- 1Área de Fisiología Vegetal, Universitat Politècnica de València, València, Spain
- 2Centro de Biotecnología y Genómica de Plantas, Madrid, Spain
- 3Departamento de Biotecnología, Universitat Politècnica de València, València, Spain
- 4Departamento de Biología, Universitat de les Illes Balears, Palma, Spain
Cycling Dof Factor (CDF) transcription factors (TFs) are involved in multiple processes related to plant growth and development. A member of this family, CDF3, has recently been linked in Arabidopsis to the regulation of primary metabolism and abiotic stress responses, but its role in crop production under stress is still unknown. In this study, we characterized tomato plants overexpressing the CDF3 genes from Arabidopsis and tomato and analyzed their effects on growth and yield under salinity, additionally gaining deeper insights into the molecular function of these TFs. Our results provide evidence for higher biomass production and yield in the 35S::AtCDF3 and 35S::SlCDF3 plants, likely due to a higher photosynthetic capacity resulting in increased sucrose availability. Transcriptome analysis revealed that CDF3 genes regulate a set of genes involved in redox homeostasis, photosynthesis performance and primary metabolism that lead to enhanced biomass production. Consistently, metabolomic profiling revealed that CDF3 evokes changes in the primary metabolism triggering enhanced nitrogen assimilation, and disclosed that the amount of some protective metabolites including sucrose, GABA and asparagine were higher in vegetative tissues of CDF3 overexpressing plants. Altogether these changes improved performance of 35S::AtCDF3 and 35S::SlCDF3 plants under salinity conditions. Moreover, the overexpression of CDF3 genes modified organic acid and sugar content in fruits, improving variables related to flavor perception and fruit quality. Overall, our results associate the CDF3 TF with a role in the control of growth and C/N metabolism, and highlight that overexpression of CDF3 genes can substantially improve plant yield.
Introduction
In the twentieth century, enhancement of crop production was achieved through improving agricultural practices to optimize photoassimilate production, and to increase the harvest index by altering photoassimilate partitioning. However, these strategies appear to have peaked in many crops, and have been achieved without increasing total biomass (Paul and Foyer, 2001). Moreover, the impact of climate change, increasing urbanization, salinity of soils and aquifers, and desertification, limit food production and present an unprecedented challenge (Godfray et al., 2010). In this context, the demand for higher productivity under prevailing conditions requires improving photosynthetic efficiency and biomass production, as well as increased crop tolerance to abiotic factors, to facilitate the usage of marginal lands and partially salinized waters.
Increased leaf photosynthesis is closely associated with increased yields when assaying the growth of a genotype under diverse environmental conditions or a narrow range of germplasm within a certain species (Long et al., 2006). Six potential routes to improve photosynthetic efficiency, which ranged from altered canopy architecture to the improved regeneration of the acceptor molecule for CO2, have been explored by Long et al. (2015). In relation to the light reactions of photosynthesis, the extension of the usable spectrum of light and the improvement of heat dissipation pathways have been proposed as biotechnological targets. Furthermore, the engineering of CO2 concentration pathways, improved Rubisco forms, photorespiration bypasses and the optimization of RuBP regeneration systems have been suggested for the dark reactions of photosynthesis. These authors concluded that the changes to improve the efficiency of converting intercepted light into biomass and, therefore, yield, could be achieved by transgenic technology. Nonetheless, transferring different individual genes of interest is not practical given the number of enzymes involved in a metabolic pathway. Several recent studies have indicated the possibility of using transcription factors (TFs), which control the expression of several genes, to enhance photosynthesis and biomass production (Yanagisawa et al., 2004; Saibo et al., 2009).
Drought, salinity and low temperature are environmental constraints that diminish photosynthetic efficiency and adversely affect plant growth and productivity. These unfavorable growth conditions impose water deficit, and stomatal closure as well as lower CO2 diffusion are the earliest responses (Tezara et al., 1999; Flexas and Medrano, 2002; Saibo et al., 2009). Furthermore, membranes may become disorganized, proteins may undergo loss of activity or be denatured, and oxidative damage often occurs. As a result, inhibition of photosynthesis, a metabolic dysfunction, and damage to cellular structures contribute to stunted growth (Krasensky and Jonak, 2012). Premature senescence in photosynthetic source tissues lowers the number and limits the growth of harvestable sink organs (Albacete et al., 2014). Plants respond to abiotic stresses by means of biochemical and physiological adaptations, which involve changes in gene expression, including genes that encode the enzymes responsible for the biosynthesis of osmolytes, detoxification enzymes, transport proteins, late-embryogenesis-abundant proteins or genes that regulate phytohormone levels (Pareek et al., 2010). In addition, plants have evolved specific strategies to maintain photosynthetic efficiency. This could involve several processes at different levels, including CO2 diffusion, light harvesting, redox homeostasis, carbon assimilation and carbohydrate metabolism (Abreu et al., 2013), and changes in the expression of many genes (Saibo et al., 2009; Abreu et al., 2013).
Altogether, this differential gene expression is regulated by specific TFs (Yamaguchi-Shinozaki and Shinozaki, 2006; Saibo et al., 2009) and, in line with this, altering the expression of these TFs can increase crop tolerance (Varshney et al., 2011; Datta et al., 2012; Hichri et al., 2016). The observed effects on photosynthesis efficiency or photosynthetic machinery are normally positive in these tolerant plants, as indicated by Saibo et al. (2009). However, these results must be interpreted with caution because abiotic stress tolerance has been evaluated mostly under laboratory conditions and very little is known about plant responses to adverse field conditions. Very few cases have been reported in which physiological research has led to improve crop cultivars to produce increased yields (Sinclair et al., 2004; Datta et al., 2012). The work of Gupta et al. (2012) in millet has reported greater photosynthetic efficiency, and also better growth, yield and crude grain protein content by overexpressing the DOF1 gene. Improved nitrogen assimilation and growth under low-nitrogen conditions in plants of Arabidopsis and rice that overexpress maize DOF1 have been previously described (Yanagisawa et al., 2004; Kurai et al., 2011). DOF TFs are of particular interest if we take into account the relation between their higher expression and increased photosynthetic rate, but also improved agronomic yield.
DNA binding with One Finger (DOF) proteins are a group of plant-specific TFs that contain a highly conserved domain that binds specifically to a 5′-T/AAAAG-3′ sequence motif in the promoters of direct target genes (Yanagisawa and Schmidt, 1999). DOF proteins have been reported to be involved in the control of very different growth and development processes in plants (Yanagisawa, 2002, 2004). The characterization of five DOF genes from tomato, homologous to Arabidopsis Cycling DOF Factors (CDFs), has been recently published (Corrales et al., 2014). SlCDF1–5 genes were differentially induced in response to osmotic, salt, heat, and low-temperature stress. The Arabidopsis plants that overexpressed tomato orthologous genes SlCDF1 or SlCDF3 showed increased drought and salt tolerance. The metabolic analyses of these plants presented higher levels of sucrose and different amino acids, which indicates increased nitrogen assimilation, as reported previously for other DOF TFs (Yanagisawa et al., 2004). A multifaceted role for the Arabidopsis AtCDF3 gene in stress responses and development in Arabidopsis has also been established (Corrales et al., 2017). In this work, the authors revealed that AtCDF3 regulates a set of genes involved in cellular osmoprotection and oxidative stress, including key stress tolerance TFs like CBFs, DREB2A and ZAT12. In addition, Fornara et al. (2015) reported that Arabidopsis CDFs promote plant growth through the action of PIF4 and IAA29 transcription factors. Together all these data highlight the impact of CDFs on plant development and on the interaction with environmental cues.
Based on these results it appeared tempting to us to investigate the possibility to use the CDF genes as a biotechnological tool in tomato breeding. With this aim in mind, we generated tomato plants overexpressing the CDF3 genes from tomato and Arabidopsis to address the question whether by this it is possible to increase salt tolerance and improve yield. Here we demonstrate that the overexpression of AtCDF3 or the orthologous tomato gene SlCDF3 in tomato plants increases photosynthetic rate and biomass production, resulting in higher yields. While non-transformed plants suffered a considerable performance decrease under salinity stress conditions, photosynthesis and yield remained significantly higher in the 35S::CDF3 tomato plants. In addition to the improved performance under salinity (Corrales et al., 2014, 2017), our data suggest that the ectopic expression of CDF3 improves the photosynthetic capacity which, in turn, leads to higher sucrose availability and changes in the plant's primary metabolism facilitating enhanced N assimilation, being the reason for the increased biomass production.
Materials and Methods
Plant Materials and Growth Conditions
Non-transformed (NT) Solanum lycopersicum cv. Moneymaker was used as the WT. Tomato seeds were germinated and cultured as described in Renau-Morata et al. (2014). Plants were grown in hydroponic culture with Hoagland no. 2 solution (Hoagland and Arnon, 1950) at 16/8 h light/dark photoperiod, 200 μmol m−2 s−1 light irradiance and 25/18°C light/dark.
For the greenhouse assays, imbibed seeds were germinated on a moistened mixture of peat moss and sand in growth chambers at 25/18°C and a 16/8 h photoperiod. Seedlings were transferred to 15 L pots that contained coconut coir fiber and were irrigated with Hoagland no. 2 nutrient solution.
Constructs and Tomato Transformation
The ORFs of the AtCDF3 (Arabidopsis thaliana Col-0) and SlCDF3 (Solanum lycopersicum cv. Moneymaker) genes were amplified by PCR using cDNA as a template. They were cloned into a binary vector under the control of the CaMV35S promoter, followed at the 3′end by the nopaline synthase gene (NOS) terminator (Figure S1). The resultant plasmids were used to transform tomato plants cv. Moneymaker following the method described by Ellul et al. (2003). The seeds from the transformed plants were harvested and plated on selective medium and the kanamycin-resistant seedlings were transplanted to soil. The next generation seeds were subjected to a second round of selection to determine the homozygous lines.
Salt Stress Assays
Thirty-day-old plants (three to four leaves) grown in hydroponic culture were used for the stress assays. Saline stress experiments were performed by adding sodium chloride at 75 mM to the nutrient solution (EC 7–8 dS m−1), as described in the standardized procedures by Renau-Morata et al. (2014). Tolerance was determined by measuring biomass and photosynthetic capacity after 15 days of treatment.
A second experiment was conducted under the above-described conditions. The behavior of the 30-day-old plants was compared for 15 days under the salinity and control conditions in growth chambers. Twelve plants were used for each genotype and treatment. For each treatment and genotype, plant material was pooled in three independent extracts (leaves of 4 plants per extract) of 45-day-old-plants, was immediately frozen (N2) after sampling and stored at −80°C until use. Sampling was performed at zeitgeber time 2 (ZT2). The transcriptomic analysis, and the metabolomic, hormones and mineral element determinations, were performed with the same plant material.
Biomass Quantification
The fresh weights of shoots and roots, leaf area and number of leaves were measured after 15 days of stress treatment (salt and low temperature conditions). Determinations were made on 10 different plants for each genotype and treatment. The dry weights of shoots and roots were measured after drying at 60°C for 48 h.
Measurement of Photosynthetic Activity
The instantaneous values of net CO2 assimilation rate (AN, μmol m−2 s−1), stomatal conductance (gs, mol m−2 s−1), substomatal CO2 concentration (Ci, μmol mol−1) and transpiration rate (E, mmol m−2 s−1) were determined with an LI-6400 infrared gas analyser (LICOR Biosciences, Lincoln, USA). One measurement per plant was taken on the third or fourth leaf from the apex. Eight to ten different plants were used. The conditions in the measuring chamber were controlled at a flow rate of 500 mol s−1, a saturating PAR of 1,200 μmol m−2 s−1, 400 ppm CO2 and 60–70% relative humidity. Maximum photochemical efficiency (Fv/Fm) on the dark-adapted leaves was measured by a portable pulse amplitude modulation fluorometer (MINI PAM, Walz, Effeltrich, Germany).
After inducing steady-state photosynthesis, AN-Ci curves were measured as described in Nebauer et al. (2011). Corrections for leakage of CO2 into and out of the leaf chamber of the LI-6400 were applied to all the gas exchange data (Flexas et al., 2007). From the combined gas exchange and chlorophyll a fluorescence measurements, mesophyll conductance to CO2 (gm) was estimated according to Harley et al. (1992). Six independent curves were produced per treatment and genotype.
RNA Isolation and RT-qPCR Analyses
Total RNA was extracted using RNeasy Plant Mini Kit (Qiagen). The gene expression levels in the transgenic tomato plants were determined by RT-qPCR following the procedures described in Corrales et al. (2014). The primer pairs used for amplification are described in Table S1. The UBIQUITIN3 gene from S. lycopersicum was used as the reference gene (Hoffman et al., 1991). The relative expression levels of the target genes were calculated by the 2−ΔΔCT method (Livak and Schmittgen, 2001). Presence of Dof binding sites in the promoters (1,500 pb) of the selected genes was established with the PlantPan2 software (Chow et al., 2016).
Transcriptomic Analysis
Differences in gene expression were studied by RNA-Seq in the 45-day-old NT and 35S::AtCDF3 (L 2.3) plants grown under the control and salinity conditions (75 mM NaCl) using an Illumina HiSeq2000 platform (BGI, China). Total RNA was extracted from leaves and purified with the Qiagen RNeasy extraction kit according to the manufacturer's instructions, and was treated with RNase-free DNase (Ambion). RNA integrity and quantity were confirmed with the Bioanalyzer 2100 (Agilent). cDNA synthesis, adaptor ligation and sequencing were performed by BGI. The raw Illumina sequencing data are deposited in GEO (http://www.ncbi.nlm.nih.gov/geo/) at NCBI.
The S. lycopersicum genome and gene information were downloaded from Solgenomics (ftp://ftp.solgenomics.net/genomes/Solanum_lycopersicum/annotation/ITAG2.4_release/ITAG2.4 Release genomic annotation). After removing the reads that contained sequencing adapters and reads of low quality (reads that contained Ns >5), the remaining reads were aligned to the S. lycopersicum genome using SOAP (Li et al., 2009). The reads that failed to be mapped and the paired-end reads were processed as described by Wang et al. (2010).
The gene expression level by RNA-Seq was normalized by the number of reads per kilobase of exon region per million mapped reads (RPKM) (Mortazavi et al., 2008). The cut-off value for the determining gene transcriptional activity was determined based on a 95% confidence interval for all the RPKM values of each gene. The transcript fold change was calculated as the log2 of the ratio between two samples. Here, genes with a fold change >2.0 were considered to be differentially expressed.
The common genes that are represented in one or more data sets were identified using the web-based VENNY tool (http://bioinfogp.cnb.csic.es/tools/venny/) Finally, Gene Ontology analyses were performed using the agriGO (http://bioinfo.cau.edu.cn/agriGO/; Du et al., 2010) and REVIGO (http://revigo.irb.hr/; Supek et al., 2011) software.
Metabolomic Analyses
The total soluble sugars and starch content in leaves and roots were determined colorimetrically by the anthrone method, as described in Nebauer et al. (2011). The NT and 35S::AtCDF3 (L 2.3 and 10.1) plants were grown under the control and salinity conditions for 15 days. Three independent leaf extracts were used for the analyses in any treatment and genotype. Targeted metabolomics analyses were also performed. Extraction, manipulation and mass spectrometric analyses of samples followed an adapted protocol described in Corrales et al. (2014).
Determination of Mineral Elements Content
Sodium, calcium, magnesium, potassium, and phosphate contents were determined in the leaves, stems and roots of 45-day-old NT and 35S::AtCDF3 (L 2.3) plants grown under control and salinity (75 mM NaCl) conditions for 15 days with an ICP-AES (Thermo Scientific, USA). For each treatment and genotype, plant material was dried for 48 h at 60°C and finely ground in a Pulverisette mill (Fristch, Germany). Acid digestion of samples was done by the method explained in Nebauer et al. (2011).
Hormone Determinations
Hormones (jasmonic acid, indole-3-acetic acid, isopentenyl adenine, abscisic acid and GA12, GA15, GA24, GA9, GA51, GA4, GA34, GA53, GA44, GA19, GA20, GA29, GA1, and GA8 gibberellins) were analyzed in leaves of NT and 35S::AtCDF3 (L 2.3) plants grown under control and salinity conditions for 15 days by liquid chromatography-electrospray ionization-tandem mass spectrometry (LC-ESI-MS/MS) using a Q-Exactive spectrometer (Orbitrap detector; ThermoFisher Scientific) by the Plant Hormone Quantification Service, IBMCP, Valencia, Spain.
Determination of Tomato Production and Fruit Quality
The agronomic performance of the transgenic lines was assessed in a third experiment by measuring total yield (g plant−1), number of fruits and fruit weight (g fruit−1). All fruits were harvested at maturity in each plant until the 6th truss. Plants were obtained as described above (Renau-Morata et al., 2014) and seedlings were transferred to 15 L pots that contained coconut fiber, and irrigated with half-strength Hoagland no. 2 nutrient solution (Hoagland and Arnon, 1950). Plants were cultured in the greenhouse from December to mid-August. Salinity was imposed by adding 75 mM of NaCl to the irrigation solution. Plants were irrigated twice a week with excess solution to ensure optimal watering and to minimize salt accumulation in the substrate during the experiment. No significant incidence of Blossom-End Rot (BER) was observed among the studied lines.
Four representative fruits were collected from each plant in the mature-red stage (only from the first three trusses to minimize intraplant variability). Soluble solids content was measured by refractometry. Taste components were determined by capillary electrophoresis, as described by Cebolla-Cornejo et al. (2012). Sugars fructose, glucose and sucrose, and organic acids malic, citric, glutamic acid and γ-amino butyric acid (GABA), were quantified. The derived sucrose equivalents, sucrose equivalents/citric acid and sucrose equivalents/malic acid ratios were calculated. Carotenoids β-carotene and lycopene were determined by reversed phase HPLC, as described in Cortés-Olmos et al. (2014).
Statistical Analyses
Data were analyzed by a two-way ANOVA (genotype and treatment) or one-way ANOVA with the Statgraphics statistical software (Statgraphics Centurion XVI, Statpoint Tech, Inc., USA). The treatment mean values were compared (P < 0.05) by Fisher's least significant difference (LSD) procedure. A regression analysis (P < 0.05) was used to evaluate the relationships between parameters.
Results
Generation of Tomato Plants that Overexpress AtCDF3 and SlCDF3 Genes
We have previously identified a group of tomato DOF TFs (SlCDFs) that exhibit specific expression patterns in response to diverse environmental stresses and whose functions are related to abiotic stress tolerance and flowering time (Corrales et al., 2014). Among them, SlCDF3 was particularly interesting for its high induction under precise stress conditions. Similar observations have been recently reported for the putative orthologous Arabidopsis AtCDF3 gene (Corrales et al., 2017), assigning an important role to this TF in regulating abiotic stress responses in plants.
To further study the function of CDF3 and to determine its impact on plant growth and production, a phenotypic characterization of CDF3 gain-of-function plants was accomplished by analyzing their performance under control and abiotic stress conditions (e.g., salinity). Tomato plants (cv. Moneymaker) overexpressing AtCDF3 or SlCDF3 genes were obtained after transformation with a binary vector harboring the corresponding coding sequences under control of the constitutive CaMV 35S promoter.
From each transformation, individual lines were selected (L 2, 4, 5, 10, and 16 of 35S::AtCDF3 plants; and L 11, 15, 23, and 25 of 35S::SlCDF3 plants). These lines were analyzed by RT-qPCR to test the expression levels of the inserted CDF3 genes (Figure 1A). The transcript levels of both genes were significantly higher in the tested transgenic lines compared to NT (non-transformed) plants.
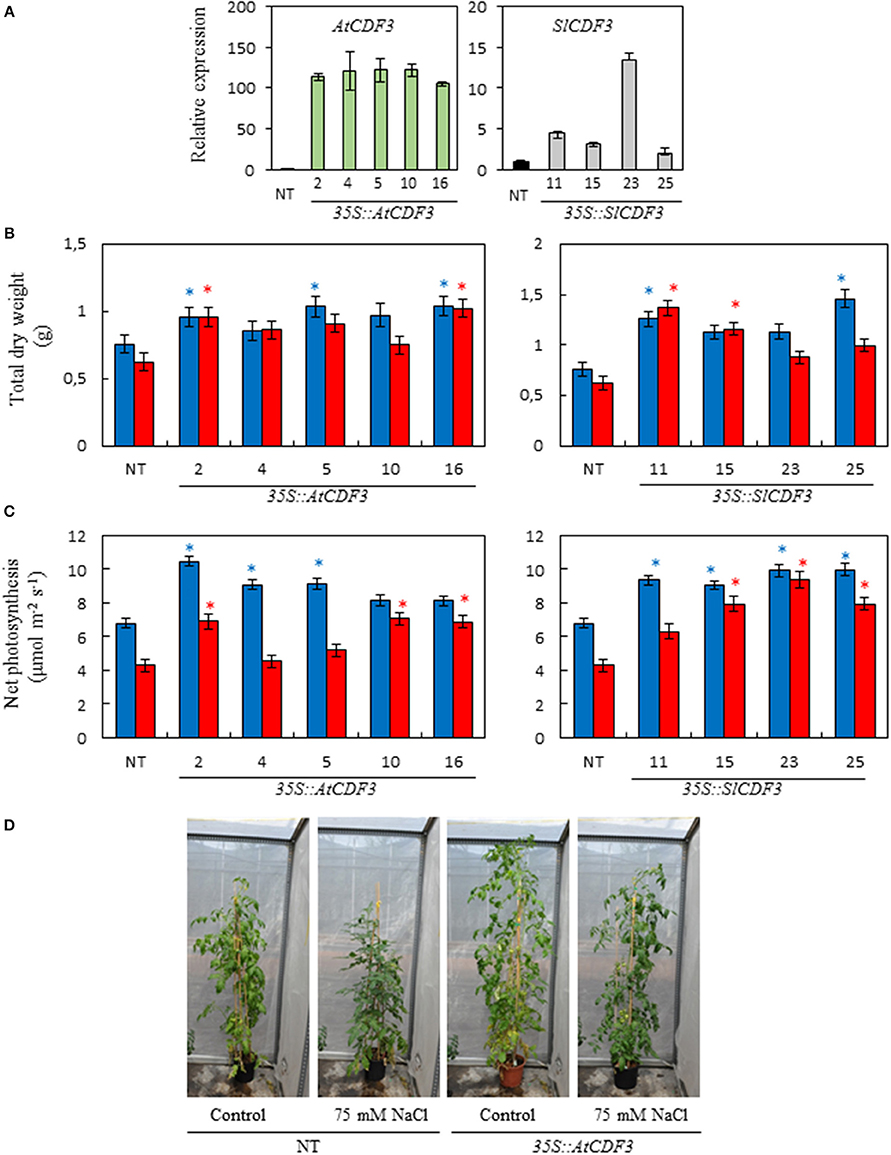
Figure 1. Phenotypic characterization of the 35S::AtCDF3 and 35S::SlCDF3 tomato lines. (A) Expression levels of the corresponding gene AtCDF3 or SlICDF3 analyzed by RT-qPCR. (B) Biomass and (C) photosynthetic rate of the 35S::AtCDF3, 35S::SlCDF3 and NT tomato lines grown under the control (blue bars) and 75 mM NaCl (red bars) conditions. Thirty-day-old plants grown in hydroponic culture were subjected to moderate salinity (75 mM NaCl). Photosynthesis and growth determinations were performed after 15 days of treatment. (D) Representative examples of the NT and 35S::AtCDF3 (line 2.3) plants grown under the control or salt stress conditions in the greenhouse. Asterisks indicate significant differences (P < 0.05) of the transgenic plants with the non-transformed plants (NT) under the same growth condition. NT, non-transformed.
Overexpression of AtCDF3 and SlCDF3 Improves Biomass Production under Control and Salinity Stress Conditions
A phenotypic characterization of the CDF3 overexpressing plants was conducted by analyzing their responses under control and salinity conditions. Plant biomass was determined in the 35S::AtCDF3 (L 2, 4, 5, 10, and 16) and 35S::SlCDF3 (L 11, 15, 23, and 25) and NT plants that were either grown under control conditions or treated with 75 mM NaCl. 35S::CDF3 and NT tomato plants grown under control conditions in hydroponic cultures did not show apparent developmental differences. However, CDF3 overexpressing plants consistently exhibited a larger size than the NT plants (Figure 1B). Accordingly, the majority of the 35S::CDF3 plants displayed significantly higher dry weight values than the NT plants under these conditions. This higher total dry weight was due to the increased size of all the plant organs (r > 0.90**, data not shown).
35S::AtCDF3 and 35S::SlCDF3 plants also displayed increased biomass under the salinity conditions (Figures 1B,D). Although salinity reduced plant growth in some lines under the assayed conditions, the plants overexpressing CDF3 genes consistently exhibited higher total biomass than the NT plants.
Enhanced Photosynthetic Capacity of AtCDF3 and SlCDF3 Overexpressing Tomato Plants
To investigate the underlying mechanisms involved in the observed growth responses of 35S::AtCDF3 and 35S::SlCDF3 plants, we examined different physiological parameters such as net photosynthesis and related gas exchange variables, stomatal conductance and substomatal CO2 concentration using a LI-6400 infrared gas analyser. NT and 35S::CDF3 plants were grown in hydroponic culture for 30 days, exposed to salinity (75 mM NaCl) for 15 days, and the photosynthetic parameters were measured.
As shown in Figure 1C, under the control conditions most of the 35S::AtCDF3 and 35S::SlCDF3 plants displayed higher photosynthetic rates than the NT plants. Although salt stress conditions reduced the photosynthetic rate values, most of the AtCDF3 and SlCDF3 overexpressing lines also exhibited higher CO2 fixation rate values than the NT plants in these conditions (Figure 1C). Both the Arabidopsis and tomato CDF3 genes consistently promoted a similar effect (P < 0.05).
The high correlation between the photosynthetic rate and total plant biomass observed (Total dry weight = 0.075*AN+ 0.40; r = 0.85; P = 0.00), indicates that increased photosynthesis sustains the higher growth rate observed in the plants overexpressing CDF3 genes.
We further investigated diverse photosynthetic parameters (Table 1) from combined gas exchange and chlorophyll fluorescence analyses in the 35S::AtCDF3 plants (L 2.3). The higher photosynthetic rate in the plants that overexpress AtCDF3, compared to the NT plants under the control conditions (10.8 vs. 8.5 μmol m−2 s−1) could be related to higher stomatal conductance (Table 1), suggesting lower limitations to CO2 diffusion to the mesophyll in transgenic plants. The 35S::AtCDF3 plants exhibited a higher effective quantum yield of PSII (PhiPS2) indicating a higher proportion of absorbed light used in photochemistry. Both the higher performance of the electron transport chain in the thylakoid membranes, and the higher maximum carboxylation rate of Rubisco (Vc max) in the CO2 fixation reactions (Table 1), support an improved overall photosynthesis.
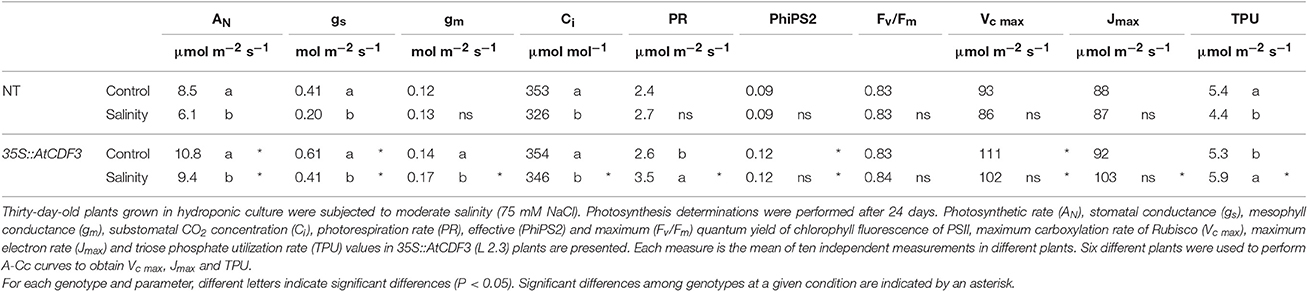
Table 1. Effect of salinity on photosynthetic parameters in Moneymaker tomato (NT) and 35S::AtCDF3 plants.
Salinity caused the photosynthetic rate to drop in both the NT and 35S::AtCDF3 (L 2.3) plants (Table 1). However, this reduction was less marked in the transgenic plants (11 vs. 28% in the NT plants). Although stomatal closure was also provoked by salinity, stomatal conductance in the transgenic plants was also higher than in the NT plants, which allowed better CO2 diffusion into leaves. The greater mesophyll conductance observed in the 35S::AtCDF3 plants also improved the CO2 supply from substomatal air spaces to the stroma in the chloroplast. Under salinity, the transgenic plants also exhibited the higher effective quantum yield of PSII and the maximum carboxylation rates of Rubisco reported under the control conditions. Interestingly, the plants that overexpress the AtCDF3 gene showed higher triose phosphate utilization rates under these stress conditions (Table 1), which suggests that the carbon flux to sucrose and starch synthesis is maintained in these plants.
Taken together, our data indicate that the greater biomass accumulation observed in the plants that overexpress the AtCDF3 and SlCDF3 genes is sustained by a higher photosynthetic rate, and that the greater photosynthetic capacity of these plants could be related to both improved diffusional and biochemical factors.
Transcriptome Analysis of Transgenic Tomato Plants Overexpressing CDF3 Genes
Transcriptome analysis of 45-day-old NT and 35S::AtCDF3 (L 2.3) plants grown under control and salinity conditions was performed by RNA-seq. Leaf transcriptomes of 35S::AtCDF3 and NT plants grown under control conditions revealed 385 differentially expressed genes (DEGs: >2-fold change, P < 0.05, see Figures 2A,B). Of them, 252 were up-regulated and 133 down-regulated (Table 2; Tables S2, S3). Furthermore, GO (gene ontology) analyses revealed that CDF3-regulated genes are highly enriched in terms related to the primary metabolism and regulation processes (Figures 2C,D). Among the up-regulated genes in the transgenic plants and directly linked to the phenotypes observed are the genes involved in carbon and nitrogen metabolism, like phosphoenolpyruvate carboxykinase (PEPCK, Solyc04g076880.2.1) and pyruvate kinase (PK, Solyc03g007810.2.1) participating in glycolysis and gluconeogenesis; and glutamate synthase (GS, Solyc01g080280.2) and glutamate decarboxylase (GAD, Solyc11g011920.1) involved in nitrogen assimilation. Likewise, a set of genes that improve photosynthetic efficiency and redox balance, including DNAJ chaperones (DnaJ8, Solyc11g011920.1, DnaJ11, Solyc07g065970.1.1, and DnaJ20, Solyc09g092260.2.1), TRX thioredoxin (Cxx1, Solyc02g079960.2.1) and peroxidases (Solyc03g119060.2.1, Solyc02g092580.2.1 and Solyc04g071890.2.1), are also up-regulated in the 35S::AtCDF3 plants (Table 2).
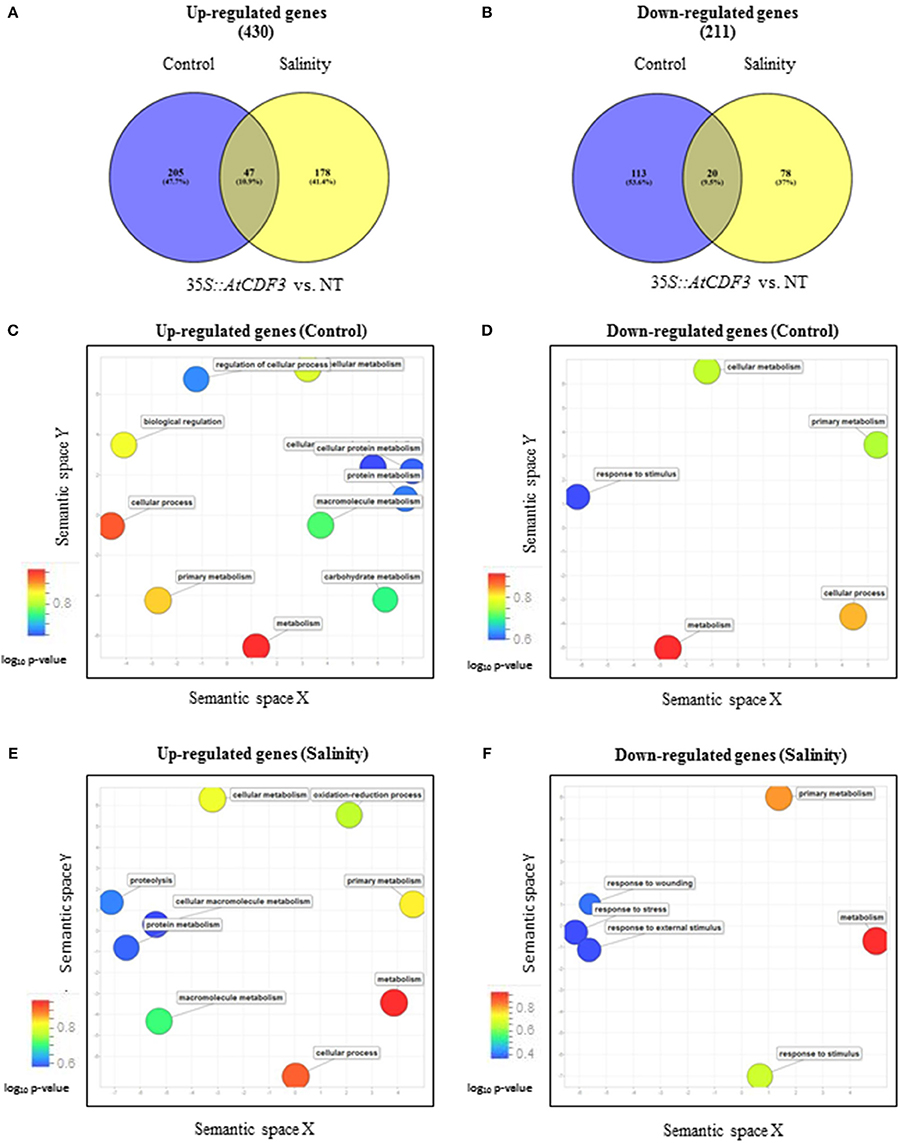
Figure 2. Classification and gene ontology analyses of the genes differentially expressed in the 35S::AtCDF3 plants compared to the NT plants under control and salinity conditions. Thirty-day-old NT and 35S::AtCDF3 plants grown in hydroponic culture were subjected to moderate salinity (75 mM NaCI). Leaf transcriptomic analysis was performed after 15 days. (A,B) Venn diagrams showing the overlap of the up-regulated and down-regulated genes expressed in the 35S::CDF3 transgenic plants vs. the NT plants in response to salinity. (C–F) Scatter plot of the genes significantly (P < 0.05) up- or down-regulated in the 35S::AtCDF3 plants compared with the non-transformed NT plants under control and salinity conditions. The GO analysis was performed using the AgriGO and Revigo tools. Bubble color indicates the P-value for the false discovery rates derived from the AgriGO analysis as well as biological processes. NT, non-transformed.
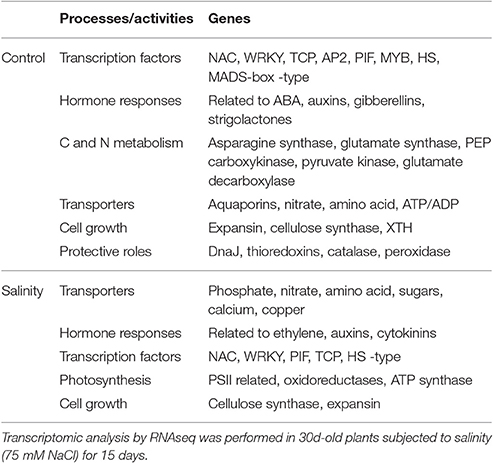
Table 2. Summary of representative up-regulated genes in the transgenic 35S::AtCDF3 (L 2.3) tomato plants under control and salinity conditions.
Other important genes related to plant growth are induced in the 35S::AtCDF3 plants. Among them, trehalose-6-phosphate (T6P) synthase (Solyc07g006500.2.1), which is involved in the synthesis of the sucrose sensor molecule T6P that modulates growth and stress responses (Ruan, 2014), or genes that participate in cell wall expansion, like expansins (Solyc08g077900.2.1 and Solyc08g077330.2.1), xyloglucan endo transglycosylase/hydrolase (XTH, Solyc05g046290.2.1) and cellulose synthase (CESA, Solyc03g005450.2.1). Among the up-regulated transcription factors, IAA29 (Solyc08g021820.2.1) and PIF1 (Solyc06g008030.2.1) have been reported to participate in the control of plant growth (Leivar and Monte, 2014; Huang et al., 2015; Shimizu et al., 2016), and other TFs like NAC29, MYB44 (Solyc04g078420.1.1) and WRKY33 (Solyc06g066370.2.1) involved in ABA and stress responses. Altogether, these data suggest that CDF3 might function in the regulation of plant growth through processes related to photosynthesis, C/N metabolism and cell growth under variable environmental conditions.
A similar analysis of plants grown under the salinity conditions, also revealed differentially expressed genes between the NT and 35S::AtCDF3 plants (225 up-regulated and 98 down-regulated genes, see Figures 2A,B). Primary metabolism, transport and regulation processes were over-represented terms in the GO analyses of DEGs (Figures 2E,F). Notably, several transporter activities, TFs related to growth and stress responses like WRKY40 (Solyc08g067340.2.1), PIF1, NAC22 (Solyc07g066330.2.1) and TCP12 (Solyc11g020670.1.1), and genes related to photosynthesis performance, including thylakoid proteins or redox components, were also up-regulated in the 35S::AtCDF3 plants (Table 2; Tables S4, S5).
Quantitative RT-PCR was performed to confirm some of the identified differentially expressed genes in the tomato 35S::AtCDF3 plants. This analysis included the genes that codify for the TFs involved in the regulation of growth and stress responses, like PIF1 and MYB44, genes related to C/N metabolism, like glutamate synthase GS2 and glutamate decarboxylase GAD, and genes related to the protection of the photosynthetic process, like CxxS1 thiorredoxin and the DnaJ8 chaperone. Figure 3 shows the expression levels of the analyzed genes in the 35S::AtCDF3 transgenic plants, where they exhibit higher values (from 2- to 4-fold) than in the NT plants. These data confirmed the results of the RNA-seq analyses and points to CDF3 as a potential upstream activator in photosynthesis, growth and stress pathways. Interestingly, several DOF binding sites were found in the promoters of the analyzed genes in Figure 3 using the PlantPan2 software (data not shown), suggesting that they might be direct target genes of the CDF3 transcription factor.
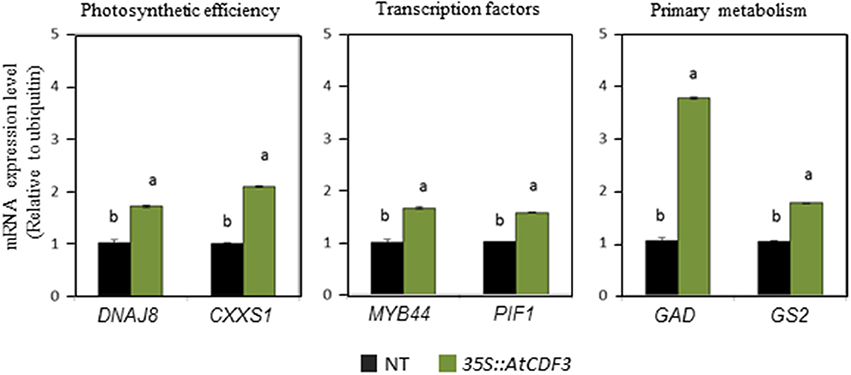
Figure 3. AtCDF3 regulates the expression of genes involved in photosynthesis, primary metabolism and growth-and stress- related transcription factors. Transcription analysis by RT-qPCR of genes DNAJ8, CxxSl, MYB44, PIFl, GAD, and GS2 in the leaves of the 35S::AtCDF3 (green bars) and NT (black bars) tomato plants grown under the control conditions for 30 days. UBIQUITIN3 gene expression was used as the reference gene. Data are expressed as mean ± SE of three independent extractions. Three technical replicates were performed for each extraction. For each gene, different letters indicate significant differences (P < 0.05).
Overexpression of AtCDF3 in Vegetative Tissues Impacts Sugar and Amino Acid Metabolism in Tomato
We performed a targeted metabolomic profiling by gas chromatography-mass spectrometry (GC-MS) to study the relative levels of the different polar compounds, including proteinogenic amino acids, and other amino acids and distinct sugars, extracted from the 45-day-old NT and two 35S::AtCDF3 tomato lines (L 2.3 and 10.1) either grown under the control conditions or treated with 75 mM of NaCl for 15 days (Figure 4; Figure S2).
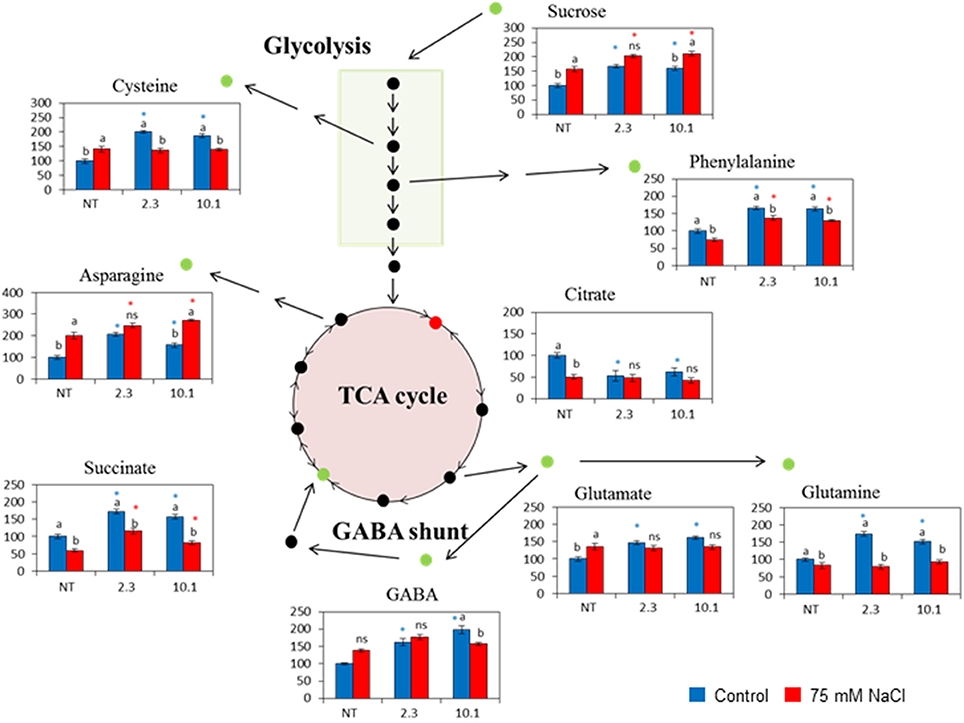
Figure 4. Schematic representation of the altered metabolism in the 35S::AtCDF3 plants (lines 2.3 and 10.1) under the control (blue bars) and salinity (red bars) conditions. Thirty-day-old NT and 35S::AICDF3 plants (L 2.3 and 10.1) were grown in hydroponic culture under control and salinity (75 mM NaCl) conditions. Metabolomic determinations in leaves were performed after 15 days. The relative quantities (% of NT) of the selected metabolites analyzed by gas chromatography-selected ion monitoring-mass spectrometry are displayed. Different letters indicate the significant differences (P < 0.05) within each genotype for the stress effect. Differences between genotypes per treatment are indicated by an asterisk. NT, non-transformed.
Figure 4 shows metabolite levels under control conditions, whereby the overexpression of AtCDF3 induced the accumulation of sugars like sucrose, organic acids like succinate and amino acids like glutamate, glutamine, asparagine, phenylalanine, cysteine and GABA in both lines, while the amounts of tryptophan, citrate and gluconate decreased in comparison to the NT plants. No changes in leaf starch were observed (data not shown) and glucose and fructose amounts remained unchanged compared to NT plants (Figure S2). Overall, the increased photosynthetic rate in the 35S::AtCDF3 plants favors the production of sucrose available for transport and growth, and increased the content of amino acids glutamate and glutamine, related to N assimilation.
Under the salt stress conditions, a reduction in fructose, citrate, tryptophan and aspartate was observed, but only in the NT plants (Figure 4; Figure S2). Succinate, phenylalanine and glutamine levels lowered in all genotypes. Sucrose and glutamate accumulated under salinity in the NT plants at similar levels to those observed in the overexpressing plants. Interestingly, under these lower C availability conditions, N assimilation was channeled in the form of asparagine in the 35S::AtCDF3 plants.
The Overexpression of CDF3 Genes in Tomato Increases Tomato Yield under the Control and Saline Conditions and Improves Fruit Quality Traits
Since the AtCDF3 and SlCDF3 overexpression in tomato promoted higher photosynthesis and biomass production, we investigated the impact of these TFs on plant yield. To this end, greenhouse assays were performed to characterize the agronomic performance of the 35S::AtCDF3 (L 2.3 and 10.1), 35S::SlCDF3 (L 11.2 and 23.1) and NT plants. Total yield, number of fruits and fruit weight were measured in the selected lines, both under control and saline conditions (75 mM of NaCl).
The results in Figure 5A show that under the non-stress control conditions both the 35S::AtCDF3 and 35::SlCDF3 plants present improved fruit yield (up to 550% of yield in NT plants). Notably, the production was related to the photosynthetic rate (Total yield = 36.1*AN-68.4; r = 0.74; P = 0.02) and the improved yield in the tomato transgenic plants resulted mainly from a larger number of fruits than the NT plants. In addition, the fruit size was larger (up to 137%) in the plants overexpressing CDF3 than in the NT plants (Figures 5B,C). However, increased fruit size influenced the agronomic performance of transgenic plants, but to a lesser extent than number of fruits.
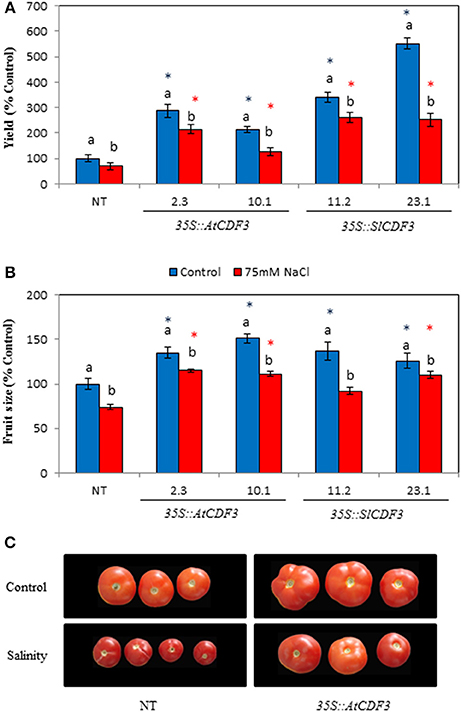
Figure 5. Agronomic performance of tomato plants overexpressing AtCDF3 or SlCDF3 genes under control and salinity conditions. (A) Yield and (B) fruit size of tomato plants under control (blue bars) and salinity (75 mM NaCl; red bars) conditions. Plants were cultured in the greenhouse from December to mid-August. All fruits were harvested at maturity in each plant until the 6th truss. The results are provided as a percentage of yield or fruit size in relation to the NT plants grown under the control conditions. (C) Representative examples of the tomatoes from the NT or 35S::AtCDF3 (line 2.3) plants grown under control or salt stress. Asterisks indicate significant differences (P < 0.05) with the NT plants grown under the same condition. Letters indicate significant differences for a given genotype between the control and salinity conditions. NT, non-transformed.
Under salt stress conditions, tomato yield decreased in almost all the lines, but was always higher in the 35S::CDF3 plants, which achieved up to 250–280% of NT plants. These results were confirmed in a second experiment performed with two additional 35S::AtCDF3 lines (L 2.3 and 10.1) in a different year (data not shown). As expected, tomato fruit size was reduced when the transgenic plants were exposed to salt stress. Under these conditions, the fruit size of the overexpressing lines was, nevertheless, greater than that of the NT plants.
We further analyzed metabolites and variables related to organoleptic quality in the fruits of the 35S::AtCDF3 (L 2.3 and 10.1) and 35S::SlCDF3 (L 11.2 and 23.1) plants under control and salinity conditions. Remarkably, overexpression of CDF3 genes had a strong impact on organic acid and sugar accumulation, and in the derived variables related to flavor perception. Figure 6 and Table S6 display important changes in the transgenic lines compared to the NT plants, as a higher malic to citric acid ratio or higher GABA and glucose content (e.g., 35% higher glucose content in the 35S::SlCDF3 line 23; Table S6). In general, overexpression of the CDF3 genes led to higher sucrose equivalents to the citric and glutamic acid ratios, parameters that strongly correlate with sensorial perception.
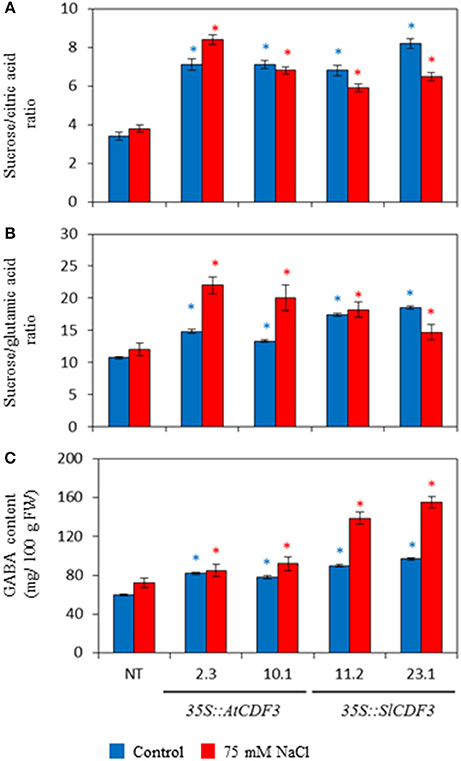
Figure 6. Effect of the overexpression of the Arabidopsis and tomato CDF3 genes on the compounds and variables related to organoleptic quality in tomato fruits. 35S::AtCDF3 (lines 2.3 and 10.1) or 35S:SlCDF3 (lines 11.2 and 23.1) and NT tomato plants were cultured under control (blue bars) and salinity (75 mM NaCl, red bars) in the greenhouse from December to mid-August. Four representative fruits, until the 3rd truss, were collected from each plant in the mature-red stage. The sucrose equivalents/citric acid (A) and sucrose equivalents/glutamic acid (B) ratios and GABA content (C) are displayed. Asterisks indicate significant differences (P < 0.05) of the transgenic plants with the non-transformed plants (NT) under the same growth condition.
Under salinity stress, dry matter, soluble solids and glucose content of fruits of the NT and CDF3 overexpressing plants moderately increased. Likewise, organic acids and fructose also increased (Figure 6; Table S6), but the reported lower citric acid and increased malic acid contents were maintained in the fruits of the transgenic plants. These plants also presented higher fructose and glucose levels, as well as higher sucrose and sucrose equivalents to the citric and glutamic acid ratios, as observed in the control media.
Overall, these data indicate that overexpression of the CDF3 genes in tomato improves yield due to increased fruit number and weight, and also improves quality under control conditions. As expected, salinity reduces yield in both the NT and most 35S::CDF3 plants, although fruit production always remained higher in the transgenic lines.
Effects of AtCDF3 Overexpression on Ion Accumulation
Since salinity reduces nutrient uptake and affects nutrient partitioning within plants (Grattan and Grieve, 1999), we wanted to investigate whether the improved yield of the 35S::CDF3 plants under salt stress was due to reduced sodium accumulation and lower toxicity, and test the mineral composition in the transgenic plants. Growth inhibition is often correlated with a high internal Na+ and K+ deficiency in vegetative tissues. Total content of sodium, calcium, magnesium, potassium and phosphate was determined in leaves, stems and roots of the 45-day-old 35S::AtCDF3 (L 2.3) and NT plants grown under control and salinity conditions for 15 days (Table S7). Notably, no significant differences were observed between the transgenic and NT plants under the control conditions. Under salinity both genotypes accumulated similar levels of sodium in the different analyzed organs, and although some reduction in the amount of potassium was triggered, no differences were observed between the NT and transgenic plants (Figure 7; Table S7). It should be noted that salinity provoked smaller leaf amounts of essential elements magnesium, calcium, phosphor and sulfur in the NT plants (Table S7). In contrast, the content of these elements were maintained in the 35S::AtCDF3 plants, which thus avoided mineral deficiency triggered by salt stress.
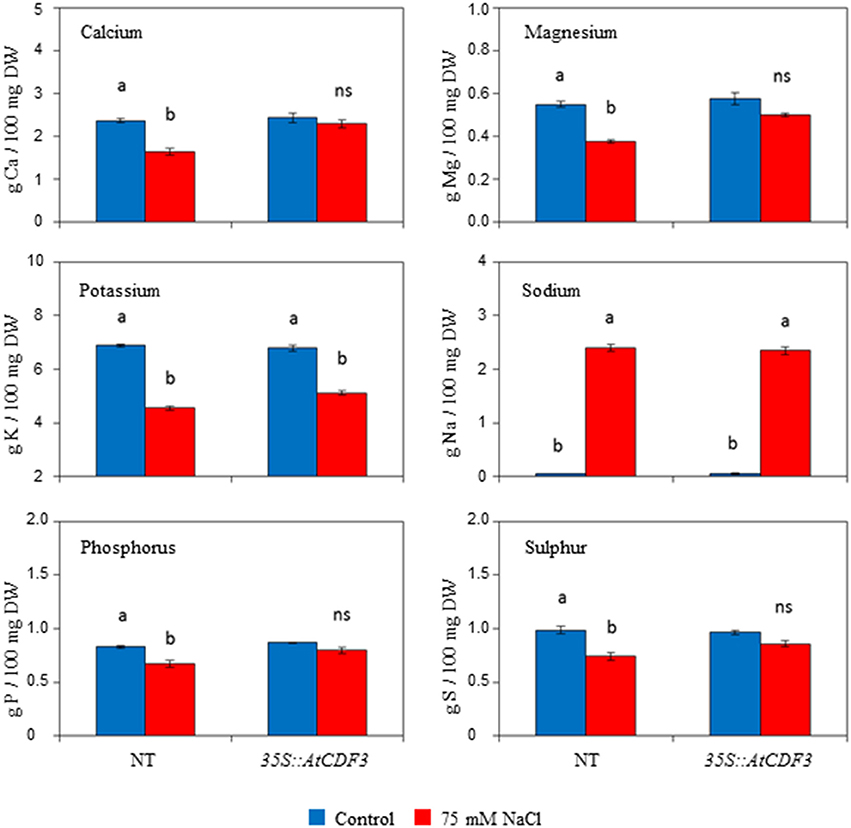
Figure 7. Effect of AtCDF3 overexpression on leaf mineral elements content in tomato. Thirty-day-old plants grown in hydroponic culture were subjected to moderate salinity (75 mM NaCl). Mineral elements determinations were performed after 15 days of treatment. The calcium, magnesium, sodium, potassium, phosphor, and sulfur levels were shown under the control (blue bars) and salinity (red bars) conditions in the NT and 35S::AtCDF3 plants (line 2.3). Different letters indicate the significant differences (P < 0.05) within each genotype for the stress effect. NT, non-transformed.
Tomato Plants Overexpressing AtCDF3 Show Increased Content of Active Gibberellins
The role of hormones in the regulation of plant growth and development and in responses to stresses is well-known (Albacete et al., 2014). To investigate whether the improved growth and yield observed in the 35S::CDF3 plants could be related to changes in hormone contents, gibberellins (GAs), jasmonic acid (JA), isopentenyladenine (IP), indole acetic acid (IAA), and abscisic acid (ABA) contents were measured in the leaves of the 45-day-old NT and 35S::AtCDF3 (L 2.3) plants grown under the control and salinity conditions (Figure 8; Figure S3). No differences were observed between the genotypes in the IP, IAA and ABA levels under the control conditions (Figure 8). Interestingly, active GA1 gibberellin content was higher (P < 0.05) in the transgenic plants. Higher active gibberellin levels have been related to growth promotion through increased source and sink activities (Iqbal et al., 2011). Remarkably, transcriptomic analyses of tomato AtCDF3 overexpressing lines show an increased expression of the 3-hydroxy-3-methylglutaryl coenzyme A reductase (HMGR), catalyzing a rate-limiting step of isoprenoid synthesis through the mevalonate pathway (Pulido et al., 2012) and leading to the synthesis of gibberellins when the chloroplastic MEP pathway is limiting (Kasahara et al., 2002).
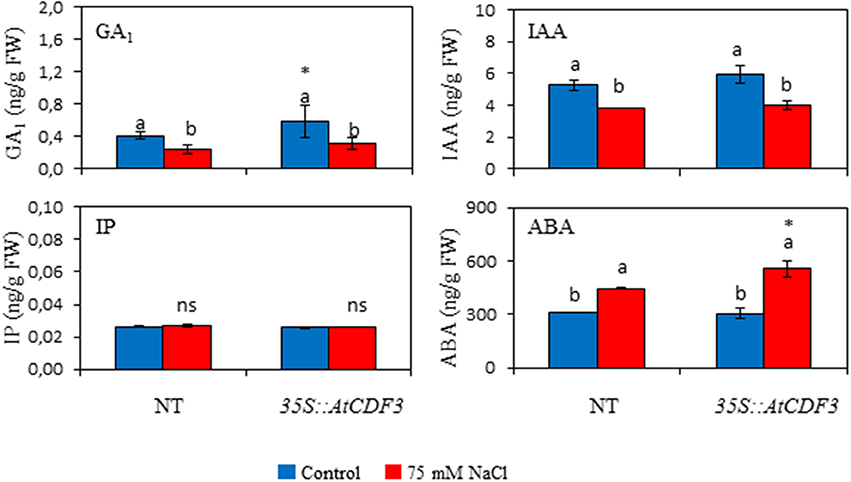
Figure 8. Effect of AtCDF3 overexpression on leaf hormone content in tomato. Thirty-day-old NT and 35S::AtCDF3 (L 2.3) plants grown in hydroponic culture were subjected to control and moderate salinity (75 mM NaCl) conditions. Hormone determinations were performed after 15 days of treatment. The GA1, IAA, IP, and ABA levels in leaves were shown under the control (blue bars) and salinity (red bars) conditions. Different letters indicate the significant differences (P < 0.05) within each genotype for the stress effect. Differences between genotypes per treatment are indicated by an asterisk. NT, non-transformed.
Under the salinity conditions, IAA and GA1 content lowered in both the NT and 35S::AtCDF3 plants. This decrease could be related to the reported drop in growth and production. ABA levels rose in both genotypes, but this increase was more prominent in the transgenic plants. Accordingly, ABA has been shown to alleviate salt stress in several species (Sah et al., 2016).
Discussion
CDF transcription factors have been reported to participate in the regulation of processes related to plant growth and development, with special relevance on responses to abiotic stress conditions (Corrales et al., 2014, 2017; Fornara et al., 2015). In this work we have overexpressed Arabidopsis and tomato CDF3 genes in tomato plants and assessed their precise impact on growth, yield and tomato fruit quality, under control and salt stress conditions. We reported that the plants overexpressing CDF3 genes display increased growth and yield. Our results indicate that the higher biomass production is supported by higher photosynthetic rates and sucrose levels, but also by changes in carbon and nitrogen metabolism. Interestingly, despite photosynthesis, growth and yield were reduced by salinity; they were consistently higher in the transgenic plants than in the NT plants.
Enhanced Biomass Production in Plants Overexpressing CDF3 Transcription Factors
In this work we have generated transgenic tomato plants overexpressing CDF3 genes from tomato and Arabidopsis. Further, their phenotypic characterization shows that they present higher biomass production compared to the non-transformed plants (Figure 1). Since plant growth relies largely on photosynthesis and the production of carbohydrates and nitrogen compounds to provide energy and biomass, we performed combined gas exchange-chlorophyll fluorescence determinations and metabolomic analyses to gain insight into the physiological processes underlying the phenotypes observed.
Photosynthetic efficiency can be improved through the optimization of any of the limiting processes involved, including CO2 diffusion, light harvesting processes, CO2 fixation and assimilation, and carbon metabolism (Long et al., 2015). Several reports have indicated that stomatal conductance is critical for growth and yield, and greater conductance is related to higher fixation rate under non-limiting water conditions (Roche, 2015). Notably, CDF3 overexpressing plants show higher stomatal conductance, thus improving CO2 diffusion to the mesophyll (Table 1). In addition, the transgenic plants display higher effective quantum yield of PSII (PhiPS2), related to the electron transport through PSII, and a higher maximum carboxylation rate of Rubisco. Taken together, these data indicate an improved photosynthetic capacity in the CDF3 overexpressing plants.
Likewise, our results related to metabolite analyses in leaves show that the overexpression of the CDF3 genes triggers manifold changes in carbon-skeleton production and nitrogen assimilation pathways (Figure 4). These plants contain higher sucrose levels, in agreement with the higher reported CO2 fixation rate. Starch levels remained almost unchanged, which suggests that C allocation favors the TCA anaplerotic flux in these plants. The marked increase in several amino acids, specially glutamine, glutamate, asparagine and GABA, indicates enhanced nitrogen assimilation. Both glutamine and glutamate are good indicators of nitrogen utilization (Stitt and Krapp, 1999; Foyer et al., 2006). The drop in citric acid content is in support of an increase of C skeletons, which diverge to amino acid synthesis through intermediate 2-oxoglutarate (2-OG), as expected from the increase in both the GS2 and AS expression in transcriptomic data and the observed glutamate/glutamine and asparagine levels. As previously reported for the heterologous expression of ZmDOF1 in Arabidopsis or rice, which modulates the expression of genes involved in C skeleton production for amino acid biosynthesis (Yanagisawa et al., 2004; Kurai et al., 2011), our findings also suggest the cooperative modification of carbon and nitrogen metabolism by the overexpression of CDF3 Dof-type TFs. In line with this, our study also revealed higher succinate and γ-amino butyric acid (GABA) content in the transgenic plants, and GABA is involved in nitrogen storage, among other roles (Shelp et al., 1999). The pathway that converts glutamate into succinate via GABA (GABA shunt) compensates the high flux rate from 2-oxoglutarate to amino acid metabolism (Araujo et al., 2012). Similarly, increased PEPCK expression is also observed, as previously associated with tissues in which the metabolism of nitrogenous compounds is enhanced (Chen et al., 2004).
The above-described metabolic changes have also been observed in Arabidopsis plants that overexpress SlCDF3 (Corrales et al., 2014), which suggests that CDF3s may play a key conserved role in controlling the genes involved in carbon fixation and nitrogen assimilation.
AtCDF3 Regulates Genes Associated to Plant Growth, Mostly Involved in Photosynthetic Activity and C/N Metabolism
Leaf transcriptomic analyses of AtCDF3 overexpressing plants revealed increased expression levels of important target genes involved in photosynthesis and primary metabolism (Table 2; Tables S2–S5).
Noticeably, DnaJ chaperones DnaJ8, DnaJ11, and DnaJ20, and thioredoxins (CxxS1 and TRX3.1), are up-regulated in the plants that overexpress the AtCDF3 gene. The higher DnaJ expression levels in the 35S::AtCDF3 tomato plants, which have also been reported in 35S::AtCDF3 Arabidopsis plants (Corrales et al., 2017) can be linked to the improved photosynthetic performance observed, since these proteins have been reported to be involved in the optimization of CO2 fixation, the stabilization of PSII complexes and the balancing of electron transfer reactions (Chen et al., 2010). Thioredoxins (TRXs) are members of sensing systems that monitor and maintain optimal redox conditions (König et al., 2012). Many essential plastid processes, such as carbon metabolism, nitrogen metabolism, lipid biosynthesis or protein folding, are regulated by TRXs (Serrato et al., 2013). Interestingly, a Dof TF (PsDOF7) has been reported to control TRX expression in response to sugars (Barajas-López et al., 2012), as observed in our work. Altogether, these data agree with the higher maximum carboxylation rate of Rubisco and the higher electron transport rate through the PSII observed under the control conditions in the plants that overexpress CDF3.
Key genes involved in C/N metabolism are also upregulated in the CDF3 overexpressing plants. Among them, pyruvate kinase (PK) and PEP carboxykinase (PEPCK), participate in the glycolysis and gluconeogenesis pathways and probably confer greater metabolic flexibility to these plants. Interestingly, the 35S::AtCDF3 plants show higher expression of the glutamate synthase (GS) and glutamate decarboxylase (GAD) genes (Figure 3) in support of the altered metabolic profiles observed in these plants (Figure 4).
Additionally, other important genes related to plant growth are upregulated in the CDF3 overexpressing plants (Table 2). Of special interest are different transcription factors, like PIFs, which are involved in growth promotion (Lucas and Prat, 2014) acting as central hubs of a regulatory programme that integrates internal (e.g., sucrose and gibberellins) and environmental signals (Leivar and Monte, 2014). It has to be noted that T6P and sucrose mediate the induction of PIFs (Liu et al., 2011) and both T6P synthase and PIF1 genes are up-regulated in the 35S::CDF3 plants. Several PIF targets are implicated in cell elongation and the control of photosynthetic capacity (Lucas and Prat, 2014). Accordingly, Fornara et al. (2015) have related greater growth in Arabidopsis, mediated by PIF4, to the activity of CDFs.
Of the different hormones measured in the CDF3 overexpressing plants we could detect higher levels of active GAs, which are known to induce the expression of cellulose synthase and expansin, involved in cell elongation and growth (Kordel and Kutschera, 1999; Lee and Kende, 2002). Interestingly, our transcriptomic analyses revealed higher expression levels of such genes: cellulose synthase, xyloglucan endo transglycosylase/hydrolase (XTH) and expansins (Table S2). Higher bioactive GA levels are reported to increase tomato fruit set and early fruit development through cell expansion (Mariotti et al., 2011; Ariizumi et al., 2013). Interestingly, an increased number of fruits with larger size were found in the plants that overexpress CDF3 genes (Figure 5). Larger fruit size and sink demand have been linked to the activation of invertases induced by gibberellins (Roitsch and González, 2004; Albacete et al., 2014).
Altogether, our results highlight the potential role of CDF3 in the regulation of the genes related to C/N metabolism, photosynthetic efficiency and growth.
Overexpression of SlCDF3 or AtCDF3 Genes Results in Changes in Fruit Composition Associated With Fruit Quality Improvement
The altered sugar and organic acid profiles in the CDF3 overexpressing lines result in higher levels of sucrose equivalents, and in variables related to sweetness perception and overall acceptability of fruits (Baldwin et al., 1998; Bucheli et al., 1999). Increased GABA content was also observed in fruits, along with a considerable reduction in citrate content, which seems compatible with altered GABA shunt regulation, as pointed out in leaves. A similar profile has also been observed in GABA-rich tomato cultivars (Akihiro et al., 2008).
CDF3 overexpressing lines show increased levels of malate, most likely due to the GABA shunt. Malate plays a key role in gluconeogenesis via PEPCK activity, which increases in the ripening stage of tomato fruits (Yin et al., 2010; Osorio et al., 2013) and would likely explain the mild rise in hexose content observed in some transgenic lines. An altered malate concentration has also been described to influence cellular redox status, and to result in altered carotenoid biosynthesis (Centeno et al., 2011), as observed in the fruits analyzed in the present study. Taken together, our results indicate that CDF3 overexpression induces changes in the composition of sugars and organic acids in fruits associated to the improvement in fruit quality, and highlights the interest of CDF genes in tomato breeding given their effects on fruit production and quality.
Tomato Plants Overexpressing CDF3 Genes Maintain Increased Growth and Yield under Salinity Conditions
One of the major outputs of our work is the capacity of using CDF TFs in engineering plant metabolism for the improvement of tomato plants in their production and fruit quality under usual growing conditions. However, they are specially interesting when considering tomato growth and production under salinity stress. In Figure 5, although some reduction was observed compared to the control conditions, yield values under salinity (75 mM of NaCl) were higher in the transgenic plants than those of the NT plants. Previous work, based on the introgression of genes involved in the stress responses of tomato (Pandey et al., 2011), have shown their potential use to alleviate short-term applied drought and salinity stresses (usually measured as plant survival). Nevertheless, in very few cases an effect on crop yield stability has been proved (Albacete et al., 2014).
Increasing crop yield involves diverse physiological and biochemical processes, including photosynthesis, assimilate partitioning to reproductive structures and nitrogen uptake and metabolism (Sinclair et al., 2004). Under salinity, the improved responses of the CDF3 overexpressing lines could be supported by the maintenance of greater carbon and nitrogen assimilation compared to NT plants. Moreover, these lines also display enhanced photosynthetic rates under this stress condition (Figure 1; Table 1). The minimal impact of salinity on the photosynthetic rate in the 35S::CDF3 plants could be explained by the maintained carboxylation rate of Rubisco and the electron transport rate of PSII (Table 1). In addition, the CO2 supply to the chloroplast in these plants appears ensured through high stomatal and mesophyll conductances. Furthermore, the increased photorespiration rate and the rise in the expression of genes related to protective activities and electron transport chain components, as observed in the transgenic plants, prevent oxidative damage under stress, reduce biochemical limitations and optimize photosynthesis (Voss et al., 2013).
The amount of sucrose, asparagine, phenylalanine and GABA also remained higher in the CDF3 overexpressing plants (Figure 4). The change in the glutamine-glutamate/asparagine ratio was as expected (Lam et al., 1996) since energy-limited conditions favor nitrogen assimilation into asparagine. The increased amounts of the above-mentioned compounds have been related to the responses of plants to salt stress. Higher levels of asparagine and sucrose can improve osmotic adjustment under salinity. The importance of a functional GABA shunt for stress has also been observed in tomato under salinity (Zushi and Matsuzoe, 2006; Bao et al., 2015). A larger amount of phenylalanine can be related to phenolic compounds scavenge of free radicals and other oxidative species (Dicko et al., 2005).
Transcriptomic analyses of CDF3 overexpressing plants grown under salinity, also showed an increased expression of certain TFs (Table 2), previously associated to abiotic stress tolerance (Agarwal et al., 2016; Hong et al., 2016). The fact that these TFs are also up-regulated in these plants under the control conditions, suggests that CDF3 is an upstream regulator of these genes, in accordance with similar results obtained in Arabidopsis plants that overexpress the AtCDF3 gene (Corrales et al., 2017), and reinforces the role of the CDF3 genes in the control of responses to stresses.
Conclusion
The overexpression of CDF3 genes from either Arabidopsis or tomato increases growth rate and, ultimately, the yield of tomato plants. The increased growth observed could be associated to higher photosynthetic rates, which lead to higher sucrose levels, and to changes in the C/N metabolism, including nitrogen assimilation. In addition, CDF3 induced the up-regulation of genes involved in cell growth, photosynthesis efficiency, primary metabolism and stress responses. Our data revealed that CDFs are potential tools for improving tomato production under regular and salinity conditions.
Author Contributions
RM, JV, JM, and SN conceived and designed the experiments. BR, LC, JC, MS, JD, SP, AC, and SN performed the experiments. RM, JC, JF, JV, JM, and SN analyzed the data. All the authors contributed to the writing, discussion and approval of the final manuscript. BR, LC, and RM are to be considered equal joint first authors.
Funding
This work has been supported by grants from the Instituto Nacional de Investigación y Tecnología Agraria y Alimentaria (Projects 2009-0004-C01 and 2012-0008-C01) and the Ministerio de Economía, Industria y Competitividad (Projects BFU2013-49665-EXP and BIO2014-53181-R). JD was supported by an INIA predoctoral fellowship.
Conflict of Interest Statement
The authors declare that the research was conducted in the absence of any commercial or financial relationships that could be construed as a potential conflict of interest.
The reviewer CJDO and handling Editor declared their shared affiliation, and the handling Editor states that the process nevertheless met the standards of a fair and objective review.
Acknowledgments
The authors would like to thank Prof. Díez and Victor Lizondo for their assistance in the characterization assays, and Helen Warburton for revising the English language. We thank Dr. García-Martínez, Dr. Lopez-Díaz, and Carrera for the hormone quantification carried out at the Plant Hormone Quantification Service, IBMCP in Valencia, Spain. We thank Mar González for technical assistance and handling of transgenic tomato plants.
Supplementary Material
The Supplementary Material for this article can be found online at: http://journal.frontiersin.org/article/10.3389/fpls.2017.00660/full#supplementary-material
References
Abreu, I. A., Farinha, A. P., Negrao, S., Gonçalves, N., Fonseca, C., Rodrigues, M., et al. (2013). Coping with abiotic stress: proteome changes for crop improvement. J. Proteomics 93, 145–168. doi: 10.1016/j.jprot.2013.07.014
Agarwal, P., Dabi, M., Sapara, K. K., Joshi, P. S., and Agarwal, P. K. (2016). Ectopic expression of JcWRKY Transcription Factor Confers salinity tolerance via salicylic acid signaling. Front. Plant Sci. 7:1541. doi: 10.3389/fpls.2016.01541
Akihiro, T., Koike, S., Tani, R., Tominaga, T., Watanabe, S., Iijima, Y., et al. (2008). Biochemical mechanism on GABA accumulation during fruit development in tomato. Plant Cell Physiol. 49, 1378–1389. doi: 10.1093/pcp/pcn113
Albacete, A. A., Martínez-Andujar, C., and Perez-Alfocea, F. (2014). Hormonal and metabolic regulation of tomato fruit sink activity and yield under salinity. Biotech. Adv. 32, 12–30. doi: 10.1016/j.biotechadv.2013.10.005
Araujo, W. L., Tohge, T., Nunes-Nesi, A., Daloso, D. M., Nimick, M., Krahnert, I., et al. (2012). Phosphonate analogs of 2-oxoglutarate perturb metabolism and gene expression in illuminated Arabidopsis leaves. Front. Plant Sci. 3:114. doi: 10.3389/fpls.2012.00114
Ariizumi, T., Shinozaki, Y., and Ezura, H. (2013). Genes that influence yield in tomato. Breeding Sci. 63, 3–13. doi: 10.1270/jsbbs.63.3
Baldwin, E. A., Scott, J. W., Einstein, M. A., Malundo, T. M. M., Carr, B. T., Shewfelt, R. L., et al. (1998). Relationship between sensory and instrumental analysis for tomato flavor. J. Amer. Soc. Hortic. Sci. 123, 906–915.
Bao, H., Chen, X., Lv, S., Jiang, P., Feng, J., Fan, P., et al. (2015). Virus-induced gene silencing reveals control of reactive oxygen species accumulation and salt tolerance in tomato by γ-aminobutyric acid metabolic pathway. Plant Cell Environ. 38, 600–613. doi: 10.1111/pce.12419
Barajas-López, J., Tezycka, J., Travaglia, C. N., Serrato, A. J., Chueca, A., Thormahlen, I., et al. (2012). Expression of the chloroplast thioredoxins f and m is linked to short-term changes in the sugar and thiol status in leaves of Pisum sativum. J. Exp. Bot. 63, 4887–4900. doi: 10.1093/jxb/ers163
Bucheli, P., Voirol, E., de la Torre, R., Lopez, J., Rytz, A., Tanksley, S. D., et al. (1999). Definition of nonvolatile markers for flavor of tomato (Lycopersicon esculentum Mill.) as tools in selection and breeding. J. Agric. Food Chem. 47, 659–664. doi: 10.1021/jf980875l
Cebolla-Cornejo, J., Valcarcel, M., Herrero-Martinez, J., Rosello, S., and Nuez, F. (2012). High efficiency joint CZE determination of sugars and acids in vegetables and fruits. Electrophoresis 33, 2416–2423. doi: 10.1002/elps.201100640
Centeno, D. C., Osorio, S., Nunes-Nesi, A., Bertolo, A. L. F., Carneiro, R. T., Araujo, W. L., et al. (2011). Malate plays a crucial role in starch metabolism, ripening, and soluble solid content of tomato fruit and affects postharvest softening. Plant Cell 23, 162–184. doi: 10.1105/tpc.109.072231
Chen, K. M., Holmström, M., Raksajit, W., Suorsa, M., Piippo, M., and Aro, E. M. (2010). Small chloroplast-targeted DnaJ proteins are involved in optimization of photosynthetic reactions in Arabidopsis thaliana. BMC Plant Biol. 10:43. doi: 10.1186/1471-2229-10-43
Chen, Z. H., Walker, R. P., Tecsi, L. I., Lea, P. J., and Leegood, R. C. (2004). Phosphoenolpyruvate carboxykinase in cucumber plants is increased both by ammonium and by acidification, and is present in the phloem. Planta 219, 48–58. doi: 10.1007/s00425-004-1220-y
Chow, C.-N., Zheng, H.-Q., Wu, N.-Y., Chien, C.-H., Huang, H.-D., Lee, T.-Y., et al. (2016). PlantPAN 2.0: an update of plant promoter analysis navigator for reconstructing transcriptional regulatory networks in plants. Nucl. Acids Res. 4, D1154–D1160. doi: 10.1093/nar/gkv1035
Corrales, A.-R., Carrillo, L., Lasierra, P., Nebauer, S. G., Domínguez-Figueroa, J., Renau-Morata, B., et al. (2017). Multifaceted role of cycling Dof Factor 3 (CDF3) in the regulation of flowering time and abiotic stress responses in Arabidopsis. Plant Cell Environ. 40, 748–764. doi: 10.1111/pce.12894
Corrales, A. R., Nebauer, S. G., Carrillo, L., Fernandez-Nohales, P., Marques, J., Renau-Morata, B., et al. (2014). Characterization of tomato Cycling Dof Factors reveals conserved and new functions in the control of flowering time and abiotic stress responses. J. Exp. Bot. 65, 995–1012. doi: 10.1093/jxb/ert451
Cortés-Olmos, C., Leiva-Brondo, M., Roselló, J., Raigon, M. D., and Cebolla-Cornejo, J. (2014). The role of traditional varieties of tomato as sources of functional compounds. J. Sci. Food Agric. 94, 2888–2904. doi: 10.1002/jsfa.6629
Datta, K., Baisakh, N., Ganguly, M., Krishnan, S., Shinozaki, K. Y., and Datta, S. K. (2012). Overexpression of Arabidopsis and Rice stress genes' inducible transcription factor confers drought and salinity tolerance to rice. Plant Biotech. J. 10, 579–586. doi: 10.1111/j.1467-7652.2012.00688.x
Dicko, M. H., Gruppen, H., Barro, C., Traore, A. S., van Berkel, W. J. H., and Voragen, A. G. J. (2005). Impact of phenolic compounds and related enzymes in sorghum varieties for resistance and susceptibility to biotic and abiotic stresses. J. Chem. Ecol. 31, 2671–2688. doi: 10.1007/s10886-005-7619-5
Du, Z., Zhou, X., Ling, Y., Zhang, Z., and Su, Z. (2010). agriGO: a GO analysis toolkit for the agricultural community. Nucl. Acids Res. 38, 64–70. doi: 10.1093/nar/gkq310
Ellul, P., Garcia-Sogo, B., Pineda, P., Ríos, G., Roig, L. A., and Moreno, V. (2003). The ploidy level of transgenic plants in Agrobacterium-mediated transformation of tomato cotyledons (Lycopersicon esculentum L. Mill.) is genotype and procedure dependent. Theor. Appl. Gen. 106, 231–238. doi: 10.1007/s00122-002-0928-y
Flexas, J., and Medrano, H. (2002). Drought-inhibition of Photosynthesis in C3 Plants: stomatal and non-stomatal limitations revisited. Ann. Bot. 89, 183–189. doi: 10.1093/aob/mcf027
Flexas, J., Ribas-Carbó, M., Díaz-Espejo, A., Galmés, J., and Medrano, H. (2007). Rapid variations of mesophyll conductance in response to changes in CO2 concentration around leaves. Plant Cell Environ. 30, 1284–1298. doi: 10.1111/j.1365-3040.2007.01700.x
Fornara, F., Montaigu, A., Sánchez-Villarreal, A., Takahashi, Y., van Termaat, E. V. L., Huettel, B., et al. (2015). The GI-CDF module of Arabidopsis affects freezing tolerance and growth as well as flowering. Plant J. 81, 695–706. doi: 10.1111/tpj.12759
Foyer, C. H., Noctor, G., and Verrier, P. (2006). “Photosynthetic carbon-nitrogen interactions: modelling inter-pathway control and signaling,” in Annual Plant Reviews Volume 22: Control of Primary Metabolism in Plants, eds W. C. Plaxton and M. T. McManus (Oxford: Blackwell Publishing Ltd.), 325–347.
Godfray, H. C. J., Crute, I. R., Haddad, L., Lawrence, D., Muir, J. F., Nisbett, N., et al. (2010). The future of the global food system. Philos. Trans. R. Soc. Biol. Sci. 365, 2769–2777. doi: 10.1098/rstb.2010.0180
Grattan, S. R., and Grieve, C. M. (1999). Salinity mineral nutrient relations in horticultural crops. Sci. Hortic. 78, 1–4.
Gupta, N., Gupta, A. K., and Kumar, A. (2012). Spatial distribution pattern analysis of Dof1 transcription factor in different tissues of three Eleusine coracana genotypes differing in their grain colour, yield and photosynthetic efficiency. Mol. Biol. Rep. 39, 2089–2095. doi: 10.1007/s11033-011-0956-2
Harley, P. C., Loreto, F., Di Marco, G., and Sharkey, T. D. (1992). Theoretical considerations when estimating the mesophyll conductance to CO2 flux by the analysis of the response of photosynthesis to CO2. Plant Physiol. 98, 1429–1436. doi: 10.1104/pp.98.4.1429
Hichri, I., Muhovski, Y., Clippe, A., Zizkova, E., Dobrev, P. I., Motyka, V., et al. (2016). SlDREB2, atomato dehydration-responsive element-binding 2 transcription factor, mediates salt stress tolerance in tomato and Arabidopsis. Plant Cell Environ. 39, 62–79. doi: 10.1111/pce.12591
Hoagland, D. R., and Arnon, D. I. (1950). The water-culture method for growing plants without soil. Calif. Agric. Exp. Stn. 347, 1–32.
Hoffman, N. E., Ko, K., Milkowski, D., and Pichersky, E. (1991). Isolation and characterization of tomato cDNA and genomic clones encoding the ubiquitin gene ubi3. Plant Mol. Biol. 17, 1189–1201. doi: 10.1007/BF00028735
Hong, Y., Zhang, H., Huang, L., Li, D., and Song, F. (2016). Overexpression of a stress-responsive NAC transcription factor gene ONAC022 improves drought and salt tolerance in rice. Front. Plant Sci. 7:4. doi: 10.3389/fpls.2016.00004
Huang, D., Wang, S., Zhang, B., Shang-Guan, K., Shi, Y., Zhang, D., et al. (2015). A gibberellin-mediated DELLA-NAC signaling cascade regulates cellulose synthesis in rice. Plant Cell 27, 1681–1696. doi: 10.1105/tpc.15.00015
Iqbal, N., Nazar, R., Khan, M. I. R., Masood, A., and Khan, N. A. (2011). Role of gibberellins in regulation of source–sink relations under optimal and limiting environmental conditions. Curr. Sci. 100, 998–1007.
Kasahara, H., Hanada, A., Kuzuyama, T., Takagi, M., Kamiya, Y., and Yamaguchi, S. (2002). Contribution of the mevalonate and methylerythritol phosphate pathways to the biosynthesis of gibberellins in Arabidopsis. J. Biol. Chem. 277, 45188–45194. doi: 10.1074/jbc.M208659200
König, J., Muthuramalingam, M., and Dietz, K. J. (2012). Mechanisms and dynamics in the thiol/disulphide redox regulatory network: transmitters, sensors and targets. Curr. Opin. Plant Biol. 15, 261–268. doi: 10.1016/j.pbi.2011.12.002
Kordel, B., and Kutschera, U. (1999). Effects of gibberellin on cellulose biosynthesis and membrane-associated sucrose synthase activity in pea internodes. J. Plant Physiol. 156, 570–573. doi: 10.1016/S0176-1617(00)80176-5
Krasensky, J., and Jonak, C. (2012). Drought, salt, and temperature stress-induced metabolic rearrangements and regulatory networks. J. Exp. Bot. 63, 1593–1608. doi: 10.1093/jxb/err460
Kurai, T., Wakayama, M., Abiko, T., Yanagisawa, S., Aoki, N., and Ohsugi, R. (2011). Introduction of the ZmDof1 gene into rice enhances carbon and nitrogen assimilation under low-nitrogen conditions. Plant Biotech. J. 9, 826–837. doi: 10.1111/j.1467-7652.2011.00592.x
Lam, H. M., Coschigano, K. T., Oliveira, I. C., Melo-Oliveira, R., and Coruzzi, G. M. (1996). The molecular-genetics of nitrogen assimilation into amino acids in higher plants. Annu. Rev. Plant Physiol. Plant Mol. Biol. 47, 569–593. doi: 10.1146/annurev.arplant.47.1.569
Lee, Y., and Kende, H. (2002). Expression of alpha-expansin and expansin-like genes in deepwater rice. Plant Physiol. 130, 1396–1405. doi: 10.1104/pp.008888
Leivar, P., and Monte, E. (2014). PIFs: systems integrators in plant development. Plant Cell 26, 56–78. doi: 10.1105/tpc.113.120857
Li, R., Yu, C., Li, Y., Lam, T., Yiu, S. M., Kristiansen, K., et al. (2009). SOAP2: an improved ultrafast tool for short read alignment. Bioinformatics 25, 1966–1967. doi: 10.1093/bioinformatics/btp336
Liu, Z. J., Zhang, Y. Q., Liu, R. Z., Hao, H. L., Wang, Z., and Bi, Y. R. (2011). Phytochrome interacting factors (PIFs) are essential regulators for sucrose-induced hypocotyl elongation in Arabidopsis. J. Plant Physiol. 168, 1771–1779. doi: 10.1016/j.jplph.2011.04.009
Livak, K. J., and Schmittgen, T. D. (2001). Analysis of relative gene expression data using real-time quantitative PCR and the 2−ΔΔCT method. Methods 25, 402–408. doi: 10.1006/meth.2001.1262
Long, S. P., Marshall-Colon, A., and Zhu, X. G. (2015). Meeting the global food demand of the future by engineering crop photosynthesis and yield potential. Cell 161, 55–66. doi: 10.1016/j.cell.2015.03.019
Long, S. P., Zhu, X. G., Naidu, S. L., and Ort, D. R. (2006). Can improvement in photosynthesis increase crop yields? Plant Cell Environ. 29, 315–330. doi: 10.1111/j.1365-3040.2005.01493.x
Lucas, M., and Prat, S. (2014). PIFs get BRright: PHYTOCHROME INTERACTING FACTORs as integrators of light and hormonal signals. New Phytol. 4, 1126–1141. doi: 10.1111/nph.12725
Mariotti, L., Picciarelli, P., Lombardi, L., and Ceccarelli, N. (2011). Fruit-set and early fruit growth in tomato are associated with increases in indoleacetic acid, cytokinin, and bioactive gibberellin contents. J. Plant Growth Regul. 30, 405–415. doi: 10.1007/s00344-011-9204-1
Mortazavi, A., Williams, B. A., McCue, K., Schaeffer, L., and Wold, B. (2008). Mapping and quantifying mammalian transcriptomes by RNA-Seq. Nature Methods 5, 621–628. doi: 10.1038/nmeth.1226
Nebauer, S. G., Renau-Morata, B., Guardiola, J. L., and Molina, R. V. (2011). Photosynthesis down-regulation precedes carbohydrate accumulation under sink limitation in Citrus. Tree Physiol. 31, 169–177. doi: 10.1093/treephys/tpq103
Osorio, S., Vallarino, J. G., Szecowka, M., Ufaz, S., Tzin, V., Angelovici, R., et al. (2013). Alteration of the interconversion of pyruvate and malate in the plastid or cytosol of ripening tomato fruit invokes diverse consequences on sugar but similar effects on cellular organic acid, metabolism, and transitory starch accumulation. Plant Physiol. 161, 628–643. doi: 10.1104/pp.112.211094
Pandey, S. K., Nookaraju, A., Upadhyaya, C. P., Gururani, M. A., Venkatesh, J. J., Kim, D. H., et al. (2011). An update on biotechnological approaches for improving abiotic stress tolerance in tomato. Crop Sci. 51, 2303–2324. doi: 10.2135/cropsci2010.10.0579
Pareek, A., Sopory, S. K., Bohnert, H. J., and Govindjee. (eds.). (2010). Abiotic stress Adaptation in Plants. Dordrecht: Springer. doi: 10.1007/978-90-481-3112-9
Paul, M. J., and Foyer, C. H. (2001). Sink regulation of photosynthesis. J. Exp. Bot. 52, 1383–1400. doi: 10.1093/jexbot/52.360.1383
Pulido, P., Perello, C., and Rodriguez-Concepcion, M. (2012). New insights into plant isoprenoid metabolism. Mol. Plant 5, 964–967. doi: 10.1093/mp/sss088
Renau-Morata, B., Sánchez-Perales, M., Medina, J., Molina, R. M., Corrales, R., Carrillo, L., et al. (2014). Salinity assay in tomato. Bioprotocols 4:1215e. doi: 10.21769/bioprotoc.1215
Roche, D. (2015). Stomatal conductance is essential for higher yield potential of C3 crops. Crit. Rev. Plant Sci. 34, 429–453. doi: 10.1080/07352689.2015.1023677
Roitsch, T., and González, M. C. (2004). Function and regulation of plant invertases: sweet sensations. Trends Plant Sci. 9, 606–613. doi: 10.1016/j.tplants.2004.10.009
Ruan, Y. L. (2014). Sucrose metabolism: Gateway to diverse carbon use and sugar signaling. Annu. Rev. Plant Biol. 65, 33–67. doi: 10.1146/annurev-arplant-050213-040251
Sah, S. K., Reddy, K. R., and Li, J. (2016). Abscisic acid and abiotic stress tolerance in crop plants. Front. Plant Sci. 7:571. doi: 10.3389/fpls.2016.00571
Saibo, N. J. M., Lourenco, T., and Oliveira, M. M. (2009). Transcription factors and regulation of photosynthetic and related metabolism under environmental stresses. Ann. Bot. 103, 609–623. doi: 10.1093/aob/mcn227
Serrato, A., Fernández-Trijueque, J., Barajas-López, J., Chueca, A., and Sahrawy, M. (2013). Plastid thioredoxins: a “one-for-all” redox-signaling system in plants. Front. Plant Sci. 4:463. doi: 10.3389/fpls.2013.00463
Shelp, B. J., Bown, A. W., and McLean, M. D. (1999). Metabolism and functions of gamma-aminobutyric acid. Trends Plant Sci. 4, 446–452. doi: 10.1016/S1360-1385(99)01486-7
Shimizu, H., Torii, K., Araki, T., and Endo, M. (2016). Importance of epidermal clocks for regulation of hypocotyl elongation through PIF4 and IAA29. Plant Signal. Behav. 11:e1143999. doi: 10.1080/15592324.2016.1143999
Sinclair, T. R., Purcell, L. C., and Sneller, C. H. (2004). Crop transformation and the challenge to increase yield potential. Trends Plant Sci. 9, 70–75. doi: 10.1016/j.tplants.2003.12.008
Stitt, M., and Krapp, A. (1999). The interaction between elevated carbon dioxide and nitrogen nutrition: the physiological and molecular background. Plant Cell Environ. 22, 583–621. doi: 10.1046/j.1365-3040.1999.00386.x
Supek, F., Bošnjak, M., Škunca, N., and Šmuc, T. (2011). REVIGO summarizes and visualizes long lists of gene ontology terms. PLoS ONE 6:e21800. doi: 10.1371/journal.pone.0021800
Tezara, W., Mitchell, V. J., Driscoll, S. D., and Lawlor, D. W. (1999). Water stress inhibits plant photosynthesis by decreasing coupling factor and ATP. Nature 401, 914–917. doi: 10.1038/44842
Varshney, R. K., Bansal, K. C., Aggarwal, P. K., Datta, S. K., and Craufurd, P. Q. (2011). Agricultural biotechnology for crop improvement in a variable climate: hope or hype? Trends Plant Sci. 16, 363–371. doi: 10.1016/j.tplants.2011.03.004
Voss, I., Sunil, B., Scheibe, R., and Raghavendra, A. S. (2013). Emerging concept for the role of photorespiration as an important part of abiotic stress response. Plant Biol. 15, 713–722. doi: 10.1111/j.1438-8677.2012.00710.x
Wang, B., Guo, G., Wang, C., Lin, X., Wang, X., Zhao, M., et al. (2010). Survey of the transcriptome of Aspergillus oryzae via massively parallel mRNA sequencing. Nucl. Acids Res. 38, 5075–5087. doi: 10.1093/nar/gkq256
Yamaguchi-Shinozaki, K., and Shinozaki, K. (2006). Transcriptional regulatory networks in cellular responses and tolerance to dehydration and cold stresses. Annu. Rev. Plant Biol. 57, 781–803. doi: 10.1146/annurev.arplant.57.032905.105444
Yanagisawa, S. (2002). The Dof family of plant transcription factors. Trends Plant Sci. 7, 555–560. doi: 10.1016/S1360-1385(02)02362-2
Yanagisawa, S. (2004). Dof domain proteins: plant-specific transcription factors associated with diverse phenomena unique to plants. Plant Cell Physiol. 45, 386–391. doi: 10.1093/pcp/pch055
Yanagisawa, S., Akiyama, A., Kisaka, H., Uchimiya, H., and Miwa, T. (2004). Metabolic engineering with Dof1 transcription factor in plants: Improved nitrogen assimilation and growth under low-nitrogen conditions. Proc. Natl. Acad. Sci. U.S.A. 101, 7833–7838. doi: 10.1073/pnas.0402267101
Yanagisawa, S., and Schmidt, R. J. (1999). Diversity and similarity among recognition sequences of Dof transcription factors. Plant J. 17, 209–214. doi: 10.1046/j.1365-313X.1999.00363.x
Yin, Y. G., Tominaga, T., Iijima, Y., Aoki, K., Shibata, D., Ashihara, H., et al. (2010). Metabolic alterations in organic acids and gamma-aminobutyric acid in developing tomato (Solanum lycopersicum L.) fruits. Plant Cell Physiol. 51, 1300–1314. doi: 10.1093/pcp/pcq090
Keywords: CDF, tomato, photosynthesis, abiotic stress, crop yield, C/N metabolism, transcriptome
Citation: Renau-Morata B, Molina RV, Carrillo L, Cebolla-Cornejo J, Sánchez-Perales M, Pollmann S, Domínguez-Figueroa J, Corrales AR, Flexas J, Vicente-Carbajosa J, Medina J and Nebauer SG (2017) Ectopic Expression of CDF3 Genes in Tomato Enhances Biomass Production and Yield under Salinity Stress Conditions Front. Plant Sci. 8:660. doi: 10.3389/fpls.2017.00660
Received: 02 November 2016; Accepted: 11 April 2017;
Published: 03 May 2017.
Edited by:
Vicent Arbona, Jaume I University, SpainReviewed by:
Carlos Jose De Ollas, Jaume I University, SpainAaron M. Rashotte, Auburn University, USA
Copyright © 2017 Renau-Morata, Molina, Carrillo, Cebolla-Cornejo, Sánchez-Perales, Pollmann, Domínguez-Figueroa, Corrales, Flexas, Vicente-Carbajosa, Medina and Nebauer. This is an open-access article distributed under the terms of the Creative Commons Attribution License (CC BY). The use, distribution or reproduction in other forums is permitted, provided the original author(s) or licensor are credited and that the original publication in this journal is cited, in accordance with accepted academic practice. No use, distribution or reproduction is permitted which does not comply with these terms.
*Correspondence: Rosa V. Molina, cnZtb2xpbmFAYnZnLnVwdi5lcw==
Sergio G. Nebauer, c2VyZ29ubmVAYnZnLnVwdi5lcw==
†These authors have contributed equally to this work.