29 for UW140, 0.30 for UW418, and 0.30 for UW422 (Figure 3C).
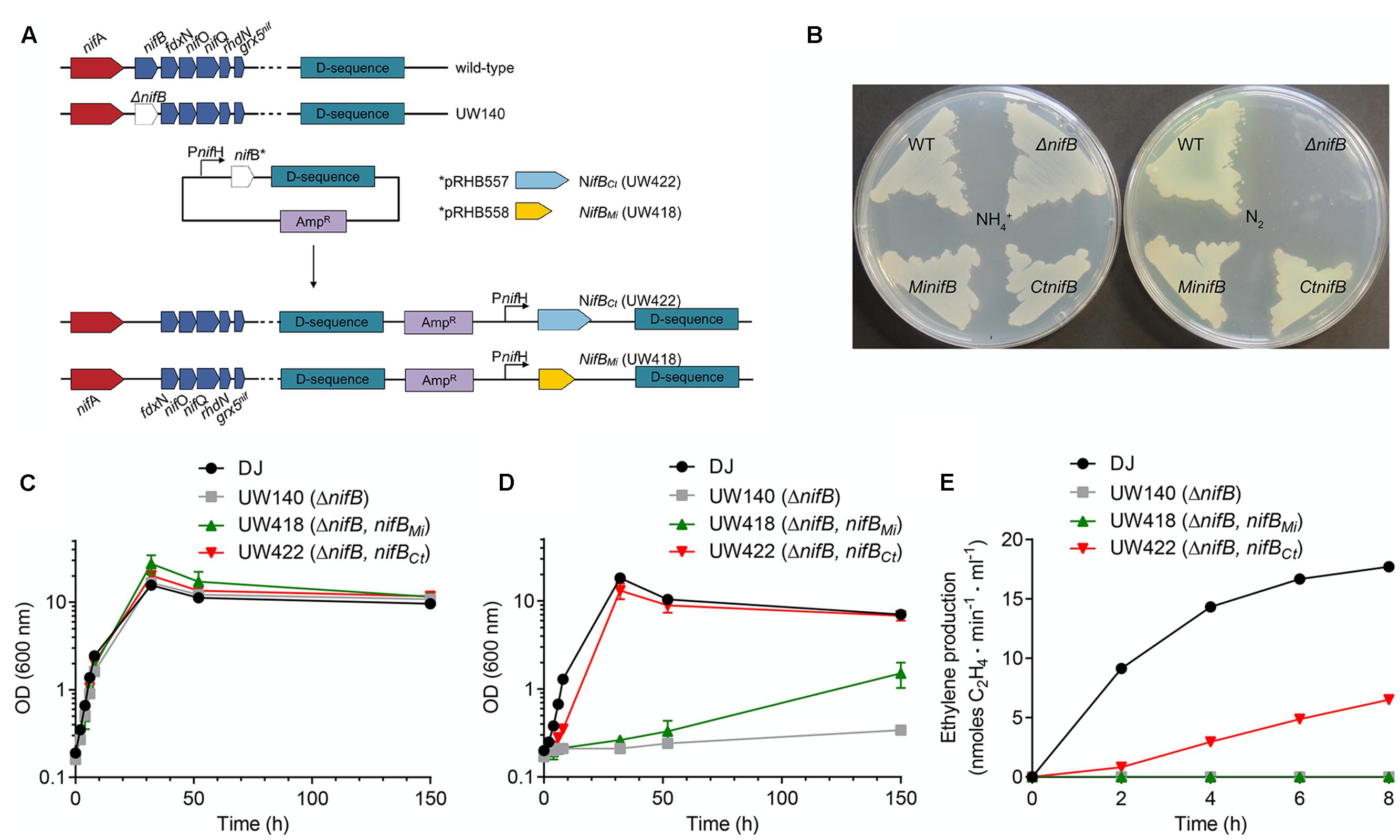
FIGURE 3. Genetic complementation of A. vinelandii ΔnifB with nifBCt and nifBMi genes. (A) Scheme showing the construction of A. vinelandii ΔnifB derivative strains carrying nifBMi (UW418) and nifBCt UW422 inserted in their chromosome under the control of a copy of the nifH promoter. (B) Petri dishes with solid Burk media showing growth of A. vinelandii DJ (wild-type strain, WT), UW140 (ΔnifB), UW418 (ΔnifB, PnifH::nifBMi) and UW422 (ΔnifB, PnifH::nifBCt) in the presence (left plate) or absence (right plate) of ammonium as nitrogen source. (C,D) Growth curves of DJ, UW140, UW418 and UW422 strains using ammonium (C) or N2 (D) as source of nitrogen. (E) In vivo acetylene reduction activity of nif derepressed A. vinelandii strains shown as nmol ethylene formed⋅min-1⋅ml-1 at a normalized OD of 1. UW140 and UW422 strains did not exhibit detectable activities during the 8-h period following derepression. Data in (C–E) represent means ± SD (n ≥ 2).
In vivo nitrogenase activities determined by the acetylene reduction assay showed significant activity in UW422 in the 8 h period following nitrogenase derepression (Figure 3E). No activity was detected in UW418 within this period of time, consistent with its significantly lower diazotrophic growth rate.
Purification and Biochemical Characterization of NifBCt
NifBCt was expressed and purified from a recombinant A. vinelandii strain (Figure 4A). The yield of pure NifBCt from A. vinelandii cells was 0.3 μg NifBCt per gram of cell, 15-fold higher than that of overexpressed NifBAv (Curatti et al., 2006). Purity of the NifBCt preparations exceeded 95%, as determined by Coomassie stained SDS-gels, and the identity of NifBCt was confirmed by MALDI-TOF analysis with 60% sequence coverage (Supplementary Table 3). NifBCt migrated as a monomer of 46.5 kDa in anaerobic size exclusion chromatography (Figure 4B), in good agreement with theoretical mass determined by the amino acid sequence (46.8 kDa). As isolated NifBCt contained 3.05 Fe atoms per monomer. In vitro reconstitution of its [Fe–S] clusters under reducing conditions increased Fe contents to 10.1 ± 0.07 Fe atoms (n = 3). Consistently, features characteristic of [Fe–S] proteins (especially the broad shoulder at 400-420 nm) were more prominent in the reconstituted NifBCt UV-vis spectrum (Figure 4C). Reconstituted NifBCt was active in the in vitro FeMo-co synthesis and nitrogenase activation assay: 5.2 ± 2.2 nmol ethylene formed⋅min-1⋅assay-1 (n = 2) compared to 8.2 ± 1.5 nmol ethylene formed⋅min-1⋅assay-1 (n = 2) when using pure NifB-co.
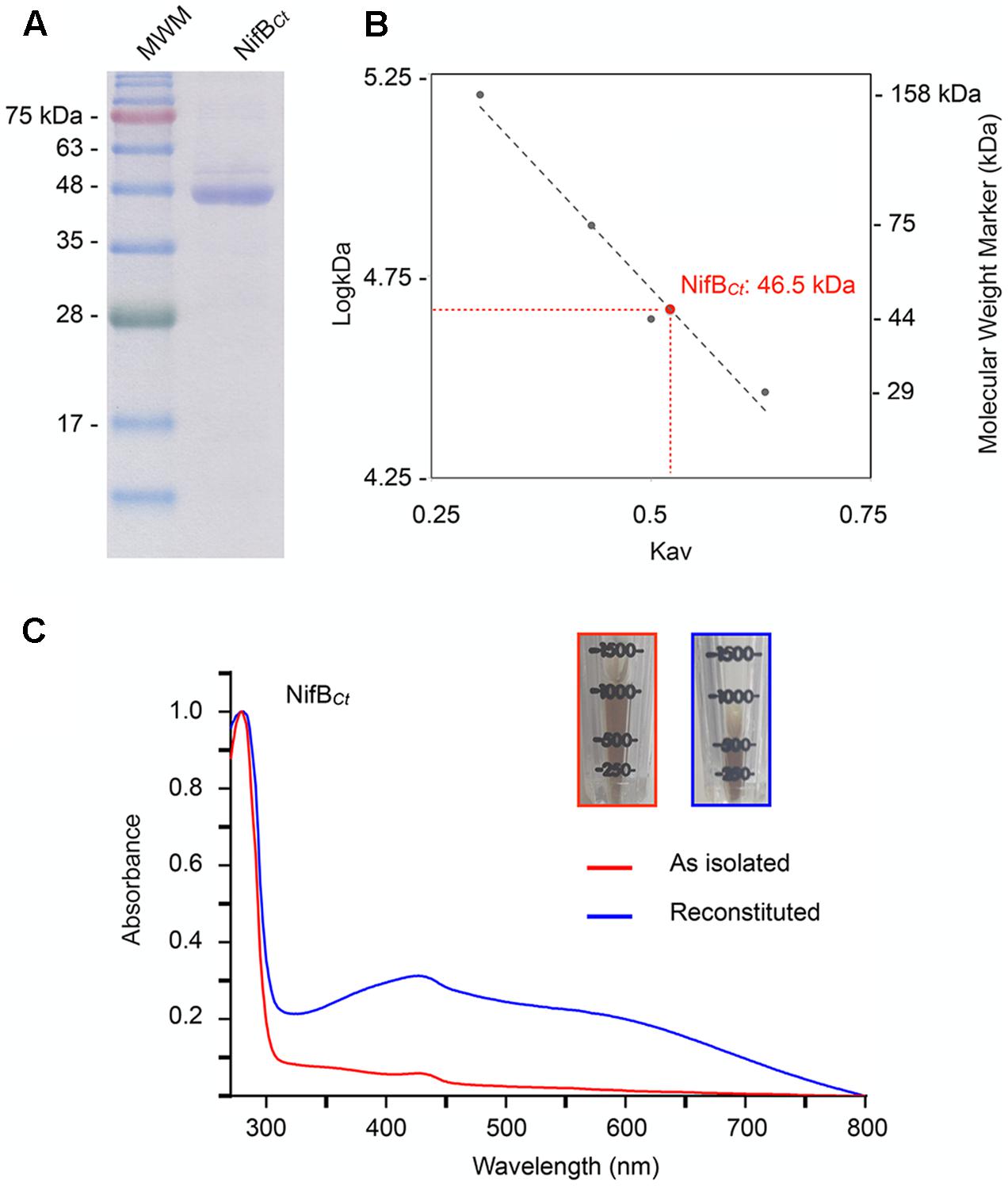
FIGURE 4. Molecular mass and UV-vis spectra of purified NifBCt preparations. (A) SDS-PAGE analysis of NifBCt purified from recombinant A. vinelandii UW422. (B) NifBCt native molecular weight as determined by size-exclusion chromatography against known protein weight markers. (C) UV-vis spectra of the as isolated (red) and [Fe–S] cluster reconstituted (blue) preparations of pure NifBCt. The inset pictures show the increase in color intensity upon [Fe–S] cluster reconstitution.
The NifX-like Domain of NifBKo Is Not Essential for Nitrogenase Activity or Diazotrophic Growth
The capacity of nifBMi to complement the ΔnifB strain strongly suggests that the SAM-radical domain of NifB is the only one required for the synthesis of the FeMo-co precursor, but this could be a property specific to the stand-alone SAM-radical domain subfamily. To determine whether the NifX-like domain naturally present in the two-domain NifB architecture is required for NifB-co synthesis, a truncated NifBKo variant lacking the entire NifX-like domain (nifBKo-ΔC) was generated, introduced in K. oxytoca UC9 (ΔnifB) and expressed under the control of a tac promoter (Figure 5A and Supplementary Figure 3). Additionally, as this truncated version would mimic a mesophilic single-domain NifB, we could test whether presence of the NifX-like domain is important for growth under moderate, non-thermophilic, temperatures. Diazotrophic growth and in vivo nitrogenase activity of UC28 (ΔnifB, Ptac::nifBKo-ΔC) were measured at 3 h intervals in a 24 h time course following derepression and compared to those of UC16 (ΔnifB, Ptac::nifBKo), a control strain expressing full-length NifBKo. Surprisingly, UC28 exhibited diazotrophic growth similar to UC16 and in vivo nitrogenase activity higher than UC16 (Figures 5B,C). The UC9 parental strain did not exhibit nitrogenase activity or diazotrophic growth, confirming that the functionality of the expressed NifBKo variants and suggesting that the NifX-like extension of NifBKo is not required for NifB-co synthesis, at least under the growth conditions tested in this study, and that this could be a general rule for the two-domain family of NifB proteins.
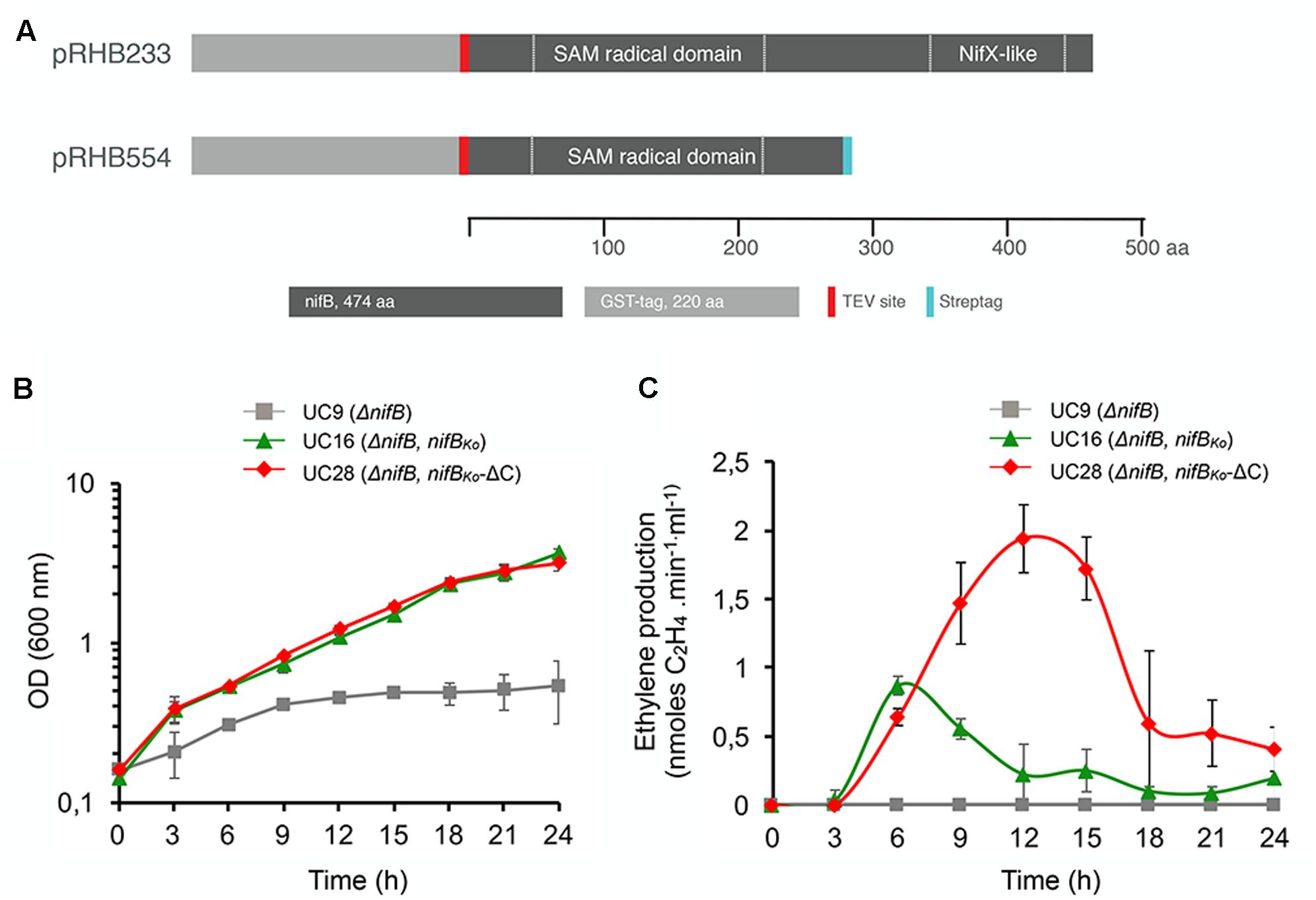
FIGURE 5. (A) Scheme of full-length GST-NifBKo and GST-NifBKo-ΔC in expression plasmids carried by UC16 and UC28, respectively. (B,C) Diazotrophic growth (B) and in vivo acetylene reduction activity (C) of K. oxytoca UC16 and UC28 expressing full-length and truncated NifB variants, respectively, compared to the UC9 (ΔnifB) strain. Data represent means ± SD (n = 3). Maximum ethylene production of K. oxytoca UN (wild-type) was 12.6 nmol⋅min-1⋅ml-1 (n = 3).
Discussion
NifB Phylogeny and Architecture
To our knowledge, this work presents the largest compilation of NifB proteins described to date. The NifB database was stringently filtered to exclude faulty annotated proteins and the curated dataset provides insights about NifB origin, taxonomy and architecture that complement previous work (Soboh et al., 2010; Boyd et al., 2011; Boyd and Peters, 2013). In this study we demonstrate that the SAM-radical domain of NifB is sufficient to support FeMo-co biosynthesis in vivo in the model organisms A. vinelandii and K. oxytoca.
A strict filter, based on motifs exhibited by experimentally confirmed NifB proteins, was applied to the initial database. As a result, 28% NifB sequences were excluded from further analysis. Although these criteria might be too strict, we reasoned that it was better to miss some true-positives than to risk including false-positives. Most excluded NifB proteins lacked the conserved Cx3Cx2C motif required for SAM-radical catalysis. In contrast, the NifX domain was identified in each one of them and we think that these faulty annotated NifB proteins are instead NifX. This confusion originates from the fact that the NifX domain is present in NifB, NafY, NifY as well as NifX proteins.
Three distinct NifB protein architectures exist. The most widespread in Bacteria consists of an N-terminal SAM-radical domain followed by a C-terminal NifX-like domain. However, this configuration is absent in Archaea, which present smaller NifB proteins consisting of a stand-alone SAM-radical domain. Boyd and collaborators investigated the lineage of the stand-alone SAM-radical domain in Archaea NifB proteins and compared it to the two-domain architecture favored in Bacteria (Boyd et al., 2011). The authors traced an event that suggested that a methanogen donated its nif cluster via LGT to a Firmicutes ancestor that co-existed in the same ecological niche. Then, a fusion event happened that resulted in the nifB-nifX protein occurring in Firmicutes. It was later suggested that the wide spread of the nifB-nifX fusion protein in Bacteria was independent of the selective pressure associated with aerobic diazotrophy (Boyd et al., 2015). An additional fusion event between nifN and nifB-nifX also occurred in Firmicutes leading to the three-domain NifB architecture. This last event was confined to Firmicutes, which is the only phylum presenting all three types of NifB architecture. It is surprising that the three-domain NifB was not widespread in Bacteria. From knowledge gained through in vitro FeMo-co synthesis studies (Curatti et al., 2007), it could be assumed that a NifENB fusion protein would be beneficial by protecting labile NifB-co and streamlining FeMo-co synthesis. However, it is possible that a NifENB fusion might not allow fine-tuning of precursor biosynthesis.
Based on the phylogeny of independent NifX proteins, another early nifB LGT was detected between Methanosarcinales and Chloroflexi. This event was also apparent in the SAM-radical domain phylogenetic tree, with Chloroflexi rooting deeper than any other group. The short distance between Methanosarcinales and Chloroflexi NifB lineages was also observed by Boyd and colleagues (Boyd et al., 2011).
Ancestral NifB Proteins from Strict Anaerobic and Thermophilic Organisms that Function in Vivo in an Aerobic Mesophilic Host
Stand-alone SAM-radical domain NifB proteins catalyze NifB-co synthesis in vitro (Fay et al., 2015; Wilcoxen et al., 2016). However, they have not yet been proven capable of sustaining diazotrophic growth of M. thermautotrophicus, M. acetivorans, and M. infernus (which also are not yet experimentally confirmed to be diazotrophs). It was also not clear whether this NifB family would function in a mesophilic and aerobic environment, which could prevent their use for plant nitrogenase engineering. Therefore, the Nif+ phenotype exhibited by the A. vinelandii ΔnifB strain complemented with nifBMi presented in this study is convincing evidence of its in vivo functionality in a mesophilic and aerobic bacterium.
As expected, stronger Nif+ phenotype was achieved by complementation with NifBCt. C. tepidum is a mild thermophile with optimum growth temperature of 48°C and therefore much closer to the 30°C optimum of A. vinelandii. In addition, NifBCt has a two-domain NifB architecture similar to NifBAv. Interestingly, NifBCt was a monomer, similar to the archaeal single-domain NifB proteins and different from the NifBAv and NifBKo homodimers. Although constrained by the limited set of available experimental data, it appears that NifB monomers might be more stable and therefore favored in thermophilic organisms regardless of protein architecture. Importantly, both configurations are functional in vivo in a mesophilic host. The strong diazotrophic growth of UW418 in plates compared to liquid medium suggests that there are other factors limiting NifBMi activity A. vinelanii in addition to operational temperature. One possibility is that oxygen limitation during growth in plate has a positive effect on NifBMi that is not observed in liquid medium.
The NifX-like Domain of NifBKo May Have a Role Regulating the Flux of NifB-co during FeMo-Co Biosynthesis
It was suggested that the distinct NifBAv domain architecture (the N-terminal SAM-radical domain and the C-terminal NifX-like domain) could be required to coordinate [Fe–S] cluster precursors prior to catalysis resulting in NifB-co synthesis (Curatti et al., 2006). This possibility was put into question when stand-alone SAM-radical domain archaeal NifB were found active in vitro (Arragain et al., 2014; Fay et al., 2015; Wilcoxen et al., 2016). Here, we demonstrate that the NifX-like domain of NifBKo is not essential for catalytic activity in vivo. A truncated NifBKo lacking the NifX-like domain supported in vivo nitrogenase (ethylene production) rates even higher than full-length NifB. It is thus reasonable to think that NifB catalysis only requires the SAM-radical domain, and that other domains may perform complementary functions that are beneficial but not essential for FeMo-co biosynthesis. A critical role in cofactor biosynthesis for alternative nitrogenases is not likely as this domain is absent in NifB from M. acetivorans, which carries all three types of nitrogenase (Galagan et al., 2002).
Prospects to Implement NifB Activity in Eukaryotes
The successful purification of active NifH from yeast mitochondria, when co-expressed with NifU, NifS and NifM, represented a first advance toward implementing BNF in eukaryotic systems (Lopez-Torrejon et al., 2016). However, major steps are still required to engineer active nitrogenase in a eukaryote. In this regard, expression of functional NifB is expected to be a major barrier to overcome. This is not only because NifB catalyzes a reaction unique and essential to diazotrophs, but also because of the O2-labilility of its [Fe-S] clusters, including NifB-co.
NifB from well-established model organisms, such as A. vinelandii and K. oxytoca, might be difficult to use in the harsh environment provided by a eukaryotic cell. There is evidence that NifB catalysis makes it susceptible to proteolysis (Martinez-Noel et al., 2011). Screening for simpler, but more suitable variants from less “sophisticated” diazotrophs may be a rewarding strategy. In this aspect, the use of less labile, monomeric, and temperature-resistant NifB from Archaea or Bacteria, such as the two examples shown in this study, may help engineering FeMo-co biosynthesis in Eukaryotic (plant) cells. The accompanying paper (Burén et al., 2017) describes the first successful step in this direction.
Materials and Methods
Data Mining and Phylogenetic Analysis
The 390 annotated NifB sequences retrieved from the Structure and Function Linkage Database (SFLD) (Akiva et al., 2014) and UniProt1 are shown in Supplementary Table 1. To exclude potentially faulty annotated sequences, the following filtering procedure was applied to the dataset. First, amino acid sequences of experimentally proven NifB proteins, including A. vinelandii (NifBAv) (Curatti et al., 2006), K. oxytoca (NifBKo) (Zhao et al., 2007), Clostridium pasteurianum (NifBCp) (Chen et al., 2001; Wiig et al., 2011), M. infernus (NifBMi) (Wilcoxen et al., 2016), Methanosarcina acetivorans (NifBMa) (Fay et al., 2015), Methanobacterium thermoautotrophicum (NifBMt) (Fay et al., 2015), and C. tepidum (this work) were aligned to determine conserved motifs. These NifB fingerprint motifs localized in the SAM-radical domain and included an HPC motif, the AdoMet Cx3Cx2C motif, an ExRP motif, an AGPG motif, a TxTxN motif, and a Cx2CRxDAxG motif (Figure 1). The full NifB dataset was then analyzed for the presence of these fingerprints, reducing the initial 390 sequences to 289 (Supplementary Table 1). Protein domain architecture was analyzed using the PFAM database2 (Finn et al., 2016). The frequency of appearance of each one of the different NifB domains in diazotrophic phyla shown in Figure 1 was represented by overlapping data from Supplementary Table 1 with a 3-domain taxonomic tree of life (modified from Boyd and Peters, 2013).
Twenty-eight NifB proteins representing all phylogenetic groups known to contain diazotrophs (Boyd and Peters, 2013) (Supplementary Table 2) were selected from the reduced list and used to investigate taxonomy versus architecture correlation. The taxonomy of diazotrophic groups was resolved using PhyloT3, an online tool that uses the full NCBI taxonomy to generate phylogenetic trees (Figure 2A).
Clustal Omega4 was used to generate protein alignments and neighbor joining (NJ) phylogenetic trees (Sievers et al., 2011). Maximum likehood (ML) trees shown in Figures 2B–E were produced using the IQ-Tree web server5 (Trifinopoulos et al., 2016). Gblocks (Talavera and Castresana, 2007) was used to remove non-conserved aligned segments leaving a 315 contiguous amino acid sequence that was used to generate the SAM-radical domain tree (Figures 2B,C) and a 64 contiguous amino acid sequence used to generate the NifX-like domain trees (Figures 2D,E). Phylogenetic trees shown in Figures 2B–E were resolved using the Interactive Tree of Life online tool6 (Letunic and Bork, 2007) and FigTree.
Plasmids, Strains and Growth Conditions
The strains and plasmids used in this work are listed in Supplementary Table 4. A. vinelandii strains DJ (wild-type) (D.R. Dean, Virginia Tech) and UW140 (ΔnifB) (Hernandez et al., 2007) have been described. K. oxytoca strains UC9 (ΔnifB) and UC16 (ΔnifB, Ptac::gst-nifBKo) (Zhao et al., 2007) have been described.
The M. infernus (nifBMi, accession number D5VRM1) and C. tepidum (nifBCt, accession number. CT1540) nifB sequences were codon-optimized and synthesized by GenScript (Piscataway, NJ, United States) for expression in E. coli. Plasmids pRHB557 and pRHB558 contained the nifBCt and nifBMi genes, respectively, cloned into the NdeI and EcoRI sites of pRHB258 for the expression of His9-tagged proteins under the control of the nifH promoter (Curatti et al., 2007). Plasmids pRHB557 and pRHB558 were inserted into the chromosome of A. vinelandii UW140 (ΔnifB) by homologous recombination at the D-sequence, a 1.1-kb DNA fragment from the chromosomal region downstream of Avin02530 (Hernandez et al., 2008), to generate strains UW422 and UW418, respectively (Figure 3A). Transformants were selected in agar plates of NH4+-free Burk’s modified medium (Shah et al., 1972) containing 50 μg/ml ampicillin.
For diazotrophic growth rate A. vinelandii strains were grown at 30°C on N-free Burk’s medium. When a fixed nitrogen source was required, ammonium acetate was added to a final concentration of 29 mM. Growth was estimated as OD600 using an Ultrospec 3300 Pro spectrophotometer (Amersham). The exponential growth rate constant corresponds to ln2/td, where td represents the doubling time.
For A. vinelandii in vivo nitrogenase activity determinations strains were grown at 30°C on NH4+ supplemented Burk’s medium and then collected, washed and derepressed for nitrogenase as previously described (Shah et al., 1972). Acetylene reduction was determined as described in (Stewart et al., 1967).
Expression plasmid pRHB233 (Ptac::gst-nifBKo) is a derivative of pGEX-4T-3 (GE Healthcare) that contains the entire nifBKo gene (1404 nucleotides encoding a 468 amino-acid polypeptide; UniProt accession number P10390) fused to a gst-encoding gene (Zhao et al., 2007). Plasmid pRHB233 was used as template to amplify a truncated nifBKo variant using oligonucleotides 5′-CCCCATATGACTACTTCCTGCTCCTCTTTTTCTGGCGGC-3′ and 5′-GGGCTCGAGTCAATGATGATGATGATGATGATGATGATGCGCGGGTCGCAATGCTGGCGTGCAG-3′. The resulting 1008 bp fragment, encoding a 336 amino acid NifBKo polypeptide that lacked the C-terminal NifX-like domain (NifBKo-ΔC), was cloned into the NdeI and XhoI sites of pGEX-4T-3 to generate plasmid pRHB554. K. oxytoca UC9 (ΔnifB) strain was transformed with pRHB554 to generate strain UC28 (ΔnifB, Ptac::gst-nifBKo-ΔC). Positive transformants were selected in LC agar plates containing ampicillin (150 μg/ml) and carbenicillin (800 μg/ml).
For diazotrophic growth rate and in vivo nitrogenase activity determinations, K. oxytoca strains were grown overnight at 30°C in minimal medium supplemented with 28.5 μM ammonium acetate (Shah et al., 1994). Cells were washed three times using N-free medium and finally resuspended at a final OD600 value of 0.15 in N-free medium supplemented with 0.1% serine, 150 μg/ml ampicillin, 800 μg/ml carbenicillin, and 5 μM IPTG in dual-sealed 100-ml vials under O2-free conditions. At 3-h intervals during a period of 24 h, culture growth was monitored by OD600 using an Ultrospec 3300 Pro spectrophotometer (Amersham), and the in vivo nitrogenase activity was determined by ethylene production at 30°C for 30 min in 1-ml culture samples at a normalized OD600 value of 1, as previously described (Stewart et al., 1967). The growth rate constant corresponds to ln2/td, where td represents the doubling time.
Purification of NifBCt from A. vinelandii Recombinant Cells
Azotobacter vinelandii UW422 cells overexpressing NifBCt under the control of a nifH promoter were grown in 32-l batches in a 300-l fermenter (Bioprocess Technology). Nitrogenase derepression and cell collection were carried out as described in (Echavarri-Erasun et al., 2014).
Purification of His-NifBCt from A. vinelandii cells was as follows: 150 g of cells were resuspended in 450 ml buffer A (50 μM Na2HPO4, pH 7.6, 4 M glycerol, 5 μM 2-mercaptoethanol and 2 μM Na2S2O4) supplemented with protease inhibitors (200 μM PMSF and 1 μg/ml leupeptin) and 5 μg/ml DNAse inside a Coy Labs glovebox for 30 min. Cells were pelleted at 14,000 × g for 10 min at 4°C and then transferred back inside the glovebox. Pellets were lysed by osmotic shock in 450 ml buffer B (50 μM Na2HPO4, pH 7.6, 0.05% n-dodecyl-β-D-maltoside, 5 μM 2-mercaptoethanol and 2 μM Na2S2O4). A cell-free extract was obtained by collecting the supernatant after centrifugation at 70,000 × g for 1 h at 4°C. The cell-free extract was supplemented with NaCl to a final concentration of 180 μM and loaded onto a 25-ml IMAC column (GE Healthcare) previously charged with Co2+ and equilibrated in buffer C (50 μM Na2HPO4, pH 7.6, 180 μM NaCl, 0.05% n-dodecyl-β-D-maltoside, 5 μM 2-mercaptoethanol, 10% glycerol and 2 μM Na2S2O4) at 4°C. Column was washed with 3 column volumes of buffer C, followed by 7 column volumes of buffer C supplemented with 50 μM imidazole. NifBCt was eluted using buffer C supplemented with 300 μM imidazole. Eluted fractions were analyzed by SDS-PAGE and Coomassie staining. Fractions containing pure NifBCt were pooled and desalted using a HiPrep 26/10 desalting column (GE Healthcare) previously equilibrated with buffer C. Purified NifBCt was stored in liquid N2 as pellets.
Determination of NifBCt Native Molecular Weight
NifBCt Native Molecular Weight was determined by size-exclusion chromatography using a HiLoad 16/600 Superdex 200 column attached to an AKTA FPLC (GE Healthcare). The column was equilibrated with 50 μM Na2HPO4, pH 7.6, 180 μM NaCl, 10% glycerol, 5 μM 2-mercaptoethanol and 2 μM Na2S2O4 and the chromatography was run with the same buffer at a flow rate of 1 ml/min. The column was calibrated for molecular mass determination by using the molecular weight standard proteins aldolase (158 kDa), conalbumin (75 kDa), ovalbumin (44 kDa), and carbonic anhydrase (29 kDa) (GE Healthcare).
NifBCt [Fe–S] Cluster Reconstitution
As isolated NifBCt samples were diluted in 50 μM Tris-HCl (pH 8) buffer containing 200 mM KCl and 10% glycerol to a final concentration of 10 μM NifBCt. Samples were then incubated during 2 h at 37°C with a 12-fold molar excess of Fe2+ [(NH4)2Fe(SO4)2] and S2- (Na2S), in the presence of 10 μM DTT. The Fe and S excess was removed from reconstituted preparations by filtration in a HiPrep 26/10 desalting column (GE Healthcare) equilibrated in dilution buffer. After desalting, Fe content of reconstituted NifBCt samples was quantified as described by Fish (1988).
NifBCt-Dependent in Vitro Synthesis of FeMo-co
Azotobacter vinelandii UW140 (ΔnifB) cell-free extracts were obtained as described above and used for biochemical complementation assays. Purified NifBCt (0.16 μM) was added to reaction mixtures containing 0.2 ml of UW140 cell-free extract (4.4 μg protein/ml) in 22 μM Tris-HCl (pH 7.4), 17.5 μM Na2MoO4, 175 μM R-homocitrate, 400 μM (NH4)2FeSO4, 400 μM Na2S, 880 μM SAM, 1.32 μM ATP, 18 μM phosphocreatine, 2.2 μM MgCl2, 3 μM Na2S2O4, 3.5% glycerol, 40 μg creatine phosphokinase, and 2 μM NifH at a final volume of 400 ml. Control reactions contained 1.4 μM pure NifB-co instead of NifBCt. Reactions were incubated at 30°C for 45 min inside an MBraun glovebox (O2 < 0.1 ppm) to allow for FeMo-co synthesis and insertion into apo-NifDK present in the UW140 extract. Acetylene reduction activity of reconstituted NifDK protein was quantified after addition of 0.1 μg NifH, changing the vial gas phase to 100% argon, and finally injecting 0.5 ml acetylene. Reaction mixtures were incubated in a water bath at 30°C for 15 min and 600 rpm shaking and then stopped by addition of 0.1 ml of 8 M NaOH. Ethylene formation was measured in a Shimadzu GC-2014 gas chromatograph equipped with a Porapak N80/100 column.
Author Contributions
CE-E, SA, EJ-V, and AS carried out experimental work; CE-E, SA, EJ-V, SB, and LR carried out experimental design and data analysis; CE-E, SB, and LR wrote the paper.
Funding
Funding for this research was provided by Bill & Melinda Gates Foundation OPP1143172, ERC Starting Grant 205442, and MINECO BIO2014-59131-R. AS was recipient of FPI Fellowship BES-2010-038322.
Conflict of Interest Statement
The authors declare that the research was conducted in the absence of any commercial or financial relationships that could be construed as a potential conflict of interest.
Acknowledgment
We thank Jose María Buesa for A. vinelandii fermentations.
Supplementary Material
The Supplementary Material for this article can be found online at: https://www.frontiersin.org/articles/10.3389/fpls.2017.01947/full#supplementary-material
Footnotes
- ^http://uniprot.org
- ^http://pfam.xfam.org
- ^http://phylot.biobyte.de/
- ^http://www.ebi.ac.uk/Tools/msa/clustalo/
- ^http://www.iqtree.org
- ^http://itol.embl.de
References
Akiva, E., Brown, S., Almonacid, D. E., Barber, A. E. II, Custer, A. F., Hicks, M. A., et al. (2014). The structure-function linkage database. Nucleic Acids Res. 42, D521–D530. doi: 10.1093/nar/gkt1130
Allen, R. M., Chatterjee, R., Ludden, P. W., and Shah, V. K. (1995). Incorporation of iron and sulfur from NifB cofactor into the iron-molybdenum cofactor of dinitrogenase. J. Biol. Chem. 270, 26890–26896. doi: 10.1074/jbc.270.45.26890
Arragain, S., Scandurra, A. A., Jimenez-Vicente, E., Echavarri-Erasun, C., and Rubio, L. M. (2014). “Methanocaldococcus infernus NifB: a new model to study NifB-co formation,” in Proceedings of the XI European Nitrogen Fixation Conference, Tenerife.
Bishop, P. E., and Joerger, R. D. (1990). Genetics and molecular biology of alternative nitrogen fixation systems. Annu. Rev. Plant Physiol. Plant Mol. Biol. 41, 109–125. doi: 10.1146/annurev.pp.41.060190.000545
Boyd, E. S., Anbar, A. D., Miller, S., Hamilton, T. L., Lavin, M., and Peters, J. W. (2011). A late methanogen origin for molybdenum-dependent nitrogenase. Geobiology 9, 221–232. doi: 10.1111/j.1472-4669.2011.00278.x
Boyd, E. S., Costas, A. M., Hamilton, T. L., Mus, F., and Peters, J. W. (2015). Evolution of molybdenum nitrogenase during the transition from anaerobic to aerobic metabolism. J. Bacteriol. 197, 1690–1699. doi: 10.1128/JB.02611-14
Boyd, E. S., and Peters, J. W. (2013). New insights into the evolutionary history of biological nitrogen fixation. Front. Microbiol. 4:201. doi: 10.3389/fmicb.2013.00201
Bulen, W. A., and Lecomte, J. R. (1966). The nitrogenase system from Azotobacter: two enzyme requirements for N2 reduction, ATP dependent H2 evolution and ATP hydrolysis. Proc. Natl. Acad. Sci. U.S.A. 56, 979–986. doi: 10.1073/pnas.56.3.979
Burris, R. H., and Roberts, G. P. (1993). Biological nitrogen fixation. Annu. Rev. Nutr. 13, 317–335. doi: 10.1146/annurev.nu.13.070193.001533
Burén, S., Jiang, X., López-Torrejón, G., Echavarri-Erasun, C., and Rubio, L. M. (2017). Purification and in vitro activity of mitochondria targeted nitrogenase cofactor maturase NifB. Front. Plant Sci. 8:1567. doi: 10.3389/fpls.2017.01567
Canfield, D. E., Glazer, A. N., and Falkowski, P. G. (2010). The evolution and future of Earth’s nitrogen cycle. Science 330, 192–196. doi: 10.1126/science.1186120
Chen, J. S., Toth, J., and Kasap, M. (2001). Nitrogen-fixation genes and nitrogenase activity in Clostridium acetobutylicum and Clostridium beijerinckii. J. Ind. Microbiol. Biotechnol. 27, 281–286. doi: 10.1038/sj.jim.7000083
Curatti, L., Hernandez, J. A., Igarashi, R. Y., Soboh, B., Zhao, D., and Rubio, L. M. (2007). In vitro synthesis of the iron-molybdenum cofactor of nitrogenase from iron, sulfur, molybdenum, and homocitrate using purified proteins. Proc. Natl. Acad. Sci. U.S.A. 104, 17626–17631. doi: 10.1073/pnas.0703050104
Curatti, L., Ludden, P. W., and Rubio, L. M. (2006). NifB-dependent in vitro synthesis of the iron-molybdenum cofactor of nitrogenase. Proc. Natl. Acad. Sci. U.S.A. 103, 5297–5301. doi: 10.1073/pnas.0601115103
Dos Santos, P. C., Fang, Z., Mason, S. W., Setubal, J. C., and Dixon, R. (2012). Distribution of nitrogen fixation and nitrogenase-like sequences amongst microbial genomes. BMC Genomics 13:162. doi: 10.1186/1471-2164-13-162
Eady, R. R. (1996). Structure-function relationships of alternative nitrogenases. Chem. Rev. 96, 3013–3030. doi: 10.1021/cr950057h
Echavarri-Erasun, C., Arragain, S., Scandurra, A. A., and Rubio, L. M. (2014). Expression and purification of NifB proteins from aerobic and anaerobic sources. Methods Mol. Biol. 1122, 19–31. doi: 10.1007/978-1-62703-794-5_3
Einsle, O., Tezcan, F. A., Andrade, S. L., Schmid, B., Yoshida, M., Howard, J. B., et al. (2002). Nitrogenase MoFe-protein at 1.16 Å resolution: a central ligand in the FeMo-cofactor. Science 297, 1696–1700. doi: 10.1126/science.1073877
Eisen, J. A., Nelson, K. E., Paulsen, I. T., Heidelberg, J. F., Wu, M., Dodson, R. J., et al. (2002). The complete genome sequence of Chlorobium tepidum TLS, a photosynthetic, anaerobic, green-sulfur bacterium. Proc. Natl. Acad. Sci. U.S.A. 99, 9509–9514. doi: 10.1073/pnas.132181499
Fay, A. W., Wiig, J. A., Lee, C. C., and Hu, Y. (2015). Identification and characterization of functional homologs of nitrogenase cofactor biosynthesis protein NifB from methanogens. Proc. Natl. Acad. Sci. U.S.A. 112, 14829–14833. doi: 10.1073/pnas.1510409112
Finn, R. D., Coggill, P., Eberhardt, R. Y., Eddy, S. R., Mistry, J., Mitchell, A. L., et al. (2016). The Pfam protein families database: towards a more sustainable future. Nucleic Acids Res. 44, D279–D285. doi: 10.1093/nar/gkv1344
Fish, W. W. (1988). Rapid colorimetric micromethod for the quantitation of complexed iron in biological samples. Methods Enzymol. 158, 357–364. doi: 10.1016/0076-6879(88)58067-9
Galagan, J. E., Nusbaum, C., Roy, A., Endrizzi, M. G., Macdonald, P., Fitzhugh, W., et al. (2002). The genome of M. acetivorans reveals extensive metabolic and physiological diversity. Genome Res. 12, 532–542. doi: 10.1101/gr.223902
George, S. J., Igarashi, R. Y., Xiao, Y., Hernandez, J. A., Demuez, M., Zhao, D., et al. (2008). Extended X-ray absorption fine structure and nuclear resonance vibrational spectroscopy reveal that NifB-co, a FeMo-co precursor, comprises a 6Fe core with an interstitial light atom. J. Am. Chem. Soc. 130, 5673–5680. doi: 10.1021/ja0755358
Georgiadis, M. M., Komiya, H., Chakrabarti, P., Woo, D., Kornuc, J. J., and Rees, D. C. (1992). Crystallographic structure of the nitrogenase iron protein from Azotobacter vinelandii. Science 257, 1653–1659. doi: 10.1126/science.1529353
Guo, Y., Echavarri-Erasun, C., Demuez, M., Jimenez-Vicente, E., Bominaar, E. L., and Rubio, L. M. (2016). The nitrogenase FeMo-cofactor precursor formed by NifB is a diamagnetic 8 iron-containing cluster. Angew. Chem. Int. Ed. Engl. 55, 12764–12767. doi: 10.1002/anie.201606447
Hernandez, J. A., Curatti, L., Aznar, C. P., Perova, Z., Britt, R. D., and Rubio, L. M. (2008). Metal trafficking for nitrogen fixation: NifQ donates molybdenum to NifEN/NifH for the biosynthesis of the nitrogenase FeMo-cofactor. Proc. Natl. Acad. Sci. U.S.A. 105, 11679–11684. doi: 10.1073/pnas.0803576105
Hernandez, J. A., Igarashi, R. Y., Soboh, B., Curatti, L., Dean, D. R., Ludden, P. W., et al. (2007). NifX and NifEN exchange NifB cofactor and the VK-cluster, a newly isolated intermediate of the iron-molybdenum cofactor biosynthetic pathway. Mol. Microbiol. 63, 177–192. doi: 10.1111/j.1365-2958.2006.05514.x
Hoffman, B. M., Lukoyanov, D., Yang, Z. Y., Dean, D. R., and Seefeldt, L. C. (2014). Mechanism of nitrogen fixation by nitrogenase: the next stage. Chem. Rev. 114, 4041–4062. doi: 10.1021/cr400641x
Jeanthon, C., L’Haridon, S., Reysenbach, A. L., Vernet, M., Messner, P., Sleytr, U. B., et al. (1998). Methanococcus infernus sp. nov., a novel hyperthermophilic lithotrophic methanogen isolated from a deep-sea hydrothermal vent. Int. J. Syst. Bacteriol. 48, 913–919. doi: 10.1099/00207713-48-3-913
Joerger, R. D., and Bishop, P. E. (1988). Nucleotide sequence and genetic analysis of the nifB-nifQ region from Azotobacter vinelandii. J. Bacteriol. 170, 1475–1487. doi: 10.1128/jb.170.4.1475-1487.1988
Letunic, I., and Bork, P. (2007). Interactive tree of life (iTOL): an online tool for phylogenetic tree display and annotation. Bioinformatics 23, 127–128. doi: 10.1093/bioinformatics/btl529
Lopez-Torrejon, G., Jimenez-Vicente, E., Buesa, J. M., Hernandez, J. A., Verma, H. K., and Rubio, L. M. (2016). Expression of a functional oxygen-labile nitrogenase component in the mitochondrial matrix of aerobically grown yeast. Nat. Commun. 7:11426. doi: 10.1038/ncomms11426
Martinez-Noel, G., Curatti, L., Hernandez, J. A., and Rubio, L. M. (2011). NifB and NifEN protein levels are regulated by ClpX2 under nitrogen fixation conditions in Azotobacter vinelandii. Mol. Microbiol. 79, 1182–1193. doi: 10.1111/j.1365-2958.2011.07540.x
Mcglynn, S. E., Boyd, E. S., Peters, J. W., and Orphan, V. J. (2013). Classifying the metal dependence of uncharacterized nitrogenases. Front. Microbiol. 3:419. doi: 10.3389/fmicb.2012.00419
Peters, J. W., Boyd, E. S., Hamilton, T. L., and Rubio, L. M. (2011). “Biochemistry of Mo-nitrogenase,” in Nitrogen Cycling in Bacteria: Molecular Analysis, ed. J. W. B. Moir (Norfolk, VA: Caister Academic Press).
Raymond, J., Siefert, J. L., Staples, C. R., and Blankenship, R. E. (2004). The natural history of nitrogen fixation. Mol. Biol. Evol. 21, 541–554. doi: 10.1093/molbev/msh047
Shah, V. K., Allen, J. R., Spangler, N. J., and Ludden, P. W. (1994). In vitro synthesis of the iron-molybdenum cofactor of nitrogenase. Purification and characterization of NifB cofactor, the product of NIFB protein. J. Biol. Chem. 269, 1154–1158.
Shah, V. K., Davis, L. C., and Brill, W. J. (1972). Nitrogenase. I. Repression and derepression of the iron-molybdenum and iron proteins of nitrogenase in Azotobacter vinelandii. Biochim. Biophys. Acta 256, 498–511. doi: 10.1016/0005-2728(72)90078-3
Sievers, F., Wilm, A., Dineen, D., Gibson, T. J., Karplus, K., Li, W., et al. (2011). Fast, scalable generation of high-quality protein multiple sequence alignments using Clustal Omega. Mol. Syst. Biol. 7, 539. doi: 10.1038/msb.2011.75
Soboh, B., Boyd, E. S., Zhao, D., Peters, J. W., and Rubio, L. M. (2010). Substrate specificity and evolutionary implications of a NifDK enzyme carrying NifB-co at its active site. FEBS Lett. 584, 1487–1492. doi: 10.1016/j.febslet.2010.02.064
Spatzal, T., Aksoyoglu, M., Zhang, L., Andrade, S. L., Schleicher, E., Weber, S., et al. (2011). Evidence for interstitial carbon in nitrogenase FeMo cofactor. Science 334, 940. doi: 10.1126/science.1214025
Stewart, W. D., Fitzgerald, G. P., and Burris, R. H. (1967). In situ studies on N2 fixation using the acetylene reduction technique. Proc. Natl. Acad. Sci. U.S.A. 58, 2071–2078. doi: 10.1073/pnas.58.5.2071
Talavera, G., and Castresana, J. (2007). Improvement of phylogenies after removing divergent and ambiguously aligned blocks from protein sequence alignments. Syst. Biol. 56, 564–577. doi: 10.1080/10635150701472164
Trifinopoulos, J., Nguyen, L. T., Von Haeseler, A., and Minh, B. Q. (2016). W-IQ-TREE: a fast online phylogenetic tool for maximum likelihood analysis. Nucleic Acids Res. 44, W232–W235. doi: 10.1093/nar/gkw256
Wahlund, T. M., and Madigan, M. T. (1993). Nitrogen fixation by the thermophilic green sulfur bacterium Chlorobium tepidum. J. Bacteriol. 175, 474–478. doi: 10.1128/jb.175.2.474-478.1993
Wiig, J. A., Hu, Y., Lee, C. C., and Ribbe, M. W. (2012). Radical SAM-dependent carbon insertion into the nitrogenase M-cluster. Science 337, 1672–1675. doi: 10.1126/science.1224603
Wiig, J. A., Hu, Y., and Ribbe, M. W. (2011). NifEN-B complex of Azotobacter vinelandii is fully functional in nitrogenase FeMo cofactor assembly. Proc. Natl. Acad. Sci. U.S.A. 108, 8623–8627. doi: 10.1073/pnas.1102773108
Wilcoxen, J., Arragain, S., Scandurra, A. A., Jimenez-Vicente, E., Echavarri-Erasun, C., Pollmann, S., et al. (2016). Electron paramagnetic resonance characterization of three iron-sulfur clusters present in the nitrogenase cofactor maturase NifB from Methanocaldococcus infernus. J. Am. Chem. Soc. 138, 7468–7471. doi: 10.1021/jacs.6b03329
Keywords: nitrogenase, iron-molybdenum cofactor, SAM-radical, nitrogen fixation, Azotobacter, methanogens
Citation: Arragain S, Jiménez-Vicente E, Scandurra AA, Burén S, Rubio LM and Echavarri-Erasun C (2017) Diversity and Functional Analysis of the FeMo-Cofactor Maturase NifB. Front. Plant Sci. 8:1947. doi: 10.3389/fpls.2017.01947
Received: 31 May 2017; Accepted: 30 October 2017;
Published: 14 November 2017.
Edited by:
Nikolai Provorov, All-Russian Research Institute of Agricultural Microbiology of the Russian Academy of Agricultural Sciences, RussiaReviewed by:
Oswaldo Valdes-Lopez, Universidad Nacional Autónoma de México, MexicoTeresa Thiel, University of Missouri–St. Louis, United States
Copyright © 2017 Arragain, Jiménez-Vicente, Scandurra, Burén, Rubio and Echavarri-Erasun. This is an open-access article distributed under the terms of the Creative Commons Attribution License (CC BY). The use, distribution or reproduction in other forums is permitted, provided the original author(s) or licensor are credited and that the original publication in this journal is cited, in accordance with accepted academic practice. No use, distribution or reproduction is permitted which does not comply with these terms.
*Correspondence: Carlos Echavarri-Erasun, Y2FybG9zLmVjaGF2YXJyaUB1cG0uZXM= Luis M. Rubio, bG0ucnViaW9AdXBtLmVz
†Present address: Simon Arragain, Department of Chemistry, University of California, Davis, Davis, CA, United States; Emilio Jiménez-Vicente, Department of Biochemistry, Virginia Tech, Blacksburg, VA, United States; Alessandro A. Scandurra, Merck, Cambridge, United Kingdom
‡These authors have contributed equally to this work.