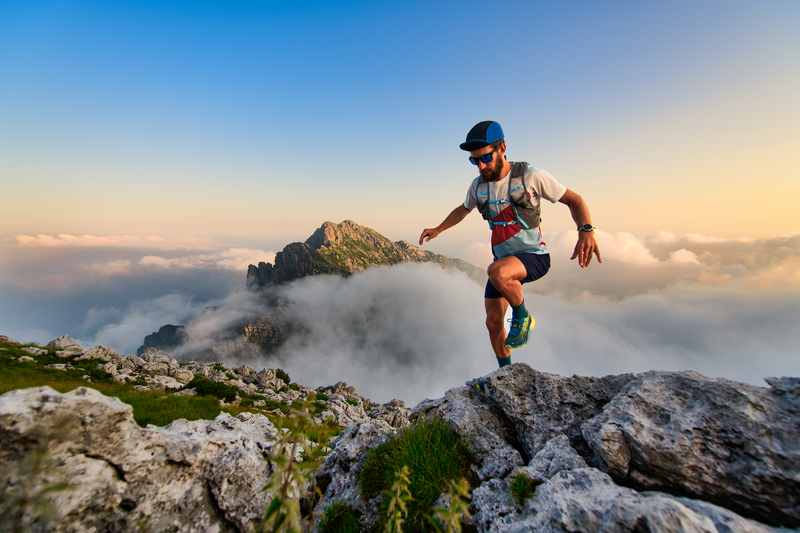
95% of researchers rate our articles as excellent or good
Learn more about the work of our research integrity team to safeguard the quality of each article we publish.
Find out more
ORIGINAL RESEARCH article
Front. Plant Sci. , 25 October 2017
Sec. Plant Development and EvoDevo
Volume 8 - 2017 | https://doi.org/10.3389/fpls.2017.01812
BIG GRAIN1 (BG1) is an auxin-regulated gene which functions in auxin pathway and positively regulates biomass, grain size and yield in rice. However, the evolutionary origin and divergence of these genes are still unknown. In this study, we found that BG genes are probably originated in seed plants. We also identified that seed plants evolved a class of BIG GRAIN LIKE (BGL) genes which share conserved middle and C-terminal motifs with BG. The BG genes were present in all monocot and eudicot species analyzed; however, the BGL genes were absent in few monocot lineages. Both BG and BGL were found to be serine-rich proteins; however, differences in expansion and rates of retention after whole genome duplication events were observed. Promoters of BG and BGL genes were found to be enriched with auxin-responsive elements and the Arabidopsis thaliana BG and BGL genes were found to be auxin-regulated. The auxin-induced expression of AthBG2 was found to be dependent on the conserved ARF17/19 module. Protein-protein interaction analysis identified that AthBG2 interact with regulators of splicing, transcription and chromatin remodeling. Taken together, this study provides interesting insights about BG and BGL genes and incentivizes future work in this gene family which has the potential to be used for crop manipulation.
In seed crops like rice, the grain size is an important agronomic trait which determines yield. Studies identified many important determinants of grain size in rice which has the potential to be used for crop manipulation (Zuo and Li, 2014). The BIG GRAIN 1 (OsaBG1) has been recently identified as a positive regulator of grain size in rice. Interestingly, the constitutive expression of OsaBG1 in Arabidopsis thaliana also resulted in an increase in seed size (Liu et al., 2015).
Apart from increase in seed size, enhanced expression of BG1 in rice and A. thaliana resulted in an increase in biomass suggesting that this gene is a general promoter of growth (Liu et al., 2015). OsaBG1 is a primary auxin response gene and increased expression resulted in enhanced auxin sensitivity suggesting that this gene is involved in auxin signaling. OsaBG1 is predominantly localized in the plasma membrane and overexpression lines showed enhanced auxin levels and basipetal transport suggesting that this gene might be involved in the regulation of auxin transport. These results suggest that OsaBG1 is involved in enhancing biomass and grain size through regulating auxin pathway in rice (Liu et al., 2015). Different studies have already implicated auxin signaling in regulating biomass and seed size in both monocots and eudicots. The loss of AUXIN RESPONSIVE FACTOR 2 of A. thaliana results in an increase in seed size due to enhanced cell proliferation while ectopic expression of AUXIN RESPONSIVE FACTOR 19 (ARF19) from Jatropha curcas promotes seed size and yield (Schruff et al., 2005; Sun et al., 2017). Decreased synthesis of auxin in Pisum sativum resulted in small and wrinkled seeds with reduced starch content (McAdam et al., 2017). In rice, the NARROW LEAF1, an inhibitor of polar auxin transport was found to be a suitable candidate gene for crop engineering for better yield (Qi et al., 2008; Fujita et al., 2013; Takai et al., 2013; Zhang et al., 2014).
In field trials, the OsaBG1 overexpressing transgenic lines showed a significant increase in yield suggesting that BG genes are ideal candidates for crop improvement (Liu et al., 2015). The BG homologs were found to be present in eudicots. However, except the single study in rice, so far, no attempt has been made to study the BG genes in eudicots or any other monocot species. The enhanced biomass and bigger seed phenotype of OsaBG1-expressing A. thaliana lines suggest that the BG-dependent pathway of growth regulation might be conserved in all angiosperms (Liu et al., 2015). Many important seed crops such as legumes belong to eudicotyledonae and the potential of BG genes in crop improvement makes them ideal candidates to be studied in eudicots. Although BG genes were identified from selected monocots and eudicots, the origin and evolution of these genes in plants are yet to be identified.
In this study, we made use of the availability of a large number of sequenced genomes in the plant lineage to trace the evolutionary origin and evolution of BG genes. The comprehensive phylogenetic analysis suggest that BG genes are originated in seed plants. We also identified a group of BIG GRAIN LIKE (BGL) genes, which show sequence similarity with BG in some motifs and seems to be originated along with BG genes. Detailed phylogenetic analysis identified difference in the rate of retention and expansion among BG and BGL genes. Expression analysis of A. thaliana BG and BGL genes in different developmental stages was done and the spatiotemporal expression dynamics of these genes were identified. Further, the promoter analysis and auxin-dependent expression analysis of A. thaliana BG and BGL genes suggest the conservation of auxin-dependent transcriptional control of BG and BGL genes. Finally, we identified that AthBG2, a member of A. thaliana BG family; interacts with conserved regulators of splicing, transcription and chromatin remodeling. This comprehensive analysis of BG and BGL genes in plants can serve as a springboard for future work in this agronomically important class of genes.
The BG and BGL proteins from different sequenced plant genomes were identified by BLASTP, iterated BLAST and profile-HMM based searches in various databases. The remaining BG proteins from Oryza sativa were identified from MSU Rice Genome Annotation Project Database using OsaBG1 as the query with default parameters (BLASTP, e-value: 1e-5) (Ouyang et al., 2007). The BG and BGL proteins from Amborella trichopoda were identified from Amborella Genome Database using OsaBG1 as the query (BLASTP, e-value: 1e-5) (Albert et al., 2013). The BG and BGL proteins from the remaining species were identified using AtrBG1 or AtrBGL1 as the query in BLASTP. The proteins from Picea abies were identified from ConGenIE (BLASTP, e-value: 1e-3) (Sundell et al., 2015). The BG and BGL proteins from Nelumbo nucifera and Phoenix dactylifera were identified from NCBI RefSeq database (BLASTP, e-value: 10) (Pruitt et al., 2007). The proteins from Lotus japonicus were identified from Lotus Base (BLASTP, e-value: 1e-5) (Mun et al., 2016). The proteins from the remaining species were identified from Phytozome v12 (BLASTP, e-value: -1) (Goodstein et al., 2012). The retrieved sequences were manually curated to remove repeats and partial sequences. These sequences were aligned and used for profile-HMM based search in hmmsearch available in HMMER web server v2.15.0 with default parameters in the respective reference proteomes (significance e-value: 0.01 for sequence and 0.03 for hits). Further, three rounds of iterated BLAST search was performed using the aligned sequences in different reference proteomes using jackhmmer available in HMMER web server v2.15.0 with default parameters (significance e-value: 0.01 for sequence and 0.03 for hits) (Finn et al., 2015). Finally, to identify the true BG/BGL hits, the hits obtained from profile-HMM and iterative searches were subjected to BLAST search in A. thaliana and/or O. sativa proteomes. The final set of verified protein sequences used for phylogenetic analysis is given in Supplementary Table S1. The corresponding CDS sequences were retrieved from the respective databases using the protein identifiers. The final set of CDS sequences used for phylogenetic analysis is given in Supplementary Table S2.
Presence of characterized domains in BG and BGL proteins was analyzed by batch CD search tool against CDD v3.16 using the default parameters (e-value: 0.01) (Marchler-Bauer et al., 2015). The conserved motifs of BG and BGL proteins were identified from analyzing the aligned sequences of BG and BGL proteins from eudicots and monocots individually in Jalview (Waterhouse et al., 2009). The sequences of conserved motifs were exported from Jalview and used for HMM logo construction in Skylign (Wheeler et al., 2014). The fold recognition, topology and disorder prediction analysis was done using Phyre v2.0 with default parameters (Kelley et al., 2015).
The phylogenetic trees were reconstructed based on Bayesian (MrBayes v3) and maximum likelihood (PhyML) estimation methods in TOPALI v2.5 (Milne et al., 2009). The protein and CDS sequences were aligned by ClustalX v2.1 (Larkin et al., 2007). The aligned file was used to identify the best-suited model of amino acid and DNA substitution in TOPALI v2.5 (Milne et al., 2009). JTT + I + G model was used for reconstruction of all protein-based phylogenetic trees (Jones et al., 1992). GTR + I + G model was used for the reconstruction of all DNA-based phylogenetic trees (Tavaré, 1986). For Bayesian phylogeny, two Metropolis-Coupled Markov Chain Monte Carlo runs with 16 chains were performed with the given settings [sample frequency: 10; burn in (%): 25] for 100,000 generations unless specified. The protein-based phylogenetic tree of eudicots was run for 500,000 generations to attain convergence. The protein-based phylogenetic tree of eudicots was run for 300,000 generations to attain convergence. The CDS-based phylogenetic tree of eudicots and monocots was run for 1,000,000 generations to attain convergence. To verify the convergence of the Bayesian phylogenetic reconstruction, potential scale reduction factor was confirmed to reach 1.0 as runs converged in MrBayes v3 (Ronquist et al., 2012). The maximum likelihood trees were constructed with 1000 bootstrap replicates. The species phylogenetic tree was obtained from NCBI Taxonomy Common Tree tool. The phylogenetic trees were edited and visualized with midpoint rooting in FigTree v1.4.3 (Rambout, 2016). The alignment files used for phylogenetic analysis and tree output obtained are deposited in Figshare1.
The PAL2NAL program was used for Ka, Ks and Ka/Ks calculations (Suyama et al., 2006). The Ka/Ks ratios of paralogous and orthologous genes identified from the phylogenetic reconstruction were calculated.
The AtGenExpress expression data of A. thaliana BG and BGL genes in different developmental stages and auxin treatments were retrieved from Arabidopsis eFP browser (Schmid et al., 2005; Winter et al., 2007; Goda et al., 2008). The response of BG and BGL genes towards auxin treatments were calculated from this data. The values were plotted as heat maps using MultiExperiment Viewer (MeV, v4.8) (Saeed et al., 2006). For the qRT-PCR analysis of AthBG2 in different developmental stages, the tissue and developmental stage-specific samples previously prepared in the lab were used (Jamsheer et al., 2015). The cDNA was prepared from 2 μg of total RNA using High-Capacity cDNA Reverse Transcription kit (Applied Biosystems, United States). The 1:20 diluted cDNA samples were used for qRT-PCR reaction in 7500 Fast Real-Time PCR System using SYBR-Green chemistry as per the manufacture’s protocol (Applied Biosystems, United States). The qRT-PCR primers of AthBG2 and the endogenous reference UBQ10 were designed from the transcript region using Primer Express v3.0 (Applied Biosystems, United States). The primers used for qRT-PCR are given in Supplementary Table S4. The relative quantification was performed using ΔΔCT method (Livak and Schmittgen, 2001).
For the promoter analysis, 1kb upstream sequences from the TSS of A. trichopoda, A. thaliana and O. sativa BG and BGL genes were retrieved from Phytozome v12 (Goodstein et al., 2012) (Supplementary Table S3). The presence of different auxin responsive elements reported in the literature (TGTCNN, NNGACA, ACTTTA, CACGCAAT, TGACG, and KGTCCCAT) was analyzed and plotted according to the scale. For the expression analysis of AthBG2 toward auxin treatment in Col-0, 5 days old seedling grown under standard growth conditions (16:8 h photoperiod, 60 μmol m-2 s-1 light and 22°C ± 2°C temperature) were subjected to 1 μM IAA treatment in 0.5X liquid MS medium for different time points. For gene expression comparison between WT and arf7arf19 mutant, 7 days old seedlings grown under standard growth conditions were subjected to 1 μM IAA treatment in 0.5X liquid MS medium for different time points. The tissue was harvested at the end of time points in liquid nitrogen and stored in -80°C. RNA isolation and cDNA preparation from this tissue were performed as described previously (Mishra et al., 2009). The qRT-PCR experiment and calculation were performed as described above. The seeds of arf7arf19 were obtained from ABRC (CS24625) (Okushima et al., 2005).
For the promoter: GUS line construction of AthBG2, 2 kb upstream region from the TSS was cloned in pENTR/D-TOPO using the primer given in Supplementary Table S4 and mobilized to pMDC164 (Curtis and Grossniklaus, 2003) vector using Gateway technology (Invitrogen, Carlsbad, CA, United States). The pAthBG2:: GUSA construct was transformed in Col-0 grown in standard growth conditions using floral dip method (Clough and Bent, 1998). The transformants were identified by screening the seeds in 0.5X MS medium with 15 μg/ml hygromycin A and homozygous lines were identified in the third generation. For GUS assay, the homozygous lines were grown under the standard growth conditions. The tissues were harvested and GUS assay was performed as described previously (Jefferson et al., 1987).
The AthBG2 CDS cloned in pGBKT7 (BD vector) and transformed into Y2H gold strain was used as the bait to screen the normalized Mate & Plate Universal Arabidopsis Y2H cDNA library as per the manufactures’ protocol (Clontech, Mountain View, CA, United States). The primers used for cloning are given in Supplementary Table S4. Before the Y2H screening, the construct was tested for auto activation and toxicity respectively on SD/–Leu/X-α-Gal/AbA (SDO/X/A) and SD/–Leu (SDO) plates. After identifying that the construct lacked auto activation and toxicity properties, it was used for Y2H library screening. The colonies obtained from SD/–Leu/–Trp/X-α-Gal/AbA (DDO/X/A) after Y2H library screening were further screened on SD/–Ade/–His/–Leu/–Trp/X-α-Gal/AbA (QDO/X/A) medium. From the colonies survived on QDO/X/A medium, prey plasmids (pGADT7; AD vector) were isolated and sequenced to identify the interacting protein. The interaction was further confirmed by co-transforming bait and recovered prey plasmids in Y2H gold strain and screening on QDO/X/A medium. A negative control experiment was conducted along with this experiment using bait vector and prey plasmids to identify the false interactors.
For the BiFC experiments, AthBG2 and Y14 CDS were cloned into pENTR/D-TOPO vector and mobilized respectively to pSAT4A-DEST-n(1-174)EYFP-N1 and pSAT5-DEST-c(175-END)EYFP-C1 (Tzfira et al., 2005) vectors using Gateway technology (Invitrogen, Carlsbad, CA, United States). Primers used for cloning are given in Supplementary Table S4. The clones were cotransformed in onion peel using PDS-1000 Helios Gene Gun (Biorad). The transformed onion peels were incubated at 22°C in darkness for 24 h and fluorescence was analyzed in TCS SP2 (AOBS) laser confocal scanning microscope after the incubation (Leica Microsystems). Negative control experiments were conducted along with this experiment.
For the subcellular localization of AthBG2, the CDS cloned into pENTR/D-TOPO vector was mobilized to pEG104 (Earley et al., 2006) vector using Gateway technology (Invitrogen, Carlsbad, CA, United States). The vector and YFP-CDS construct were transformed individually in onion peels and fluorescence was analyzed as described above.
A combination of BLAST and HMM-based methods were employed to identify BG proteins from the selected sequenced viridiplantae members. Homology search in some of the sequenced chlorophyte genomes (Chlamydomonas reinhardtii, Ostreococcus lucimarinus, Volvox carteri, and Micromonas pusilla) could not identify any homologs of OsaBG1. We also searched for the BG proteins in Klebsormidium flaccidum, which belongs to Charophyta and shows prototypes of signaling pathways required for terrestrial life (Hori et al., 2014). However, we couldn’t retrieve any homologs of OsaBG1 from this genome either. Further, iterative searches also failed to identify any hits of BG proteins in the algal genomes we analyzed, indicating that BG proteins are absent in algae.
We next analyzed the presence of BG1 homologs in embryophytes. The searches in Bryophyta (Marchantia polymorpha, Physcomitrella patens and Sphagnum fallax) and Pteridophyta (Selaginella moellendorffii) could not identify any hits of BG1. Interestingly, 9 hits of BG proteins were identified from the gymnosperm, Picea abies (Figure 1). Further, a hit of BG1 was identified from A. trichopoda, which is the only living species belonging to the sister lineage of angiosperms (Albert et al., 2013). In both P. abies and A. trichopoda, BLAST search identified another protein which has similarity with the BG proteins in the middle and C-terminal regions (Figure 1, Supplementary Figure S1). We named these proteins as BIG GRAIN LIKE (BGL). Consistent with the sequence divergence observed among BG and BGL proteins, in the Bayesian phylogenetic interference of A. trichopoda and P. abies BG and BGL proteins, they recovered to distinct clades (Supplementary Figure S2). Relatively large number of BG proteins in P. abies indicates the possible contribution of whole genome duplications (WGD) in the expansion of BG genes in spermatophytes. In the plant lineage, there is evidence for an ancient WGD event (ζ) shared by seed plants and another WGD event (𝜀) common to angiosperms (Jiao et al., 2011). The absence of lineage-specific WGD in the Amborella lineage and the occurrence of Pinaceae-specific WGD event can be attributed to the increase in the number of BG genes in P. abies (Albert et al., 2013; Li Z. et al., 2015).
FIGURE 1. The distribution of BG and BGL genes in different plant genomes. The numbers represent the distribution of BG and BGL genes in each species. The asterisks indicates the WGD events in the plant lineages. The green and red asterisks indicate genome duplication and triplication respectively.
To dissect the evolutionary history of BG and BGL genes in angiosperms, we identified the BG and BGL genes from 17 eudicots and 9 monocots which represent important taxonomical positions (Figure 1). In eudicots, BG and BGL genes were found to be present in all species analyzed. Among the early diverging eudicots species analyzed, Aquilegia coerulea was found to have single BG and BGL genes each while their number was found to be increased to 2 in Nelumbo nucifera. Interestingly, both these genomes underwent lineage-specific WGD (Ming et al., 2013; Tiley et al., 2016). Further, the number of BG genes in majority of eudicot genomes without independent WGD (Vitis vinifera, Fragaria vesca, Ricinus communis, Carica papaya and Theobroma cacao) (Jaillon et al., 2007; Ming et al., 2008; Chan et al., 2010; Argout et al., 2011; Shulaev et al., 2011) events were found to be increased to 3 suggesting that the core eudicot genome triplication event contributed to this expansion (Jiao et al., 2012). However, in these species, the number of BGL genes remained 1 as in A. trichopoda. An expansion in the number of BG genes was observed in Mimulus guttatus, which can be attributed to the Lamiales-specific WGD event (Edger et al., 2016). Even in this species, only modest increase in the number of BGL genes was observed (Figure 1). These results indicate the difference in the rate of retention of BG and BGL genes after WGD events.
Legumes share an ancient papilionoid-specific WGD event that occurred ∼ 54 Mya (Pfeil et al., 2005; Bertioli et al., 2009). Apart from this ancestral WGD, Glycine max underwent an independent WGD event ∼ 13 Mya (Schmutz et al., 2010). Consistent with this, the legumes without independent WGD (Lotus japonicus and Medicago truncatula) (Sato et al., 2008; Young et al., 2011) possess 4 BG genes and 1 BGL gene each while G. max possesses 8 BG genes and 2 BGL genes (Figure 1). A similar increase in the number of BG and BGL genes was also observed in other species with independent WGD (Malus domestica, Manihot esculenta, Populus trichocarpa and Brassica rapa) (Tuskan et al., 2006; Velasco et al., 2010; Wang et al., 2011; Bredeson et al., 2016) events. The modest increase in the number of BG and BGL genes in C. rubella and A. thaliana could be due to the β and α duplications in crucifers (Bowers et al., 2003; Panchy et al., 2016).
In monocots, among the species analyzed, we could not identify BGL proteins from Zostera marina and Brachypodium distachyon even after repeated searches using various strategies (Figure 1). However, BGL proteins were found to be present in all other monocot species. The available evidence suggests that the monocot-specific τ duplication occurred after the divergence of Alismatales from other monocots (Jiao et al., 2014; Ming et al., 2015). The modest increase in the number of BG genes in species belonging to Alismatales (Zostera marina and Spirodela polyrhiza) can be attributed to the lineage-specific WGD event(s) (Wang et al., 2014; Olsen et al., 2016). The σ WGD shared by Poales and ρ WGD common to grasses might have contributed to the expansion of BG genes in Ananas comosus, Zea mays, Sorghum bicolor, Brachypodium Distachyon, and Oryza sativa (Paterson et al., 2004; Tang et al., 2010; Ming et al., 2015). As observed in eudicots, in the monocots with independent WGD (P. dactylifera, Musa acuminata and Z. mays) (Schnable et al., 2009; D’Hont et al., 2012; Al-Mssallem et al., 2013) event(s), the number of BG genes are further increased. However, except in M. acuminata, not much increase in the number of BGL genes was observed indicating the difference in the rate of retention in BG and BGL genes in monocots also.
The preliminary analysis of representative BG proteins from eudicots and monocots suggest that this is a novel class of protein with no known protein domains (Liu et al., 2015). To find out whether the BG and BGL proteins acquired any known domains, we analyzed all 124 BG and 40 BGL proteins identified from different species for the presence of known protein domains. Almost in all species we analyzed, the size of BGL proteins was found to be smaller than BG (Supplementary Figure S3). Our analysis revealed that except two BG proteins from Z. mays and one protein from S. bicolor, BG and BGL proteins do not possess any known functional domains (Supplementary Figure S4). The middle region of SbiBG2 and ZmaBG3 show partial similarity with the domain present in γ and τ subunits of DNA polymerase III (Jergic et al., 2007) (Supplementary Figures S4A,C,D). Similarly, the mid region of ZmaBG2 shows partial similarity with the domain present in the δ subunit of DNA polymerase III (Song et al., 2001) (Supplementary Figures S4B,D). Apart from these remote similarities, BG and BGL proteins did not show any significant similarities with any known protein domains suggesting that they belong to a novel class of proteins.
Since the domain search revealed that BG and BGL proteins do not possess any known domains, we analyzed the BG and BGL proteins from eudicots and monocots individually for the presence of conserved motifs shared across different species. As observed in the BLAST results, BG and BGL proteins in both eudicots and monocots show the presence of a conserved C-terminal region (Figure 2). This region was also found to be conserved among the P. abies BG and BGL proteins (Supplementary Figure S1). The N-terminal of this region was found to be enriched with glutamic acid and aspartic acid repeats and showed a propensity for disorder in BG and BGL proteins we analyzed (Supplementary Figure S5). Further, many other amino acids were also found to be conserved in this C-terminal motif in the BG and BGL proteins in both eudicots and monocots (Figure 2). The topology prediction of the C-terminal motif of BG proteins from A. trichopoda, O. sativa and A. thaliana identified that this region has high propensity to form helices (Supplementary Figure S5A). However, analysis of fold similarity with known structures identified very low similarity suggesting that this C-terminal region is a novel domain (Supplementary Table S5). Topology prediction of the C-terminal motif of BGL proteins from A. trichopoda and A. thaliana predicted different topologies. The C-terminal motif of AtrBGL1 and AthBGL1 showed an N-terminal repeat of three small beta sheets followed by a C-terminal short helix while the shorter C-terminal motif of AthBGL2 predicted to have only the repeats of beta sheets (Supplementary Figure S5B). Analysis of fold similarity of the C-terminal motif of BGL2 also showed weak similarities with known structures (Supplementary Table S5).
FIGURE 2. The conserved C-terminal motif in BG and BGL protein family in eudicots and monocots. The sample alignment is given on the top and HMM logo of the motif is given in the bottom for each group.
Further, we identified four other conserved motifs present in BG proteins in both eudicots and monocots (Supplementary Figure S6). Similarly, conservation analysis identified two other conserved motifs in BGL proteins in both eudicots and monocots (Supplementary Figure S7). The motif III of BG proteins was found to have similarity with the N-terminus of the long motif II of BGL indicating that this is another region where the BG and BGL protein show similarity. The motif analysis identified that both BG and BGL proteins show many repeats of serine, glutamic acid, aspartic acid, arginine and lysine. As observed in the motif analysis, amino acid composition analysis of BG and BGL protein found the highest enrichment of serine followed by lysine, glutamine, arginine etc (Supplementary Figure S8).
In order to get more insight into the protein evolution, Bayesian phylogenetic reconstruction was used to decipher the evolutionary relationship of BG and BGL proteins in eudicots and monocots. The eudicot and monocot phylogenetic tree of BG and BGL protein was constructed individually along with the BG and BGL proteins from A. trichopoda. The eudicot BG and BGL proteins were recovered to separate clades in the phylogram (Figure 3). In the BGL clade, one protein each from V. vinifera, C. rubella and A. thaliana formed a clade with strong probability (posterior probability support value: 1) from other BGL proteins. We annotated them as BGL proteins because sequence alignment shows that it shares similarity with other BGL proteins in many regions. However, they also showed more sequence divergence compared to other BGL proteins (Supplementary Figure S9A). Further, in the individual phylogenetic tree with A. trichopoda BG and BGL proteins, these proteins were closely positioned with AtrBGL1 (Supplementary Figures S9B–D). The formation of separate clade from other BGL proteins can be due to the increased sequence divergence observed in these proteins and the consequent long-branch attraction (Kolaczkowski et al., 2009). Interestingly, in the BG proteins, we recovered four major sub-clades with strong probability (posterior probability support value: 0.8–1) suggesting sequence variation among the eudicot BG proteins. The BG proteins from eudicots without independent WGD were found to be positioned in most of these sub-clades suggesting the early origin of this sequence divergence. In the sub-clade recovered in our analysis, the positioning of BG proteins from different species fairly reflect the eudicot phylogeny (Zeng et al., 2017), suggesting the involvement of concerted evolution in protein divergence. The same pattern was also observed in the BGL sub-clades. The recovery of clades of BG and BGL proteins and different sub-clades in both groups is also observed in a maximum likelihood based estimation of the phylogenetic tree supporting the inferences obtained from the Bayesian phylogenetic reconstruction (Supplementary Figure S10). Further, we reconstructed the Bayesian phylogram of BG and BGL genes of eudicots using CDS sequences. As observed in the protein phylograms, the BG and BGL genes recovered to separate clades in the CDS-based phylogram (Supplementary Figure S11).
FIGURE 3. Phylogenetic analysis of BG and BGL proteins from eudicots. Bayesian phylogenetic reconstruction of BG and BGL proteins from eudicots and A. trichopoda based on JTT + I + G model. The posteriori probability values are given adjacent to the branches. The BG and BGL proteins are marked by red and green color respectively.
The recovery of BG and BGL proteins into separate clades was also observed in the Bayesian phylogram of monocots (Figure 4A). Further, BG proteins from monocots were also recovered to sub-clades with strong probabilities (posterior probability support value: 0.71–1). The maximum likelihood based estimation of the phylogenetic tree also supported these inferences (Supplementary Figure S12). However, in the Bayesian phylogenetic reconstruction using CDS sequences, the BGL genes from Poaceae were recovered to a clade closer to BG genes while other BGL genes formed another clade. Further, the solitary BG gene from A. trichopoda and one BG gene each from Z. marina and S. polyrhiza positioned a branch in between the two clades of BGL genes (Supplementary Figure S13).
FIGURE 4. Phylogenetic analysis of BG and BGL proteins from monocots and eudicots. (A) Bayesian phylogenetic reconstruction of BG and BGL proteins from monocots and A. trichopoda. (B) Bayesian phylogenetic reconstruction of selected BG and BGL proteins from angiosperms. The phylograms were reconstructed on the basis of JTT + I + G model and the posteriori probability values are given adjacent to the branches. The BG and BGL proteins are marked by red and green color respectively.
Taken together, the phylogenetic analysis of BG and BGL proteins from eudicots and monocots indicates the possible role of concerted evolution in the protein divergence. Strikingly, the recovery of BG and BGL proteins into distinct clades in the phylogenetic analysis supporting the occurrence of distinct motifs specific to BG and BGL proteins. To further validate this observation, a phylogram was constructed with eudicot and monocot BG and BGL proteins (Figure 4B). Irrespective of the ancient monocot-eudicot divergence, the BG and BGL proteins recovered to separate clades supporting the hypothesis that the origin of both types of proteins predates the divergence of angiosperms.
To identify the nature of evolutionary pressure acting on BG genes over time, the Ka/Ks ratio of 23 eudicot and 11 monocot putative paralogous gene pairs identified from the phylogenetic reconstruction was calculated. The Ka/Ks ratio of paralogous BG genes from eudicot was found to be ranging from 0.0108 to 0.7304 (Avg. 0.246) (Figure 5A). The Ka/Ks ratio of monocot BG genes was found to be ranging from 0.0601 to 0.3519 (Avg. 0.185) (Figure 5A). These results indicate strong purifying selection acting on BG genes in both eudicots and monocots. Similarly, we calculated the Ka/Ks ratios of 6 eudicots and 1 monocot putative paralogous BGL gene pairs. The Ka/Ks ratios of BGL genes were found to be ranging from 0.238 to 0.434 (Avg. 0.326) in eudicots. The Ka/Ks ratio of MacBGL1 and MacBGL2 was estimated to be 0.435 (Figure 5A). These results suggest that both BG and BGL genes are under purifying selection in angiosperms.
FIGURE 5. Analysis of selection pressure on BG and BGL genes. (A) The Ka/Ks ratio of paralogous BG and BGL genes from eudicots and monocots. (B) The Ka/Ks ratio of orthologous BG and BGL genes from eudicots and monocots.
To get better insight into the nature of selection in BG and BGL genes in closely related species, we calculated Ka/Ks ratio of putative orthologous genes inferred from the phylogenetic reconstruction (Figure 5B). The Ka/Ks ratio of 22 orthologous BG genes from eudicot was found to be ranging from 0.0917 to 0.3008 (Avg. 0.168). Similarly, the Ka/Ks ratio of 12 orthologous BG genes from monocots was found to be ranging from 0.0116 to 0.1851 (Avg. 0.116) confirming the purifying selection among BG genes. In 9 orthologous BGL eudicot genes analyzed, the Ka/Ks ratio varied from 0.0941 to 0.3856 (Avg. 0.177). Similarly, in the 3 orthologous BGL genes analyzed, the Ka/Ks ratio varied from 0.2065 to 0.4864 (Avg. 0.370) (Figure 5B). Taken together, these results confirm that both BG and BGL genes are under purifying selection in angiosperms.
For further analysis of BG and BGL gene family, we chose the model system A. thaliana which possess 4 BG and 2 BGL genes (Figure 1). The expression analysis of A. thaliana BG and BGL genes based on microarray data identified that these genes are expressed more in the seedling stages. Further, the expression of these genes was found to be more in the root, leaf, and flower and seed stages (Figure 6A). To validate the microarray expression data, we checked the expression of AthBG2 in different tissues and developmental stages using qRT-PCR (Figure 6B). In microarray data, the expression of AthBG2 was found to be high in the roots of different stages, leaf, flower etc. In qRT-PCR analysis, expression of AthBG2 was found to be high in seedling of cotyledon and 2 leaves stage. The expression was found to be relatively high during seed germination, flower and root of the mature rosette. However, the expression was found to be relatively less in the rosette before and after bolting (Figure 6B). We further analyzed the promoter activity of AthBG2 using promoter: GUS assay (Figure 6C). In seedling stage, the AthBG2 promoter was found to be active at cotyledon tip and stelar region of root and at the root tip. In mature leaves, the promoter activity was mainly restricted to serration tips. A small amount of promoter activity was observed in the stigma region. Further, promoter activity of AthBG2 was observed during lateral root development in the young primordia and enhanced expression was observed in young lateral root (Figure 6D). Taken together, the expression analysis of A. thaliana BG and BGL genes identified that these genes are expressed profusely in the seedling stages. Interestingly, the promoter: GUS assay of AthBG2 identified that the promoter activity of this gene is overlapping with the activity of DR5: GUS auxin-responsive reporter, which show maximum activity in the regions of auxin accumulation such as root tip and cotyledon tip (Ulmasov et al., 1997). Further, the promoter activity of AthBG2 overlaps with the expression domain of genes such as ARF7, ARF19, INDOLE-3-ACETIC ACID INDUCIBLE 2, LATERAL ORGAN BOUNDARIES-DOMAIN 16 (LBD16), LBD29, and LBD33 which are involved in the regulation of auxin-dependent development (Okushima et al., 2005, 2007; Hay et al., 2006).
FIGURE 6. Tissue and developmental stage-specific expression analysis of A. thaliana BG and BGL genes. (A) Heat map showing the expression of A. thaliana BG and BGL genes in different tissues and developmental stages (B) The qRT-PCR expression analysis of AthBG2 in different developmental stages and tissues. The expression in radicle stage was taken as control to compare the expression in other stages and tissues. UBQ10 was used as the endogenous control. The graph was plotted from the values of two biological replicates with three technical replicates each. The abbreviation of samples: RS, seeding radicle stage; CS, seeding cotyledon stage; seedling two-leaf stage; R22, rosette 22 days old; R32, rosette 32 days old; FB, flower bud; FO, flower open; MS, mature silique; MR, mature root. (C) Promoter activity of AthBG2 in different tissues and developmental stages of A. thaliana. (D) Promoter activity of AthBG2 during lateral root development.
The OsaBG1 is a primary auxin-responsive gene (Liu et al., 2015). The promoter: GUS assay of AthBG2 suggests that this gene might be involved in auxin signaling (Figures 6C,D). These results indicate that the auxin-dependent regulation of BG genes might be evolutionarily conserved. As the first step to test this hypothesis, we analyzed the presence of different auxin-responsive elements in the promoter and 5′UTR region of BG and BGL genes of A. trichopoda, O. sativa and A. thaliana (Figure 7A). The promoter and 5’UTR regions of both AtrBG1 and AtrBGL1 were found to be enriched with various auxin-responsive elements suggesting that the auxin-responsive expression of BG and BGL1 genes predates the angiosperm divergence. In rice, similar to OsaBG1, the promoter of other BG genes were also found to be enriched with auxin-responsive elements. The same pattern is also observed in the promoters of BG and BGL genes from A. thaliana (Figure 7A). As evident in the promoter analysis, the auxin-dependent expression analysis of A. thaliana BG and BGL genes using the available microarray data identified that these genes are responsive to auxin in various degrees (Figure 7B). The expression of AthBG1 and AthBG2 was found to be induced in response to 30 min of auxin treatment and increased expression was retained in treatments of 1 and 3 h. The expression of AthBG3 was induced in 30 min and in 1- and 3-h time points, expression was found to be almost similar to control levels. The expression of AthBG4 was slightly induced in 30 min treatment and showed a small decrease in expression in the later time points. Among the BGL genes, AthBGL1 expression was induced in response to 30 min treatment and repressed in response to 3 h treatment. In contrast, the expression of AthBGL2 was induced in response to 1-h treatment (Figure 7B). These results indicate that the auxin-dependent regulation of expression of BG genes is conserved in monocots and eudicots. Further, the expression of BGL genes was also found to be responsive to auxin. In order to validate the microarray-based expression data, qRT-PCR expression analysis of AthBG2 was done at various time points of auxin treatments (Figure 7C). As observed in the microarray data, the expression of AthBG2 was found to be induced in 30 min and the increase in expression was found to be retained in all other time points. In rice, the increased expression of OsaBG1 results in promotion of growth and increased grain size possibly through regulating auxin signaling pathway (Liu et al., 2015). In response to auxin, ARF7-ARF19 module redundantly activates cell cycle and positively regulates lateral and adventitious root formation and leaf cell expansion (Wilmoth et al., 2005; Okushima et al., 2007; Ito et al., 2016). Similar to OsaBG1, expression of AthBG2 was found to be constitutively induced in response to auxin treatment at different time points (Figure 7C). Since OsaBG1 is involved in the promotion of growth, we analyzed whether the auxin-dependent expression of AthBG2 is dependent on ARF7-ARF19 module using the arf7 arf19 mutant (Okushima et al., 2005) (Figure 7D). In the qRT-PCR analysis, the expression of AthBG2 was found to be similar in the mutant and WT suggesting that ARF7 and ARF19 are not involved in regulating the transcription of AthBG2 in normal conditions. As observed in previous experiment, the expression of AthBG2 was found to be induced in WT in response to auxin; however, this induction was found to be significantly abolished in the arf7 arf19 mutant in both time points studied suggesting the role of ARF7-ARF19 module in regulating the auxin-dependent expression of AthBG2 (Figure 7D).
FIGURE 7. Promoter analysis and auxin-responsive expression study of BG and BGL genes. (A) Auxin-responsive elements in the BG and BGL promoters A. trichopoda, A. thaliana and O. sativa along with the Bayesian phylogram of proteins. (B) Heat map showing the auxin-dependent expression of A. thaliana BG and BGL genes. (C) The qRT-PCR expression analysis of AthBG2 in response to IAA treatments. Asterisk indicates a significant difference in expression in treatment in comparison to untreated control (P < 0.05, Student’s t-test). (D) The qRT-PCR expression analysis of AthBG2 in response to IAA treatments in Col-0 and arf7arf19 double mutant. Asterisk indicates a significant difference in expression in the mutant in response to 30 or 180 min IAA treatment in comparison to the response in the WT (P < 0.05, Student’s t-test). The graphs of qRT-PCR experiments were plotted from the values of two biological replicates with three technical replicates each. UBQ10 was used as the endogenous control for both experiments.
In order to get clues about the molecular function of AthBG2, we screened Arabidopsis Y2H library using AthBG2 as bait. We obtained 68 colonies after screening on QDO/X/A medium. Sequencing of these colonies identified 6 proteins in repeats (Figure 8A). Further screening to identify positive interaction confirmed 4 genuine interacting proteins of AthBG2. The 4 interacting proteins of AthBG2 were found to have conserved molecular functions. The Y14 is a conserved eukaryotic protein which is a part of exon junction complex (EJC) and involved in RNA splicing (Park and Muench, 2007; Koroleva et al., 2009; Mufarrege et al., 2011; Cilano et al., 2016). SIGMA FACTOR 6 (SIG6) is a chloroplast sigma factor of RNA polymerase involved in chloroplast gene expression (Schweer et al., 2009; Chi et al., 2010). BRAHMA (BRM) is an SWI/SNF chromatin remodeling ATPase which is involved in the regulation of gene expression in response to many extrinsic and intrinsic cues (Han et al., 2015). KNOTTED1-LIKE HOMEOBOX GENE 5 (KNAT5) is a homeobox transcription factor expressed majorly in the distal end of the epidermal cells in the elongation zone of primary root (Truernit et al., 2006).
FIGURE 8. Protein-protein interaction and localization analysis of AthBG2. (A) The interacting proteins of AthBG2 identified from the Arabidopsis Y2H library screening. (B–D) BiFC confirmation of AthBG2-Y14 interaction with negative control experiments. (E) Subcellular localization of AthBG2. For both BiFC and localization experiments, YFP was excited at 514 nm and fluorescence emission was recorded at 530 nm.
In order to validate the Y2H result, we analyzed the interaction of AthBG2 with Y14 using BiFC assay. The AthBG2-Y14 interaction was found in both cytoplasm and nucleus confirming the Y2H result (Figure 8B). The removal of AthBG2 or Y14 abolished fluorescent signal confirming that this interaction is specific (Figures 8C,D). In the nucleus, the AthBG2-Y14 interaction was found to form speckle-like bodies. Y14 is reported to be localized predominantly in the nucleus (Park and Muench, 2007). Further, in the nucleus, Y14 was found in nucleolus and speckles (Koroleva et al., 2009). The subcellular localization analysis found that AthBG2 is predominantly localized in the nucleus, both in diffused and speckle-like form (Figure 8E).
The BG genes are recently identified as positive regulators of growth and grain size in rice (Liu et al., 2015). In this study, through homology-based searches in 39 viridiplantae genome, we suggest that BG genes are probably originated in seed plants. BG genes are absent in S. moellendorffii, which belongs to Lycopodiophyta. Since there is no sequenced monilophyte genome available, at this point we cannot rule out whether the origin of BG genes predates the dawn of seed plants. Interestingly, a class of BGL genes were also found to be originated at the same time. The BG and BGL proteins share a conserved C-terminal motif and a middle motif. Apart from these shared motifs, BG and BGL proteins possess many unique conserved regions which resulted in their recovery to separate clades in the phylogenetic reconstruction. In the CDS-based phylogenetic reconstruction of BG and BGL genes from monocots, the recovery to specific BG and BGL clades was not that distinct, which could be due to the accumulation of synonymous mutations in the coding region. However, even in monocots, the phylogenetic reconstruction based on protein sequences using both Bayesian and maximum likelihood methods recovered distinct clades of BG and BGL proteins confirming the early divergence of BG and BGL proteins.
Unlike the animal and fungi genomes, plant genomes are characterized by many ancestral and recent WGD events. As a consequence of this, on an average, approximately 65% of genes in plant genomes have duplicated copies (Panchy et al., 2016). The genome sequencing of many plants identified specific gain or loss of many genes and gene families which ensure the survival of the species in their local environment (Tuskan et al., 2006; Jaillon et al., 2007; Chan et al., 2010; Young et al., 2011; Hori et al., 2014). The functional analysis in rice identified that OsaBG1 is a general promoter of growth and seed size (Liu et al., 2015). Our study predicts that origin of these genes coincide with the origin of seed habit (Linkies et al., 2010). In our analysis, we found that ancient and recent WGD increased the number of BG genes in most of the species. Interestingly, although BGL genes are also originated along with BG genes in seed plants before the divergence of angiosperms into eudicots and monocots, in eudicot species without independent WGD, their number is not increased suggesting the selective loss of BGL genes after the ancient eudicot genome triplication. Characteristically, in species with recent WGD event(s), the numbers of BGL genes have been increased suggesting the contribution of recent WGD events in the expansion of BGL genes in such species. Consistent with this, analysis of retention rate of duplicated genes after WGD events in A. thaliana identified that the rate of retention of duplicated genes is high in recent WGD events (Panchy et al., 2016).
Increased gene dosage provides a selective advantage for the organisms and therefore polyploids generally show hybrid vigor and increased resilience (del Pozo and Ramirez-Parra, 2015; Panchy et al., 2016). Since BG1 gene in rice is a general growth promoter, the increased retention of these genes in comparison to BGL genes could be because of the selective advantage it can provide for the organisms. Along the same line, the reduced retention of BGL genes in angiosperms might have occurred because these genes might be acting as a competitive inhibitor of BG functions in a manner similar to microProteins (Eguen et al., 2015). This preliminary hypothesis can be tested in model species like A. thaliana which will provide interesting insights about the molecular connection between BG and BGL genes.
In our intra- and inter-species analysis, both BG and BGL genes were found to be under purifying selection. Consistent with this, although both these protein families do not have any known domains, they possess many novel motifs which are conserved across the plant kingdom. The gene duplication relaxes the selection pressure which results in the concomitant neo-functionalization or sub-functionalization and hypo-functionalization or non-functionalization in one or both duplicated genes. Eventually, the survivors of this selection would undergo strong purifying selection. In BG genes, the paralogous gene pairs from species without recent WGD (V. vinifera, T. cacao, R. communis, and F. vesca) events were found to be under more strong purifying selection than the species with recent lineage-specific or independent WGD (A. thaliana, C. rubella, B. rapa, M. esculenta, M. domestica, M. truncatula, G. max, Z. mays, and M. acuminata). Further, difference in the Ka/Ks values observed among paralogous gene pairs from different species can be due to the difference in the timing of WGD event in particular lineage and slow nucleotide substitution rate observed in species such as P. dactylifera and N. nucifera (Wilson et al., 1990; Ming et al., 2013).
The diverse expression pattern of A. thaliana BG and BGL genes in different developmental stages indicates their general role in regulating plant growth. The OsaBG1 is expressed abundantly in the vascular tissue of culm and young panicles (Liu et al., 2015). Our expression analysis identified that A. thaliana BG and BGL genes show high expression in seedling tissues and flowers suggesting common expression domains in eudicots and monocots. Further, the promoter: GUS assay identified that similar to OsaBG1, AthBG2 is expressed profusely in the vascular tissues. Interestingly, the expression of A. thaliana BG and BGL genes was high in roots while the expression of OsaBG1 was found relatively low in roots indicating differences in the expression domain in eudicots and monocots. The divergence in the promoter regions in monocots and eudicots might have contributed to this divergence in expression domain.
Among the phytohormones, auxin acts as the major regulator of plant development and OsaBG1 is found to enhance growth through promoting auxin signaling (Ljung, 2013; Liu et al., 2015). Our expression study identified that A. thaliana BG and BGL genes are responsive to auxin in varying degrees suggesting that the auxin-dependent transcriptional regulation is a conserved mechanism by which the transcription of BG and BGL genes are controlled. Promoter analysis identified significant enrichment of auxin-responsive elements in the promoters of AtrBG1 and AtrBGL1 suggesting that the auxin-dependent transcriptional regulation predates the angiosperm divergence. The promoter: GUS assay of AthBG2 identified that the promoter activity of this gene is high wherever localized auxin maxima is present. Further, the auxin-dependent expression of AthBG2 was found to be mediated through the ARF7-ARF19 module. These results indicate auxin as a major regulator of transcription of BG and BGL genes. The root-specific expression and the role of ARF7-ARF19 in the regulation of auxin-dependent expression of AthBG2 gene suggest the possible involvement of this gene in primary and lateral root development. Further, ARF19 from J. curcas is already implicated in the regulation of seed size and yield suggesting the interconnection between ARF19 pathway and seed development (Sun et al., 2017).
The localization analysis identified the predominant localization of AthBG2 in the nucleus. OsaBG1 is predominantly localized in the plasma membrane (Liu et al., 2015). These results suggest that BG genes from monocots and eudicots may have undergone divergence in the localization pattern which might be contributing to differences in the molecular functions. Protein-protein interaction analysis identified that AthBG2 interact with nuclear proteins with conserved molecular functions. AthBG2 interacts with Y14, which is the component of EJC, in the cytoplasm and nuclear speckles suggesting the role of AthBG2 in mRNA splicing. Further, both BG and BGL proteins were found to be serine-rich with multiple conserved serine repeats. This is a characteristic feature of proteins involved in splicing (Richardson et al., 2011). The interaction with SIG6 and KNAT5 suggest the possible role of AthBG2 in regulating transcription. The other interacting protein we identified from the Y2H library screening is BRM; which is an SWI/SNF chromatin remodeling ATPase reported to be essential for the maintenance of root stem cell niche through positively regulating auxin level and the transcription of specific PIN and PLETHORA genes (Yang et al., 2015). Further, BRM is involved in all aspects of plant growth from the embryonic to reproductive development and stress mitigation (Tang et al., 2008; Han et al., 2012; Li C. et al., 2015; Peirats-Llobet et al., 2016; Xu et al., 2016). The interaction of AthBG2 with BRM suggests its possible involvement in auxin-regulated processes like embryonic, root, shoot and reproductive development (Ljung, 2013). This comprehensive analysis of BG and BGL proteins reveals insights into their origin and expansion in plants. Further, the expression, localization and protein-protein interaction analyses highlight the possible differences between eudicot and monocot BG proteins and encourage more functional analysis in this gene family.
AL, BM, and MJ conceived and designed the study. MJ performed phylogenetic and bioinformatic analysis. BM performed cloning, promoter: GUS analysis and BiFC. MJ and BM performed the Y2H analysis. BM, DS, and MS performed qRT-PCR analysis. MJ, DS, and MS performed the Ka/Ks estimation. AL, BM, and MJ analyzed the data. MJ wrote the manuscript. All authors reviewed the manuscript
The authors declare that the research was conducted in the absence of any commercial or financial relationships that could be construed as a potential conflict of interest.
MJ acknowledges National Institute of Plant Genome Research for the Post-Doctoral Fellowship. DS and MS acknowledge the University Grant Commission, Government of India for the Senior Research Fellowship.
The Supplementary Material for this article can be found online at: https://www.frontiersin.org/articles/10.3389/fpls.2017.01812/full#supplementary-material
Albert, V. A., Barbazuk, W. B., dePamphilis, C. W., Der, J. P., Leebens-Mack, J., Ma, H., et al. (2013). The Amborella genome and the evolution of flowering plants. Science 342, 1241089–1241089. doi: 10.1126/science.1241089
Al-Mssallem, I. S., Hu, S., Zhang, X., Lin, Q., Liu, W., Tan, J., et al. (2013). Genome sequence of the date palm Phoenix dactylifera L. Nat. Commun. 4, 520–524. doi: 10.1038/ncomms3274
Argout, X., Salse, J., Aury, J.-M., Guiltinan, M. J., Droc, G., Gouzy, J., et al. (2011). The genome of Theobroma cacao. Nat. Genet. 43, 101–108. doi: 10.1038/ng.736
Bertioli, D. J., Moretzsohn, M. C., Madsen, L. H., Sandal, N., Leal-Bertioli, S. C., Guimaraes, P. M., et al. (2009). An analysis of synteny of Arachis with Lotus and Medicago sheds new light on the structure, stability and evolution of legume genomes. BMC Genomics 10:45. doi: 10.1186/1471-2164-10-45
Bowers, J. E., Chapman, B. A., Rong, J., and Paterson, A. H. (2003). Unravelling angiosperm genome evolution by phylogenetic analysis of chromosomal duplication events. Nature 422, 433–438. doi: 10.1038/nature01521
Bredeson, J. V., Lyons, J. B., Prochnik, S. E., Wu, G. A., Ha, C. M., Edsinger-Gonzales, E., et al. (2016). Sequencing wild and cultivated cassava and related species reveals extensive interspecific hybridization and genetic diversity. Nat. Biotechnol. 34, 562–570. doi: 10.1038/nbt.3535
Chan, A. P., Crabtree, J., Zhao, Q., Lorenzi, H., Orvis, J., Puiu, D., et al. (2010). Draft genome sequence of the oilseed species Ricinus communis. Nat. Biotechnol. 28, 951–956. doi: 10.1038/nbt.1674
Chi, W., Mao, J., Li, Q., Ji, D., Zou, M., Lu, C., et al. (2010). Interaction of the pentatricopeptide-repeat protein DELAYED GREENING 1 with sigma factor SIG6 in the regulation of chloroplast gene expression in Arabidopsis cotyledons. Plant J. 64, 14–25. doi: 10.1111/j.1365-313X.2010.04304.x
Cilano, K., Mazanek, Z., Khan, M., Metcalfe, S., and Zhang, X.-N. (2016). A new mutation, hap1-2, reveals a C terminal domain function in AtMago protein and its biological effects in male gametophyte development in Arabidopsis thaliana. PLOS ONE 11:e0148200. doi: 10.1371/journal.pone.0148200
Clough, S. J., and Bent, A. F. (1998). Floral dip: a simplified method for Agrobacterium-mediated transformation of Arabidopsis thaliana. Plant J. 16, 735–743.
Curtis, M. D., and Grossniklaus, U. (2003). A gateway cloning vector set for high-throughput functional analysis of genes in planta. Plant Physiol. 133, 462–469. doi: 10.1104/pp.103.027979
del Pozo, J. C., and Ramirez-Parra, E. (2015). Whole genome duplications in plants: an overview from Arabidopsis. J. Exp. Bot. 66, 6991–7003. doi: 10.1093/jxb/erv432
D’Hont, A., Denoeud, F., Aury, J.-M., Baurens, F.-C., Carreel, F., Garsmeur, O., et al. (2012). The banana (Musa acuminata) genome and the evolution of monocotyledonous plants. Nature 488, 213–217. doi: 10.1038/nature11241
Earley, K. W., Haag, J. R., Pontes, O., Opper, K., Juehne, T., Song, K., et al. (2006). Gateway-compatible vectors for plant functional genomics and proteomics. Plant J. 45, 616–629. doi: 10.1111/j.1365-313X.2005.02617.x
Edger, P. P., Smith, R. D., McKain, M. R., Cooley, A. M., Vallejo-Marin, M., Yuan, Y., et al. (2016). Subgenome Dominance in an Interspecific Hybrid, Synthetic Allopolyploid, and a 140 Year Old Naturally Established Neo-Allopolyploid Monkeyflower. Available at: http://www.biorxiv.org/content/early/2016/12/16/094797 [accessed August 9, 2017].
Eguen, T., Straub, D., Graeff, M., and Wenkel, S. (2015). MicroProteins: small size – big impact. Trends Plant Sci. 20, 477–482. doi: 10.1016/j.tplants.2015.05.011
Finn, R. D., Clements, J., Arndt, W., Miller, B. L., Wheeler, T. J., Schreiber, F., et al. (2015). HMMER web server: 2015 update. Nucleic Acids Res. 43, W30–W38. doi: 10.1093/nar/gkv397
Fujita, D., Trijatmiko, K. R., Tagle, A. G., Sapasap, M. V., Koide, Y., Sasaki, K., et al. (2013). NAL1 allele from a rice landrace greatly increases yield in modern indica cultivars. Proc. Natl. Acad. Sci. U.S.A. 110, 20431–20436. doi: 10.1073/pnas.1310790110
Goda, H., Sasaki, E., Akiyama, K., Maruyama-Nakashita, A., Nakabayashi, K., Li, W., et al. (2008). The AtGenExpress hormone- and chemical-treatment data set: experimental design, data evaluation, model data analysis, and data access. Plant J. 0, 80414150319983. doi: 10.1111/j.0960-7412.2008.03510.x
Goodstein, D. M., Shu, S., Howson, R., Neupane, R., Hayes, R. D., Fazo, J., et al. (2012). Phytozome: a comparative platform for green plant genomics. Nucleic Acids Res. 40, D1178–D1186. doi: 10.1093/nar/gkr944
Han, S.-K., Sang, Y., Rodrigues, A., Wagner, D., Wu, M.-F., Rodriguez, P. L., et al. (2012). The SWI2/SNF2 chromatin remodeling ATPase BRAHMA represses abscisic acid responses in the absence of the stress stimulus in Arabidopsis. Plant Cell 24, 4892–4906. doi: 10.1105/tpc.112.105114
Han, S.-K., Wu, M.-F., Cui, S., and Wagner, D. (2015). Roles and activities of chromatin remodeling ATPases in plants. Plant J. 83, 62–77. doi: 10.1111/tpj.12877
Hay, A., Barkoulas, M., and Tsiantis, M. (2006). ASYMMETRIC LEAVES1 and auxin activities converge to repress BREVIPEDICELLUS expression and promote leaf development in Arabidopsis. Development 133, 3955–3961. doi: 10.1242/dev.02545
Hori, K., Maruyama, F., Fujisawa, T., Togashi, T., Yamamoto, N., Seo, M., et al. (2014). Klebsormidium flaccidum genome reveals primary factors for plant terrestrial adaptation. Nat. Commun. 5, 2914–2927. doi: 10.1038/ncomms4978
Ito, J., Fukaki, H., Onoda, M., Li, L., Li, C., Tasaka, M., et al. (2016). Auxin-dependent compositional change in mediator in ARF7- and ARF19-mediated transcription. Proc. Natl. Acad. Sci. U.S.A. 113, 6562–6567. doi: 10.1073/pnas.1600739113
Jaillon, O., Aury, J.-M., Noel, B., Policriti, A., Clepet, C., Casagrande, A., et al. (2007). The grapevine genome sequence suggests ancestral hexaploidization in major angiosperm phyla. Nature 449, 463–467. doi: 10.1038/nature06148
Jamsheer, K. M., Mannully, C. T., Gopan, N., and Laxmi, A. (2015). Comprehensive evolutionary and expression analysis of FCS-like zinc finger gene family yields insights into their origin, expansion and divergence. PLOS ONE 10:e0134328. doi: 10.1371/journal.pone.0134328
Jefferson, R. A., Kavanagh, T. A., and Bevan, M. W. (1987). GUS fusions: beta-glucuronidase as a sensitive and versatile gene fusion marker in higher plants. EMBO J. 6, 3901–3907.
Jergic, S., Ozawa, K., Williams, N. K., Su, X.-C., Scott, D. D., Hamdan, S. M., et al. (2007). The unstructured C-terminus of the subunit of Escherichia coli DNA polymerase III holoenzyme is the site of interaction with the subunit. Nucleic Acids Res. 35, 2813–2824. doi: 10.1093/nar/gkm079
Jiao, Y., Leebens-Mack, J., Ayyampalayam, S., Bowers, J. E., McKain, M. R., McNeal, J., et al. (2012). A genome triplication associated with early diversification of the core eudicots. Genome Biol. 13:R3. doi: 10.1186/gb-2012-13-1-r3
Jiao, Y., Li, J., Tang, H., and Paterson, A. H. (2014). Integrated syntenic and phylogenomic analyses reveal an ancient genome duplication in monocots. Plant Cell 26, 2792–2802. doi: 10.1105/tpc.114.127597
Jiao, Y., Wickett, N. J., Ayyampalayam, S., Chanderbali, A. S., Landherr, L., Ralph, P. E., et al. (2011). Ancestral polyploidy in seed plants and angiosperms. Nature 473, 97–100. doi: 10.1038/nature09916
Jones, D. T., Taylor, W. R., and Thornton, J. M. (1992). The rapid generation of mutation data matrices from protein sequences. Comput. Appl. Biosci. 8, 275–282.
Kelley, L. A., Mezulis, S., Yates, C. M., Wass, M. N., and Sternberg, M. J. E. (2015). The Phyre2 web portal for protein modeling, prediction and analysis. Nat. Protoc. 10, 845–858. doi: 10.1038/nprot.2015.053
Kolaczkowski, B., Thornton, J. W., Philippe, H., Raucourt, G., de, and Philippe, H. (2009). Long-branch attraction bias and inconsistency in bayesian phylogenetics. PLOS ONE 4:e7891. doi: 10.1371/journal.pone.0007891
Koroleva, O. A., Calder, G., Pendle, A. F., Kim, S. H., Lewandowska, D., Simpson, C. G., et al. (2009). Dynamic behavior of Arabidopsis eIF4A-III, putative core protein of exon junction complex: fast relocation to nucleolus and splicing speckles under hypoxia. Plant Cell 21, 1592–1606. doi: 10.1105/tpc.108.060434
Larkin, M. A., Blackshields, G., Brown, N. P., Chenna, R., McGettigan, P. A., McWilliam, H., et al. (2007). Clustal W and Clustal X version 2.0. Bioinformatics 23, 2947–2948. doi: 10.1093/bioinformatics/btm404
Li, C., Chen, C., Gao, L., Yang, S., Nguyen, V., Shi, X., et al. (2015). The Arabidopsis SWI2/SNF2 chromatin remodeler BRAHMA regulates polycomb function during vegetative development and directly activates the flowering repressor gene SVP. PLOS Genet. 11:e1004944. doi: 10.1371/journal.pgen.1004944
Li, Z., Baniaga, A. E., Sessa, E. B., Scascitelli, M., Graham, S. W., Rieseberg, L. H., et al. (2015). Early genome duplications in conifers and other seed plants. Sci. Adv. 10:e1501084. doi: 10.1126/sciadv.1501084
Linkies, A., Graeber, K., Knight, C., and Leubner-Metzger, G. (2010). The evolution of seeds. New Phytol. 186, 817–831. doi: 10.1111/j.1469-8137.2010.03249.x
Liu, L., Tong, H., Xiao, Y., Che, R., Xu, F., Hu, B., et al. (2015). Activation of Big Grain1 significantly improves grain size by regulating auxin transport in rice. Proc. Natl. Acad. Sci. U.S.A. 112, 11102–11107. doi: 10.1073/pnas.1512748112
Livak, K. J., and Schmittgen, T. D. (2001). Analysis of relative gene expression data using real-time quantitative PCR and the 2-ΔΔCT method. Methods 25, 402–408. doi: 10.1006/meth.2001.1262
Ljung, K. (2013). Auxin Metabolism and Homeostasis During Plant Development. Available at: http://dev.biologists.org/content/140/5/943 [accessed May 16, 2017].
Marchler-Bauer, A., Derbyshire, M. K., Gonzales, N. R., Lu, S., Chitsaz, F., Geer, L. Y., et al. (2015). CDD: NCBI’s conserved domain database. Nucleic Acids Res. 43, D222–D226. doi: 10.1093/nar/gku1221
McAdam, E. L., Meitzel, T., Quittenden, L. J., Davidson, S. E., Dalmais, M., Bendahmane, A. I., et al. (2017). Evidence that auxin is required for normal seed size and starch synthesis in pea. New Phytol. 216, 193–204. doi: 10.1111/nph.14690
Milne, I., Lindner, D., Bayer, M., Husmeier, D., McGuire, G., Marshall, D. F., et al. (2009). TOPALi v2: a rich graphical interface for evolutionary analyses of multiple alignments on HPC clusters and multi-core desktops. Bioinformatics 25, 126–127. doi: 10.1093/bioinformatics/btn575
Ming, R., Hou, S., Feng, Y., Yu, Q., Dionne-Laporte, A., Saw, J. H., et al. (2008). The draft genome of the transgenic tropical fruit tree papaya (Carica papaya Linnaeus). Nature 452, 991–996. doi: 10.1038/nature06856
Ming, R., VanBuren, R., Liu, Y., Yang, M., Han, Y., Li, L.-T., et al. (2013). Genome of the long-living sacred lotus (Nelumbo nucifera Gaertn.). Genome Biol. 14:R41. doi: 10.1186/gb-2013-14-5-r41
Ming, R., VanBuren, R., Wai, C. M., Tang, H., Schatz, M. C., Bowers, J. E., et al. (2015). The pineapple genome and the evolution of CAM photosynthesis. Nat. Genet. 47, 1435–1442. doi: 10.1038/ng.3435
Mishra, B. S., Singh, M., Aggrawal, P., and Laxmi, A. (2009). Glucose and auxin signaling interaction in controlling Arabidopsis thaliana seedlings root growth and development. PLOS ONE 4:e4502. doi: 10.1371/journal.pone.0004502
Mufarrege, E. F., Gonzalez, D. H., and Curi, G. C. (2011). Functional interconnections of Arabidopsis exon junction complex proteins and genes at multiple steps of gene expression. J. Exp. Bot. 62, 5025–5036. doi: 10.1093/jxb/err202
Mun, T., Bachmann, A., Gupta, V., Stougaard, J., and Andersen, S. U. (2016). Lotus base: an integrated information portal for the model legume Lotus japonicus. Sci. Rep. 6:39447. doi: 10.1038/srep39447
Okushima, Y., Fukaki, H., Onoda, M., Theologis, A., and Tasaka, M. (2007). ARF7 and ARF19 regulate lateral root formation via direct activation of LBD/ASL genes in Arabidopsis. Plant Cell 19, 118–130. doi: 10.1105/tpc.106.047761
Okushima, Y., Overvoorde, P. J., Arima, K., Alonso, J. M., Chan, A., Chang, C., et al. (2005). Functional genomic analysis of the AUXIN RESPONSE FACTOR gene family members in Arabidopsis thaliana: unique and overlapping functions of ARF7 and ARF19. Plant Cell 17, 444–463. doi: 10.1105/tpc.104.028316
Olsen, J. L., Rouzé, P., Verhelst, B., Lin, Y.-C., Bayer, T., Collen, J., et al. (2016). The genome of the seagrass Zostera marina reveals angiosperm adaptation to the sea. Nature 530, 331–335. doi: 10.1038/nature16548
Ouyang, S., Zhu, W., Hamilton, J., Lin, H., Campbell, M., Childs, K., et al. (2007). The TIGR rice genome annotation resource: improvements and new features. Nucleic Acids Res. 35, D883–D887. doi: 10.1093/nar/gkl976
Panchy, N., Lehti-Shiu, M., and Shiu, S.-H. (2016). Evolution of gene duplication in plants. Plant Physiol. 171, 2294–2316. doi: 10.1104/pp.16.00523
Park, N., and Muench, D. G. (2007). Biochemical and cellular characterization of the plant ortholog of PYM, a protein that interacts with the exon junction complex core proteins Mago and Y14. Planta 225, 625–639. doi: 10.1007/s00425-006-0385-y
Paterson, A. H., Bowers, J. E., and Chapman, B. A. (2004). Ancient polyploidization predating divergence of the cereals, and its consequences for comparative genomics. Proc. Natl. Acad. Sci. U.S.A. 101, 9903–9908. doi: 10.1073/pnas.0307901101
Peirats-Llobet, M., Han, S.-K., Gonzalez-Guzman, M., Jeong, C. W., Rodriguez, L., Belda-Palazon, B., et al. (2016). A direct link between abscisic acid sensing and the chromatin-remodeling ATPase BRAHMA via core ABA signaling pathway components. Mol. Plant 9, 136–147. doi: 10.1016/j.molp.2015.10.003
Pfeil, B., Schlueter, J., Shoemaker, R., and Doyle, J. (2005). Placing paleopolyploidy in relation to taxon divergence: a phylogenetic analysis in legumes using 39 gene families. Syst. Biol. 54, 441–454. doi: 10.1080/10635150590945359
Pruitt, K. D., Tatusova, T., and Maglott, D. R. (2007). NCBI reference sequences (RefSeq): a curated non-redundant sequence database of genomes, transcripts and proteins. Nucleic Acids Res. 35, D61–D65. doi: 10.1093/nar/gkl842
Qi, J., Qian, Q., Bu, Q., Li, S., Chen, Q., Sun, J., et al. (2008). Mutation of the rice Narrow leaf1 gene, which encodes a novel protein, affects vein patterning and polar auxin transport. Plant Physiol. 147, 1947–1959. doi: 10.1104/pp.108.118778
Rambout, A. (2016). FigTree. Available at: http://tree.bio.ed.ac.uk/software/figtree/ [accessed May 8, 2017].
Richardson, D. N., Rogers, M. F., Labadorf, A., Ben-Hur, A., Guo, H., Paterson, A. H., et al. (2011). Comparative analysis of serine/arginine-rich proteins across 27 eukaryotes: insights into sub-family classification and extent of alternative splicing. PLOS ONE 6:e24542. doi: 10.1371/journal.pone.0024542
Ronquist, F., Teslenko, M., van der Mark, P., Ayres, D. L., Darling, A., Höhna, S., et al. (2012). MrBayes 3.2: efficient Bayesian phylogenetic inference and model choice across a large model space. Syst. Biol. 61, 539–542. doi: 10.1093/sysbio/sys029
Saeed, A. I., Bhagabati, N. K., Braisted, J. C., Liang, W., Sharov, V., Howe, E. A., et al. (2006). TM4 microarray software suite. Methods Enzymol. 411, 134–193. doi: 10.1016/S0076-6879(06)11009-5
Sato, S., Nakamura, Y., Kaneko, T., Asamizu, E., Kato, T., Nakao, M., et al. (2008). Genome structure of the legume, Lotus japonicus. DNA Res. 15, 227–239. doi: 10.1093/dnares/dsn008
Schmid, M., Davison, T. S., Henz, S. R., Pape, U. J., Demar, M., Vingron, M., et al. (2005). A gene expression map of Arabidopsis thaliana development. Nat. Genet. 37, 501–506. doi: 10.1038/ng1543
Schmutz, J., Cannon, S. B., Schlueter, J., Ma, J., Mitros, T., Nelson, W., et al. (2010). Genome sequence of the palaeopolyploid soybean. Nature 463, 178–183. doi: 10.1038/nature08670
Schnable, P. S., Ware, D., Fulton, R. S., Stein, J. C., Wei, F., Pasternak, S., et al. (2009). The B73 maize genome: complexity, diversity, and dynamics. Science 326, 1112–1115. doi: 10.1126/science.1178534
Schruff, M. C., Spielman, M., Tiwari, S., Adams, S., Fenby, N., and Scott, R. J. (2005). The AUXIN RESPONSE FACTOR 2 gene of Arabidopsis links auxin signalling, cell division, and the size of seeds and other organs. Development 133, 251–261. doi: 10.1242/dev.02194
Schweer, J., Geimer, S., Meurer, J., and Link, G. (2009). Arabidopsis mutants carrying chimeric sigma factor genes reveal regulatory determinants for plastid gene expression. Plant Cell Physiol. 50, 1382–1386. doi: 10.1093/pcp/pcp069
Shulaev, V., Sargent, D. J., Crowhurst, R. N., Mockler, T. C., Folkerts, O., Delcher, A. L., et al. (2011). The genome of woodland strawberry (Fragaria vesca). Nat. Genet. 43, 109–116. doi: 10.1038/ng.740
Song, M.-S., Pham, P. T., Olson, M., Carter, J. R., Franden, M. A., Schaaper, R. M., et al. (2001). The delta and delta subunits of the DNA polymerase III holoenzyme are essential for initiation complex formation and processive elongation. J. Biol. Chem. 276, 35165–35175. doi: 10.1074/jbc.M100389200
Sun, Y., Wang, C., Wang, N., Jiang, X., Mao, H., Zhu, C., et al. (2017). Manipulation of auxin response factor 19 affects seed size in the woody perennial Jatropha curcas. Sci. Rep. 7:40844. doi: 10.1038/srep40844
Sundell, D., Mannapperuma, C., Netotea, S., Delhomme, N., Lin, Y.-C., Sjödin, A., et al. (2015). The plant genome integrative explorer resource: PlantGenIE.org. New Phytol. 208, 1149–1156. doi: 10.1111/nph.13557
Suyama, M., Torrents, D., and Bork, P. (2006). PAL2NAL: robust conversion of protein sequence alignments into the corresponding codon alignments. Nucleic Acids Res. 34, W609–W612. doi: 10.1093/nar/gkl315
Takai, T., Adachi, S., Taguchi-Shiobara, F., Sanoh-Arai, Y., Iwasawa, N., Yoshinaga, S., et al. (2013). A natural variant of NAL1, selected in high-yield rice breeding programs, pleiotropically increases photosynthesis rate. Sci. Rep. 3, 2149. doi: 10.1038/srep02149
Tang, H., Bowers, J. E., Wang, X., and Paterson, A. H. (2010). Angiosperm genome comparisons reveal early polyploidy in the monocot lineage. Proc. Natl. Acad. Sci. U.S.A. 107, 472–477. doi: 10.1073/pnas.0908007107
Tang, X., Hou, A., Babu, M., Nguyen, V., Hurtado, L., Lu, Q., et al. (2008). The Arabidopsis BRAHMA chromatin-remodeling ATPase is involved in repression of seed maturation genes in leaves. Plant Physiol. 147, 1143–1157. doi: 10.1104/pp.108.121996
Tavaré, S. (1986). Some Probabilistic and Statistical Problems in the Analysis of DNA Sequences. Available at: http://www.citeulike.org/user/aprasad/article/4801403 [accessed August 17, 2017].
Tiley, G. P., Ané, C., and Burleigh, J. G. (2016). Evaluating and characterizing ancient whole-genome duplications in plants with gene count data. Genome Biol. Evol. 8, 1023–1037. doi: 10.1093/gbe/evw058
Truernit, E., Siemering, K. R., Hodge, S., Grbic, V., and Haseloff, J. (2006). A map of KNAT gene expression in the Arabidopsis root. Plant Mol. Biol. 60, 1–20. doi: 10.1007/s11103-005-1673-9
Tuskan, G. A., DiFazio, S., Jansson, S., Bohlmann, J., Grigoriev, I., Hellsten, U., et al. (2006). The genome of black cottonwood, Populus trichocarpa (Torr. & Gray). Science 313, 1596–1604. doi: 10.1126/science.1128691
Tzfira, T., Tian, G.-W., Lacroix, B., Vyas, S., Li, J., Leitner-Dagan, Y., et al. (2005). pSAT vectors: a modular series of plasmids for autofluorescent protein tagging and expression of multiple genes in plants. Plant Mol. Biol. 57, 503–516. doi: 10.1007/s11103-005-0340-5
Ulmasov, T., Murfett, J., Hagen, G., and Guilfoyle, T. J. (1997). Aux/IAA proteins repress expression of reporter genes containing natural and highly active synthetic auxin response elements. Plant Cell 9, 1963–1971. doi: 10.1105/tpc.9.11.1963
Velasco, R., Zharkikh, A., Affourtit, J., Dhingra, A., Cestaro, A., Kalyanaraman, A., et al. (2010). The genome of the domesticated apple (Malus × domestica Borkh.). Nat. Genet. 42, 833–839. doi: 10.1038/ng.654
Wang, W., Haberer, G., Gundlach, H., Gläßer, C., Nussbaumer, T., Luo, M. C., et al. (2014). The Spirodela polyrhiza genome reveals insights into its neotenous reduction fast growth and aquatic lifestyle. Nat. Commun. 5:3311. doi: 10.1038/ncomms4311
Wang, X., Wang, H., Wang, J., Sun, R., Wu, J., Liu, S., et al. (2011). The genome of the mesopolyploid crop species Brassica rapa. Nat. Genet. 43, 1035–1039. doi: 10.1038/ng.919
Waterhouse, A. M., Procter, J. B., Martin, D. M. A., Clamp, M., and Barton, G. J. (2009). Jalview Version 2–a multiple sequence alignment editor and analysis workbench. Bioinformatics 25, 1189–1191. doi: 10.1093/bioinformatics/btp033
Wheeler, T. J., Clements, J., and Finn, R. D. (2014). Skylign: a tool for creating informative, interactive logos representing sequence alignments and profile hidden Markov models. BMC Bioinformatics 15:7. doi: 10.1186/1471-2105-15-7
Wilmoth, J. C., Wang, S., Tiwari, S. B., Joshi, A. D., Hagen, G., Guilfoyle, T. J., et al. (2005). NPH4/ARF7 and ARF19 promote leaf expansion and auxin-induced lateral root formation. Plant J. 43, 118–130. doi: 10.1111/j.1365-313X.2005.02432.x
Wilson, M. A., Gaut, B., and Clegg, M. T. (1990). Chloroplast DNA evolves slowly in the palm family (Arecaceae). Mol. Biol. Evol. 7, 303–314.
Winter, D., Vinegar, B., Nahal, H., Ammar, R., Wilson, G. V., and Provart, N. J. (2007). An “electronic fluorescent pictograph” browser for exploring and analyzing large-scale biological data sets. PLOS ONE 2:e718. doi: 10.1371/journal.pone.0000718
Xu, Y., Guo, C., Zhou, B., Li, C., Wang, H., Zheng, B., et al. (2016). Regulation of vegetative phase change by SWI2/SNF2 chromatin remodeling ATPase BRAHMA. Plant Physiol. 172, 2416–2428. doi: 10.1104/pp.16.01588
Yang, S., Li, C., Zhao, L., Gao, S., Lu, J., Zhao, M., et al. (2015). The Arabidopsis SWI2/SNF2 chromatin remodeling ATPase BRAHMA targets directly to PINs and Is required for root stem cell niche maintenance. Plant Cell 27, 1670–1680. doi: 10.1105/tpc.15.00091
Young, N. D., Debellé, F., Oldroyd, G. E. D., Geurts, R., Cannon, S. B., Udvardi, M. K., et al. (2011). The Medicago genome provides insight into the evolution of rhizobial symbioses. Nature 480, 520–524. doi: 10.1038/nature10625
Zeng, L., Zhang, N., Zhang, Q., Endress, P. K., Huang, J., and Ma, H. (2017). Resolution of deep eudicot phylogeny and their temporal diversification using nuclear genes from transcriptomic and genomic datasets. New Phytol. 214, 1338–1354. doi: 10.1111/nph.14503
Zhang, G.-H., Li, S.-Y., Wang, L., Ye, W.-J., Zeng, D.-L., Rao, Y.-C., et al. (2014). LSCHL4 from japonica cultivar, which is allelic to NAL1, increases yield of indica super rice 93-11. Mol. Plant 7, 1350–1364. doi: 10.1093/mp/ssu055
Keywords: BIG GRAIN, BIG GRAIN LIKE, Phylogenetic analysis, gene family, auxin, ARF7/19
Citation: Mishra BS, Jamsheer K M, Singh D, Sharma M and Laxmi A (2017) Genome-Wide Identification and Expression, Protein–Protein Interaction and Evolutionary Analysis of the Seed Plant-Specific BIG GRAIN and BIG GRAIN LIKE Gene Family. Front. Plant Sci. 8:1812. doi: 10.3389/fpls.2017.01812
Received: 06 June 2017; Accepted: 05 October 2017;
Published: 25 October 2017.
Edited by:
Elena M. Kramer, Harvard University, United StatesReviewed by:
Michael R. McKain, University of Alabama, United StatesCopyright © 2017 Mishra, Jamsheer K, Singh, Sharma and Laxmi. This is an open-access article distributed under the terms of the Creative Commons Attribution License (CC BY). The use, distribution or reproduction in other forums is permitted, provided the original author(s) or licensor are credited and that the original publication in this journal is cited, in accordance with accepted academic practice. No use, distribution or reproduction is permitted which does not comply with these terms.
*Correspondence: Ashverya Laxmi, YXNodmVyeWFfbGF4bWlAbmlwZ3IuYWMuaW4=
†Present address: Bhuwaneshwar S. Mishra, Department of Plant Molecular Biology, University of Delhi, New Delhi, India
‡These authors have contributed equally to this work as first authors.
§These authors have contributed equally to this work as second authors.
Disclaimer: All claims expressed in this article are solely those of the authors and do not necessarily represent those of their affiliated organizations, or those of the publisher, the editors and the reviewers. Any product that may be evaluated in this article or claim that may be made by its manufacturer is not guaranteed or endorsed by the publisher.
Research integrity at Frontiers
Learn more about the work of our research integrity team to safeguard the quality of each article we publish.