- 1College of Horticulture, Fujian Agriculture and Forestry University, Fuzhou, China
- 2Department of Environmental Horticulture, Mid-Florida Research and Education Center, Institute of Food and Agricultural Sciences, University of Florida, Apopka, FL, USA
- 3Tropical Crops Genetic Resources Institute, Chinese Academy of Tropical Agricultural Sciences, Danzhou, China
- 4Hunan Key Laboratory for Breeding of Clonally Propagated Forest Trees, Hunan Academy of Forestry, Changsha, China
Titanium (Ti) is considered a beneficial element for plant growth. Ti applied via roots or leaves at low concentrations has been documented to improve crop performance through stimulating the activity of certain enzymes, enhancing chlorophyll content and photosynthesis, promoting nutrient uptake, strengthening stress tolerance, and improving crop yield and quality. Commercial fertilizers containing Ti, such as Tytanit and Mg-Titanit, have been used as biostimulants for improving crop production; however, mechanisms underlying the beneficial effects still remain unclear. In this article, we propose that the beneficial roles Ti plays in plants lie in its interaction with other nutrient elements primarily iron (Fe). Fe and Ti have synergistic and antagonistic relationships. When plants experience Fe deficiency, Ti helps induce the expression of genes related to Fe acquisition, thereby enhancing Fe uptake and utilization and subsequently improving plant growth. Plants may have proteins that either specifically or nonspecifically bind with Ti. When Ti concentration is high in plants, Ti competes with Fe for ligands or proteins. The competition could be severe, resulting in Ti phytotoxicity. As a result, the beneficial effects of Ti become more pronounced during the time when plants experience low or deficient Fe supply.
Introduction
Titanium (Ti), which has an atomic number 22 and atomic weight 47.88, is a transition element belonging to Group 4 (IVB) in the middle of the Periodical Table. It is the ninth most abundant element in the earth's crust and makes up about 0.25% by moles and 0.57% by weight of the crust (Buettner and Valentine, 2012). Ti is the second most abundant transition metal, after iron (Fe), and the elemental abundance of Ti is about 5 times less than Fe and 100 times greater than copper (Cu). Ti exhibits oxidation states of Ti2+, Ti3+ (titanous), and Ti4+ (titanic), of which Ti2+ and Ti3+ are unstable, while Ti4+ is the most stable ion. The most important compound is TiO2, which is mainly used in paints. TiCl4 is water soluble but is highly volatile and forms spectacular opaque clouds upon contact with humid air. Ti ascorbate is a synthesized compound which is soluble in water and stable up to pH 8.0.
The mineral sources of Ti include anatase, rutile, and brookite, each encompassing about 95% TiO2 as well as ilmenite (FeOTiO3) comprising 40–65% TiO2 and leucoxene (Fe2O3 nTiO3) containing more than 65% TiO2 (Zhang et al., 2011). These minerals are generally not soluble; thus Ti has been conventionally considered to be inert in the environment. Increasing evidence in the literature, however, suggests that Ti is mobile in rocks under weathering conditions (Kaup and Carter, 1987; Du et al., 2012). Ti may be mobile at the centimeter scale as well as at the profile scale under strong tropical weathering conditions (Cornu et al., 1999). Higher Ti contents occur in tropical soils, particularly in lateritic soils and laterites, such as 15% in Hawaii soils (Sherman, 1952); 15% in Norfolk Island soils (Hutton and Stephens, 1956), and 3.4% in Australian soils (Stace et al., 1968). Ti in surface soils worldwide ranges from 0.02 to 2.4% with a mean of 0.33%; Ti in soil solutions is about 30 mg L−1 (Kabata-Pendias and Mukherjee, 2007). Ti in river waters ranges from 0.02 to 2.3 μg L−1, and the worldwide average is estimated to be 0.49 μg L−1 (Kabata-Pendias and Pendias, 2001). Drinking waters in the US contain Ti from 0.5 to 15 μg L−1 (Anke and Seifert, 2004). Ti also exists in the atmosphere with global median values of 7 ng m−3 in the remote regions (away from anthropogenic releases) and 85 ng m−3 in polluted zones. Ti concentrations in the air of the US vary from 10 to 100 ng m−3 and can increase up to ≤1,000 ng m−3 in industrial regions (Kabata-Pendias and Mukherjee, 2007).
Titanium dioxide nanoparticles (TiO2NPs) are another form of Ti in the environment. TiO2NPs are produced worldwide at an estimated 88,000 t per year (Keller et al., 2013) and are utilized widely in the cosmetic, food, painting, and plastic industries. Due to their photoprotective and photocatalytic roles, TiO2NPs are also used for plant protection and environmental remediation. It is estimated that the concentrations of TiO2NPs in soils could reach 0.13 μg kg−1 yr−1 in Europe, and TiO2NPs in soils amended with sewage could be much higher up to 1,200 μg kg−1 yr−1 (Sun et al., 2014). With the increased exploration of nanomaterials for novel commercial applications, TiO2NPs in soils could increase from 3 to more than 5,000 μg kg−1 yr−1 (Gogos et al., 2012; Kah et al., 2013).
Ti in Higher Plants
The earth contains 92 elements, of which 82 can be found in plants (Reimann et al., 2001). Ti contents in plants range from 1 to 578 mg kg−1 with a mean of 33.4 mg kg−1 across the listed species (Table 1) excluding two Ti accumulators: horsetail (Equisetum spp.) and beach morning glory [Ipomoea pes-caprae (L.) R. Br.]. There are several factors affecting plant absorption of Ti: (1) Plant species differ in Ti uptake. Ti concentrations vary from 20 mg kg−1 in red cabbage (Brassica oleracea var. capitata f. rubra) to 1,900 mg kg−1 in the wood of pedunculate oak (Quercus robur L.) (Dumon and Ernst, 1988). Ti in horsetail ranged from 42 to 14,000 mg kg−1 when grown in soils rich in lead and zinc (Cannon et al., 1968). (2) Plants respond to Ti addition regardless of soil application or hydroponic culture. Increased Ti application elevates Ti concentrations in crops, such as cabbage (Hara et al., 1976), common bean (Phaseolus vulgaris L.) (Ram et al., 1983), corn (Zea mays L.) (Pais, 1983), and pepper (Capsicum annuum L.) (Giménez et al., 1990). Plant roots accumulate more Ti with a small amount transported to shoots (Kelemen et al., 1993). (3) Soil pH significantly affects the absorption of Ti in plants. Acid sandy soil (pH 3.1) increased Ti solubility resulting in Ti concentrations in leaves of gray hair grass (Corynephorus canescens P. Beauv.) and Sheep's sorrel (Rumex acetosella L.) up to 142 and 207 mg kg−1, respectively; however, leaf Ti concentrations of the same species were only 2.4 and 4.8 mg kg−1, respectively when grown in a soil with nearly identical total Ti concentrations but a pH at 4.9 (Ernst, 1985). Ti concentration in beach morning glory ranged from 310 to 480 mg kg−1 when grown in the ilmenite soil with a pH range of 7.8–7.9, whereas Ti concentration was 910 to 1,300 mg kg−1 in a pH range from 7.3 to 7.4 (Ramakrishna et al., 1989). (4) Foliar application is more effective for Ti absorption. Ti content in leaves and stems increased with Ti sprays but the increase was limited in soil application (Wojcik and Wojcik, 2001). Tapertip hawksbeard (Crepis acuminata Nutt.) is a dust-indicator plant, and seedlings of this species showed an 11-fold increase in Ti after being exposed to contaminated soil dusts (Cook et al., 2009). (5) Ti deficiency symptoms have not been described in plants. Ti supplied at low concentrations has been shown to positively affect plant growth (Figure 1) but causes phytotoxicity at high concentrations (Wallace et al., 1977).
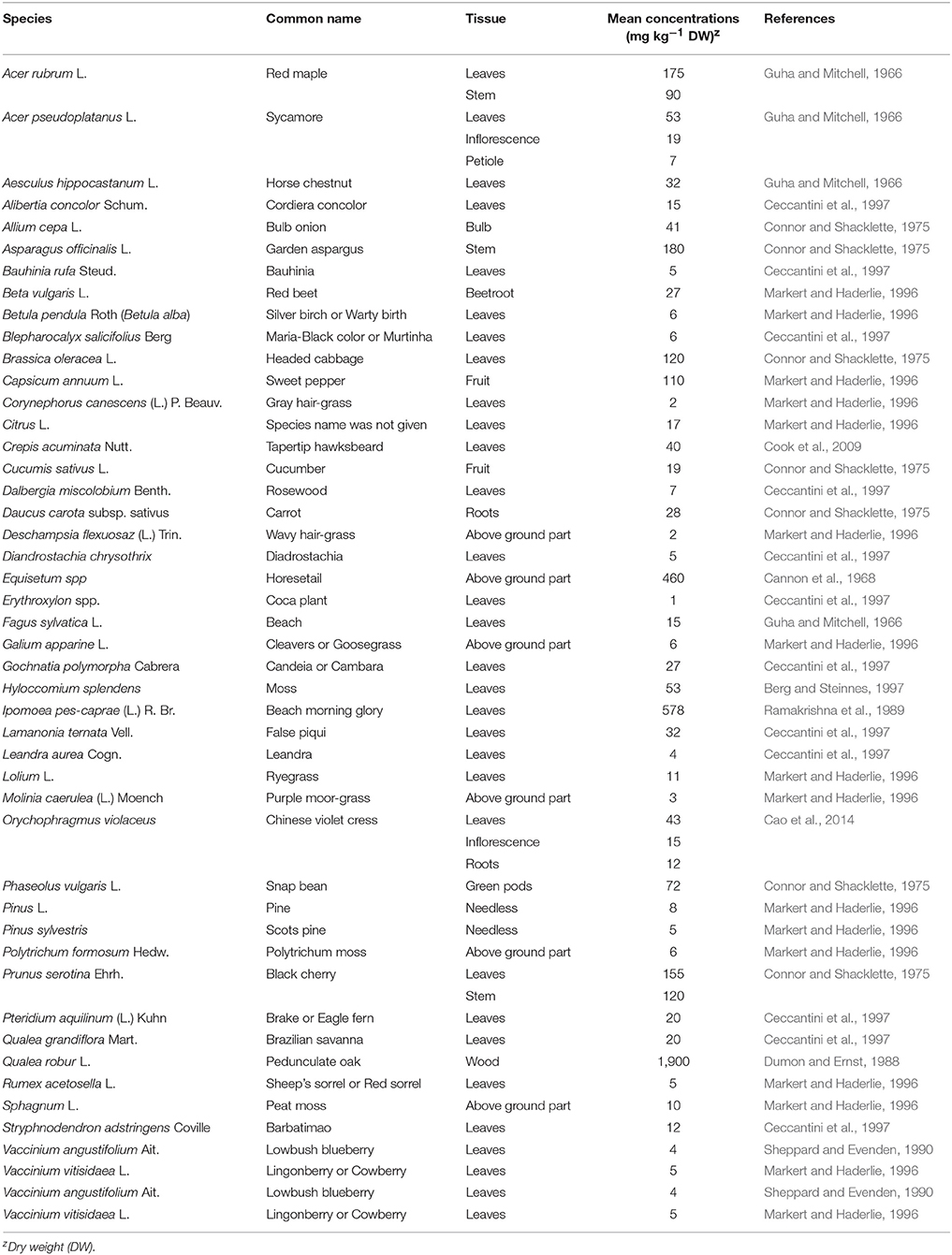
Table 1. Concentration of titanium in plants grown in soils where titanium was not applied via roots or leaves.
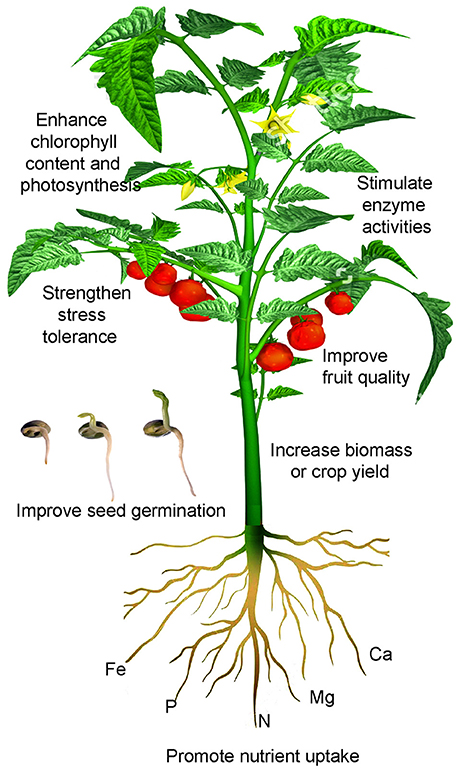
Figure 1. A schematic illustration of Ti effects on crop performance. Ti applied via roots or leaves at appropriately low concentrations has been shown to promote seed germination, enhance root uptake of other nutrient elements, stimulate the activity of some enzymes, increase chlorophyll biosynthesis and photosynthesis, strengthen stress tolerance, and improve crop quality and yield.
Ti Improves Plant Performance
The biological role of Ti in plants has been studied for more than 100 years. Pellet and Fribourg (1905) were the first to study Ti in soils and sugar cane (Saccharum spp.) and sugar beets (Beta vulgaris L.). Traetta-Mosca (1913) observed that Ti enhanced the growth of tobacco (Nicotiana tabacum L.) leaves and believed that Ti was an inherent constituent of the ash from all plants. They proposed that Ti might participate in plant metabolism as a redox catalyst. Geilmann (1920) found that Ti mainly accumulated in assimilation organs. A systematic study of plant responses to different concentrations of Ti by Němec and Káš (1923) showed that optimal levels of Ti caused increased plant growth and development and increased the intensity of green color (higher chlorophyll content) of mustard (Brassica arvensis L.), pea (Pisum sativum L.), and alfalfa (Medicago sativa L.). Subsequently, a great deal of attention from the 1920s to early 1970s has been focused on the analysis of Ti contents in wild and cultivated plants (Dumon and Ernst, 1988). Pais et al. (1977) synthesized a Ti compound called Ti-ascorbate with a trade name of Titavit. It was produced by chelating TiCl4 with ascorbic acid in the presence of gaseous HCl. Ti-ascorbate is water soluble, stable up to pH 8, and also not toxic to animals. Since then, Ti-ascorbate has been widely used for Ti-related plant experiments (Pais, 1983; Carvajal and Alcaraz, 1998; Hrubý et al., 2002; Cigler et al., 2010). A commercial product called Tytanit® containing 5% MgO, 10% SO3, and 0.85% other titanium complex was developed and used in central and eastern European countries for improving crop production. Ti has also been used as a beneficial element in China for crop production (Li et al., 2011).
Effects of Ti Compounds
Chelated Ti compounds applied to soils or onto leaves have been shown to increase plant biomass or crop yield (Table 2). Foliar spray of water-soluble Ti at 1 mg L−1 led to a 20% increase of dry matter of common bean (Ram et al., 1983). Application of 0.04% Ti increased total yield of wandflower (Sparaxis tricolor Ker. Gawl.) corms by 20% and commercial yield by 7% (Marcinek and Hetman, 2008). Kleiber and Markiewicz (2013) investigated Ti effects on tomato plants (Solanum lycopersicum L.) and reported that soil addition of 960 g Ti ha−1 for 1 year increased the yield of fruits, but had no significant effects on dry matter and sugars in fruits. Ti addition increased height of some annual bedding plants (Whitted-Haag et al., 2014). Different tissue dry weights of apple trees (Malus pumila Mill.) grown in the Brzenza region of Poland increased after Ti fertilization (Wojcik and Wojcik, 2001). Pais (1983) summarized Ti experiments conducted from 1974 to 1983 in Hungary and found that more than 90% of the described experiments showed yield increase ranging from 10 to 20% in different crops.
Plant biomass or crop yield increase has been attributed to Ti-enhanced chlorophyll biosynthesis and enzymatic activities and increased photosynthesis and nutrient uptake (Dumon and Ernst, 1988; Cigler et al., 2010). Ti application increased the concentration of chlorophyll a and b as well as total chlorophyll in common bean (Ram et al., 1983), wheat (Triticum aestivum L.) (Kovacik et al., 2014), and other plant species (Traetta-Mosca, 1913; Bottini, 1964; Pais et al., 1969, 1977). Ti enhanced photosynthetic oxygen evolution and generated a three-fold increase of fructose-1,6-biphosphatase in blue green algae (Anacystis nidulans Drouet and Daily) (Kiss et al., 1985). Ti stimulates the activity of nitrate reductase in common bean (Nautsch-Laufer, 1974). Catalase was activated by Ti-ascorbate and TiCl4 at all development stages of embryos, seeds, and seedlings of red pepper (Capsicum annuum L.) (Carvajal et al., 1994). Lipoxygenase (Daood et al., 1988) and phosphofructokinase activities (Simon et al., 1988) were enhanced in tomato plants after Ti addition. Ti application also boosted plants' abilities to take up other nutrients. The contents of N, P, Ca, and Mg of greenhouse-grown tomato plants increased after Ti application (Kleiber and Markiewicz, 2013). Leaves of paprika pepper (Capsicum annuum L.) sprayed with Ti-ascorbate showed a significant increase of Fe and Ti concentrations (Carvajal et al., 1995).
Application of Ti can also improve crop quality. Spice red pepper (Capsicum annuum L. cv. Mihalyteleki) treated with Ti-ascorbate showed increased concentrations of β-carotene and xanthophylls; capsanthin content also increased 1.4 times as a function of Ti addition (Biacs et al., 1997). Tomato plants grown on rockwool supplied with a nutrient solution containing Ti equivalent to 80 g per hectare a year had elevated levels of vitamin C and total sugar in the fruits (Kleiber and Markiewicz, 2013). Foliar spray of Ti increased vitamin C biosynthesis in fruits of peppers (Martinez-Sanchez et al., 1993). Ti application also increased vitamin C contents in six cultivars of strawberries (Fragaria x ananassa Duch.) and anthocyanin contents in three cultivars (Skupień and Oszmiański, 2007). Fruit soluble solids, firmness and size of three primocane raspberry (Rubus idaeus L.) cultivars increased after the fruits were sprayed with Tytanit before harvest (Grajkowski and Ochmian, 2007). Pre-harvest spraying of a solution containing 0.1 mM Ca2+, 0.103 mM Mg2+, or 0.042 mM Ti4+ to peaches (Prunus persica L.) and nectarines (Prunus persica L., Batsch, var. nucipersica) improved fruit color, ripening index and firmness at harvest (Serrano et al., 2004). Peach fruit weight and firmness significantly increased, and weight loss during storage significantly decreased after foliar application of Ti, or Ti with Ca and/or Mg before harvest (Alcaraz-Lopez et al., 2004a,b).
Effects of TiO2NPs
There has been an increasing amount of attention in the literature regarding effects of TiO2NPs on plant performance (Tables 3, 4). TiO2NPs have been studied for influence on seed germination. Seeds treated with TiO2NPs suspensions exhibited increased germination rates, enhanced root lengths or improved seedling growth of Arabidopsis thaliana (L.) Heynh. (Szymanska et al., 2016), cabbage (Andersen et al., 2016), oilseed rape or canola (Brassica napus L.) (Mahmoodzadeh et al., 2013), corn (Andersen et al., 2016), cucumber (Servin et al., 2012), fennel (Foeniculum vulgare Mill.) (Feizi et al., 2013), lettuce (Lactuca sativa L.) (Andersen et al., 2016), oat (Avena sativa L.) (Andersen et al., 2016), onion (Allium cepa L.) (Haghighi and Teixeira da Silva, 2014), parsley (Petroselinum crispum Mill.) (Dehkourdi and Mosavi, 2013), red clover (Trifolium pretense L.) (Gogos et al., 2016), soybean (Glycine max Merr.) (Rezaei et al., 2015), spinach (Spinacia oleracea L.) (Zheng et al., 2005), tomato (Haghighi and Teixeira da Silva, 2014), and wheat (Feizi et al., 2012; Mahmoodzadeh and Aghili, 2014; Gogos et al., 2016). Application of TiO2NPs increased plant tolerance to abiotic and biotic stresses, including cold stress in chickpea (Cicer arietinum L.) (Mohammadi et al., 2013, 2014), heat stress in tomato (Qi et al., 2013), drought in wheat (Jaberzadeh et al., 2013) and flax (Linum usitatissium L.) (Aghdam et al., 2016), cadmium toxicity in green algae (Chlamydomonas reinhardtii P.A. Dang) and soybean (Yang et al., 2012; Singh and Lee, 2016), and bacterial spot disease caused by Xanthomonas perforans in tomato (Paret et al., 2013). Foliar spray of TiO2NPs increased chlorophyll content in tomato (Raliya et al., 2015a) and oilseed rape (Li et al., 2015), enhanced the activity of Rubisco (Ribulose-1,5-bisphosphate carboxylase/oxygenase), and promoted net photosynthesis in Arabidopsis (Ze et al., 2011), spinach (Hong et al., 2005a,b; Lei et al., 2007, 2008), tomato (Qi et al., 2013), and basil (Ocimum basilicum L.) (Kiapour et al., 2015). TiO2NPs treatments significantly increased crop yield or biomass of barley (Moaveni et al., 2011), corn (Moaveni and Kheiri, 2011; Morteza et al., 2013), mung bean (Vigna radiate L.), snail clover (Medicago scutellata Mil.), tomato (Raliya et al., 2015a,b), and wheat (Rafique et al., 2015).
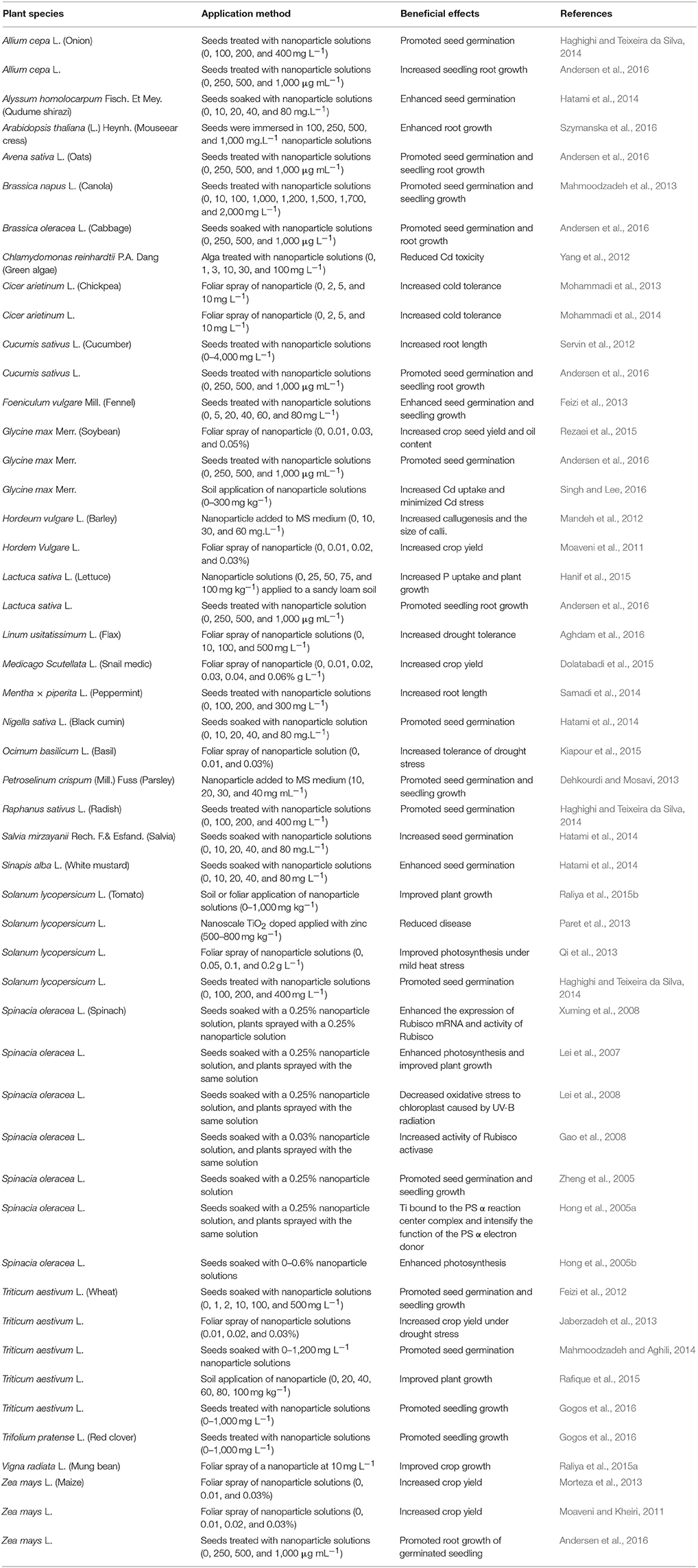
Table 3. Beneficial effects of titanium dioxide nanoparticles (TiO2NPs) on seed germination and plant growth.
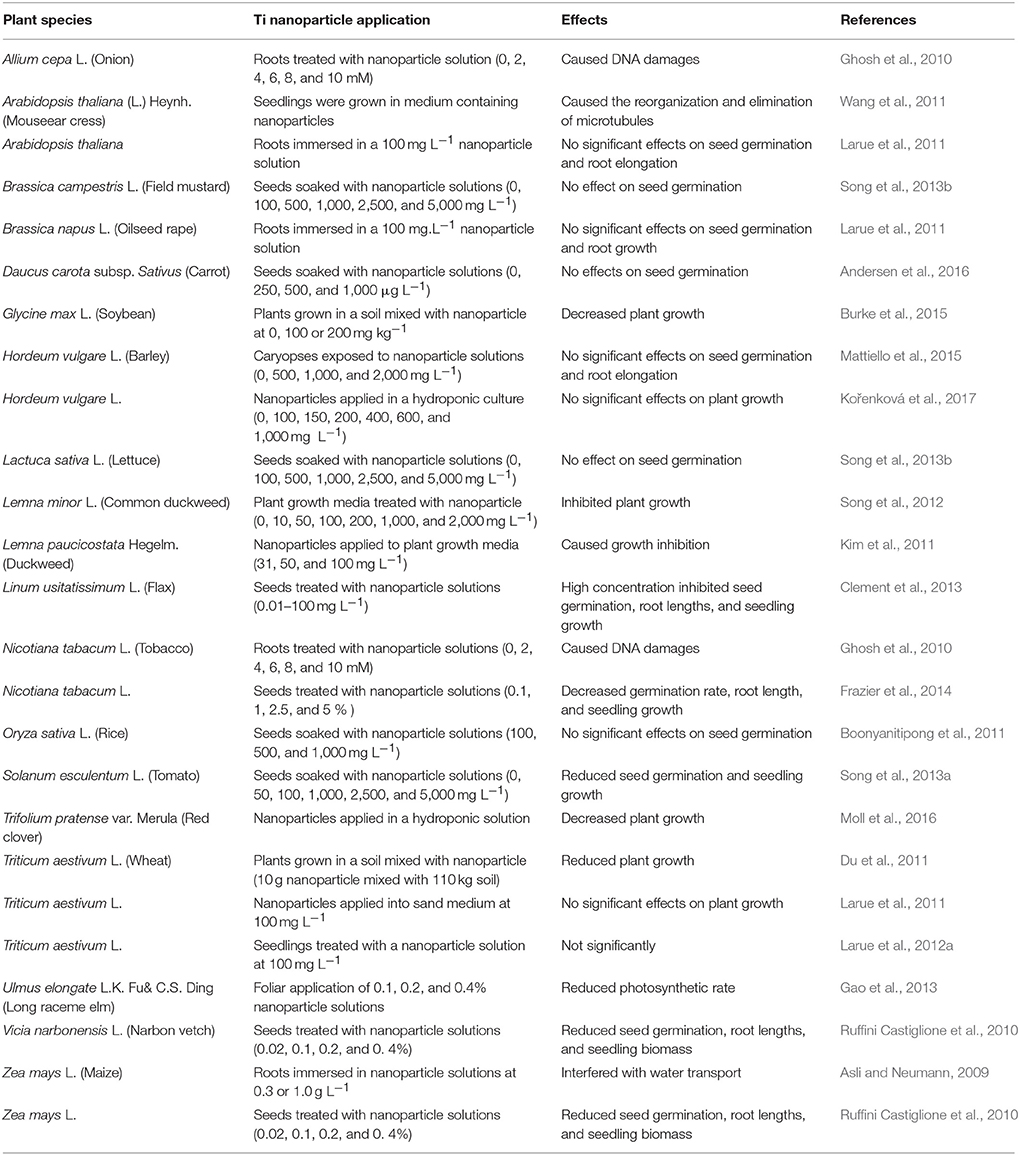
Table 4. Negative or neutral effects of titanium dioxide nanoparticles (TiO2NPs) on seed germination and plant growth.
Application of TiO2NPs may not produce positive results. As presented in Table 4, some effects were neutral or negative. Less positive results could be attributed to several factors including differences in plant species, physiological status of plants at the time being evaluated, seed quality, TiO2NPs sizes and their uniformity, and experimental objectives and methods. For example, some experiments used TiO2NPs at concentrations up to 5,000 μg mL−1; such high concentrations may not occur naturally in the environment, and results from the studies may not provide complete information about the roles of TiO2NPs in plants. However, attention does need to be given to the fate and consequence of applied TiO2NPs within the environment and food chain (Cox et al., 2016; Tripathi et al., 2017); more thorough research in this regard should be pursued.
Ti as a Beneficial Element to Crop Production
Results from the literature in general suggest that Ti has positive effects on plant growth and crop quality. Ti, however, is not an essential element for plant nutrition based on the criteria for essentiality (Arnon and Stout, 1939). Plants can complete their life cycle without Ti; there is no reported Ti deficiency in plants; and mechanisms of Ti action are still uncertain. As a result, Ti is considered a beneficial element proposed by Pais (1992) because it improves plant health status at low concentrations but has toxic effects at high concentrations.
As far as is known, critical tissue concentrations for Ti that are considered to be appropriate for enhancing plant growth or potentially toxic to plants have not been well determined (Huang et al., 1993; Kuzel et al., 2007). Ceccantini et al. (1997) and Tlustoš et al. (2005) stated that Ti content in plants usually varies from 0.1 to 12.0 mg kg−1 of dry matter. The growth of bush bean plants was not significantly different when leaf Ti contents varied from 1.2 to 11.7 mg kg−1 (Wallace et al., 1977). Table grape (Vitis vinifera L.) plants were healthy with a mean Ti content of 17.8 mg kg−1 in leaves (Alcaraz-Lopez et al., 2005). Oilseed rape plants grew healthily with Ti content in shoots ranging from 16.8 to 66.7 mg kg−1 during their flowering period (Kovacik et al., 2016). The mean Ti content in plants listed in Table 1 is 33.4 mg kg−1 excluding two Ti accumulators: horsetail and beach morning glory. We propose that Ti contents in leaf tissues below 15 mg kg−1 based on dry weight could be appropriate for plant growth. So far, limited information is available regarding critical levels of Ti in plant toxicity. Wallace et al. (1977) reported dramatic decrease in bush bean growth when Ti in leaf tissue was 202 mg kg−1. Kabata-Pendias and Pendias (2001) suggested that Ti content in mature leaves ranging from 50 to 200 mg kg−1 could be excessive or toxic. We propose that Ti contents in leaf tissues above 50 mg kg−1 could potentially be toxic to plants. Morphological symptoms of Ti toxicity include chlorotic and necrotic spots on leaves (Wallace et al., 1977) and reduced plant growth and crop yield.
Mechanisms of Action
Several explanations have been proposed concerning the actions of Ti as a beneficial element to plants, including (1) participation in N fixation in the nodules of legumes (Konishi and Tsuge, 1936); (2) influence on plant metabolism by increasing absorption of other nutrient elements, such as Fe and Mg (Dumon and Ernst, 1988; Simon et al., 1988); (3) involvement in redox system reactions (Ti4+/Ti3+ with Fe3+/Fe2+) thus improving the Fe activity in plant tissues (Carvajal et al., 1995) or interaction with Fe in electron transport chain and decrease of the photosystem II efficiency at a high Ti concentration (Cigler et al., 2010); (4) stimulation of enzymatic activities and photosynthesis (Carvajal and Alcaraz, 1998); and (5) hormesis (Hrubý et al., 2002; Kuzel et al., 2003). Among these claims, Ti participation in N fixation has not been documented thereafter the initial report (Konishi and Tsuge, 1936); as a result, this claim may not be valid. Hormesis is a term used by toxicologists to refer to a biphasic dose response to an environmental agent characterized by low dose stimulation or beneficial effects and a high dose inhibitory or toxic effect (Mattson, 2008). It is a biological phenomenon for almost any chemical element or drug in living things, and it cannot be considered a specific mechanism for Ti actions in plants. The other explanations are mainly focused on the physiological roles of Ti in plants and have not explored any cellular or molecular mechanisms underpinning its actions.
A common characteristic of beneficial elements is their ability to positively interact with one or more essential elements, primarily by partial substitution of essential elements: such as sodium (Na) with potassium (K), selenium (Se) with sulfur (S), cobalt (Co) with nickel (Ni), and silicon (Si) with boron (B), manganese (Mn), and phosphorus (P). Such interactions could be synergistic at a certain concentration range but may become antagonistic when the concentration is too high. For example, when K supply becomes limited in soils, Na can partially substitute for K in osmoregulation (Marschner, 2011). Both elements are alkali metals in the Group 1 column of the Periodic Table and have similar physical and chemical properties. Like K, Na can enter plant cells through K channels (Demidchik et al., 2002). Se and S are both Group VIA elements in the Periodic Table and share similar chemical properties. Se is absorbed by plants in the form of selenate through sulfate transporters (Cabannes et al., 2011). S uptake is enhanced by rhizosphere selenate; however, Se toxicity occurs if Se and S compete for a biochemical process (White et al., 2004). Co and Ni are both transition metals and are generally found together in nature. Co is synergistically related to Ni, and reports showed that toxic Co levels of 10–20 mg kg−1 dry mater were associated with excess Ni (Anderson et al., 1973). This is because Co and Ni share the same plasma membrane carriers (Pilon-Smits et al., 2009).
We here propose that the beneficial roles Ti plays in plants lie in its interaction with other nutrient elements, primarily Fe. This proposal is not new and has been postulated by Simon et al. (1988), Carvajal and Alcaraz (1998), and Cigler et al. (2010). More specifically, we hypothesize that Ti and Fe have synergistic and antagonistic relationships. When plants encounter Fe deficiency, Ti could induce the expression of genes related to Fe acquisition, enhancing Fe uptake and utilization and subsequently improving plant growth. Plants could have proteins that either specifically or nonspecifically bind with Ti. When Ti concentration is high in plants, it may compete with Fe for ligands or proteins. The competition could be severe, resulting in Ti phytotoxicity. As such, the beneficial effects of Ti could be particularly visible or measurable during the time when plants are near to or are experiencing Fe deficiency. This hypothesis relies on the beneficial effects of Ti that have been reviewed above and will be elaborated further in subsequent sections of this review.
Ti and Fe have similar physical and chemical properties. Both Ti and Fe are transition metals. The ionic radius and Pauling electronegativity of Ti are 0.7 Å and 1.54; the same parameters for Fe are 0.9 to 0.7 Å and 1.83. Ti and Fe occur together in nature. During magmatic processes, Ti follows Fe in magmatic crystallization. Ti4+ is predominantly partitioned into Fe-Ti or Fe oxides, such as ilmenite (FeTiO3) and magnetite (Fe3O4), or into one or more of the TiO2 phases, rutile (TiO2), and anatase (TiO2). The Ti-Fe-oxides and their relationships have been illustrated by triangular FeO-TiO2-Fe2O3 diagrams (Bowles et al., 2011). Ilmenite (FeTiO3) is the most widespread form of TiO2-bearing mineral around the world, and it provides 90% of the total world Ti. Ti has been shown to be mobile in rocks under weathering conditions and also in soils (Cornu et al., 1999). It could be possible that adaptation of plants to soils containing heavy mineral sands (derived from the weathering of ilmenite) might enable roots to absorb both Fe and Ti. Due to the abundance of Fe in soil relative to Ti and its biological functionality, more Fe is absorbed by and translocated in plants. As a result, Fe has been fulfilling much more important roles in plants. Fe is thus considered an essential element to plants, while Ti plays a complementary role, i.e., it is often found along with Fe and plays both synergistic and antagonistic roles depending on Fe concentrations in plant cells.
Ti Uptake by Plants
Plant uptake of ions through roots or leaves involves both passive absorption and active transport. Passive absorption is facilitated by concentration gradients of an ion, while active transport is driven by the electrochemical gradient generated by H+-ATPase to allow selective ions to move across the plasma membrane through specific carriers or transporters.
Root Uptake
There has been no report about how Ti in bulk form is absorbed by roots. Plant uptake of Fe, however, has been well studied. Plant roots use two strategies for acquisition of Fe from soils: the reduction-based strategy I in non-graminaceous plants and the chelation-based strategy II in graminaceous plants (Takagi, 1976; Römheld and Marschner, 1986). In non-graminaceous plants, Fe deficiency induces the activity of ferric reduction oxidase 2 (FRO2), which results in the reduction of Fe3+ to Fe2+, and Fe2+ is then transported inside the root cells by an iron-regulated transporter (IRT1) located at the plasmalemma of root epidermal cells. The IRT1/FRO2 system is subjected to complex transcriptional and post-transcriptional regulations, involving Fe itself as a local inducer, and also uncharacterized systemic signals (Kobayashi and Nishizawa, 2012). In graminaceous plants, such as maize, Fe deficiency induces root secretion of deoxymugineic acid (DMA), which is synthesized from nicotianamine, a secondary amino-acid derived from methionine. DMA has a strong affinity for Fe3+, and the Fe3+-DMA chelate is transported inside the root cells by a specific transporter YS1 (yellow stripe 1). As we proposed above, the roles Ti plays in plants lie in its interaction with Fe. We hypothesize that root uptake of Ti could occur as follows: In roots of non-graminaceous plants, the applied Ti (Ti-ascorbate) could be reduced by FRO or not be reduced and could enter plant cells through the IRT1. In roots of graminaceous plants, since Ti is often applied as Ti-ascorbate, it may not be chelated with phytosiderphore, and Ti-ascorbate could directly enter cells via YS1.
Root uptake of TiO2NPs appears to be size selective (Tripathi et al., 2017). Larue et al. (2012a,b) proposed that threshold diameters for movement of TiO2NPs through root epidermis of wheat plants should be smaller than 140 nm; thresholds for transferring through parenchyma are 36 nm or less; and for passing through the Casparian band (CB), particle diameters should be strictly smaller than 36 nm. The authors further observed that TiO2NPs smaller than 36 nm could be transported to the stele in two ways: direct penetration of CB, this was based on the transmission electron microscopy observation that 14 nm TiO2NPs were inside thick CB walls of wheat roots, implying the TiO2NPs had crossed the CB. The other pathway is through plasmodesmata (Larue et al., 2012a,b). Additionally, TiO2NPs may enter plant cells through endocytosis as NPs have been shown to activate membrane receptors and induce endocytosis (Iversen et al., 2011). So far, there are no reports regarding active transport of TiO2NPs through either carriers or transporters as mentioned for bulk materials. Root absorption of an ultrasmall TiO2NP (<5 nm) was reported to be complexed with Alizarin red S nanoconjugate in Arabidopsis (Kurepa et al., 2010). Whether or not such a complex was absorbed through transporters or carriers is unclear.
Leaf Absorption
Ti in both bulk and nanoparticles has been applied as liquid form to above-ground plant parts, commonly known as foliar spray or foliar application (Tables 2–4). Leaf absorption initially is a nonselective and passive process driven by concentration gradients between the outside and inside of the leaf surface (Eichert and Fernandez, 2012; Fallahi and Eichert, 2013). Since foliar applied Ti is chelated with either ascorbate or citrate, it could be likely that Ti may enter the leaf apoplast through the same routes as Fe, i.e., stomata, cuticular cracks (cracks on the cuticular surface), ectodesmata, lenticels or aqueous pores (Pandey et al., 2013). After arriving in the apoplast, Ti could be transported to symplast through the active process. The mechanism by which Ti crosses cell membranes is unknown; we assume that it could be similar to root absorption of Ti through Fe transporters.
Leaf absorption of TiO2NPs to apoplast could be via the same paths as the bulk materials. Due to the size effects, however, small-diameter TiO2NPs may gain access to symplast through direct penetration. In an experiment with TiO2NPs, Fe2O3NPs, and MgONPs, Wang et al. (2013) found that NPs entered leaf symplast of watermelon (Citrullus lanatus Matsum. & Nakai) via stomata. Raliya et al. (2015a,b) studied effects of TiO2NPs and ZnO2NPs on tomato plants and reported that foliar-applied TiO2NPs and ZnO2NPS may enter leaf cells through stomata, cuticle wounds, and direct penetration.
Seed Absorption
TiO2NPs have been used for seed treatment. Seeds soaked in TiO2NPs solutions exhibited higher germination rates, increased root elongation, and improved seedling growth (Table 3). It is generally agreed that nanoparticles are able to penetrate the seed coat, resulting in increased water/nutrient absorption and improved seed germination (Hatami et al., 2014; Zhang et al., 2015; Cox et al., 2016). However, negative effects, mainly phytotoxicities, have been reported (Table 4). The negative effects could be due in part to the penetration-resultant injury. TiO2NPs randomly penetrate seeds. If the penetration damaged cell membranes or embryos, seed germination and subsequent growth could be adversely affected. It is worth mentioning that physiochemical properties of TiO2NPs rely on the NP size, morphology, and surface area (Dietz and Herth, 2011); these properties along with TiO2NPs concentrations are critically important for evaluation of biological materials. Some of the reported evaluations used TiO2NPs with variable particle sizes, and others used concentrations much higher than those commonly encountered in the environment or normally used for evaluating other nutrient elements. These may contribute to the negative effects of TiO2NPs on seed germination.
Ti Translocation in Plants
Ti absorbed via roots or leaves is translocated to the other organs. Like most transition elements, root-absorbed Ti is largely accumulated in the roots with a small amount transported to shoots through xylem stream (Kelemen et al., 1993). Ti absorbed by leaves is translocated via phloem flow.
Ti Distribution in Plants
Nautsch-Laufer (1974) was first to report the cellular distribution of Ti in plants. When corn plants were grown in a nutrient solution containing 144 mg L−1 Ti, 65% of cellular Ti was found in the cell wall, 27.7% in leaf cell vacuoles, and 5.1% in root cell vacuoles. Later, Kelemen et al. (1993) studied the distribution and intracellular location of Ti in wheat plants. Foliar-applied Ti was found to be unidirectionally translocated from shoots into roots, and the majority of Ti in treated cells was in a diffusible form except for those bound firmly with nuclei. Since then, there has been no report concerning the cellular distribution of bulk Ti compounds in plants.
Recently, several studies documented the distribution of TiO2NPs in plants. Larue et al. (2012a,b) reported that root-absorbed TiO2NPs with a diameter of 14 nm were translocated to entire wheat plants without modification of crystal phase. Aerosolized TiO2NPs with particle diameter less than 100 nm could enter leaf cells through stomata and then be distributed to stem and roots of watermelon (Wang et al., 2013). The contents of TiO2 in leaves, shoots, and roots of watermelon were 61.25, 33.3, and 5.45%, respectively. When TiO2NPs consisting of 82% anatase and 18% rutile were used for hydroponic production of cucumber, root-absorbed TiO2NPs were translocated to shoots (Servin et al., 2012, 2013). Ti was found in dermal cells, mesophyll, vascular systems, and trichomes of leaves as well as cucumber fruit. Ti in rutile phase was observed mainly in aerial tissues, but anatase remained in root tissues due to the size difference. Raliya et al. (2015a,b) reported that foliar applied TiO2NPs were transported in a bidirectional manner, and the concentration of Ti in tomato plant tissues was in an order of stem > roots > leaves > fruits.
The distribution of Ti has been documented, but how it is translocated in plants is unclear. Fe is translocated from roots to leaves by chelating with citrate through xylem vessels. Small organic molecules and various transporters, such as NRAMPs (natural resistance-associated macrophage protein) and VIT1 (vacuolar iron transporter 1), are then responsible for Fe distribution among various organs and among various subcellular compartments (Kobayashi and Nishizawa, 2012). We assume that root-absorbed Ti-ascorbate could be directly transported to leaves through xylem vessels and the transporters that facilitate Fe distribution might also be able to translocate Ti to different organs and various subcellular locations.
Ti Binding Proteins
The most stable oxidation state of Ti in an aqueous oxygenated environment is Ti4+, which shares the ionic radius of Fe3+. Ti and Fe also share a thermodynamic preference for similar binding sites, though Ti4+ is more strongly Lewis acidic (Zierden and Valentine, 2016). In animal cells, Ti4+ has been shown to bind tightly to universal iron-carrier proteins (transferrins) which carried them into the tumor cell (Guo et al., 2000). Typical animal transferrins are about 80-kDa soluble proteins involved in binding, mobilizing, and delivering Fe. Tinoco and Valentine (2005) also found that in vitro Ti4+ binds more tightly than Fe3+ to human transferrins. A novel transferrin-like protein was identified in unicellular green alga (Dunaliella salina Teodor) (Fisher et al., 1997, 1998). However, such types of proteins have not yet been identified in higher plants.
The roles Ti exhibits in plants are similar to those of rare earth elements (REEs). REEs have been widely used in agriculture as plant growth stimulants (Hu et al., 2004; Tyler, 2004). Research on the roles of REEs identified a REE-binding protein in corn (Yuan et al., 2001), two from coral fern [Dicranoptris dichotoma (Thunb.) Dernh.] (Guo et al., 1996), and a REE-binding peptide also from coral fern (Wang et al., 2003). Recent studies showed that REEs lanthanum and terbium can activate plant endocytosis and their entrance to cells by endocytosis (Wang et al., 2014, 2016; Yang et al., 2016). REEs in soil solutions and their contents in plant tissues are much lower than Ti (Tyler, 2004). It is possible that plants may also have proteins that interact with Ti.
We hypothesize that Ti binding proteins occur in plants. Some of them could specifically bind with Ti while others may bind not only with Ti but also with Fe. Like other ions, Ti4+ inclines to hydrolysis and hydrolytic precipitation (Buettner and Valentine, 2012). Binding to biomolecules that are either small or large will significantly increase its solubility. As indicated by Zierden and Valentine (2016), Ti4+ complexes can kinetically display a wide range of ligand exchange rates. Hydroxyl and water ligands are very labile and exchange with rate constants on the order of thousands per second (Comba and Merbach, 1987); whereas the rates for exchange with small bioligands such as ascorbate or citrate, or with transferrin-like proteins transferrins are over minutes to hours (Tinoco and Valentine, 2005; Buettner et al., 2012). As such, Ti may bind with some organic acids, such as citric acid and ascorbic acid to allow the chelated Ti to be easily translocated in plants. Additionally, Fe storage protein ferritins can biomineralize Ti (Klem et al., 2008; Amos et al., 2013). Furthermore, Ti may interact with other proteins. TiO2NPs have been shown to bind to the PSII reaction center complex and enhance the role of the PSII electron donor (Hong et al., 2005a). A recent microarray analysis of TiO2NPs treated Arabidopsis has shown that a series of genes, particularly those associated with photosynthesis were highly upregulated (Tumburu et al., 2015), which provides some fundamental information for further investigation of Ti effects on plants. Nevertheless, we believe that Ti binding proteins could be identified with the advances in omics technologies, and the identification should provide theoretical explanations for the roles Ti plays and its phytotoxicity in plants.
Contributions to Fe Homeostasis
Plant cells contain numerous iron-containing proteins which can be mainly classified into three groups: iron-sulfur cluster proteins, hemeproteins, and non-heme/non-Fe-S proteins (Zhang, 2015). These proteins use Fe as a cofactor and perform critical roles in photosynthesis, genome stability, electron transfer, and oxidation-reduction reactions. Plants have evolved sophisticated mechanisms to maintain iron homeostasis for the assembly of functional iron-containing proteins, thereby ensuring genome stability, cell development, electron transport chain of photosynthesis and respiration in chloroplasts and mitochondria, respectively (Kobayashi and Nishizawa, 2012). Fe is also essential for reactive oxygen species (ROS) detoxification, chlorophyll biosynthesis, period length control of circadian rhythm, and activity of numerous metal-dependent enzymes (Alscher et al., 2002; Moseley et al., 2002; Chen et al., 2013). Most of the Fe in leaves is found within the chloroplasts where photosynthesis takes place to assimilate C and produce O2. In addition to the general mitochondrial Fe-S cluster synthesis pathway, chloroplasts are autonomous for their Fe-S cluster synthesis (Zhang, 2015). It is within this plant specific subcellular compartment that ferritins store and buffer Fe, thereby participating in remediating oxidative stress. Ferritins are plastid proteins whose abundance is strictly controlled at a transcriptional level by the Fe status of the cells (Kobayashi and Nishizawa, 2012; Zhang, 2015).
In the case of Fe and Ti interactions, Ti effects could become more pronounced when plants had deficient supply of Fe. Under such conditions, application of Ti could induce the expression of IRT in nongraminaceous tobacco plants and YS1 in graminaceous corn plants. The expression of ferritin genes could also be enhanced by Ti application. The induced expression of these genes under limited Fe supply might suggest that some roles Ti would play could be the maintenance of Fe homeostasis at the cellular level, thus improving plant growth. Carvajal and Alcaraz (1995) demonstrated that foliar application of Ti-ascorbate resulted in an increase of Fe concentrations in leaves, fruits, chloroplasts, and chromoplasts of red pepper plants. Foliar application of Ti resulted in 39% and 35.7% increase of Fe in peel and flesh of peach fruit (Alcaraz-Lopez et al., 2004a,b). Leaves of paprika pepper sprayed with Ti-ascorbate increased Fe uptake by 50% in a greenhouse experiment and close to 100% in a field experiment, and leaf peroxidase and catalase activities also significantly increased due to the Ti-ascorbate application (Carvajal et al., 1995). These results provide further evidence supporting our hypothesis that the synergetic roles Ti plays become more noticeable when plants encounter low Fe supply. Under a limited Fe supply, application of an appropriate concentration of Ti would induce IRT or YS1 expression, thus enhancing Fe uptake. Increased Fe uptake would increase chlorophyll biosynthesis, subsequently increasing net photosynthesis. Increased photosynthesis directly couples with assimilation in chloroplasts, which is known as nitrate photoassimilation (Searles and Bloom, 2003). The increased photosynthesis would enhance the expression of nitrate transporter genes, consequently increasing N uptake. The increased uptake of could improve plant growth and in turn enhance absorption of other ions. For example, a 7-fold increase in N uptake by rhododendron (Rhododendron spp. cv. P.J.M. Compact) was associated with a 3 to 4-fold increase in the uptake rate of phosphorus, potassium, and sulfur, and ~2-fold increase in the uptake rate of magnesium and calcium (Scagel et al., 2008). Additionally, IRT1 belongs to the ZRT/IRT-like protein (ZIP) gene family, which plays a major role in Fe/Zn (zinc) uptake (Guerinot, 2000). IRT1 can also transport Zn, Co, Mn, and cadmium (Cd) (Eide et al., 1996; Connolly et al., 2002; Varotto et al., 2002; Vert et al., 2002). YS1 functions as a proton-coupled symporter for various DMA-bound metals, including Fe3+, Zn2+, Cu2+, and Ni2+ (Kakei et al., 2012). This may explain why the application of Ti also increases plant uptake of other nutrient elements.
Ti may act antagonistically with Fe resulting in Ti toxicity in plants. If Ti concentration is too high, it could interfere with biological roles of Fe, resulting in Ti toxicity. Cigler et al. (2010) measured chlorophyll fluorescence of spinach plants after treatment by a combination of Fe and Ti. They found that Ti at a high level affects Fe-containing proteins in electron transport, primarily the PSI, slowing down the PSII efficiency. If Ti and Fe were equally present in the medium, the Ti impact on the PSI was lowered, probably due to competition for binding sites.
Photocatalysis and Antimicrobial Roles
Ti in both bulk and nanoparticle forms has been used for suppressing crop diseases (Paret et al., 2013; Servin et al., 2015). Chao and Choi (2005) reported that severity and incidence of curvularia leaf spot [Curvularia lunata (Wakker) Boedijn] and bacterial leaf blight (Xanthomonas oryzae pv. oryzae) in cereal crops were reduced with TiO2 application. Similar results were observed on field-grown cowpea (Vigna unguiculata Walp.) where cercospora leaf spots caused by Cercospora rosicola Pass. and brown blotch caused by Mycosphaerella cruenta Sacc. were significantly suppressed by application of TiO2 (Owolade and Ogunleti, 2008). TiO2 has been shown to control bacterial leaf spot (Xanthomonas hortorum pv. pelargonii) on geranium (Pelargonium x hortorum L.H. Bariley) and (Xanthomonas axonopodis pv. poinsettiicola) on poinsettia (Euphorbia pulcherrima Willd. Ex klotzsch.) (Norman and Chen, 2011). Additionally, the use of TiO2 in recycled irrigation water was shown to eliminate both fungal and bacterial pathogens (Yao et al., 2007).
The antimicrobial roles of TiO2 are related to the oxidation processes even though the role of Ti-uptake resultant biological activities could not be ruled out. Recently, the photocatalytic process by UV/TiO2 is receiving increased attention due to the low cost and relatively high chemical stability of TiO2, especially in aqueous environments. It generates singlet oxygen and superoxide anion which both cause damaging cellular oxidation. Therefore, TiO2 has been used for controlling some bacterial and fungal pathogens in crop production (Yao et al., 2007; Owolade and Ogunleti, 2008; Norman and Chen, 2011) and also for decontaminating toxic organic pollutants in water treatment (Lazar et al., 2012). TiO2NPs have been shown to degrade organic pesticides and herbicides in soils via redox reactions, photocatalysis, and thermal destruction under irradiation (Mir et al., 2014; Li et al., 2016). Photocatalytic TiO2 has been used to kill cancer cells in human (Thevenot et al., 2008), and biomedical applications of TiO2NPs are promising and could play important roles for improving health care, especially cancer treatment (Yin et al., 2013).
Conclusion
Evidence accumulated over the last 100 years suggests that Ti is relatively mobile in soils, occurs in soil solution, and is available to plants. Plants are able to absorb Ti through either roots or leaves, and Ti concentrations in plant tissues are either equal to or higher than some essential nutrient elements. Ti has been shown to improve plant performance at low concentrations. In the present article, we propose Ti and Fe have synergistic and antagonistic relationships. Ti may induce the expression of genes related to Fe acquisition, enhancing Fe uptake and utilization when plants encounter Fe deficiency. The interaction of plants with Ti as well as with Fe may result in the occurrence of Ti binding proteins in plants that either specifically bind with Ti or nonspecifically share with Fe or other elements. When Ti levels are high in plants, Ti may cause phytotoxicity. This hypothesis is not new but is updated based on the current available information. With the advances in omics technologies, we anticipate that this hypothesis will be tested and improved.
Author Contributions
All authors contributed to the acquisition and interpretation of available literature and the conception of the work. JC, SL, and XYW wrote the manuscript, and all authors revised the manuscript and approved this final version.
Conflict of Interest Statement
The authors declare that the research was conducted in the absence of any commercial or financial relationships that could be construed as a potential conflict of interest.
Acknowledgments
The authors would like to thank the Fujian Science and Technology Key Projects (2013NZ0002-1B), Construction of High-level University program of Fujian Agriculture and Forestry University: “Construction of High-level Horticulture Science Discipline” (612014007), and Scientific Research Foundation of Graduate School at the Fujian Agriculture and Forestry 609 University (324-1122YB026) for supporting this study.
References
Aghdam, M. T. B., Mohammadi, H., and Ghorbanpour, M. (2016). Effects of nanoparticulate anatase titanium dioxide on physiological and biochemical performance of Linum usitatissimum (Linaceae) under well-watered and drought stress conditions. Braz. J. Bot. 39, 139–146. doi: 10.1007/s40415-015-0227-x
Alcaraz-Lopez, C., Botia, M., Alcaraz, C. F., and Riquelme, F. (2003). Effects of foliar sprays containing calcium, magnesium and titanium on plum (Prunus domestica L.) fruit quality. J. Plant Physiol. 160, 1441–1446. doi: 10.1078/0176-1617-00999
Alcaraz-Lopez, C., Botia, M., Alcaraz, C. F., and Riquelme, F. (2004a). Effect of the in-season combined leaf supply of calcium, magnesium and titanium on peach (Prunus persica L). J. Sci. Food Agric. 84, 949–954.
Alcaraz-Lopez, C., Botia, M., Alcaraz, C. F., and Riquelme, F. (2004b). “Effects of titanium-containing foliar sprays on calcium assimilation in nectarine fruits,” in Nutriçao Mineral: Causas e Consequências da Dependencia da Fertilaçao, eds M. A. Martin-Luçao and C. Cruz (Faculdade de Ciencias da Universidade de Lisboa), 66–72.
Alcaraz-Lopez, C., Botia, M., Alcaraz, C. F., and Riquelme, F. (2005). Induction of fruit calcium assimilation and its influence on the quality of tabel grapes. Span. J. Agr. Res. 3, 335–343. doi: 10.5424/sjar/2005033-156
Alscher, R. G., Erturk, N., and Heath, L. S. (2002). Role of superoxide dismutases (SODs) in controlling oxidative stress in plants. J. Exp. Bot. 53, 1331–1341. doi: 10.1093/jxb/53.372.1331
Amos, F. F., Cole, K. E., Meserole, R. L., Gaffney, J. P., and Valentine, A. M. (2013). Titanium mineralization in ferritin: a room temperature nonphotochemical preparation and biophysical characterization. J. Biol. Inorg. Chem. 18, 145–152. doi: 10.1007/s00775-012-0959-z
Andersen, C. P., King, G., Plocher, M., Storm, M., Pokhrel, L. R., Johnson, M. G., et al. (2016). Germination and early plant development of ten plant species exposed to titanium dioxide and cerium oxide nanoparticles. Environ. Toxicol. Chem. 35, 2223–2229. doi: 10.1002/etc.3374
Anderson, A. J., Mayer, D. R., and Mayer, F. K. (1973). Heavy metal toxicity: levels of nickel, cobalt, and chromium in the soil and plants associated with visual symptoms and variations in the growth of an oat crop. Aust. J. Agri. Res. 24, 557–571. doi: 10.1071/AR9730557
Anke, M., and Seifert, M. (2004). “Titanium,” in Elements and Their Compounds in the Environment, 2nd Edn., eds E. Merian, M. Anke, M. Ihnat, and M. Stoeppler (Weinheim: Wiley-VCH), 1125–1140. doi: 10.1002/9783527619634.ch45
Arnon, D. I., and Stout, P. R. (1939). The essentiality of certain elements in minute quantity for plants with special reference to copper. Plant Physiol. 14, 371–375. doi: 10.1104/pp.14.2.371
Asli, S., and Neumann, P. M. (2009). Colloidal suspensions of clay or titanium dioxide nanoparticles can inhibit leaf growth and transpiration via physical effects on root water transport. Plant Cell Environ. 32, 577–584. doi: 10.1111/j.1365-3040.2009.01952.x
Berg, T., and Steinnes, E. (1997). Recent trends in atmospheric deposition of trace elements in Norway as evident from the 1995 moss survey. Sci. Total Environ. 208, 197–206. doi: 10.1016/S0048-9697(97)00253-2
Biacs, P. A., Daood, H. G., and Keresztes, A. (1997). “Biochemical aspect on the effect of Titavit treatment on carotenoids, lipds and antioxiants in spice red pepper,” in Physiology, Biochemistry and Molecular Biology of Plant Lipids, eds J. P. Williams, M. U. Khan, and N. W. Lem (Dordrecht: Springer Science + Business Media), 215–217.
Boonyanitipong, P., Kositsup, B., Kumar, P., Baruah, S., and Dutta, J. (2011). Toxicity of ZnO and TiO2 nanoparticles on germinating rice seed Oryza sativa L. Int. J. Biosci. Biochem. Bioinform. 1:282. doi: 10.7763/IJBBB.2011.V1.53
Bowles, F. W., Howie, R. A., Vaughan, D. J., and Zussman, J. (2011). Rock-Forming Minerals. vol. 5. Non-silicates Oxides, Hydroxides and Sulphides. 2nd Edn. Bath: The Geological Society Pubishing House.
Buettner, K. M., Collins, J. M., and Valentine, A. M. (2012). Titanium (IV) and vitamin C: aqueous complexes of a bioactive form of Ti (IV). Inorg. Chem. 51, 11030–11039. doi: 10.1021/ic301545m
Buettner, K. M., and Valentine, A. M. (2012). Bioinorganic chemistry of titanium. Chem. Rev. 112, 1863–1881. doi: 10.1021/cr1002886
Burke, D. J., Pietrasiak, N., Situ, S. F., Abenojar, E. C., Porche, M., Kraj, P., et al. (2015). Iron oxide and titanium dioxide nanoparticle effects on plant performance and root associated microbes. Int. J. Mol. Sci. 16, 23630–23650. doi: 10.3390/ijms161023630
Cabannes, E., Buchner, P., Broadley, M. R., and Hawkesford, M. J. (2011). A comparison of sulfate and selenate accumulation in relation to the expression of sulfate transporter genes in Astragalus species. Plant Physiol. 187, 2227–2239. doi: 10.1104/pp.111.183897
Cannon, H. L., Shacklette, H. T., and Bastron, H. (1968). Metal Absorption by Equisetum (Horsetail). Geological Survey Bulletin 1278-A. Washington, DC: United State Govement Printing Office.
Cao, W., Rui, Y., and Li, X. (2014). Determination of forty six elements in different organs of Orychophragmus violaceus in agricultural farm. Asian J. Chem. 26, 1038–1040. doi: 10.14233/ajchem.2014.15837
Carvajal, M., and Alcaraz, C. F. (1995). Effect of Ti (IV) on Fe activity in Capsicum anuum. Phytochemistry 39, 977–980. doi: 10.1016/0031-9422(95)00095-O
Carvajal, M., and Alcaraz, C. F. (1998). Why titanium is a beneficial element for plants. J. Plant Nutr. 21, 655–664. doi: 10.1080/01904169809365433
Carvajal, M., Martinez-Sanchez, F., and Alcaraz, C. F. (1994). Effect of titanium (IV) application on some enzymatic activities in several developing stages of red pepper plants. J. Plant Nutr. 17, 243–253. doi: 10.1080/01904169409364724
Carvajal, M., Martínez-Sánchez, F., Pastor, J. J., and Alcaraz, C. F. (1995). “Leaf spray with Ti(IV) ascorbate improves the iron uptake and iron activity in Capsicum annuum L. plants,” in Iron Nutrition in Soils and Plants, ed J. Abadía (Dordrecht: Kluwer Academic Publishers), 1–5. doi: 10.1007/978-94-011-0503-3_1
Ceccantini, G., Figuriredo, A. M. G., Sondag, F., and Soubies, F. (1997). “Rare earth elments and titanium in plants, soils and groundwaters in the alkaline-ultramafic complex of Salitre, MG, Brazil,” in Contaminated Soils, 3rd International Conference on the Biogeochemistry of Trace Elements, ed R. Prost (Paris).
Chao, S. H. L., and Choi, H. S. (2005). Method for Providing Enhanced Photosynthesis. Jeonju: Korea Research Institute of Chemical Technology Bulletin.
Chen, Y. Y., Wang, Y., Shin, L. J., Wu, J. F., Shanmugam, V., Tsednee, M., et al. (2013). Iron is involved in the maintenance of circadian period length in Arabidopsis. Plant Physiol. 161, 1409–1420. doi: 10.1104/pp.112.212068
Cigler, P., Olejnickova, J., Hruby, M., Csefalvay, L., Peterka, J., and Kuzel, S. (2010). Interactions between iron and titanium metabolism in spinach: a chlorophyll fluorescence study in hydropony. J. Plant Physiol. 167, 1592–1597. doi: 10.1016/j.jplph.2010.06.021
Clement, L., Hurel, C., and Marmier, N. (2013). Toxicity of TiO2 nanoparticles to cladocerans, algae, rotifers and plants - effects of size and crystalline structure. Chemosphere 90, 1083–1090. doi: 10.1016/j.chemosphere.2012.09.013
Comba, P., and Merbach, A. (1987). The titanyl question revisited. Inorg. Chem. 26, 1315–1323. doi: 10.1021/ic00255a024
Connolly, E. L., Fett, J. P., and Guerinot, M. L. (2002). Expression of the IRT1 metal transporter is controlled by metals at the levels of transcript and protein accumulation. Plant Cell 14, 1347–1357. doi: 10.1105/tpc.001263
Connor, J. H., and Shacklette, H. T. (1975). “Background geochemistry of some rocks, soils plants and vegetables in the conterminous United States,” in Statistical Studies in Field Geochemistry, Geological Survey Professional Paper 574F (Washington, DC: United States Government Printing Office).
Cook, L. L., McGonigle, T. P., and Inouye, R. S. (2009). Titanium as an indicator of residual soil on arid-land plants. J. Environ. Qual. 38, 188–199. doi: 10.2134/jeq2007.0034
Cornu, S., Lucas, Y., Lebon, E., Ambrosi, J. P., Luizao, F., Rouiller, J., et al. (1999). Evidence of titatnium mobility in soil profiles, Manaus, central Amazonia. Geoderma 91, 281–295. doi: 10.1016/S0016-7061(99)00007-5
Cox, A., Venkatachalam, P., Sahi, S., and Sharm, N. (2016). Siliver and titanium nanoparticle toxicity in plants: a review of current research. Plant Physiol. Biochem. 107, 147–163. doi: 10.1016/j.plaphy.2016.05.022
Daood, H. G., Biacs, P., Fehér, M., Hajdu, F., and Pais, I. (1988). Effect of titanium on the activity of lipoxygenase. J. Plant Nutr. 11, 505–516. doi: 10.1080/01904168809363818
Dehkourdi, E. H., and Mosavi, M. (2013). Effect of anatase nanoparticles (TiO2) on parsley seed germination (Petroselinum crispum) in vitro. Biol. Trace Elem. Res. 155, 283–286. doi: 10.1007/s12011-013-9788-3
Demidchik, V., Davenport, R. J., and Tester, M. (2002). Nonselective cation channels in plants. Annu. Rev. Plant Biol. 53, 67–107. doi: 10.1146/annurev.arplant.53.091901.161540
Dietz, K. J., and Herth, S. (2011). Plant nanotoxicology. Trends Plant Sci. 16, 582–589. doi: 10.1016/j.tplants.2011.08.003
Dolatabadi, A., Sani, B., and Moaveni, P. (2015). Impact of nanosized titanium dioxide on agronomical and physiological characteristics of annual medic (Medicago scutellata L.). Cercetari Agronomice Moldova 48, 53–61. doi: 10.1515/cerce-2015-0041
Du, W., Sun, Y., Ji, R., Zhu, J., Wu, J., and Guo, H. (2011). TiO2 and ZnO nanoparticles negatively affect wheat growth and soil enzyme activities in agricultural soil. J. Environ. Monit. 13, 822–828. doi: 10.1039/c0em00611d
Du, X., Rate, A. W., and Gee, M. A. M. (2012). Redistribution and mobilization of titaunium, zirconium and thorium in an intensely weathered lateritic profile in Western Australia. Chem. Geol. 330, 101–115. doi: 10.1016/j.chemgeo.2012.08.030
Dumon, J. C., and Ernst, W. H. O. (1988). Titanium in plants. J. Plant Physiol. 133, 203–209. doi: 10.1016/S0176-1617(88)80138-X
Eichert, T., and Fernandez, V. (2012). “Uptake and release of elements by leaves and other aerial plant parts,” in Marschner's Minearl Nutrition of Higher Plants, ed P. Marschner (Oxford: Academic Press), 71–84. doi: 10.1016/B978-0-12-384905-2.00004-2
Eide, D., Broderius, M., Fett, J., and Guerinot, M. L. (1996). A novel iron-regulated metal transporter from plants identified by functional expression in yeast. Proc. Natl. Acad. Sci. U.S.A. 93, 5624–5628. doi: 10.1073/pnas.93.11.5624
Ernst, W. H. O. (1985). Bedeutung einer veranderten Mineralstoffverfiigbarkeit (Schwer-metalle, AI, Ti) fiir Wachsrums und Selektionsprozesse in Waldern. Bielefelder Okol. Beitr. 1, 143–158.
Fallahi, E., and Eichert, T. (2013). Principles and practices of foliar nutrients with an emphasis on nitrogen and calcium sprays in apple. Horttechnology 23, 542–547.
Feizi, H., Kamali, M., Jafari, L., and Rezvani Moghaddam, P. (2013). Phytotoxicity and stimulatory impacts of nanosized and bulk titanium dioxide on fennel (Foeniculum vulgare Mill). Chemosphere 91, 506–511. doi: 10.1016/j.chemosphere.2012.12.012
Feizi, H., Rezvani Moghaddam, P., Shahtahmassebi, N., and Fotovat, A. (2012). Impact of bulk and nanosized titanium dioxide (TiO2) on wheat seed germination and seedling growth. Biol. Trace. Elem. Res. 146, 101–106. doi: 10.1007/s12011-011-9222-7
Fisher, M., Gokhman, I., Pick, U., and Zamir, A. (1997). A structurally novel transferrin-like protein accumulates in the plasma membrane of the unicellular green alga Dunaliella salina grown in high salinities. J. Biol. Chem. 272, 1565–1570. doi: 10.1074/jbc.272.3.1565
Fisher, M., Zamir, A., and Pick, U. (1998). Iron uptake by the halotolerant alga Dunaliella is mediated by a plasma membrane transferrin. J. Biol. Chem. 273, 17553–17558. doi: 10.1074/jbc.273.28.17553
Frazier, T. P., Burklew, C. E., and Zhang, B. (2014). Titanium dioxide nanoparticles affect the growth and microRNA expression of tobacco (Nicotiana tabacum). Funct. Integr. Genomics 14, 75–83. doi: 10.1007/s10142-013-0341-4
Frutos, M. J., Pastor, J. J., Martínez-Sánchez, F., and Alcaraz, C. F. (1996). Improvement of the nitrogen uptake induced by titanium (IV) leaf supply in nitrogen-stressed pepper seedlings. J. Plant Nutr. 19, 771–783. doi: 10.1080/01904169609365159
Gao, F., Liu, C., Qu, C., Zheng, L., Yang, F., Su, M., et al. (2008). Was improvement of spinach growth by nano-TiO2 treatment related to the changes of Rubisco activase? Biometals 21, 211–217. doi: 10.1007/s10534-007-9110-y
Gao, J., Xu, G., Qian, H., Liu, P., Zhao, P., and Hu, Y. (2013). Effects of nano-TiO2 on photosynthetic characteristics of Ulmus elongata seedlings. Environ. Pollut. 176, 63–70. doi: 10.1016/j.envpol.2013.01.027
Geilmann, W. (1920). Uber die Verbreitung des Titans in Boden und Pflanzen. J. Landwinsch. 68, 107–124.
Ghosh, M., Bandyopadhyay, M., and Mukherjee, A. (2010). Genotoxicity of titanium dioxide (TiO2) nanoparticles at two trophic levels: plant and human lymphocytes. Chemosphere 81, 1253–1262. doi: 10.1016/j.chemosphere.2010.09.022
Giménez, J. L., Martinez-Sanchez, F., Moreno, J. L., Fuentes, J. L., and Alcaraz, C. F. (1990). “Titanium in plant nutrition. III. Effect of Ti (IV) on yield of Capsicum annuum L.,” in Nutricion Mineral Bajo Condiciones de Estres, ed J. Barcelo (Palma de Mallorca: SPIC-UIB), 123–128.
Gogos, A., Knauer, K., and Bucheli, T. D. (2012). Nanomaterials in plant protection and fertilization: current state, foreseen applications, and research priorities. J. Agric. Food Chem. 60, 9781–9792. doi: 10.1021/jf302154y
Gogos, A., Moll, J., Klingenfuss, F., van der Heijden, M., Irin, F., Green, M. J., et al. (2016). Vertical transport and plant uptake of nanoparticles in a soil mesocosm experiment. J. Nanobiotechnol. 14:40. doi: 10.1186/s12951-016-0191-z
Grajkowski, J., and Ochmian, I. (2007). Influence of three biostimulants on yielding and fruit quality of three primocane raspberry cultivars. Acta. Sci. Pol. Hortorum Cult. 6, 29–36.
Guerinot, M. L. (2000). The ZIP family of metal transporters. Biochim. Biophys. Acta 1465, 190–198. doi: 10.1016/S0005-2736(00)00138-3
Guha, M. M., and Mitchell, R. L. (1966). The trace and major element composition of the leaves of some deciduous trees. ll. Seasonal changes. Plant Soil 24, 90–112. doi: 10.1007/BF01373076
Guo, F. Q., Wang, Y. Q., Sun, J. X., and Chen, H. M. (1996). Preliminary study on rare earth bound proteins in natural plant fern Dicranopteris dichotoma. J. Radioanal. Nucl. Chem. 209, 91–99. doi: 10.1007/BF02063534
Guo, M., Sun, H., McArdle, H. J., Gambling, L., and Sadler, P. J. (2000). TiIV uptake and release by human serum transferrin and recognition of TiIV-transferrin by cancer cells: understanding the mechanism of action of the anticancer drug titanocene dichloride. Biochemistry 39, 10023–10033. doi: 10.1021/bi000798z
Haghighi, M., Heidarian, S., and Teixeira da Silva, J. A. (2012). The effect of titanium amendment in N-withholding nutrient solution on physiological and photosynthesis attributes and micronutrient uptake of tomato. Biol. Trace. Elem. Res. 150, 381–390. doi: 10.1007/s12011-012-9481-y
Haghighi, M., and Teixeira da Silva, J. A. (2014). The effect of N-TiO2 on tomato, onion, and radish seed germination. J. Crop Sci. Biotechnol. 17, 221–227. doi: 10.1007/s12892-014-0056-7
Hanif, H. U., Arshad, M., Ali, M. A., Ahmed, N., and Qazi, I. A. (2015). Phyto-availability of phosphorus to Lactuca sativa in response to soil applied TiO2 nanoparticles. Pakistan J. Agri. Sci. 52, 177–182.
Hara, T., Sonoda, Y., and Iwai, I. (1976). Growth response of cabbage plants to transition elements under water culture conditions. Soil Sci. Plant Nutr. 22, 307–315. doi: 10.1080/00380768.1976.10432993
Hatami, M., Ghorbanpour, M., and Salehiarjomand, H. (2014). Nano-anatase TiO2 modulates the germination behavior and seedling vigority of some commercially important medicinal and aromatic plants. J. Biol. Environ. Sci. 8, 53–59.
Hong, F., Yang, P., Gao, F., Liu, C., Zheng, L., Yang, F., et al. (2005a). Effect of nano-anatase TiO2 on spectral characterization of photosystem particles from spinach. Chem. Res. China Univ. 21, 196–200.
Hong, F., Zhou, J., Liu, C., Yang, F., Wu, C., Zheng, L., et al. (2005b). Effect of nano-TiO2 on photochemical reaction of chloroplasts of spinach. Biol. Trace Element Res. 105, 269–279. doi: 10.1385/BTER:105:1-3:269
Hrubý, M., Cígler, P., and Kuzel, S. (2002). Contribution to understanding the mechanism of Titanium action in plant. J. Plant Nutr. 25, 577–598. doi: 10.1081/PLN-120003383
Hu, Z., Richter, H., Sparovek, G., and Schnug, E. (2004). Physiological and biochemical effects of rare earth elements on plants and their agricultural significance: a review. J. Plant Nutr. 27, 183–220. doi: 10.1081/PLN-120027555
Huang, M. L., Tang, X. D., and Lu, L. (1993). “The use of titanium chelate in agriculture,” in Titanium 92 Science and Technology, Proc. Symp. 3. Miner. Met. Mater. Soc. eds F. H. Froes and I. L. Caplan (Warrendale: PA), 2779–2786.
Hutton, J. T., and Stephens, C. G. (1956). The paleopedology of Norfolk Island. Eur. J. Soil Sci. 7, 255–267. doi: 10.1111/j.1365-2389.1956.tb00883.x
István, P., Fehér, M., Bokori, J., and Nagy, B. (1991). Physilogically beneficial effects of titanium. Water Air Soil Pollut. 57, 675–680. doi: 10.1007/BF00282931
Iversen, T.-G., Skotland, T., and Sandvig, K. (2011). Endocytosis and intracellular transport of nanoparticles: present knowledge and need for future studies. Nano Today 6, 176–185. doi: 10.1016/j.nantod.2011.02.003
Jaberzadeh, A., Moaveni, P., Moghadam, H. R. T., and Zahedi, H. (2013). Influence of bulk and nanoparticles titanium foliar application on some agronomic traits, seed gluten and starch contents of wheat subjected to water deficit stress. Not. Bot. Horti Agrobot. Cluj Napoca 41, 201–207.
Kabata-Pendias, A., and Mukherjee, A. B. (2007). Trace Elements from Soils to Human. Berlin; Heidelberg: Springer-Verlag. doi: 10.1007/978-3-540-32714-1
Kabata-Pendias, A., and Pendias, H. (2001). Trace Elements in Soils and Plants. Boca Raton, FL: CRC Press.
Kah, M., Beulke, S., Tiede, K., and Hofmann, T. (2013). Nanopesticides: state of knowledge, environmental fate, and exposure modeling. Crit. Rev. Environ. Sci. Technol. 43, 1823–1867. doi: 10.1080/10643389.2012.671750
Kakei, Y., Ishimaru, Y., Kobayashi, T., Yamakawa, T., Nakanishi, H., and Nishizawa, N. K. (2012). OsYSL16 plays a role in the allocation of iron. Plant Mol. Biol. 79, 583–594. doi: 10.1007/s11103-012-9930-1
Kaup, B. S., and Carter, B. J. (1987). Determining Ti source and distribution within a pleustalf by micromorphology, submicroscopy and elemental analysis. Geoderma 40, 141–156. doi: 10.1016/0016-7061(87)90019-X
Kelemen, G., Keresztes, A., Bacsy, E., Feher, M., Fodor, P., and Pais, I. (1993). Distribution and intracellular localization of titanium in plants after titanium treatment. Food Struct. 12, 67–72.
Keller, A. A., McFerran, S., Lazareva, A., and Suh, S. (2013). Global life cycle releases of engineered nanomaterials. J. Nanopart. Res. 15, 1–17. doi: 10.1007/s11051-013-1692-4
Kiapour, H., Moaveni, P., Habibi, D., and Sani, B. (2015). Evaluation of the application of gibbrellic acid and titanium dioxide nanoparticles under drought stress on some traits of basil (Ocimum basilicum L.). Int. J. Agron. Agric. Res. 6, 138–150.
Kim, E., Kim, S.-H., Kim, H.-C., Lee, S. G., Lee, S. J., and Jeong, S. W. (2011). Growth inhibition of aquatic plant caused by silver and titanium oxide nanoparticles. Toxicol. Environ. Health Sci. 3, 1–6. doi: 10.1007/s13530-011-0071-8
Kiss, F., Deak, G., Feher, M., Balough, A., Szabolcsi, L., and Pais, I. (1985). The effect of titanium and gallium on photosynthetic rate of algae. J. Plant Nutr. 8, 825–831. doi: 10.1080/01904168509363387
Kleiber, T., and Markiewicz, B. (2013). Application of “Tytanit” in greenhouse tomato growing. Acta Sci. Pol. Hortorum Cult. 12, 117–126.
Klem, M. T., Mosolf, J., Young, M., Douglas, T., and Science, N. J. (2008). Photochemical mineralization of europium, titanium, and iron oxyhydroxide nanoparticles in the ferritin protein cage. Inorg Chem. 47, 2237–2239. doi: 10.1021/ic701740q
Kobayashi, T., and Nishizawa, N. K. (2012). Iron uptake, translocation, and regulation in higher plants. Annu. Rev. Plant Biol. 63, 131–152. doi: 10.1146/annurev-arplant-042811-105522
Konishi, K., and Tsuge, T. (1936). Inorganic constituents of green-manure crops. J. Agri. Chem. Soc. 12, 916–930. doi: 10.1271/nogeikagaku1924.12.328
Kořenková, L., Šebesta, M., Urík, M., Kolenčík, M., Kratošová, G., Bujdoš, M., et al. (2017). Physiological response of culture media-grown barley (Hordeum vulgare L.) to titanium oxide nanoparticles. Acta Agric. Scand. B Soil Plant Sci. 67, 285–291. doi: 10.1080/09064710.2016.1267255
Kovacik, P., Hudec, J., Ondrisik, P., and Poliakova, N. (2014). The effect of liquid Mg-Titanit on creation of winter wheat phytomass. Res. J. Agri. Sci. 46, 125–131.
Kovacik, P., Simansky, V., Ryant, P., Renco, M., and Hudec, J. (2016). Determination of the titanium contents in the winter oilseed rape plants (Brassica napus L.) by the application of fertilizer containing titanium. Acta Univ. Agri. Silvicult. Mend. Brun. 64, 81–90. doi: 10.11118/actaun201664010081
Kurepa, J., Paunesku, T., Vogt, S., Arora, H., Rabatic, B. M., Lu, J., et al. (2010). Uptake and distribution of ultrasmall anatase TiO2 alizarin red S nanoconjugates in Arabidopsis thaliana. Nano Lett. 10, 2296–2302. doi: 10.1021/nl903518f
Kuzel, S., Hruby, M., Cigler, P., Tlustos, P., and Van, P. N. (2003). Mechanism of physiological effects of titanium leaf sprays on plants grown on soil. Biol. Trace Elem. Res. 91, 179–189. doi: 10.1385/BTER:91:2:179
Kuzel, S. P., Cigler, P., Hruby, M., Vydra, J., Pavlikova, D., and Tlustos, P. (2007). The effect of simulatanous magnesium application on the biological effects of titanium. Plant Soil Environ. 53, 16–23.
Larue, C., Khodja, H., Herlin-Boime, N., Brisset, F., Flank, A. M., Fayard, B., et al. (2011). Investigation of titanium dioxide nanoparticles toxicity and uptake by plants. J. Phys 304:012057. doi: 10.1088/1742-6596/304/1/012057
Larue, C., Laurette, J., Herlin-Boime, N., Khodja, H., Fayard, B., Flank, A. M., et al. (2012a). Accumulation, translocation and impact of TiO2 nanoparticles in wheat (Triticum aestivum spp.): influence of diameter and crystal phase. Sci. Total Environ. 431, 197–208. doi: 10.1016/j.scitotenv.2012.04.073
Larue, C., Veronesi, G., Flank, A. M., Surble, S., Herlin-Boime, N., and Carriere, M. (2012b). Comparative uptake and impact of TiO2 nanoparticles in wheat and rapeseed. J. Toxicol. Environ. Health A 75, 722–734. doi: 10.1080/15287394.2012.689800
Lazar, M. A., Varghese, S., and Nair, S. S. (2012). Photocatalytic water treatment by titanium dioxide: recent updates. Catalysts 2, 572–601. doi: 10.3390/catal2040572
Lei, Z., Mingyu, S., Chao, L., Liang, C., Hao, H., Xiao, W., et al. (2007). Effects of Nanoanatase TiO2 on photosynthesis of spinach chloroplasts under different light illumination. Biol. Trace Elem Res. 119, 68–76. doi: 10.1007/s12011-007-0047-3
Lei, Z., Mingyu, S., Xiao, W., Chao, L., Chunxiang, Q., Liang, C., et al. (2008). Antioxidant stress is promoted by nano-anatase in spinach chloroplasts under UV-B radiation. Biol. Trace Elem. Res. 121, 69–79. doi: 10.1007/s12011-007-8028-0
Leskó, K., Stefanovits-Bányai, É., Pais, I., and Simon-Sarkadi, L. (2002). Effect of cadmium and titanium-ascorbate stress on biological active compounds in wheat seedlings. J. Plant Nutr. 25, 2571–2581. doi: 10.1081/PLN-120014714
Li, J., Naeem, M. S., Wang, X., Liu, L., Chen, C., Ma, N., et al. (2015). Nano-TiO2 is not phytotoxic as revealed by the oilseed rape growth and photosynthetic appratus ultra-structural response. PLoS ONE 10:e0143885. doi: 10.1371/journal.pone.0143885
Li, Q., Chen, X., Zhuang, J., and Chen, X. (2016). Decontaminating soil organic pollutants with manufactured nanoparticles. Environ. Sci. Pollut. Res. 23, 11533–11548. doi: 10.1007/s11356-016-6255-7
Li, W., Tang, H., Xiao, A., Xie, T., Sun, Y., Liao, Y., et al. (2011). Effects of applying titanium contained trace-element fertilizer to several grain crops in Hunan. Hunan Agric. Sci. 21, 55–58.
Lopez-Moreno, J. L., Giménez, J. L., Moreno, A., Fuentes, J. L., and Alcaraz, C. F. (1995). Plant biomass and fruit yield induction by Ti(IV) in P-stressed pepper crops. Fert. Res. 43, 131–136. doi: 10.1007/BF00747692
Mahmoodzadeh, H., and Aghili, R. (2014). Effect on germination and early growth characteristics in wheat plants (Triticum aestivum L.) seeds exposed to TiO2 nanoparticles. J. Chem. Health Risks 4, 467–472.
Mahmoodzadeh, H., Nabavi, M., and Kashefi, H. (2013). Effect of nanoscale titanium dioxide particles on the germination and growth of canola (Brassica napus). J. Ornamental Hort. Plants 3, 25–32.
Mandeh, M., Omidi, M., and Rahaie, M. (2012). In vitro influences of TiO2 nanoparticles on barley (Hordeum vulgare L.) tissue culture. Biol. Trace Elem. Res. 150, 376–380. doi: 10.1007/s12011-012-9480-z
Marcinek, B., and Hetman, J. (2008). The effect of foliage feeding on the structure of yield, dry weight content and macroelements in the corms of Sparaxis tricolor Ker-Gawl. Acta. Sci. Pol. Hortorum Cult. 7, 89–99.
Markert, B., and Haderlie, B. (1996). Instrumental Element and Multi-Element Analysis of Plant Samples: Methods and Application. New York, NY: John Wiley & Sons Press.
Markiewicz, B., and Kleiber, T. (2014). The effect of Tytanit application on the content of selected microelements and the biological value of tomato fruits. J. Elem. 19, 1065–1072.
Marschner, H. (2011). Marschner's Mineral Nutrition of Higher Plants. San Diego, CA: Academic Press.
Martinez-Sanchez, F., Nunez, M., Amoros, A., Gimenez, J. L., and Alcaraz, C. F. (1993). Effect of titanium leaf spray treatments on ascorbic acid levels of Capsicum annuum L. J. Plant Nutr. 16, 975–981. doi: 10.1080/01904169309364586
Mattiello, A., Filippi, A., Poscic, F., Musetti, R., Salvatici, M. C., Giordano, C., et al. (2015). Evidence of phytotoxicity and genotoxicity in Hordeum vulgare L. exposed to CeO2 and TiO2 nanoparticles. Front. Plant Sci. 6:1043. doi: 10.3389/fpls.2015.01043
Mir, N. A., Haque, M. M., Khan, A., Muneer, M., and Vijayalakshmi, S. (2014). Photocatalytic degradation of herbicide bentazone in aqueous suspension of TiO2: mineralization, identification of intermdiates and reaction pathway. Environ. Technol. 35, 407–415. doi: 10.1080/09593330.2013.829872
Moaveni, P., and Kheiri, T. (2011). “TiO2 nano particles affected on maize (Zea mays L),” in 2nd International Conference on Agricultural and Animal Science, Vol. 22 (Singapore: IACSIT Press), 160–163.
Moaveni, P., Talebi, A., Farahani, A., and Maroufi, K. (2011). “Study of nano particles TiO2 spraying on some yield components in barley (Hordem vulgare L.),” in Intl. Conf. Environ. Agri. Eng. IPCBEE, vol. 15 (Jurong West: IACSIT Press), 115–119.
Mohammadi, R., Maali-Amiri, R., and Abbasi, A. (2013). Effect of TiO2 nanoparticles on chickpea response to cold stress. Biol. Trace Elem. Res. 152, 403–410. doi: 10.1007/s12011-013-9631-x
Mohammadi, R., Maali-Amiri, R., and Mantri, N. L. (2014). Effect of TiO2 nanoparticles on oxidative damage and antioxidant defense systems in chickpea seedlings during cold stress. Russian J. Plant Physiol. 61, 768–775. doi: 10.1134/S1021443714050124
Moll, J., Okupnik, A., Gogos, A., Knauer, K., Bucheli, T. D., van der Heijden, M. G., et al. (2016). Effects of titanium dioxide nanoparticles on red clover and its rhizobial symbiont. PLoS ONE 11:e0155111. doi: 10.1371/journal.pone.0155111
Morteza, E., Moaveni, P., Farahani, H. A., and Kiyani, M. (2013). Study of photosynthetic pigments changes of maize (Zea mays L.) under nano TiO2 spraying at various growth stages. SpringerPlus 2:247. doi: 10.1186/2193-1801-2-247
Moseley, J. L., Page, M. D., Alder, N. P., Eriksson, M., Quinn, J., Soto, F., et al. (2002). Reciprocal expression of two candidate di-iron enzymes affecting photosystem I and light-harvesting complex accumulation. Plant Cell 14, 673–688. doi: 10.1105/tpc.010420
Nautsch-Laufer, C. (1974). Die Wirkung von Titan auf den Stoffwechsel von Phaseolus vulgaris und Zea mays. Dissertation University of Münster.
Němec, A., and Káš, V. (1923). Studien uber die physiologische Bedeutung des titanium Pflanzenorganismus. Biochem. Z. 140, 583–590.
Norman, D. J., and Chen, J. (2011). Effect of foliar application of titanium dioxide on bacterial blight of geranium and Xanthomonas leaf spot of poinsettia. Hortscience 46, 426–428.
Owolade, O. F., and Ogunleti, D. O. (2008). Effects of titanium dioxide on the diseases, development and yield of edible cowpea. J. Plant Protect. Res. 48, 329–335. doi: 10.2478/v10045-008-0042-5
Pais, I. (1983). The biological importance of titanium. J. Plant Nutr. 6, 3–131. doi: 10.1080/01904168309363075
Pais, I. (1992). Criteria of essentiality, beneficality and toxicity of chemical elements. Acta Aliment. 121, 145–152.
Pais, I., Feher, M., Farkas, E., Szabo, Z., and Comides, I. (1977). Titanium as a new trace element. Comm. Soil Sci. Plant Anal. 8, 407–410. doi: 10.1080/00103627709366732
Pais, I., Somos, A., Duda, G., Tarjanyi, F., and Nagymihaly, F. (1969). Trace-element experiments with tomato and paprika. Kberletugyi Kozlem 62, 25–401.
Pandey, R., Krishnapriya, V., and Bindraban, P. S. (2013). Biochemical Nutrient Pathway in Plants Applied as Foliar Spray: Phosphorus and Iron. Washington, DC: VFRC (Virtual Fertilizer Research Center) report 2013/1.
Paret, M. L., Vallad, G. E., Averett, D. R., Jones, J. B., and Olson, S. M. (2013). Photocatalysis: effect of light-activated nanoscale formulations of TiO2 on Xanthomonas perforans and control of bacterial spot of tomato. Phytopathology 103, 228–236. doi: 10.1094/PHYTO-08-12-0183-R
Pilon-Smits, E. A., Quinn, C. F., Tapken, W., Malagoli, M., and Schiavon, M. (2009). Physiological functions of beneficial elements. Curr. Opin. Plant Biol. 12, 267–274. doi: 10.1016/j.pbi.2009.04.009
Qi, M., Liu, Y., and Li, T. (2013). Nano-TiO2 improve the photosynthesis of tomato leaves under mild heat stress. Biol. Trace Element Res. 156, 323–328. doi: 10.1007/s12011-013-9833-2
Radkowski, A., Radkowska, I., and Lemek, T. (2015). Effects of foliar application of titanium on seed yield in timothy (Phleum pratense L.). Ecol. Chem. Eng. 22, 691–701. doi: 10.1515/eces-2015-0042
Rafique, R., Arshad, M., Khokhar, M., Qazi, I., Hamza, A., and Virk, N. (2015). Growth response of wheat to titania nanoparticles application. NUST J. Eng. Sci. 7, 42–46.
Raliya, R., Biswas, P., and Tarafdar, J. C. (2015a). TiO2 nanoparticle biosynthesis and its physiological effect on mung bean (Vigna radiata L.). Biotechnol. Rep. 5, 22–26. doi: 10.1016/j.btre.2014.10.009
Raliya, R., Nair, R., Chavalmane, S., Wang, W. N., and Biswas, P. (2015b). Mechanistic evaluation of translocation and physiological impact of titanium dioxide and zinc oxide nanoparticles on the tomato (Solanum lycopersicum L.) plant. Metallomics 7, 1584–1594. doi: 10.1039/C5MT00168D
Ram, N., Verloo, M., and Cottenie, A. (1983). Response of bean to foliar spray of titanium. Plant Soil 73, 285–290. doi: 10.1007/BF02197724
Ramakrishna, R. S., Paul, A. A., and Fonseka, J. P. R. (1989). Uptake of titanium and iron by Ipomoea biloba from titaniferrous sands. Environ. Exp. Bot. 29, 293–300. doi: 10.1016/0098-8472(89)90002-6
Reimann, C., Koller, F., Frengstad, B., Kashulina, G., Niskavaara, H., and Englmaier, P. (2001). Comparison of the element compositin in several plant species and their substrate from a 1555000 km2 area in northern Europe. Sci. Total Environ. 278, 87–112. doi: 10.1016/S0048-9697(00)00890-1
Rezaei, F., Moaveni, P., and Mozafari, H. (2015). Effect of different concentrations and time of nano TiO2 spraying on quantitative and qualitative yield of soybean (Glycine max L.) at Shahr-e-Qods, Iran". Biol. Forum 7, 957–964.
Römheld, V., and Marschner, H. (1986). Evidence for a specific uptake system for iron phytosiderophore in roots of grasses. Plant Physiol. 80, 175–180. doi: 10.1104/pp.80.1.175
Ruffini Castiglione, M., Giorgetti, L., Geri, C., and Cremonini, R. (2010). The effects of nano-TiO2 on seed germination, development and mitosis of root tip cells of Vicia narbonensis L. and Zea mays L. J. Nanopart. Res. 13, 2443–2449. doi: 10.1007/s11051-010-0135-8
Samadi, N., Yahyaabadi, S., and Rezayatmand, Z. (2014). Effect of TiO2 and TiO2 nanoparticle on germination, root and shoot Length and photosynthetic pigments of Mentha piperita. Int. J. Plant Soil Sci. 3, 408–418. doi: 10.9734/IJPSS/2014/7641
Scagel, C., Bi, G., Fuchigami, L. H., and Regan, R. P. (2008). Nitrogen availability alters mineral nutrient uptake and demand in container-grown deciduous and evergreen rhododendron. J. Environ. Hort. 26, 177–187.
Searles, P. S., and Bloom, A. J. (2003). Nitrate photo-assimilation in tomato leaves under short-term exposure to elevated carbon dioxide and low oxygen. Plant Cell Environ. 26, 1247–1255. doi: 10.1046/j.1365-3040.2003.01047.x
Serrano, M., Martínez-Romero, D., Castillo, S., Guillén, F., and Valero, D. (2004). Effect of preharvest sprays containing calcium, magnesium and titanium on the quality of peaches and nectarines at harvest and during postharvest storage. J. Sci. Food Agric. 84, 1270–1276. doi: 10.1002/jsfa.1753
Servin, A. D., Castillo-Michel, H., Hernandez-Viezcas, J. A., Diaz, B. C., Peralta-Videa, J. R., and Gardea-Torresdey, J. L. (2012). Synchrotron micro-XRF and micro-XANES confirmation of the uptake and translocation of TiO2 nanoparticles in cucumber (Cucumis sativus) plants. Environ. Sci. Technol. 46, 7637–7643. doi: 10.1021/es300955b
Servin, A. D., Morales, M. L., Castillo-Michel, H., Hernandez-Viezcas, J. A., Munoz, B., Zhao, L., et al. (2013). Synchrotron verification of TiO2 nanoparticle transfer from soil into the food chain. Environ. Sci. Technol. 47, 11592–11598. doi: 10.1021/es403368j
Servin, A., Elmer, W., Mukherjee, A., Torre-Roche, R. D., Hamdi, H., White, J. C., et al. (2015). A review of the used of engineered nanomaterials to suppress plant disease and enhance crop yield. J. Nanopart. Res. 17:92. doi: 10.1007/s11051-015-2907-7
Sheppard, S. C., and Evenden, W. G. (1990). Characteristics of plant concentration ratios assessed in a 64-site field survey of 23 elements. J. Environ. Radioact. 11, 15–36. doi: 10.1016/0265-931X(90)90041-S
Sherman, G. D. (1952). The titanium content of Hawaiian soils and its significance. Soil Sci. Soc. Am. Proc. 16, 15–18. doi: 10.2136/sssaj1952.03615995001600010006x
Simon, L., Balogh, A., Hajdu, F., and Pais, I. (1988). “Effect of titanium on growth and photosynthetic pigment composition of Chlorella pyrenoidosa (Green Alga). II. Effect of titanium ascorbate on pigment content and chlorophyll metabolism of Chlorella,” in New Results in the Research of Hardly Known Trace Elements and Their Role in the Food Chain, ed I. Pais (Budapest: University of Horticultural and Food Science), 87–101.
Singh, J., and Lee, B. K. (2016). Influence of nano-TiO2 particles on the bioaccumulation of Cd in soybean plants (Glycine max): a possible mechanism for the removal of Cd from the contaminated soil. J. Environ. Manage. 170, 88–96. doi: 10.1016/j.jenvman.2016.01.015
Skupień, K., and Oszmiański, J. (2007). Influence of titanium treatment on antioxidants content and antioxidant activity of strawberries. Acta Sci. Pol. Technol. Aliment. 6, 83–93.
Song, G., Gao, Y., Wu, H., Hou, W., Zhang, C., and Ma, H. (2012). Physiological effect of anatase TiO2 nanoparticles on Lemna minor. Environ. Toxicol. Chem. 31, 2147–2152. doi: 10.1002/etc.1933
Song, U., Jun, H., Waldman, B., Roh, J., Kim, Y., Yi, J., et al. (2013a). Functional analyses of nanoparticle toxicity: a comparative study of the effects of TiO2 and Ag on tomatoes (Lycopersicon esculentum). Ecotoxicol. Environ. Saf. 93, 60–67. doi: 10.1016/j.ecoenv.2013.03.033
Song, U., Shin, M., Lee, G., Roh, J., Kim, Y., and Lee, E. J. (2013b). Functional analysis of TiO2 nanoparticle toxicity in three plant species. Biol. Trace Elem. Res. 155, 93–103. doi: 10.1007/s12011-013-9765-x
Stace, H. C. T., Hubble, G. D., Brewer, R., Northcote, K. H., Sleeman, J. R., Mulcahy, M. J., et al. (1968). A Handbook of Australian Soils. Glenside, PA: Rellim Technical Publications.
Sun, T. Y., Gottschalk, F., Hungerbühler, K., and Nowack, B. (2014). Comprehensive probabilistic modelling of environmental emissions of engineered nanomaterials. Environ. Pollut. 185, 69–76. doi: 10.1016/j.envpol.2013.10.004
Szymanska, R., Kolodziej, K., Slesak, I., Zimak-Piekarczyk, P., Orzechowska, A., Gabruk, M., et al. (2016). Titanium dioxide nanoparticles (100-1000 mg/l) can affect vitamin E response in Arabidopsis thaliana. Environ. Pollut. 213, 957–965. doi: 10.1016/j.envpol.2016.03.026
Takagi, S. (1976). Naturally occurring iron-chelating compounds in oat- and rice-root washing. I. activity measurement and preliminary characterization. Soil Sci. Plant Nutr. 22, 423–433. doi: 10.1080/00380768.1976.10433004
Thevenot, P., Cho, J., Wavhal, D., Timmons, R. B., and Tang, L. (2008). Surface chemistry influence cancer killing effect of TiO2 nanoparticles. Nanomedicine 4, 226–236. doi: 10.1016/j.nano.2008.04.001
Tinoco, A. D., and Valentine, A. M. (2005). Ti (IV) binds to human serum transferrin more tightly than does Fe (III). J. Am. Chem. Soc. 127, 11218–11219. doi: 10.1021/ja052768v
Tlustoš, P., Cigler, P., Hruby, M., Kuzel, S., Szakova, J., and Balik, J. (2005). The role of titanium in biomass production and its influence on essential elements contents in field growing crops. Plant Soil Environ. 51, 19–25.
Traetta-Mosca, F. (1913). Titanium and the rare metals in the ash of the leaves of Kentucky tobacco cultivated in Italy. Gazzetta Chimica Italiana 43, 437–440.
Tripathi, D. K., Shweta, S., Singh, S., Pandey, R., Singh, V. P., Sharma, N. C., et al. (2017). An overview on manufactured nanoparticles in plants: uptake, translocation, accumulation and phytoxicity. Plant Physiol. Biochem. 110, 2–12. doi: 10.1016/j.plaphy.2016.07.030
Tumburu, L., Andersen, C. P., Rygiewicz, P. T., and Reichman, J. R. (2015). Phenotypic and genomic responses to titanium dioxide and cerium oxide nanoparticles in Arabidopsis germinants. Environ. Toxicol. Chem. 34, 70–83. doi: 10.1002/etc.2756
Tyler, G. (2004). Rare earth elements in soil and plant systems-a review. Plant Soil 267, 191–206. doi: 10.1007/s11104-005-4888-2
Varotto, C., Maiwald, D., Pesaresi, P., Jahns, P., Salamini, F., and Leister, D. (2002). The metal ion transporter IRT1 is necessary for iron homeostasis and efficient photosynthesis in Arabidopsis thaliana. Plant J. 31, 589–599. doi: 10.1046/j.1365-313X.2002.01381.x
Vert, G., Grotz, N., Dedaldechamp, F., Gaymard, F., Guerinot, M. L., Briat, J. F., et al. (2002). IRT1, an Arabidopsis transporter essential for iron uptake from the soil and for plant growth. Plant Cell 14, 1223–1233. doi: 10.1105/tpc.001388
Wallace, A., Alexander, G. V., and Chaudhry, F. M. (1977). Phytotoxicity of cobalt, vanadium, titanium, silver, and chromium. Commun. Soil Sci. Plant Anal. 8, 751–756. doi: 10.1080/00103627709366769
Wang, H., Shan, X. Q., Zhang, S. Z., and Wen, B. (2003). Preliminary characterization of a light-rare-earth-element- binding peptide of a natural perennial fern Dicranopteris dichotoma. Anal. Bioanal. Chem. 376, 49–52. doi: 10.1007/s00216-003-1853-x
Wang, L., Cheng, M., Chu, Y., Li, X., Chen, D. D. Y., Huang, X., et al. (2016). Responses of plant calmodulin to endocytosis induced by rare earth elements. Chemosphere 154, 408–415. doi: 10.1016/j.chemosphere.2016.03.106
Wang, L., Li, J., Zhou, Q., Yang, G., Ding, X. L., Li, X., et al. (2014). Rare earth elements activate endocytosis in plant cells. Proc. Natl. Acad. Sci. U.S.A. 111, 12936–12941. doi: 10.1073/pnas.1413376111
Wang, S., Kurepa, J., and Smalle, J. A. (2011). Ultra-small TiO2 nanoparticles disrupt microtubular networks in Arabidopsis thaliana. Plant Cell Environ. 34, 811–820. doi: 10.1111/j.1365-3040.2011.02284.x
Wang, W. N., Tarafdar, J. C., and Biswas, P. (2013). Nanoparticle synthesis and delivery by an aerosol route for watermelon plant foliar uptake. J. Nanopart. Res. 15:1417. doi: 10.1007/s11051-013-1417-8
White, P. J., Bowen, H. C., Parmaguru, P., Fritz, M., Spracklen, W. P., Spiby, R. E., et al. (2004). Interactions between selenium and sulphur nutrition in Arabidopsis thaliana. J. Expt. Bot. 55, 1927–1937. doi: 10.1093/jxb/erh192
Whitted-Haag, B., Kopsell, D. E., Kopsell, D. A., and Rhykerd, R. L. (2014). Foliar silicon and titanium applications influence growth and quality characteristics of annual bedding plants. Open Hort. J. 7, 6–15. doi: 10.2174/1874840601407010006
Wojcik, P. (2002). Vigor and nutrition of apple trees in nursery as influenced by titanium sprays. J. Plant Nutr. 25, 1129–1138. doi: 10.1081/PLN-120003944
Wojcik, P., and Wojcik, M. (2001). Growth and nutrition of M.26 Emla apple rootstock as influenced by titanium fertilization. J. Plant Nutr. 24, 1575–1588. doi: 10.1081/PLN-100106022
Xuming, W., Fengqing, G., Linglan, M., Jie, L., Sitao, Y., Ping, Y., et al. (2008). Effects of nano-anatase on ribulose-1, 5-bisphosphate carboxylase/oxygenase mRNA expression in spinach. Biol. Trace Elem. Res. 126, 280–289. doi: 10.1007/s12011-008-8203-y
Yang, Q., Wang, L., He, J., Li, X., Tong, W., Yang, Z., et al. (2016). Vitronectin-like protein is a first line of defense against lanthanum (III) stress in Arabidopsis leaf cells. Environ. Exp. Bot. 130, 86–94. doi: 10.1016/j.envexpbot.2016.05.011
Yang, W. W., Miao, A. J., and Yang, L. Y. (2012). Cd2+ Toxicity to a green alga Chlamydomonas reinhardtii as influenced by its adsorption on TiO2 engineered nanoparticles. PLoS ONE 7:e32300. doi: 10.1371/journal.pone.0032300
Yao, K. S., Wang, D. Y., Ho, W. Y., Yan, J. J., and Tzeng, K. C. (2007). Photocatalytic bactericidal effect of TiO2 thin film on plant pathogens. Surf. Coat. Technol. 201, 6886–6888. doi: 10.1016/j.surfcoat.2006.09.068
Yin, Z. F., Wu, L., Yang, H. G., and Su, Y. H. (2013). Recent progress in biomedical applications of titatnium dioxide. Phys. Chem. Chem. Phys. 15:4844. doi: 10.1039/c3cp43938k
Yuan, D. A., Shan, X. Q., Wen, B., and Huai, Q. (2001). Isolation and characterization of rare earth element-binding protein in roots of maize. Biol. Trace Elem. Res. 79, 185–194. doi: 10.1385/BTER:79:2:185
Ze, Y., Liu, C., Wang, L., Hong, M., and Hong, F. (2011). The regulation of TiO2 nanoparticles on the expression of light-harvesting complex II and photosynthesis of chloroplasts of Arabidopsis thaliana. Biol. Trace Elem. Res. 143, 1131–1141. doi: 10.1007/s12011-010-8901-0
Zhang, C. (2015). Involvement of iron-containing proteins in genome integrity in Arabidopsis thaliana. Genome Integr. 6:2. doi: 10.4103/2041-9414.155953
Zhang, M., Gao, B., Chen, J., and Li, Y. (2015). Effects of graphene on seed germination and seedling growth. J. Nanopart. Res. 17:78. doi: 10.1007/s11051-015-2885-9
Zhang, W., Zhu, Z., and Cheng, C. Y. (2011). A literature review of titanium metallurgical process. Hydrometallurgy 108, 177–188. doi: 10.1016/j.hydromet.2011.04.005
Zheng, L., Hong, F., Lu, S., and Liu, C. (2005). Effect of nano-TiO2 on strength of naturally aged seeds and growth of spinach. Bio. Trace Element Res. 104, 83–91. doi: 10.1385/BTER:104:1:083
Keywords: beneficial elements, ferric chelate reductase, ferritins, iron, metal transporter, nano-TiO2 particles (TiO2NPs), titanium
Citation: Lyu S, Wei X, Chen J, Wang C, Wang X and Pan D (2017) Titanium as a Beneficial Element for Crop Production. Front. Plant Sci. 8:597. doi: 10.3389/fpls.2017.00597
Received: 16 October 2016; Accepted: 03 April 2017;
Published: 25 April 2017.
Edited by:
Fernando Carlos Gómez-Merino, Colegio de Postgraduados, MexicoReviewed by:
Stefano Cesco, Free University of Bozen-Bolzano, ItalyMarta Dell'Orto, Università degli Studi di Milano, Italy
Copyright © 2017 Lyu, Wei, Chen, Wang, Wang and Pan. This is an open-access article distributed under the terms of the Creative Commons Attribution License (CC BY). The use, distribution or reproduction in other forums is permitted, provided the original author(s) or licensor are credited and that the original publication in this journal is cited, in accordance with accepted academic practice. No use, distribution or reproduction is permitted which does not comply with these terms.
*Correspondence: Jianjun Chen, ampjaGVuQHVmbC5lZHU=
Xiaoming Wang, d3htMTk2NEAxNjMuY29t
Dongming Pan, cGRtNjY2QDEyNi5jb20=
†These authors have contributed equally to this work.