- College of Life and Environment Sciences, Shanghai Normal University, Shanghai, China
In flowering plants, male gametophyte development occurs in the anther. Tapetum, the innermost of the four anther somatic layers, surrounds the developing reproductive cells to provide materials for pollen development. A genetic pathway of DYT1-TDF1-AMS-MS188 in regulating tapetum development has been proven. Here we used laser microdissection and pressure catapulting to capture and analyze the transcriptome data for the Arabidopsis tapetum at two stages. With a comprehensive analysis by the microarray data of dyt1, tdf1, ams, and ms188 mutants, we identified possible downstream genes for each transcription factor. These transcription factors regulate many biological processes in addition to activating the expression of the other transcription factor. Briefly, DYT1 may also regulate early tapetum development via E3 ubiquitin ligases and many other transcription factors. TDF1 is likely involved in redox and cell degradation. AMS probably regulates lipid transfer proteins, which are involved in pollen wall formation, and other E3 ubiquitin ligases, functioning in degradating proteins produced in previous processes. MS188 is responsible for most cell wall-related genes, functioning both in tapetum cell wall degradation and pollen wall formation. These results propose a more complex gene regulatory network for tapetum development and function.
Introduction
Anther contains four locules, each with four layers of somatic cells surrounding the germ cells (Goldberg et al., 1993). The tapetum directly contacts the germ cells. It is the main tissue providing precursors for pollen development and pollen wall formation (Heslop-Harrison, 1962; Mariani et al., 1990; Ariizumi and Toriyama, 2011). Development of the tapetum is highly regulated. Tapetum cells are identified at anther stage 5 and undergo programmed cell death (PCD) in stage 10, then release the contents for further pollen wall development (Sanders et al., 1999). Mature pollen forms at stage 12 (Owen and Makaroff, 1995).
Several transcription factors regulating tapetum development and pollen formation have been reported. DYSFUNCTIONAL TAPETUM1 (DYT1) encodes a putative basic helix-loop-helix (bHLH) transcription factor. Defective DYT1 results in a premature vacuolation of the tapetum and the absence of pollen (Zhang et al., 2006). DEFECTIVE in TAPETAL DEVELOPMENT and FUNCTION1 (TDF1) encodes a putative R2R3 MYB transcription factor. Defective TDF1 results in a dysfunctional tapetum with irregular cell division and absence of pollen in both Arabidopsis and rice (Zhu et al., 2008; Cai et al., 2015). The transcription factor ABORTED MICROSPORES (AMS) encodes a bHLH family protein. The ams mutant shows degeneration of both tapetum and microspores at anther stage 7 (Sorensen et al., 2003; Xu et al., 2014). AtMYB103/MS188, also a member of the R2R3 MYB gene family, plays an important role in tapetum development, callose dissolution and exine formation in anthers. In myb103/ms188 mutant, the tapetal protoplast contains several irregular enlarged vacuoles after meiosis stage, indicating the abnormal tapetal development in this mutant (Higginson et al., 2003; Zhang et al., 2007; Zhu et al., 2010, 2011).
Although the results of in situ hybridization with wild-type and mutant anther sections showed these transcription factors expressed in tapetum and microsporocytes, the GFP fusion proteins were specifically localized in tapetal cells (Gu et al., 2014; Xiong et al., 2016; Ferguson et al., 2017). The mutants of these four transcription factors are male-sterile, with defective tapetum development.
DYT1 was expressed at anther stage 4, which indicates that it functions during the early stages of tapetum development (Zhu et al., 2011). Previous transcriptome analysis showed that DYT1 integrates lipid metabolism, transporter, secondary metabolism and other biological processes for pollen development (Feng et al., 2012). DYT1 directly regulates the expression of TDF1 (Gu et al., 2014). The expression of TDF1 in dyt1 restored the expression of AMS, MS188 and pollen wall-related genes although the transgenic line is still male sterile (Gu et al., 2014). TDF1 was also expressed at stage 4 and peaked in expression in the tapetum and meiocytes at stage 6, then gradually decreased after meiosis. In TDF1 knock out mutant, AMS is only minimally expressed, which indicates that TDF1 acts upstream of AMS (Zhu et al., 2008, 2011). AMS is a key regulator of pollen wall formation. It directly regulates MS188 and transposable element silencing via AT-hook (TEK) for sexine and nexine formation (Zhang et al., 2007; Lou et al., 2014). AMS expression was low at stage 5 but high at the meiosis stage and lasted until the release of pollen (Sorensen et al., 2003; Zhu et al., 2011). The expression pattern of AMS indicates that AMS might be responsible for both tapetum development at an early stage and degradation at a later stage. MS188 is a key regulator of tapetum development and pollen sexine formation. In ms188, the degradation of the tapetum cell wall is delayed, and the sexine of the pollen wall is absent. Therefore, MS188 is important for tapetum cell wall degradation and sexine formation (Zhang et al., 2007). These transcription factors form a genetic pathway, DYT1-TDF1-AMS-MS188, to regulate tapetum development and function (Zhu et al., 2008, 2011; Gu et al., 2014; Lou et al., 2014). However, the expression of TDF1 in dyt1 mutant cannot rescue their male-sterility, indicating the complexity of gene regulation network in tapetum development and pollen formation.
To better understand transcriptional regulation in the tapetum, we used laser microdissection and pressure catapulting (LMPC) to study gene expression in tapetum cells (Burgemeister et al., 2003; Zhan et al., 2015). With a comprehensive analysis of global transcriptional changes in dyt1, tdf1, ams, ms188 mutants and transcriptomes of tapetum cells, we identified several subsets of genes as targets of the transcription factors in tapetum cells. Detailed analysis of this subset revealed an expanded transcriptional regulatory network during tapetum development in Arabidopsis thaliana.
Materials and Methods
Plant Material
Plants were grown under long-day conditions (16-h light/8-h dark) in a growth room at 22°C. The dyt1-2, tdf1, and ms188 mutants were isolated from EMS mutation lines in the Ler background, then backcrossed to Col-0 more than 10 times, as described by Zhang et al. (2007), Zhu et al. (2008) and Song et al. (2009) and the ams mutant (SALK_152147) was obtained from the Arabidopsis Biological Resource Center (Ohio State University, Columbus, OH, United States) (Zhang et al., 2007; Zhu et al., 2008; Song et al., 2009).
Tissue Preparation
Farmer‘s fixative (Kerk et al., 2003) [75% (v/v) ethanol and 25% (v/v) acetic acid] was freshly prepared and chilled in a -20°C freezer for at least 20 min. In total, 20∼30 inflorescences of 6- to 8-week-old A. thaliana ecotype Columbia-0 were immediately immersed in the fixative solutions in a glass vial and fixed under a soft vacuum for 15 min on ice three times with fresh fixative.
Paraffin-embedding was followed by the microwave method reported by Inada and Wildermuth (2005) with minor modification. The fixative solution in the vial was replaced with a new pre-chilled fixative solution before the microwave method. The tissues was microwaved in the fixative solution at 450 W at 37°C for 15 min three times, each time replaced with a new fixative solution. The fixed samples were dehydrated with 75% ethanol at 67°C for 1 min 15 s, followed by dehydration steps of 1 min 15 s each in 85, 95, 100, and 100% ethanol. Several drops of 1.0% Safranin-O were added to the second 100% ethanol step (Ruzin, 1999). The 100% ethanol was replaced with ethanol: xylene 1:1 followed by 100% xylene with each step microwaved at 70°C for 1 min 30 s. Xylene was subsequently replaced with paraplast, and samples in xylene : paraplast 1:1 were microwaved at 70°C for 10 min. Then, samples were microwaved in 100% paraplast at 70°C for 10 min, then at 70°C for 2.5 h, with paraplast replaced every 30 min. The samples were placed into a Kraft dish filled with 100% new paraplast, and embedded samples were cooled to room temperature. After cooling, the paraplast blocks were stored at 4°C.
Laser Microdissection and Pressure Catapulting (LMPC)
We obtained 8-μm-thick sections by using a rotary microtome (Leica RM2235; Leica Bio-systems Nussloch). To enhance RNA preservation, a paraffin tape transfer system (Instrumedics) was used (Cai and Lashbrook, 2006). Before LMPC, the slides were illuminated for 1 min under an ultraviolent lamp (366 nm) in the paraffin tape transfer system and deparafinized twice in xylene, 10 min each time, and air dried for 2 to 3 h.
Laser microdissection and pressure catapulting involved use of a PALM MicroLaser system (PALM-Zeiss, Bernried, Germany) containing a PALM MicroBeam (driven by PALM MicroBeam software) and a PALM RoboStage and, for high-throughput sample collection, a PALM RoboMover (driven by PALM RoboSoftware v4.6). The slides were then loaded into the PALM Microbeam (Carl Zeiss, Germany). The tapetum cells of Arabidopsis anther on the slides were first selected by using the graphic tools of the PALM RoboSoftware. Then cells were collected by laser pressure, which catapults them into the cap of an AdhesiveCap (Carl Zeiss, Germany). The tapetum cells at stages 6 and 7 were placed into one cap and cells at stages 8, 9, and 10 in another cap. Approximately 1 mm2 (about 2000 cells) of tissue was obtained for each sample per library.
RNA Extraction, Amplification, and RNA-Seq
For the two samples, total RNA was extracted by using a PicoPure RNA isolation kit (Acturus) and subjected to DNase treatment (RNase-free DNA set; Qiagen) according to the manufacturer’s protocol. Next, 10 ng RNA was used for cDNA synthesis and amplification by using an Ovation RNA-seq System V2 kit (NuGen Technologies) following the manufacturer’s protocols with minor modifications. The quality and profile of the RNA and amplified cDNA was assessed by using an RNA 6000 Pico Assay Kit with an Agilent 2100 Bioanalyzer, respectively (Agilent Technologies). The cDNA libraries were then further refined by using the Illumina TruSeq RNA Sample Preparation v2 kit (Illumina) with the low-throughput protocol according to the manufacturer’s instructions. Then samples were paired-end sequenced by using an Illumina HiSeq 2500 platform (Illumina Inc.)1. The average length of the reads was 125 bp.
Read Mapping and Analysis
RNA-seq reads were mapped to the Arabidopsis genome (TAIR102) by using TOPHAT v2.0.9 (Trapnell et al., 2009). Raw reads were preprocessed to remove adaptors using an in-house developed Perl script. After filtering out low-quality (lowest base score < 30) reads using SolexaQA v3.1.3 (Cock et al., 2010). The multi-mapped read correction and fragment bias correction options of Cufflinks v2.1.1 (Trapnell et al., 2010) were used. Gene expression levels were reported as fragments per kilobase of transcripts per million mapped reads [FPKM; (Mortazavi et al., 2008)]. The upper- and lower-bound FPKM values (FPKM_conf_hi and FPKM_conf_lo, respectively) for the 95% confidence interval of each gene were provided by Cufflinks. A gene was defined as expressed in a sample if the FPKM_conf_lo was >0. Differential gene expression between stages 6–7 and stages 8–10 was evaluated using the R package edgeR.
Microarray Analysis
Closed floral buds were collected from wild-type and dyt1, tdf1, ams mutant plants for RNA isolation. Three separate microarrays were performed for each mutant. Total RNA was isolated by use of TRIzol reagent (Invitrogen), and further purified by using the RNeasy Mini kit (Qiagen). Three biological replicates of total RNA were used. Total RNA was labeled by Cy3 and Cy5 with use of the Low RNA Input Linear Amplification and Labeling kit plus (Agilent, 5184-3523). After cRNA labeling, samples were hybridized onto a 44 K Arabidopsis oligo microarray by using the Gene Expression Hybridization kit (Agilent, 5188-5242; Agilent, Santa Clara, CA, United States). Arrays were scanned by using the Agilent G2565BA laser scanner. The microarray data for the ms188 mutant were obtained from a previous study (Zhu et al., 2010). Significance analysis of microarrays (SAM) was used to calculated gene expression values and differentially expressed genes. In this study, the threshold value were fold change > 2, FDR < 0.05, and q-value < 0.05. The delta cut-off of dyt1 was 1.43, while that of tdf1, ams, ms188 was 2.2, 1.55, and 1.47, respectively.
Functional Classification and Categorization of Expression Patterns
MAPMAN analysis was performed as described (Thimm et al., 2004). Then hypergeometric distribution was used to calculate the p value for Gene Ontology (GO) enrichment analyses. Cluster analysis involved use of MEGA3. Heatmap analysis involved use of R4.
Real-time PCR
Real-time PCR analysis was performed as described (Lou et al., 2014). Three biological replicates for each sample and three technical replicates for each gene were used. The relevant primers are in Supplementary Table S19. The β-tubulin gene was used as a positive control.
Results
RNA Sequencing with LMPC Captured Tapetum Cells at Anther Stages 6–7 and 8–10
Tapetum in A. thaliana was identified at anther stage 5 and initiated degradation at stage 10 (Sanders et al., 1999). To identify genes expressed in both early- and later-stage tapetum cells, we used LMPC to capture tapetum cells at anther stages 6–7 and 8–10, respectively (Figures 1A–D). RNAs of the captured tapetum cells were reverse-transcribed to cDNA, then used to make Illumina RNA-seq libraries and sequenced. Over 99% reads could be mapped to the Arabidopsis reference CDS database (99.3% at anther stages 6–7, total reads 53 million; and 99.2% at anther stages 8–10, total reads 50 millions), covered most of the Arabidopsis reference genome (Figures 1E,F). The mapped reads were normalized by using fragments per kilobase per million reads (FPKM) (Trapnell et al., 2010). We used FPKM 95% confidence interval > 0 as the standard for an expressed gene (Zhan et al., 2015). With this criterion, 18,298 genes were expressed in the tapetum at anther stages 6–7 and 18,227 at anther stages 8–10 (Supplementary Data S1, S2). Of these genes, 15,977 (77.8%) genes were expressed both at early and later stages. Most of the known tapetum expressed genes were confirmed in the sequencing data (Supplementary Table S1). With a threshold of 300 FPKM as extremely high-expressing genes, 292 genes were found in the tapetum at anther stages 6–7 and 283 at anther stages 8–10 (Supplementary Data S3, S4). Among those genes, 187 (48.2%) genes were highly expressed both at the two stages. We used GO and MapMan analysis for these genes (Thimm et al., 2004; Usadel et al., 2005). Protein synthesis, transporters, and lipid metabolism genes were highly expressed in the tapetum at both stages 6–7 and 8–10, which indicates a highly dynamic metabolism and transportation system of tapetum for supporting pollen development (Piffanelli et al., 1998; Ariizumi and Toriyama, 2011) (Figures 1G,H and Supplementary Tables S2, S3). At anther stages 8–10, the expression of pectinases, G-protein genes and 1-aminocyclopropane-1-carboxylate synthase 4 (ACS4) were highly upregulated. The primary plant cell wall is a complex mixture of polysaccharides and proteins encasing living plant cells. The pectinases act as glycoside hydrolases for plant cell wall degradation (Cosgrove, 2005; Kirsch et al., 2012). After meiosis, the tapetal cells become a spongy shape with cell wall degradation in wild-type anthers (Zhang et al., 2007; Zhu et al., 2010). The accumulation of pectinases in tapetal cell is consistent with this cytological character. The GTP-binding proteins (G proteins) are conserved signal transduction molecules as cytokinin signaling in animals and plants. In Arabidopsis, mutation in three of extra-large G proteins (XLGs) leads to an abnormally expanded tapetum phenotype, indicating the G proteins were involved in tapetal development (Wang et al., 2017).
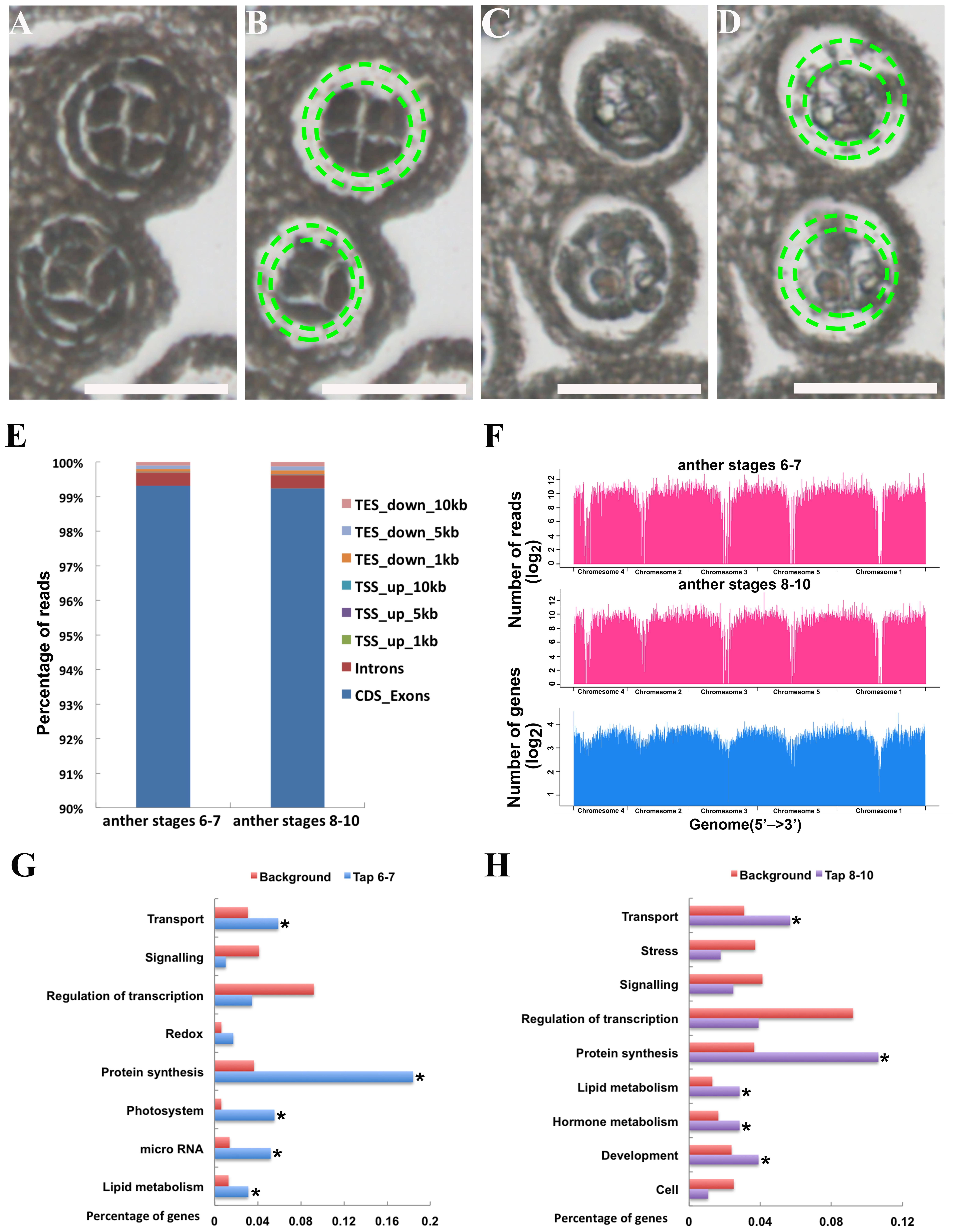
FIGURE 1. RNA sequencing and Gene Ontology (GO) enrichment analysis of transcriptome from laser microdissection and pressure catapulting (LMPC) captured tapetal cells of anther at stages 6–7 and 8–10. (A) Wild-type Col-0 anther at stages 6–7 before LMPC. (B) Wild-type Col-0 anther at stages 6–7 after LMPC, tapetal cells were isolated. (C) Wild-type Col-0 anther at stages 8–10 before LMPC. (D) Wild-type Col-0 anther at stages 8–10 after LMPC, tapetal cells were isolated. (E) Reads distribution in different gene area. Over 99% of the reads were mapped in the CDS_Exons. TES, transcription termination site; TSS, Transcription start site; CDS, coding DNA sequence. (F) Reads distribution in the whole genome, compared with the gene distribution in the whole genome. (G) GO enrichment analysis of tapetum expressed genes at anther stages 6–7. (H) GO enrichment analysis of tapetum expressed genes at anther stages 8–10. Asterisk indicates P values < 0.05 compared with background.
Identification of Tapetum-Expressed Downstream Genes of DYT1, TDF1, AMS, and MS188
Previous investigations showed that the transcription factors DYT1, TDF1, AMS, and MS188 are key regulators in tapetum development (Sorensen et al., 2003; Zhang et al., 2006, 2007; Zhu et al., 2008). As these transcription factors act as the transcriptional activators, we mainly focus on the downregulated genes in current study (Feng et al., 2012; Lou et al., 2014; Xiong et al., 2016). Recently we obtained the transcriptional profiling data of flower buds in these mutants. Significance analysis of microarrays (SAM) (Tusher et al., 2001) was used to identify differentially expressed genes in mutants more than twofold change compared with wild-type. Totally, 2179 genes in dyt1, 1953 genes in tdf1, 1508 genes in ams and 1287 genes in ms188 were identified to be down-regulated, respectively (FDR < 0.05 and q-value < 0.05). To further analyze the transcriptional regulation of tapetum development, we compared the expressed genes in tapetum transcriptome data (FPKM > 2) and the flower buds transcriptional profiling of dyt1, tdf1, ams, and ms188 mutants (Zhang et al., 2006; Zhu et al., 2008; Feng et al., 2012) (Figure 2A). In total, 1289 significantly down-regulated genes in dyt1 were expressed in the tapetum, including 1051 genes that were also down-regulated in tdf1, 825 genes that were affected in dyt1, tdf1 and ams, and 536 genes that were altered in dyt1, tdf1, ams, and ms188 (Figure 2B and Supplementary Data S5). We defined the genes expressed in tapetum and down-regulated in dyt1 but not in tdf1 as DYT1-specific regulated genes, genes down-regulated in both dyt1 and tdf1 but not in ams as TDF1-specific regulated genes. With such a definition, 238 DYT1, 226 TDF1, and 289 AMS specific genes were identified (Figure 2B and Supplementary Data S6–S8). 536 down-regulated genes in all four mutants were defined as common genes (Figure 2B and Supplementary Data S9). The transcriptomes of uninucleate microspores (UNM), bicellular pollen (BCP), immature tricellular pollen (TCP) and mature pollen grains (MPG) from Honys and Twell (2004) were analyzed in a recent study, comparing to the genes expressed in tapetal cells (Supplementary Data S10). We found thousands of microspores expressed genes were not expressed in tapetal cells. We used GO and MapMan to further analyze the DYT1-, TDF1-, AMS- specific and common genes. The subsets of these specific genes indicated that besides the activation of the downstream genes, each transcription factor might regulate several processes individually.
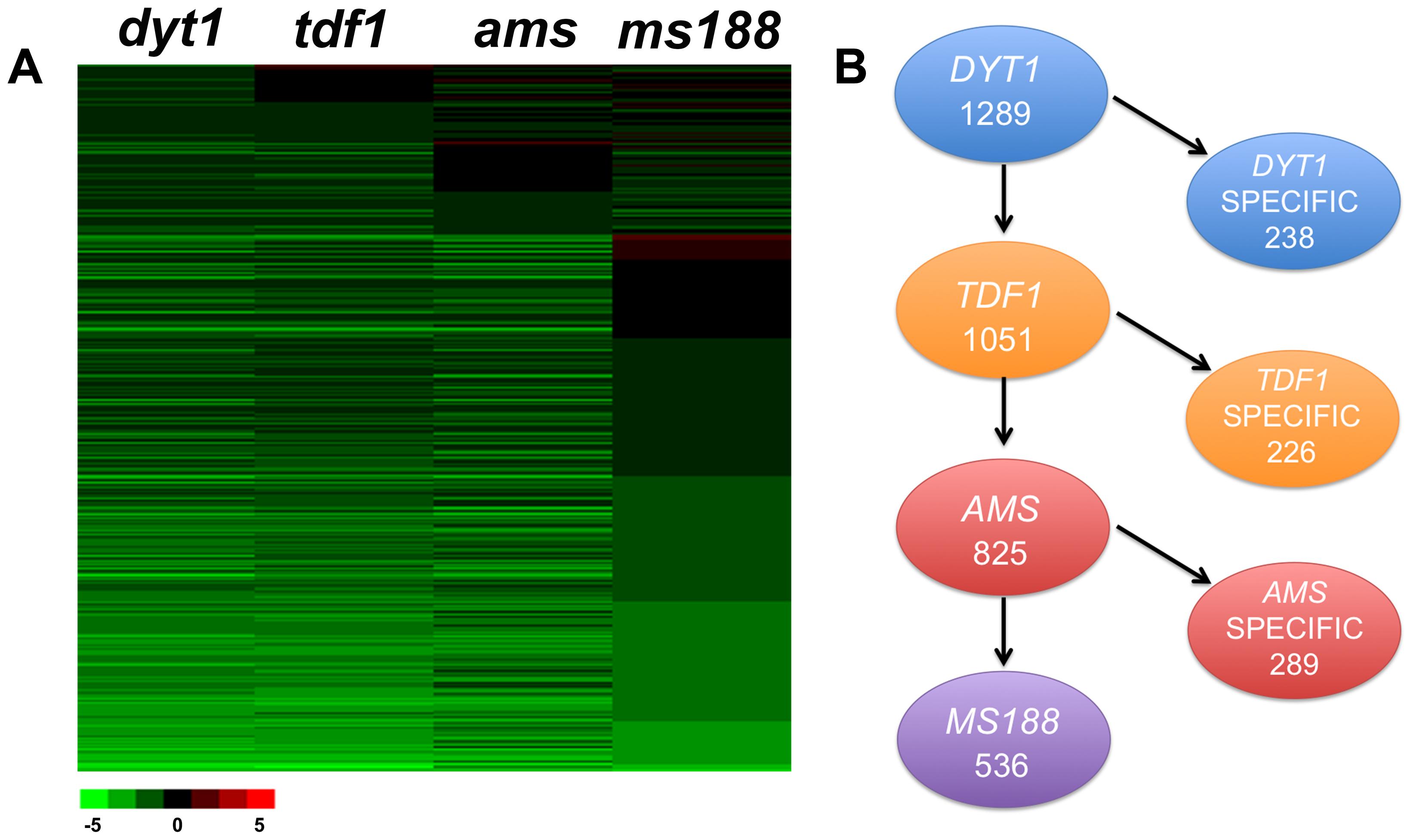
FIGURE 2. Comparative analysis of tapetum expressed genes differentially down-regulated in dyt1, tdf1, ams, and ms188 mutants. (A) Transcription changes of tapetum expressed genes differentially down-regulated in dyt1, tdf1, ams, and ms188 mutants. Green and red colors represent lower and higher log2 fold change expression in mutants compared with the wild type. (B) Subsets of total differentially and commonly expressed genes down-regulated in the four mutants.
Functional Analysis of DYT1-Specific Genes
Among the 1289 down-regulated genes in dyt1, 81.5% (1051) were also down-regulated in tdf1, including most of the lipid metabolism related genes, transporters, and cell wall related genes, indicating TDF1 is the main target of DYT1. The DYT1-specific genes included transcription factors, E3 ubiquitin ligases and transporters (Figure 3A and Supplementary Table S4). DYT1 is the earliest known regulator of the tapetum cell (Zhu et al., 2011). Although DYT1 regulates downstream genes primarily via TDF1 (Gu et al., 2014), the enrichment of transcription factors in DYT1-specific genes indicates that DYT1 might play other important functions for tapetum development independent of TDF1 (Figures 3B,C and Supplementary Table S5). Among them, two bHLH genes, bHLH089 and bHLH010 have been identified as DYT1-specific genes. Previous study showed that bHLH010, bHLH089, and bHLH091 were redundantly required for Arabidopsis anther development. DYT1 was required to activate the expression of these genes (Zhu et al., 2015; Cui et al., 2016). The enrichment of E3 ubiquitin ligases in DYT1-specific genes suggested that they may degrade some proteins to maintain tapetum-cell identity (Figure 3D and Supplementary Table S6). Previous studies has been reported that the transport and signaling of auxin are essential for anther and pollen development (Yang et al., 2013; Cardarelli and Cecchetti, 2014). In the hormone group, auxin-responsive GH3 family gene GH3.3, small auxin upregulated RNA SAUR25 and SAUR30 were enriched (Supplementary Table S7). GH3 and SAUR gene family are primary auxin response gene family (Hagen and Guilfoyle, 2002). DYT1 might affect auxin signal response in tapetum at early stages.
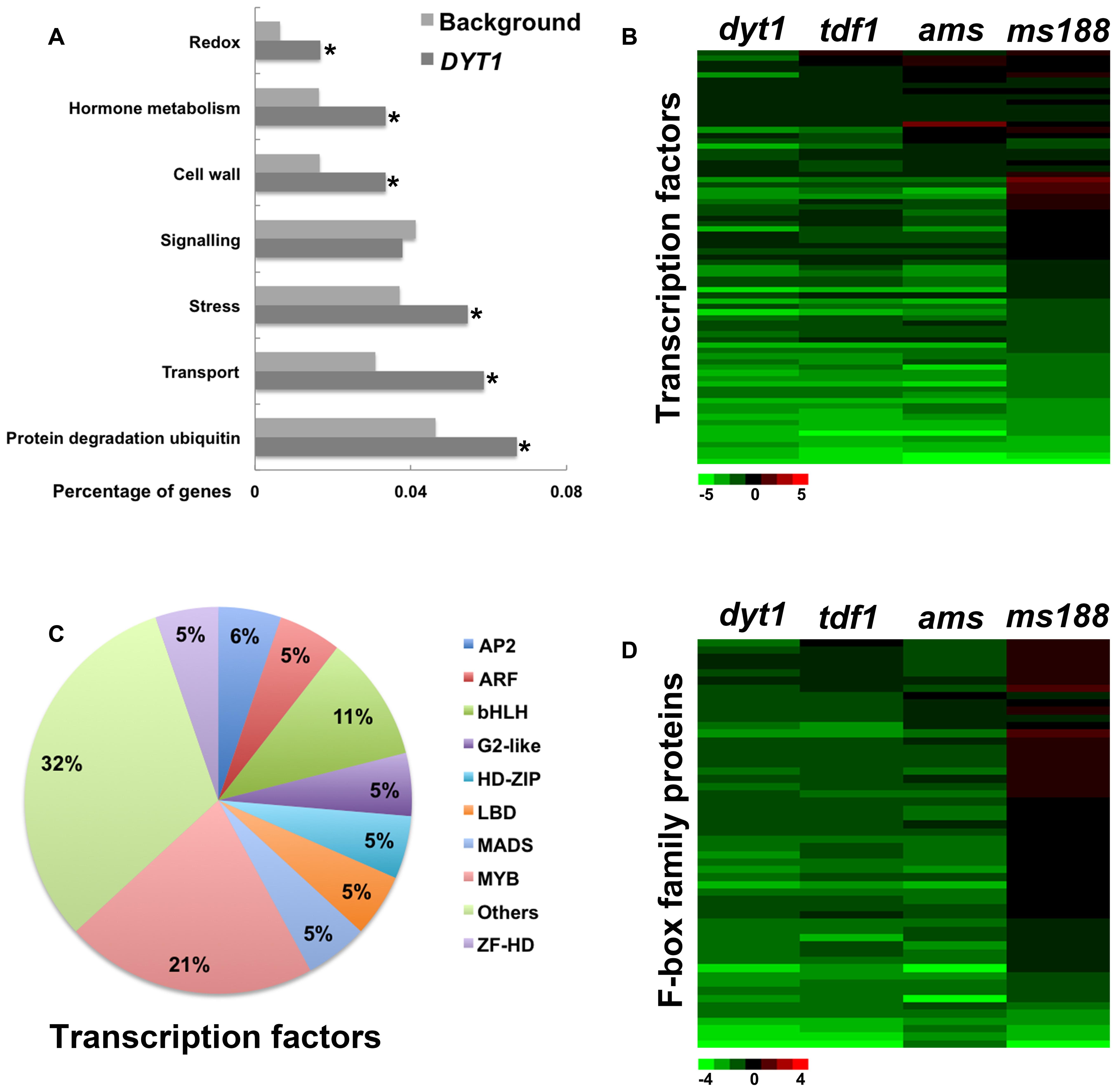
FIGURE 3. Functional analysis of DYT1-specific genes. (A) GO enrichment analysis of DYT1-specific genes. (B) Heat map of mRNA expression profiles of transcription factors of DYT1 in dyt1, tdf1, ams, and ms188 mutants. Green and red colors represent lower and higher log2 fold change of expression in mutants compared with the wild type. (C) Subsets in DYT1-specific transcription factors. (D) Heat map of mRNA expression profiles of F-box family proteins of DYT1 in dyt1, tdf1, ams, and ms188 mutants. Green and red colors represent lower and higher log2 fold change of expression in mutants compared with the wild type. Asterisk indicates P values < 0.05 compared with background.
Functional Analysis of TDF1-Specific Genes
Among the 1051 genes down-regulated in the dyt1 and tdf1 mutants, 825 (78%) were also down-regulated in ams, including most of the lipid-metabolism and cell wall-related genes (Supplementary Data S5). The remaining 226 genes were termed TDF1-specific genes. Transcription factors, protein degradation related genes, redox proteins, cell wall-related genes and transporters were enriched in TDF1-specific genes (Figure 4A and Supplementary Table S8). 11 TDF1-specific transcription factors were identified (Figure 4B and Supplementary Table S5). Tapetum degradation requires temporal control of reactive oxygen species (ROS). NADPH oxidases RESPIRATORYBURST OXIDASE HOMOLOG (RBOH) genes and glutaredoxins (GRX) genes have been proved to regulate tapetum degradation (Xing and Zachgo, 2008; Xie et al., 2014). The tapetum degradation in dyt1 and tdf1 mutants is also abnormal. The vacuolated tapetum cells were enlarged and occupied the locular at anther stage 11 in dyt1 and tdf1 mutants, suggested the irregular PCD process of them (Zhang et al., 2006; Zhu et al., 2008, 2011). Plant glutathione-S-transferase (GST) proteins can protect tissues from oxidative damage (Marrs, 1996). Peroxidases are important for the redox regulation in plants (Apel and Hirt, 2004). Catalases has been reported to be an major type of ROS-scavenging enzymes (Mittler et al., 2004). All GSTs and catalases down-regulated in dyt1 were also TDF1-specific genes (Supplementary Table S9). There were five peroxidases down-regulated in dyt1, and three of them were also TDF1-specific genes (Supplementary Figure S1 and Table S9). Thus TDF1 might functional in maintaining the redox balance in tapetum. The cysteine protease is involved in tapetum PCD and pollen development (Zhang et al., 2014). Metacaspases have been proposed to be involved in regulating PCD (Yang et al., 2014; Zhang et al., 2014; Fagundes et al., 2015; Daneva et al., 2016). We also found metacaspases and cysteine proteases enriched in TDF1-specific genes (Supplementary Table S9). These proteins may play a role in tapetum PCD. Several reports indicated that calcium-binding proteins contribute to tapetum development and degradation (Falasca et al., 2013; Wei et al., 2015; Monihan et al., 2016). Genes involved in calcium signaling were also enriched in TDF1-specific genes (Figures 4D and Supplementary Table S10). The leucine-rich repeat receptor-like kinases have been reported to be involved in anther somatic layer differentiation including tapetum in male gametogenesis (Zhao et al., 2002; Albrecht et al., 2005; Mizuno et al., 2007). Receptor kinases and protein kinases/phosphatases were enriched, so TDF1 might regulate tapetum signaling communication (Figures 4E and Supplementary Table S11). Pectin methylesterases (PMEs) remove the methylesterase of pectin. PMEs are antagonistically regulated by PME inhibitors (PMEIs) (Levesque-Tremblay et al., 2015). Most of the down-regulated PMEIs in dyt1 mutant were TDF1-specific genes (Figure 4C), indicate that TDF1 might also regulate the pectin methylesterase in tapetum cell wall.
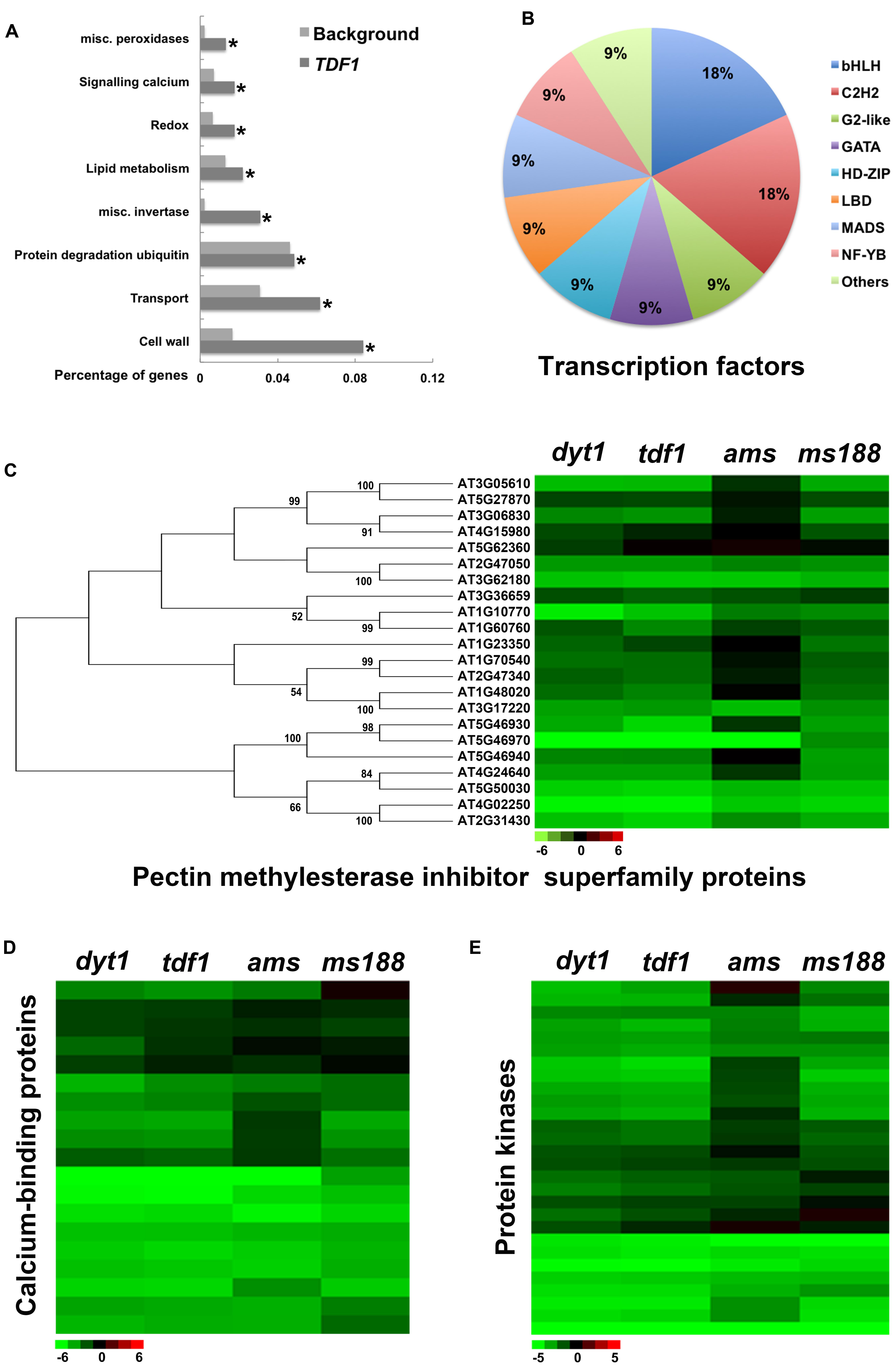
FIGURE 4. Functional analysis of TDF1-specific genes. (A) GO enrichment analysis of TDF1-specific genes. (B) Subsets of transcription factors in TDF1-specific genes. (C) Cluster and heat map of mRNA expression profiles of pectin methylesterase inhibitor superfamily proteins of TDF1 in dyt1, tdf1, ams, and ms188 mutants. (D) Heat map of mRNA expression profiles of calcium-binding proteins of TDF1 in dyt1, tdf1, ams, and ms188 mutants. Green and red colors represent lower and higher log2 fold change of expression in mutants compared with the wild type. (E) Heat map of mRNA expression profiles of protein kinases of TDF1 in dyt1, tdf1, ams and ms188 mutants. Asterisk indicates P values < 0.05 compared with background.
Functional Analysis of AMS-Specific Genes
Among the 825 genes downregulated in the tapetum of dyt1, tdf1, and ams mutants, 65% (536) were also downregulated in ms188. Therefore, MS188 is the major regulator under AMS. However, the expression of MS188 in ams cannot rescue its male-sterility phenotype (Xiong et al., 2016). Therefore, AMS-specific genes are also important for tapetum development. AMS-specific genes contain few cell-wall, hormone-metabolism and transcription-factor genes (Figures 5A,B and Supplementary Table S12). E3 ubiquitin ligases were highly enriched (Figure 3D). Among all the 53 F-box E3 ligases in dyt1, 43% were AMS-specific genes. These E3 ligases might function in tapetum degradation. Thus the expression of AMS might transfer the tapetum cell fate into cell death by terminating several key factors via protein degradation. Type III lipid transfer proteins (LTPs) are expressed in the tapetum and function in pollen exine formation (Huang et al., 2013). Lipid metabolism-related genes were enriched in AMS-specific genes. We found 15 genes coding for LTPs were down-regulated in dyt1. Six of them were AMS-specific genes, including the three type III LTPs. Some other LTPs were MS188-specific genes (Figure 5C and Supplementary Table S13). Further qRT-PCR analysis confirmed these results (Figure 5D). The expression of LTPs might be mainly regulated by AMS and MS188. As well, lipid transfer might start at the AMS stage, which might function in preparation for pollen wall formation.
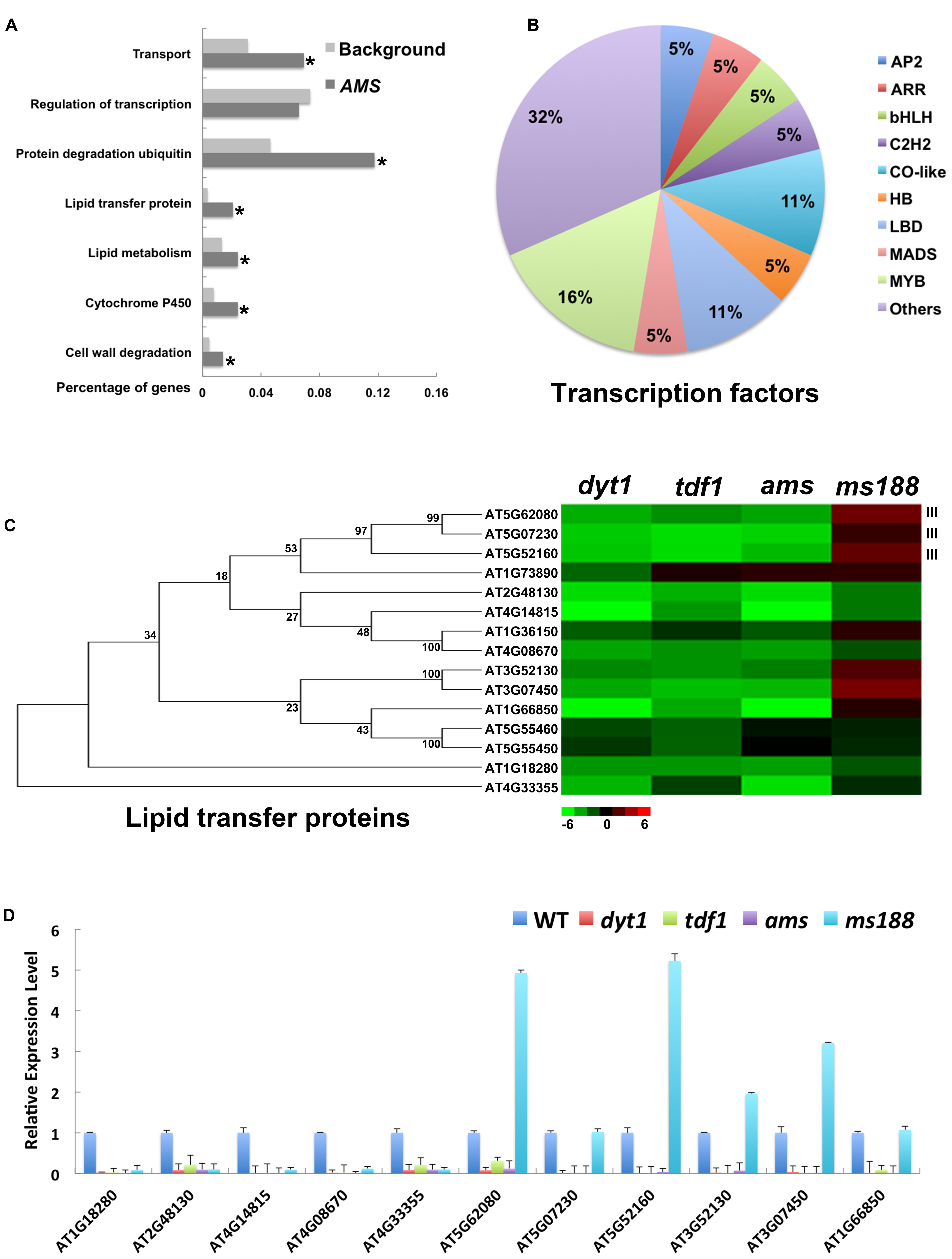
FIGURE 5. Functional analysis of AMS-specific genes. (A) GO enrichment analysis of AMS-specific genes. (B) Subsets of transcription factors in AMS-specific genes. (C) Heat map of mRNA expression profiles of lipid transfer proteins of AMS in dyt1, tdf1, ams, and ms188 mutants. Green and red colors represent lower and higher log2 fold changes of expression in mutants compared with the wild type. (D) Q-RT-PCR analysis of the lipid transfer proteins. Asterisk indicates P values < 0.05 compared with background.
Functional Analysis of the Common Genes
Among the 536 genes down-regulated in all the four mutants, cell-wall related genes were highly enriched and E3 ubiquitin ligases were reduced (Figure 6A and Supplementary Table S14). At late stage of anther development, tapetum cell wall is degraded, and MS188 is important for this degradation (Zhang et al., 2007). Pectate lyases and polygalacturonases (PGs), and xyloglucan endo-transglucosylases are typical cell wall hydrolytic enzymes (Carpita and McCann, 2000; Kim et al., 2006). These proteins contributed to 36% of all cell wall related genes (Figure 6B and Supplementary Table S15). They may be responsible for tapetum cell wall degradation. Arabinogalactan proteins (AGPs), and fasciclin-like AGPs (FLAs) function in pollen wall formation (Li H. et al., 2010; Li J. et al., 2010; MacMillan et al., 2010; Tan et al., 2013; Jia et al., 2015; Showalter and Basu, 2016). We found AGPs and FLAs enriched in common cell wall genes (Figure 6B). AGPs are essential for TEK-mediated pollen wall formation (Jia et al., 2015). TEK is highly expressed in the tapetum at stage 7 and greatly reduced at stage 8 (Lou et al., 2014). AGPs were also down-regulated in ms188, so AGPs might contribute to the pollen wall formation after stage 7, and MS188 might regulate the transcription of AGPs at later stages. Cytochrome P450 is a superfamily of heme-containing proteins that catalyze NADPH and O2-dependent monooxygenase (Chapple, 1998; Coon, 2005). Several cytochrome P450s were reported to contribute to the development of pollen wall (Morant and Bak, 2007; Dobritsa et al., 2009; Li H. et al., 2010; Amrad et al., 2016; Xiong et al., 2016). Here we found 17 cytochrome P450s down-regulated in dyt1 in the tapetum, and most of them were common genes (Figure 6C and Supplementary Table S16). MS188 functions in both degradation of the tapetum cell wall and formation of the pollen wall (Zhou et al., 2015; Xu et al., 2016). Further qRT-PCR analysis confirmed these results (Figure 6D). Many transcription factors, especially zinc finger and LOB domain-containing protein (LBD) family transcription factors were common genes (Figure 6E and Supplementary Table S5). The receptor kinases, calcium-related proteins, and G-proteins were still enriched in common genes. Rapid alkalization factor (RALF) proteins are peptides that function to regulate plant development (Sharma et al., 2016). We also found RALF proteins enriched in MS188-specific genes (Supplementary Figure S2). The tapetum might be both a target and a source of peptide signaling at the MS188 stage.
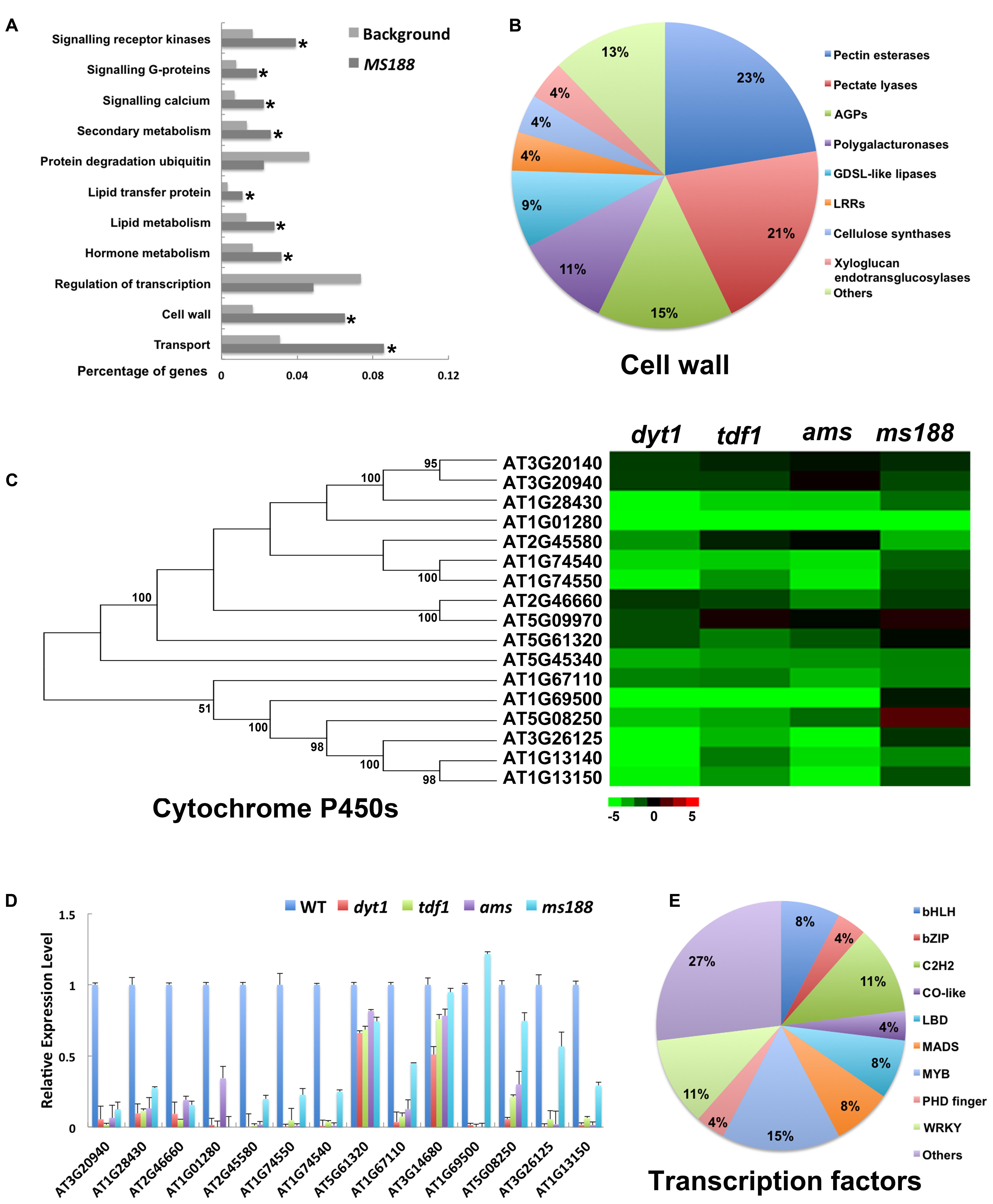
FIGURE 6. Functional analysis of commonly expressed genes down-regulated in the four mutants. (A) GO enrichment analysis of common genes. (B) Subsets of cell wall related genes in common genes. (C) Heat map of mRNA expression profiles of cytochrome P450 family genes in dyt1, tdf1, ams, and ms188 mutants. (D) Q-RT-PCR analysis of the cytochrome P450 family genes. (E) Subsets of transcription factors in common genes. Asterisk indicates P values < 0.05 compared with background.
Discussion
During anther development, tapetum provides nutrition for pollen development and materials for pollen wall formation. It only lasts for several days from its differentiation from inner secondary parietal cells to its completely degradation of PCD (Feng and Dickinson, 2010a). In Arabidopsis, DYT1-TDF1-AMS-MS188 form a genetic pathway for tapetum development and pollen wall formation (Zhu et al., 2011). After laser microdissection and sequencing of tapetum cells, and integration of transcriptional profiling of dyt1, tdf1, ams, and ms188 mutants, we identified reliable tapetum expressed genes, and specific genes regulated by these transcription factors (Figure 7). Although only a subset of the specific genes for each transcription factor are likely to be direct targets of the TFs, these informations provide novel understandings of the DYT1-TDF1-AMS-MS188 pathway in a highly ordered and precisely regulated network for tapetum and pollen development.
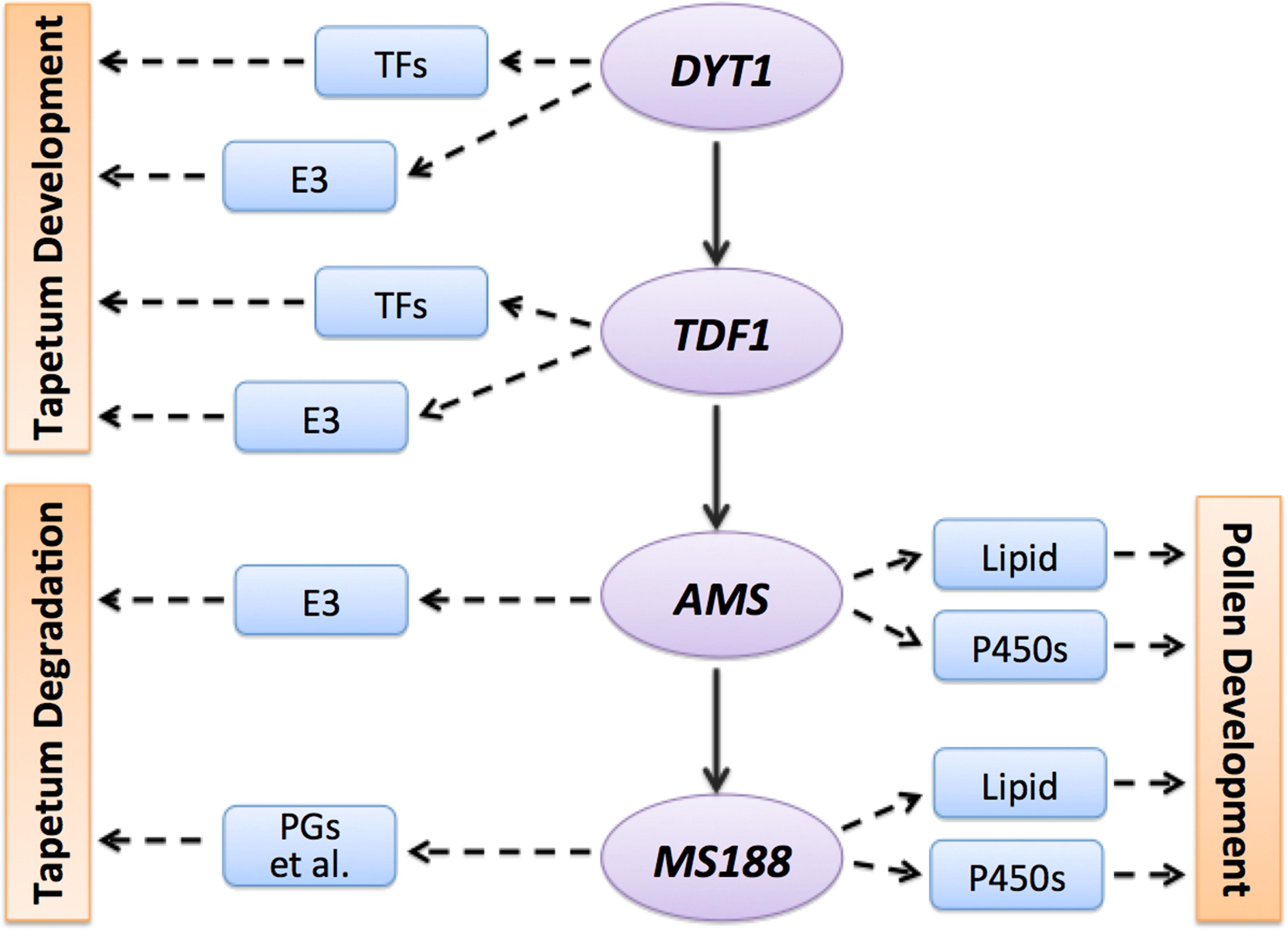
FIGURE 7. A working model of DYT1-TDF1-AMS-MS188 pathway. Combined of our analysis, here we hypothesis a simple model of how different transcription factor regulate the tapetum and pollen development. TFs, transcription factors; E3, E3 ubiquitin ligases; P450s, cytochrome P450 genes; PGs, polygalacturonases.
In this work, we found that 536 tapetum genes were common downstream genes of all the four transcription factors, and each factor regulated hundreds of specific genes independent of the downstream components of the pathway. The down-regulated transcriptional data of tapetum genes were highly similar between dyt1 and tdf1 mutant (81.5%); tdf1 and ams mutant (78%), ams and ms188 mutant (65%). This indicates that these transcription factors play their functions mainly through downstream key regulators for tapetum development. However, each transcription factor should play some specific functions as the downstream genes could not rescue the pollen formation (Gu et al., 2014; Xiong et al., 2016). Besides the common regulated genes, there were 238 DYT1-specific genes, 226 TDF1-specific genes, 289 AMS-specific genes. The identification of these specific genes is helpful to understand the specific functions of these regulators. Of the 74 transcription factors down-regulated in dyt1 mutant, 19 were DYT1-specific genes, 11 were TDF1-specific genes, and 19 were AMS-specific genes (Supplementary Table S5). This indicates that besides the primary targets, each factor might regulate other TFs for tapetum development. E3 ubiquitin ligase is widely reported to degrade proteins during plant development. In Arabidopsis, E3 ligase PUB4 was reported to involve in tapetal cell development (Wang et al., 2013). DYT1, TDF1, and AMS are early regulators for tapetum development. All these transcription factors regulate some specific E3 ligases (Supplementary Table S6). E3 protein-degradation system may degrade some of the former-stage proteins to maintain tapetum cell identity at a specific stage to ensure successful tapetum development. AMS is critical for tapetum development as the ams tapetum cell is enlarged to occupy the anther locule (Sorensen et al., 2003). Most of the E3 ligase were AMS-specific genes (Figure 3D). It’s likely that AMS plays the role in determining the tapetum cell fate via this E3 ligase protein degradation. Many receptor kinases are important regulators in Arabidopsis tapetum and pollen development (Zhao et al., 2002; Hord et al., 2006), which suggests that cell–cell signaling events are critical for tapetum and pollen development (Feng and Dickinson, 2010b). We found 26 receptor kinases down-regulated in dyt1 mutant, 3 of them were DYT1-specific genes, 2 of them were TDF1-specific genes, 21 of them were common genes (Supplementary Table S17). We also found RALF type peptides were common genes (Supplementary Figure S2). These suggest that tapetum cell keeps the cell-cell communication during different development stage. Genes functioned in transport were differentially and commonly expressed in these mutants (Supplementary Table S18), indicating these factors controls a highly ordered transport system in tapetum for pollen development. Therefore, tapetum cells evolve a complicated gene regulatory network for its ordered development and finally PCD while efficiently communicates with other plant cells for pollen formation.
Accession Number
Microarray data from this article can be found in Gene Expression Omnibus (GEO) under the accession number GSE102528. The RNA-Seq data for tapetal cells of Arabidopsis stages 6–7 anthers and stages 8–10 anthers have been deposited in Sequence Read Archive (SRA) repository under the accession number SRS2426392 and SRS2426393.
Author Contributions
The experiments were conceived and designed by: Z-NY. The experiments were performed by: D-DL, JZ. The data were analyzed by: D-DL, J-SX, and JZ. The paper was written by: J-SX, D-DL, and Z-NY.
Conflict of Interest Statement
The authors declare that the research was conducted in the absence of any commercial or financial relationships that could be construed as a potential conflict of interest.
Acknowledgments
We thank Dr. Wei-Hua Tang, Dong Zhang, Ting-Lu Yuan at the Shanghai Institutes for Biological Sciences at the Chinese Academy of Sciences for their help in the methods of tissue preparation and LMPC. This work was supported by the grant from the National Science Foundation of China (31670314).
Supplementary Material
The Supplementary Material for this article can be found online at: http://journal.frontiersin.org/article/10.3389/fpls.2017.01559/full#supplementary-material
FIGURE S1 | Heat map of mRNA expression profiles of ralf-like genes of MS188 in dyt1, tdf1, ams, and ms188 mutants.
FIGURE S2 | Heat map of mRNA expression profiles of peroxidases of TDF1 in dyt1, tdf1, ams, and ms188 mutants.
Footnotes
- ^ http://www.illumina.com/
- ^ http://www.arabidopsis.org
- ^ http://www.megasoftware.net/
- ^ https://www.r-project.org/
References
Albrecht, C., Russinova, E., Hecht, V., Baaijens, E., and de Vries, S. (2005). The Arabidopsis thaliana SOMATIC EMBRYOGENESIS RECEPTOR-LIKE KINASES1 and 2 control male sporogenesis. Plant Cell 17, 3337–3349. doi: 10.1105/tpc.105.036814
Amrad, A., Moser, M., Mandel, T., de Vries, M., Schuurink, R. C., Freitas, L., et al. (2016). Gain and loss of floral scent production through changes in structural genes during pollinator-mediated speciation. Curr. Biol. 26, 3303–3312. doi: 10.1016/j.cub.2016.10.023
Apel, K., and Hirt, H. (2004). Reactive oxygen species: metabolism, oxidative stress, and signal transduction. Annu. Rev. Plant Biol. 55, 373–399. doi: 10.1146/annurev.arplant.55.031903.141701
Ariizumi, T., and Toriyama, K. (2011). Genetic regulation of sporopollenin synthesis and pollen exine development. Annu. Rev. Plant Biol. 62, 437–460. doi: 10.1146/annurev-arplant-042809-112312
Burgemeister, R., Gangnus, R., Haar, B., Schütze, K., and Sauer, U. (2003). High quality RNA retrieved from samples obtained by using LMPC (laser microdissection and pressure catapulting) technology. Pathol. Res. Pract. 199, 431–436. doi: 10.1078/0344-0338-00442
Cai, C. F., Zhu, J., Lou, Y., Guo, Z. L., Xiong, S. X., Wang, K., et al. (2015). The functional analysis of OsTDF1 reveals a conserved genetic pathway for tapetal development between rice and Arabidopsis. Sci. Bull. 60, 1073–1082. doi: 10.1007/s11434-015-0810-3
Cai, S., and Lashbrook, C. C. (2006). Laser capture microdissection of plant cells from tape-transferred paraffin sections promotes recovery of structurally intact RNA for global gene profiling. Plant J. 48, 628–637. doi: 10.1111/j.1365-313X.2006.02886.x
Cardarelli, M., and Cecchetti, V. (2014). Auxin polar transport in stamen formation and development: how many actors? Front. Plant Sci. 5:333. doi: 10.3389/fpls.2014.00333
Carpita, N., and McCann, M. C. (2000). “The cell wall,” in Biochemistry & Molecular Biology of Plants, eds B. B. Buchanan, G. Wilhelm, and R. L. Jones (Chichester: John Wiley & Sons Ltd. Press), 52–108.
Chapple, C. (1998). Molecular-genetic analysis of plant Cytochrome P450-dependent monooxygenases. Annu. Rev. Plant Physiol. Plant Mol. Biol. 49, 311–343. doi: 10.1146/annurev.arplant.49.1.311
Cock, P. J. A., Fields, C. J., Goto, N., Heuer, M. L., and Rice, P. M. (2010). The Sanger FASTQ file format for sequences with quality scores, and the Solexa/Illumina FASTQ variants. Nucleic Acids Res. 38, 1767–1771. doi: 10.1093/nar/gkp1137
Coon, M. J. (2005). Cytochrome P450: nature’s most versatile biological catalyst. Annu. Rev. Pharmacol. Toxicol. 45, 1–25. doi: 10.1146/annurev.pharmtox.45.120403.100030
Cosgrove, D. J. (2005). Growth of the plant cell wall. Nat. Rev. Mol. Cell Biol. 6, 850–861. doi: 10.1038/nrm1746
Cui, J., You, C. J., Zhu, E. G., Huang, Q., Ma, H., and Chang, F. (2016). Feedback regulation of DYT1 by interactions with downstream bHLH factors promotes DYT1 nuclear localization and anther development. Plant Cell 28, 1078–1093. doi: 10.1105/tpc.15.00986
Daneva, A., Gao, Z., Van Durme, M., and Nowack, M. K. (2016). Functions and regulation of programmed cell death in plant development. Annu. Rev. Cell Dev. Biol. 32, 441–468. doi: 10.1146/annurev-cellbio-111315-124915
Dobritsa, A. A., Shrestha, J., Morant, M., Pinot, F., Matsuno, M., Swanson, R., et al. (2009). CYP704B1 is a long-chain fatty acid ω-hydroxylase essential for sporopollenin synthesis in pollen of Arabidopsis. Plant Physiol. 151, 574–589. doi: 10.1104/pp.109.144469
Fagundes, D., Bohn, B., Cabreira, C., Leipelt, F., Dias, N., Bodanesezanettini, M. H., et al. (2015). Caspases in plants: metacaspase gene family in plant stress responses. Funct. Integr. Genomics 15, 639–649. doi: 10.1007/s10142-015-0459-7
Falasca, G., D’Angeli, S., Biasi, R., Fattorini, L., Matteucci, M., Canini, A., et al. (2013). Tapetum and middle layer control male fertility in Actinidia deliciosa. Ann. Bot. 112, 1045–1055. doi: 10.1093/aob/mct173
Feng, B. M., Lu, D. H., Ma, X., Peng, Y. B., Sun, Y. J., Ning, G., et al. (2012). Regulation of the Arabidopsis anther transcriptome by DYT1 for pollen development. Plant J. 72, 612–624. doi: 10.1111/j.1365-313X.2012.05104.x
Feng, X. Q., and Dickinson, H. G. (2010a). Tapetal cell fate, lineage and proliferation in the Arabidopsis anther. Development 137, 2409–2416. doi: 10.1242/dev.049320
Feng, X. Q., and Dickinson, H. G. (2010b). Cell-cell interactions during patterning of the Arabidopsis anther. Biochem. Soc. Trans. 38, 571–576. doi: 10.1042/BST0380571
Ferguson, A. C., Pearce, S., Band, L. R., Yang, C., Ferjentsikova, I., King, J., et al. (2017). Biphasic regulation of the transcription factor ABORTED MICROSPORES (AMS) is essential for tapetum and pollen development in Arabidopsis. New Phytol. 213, 778–790. doi: 10.1111/nph.14200
Goldberg, R. B., Beals, T. P., and Sanders, P. M. (1993). Anther development: basic principles and practical applications. Plant Cell 5, 1217–1229. doi: 10.1105/tpc.5.10.1217
Gu, J. N., Zhu, J., Yu, Y., Teng, X. D., Lou, Y., Xu, X. F., et al. (2014). DYT1 directly regulates the expression of TDF1 for tapetum development and pollen wall formation in Arabidopsis. Plant J. 80, 1005–1013. doi: 10.1111/tpj.12694
Hagen, G., and Guilfoyle, T. (2002). Auxin-responsive gene expression: genes, promoters and regulatory factors. Plant Mol. Biol. 49, 373–385.
Higginson, T., Li, S. F., and Parish, R. W. (2003). AtMYB103 regulates tapetum and trichome development in Arabidopsis thaliana. Plant J. 35, 177–192. doi: 10.1046/j.1365-313X.2003.01791.x
Honys, D., and Twell, D. (2004). Transcriptome analysis of haploid male gametophyte development in Arabidopsis. Genome Biol. 5:R85. doi: 10.1186/gb-2004-5-11-r85
Hord, C. L. H., Chen, C., Deyoung, B. J., Clark, S. E., and Ma, H. (2006). The BAM1/BAM2 receptor-like kinases are important regulators of Arabidopsis early anther development. Plant Cell 18, 1667–1680. doi: 10.1105/tpc.105.036871
Huang, M. D., Chen, T. L., and Huang, A. H. (2013). Abundant type III lipid transfer proteins in Arabidopsis tapetum are secreted to the locule and become a constituent of the pollen exine. Plant Physiol. 163, 1218–1229. doi: 10.1104/pp.113.225706
Inada, N., and Wildermuth, M. C. (2005). Novel tissue preparation method and cell-specific marker for laser microdissection of Arabidopsis mature leaf. Planta 221, 9–16. doi: 10.1007/s00425-004-1427-y
Jia, Q. S., Zhu, J., Xu, X. F., Lou, Y., Zhang, Z. L., Zhang, Z. P., et al. (2015). Arabidopsis AT-hook protein TEK positively regulates the expression of arabinogalactan proteins for Nexine formation. Mol. Plant 8, 251–260. doi: 10.1016/j.molp.2014.10.001
Kerk, N. M., Ceserani, T., Tausta, S. L., Sussex, I. M., and Nelson, T. M. (2003). Laser capture microdissection of cells from plant tissues. Plant Physiol. 132, 27–35. doi: 10.1104/pp.102.018127.1
Kirsch, R., Wielsch, N., Vogel, H., Svatoš, A., Heckel, D. G., and Pauchet, Y. (2012). Combining proteomics and transcriptome sequencing to identify active plant-cell-wall-degrading enzymes in a leaf beetle. BMC Genomics 13:587. doi: 10.1186/1471-2164-13-587
Kim, J., Shiu, S. H., Thoma, S., Li, W. H., and Patterson, S. E. (2006). Patterns of expansion and expression divergence in the plant polygalacturonase gene family. Genome Biol. 7:R87. doi: 10.1186/gb-2006-7-9-r87
Levesque-Tremblay, G., Pelloux, J., Braybrook, S. A., and Müller, K. (2015). Tuning of pectin methylesterification: consequences for cell wall biomechanics and development. Planta 242, 791–811. doi: 10.1007/s00425-015-2358-5
Li, H., Pinot, F., Sauveplane, V., Werck-Reichhart, D., Diehl, P., Schreiber, L., et al. (2010). Cytochrome P450 family member CYP704B2 catalyzes the w-hydroxylation of fatty acids and is required for anther cutin biosynthesis and pollen exine formation in rice. Plant Cell 22, 173–190. doi: 10.1105/tpc.109.070326
Li, J., Yu, M., Geng, L. L., and Zhao, J. (2010). The fasciclin-like arabinogalactan protein gene, FLA3, is involved in microspore development of Arabidopsis. Plant J. 64, 482–497. doi: 10.1111/j.1365-313X.2010.04344.x
Lou, Y., Xu, X. F., Zhu, J., Gu, J. N., Blackmore, S., and Yang, Z. N. (2014). The tapetal AHL family protein TEK determines nexine formation in the pollen wall. Nat. Commun. 5:3855. doi: 10.1038/ncomms4855
MacMillan, C. P., Mansfield, S. D., Stachurski, Z. H., Evans, R., and Southerton, S. G. (2010). Fasciclin-like arabinogalactan proteins: specialization for stem biomechanics and cell wall architecture in Arabidopsis and Eucalyptus. Plant J. 62, 689–703. doi: 10.1111/j.1365-313X.2010.04181.x
Mariani, C., Debeuckeleer, M., Truettner, J., Leemans, J., and Goldberg, R. B. (1990). Induction of male-sterility in plants by a chimeric ribonuclease gene. Nature 347, 737–741. doi: 10.1038/347737a0
Marrs, K. A. (1996). The functions and regulation of glutathione S-transferases in plants. Annu. Rev. Plant Biol. 47, 127–158. doi: 10.1146/annurev.arplant.47.1.127
Mittler, R., Vanderauwera, S., Gollery, M., and Frank, V. B. (2004). Reactive oxygen gene network of plants. Trends Plant Sci. 9, 490–498. doi: 10.1016/j.tplants.2004.08.009
Mizuno, S., Osakabe, Y., Maruyama, K., Ito, T., Osakabe, K., Sato, T., et al. (2007). Receptor-like protein kinase 2 (RPK 2) is a novel factor controlling anther development in Arabidopsis thaliana. Plant J. 50, 751–766. doi: 10.1111/j.1365-313X.2007.03083.x
Monihan, S. M., Magness, C. A., Yadegari, R., Smith, S. E., and Schumaker, K. S. (2016). Arabidopsis CALCINEURIN B-LIKE10 functions independently of the SOS pathway during reproductive development in saline conditions. Plant Physiol. 171, 369–379. doi: 10.1104/pp.16.00334
Morant, M., and Bak, S. (2007). CYP703 is an ancient Cytochrome P450 in land plants catalyzing in-chain hydroxylation of lauric acid to provide building blocks for sporopollenin synthesis in pollen. Plant Cell 19, 1473–1487. doi: 10.1105/tpc.106.045948
Mortazavi, A., Williams, B. A., McCue, K., Schaeffer, L., and Wold, B. (2008). Mapping and quantifying mammalian transcriptomes by RNA-Seq. Nat. Methods 5, 621–628. doi: 10.1038/nmeth.1226
Owen, H. A., and Makaroff, C. A. (1995). Ultrastructure of microsporogenesis and microgametogenesis in Arabidopsis thaliana (L.) Heynh. ecotype Wassilewskija (Brassicaceae). Protoplasma 185, 7–21. doi: 10.1007/BF01272749
Piffanelli, P., Ross, J. H. E., and Murphy, D. J. (1998). Biogenesis and function of the lipidic structures of pollen grains. Plant Reprod. 11, 65–80. doi: 10.1007/s004970050122
Sanders, P. M., Bui, A. Q., Weterings, K., Mcintire, K. N., Hsu, Y. C., Pei, Y. L., et al. (1999). Anther developmental defects in Arabidopsis thaliana male-sterile mutants. Sex. Plant Reprod. 11, 297–322. doi: 10.1007/s004970050158
Sharma, A., Hussain, A., Mun, B. G., Imran, Q. M., Falak, N., Lee, S. U., et al. (2016). Comprehensive analysis of plant rapid alkalization factor (RALF) genes. Plant Physiol. Biochem. 106, 82–90. doi: 10.1016/j.plaphy.2016.03.037
Showalter, A. M., and Basu, D. (2016). Extensin and arabinogalactan-protein biosynthesis: glycosyltransferases, research challenges, and biosensors. Front. Plant Sci. 7:814. doi: 10.3389/fpls.2016.00814
Song, Y., Li, H., Shi, Q. L., Jiang, H., Chen, H., Zhong, X. L., et al. (2009). The Arabidopsis bHLH transcription factor DYT1 is essential for anther development by regulating callose dissolution. J. Shanghai Normal Univ. Nat. Sci. 38, 174–182. doi: 10.3969/j.issn.1000-5137.2009.02.011
Sorensen, A. M., Krober, S., Unte, U. S., Huijser, P., Dekker, K., and Saedler, H. (2003). The Arabidopsis ABORTED MICROSPORES (AMS) gene encodes a MYC class transcription factor. Plant J. 33, 413–423. doi: 10.1046/j.1365-313X.2003.01644.x
Tan, L., Eberhard, S., Pattathil, S., Warder, C., Glushka, J., Yuan, C., et al. (2013). An Arabidopsis cell wall proteoglycan consists of pectin and arabinoxylan covalently linked to an arabinogalactan protein. Plant Cell 25, 270–287. doi: 10.1105/tpc.112.107334
Thimm, O., Blasing, O., Gibon, Y., Nagel, A., Meyer, S., Kruger, P., et al. (2004). MAPMAN: a user-driven tool to display genomics data sets onto diagrams of metabolic pathways and other biological processes. Plant J. 37, 914–939. doi: 10.1111/j.1365-313X.2004.02016.x
Trapnell, C., Pachter, L., and Salzberg, S. L. (2009). TopHat: discovering splice junctions with RNA-Seq. Bioinformatics 25, 1105–1111. doi: 10.1093/bioinformatics/btp120
Trapnell, C., Williams, B. A., Pertea, G., Mortazavi, A., Kwan, G., van Baren, M. J., et al. (2010). Transcript assembly and quantification by RNA-Seq reveals unannotated transcripts and isoform switching during cell differentiation. Nat. Biotechnol. 28, 511–515. doi: 10.1038/nbt.1621
Tusher, V. G., Tibshirani, R., and Chu, G. (2001). Significance analysis of microarrays applied to the ionizing radiation response. Proc. Natl. Acad. Sci. U.S.A. 98, 5116–5121.
Usadel, B., Nagel, A., Thimm, O., Redestig, H., Blaesing, O. E., Palacios-Rojas, N., et al. (2005). Extension of the visualization tool MapMan to allow statistical analysis of arrays, display of corresponding genes, and comparison with known responses. Plant Physiol. 138, 1195–1204. doi: 10.1104/pp.105.060459
Wang, H., Lu, Y., Jiang, T., Berg, H., Li, C., and Xia, Y. (2013). The Arabidopsis U-box/ARM repeat E3 ligase AtPUB4 influences growth and degeneration of tapetal cells, and its mutation leads to conditional male sterility. Plant J. 74, 511–523. doi: 10.1111/tpj.12146
Wang, Y. P., Wu, Y. Y., Yu, B. Y., Yin, Z., and Xia, Y. (2017). Extra-large G proteins interact with E3 ligases PUB4 and PUB2 and function in cytokinin and developmental processes. Plant Physiol. 173, 1235–1246. doi: 10.1104/pp.16.00816
Wei, D. M., Gao, C., and Yuan, D. Y. (2015). Calcium distribution during anther development in oil tea (Camellia oleifera Abel.). J. Am. Soc. Hortic. Sci. 140, 88–93.
Xie, H. T., Zhang, Y. W., Li, S., and Zhang, Y. (2014). Spatiotemporal production of reactive oxygen species by NADPH oxidase is critical for tapetal programmed cell death and pollen development in Arabidopsis. Plant Cell 26, 193–201. doi: 10.1105/tpc.114.125427
Xing, S. P., and Zachgo, S. (2008). ROXY1 and ROXY2, two Arabidopsis glutaredoxin genes, are required for anther development. Plant J. 53, 790–801. doi: 10.1111/j.1365-313X.2007.03375.x
Xiong, S. X., Lu, J. Y., Lou, Y., Teng, X. D., Gu, J. N., Zhang, C., et al. (2016). The transcription factors MS188 and AMS form a complex to activate the expression of CYP703A2 for sporopollenin biosynthesis in Arabidopsis thaliana. Plant J. 88, 936–946. doi: 10.1111/tpj.13284
Xu, J., Ding, Z. W., Vizcay-Barrena, G., Shi, J. X., Liang, W. Q., Yuan, Z., et al. (2014). ABORTED MICROSPORES acts as a master regulator of pollen wall formation in Arabidopsis. Plant Cell 26, 1544–1556. doi: 10.1105/tpc.114.122986
Xu, T., Zhang, C., Zhou, Q., and Yang, Z. N. (2016). Pollen wall pattern in Arabidopsis. Sci. Bull. 61, 832–837. doi: 10.1007/s11434-016-1062-6
Yang, J., Tian, L., Sun, M. X., Huang, X. Y., Zhu, J., Guan, Y. F., et al. (2013). AUXIN RESPONSE FACTOR17 is essential for pollen wall pattern formation in Arabidopsis. Plant Physiol. 162, 720–731. doi: 10.1104/pp.113.214940
Yang, Y. X., Dong, C. H., Yu, J. Y., Shi, L., Tong, C. B., Li, Z. B., et al. (2014). Cysteine Protease 51(CP51), an anther-specific cysteine protease gene, is essential for pollen exine formation in Arabidopsis. Plant Cell Tissue Organ Cult. 119, 383–397. doi: 10.1007/s11240-014-0542-0
Zhan, J. P., Thakare, D., Ma, C., Lloyd, A., Nixon, N. M., Arakaki, A. M., et al. (2015). RNA sequencing of laser-capture microdissected compartments of the maize kernel identifies regulatory modules associated with endosperm cell differentiation. Plant Cell 27, 513–531. doi: 10.1105/tpc.114.135657
Zhang, D. D., Liu, D., Lv, X. M., Wang, Y., Xun, Z. L., Liu, Z. X., et al. (2014). The cysteine protease CEP1, a key executor involved in tapetal programmed cell death, regulates pollen development in Arabidopsis. Plant Cell 26, 2939–2961. doi: 10.1105/tpc.114.127282
Zhang, W., Sun, Y. J., Timofejeva, L., Chen, C. B., Grossniklaus, U., and Ma, H. (2006). Regulation of Arabidopsis tapetum development and function by DYSFUNCTIONAL TAPETUM1 (DYT1) encoding a putative bHLH transcription factor. Development 133, 3085–3095. doi: 10.1242/dev.02463
Zhang, Z. B., Zhu, J., Gao, J. F., Wang, C., Li, H., Li, H., et al. (2007). Transcription factor AtMYB103 is required for anther development by regulating tapetum development, callose dissolution and exine formation in Arabidopsis. Plant J. 52, 528–538. doi: 10.1111/j.1365-313X.2007.03254.x
Zhao, D. Z., Wang, G. F., Speal, B., and Ma, H. (2002). The EXCESS MICROSPOROCYTES1 gene encodes a putative leucine-rich repeat receptor protein kinase that controls somatic and reproductive cell fates in the Arabidopsis anther. Genes Dev. 16, 2021–2031. doi: 10.1101/gad.997902
Zhou, Q., Zhu, J., Cui, Y. L., and Yang, Z. N. (2015). Ultrastructure analysis reveals sporopollenin deposition and nexine formation at early stage of pollen wall development in Arabidopsis. Sci. Bull. 60, 273–276. doi: 10.1007/s11434-014-0723-6
Zhu, E. G., You, C. J., Wang, S. S., Cui, J., Niu, B. X., Wang, Y. X., et al. (2015). The DYT1-interacting proteins bHLH010, bHLH089 and bHLH091 are redundantly required for Arabidopsis anther development and transcriptome. Plant J. 83, 976–990. doi: 10.1111/tpj.12942
Zhu, J., Chen, H., Li, H., Gao, J. F., Jiang, H., Wang, C., et al. (2008). Defective in Tapetal Development and Function 1 is essential for anther development and tapetal function for microspore maturation in Arabidopsis. Plant J. 55, 266–277. doi: 10.1111/j.1365-313X.2008.03500.x
Zhu, J., Lou, Y., Xu, X. F., and Yang, Z. N. (2011). A genetic pathway for tapetum development and function in Arabidopsis. J. Integr. Plant Biol. 53, 892–900. doi: 10.1111/j.1744-7909.2011.01078.x
Keywords: anther, tapetum, DYT1, TDF1, AMS, MS188
Citation: Li D-D, Xue J-S, Zhu J and Yang Z-N (2017) Gene Regulatory Network for Tapetum Development in Arabidopsis thaliana. Front. Plant Sci. 8:1559. doi: 10.3389/fpls.2017.01559
Received: 20 March 2017; Accepted: 28 August 2017;
Published: 12 September 2017.
Edited by:
Changbin Chen, University of Minnesota, United StatesReviewed by:
Yingxiang Wang, Fudan University, ChinaMatthew R. Willmann, Cornell University, United States
Copyright © 2017 Li, Xue, Zhu and Yang. This is an open-access article distributed under the terms of the Creative Commons Attribution License (CC BY). The use, distribution or reproduction in other forums is permitted, provided the original author(s) or licensor are credited and that the original publication in this journal is cited, in accordance with accepted academic practice. No use, distribution or reproduction is permitted which does not comply with these terms.
*Correspondence: Zhong-Nan Yang, em55YW5nQHNobnUuZWR1LmNu
†These authors have contributed equally to this work.