- 1Plant Quality and Food Security, Leibniz Institute of Vegetable and Ornamental Crops, Grossbeeren, Germany
- 2Food Chemistry, Institute of Nutritional Science, University of Potsdam, Nuthethal, Germany
- 3Functional Plant Biology, Leibniz Institute of Vegetable and Ornamental Crop, Grossbeeren, Germany
- 4Department of Molecular Nutritional Physiology, Institute of Nutrition, Friedrich Schiller University Jena, Jena, Germany
- 5Food Innovation, The New Zealand Institute for Plant & Food Research Limited, Palmerston North, New Zealand
Selenium (Se) is an essential micronutrient for human health. Se deficiency affects hundreds of millions of people worldwide, particularly in developing countries, and there is increasing awareness that suboptimal supply of Se can also negatively affect human health. Selenium enters the diet primarily through the ingestion of plant and animal products. Although, plants are not dependent on Se they take it up from the soil through the sulphur (S) uptake and assimilation pathways. Therefore, geographic differences in the availability of soil Se and agricultural practices have a profound influence on the Se content of many foods, and there are increasing efforts to biofortify crop plants with Se. Plants from the Brassicales are of particular interest as they accumulate and synthesize Se into forms with additional health benefits, such as methylselenocysteine (MeSeCys). The Brassicaceae are also well-known to produce the glucosinolates; S-containing compounds with demonstrated human health value. Furthermore, the recent discovery of the selenoglucosinolates in the Brassicaceae raises questions regarding their potential bioefficacy. In this review we focus on Se uptake and metabolism in the Brassicaceae in the context of human health, particularly cancer prevention and immunity. We investigate the close relationship between Se and S metabolism in this plant family, with particular emphasis on the selenoglucosinolates, and consider the methodologies available for identifying and quantifying further novel Se-containing compounds in plants. Finally, we summarize the research of multiple groups investigating biofortification of the Brassicaceae and discuss which approaches might be most successful for supplying Se deficient populations in the future.
Introduction
Awareness of malnutrition, e.g., deficiencies in iron, iodine, vitamin A, and zinc, in the developing world is high, but micronutrient deficiency is rarely discussed in developed countries. This reduced awareness is surprising, especially since micronutrient deficiency or suboptimal supply of the essential micronutrient selenium (Se) is seen in several developed countries such as New Zealand to (Thomson, 2004; Curtin et al., 2006), Australia (Oldfield, 2002) as well as in Europe, e.g., United Kingdom (Lyons et al., 2003), Germany (Hartfiel et al., 2010), and Finland (Alfthan et al., 2015), and is estimated affect about one billion persons worldwide (Haug et al., 2007). Micronutrient deficiency is usually regarded as having minor effects in developed countries where the diet is more diverse and the food comes from a range of sources rather than being limited to local produce. Therefore, Se deficiency in developed countries, such as New Zealand, is not as extreme as in the Se deficient areas of China and Tibet where local populations have suffered Se deficiency related disease that can be highly debilitating and sometimes fatal (Chen et al., 1980; Moreno-Reyes et al., 1998). However, there is increasing awareness that suboptimal amounts of Se can also be damaging to human health, in particular when coupled with malnutrition. Micronutrient deficiency may likely occur also in developed countries in the future, due to the consumption of fast-foods and the associated intake of so called “empty calories.”
As Se is an essential micronutrient, under-supply has direct and indirect consequences for human health. Direct disorders include a destabilized immune system, hypothyroidism and cardiomyopathy (Whanger, 2004; Rayman, 2012). Pathologic symptoms are developed as a consequence of a daily Se intake <10 μg day−1. Indirectly, Se deficiency results in loss of protective anti-cancerogenic effects through reduced expression of antioxidant selenoproteins and reduced availability of several seleno-compounds (Whanger, 2004). The dietary reference Se intake is 55 μg d−1 for adult humans in the USA, according to the National Institutes of Health1, whereas in Europe the recommended daily intake is 70 μg d−1 according to the European Food Safety Authority (EFSA Panel on Dietetic Products, Nutrition and Allergies (NDA), 2014).
Consequently, agricultural and horticultural food production systems should develop to improve access to more Se nutritious food. One promising approach is to promote the production and consumption of Se-biofortified plant-based food. It is remarkable that about 10% of the world's vegetable production is generated from Brassicales species (Augustine et al., 2014) including the most economically important family Brassicaceae. Brassicales species are able to accumulate Se (White, 2016) and, furthermore, are characterized by a certain group of secondary plant metabolites—the glucosinolates—found almost exclusively in the order Brassicales (Verkerk et al., 2009). Certain individual glucosinolates are known to confer health-promoting effects, mainly due to the anti-carcinogenic and antidiabetogenic properties of their hydrolysis products (e.g., Lippmann et al., 2014; Guzmán-Pérez et al., 2016). In contrast to other plant species, Brassicales species demonstrate the ability to synthesize not only seleno-amino acids and selenoproteins but also selenoglucosinolates. Moreover, synthetic breakdown products of selenoglucosinolates are reported to be distinctly more protective in cancer prevention compared to their S-containing analogs (Sharma et al., 2008; Emmert et al., 2010). It also seems likely that the antioxidant selenoproteins may be of benefit in counteracting diseases of oxidative stress such as cancer (Rayman et al., 2008). Previously several novel selenoglucosinolates have been identified from Brassica species (Matich et al., 2012, 2015; McKenzie et al., 2015b), and it was demonstrated that ingestion of Se-enriched broccoli, which contains these seleno-compounds alongside others, may have also a beneficial role in the human immune response (Bentley-Hewitt et al., 2014).
The focus of this review is to highlight the human health benefits implicit in the presence of unique Se-containing metabolites produced by Brassica species. This is an important and novel set of circumstances not present in other plant families—or contained in other reviews. The selenoglucosinolates in particular have not previously been reviewed in any depth, nor have their potential roles in human health. The review also highlights the latest methodology specific to the identification of Se containing compounds. Therefore, we aim to provide not only an up-to-date overview of previous and current research on Se metabolism in the Brassicales and its association with human health, but also provide new insight and motivation to further investigation.
Selenium in Brassicales
In recent years several reviews have been published on the importance of Se in higher plants, such as Terry et al. (2000), Pilon-Smits (2005), Zhu et al. (2009), Pilon-Smits and Quinn (2010), Feng et al. (2013), El-Ramady et al. (2015), Malagoli et al. (2015), Winkel et al. (2015), White (2016), and Schiavon and Pilon-Smits (2017). The review of El-Ramady et al. (2015) gives an overview regarding Se physiology and biology in higher plants and describes many aspects of Se fertilization, whereas Winkel et al. (2015) deals with Se uptake and pathways, but also with Se sources and distribution in water, air and soil. The groups round Terry and Pilon-Smits have established the critical nature of the selenocysteine methyltransferase (SMT) gene in the biosynthesis of methylselenocysteine (MeSeCys) and the role Se plays throughout the plants' wider ecosystem. White's (2016) latest review provides an excellent overview of Se uptake, translocation and metabolism in plants in general concluding in the demand to breed crops with greater Se concentrations in their edible tissue.
Selenium Uptake
As in most plant genera, the Brassicales take up Se primarily as the selenate anion (), the predominant form occurring in alkaline and well-oxidized soils, as the selenite anion () existing in well-drained mineral soils, and also as selenocysteine (SeCys) and selenomethionine (SeMet; Ajwa et al., 1998).
Generally, the amount of Se taken up is related directly to the amount present in the soil (Brown and Shrift, 1982; Zhao et al., 2005) or the nutrient solution the plants grow on (Bañuelos, 1996). However, this is not always the case; for example in the Brassica vegetable rutabaga (Brassica napus L.) there was a poor correlation between Se uptake and Se soil content grown on a landfill (Arthur et al., 1992). Also, after two plantings of canola (B. napus), 80% of the Se remained in soils (Ajwa et al., 1998).
Se uptake has been shown to be greater when supplied in nutrient solution. While some experiments report data related to Se uptake from natural soils, most data originate from experiments in the context of biofortification (see Section Selenium Biofortification). The amount of Se taken up varies between species of Brassicales (Table 1). Concentrations may reach up to 2,000 μg/g dry weight (DW) (Ximenez-Embun et al., 2004; Manion et al., 2014). Significant genetic effects on Se concentration in Brassicales have been observed for leaves of rapid-cycling B. oleracea L. (Kopsell and Randle, 2001), broccoli florets [B. oleracea L. Italica Group (Bañuelos et al., 2003; Farnham et al., 2007; Ramos et al., 2011)], sprouts of cauliflower (B. oleracea L. Botrytis Group), kale (B. oleracea L. acephala Group), cabbage (B. oleracea Capitata Group) and Chinese cabbage [B. rapa L. (Ávila et al., 2014)], as well as shoots of Indian mustard [B. juncea (L.) Czern (Bañuelos et al., 1997)]. Comparing different Brassica species cultivated on natural soils with a comparable Se concentration of about 0.32 mg kg−1 the order of precedence in uptake (in μg g−1 DW) was Brussels sprouts (B. oleracea Gemnifera Group) (0.247), broccoli (0.129), savoy cabbage (B. oleracea Savoy Cabbage Group) (0.104), cauliflower (0.102), red cabbage (0.091), white cabbage (0.085), kale (0.046), kohlrabi (B. oleracea var. gongylodes L.) (0.037), and finally turnip (B. rapa var. rapa L.) (0.029; De Temmerman et al., 2014). This demonstrates the wide range of Se uptake within the Brassicales. As an example of the potential differences within a single species, cultivars of Indian mustard originating from different countries were compared under the same growing conditions. The amount of Se taken up doubled between the cultivar with the lowest and the highest Se concentrations independent of the supply form (soil or hydroponics) and the plant tissue (root or shoot; Bañuelos et al., 1997).
The form in which Se is provided is also important, with selenate being taken up two-fold faster than selenite in Indian mustard (De Souza et al., 1998). The order of preference when Indian mustard was supplied over an 8-day period with different Se forms at 20 μM was dimethylselenoproprionate (DMSeP) > SeMet > selenate > SeCys > selenite (Terry et al., 2000).
Although abiotic effects seem to be less influential than genetic effects, Se uptake also depends on environmental conditions and on the interaction with other nutrients supplied (see Section Promotion of Selenoglucosinolate Formation by Targeted Supply of N and S). In rapid-cycling B. oleracea, Se accumulation was clearly temperature-dependent (Chang and Randle, 2006), with Se concentrations increasing linearly with increasing temperature from 10 to 30°C in the leaves, ranging from 1.73 to 2.54 mg g−1 DW. Conversely, Se content decreased linearly with increasing temperature in the roots and ranged from 2.87 to 2.17 mg Se g−1 DW. Within environmental conditions, the microbiome also seems to be important for Se uptake. Inoculation of bacterial isolates into the rhizosphere of axenic plants (B. juncea) led to increased Se accumulation in shoots and roots following supply with 20 μmol selenate (De Souza et al., 1999). Depending on the strain selected, the tissue Se concentration increased up to three-fold in shoots and five-fold in roots. The influence of environmental factors has not yet been fully explored. However, knowledge in this area will become a crucial topic when Se-biofortification in crops is investigated.
Selenate enters root cells through sulphate transporters in their plasma membranes (Terry et al., 2000; White et al., 2004). sulphate transporters are encoded by a small family of genes; e.g., 14 in the genome of Arabidopsis thaliana L., and a similar number in other Brassicaceae species (Buchner et al., 2004; Hawkesford et al., 2005). A detailed overview of the sulphate transporters identified in Arabidopsis and their function are given in White (2016). Briefly, all sulphate transporters can be placed into one of four groups based on their protein sequences and distinct functional characteristics (Hawkesford, 2003; Hawkesford et al., 2005; Gigolashvili and Kopriva, 2014). Group 1 contains high-affinity sulphate transporters (HAST) that are thought to catalyse most selenate influx to cells (Hawkesford et al., 2005), sulphate transporters from group 2 are thought to catalyse selenate uptake into cells within the stele. Further, group 3 transporter AtSULTR3;5 appears to modulate the activity of a group 2 transporter, but does not catalyse transport itself (White, 2016). In contrast to group 1 transporters, sulphate transporters of group 4 (AtSULTR4;1 and AtSULTR4;2) might be responsible for catalysing the selenate efflux from the vacuoles (Gigolashvili and Kopriva, 2014). However, while these transporters are well-characterized in Arabidopsis, transporters and their mode of action need to be more fully investigated in brassicaceous vegetables.
For selenite uptake, P transporters are activated as well (Winkel et al., 2015). The involvement of the phosphate transport system in the movement of selenite throughout a plant has been reported based on the observation that increasing P concentration reduced selenite uptake rates in different plant species (Broyer et al., 1972; Hopper and Parker, 1999), however, this has not yet been found in Brassicales.
Selenium Mobilization and Distribution
Selenate and selenite transport processes in all plants are energy-dependent (Hawkesford et al., 1993; Sors et al., 2005; Li et al., 2008). Selenate is rapidly translocated from the root to the shoot, whereas only ~10% of selenite is translocated in this way (De Souza et al., 1998). After uptake, Se is distributed within the plant to the different organs. The sites of accumulation depend on the species, its phase of development, and its physiological conditions. Overall, it follows: seeds > flowers > leaves > roots > stems (Terry et al., 2000; Quinn et al., 2011). A portion of the Se transported into the plant is volatilized as dimethyl selenide. Se-volatilisation rates of Indian mustard pre-treated for 7 d with 20 μg selenate amounted to 7 μg (g d)−1 DW and were two- to three-fold higher from plants pre-treated with 20 μg selenite (De Souza et al., 1999). A clear correlation was found between Se-volatilization rates and total Se concentrations.
Selenium—a Non-essential Element for Plants That Has Beneficial or Toxic Effects
Unlike in animals and some green algae (Araie and Shiraiwa, 2016), Se is considered a non-essential element for the healthy growth of crops (Zhang and Gladyshev, 2009). Brassicales as well as many other plant species exposed to high concentrations of Se in their root environment exhibit symptoms of injury. Visible and often initial symptoms are stunting of growth, root shortening, chlorosis, withering, and drying of leaves accompanied by decreased protein synthesis and ending in premature death of the plant (Terry et al., 2000). Toxicity thresholds are very different depending on the species and the environment.
In contrast, several authors report beneficial effects of increased Se content in the Brassicales, where low-dose Se supplementation has been shown to increase growth in Stanleya (Cappa et al., 2015), broccoli, radish (Raphanus raphanistrum ssp. sativus (L.) Domin), and turnip. This beneficial effect has been suggested to be due to Se-induced mimicry of S-deficiency resulting in increased S uptake by S transporters (Boldrin et al., 2016), increased anti-oxidant activity (Hartikainen et al., 2000; Proietti et al., 2013), and decreased lipid peroxidation (Xue et al., 2001; Abd Allah et al., 2016). Further, benefit of increased Se content derives from herbivory protection from insects, as shown in S. pinnata, B. juncea and B. oleracea Italica Group (Freeman et al., 2006a, 2007).
Based on their capacity for Se-uptake and tolerance plants are divided into three groups: Se non-accumulators, Se-indicators, and Se-accumulators (Brown and Shrift, 1982; Terry et al., 2000; White, 2016). The majority of plants are non-accumulating species, which cannot tolerate Se tissue concentrations of more than 10–100 μg g−1 DW, and rapidly show signs of Se toxicity (Hartikainen et al., 2001) on exposure to higher concentrations of Se than this. This toxicity is due to the non-specific incorporation of seleno-amino acids into proteins, replacing Cys and Met and thus disrupting protein function, and causing toxicity to the plant (Van Hoewyk, 2013).
Several members of the Brassicaceae fall into the category of Se indicator plants (also known as Se secondary accumulator plants) and are able to tolerate Se concentrations up to 1,000 μ g g−1 DW in their tissues and can therefore colonize soils described as seleniferous. These include broccoli (Lyi et al., 2005; Ramos et al., 2011; Ávila et al., 2013), Indian mustard (Bañuelos and Meek, 1989), kale (Maneetong et al., 2013), turnip, and headed cabbage (Sugihara et al., 2004; see Table 1).
Se-accumulator plants (also known as Se hyper-accumulators) are able to accumulate Se concentrations of >1,000 μg g−1 DW in their tissues with no apparent ill-effects (Pickering et al., 2003; Broadley et al., 2006; Freeman et al., 2006b; El Mehdawi and Pilon-Smits, 2012). Indeed, Se hyper-accumulators show a particularly strong growth effect which may exceed a two-fold increase in biomass production (El Mehdawi and Pilon-Smits, 2012). They are also the only plants able to colonize highly-seleniferous soils. Hyperaccumulation among the Brassicaceae family is found for Cardamine hupingshanesis (Yuan et al., 2013) and species within the genus Stanleya and Thelypodium, such as S. pinnata and T. laciniatum Endl. (Death et al., 1940; Galeas et al., 2007; Cappa and Pilon-Smits, 2014; Winkel et al., 2015). Although stems and leaves of the wildflower princesplume (S. pinnata) are edible and have been used as cooked greens and as medicine, when crushed they may have an unpleasant odor, are bitter and basically disliked (Whiting, 1985). It is intriguing that these species are able to accumulate an element that is not essential for higher plants (Zhang and Gladyshev, 2009), and that they not only tolerate but even grow better at tissue Se levels that are lethal for other plant species (Winkel et al., 2015).
Metabolism of Specialized Selenocompounds in the Brassicaceae
Methyl Selenocysteine
Se-indicator or -accumulating Brassicaceae are able to take up and store excess Se due to the expression of an additional Se metabolism gene, SMT, which specifically methylates selenocysteine producing MeSeCys. MeSeCys is not incorporated into the plant's proteins, as SeCys or SeMet are, and therefore does not contribute to Se toxicity (Brown and Shrift, 1981). Instead it allows the safe storage of Se away from the plant's biosynthetic machinery. The SMT gene is inducible by selenate in broccoli (Lyi et al., 2005) and has been confirmed as the key to Se-tolerance in Se-accumulating plants (Neuhierl and Bock, 1996; Neuhierl et al., 1999). Its over-expression in non-Se accumulator species, such as tobacco and tomato, has been shown to convert such plants into Se-accumulators with up to 25% of the Se in these plants found as MeSeCys (McKenzie et al., 2009; Brummell et al., 2011).
MeSeCys content has been reported for many brassicaceous crops fertilized with Se (Table 1). Ávila et al. (2014) hydroponically fertilized sprouts from six different Brassica species with 50 mM selenate for 1 week and reported MeSeCys concentrations of up to 50–100 μg g−1 DW (Table 1). Sugihara et al. (2004) have also reported high quantities of Se as MeSeCys in broccoli, Chinese cabbage, radish, and turnip sprouts. There have been few reports of MeSeCys concentration in mature crop plants, though 108 μg g−1 DW (Ávila et al., 2013), 1.5 μmol g−1 DW (Lyi et al., 2005), and 3.4 μmol g−1 DW (Mahn, 2017) have been reported in broccoli florets. MeSeCys and its derivative γ-glutamyl methylselenocysteine, have been shown to have greater bioefficacy in preventing cancer cell proliferation than other Se-containing compounds (Whanger, 2002). These compounds are also believed to be responsible for the reported decreased rate of pre-cancerous cell production in rat models following ingestion of Se-enriched broccoli (Finley et al., 2001). Thus, the presence of MeSeCys is important when considering Se-metabolism in the Brassicales in the context of human health.
Selenoglucosinolates
Selenium Brassica accumulators contain glucosinolates, a group of secondary plant metabolites containing sulphur. Glucosinolates are β-D-thioglucoside-N-hydroxysulphates with a variable side chain. So far more than 130 glucosinolates have been reported (Agerbirk and Olsen, 2012). Due to their variable side chain glucosinolates can be classified into aliphatic, aromatic, or indole forms. The aliphatic glucosinolates can be subdivided into straight or branched chain aliphatics, alcohols or unsaturated alkenyl glucosinolates, as well into the sulphur containing aliphatic methylsulphanylalkyl (SII), methylsulphinylalkyl (SIV), or methylsulphonylalkyl glucosinolates (SVI; Hanschen et al., 2014). Thus, according to their structure, glucosinolates contain at least two, very often three, and sometimes four sulphur atoms that might be replaced by Se in plants grown in Se-rich soils. Upon cell disruption, glucosinolates are hydrolyzed by the endogenous plant enzyme myrosinase, resulting in the formation of volatile hydrolysis products such as nitriles and isothiocyanates (Kissen et al., 2009). Possible breakdown products are shown in Figure 1. Isothiocyanates are valued as pleiotropic agents that exert a multitude of cancer-preventive actions, among them chemopreventive phase-I enzyme inhibition and phase-II enzyme induction as well the induction of apoptosis and cell cycle arrest. Thus, these compounds are linked to the cancer-preventive effects of Brassica consumption (Veeranki et al., 2015).
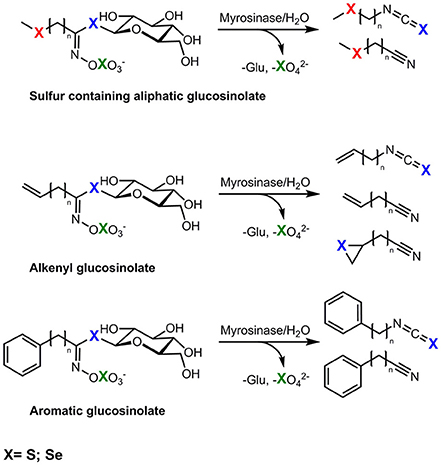
Figure 1. Possible exchange positions (X) of sulphur (S) by selenium (Se) in seleno-glucosinolates and the formation of possible corresponding hydrolysis products, red, incorporation in side chain; blue, incorporation in thioglucose, green, incorporation in sulphate group.
As glucosinolates are precursors to cancer preventing substances and exchanging sulphur with Se might further enhance the bioactivity of glucosinolate hydrolysis products (Emmert et al., 2010), it is of great interest to study the effect of Se on glucosinolate production and the formation of selenoglucosinolates.
More than 40 years ago Stewart et al. (1974) reported a Se-containing sinigrin (2-propenyl glucosinolate) in horseradish (Armoracia lapathifolia Gilib.). In 1988, Kjær and Skrydstrup (1987) synthesized the first Se-containing glucosinolates by replacing the thioglucosidic S with Se in order to study their properties and their enzymatic hydrolysis. One year later, their group identified traces of selenogluconapin (Se-3-butenyl glucosinolate) and the corresponding isoselenocyanates in plants of S. pinnata after 3-weeks of Se fertilization with 100 ppm sodium selenite (Bertelsen et al., 1988), indicating incorporation of Se into the glucose moiety. However, the ratio of Se-glucosinolate to the normal glucosinolate did not exceed 1:50,000. Further, the authors did not detect significant Se incorporation in Lepidium sativum L., A. lapathifolia nor in S. pinnata grown at low Se-levels (Bertelsen et al., 1988). Higher incorporation of Se into glucosinolates of Brassica species was reported by Matich and co-workers in 2012. Following treatment with sodium selenate (20 mL of 5 mM, twice weekly to the soil for 4 weeks) to forage rape (cv. Maxima), cauliflower (cv. Liberty), and broccoli (cv. Triathlon) the accumulation of methylselenoalkyl glucosinolates with up to 40% of the respective glucosinolate containing Se was reported (Matich et al., 2012). Further, they analyzed the respective volatile hydrolysis products and identified methylselenoalkyl nitriles and isothiocyanates indicating that Se was incorporated into the methylselenoalkyl side chain of the glucosinolate (Matich et al., 2012). For example, the main isothiocyanate, the 4-methylselenobutyl isothiocyanate was found in 3-times higher concentrations compared to the sulphur analog (Matich et al., 2012). In 2015, that group went on to study the distribution of Se-glucosinolates and their metabolites in these plants and reported that broccoli florets particularly accumulate methylselenoalkyl glucosinolates with up to 32.4 μg g−1 FW and about 50% of the glucosinolates present being selenised (Matich et al., 2015). Moreover, incorporation of Se into aromatic glucosinolates such as gluconasturtiin (2-phenylethyl glucosinolate) was observed. However, this was at very low rates (only 0.04% of the glucosinolate) and the authors observed no isoselenocyanate formation (Matich et al., 2015). Thus, Matich and coworkers concluded that selenoglucosinolate biosynthesis in Brassica via SeMet is the only efficient route (Matich et al., 2012, 2015).
Recently, Ouerdane et al. (2013) tentatively identified several selenoglucosinolates in seeds of B. nigra. As well as methylselenoalkyl glucosinolates such as glucoselenoerucin or glucoselenoiberin [4-(methylseleno)butyl- and 3-(methylseleninyl)propyl glucosinolate], they reported the detection of several non-typical glucosinolates. They postulated methylseleno-indole glucosinolates and glucosinolates acylated with methylselenoacetic acid or with methylselenosinapinic acid at position 6' of the thioglucose moiety. Typically, acylated glucosinolates are only found in seeds and not in other plant parts (Agerbirk and Olsen, 2012).
Promotion of Selenoglucosinolate Formation by Targeted Supply of N and S
The amounts of N- and S-containing compounds in plants, such as glucosinolates, can be highly variable and are strongly influenced by S and N supply (e.g., Kim et al., 2002; Li et al., 2007; Schonhof et al., 2007). Thus, Brassica species-specific N/S ratios distinctly stimulate the formation of glucosinolates (Fallovo et al., 2011). For example, N/S ratios between 7:1 and 10:1 promoted aliphatic alkyl and indole glucosinolates concentrations in broccoli florets (Schonhof et al., 2007), whereas high concentrations of aromatic glucosinolates occurred in turnip roots at N/S ratios <5 (Li et al., 2007). It seems that within these ranges of N/S the corresponding glucosinolate precursors of the amino acid-derived glucosinolates would be preferentially available for glucosinolate synthesis (and not for protein synthesis), such as Met for aliphatic glucosinolates and phenylalanine and tryptophan for aromatic and indole glucosinolates, respectively. Matich et al. (2015) suggested that SeMet is the decisive Se-precursor bottleneck or the major Se-precursor for the formation of selenoglucosinolates. Consequently, a targeted strategy for selenoglucosinolate production in Brassica plants could include the identification of a N/S/Se ratio and how to balance it for the promotion of selenoglucosinolate synthesis.
Selenium Biofortification
As Se is lacking in many diets, consumption of plants containing Se may be an effective way to increase dietary Se (McKenzie et al., 2015a). Furthermore, Se enrichment of the Brassicales produces Se-containing compounds with added bioefficacy, such as MeSeCys and potentially the selenoglucosinolates. To reach RDIs of >55 μg d−1 as recommend for adult humans a 100 g serving of fresh Brassica food with a Se concentration of at least 5 μg g−1 DW is necessary. This can be achieved via biofortification; the idea of enhancing nutrients in food crops. Three methods of Se-biofortification have been used for the Brassicales; hydroponic culture, soil fertilization and foliar spraying, resulting in varying amounts of Se uptake (for references, see Table 1).
The highest reported Se concentration in the Brassicaceae have been recorded following hydroponic culture (Table 1), with Se concentrations of 1,200 and up to 1,800 μg Se g−1 DW reported in the florets and leaves of broccoli (Lyi et al., 2005; Ramos et al., 2011), up to 1,900 μg Se g−1 DW in rapid cycling cabbage leaves (Kopsell and Randle, 1999), and up to 1,800 and 2,000 μg Se g−1 DW in B. juncea shoots and seedlings, respectively (Bañuelos et al., 1990; Pilon-Smits et al., 1999; Ximenez-Embun et al., 2004). Presumably, the constant exposure of the plant's root system to the Se-enriched solution and the lack of Se-soil interactions act together to make hydroponic culture particularly efficient. The Se concentrations achieved are dependent on the concentration of Se in the hydroponic solution and the length of time the plant is exposed, as well as the Se-accumulating capacity of the plant species, therefore direct comparisons between different experiments are difficult. However, broccoli, Indian mustard, and rapid-cycling B. oleracea appear to accumulate the highest Se contents following hydroponic culture (1,800–1,900 μg g−1 DW in the shoot tissue, Table 1), taking them into the realm of the Se-hyper-accumulators. By comparison, the hydroponic culture of Brussels sprouts, cabbage, cauliflower, Chinese cabbage, kale, radish, and turnip for similar lengths of time and Se concentrations result in lower Se accumulation (50–386 μg g−1 DW; Table 1).
Soil fertilization has also been used for Se enrichment, particularly for broccoli, resulting in a maximum reported content of 879 μg g−1 DW in the florets of mature, flowering plants fertilized with Se every second day for 12 days (Lee et al., 2005; Table 1). Recently, material from the Se-hyper-accumulator S. pinnata that had been grown on seleniferous soils was used to enrich the Se content of the soil broccoli plants were subsequently grown in. This resulted in a Se content of 3.5 μg g−1 DW in the leaves and floret material of the broccoli (Bañuelos et al., 2015). Indian mustard plants grown on naturally Se-rich soils have been shown to accumulate Se up to 150 μg g−1 DW in their shoot material (Van Huysen et al., 2003). Soil fertilization of other brassicaceous crops resulted in Se contents of 30–60 μg g−1 DW in cauliflower, radish and turnip (Table 1). Notably, when cabbage was grown on peat fertilized with Se at 158 mg kg−1 soil for 6 months Se accumulated to 1,600 μg g−1 DW in the leaves with no toxicity symptoms (Funes-Collado et al., 2013). This is a high concentration and presumably due to the length of time the plants were exposed to the Se treatment.
In order to develop a commercial regime for Se-enrichment of broccoli, Hsu et al. (2011) investigated foliar application of sodium selenate as a single dose to the leaves of plants growing in the field, resulting in head and upper stem tissue containing 5 μg g−1 DW Se. Recently, Palomo-Siguero et al. (2015) investigated the bioefficiency of Se supplied as Se-nanoparticles to the roots of radish plants hydroponically. There was no sign of Se toxicity in plants treated this way and the Se were incorporated into MeSeCys and SeMet. Se accumulation was 25% less when Se nanoparticles were used compared with selenite (Table 1). Nevertheless, this is the first report of Se being biotransformed from nanoparticles in plants.
Sodium selenate and sodium selenite are the most commonly used Se-sources for the enrichment of Brassicas. However, plants exposed to selenite have a much reduced Se content compared to those fertilized with selenate (Ximenez-Embun et al., 2004; Lyi et al., 2005). This is because selenate is taken up directly by high efficiency S transporters in the roots compared with the less efficient phosphate transporters used to take up selenite (see Section Selenium Uptake).
Transgenic approaches have also been used to successfully increase the Se and MeSeCys content of Brassica species. Over-expression of ATP-sulphurylase, the rate limiting step for Se uptake and assimilation, in B. juncea resulted in a two- to three-fold increase in Se content in the shoots (Pilon-Smits et al., 1999). A similar approach was used for SMT in B. juncea, resulting in up to 4,000 Se μg g−1 DW accumulating in seedlings, and 100 μg Se g−1 FW as MeSeCys; a four-fold increase compared with controls (Leduc et al., 2004). However, although effective, a transgenic approach to increasing Se content in crops is unlikely to be acceptable by consumers in the near future. Gene editing technologies such as CRISPR/Cas9, may offer an alternative, and ultimately more palatable method, though this technology is not so easily applied to the upregulation of genes as to down.
When producing vegetables with enhanced Se concentrations it is necessary to consider that toxic conditions might be reached in the diet of some consumer groups such as children or people with particularly high vegetable consumption. Therefore, it is important to produce plants with stable and defined Se contents so that food produced from these are safe and where advice on quantities for consumption can be relied upon. Careful consideration should be given to the amount of Se taken up by different Brassicales as well as reproducibility over the growing season. The amount of Se taken up can most effectively be controlled under hydroponic conditions. However, it is important to note that even using this method substantial differences have been noted for Se uptake in the same species (Table 1). Therefore, the amount of Se applied to a Brassica crop should be carefully determined in each case and over several growing seasons. Despite this, biofortification of crops through Se-enriched fertilizers has been conducted in Finland for the past two decades as the population was Se-deficient. Currently, the daily Se intake for the Finish population is considered to meet the Nordic and EU RDI (Alfthan et al., 2011). Thus, understanding mechanisms of Se-uptake into crop plants and how this is affected by environmental conditions is of great importance in producing biofortified plant foods.
Instrumental Approaches for Detecting, Measuring, and Monitoring Selenium and its Metabolites within Brassica Species
Selenium readily substitutes for S in a non-specific manner in biological systems and is thus readily incorporated by living organisms into a variety of organic compounds which would normally contain sulphur. This process results in the biosynthesis of Se-containing amino acids, proteins and plant secondary metabolites such as glucosinolates. Biogenic production of methylselenol (MeSeH) from selenoamino acids may also result in the production of further Se-containing metabolites including selenosugars, selenosinapine, and selenourea derivatives as shown in mustard seeds (Ouerdane et al., 2013). We briefly summarize instrumental approaches for detecting, measuring, and monitoring Se and Se-containing metabolites.
Total and Inorganic Selenium
Inductively coupled plasma-optical emission spectrometry (ICP-OES) provides quantification by measuring the light emitted from excited ions and atoms at characteristic wavelengths. Excited ions and atoms are formed by reaction of the sample in an inductively-coupled plasma (ICP) and detected by atomic emission. For Se, the detection limit is relatively high due to the poor emission intensity of Se compared to other elements. The primary detection wavelength is 196.026 nm with small interference with iron complicating the analysis of Se in iron rich samples (Ralston et al., 2008). ICP can be also coupled to mass analysers, typically a quadrupole mass spectrometer (MS) in ICP-MS. In addition, high resolution ICP-MS systems can be used to resolve Se-isotopes. Important in ICP-MS is the consideration of potential interferences and to minimize biases created by argon, germanium, and krypton isotopes. For example, the most abundant Se-isotope 80Se cannot be used to measure trace Se concentrations because of the severe interference with argon-dimers, also m/z 80, that are also formed in the inductively-coupled plasma (Ralston et al., 2008; Pettine et al., 2015). Moreover, organic carbon and high sodium concentrations can non-specifically affect the Se-signal. Due to the high ionization potential of Se, the addition of organic carbon compounds such as methanol, ethanol, or propanol can enhance the ICP-MS signal for Se. Therefore, for the ICP-MS analysis of Se, it is essential to work with internal standards and use standardized approved methods. In order to reduce or eliminate polyatomic interference, devices equipped with additional collision/reaction cell (CRC) technology have been introduced. These devices allow the detection of the most abundant Se-isotopes by avoiding the interference with argon from the plasma.
Organic Selenium
In planta, Se is involved in biochemical pathways that are analogous to S, resulting in the potential production of a large number of Se-containing metabolites with widely different physical properties which make their analysis a complex task. Different strategies need to be applied to identify and quantify these chemically diverse Se-species. Most frequently chromatographic separation methods are used for the physical separation of volatile or non-volatile Se-metabolites which are then detected, identified and measured by mass spectrometry (MS). The relatively large mass deficiency, coupled with a distinctive isotope pattern (Figure 2), and the high atomic mass relative to carbon and oxygen, greatly facilitates the detection, assignment of molecular formulae and identification of Se-containing compounds by high resolution mass spectrometry. The chromatographic methods include high performance liquid chromatography (HPLC or UHPLC), gas chromatography (GC), capillary electrophoresis (CE), and gel electrophoresis. For HPLC separations, reversed phase chromatography, ion pair chromatography, ion exchange chromatography, and size exclusion chromatography have all been employed (e.g., summarized in Lobinski et al., 2000; Uden, 2002). Among many others, anion exchange (Pedrero et al., 2007) and reversed phase column chromatography (McKenzie et al., 2009; Peñas et al., 2012) have been used for the separation of selenate, selenite, SeMet, SeMeCys, SeCys and other metabolites. More recently hydrophilic interaction liquid chromatography (HILIC) chromatography has been explored as method for analysis of amino acids and selenosugars (Aureli et al., 2012).
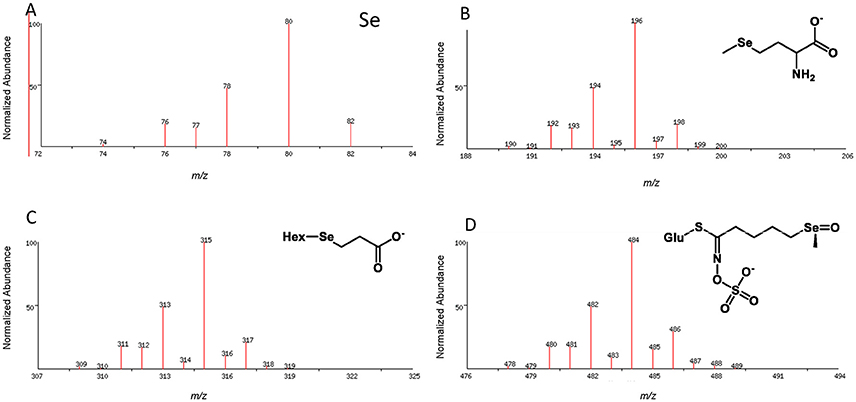
Figure 2. Isotope pattern for elemental selenium (A) and as observed in the pseudomolecular ion (M-H)− for organoselenium species, e.g., selenomethionine selenosugar derivative (B), Deamino-selenocysteine-selenosugar (C), Se-glucoraphanin (D); Hex, hexose; Glu, glucose.
Selenoglucosinolates
Brassica glucosinolates may contain up to four S atoms. Substitution with Se is possible at any of these sites with the Se entering as SeMet leading to a methylselenide sidechain, as SeCys (proposed for the thioglucose moiety) or directly as selenate. To date mainly incorporation of Se via SeMet has been observed (Matich et al., 2012), but an incorporation via the SeCys into the glucose moiety was also observed (Bertelsen et al., 1988). Glucosinolates may be analyzed by HPLC or LC-MS either directly or after removal of the sulphate group. Se-glucosinolates have been successfully analyzed by LC-MS (Matich et al., 2012, 2015). The location of Se in selenoglucosinates may be determined by LC-MS/MS analysis using well-established negative ion fragmentations (Figure 3). The elemental composition of these fragment ions has been validated using tandem MS and ion trap analysis of the 32S and 34S isotope distributions in daughter ions derived from the glucosinolate M+2 (32S and 34S) pseudomolecular ion (Cataldi et al., 2010; Figure 3).
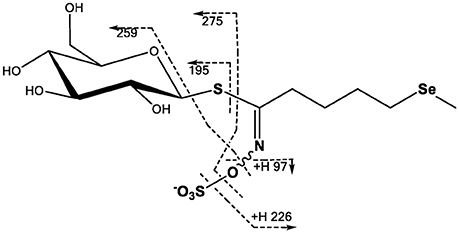
Figure 3. Proposed MS/MS fragmentation pathway for glucoselenoerucin based on fragmentation analysis of glucosinolates (Cataldi et al., 2010; Lelario et al., 2012; Matich et al., 2012).
The increasing power of very high mass resolution LC-MS instruments to identify Se-containing metabolites in Brassicas has been demonstrated by Ouerdane et al. (2013). Selenosugars, selenosinapine, and selenourea derivatives have also been reported in seed of black mustard (B. nigra), grown on naturally Se-rich soil. These identifications are based on high resolution LC-MS, however, the exact structures of these compounds cannot be determined from the mass spectral data alone.
Selenium-containing Volatiles
Incorporation of Se into amino acids, glucosinolates, and other precursors, provides the potential for the formation of novel Se containing volatiles when these precursors are subjected to enzymatic action or non-biotic degradation. Se containing volatiles may arise via three biosynthetic pathways. Firstly direct substitution of Se for S in the amino acids cysteine, SeMeCys and Met leads to the production of dimethylselenide (Me2Se), dimethyldiselenide (Me2Se2) and dimethylthioselenide (MeSSeMe) in transgenic tobacco (Nicotiana benthamiana; Matich et al., 2009), Se-enhanced green onions (Allium fistulosum; Shah et al., 2007) and Brassicas (Matich et al., 2012; Ouerdane et al., 2013). Enzymatic degradation of MeSeCys or SeMet may also result in the production of 2-(methylseleno)acetaldehyde, or the methylselenides so produced may react further with unsaturated aldehydes (2-alkenals) resulting from lipid oxidation (Matich et al., 2009). Thirdly, enzymatic hydrolysis of methylselenoalkyl-glucosinolates leads to methylselenoalkylnitriles and methylselenoalkylisothiocyanates (Matich et al., 2012, 2015).
Many Se-containing volatiles can be readily analyzed by standard GC-MS methods as used for their S-containing analogs. Gas chromatography has been used to separate Me2Se and Me2Se2 and others (Kubachka et al., 2007) as well as Se-containing glucosinolate breakdown products (Matich et al., 2012, 2015). However, selenoxides (e.g., dimethylselenoxide Me2SeO analogous to dimethylsulphoxide Me2SO) containing beta hydrogens (R2CHC(SeO)R2) and selenones (analogous to sulphones such as dimethylsulphone Me2SO2) are thermally unstable above room temperatures and are not suitable for standard GC-MS analysis. For highly volatile selenides such as Me2Se and Me2Se2, headspace analysis using highly adsorbent graphite based SPME phases such as CarboxenTM or CarboxenTM-PDMS hybrid fibers (Matich et al., 2009) should be preferred. Further increases in sensitivity could be expected by the application of Stir Bar Sorptive Extraction SBSE (Twister) combined with cyrofocusing of volatiles onto the GC column.
New Methods for Discovery of Selenometabolites
The finding and measurement of trace amounts of novel Se compounds in complex plant extracts can be difficult and tedious. Chromatographic separations coupled with ICP-MS reliably identify HPLC fractions containing Se but do not provide the molecular mass or information about the structure of the Se-containing molecules. This identification relies on the comparison of retention times with authentic reference compounds. Electrospray ionization mass spectrometry (ESI-MS) is very useful for the identification of Se-containing metabolites, however finding minor Se containing species in complex plant extracts is tedious and has disadvantages such as the oxidation of small Se-molecules and lower sensitivity engendered by the complex isotope distribution of Se. A robust approach, which should be also applied for the analysis of Brassica vegetables, is the combination of ICP-MS and ESI-MS. Such an approach has been used to investigate Se metabolites in kale (Chan et al., 2010).
Alternatively, bioinformatics approaches, based on the mass defect and Se isotopic ratios, may be used to identify Se-containing metabolites in complex plant extracts. Such strategies are susceptible to automation and the mass defect approach has been used to find Se-containing volatiles in Se-enriched green onions (A. fistulosum; Shah et al., 2007). The alternative would be to use the Se isotope pattern as a search requirement for the identification of putative Se-containing metabolites. Such an approach, based on relative isotope abundance and ultra-high resolution FT mass spectrometry, has been implemented to identify all S-containing metabolites in Allium species (Nakabayashi et al., 2013) but has not yet been applied to Se-containing metabolites. The application of such analytical approaches will help elucidate the principles that govern relationships between biological metabolites in brassicaceous vegetables and provide important tools for gaining deeper insight into Se metabolism in plants and humans.
Selenoglucosinolates for Human Nutrition
Bioavailability and Metabolism of Selenoglucosinolates
Selenium is an essential micronutrient for humans, and is part of the 21st amino acid, SeCys, and therefore of selenoproteins. In most Se-dependent enzymes, SeCys is part of the active site, and Se often functions as a redox center in these enzymes. An detailed overview about the Se metabolism in humans is given by Roman et al. (2014). The bioavailability of Se strongly depends on the chemical form in the food. In plants a multitude of different species have been identified such as selenate, selenite, selenocystine, SeMet, selenohomocysteine, MeSeCys, γ-glutamyl-selenocystathionine, SeMet selenoxide, γ-glutamyl-MeSeCys, selenocysteineselenic acid, Se-proponylselenocysteine selenoxide, Se-methylselenomethionine, selenocystathionine, Me2Se2, selenosinigrin, and other selenoglucosinolates, selenopeptides and selenowax (Navarro-Alarcon and Cabrera-Vique, 2008). In animal tissues selenocompounds are SeCys, SeMet, selenotrisulphides of cysteine, selenosugars, selenite, and selenate.
In humans, Se is mainly ingested and absorbed as SeMet, but also as selenate and selenite. The absorption efficiency of those compounds is supposed to range between 80 and 90% (Patterson et al., 1993; FAO/WHO, 2002). For all other Se-compounds, and especially for selenoglucosinolates, no studies on bioavailability have been systematically conducted so far. With regard to bioavailablility, the limiting step is not the absorption of Se but rather its conversion into metabolically active forms. In general, the human body metabolizes the various Se forms into hydrogen selenide (H2Se). H2Se is the key metabolite formed from inorganic sodium selenite via selenodiglutathione through reduction by thiols and NADPH-dependent reductases and released from SeCys by a lyase-dependent reaction (Bjornstedt et al., 1992). H2Se provides Se for the synthesis of selenoproteins (Ganther, 1999, Figure 4). Also at this stage, it is unclear if and how selenoglucosinolates could be metabolized to release Se for selenoprotein synthesis. Alternatively, they could exert their effects only directly without modulating selenoprotein expression. In addition, their metabolism has not been studied yet.
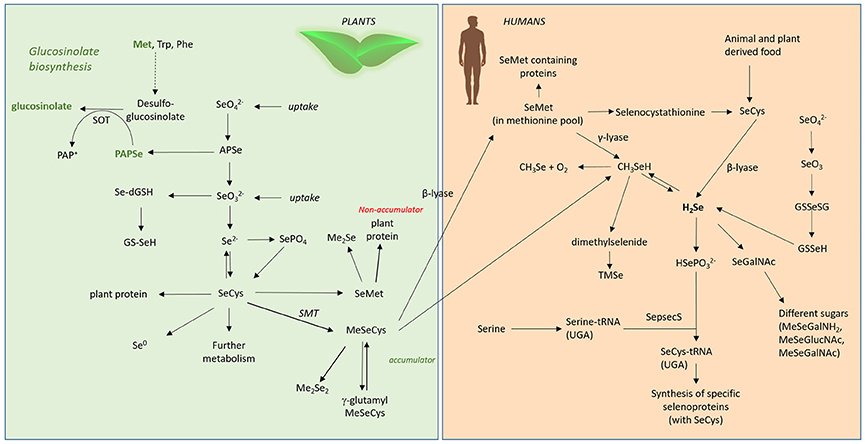
Figure 4. Se metabolism in plants and humans. Met, methionine; Trp, tryptophane; Phe, phenylalanine; SOT, sulphotransferase; , selenate; , selenite; APSe, Adenosine-5′-phospho-selenate; Se-dGSH, seleno-diglutathione; GS-SeH, glutathioselenol; SePO4, selenophosphate; SeCys, selenocysteine; SMT, selenocysteinemethyltransferase; SeMet, selenomethionine; MeSeCys, methylselenocysteine; Me2Se, dimethylselenide; Me2Se2, dimethyldiselenide; γ-glutamyl-MeSeCys, γ-glutamyl-methylselenocysteine; H2Se, hydrogen selenide; CH3Se, methylselanyl; CH3SeH, methylselenol; SeGalNAc, seleno N-acetylgalactosamine; SepsecS, Sep (O-phosphoserine) tRNA:Sec (selenocysteine) tRNA synthase; , selenophosphate; TMSe, trimethylselenonium.
It is also important to consider that many brassicaceous vegetables are processed by chopping, cooking or freezing. Such processing has been shown to severely influence the glucosinolate profile (Hanschen et al., 2014), and would also be expected to modulate the selenoglucosinolates within the vegetable matrix. To provide knowledge about the amount of their corresponding health-promoting breakdown products, the effect of various processing procedures on the degradation of selenoglucosinolates must be considered as well.
Chemo-Preventive Effects of Selenoglucosinolate Related Products
Selenium, often labeled as “antioxidant,” is actually not an antioxidant compound by itself, but rather an essential part of the catalytic center of selenoproteins, which are involved in protection against oxidative stress (Steinbrenner and Sies, 2009). Among the selenoproteins there are well-known redox-active selenoenzymes, such as glutathione peroxidase (GPx), thioredoxin reductase (TrxR), and methionine sulphoxide reductase B (MsrB). GPx isoenzymes reduce hydrogen peroxide (H2O2), organic hydroperoxides, and phospholipid hydroperoxides (only GPx4) using reduced glutathione as co-substrate (Papp et al., 2007; Brigelius-Flohe and Maiorino, 2013). TrxR isoenzymes reduce a wide variety of substrates, including oxidized thioredoxins, H2O2 and organic hydroperoxides (Bjornstedt et al., 1995), MsrB reduces free and protein-bound methionine sulphoxide to methionine (Moskovitz et al., 2002). Links between sulphur-containing isothiocyanates and selenoprotein production have been described in Barrera et al. (2012). Se in combination with isothiocyanates increased the expression of TrxR1 and GPx2 in colonic cell lines more strongly than Se or isothiocyanates alone (Barrera, 2010). In mice, the combination of the isothiocyanate sulphoraphane and a super-nutritional Se supply was most efficient in upregulating TrxR1 and glutathione-S-transferase activity in the colon (Krehl et al., 2012; see Section Selenoglucosinolates). A similar effect was observed in endothelial cells lines (Campbell et al., 2007) and in a colonic cell line (Wang et al., 2015).
Any excess supply of Se results in increased metabolism, but marginal or no further increases in selenoprotein biosynthesis. Many of the metabolites including H2Se and monomethylselenol are highly redox active and generate reactive oxygen species (ROS) upon reaction with and oxidation of thiols. These compounds are therefore termed as redox-active Se compounds (e.g., selenite, selenocystine, methylseleninic acid, MeSeCys that are known to exert oxidative stress; Spallholz, 1994). Such pro-oxidative properties reflect the opposite spectrum of a common consensus that Se is just an antioxidant (Jukes, 1983). Based on this, selenoglucosinolate related compounds were analyzed for their putative redox-modulatory properties: Preliminary studies focused on the effects of artificial phenylalkyl isoselenocyanates. In cell culture, these compounds were shown to be more cytotoxic compared to natural phenylalkyl isothiocyanates, to induce more apoptosis, and to inhibit cell proliferation of human melanoma cells more strongly (Sharma et al., 2008). Further, in a melanoma mouse model they reduced tumor size more efficiently than S-containing analogs (Sharma et al., 2008). The higher anticancer activity of isoselenocyanates was linked to their faster reaction with thiols such as glutathione and their more efficient modulation of the cellular redox status compared to isothiocyanates (Crampsie et al., 2012). Moreover, these artificial compounds effectively decrease Akt-3 signaling in mouse melanoma cells (Sharma et al., 2009) as well as in different xenograft models (Nguyen et al., 2011). Prostate apoptosis protein-4 can further enhance the antitumor activity of the phenylbutyl isoselenocyanate (ISC-4; Sharma et al., 2011) and a synergistic interaction of ISC-4 with the tumor therapeutic agent cetuximab was reported for colon cancer cells and a related xenograft model (Allen et al., 2013). The artificial 4-(methylsulphinyl)butyl isoselenocyanate (ISC-SFN) showed stronger induction of the redox-sensitive transcription factor Nrf2 compared to the corresponding isothiocyanate sulphoraphane (SFN; Emmert et al., 2010). Moreover, ISC-SFN was more cytotoxic to malignant cells but less toxic to non-cancer cells compared to SFN (Emmert et al., 2010). Recently, it was shown that derivatization of ISC-SFN with organofluorine substitutes can further enhance the selective toxicity toward tumor cells (Cierpial et al., 2016). Thus, selenoglucosinolates as possible precursors for related compounds studied in in vitro and xenograft models might have a relevant anti-cancer potential, which should be further analyzed in the future.
Selenoglucosinolate Related Compounds and Their Effects on the Immune System
Dietary Se plays an important role in inflammation and immunity. Selenium deficiency can lead to significant impairment of immune function and an increased susceptibility to infection and chronic disease (Calder and Kew, 2002). Our current knowledge suggests the effects of Se on the immune system are predominantly mediated through Se incorporation into selenoproteins (Huang et al., 2012). These selenoproteins can initiate or enhance immunity and some are involved in immune regulation.
Se enrichment of the diet is a subject of considerable debate. There is good evidence that supplementation between 100 and 200 μg day−1 can be beneficial to immune function, e.g., enhanced cellular immune response and restored age-related decline in immune response in elderly patients (reviewed in Rayman et al., 2008). A study looking at Se supplementation in prawns showed increased phagocytic activity and increased respiratory burst, whilst also inducing a range of antioxidant selenoproteins (Chiu et al., 2010). This highlights the interesting paradox of Se regulating oxidation status (redox tone) in either direction e.g., reducing oxidation status or triggering oxidation. Selenylation of plant polysaccharides, known to be immune-stimulatory, were found to have enhanced immune activity in vitro (peripheral lymphocytes) and in vivo (chickens) compared to their un-selenated forms (Li et al., 2016), whilst Se-enriched Lactobacillus brevis induced interferon γ and interleukin 17 secretion and increased natural killer cell activity in mice compared to L. brevis alone (Yazdi et al., 2012). Both studies indicate the Se predominate effect may be immune enhancement rather than regulation.
Substantial evidence exists that both Se and Brassica compounds, mainly isothiocyanates, impact the immune response through mechanisms predominantly involving oxidation status (redox tone). There does appear to be some opposing effects of Se and isothiocyanates. The former is more commonly related to immune enhancing effects (Hoffmann and Berry, 2008) whilst the latter is linked to downregulation of immune signals (Wagner et al., 2013). Research investigating the effects of Se-enriched broccoli on immune response produced by peripheral blood mononuclear cells challenged ex vivo indicated that the overriding effect was immune-stimulatory (Bentley-Hewitt et al., 2014). This study involved participants consuming one serving of control broccoli or Se-enriched broccoli (200 μg Se) for 3 days with a wash-out period between dietary interventions. Plasma Se significantly increased from a baseline of 96 ± 4 ng ml−1 to 110 ± 3 ng ml−1 after Se-enriched broccoli consumption, along with cytokines interleukin-2 and interleukin-4 production from participants' peripheral blood mononuclear cells when stimulated with phorbol 12-myristate 13-acetate and ionomycin, whilst no increases were observed following consumption of control broccoli (Figure 5). Additionally Se-enriched radish sprouts were found to be immune-stimulatory in hens (Hossain et al., 2010). In contrast, a study testing Se-enriched sauerkraut extracts on a macrophage cell line in vitro showed anti-inflammatory effects (Peñas et al., 2012), highlighting a major discrepancy between in vivo and in vitro studies. It appears that the anti-inflammatory effects of Brassica phytochemicals may predominate in vitro. This does not explain why Se supplementation of Brassicas in Peñas et al. (2012) have enhanced anti-inflammatory activity compared to the same Brassica extracts that were not supplemented with Se. However, it suggests that digestion, absorption, Se status, modification of the bioactive compounds by other cell types and/or the complexity of cellular cross talk in vivo may have a great influence on how immune cells respond to the bioactive compounds in Se-enriched Brassicas. The in vitro study by Peñas et al. (2012) includes an extraction procedure in an attempt to isolate the glucosinolate hydrolysis products for testing on a macrophage cell line. Therefore, it is possible that alternative seleno compounds that were removed in the process may drive the immuno-stimulatory effect in vivo e.g., seleno amino acids. To start to elucidate the reasons for the discrepancy between in vitro and in vivo results, one could use in vitro digestion of Se-enriched Brassica prior to exposure to cells in vitro and extract material to include seleno amino acids. Previous research, utilized in vitro digestion of Se-enriched broccoli before testing with colon cancer cells and found a reduction in H2O2 production, however no inflammatory markers were measured (Tsai et al., 2013). At present, information into the effects of Se-enriched Brassicas on immune respones particulary human in vivo data is limited.
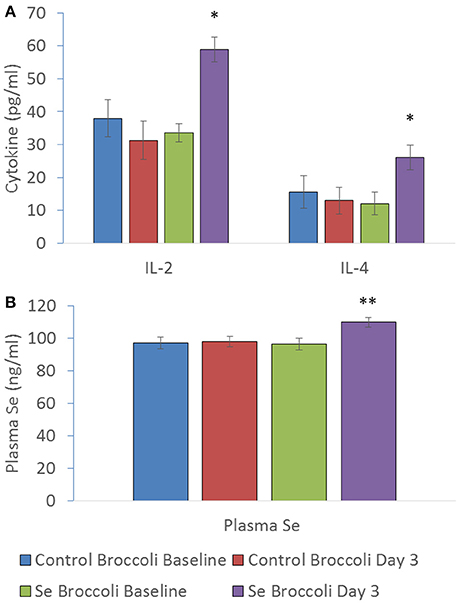
Figure 5. (A) IL-2 (pg/ml), IL-4 (pg/ml) and (B) plasma selenium (ng/ml) at baseline of control broccoli feeding (C0), end of 3 days of control broccoli feeding (C3), baseline of Se-enriched broccoli feeding (S0) and end of 3 days of Se-enriched broccoli feeding (S3). Results are shown as mean (n = 18) ± SE and significance of change between differences seen in both weeks ((S3-S0)-(C3-C0)) are indicated by *p < 0.05 and **p < 0.001 (reformatted from Bentley-Hewitt et al., 2014).
We still do not know the impact of all the Se-containing bioactives, which may be driving the immune response. Research should focus on whether naturally occuring selenoglucosinolate hydrolysis products and amino acids are more bioactive than the S-containing analogs. Additionally, research is required to ascertain whether modification to immune signals, such as increased levels of cytokines, results in a more robust immune response in humans.
Conclusion/Future View
Selenium deficiency or suboptimal Se intake is still regarded as a major health problem for about one billion people worldwide, while an even larger number may consume less Se than required for optimal protection against cancer, cardiovascular diseases, and severe infectious diseases (Haug et al., 2007). Furthermore, due to climate change and climate-soil-interactions, a global Se soil loss of about 8% is predicted by 2099 (Jones et al., 2017). These Se losses will have a higher impact on human health than predicted because Se losses for cropland and pasture are predicted to be 66 and 61%, respectively. These Se losses would be expected to increase global Se deficiency in humans further since the main Se sources for humans are plants and livestock grown on this land.
Due to their distinct biodiversity, Brassica vegetables are consumed regularly worldwide. Thus, Se-biofortification of Brassica crops is an important biotechnological tool that can be used to benefit of Se nutrition in humans. Furthermore, beside the general Se-metabolites, such as seleno-proteins and seleno aminoacids, which can be found in most plant species, Brassicales also contain specialized Se-containing compounds with health benefiting properties such as MeSeCys. Also, the recent discovery of significant amounts of the selenoglucosinolates in biofortified broccoli is encouraging as it opens a further avenue for the production of potentially health promoting compounds in this genera. However, so far no investigations have been conducted on the biosynthesis of selenoglucosinolates or on their bioefficacy in human health, which would be of particular interest due to the distinct protective potential of their potential hydrolysis products.
Increasing Se uptake by the Brassicales is best achieved via hydroponic means, where Se exposure can be carefully controlled and uptake maximized. For crops already grown using hydroponic or similar systems, such as drip lines, Se biofortification should be relatively easy to implement. However, alongside this it will be critical to consider issues relating to Se toxicity, as even Brassicales of the same species can have variable Se uptake rates. This means that a “one size fits all” approach cannot be implemented and biofortificaiton regimes will have to be established for each crop and over the entire plant growth period in order to produce material with a known and stable Se content. Despite this, Se fertilization strategies have been successfully achieved in Finland to counter Se deficiency and should be investigated by other countries where the malnutrition of Se effects the human health of their population.
Although, critical Se intake levels have already been determined with respect to Se undernourishment, we still need to understand the modes of action of individual Se-compounds in human metabolism before recommendations can be made for specific diseases. So far, no daily intake recommendation is established regarding the prevention of chronic diseases such as cancer or the maintenance of a well-regulated immune system. Moreover, further investigations are required to better understand the bioavailability and molecular effects of the selenoglucosinolates before determining their effective concentration for protection against chronic diseases. Achieving these goals will further establish the role Se plays in supporting human health, particularly through members of the Brassicales.
Author Contributions
MW: Corresponding author; MS, FSH, DS, MM: Section Selenium in Brassicales; SB and DR: Section Instrumental Approaches for Detecting, Measuring, and Monitoring Selenium and Its Metabolites within Brassica Species; AK, KBH, FSH, MW: Section Selenoglucosiolates for Human Nutrition.
Conflict of Interest Statement
The authors declare that the research was conducted in the absence of any commercial or financial relationships that could be construed as a potential conflict of interest.
Acknowledgments
The authors thank Royal Society Te Aparangi for funding provided by the Catalyst Seeding Fund (Project number 16-PAF-003-CSG) as well as the German Federal Office for Agriculture and Food (Project number 03/14-15-NZL) allowing this review to be written. The authors declare that all appropriate permissions have been obtained from the copyright holders of any work that has been reproduced in the manuscript (Figures 3, 5).
Footnotes
References
Abd Allah, E. F., Abeer, H., and Alqarawi, A. A. (2016). Mitigation of cadmium induced stress in tomato (Solanum lycopersicum L.) by selenium. Pakistan J. Bot. 48, 953–961.
Agerbirk, N., and Olsen, C. E. (2012). Glucosinolate structures in evolution. Phytochemistry 77, 16–45. doi: 10.1016/j.phytochem.2012.02.005
Ajwa, H. A., Banuelos, G. S., and Mayland, H. F. (1998). Selenium uptake by plants from soils amended with inorganic and organic materials. J. Environ. Qual. 27, 1218–1227. doi: 10.2134/jeq1998.00472425002700050029x
Alfthan, G., Aspila, P., Ekholm, P., Eurola, M., Hartikainen, H., Hero, H., et al. (2011). “Nationwide supplementation of sodium selenate to commercial fertilizers: history and 25-year results from the Finnish Selenium Monitoring Programme,” in Combating Micronutrient Deficiencies: Food Based Approaches, eds. B. Thompson and L. Amoroso (Rome: Food and Agriculture Organization of the United Nations CAB International), 400.
Alfthan, G., Eurola, M., Ekholm, P., Venäläinen, E.-R., Root, T., Korkalainen, K., et al. (2015). Effects of nationwide addition of selenium to fertilizers on foods, and animal and human health in finland: from deficiency to optimal selenium status of the population. J. Trace Elem. Med. Biol. 31, 142–147. doi: 10.1016/j.jtemb.2014.04.009
Allen, J. E., Gallant, J.-N., Dicker, D. T., Amin, S., Irby, R. B., Sharma, A. K., et al. (2013). The akt inhibitor isc-4 synergizes with cetuximab in 5-fu-resistant colon cancer. PLoS ONE 8:e59380. doi: 10.1371/annotation/aaa12360-0c90-42c3-bc43-d00022b68b81
Araie, H., and Shiraiwa, Y. (2016). “Selenium in algae,” in The Physiology of Microalgae, eds M. A. Borowitzka, J. Beardall, and J. A. Raven (Heidelberg: Springer), 281–288.
Arscott, S., and Goldman, I. (2012). Biomass effects and selenium accumulation in sprouts of three vegetable species grown in selenium-enriched conditions. Hortscience 47, 497–502.
Arthur, M. A., Rubin, G., Woodbury, P. B., Schneider, R. E., and Weinstein, L. H. (1992). Uptake and accumulation of selenium by terrestrial plants growing on a coal fly-ash landfill 2. Forage and root crops. Environ. Toxicol. Chem. 11, 1289–1299. doi: 10.1002/etc.5620110909
Augustine, R., Arya, G. C., Nambiar, D. M., Kumar, R., and Bisht, N. C. (2014). Translational genomics in Brassica crops: challenges, progress, and future prospects. Plant Biotechnol. Rep. 8, 65–81. doi: 10.1007/s11816-013-0298-8
Aureli, F., Ouerdane, L., Bierla, K., Szpunar, J., Prakash, N. T., and Cubadda, F. (2012). Identification of selenosugars and other low-molecular weight selenium metabolites in high-selenium cereal crops. Metallomics 4, 968–978. doi: 10.1039/c2mt20085f
Ávila, F. W., Faquin, V., Yang, Y., Ramos, S. J., Guilherme, L. R. G., Thannhauser, T. W., et al. (2013). Assessment of the anticancer compounds Se-methylselenocysteine and glucosinolates in Se-biofortified broccoli (Brassica oleracea L. var. italica) sprouts and florets. J. Agric. Food Chem. 61, 6216–6223. doi: 10.1021/jf4016834
Ávila, F. W., Yang, Y., Faquin, V., Ramos, S. J., Guilherme, L. R. G., Thannhauser, T. W., et al. (2014). Impact of selenium supply on Se-methylselenocysteine and glucosinolate accumulation in selenium-biofortified Brassica sprouts. Food Chem. 165, 578–586. doi: 10.1016/j.foodchem.2014.05.134
Bañuelos, G. S. (1996). Managing high levels of boron and selenium with trace element accumulator crops. J. Environ. Sci. Health A Environ. Sci. Eng. Toxic Hazard. Subst. Control 31, 1179–1196.
Bañuelos, G. S. (2002). Irrigation of broccoli and canola with boron- and selenium-laden effluent. J. Environ. Qual. 31, 1802–1808.
Bañuelos, G. S., Ajwa, H. A., Wu, L., Guo, X., Akohoue, S., and Zambrzuski, S. (1997). Selenium-induced growth reduction in Brassica land races considered for phytoremediation. Ecotoxicol. Environ. Saf. 36, 282–287.
Bañuelos, G. S., and Meek, D. W. (1989). Selenium accumulation in selected vegetables. J. Plant Nutr. 12, 1255–1272.
Bañuelos, G. S., Arroyo, I. S., Dangi, S. R., and Zambrano, M. C. (2016). Continued selenium biofortification of carrots and broccoli grown in soils once amended with se-enriched S. pinnata. Front. Plant Sci. 7:1251. doi: 10.3389/fpls.2016.01251
Bañuelos, G. S., Arroyo, I., Pickering, I. J., Yang, S. I., and Freeman, J. L. (2015). Selenium biofortification of broccoli and carrots grown in soil amended with Se-enriched hyperaccumulator Stanleya pinnata. Food Chem. 166, 603–608. doi: 10.1016/j.foodchem.2014.06.071
Bañuelos, G. S., Meek, D. W., and Hoffman, G. J. (1990). The influence of selenium, salinity, and boron on se uptake in wild mustard. Plant Soil 127, 201–206.
Bañuelos, G. S., Pasakdee, S., and Finley, J. W. (2003). Growth response and selenium and boron distribution in broccoli varieties irrigated with poor quality water. J. Plant Nutr. 26, 2537–2549. doi: 10.1081/PLN-120025477
Bañuelos, G. S., Walse, S. S., Yang, S. I., Pickering, I. J., Fakra, S. C., Marcus, M. A., et al. (2012). Quantification, localization, and speciation of selenium in seeds of canola and two mustard species compared to seed-meals produced by hydraulic press. Anal. Chem. 84, 6024–6030. doi: 10.1021/ac300813e
Bañuelos, G. S., Zambrzuski, S., and Mackey, B. (2000). Phytoextraction of selenium from soils irrigated with selenium-laden effluent. Plant Soil 224, 251–258. doi: 10.1023/A:1004881803469
Bañuelos, G. S., Zayed, A., Terry, N., Wu, L., Akohoue, S., and Zambrzuski, S. (1996). Accumulation of selenium by different plant species grown under increasing sodium and calcium chloride salinity. Plant Soil 183, 49–59.
Bañuelos, G., Terry, N., Leduc, D. L., Pilon-Smits, E. A., and Mackey, B. (2005). Field trial of transgenic indian mustard plants shows enhanced phytoremediation of selenium-contaminated sediment. Environ. Sci. Technol. 39, 1771–1777. doi: 10.1021/es049035f
Barrera, L. N. (2010). Effect of Isothiocyanates and Selenium on Antioxidant Enzyme Expression and DNA Methylationin Colon Cancer Cells In vitro. Ph.D. thesis, University of East Anglia.
Barrera, L. N., Cassidy, A., Johnson, I. T., Bao, Y., and Belshaw, N. J. (2012). Epigenetic and antioxidant effects of dietary isothiocyanates and selenium: potential implications for cancer chemoprevention. Proc. Nutr. Soc. 71, 237–245. doi: 10.1017/S002966511200016X
Bentley-Hewitt, K. L., Chen, R. K. Y., Lill, R. E., Hedderley, D. I., Herath, T. D., Matich, A. J., et al. (2014). Consumption of selenium-enriched broccoli increases cytokine production in human peripheral blood mononuclear cells stimulated ex vivo, a preliminary human intervention study. Mol. Nutr. Food Res. 58, 2350–2357. doi: 10.1002/mnfr.201400438
Bertelsen, F., Gissel-Nielsen, G., Ki1R,, A., and Skrydstrup, T. (1988). Selenoglucosinolates in nature: fact or myth? Phytochemistry 27, 3743–3749. doi: 10.1016/0031-9422(88)83010-3
Bjornstedt, M., Hamberg, M., Kumar, S., Xue, J., and Holmgren, A. (1995). Human thioredoxin reductase directly reduces lipid hydroperoxides by NADPH and selenocystine strongly stimulates the reaction via catalytically generated selenols. J. Biol. Chem. 270, 11761–11764. doi: 10.1074/jbc.270.20.11761
Bjornstedt, M., Kumar, S., and Holmgren, A. (1992). Selenodiglutathione is a highly efficient oxidant of reduced thioredoxin and a substrate for mammalian thioredoxin reductase. J. Biol. Chem. 267, 8030–8034.
Boldrin, P. F., De Figueiredo, M. A., Yang, Y., Luo, H. M., Giri, S., Hart, J. J., et al. (2016). Selenium promotes sulfur accumulation and plant growth in wheat (Triticum aestivum). Physiol. Plant. 158, 80–91. doi: 10.1111/ppl.12465
Brigelius-Flohe, R., and Maiorino, M. (2013). Glutathione peroxidases. Biochim. Biophys. Acta 1830, 3289–3303. doi: 10.1016/j.bbagen.2012.11.020
Broadley, M. R., White, P. J., Bryson, R. J., Meacham, M. C., Bowen, H. C., Johnson, S. E., et al. (2006). Biofortification of uk food crops with selenium. Proc. Nutr. Soc. 65, 169–181. doi: 10.1079/PNS2006490
Brown, T. A., and Shrift, A. (1981). Exclusion of selenium from proteins of selenium-tolerant Astragalus species. Plant Physiol. 67, 1051–1053. doi: 10.1104/pp.67.5.1051
Brown, T. A., and Shrift, A. (1982). Selenium: toxicity and tolerance in higher plants. Biol. Rev. 57, 59–84. doi: 10.1111/j.1469-185X.1982.tb00364.x
Broyer, T. C., Johnson, C. M., and Huston, R. P. (1972). Selenium and nutrition of Astragalus. Plant Soil 36, 635–649. doi: 10.1007/BF01373513
Brummell, D. A., Watson, L. M., Pathirana, R., Joyce, N. I., West, P. J., Hunter, D. A., et al. (2011). Biofortification of tomato (Solanum lycopersicum) fruit with the anticancer compound methylselenocysteine using a selenocysteine methyltransferase from a selenium hyperaccumulator. J. Agric. Food Chem. 59, 10987–10994. doi: 10.1021/jf202583f
Buchner, P., Stuiver, C. E., Westerman, S., Wirtz, M., Hell, R., Hawkesford, M. J., et al. (2004). Regulation of sulfate uptake and expression of sulfate transporter genes in Brassica oleracea as affected by atmospheric H(2)S and pedospheric sulfate nutrition. Plant Physiol. 136, 3396–3408. doi: 10.1104/pp.104.046441
Calder, P. C., and Kew, S. (2002). The immune system: a target for functional foods? Br. J. Nutr. 88(Suppl. 2), S165–177. doi: 10.1079/BJN2002682
Campbell, L., Howie, F., Arthur, J. R., Nicol, F., and Beckett, G. (2007). Selenium and sulforaphane modify the expression of selenoenzymes in the human endothelial cell line EA.hy926 and protect cells from oxidative damage. Nutrition 23, 138–144. doi: 10.1016/j.nut.2006.10.006
Cappa, J. J., and Pilon-Smits, E. A. (2014). Evolutionary aspects of elemental hyperaccumulation. Planta 239, 267–275. doi: 10.1007/s00425-013-1983-0
Cappa, J. J., Yetter, C., Fakra, S., Cappa, P. J., Detar, R., Landes, C., et al. (2015). Evolution of selenium hyperaccumulation in stanleya (brassicaceae) as inferred from phylogeny, physiology and x-ray microprobe analysis. New Phytol. 205, 583–595. doi: 10.1111/nph.13071
Cataldi, T. R., Lelario, F., Orlando, D., and Bufo, S. A. (2010). Collision-induced dissociation of the A + 2 isotope ion facilitates glucosinolates structure elucidation by electrospray ionization-tandem mass spectrometry with a linear quadrupole ion trap. Anal. Chem. 82, 5686–5696. doi: 10.1021/ac100703w
Chan, Q. L., Afton, S. E., and Caruso, J. A. (2010). Investigation of selenium metabolites in se-enriched kale, Brassica oleracea a, via HPLC-ICPMS and nanoESI-ITMS. J. Anal. Atom. Spectrom. 25, 186–192. doi: 10.1039/B914157J
Chang, P., and Randle, W. M. (2006). Influence of temperature on selenium and sulphur accumulation in Brassica oleracea L. J. Horticult. Sci. Biotechnol. 81, 754–758. doi: 10.1080/14620316.2006.11512133
Charron, C. S., Kopsell, D. A., Randle, W. M., and Sams, C. E. (2001). Sodium selenate fertilisation increases selenium accumulation and decreases glucosinolate concentration in rapid-cycling Brassica oleracea. J. Sci. Food Agric. 81, 962–966. doi: 10.1002/jsfa.906
Chen, X., Yang, G., Chen, J., Chen, X., Wen, Z., and Ge, K. (1980). Studies on the relations of selenium and Keshan disease. Biol. Trace Elem. Res. 2, 91–107. doi: 10.1007/BF02798589
Chiu, S.-T., Hsieh, S.-L., Yeh, S.-P., Jian, S.-J., Cheng, W., and Liu, C.-H. (2010). The increase of immunity and disease resistance of the giant freshwater prawn, Macrobrachium rosenbergii by feeding with selenium enriched-diet. Fish Shellfish Immunol. 29, 623–629. doi: 10.1016/j.fsi.2010.06.012
Cierpial, T., Luczak, J., Kwiatkowska, M., Kielbasinski, P., Mielczarek, L., Wiktorska, K., et al. (2016). Organofluorine isoselenocyanate analogues of sulforaphane: Synthesis and anticancer activity. ChemMedChem 11, 2398–2409. doi: 10.1002/cmdc.201600442
Crampsie, M. A., Pandey, M. K., Desai, D., Spallholz, J., Amin, S., and Sharma, A. K. (2012). Phenylalkyl isoselenocyanates vs phenylalkyl isothiocyanates: thiol reactivity and its implications. Chem. Biol. Interact. 200, 28–37. doi: 10.1016/j.cbi.2012.08.022
Curtin, D., Hanson, R., Lindley, T., and Butler, R. (2006). Selenium concentration in wheat (Triticum aestivum) grain as influenced by method, rate, and timing of sodium selenate application. N. Z. J. Crop Hortic. Sci. 34, 329–339. doi: 10.1080/01140671.2006.9514423
De Souza, M. P., Chu, D., Zhao, M., Zayed, A. M., Ruzin, S. E., Schichness, D., et al. (1999). Rhizosphere bacteria enhance selenium accumulation and volatilization by Indian mustard. Plant Physiol. 119, 565–573. doi: 10.1104/pp.119.2.565
De Souza, M. P., Pilon-Smits, E. A., Lytle, C. M., Hwang, S., Tai, J., Honma, T. S. U., et al. (1998). Rate-limiting steps in selenium assimilation and volatilization by Indian mustard. Plant Physiol. 117, 1487–1494. doi: 10.1104/pp.117.4.1487
De Temmerman, L., Waegeneers, N., Thiry, C., Du Laing, G., Tack, F., and Ruttens, A. (2014). Selenium content of Belgian cultivated soils and its uptake by field crops and vegetables. Sci. Total Environ. 468, 77–82. doi: 10.1016/j.scitotenv.2013.08.016
Death, O. A., Gilbert, C. S., and Epson, H. F. (1940). The use of indicator plants in locating seleniferous areas in western United States. III. Further studies. Am. J. Bot. 27, 564–573. doi: 10.2307/2437092
Dhillon, K. S., and Dhillon, S. K. (2009). Accumulation and distribution of selenium in some vegetable crops in selenate-se treated clay loam soil. Front Agric. China 3, 366–373. doi: 10.1007/s11703-009-0070-6
EFSA Panel on Dietetic Products, Nutrition and Allergies (NDA). (2014). Scientific opinion on dietary reference values for selenium. EFSA J. 12:3846. doi: 10.2903/j.efsa.2014.3846
El Mehdawi, A. F., and Pilon-Smits, E. A. (2012). Ecological aspects of plant selenium hyperaccumulation. Plant Biol. 14, 1–10. doi: 10.1111/j.1438-8677.2011.00535.x
El-Ramady, H., Abdalla, N., Taha, H. S., Alshaal, T., El-Henawy, A., Faizy, S. E. D. A., et al. (2015). Selenium and nano-selenium in plant nutrition. Environ. Chem. Lett. 14, 123–147. doi: 10.1007/s10311-015-0535-1
Emmert, S. W., Desai, D., Amin, S., and Richie Jr, J. P. (2010). Enhanced Nrf2-dependent induction of glutathione in mouse embryonic fibroblasts by isoselenocyanate analog of sulforaphane. Bioorg. Med. Chem. Lett. 20, 2675–2679. doi: 10.1016/j.bmcl.2010.01.044
Fallovo, C., Schreiner, M., Schwarz, D., Colla, G., and Krumbein, A. (2011). Phytochemical changes induced by different nitrogen supply forms and radiation levels in two leafy Brassica species. J. Agric. Food Chem. 59, 4198–4207. doi: 10.1021/jf1048904
FAO/WHO (2002). “Selenium,” in Human Vitamin and Mineral Requirements, eds World Health Organization and Food and Agriculture Organization of the United Nations (Rome: FAO), 235–255.
Farnham, M. W., Hale, A. J., Grusak, M. A., and Finley, J. W. (2007). Genotypic and environmental effects on selenium concentration of broccoli heads grown without supplemental selenium fertilizer. Plant Breed. Z. Pflanzenzucht. 126, 195–200. doi: 10.1111/j.1439-0523.2007.01294.x
Feng, R., Wei, C., and Tu, S. (2013). The roles of selenium in protecting plants against abiotic stresses. Environ. Exp. Bot. 87, 58–68. doi: 10.1016/j.envexpbot.2012.09.002
Finley, J. W., Ip, C., Lisk, D. J., Davis, C. D., Hintze, K. J., and Whanger, P. D. (2001). Cancer-protective properties of high-selenium broccoli. J. Agric. Food Chem. 49, 2679–2683. doi: 10.1021/jf0014821
Freeman, J. L., Lindblom, S. D., Quinn, C. F., Fakra, S., Marcus, M. A., and Pilon-Smits, E. A. (2007). Selenium accumulation protects plants from herbivory by Orthoptera via toxicity and deterrence. New Phytol. 175, 490–500. doi: 10.1111/j.1469-8137.2007.02119.x
Freeman, J. L., Quinn, C. F., Marcus, M. A., Fakra, S., and Pilon-Smits, E. A. (2006a). Selenium-tolerant diamondback moth disarms hyperaccumulator plant defense. Curr. Biol. 16, 2181–2192. doi: 10.1016/j.cub.2006.09.015
Freeman, J. L., Zhang, L. H., Marcus, M. A., Fakra, S., McGrath, S. P., and Pilon-Smits, E. A. (2006b). Spatial imaging, speciation, and quantification of selenium in the hyperaccumulator plants Astragalus bisulcatus and Stanleya pinnata. Plant Physiol. 142, 124–134. doi: 10.1104/pp.106.081158
Funes-Collado, V., Morell-Garcia, A., Rubio, R., and Lopez-Sanchez, J. F. (2013). Selenium uptake by edible plants from enriched peat. Sci. Horticult. 164, 428–433. doi: 10.1016/j.scienta.2013.09.052
Galeas, M. L., Zhang, L. H., Freeman, J. L., Wegner, M., and Pilon-Smits, E. A. (2007). Seasonal fluctuations of selenium and sulfur accumulation in selenium hyperaccumulators and related nonaccumulators. New Phytol. 173, 517–525. doi: 10.1111/j.1469-8137.2006.01943.x
Ganther, H. E. (1999). Selenium metabolism, selenoproteins and mechanisms of cancer prevention: complexities with thioredoxin reductase. Carcinogenesis 20, 1657–1666. doi: 10.1093/carcin/20.9.1657
Gigolashvili, T., and Kopriva, S. (2014). Transporters in plant sulfur metabolism. Front. Plant Sci. 5:442. doi: 10.3389/fpls.2014.00442
Guzmán-Pérez, V., Bumke-Vogt, C., Schreiner, M., Mewis, I., Borchert, A., and Pfeiffer, A. F. (2016). Benzylglucosinolate derived isothiocyanate from Tropaeolum majus reduces gluconeogenic gene and protein expression in human cells. PLoS ONE 11:e0162397. doi: 10.1371/journal.pone.0162397
Hanschen, F. S., Lamy, E., Schreiner, M., and Rohn, S. (2014). Reactivity and stability of glucosinolates and their breakdown products in foods. Angewandte Chemie. International Ed. 53, 11430–11450. doi: 10.1002/anie.201402639
Hartfiel, W., Lux, H., Lux, J., and Gentges, K. (2010). Bedeutung von Selen. Ernährung Medizin 25, 129–133. doi: 10.1055/s-0030-1248863
Hartikainen, H., Pietola, L., and Simojoki, A. (2001). Quantification of fine root responses to selenium toxicity. Agric. Food Sci. 10, 53–58.
Hartikainen, H., Xue, T. L., and Piironen, V. (2000). Selenium as an anti-oxidant and pro-oxidant in ryegrass. Plant Soil 225, 193–200. doi: 10.1023/A:1026512921026
Haug, A., Graham, R. D., Christophersen, O. A., and Lyons, G. H. (2007). How to use the world's scarce selenium resources efficiently to increase the selenium concentration in food. Microb. Ecol. Health Dis. 19, 209–228. doi: 10.1080/08910600701698986
Hawkesford, M. J. (2003). Transporter gene families in plants: the sulphate transporter gene family — redundancy or specialization? Physiol. Plant. 117, 155–163. doi: 10.1034/j.1399-3054.2003.00034.x
Hawkesford, M. J., Buchner, P., Howarth, J. R., and Lu, C. (2005). Understanding the regulation of sulfur nutrition - from sulfate transporter genes to the field. Phyton 45, 57–67.
Hawkesford, M. J., Davidian, J.-C., and Grignon, C. (1993). Sulphate/proton cotransport in plasma-membrane vesicles isolated from roots of Brassica napus L.: increased transport in membranes isolated from sulphur-starved plants. Planta 190, 297–304. doi: 10.1007/BF00196957
Hoffmann, P. R., and Berry, M. J. (2008). The influence of selenium on immune responses. Mol. Nutr. Food Res. 52, 1273–1280. doi: 10.1002/mnfr.200700330
Hopper, J. L., and Parker, D. R. (1999). Plant availability of selenite and selenate as influenced by the competing ions phosphate and sulfate. Plant Soil 210, 199–207. doi: 10.1023/A:1004639906245
Hossain, M. S., Afrose, S., Takeda, I., and Tsujii, H. (2010). Effect of selenium-enriched Japanese radish sprouts and Rhodobacter capsulatus on the cholesterol and immune response of laying hens. Asian Austral. J. Anim. 23, 630–639. doi: 10.5713/ajas.2010.90394
Hsu, F., Wirtz, M., Heppel, S. C., Bogs, J., Kramer, U., Khan, M. S., et al. (2011). Generation of Se-fortified broccoli as functional food: impact of Se fertilization on S metabolism. Plant Cell Environ. 34, 192–207. doi: 10.1111/j.1365-3040.2010.02235.x
Huang, Z., Rose, A. H., and Hoffmann, P. R. (2012). The role of selenium in inflammation and immunity: from molecular mechanisms to therapeutic opportunities. Antioxid. Redox Signal. 16, 705–743. doi: 10.1089/ars.2011.4145
Jaiswal, S. K., Prakash, R., Acharya, R., Reddy, A. V. R., and Prakash, N. T. (2012). Selenium content in seed, oil and oil cake of se hyperaccumulated Brassica juncea (Indian mustard) cultivated in a seleniferous region of India. Food Chem. 134, 401–404. doi: 10.1016/j.foodchem.2012.02.140
Jones, G. D., Droz, B., Greve, P., Gottschalk, P., Poffet, D., McGrath, S. P., et al. (2017). Selenium deficiency risk predicted to increase under future climate change. Proc. Natl. Acad. Sci. U.S.A. 114, 2848–2853. doi: 10.1073/pnas.1611576114
Kim, S.-J., Matsuo, T., Watanabe, M., and Watanabe, Y. (2002). Effect of nitrogen and sulphur application on the glucosinolate content in vegetable turnip rape (Brassica rapa L.). Soil Sci. Plant Nutr. 48, 43–49. doi: 10.1080/00380768.2002.10409169
Kissen, R., Rossiter, J. T., and Bones, A. M. (2009). The ‘mustard oil bomb’: Not so easy to assemble?! Localization, expression and distribution of the components of the myrosinase enzyme system. Phytochem. Rev. 8, 69–86. doi: 10.1007/s11101-008-9109-1
Kjær, A., and Skrydstrup, T. (1987). Selenoglucosinolates: SYNTHESIS and enzymatic hydrolysis. Acta Chem. Scand. 41b, 29–33. doi: 10.3891/acta.chem.scand.41b-0029
Kopsell, D. A., and Randle, W. M. (1999). Selenium accumulation in a rapid-cycling Brassica oleracea population responds to increasing sodium selenate concentrations. J. Plant Nutr. 22, 927–937. doi: 10.1080/01904169909365683
Kopsell, D. A., and Randle, W. M. (2001). Genetic variances and selection potential for selenium accumulation in a rapid-cycling Brassica oleracea population. J. Am. Soc. Hort. Sci. 126, 329–335.
Krehl, S., Loewinger, M., Florian, S., Kipp, A. P., Banning, A., Wessjohann, L. A., et al. (2012). Glutathione peroxidase-2 and selenium decreased inflammation and tumors in a mouse model of inflammation-associated carcinogenesis whereas sulforaphane effects differed with selenium supply. Carcinogenesis 33, 620–628. doi: 10.1093/carcin/bgr288
Kubachka, K. M., Meija, J., Leduc, D. L., Terry, N., and Caruso, J. A. (2007). Selenium volatiles as proxy to the metabolic pathways of selenium in genetically modified Brassica juncea. Environ. Sci. Technol. 41, 1863–1869. doi: 10.1021/es0613714
Leduc, D. L., Abdelsamie, M., Montes-Bayon, M., Wu, C. P., Reisinger, S. J., and Terry, N. (2006). Overexpressing both atp sulfurylase and selenocysteine methyltransferase enhances selenium phytoremediation traits in indian mustard. Environ. Pollut. 144, 70–76. doi: 10.1016/j.envpol.2006.01.008
Leduc, D. L., Tarun, A. S., Montes-Bayon, M., Meija, J., Malit, M. F., Wu, C. P., et al. (2004). Overexpression of selenocysteine methyltransferase in Arabidopsis and Indian mustard increases selenium tolerance and accumulation. Plant Physiol. 135, 377–383. doi: 10.1104/pp.103.026989
Lee, J., Finley, J. W., and Harnly, J. A. (2005). Effect of selenium fertilizer on free amino acid composition of broccoli (Brassica oleracea cv. Majestic) determined by gas chromatography with flame ionization and mass selective detection. J. Agric. Food Chem. 53, 9105–9111. doi: 10.1021/jf051221x
Lelario, F., Bianco, G., Bufo, S. A., and Cataldi, T. R. I. (2012). Establishing the occurrence of major and minor glucosinolates in Brassicaceae by LC-ESI-hybrid linear ion-trap and Fourier-transform ion cyclotron resonance mass spectrometry. Phytochemistry 73, 74–83. doi: 10.1016/j.phytochem.2011.09.010
Li, H. F., McGrath, S. P., and Zhao, F. J. (2008). Selenium uptake, translocation and speciation in wheat supplied with selenate or selenite. New Phytol. 178, 92–102. doi: 10.1111/j.1469-8137.2007.02343.x
Li, S., Schonhof, I., Krumbein, A., Li, L., Stützel, H., and Schreiner, M. (2007). Glucosinolate concentration in turnip (Brassica rapa ssp. rapifera L.) roots as affected by nitrogen and sulfur supply. J. Agric. Food Chem. 55, 8452–8457. doi: 10.1021/jf070816k
Li, X., Hou, R., Yue, C., Liu, J., Gao, Z., Chen, J., et al. (2016). The selenylation modification of epimedium polysaccharide and isatis root polysaccharide and the immune-enhancing activity comparison of their modifiers. Biol. Trace Elem. Res. 171, 224–234. doi: 10.1007/s12011-015-0511-4
Lippmann, D., Lehmann, C., Florian, S., Barknowitz, G., Haack, M., Mewis, I., et al. (2014). Glucosinolates from pak choi and broccoli induce enzymes and inhibit inflammation and colon cancer differently. Food Funct. 5, 1073–1081. doi: 10.1039/C3FO60676G
Lobinski, R., Edmonds, J. S., Suzuki, K. T., and Uden, P. C. (2000). Species-selective determination of selenium compounds in biological materials (Technical Report). Pure Appl. Chem. 72, 447–461. doi: 10.1351/pac200072030447
Lyi, S. M., Heller, L. I., Rutzke, M., Welch, R. M., Kochian, L. V., and Li, L. (2005). Molecular and biochemical characterization of the selenocysteine Se-methyltransferase gene and Se-methylselenocysteine synthesis in broccoli. Plant Physiol. 138, 409–420. doi: 10.1104/pp.104.056549
Lyons, G., Stangoulis, J., and Graham, R. (2003). High-selenium wheat: biofortification for better health. Nutr. Res. Rev. 16, 45–60. doi: 10.1079/NRR200255
Mahn, A. (2017). Modelling of the effect of selenium fertilization on the content of bioactive compounds in broccoli heads. Food Chem. 233, 492–499. doi: 10.1016/j.foodchem.2017.04.144
Malagoli, M., Schiavon, M., Dall'acqua, S., and Pilon-Smits, E. A. (2015). Effects of selenium biofortification on crop nutritional quality. Front. Plant Sci. 6:280. doi: 10.3389/fpls.2015.00280
Maneetong, S., Chookhampaeng, S., Chantiratikul, A., Chinrasri, O., Thosaikham, W., Sittipout, R., et al. (2013). Hydroponic cultivation of selenium-enriched kale (Brassica oleracea var. alboglabra L.) seedling and speciation of selenium with HPLC-ICPMS. Microchem. J. 108, 87–91. doi: 10.1016/j.microc.2013.01.003
Manion, L. K., Kopsell, D. E., Kopsell, D. A., Sams, C. E., and Rhykerd, R. L. (2014). Selenium fertilization influences biomass, elemental accumulations, and phytochemical concentrations in watercress. J. Plant Nutr. 37, 327–342. doi: 10.1080/01904167.2013.789110
Matich, A. J., McKenzie, M. J., Brummell, D. A., and Rowan, D. D. (2009). Organoselenides from Nicotiana tabacum genetically modified to accumulate selenium. Phytochemistry 70, 1098–1106. doi: 10.1016/j.phytochem.2009.06.001
Matich, A. J., McKenzie, M. J., Lill, R. E., Brummell, D. A., McGhie, T. K., Chen, R. K., et al. (2012). Selenoglucosinolates and their metabolites produced in Brassica spp. fertilised with sodium selenate. Phytochemistry 75, 140–152. doi: 10.1016/j.phytochem.2011.11.021
Matich, A. J., McKenzie, M. J., Lill, R. E., McGhie, T. K., Chen, R. K., and Rowan, D. D. (2015). Distribution of selenoglucosinolates and their metabolites in Brassica treated with sodium selenate. J. Agric. Food Chem. 63, 1896–1905. doi: 10.1021/jf505963c
McKenzie, M. J., Hunter, D. A., Pathirana, R., Watson, L. M., Joyce, N. I., Matich, A. J., et al. (2009). Accumulation of an organic anticancer selenium compound in a transgenic Solanaceous species shows wider applicability of the selenocysteine methyltransferase transgene from selenium hyperaccumulators. Transgenic Res. 18, 407–424. doi: 10.1007/s11248-008-9233-0
McKenzie, M. J., Lill, R. E., Trolove, S. N., and Brummell, D. A. (2015a). “Biofortification of fruit and vegetables with selenium,” in Selenium: Chemistry, Analysis, Function and Effects, ed V. E. Preedy (Cambridge, UK: Royal Society of Chemistry), 304–323.
McKenzie, M. J., Matich, A. J., Chen, R. K.-Y., Lill, R. E., McGhie, T. K., and Rowan, D. D. (2015b). “Identification and distribution of selenium-containing glucosinolate analogues in tissues of three brassicaceae species,” in Molecular Physiology and Ecophysiology of Sulfur, eds L. J. De Kok, M. Hawkesford, H. Rennenberg, K. Saito, and E. Schnug. (Cham: Springer International Publishing), 239–246.
Moreno-Reyes, R., Suetens, C., Mathieu, F., Begaux, F., Zhu, D., Rivera, M. T., et al. (1998). Kashin–Beck Osteoarthropathy in Rural Tibet in Relation to Selenium and Iodine Status. N. Engl. J. Med. 339, 1112–1120. doi: 10.1056/NEJM199810153391604
Moskovitz, J., Singh, V. K., Requena, J., Wilkinson, B. J., Jayaswal, R. K., and Stadtman, E. R. (2002). Purification and characterization of methionine sulfoxide reductases from mouse and Staphylococcus aureus and their substrate stereospecificity. Biochem. Biophys. Res. Commun. 290, 62–65. doi: 10.1006/bbrc.2001.6171
Nakabayashi, R., Sawada, Y., Yamada, Y., Suzuki, M., Hirai, M. Y., Sakurai, T., et al. (2013). Combination of liquid chromatography–fourier transform ion cyclotron resonance-mass spectrometry with 13C-labeling for chemical assignment of sulfur-containing metabolites in onion bulbs. Anal. Chem. 85, 1310–1315. doi: 10.1021/ac302733c
Navarro-Alarcon, M., and Cabrera-Vique, C. (2008). Selenium in food and the human body: a review. Sci. Tot. Environ. 400, 115–141. doi: 10.1016/j.scitotenv.2008.06.024
Neuhierl, B., and Bock, A. (1996). On the mechanism of selenium tolerance in selenium-accumulating plants - purification and characterization of a specific selenocysteine methyltransferase from cultured cells of Astragalus bisculatus. Eur. J. Biochem. 239, 235–238. doi: 10.1111/j.1432-1033.1996.0235u.x
Neuhierl, B., Thanbichler, M., Lottspeich, F., and Bock, A. (1999). A family of S-methylmethionine-dependent thiol/selenol methyltransferases - role in selenium tolerance and evolutionary relation. J. Biol. Chem. 274, 5407–5414. doi: 10.1074/jbc.274.9.5407
Nguyen, N., Sharma, A., Nguyen, N., Sharma, A. K., Desai, D., Huh, S. J., et al. (2011). Melanoma chemoprevention in skin reconstructs and mouse xenografts using isoselenocyanate-4. Cancer Prev. Res. 4, 248–258. doi: 10.1158/1940-6207.CAPR-10-0106
Oldfield, J. (2002). Selenium World Atlas, Updated Edn. Grimbergen: Selenium-Tellurium Development Association (STDA).
Ouerdane, L., Aureli, F., Flis, P., Bierla, K., Preud'homme, H., Cubadda, F., et al. (2013). Comprehensive speciation of low-molecular weight selenium metabolites in mustard seeds using hplc - electrospray linear trap/orbitrap tandem mass spectrometry. Metallomics 5, 1294–1304. doi: 10.1039/c3mt00113j
Palomo-Siguero, M., Lopez-Heras, M. I., Camara, C., and Madrid, Y. (2015). Accumulation and biotransformation of chitosan-modified selenium nanoparticles in exposed radish (Raphanus sativus). J. Anal. Atom. Spectrom. 30, 1237–1244. doi: 10.1039/C4JA00407H
Papp, L. V., Lu, J., Holmgren, A., and Khanna, K. K. (2007). From selenium to selenoproteins: synthesis, identity, and their role in human health. Antioxid. Redox Signal. 9, 775–806. doi: 10.1089/ars.2007.1528
Patterson, B. H., Zech, L. A., Swanson, C. A., and Levander, O. A. (1993). Kinetic modeling of selenium in humans using stable-isotope tracers. J. Trace Elem. Electrolytes Health Dis. 7, 117–120.
Pedrero, Z., Elvira, D., Camara, C., and Madrid, Y. (2007). Selenium transformation studies during broccoli (Brassica oleracea) growing process by liquid chromatography-inductively coupled plasma mass spectrometry (LC-ICP-MS). Anal. Chim. Acta 596, 251–256. doi: 10.1016/j.aca.2007.05.067
Peñas, E., Martinez-Villaluenga, C., Frias, J., Sánchez-Martínez, M. J., Pérez-Corona, M. T., Madrid, Y., et al. (2012). Se improves indole glucosinolate hydrolysis products content, se-methylselenocysteine content, antioxidant capacity and potential anti-inflammatory properties of sauerkraut. Food Chem. 132, 907–914. doi: 10.1016/j.foodchem.2011.11.064
Pettine, M., McDonald, T. J., Sohn, M., Anquandah, G. A. K., Zboril, R., and Sharma, V. K. (2015). A critical review of selenium analysis in natural water samples. Trends Environ. Anal. Chem. 5, 1–7. doi: 10.1016/j.teac.2015.01.001
Pickering, I. J., Wright, C., Bubner, B., Ellis, D., Persans, M. W., Yu, E. Y., et al. (2003). Chemical form and distribution of selenium and sulfur in the selenium hyperaccumulator Astragalus bisulcatus. Plant Physiol. 131, 1460–1467. doi: 10.1104/pp.014787
Pilon-Smits, E. A. (2005). Phytoremediation. Annu. Rev. Plant Biol. 56, 15–39. doi: 10.1146/annurev.arplant.56.032604.144214
Pilon-Smits, E. A., and Quinn, C. F. (2010). “Selenium metabolism in plants,” in Cell Biology of Metal and Nutrients, eds R. Hell and R. Mendel (Berlin: Springer), 225–241.
Pilon-Smits, E. A., Hwang, S. B., Lytle, C. M., Zhu, Y. L., Tai, J. C., Bravo, R. C., et al. (1999). Overexpression of atp sulfurylase in indian mustard leads to increased selenate uptake, reduction, and tolerance. Plant Physiol. 119, 123–132. doi: 10.1104/pp.119.1.123
Proietti, P., Nasini, L., Del Buono, D., D'amato, R., Tedeschini, E., and Businelli, D. (2013). Selenium protects olive (Olea europaea L.) from drought stress. Sci. Horticult. 164, 165–171. doi: 10.1016/j.scienta.2013.09.034
Quinn, C. F., Prins, C. N., Freeman, J. L., Gross, A. M., Hantzis, L. J., Reynolds, R. J., et al. (2011). Selenium accumulation in flowers and its effects on pollination. New Phytol. 192, 727–737. doi: 10.1111/j.1469-8137.2011.03832.x
Ralston, N. V., Unrine, J., and Wallschläger, D. (2008). Biogeochemistry and Analysis of Selenium and Its Species. Washington, DC: North American Metals Council.
Ramos, S. J., Yuan, Y., Faquin, V., Guilherme, L. R. G., and Li, L. (2011). Evaluation of genotypic variation of broccoli (Brassica oleracea var. italic) in response to selenium treatment. J. Agric. Food Chem. 59, 3657–3665. doi: 10.1021/jf104731f
Rayman, M. P. (2012). Selenium and human health. Lancet 379, 1256–1268. doi: 10.1016/S0140-6736(11)61452-9
Rayman, M. P., Infante, H. G., and Sargent, M. (2008). Food-chain selenium and human health: spotlight on speciation. Br. J. Nutr. 100, 238–253. doi: 10.1017/S0007114508922522
Roman, M., Jitaru, P., and Barbante, C. (2014). Selenium biochemistry and its role for human health. Metallomics 6, 25–54. doi: 10.1039/C3MT00185G
Schiavon, M., and Pilon-Smits, E. A. (2017). The fascinating facets of plant selenium accumulation - biochemistry, physiology, evolution and ecology. New Phytol. 213, 1582–1596. doi: 10.1111/nph.14378
Schonhof, I., Blankenburg, D., Müller, S., and Krumbein, A. (2007). Sulfur and nitrogen supply influence growth, product appearance, and glucosinolate concentration of broccoli. J. Plant Nutr. Soil Sci. 170, 65–72. doi: 10.1002/jpln.200620639
Shah, M., Meija, J., and Caruso, J. A. (2007). Relative mass defect filtering of high-resolution mass spectra for exploring minor selenium volatiles in selenium-enriched green onions. Anal. Chem. 79, 846–853. doi: 10.1021/ac060703k
Sharma, A. K., Kline, C. L., Berg, A., Amin, S., and Irby, R. B. (2011). The akt inhibitor isc-4 activates prostate apoptosis response protein-4 and reduces colon tumor growth in a nude mouse model. Clin. Cancer. Res. 17, 4474–4483. doi: 10.1158/1078-0432.CCR-10-2370
Sharma, A. K., Sharma, A., Desai, D., Madhunapantula, S. V., Huh, S. J., Robertson, G. P., et al. (2008). Synthesis and anticancer activity comparison of phenylalkyl isoselenocyanates with corresponding naturally occurring and synthetic isothiocyanates. J. Med. Chem. 51, 7820–7826. doi: 10.1021/jm800993r
Sharma, A., Sharma, A. K., Madhunapantula, S. V., Desai, D., Huh, S. J., Mosca, P., et al. (2009). Targeting akt3 signaling in malignant melanoma using isoselenocyanates. Clin. Cancer. Res. 15, 1674–1685. doi: 10.1158/1078-0432.CCR-08-2214
Sindelarova, K., Szakova, J., Tremlova, J., Mestek, O., Praus, L., Kana, A., et al. (2015). The response of broccoli (Brassica oleracea convar. italica) varieties on foliar application of selenium: Uptake, translocation, and speciation. Food Addit. Contam. Part A Chem. Anal. Control Expo. Risk Assess. 32, 2027–2038. doi: 10.1080/19440049.2015.1099744
Sors, T. G., Ellis, D. R., and Salt, D. E. (2005). Selenium uptake, translocation, assimilation and metabolic fate in plants. Photosynth. Res. 86, 373–389. doi: 10.1007/s11120-005-5222-9
Spallholz, J. E. (1994). On the nature of selenium toxicity and carcinostatic activity. Free Radic. Biol. Med. 17, 45–64. doi: 10.1016/0891-5849(94)90007-8
Steinbrenner, H., and Sies, H. (2009). Protection against reactive oxygen species by selenoproteins. Biochim. Biophys. Acta 1790, 1478–1485. doi: 10.1016/j.bbagen.2009.02.014
Stewart, J. M., Nigiam, S. N., and McConnel, W. B. (1974). Metabolism of SeO4 in horseradish: formation of selenosinigrin. Can. J. Biochem. 52, 144–145. doi: 10.1139/o74-023
Sugihara, S., Kondo, M., Chihara, Y., Yuji, M., Hattori, H., and Yoshida, M. (2004). Preparation of selenium-enriched sprouts and identification of their selenium species by high-performance liquid chromatography-inductively coupled plasma mass spectrometry. Biosci. Biotechnol. Biochem. 68, 193–199. doi: 10.1271/bbb.68.193
Terry, N., Zayed, A. M., De Souza, M. P., and Tarun, A. S. (2000). Selenium in higher plants. Annu. Rev. Plant Physiol. Plant Mol. Biol. 51:401432. doi: 10.1146/annurev.arplant.51.1.401
Thomson, C. D. (2004). Selenium and iodine intakes and status in New Zealand and Australia. Br. J. Nutr. 91, 661–672. doi: 10.1079/BJN20041110
Tian, M., Xu, X., Liu, Y., Xie, L., and Pan, S. (2016). Effect of Se treatment on glucosinolate metabolism and health-promoting compounds in the broccoli sprouts of three cultivars. Food Chem. 190, 374–380. doi: 10.1016/j.foodchem.2015.05.098
Toler, H. D., Charron, C. S., Sams, C. E., and Randle, W. R. (2007). Selenium increases sulfur uptake and regulates glucosinolate metabolism in rapid-cycling Brassica oleracea. J. Am. Soc. Hort. Sci. 132, 14–19.
Tsai, C. F., Ou, B. R., Liang, Y. C., and Yeh, J. Y. (2013). Growth inhibition and antioxidative status induced by selenium-enriched broccoli extract and selenocompounds in DNA mismatch repair-deficient human colon cancer cells. Food Chem. 139, 267–273. doi: 10.1016/j.foodchem.2013.02.001
Uden, P. C. (2002). Modern trends in the speciation of selenium by hyphenated techniques. Anal. Bioanal. Chem. 373, 422–431. doi: 10.1007/s00216-002-1405-9
Van Hoewyk, D. (2013). A tale of two toxicities: malformed selenoproteins and oxidative stress both contribute to selenium stress in plants. Ann. Bot. 112, 965–972. doi: 10.1093/aob/mct163
Van Huysen, T., Abdel-Ghany, S., Hale, K. L., Leduc, D., Terry, N., and Pilon-Smits, E. A. (2003). Overexpression of cystathionine-gamma-synthase enhances selenium volatilization in Brassica juncea. Planta 218, 71–78. doi: 10.1007/s00425-003-1070-z
Van Huysen, T., Terry, N., and Pilon-Smits, E. A. (2004). Exploring the selenium phytoremediation potential of transgenic indian mustard overexpressing atp sulfurylase or cystathionine-gamma-synthase. Int. J. Phytoremed. 6, 111–118. doi: 10.1080/16226510490454786
Veeranki, O., Bhattacharya, A., Tang, L., Marshall, J., and Zhang, Y. (2015). Cruciferous vegetables, isothiocyanates, and prevention of bladder cancer. Curr. Pharmacol. Rep. 1, 272–282. doi: 10.1007/s40495-015-0024-z
Verkerk, R., Schreiner, M., Krumbein, A., Ciska, E., Holst, B., Rowland, I., et al. (2009). Glucosinolates in Brassica vegetables: the influence of the food supply chain on intake, bioavailability and human health. Mol. Nutr. Food Res. 53:S219. doi: 10.1002/mnfr.200800065
Wagner, A. E., Terschluesen, A. M., and Rimbach, G. (2013). Health promoting effects of Brassica-derived phytochemicals: from chemopreventive and anti-inflammatory activities to epigenetic regulation. Oxid. Med. Cell. Longev. 2013:964539. doi: 10.1155/2013/964539
Wang, Y., Dacosta, C., Wang, W., Zhou, Z., Liu, M., and Bao, Y. (2015). Synergy between sulforaphane and selenium in protection against oxidative damage in colonic CCD841 cells. Nutr. Res. 35, 610–617. doi: 10.1016/j.nutres.2015.05.011
Whanger, P. (2004). Selenium and its relationship to cancer: an update. Br. J. Nutr. 91, 11–28. doi: 10.1079/BJN20031015
Whanger, P. D. (2002). Selenocompounds in plants and animals and their biological significance. J. Am. Coll. Nutr. 21, 223–232. doi: 10.1080/07315724.2002.10719214
White, P. J. (2016). Selenium accumulation by plants. Ann. Bot. 117, 217–235. doi: 10.1093/aob/mcv180
White, P. J., Bowen, H. C., Parmaguru, P., Fritz, M., Spracklen, W. P., Spiby, R. E., et al. (2004). Interactions between selenium and sulphur nutrition in Arabidopsis thaliana. J. Exp. Bot. 55, 1927–1937. doi: 10.1093/jxb/erh192
Whiting, A. F. (1985). Havasupai Habitat: A. F. Whiting's Ethnography of a Traditional Indian Culture. Tuscon: University of Arizona Press.
Winkel, L. H. E., Vriens, B., Jones, G. D., Schneider, L. S., Pilon-Smits, E., and Banuelos, G. S. (2015). Selenium cycling across soil-plant-atmosphere interfaces: a critical review. Nutrients 7, 4199–4239. doi: 10.3390/nu7064199
Ximenez-Embun, P., Alonso, I., Madrid-Albarran, Y., and Camara, C. (2004). Establishment of selenium uptake and species distribution in lupine, Indian mustard, and sunflower plants. J. Agric. Food Chem. 52, 832–838. doi: 10.1021/jf034835f
Xue, T. L., Hartikainen, H., and Piironen, V. (2001). Antioxidative and growth-promoting effect of selenium on senescing lettuce. Plant Soil 237, 55–61. doi: 10.1023/A:1013369804867
Yazdi, M., Mahdavi, M., Kheradmand, E., and Shahverdi, A. (2012). The preventive oral supplementation of a selenium nanoparticle-enriched probiotic increases the immune response and lifespan of 4T1 breast cancer bearing mice. Arzneimittelforschung 62, 525–531. doi: 10.1055/s-0032-1323700
Yuan, L., Zhu, Y., Lin, Z. Q., Banuelos, G., Li, W., and Yin, X. (2013). A novel selenocystine-accumulating plant in selenium-mine drainage area in Enshi, China. PLoS ONE 8:e65615. doi: 10.1371/journal.pone.0065615
Zhang, Y., and Gladyshev, V. N. (2009). Comparative genomics of trace elements: emerging dynamic view of trace element utilization and function. Chem. Rev. 109, 4828–4861. doi: 10.1021/cr800557s
Zhao, C. Y., Ren, J. G., Xue, C. Z., and Lin, E. D. (2005). Study on the relationship between soil selenium and plant selenium uptake. Plant Soil 277, 197–206. doi: 10.1007/s11104-005-7011-9
Keywords: Brassica vegetables, selenium, biofortification, glucosinolates, human health, immune system, cancer, analytical methods
Citation: Wiesner-Reinhold M, Schreiner M, Baldermann S, Schwarz D, Hanschen FS, Kipp AP, Rowan DD, Bentley-Hewitt KL and McKenzie MJ (2017) Mechanisms of Selenium Enrichment and Measurement in Brassicaceous Vegetables, and Their Application to Human Health. Front. Plant Sci. 8:1365. doi: 10.3389/fpls.2017.01365
Received: 13 March 2017; Accepted: 21 July 2017;
Published: 03 August 2017.
Edited by:
Nadia Bertin, Plantes et Système de cultures Horticoles (INRA), FranceReviewed by:
Daniel A. Jacobo-Velázquez, Tecnológico de Monterrey, MexicoKarl Kunert, University of Pretoria, South Africa
Copyright © 2017 Wiesner-Reinhold, Schreiner, Baldermann, Schwarz, Hanschen, Kipp, Rowan, Bentley-Hewitt and McKenzie. This is an open-access article distributed under the terms of the Creative Commons Attribution License (CC BY). The use, distribution or reproduction in other forums is permitted, provided the original author(s) or licensor are credited and that the original publication in this journal is cited, in accordance with accepted academic practice. No use, distribution or reproduction is permitted which does not comply with these terms.
*Correspondence: Melanie Wiesner-Reinhold, wiesner@igzev.de